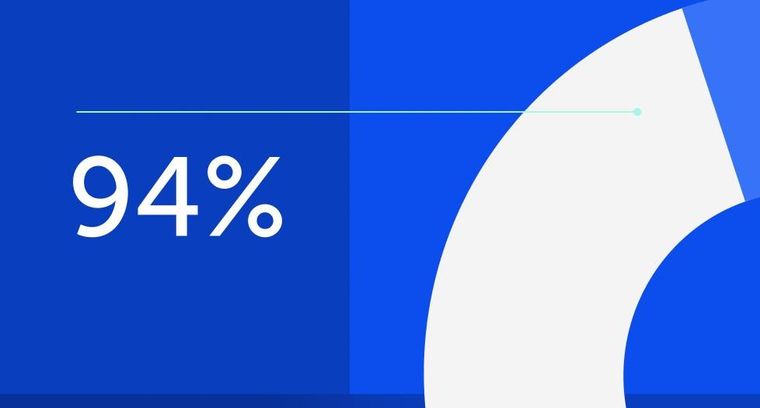
94% of researchers rate our articles as excellent or good
Learn more about the work of our research integrity team to safeguard the quality of each article we publish.
Find out more
REVIEW article
Front. Cell Dev. Biol., 02 October 2023
Sec. Stem Cell Research
Volume 11 - 2023 | https://doi.org/10.3389/fcell.2023.1255697
Multipotent mesenchymal stem cells (MSCs) are widely accepted as a useful tool for cell-based therapy of various diseases including malignancies. The therapeutic effects of MSCs are mainly attributed to their immunomodulatory and immunosuppressive properties. Despite the promising outcomes of MSCs in cancer therapy, a growing body of evidence implies that MSCs also show tumorigenic properties in the tumor microenvironment (TME), which might lead to tumor induction and progression. Owing to the broad-spectrum applications of MSCs, this challenge needs to be tackled so that they can be safely utilized in clinical practice. Herein, we review the diverse activities of MSCs in TME and highlight the potential methods to convert their protumorigenic characteristics into onco-suppressive effects.
Cancer is the major obstacle to improving life expectancy in the 21st century (Moslemi et al., 2021; Nagy et al., 2023). The morbidity and mortality of cancer are rising expeditiously because of aging and population growth (Sohrabi et al., 2021; Bisht et al., 2023). Cancer therapy is among the most crucial clinical challenges. Surgical intervention and chemotherapy, as the most common therapeutic methods, may be associated with different complications (Debela et al., 2021; Zeng et al., 2023). Despite the development of different therapy methods, metastatic tumors are mainly untreatable and are responsible for the preponderance of deaths due to cancer (Espona-Fiedler et al., 2023). A major barrier to the development of efficient therapies is the complexity of tumors. The tumor heterogeneity increases as cancer progresses and the components of the tumor microenvironment (TME) become fully developed. The TME contains extracellular matrix and stromal cells, as well as immune cells, thereby playing a substantial role in the evolution of malignant tumors (Roma-Rodrigues et al., 2019). In recent years, novel therapeutic approaches including stem cell therapy, targeted therapy, nanoparticles, ablation therapy, radionics, natural antioxidants, and chemodynamic therapy have been introduced. These methods have improved the outcomes of patients, nevertheless, further advancements in drug delivery systems are required to refine therapeutic outcomes (Debela et al., 2021).
Recently, mesenchymal stem cells (MSCs) have been of great interest in the field of cancer therapy. MSCs are precursor cells that have the ability to self-regulate and proliferate. Under particular circumstances, they can differentiate into numerous mesenchymal tissues (Chen et al., 2019; Mahjoor et al., 2021). MSCs are obtained from different tissues, such as bone marrow, adipose tissue, skin, salivary gland, limb buds, dental tissues, menstrual blood, and placenta. MSCs are primarily isolated as plastic-adherent cells via tissue mincing, enzymatic digestion, and cell outgrowth. The most commonly used procedures are enzymatic and explant techniques. In the explant protocol, the source tissue is rinsed and cut into small fragments. Afterward, the tissue fragments are transferred to plastic culture vessels containing growth medium. In the enzymatic technique, tissue pieces are incubated with enzymes that degrade the extracellular matrix (Mushahary et al., 2018; Mahjoor et al., 2022). MSCs express specific adhesion molecules (e.g., CD13, CD29, CD44, CD49b, CD58, CD73, CD105, and CD166). Besides, MSCs derived from different sources express specific markers. For instance, CD29 and CD49b are mainly expressed by MSCs isolated from the placenta, while bone marrow-MSCs (BM-MSCs) demonstrate higher levels of CD90 (Montesinos et al., 2009).
Immunomodulatory properties of MSCs are mediated by various cytokines, including transforming growth factor (TGF-β), hepatic growth factors (HGF), prostaglandin E2 (PGE2), interleukins (Khakoo et al., 2006), indolamine 2,3-dioxygenase (IDO), and nitric oxide (NO) (Soleymaninejadian et al., 2012; Mahmoudvand et al., 2023; Mirshekar et al., 2023). MSCs are divided into various subtypes, which show different features, accordingly can both boost and suppress tumor progression by exerting influence on tumor cells through different mediators and intercellular interactions as well as adjusting the innate and acquired immune response (Galland and Stamenkovic, 2020; Janmohammadi et al., 2023). MSC1 and MSC2 are two important phenotypes of MSCs. The former shows pro-inflammatory properties while the latter exerts immunosuppressive effects. Strong evidence confirms that MSC1 is primarily anti-tumorigenic, while MSC2 favors tumor cell growth. Tumor growth-promoting effects of MSCs include expression of growth factors, improvement of tumor angiogenesis, and formation of tumor stem cell micro-environment (Ramdasi et al., 2015). On the other hand, antitumorigenic effects of MSCs are exerted through several pathways including, promotion of the immune response, suppression of angiogenesis, control of cellular signaling, and induction of cancer components apoptosis (Atiya et al., 2020). In this review article, the anti-tumorigenic and protumorigenic properties of MSCs will be highlighted first and we further discuss the solutions that have been proposed to eliminate the protumorigenic activity of MSCs and convert them to anti-cancer features.
Investigations have revealed that despite the positive impact of MSCs on tumorigenesis, they can limit tumor growth. These effects may be exerted via different mechanisms.
• Effects of MSCs on TME
Via their strong proinflammatory properties, the combination of MSCs and tumor cells enhances the infiltration of monocytes, granulocytes, and T lymphocytes. The elevated infiltration of inflammatory cells facilitates interaction between immune cells and the adjacent tissues. These immune cells and the inflamed tissues enclosing them can produce several chemokines that recruit activated lymphocytes with their correlating receptors, hence provoking antitumor immunity (Figure 1A) (Ohlsson et al., 2003). Researchers have reported that iNOS-expressing MSCs successfully hinder the growth of fibrosarcoma cells (Xiang et al., 2009). As a matter of fact, iNOS synthesized by stromal cells plays a dual role in TME. M1 and M2 macrophages are essential determiners in the early and late stages of tumor growth (Trivanović et al., 2016). A similar behavior can be attributable to MSCs. Despite the lack of convincing evidence for MSC’s involvement in M1 polarization, the existence of iNOS-expressing M1 macrophages in tumor milieu suggests that MSCs may have the ability to acquire an M1 phenotype. Hence, it is reasonable to conclude that iNOS acts as a switch molecule of phenotypes of MSCs and macrophages in the tumor milieu. Overall, these findings point to the intricate cross-talks between macrophages and MSCs in TME (Sainz et al., 2016).
• Antitumor properties exerted through signaling pathways
FIGURE 1. (A). Mesenchymal stem cells strengthen the body’s immune system to combat tumors (B). The immune system, with its immunomodulatory and anti-inflammatory properties, shuts down the immune system, as a result of which we see the progress of the tumor.
Khakoo and co-authors (Khakoo et al., 2006) found that MSCs inhibited tumor progression in vivo by reducing target cell AKT activation in Kaposi’s sarcoma (KS). Nevertheless, they observed that when KS tumor cells were modified to express active AKT constantly, KS tumors were no longer susceptible to MSC treatment. These results imply that MSCs produce significant antitumorigenic properties via blocking AKT signaling. Furthermore, others have found that MSCs decrease breast cancer cell growth through the Wnt pathway, which is critical in oncogenesis (Qiao et al., 2008). MSCs have been administered systemically to deliver a binary vector containing an OAd along with a helper-dependent Ad that expresses IL-12 and programmed death-ligand 1 blocker (PD-L1). These MSCs deliver and synthesize viruses to invade and destroy lung tumor cells while triggering the onco-suppressive properties of chimeric antigen receptor-T (CAR-T) cells by producing IL-12 and PD-L1 blockers. In vivo, administration of combinatorial Ad vector MSCs causes a more significant rise in the number of T cells compared to CAR-T cells and propagates their polyfunctional cytokine release (McKenna et al., 2021). Moreover, in a study by Lu et al. (Lu et al., 2008), In cancerous cells, injection of MSCs increased the messenger ribonucleic acid (mRNA) expression of p21 (cell cycle negative regulator) and caspase 3 (apoptosis-related protease). Their results indicated that MSCs may suppress the growth of cancer in vitro and in vivo by enhancing cancer cell apoptosis and G0/G1 phase arrest. In addition, research has demonstrated that MSCs control cancer by decreasing tumor angiogenesis employing endothelial cell death and capillary degeneration (Otsu et al., 2009). As shown in a study, bone marrow MSCs inhibited vascular development in 1Gli36 glioma xenografts through suppression of the platelet-derived growth factor/platelet-derived growth factor receptor (PDGF/PDGFR) axis. Particularly, the expression of PDGF-BB protein considerably decreased in tumor lysates when treated with MSCs, which was associated with diminished concentrations of activated PDGFR-b and its subsequent target AKT isoform (Ho et al., 2013a). Lately, Gu and co-authors (Gu et al., 2021) discovered that MSCs-derived exosome could suppress hepatocellular cancer stem cells (CSCs) malignancy through a long noncoding RNAs (lncRNAs) C5orf66-AS1/micro-RNA-127-3p/dual-specificity phosphatase 1 (DUSP1)/ERK axis. Given the role of exosomes in both tumorigenic and anti-tumor activities of MSCs, hepatocellular CSCs were treated with exosomes, leading to a marked decrease in the proliferation, migration, invasion, angiogenesis-inducing, and self-regeneration capacities of CSCs via lncRNA C5orf66-AS1/microRNA-127-3p/DUSP1 axis and blockage of the phosphorylation of ERK in vitro. Similarly, in vivo investigation revealed that exosomes diminished the growth of xenografts made by CSCs in nude mice (Xuan et al., 2021). MSCs produce cytotoxic factors, including TNF-related apoptosis-inducing ligand (TRAIL) that selectively drives apoptosis in several types of malignancies (Hao et al., 2001; Takeda et al., 2001). In vivo investigations in the murine xenograft model of intraperitoneal human mesothelioma revealed the potential of TRAIL-expressing MSCs for dampening inflammatory responses in TME (Lathrop et al., 2015). Findings of another study conducted in 2019 showed that bone marrow MSCs could enhance apoptosis and inhibit the progression of glioma U251 cells via downregulating the PI3K/AKT signaling cascade (Lu et al., 2019). IFN-β-releasing BM-MSCs have been observed to diminish the growth of hepatocellular carcinoma cells primarily by affecting their cell cycle, reducing the expression of cyclin D1 and phosphorylation of Rb via silenced Akt and promoting FOXO3a activity (Xie et al., 2013).
