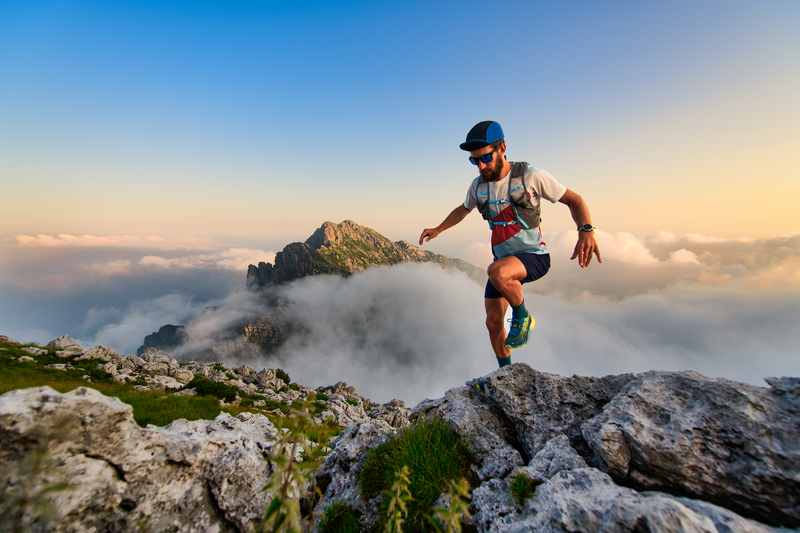
94% of researchers rate our articles as excellent or good
Learn more about the work of our research integrity team to safeguard the quality of each article we publish.
Find out more
MINI REVIEW article
Front. Cell Dev. Biol. , 28 August 2023
Sec. Signaling
Volume 11 - 2023 | https://doi.org/10.3389/fcell.2023.1264852
Extracellular vesicles (EVs) are lipid-enclosed structures that facilitate intercellular communication by transferring cargo between cells. Although predominantly studied in mammals, extracellular vesicles are ubiquitous across metazoans, and thus research in non-mammalian models is critical for fully elucidating extracellular vesicles biology. Recent advances demonstrate that extracellular vesicles mediate diverse physiological processes in non-mammalian vertebrates, including fish, amphibians, and reptiles. Piscine extracellular vesicles promote fin regeneration in zebrafish and carry heat shock proteins regulated by stress. Frog extracellular vesicles containing microRNAs modulate angiogenesis, while turtle extracellular vesicles coordinate reproductive functions. Venom from snakes contains extracellular vesicles that mirror the whole venom composition and interact with mammalian cells. Invertebrates also possess extracellular vesicles involved in immunity, development, and pathogenesis. Molluscan extracellular vesicles participate in shell formation and host interactions. Arthropod models, including Drosophila, genetically dissect conserved pathways controlling extracellular vesicles biogenesis and signalling. Nematode extracellular vesicles regulate larval development, animal communication, and ageing via conserved extracellular vesicles proteins. Ancient metazoan lineages utilise extracellular vesicles as well, with cnidarian extracellular vesicles regulating immunity and regeneration. Ultimately, expanding extracellular vesicles research beyond typical biomedical models to encompass phylogenetic diversity provides an unparalleled perspective on the conserved versus specialised aspects of metazoan extracellular vesicles roles over ∼500 million years. With a primary focus on the literature from the past 5 years, this review aims to reveal fundamental insights into EV-mediated intercellular communication mechanisms shaping animal physiology.
Extracellular vesicles (EVs) mediate intercellular communication across the animal kingdom by transferring biomolecular cargo that can alter recipient cell states. First identified in mammals over 40 years ago in rats (Harding et al., 1983), EVs have been extensively characterised in rodents and humans in vivo and in vitro contexts, providing significant insights into their origin, categorization, distribution, and impacts on immunity or tissue regeneration (van Niel et al., 2018). However, EV research in non-mammalian animals remains limited despite their vast phylogenetic diversity spanning over 500 million years of evolution. This review aims to bridge this knowledge gap by focusing on non-mammalian vertebrates, invertebrates, and early-diverging metazoans, which are often overlooked in EV studies. Examining EVs across this diversity presents an exceptional opportunity to elucidate universally conserved as well as lineage-specific aspects of EV biology adapted to diverse environments.
In general, EVs are membrane bound vesicles encompassing exosomes (Janouskova et al., 2022), microvesicles and other subtypes. They contain proteins, nucleic acids, lipids and other biomolecular cargo that can modulate signalling and function in recipient cells. EVs are produced by all cell types and found in all bodily fluids. They play key roles in health and disease by mediating local and systemic cell-cell communication (van Niel et al., 2018).
Looking beyond typical biomedically relevant models is critical for a comprehensive understanding of the role of metazoan EVs. This review aims to elucidate shared and distinct EV characteristics that were adapted to varying ecologies throughout animal evolution. Integrating discoveries in understudied organisms would provide valuable insights into EV formation, biodistribution, categorization and signalling in multicellular animals. Advancing the rapidly growing field requires prioritising comparative EV biology in diverse, understudied species.
