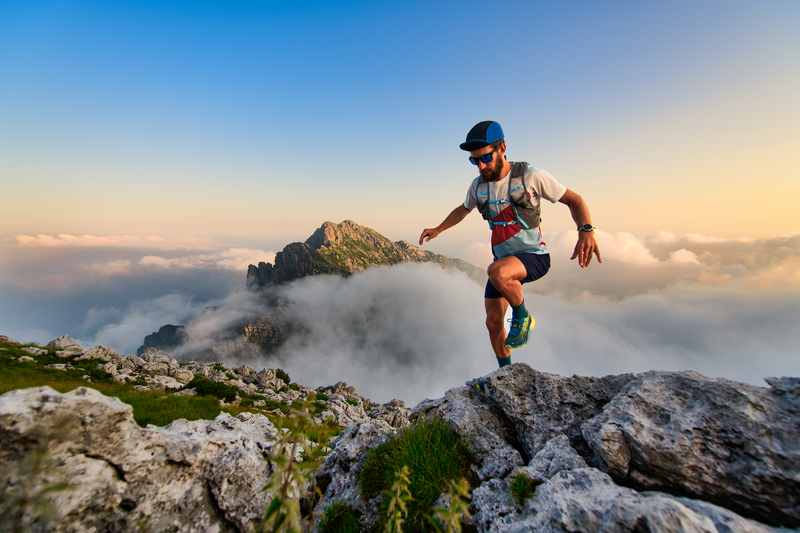
95% of researchers rate our articles as excellent or good
Learn more about the work of our research integrity team to safeguard the quality of each article we publish.
Find out more
REVIEW article
Front. Cell Dev. Biol. , 11 September 2023
Sec. Molecular and Cellular Pathology
Volume 11 - 2023 | https://doi.org/10.3389/fcell.2023.1252318
Mitochondria are intracellular organelles that play a critical role in numerous cellular processes including the regulation of metabolism, cellular stress response, and cell fate. Mitochondria themselves are subject to well-orchestrated regulation in order to maintain organelle and cellular homeostasis. Wound healing is a multifactorial process that involves the stringent regulation of several cell types and cellular processes. In the event of dysregulated wound healing, hard-to-heal chronic wounds form and can place a significant burden on healthcare systems. Importantly, treatment options remain limited owing to the multifactorial nature of chronic wound pathogenesis. One area that has received more attention in recent years is the role of mitochondria in wound healing. With regards to this, current literature has demonstrated an important role for mitochondria in several areas of wound healing and chronic wound pathogenesis including metabolism, apoptosis, and redox signalling. Additionally, the influence of mitochondrial dynamics and mitophagy has also been investigated. However, few studies have utilised patient tissue when studying mitochondria in wound healing, instead using various animal models. In this review we dissect the current knowledge of the role of mitochondria in wound healing and discuss how future research can potentially aid in the progression of wound healing research.
Wound healing is a complex and conserved process consisting of four concurrent and overlapping phases: 1) haemostasis, 2) immune response/inflammation, 3) proliferation, and 4) tissue remodelling (Velnar and Bailey, 2009), with the end goal to restore tissue integrity and homeostasis. In the event of disruption in any of four phases individually or collectively, this highly regulated process becomes dysfunctional and can subsequently lead to the formation of chronic wounds (Falanga et al., 2022). These chronic wounds are defined as a wound that does not heal within 3 months, does not heal in the orderly phases of wound healing, or does not heal after 4 weeks of treatment (Atkin et al., 2019). Chronic wounds place an enormous burden on the healthcare system, accounting for approximately 2%–4% of healthcare budgets worldwide (Olsson et al., 2019). Therefore, a greater understanding of the pathogenesis of chronic wounds and dysfunction in the wound healing process that underpins them are essential in order to improve their treatment. Additionally, due to the fact that the wound healing cycle shares similarities to healing in other tissues such as muscle (three stages of inflammation, proliferation, and remodelling) (Ciciliot and Schiaffino, 2010), liver (three stages of priming, proliferation, and termination) (Tao et al., 2017), and bone (four stages of hematoma, fibrocartilaginous callus and bony callus, as well as remodelling) (Ghiasi et al., 2017), it could be hypothesised that a better understanding of wound healing pathophysiology may also benefit research in other diseases.
Wound healing is a highly metabolically demanding process, and mitochondria play a significant role in the wound healing cycle. Mitochondria are organelles that exist in large numbers within eukaryotic cells, having originated as α-proteobacterium which became incorporated into an archaeal cell via endocytosis nearly 2 billion years ago (Sicheritz-Pontén and Andersson, 1998; Margulis et al., 2006). Human mitochondria contain their own double-stranded genome (mtDNA) roughly 16.5 kbp in size, which encodes 13 polypeptides, 22 transfer RNAs (tRNA), and two ribosomal RNAs (rRNAs). The 13 polypeptides encoded by mtDNA collectively make up a portion of the subunits of the electron transport chain (ETC). In addition to mtDNA being essential for the function of mitochondria, roughly 1,500 nuclear-encoded (nDNA) polypeptides contribute to the mitochondrial proteome (Pfanner et al., 2019). These include mtDNA and RNA polymerases, transcription factors, ribosomal proteins, and enzymes for the TCA cycle and nucleic acids, among others (Pfanner et al., 2019; Stewart and Chinnery, 2021).
Mitochondria are well known as being the cellular hubs for the generation and storage of energy in the form of adenosine triphosphate (ATP) (Spinelli and Haigis, 2018), however they are also responsible for several other key cellular processes including stress response, immunity, redox state, calcium (Ca2+) homeostasis, and cell fate (Shen et al., 2022). In all cases, communication between mitochondria and the nucleus is essential, in what is known as mito-nuclear crosstalk or retrograde signalling (Cardamone et al., 2018; Kim et al., 2018). In this important feedback mechanism, the mitochondria sense stress signals and relay them to the nucleus, whereby the nucleus responds by activating one or more stress response pathways (Picard and Shirihai, 2022). This can lead to alterations in mitochondrial structure and function, allowing mitochondria to adapt to what is required in order to maintain mitochondrial homeostasis. One important aspect of this is changes to mitochondrial shape (mitochondrial dynamics) and number (mitochondrial biogenesis and mitophagy) (Ma et al., 2020). In the context of wound healing, all these cellular processes are important for normal functioning, as is highlighted by a range of literature in the field and reports of mitochondrial involvement in various skin pathologies with wound healing-related characteristics (Table 1). However, in this review, we will focus on what is known about the role of mitochondria in the regulation of metabolism in wound healing, redox signalling, mitochondrial control of apoptosis, and the underlying regulation of mitochondrial homeostasis through mitochondrial dynamics and mitophagy.
As previously mentioned, wound healing is a highly dynamic process involving the four sequential and overlapping phases of haemostasis (0-several hours following wounding), inflammation (1–3 days), proliferation (4–21 days), and tissue repair (21 days–1 year) (Reinke and Sorg, 2012) (Figure 1).
FIGURE 1. Stages of the wound healing cycle. Wound healing consists of four concurrent and overlapping stages. These include haemostasis (occurring 0 to several hours after wounding), inflammation (from 1 to 3 days post wounding), proliferation (from 4 to 21 days following wounding), and tissue repair (occurring roughly 21 days up to a year post wounding). During the haemostasis phase, inflammatory cells, growth factors and fibrin accumulate in and around the wound bed, and induce clot formation and vasoconstriction. In the inflammatory phase, several other immune cells are recruited and act as immune effector cells as well as producing growth factors. Next, in the proliferative phase, re-epithelialisation of the wound occurs, as well as formation and expansion of vasculature and granulation tissue. Finally, in the remodelling phase, contraction of the wound occurs and scar tissue is formed.
In the haemostasis phase, which occurs immediately after wounding, platelets are recruited to the wound site and aggregate, leading to clot formation and vasoconstriction. Platelets here require mitochondria for both activation and metabolic activities (Baccarelli and Byun, 2015; Melchinger et al., 2019). This subsequently induces hypoxia, with the resulting effects of pH changes and increased glycolysis (Martin, 1997; Eming et al., 2007). Following degranulation of platelets, the complement cascade is activated, leading to the stimulation of inflammatory cells and the beginning of the inflammatory phase (Sinno and Prakash, 2013). Here, in response to the hypoxic environment of the wound whereby proinflammatory mediators and damage associated molecular pattern molecules (DAMPs) are released, local immune cells are activated. Of these, leukocytic infiltration is amongst the first action (Ross and Odland, 1968). Additionally, circulating monocytes are recruited and differentiate into mature wound macrophages (Ross and Odland, 1968), as well as mast cells being recruited to the wound site from adjacent tissue (Artuc et al., 1999). In the later stages of the inflammatory phase, B and T lymphocytes are then recruited to the wound site, where they produce various cytokines and growth factors, as well as acting as immune effector cells (Barbul et al., 1989; Gillitzer and Goebeler, 2001). Leukocytes, in particular neutrophils, as well as macrophages, are responsible for clearing the wound of bacteria and pathogens. Additionally, the macrophages produce growth factors responsible for activating cells involved in the proliferation stage, such as local keratinocytes, fibroblasts, and endothelial cells, while the neutrophils are cleared by apoptosis (Ramasastry, 2005; Velnar and Bailey, 2009; Holzer-Geissler et al., 2022).
During the next phase of wound healing, proliferation, re-epithelialisation occurs. This is in addition to formation of granulation tissue and neoangiogenesis. With regards to the formation of granular tissue, collagen bundles containing fibroblasts, granulocytes, blood vessels, and macrophages create a new structure to replace the matrix that was generated during haemostasis (Schultz and Wysocki, 2009). Importantly, the primary drivers are fibroblasts. Here, fibroblasts which proliferate and migrate from the dermis produce proteinases in order to degrade the existing matrix (Hinz et al., 2007). In addition, they deposit extracellular matrix (ECM) components such as collagen, hyaluronic acid, fibronectin, and proteoglycans. These components provide a scaffold for other processes of wound healing (Barker, 2011; Xue and Jackson, 2015; Eckes et al., 2023). During re-epithelialisation, keratinocytes at the basal layer of the wound edge begin proliferating and migrating until contact, whereby new adhesion structures are formed. At this point, the keratinocytes begin to form a new basement membrane (Jacinto et al., 2001). Alongside re-epithelialisation, angiogenesis is triggered by growth factors such as vascular endothelial growth factor (VEGF). These growth factors trigger the recruitment of endothelial cells from existing vessels, where they proliferate and migrate towards the angiogenic stimulus (Li et al., 2003).
Finally, in the remodelling phase, which coincides with the end of the proliferation phase and cessation of epidermal keratinocyte migration, wound tension and contraction is driven by fibroblasts which differentiate into myofibroblasts and convert collagen III into collagen I (Hinz et al., 2007). Here, complete resolution of wound inflammation occurs, and in an attempt to recover the normal structure of tissue, granulation tissue is remodelled alongside the cell death, primarily through apoptosis, of remaining fibroblasts to form scar tissue. This new tissue contains more, and thicker, collagen than in normal tissue, and is both less vascular and less cellular than the previous tissue (Gonçalves et al., 2010; Gonzalez et al., 2016).
Chronic wounds are the pathological result of partial, superficial, or full-thickness wounds failing to heal. Chronic wounds are heterogeneous with regard to several factors, such as pathophysiology, aetiology, and body location. In terms of pathogenesis, although there is no one factor that causes chronic wounds in the whole population, several factors are known to be significantly implicated. These include diabetes, neuropathies, genetic factors, arterial and venous insufficiency, certain medications, or ageing (Falanga et al., 2022). Because the wound healing process is multifactorial and highly coordinated, any disturbance in the process can lead to a failure to heal. One example where this occurs is in the inflammatory phase. Here, either a significantly enhanced inflammatory response, whereby overstimulation by immune and non-immune cells can lead to prolonged tissue inflammation (Hesketh et al., 2017; Shook et al., 2018; Januszyk et al., 2020; Kirchner et al., 2020), or an abnormal response, whereby immune cells are unable to clear invading pathogens (Falanga et al., 2022), can be common a pathophysiological cause. With regards to the tissue repair phase, dysfunction in basal cells as well as immune cells can prevent angiogenesis and re-epithelialisation (Lucas et al., 2010; Shook et al., 2016). In addition, disturbed vascularisation and angiogenesis can cause a prolonged hypoxic microenvironment which leads to excess cell death and potentially necrosis (Krisp et al., 2013). Cell proliferation and migration can also be impaired and lead to chronic wound formation due to insufficient re-epithelialisation (Makrantonaki et al., 2017a; Falanga et al., 2022).
Due to the fact that the pathophysiology of chronic wounds is so complex and multifactorial, and that effective treatment needs to be based on a multidisciplinary approach, the effort to improve knowledge in the field has proved difficult. Although several preclinical in vivo models of wound healing exist, such as pigs and mice, they do not display exactly the same pathophysiological mechanisms as humans (Buck et al., 2011; Dhall et al., 2014; Seaton et al., 2015; Fadini et al., 2016; Olson and Nechiporuk, 2018). A key example being that rodents predominantly heal through contraction, as opposed to re-epithelialisation (Dunn et al., 2013). Additionally, although similarities exist in invertebrate models such as Caenorhabditis elegans or Drosophila, for example, innate immune system activation, the lack of organism complexity and skin structure diversity to humans means that important processes such as angiogenesis or cell division cannot be accurately studied (Eming et al., 2014). Another point to note is that processes such as mitochondrial fragmentation or mitochondrial Ca2+ uptake occur at a much faster rate in these invertebrates than in humans (Ma et al., 2021). However, advancements in technologies such as improved single cell transcriptomics have allowed for more effective studies into the field, such as those studying the role of immunity (Theocharidis et al., 2022). In order to gain a more comprehensive understanding and allow for the advancement of more targeted treatments and preventions of chronic wounds, further research into specific areas of significance in the wound healing pathophysiology paradigm are needed. One such area is the role of mitochondria in wound healing. As such, in this review we will discuss in detail the current knowledge in this field and how it could be targeted in the future.
Due to the complex multifactorial nature of wound healing–whereby many cellular processes such as regeneration of tissue (Rousselle et al., 2019), immune response (Hall et al., 2014; Willenborg et al., 2020), replenishment of intracellular contents and phospholipid membranes (Xu and Huang, 2020), as well as proliferation and migration of various cell types (Onida et al., 2019), require vast amounts of energy–wound healing is a highly metabolically demanding process.
As previously mentioned, mitochondria are the principle suppliers of energy in the cell. They produce ATP as well as metabolic precursors. In addition, mitochondria also generate metabolic by-products essential for signalling and cellular homeostasis such as ammonia and reactive oxygen species (ROS) (Spinelli and Haigis, 2018).
The most widely known process by which mitochondria supply energy is through the metabolism of nutrients into ATP via the TCA cycle and ETC (Watt et al., 2010; Walsh et al., 2017). In addition to ATP, various other metabolites are produced by mitochondria. These include pyruvate, glutamine, branch-chain amino acids, and fatty acids (FAs). Pyruvate is produced in the cytosol via glycolysis in normoxic conditions and shuttled through the inner mitochondrial membrane (IMM) into mitochondria. However, during hypoxia, pyruvate picks up electrons and generates lactate in the cytosol (Papandreou et al., 2006). Branched-chain amino acids are sources of ATP for cells such as myocytes and adipocytes during differentiation (Green et al., 2016; Shimomura et al., 2023). FAs, such as palmitate, are significant sources of energy for cellular function, particularly in nutrient stress conditions (Rohrig and Schulze, 2016).
An alternative form of energy production in cells is through the process of aerobic glycolysis, which is advantageous for proliferating cells as well as cells in hypoxic or tumour environments where the availability of amino acids, FAs, and nucleotides is more important than that of ATP (Li et al., 2014). During glycolysis, glucose is converted into pyruvate in the cytoplasm, producing ATP (Li et al., 2014). In addition to aerobic glycolysis, glutamine is converted into glutamate, lactate, and ammonia by glutaminase (GLS) in a process termed glutaminolysis (Divakaruni et al., 2013; Schell et al., 2014). This process is critical in order to maintain the energy requirements of proliferating cells (DeBerardinis, 2007; Wise et al., 2008; Wang, 2011a).
As stated above, mitochondria also act as hubs for the generation and storage of other biometabolic molecules. One example is that mitochondria generate citrate as a TCA cycle intermediate. Citrate is important as a regulator of anaerobic respiration by acting as a carbon source (Hatzivassiliou et al., 2005) and is involved in the growth and proliferation of various cell types (Ben-Sahra et al., 2016; Ron-Harel et al., 2016).
In general, wound healing is associated with a switch in metabolism towards anaerobic metabolism, due to the hypoxic nature of the wound milieu (Mannello et al., 2014; Eming et al., 2021) (Figure 2). A recent paper by our lab assessed the metabolic profile of wounding in human skin. In it, it was confirmed that glycolysis, and to a lesser extent glutaminolysis, are vital for the normal regulation of wound healing, and that defects in these pathways may be a pathogenic factor in chronic wound formation (Manchanda et al., 2023). In this study it was demonstrated using various techniques such as metabolomics and transcriptomics that there is an upregulation of glycolysis, glutaminolysis, the TCA cycle, and β-oxidation in both acute and chronic wound skin samples. In concert with this, levels of mtDNA, encoding oxidative phosphorylation (OXPHOS) subunits, and nDNA genes involved in fatty acid oxidation, were significantly downregulated in the wounded samples compared to intact skin (Manchanda et al., 2023). Additionally, explant work further demonstrated the significance of upregulated glycolysis and glutaminolysis in efficient wound healing and in particular, the proliferation and migration of fibroblasts and keratinocytes.
FIGURE 2. Metabolism in wound healing. During the early stages of wound healing, primarily the haemostasis and inflammation stages, glycolytic metabolism is upregulated, and there is a simultaneous downregulation of oxidative metabolism. Later, during the proliferation and remodelling stages, there is a switch from glycolytic metabolism (blue bar) towards more oxidative metabolism (yellow bar).
This paper has built upon previous studies which demonstrated greater glucose utilisation and lactate production in both burn patients and animal models (Im and Hoopes, 1970; Vinaik et al., 2020). In another recent study which investigated the differences of cells involved in wound healing at the single-cell level in wounded mice, the cells in the neo-epidermis 4 days post wounding exhibited a high glycolysis state, compared to unwounded cells which exhibited a low glycolysis state (Haensel et al., 2020). Accordingly, basal cells in unwounded skin showed the highest levels for OXPHOS, whereas the cells in the wounded skin had significantly lower levels of OXPHOS. Additionally, wound edge keratinocytes with increased migration and differentiation have been shown to have elevated levels of the polyamine spermine, an endogenous polyamine metabolite which increases cell proliferation and differentiation through DNA synthesis (Zhan et al., 2015; Lim et al., 2018; Ito et al., 2021). Polyamines were also found to be elevated in chronic wound samples in the recent Manchanda et al. study (Manchanda et al., 2023). Other studies have also demonstrated the beneficial effects of inhibiting OXPHOS on wound healing (Chen et al., 2003; Ved et al., 2005; Xu and Chisholm, 2014).