• Cytokine-mediated mechanisms
IL-18-overexpressing umbilical cord MSCs (UC-MSCs) have been shown to attenuate the growth and spread of breast cancer cells, probably by modifying the cell cycle of cancerous cells (Liu et al., 2015). Additionally, by producing inflammatory cytokines such as the multifunctional cytokine TGF-β, MSCs have been shown to trigger anti-tumor immune responses. TGF-β signaling has inhibitory effects in cancer. Although the expression of the type III TGF-β receptor (TbRIII) drops throughout the evolution of breast cancer, restoring TbRIII expression inhibits tumorigenicity. This is even though TbRIII expression drops during progression. (Dong et al., 2007; Guasch et al., 2007) (Table 1).
• Tumorigenic effects of MSCs on TME
Heterogeneity within the TME is an important factor with a detrimental effect on the development of tumors. The TME is an intricate environment, composed of stromal cells and components of the extracellular matrix, along with secreted factors (Nilendu et al., 2018). Stromal cells in TME combine endothelial cells, adipocytes, cancer-associated fibroblasts, immune cells, and MSCs (Spaw et al., 2017; Tao et al., 2023). Notably, MSCs exhibit significant tropism to tumor sites, which may either speed up or slow down the progression of cancer (Xuan et al., 2021). Toll-like receptors (TLRs) exist in MSCs, among other cell types. TLRs are able to recognize signals of ‘danger,’ and once they are activated, a wide range of cells, particularly immune cells and MSCs, are drawn to the injury site. It is noteworthy that activation of TLR3 drives MSCs to produce factors that primarily have an immunomodulatory effect on the tumor cells (such as IL-1 receptor antagonist and IL10), whereas activation of TLR4 results in the production of inflammatory and proapoptotic factors by MSCs (such as IL17, granulocyte-macrophage colony-stimulating factor, and TRAIL). Degrading tryptophan is another process through which IDO synthesized by MSCs was able to block allogeneic T-cell responses (Meisel et al., 2004). In particular, in naive CD4+T cells, tryptophan catabolism induced the production of the forkhead box P3-positive regulatory T cells (Fallarino et al., 2006). These cells impeded the responses of effector T cells, which led to a decrease in anti-tumor immunity. Current research has introduced an innovative method through which MSCs control the activity of the immune system. In fact, MSCs attracted myeloid-derived suppressor cells (MDSCs) in a C-C motif chemokine ligand 2 (CCL2)-dependent mechanism, hence lowering the activity of anti-cancer T cells even further (Lee et al., 2015). The MDSC is considered the main protector of the TME, providing an immunosuppressive shield that protects the cancerous cells from the host’s immune system (Tesi, 2019). MSCs have the ability to decrease the activities of both T cells and B cells as part of the adaptive immune response. MSCs were able to restrict B cell activity by producing humoral chemicals via reducing B cell terminal differentiation (Asari et al., 2009). Overall, MSCs have powerful inhibitory effects on adaptive immune response, which can be used by cancer cells inside TME. MSCs not only suppress the adaptive immune response, but they also inhibit the innate immune cells, causing a reduction in the effectiveness of the basic immunological responses against cancer. MSCs inhibited the formation and function of monocyte-derived DCs, causing a reduction in the expression of the costimulatory molecules CD80 and CD86. This resulted in a limitation of the allogeneic T cell’s potential for allostimulation (Jiang et al., 2005). Importantly, macrophage functioning in the TME was directly suppressed by MSCs. It has been reported that the conditioned medium formed from MSC may inhibit the phagocytic activity of macrophages, hence further lowering anti-cancer immunity (Chen et al., 2018). Moreover, the activity of neutrophils was affected by the presence of MSCs. In a model of breast cancer, CD11b+Ly6G + neutrophils were cocultured with MSCs and then taught to obtain immunomodulatory properties. This training resulted in the neutrophils inhibiting the proliferation of T cells in vitro and accelerating tumor growth in vivo (Hu et al., 2014). The mesenchymal niche might also be implicated in cancer metastasis. Growing shreds of evidence show that MSCs have the capacity to migrate to tumor locations, including both primary and pre-metastatic sites (Kaplan et al., 2005). Tumor-secreted elements might move to surrounding tissues (Bergfeld and DeClerck, 2010) and draw MSCs to aid in the formation of mesenchymal niche, propagating tumor cell migration. Breast cancer cells promote the synthesis of CCL5 (also called RANTES) from MSCs by communicating with C-C chemokine receptor type 5, enhancing cancer cell motility, invasion, and distant spread in vitro and in vivo (Karnoub et al., 2007). Once affected by oxidative stress in the TME, MSCs can release lactate, and when lactate is absorbed by cancer cells, they can migrate more efficiently by producing ATP (Bonuccelli et al., 2014). In particular, MSCs were found to differentiate into cancer-associated fibroblasts in vitro, which promotes tumor heterogeneity and aids in cancer growth and drug resistance (Miyazaki et al., 2020). Several researchers have also shown that noncoding RNAs are involved in tumorigenesis and drug resistance (Asari et al., 2009; Wang et al., 2015; Yuan et al., 2016). Taken together, the evidence revealed the role of MSCs in boosting cancer progression via different mechanisms, hence targeting MSCs can be a potential strategy for cancer therapy (Xuan et al., 2021).
• Cytokine-mediated mechanisms
MSCs were found to block the oncosuppressive innate and adaptive immune responses, via releasing several soluble factors and mediators (e.g., PGE2, interferon-gamma (IFNγ), IL-4, indoleamine 2,3-dioxygenase (Kaplan et al., 2005), TGF-β1, IL-6) and cross-talking with a wide range of immune cell types (e.g., T cell, B cells, macrophages, dendritic cells, natural killer (NK) cells, and neutrophils) (Rivera-Cruz et al., 2017). MSCs inhibit both the activation and proliferation of T cells, which serve a substantial role in adaptive immunological responses. PGE2 is released by MSCs, which subsequently binds to prostaglandin EP2 and EP4 receptors on macrophages, causing them to produce the anti-inflammatory cytokine IL-10 and limit T cell activity (Németh et al., 2009). Besides, T helper 2 (Th2)-polarized immune response is evoked by MSCs. In fact, they cause a reduction in inflammatory T cells and their related cytokines (Th1 cells-IFNγ), while elevating anti-inflammatory T cells and related cytokines (Th2 cells-IL4) (Bai et al., 2009). MSCs were also able to suppress T cell activation via secreting immunosuppressive TGF-β1, which adheres to glycoprotein A repetitions predominant (GARP) located on MSCs (Niu et al., 2017). IFNγ-activated MSCs were accompanied by an upregulation in the expression of galectin-9, resulting in suppressed antigen-driven immunoglobulin secretion and lowered B cell proliferation (Ungerer et al., 2014). The functions of NK cells are inhibited by MSC-originated PGE2 and IL-6. Moreover, MSCs were shown to largely suppress the synthesis of IFN-γ in NK cells, which reduced the anti-cancer efficacy of the NK cells (Galland et al., 2017). Dendritic cells (DCs), which play a role in the process of presenting antigens, are intricately associated with anti-cancer activity. It has been shown that the maturation and function of DCs were impeded when PGE2 produced by MSCs was present in the environment (Spaggiari et al., 2009). Also, MSC-derived PGE2 stimulated a switch from inflammatory M1 macrophages to a pro-tumorigenic M2 state, which was associated with increased concentrations of immune-inhibitory IL-10 (Vasandan et al., 2016). The aforementioned evidence suggests that MSCs are able to inhibit the immune response to tumors, which in turn promotes the progression of tumors. In addition, MSCs exhibited the ability to promote the proliferation of cancer cells as well as neovascularization. In breast and prostate cancers, for example, MSCs increased the levels of pro-angiogenic factors such as vascular endothelial growth factor (VEGF), macrophage inflammatory protein-2 (MIP-2), TGF-β, and IL-6. Owing to the effects of these substances, tumor proliferation and angiogenesis were triggered, thereby solid tumor formation was sped up both in vitro and in vivo (Zhang et al., 2013a). Tumor cell apoptosis is also inhibited by MSCs. Hypoxia, malnutrition, and inflammation all contribute to tumor pathogenesis. Under this circumstance, MSCs maintain their self-survival via autophagy and secreting a variety of pro-survival or anti-apoptotic factors, such as basic fibroblast growth factor (bFGF), PDGF, VEGF, TGF-β, stromal cell-derived factor 1 alpha (SDF-1α), NO, and hepatocyte growth factor (HGF) (Hung et al., 2007). As an illustration, vascular VEGFs and bFGF, can promote Bcl-2 expression (König et al., 1997; Dias et al., 2002); on the other hand, PDGF and TGF-β upregulate VEGF and bFGF gene expression (Brogi et al., 1994). SDF-1α is able to defend leukemia cells against spontaneous apoptosis (Burger et al., 2000), and HGF improves the angiogenic and anti-apoptotic effects (Efimenko et al., 2011). Besides, NO has been proposed to act as a dual-function apoptotic regulator; At large doses, NO exerts proapoptotic effects, while at low doses, it has antiapoptotic function (Stamler, 1994) (Table 2).