While extracellular vesicles (EVs) have been extensively characterised in mammals, studies elucidating EVs in avian species remain scarce. Recent proteomic analysis (Luo et al., 2022) of EVs from rooster seminal plasma identified over 3,000 proteins, including many involved in sperm maturation and antimicrobial protection. This suggests avian EVs may regulate fertility, akin to prostasomes in mammals (Muñoz et al., 2022). Separately, EVs isolated from chicken egg yolks were shown to contain diverse miRNA species (Fratantonio et al., 2023). Feeding studies revealed these egg EVs accumulated in the mouse intestines, brain, and lungs post-ingestion. Egg EV depletion also impaired mouse cognition, while egg consumption increased plasma miRNAs and PBMCs gene expression in humans. Together, these findings provide initial glimpses into the cargo and potential physiological roles of EVs from avian reproductive secretions. Both egg and seminal EVs appear to mediate critical processes in the producer while transferring bioactive molecules to consumers. Further interrogation of the specific bioactive cargoes and functions of these avian EVs is greatly needed. Additional multi-omics profiling and functional validation studies across avian taxa would provide tremendous insights into the conserved versus specialised roles of non-mammalian EVs. Elucidating the contributions of these EVs to avian biology and cross-species interaction could uncover innovative approaches to enhance poultry production, egg nutritional value, and human health.
Criscitiello et al. have uncovered extracellular vesicles (EVs) in American alligator plasma, providing the first insights into EV biology in crocodilians (Criscitiello et al., 2020). The characterization of alligator EVs revealed a heterogeneous population with proteomic cargo involved in metabolism, immunity, and epigenetic control. EVs may facilitate moonlighting functions of proteins involved in alligator physiology, like cancer resistance. This establishes a model for elucidating EV mechanisms in ancient reptiles. Expanded EV analysis in diverse reptiles will uncover conserved and unique aspects of their biology.
Work in the Chinese softshell turtle showed biliary EVs originate from hepatocytes, supporting conserved EV roles in cell communication along the biliary system (Zhu et al., 2019). In turtle reproductive tracts, EVs from Sertoli and telocyte sources appear to coordinate spermatogenesis and sperm maturation (Chen and Holt, 2021). Further research into turtle EV cargos and functions will provide evolutionary insights into this signalling mechanism in amniotes.
Proteomic characterization of viper (Carregari et al., 2018) and cobra (Manuwar et al., 2020) venoms revealed the presence of EV-related proteins and cargo, suggesting EVs are an inherent component. Looking beyond venomics, Ogawa et al. (Ogawa et al., 2008) provided the first direct isolation and visualisation of EVs from Gloydius blomhoffii snake venom, determining they harbour bioactive cargo that degrades peptides. Expanding on this, Willard et al. (Willard et al., 2021) demonstrated quantitative proteomics of venom EVs can identify biomarkers of envenomation, while another study (Gonçalves-Machado et al., 2022) showed venom EVs containing toxin-processing enzymes are internalised by mammalian cells. Together, these pioneering studies indicate venom EVs may facilitate toxin spread and host effects. Further research on their biogenesis, proteomic content, and pathophysiological roles across diverse snake families is needed to elucidate venom EV biology.
The large fluid compartments in developing Xenopus embryos enabled the isolation of EVs for characterization, revealing dynamic changes in EV composition during embryogenesis (Danilchik and Tumarkin, 2017). Frog thrombocytes were also shown to secrete CD41+ EVs containing regulatory miRNAs that can modulate gene expression related to angiogenesis in target cells (Sugimoto and Toume, 2021). Additionally, EVs were isolated from the skin secretions of the amphibian Bombina maxima and found to be enriched in proteins involved in the stress response (Wei et al., 2022; Wang et al., 2023). Together, these studies demonstrate that amphibians are a rich source of EVs that likely play important physiological roles. Further research characterising the biogenesis, cargo, and functions of amphibian EVs, both in vitro and in vivo, promises to provide evolutionary insights into EV biology and intercellular communication in vertebrates. Studies in amphibian models can elucidate the conserved versus specialised roles of EVs in development, haemostasis, and innate immunity across species.
Recent studies in zebrafish models have begun to elucidate the roles of extracellular vesicles (EVs) in development, tissue homeostasis, and regeneration in vivo (reviewed in Verweij et al., 2019). Zebrafish embryos and larvae provide a powerful system to visualise and track endogenous EVs, revealing their biodistribution and clearance dynamics. Research uncovered tissue-specific EV impacts, like proposed regulation within intestinal epithelia (Bai et al., 2019) and promotion of osteoclast differentiation during bone fracture healing (Kobayashi-Sun et al., 2020). EVs have been tracked in the developing brain (Xu et al., 2017) and heart (Scott, Sueiro Ballesteros et al., 2021), establishing zebrafish as a versatile model to investigate endogenous EVs across development, homeostasis, and disease states.