With regards to the inflammatory phase of wound healing, previous studies have demonstrated alterations in epidermal cell metabolism in the immune response (Hall et al., 2014; Willenborg et al., 2020). Recent studies have examined the differences between the two types of macrophages involved in wound healing. Here it was shown that type-1 macrophages, primarily involved in the inflammatory phase and so with antimicrobial functions and proinflammatory actions, utilised glycolysis (Eming et al., 2017; Willenborg et al., 2020). Conversely, M2 macrophages that are involved in the later phases of wound healing utilised OXPHOS. They also found that these macrophages had signatures for anabolic metabolism. Due to the distinct differences in metabolic signature between and M1 and M2 macrophages, metabolic reprogramming is of vital significance, and disturbances in this metabolic reprogramming may affect the proper transition of macrophage subtype (Mills and O'Neill, 2016). Studies have also provided evidence that there is a specific composition of lipids required in the inflammatory stage (Wijesinghe et al., 2014; Wijesinghe et al., 2016; Yasukawa et al., 2020), and so it could be hypothesised that fatty acid oxidation plays an important role in wound healing.
As with the upregulation of glycolysis required for the proinflammatory effects of the M1 macrophages involved in the inflammatory phase, these macrophages seemingly also utilise glycolysis in order to promote angiogenesis (Willenborg et al., 2020). Metabolism is also critically involved in endothelial cell function in angiogenesis (De Bock et al., 2013; Schoors et al., 2015; Kim et al., 2017; Eelen et al., 2018; Diebold et al., 2019). Here, OXPHOS, which is upregulated in the latter stages of wound healing, is required for efficient migration and proliferation of endothelial cells and therefore angiogenesis, which also occurs in the more latter stages of wound healing (Vandekeere et al., 2018; Schiffmann et al., 2020).
Another process that metabolism is important in wound healing for is in matrix remodelling. Previously, glucose treatment was found to accelerate the formation of collagen and therefore re-epithelialisation in ex vivo wounded skin, while collagen genes were upregulated in 2-Deoxy-D-glucose (2-DG) treated fibroblasts (Manchanda et al., 2023). In a separate study, L-glutamine treatment was shown to enhance the rate of collagen biosynthesis and re-epithelialisation in a rat incision wound model (Goswami et al., 2016). Thus, modulating metabolism may be a potential target for treating chronic wounds.
Due to the fact that metabolism plays such an important role in wound healing, it is of no surprise that defects in the regulation of metabolism may be involved in the pathogenesis of chronic wounds. One possible mechanism may be the long-term upregulation of lipids derived from free FAs even after the normal course of wound healing has finished, thereby prolonging inflammation in the wound (Yasukawa et al., 2020; Manchanda et al., 2023). As mentioned earlier, this is significant, as prolonged inflammation can render wound healing stuck in the inflammatory stage. Prolonged inflammation may also arise in chronic wounds by the elevated levels of polyamines (Zhan et al., 2015; Lim et al., 2018).
The reports detailed in this section offer a reasoning to the hypothesis that dysregulated metabolism may be important in the formation of chronic wounds, and therefore that methods to target this dysfunction may be a viable treatment option for chronic wounds. Some examples of this include evidence of topical insulin treatment increasing glucose availability in a type II diabetic ischemic rat wound model (Yu et al., 2017), as well as honey acting as a beneficial fuel for glycolytic metabolism in wound healing (Sumitra et al., 2009). However, in the latter example, it should also be stated that other components of honey may also contribute to the beneficial wound healing effect.
Mitochondria are the primary producers of reactive oxygen species (mtROS) and of redox signalling in the cell, producing roughly 90% of ROS (Balaban et al., 2005a). As a consequence of proton transfer during OXPHOS, electrons leak from the ETC, complexes I, II, III, and IV. This results in the production of superoxide (Quinlan et al., 2013). Superoxide is then converted into hydrogen peroxide (H2O2) by superoxide dismutases. Subsequently, H2O2 is detoxified, generating water and oxygen (Shadel and Horvath, 2015). Importantly, following diffusion through the mitochondrial membranes, H2O2 that is not detoxified is then responsible for regulating redox signalling (Shadel and Horvath, 2015).
As previously mentioned, the wound milieu is hypoxic due to the disruption of vasculature around the wound and subsequent decrease in oxygen supply (Haensel et al., 2020). As such, there is a significant decrease in oxidative metabolism, and an upregulation in aerobic glycolysis (Im and Hoopes, 1970; Haensel et al., 2020). The result of this is the formation of a microenvironment whereby metabolic intermediates required for cell proliferation and migration such as lactate are more readily available (Vinaik et al., 2020). Hypoxia is known to induce the stabilisation of HIF-1α and the upregulation of mtROS (Chandel et al., 1998). Together these lead to the downstream activation of AMP-activated protein kinase (AMPK), mitogen-activated protein kinase (MAPK), and nuclear factor erythroid-derived 2-like 2 (Nrf2) pathways, which are critically involved in apoptosis (see Section 5), differentiation, motility, and proliferation of cells (Lamb et al., 2003; Lu and Xu, 2006; Kim and Choi, 2015; Atay and Skotheim, 2017). However, studies have indicated that the concentration of H2O2 in the wound is between 100 and 250 μM, and the various processes of wound healing require precise levels of ROS. As such, imbalances in the ROS equilibrium either way can be deleterious, and often lead to the formation of chronic wounds (Polaka et al., 2022).
mtROS production at the early stages of wound healing has pleiotropic effects, and is critical for the efficiency of the wound healing process (Gauron et al., 2013; Love et al., 2013) (Figure 3). Importantly though, whilst low levels of ROS are essential in wound healing, excessive production of ROS can lead to oxidative damage and impaired wound healing (Cano Sanchez et al., 2018). One beneficial example of mtROS is that of H2O2, which recruits inflammatory cells such as phagocytes to the wound site (Niethammer et al., 2009a; West et al., 2011; Yoo et al., 2011; Pase et al., 2012; Razzell et al., 2013; van der Vliet and Janssen-Heininger, 2014). In the recent Willenborg et al. paper, which profiled early and late wound stage macrophages in mice, it was confirmed that macrophages involved in the early events of wound healing have high levels of mtROS production, high expression of mtROS detoxification genes, high levels of succinate metabolism, and subsequently high HIF-1α stabilisation, coinciding with upregulated glycolysis (Willenborg et al., 2020). In addition to its role in the recruitment of immune cells in wound healing, mtROS such as H2O2 also aid in antimicrobial mechanisms of immune cells (Cao et al., 2022). mtROS also play an important role in the signalling, survival, and cytokine production of immune cells, which are essential functions required to prevent infections and therefore prolonged inflammation (Allaoui et al., 2009; Segal et al., 2012; van der Vliet and Janssen-Heininger, 2014; Dunnill et al., 2017; Hoffmann and Griffiths, 2018).
FIGURE 3. Mitochondrial redox signalling in wound healing. Following wounding, a burst of intracellular Ca2+ is released as the initial damage signal, and is detected by mitochondria. Among several subsequent actions, increase in mitochondrial Ca2+ can lead to the increased leakage of ROS from the ETC. After conversion of superoxide (O2−.) into H2O2, the H2O2 is translocated out of the mitochondria though its membrane pores including VDAC, and into the cytosol. Here, this H2O2 can have several effects that contribute to the regulation of wound healing, including involvement in the migration of immune and non-immune cells, as well as antimicrobial activities of immune cells, accelerating re-epithelialisation, and regulating platelet infiltration in the initial haemostasis phase and later, accelerating the migration of endothelial cells involved in angiogenesis.
mtROS have also been shown to be important in migration and adhesion of non-immune cells during both development and wound healing in various in vitro models (Chiarugi et al., 2003; Kwon et al., 2016; Weidong P et al., 2022). During cell migration, cells require signals which trigger the polarisation and extension of protrusions in the direction of chemoattractants, of which H2O2 is a key one (Klyubin et al., 1996; Hurd et al., 2012). Previous studies using cell and mouse models have demonstrated the beneficial effect of hypoxia and production of mtROS on keratinocyte motility and migration via p38 and JNK/MAPK signalling during wound healing (O’Toole et al., 2008; Rodriguez et al., 2008; Zhang et al., 2019). With regards to dermal fibroblasts, hypoxic conditions significantly enhance their proliferation as well as their differentiation into myofibroblasts, as shown in previous studies on various disease rodent models and cells (Falanga and Kirsner, 1993; Sen and Roy, 2010; Janda et al., 2016; Levigne et al., 2016; Hoffmann and Griffiths, 2018).
During re-epithelialisation of skin during wound repair, several proteins act to aid in keratinocyte migration and proliferating, as well as the formation and regulation of the cytoskeleton. These include F-actin, myosin, and E-cadherin (Rousselle et al., 2019). Importantly, studies have shown that mtROS promotes wound healing by regulating F-actin and myosin at the wound edge (Xu and Chisholm, 2011; Muliyil and Narasimha, 2014; Xu and Chisholm, 2014; Hunter et al., 2018; Fung et al., 2023). Of note, in the later Xu and Chisholm study they demonstrated that wounding in C. elegans induced a rapid release of Ca2+ around the wound site and uptake into mitochondria, subsequently inducing mtROS production. This mtROS then inhibits RHO-1 activity via oxidation of cysteine residues (Lambeth and Neish, 2014) to enhance F-actin build up and accelerate wound closure (Xu and Chisholm, 2014). The more recent study by Hunter et al. confirmed the key role of mtROS in the promotion of E-cadherin trafficking as well as junction and cytoskeleton polarisation in wound closure. Again, it was shown that Ca2+ signals released immediately after wounding induced a greater production of mtROS, leading to cytoskeleton remodelling mediated by redox signalling (Hunter et al., 2018).
Angiogenic factors are released into the wound bed immediately following wounding and granulation tissue begins to form between day three and five post wounding, allowing for the formation of new blood vessels via angiogenesis, as well as fibroblast proliferation, and collagen production (Tonnesen et al., 2000). Along with NADPH oxidase (NOX), mtROS play an important role in angiogenesis in the wound healing response (Knighton et al., 1983; Guo et al., 2010; Schreml et al., 2010; Aldosari et al., 2018). In addition, mtROS-sensitive pathways mentioned previously, such as the HIF-1α, NFκB, MAPK, JAK, PI3 kinase, and Akt pathways are involved in angiogenesis in various other tissues (Chandel et al., 2000; Guzy et al., 2005; Klimova and Chandel, 2008; Fukai and Ushio-Fukai, 2020).
In the early stages of wound healing, mtROS induce the recruitment of platelets and other immune cells to the wound site (Hoffmann and Griffiths, 2018). mtROS is also significantly involved in the migration of endothelial cells in wound healing. In particular, mtROS promote endothelial cell migration as a downstream effect of VEGF (Wang et al., 2011b). In both these instances, the effects of mtROS are dependant on wounding-induced hypoxia, and although these studies show a positive correlation, in some instances it is difficult to uncouple the role of mtROS from hypoxia. Although there are few studies describing the specific role of mtROS in angiogenesis, current thinking supports the suggestion that mtROS is critical agent in angiogenesis occurring during wound healing.
Wound healing is recognised to be delayed in aged skin due to a multitude of factors (Ding et al., 2021). Importantly, aged skin is structurally different to younger skin, characterised by having decreased keratinocyte turnover (Biniek, 2015), impaired cellular plasticity (Ocampo et al., 2016), decreased fibroblast density (Salzer et al., 2018), less efficient collagen maturation as well as organisation (Kobayashi et al., 2019), and thinner vasculature (Bentov and Reed, 2015). Immune responses and immune cell abundance (Nishio et al., 2008; Vu et al., 2022) as well as keratinocyte dynamics (Keyes et al., 2016) are also dysregulated in wound healing in aged skin. In addition, inefficient metabolism was found to contribute to delayed wound healing in aged mice (Jones et al., 2020), and in a separate study macrophage metabolism was found to be altered in aged wounded skin compared to young wound skin (Vu et al., 2022). Current thinking supports the idea that mitochondria are implicated in several of these age-related dysregulations in aged skin wound healing (Schiffmann et al., 2020; Sreedhar et al., 2020). Indeed, mitochondrial abnormalities are a common feature of aged skin in general (Stout and Birch-Machin, 2019; Sreedhar et al., 2020), whereby mtDNA deletions such as the ‘common mutation’ accumulate and are accompanied by impaired mitochondrial function, characterised broadly by elevated oxidative stress and loss of mitochondrial membrane potential. In particular, the role of mitochondria in metabolic dysfunction is of interest, as impaired vascularisation in aged skin occurs as a consequence of decreased HIF-1α expression (Bonham et al., 2020). Indeed, studies in aged mouse skin demonstrated that increased HIF-1α stabilization through topical deferoxamine (DFP) administration accelerated wound healing (Duscher et al., 2017). DFP is also accepted to be a mitophagy inducer (Allen et al., 2013), and so although not researched in the context of wound healing, this increased DFP-mediated vascularisation may also be related to accompanying elevated mitophagy. The most seemingly promising other results with regard to targetting mitochondrial function in aged skin as a potential treatment for wound healing is metformin, a caloric restriction mimitec. Here, metformin accelerated wound healing in aged mice through improved AMPK-mediated angiogeneisis (Zhao et al., 2017).
Cellular senescence is a state of irreversible cell-cycle arrest brought about in response to various cellular stressors, and is characterised by a pro-inflammatory phenotype termed the senescence-associated secretory phenotype (SASP). The SASP is known to increase in aged tissue, particularly that of proliferative cells, and is implicated in pathogenic defects which contribute to various physiologies, including wound healing (Gorgoulis et al., 2019; von Zglinicki et al., 2021). In recent years, mitochondria and mitochondrial dynamics have been significantly implicated in cellular senescence in various contexts (Westermann, 2010), and in particular in aged and UV-damaged skin (Sreedhar et al., 2020). Here, as the result of oxidative damage, mutations in mtDNA can accumulate and lead to aberrations in various aspects of mitochondrial features (Yang et al., 1995; Berneburg and Krutmann, 2000; Berneburg and Lehmann, 2001; Balaban et al., 2005b; Krishnan et al., 2005; Schroeder et al., 2008; Kaneko et al., 2012; Sreedhar et al., 2020) and subsequently induce cellular senescence (Martini and Passos, 2022).
Transient senescence has been shown to be important in wound healing (Wilkinson and Hardman, 2020), for example, by preventing excessive fibrosis (Krizhanovsky et al., 2008; Jun and Lau, 2017; Hiebert et al., 2018). However, chronic senescence in various cell types can propagate impaired wound healing through various mechanisms, such as prolonged inflammation, epidermal hyperproliferation, matrix proteolysis, and impaired anti-microbial activities (Swift et al., 2001; Ressler et al., 2006; Wall et al., 2008; Makrantonaki et al., 2017b; Albanesi et al., 2018; Wilkinson et al., 2019). As such, targeting uncontrolled senescence may be a potential therapeutic target for future treatments.
With regards to UV-induced damage in skin, it has been shown that UVA-induced mtDNA deletions in both fibroblasts and keratinocytes lead to the increased activity of collagen-degrading enzymes (Berneburg and Krutmann, 2000), downregulation of collagen biosynthesis genes (Blatt and Littarru, 2011), and ECM degradation (Oyewole et al., 2014). In addition to the common phenotypes of aged skin, such as impaired proliferative, migratory, and differentiation ability of various cell types (Sreedhar et al., 2020), UV-induced and aged skin-induced mitochondrial damage and senescence can lead to chronic inflammation, which is a common phenotype of chronic wound pathophysiology (D’Orazio et al., 2013).
As mentioned previously, mtROS play a pleiotropic role in wound healing, and persistently exist at low levels throughout the process. Precise, low-level ROS is beneficial at several adaptive stages of wound healing (Polaka et al., 2022). However, excessive ROS production can be damaging and impair wound healing. For example, at the inflammatory stage, where excessive ROS levels can cause chronic inflammation (Sen and Roy, 2010; Bryan et al., 2012). Prolonged ROS production can also enhance the activation of pro-apoptotic proteins, leading to excessive apoptosis and necrosis (Trachootham et al., 2008). In line with this, several studies have described the beneficial effects of targeting mtROS in wound healing in an effort to prevent excessive ROS production and treat chronic wounds (Kunkemoeller and Kyriakides, 2017; Cano Sanchez et al., 2018). Endogenous antioxidant compounds such as taurine, N-acetyltaurin and hypotaurine have been found in wound samples from humans (Manchanda et al., 2023). Pharmaceutical regulation of ROS has also been shown to be beneficial in wound healing in diabetic mice. For example, administration of SkQ1, which normalises ROS levels in diabetic mice, accelerated wound healing by enhancing re-epithelialisation, vascularisation, and granulation tissue formation, as well as improving immune cell regulation (Demyanenko et al., 2017). It was also found to accelerate granulation tissue formation, vascularisation and inflammatory phase resolution in aged mouse models (Demyanenko et al., 2015). Other studies investigating the impact of various antioxidants in diabetic wounds include the genetic inhibition of XO, or activation of Nrf2-mediatiated antioxidants, which significantly improved wound healing (Bitar and Al-Mulla, 2011; Weinstein et al., 2015; Soares et al., 2016; David et al., 2017). In addition to various chemicals, extensive research has gone into developing novel applications to regulate ROS levels in wounds. One such example are oxygen-releasing polymeric microspheres embedded in alginate hydrogels (Choi et al., 2018). These microspheres were found to enhance cell growth and thus wound healing, primarily through upregulating angiogenesis via increased oxygenation around the wound. In a recent review by Polaka et al., the various current treatment possibilities for modulating ROS in wound healing are described in more detail (Polaka et al., 2022).