• Anti-tumor effects via signaling cascades
In a gastric cancer model, chemotaxis, survival, and stimulation of neutrophils were modulated by IL6-STAT3-ERK1/2 signaling (Zhu et al., 2014). In a hepatocellular carcinoma model, Li and colleagues observed a remarkable increase in the microvessel density and TGFβ1 mRNA levels, as well as a noticeable reduction in Smad7 mRNA in the subjects treated with MSC. These findings insinuated that MSCs might serve pro-angiogenic effects via the TGFβ1/Smad pathway (Li et al., 2016). Similarly, in a gastric cancer model, TGF-β1 secreted by MSCs stimulated the SMAD2/3 pathway and enhanced tumor growth via the lncRNA MACC1-AS1/miR-145-5p/fatty acid oxidation axis in cancer cells (He et al., 2019). Yuan et al. also discovered that LncRNA H19 contributes to MSC-mediated angiogenesis (Yuan et al., 2019a). Their results pointed to the fact that LncRNA H19 knockdown in MSCs blocked neovascularization by interacting with histone methyltransferase EZH2 and inducing the angiogenesis inhibitor gene VASH1, diminishing angiogenesis factors release, and promoting the formation of angiogenesis inhibitors. Importantly, MSCs can induce the spread of cancerous cells; Breast cancer cells treated with MSCs showed overexpression of oncogenes (NCOA4, FOS), proto-oncogenes (FYN, JUN), and EMT-specific markers, leading to breast cancer metastasis (Martin et al., 2010). MSCs also increase tumor growth by modifying their metabolic state. In lymphoblastic leukemia, MSCs-derived PGE2 activated cAMP-PKA signaling in tumor blasts and blocked the antitumor role of wild-type p53, thus promoting leukaemogenesis (Naderi et al., 2015) (Table 3).
As mentioned before, the role of MSCs in cancer progression is controversial. In vivo and in vitro studies have demonstrated that MSCs have the ability to suppress tumor growth. However, there is robust evidence that confirms the substantial role of MSCs in promoting cancer and metastasis through various pathways (Atiya et al., 2020; Bui et al., 2023). Since decades ago, various therapeutic advantages have been proposed for exogenous MSCs. Application of MSCs in the field of tissue regeneration has shown promising outcomes in the treatment of cardiovascular diseases, stroke, lung disorders, renal failure, rheumatic diseases, neurological disorders, etc. Nevertheless, emerging evidence on the tumorigenic function of MSCs raises concerns about their safety in clinical applications (Galland and Stamenkovic, 2020). As reported in an article, a boy diagnosed with ataxia-telangiectasia who received human fetal neural SCs developed a glioneuronal tumor 4 years after the first SC- injection. Further assessment revealed that the tumor was of non-host origin, implying that the tumor arose from the transplanted neural SCs (Amariglio et al., 2009). The findings of another survey suggested that chronic infection of C57BL/6 mice with Helicobacter triggers repopulation of the stomach with BM-MSCs, which then undergo metaplasia and dysplasia to induce intraepithelial cancer (Houghton et al., 2004). These and other similar reports persuaded scientists to find methods for enhancing the anti-tumor properties of MSCs relative to their tumorigenic activities and converting them into unquestionable therapeutic agents (Liang et al., 2021).
• Genetic modification of MSCs
Tumor specificity is the main barrier to the effectiveness of conventional cancer therapy. MSC’s tendency towards tumor sites improves drug specificity by resolving the issues of stability, dosing, and toxicity related to systemic administration of drugs. This approach has been previously used for the controlled release of anti-cancer agents and has shown promising results when using genetically modified MSC (GM-MSC) against various cancers in animal models (Hagenhoff et al., 2016; Christodoulou et al., 2018). Several studies used GM-MSCs as a tool to transfer and express different onco-suppressive agents such as IFN α and β, IL-2, IL-12, CXCL1, TRAIL, and oncolytic virus. Since GM-MSCs elevate the local concentration of these agents, their anti-tumorigenic function is more effective relative to their function when applied systematically. Furthermore, manipulated MSCs can express certain enzymes that may modify inactive systemically used prodrugs such as ganciclovir into active cytotoxic medications (Liang et al., 2021). von Einem et al. investigated the efficacy of autologous GM-MSC combined with ganciclovir in the treatment of advanced gastrointestinal adenocarcinoma. The results showed that this combination was tolerable and safe which led to clinical stabilization of malignancy and a higher overall survival rate than expected in patients (von Einem et al., 2019). Other studies have similarly reported the therapeutic effectiveness of GM-MSCs against lung, brain, and breast cancers (Christodoulou et al., 2018). By way of illustration, Fei et al. investigated the effects of cytosine deaminase-expressing MSCs in a rat model of C6 glioma. This strategy reduced the tumor volume, propagated tumor cell apoptosis, and improved the survival time (Fei et al., 2012). Gene-directed enzyme/prodrug therapy using adipose MSCs that expressed herpes simplex virus thymidine kinase (TK) demonstrated a great potential for glioblastoma therapy. A group of researchers showed that canine adipose MSCs can be treated with a lentiviral vector to express TK. Combined with ganciclovir, this prodrug exerted antitumor effects on human glioblastoma cell line U87 in a murine model (Villatoro et al., 2022). Adipose SCs were also genetically modified to express recombinant secretory human carboxylesterase-2 and nanoluciferase genes. These cells effectively targeted and localized at tumor stroma and necrotic tissues, and when used together with irinotecan, destroyed all intraperitoneal tumor cells and ameliorated the survival (Malekshah et al., 2019). In another experiment, the combination of the suicide gene CYP2B6TM-RED (a fusion of a triple mutant of CYP2B6 with NADPH cytochrome P450 reductase) and cyclophosphamide showed promising results in treating solid tumors. MSCs as cellular vehicles for the delivery of our suicide genes. MSCs expressing CYP2B6TM-RED could activate cyclophosphamide and eliminate the surrounding tumor cells (Amara et al., 2016).
• Preconditioning with pro-inflammatory cytokines
Macrophages, which can be present as pro-inflammatory M1 and alternatively activated M2 cells, contribute to different inflammatory responses. MSCs can steer monocytes to differentiate into anti-inflammatory M2 phenotypes. Thus, MSCs have the ability to inhibit excessive immune response. MSCs feature immunosuppressive effects that need to be promoted by supportive signals. The immunosuppressive properties of MSCs can be affected by certain pro-inflammatory cytokines IFN-γ, TNF-α, and IL-1α. The stimulation of MSCs by these cytokines is essential for the demonstration of their immunosuppressive behavior (Kim et al., 2018a). Philipp and colleagues found that MSCs preconditioned with IL-1ß and IFN- γ released high amounts of PGE2, NO, and IL-6. Additionally, co-culture with M0 macrophages under the influence of M1 inducers, lipopolysaccharide, and IFN-γ, caused a marked drop of CD86 and iNOS protein in macrophages and reduced TNF-α release. Overall, this method was highly effective in promoting the immunosuppression behavior of MSCs (Philipp et al., 2018). The elevated immunosuppressive activity of MSCs following POLY-IC stimulation that has been observed in some studies is also a promising approach to improve conventional SC-based therapies (Sangiorgi and Panepucci, 2016).
• MSC-extracellular vesicles (MSC-EVs)
Recently, the application of MSC-EVs has been suggested as a potential cell-free therapeutic agent (Galland and Stamenkovic, 2020). EVs are defined as heterogeneous vesicles that act as mediators of intercellular interaction through their loaded proteins, or nucleic acids. Although significantly smaller in size, MSC-derived EVs show most of the features of MSC. MSC-EVs excel in many ways such as in vivo stability and long half-life (Lai et al., 2019). They play a major role in TME communications and akin to MSCs, MSC-EVs may demonstrate both onco-suppressive and protumorigenic activities (Shojaei et al., 2019). Various researchers have proposed that the cell source can condition EV homing to particular sites and that their membrane could be manipulated to elevate tissue-specific targeting. Hence, MSC-EVs can be utilized as biocompatible tools to deliver mRNA, microRNA (miRNA), non-coding RNAs, prodrugs, and peptides to the desired cells (Galland and Stamenkovic, 2020; Guo et al., 2022). By way of illustration, a group of researchers evaluated the application of membrane surface manipulation along with targeting EVs for reinforced uptake in cardiac tissues affected by ischemia via admixture of tissue-targeting antibodies, fluorescent tags, and homing peptide surface cloaks. Their findings showed that EV targeting could be boosted both by a surface display and cloaking (Antes et al., 2018). The activities of MSC-EVs have been investigated in different types of malignant tumors and promising anti-tumorigenic effects have been observed. Del Fattore et al. (Del Fattore et al., 2015) investigated the effects of MSC-EVs on glioblastoma cells. The results showed that UC- and BM-MSC-EVs reduced cell proliferation and increased apoptosis of glioblastoma cells. Some researchers have demonstrated improved efficacy of EVs when applied in combination with gene therapy methods. Gene therapy can be a beneficial method by providing ways to control and correct gene expression. Small interfering RNA (siRNA) and miRNA are among the main molecules applied to trigger gene suppression (Hu et al., 2020). For instance, Dong and colleagues (Dong et al., 2019) enriched human umbilical cord MSCs (UC-MSCs) with siRNA-ELFN1-AS1 and observed that EVs from these treated cells could suppress colon adenocarcinoma cell proliferation and migration in vitro. Kamerkar and co-authors (Kamerkar et al., 2017) evaluated the impact of siRNA carried by exosomes against oncogenic KRAS in human pancreatic cancer. They observed that this strategy remarkably reduced mRNA levels and phosphorylated-ERK protein concentrations in PANC-1 cells. Another group of researchers reported that the administration of anti-miRNA via MSC exosomes targeting glioblastoma multiforme was effective in the restoration of chemosensitivity of multidrug-resistant cells (Munoz et al., 2013). MSC-EVs have also been suggested as an excellent vehicle for drug therapy against malignant cells. As an illustration, the use of UC-MSC-EVs loaded with Vincristine has led to a further increase in cytotoxicity against glioblastoma cells compared with both free drugs and intact EVs (Del Fattore et al., 2015). Hence, the use of EVs seems to be an effective approach to enhance the onco-suppressive effects of MSCs.