Exosomes from olive flounder plasma exhibited wound healing and tissue regeneration activities in vitro and in vivo, accelerating fin regeneration in zebrafish larvae (Jayathilaka et al., 2022). Gene expression analysis indicated immunomodulatory effects, revealing the potential of fish plasma EVs as therapeutic biomaterials. Rainbow trout plasma exosomes were found to be enriched in the heat shock protein Hsp70, which increased after heat stress. However, the stress hormone cortisol reduced Hsp70 in exosomes, highlighting dynamic cargo regulation (Faught et al., 2017). Atlantic halibut serum EVs contained diverse cargo involved in immunity, gene regulation, metabolism, and development (Magnadóttir et al., 2021). Some complement proteins were post-translationally deiminated, including immune factor C3. Selective modification indicates regulated packaging of native and altered proteins via EVs.
Overall, these studies demonstrate the conservation of fundamental EV biology in fish while highlighting its importance in aquatic physiology and tissue repair. Fish provide tractable models to assess EV functions. Understanding piscine EVs has implications for biomedicine, evolutionary biology, and environmental toxicology.
Recent work has identified deiminated proteins and characterised extracellular vesicles (EVs) in the plasma of sea lamprey (Petromyzon marinus) (Rast et al., 2021). Proteomic analysis revealed 37 deiminated proteins in lamprey EVs, involved in pathways related to immunity, metabolism, muscle regulation, and cellular stress response. The findings provide the first evidence of post-translational deimination of key immune and regulatory proteins in lamprey plasma and EVs. Research into such an evolutionary ancient organism provides insights into the conserved roles of protein deimination and EVs throughout vertebrate phylogeny.
In nurse sharks (Ginglymostoma cirratum), six deiminated proteins were found in plasma, including complement C3, immunoglobulin, and hemopexin (Criscitiello et al., 2019). Plasma EVs, validated by morphology and EV markers, contained three deiminated proteins: novel antigen receptor (NAR), haptoglobin, and hemopexin. Together, this reveals conservation of protein deimination and EV-mediated export as mechanisms for immune regulation in early vertebrates. The study of these phylogenetically distant elasmobranchs provides evolutionary insight into the functional importance of post-translational modifications and EVs.
Arthropods comprise the most abundant and diverse animal phylum, including insects, arachnids, crustaceans, and other invertebrates. Research across select arthropod models has recently revealed important roles for extracellular vesicles (EVs) in arthropod biology.
In the commercially fished American lobster Homarus americanus, EVs isolated from haemolymph were found to range from 10 to 500 nm, with most under 115 nm (Bowden et al., 2020a). Haemolymph proteomics identified deiminated forms of nine proteins involved in metabolism, defence, and the regulation of vesicle release. KEGG pathway analysis revealed that deiminated proteins are involved in viral, bacterial, and fungal defence pathways. The authors suggest lobster EVs and protein deimination may facilitate immune responses and intercellular communication.
EVs isolated from the venom of the Chinese bird spider Ornithoctonus hainana ranged from 50 to 150 nm and expressed the EV marker Tsp29Fb (Xun et al., 2021). Proteomics identified 150 proteins related to vesicle transport, virulence factors, neurotoxins, and enzymes such as hyaluronidase. Functional assays showed the EVs exhibit hyaluronidase activity and inhibit human endothelial cell proliferation. The data shed light on the potential contributions of EVs to spider envenomation pathology.
In the tick Ixodes scapularis, EVs from salivary glands contained proteins involved in modulating host immunity and physiology (Oliva Chávez et al., 2021). RNAi silencing the EV biogenesis genes vamp33 and synaptobrevin-2 in tick cells reduced EV secretion and impaired tick feeding on mice. The EV-mediated feeding effect depended on modulating dermal gamma-delta T cells. I. scapularis EVs also enhanced the transmission of Anaplasma phagocytophilum in mice. Tick cell-derived EVs in the 30–200 nm range transmitted the flavivirus Langat virus to human keratinocytes and endothelial cells (Zhou et al., 2018). Cryo-EM imaging verified the purification of exosomes containing viral envelope proteins. The release of EVs from infected tick cells was reduced by the inhibitor GW4869. The authors propose EVs mediate transmission of tick-borne flaviviruses from arthropod to human cells.
The genetic model Drosophila has proven instrumental for elucidating fundamental EV biology, including biogenesis, cargo sorting, and roles in development and physiology (reviewed in Beer and Wehman, 2017). Drosophila EVs comprise exosomes and microvesicles that transport diverse cargos. Comparative analysis found similarities in size, morphology, and RNA contents between fly and human EVs (Lefebvre, Benoit Bouvrette et al., 2016). However, Drosophila EVs were enriched in small nucleolar RNAs, while human EVs contained more Y and vault RNAs. In vivo studies revealed EVs carry morphogens like Wingless that pattern tissues during development (Gross et al., 2012). Endocytic trafficking pathways control Wingless sorting into EVs. EVs also mediate physiological changes in the female reproductive tract in response to mating (Sanchez-Lopez et al., 2022). A specific tracheal EV subpopulation associates with fusion events, regulated by Rab GTPases and SNAREs (Camelo, Körte et al., 2022).