Apoptosis is a non-inflammatory process that leads to programmed cell death and is essential for tissue homeostasis, defence, and development. It is particularly important in wound healing for aspects such as the removal of inflammatory cells in early healing and the formation of the scar (Greenhalgh, 1998). The apoptotic process is characterised by specific morphological changes. First, the cell shrinks and ceases to adhere to the neighbouring cells, albeit maintaining an intact cell membrane. Then, chromatin condensation, membrane blebbing, and nuclear fragmentation take place (Kerr et al., 1972). In the meantime, cytochrome c is released from a ruptured mitochondrial membrane and activates a series of degradation reactions that lead to the formation of apoptotic bodies. The remaining particles are quickly cleared by phagocytosis, without triggering inflammation (Taylor et al., 2008). Apoptosis is a balanced state between the action of pro- and anti-apoptotic factors. Activation can occur via an extrinsic pathway, through ligand-binding activation of death receptors (CD95/Fas, tumour necrosis factor (TNF) receptor-1) (Ashkenazi and Dixit, 1998), or via an intrinsic pathway through an intracellular cascade of reactions dependent on mitochondrial permeabilisation changes and the release of cytochrome c (Scaffidi et al., 1998). The regulation of apoptosis was initially thought to happen at the nuclear level. However, when Bcl-2 was reported to be localised in the mitochondria (Hockenbery et al., 1990), the mitochondrial permeability transition pore (mPTP) was reported to generate the “point of no return” for apoptosis (Kroemer et al., 1995), and cytochrome c was determined essential for the activation of caspases (Liu et al., 1996), mitochondria ended up at the centre of the apoptotic regulation process, as well as a means of amplification of the signal generated via the extrinsic pathway (Bossy-Wetzel and Green, 1999).
Wound healing consists of overlapping phases and the correct transition from one phase to the next, following the required timeline, is necessary for adequate healing. Apoptosis has an essential role in this context. It is necessary to remove inflammatory cells during inflammation, and the absence of this response leads to persistent inflammation and non-healing wounds. Furthermore, apoptosis balances the proliferative phase by not allowing excessive cell proliferation and by reducing the cell load on granulation tissue at a later stage, to allow scar formation. Several mice and pig studies showed the impact of apoptosis in wound healing and the impact of non-efficient apoptosis in disease models, such as in diabetes.
Brown et al. (Brown et al., 1997) demonstrated that apoptosis is involved in downregulating inflammation while the re-epithelialisation tissue is generated at the edges of the wound. They assessed wounds in diabetic and non-diabetic mice and detected (in normal mice) early apoptosis in inflammatory cells (earliest 12 h), peaking at day 5 and then regressing. Apoptosis was consistently seen in inflammatory cells underneath the edge of re-epithelialisation tissue or tongue, which suggests that apoptosis may be an important source of signalling in the transition between the inflammatory and proliferative phases. Diabetic mice in this study showed delayed apoptosis and slower healing. Further studies explored apoptosis of inflammatory cells and their role in guiding re-epithelialisation. Kane et al. (Kane and Greenhalgh, 2000) compared apoptosis between diabetic and non-diabetic mice for 42 days and assessed the expression of p53 and Bcl-2. In normal mice they observed an inversed correlation between the levels of Bcl-2 and p53 over time, where Bcl-2 levels increased just after wounding (in parallel to decreasing p53) to allow cell proliferation. When the inflammation phase decreased, p53 levels raised in contrast to Bcl-2, and the rate of apoptosis seemed to be consistent with the levels of p53. This was not seen in diabetic mice, where a consistently higher expression of p53 in relation to Bcl-2 was observed. Nagata et al. (Nagata et al., 1999), in a study using pig burn wounds, also showed that apoptosis and the expression of p53 were higher during the proliferative phase, later declining during the remodelling phase. The importance of apoptosis in the maturation and evolution of granulation tissue was demonstrated by Desmouliere et al. (Desmoulière et al., 1995). In a rat model (8 weeks old), wounds were inflicted dorsally, and granulation tissue was sampled and analysed from day 2–60 (at various time points). Apoptosis was observed initially after wounding, became more evident at day 8, and peaked between day 12 and 25. During this last period, the frequency of apoptotic myofibroblasts and vascular cells was the highest, which suggests that the apoptosis of the granulation tissue occurs after wound closure and takes place in a consecutive manner, leading to a gradual clearance of the granulation tissue over time. Other aspects linked to dysregulation of apoptosis in skin wound healing were, for example, hypertrophic scars (Wassermann et al., 1998) but also decreased myofibroblast differentiation leading to delayed wound contraction (Sindrilaru et al., 2009).
Mitochondrial permeability transition (MPT) is a state characterised by an increase of the inner membrane permeability, mediated by voltage-dependent channels located in the inner membrane such as the mPTP. The exact composition of the mPTP is still not fully understood, but it is recognised that the pore is composed of several proteins such as the adenine nucleotide translocator (ANT) and Cyclophilin D (CypD), a target for the drug Cyclosporine A, which inhibits CypD and mediates the closure of mPTP (Beutner et al., 1997). A transient loss of membrane potential in the IMM occurs in certain physiological states and leads to outcomes such as muscle contraction or saliva secretion (Altschuld et al., 1992; Ryu et al., 2010). It can also mediate cell signalling by generating a burst in ROS production (Wang et al., 2008). However, a prolonged dissipation of the inner membrane potential is usually linked to cell death (Marchetti et al., 1996; Zamzami et al., 2005).
During the intrinsic apoptotic pathway, there is a tight regulatory system in place, led by the Bcl-2 protein family (Kroemer et al., 2007). Bax and Bak are necessary for the machinery behind membrane permeabilisation in apoptosis. On the contrary, Bcl-2 and Bcl-xL act as anti-apoptotic factors. In addition, BH3-proteins can interact with both anti- and pro-apoptotic effectors (Chipuk and Green, 2008). This way, the Bcl-2 protein family has a direct effect on cell fate depending on the upstream stimulus, deciding whether the membrane will be permeabilised or not, which will be followed by outer membrane permeabilisation and release of cytochrome c. The outer membrane permeabilisation occurs via the mitochondrial apoptosis-induced channel (MAC) which is suggested to interact and activate the mPTP (in the inner membrane) via positive reinforcement loops, leading to matrix swelling, loss of folding and cristae, rupture of the inner membrane and more cytochrome c release (Kinnally et al., 2011). Other outer membrane channels, such as VDAC1, have also been implicated in the facilitation of the mPTP opening, due to an increased Ca2+ influx and activation of the calcium-sensitive mPTP. VDACs are also under the influence of the Bcl-2 protein family (Heiden et al., 2001).
Few studies have investigated the role of mitochondria in apoptosis and wound healing. Herein, a study in C. elegans wounding model has suggested a possible role of mPTP in wound healing (Xu and Chisholm, 2014). Xu et al. showed that mitochondrial calcium induces mtROS upon injury, which is then released via the mPTP and accelerates wound healing. As such, further studies are required to further elucidate the role of mPTP in wound healing. Additionally, investigations into other mechanisms mentioned in this review such as the metabolism of apoptosis, as well as the interaction of mitochondrial dynamics and mitophagy with apoptosis in the context of wound healing are required.
Mitochondria can rapidly adapt in order to meet changing metabolic demands in the cell or respond to cell stressors. In general, greater demand is met with increased mitochondrial biogenesis (mitogenesis) as well as fusion of mitochondria in order to expand the network. In contrast, decreased demand or certain stressors induce the fission of mitochondria and in some cases degradation through mitochondrial autophagy (mitophagy) (Herst et al., 2017; Ma et al., 2020) (Figure 4).
FIGURE 4. The life cycle of mitochondria and mitochondrial homeostasis and wound healing. In response to increased energetic demand in the cell, mitochondria can fuse with other mitochondria as well as increase their number through mitochondrial biogenesis (mitogenesis). In contrast, when the energy requirements in the cell are diminished or the cell is stressed, mitochondria can undergo fission/fragmentation in order to reprogramme the cellular metabolic state. Further, mitochondria can undergo degradation through mitophagy in order to clear damaged mitochondria and to prevent cellular stress.
Mitochondria undergo constant cycles of fission and fusion events in what is termed ‘mitochondrial dynamics’. Mitochondrial dynamics control the morphology of mitochondria, as well as the number, size, distribution, transport, and quality control (Chen and Chan, 2009). Correct maintenance of these factors is important in order for the regulation of mitochondrial function. Over the past decades evidence has supported the fact that mitochondrial dynamics is important for several cellular processes, such as proliferation, cell-cycle progression, Ca2+ homeostasis, and cell death, and that dysregulation in mitochondrial dynamics is associated with several diseases (Roy et al., 2015; Chen and Chan, 2017; Trotta and Chipuk, 2017; Altieri, 2018). Dynamin-related protein 1 (Drp1) is the master regulator of mitochondrial fission and is recruited to the outer mitochondrial membrane (OMM) by its receptors Fis1, Mff, MIEF1, and MIEF2. Once recruited, Drp1 wraps around the mitochondria and induces constriction through GTPase activity (Otera et al., 2013). With regards to mitochondrial fusion, there are three key proteins–Opa1, Mfn1, and Mfn2. Mfn1 and Mfn2 lie on the OMM and are responsible for forming a dock between two adjacent mitochondria followed by fusion through GTP hydrolysis (Brandt et al., 2016). In addition to its fusion functions, Mfn2 is also involved in insulin signalling, energy metabolism, ER-mitochondrial connections, and mitophagy (de Brito and Scorrano, 2008; Perumalsamy et al., 2010; Chen and Dorn, 2013; Zorzano et al., 2015). Opa1 on the other hand is located in the IMM, and is responsible for the fusion of the IMM and matrixes of two adjacent mitochondria (Ishihara et al., 2006; Mishra et al., 2014).
In recent years, studies have shown that mitochondrial fission and therefore a fragmented mitochondrial phenotype, where the mitochondria are more spherical and unfused, and the mitochondrial network is less tubular and branched, is important for wound healing (Muliyil et al., 2011; Muliyil and Narasimha, 2014; Fu et al., 2020; Ponte et al., 2020). In the Ponte et al. study, in which wound healing was characterised in Drosophila with various mutations in mitochondrial dynamic genes, they found that Drp1-mediated fission and an increased fragmented phenotype regulated wound healing by controlling ROS production, Ca2+ homeostasis, and the accumulation of F-actin at the wound edge (Ponte et al., 2020). In this study it was also found that mutations in Fis1 leading to mitochondrial fragmentation also impaired wound healing. Similarly, in the Fu et al. study it was shown that mitochondrial fission is important in wound healing in C.elegans and zebrafish, however in this study they found that mitochondrial fragmentation that occurs immediately after wounding was Drp1-independent, and instead dependant on the Rho GTPase MIRO-1 (Fu et al., 2020). It was also demonstrated that this mitochondrial fragmentation-mediated acceleration of wound healing relied on mtROS production and Ca2+ signalling, as well as being associated with a more glycolytic metabolism (Fu et al., 2020). It is important to note however that neither of these studies looked at the role of mitochondrial dynamics in human tissue, which may be a factor in the differences in findings. In addition, few of these studies used mouse models, which although considerable differences exist between wound healing in human and mice, such as the significant presence of panniculus carnosus (Gerber et al., 2014) and stem cells niches from increased hair follicle frequency in mice (Eming et al., 2014), these models offer an important opportunity to study wound healing pathophysiology at a higher organismal level (Zomer and Trentin, 2018), and also strongly supports the notion that studies into human tissue are required. Importantly, a lack of electron microscopy analysis of the ultrastructure of mitochondrial morphology in these studies limits the knowledge of cristae structure and complexity in wound healing contexts.
Previous studies have shown that mitochondria in differentiating epidermal keratinocytes have a fragmented phenotype (Mellem et al., 2017; Ipponjima et al., 2020). This mitochondrial fragmentation coincides with the reduced energetic requirement of the keratinocytes involved in differentiation, as opposed to proliferating basal keratinocytes, and aids in the degradation of mitochondria required in keratinocyte differentiation, as discussed in the Section 6.3 later.
Mitochondrial fragmentation is known to promote apoptosis of macrophages (Wang et al., 2017), cell proliferation (Parker et al., 2015), and support glucose homeostasis and glycolytic metabolism (Jakobs et al., 2003; Toda et al., 2016; Seo et al., 2018) in a variety of tissues. Although the role of mitochondrial fragmentation in these processes has not been studied in the wound healing context in particular, appropriate regulation of these physiological processes is known to be critical to well-coordinated wound healing.
Mitogenesis is commonly initiated in cases of decreased ATP levels, specifically through the AMPK-peroxisome proliferator-activated receptor γ coactivator 1α (PGC-1α) pathway. PGC-1α subsequently interacts with nuclear respiratory factor 1 (NRF1) to activate the mitochondrial transcription factor A (TFAM) and induce transcription of mtDNA (Chung et al., 2017). The AMPK-PGC1α pathway also inhibits mammalian target of rapamycin (mTOR) signalling to decrease anabolic processes and promote oxidative metabolism (Arnould et al., 2015).
The role of mitogenesis in wound healing has not been extensively investigated, and so requires further study. However, it has been examined in some studies, and from current knowledge of mitochondrial homeostasis it can be suggested that decreased mitogenesis is seen in the early stages of wound healing where glycolysis is the main source of metabolism. One such previous report that has investigated mitochondrial homeostasis in wound healing found that macrophages involved in the later stages of wound healing had higher mitochondrial mass, as determined by MitoTracker Green staining, as well as a transcriptional upregulation of genes involved in mtDNA replication and biogenesis, when compared to macrophages involved in the inflammatory phase (Willenborg et al., 2020). Conversely though, higher mitochondrial mass was observed in dermal fibroblasts in day 1, 7, and CW patient tissue in another study (Manchanda et al., 2023).
Increased mitogenesis has been described in other tissues such as in rotator cuff tendon injury muscle healing (Thankam et al., 2018). However, in this tissue mitogenesis and OXPHOS are upregulated in response to hypoxia, which is seemingly the opposite of what happens in the skin wound healing context.
Mitophagy is the selective clearance of mitochondria by autophagy, and effective regulation of mitophagy is essential for the functional integrity of the mitochondrial network and cell homeostasis (Levine and Kroemer, 2008; Ma et al., 2020). Indeed, defective mitophagy and the subsequent accumulation of dysfunctional mitochondria is implicated in several diseases such as Parkinson’s, neurodegenerative diseases, and type 2 diabetes, among others (Doblado et al., 2021). Numerous pathways of mitophagy have been described, such as PINK1/Parkin ubiquitin-dependant mitophagy, as well as receptor-dependent pathways, which include BNIP3L/NIX (herein referred to as NIX), BNIP3, and FUNDC1, which interact with LC3 to induce mitochondrial degradation (Ma et al., 2020).
Mitophagy is known to promote cell proliferation by helping switch metabolism from OXPHOS toward aerobic glycolysis (Doblado et al., 2021). Mitophagy has also been shown to drive metabolism towards glycolysis in order to promote cell growth and survival in cancer cells (Domenech et al., 2015; Esteban-Martinez et al., 2015; Agnihotri et al., 2016), as well as the metabolic reprogramming required during somatic stem cell differentiation. Additionally, it is known that HIF-1α induces the transcription of mitophagy receptors BNIP3, NIX, and FUNDC1 (Sowter et al., 2001; Esteban-Martínez et al., 2017). Although the role of mitophagy in metabolism in wound healing has not been investigated, current thinking supports the idea that it may play a critical role (Liu et al., 2020).
Various studies have investigated the role of mitophagy in different aspects of wound healing, however, few studies have undertaken studies using human wounded tissue. One such aspect that has been studied is the migration of epidermal cells during re-epithelialisation. Here, previous studies in mice have demonstrated the role of BNIP3-mediated mitophagy in accelerating keratinocyte migration, and that this upregulation of BNIP3 was controlled by hypoxia (Zhang et al., 2017; Zhang et al., 2019). Another recent study found that hypoxia-induced mitophagy promoted keratinocyte migration and proliferation by degrading p-MAP4 (Feng et al., 2023). Although not investigated in the context of wound healing, mitophagy and autophagy in general are known to regulate the acquisition of mesenchymal markers, focal adhesion disassembly, maintenance of the cytoskeleton organisation as well as focal adhesion, and β1 integrin membrane recycling to promote cell migration (Tuloup-Minguez et al., 2013; Maes et al., 2014; Sharifi et al., 2016; Liu et al., 2017; Sun et al., 2018). Interestingly, autophagy has been shown to be important epithelial-to-mesenchymal (EMT) transition in some cancers, although the molecular mechanism behind this is not fully known (Gugnoni et al., 2016).
Significantly, BNIP3 (Moriyama et al., 2014; Moriyama et al., 2017) and NIX (Simpson et al., 2021) mediated mitophagy has been shown to be essential for the differentiation of keratinocytes in the epidermis. General autophagy has also been demonstrated to play a role in epidermal keratinocyte differentiation (Aymard et al., 2010; Chatterjea et al., 2011; Yoshihara et al., 2015; Akinduro et al., 2016; Sil et al., 2018; Ipponjima et al., 2020). However, studies by Moriyama et al. and the Simpson et al. study were the first to specifically describe mitophagy (Moriyama et al., 2014; Moriyama et al., 2017; Simpson et al., 2021). Additionally, they showed that the mitochondria in differentiating keratinocytes were more depolarised, had higher levels of HIF-1α expression, upregulated Drp1-mediated fragmentation, and were using glycolysis for metabolism–linking previously discussed aspects of mitochondrial homeostasis. Importantly, through inhibition of these functions, the keratinocytes displayed abnormal differentiation. This suggests that dysfunctional mitophagy could be implicated in abnormal re-epithelialisation and therefore in the pathogenesis of chronic wounds (Simpson et al., 2021).
Previous studies have shown that BNIP3 mitophagy protects against UVA (Zhao et al., 2013) and UVB-induced as well as ROS-mediated epidermal damage by preventing excessive apoptosis (Moriyama et al., 2014; Moriyama et al., 2017). Additionally, clinical trials in which the mitophagy inducer urolithin A (UA) was applied topically demonstrated beneficial effects of mitophagy on skin ageing and protection from UVB-induced photodamage (D’Amico et al., 2023; Liu et al., 2022). One potential mechanism could be that mitophagy aids in preventing the accumulation of damaged and oxidised proteins, thereby supressing proteolytic stress (Ogata et al., 2006; Mera et al., 2010; Song et al., 2017). Although not confirmed in studies, this would suggest that functioning mitophagy may be important in preventing chronic wound pathogenesis by inhibiting excessive apoptosis and the pathophysiological issues that arise with that (Ogata et al., 2006; Mera et al., 2010; Burton et al., 2013).
PINK1 has been shown to be important to the function of astrocytes in neurological wound healing (Choi et al., 2013), however, through screenings of altered signalling pathways, PINK1/Parkin mediated mitophagy has been shown to not play an important role in skin wound healing. This suggests that the receptor-dependent pathways of mitophagy, such as the BNIP3, NIX, and FUNDC1 pathways, as opposed to the PINK1/Parkin pathway, are involved in wound healing. This agrees with the fact that receptor-dependant pathways are known to be upregulated by hypoxia-induced HIF-1α activation (Zhang and Ney, 2009; Liu et al., 2012). However, future studies would benefit from utilising mouse models with knockouts of mitophagy receptors.