• Manipulating the protumorigenic signaling pathways
Therapeutic blockade of signaling cascade molecules involved in tumorigenesis is another approach to suppress protumorigenic activities of MSCs. In this regard, both chemical and herbal products have been suggested to hinder the protumorigenic properties of MSCs. To give an example, MSCs have been shown to induce ovarian carcinoma STAT3 signaling through IL6 and LIF. A group of researchers used Ruxolitinib to target this signaling and observed increased survival in subjects following this therapy (McLean et al., 2019). Recently, the usage of herbal products in the suppression of protumorigenic activities of MSCs has also gained attention. The evaluation of the function of curcumin in adjusting gastric cancer cells-derived MSCs mediated angiogenesis has shown that this product can inhibit angiogenesis via suppressing NF-κB/VEGF signaling (Huang et al., 2017). Treatment with Astragalus polysaccharide, a traditional Chinese herb, has led to a protective impact on morphological changes in BM-MSCs triggered by lung cancer cells (Zhang et al., 2019). Wensheng Zhuanggu Formula inhibits BM-MSC-induced EMT and metastasis in breast cancer via downregulating TGF-β1/Smads signaling (Ma et al., 2020). Similarly, ginseng extract has been shown to inhibit the invasion of colon cancer cells by suppressing ERK1/2 and NF-κB pathways (Kim et al., 2018b)
As mentioned earlier, the influence of MSCs on the tumor milieu is extensive and occasionally paradoxical. The majority of studies that have shown antitumorigenic effects for MSCs have applied MSCs with no previous exposure to cancer cells. This may reflect that cancer-naïve MSCs and cancer-educated MSCs have different functions (Atiya et al., 2020). Moreover, the anti-cancer activity of different types of MSC has been investigated compared to each other. The results have shown that UC-MSCs have significantly higher onco-suppressive effects compared to BM- MSCs and adipose tissue-MSCs (Christodoulou et al., 2018). The complex cellular and molecular interplays between MSCs and the TME can also cause discrepancies in results. MSCs can migrate to tumors and differentiate into various types of cells, including tumor-associated MSCs and tumor-associated fibroblasts (Quante et al., 2011). Neoplasm-derived signals can affect the phenotype of the recruited MSCs, making them a component of tumor tissue; these MSCs carry characteristics that are different from tissue-associated MSCs and BM-MSCs (Zhao et al., 2020). MSCs that have been primed with TLR4 are referred to as MSC1 and display an antitumorigenic effect, while MSCs that have been primed with TLR3 are known as MSC2 and have a tumor-supportive role (Figure 1B) (Waterman et al., 2012). Ruth et al. found that MSC1 was able to suppress tumor progression, while tumor growth and metastasis were promoted by MSC2 both in vivo and in vitro (Waterman et al., 2010). It is interesting to note that the specific TLR agonist that MSCs are exposed to facilitates the shift between MSC1 and MSC2. To further clarify, TLR4 agonists polarize MSCs in the direction of the pro-inflammatory MSC1 population, which is essential for early injury responses. On the other hand, TLR3 agonist exposure would polarize MSCs towards the immunosuppressive MSC2 population, which is essential for facilitating tissue repair. This might partly clarify why MSCs have such a wide function in the treatment of different forms of cancer. Co-culture with MSCs causes more breast, pancreas, and ovarian tumor cell colonies and larger masses compared to untreated controls. MSC2 co-culture leads to the most expanded colonies. On the contrary, co-culture with MSC1 is accompanied by fewer cancer colonies and smaller masses. Overall, these findings insinuate that MSCs and MSC2 promote tumor progression, while MSC1 hurdles tumor cell growth (Waterman et al., 2012). This is partially true of MSC-EVs as well as MSCs. Since MSC-EVs are non-living components, they lack the ability to cause neoplasms. Nevertheless, they may exert an influence on tumor progression. The impact of MSC-EVs on tumor growth is a matter of debate. Some investigations reported that MSC-EVs dampened tumor growth; on the other hand, there are pieces of evidence for the implication of MSC-EV in tumor progression and spread. Interestingly, all of the MSC-EVs used in these surveys also originated from naïve MSCs. Where MSC exosomes synthesized by MYC-transformed MSCs, E1-MYC cells were used, no inhibitory or promoting effects were observed on tumor growth. These controversial findings may be justified by the heterogeneity of MSC sources, different methods used for EV isolation, or discrepancy in tumor models studied (Tan et al., 2021) (Table 4). Further studies may help to explain the intricate interactions between MSCs and tumor components.
MSCs can be considered the major regulators of tissue homeostasis. Evaluating the level of the inflammatory response to injury, MSCs can adapt effective functions to suppress or promote the response. Pro-tumorigenic effects of MSCs are exerted through various mechanisms in the TME, including differentiation into stromal components of the TME, suppression of immune response, enhancement of angiogenesis, improving tumor cell survival, and promotion of metastasis. On the other hand, many studies have suggested that MSCs have the potential to suppress tumor progression via modulation of the immune system, suppression of angiogenesis, induction of apoptosis, and regulation of cellular signaling pathways. Despite the controversy on the role of MSCs in tumor promotion or inhibition, it is obvious that they have a dynamic role in the TME. Considering the wide range of therapeutic applications of MSCs, numerous studies have tried to identify the anti-cancer properties of different types of MSCs. A great body of evidence shows that cancer-naïve MSCs and UC-MSCs have remarkably higher onco-suppressive effects compared with other subtypes. Additionally, some researchers have taken a step further and proposed techniques to convert the tumorigenic function of MSCs into onco-suppressive effects. The existing methods include the application of GM-MSCs, which can help to transfer anti-cancer agents in a highly effective way compared with systemic administration, using MSC-EVs as biocompatible tools to deliver mRNA, miRNAs, prodrugs, and peptides to the target cells, autologous injection of MSCs, which can be administered in combination with prodrugs, therapeutic blockade of cell signaling, and the use of herbal such as curcumin and ginseng. Further studies are suggested to explain the complex interaction between MSCs and tumor components more precisely. Since different subpopulations of MSCs show varied effects, further research should be conducted to evaluate the role of these subpopulations in the progression of different types of cancer which can help to develop more effective methods to convert these unfavorable activities to onco-suppressive effects.
HA: Writing–original draft. GM: Writing–review and editing. AF: Writing–review and editing. MM: Writing–original draft. TK: Supervision, Writing–original draft.
The authors declare that the research was conducted in the absence of any commercial or financial relationships that could be construed as a potential conflict of interest.
All claims expressed in this article are solely those of the authors and do not necessarily represent those of their affiliated organizations, or those of the publisher, the editors and the reviewers. Any product that may be evaluated in this article, or claim that may be made by its manufacturer, is not guaranteed or endorsed by the publisher.
Amara, I., Pramil, E., Senamaud-Beaufort, C., Devillers, A., Macedo, R., Lescaille, G., et al. (2016). Engineered mesenchymal stem cells as vectors in a suicide gene therapy against preclinical murine models for solid tumors. J. Control. Release 239, 82–91. doi:10.1016/j.jconrel.2016.08.019
Amariglio, N., Hirshberg, A., Scheithauer, B. W., Cohen, Y., Loewenthal, R., Trakhtenbrot, L., et al. (2009). Donor-derived brain tumor following neural stem cell transplantation in an ataxia telangiectasia patient. PLoS Med. 6 (2), e1000029. doi:10.1371/journal.pmed.1000029
Antes, T. J., Middleton, R. C., Luther, K. M., Ijichi, T., Peck, K. A., Liu, W. J., et al. (2018). Targeting extracellular vesicles to injured tissue using membrane cloaking and surface display. J. Nanobiotechnology 16 (1), 61. doi:10.1186/s12951-018-0388-4
Arena, S., Salati, M., Sorgentoni, G., Barbisan, F., and Orciani, M. (2018). Characterization of tumor-derived mesenchymal stem cells potentially differentiating into cancer-associated fibroblasts in lung cancer. Clin. Transl. Oncol. 20 (12), 1582–1591. doi:10.1007/s12094-018-1894-4
Asari, S., Itakura, S., Ferreri, K., Liu, C. P., Kuroda, Y., Kandeel, F., et al. (2009). Mesenchymal stem cells suppress B-cell terminal differentiation. Exp. Hematol. 37 (5), 604–615. doi:10.1016/j.exphem.2009.01.005
Atiya, H., Frisbie, L., Pressimone, C., and Coffman, L. (2020). “Mesenchymal stem cells in the tumor microenvironment,” in Tumor microenvironment: Non-hematopoietic cells. Editor A. Birbrair (Cham: Springer International Publishing), 31–42.
Bai, L., Lennon, D. P., Eaton, V., Maier, K., Caplan, A. I., Miller, S. D., et al. (2009). Human bone marrow-derived mesenchymal stem cells induce Th2-polarized immune response and promote endogenous repair in animal models of multiple sclerosis. Glia 57 (11), 1192–1203. doi:10.1002/glia.20841
Battula, V. L., Evans, K. W., Hollier, B. G., Shi, Y., Marini, F. C., Ayyanan, A., et al. (2010). Epithelial-mesenchymal transition-derived cells exhibit multilineage differentiation potential similar to mesenchymal stem cells. Stem cells 28 (8), 1435–1445. doi:10.1002/stem.467
Bergfeld, S. A., and DeClerck, Y. A. (2010). Bone marrow-derived mesenchymal stem cells and the tumor microenvironment. Cancer Metastasis Rev. 29 (2), 249–261. doi:10.1007/s10555-010-9222-7
Bisht, J., Rawat, P., Sehar, U., and Reddy, P. H. (2023). Caregivers with cancer patients: focus on hispanics. Cancers 15 (3), 626. doi:10.3390/cancers15030626
Bonuccelli, G., Avnet, S., Grisendi, G., Salerno, M., Granchi, D., Dominici, M., et al. (2014). Role of mesenchymal stem cells in osteosarcoma and metabolic reprogramming of tumor cells. Oncotarget 5 (17), 7575–7588. doi:10.18632/oncotarget.2243
Brogi, E., Wu, T., Namiki, A., and Isner, J. M. (1994). Indirect angiogenic cytokines upregulate VEGF and bFGF gene expression in vascular smooth muscle cells, whereas hypoxia upregulates VEGF expression only. Circulation 90 (2), 649–652. doi:10.1161/01.cir.90.2.649
Bui, Q. T., Lee, K-D., Fan, Y-C., Lewis, B. S., Deng, L-W., and Tsai, Y-C. (2023). Disruption of CCL2 in mesenchymal stem cells as an anti-tumor approach against prostate cancer. Cancers 15 (2), 441. doi:10.3390/cancers15020441
Burger, J. A., Tsukada, N., Burger, M., Zvaifler, N. J., Dell'Aquila, M., and Kipps, T. J. (2000). Blood-derived nurse-like cells protect chronic lymphocytic leukemia B cells from spontaneous apoptosis through stromal cell-derived factor-1. Blood 96 (8), 2655–2663. doi:10.1182/blood.v96.8.2655.h8002655_2655_2663
Cavarretta, I. T., Altanerova, V., Matuskova, M., Kucerova, L., Culig, Z., and Altaner, C. (2010). Adipose tissue–derived mesenchymal stem cells expressing prodrug-converting enzyme inhibit human prostate tumor growth. Mol. Ther. 18 (1), 223–231. doi:10.1038/mt.2009.237
Chen, B., Ni, Y., Liu, J., Zhang, Y., and Yan, F. (2018). Bone marrow-derived mesenchymal stem cells exert diverse effects on different macrophage subsets. Stem Cells Int. 2018, 8348121. doi:10.1155/2018/8348121
Chen, J., Ji, T., Wu, D., Jiang, S., Zhao, J., Lin, H., et al. (2019). Human mesenchymal stem cells promote tumor growth via MAPK pathway and metastasis by epithelial mesenchymal transition and integrin α5 in hepatocellular carcinoma. Cell. Death Dis. 10 (6), 425. doi:10.1038/s41419-019-1622-1
Christodoulou, I., Goulielmaki, M., Devetzi, M., Panagiotidis, M., Koliakos, G., and Zoumpourlis, V. (2018). Mesenchymal stem cells in preclinical cancer cytotherapy: A systematic review. Stem Cell. Res. Ther. 9 (1), 336–338. doi:10.1186/s13287-018-1078-8
Cousin, B., Ravet, E., Poglio, S., De Toni, F., Bertuzzi, M., Lulka, H., et al. (2009). Adult stromal cells derived from human adipose tissue provoke pancreatic cancer cell death both in vitro and in vivo. PloS one 4 (7), e6278. doi:10.1371/journal.pone.0006278
de Melo, S. M., Bittencourt, S., Ferrazoli, E. G., da Silva, C. S., da Cunha, F. F., da Silva, F. H., et al. (2015). The anti-tumor effects of adipose tissue mesenchymal stem cell transduced with HSV-Tk gene on U-87-driven brain tumor. PLoS One 10 (6), e0128922. doi:10.1371/journal.pone.0128922
Debela, D. T., Muzazu, S. G., Heraro, K. D., Ndalama, M. T., Mesele, B. W., Haile, D. C., et al. (2021). New approaches and procedures for cancer treatment: current perspectives. SAGE Open Med. 9, 20503121211034366. doi:10.1177/20503121211034366
Del Fattore, A., Luciano, R., Saracino, R., Battafarano, G., Rizzo, C., Pascucci, L., et al. (2015). Differential effects of extracellular vesicles secreted by mesenchymal stem cells from different sources on glioblastoma cells. Expert Opin. Biol. Ther. 15 (4), 495–504. doi:10.1517/14712598.2015.997706
Deng, Y., Zhang, Y., Ye, L., Zhang, T., Cheng, J., Chen, G., et al. (2016). Umbilical cord-derived mesenchymal stem cells instruct monocytes towards an IL10-producing phenotype by secreting IL6 and HGF. Sci. Rep. 6 (1), 37566. doi:10.1038/srep37566
Dias, S., Choy, M., Alitalo, K., and Rafii, S. (2002). Vascular endothelial growth factor (VEGF)-C signaling through FLT-4 (VEGFR-3) mediates leukemic cell proliferation, survival, and resistance to chemotherapy. Blood 99 (6), 2179–2184. doi:10.1182/blood.v99.6.2179
Dong, L., Ding, C., Zheng, T., Pu, Y., Liu, J., Zhang, W., et al. (2019). Extracellular vesicles from human umbilical cord mesenchymal stem cells treated with siRNA against ELFN1-AS1 suppress colon adenocarcinoma proliferation and migration. Am. J. Transl. Res. 11 (11), 6989–6999.