Studies in honeybees have revealed therapeutic bioactivities of EVs from bee products (reviewed in Peršurić and Pavelić, 2021). Bee gland secretomal products (honey, royal jelly, and bee pollen) EVs exhibited antimicrobial effects on Staphylococcus aureus and were internalised by human mesenchymal stem cells to increase migration (Schuh et al., 2019). Royal jelly EVs contained cargo proteins, and uptake into mammalian cells modulated mesenchymal stem cell differentiation (Álvarez et al., 2023). Anti-inflammatory effects were shown in macrophages, and acceleration of wound closure by royal jelly EVs was confirmed in vivo.
Overall, studies in Arthropods establish diverse EV roles in development and physiology. Drosophila enables the systematic dissection of molecular regulators controlling EV functions, providing insights into conserved metazoan communication. Analyses of therapeutic bee EVs warrant further research but show great potential for biomedical applications.
Molluscs possess a complex system of EVs involved in physiology, immunity, and biomineralization. Proteomic profiling revealed exosomal markers and immune cargo in the haemolymph EVs of four commercially valuable bivalve species (Bowden et al., 2020b). Species-specific variations in yield and size were observed, and deiminated proteins were identified. In freshwater mussels, mantle EVs containing miRNAs were isolated (Chen et al., 2019). Comparative sequencing suggested involvement in shell pigmentation. Mucus from the invasive slug Arion vulgaris contained abundant EVs, which could be internalised by plant and human cells and loaded with chemotherapy agents (Liegertová et al., 2022).
These findings demonstrate that molluscs have complex EV communication systems that modulate immunity, biomineralization, and host interactions. Ongoing isolation and sequencing of EVs from diverse mollusc tissues/species is needed to elucidate proteins/RNAs in shell formation. Studying EVs in emerging pests like Arion slugs could reveal novel signalling in plant pathogenesis. Overall, molluscan EVs remain an exciting research frontier at the intersection of marine biology, immunology, materials science, and biotechnology.
Research utilising the model nematode C. elegans has provided significant insights into the mechanisms of EV biogenesis and the functions of EVs in development and physiology. Studies in C. elegans demonstrate that both exosomes and microvesicles play critical roles during larval development and adult behaviour. Exosomes containing Hedgehog-related proteins that are essential for proper cuticle formation (Liégeois et al., 2006). The V-ATPase V0 subunit mediates fusion of multivesicular bodies with the plasma membrane for exosome release and secretion of Hedgehog signals. Additionally, ciliated sensory neurons selectively shed EVs in an intraflagellar transport-dependent manner (Wang and Barr, 2016), which could provide valuable insight into human ciliopathies. These neuronal EVs contain polycystins and influence animal-to-animal communication and mating behaviours.
Large-scale proteomic and RNA analysis of nematode EVs identified diverse coding and non-coding RNA and protein cargo types commonly found in human EVs, underscoring the conservation of EV composition (Russell et al., 2020). The EV cargo spectrum suggested that protein and RNA cargoes are actively recruited to EVs. The enrichment for ageing-related factors suggests EVs may modulate longevity and health span. The conservation of EV composition underscores the potential for C. elegans to elucidate fundamental mechanisms of EV signalling in ageing.
Recent work found that EVs derived from C. elegans dauer larvae, a stress-resistant form, could extend lifespan and health span when fed to normal worms (Ma et al., 2023). The anti-aging effects were associated with reduced oxidative stress and fat accumulation. Together, these findings establish C. elegans as a powerful genetic model to elucidate mechanisms of EV biogenesis as well as the functional roles of EVs in development, cell signalling, and ageing. Further exploration of EV biology in this simple organism would provide fundamental insights into conserved metazoan EV pathways.
Echinoderm studies have provided insights into multifunctional EVs roles in immunity, regeneration, and signalling. Proteomics of starfish coelomic epithelium revealed numerous exosomal diverse signalling proteins likely participating in immune responses and regeneration abilities (Guatelli et al., 2022). Sea urchin coelomic fluid EVs contained evolutionarily conserved proteins involved in immunity, the cytoskeleton, nuclear functions, metabolism, and stress pathways (D'Alessio et al., 2021). Echinoderm EVs appear to carry both conserved and specialised cargo. For instance, immune proteins underwent post-translational deimination in sea urchin EVs, suggesting regulatory roles in modulating responses. Both studies provided foundational isolation and profiling of echinoderm coelomic fluid EVs to inform future research. Overall, echinoderm EVs reveal intriguing similarities and key specialisations versus mammalian EVs. Further analyses across taxa will provide evolutionary perspectives on conserved and specialised EV biology. Studying EVs in regeneration could uncover therapeutic signalling pathways. Unique immune proteins warrant further study for the development of antimicrobial or immunoregulatory biotechnology. Continued echinoderm EV research holds potential for fundamental biological discovery and biomedical innovation.