With regards to mitophagy in inflammation, although it has not been studied in the wound healing context, mitophagy has been shown to prevent the activation of the inflammasome by clearing damaged mitochondria and thereby preventing the excess release of DAMPs (Lupfer et al., 2013; Guo et al., 2014; West et al., 2015; Kim et al., 2016; Zhong et al., 2016). In addition, mitophagy contributes to the metabolic rewiring of macrophages in order to stimulate either M1 or M2 macrophage functions (Wu et al., 2014; Gupta et al., 2019; Lampert et al., 2019). It is feasible therefore that mitophagy plays an important role in the inflammatory stage of wound healing, and as such, dysregulated mitophagy may contribute to excess inflammation and the pathogenesis of chronic wounds.
Finally, another recent study found that pharmacological induction of mitophagy promoted angiogenesis and the deposition of mature collagen fibres to enhance wound healing (Feng et al., 2022).
Ca2+ plays an essential role in wound healing, and mitochondria are one of the key regulators of Ca2+ signalling in cells. However, few studies have specifically investigated the relationship of mitochondria and Ca2+ in wound healing.
The elevation of intracellular Ca2+ is one of the first damage signals in the wound healing process (Lansdown, 2002) and is essential for the initiation and regulation of wound healing (Xu and Chisholm, 2011; Yoo et al., 2011; Razzell et al., 2013; Xu and Chisholm, 2014). As mentioned previously in this review, this propagation of Ca2+ is responsible for various processes such as being inflammatory mediators (Niethammer et al., 2009a; Niethammer et al., 2009b; Razzell et al., 2013) and modulating proliferation, migration, and differentiation of fibroblasts and keratinocytes during re-epithelialisation (Hennings and Holbrook, 1983; Ko et al., 2001; Huang et al., 2006; Bikle et al., 2012; Pastar et al., 2014; Xu and Chisholm, 2014; Law et al., 2015; Xue and Jackson, 2015). Ca2+ is also involved in angiogenesis in wound healing (Berridge et al., 1998), as well as triggering an increased generation and release of mtROS (Xu and Chisholm, 2011; Xu and Chisholm, 2014). Additionally, Ca2+ signalling pathways contribute to the regulation of metabolism and extracellular matrix formation (Subramaniam et al., 2021).
As stated above, mitochondria are significantly responsible for regulating cellular Ca2+ storage and propagation, and mitochondrial Ca2+ is essential for various cellular signalling pathways as well as in cellular bioenergetics (Pathak and Trebak, 2018). Ca2+ is imported into the mitochondria through the mitochondrial Ca2+ uniporter (MCU) and effluxed via the mitochondrial Na+/Ca2+/Li+ exchanger (NCLX). However, because mitochondria have a low affinity for Ca2+, Ca2+ transfer into the mitochondria occurs through close contacts with the ER, termed mitochondrial associated membranes (MEM) (Mesmin, 2016). These MEMs are essential for various mitochondrial functions, such as glucose sensing, mitophagy, apoptosis, the unfolded protein response (UPR), insulin signalling, and ROS signalling (Rieusset, 2018). Recent studies have described the important role of Ca2+ in regulating oxidative stress, as well as mitochondrial dynamics and biogenesis, in aiding wound healing, particularly re-epithelialisation (Xu and Chisholm, 2011; Xu and Chisholm, 2014; Hunter et al., 2018; Ponte et al., 2020). In addition, a study by Parnis et al. demonstrated that mitochondrial calcium homeostasis was important in cell proliferation and wound healing in astrocytes (Parnis et al., 2013). However, much is still unknown about the roles of mitochondrial Ca2+ homeostasis in skin wound healing, such as its role in apoptosis and immune responses, particularly in humans.
Mitochondria play an essential role in the regulation of immune cells, primarily through acting as hubs for metabolism regulation, which is necessary for the activation of several immune cell types of both the innate and acquired immune systems. In addition, they participate in various inflammatory signalling pathways via ROS mediators (Chandel et al., 2000; Gurung et al., 2015), can form scaffolding required for interactions between various immune cells and respective proteins (Jacobs and Coyne, 2013), and components of mitochondria can activate several types of inflammatory responses themselves (Marchi et al., 2023).
In general, inflammation is initiated by the activation of pattern recognition receptors (PRRs), which can be achieved by bacterial and viral molecules, as well as endogenous DAMPs (Kroemer et al., 2022). DAMPs are normally restricted physical access to PRRs in physiological conditions, however, in various cellular stress environments whereby alterations in the permeability of cell compartments occurs, DAMPs can interact with PRRs and initiate immune responses (Elliott et al., 2009; Ghiringhelli et al., 2009). Notably, mitochondria contain DAMPs (mtDAMPs) which can activate immune responses in different tissues. A notable example which has gathered significant interest in recent years is the role of mtDNA in the cyclic GMP-AMP synthase (cGAS), stimulator of interferon response cGAMP interactor 1 (STING1) pathway (Decout et al., 2021). cGAS is a cytosolic protein that responds to dsDNA in the cytosol by catalysing the formation of cyclic GMP-AMP (cGAMP), which then binds to ER-bound STING1 to initiate interferon responses (Decout et al., 2021). Subsequently, studies in recent years have shown that mtDNA in the cytosol is also a potent activator of the cGAS-STING pathway, and has been shown to contribute to various diseases, such as systemic lupus erythematosus, liver damage, and ageing itself (West et al., 2015; Kim et al., 2019; Miller et al., 2021; He et al., 2022). There have been numerous methods proposed to as to how mtDNA is released into the cytosol, including following mitochondrial outer membrane permalisation (MOMP) (Fuchs and Steller, 2015; Li et al., 2021), which involves the BAX-BAK1 pores (McArthur et al., 2018; Riley et al., 2018; Yamazaki et al., 2020; Willemsen et al., 2021), or BH3-interacting domain death agonist (BID)-induced mitochondrial membrane pores (Flores-Romero et al., 2022). Additionally, cytosolic mtDNA translocation has also been shown to occur without significant mitochondrial stress through MPT responses via VDAC pores (Kim et al., 2019; Yu et al., 2020; Domizio et al., 2022). As well as being activators of the cGAS-STING1 pathway, mtDNA can also activate inflammasome signalling, in particular NLRP3 and AIM2 containing inflammasomes (Shimada et al., 2012; Dang et al., 2017). mtDNA involved in these inflammasome pathways are generally oxidised, released via MPT (Nakahira et al., 2011; Renaudin, 2021), and can initiate a feed-forward loop whereby increased ROS further increased mtDNA release into the cytosol. As well as the inflammatory pathways mentioned above, mtDNA can also activate Toll-like receptor 9 (TLR9) and advanced glycosylation end product-specific receptors (AGER/RAGE) to subsequently activate myeloid cells (Vanpouille-Box et al., 2019).
Another mtDAMP is mtRNA, which has been shown to activate RIG-1 like receptors (Hur, 2019). As with mtDNA release into the cytosol, mtRNA can be released via BAX-BAK1 induced MOMP (Dhir et al., 2018). Other components of mitochondria are also known to induce various forms of inflammation. These include SMAC, which is released downstream of MOMP and can modulate T cells (Gyrd-Hansen and Meier, 2010; Rizk et al., 2019), N-formyl peptides and cardiolipin, which activate neutrophils (Boada-Romero et al., 2020) and T cells (Leslie et al., 2008; Dieudé et al., 2011) respectively, as well as cytochrome c, which has been shown to stimulate TLR4 inflammation (Gouveia et al., 2017).
Several of the immune systems mentioned above are essential in the wound healing process, including TLR9-mediated inflammation (Yamamoto et al., 2011) and inflammasomes (Ito et al., 2018). In addition, the interferon response plays an important role in wound healing, in particular through antimicrobial activities in early wound healing, and by promoting re-epithelialisation (Decker et al., 2005; Stetson and Medzhitov, 2006). In a recent study on mice topically treated with cGAMP, the cGAS-STING1 pathway was shown to accelerate wound closure, in particular by increasing cell migration to the wound site (Mizutani et al., 2020). Importantly, treatment with IFNR inhibitors also significantly impaired wound healing in these mice. Although few studies have looked at the specific role of mitochondria in these immune responses in the wound healing process, current knowledge supports the theory that mitochondria are involved. This suggests that future studies should look to elucidate the potential contribution of mitochondria to the activation of immune pathways in wound healing.
With regards to the role of mitochondrial metabolism in immune signalling, increased glycolysis at the early stages and a shift towards more oxidative metabolism towards the later wound healing stages has been shown to control macrophage phenotype and polarisation in wound healing, as discussed previously (Willenborg et al., 2020). Mitochondrial metabolism is also essential for the proper function of neutrophils. In particular, they play a role in antimicrobial activities through respiratory burst, as well as the development, adhesion, migration, and ultimately death of neutrophils (Cao et al., 2022). For example, pharmacological inhibition of OXPHOS reduced neutrophil ROS production to decrease the potency of their respiratory burst (Bao et al., 2014; Dunham-Snary et al., 2022). Similar pharmacological OXPHOS inhibition also reduced neutrophil migration and chemotaxis (Chen et al., 2006; Bao et al., 2015). Importantly however, too much ATP production also reduced chemotaxis in neutrophils (Kondo et al., 2019), suggesting that there is a well-regulated balance in the regulation of metabolism required. Finally, mitochondrial metabolism is required for the induction of apoptosis of another important immune cell type in wound healing, platelets (Melchinger et al., 2019). However, apart from the examples listed, few studies have investigated the role of mitochondrial metabolism in immune cells in the context of wound healing.
In summary, even though there is a large body of literature describing the importance of inflammation in wound healing as well as that of mitochondria in inflammation, little is known about the specific role of mitochondria in inflammation in wound healing. Therefore, future studies should look to bridge the gap in scientific knowledge in this area.
Another aspect of mitochondrial quality control is the mitochondrial unfolded protein response (UPRmt). Like mitophagy, the UPRmt is responsible for orchestrating responses to mitochondrial stresses such as the aggregation of misfolded mitochondrial proteins or mutated mtDNA (Zhao et al., 2002), although unlike mitophagy, the UPRmt does not result in the degradation of damaged mitochondria, but instead, promotes the repair of individual mitochondria and the mitochondrial network as a whole (Shpilka and Haynes, 2018). The UPRmt is also important in the context of mitochondria in ageing (Lima et al., 2022). Mechanistically, the UPRmt involves the activation of the nuclear localising sequence (NLS) of the activating transcription factor associated with stress (ATFS-1). In a healthy mitochondrial network context, the mitochondrial localising sequence (MLS), predominates over the NLS, and directs ATFS-1 towards import into the mitochondria, where it is degraded by the protease LON (Nargund et al., 2012; Rauthan et al., 2013). However, in the event of mitochondrial stress, such as an accumulation of OXPHOS complexes (Houtkooper et al., 2013), ATFS-1 is activated as a nuclear transcription factor and leads to the subsequent activation of the UPRmt, in order to avoid toxicity (Wang and Chen, 2015; Wrobel et al., 2015).
The UPRmt is similar to the ER-directed UPR (herein referred to simply as UPR), in that they contain several of the same proteases and chaperones, including CHOP, and both the UPR and UPRmt reduce cellular damage by clearing misfolded proteins (Hetz, 2012). Additionally, the UPR is significantly involved in the induction of apoptosis, cell differentiation, inflammatory responses, and glucose and lipid metabolism–all of which are important in the context of mitochondria in wound healing (Hetz, 2012). Indeed, mild UPR has been shown to be beneficial to wound healing, however, excessive UPR induces cell death and can be detrimental to wound healing (Bachar-Wikstrom et al., 2021). Currently, little is known about the role of the UPRmt in wound healing or skin ageing in general. Therefore, future studies should aim to investigate its role, as well as the potential link between the UPR and UPRmt in the context of wound healing.
In recent years, mitochondria have become the most studied organelle in biomedical research (Picard and Shirihai, 2022; Monzel et al., 2023), and significant research has collectively demonstrated that mitochondria play a vital role in various aspects of the wound healing process. Indeed, dysfunction in numerous aspects of mitochondrial behaviours and functions is significantly implicated in the pathogenesis of chronic wounds. Additionally, although wound healing has not been noted in many cases of mitochondrial disease patients, approximately 10% of these patients present with some form of skin pathology (Hussain et al., 2021). This review has highlighted the several ways in which mitochondria are involved in wound healing, notably by regulating cellular metabolism, as well as redox signalling and apoptosis, which are critical mediators of correct wound healing function (Figure 5). In addition, we have discussed how control of mitochondrial homeostasis through the regulation of mitochondrial dynamics, mitogenesis, and mitophagy are also important in wound healing.
FIGURE 5. Summary of the role of mitochondria in wound healing. Current knowledge about the role of mitochondria in wound healing can be divided into seven sections–mitochondrial metabolism, oxidative stress/ROS signalling, mitochondrial dynamics and mitophagy, apoptosis, inflammation, mtUPR, and Ca2+ homeostasis. Although a considerable amount of knowledge has been learned in the past decade, many questions remain about its role, and further research is needed in order to develop translational options for the treatment of mitochondrial parameters in chronic wounds.
However though, one of the significant limitations of research in the field of mitochondria in wound healing, and of wound healing as a whole, is the fact that few studies use human tissue. This can limit the translational potential of many findings, as human skin and various factors of the wound healing process itself varies considerably to that of animal models such as mice, Drosophila, or C.elegans. These studies are still important in furthering knowledge in physiological and pathological mechanisms of wound healing, however future studies should aim to also use human tissue when studying mitochondria in wound healing (Zomer and Trentin, 2018). It would be hoped that in the near future more studies can look to target various aspects of mitochondrial function in order to explore the therapeutic potential of targeting mitochondria in chronic wounds. Such targeting may include promoting glycolysis for improved haemostasis and inflammation stage function, ROS dynamics, and ensuring proper mitochondrial dynamics, particularly in the case of elderly individuals. With regard to targeting mitochondrial metabolism, several chemicals that inhibit specific forms of mitochondrial metabolism, such as metformin, which inhibits the ETC, are available and undergoing clinical trials for various diseases including some forms of cancer (Vasan et al., 2020; Sainero-Alcolado et al., 2022). There are also numerous ways to modulate mtROS levels therapeutically, including by inhibiting the ETC (Perillo et al., 2020), or the glutathione disulphide mimetic NOV-002, which has also been used in clinical trails for various cancers (Montero and Jassem, 2011). Finally, one exciting, although yet not fully validated, potential treatment of mitochondrial defect-related pathology is that of mitochondrial transplantation (Ulger and Kubat, 2022). Broadly, there are several alternative in vitro methodologies for this application including microinjection (Capecchi, 1980), magnetomitotransfer (Macheiner et al., 2016), the photothermal nanoblade method (Wu et al., 2011), or direct transfer into tissue in an in vivo manner. With regards to the latter method, early studies have shown beneficial impacts of directly transferring mitochondria into mouse models of ischemic heart disease (McCully et al., 2009), liver ischemia-reperfusion (Lin et al., 2013), ischemic spinal chords (Fang et al., 2021), and Parkinson’s disease (Shi et al., 2017). As such, due to the partial overlap in pathophysiological factors of these diseases and chronic wounds, for example, chronic inflammation and oxidative stress, as well as metabolic imbalances, the application of mitochondrial transplantation should be considered as a potential future treatment option.
Importantly though, as the pathogenesis and pathophysiology of chronic wounds can vary greatly between patients as a result of differences in genetic backgrounds, chronic wound type, or lifestyle factors (Falanga et al., 2022), future therapeutic targeting should look at how any rational therapy specifically impacts mitochondrial function, with the aim for specific and targeted benefits. This type of personalised medicine is essential in treating cancer, and this approach should be adopted for treating chronic wounds. For instance, a treatment targeted at increasing glycolytic metabolism with the aim of improving immune cell function should be assessed to see whether this can have harmful off-target effects, and the specific duration and timeline of the treatment should be determined. With regard to that, advances in emerging technologies such as combining metabolomics and proteomics on human wound samples at various stages of the wound healing cycle could provide hints to how active metabolic pathways vary at different time points (Karczewski and Snyder, 2018). In addition, single cell studies such as single-cell proteomics or deep visual proteomics (DVP) on human tissue may provide insights into the relationship of specific cells types such as fibroblasts or ECM cells and arrays of mitochondrial parameters (Rosenberger et al., 2023).
MH, EB-W and JW conceptualised and wrote the manuscript. MT wrote the manuscript and drafted the figures. All authors contributed to the article and approved the submitted version.
This work was supported by grants from Hudfonden, Swedish Science Council, Swedish Society for Medical Research, Leo foundation, ALF medicin Stockholm, Jeanssons stiftelse, Wallenberg foundation and Tore Nilssons Stiftelse. The grants were all awarded to JW.
The authors declare that the research was conducted in the absence of any commercial or financial relationships that could be construed as a potential conflict of interest.
All claims expressed in this article are solely those of the authors and do not necessarily represent those of their affiliated organizations, or those of the publisher, the editors and the reviewers. Any product that may be evaluated in this article, or claim that may be made by its manufacturer, is not guaranteed or endorsed by the publisher.