Dong, M., How, T., Kirkbride, K. C., Gordon, K. J., Lee, J. D., Hempel, N., et al. (2007). The type III TGF-beta receptor suppresses breast cancer progression. J. Clin. Invest. 117 (1), 206–217. doi:10.1172/JCI29293
Efimenko, A., Starostina, E., Kalinina, N., and Stolzing, A. (2011). Angiogenic properties of aged adipose derived mesenchymal stem cells after hypoxic conditioning. J. Transl. Med. 9, 10. doi:10.1186/1479-5876-9-10
Espona-Fiedler, M., Manuel-Manresa, P., Benítez-García, C., Fontova, P., Quesada, R., Soto-Cerrato, V., et al. (2023). Antimetastatic properties of prodigiosin and the BH3-mimetic obatoclax (GX15-070) in melanoma. Pharmaceutics 15 (1), 97. doi:10.3390/pharmaceutics15010097
Fallarino, F., Grohmann, U., You, S., McGrath, B. C., Cavener, D. R., Vacca, C., et al. (2006). The combined effects of tryptophan starvation and tryptophan catabolites down-regulate T cell receptor zeta-chain and induce a regulatory phenotype in naive T cells. J. Immunol. 176 (11), 6752–6761. doi:10.4049/jimmunol.176.11.6752
Fei, S., Qi, X., Kedong, S., Guangchun, J., Jian, L., and Wei, Q. (2012). The antitumor effect of mesenchymal stem cells transduced with a lentiviral vector expressing cytosine deaminase in a rat glioma model. J. Cancer Res. Clin. Oncol. 138 (2), 347–357. doi:10.1007/s00432-011-1104-z
François, S., Usunier, B., Forgue-Lafitte, M-E., L’Homme, B., Benderitter, M., Douay, L., et al. (2018). Mesenchymal stem cell administration attenuates colon cancer progression by modulating the immune component within the colorectal tumor microenvironment. Stem Cells Transl. Med. 8 (3), 285–300. doi:10.1002/sctm.18-0117
Galland, S., and Stamenkovic, I. (2020). Mesenchymal stromal cells in cancer: A review of their immunomodulatory functions and dual effects on tumor progression. J. pathology 250 (5), 555–572. doi:10.1002/path.5357
Galland, S., Vuille, J., Martin, P., Letovanec, I., Caignard, A., Fregni, G., et al. (2017). Tumor-derived mesenchymal stem cells use distinct mechanisms to block the activity of natural killer cell subsets. Cell. Rep. 20 (12), 2891–2905. doi:10.1016/j.celrep.2017.08.089
Gao, P., Ding, Q., Wu, Z., Jiang, H., and Fang, Z. (2010). Therapeutic potential of human mesenchymal stem cells producing IL-12 in a mouse xenograft model of renal cell carcinoma. Cancer Lett. 290 (2), 157–166. doi:10.1016/j.canlet.2009.08.031
Gu, H., Yan, C., Wan, H., Wu, L., Liu, J., Zhu, Z., et al. (2021). Mesenchymal stem cell-derived exosomes block malignant behaviors of hepatocellular carcinoma stem cells through a lncRNA C5orf66-AS1/microRNA-127-3p/DUSP1/ERK axis. Hum. Cell. 34 (6), 1812–1829. doi:10.1007/s13577-021-00599-9
Guasch, G., Schober, M., Pasolli, H. A., Conn, E. B., Polak, L., and Fuchs, E. (2007). Loss of TGFbeta signaling destabilizes homeostasis and promotes squamous cell carcinomas in stratified epithelia. Cancer Cell. 12 (4), 313–327. doi:10.1016/j.ccr.2007.08.020
Guo, Y., Zhai, Y., Wu, L., Wang, Y., Wu, P., and Xiong, L. (2022). Mesenchymal stem cell-derived extracellular vesicles: pleiotropic impacts on breast cancer occurrence, development, and therapy. Int. J. Mol. Sci. 23 (6), 2927. doi:10.3390/ijms23062927
Hagenhoff, A., Bruns, C. J., Zhao, Y., von Lüttichau, I., Niess, H., Spitzweg, C., et al. (2016). Harnessing mesenchymal stem cell homing as an anticancer therapy. Expert Opin. Biol. Ther. 16 (9), 1079–1092. doi:10.1080/14712598.2016.1196179
Han, I., Yun, M., Kim, E-O., Kim, B., Jung, M-H., and Kim, S-H. (2018). Retraction note: umbilical cord tissue-derived mesenchymal stem cells induce apoptosis in PC-3 prostate cancer cells through activation of JNK and downregulation of PI3K/AKT signaling. Stem Cell. Res. Ther. 9 (1), 354. doi:10.1186/s13287-018-1113-9
Hao, C., Beguinot, F., Condorelli, G., Trencia, A., Van Meir, E. G., Yong, V. W., et al. (2001). Induction and intracellular regulation of tumor necrosis factor-related apoptosis-inducing ligand (TRAIL) mediated apotosis in human malignant glioma cells. Cancer Res. 61 (3), 1162–1170.
He, W., Liang, B., Wang, C., Li, S., Zhao, Y., Huang, Q., et al. (2019). MSC-regulated lncRNA MACC1-AS1 promotes stemness and chemoresistance through fatty acid oxidation in gastric cancer. Oncogene 38 (23), 4637–4654. doi:10.1038/s41388-019-0747-0
Ho, I. A., Toh, H. C., Ng, W. H., Teo, Y. L., Guo, C. M., Hui, K. M., et al. (2013a). Human bone marrow-derived mesenchymal stem cells suppress human glioma growth through inhibition of angiogenesis. Stem Cells 31 (1), 146–155. doi:10.1002/stem.1247
Ho, I. A. W., Toh, H. C., Ng, W. H., Teo, Y. L., Guo, C. M., Hui, K. M., et al. (2013b). Human bone marrow-derived mesenchymal stem cells suppress human glioma growth through inhibition of angiogenesis. Stem Cells 31 (1), 146–155. doi:10.1002/stem.1247
Holan, V., Cechova, K., Zajicova, A., Kossl, J., Hermankova, B., Bohacova, P., et al. (2018). The impact of morphine on the characteristics and function properties of human mesenchymal stem cells. Stem Cell. Rev. Rep. 14 (6), 801–811. doi:10.1007/s12015-018-9843-8
Houghton, J., Stoicov, C., Nomura, S., Rogers, A. B., Carlson, J., Li, H., et al. (2004). Gastric cancer originating from bone marrow-derived cells. Science 306 (5701), 1568–1571. doi:10.1126/science.1099513
Hsu, H-S., Lin, J-H., Hsu, T-W., Su, K., Wang, C-W., Yang, K-Y., et al. (2012). Mesenchymal stem cells enhance lung cancer initiation through activation of IL-6/JAK2/STAT3 pathway. Lung cancer 75 (2), 167–177. doi:10.1016/j.lungcan.2011.07.001
Hu, B., Zhong, L., Weng, Y., Peng, L., Huang, Y., Zhao, Y., et al. (2020). Therapeutic siRNA: state of the art. Signal Transduct. Target. Ther. 5 (1), 101. doi:10.1038/s41392-020-0207-x
Hu, X., Zhou, Y., Dong, K., Sun, Z., Zhao, D., Wang, W., et al. (2014). Programming of the development of tumor-promoting neutrophils by mesenchymal stromal cells. Cell. Physiol. Biochem. 33 (6), 1802–1814. doi:10.1159/000362959
Huang, F., Yao, Y., Wu, J., Liu, Q., Zhang, J., Pu, X., et al. (2017). Curcumin inhibits gastric cancer-derived mesenchymal stem cells mediated angiogenesis by regulating NF-κB/VEGF signaling. Am. J. Transl. Res. 9 (12), 5538–5547.