Recent studies demonstrate the presence and importance of EVs as an ancient communication mode in early-diverging metazoans like cnidarians. In the freshwater polyp Hydra, EVs were thoroughly characterised and shown to exhibit exosomal morphology and markers (Moros et al., 2021). Proteomic and RNA sequencing revealed the EVs contained cytoskeletal, extracellular matrix, signalling, and metabolism-related proteins and transcripts, tracing back to multiple cell origins. Excitingly, EVs modulated developmental processes like head and foot regeneration in amputated Hydra. In corals, genes involved in exosome release were upregulated during infection, suggesting exosomes transport immune signals between cells (Takagi et al., 2020). Extracellular matrix genes were also increased, pointing to exosome-ECM interplay in the coral innate immune response.
These studies demonstrate that EVs are an important communication and immune regulation mode that might represent the first language of cell-cell communication to emerge during evolution. Further elucidating the bioactive molecules and physiological functions of cnidarian EVs promises to reveal fundamental metazoan signalling principles. A better understanding of coral innate immunity and EV pathways could support conservation efforts. The emerging picture is that EVs are an ancient language mediating developmental and immune signalling across animal evolution.
Research across diverse non-mammalian species reveals both highly conserved and uniquely adapted aspects of EV biology. From vertebrates to invertebrates, common themes emerge regarding EVs mediating immune regulation, tissue homeostasis, regeneration, and developmental signalling. Conserved EV biogenic pathways underlie EV release from Hydra to zebrafish. Cargo sorting mechanisms also display conservation, with small RNAs, heat shock proteins, morphogens, and immune factors consistently detected in EVs of organisms separated by hundreds of millions of years. Yet intriguing differences become apparent between mammal and non-mammal EVs. The specific bioactive proteins and RNAs often reflect adaptations to each species’ physiology or environment. Deiminated proteins in ancient vertebrate EVs highlight immune regulation. Enrichment of small nucleolar RNAs in fly EVs contrasts abundance of Y RNAs in human EVs, exemplifying divergence. Unique EVs from nematode neurons demonstrate cell type-specific biogenesis. Moving forward, elucidating EV cargo and impacts across phylogeny remains critical to uncovering new signalling paradigms and biomolecules translatable to biomedicine.
ML: Conceptualization, Writing–original draft, Writing–review and editing. OJ: Writing–review and editing.
The author(s) declare that no financial support was received for the research, authorship, and/or publication of this article.
The text was proof-read and refined by online proofreader Quillbot.
The authors declare that the research was conducted in the absence of any commercial or financial relationships that could be construed as a potential conflict of interest.
All claims expressed in this article are solely those of the authors and do not necessarily represent those of their affiliated organizations, or those of the publisher, the editors and the reviewers. Any product that may be evaluated in this article, or claim that may be made by its manufacturer, is not guaranteed or endorsed by the publisher.
Álvarez, S., Contreras-Kallens, P., Aguayo, S., Ramírez, O., Vallejos, C., Ruiz, J., et al. (2023). Royal jelly extracellular vesicles promote wound healing by modulating underlying cellular responses. Mol. Ther. Nucleic Acids 31, 541–552. doi:10.1016/j.omtn.2023.02.008
Bai, X., Guo, Y., Shi, Y., Lin, J., Tarique, I., Wang, X., et al. (2019). In vivo multivesicular bodies and their exosomes in the absorptive cells of the zebrafish (Danio Rerio) gut. Fish. Shellfish Immunol. 88, 578–586. doi:10.1016/j.fsi.2019.03.030
Beer, K. B., and Wehman, A. M. (2017). Mechanisms and functions of extracellular vesicle release in vivo-What we can learn from flies and worms. Cell Adh Migr. 11 (2), 135–150. doi:10.1080/19336918.2016.1236899
Bowden, T. J., Kraev, I., and Lange, S. (2020a). Extracellular vesicles and post-translational protein deimination signatures in haemolymph of the American lobster (Homarus americanus). Fish. Shellfish Immunol. 106, 79–102. doi:10.1016/j.fsi.2020.06.053