Agnihotri, S., Golbourn, B., Huang, X., Remke, M., Younger, S., Cairns, R. A., et al. (2016). PINK1 is a negative regulator of growth and the warburg effect in glioblastoma. Cancer Res. 76, 4708–4719. doi:10.1158/0008-5472.CAN-15-3079
Akinduro, O., Sully, K., Patel, A., Robinson, D., Chikh, A., Mcphail, G., et al. (2016). Constitutive autophagy and nucleophagy during epidermal differentiation. J. Invest. Dermatol 136, 1460–1470. doi:10.1016/j.jid.2016.03.016
Albanesi, C., Madonna, S., Gisondi, P., and Girolomoni, G. (2018). The interplay between keratinocytes and immune cells in the pathogenesis of psoriasis. Front. Immunol. 9, 1549. doi:10.3389/fimmu.2018.01549
Aldosari, S., Awad, M., Harrington, E., Sellke, F., and Abid, M. (2018). Subcellular reactive oxygen species (ROS) in cardiovascular pathophysiology. Antioxidants (Basel) 7, 14. doi:10.3390/antiox7010014
Allaoui, A., Botteaux, A., Dumont, J., Hoste, C., and De Deken, X. (2009). Dual oxidases and hydrogen peroxide in a complex dialogue between host mucosae and bacteria. Trends Mol. Med. 15, 571–579. doi:10.1016/j.molmed.2009.10.003
Allen, G., Toth, R., James, J., and Ganley, I. (2013). Loss of iron triggers PINK1/Parkin-independent mitophagy. EMBO Rep. 14 (12), 1127–1135. doi:10.1038/embor.2013.168
Altieri, D. (2018). Mitochondrial dynamics and metastasis. Cell Mol. Life Sci. 76, 827–835. doi:10.1007/s00018-018-2961-2
Altschuld, R., Hohl, C., Castillo, L., Garleb, A., Starling, R., and Brierley, G. (1992). Cyclosporin inhibits mitochondrial calcium efflux in isolated adult rat ventricular cardiomyocytes. Am. J. Physiol-heart C 262 (6), H1699–H1704. doi:10.1152/ajpheart.1992.262.6.H1699
Arnould, T., Michel, S., and Renard, P. (2015). Mitochondria retrograde signaling and the UPR mt: where are we in mammals? Int. J. Mol. Sci. 16 (8), 18224–18251. doi:10.3390/ijms160818224
Artuc, M., Hermes, B., Steckelings, U. M., Grützkau, A., and Henz, B. M. (1999). Mast cells and their mediators in cutaneous wound healing-active participants or innocent bystanders? Exp. Dermatol 8, 1–16. doi:10.1111/j.1600-0625.1999.tb00342.x
Ashkenazi, A., and Dixit, V. (1998). Death receptors: signaling and modulation. Science 281 (5381), 1305–1308. doi:10.1126/science.281.5381.1305
Atay, O., and Skotheim, J. (2017). Spatial and temporal signal processing and decision making by MAPK pathways. J. Cell Biol. 216, 317–330. doi:10.1083/jcb.201609124
Atkin, L., Bućko, Z., Conde Montero, E., Cutting, K., Moffatt, C., Probst, A., et al. (2019). Implementing TIMERS: the race against hard-to-heal wounds. J. Wound Care 23, S1-S50–S50. doi:10.12968/jowc.2019.28.Sup3a.S1
Aymard, E., Barruche, V., Naves, K., Bordes, S., C Loss, B., Verdier, M., et al. (2010). Autophagy in human keratinocytes: an early step of the differentiation? Exp. Derm. 20, 263–268. doi:10.1111/j.1600-0625.2010.01157.x
Baccarelli, A., and Byun, H. (2015). Platelet mitochondrial DNA methylation: A potential new marker of cardiovascular disease. Clin. Epigenet 7, 44. doi:10.1186/s13148-015-0078-0
Bachar-Wikstrom, E., Manchanda, M., Bansal, R., Karlsson, M., Kelly-Pettersson, P., Sköldenberg, O., et al. (2021). Endoplasmic reticulum stress in human chronic wound healing: rescue by 4-phenylbutyrate. Int. Wound J. 18 (1), 49–61. doi:10.1111/iwj.13525
Balaban, R., Nemoto, S., and Finkel, T. (2005a). Mitochondria, oxidants, and aging. Cell 120 (4), 483–495. doi:10.1016/j.cell.2005.02.001
Balaban, R., Nemoto, S., and Finkel, T. (2005b). Mitochondria, oxidants, and aging. Cell 25, 483–495. doi:10.1016/j.cell.2005.02.001
Bao, Y., Ledderose, C., Graf, A., Brix, B., Birsak, T., Lee, A., et al. (2015). MTOR and differential activation of mitochondria orchestrate neutrophil chemotaxis. J. Cell Biol. 210 (7), 1153–1164. doi:10.1083/jcb.201503066
Bao, Y., Ledderose, C., Seier, T., Graf, A., Brix, B., Chong, E., et al. (2014). Mitochondria regulate neutrophil activation by generating ATP for autocrine purinergic signaling. J. Biol. Chem. 289 (39), 26794–26803. doi:10.1074/jbc.M114.572495
Barbul, A., Shawe, T., Rotter, S. M., Efron, J. E., Wasserkrug, H. L., and Badawy, S. B. (1989). Wound healing in nude mice: A study on the regulatory role of lymphocytes in fibroplasia. Surgery 105, 764–769. doi:10.1101/cshperspect.a023267
Barker, T. (2011). The role of ECM proteins and protein fragments in guiding cell behavior in regenerative medicine. Biomaterials 32 (18), 4211–4214. doi:10.1016/j.biomaterials.2011.02.027
Barnérias, C., Giurgea, I., Hertz-Pannier, L., Bahi-Buisson, N., Boddaert, N., Rustin, P., et al. (2006). Respiratory chain deficiency in a female with Aicardi-Goutières syndrome. Dev. Med. Child. Neurol. 48 (3), 227–230. doi:10.1017/S001216220600048X
Ben-Sahra, I., Hoxhaj, G., Ricoult, S., Asara, J., and Manning, B. (2016). mTORC1 induces purine synthesis through control of the mitochondrial tetrahydrofolate cycle. Science 351, 728–733. doi:10.1126/science.aad0489
Bentov, I., and Reed, M. (2015). The effect of aging on the cutaneous microvasculature. Microvasc. Res. 100, 25–31. doi:10.1016/j.mvr.2015.04.004
Berneburg, M., and Krutmann, J. (2000). Photoaging-associated large-scale deletions of mitochondrial DNA. Methods Enzymol. 319, 366–376. doi:10.1016/s0076-6879(00)19036-6
Berneburg, M., and Lehmann, A. (2001). Xeroderma pigmentosum and related disorders: defects in DNA repair and transcription. Adv. Genet. 43, 71–102. doi:10.1016/s0065-2660(01)43004-5
Berquist, B., Canugovi, C., Sykora, P., Wilson, D. R., and Bohr, V. (2012). Human Cockayne syndrome B protein reciprocally communicates with mitochondrial proteins and promotes transcriptional elongation. Nucleic Acids Res. 40 (17), 8392–8405. doi:10.1093/nar/gks565
Berridge, M., Bootman, M., and Lipp, P. (1998). Calcium—a life and death signal. Nature 395, 645–648. doi:10.1038/27094
Beutner, G., Rück, A., Riede, B., and Brdiczka, D. (1997). Complexes between hexokinase, mitochondrial porin and adenylate translocator in brain: regulation of hexokinase, oxidative phosphorylation and permeability transition pore. Biochem. Soc. T 25 (1), 151–157. doi:10.1042/bst0250151
Bikle, D., Xie, Z., and Tu, C. (2012). Calcium regulation of keratinocyte differentiation. Expert Rev. Endocrinol. Metab. 7, 461–472. doi:10.1586/eem.12.34
Biniek, K. E. A., Kaczvinsky, J., Matts, P., and Dauskardt, R. H. (2015). Understanding age-induced alterations to the biomechanical barrier function of human stratum corneum. J. Dermatol Sci. 80, 94–101. doi:10.1016/j.jdermsci.2015.07.016
Bitar, M., and Al-Mulla, F. (2011). A defect in Nrf2 signaling constitutes a mechanism for cellular stress hypersensitivity in a genetic rat model of type 2 diabetes. Am. J. Physiol. Endocrinol. Metab. 301, E1119–H1129. doi:10.1152/ajpendo.00047.2011
Blatt, T., and Littarru, G. (2011). Biochemical rationale and experimental data on the antiaging properties of CoQ(10) at skin level. Biofactors 37, 381–385. doi:10.1002/biof.169
Boada-Romero, E., Martinez, J., Heckmann, B., and Green, D. (2020). The clearance of dead cells by efferocytosis. Nat. Rev. Mol. Cell Biol. 21, 398–414. doi:10.1038/s41580-020-0232-1
Bonham, C., Kuehlmann, B., and Gurtner, G. (2020). Impaired neovascularization in aging. Adv. Wound Care 9 (3), 111–126. doi:10.1089/wound.2018.0912
Bossy-Wetzel, E., and Green, D. (1999). Apoptosis: checkpoint at the mitochondrial frontier. Mutat. Res. Dna Repair 434 (3), 243–251. doi:10.1016/s0921-8777(99)00032-4
Brandt, T., Cavellini, L., Kuhlbrandt, W., and Cohen, M. (2016). A mitofusin-dependent docking ring complex triggers mitochondrial fusion in vitro. Elife 5, e14618. doi:10.7554/eLife.14618
Brown, D., Kao, W.-Y., and Greenhalgh, D. (1997). Apoptosis down-regulates inflammation under the advancing epithelial wound edge: delayed patterns in diabetes and improvement with topical growth factors. Surgery 121 (4), 372–380. doi:10.1016/s0039-6060(97)90306-8
Bryan, N., Ahswin, H., Smart, N., Bayon, Y., Wohlert, S., and Hunt, J. (2012). Reactive oxygen species (ROS)—a family of fate deciding molecules pivotal in constructive inflammation and wound healing. Eur. Cells Mater 24, 249–265. doi:10.22203/ecm.v024a18
Buck, D., Jin, D. P., Geringer, M., Hong, S. J., Galiano, R. D., and Mustoe, T. A. (2011). The TallyHo polygenic mouse model of diabetes: implications in wound healing. Plast. Reconstr. Surg. 128, 427e–437e. doi:10.1097/PRS.0b013e31822b7333
Burton, T., Henson, E., Azad, M., Brown, M., Eisenstat, D., and Gibson, S. (2013). BNIP3 acts as transcriptional repressor of death receptor-5 expression and prevents TRAIL-induced cell death in gliomas. Cell Death Dis. 4, e587. doi:10.1038/cddis.2013.100
Cano Sanchez, M., Lancel, S., Boulanger, E., and Neviere, R. (2018). Targeting oxidative stress and mitochondrial dysfunction in the treatment of impaired wound healing: A systematic review. Antioxidants (Basel) 7 (8), 98. doi:10.3390/antiox7080098
Cao, Z., Zhao, M., Sun, H., Hu, L., Chen, Y., and Fan, Z. (2022). Roles of mitochondria in neutrophils. Front. Immunol. 13, 934444. doi:10.3389/fimmu.2022.934444
Capecchi, M. (1980). High efficiency transformation by direct microinjection of DNA into cultured mammalian cells. Cell 22, 479–488. doi:10.1016/0092-8674(80)90358-x
Cardamone, M., Tanasa, B., Cederquist, C., Huang, J., Mahdaviani, K., Li, W., et al. (2018). Mitochondrial retrograde signaling in mammals is mediated by the transcriptional cofactor GPS2 via direct mitochondria-to-nucleus translocation. Mol. Cell 69, 757–772. doi:10.1016/j.molcel.2018.01.037
Chandel, N., Maltepe, E., Goldwasser, E., Mathieu, C., Simon, M., and Schumacker, P. (1998). Mitochondrial reactive oxygen species trigger hypoxia-induced transcription. Proc. Natl. Acad. Sci. U. S. A. 95 (20), 11715–11720. doi:10.1073/pnas.95.20.11715
Chandel, N., Mcclintock, D., Feliciano, C., Wood, T., Melendez, J., Rodriguez, A., et al. (2000). Reactive oxygen species generated at mitochondrial complex III stabilize hypoxia-inducible factor-1alpha during hypoxia: A mechanism of O2 sensing. J. Biol. Chem. 275, 25130–25138. doi:10.1074/jbc.M001914200
Charlesworth, A., Chiaverini, C., Chevrant-Breton, J., Meneguzzi, G., Diociaiuti, A., Dupuis, R. P., et al. (2013). Epidermolysis bullosa simplex with PLEC mutations: new phenotypes and new mutations. Br. J. Dermatol 168 (4), 808–814. doi:10.1111/bjd.12202
Chatre, L., Biard, D., Sarasin, A., and Ricchetti, M. (2015). Reversal of mitochondrial defects with CSB-dependent serine protease inhibitors in patient cells of the progeroid Cockayne syndrome. Proc. Natl. Acad. Sci. U. S. A. 112 (22), E2910–E2919. doi:10.1073/pnas.1422264112
Chatterjea, S., Resing, K., Old, W., Nirunsuksiri, W., and Fleckman, P. (2011). Optimization of filaggrin expression and processing in cultured rat keratinocytes. J. Dermatol Sci. 61 (1), 51–59. doi:10.1016/j.jdermsci.2010.11.003
Chen, H., and Chan, D. (2017). Mitochondrial dynamics in regulating the unique phenotypes of cancer and stem cells. Cell Metab. 26, 39–48. doi:10.1016/j.cmet.2017.05.016
Chen, H., and Chan, D. (2009). Mitochondrial dynamics–fusion, fission, movement, and mitophagy–in neurodegenerative diseases. Hum. Mol. Genet. 18, R169–R176. doi:10.1093/hmg/ddp326
Chen, Q., Vazquez, E., Moghaddas, S., Hoppel, C., and Lesnefsky, E. (2003). Production of reactive oxygen species by mitochondria: central role of complex III. J. Biol. Chem. 278, 36027–36031. doi:10.1074/jbc.M304854200
Chen, Y., Corriden, R., Inoue, Y., Yip, L., Hashiguchi, N., Zinkernagel, A., et al. (2006). ATP release guides neutrophil chemotaxis via P2Y2 and A3 receptors. Science 314 (5806), 1792–1795. doi:10.1126/science.1132559
Chen, Y., and Dorn, G. I. (2013). PINK1-phosphorylated mitofusin 2 is a parkin receptor for culling damaged mitochondria. Science 340, 471–475. doi:10.1126/science.1231031
Chernyavsky, A., Chen, Y., Wang, P., and Grando, S. (2015). Pemphigus vulgaris antibodies target the mitochondrial nicotinic acetylcholine receptors that protect keratinocytes from apoptolysis. Int. Immunopharmacol. 29 (1), 76–80. doi:10.1016/j.intimp.2015.04.046
Chiarugi, P., Pani, G., Giannoni, E., Taddei, L., Colavitti, R., Raugei, G., et al. (2003). Reactive oxygen species as essential mediators of cell adhesion: the oxidative inhibition of a FAK tyrosine phosphatase is required for cell adhesion. J. Cell Biol. 161, 933–944. doi:10.1083/jcb.200211118
Chipuk, J., and Green, D. (2008). How do BCL-2 proteins induce mitochondrial outer membrane permeabilization? Trends Cell Biol. 18 (4), 157–164. doi:10.1016/j.tcb.2008.01.007
Choi, I., Kim, J., Jeong, H., Kim, B., Jou, I., Park, S., et al. (2013). PINK1 deficiency attenuates astrocyte proliferation through mitochondrial dysfunction, reduced AKT and increased p38 MAPK activation, and downregulation of EGFR. Glia 61 (5), 800–812. doi:10.1002/glia.22475
Choi, J., Hong, G., Kwon, T., and Lim, J. (2018). Fabrication of oxygen releasing scaffold by embedding H2O2-PLGA microspheres into alginate-based hydrogel sponge and its application for wound healing. Appl. Sci. 8 (9), 1492. doi:10.3390/app8091492
Chung, N., Park, J., and Lim, K. (2017). The effects of exercise and cold exposure on mitochondrial biogenesis in skeletal muscle and white adipose tissue. J. Exerc. Nutr. Biochem. 21, 39–47. doi:10.20463/jenb.2017.0020
Ciciliot, S., and Schiaffino, S. (2010). Regeneration of mammalian skeletal muscle. Basic mechanisms and clinical implications. Curr. Pharm. Des. 16 (8), 906–914. doi:10.2174/138161210790883453
Croteau, D., Rossi, M., Canugovi, C., Tian, J., Sykora, P., Ramamoorthy, M., et al. (2012). RECQL4 localizes to mitochondria and preserves mitochondrial DNA integrity. Aging Cell 11 (3), 456–466. doi:10.1111/j.1474-9726.2012.00803.x
Dale, R., Gornall, H., Singh-Grewal, D., Alcausin, M., Rice, G., and Crow, Y. (2010). Familial Aicardi-Goutières syndrome due to SAMHD1 mutations is associated with chronic arthropathy and contractures. Am. J. Med. Genet. A 152A (4), 938–942. doi:10.1002/ajmg.a.33359
D’Amico, D., Fouassier, A., Faitg, J., Hennighausen, N., Brandt, M., Konstantopoulos, D., et al. (2023). Topical application of urolithin A slows intrinsic skin aging and protects from UVB-mediated photodamage: findings from randomized clinical trials. https://www.medrxiv.org/content/10.1101/2023.06.16.23291378v1#:∼:text=In%20the%20third%20trial%2C%20investigating,well%2Dtolerated%20in%20all%20studies.
Dang, E., Mcdonald, J. G., Russell, D., and Cyster, J. (2017). Oxysterol restraint of cholesterol synthesis prevents AIM2 inflammasome activation. Cell 171, 1057–1071.e11. doi:10.1016/j.cell.2017.09.029
David, J., Rifkin, W., Rabbani, P., and Ceradini, D. (2017). The nrf2/keap1/ARE pathway and oxidative stress as a therapeutic target in type II diabetes mellitus. J. Diabetes Res. 2017, 4826724. doi:10.1155/2017/4826724
De Bock, K., Georgiadou, M., Schoors, S., Kuchnio, A., Wong, B. W., Cantelmo, A. R., et al. (2013). Role of PFKFB3-driven glycolysis in vessel sprouting. Cell 154, 651–663. doi:10.1016/j.cell.2013.06.037
De Brito, O., and Scorrano, L. (2008). Mitofusin 2 tethers endoplasmic reticulum to mitochondria. Nature 456, 605–610. doi:10.1038/nature07534
Deberardinis, R. E. A., Mancuso, A., Daikhin, E., Nissim, I., Yudkoff, M., Wehrli, S., et al. (2007). Beyond aerobic glycolysis: transformed cells can engage in glutamine metabolism that exceeds the requirement for protein and nucleotide synthesis. Proc. Natl. Acad. Sci. U. S. A. 104, 19345–19350. doi:10.1073/pnas.0709747104
Decker, P., Singh-Jasuja, H., Haager, S., Kotter, I., and Rammensee, H. G. (2005). Nucleosome the main autoantigen in systemic lupus erythematosus, induces direct dendritic cell activation via a MyD88-independent pathway: consequences on inflammation. J. Immunol. 174, 3326–3334. doi:10.4049/jimmunol.174.6.3326
Decout, A., Katz, J., Venkatraman, S., and Ablasser, A. (2021). The cGAS-STING pathway as a therapeutic target in inflammatory diseases. Nat. Rev. Immunol. 21, 548–569. doi:10.1038/s41577-021-00524-z
Demyanenko, I., Popova, E., Zakharova, V., Pletjushkina, O., Vasilieva, T. V., Romashchenko, V. P., et al. (2015). Mitochondria-targeted antioxidant SkQ1 improves impaired dermal wound healing in old mice. Aging (Albany NY) 7 (7), 475–485. doi:10.18632/aging.100772
Demyanenko, I., Zakharova, V., Ilyinskaya, O., Vasilieva, T., Fedorov, A., Manskikh, V., et al. (2017). Mitochondria-targeted antioxidant SkQ1 improves dermal wound healing in genetically diabetic mice. Oxid. Med. Cell Longev. 2017, 6408278. doi:10.1155/2017/6408278
Desmoulière, A., Redard, M., Darby, I., and Gabbiani, G. (1995). Apoptosis mediates the decrease in cellularity during the transition between granulation tissue and scar. Am. J. Pathol. 146 (1), 56–66.