Hung, S. C., Pochampally, R. R., Chen, S. C., Hsu, S. C., and Prockop, D. J. (2007). Angiogenic effects of human multipotent stromal cell conditioned medium activate the PI3K-Akt pathway in hypoxic endothelial cells to inhibit apoptosis, increase survival, and stimulate angiogenesis. Stem Cells 25 (9), 2363–2370. doi:10.1634/stemcells.2006-0686
Janmohammadi, M., Nazemi, Z., Salehi, A. O. M., Seyfoori, A., John, J. V., Nourbakhsh, M. S., et al. (2023). Cellulose-based composite scaffolds for bone tissue engineering and localized drug delivery. Bioact. Mater. 20, 137–163. doi:10.1016/j.bioactmat.2022.05.018
Jia, Y., Ding, X., Zhou, L., Zhang, L., and Yang, X. (2021). Mesenchymal stem cells-derived exosomal microRNA-139-5p restrains tumorigenesis in bladder cancer by targeting PRC1. Oncogene 40 (2), 246–261. doi:10.1038/s41388-020-01486-7
Jiang, X-X., Zhang, Y., Liu, B., Zhang, S-X., Wu, Y., Yu, X-D., et al. (2005). Human mesenchymal stem cells inhibit differentiation and function of monocyte-derived dendritic cells. Blood 105 (10), 4120–4126. doi:10.1182/blood-2004-02-0586
Kamerkar, S., LeBleu, V. S., Sugimoto, H., Yang, S., Ruivo, C. F., Melo, S. A., et al. (2017). Exosomes facilitate therapeutic targeting of oncogenic KRAS in pancreatic cancer. Nature 546 (7659), 498–503. doi:10.1038/nature22341
Kaplan, R. N., Riba, R. D., Zacharoulis, S., Bramley, A. H., Vincent, L., Costa, C., et al. (2005). VEGFR1-positive haematopoietic bone marrow progenitors initiate the pre-metastatic niche. Nature 438 (7069), 820–827. doi:10.1038/nature04186
Karnoub, A. E., Dash, A. B., Vo, A. P., Sullivan, A., Brooks, M. W., Bell, G. W., et al. (2007). Mesenchymal stem cells within tumour stroma promote breast cancer metastasis. Nature 449 (7162), 557–563. doi:10.1038/nature06188
Khakoo, A. Y., Pati, S., Anderson, S. A., Reid, W., Elshal, M. F., Rovira, , et al. (2006). Human mesenchymal stem cells exert potent antitumorigenic effects in a model of Kaposi's sarcoma. J. Exp. Med. 203 (5), 1235–1247. doi:10.1084/jem.20051921
Kim, D. S., Jang, I. K., Lee, M. W., Ko, Y. J., Lee, D-H., Lee, J. W., et al. (2018a). Enhanced immunosuppressive properties of human mesenchymal stem cells primed by interferon-γ. EBioMedicine 28, 261–273. doi:10.1016/j.ebiom.2018.01.002
Kim, E. J., Kwon, K. A., Lee, Y. E., Kim, J. H., Kim, S-H., and Kim, J. H. (2018b). Korean Red Ginseng extract reduces hypoxia-induced epithelial-mesenchymal transition by repressing NF-κB and ERK1/2 pathways in colon cancer. J. Ginseng Res. 42 (3), 288–297. doi:10.1016/j.jgr.2017.03.008
König, A., Menzel, T., Lynen, S., Wrazel, L., Rosén, A., Al-Katib, A., et al. (1997). Basic fibroblast growth factor (bFGF) upregulates the expression of bcl-2 in B cell chronic lymphocytic leukemia cell lines resulting in delaying apoptosis. Leukemia 11 (2), 258–265. doi:10.1038/sj.leu.2400556
Kucerova, L., Skolekova, S., Matuskova, M., Bohac, M., and Kozovska, Z. (2013). Altered features and increased chemosensitivity of human breast cancer cells mediated by adipose tissue-derived mesenchymal stromal cells. BMC cancer 13, 1–13. doi:10.1186/1471-2407-13-535
Lai, P., Weng, J., Guo, L., Chen, X., and Du, X. (2019). Novel insights into MSC-EVs therapy for immune diseases. Biomark. Res. 7 (1), 6. doi:10.1186/s40364-019-0156-0
Larmonier, N., Ghiringhelli, F., Larmonier, C. B., Moutet, M., Fromentin, A., Baulot, E., et al. (2003). Freshly isolated bone marrow cells induce death of various carcinoma cell lines. Int. J. cancer 107 (5), 747–756. doi:10.1002/ijc.11463
Lathrop, M. J., Sage, E. K., Macura, S. L., Brooks, E. M., Cruz, F., Bonenfant, N. R., et al. (2015). Antitumor effects of TRAIL-expressing mesenchymal stromal cells in a mouse xenograft model of human mesothelioma. Cancer Gene Ther. 22 (1), 44–54. doi:10.1038/cgt.2014.68
Lee, H. J., Ko, J. H., Jeong, H. J., Ko, A. Y., Kim, M. K., Wee, W. R., et al. (2015). Mesenchymal stem/stromal cells protect against autoimmunity via CCL2-dependent recruitment of myeloid-derived suppressor cells. J. Immunol. 194 (8), 3634–3645. doi:10.4049/jimmunol.1402139
Li, G. C., Zhang, H. W., Zhao, Q. C., Sun, L. I., Yang, J. J., Hong, L., et al. (2016). Mesenchymal stem cells promote tumor angiogenesis via the action of transforming growth factor β1. Oncol. Lett. 11 (2), 1089–1094. doi:10.3892/ol.2015.3997
Liang, W., Chen, X., Zhang, S., Fang, J., Chen, M., Xu, Y., et al. (2021). Mesenchymal stem cells as a double-edged sword in tumor growth: focusing on MSC-derived cytokines. Cell. Mol. Biol. Lett. 26 (1), 3–25. doi:10.1186/s11658-020-00246-5
Liu, S., Ginestier, C., Ou, S. J., Clouthier, S. G., Patel, S. H., Monville, F., et al. (2011). Breast cancer stem cells are regulated by mesenchymal stem cells through cytokine networks. Cancer Res. 71 (2), 614–624. doi:10.1158/0008-5472.CAN-10-0538
Liu, X., Hu, J., Sun, S., Li, F., Cao, W., Wang, Y. U., et al. (2015). Mesenchymal stem cells expressing interleukin-18 suppress breast cancer cells in vitro. Exp. Ther. Med. 9 (4), 1192–1200. doi:10.3892/etm.2015.2286
Lu, L., Chen, G., Yang, J., Ma, Z., Yang, Y., Hu, Y., et al. (2019). Bone marrow mesenchymal stem cells suppress growth and promote the apoptosis of glioma U251 cells through downregulation of the PI3K/AKT signaling pathway. Biomed. Pharmacother. 112, 108625. doi:10.1016/j.biopha.2019.108625
Lu, Y., Yuan, Y., Wang, X., Wei, L., Chen, Y., Cong, C., et al. (2008). The growth inhibitory effect of mesenchymal stem cells on tumor cells in vitro and in vivo. Cancer Biol. Ther. 7 (2), 245–251. doi:10.4161/cbt.7.2.5296
Ma, J., Li, J., Wang, Y., Chen, W., Zheng, P., Chen, Y., et al. (2020). WSZG inhibits BMSC-induced EMT and bone metastasis in breast cancer by regulating TGF-β1/Smads signaling. Biomed. Pharmacother. 121, 109617. doi:10.1016/j.biopha.2019.109617
Mahjoor, M., Afkhami, H., Mollaei, M., Nasr, A., Shahriary, S., and Khorrami, S. J. L. S. (2021). MicroRNA-30c delivered by bone marrow-mesenchymal stem cells induced apoptosis and diminished cell invasion in U-251 glioblastoma cell line. Life Sci. 279, 119643. doi:10.1016/j.lfs.2021.119643
Mahjoor, M., Afkhami, H., Najafi, M., Nasr, A., and Khorrami, S. (2022). The role of microRNA-30c in targeting interleukin 6, as an inflammatory cytokine, in the mesenchymal stem cell: A therapeutic approach in colorectal cancer. J. Cancer Res. Clin. Oncol. 149, 1–12. doi:10.1007/s00432-022-04123-w
Mahmoudvand, G., Karimi Rouzbahani, A., Razavi, Z. S., Mahjoor, M., and Afkhami, H. J. FiB. (2023). Mesenchymal stem cell therapy for non-healing diabetic foot ulcer infection: new insight. New insight 11, 1158484. doi:10.3389/fbioe.2023.1158484
Malekshah, O. M., Sarkar, S., Nomani, A., Patel, N., Javidian, P., Goedken, M., et al. (2019). Bioengineered adipose-derived stem cells for targeted enzyme-prodrug therapy of ovarian cancer intraperitoneal metastasis. J. Control. Release 311-312, 273–287. doi:10.1016/j.jconrel.2019.09.006
Martin, F. T., Dwyer, R. M., Kelly, J., Khan, S., Murphy, J. M., Curran, C., et al. (2010). Potential role of mesenchymal stem cells (MSCs) in the breast tumour microenvironment: stimulation of epithelial to mesenchymal transition (EMT). Breast Cancer Res. Treat. 124 (2), 317–326. doi:10.1007/s10549-010-0734-1
McKenna, M. K., Englisch, A., Brenner, B., Smith, T., Hoyos, V., Suzuki, M., et al. (2021). Mesenchymal stromal cell delivery of oncolytic immunotherapy improves CAR-T cell antitumor activity. Mol. Ther. 29 (5), 3529–3533. doi:10.1016/j.ymthe.2021.10.007
McLean, K., Tan, L., Bolland, D. E., Coffman, L. G., Peterson, L. F., Talpaz, M., et al. (2019). Leukemia inhibitory factor functions in parallel with interleukin-6 to promote ovarian cancer growth. Oncogene 38 (9), 1576–1584. doi:10.1038/s41388-018-0523-6
Meisel, R., Zibert, A., Laryea, M., Göbel, U., Däubener, W., and Dilloo, D. (2004). Human bone marrow stromal cells inhibit allogeneic T-cell responses by indoleamine 2,3-dioxygenase-mediated tryptophan degradation. Blood 103 (12), 4619–4621. doi:10.1182/blood-2003-11-3909
Mirshekar, M., Afkhami, H., Razavi, S., Jazi, F. M., Darban-Sarokhalil, D., Ohadi, E., et al. (2023). Potential antibacterial activity and healing effect of topical administration of bone marrow and adipose mesenchymal stem cells encapsulated in collagen-fibrin hydrogel scaffold on full-thickness burn wound infection caused by Pseudomonas aeruginosa. Burns. doi:10.1016/j.burns.2023.01.005
Miyazaki, Y., Oda, T., Mori, N., and Kida, Y. S. (2020). Adipose-derived mesenchymal stem cells differentiate into pancreatic cancer-associated fibroblasts in vitro. FEBS Open Bio 10 (11), 2268–2281. doi:10.1002/2211-5463.12976