Bowden, T. J., Kraev, I., and Lange, S. (2020b). Extracellular vesicles and post-translational protein deimination signatures in Mollusca-the blue mussel (Mytilus edulis), soft shell clam (mya arenaria), eastern oyster (Crassostrea virginica) and atlantic jacknife clam (Ensis leei). Biol. (Basel) 9 (12), 416. doi:10.3390/biology9120416
Camelo, C., Körte, A., Jacobs, T., and Luschnig, S. (2022). Tracheal tube fusion in Drosophila involves release of extracellular vesicles from multivesicular bodies. J. Cell Sci. 135 (3), jcs259590. doi:10.1242/jcs.259590
Carregari, V. C., Rosa-Fernandes, L., Baldasso, P., Bydlowski, S. P., Marangoni, S., Larsen, M. R., et al. (2018). Author correction: snake venom extracellular vesicles (SVEVs) reveal wide molecular and functional proteome diversity. Sci. Rep. 8 (1), 15908. doi:10.1038/s41598-018-33284-3
Chen, Q., and Holt, W. V. (2021). Extracellular vesicles in the male reproductive tract of the softshell turtle. Reprod. Fertil. Dev. 33, 519–529. doi:10.1071/RD20214
Chen, X., Bai, Z., and Li, J. (2019). The mantle exosome and MicroRNAs of hyriopsis cumingii involved in nacre color formation. Mar. Biotechnol. (NY) 21 (5), 634–642. doi:10.1007/s10126-019-09908-8
Criscitiello, M. F., Kraev, I., and Lange, S. (2019). Deiminated proteins in extracellular vesicles and plasma of nurse shark (Ginglymostoma cirratum) - novel insights into shark immunity. Fish. Shellfish Immunol. 92, 249–255. doi:10.1016/j.fsi.2019.06.012
Criscitiello, M. F., Kraev, I., Petersen, L. H., and Lange, S. (2020). Deimination protein profiles in Alligator mississippiensis reveal plasma and extracellular vesicle-specific signatures relating to immunity, metabolic function, and gene regulation. Front. Immunol. 11, 651. doi:10.3389/fimmu.2020.00651
D'Alessio, S., Buckley, K. M., Kraev, I., Hayes, P., and Lange, S. (2021). Extracellular vesicle signatures and post-translational protein deimination in purple sea urchin (Strongylocentrotus purpuratus) coelomic fluid-novel insights into echinodermata biology. Biol. (Basel) 10 (9), 866. doi:10.3390/biology10090866
Danilchik, M., and Tumarkin, T. (2017). Exosomal trafficking in Xenopus development. Genesis 55 (1-2), e23011. doi:10.1002/dvg.23011
Faught, E., Henrickson, L., and Vijayan, M. M. (2017). Plasma exosomes are enriched in Hsp70 and modulated by stress and cortisol in rainbow trout. J. Endocrinol. 232 (2), 237–246. doi:10.1530/JOE-16-0427
Fratantonio, D., Munir, J., Shu, J., Howard, K., Baier, S. R., Cui, J., et al. (2023). The RNA cargo in small extracellular vesicles from chicken eggs is bioactive in C57BL/6 J mice and human peripheral blood mononuclear cells ex vivo. Front. Nutr. 10, 1162679. doi:10.3389/fnut.2023.1162679
Gonçalves-Machado, L., Verçoza, B. R. F., Nogueira, F. C. S., Melani, R. D., Domont, G. B., Rodrigues, S. P., et al. (2022). Extracellular vesicles from Bothrops jararaca venom are diverse in structure and protein composition and interact with mammalian cells. Toxins 14 (11), 806. doi:10.3390/toxins14110806
Gross, J. C., Chaudhary, V., Bartscherer, K., and Boutros, M. (2012). Active Wnt proteins are secreted on exosomes. Nat. Cell Biol. 14 (10), 1036–1045. doi:10.1038/ncb2574
Guatelli, S., Ferrario, C., Bonasoro, F., Anjo, S. I., Manadas, B., Candia Carnevali, M. D., et al. (2022). More than a simple epithelial layer: multifunctional role of echinoderm coelomic epithelium. Cell Tissue Res. 390 (2), 207–227. doi:10.1007/s00441-022-03678-x
Harding, C., Heuser, J., and Stahl, P. (1983). Receptor-mediated endocytosis of transferrin and recycling of the transferrin receptor in rat reticulocytes. J. Cell Biol. 97 (2), 329–339. doi:10.1083/jcb.97.2.329
Janouskova, O., Herma, R., Semeradtova, A., Poustka, D., Liegertova, M., Hana Auer, M., et al. (2022). Conventional and nonconventional sources of exosomes-isolation methods and influence on their downstream biomedical application. Front. Mol. Biosci. 9, 846650. doi:10.3389/fmolb.2022.846650
Jayathilaka, E., Edirisinghe, S. L., Lee, J., Nikapitiya, C., and De Zoysa, M. (2022). Isolation and characterization of plasma-derived exosomes from olive flounder (Paralichthys olivaceus) and their wound healing and regeneration activities. Fish. Shellfish Immunol. 128, 196–205. doi:10.1016/j.fsi.2022.07.076