Dhall, S., Garcia, M., Kim, J., Mirebrahim, S. H., and Lyubovitsky, J., (2014). Generating and reversing chronic wounds in diabetic mice by manipulating wound redox parameters. J. Diabetes Res. 2014, 562625. doi:10.1155/2014/562625
Dhir, A., Al, E., Borowski, L. S., Jimenez, L., Teitell, M., Rötig, A., et al. (2018). Mitochondrial double-stranded RNA triggers antiviral signalling in humans. Nature 560, 238–242. doi:10.1038/s41586-018-0363-0
Di Zenzo, G., Di Lullo, G., Corti, D., Lanzavecchia, A., Sinistro, A., Vanzetta, F., et al. (2012). Pemphigus autoantibodies generated through somatic mutations target the desmoglein-3 cis-interface. J. Clin. Invest 122 (10), 3781–3790. doi:10.1172/JCI64413
Diebold, L., Gil, H. J., Gao, P., Martinez, C. A., Weinberg, S. E., and Chandel, N. S. (2019). Mitochondrial complex III is necessary for endothelial cell proliferation during angiogenesis. Nat. Metab. 1, 158–171. doi:10.1038/s42255-018-0011-x
Dieudé, M., AL, E., Tyznik, A. J., Wang, J., Behar, S. M., Piccirillo, C. A., et al. (2011). Cardiolipin binds to CD1d and stimulates CD1d-restricted γδ T cells in the normal murine repertoire. J. Immunol. 186, 4771–4781. doi:10.4049/jimmunol.1000921
Ding, X., Kakanj, P., Leptin, M., and Eming, S. (2021). Regulation of the wound healing response during aging. J. Invest Dermatol 141 (4S), 1063–1070. doi:10.1016/j.jid.2020.11.014
Divakaruni, A., Wiley, S. E., Rogers, G. W., Andreyev, A. Y., Petrosyan, S., Loviscach, M., et al. (2013). Thiazolidinediones are acute, specific inhibitors of the mitochondrial pyruvate carrier. Proc. Natl. Acad. Sci. U. S. A. 110, 5422–5427. doi:10.1073/pnas.1303360110
Doblado, L., Lueck, C., Rey, C., Samhan-Arias, A., Prieto, I., Stacchiotti, A., et al. (2021). Mitophagy in human diseases. Int. J. Mol. Sci. 22 (8), 3903. doi:10.3390/ijms22083903
Domenech, E., Maestre, C., Esteban-Martinez, L., Partida, D., Pascual, R., Fernández-Miranda, G., et al. (2015). AMPK and PFKFB3 mediate glycolysis and survival in response to mitophagy during mitotic arrest. Nat. Cell Biol. 17, 1304–1316. doi:10.1038/ncb3231
Domizio, J., AL, E., Saidoune, F., Thacker, V. V., Yatim, A., Sharma, K., et al. (2022). The cGAS-STING pathway drives type I IFN immunopathology in COVID-19. Nature 603, 145–151. doi:10.1038/s41586-022-04421-w
D’Orazio, J., Jarrett, S., Amaro-Ortiz, A., and Scott, T. (2013). UV radiation and the skin. Int. J. Mol. Sci. 14, 12222–12248. doi:10.3390/ijms140612222
Dragoni, F., Garau, J., Orcesi, S., Gagliardi, S., Bordoni, M., Scarian, E., et al. (2023). Comparison between D-loop methylation and mtDNA copy number in patients with Aicardi-Goutières Syndrome. Front. Endocrinol. (Lausanne) 14, 1152237. doi:10.3389/fendo.2023.1152237
Dragoni, F., Garau, J., Sproviero, D., Orcesi, S., Varesio, C., De Siervi, S., et al. (2022). Characterization of mitochondrial alterations in aicardi-goutières patients mutated in RNASEH2A and RNASEH2B genes. Int. J. Mol. Sci. 23 (22), 14482. doi:10.3390/ijms232214482
Dunham-Snary, K., Surewaard, B., Mewburn, J., Bentley, R., Martin, A., Jones, O., et al. (2022). Mitochondria in human neutrophils mediate killing of staphylococcus aureus. Redox Biol. 49, 102225. doi:10.1016/j.redox.2021.102225
Dunn, L., Prosser, H., Tan, J., Vanags, L. Z., Ng, M. K. C., and Bursill, C. A. (2013). Murine model of woundhealing. J. Vis. Exp. 75, e50265. doi:10.3791/50265
Dunnill, C., Patton, T., Brennan, J., Barrett, J., Dryden, M., Cooke, J., et al. (2017). Reactive oxygen species (ROS) and wound healing: the functional role of ROS and emerging ROS-modulating technologies for augmentation of the healing process. Int. Wound J. 14, 89–96. doi:10.1111/iwj.12557
Duscher, D., Longaker, M., Gurtner, G., Whittam, A. J., Hu, M. S., Walmsley, G. G., et al. (2017). Comparison of the hydroxylase inhibitor dimethyloxalylglycine and the iron chelator deferoxamine in diabetic and aged wound healing. Plastic Reconstr. Surg. 139 (3), 695e–706e. doi:10.1097/PRS.0000000000003072
Eckes, B., Nischt, R., and Krieg, T. (2023). Cell-matrix interactions in dermal repair and scarring. Fibrogenes. Tissue Repair 3, 4. doi:10.1186/1755-1536-3-4
Eelen, G., de Zeeuw, P., Treps, L., Harjes, U., Wong, B. W., and Carmeliet, P. (2018). Endothelial cell metabolism. Physiol. Rev. 98, 3–58. doi:10.1152/physrev.00001.2017
Elliott, M., AL, E., Trampont, P. C., Lazarowski, E. R., Kadl, A., Walk, S. F., et al. (2009). Nucleotides released by apoptotic cells act as a find-me signal to promote phagocytic clearance. Nature 461, 282–286. doi:10.1038/nature08296
Eming, S., Krieg, T., and Davidson, J. (2007). Inflammation in wound repair: molecular and cellular mechanisms. J. Invest Dermatol 127 (3), 514–525. doi:10.1038/sj.jid.5700701
Eming, S., Martin, P., and Tomic-Canic, M. (2014). Wound repair and regeneration: mechanisms, signaling, and translation. Sci. Transl. Med. 6, 265sr6. doi:10.1126/scitranslmed.3009337
Eming, S., Murray, P., and Pearce, E. (2021). Metabolic orchestration of the wound healing response. Cell Metab. 33 (9), 1726–1743. doi:10.1016/j.cmet.2021.07.017
Eming, S., Wynn, T., and Martin, P. (2017). Inflammation and metabolism in tissue repair and regeneration. Science 56 (6342), 1026–1030. doi:10.1126/science.aam7928
Esteban-Martinez, L., Domenech, E., Boya, P., Salazar-Roa, M., and Malumbres, M. (2015). Mitophagy in mitosis: more than a myth. Autophagy 11, 2379–2380. doi:10.1080/15548627.2015.1108509
Esteban-Martínez, L., Sierra-Filardi, E., Mcgreal, R., Salazar-Roa, M., Mariño, G., Seco, E., et al. (2017). Programmed mitophagy is essential for the glycolytic switch during cell differentiation. EMBO J. 36 (12), 1688–1706. doi:10.15252/embj.201695916
Fadini, G., Menegazzo, L., Rigato, M., Scattolini, V., Poncina, N., Bruttocao, A., et al. (2016). NETosis delays diabetic wound healing in mice and humans. Diabetes 65, 1061–1071. doi:10.2337/db15-0863
Falanga, V., Isseroff, R., Soulika, A., Romanelli, M., Margolis, D., Kapp, S., et al. (2022). Chronic wounds. Nat. Rev. Dis. Prim. 8, 50. doi:10.1038/s41572-022-00377-3
Falanga, V., and Kirsner, R. (1993). Low oxygen stimulates proliferation of fibroblasts seeded as single cells. J. Cell Physiol. 154, 506–510. doi:10.1002/jcp.1041540308
Fang, E., Hou, Y., Lautrup, S., Jensen, M., Bohr, V., SenGupta, T., et al. (2019). NAD + augmentation restores mitophagy and limits accelerated aging in Werner syndrome. Nat. Commun. 10 (1), 5284. doi:10.1038/s41467-019-13172-8
Fang, S., Roan, J. N., Lee, J. S., Chiu, M. H., Lin, M. W., Liu, C. C., et al. (2021). Transplantation of viable mitochondria attenuates neurologic injury after spinal cord ischemia. J. Thorac. Cardiovasc. Surg. 161 (5), e337–e347. doi:10.1016/j.jtcvs.2019.10.151
Feng, Y., Li, L., Zhang, Q., He, Y., Huang, Y., Zhang, J., et al. (2023). Mitophagy associated self-degradation of phosphorylated MAP4 guarantees the migration and proliferation responses of keratinocytes to hypoxia. Cell Death Discov. 9 (1), 168. doi:10.1038/s41420-023-01465-3
Feng, Z., Chen, J., Yuan, P., Ji, Z., Tao, S., Zheng, L., et al. (2022). Urolithin A promotes angiogenesis and tissue regeneration in a full-thickness cutaneous wound model. Front. Pharmacol. 13, 806284. doi:10.3389/fphar.2022.806284
Flores-Romero, H., AL, E., John, M., Albert, M. C., King, L. E., Beckmann, L., et al. (2022). BCL-2-family protein tBID can act as a BAX-like effector of apoptosis. EMBO J. 41, e108690. doi:10.15252/embj.2021108690
Fu, H., Zhou, H., Yu, X., Xu, J., Zhou, J., Meng, X., et al. (2020). Wounding triggers MIRO-1 dependent mitochondrial fragmentation that accelerates epidermal wound closure through oxidative signaling. Nat. Commun. 11, 1050. doi:10.1038/s41467-020-14885-x
Fuchs, Y., and Steller, H. (2015). Live to die another way: modes of programmed cell death and the signals emanating from dying cells. Nat. Rev. Mol. Cell Biol. 16, 329–344. doi:10.1038/nrm3999
Fukai, T., and Ushio-Fukai, M. (2020). Cross-talk between NADPH oxidase and mitochondria: role in ROS signaling and angiogenesis. Cells 9 (8), 1849. doi:10.3390/cells9081849
Fung, T., Chakrabarti, R., and Higgs, H. (2023). Publisher Correction: the multiple links between actin and mitochondria. Nat. Rev. Mol. Cell Biol. 24, 688. doi:10.1038/s41580-023-00632-9
Gabr, S., and AL-Ghadir, A. (2012). Role of cellular oxidative stress and cytochrome c in the pathogenesis of psoriasis. Arch. Dermatol Res. 304 (6), 451–457. doi:10.1007/s00403-012-1230-8
Gauron, C., Rampon, C., Bouzaffour, M., Ipendey, E., Teillon, J., Volovitch, M., et al. (2013). Sustained production of ROS triggers compensatory proliferation and is required for regeneration to proceed. Sci. Rep. 3, 2084. doi:10.1038/srep02084
Gerber, P., Buhren, B., Schrumpf, H., Homey, B., Zlotnik, A., and Hevezi, P. (2014). The top skin-associated genes: A comparative analysis of human and mouse skin transcriptomes. Biol. Chem. 395, 577–591. doi:10.1515/hsz-2013-0279
Ghiasi, M., Chen, J., Vaziri, A., Rodriguez, E., and Nazarian, A. (2017). Bone fracture healing in mechanobiological modeling: A review of principles and methods. Bone Rep. 6, 87–100. doi:10.1016/j.bonr.2017.03.002
Ghiringhelli, F., AL, E., Tesniere, A., Aymeric, L., Ma, Y., Ortiz, C., et al. (2009). Activation of the NLRP3 inflammasome in dendritic cells induces IL-1beta-dependent adaptive immunity against tumors. Nat. Med. 15, 1170–1178. doi:10.1038/nm.2028
Gillitzer, R., and Goebeler, M. (2001). Chemokines in cutaneous wound healing. J. Leukoc. Biol. 69 (4), 513–521. doi:10.1189/jlb.69.4.513
Gonçalves, R., Souza, N., Silva, P., Barbosa, F., and Neves, C. (2010). Influência do laser arseneto de gálio-alumínio em feridas cutâneas de ratos. Fisoter Mov. 23, 381–388. doi:10.1590/s0103-51502010000300005
Gonzalez, A., Costa, T., Andrade, Z., and Medrado, A. (2016). Wound healing - a literature review. An Bras Dermatol 91 (5), 614–620. doi:10.1590/abd1806-4841.20164741
Gorgoulis, V., Adams, P., Alimonti, A., Bennett, D., Bischof, O., Bishop, C., et al. (2019). Cellular senescence: defining a path forward. Cell 179, 813–827. doi:10.1016/j.cell.2019.10.005
Goswami, S., Kandhare, A., Zanwar, A., Hegde, M., Bodhankar, S., Shinde, S., et al. (2016). Oral L-glutamine administration attenuated cutaneous wound healing in Wistar rats. Int. Wound J. 13 (1), 116–124. doi:10.1111/iwj.12246
Gouveia, A., Bajwa, E., and Klegeris, A. (2017). Extracellular cytochrome c as an intercellular signaling molecule regulating microglial functions. Biochim. Biophys. Acta Gen. Subj. 1861, 2274–2281. doi:10.1016/j.bbagen.2017.06.017
Green, C., Wallace, M., Divakaruni, A. S., Phillips, S. A., Murphy, A. N., Ciaraldi, T. P., et al. (2016). Branched-chain amino acid catabolism fuels adipocyte differentiation and lipogenesis. Nat. Chem. Biol. 12, 15–21. doi:10.1038/nchembio.1961
Greenhalgh, D. (1998). The role of apoptosis in wound healing. Int. J. Biochem. Cell Biol. 30 (9), 1019–1030. doi:10.1016/s1357-2725(98)00058-2
Guo, Z., Kozlov, S., Lavin, M., Person, M., and Paull, T. (2010). ATM activation by oxidative stress. Science 330 (6003), 517–521. doi:10.1126/science.1192912
Gudmundsrud, R., Skjånes, T., Gilmour, B., Caponio, D., Lautrup, S., and Fang, E. (2021). Crosstalk among DNA damage, mitochondrial dysfunction, impaired mitophagy, stem cell attrition, and senescence in the accelerated ageing disorder werner syndrome. Cytogenet Genome Res. 161 (6-7), 297–304. doi:10.1159/000516386
Gugnoni, M., Sancisi, V., Manzotti, G., Gandolfi, G., and Ciarrocchi, A. (2016). Autophagy and epithelial-mesenchymal transition: an intricate interplay in cancer. Cell Death Dis. 7 (12), e2520. doi:10.1038/cddis.2016.415
Guo, W., Sun, Y., Liu, W., Wu, X., Guo, L., Cai, P., et al. (2014). Small molecule-driven mitophagy-mediated NLRP3 inflammasome inhibition is responsible for the prevention of colitis-associated cancer. Autophagy 10 (6), 972–985. doi:10.4161/auto.28374
Gupta, S., Sharp, R., Hofferek, C., Kuai, L., Dorn, G., Wang, J., et al. (2019). NIX mediated Mitophagy promotes effector memory formation in antigenspecific CD8(+) T cells. Cell Rep. 29 (7), 1862–1877. doi:10.1016/j.celrep.2019.10.032
Gurung, P., Lukens, J., and Kanneganti, T. (2015). Mitochondria: diversity in the regulation of the NLRP3 inflammasome. Trends Mol. Med. 21 (3), 193–201. doi:10.1016/j.molmed.2014.11.008
Guzy, R., Hoyos, B., Robin, E., Chen, H., Liu, L., Mansfield, K., et al. (2005). Mitochondrial complex III is required for hypoxia-induced ROS production and cellular oxygen sensing. Cell Metab. 1, 401–408. doi:10.1016/j.cmet.2005.05.001
Gyrd-Hansen, M., and Meier, P. (2010). IAPs: from caspase inhibitors to modulators of NF-kappaB, inflammation and cancer. Nat. Rev. Cancer 10, 561–574. doi:10.1038/nrc2889
Haensel, D., Jin, S., Sun, P., Cinco, R., Dragan, M., Nguyen, Q., et al. (2020). Defining epidermal basal cell states during skin homeostasis and wound healing using single-cell transcriptomics. Cell Rep. 30 (11), 3932–3947. doi:10.1016/j.celrep.2020.02.091
Hall, C., Boyle, R., Sun, X., Wicker, S., Misa, J., Krissansen, G., et al. (2014). Epidermal cells help coordinate leukocyte migration during inflammation through fatty acid-fuelled matrix metalloproteinase production. Nat. Commun. 5, 3880. doi:10.1038/ncomms4880