Montesinos, J., Flores-Figueroa, E., Castillo-Medina, S., Flores-Guzmán, P., Hernández-Estévez, E., Fajardo-Orduña, G., et al. (2009). Human mesenchymal stromal cells from adult and neonatal sources: comparative analysis of their morphology, immunophenotype, differentiation patterns and neural protein expression. Cytotherapy 11 (2), 163–176. doi:10.1080/14653240802582075
Moslemi, M., Moradi, Y., Dehghanbanadaki, H., Afkhami, H., Khaledi, M., Sedighimehr, N., et al. (2021). The association between ATM variants and risk of breast cancer: A systematic review and meta-analysis. BMC Cancer 21 (1), 27–12. doi:10.1186/s12885-020-07749-6
Munoz, J. L., Bliss, S. A., Greco, S. J., Ramkissoon, S. H., Ligon, K. L., and Rameshwar, P. (2013). Delivery of functional anti-miR-9 by mesenchymal stem cell-derived exosomes to glioblastoma multiforme cells conferred chemosensitivity. Mol. Ther. Nucleic Acids 2 (10), e126. doi:10.1038/mtna.2013.60
Mushahary, D., Spittler, A., Kasper, C., Weber, V., and Charwat, V. (2018). Isolation, cultivation, and characterization of human mesenchymal stem cells. Cytom. Part A 93 (1), 19–31. doi:10.1002/cyto.a.23242
Naderi, E. H., Skah, S., Ugland, H., Myklebost, O., Sandnes, D. L., Torgersen, M. L., et al. (2015). Bone marrow stroma-derived PGE2 protects BCP-ALL cells from DNA damage-induced p53 accumulation and cell death. Mol. Cancer 14 (1), 14. doi:10.1186/s12943-014-0278-9
Nagy, B., Szilberhorn, L., Győrbíró, D. M., Moizs, M., Bajzik, G., Kerpel-Fronius, A., et al. (2023). Shall we screen lung cancer with low-dose computed tomography? Cost-effectiveness in Hungary. Value Health Regional Issues 34, 55–64. doi:10.1016/j.vhri.2022.10.002
Németh, K., Leelahavanichkul, A., Yuen, P. S., Mayer, B., Parmelee, A., Doi, K., et al. (2009). Bone marrow stromal cells attenuate sepsis via prostaglandin E(2)-dependent reprogramming of host macrophages to increase their interleukin-10 production. Nat. Med. 15 (1), 42–49. doi:10.1038/nm.1905
Nilendu, P., Sarode, S. C., Jahagirdar, D., Tandon, I., Patil, S., Sarode, G. S., et al. (2018). Mutual concessions and compromises between stromal cells and cancer cells: driving tumor development and drug resistance. Cell. Oncol. (Dordr) 41 (4), 353–367. doi:10.1007/s13402-018-0388-2
Niu, J., Yue, W., Le-Le, Z., Bin, L., and Hu, X. (2017). Mesenchymal stem cells inhibit T cell activation by releasing TGF-β1 from TGF-β1/GARP complex. Oncotarget 8 (59), 99784–99800. doi:10.18632/oncotarget.21549
Ohkouchi, S., Block, G. J., Katsha, A. M., Kanehira, M., Ebina, M., Kikuchi, T., et al. (2012). Mesenchymal stromal cells protect cancer cells from ROS-induced apoptosis and enhance the warburg effect by secreting STC1. Mol. Ther. 20 (2), 417–423. doi:10.1038/mt.2011.259
Ohlsson, L. B., Varas, L., Kjellman, C., Edvardsen, K., and Lindvall, M. (2003). Mesenchymal progenitor cell-mediated inhibition of tumor growth in vivo and in vitro in gelatin matrix. Exp. Mol. pathology 75 (3), 248–255. doi:10.1016/j.yexmp.2003.06.001
Ong, H-T., Federspiel, M. J., Guo, C. M., Ooi, L. L., Russell, S. J., Peng, K-W., et al. (2013). Systemically delivered measles virus-infected mesenchymal stem cells can evade host immunity to inhibit liver cancer growth. J. Hepatology 59 (5), 999–1006. doi:10.1016/j.jhep.2013.07.010
Otsu, K., Das, S., Houser, S. D., Quadri, S. K., Bhattacharya, S., and Bhattacharya, J. (2009). Concentration-dependent inhibition of angiogenesis by mesenchymal stem cells. J. Am. Soc. Hematol. 113 (18), 4197–4205. doi:10.1182/blood-2008-09-176198
Philipp, D., Suhr, L., Wahlers, T., Choi, Y-H., and Paunel-Görgülü, A. (2018). Preconditioning of bone marrow-derived mesenchymal stem cells highly strengthens their potential to promote IL-6-dependent M2b polarization. Stem Cell. Res. Ther. 9 (1), 286. doi:10.1186/s13287-018-1039-2
Qiao, L., Xu, Z., Zhao, T., Ye, L., and Zhang, X. (2008). Dkk-1 secreted by mesenchymal stem cells inhibits growth of breast cancer cells via depression of Wnt signalling. Cancer Lett. 269 (1), 67–77. doi:10.1016/j.canlet.2008.04.032
Quante, M., Tu, S. P., Tomita, H., Gonda, T., Wang, S. S., Takashi, S., et al. (2011). Bone marrow-derived myofibroblasts contribute to the mesenchymal stem cell niche and promote tumor growth. Cancer Cell. 19 (2), 257–272. doi:10.1016/j.ccr.2011.01.020
Ramdasi, S., Sarang, S., and Viswanathan, C. (2015). Potential of mesenchymal stem cell based application in cancer. Int. J. hematology-oncology stem Cell. Res. 9 (2), 95–103.
Ren, G., Zhao, X., Wang, Y., Zhang, X., Chen, X., Xu, C., et al. (2012). CCR2-Dependent recruitment of macrophages by tumor-educated mesenchymal stromal cells promotes tumor development and is mimicked by TNFα. Cell. Stem Cell. 11 (6), 812–824. doi:10.1016/j.stem.2012.08.013
Rivera-Cruz, C. M., Shearer, J. J., Figueiredo Neto, M., and Figueiredo, M. L. (2017). The immunomodulatory effects of mesenchymal stem cell polarization within the tumor microenvironment niche. Stem Cells Int. 2017, 4015039. doi:10.1155/2017/4015039
Roccaro, A. M., Sacco, A., Maiso, P., Azab, A. K., Tai, Y-T., Reagan, M., et al. (2013). BM mesenchymal stromal cell–derived exosomes facilitate multiple myeloma progression. J. Clin. investigation 123 (4), 1542–1555. doi:10.1172/JCI66517
Roma-Rodrigues, C., Mendes, R., Baptista, P. V., and Fernandes, A. R. (2019). Targeting tumor microenvironment for cancer therapy. Int. J. Mol. Sci. 20 (4), 840. doi:10.3390/ijms20040840
Safari, F., Shakery, T., and Sayadamin, N. (2021). Evaluating the effect of secretome of human amniotic mesenchymal stromal cells on apoptosis induction and epithelial-mesenchymal transition inhibition in LNCaP prostate cancer cells based on 2D and 3D cell culture models. Cell. Biochem. Funct. 39 (6), 813–820. doi:10.1002/cbf.3654
Sainz, B., Carron, E., Vallespinós, M., and Machado, H. L. (2016). Cancer stem cells and macrophages: implications in tumor Biology and therapeutic strategies. Mediat. Inflamm. 2016, 9012369. doi:10.1155/2016/9012369
Sangiorgi, B., and Panepucci, R. A. (2016). Modulation of immunoregulatory properties of mesenchymal stromal cells by toll-like receptors: potential applications on GVHD. Stem Cells Int. 2016, 9434250. doi:10.1155/2016/9434250
Sato, K., Ozaki, K., Oh, I., Meguro, A., Hatanaka, K., Nagai, T., et al. (2007). Nitric oxide plays a critical role in suppression of T-cell proliferation by mesenchymal stem cells. Blood 109 (1), 228–234. doi:10.1182/blood-2006-02-002246
Secchiero, P., Zorzet, S., Tripodo, C., Corallini, F., Melloni, E., Caruso, L., et al. (2010). Human bone marrow mesenchymal stem cells display anti-cancer activity in SCID mice bearing disseminated non-Hodgkin's lymphoma xenografts. PloS one 5 (6), e11140. doi:10.1371/journal.pone.0011140
Shojaei, S., Hashemi, S. M., Ghanbarian, H., Salehi, M., and Mohammadi-Yeganeh, S. (2019). Effect of mesenchymal stem cells-derived exosomes on tumor microenvironment: tumor progression versus tumor suppression. J. Cell. physiology 234 (4), 3394–3409. doi:10.1002/jcp.27326
Sohrabi, E., Moslemi, M., Rezaie, E., Nafissi, N., Khaledi, M., Afkhami, H., et al. (2021). The tissue expression of MCT3, MCT8, and MCT9 genes in women with breast cancer. Genes. Genomics 43 (9), 1065–1077. doi:10.1007/s13258-021-01116-w
Soleymaninejadian, E., Pramanik, K., and Samadian, E. (2012). Immunomodulatory properties of mesenchymal stem cells: cytokines and factors. Am. J. reproductive Immunol. 67 (1), 1–8. doi:10.1111/j.1600-0897.2011.01069.x
Spaggiari, G. M., Abdelrazik, H., Becchetti, F., and Moretta, L. (2009). MSCs inhibit monocyte-derived DC maturation and function by selectively interfering with the generation of immature DCs: central role of MSC-derived prostaglandin E2. Blood 113 (26), 6576–6583. doi:10.1182/blood-2009-02-203943
Spaw, M., Anant, S., and Thomas, S. M. (2017). Stromal contributions to the carcinogenic process. Mol. Carcinog. 56 (4), 1199–1213. doi:10.1002/mc.22583
Stamler, J. S. (1994). Redox signaling: nitrosylation and related target interactions of nitric oxide. Cell. 78 (6), 931–936. doi:10.1016/0092-8674(94)90269-0
Svobodova, E., Krulova, M., Zajicova, A., Pokorna, K., Prochazkova, J., Trosan, P., et al. (2012). The role of mouse mesenchymal stem cells in differentiation of naive T-cells into anti-inflammatory regulatory T-cell or proinflammatory helper T-cell 17 population. Stem cells Dev. 21 (6), 901–910. doi:10.1089/scd.2011.0157
Takeda, K., Hayakawa, Y., Smyth, M. J., Kayagaki, N., Yamaguchi, N., Kakuta, S., et al. (2001). Involvement of tumor necrosis factor-related apoptosis-inducing ligand in surveillance of tumor metastasis by liver natural killer cells. Nat. Med. 7 (1), 94–100. doi:10.1038/83416
Tan, T. T., Lai, R. C., Padmanabhan, J., Sim, W. K., Choo, A. B., and Lim, S. K. (2021). Assessment of tumorigenic potential in mesenchymal-stem/stromal-cell-derived small extracellular vesicles (MSC-sEV). Pharmaceuticals 14 (4), 345. doi:10.3390/ph14040345