Kobayashi-Sun, J., Yamamori, S., Kondo, M., Kuroda, J., Ikegame, M., Suzuki, N., et al. (2020). Uptake of osteoblast-derived extracellular vesicles promotes the differentiation of osteoclasts in the zebrafish scale. Commun. Biol. 3 (1), 190. doi:10.1038/s42003-020-0925-1
Lefebvre, F. A., Benoit Bouvrette, L. P., Perras, L., Blanchet-Cohen, A., Garnier, D., Rak, J., et al. (2016). Comparative transcriptomic analysis of human and Drosophila extracellular vesicles. Sci. Rep. 6, 27680. doi:10.1038/srep27680
Liégeois, S., Benedetto, A., Garnier, J. M., Schwab, Y., and Labouesse, M. (2006). The V0-ATPase mediates apical secretion of exosomes containing Hedgehog-related proteins in Caenorhabditis elegans. J. Cell Biol. 173 (6), 949–961. doi:10.1083/jcb.200511072
Liegertová, M., Semerádtová, A., Kocholatá, M., Průšová, M., Němcová, L., Štofik, M., et al. (2022). Mucus-derived exosome-like vesicles from the Spanish slug (arion vulgaris): taking advantage of invasive pest species in biotechnology. Sci. Rep. 12 (1), 21768. doi:10.1038/s41598-022-26335-3
Luo, X., Guo, Y., Huang, Y., Cheng, M., Wu, X., and Gong, Y. (2022). Characterization and proteomics of chicken seminal plasma extracellular vesicles. Reprod. Domest. Anim. 57 (1), 98–110. doi:10.1111/rda.14033
Ma, J., Wang, Y. T., Chen, L. H., Yang, B. Y., Jiang, Y. Z., Wang, L. X., et al. (2023). Dauer larva-derived extracellular vesicles extend the life of Caenorhabditis elegans. Biogerontology 24 (4), 581–592. doi:10.1007/s10522-023-10030-5
Magnadóttir, B., Kraev, I., Dodds, A. W., and Lange, S. (2021). The proteome and citrullinome of Hippoglossus hippoglossus extracellular vesicles-novel insights into roles of the serum secretome in immune, gene regulatory and metabolic pathways. Int. J. Mol. Sci. 22 (2), 875. doi:10.3390/ijms22020875
Manuwar, A., Dreyer, B., Böhmert, A., Ullah, A., Mughal, Z., Akrem, A., et al. (2020). Proteomic investigations of two Pakistani Naja snake venoms species unravel the venom complexity, posttranslational modifications, and presence of extracellular vesicles. Toxins (Basel) 12 (11), 669. doi:10.3390/toxins12110669
Moros, M., Fergola, E., Marchesano, V., Mutarelli, M., Tommasini, G., Miedziak, B., et al. (2021). The aquatic invertebrate Hydra vulgaris releases molecular messages through extracellular vesicles. Front. Cell Dev. Biol. 9, 788117. doi:10.3389/fcell.2021.788117
Muñoz, E. L., Fuentes, F. B., Felmer, R. N., Yeste, M., and Arias, M. E. (2022). Extracellular vesicles in mammalian reproduction: A review. Zygote 30 (4), 440–463. doi:10.1017/S0967199422000090
Ogawa, Y., Kanai-Azuma, M., Akimoto, Y., Kawakami, H., and Yanoshita, R. (2008). Exosome-like vesicles in Gloydius blomhoffii blomhoffii venom. Toxicon 51 (6), 984–993. doi:10.1016/j.toxicon.2008.02.003
Oliva Chávez, A. S., Wang, X., Marnin, L., Archer, N. K., Hammond, H. L., Carroll, E. E. M., et al. (2021). Tick extracellular vesicles enable arthropod feeding and promote distinct outcomes of bacterial infection. Nat. Commun. 12 (1), 3696. doi:10.1038/s41467-021-23900-8
Peršurić, Z., and Pavelić, S. K. (2021). Bioactives from bee products and accompanying extracellular vesicles as novel bioactive components for wound healing. Molecules 26 (12), 3770. doi:10.3390/molecules26123770
Rast, J. P., D'Alessio, S., Kraev, I., and Lange, S. (2021). Post-translational protein deimination signatures in sea lamprey (Petromyzon marinus) plasma and plasma-extracellular vesicles. Dev. Comp. Immunol. 125, 104225. doi:10.1016/j.dci.2021.104225
Russell, J. C., Kim, T. K., Noori, A., Merrihew, G. E., Robbins, J. E., Golubeva, A., et al. (2020). Composition of Caenorhabditis elegans extracellular vesicles suggests roles in metabolism, immunity, and aging. Geroscience 42 (4), 1133–1145. doi:10.1007/s11357-020-00204-1
Sanchez-Lopez, J. A., Twena, S., Apel, I., Kornhaeuser, S. C., Chasnitsky, M., Miklosi, A. G., et al. (2022). Male-female communication enhances release of extracellular vesicles leading to high fertility in Drosophila. Commun. Biol. 5 (1), 815. doi:10.1038/s42003-022-03770-6
Schuh, C., Aguayo, S., Zavala, G., and Khoury, M. (2019). Exosome-like vesicles in Apis mellifera bee pollen, honey and royal jelly contribute to their antibacterial and pro-regenerative activity. J. Exp. Biol. 222 (20), jeb208702. doi:10.1242/jeb.208702
Scott, A., Sueiro Ballesteros, L., Bradshaw, M., Tsuji, C., Power, A., Lorriman, J., et al. (2021). In vivo characterization of endogenous cardiovascular extracellular vesicles in larval and adult zebrafish. Arterioscler. Thromb. Vasc. Biol. 41 (9), 2454–2468. doi:10.1161/ATVBAHA.121.316539