Hatzivassiliou, G., Zhao, F., Bauer, D. E., Andreadis, C., Shaw, A. N., Dhanak, D., et al. (2005). ATP citrate lyase inhibition can suppress tumor cell growth. Cancer Cell 8, 311–321. doi:10.1016/j.ccr.2005.09.008
He, B., Yu, H., Liu, S., Wan, H., Fu, S., Liu, S., et al. (2022). Mitochondrial cristae architecture protects against mtDNA release and inflammation. Cell Rep. 41 (10), 111774. doi:10.1016/j.celrep.2022.111774
Heiden, M., Li, X., Gottleib, E., Hill, R., Thompson, C., and Colombini, M. (2001). Bcl-x l promotes the open configuration of the voltage-dependent anion channel and metabolite passage through the outer mitochondrial membrane. J. Biol. Chem. 276 (22), 19414–19419. doi:10.1074/jbc.M101590200
Hennings, H., and Holbrook, K. (1983). Calcium regulation of cell-cell contact and differentiation of epidermal cells in culture: an ultrastructural study. Exp. Cell Res. 143, 127–142. doi:10.1016/0014-4827(83)90115-5
Herst, P., Rowe, M., Carson, G., and Berridge, M. (2017). Functional mitochondria in health and disease. Front. Endocrinol. (Lausanne) 8, 296. doi:10.3389/fendo.2017.00296
Hesketh, M., Sahin, K., West, Z., and Murray, R. (2017). Macrophage phenotypes regulate scar formation and chronic wound healing. Int. J. Mol. Sci. 18, 1545. doi:10.3390/ijms18071545
Hetz, C. (2012). The unfolded protein response: controlling cell fate decisions under ER stress and beyond. Nat. Rev. Mol. Cell Biol. 13, 89–102. doi:10.1038/nrm3270
Hiebert, P., Wietecha, M., Cangkrama, M., Haertel, E., Mavrogonatou, E., Stumpe, M., et al. (2018). Nrf2-mediated fibroblast reprogramming drives cellular senescence by targeting the matrisome. Dev. Cell 46, 145–161.e10. doi:10.1016/j.devcel.2018.06.012
Hinz, B., Phan, S., Thannickal, V., Galli, A., B Ochaton-Piallat, M., and Gabbiani, G. (2007). The myofibroblast: one function, multiple origins. Am. J. Pathol. 170 (6), 1807–1816. doi:10.2353/ajpath.2007.070112
Hockenbery, D., Nuñez, G., Milliman, C., Schreiber, R., and Korsmeyer, S. (1990). Bcl-2 is an inner mitochondrial membrane protein that blocks programmed cell death. Nature 348 (6299), 334–336. doi:10.1038/348334a0
Hoffmann, M., and Griffiths, H. (2018). The dual role of ROS in autoimmune and inflammatory diseases: evidence from preclinical models. Free Radic. Biol. Med. 2018. doi:10.1016/j.freeradbiomed.2018.03.016
Holzer-Geissler, J., Schwingenschuh, S., Zacharias, M., Einsiedler, J., Kainz, S., Reisenegger, P., et al. (2022). The impact of prolonged inflammation on wound healing. Biomedicines 10 (4), 856. doi:10.3390/biomedicines10040856
Houtkooper, R., Mouchiroud, L., Ryu, D., Moullan, N., Katsyuba, E., Knott, G., et al. (2013). Mitonuclear protein imbalance as a conserved longevity mechanism. Nature 497, 451–457. doi:10.1038/nature12188
Huang, Y., Wang, T., Sun, J., and Lin, F. (2006). Effect of calcium ion concentration on keratinocyte behaviors in the defined media. Biomed. Eng. 18, 37–41. doi:10.4015/s1016237206000087
Hunter, M., Willoughby, P., Aee, B., and Fernandez-Gonzalez, R. (2018). Oxidative stress orchestrates cell polarity to promote embryonic wound healing. Dev. Cell 47 (3), 377–387. doi:10.1016/j.devcel.2018.10.013
Hur, S. (2019). Double-stranded RNA sensors and modulators in innate immunity. Annu. Rev. Immunol. 37, 349–375. doi:10.1146/annurev-immunol-042718-041356
Hurd, T., Degennaro, M., and Lehmann, R. (2012). Redox regulation of cell migration and adhesion. Trends Cell Biol. 22 (2), 107–115. doi:10.1016/j.tcb.2011.11.002
Hussain, M., Krishnamurthy, S., Patel, J., Kim, E., Baptiste, B., Croteau, D., et al. (2021). Skin abnormalities in disorders with DNA repair defects, Premature aging, and mitochondrial dysfunction. J. Invest Dermatol 141 (4S), 968–975. doi:10.1016/j.jid.2020.10.019
Hutchison, D., Hosking, A., Hong, E., and Grando, S. (2022). Mitochondrial autoantibodies and the role of apoptosis in pemphigus vulgaris. Antibodies (Basel) 11 (3), 55. doi:10.3390/antib11030055
Im, M., and Hoopes, J. (1970). Energy metabolism in healing skin wounds. J. Surg. Res. 10 (10), 459–464. doi:10.1016/0022-4804(70)90070-3
Ipponjima, S., Umino, Y., Nagayama, M., and Denda, M. (2020). Live imaging of alterations in cellular morphology and organelles during cornification using an epidermal equivalent model. Sci. Rep. 10 (1), 5515. doi:10.1038/s41598-020-62240-3
Ishihara, N., Fujita, Y., Oka, T., and Mihara, K. (2006). Regulation of mitochondrial morphology through proteolytic cleavage of OPA1. EMBO J. 25, 2966–2977. doi:10.1038/sj.emboj.7601184
Ito, D., Ito, H., Ideta, T., Kanbe, A., Ninomiya, S., and Shimizu, M. (2021). Systemic and topical administration of spermidine accelerates skin wound healing. Cell Commun. Signal 19 (1), 36. doi:10.1186/s12964-021-00717-y
Ito, H., Kanbe, A., Sakai, H., and Seishima, M. (2018). Activation of NLRP3 signalling accelerates skin wound healing Exp. Dermatol 27, 80–86. doi:10.1111/exd.13441
Jacinto, A., Martinez-Arias, A., and Martin, P. (2001). Mechanisms of epithelial fusion and repair. Nat. Cell Biol. 3 (5), E117–E123. doi:10.1038/35074643
Jacobs, J., and Coyne, C. (2013). Mechanisms of MAVS regulation at the mitochondrial membrane. J. Mol. Biol. 425 (24), 5009–5019. doi:10.1016/j.jmb.2013.10.007
Jakobs, S., Martini, N., Schauss, A., Egner, A., Westermann, B., and Hell, S. (2003). Spatial and temporal dynamics of budding yeast mitochondria lacking the division component Fis1p. J. Cell Sci. 116 (10), 2005–2014. doi:10.1242/jcs.00423
Janda, J., Nfonsam, V., Calienes, F., Sligh, J., and Jandova, J. (2016). Modulation of ROS levels in fibroblasts by altering mitochondria regulates the process of wound healing. Arch. Dermatol Res. 308 (4), 239–248. doi:10.1007/s00403-016-1628-9
Januszyk, M., Chen, K., Henn, D., Foster, D. S., Borrelli, M. R., Bonham, C. A., et al. (2020). Characterization of diabetic and non-diabetic foot ulcers using single-cell RNA-sequencing. Micromachines 11, 815. doi:10.3390/mi11090815
Jones, J., Ramser, H., Woessner, A., Veves, A., and Quinn, K. (2020). Quantifying age-related changes in skin wound metabolism using in vivo multiphoton microscopy. Adv. Wound Care 9 (3), 90–102. doi:10.1089/wound.2019.1030
Jun, J., and Lau, L. (2017). CCN2 induces cellular senescence in fibroblasts. J. Cell Commun. Signal 11, 15–23. doi:10.1007/s12079-016-0359-1
Kalantari-Dehaghi, M., Chen, Y., Deng, W., Chernyavsky, A., Marchenko, S., Wang, P., et al. (2013). Mechanisms of mitochondrial damage in keratinocytes by pemphigus vulgaris antibodies. J. Biol. Chem. 288 (23), 16916–16925. doi:10.1074/jbc.M113.472100
Kane, C., and Greenhalgh, D. (2000). Expression and localization of p53 and bcl-2 in healing wounds in diabetic and nondiabetic mice. Wound Repair Regen. 8 (1), 45–58. doi:10.1046/j.1524-475x.2000.00045.x
Kaneko, N., Vierkoetter, A., Kraemer, U., Sugiri, D., Matsui, M., Yamamoto, A., et al. (2012). Mitochondrial common deletion mutation and extrinsic skin ageing in German and Japanese women. Exp. Dermatol. 21, 26–30. doi:10.1111/j.1600-0625.2012.01499.x
Karczewski, K., and Snyder, M. (2018). Integrative omics for health and disease. Nat. Rev. Genet. 19 (5), 299–310. doi:10.1038/nrg.2018.4
Kerr, J., Wyllie, A., and Currie, A. (1972). Apoptosis: A basic biological phenomenon with wide-ranging implications in tissue knetics. Brit J. Cancer 26 (4), 239–257. doi:10.1038/bjc.1972.33
Keyes, B., Liu, S., Asare, A., Naik, S., Levorse, J., Polak, L., et al. (2016). Impaired epidermal to dendritic T cell signaling slows wound repair in aged skin. Cell 167 (5), 1323–1338.e14. doi:10.1016/j.cell.2016.10.052
Kim, B., Li, J., Jang, C., and Arany, Z. (2017). Glutamine fuels proliferation but not migration of endothelial cells. EMBO J. 36, 2321–2333. doi:10.15252/embj.201796436
Kim, E., and Choi, E. (2015). Compromised MAPK signaling in human diseases: an update. Arch. Toxicol. 89, 867–882. doi:10.1007/s00204-015-1472-2
Kim, J., AL, E., Blanco, L. P., Yang, S., Shteinfer-Kuzmine, A., Wang, K., et al. (2019). VDAC oligomers form mitochondrial pores to release mtDNA fragments and promote lupus-like disease. Science 366, 1531–1536. doi:10.1126/science.aav4011
Kim, K., Son, J., Benayoun, B., and Lee, C. (2018). The mitochondrial-encoded peptide MOTS-c translocates to the nucleus to regulate nuclear gene expression in response to metabolic stress. Cell Metab. 28, 516–524.e7. doi:10.1016/j.cmet.2018.06.008
Kim, M., Bae, S., Ryu, J., Kwon, Y., Oh, J., Kwon, J., et al. (2016). SESN2/sestrin2 suppresses sepsis by inducing mitophagy and inhibiting NLRP3 activation in macrophages. Autophagy 12 (8), 1272–1291. doi:10.1080/15548627.2016.1183081
Kinnally, K., Peixoto, P., Ryu, S.-Y., and Dejean, L. (2011). Is mPTP the gatekeeper for necrosis, apoptosis, or both? Biochimica Biophysica Acta Bba - Mol Cell Res. 1813 (4), 616–622. doi:10.1016/j.bbamcr.2010.09.013
Kirchner, S., Lei, V., and Macleod, A. (2020). The cutaneous wound innate immunological microenvironment. Int. J. Mol. Sci. 21, 8748. doi:10.3390/ijms21228748
Klimova, T., and Chandel, N. (2008). Mitochondrial complex III regulates hypoxic activation of HIF. Cell Death Differ. 15, 660–666. doi:10.1038/sj.cdd.4402307
Klyubin, I., Kirpichnikova, K. M., and Gamaley, I. A. (1996). Hydrogen peroxide-induced chemotaxis of mouse peritoneal neutrophils. Eur. J. Cell Biol. 70, 347–351.
Knighton, D., Hunt, T., Scheuenstuhl, H., Halliday, B., Werb, Z., and Banda, M. (1983). Oxygen tension regulates the expression of angiogenesis factor by macrophages. Science 221, 1283–1285. doi:10.1126/science.6612342
Ko, K., Arora, P., Bhide, V., Chen, A., and Mcculloch, C. (2001). Cell-cell adhesion in human fibroblasts requires calcium signaling. J. Cell Sci. 114, 1155–1167. doi:10.1242/jcs.114.6.1155
Kobayashi, T., Naik, S., and Nagao, K. (2019). Choreographing immunity in the skin epithelial barrier. Immunity 50 (3), 552–565. doi:10.1016/j.immuni.2019.02.023
Kondo, Y., Ledderose, C., Slubowski, C., Fakhari, M., Sumi, Y., Sueyoshi, K., et al. (2019). Frontline science: escherichia coli use LPS as decoy to impair neutrophil chemotaxis and defeat antimicrobial host defense. J. Leukoc. Biol. 106 (6), 1211–1219. doi:10.1002/JLB.4HI0319-109R
Krishnan, K., Harbottle, A., and Birch-Machin, M. (2005). The use of a 3895 bp mitochondrial DNA deletion as a marker for sunlight exposure in human skin. J. Invest. Dermatol. 123, 1020–1024. doi:10.1111/j.0022-202X.2004.23457.x
Krisp, C., Jacobsen, F., Mckay, M., Molloy, M. P., Steinstraesser, L., and Wolters, D. A. (2013). Proteome analysis reveals antiangiogenic environ-ments in chronic wounds of diabetes mellitus type 2 patients. Proteomics 13 (17), 2670–2681. doi:10.1002/pmic.201200502
Krizhanovsky, V., Yon, M., Dickins, R., Hearn, S., Simon, J., Miething, C., et al. (2008). Senescence of activated stellate cells limits liver fibrosis. Cell 134, 657–667. doi:10.1016/j.cell.2008.06.049
Kroemer, G., Galassi, C., Zitvogel, L., and Galluzzi, L. (2022). Immunogenic cell stress and death. Nat. Immunol. 23, 487–500. doi:10.1038/s41590-022-01132-2
Kroemer, G., Galluzzi, L., and Brenner, C. (2007). Mitochondrial membrane permeabilization in cell death. Physiol. Rev. 87 (1), 99–163. doi:10.1152/physrev.00013.2006
Kroemer, G., Petit, P., Zamzami, N., Vayssière, J., and Mignotte, B. (1995). The biochemistry of programmed cell death. Faseb J. 9 (13), 1277–1287. doi:10.1096/fasebj.9.13.7557017
Kunkemoeller, B., and Kyriakides, T. (2017). Redox signaling in diabetic wound healing regulates extracellular matrix deposition. Antioxid. Redox Signal 27, 823–838. doi:10.1089/ars.2017.7263
Kwon, J., Wang, A., Burke, D., Boudreau, H., Lekstrom, K., Korzeniowska, A., et al. (2016). Peroxiredoxin 6 (Prdx6) supports NADPH oxidase1 (Nox1)-based superoxide generation and cell migration. Free Radic. Biol. Med. 96, 99–115. doi:10.1016/j.freeradbiomed.2016.04.009
Labbé, A., Lafleur, V., Patten, D., Robitaille, G., Garand, C., Lamalice, L., et al. (2012). The werner syndrome gene product (WRN): A repressor of hypoxia-inducible factor-1 activity. Exp. Cell Res. 318 (14), 1620–1632. doi:10.1016/j.yexcr.2012.04.010
Lamb, J., Ventura, J., Hess, P., Flavell, R., and Davis, R. (2003). JunD mediates survival signaling by the JNK signal transduction pathway. Mol. Cell 11, 1479–1489. doi:10.1016/s1097-2765(03)00203-x
Lambeth, J., and Neish, A. (2014). Nox enzymes and new thinking on reactive oxygen: A double-edged sword revisited. Annu. Rev. Pathol. 9, 119–145. doi:10.1146/annurev-pathol-012513-104651
Lampert, M., Orogo, A., Najor, R., Hammerling, B., Leon, L., Wang, B., et al. (2019). BNIP3L/NIX and FUNDC1-mediated mitophagy is required for mitochondrial network remodeling during cardiac progenitor cell differentiation. Autophagy 15 (7), 1182–1198. doi:10.1080/15548627.2019.1580095
Lansdown, A. (2002). Calcium: A potential central regulator in wound healing in the skin. Wound Repair Regen. 10 (5), 271–285. doi:10.1046/j.1524-475x.2002.10502.x
Law, J., Chowdhury, S., Aminuddin, B., and Ruszymah, B. (2015). Effect of calcium concentration on keratinocyte differentiation in 2-D culture and 3-D construct. Regen. Res. 4, 22–29.