Tao, Z., Huang, C., Wang, D., Wang, Q., Gao, Q., Zhang, H., et al. (2023). Lactate induced mesenchymal stem cells activation promotes gastric cancer cells migration and proliferation. Exp. Cell. Res. 424, 113492. doi:10.1016/j.yexcr.2023.113492
Tesi, R. (2019). MDSC; the most important cell you have never heard of. Trends Pharmacol. Sci. 40 (1), 4–7. doi:10.1016/j.tips.2018.10.008
Trivanović, D., Krstić, J., Djordjević, I. O., Mojsilović, S., Santibanez, J. F., Bugarski, D., et al. (2016). The roles of mesenchymal stromal/stem cells in tumor microenvironment associated with inflammation. Mediat. Inflamm. 2016, 7314016. doi:10.1155/2016/7314016
Tu, B., Zhu, J., Liu, S., Wang, L., Fan, Q., Hao, Y., et al. (2016). Mesenchymal stem cells promote osteosarcoma cell survival and drug resistance through activation of STAT3. Oncotarget 7 (30), 48296–48308. doi:10.18632/oncotarget.10219
Ungerer, C., Quade-Lyssy, P., Radeke, H. H., Henschler, R., Königs, C., Köhl, U., et al. (2014). Galectin-9 is a suppressor of T and B cells and predicts the immune modulatory potential of mesenchymal stromal cell preparations. Stem Cells Dev. 23 (7), 755–766. doi:10.1089/scd.2013.0335
Vasandan, A. B., Jahnavi, S., Shashank, C., Prasad, P., Kumar, A., and Prasanna, S. J. (2016). Human Mesenchymal stem cells program macrophage plasticity by altering their metabolic status via a PGE(2)-dependent mechanism. Sci. Rep. 6, 38308. doi:10.1038/srep38308
Villatoro, A. J., Alcoholado, C., Martín-Astorga, MdC., Rubio, N., Blanco, J., Garrido, C. P., et al. (2022). Suicide gene therapy by canine mesenchymal stem cell transduced with thymidine kinase in a u-87 glioblastoma murine model: secretory profile and antitumor activity. PLOS ONE 17 (2), e0264001. doi:10.1371/journal.pone.0264001
von Einem, J. C., Guenther, C., Volk, H. D., Grütz, G., Hirsch, D., Salat, C., et al. (2019). Treatment of advanced gastrointestinal cancer with genetically modified autologous mesenchymal stem cells: results from the phase 1/2 TREAT-ME-1 trial. Int. J. cancer 145 (6), 1538–1546. doi:10.1002/ijc.32230
Wang, Y., He, L., Du, Y., Zhu, P., Huang, G., Luo, J., et al. (2015). The long noncoding RNA lncTCF7 promotes self-renewal of human liver cancer stem cells through activation of Wnt signaling. Cell. Stem Cell. 16 (4), 413–425. doi:10.1016/j.stem.2015.03.003
Waterman, R. S., Henkle, S. L., and Betancourt, A. M. (2012). Mesenchymal stem cell 1 (MSC1)-based therapy attenuates tumor growth whereas MSC2-treatment promotes tumor growth and metastasis. PLoS One 7 (9), e45590. doi:10.1371/journal.pone.0045590
Waterman, R. S., Tomchuck, S. L., Henkle, S. L., and Betancourt, A. M. (2010). A new mesenchymal stem cell (MSC) paradigm: polarization into a pro-inflammatory MSC1 or an immunosuppressive MSC2 phenotype. PloS one 5 (4), e10088. doi:10.1371/journal.pone.0010088
Xiang, J., Tang, J., Song, C., Yang, Z., Hirst, D. G., Zheng, Q. J., et al. (2009). Mesenchymal stem cells as a gene therapy carrier for treatment of fibrosarcoma. Cytotherapy 11 (5), 516–526. doi:10.1080/14653240902960429
Xie, C., Xie, D. Y., Lin, B. L., Zhang, G. L., Wang, P. P., Peng, L., et al. (2013). Interferon-β gene-modified human bone marrow mesenchymal stem cells attenuate hepatocellular carcinoma through inhibiting AKT/FOXO3a pathway. Br. J. Cancer 109 (5), 1198–1205. doi:10.1038/bjc.2013.422
Xu, H., Zhao, G., Zhang, Y., Jiang, H., Wang, W., Zhao, D., et al. (2019). Mesenchymal stem cell-derived exosomal microRNA-133b suppresses glioma progression via Wnt/β-catenin signaling pathway by targeting EZH2. Stem Cell. Res. Ther. 10 (1), 1–14. doi:10.1186/s13287-019-1446-z
Xuan, X., Tian, C., Zhao, M., Sun, Y., and Huang, C. (2021). Mesenchymal stem cells in cancer progression and anticancer therapeutic resistance. Cancer Cell. Int. 21 (1), 595. doi:10.1186/s12935-021-02300-4
Yang, L., Chang, N., Liu, X., Han, Z., Zhu, T., Li, C., et al. (2012). Bone marrow-derived mesenchymal stem cells differentiate to hepatic myofibroblasts by transforming growth factor-β1 via sphingosine kinase/sphingosine 1-phosphate (S1P)/S1P receptor Axis. Am. J. Pathology 181 (1), 85–97. doi:10.1016/j.ajpath.2012.03.014
Yuan, L., Liu, Y., Qu, Y., Liu, L., and Li, H. (2019b). Exosomes derived from MicroRNA-148b-3p-overexpressing human umbilical cord mesenchymal stem cells restrain breast cancer progression. Front. Oncol. 9, 1076. doi:10.3389/fonc.2019.01076
Yuan, S. X., Wang, J., Yang, F., Tao, Q. F., Zhang, J., Wang, L. L., et al. (2016). Long noncoding RNA DANCR increases stemness features of hepatocellular carcinoma by derepression of CTNNB1. Hepatology 63 (2), 499–511. doi:10.1002/hep.27893
Yuan, Z., Bian, Y., Ma, X., Tang, Z., Chen, N., and Shen, M. (2019a). LncRNA H19 knockdown in human amniotic mesenchymal stem cells suppresses angiogenesis by associating with EZH2 and activating vasohibin-1. Stem Cells Dev. 28 (12), 781–790. doi:10.1089/scd.2019.0014
Yüce, M., and Albayrak, E. (2021). Tonsil-derived mesenchymal stem cells inhibit the proliferation of hematological cancer cells through downregulation of IL-6 gene expression under hyperthermia.
Zeng, L., Gowda, B., Ahmed, M. G., Abourehab, M. A., Chen, Z-S., Zhang, C., et al. (2023). Advancements in nanoparticle-based treatment approaches for skin cancer therapy. Mol. Cancer 22 (1), 10–50. doi:10.1186/s12943-022-01708-4
Zhang, T., Lee, Y. W., Rui, Y. F., Cheng, T. Y., Jiang, X. H., and Li, G. (2013a). Bone marrow-derived mesenchymal stem cells promote growth and angiogenesis of breast and prostate tumors. Stem Cell. Res. Ther. 4 (3), 70. doi:10.1186/scrt221
Zhang, T., Lee, Y. W., Rui, Y. F., Cheng, T. Y., Jiang, X. H., and Li, G. (2013b). Bone marrow-derived mesenchymal stem cells promote growth and angiogenesis of breast and prostate tumors. Stem Cell. Res. Ther. 4 (3), 70–15. doi:10.1186/scrt221
Zhang, Y-M., Liu, Y-Q., Liu, D., Zhang, L., Qin, J., Zhang, Z., et al. (2019). The effects of astragalus polysaccharide on bone marrow-derived mesenchymal stem cell proliferation and morphology induced by A549 lung cancer cells. Med. Sci. Monit. Int. Med. J. Exp. Clin. Res. 25, 4110–4121. doi:10.12659/MSM.914219
Zhao, R., Chen, X., Song, H., Bie, Q., and Zhang, B. (2020). Dual role of MSC-derived exosomes in tumor development. Stem Cells Int. 2020, 8844730. doi:10.1155/2020/8844730
Zhao, X., Wu, X., Qian, M., Song, Y., Wu, D., and Zhang, W. (2018). Knockdown of TGF-β1 expression in human umbilical cord mesenchymal stem cells reverts their exosome-mediated EMT promoting effect on lung cancer cells. Cancer Lett. 428, 34–44. doi:10.1016/j.canlet.2018.04.026
Zheng, H., Zou, W., Shen, J., Xu, L., Wang, S., Fu, Y-X., et al. (2016). Opposite effects of coinjection and distant injection of mesenchymal stem cells on breast tumor cell growth. Stem cells Transl. Med. 5 (9), 1216–1228. doi:10.5966/sctm.2015-0300
Keywords: mesenchymal stem cell (MSC), cell-and tissue-based therapy, stem cell transplantation, neoplasm, tumor microenvironment (TME)
Citation: Afkhami H, Mahmoudvand G, Fakouri A, Shadab A, Mahjoor M and Komeili Movahhed T (2023) New insights in application of mesenchymal stem cells therapy in tumor microenvironment: pros and cons. Front. Cell Dev. Biol. 11:1255697. doi: 10.3389/fcell.2023.1255697
Received: 09 July 2023; Accepted: 11 September 2023;
Published: 02 October 2023.
Edited by:
Yuning Hou, Emory University, United StatesReviewed by:
Shuhua Wang, Emory University, United StatesCopyright © 2023 Afkhami, Mahmoudvand, Fakouri, Shadab, Mahjoor and Komeili Movahhed. This is an open-access article distributed under the terms of the Creative Commons Attribution License (CC BY). The use, distribution or reproduction in other forums is permitted, provided the original author(s) and the copyright owner(s) are credited and that the original publication in this journal is cited, in accordance with accepted academic practice. No use, distribution or reproduction is permitted which does not comply with these terms.
*Correspondence: Tahereh Komeili Movahhed, dF9rb21laWxpX21AeWFob28uY29t, dGtvbWVpbGlAbXVxLmFjLmly
†These authors share Co-first authorship
Disclaimer: All claims expressed in this article are solely those of the authors and do not necessarily represent those of their affiliated organizations, or those of the publisher, the editors and the reviewers. Any product that may be evaluated in this article or claim that may be made by its manufacturer is not guaranteed or endorsed by the publisher.
Research integrity at Frontiers
Learn more about the work of our research integrity team to safeguard the quality of each article we publish.