Sugimoto, K., and Toume, K. (2021). Amphibian thrombocyte-derived extracellular vesicles, including microRNAs, induce angiogenesis-related genes in endothelial cells. Genes cells. 26 (10), 757–771. doi:10.1111/gtc.12882
Takagi, T., Yoshioka, Y., Zayasu, Y., Satoh, N., and Shinzato, C. (2020). Transcriptome analyses of immune system behaviors in primary polyp of coral acropora digitifera exposed to the bacterial pathogen Vibrio coralliilyticus under thermal loading. Mar. Biotechnol. (NY) 22 (6), 748–759. doi:10.1007/s10126-020-09984-1
van Niel, G., D'Angelo, G., and Raposo, G. (2018). Shedding light on the cell biology of extracellular vesicles. Nat. Rev. Mol. Cell Biol. 19 (4), 213–228. doi:10.1038/nrm.2017.125
Verweij, F. J., Hyenne, V., Van Niel, G., and Goetz, J. G. (2019). Extracellular vesicles: catching the light in zebrafish. Trends Cell Biol. 29 (10), 770–776. doi:10.1016/j.tcb.2019.07.007
Wang, J., and Barr, M. M. (2016). Ciliary extracellular vesicles: txt msg organelles. Cell Mol. Neurobiol. 36 (3), 449–457. doi:10.1007/s10571-016-0345-4
Wang, Q.-Q., Lan, X.-Q., Wei, X.-S., Xu, S.-M., Liu, L.-Z., Bian, X.-L., et al. (2023). Amphibian pore-forming protein βγ-CAT drives metabolite release from small extracellular vesicles through channel formation. Zoological Res. 44 (4), 739–742. doi:10.24272/j.issn.2095-8137.2022.510
Wei, X. S., Liu, L. Z., Bian, X. L., Zeng, L., Lee, W. H., Lin, L., et al. (2022). Protein composition of extracellular vesicles from skin secretions of the amphibian Bombina maxima. Zool. Res. 43 (4), 687–690. doi:10.24272/j.issn.2095-8137.2022.069
Willard, N. K., Salazar, E., Oyervides, F. A., Wiebe, C. S., Ocheltree, J. S., Cortez, M., et al. (2021). Proteomic identification and quantification of snake venom biomarkers in venom and plasma extracellular vesicles. Toxins (Basel) 13 (9), 654. doi:10.3390/toxins13090654
Xu, B., Zhang, Y., Du, X. F., Li, J., Zi, H. X., Bu, J. W., et al. (2017). Neurons secrete miR-132-containing exosomes to regulate brain vascular integrity. Cell Res. 27 (7), 882–897. doi:10.1038/cr.2017.62
Xun, C., Wang, L., Yang, H., Xiao, Z., Deng, M., Xu, R., et al. (2021). Origin and characterization of extracellular vesicles present in the spider venom of Ornithoctonus hainana. Toxins (Basel) 13 (8), 579. doi:10.3390/toxins13080579
Zhou, W., Woodson, M., Neupane, B., Bai, F., Sherman, M. B., Choi, K. H., et al. (2018). Exosomes serve as novel modes of tick-borne flavivirus transmission from arthropod to human cells and facilitates dissemination of viral RNA and proteins to the vertebrate neuronal cells. PLoS Pathog. 14 (1), e1006764. doi:10.1371/journal.ppat.1006764
Keywords: extracellular vesicles, exosomes, cargo, non-mammalian animal models, signalling, regeneration, development
Citation: Liegertová M and Janoušková O (2023) Bridging the extracellular vesicle knowledge gap: insights from non-mammalian vertebrates, invertebrates, and early-diverging metazoans. Front. Cell Dev. Biol. 11:1264852. doi: 10.3389/fcell.2023.1264852
Received: 21 July 2023; Accepted: 15 August 2023;
Published: 28 August 2023.
Edited by:
Leonid L Moroz, University of Florida, United StatesReviewed by:
Daria Romanova, Institute of Higher Nervous Activity and Neurophysiology (RAS), RussiaCopyright © 2023 Liegertová and Janoušková. This is an open-access article distributed under the terms of the Creative Commons Attribution License (CC BY). The use, distribution or reproduction in other forums is permitted, provided the original author(s) and the copyright owner(s) are credited and that the original publication in this journal is cited, in accordance with accepted academic practice. No use, distribution or reproduction is permitted which does not comply with these terms.
*Correspondence: Michaela Liegertová, bWljaGFlbGEubGllZ2VydG92YUB1amVwLmN6
Disclaimer: All claims expressed in this article are solely those of the authors and do not necessarily represent those of their affiliated organizations, or those of the publisher, the editors and the reviewers. Any product that may be evaluated in this article or claim that may be made by its manufacturer is not guaranteed or endorsed by the publisher.
Research integrity at Frontiers
Learn more about the work of our research integrity team to safeguard the quality of each article we publish.