Leshinsky-Silver, E., Malinger, G., Ben-Sira, L., Kidron, D., Cohen, S., Inbar, S., et al. (2011). A large homozygous deletion in the SAMHD1 gene causes atypical Aicardi-Goutiéres syndrome associated with mtDNA deletions. Eur. J. Hum. Genet. 19 (3), 287–292. doi:10.1038/ejhg.2010.213
Leslie, D., AL, E., Cembrola, K., Townes, M. A., Hava, D. L., Hugendubler, L. C., et al. (2008). Serum lipids regulate dendritic cell CD1 expression and function. Immunology 125, 289–301. doi:10.1111/j.1365-2567.2008.02842.x
Levigne, D., Modarressi, A., Krause, K., and Pittet-Cuenod, B. (2016). Nadph oxidase 4 deficiency leads to impaired wound repair and reduced dityrosine-crosslinking, but does not affect myofibroblast formation. Free Radic. Biol. Med. 96, 374–384. doi:10.1016/j.freeradbiomed.2016.04.194
Levine, B., and Kroemer, G. (2008). Autophagy in the pathogenesis of disease. Cell 132 (1), 27–42. doi:10.1016/j.cell.2007.12.018
Li, J., Zhang, Y., and Kirsner, R. (2003). Angiogenesis in wound repair: angiogenic growth factors and the extracellular matrix. Microsc. Res. Tech. 60 (1), 107–114. doi:10.1002/jemt.10249
Li, T., AL, E., Du, M., Chen, X., Du, F., Ren, J., et al. (2021). Phosphorylation and chromatin tethering prevent cGAS activation during mitosis. Science 371, eabc5386. doi:10.1126/science.abc5386
Li, X.-B., Gu, J.-D., and Zhou, Q.-H. (2014). Review of aerobic glycolysis and its key enzymes - new targets for lung cancer therapy. Thorac. Cancer 6, 17–24. doi:10.1111/1759-7714.12148
Lim, H., Rahim, A., Leo, V., Das, S., Lim, T., Uemura, T., et al. (2018). Polyamine regulator AMD1 promotes cell migration in epidermal wound healing. J. Invest Dermatol 138 (12), 2653–2665. doi:10.1016/j.jid.2018.05.029
Lima, T., Li, T., Mottis, A., and J, A. (2022). Pleiotropic effects of mitochondria in aging. Nat. Aging 2, 199–213. doi:10.1038/s43587-022-00191-2
Lin, H., Liu, S. Y., Lai, H. S., and Lai, I. R. (2013). Isolated mitochondria infusion mitigates ischemia-reperfusion injury of the liver in rats. Shock 39 (3), 304–310. doi:10.1097/SHK.0b013e318283035f
Liu, H., Ma, Y., He, H. W., Zhao, W., and Shao, R. (2017). SPHK1 (sphingosine kinase 1) induces epithelial-mesenchymal transition by promoting the autophagylinked lysosomal degradation of CDH1/E-cadherin in hepatoma cells. Autophagy 13, 900–913. doi:10.1080/15548627.2017.1291479
Liu, L., Feng, D., Chen, G., Chen, M., Zheng, Q., Song, P., et al. (2012). Mitochondrial outer-membrane protein FUNDC1 mediates hypoxia-induced mitophagy in mammalian cells. Nat. Cell Biol. 14 (2), 177–185. doi:10.1038/ncb2422
Liu, L., Liao, X., Wu, H., Li, Y., Zhu, Y., and Chen, Q. (2020). Mitophagy and its contribution to metabolic and aging-associated disorders. Antioxidants Redox Signal. 32, 906–927. doi:10.1089/ars.2019.8013
Liu, W., Yan, F., Xu, Z., Chen, Q., Ren, J., Wang, Q., et al. (2022). Urolithin A protects human dermal fibroblasts from UVA-induced photoaging through NRF2 activation and mitophagy. J. Photochem Photobiol. B 232, 112462. doi:10.1016/j.jphotobiol.2022.112462
Liu, X., Kim, C., Yang, J., Jemmerson, R., and Wang, X. (1996). Induction of apoptotic program in cell-free extracts: requirement for dATP and cytochrome c. Cell 86 (1), 147–157. doi:10.1016/s0092-8674(00)80085-9
Love, N., Chen, Y., Ishibashi, S., Kritsiligkou, P., Lea, R., Koh, Y., et al. (2013). Amputation-induced reactive oxygen species are required for successful Xenopus tadpole tail regeneration. Nat. Cell Biol. 15, 222–228. doi:10.1038/ncb2659
Lu, Z., and Xu, S. (2006). ERK1/2 MAP kinases in cell survival and apoptosis. IUBMB Life 58, 621–631. doi:10.1080/15216540600957438
Lucas, T., Waisman, A., Ranjan, R., Roes, J., Krieg, T., Müller, W., et al. (2010). Differential roles of macrophages in diverse phases of skin repair. J. Immunol. 184, 3964–3977. doi:10.4049/jimmunol.0903356
Lupfer, C., Thomas, P., Anand, P., Vogel, P., Milasta, S., Martinez, J., et al. (2013). Receptor interacting protein kinase 2-mediated mitophagy regulates inflammasome activation during virus infection. Nat. Immunol. 14 (5), 480–488. doi:10.1038/ni.2563
Ma, K., Chen, G., Li, W., Kepp, O., Zhu, Y., and Chen, Q. (2020). Mitophagy, mitochondrial homeostasis, and cell fate. Front. Cell Dev. Biol. 8, 467. doi:10.3389/fcell.2020.00467
Ma, Y., Xie, J., Wijaya, C., and Xu, S. (2021). From wound response to repair - lessons from C. elegans. elegans Cell Regen. 3 (1), 5. doi:10.1186/s13619-020-00067-z
Macheiner, T., Fengler, V. H. I., Agreiter, M., Eisenberg, T., Madeo, F., Kolb, D., et al. (2016). Magnetomitotransfer: an efficient way for direct mitochondria transfer into cultured human cells. Sci. Rep. 6, 35571. doi:10.1038/srep35571
Maes, H., Van Eygen, S., Krysko, D., Vandenabeele, P., Nys, K., Rillaerts, K., et al. (2014). BNIP3 supports melanoma cell migration and vasculogenic mimicry by orchestrating the actin cytoskeleton. Cell Death Dis. 5, e1127. doi:10.1038/cddis.2014.94
Makrantonaki, E., Wlaschek, M., and Scharffetter-Kochanek, K. (2017a). Pathogenesis of wound healing disorders in the elderly. JDDG J. der Deut Dermatol Gesell 15, 255–275. doi:10.1111/ddg.13199
Makrantonaki, E., Wlaschek, M., and Scharffetter-Kochanek, K. (2017b). Pathogenesis of wound healing disorders in the elderly. J. Dtsch. Dermatol. Ges. 15, 255–275. doi:10.1111/ddg.13199
Manchanda, M., Inuossa, F., Torres, M., Bansal, R., Kumar, R., Hunt, M., et al. (2023). Metabolic reprogramming and reliance in human skin wound healing. J. Investi Dermatol. doi:10.1016/j.jid.2023.02.039
Mannello, F., Ligi, D., Canale, M., and Raffetto, J. (2014). Omics profiles in chronic venous ulcer wound fluid: innovative applications for translational medicine. Expert Rev. Mol. Diagn 14 (6), 737–762. doi:10.1586/14737159.2014.927312
Marchetti, P., Castedo, M., Susin, S., Zamzami, N., Hirsch, T., Macho, A., et al. (1996). Mitochondrial permeability transition is a central coordinating event of apoptosis. J. Exp. Med. 184 (3), 1155–1160. doi:10.1084/jem.184.3.1155
Marchi, S., Guilbaud, E., Tait, S., Yamazaki, T., and Galluzzi, L. (2023). Mitochondrial control of inflammation. Nat. Rev. Immunol. 23 (3), 159–173. doi:10.1038/s41577-022-00760-x
Margulis, C. G. U., Chapman, M., Guerrero, R., and Hall, J. (2006). The last eukaryotic common ancestor (LECA): acquisition of cytoskeletal motility from aerotolerant spirochetes in the proterozoic eon. PNAS 103 (35), 13080–13085. doi:10.1073/pnas.0604985103
Martin, P. (1997). Wound healing—Aiming for perfect skin regeneration. Science 276 (5309), 75–81. doi:10.1126/science.276.5309.75
Martini, H., and Passos, J. (2022). Cellular senescence: all roads lead to mitochondria. FEBS J. 290, 1186–1202. doi:10.1111/febs.16361
Maselli, R., Arredondo, J., Cagney, O., Mozaffar, T., Skinner, S., Yousif, S., et al. (2011). Congenital myasthenic syndrome associated with epidermolysis bullosa caused by homozygous mutations in PLEC1 and CHRNE. Clin. Genet. 80 (5), 444–451. doi:10.1111/j.1399-0004.2010.01602.x
Mcarthur, K., AL, E., Heddleston, J. M., Li, L., Padman, B. S., Oorschot, V., et al. (2018). BAK/BAX macropores facilitate mitochondrial herniation and mtDNA efflux during apoptosis. Science 359, eaao6047. doi:10.1126/science.aao6047
Mccully, J., Cowan, D. B., Pacak, C. A., Toumpoulis, I. K., Dayalan, H., and Levitsky, S. (2009). Injection of isolated mitochondria during early reperfusion for cardioprotection. Am. J. Physiol. Heart Circ. Physiol. 296 (1), H94-H105. doi:10.1152/ajpheart.00567.2008
Mera, K., Kawahara, K., Tada, K., Kawai, K., Hashiguchi, T., Maruyama, I., et al. (2010). ER signaling is activated to protect human HaCaT keratinocytes from ER stress induced by environmental doses of UVB. Biochem. Biophys. Res. Commun. 397, 350–354. doi:10.1016/j.bbrc.2010.05.128
Melchinger, H., Jain, K., Tyagi, T., and Hwa, J. (2019). Role of platelet mitochondria: life in a nucleus-free zone. Front. Cardiovasc Med. 6, 153. doi:10.3389/fcvm.2019.00153
Mellem, D., Sattler, M., Pagel-Wolff, S., Jaspers, S., Wenck, H., Rübhausen, M., et al. (2017). Fragmentation of the mitochondrial network in skin in vivo. PLoS One 12 (6), e0174469. doi:10.1371/journal.pone.0174469
Mesmin, B. (2016). Mitochondrial lipid transport and biosynthesis: A complex balance. J. Cell Biol. 214 (1), 9–11. doi:10.1083/jcb.201606069
Miller, K., Victorelli, S., Salmonowicz, H., Dasgupta, N., Liu, T., Passos, J., et al. (2021). Cytoplasmic DNA: sources, sensing, and role in aging and disease. Cell 184 (22), 5506–5526. doi:10.1016/j.cell.2021.09.034
Mills, E., and O'Neill, L. (2016). Reprogramming mitochondrial metabolism in macrophages as an anti-inflammatory signal. Eur. J. Immunol. 46 (1), 13–21. doi:10.1002/eji.201445427
Mishra, P., Carelli, V., Manfredi, G., and Chan, D. (2014). Proteolytic cleavage of Opa1 stimulates mitochondrial inner membrane fusion and couples fusion to oxidative phosphorylation. Cell Metab. 19, 630–641. doi:10.1016/j.cmet.2014.03.011
Mizuguchi, S., Gotoh, K., Nakashima, Y., Setoyama, D., Takata, Y., Ohga, S., et al. (2021). Mitochondrial reactive oxygen species are essential for the development of Psoriatic inflammation. Front. Immunol. 12, 714897. doi:10.3389/fimmu.2021.714897
Mizutani, Y., Kanbe, A., Ito, H., and Seishima, M. (2020). Activation of STING signaling accelerates skin wound healing. J. Dermatol Sci. 97 (1), 21–29. doi:10.1016/j.jdermsci.2019.11.008
Montero, A., and Jassem, J. (2011). Cellular redox pathways as a therapeutic target in the treatment of cancer. Drugs 71, 1385–1396. doi:10.2165/11592590-000000000-00000
Monzel, A., Enríquez, J., and Picard, M. (2023). Multifaceted mitochondria: moving mitochondrial science beyond function and dysfunction. Nat. Metab. 5, 546–562. doi:10.1038/s42255-023-00783-1
Moriyama, M., Moriyama, H., Uda, J., Kubo, H., Nakajima, Y., Goto, A., et al. (2017). BNIP3 upregulation via stimulation of ERK and JNK activity is required for the protection of keratinocytes from UVB-induced apoptosis. Cell Death Dis. 8, e2576. doi:10.1038/cddis.2017.4
Moriyama, M., Moriyama, H., Uda, J., Matsuyama, A., Osawa, M., and Hayakawa, T. (2014). BNIP3 plays crucial roles in the differentiation and maintenance of epidermal keratinocytes. J. Invest Dermatol 134 (6), 1627–1635. doi:10.1038/jid.2014.11
Muliyil, S., Krishnakumar, P., and Narasimha, M. (2011). Spatial, temporal and molecular hierarchies in the link between death, delamination and dorsal closure. Development 138, 3043–3054. doi:10.1242/dev.060731
Muliyil, S., and Narasimha, M. (2014). Mitochondrial ROS regulates cytoskeletal and mitochondrial remodeling to tune cell and tissue dynamics in a model for wound healing. Dev. Cell 28 (3), 239–252. doi:10.1016/j.devcel.2013.12.019
Nagata, M., Takenaka, H., Shibagaki, R., and Kishimoto, S. (1999). Apoptosis and p53 protein expression increase in the process of burn wound healing in Guinea-pig skin. Brit J. Dermatol 140 (5), 829–838. doi:10.1046/j.1365-2133.1999.02811.x
Nakahira, K., AL, E., Rathinam, V. A. K., Lee, S. J., Dolinay, T., Lam, H. C., et al. (2011). Autophagy proteins regulate innate immune responses by inhibiting the release of mitochondrial DNA mediated by the NALP3 inflammasome. Nat. Immunol. 12, 222–230. doi:10.1038/ni.1980
Nargund, A., Pellegrino, M., Fiorese, C., Baker, B., and Haynes, C. (2012). Mitochondrial import efficiency of ATFS-1 regulates mitochondrial UPR activation. Science 337, 587–590. doi:10.1126/science.1223560
Niethammer, P., Grabher, C., Look, A., and Mitchison, T. (2009a). A tissue-scale gradient of hydrogen peroxide mediates rapid wound detection in zebrafish. Nature 459 (7249), 996–999. doi:10.1038/nature08119
Niethammer, P., Grabher, C., Look, A., and Mitchison, T. (2009b). A tissue-scale gradient of hydrogen peroxide mediates rapid wound detection in zebrafish. Nature 18 (7249), 996–999. doi:10.1038/nature08119
Nishio, N., Okawa, Y., Sakurai, H., and Isobe, K. (2008). Neutrophil depletion delays wound repair in aged mice. Age 30, 11–19. doi:10.1007/s11357-007-9043-y
Oaks, Z., and Perl, A. (2014). Metabolic control of the epigenome in systemic Lupus erythematosus. Autoimmunity 47 (4), 256–264. doi:10.3109/08916934.2013.834495
Ocampo, A., Reddy, P., Martinez-Redondo, P., Platero-Luengo, A., Hatanaka, F., Hishida, T., et al. (2016). In vivo amelioration of age-associated hallmarks by partial reprogramming. Cell 167 (7), 1719–1733.e12. doi:10.1016/j.cell.2016.11.052
Ogata, M., Hino, S., Saito, A., Morikawa, K., Kondo, S., Kanemoto, S., et al. (2006). Autophagy is activated for cell survival after endoplasmic reticulum stress. Mol. Cell Biol. 26, 9220–9231. doi:10.1128/MCB.01453-06
Okur, M., Fang, E., Fivenson, E., Tiwari, V., Croteau, D., and Bohr, V. (2020). Cockayne syndrome proteins CSA and CSB maintain mitochondrial homeostasis through NAD + signaling. Aging Cell 19 (12), e13268. doi:10.1111/acel.13268
Olson, H., and Nechiporuk, A. (2018). Using zebrafish to study collective cell migration in development and disease. Front. Cell Dev. Biol. 6, 83. doi:10.3389/fcell.2018.00083
Olsson, M., Järbrink, K., Divakar, U., Bajpai, R., Upton, Z., Schmidtchen, A., et al. (2019). The humanistic and economic burden of chronic wounds: A systematic review. Wound Rep. Reg. 27, 114–125. doi:10.1111/wrr.12683
Onida, S., Tan, M., Kafeza, M., Bergner, R., Shalhoub, J., Holmes, E., et al. (2019). Metabolic phenotyping in venous disease: the need for standardization. J. Proteome Res. 18 (11), 3809–3820. doi:10.1021/acs.jproteome.9b00460
Otera, H., Ishihara, N., and Mihara, K. (2013). New insights into the function and regulation of mitochondrial fission. Biochim. Biophys. Acta 1833, 1256–1268. doi:10.1016/j.bbamcr.2013.02.002
O’Toole, E., Van Koningsveld, R., Chen, M., and Woodley, D. (2008). Hypoxia induces epidermal keratinocyte matrix metalloproteinase-9 secretion via the protein kinase C pathway. J. Cell. Physiol. 214, 47–55. doi:10.1002/jcp.21160
Oyewole, A., Wilmot, M., Fowler, M., and Birch-Machin, M. (2014). Comparing the effects of mitochondrial targeted and localized antioxidants with cellular antioxidants in human skin cells exposed to UVA and hydrogen peroxide. FASEB J. 28, 485–494. doi:10.1096/fj.13-237008
Papandreou, I., Cairns, R., Fontana, L., Lim, A., and Denko, N. (2006). HIF-1 mediates adaptation to hypoxia by actively downregulating mitochondrial oxygen consumption. Cell Metab. 3, 187–197. doi:10.1016/j.cmet.2006.01.012
Parker, D., Iyer, A., Shah, S., Moran, A., Hjelmeland, A. B., Basu, M. K., et al. (2015). A new mitochondrial pool of cyclin E, regulated by Drp1, is linked to cell-density-dependent cell proliferation. J. Cell Sci. 128, 4171–4182. doi:10.1242/jcs.172429
Parnis, J., Montana, V., Delgado-Martinez, I., Matyash, V., Parpura, V., Kettenmann, H., et al. (2013). Mitochondrial exchanger NCLX plays a major role in the intracellular Ca2+ signaling, gliotransmission, and proliferation of astrocytes. J. Neurosci. 33 (17), 7206–7219. doi:10.1523/JNEUROSCI.5721-12.2013
Pase, L., Layton, J., Wittmann, C., Ellett, F., Nowell, C., Reyes-Aldasoro, C. C., et al. (2012). Neutrophil-delivered myeloperoxidase dampens the hydrogen peroxide burst after tissue wounding in zebrafish. Curr. Biol. 22, 1818–1824. doi:10.1016/j.cub.2012.07.060
Pastar, I., Stojadinovic, O., Yin, N., Ramirez, H., Nusbaum, A., Sawaya, A., et al. (2014). Epithelialization in wound healing: A comprehensive review. Adv. Wound Care 3, 445–464. doi:10.1089/wound.2013.0473
Pathak, T., and Trebak, M. (2018). Mitochondrial Ca 2+ signaling. Pharmacol. Ther. 192, 112–123. doi:10.1016/j.pharmthera.2018.07.001
Perillo, B., Di Donato, M., Pezone, A., Di Zazzo, E., Giovannelli, P., Galasso, G., et al. (2020). ROS in cancer therapy: the bright side of the moon. Exp. Mol. Med. 52, 192–203. doi:10.1038/s12276-020-0384-2
Perl, A., Gergely, P. J., and Banki, K. (2004). Mitochondrial dysfunction in T cells of patients with systemic lupus erythematosus. Int. Rev. Immunol. 23 (3-4), 293–313. doi:10.1080/08830180490452576
Perl, A., Hanczko, R., and Doherty, E. (2012). Assessment of mitochondrial dysfunction in lymphocytes of patients with systemic lupus erythematosus. Methods Mol. Biol. 900, 61–89. doi:10.1007/978-1-60761-720-4_4
Perumalsamy, L., Nagala, M., and Sarin, A. (2010). Notch-activated signaling cascade interacts with mitochondrial remodeling proteins to regulate cell survival. Proc. Natl. Acad. Sci. U. S. A. 107, 6882–6887. doi:10.1073/pnas.0910060107
Pfanner, N., Warscheid, B., and Wiedemann, N. (2019). Mitochondrial proteins: from biogenesis to functional networks. Nat. Rev. Mol. Cell Biol. 20, 267–284. doi:10.1038/s41580-018-0092-0
Picard, M., and Shirihai, (2022). Mitochondrial signal transduction. Cell Met. 34, 1620–1653. doi:10.1016/j.cmet.2022.10.008
Polaka, S., Katare, P., Pawar, B., Vasdev, N., Gupta, T., Rajpoot, K., et al. (2022). Emerging ROS-modulating technologies for augmentation of the wound healing process. ACS Omega 7 (35), 30657–30672. doi:10.1021/acsomega.2c02675
Ponte, S., Carvalho, L., Gagliardi, M., Campos, I., Oliveira, P., and Jacinto, A. (2020). Drp1-mediated mitochondrial fission regulates calcium and F-actin dynamics during wound healing. Biol. Open 9 (5), bio048629. doi:10.1242/bio.048629
Quinlan, C., Perevoshchikova, I., Hey-Mogensen, M., Orr, A., and Brand, M. (2013). Sites of reactive oxygen species generation by mitochondria oxidizing different substrates. Redox Biol. 1 (1), 304–312. doi:10.1016/j.redox.2013.04.005
Rauthan, M., Ranji, P., Aguilera Pradenas, N., Pitot, C., and Pilon, M. (2013). The mitochondrial unfolded protein response activator ATFS-1 protects cells from inhibition of the mevalonate pathway. Proc. Natl. Acad. Sci. U. S. A. 110, 5981–5986. doi:10.1073/pnas.1218778110
Razzell, W., Evans, I., Martin, P., and Wood, W. (2013). Calcium flashes orchestrate the wound inflammatory response through DUOX activation and hydrogen peroxide release. Curr. Biol. 23 (5), 424–429. doi:10.1016/j.cub.2013.01.058
Reinke, J. M., and Sorg, H. (2012). Wound repair and regeneration. Eur. Surg. Res. 49 (1), 35–43. doi:10.1159/000339613
Renaudin, X. (2021). Reactive oxygen species and DNA damage response in cancer. Int. Rev. Cell Mol. Biol. 364, 139–161. doi:10.1016/bs.ircmb.2021.04.001
Ressler, S., Bartkova, J., Niederegger, H., Bartek, J., Scharffetter-Kochanek, K., Jansen-Dürr, P., et al. (2006). p16INK4A is a robust in vivo biomarker of cellular aging in human skin. Aging Cell 5, 379–389. doi:10.1111/j.1474-9726.2006.00231.x
Rice, G., Bond, J., Asipu, A., Brunette, R., Crow, Y., Carr, I. M., et al. (2009). Mutations involved in Aicardi-Goutières syndrome implicate SAMHD1 as regulator of the innate immune response. Nat. Genet. 41 (7), 829–832. doi:10.1038/ng.373
Rieusset, J. (2018). The role of endoplasmic reticulum-mitochondria contact sites in the control of glucose homeostasis: an update. Cell Death Dis. 9 (3), 388. doi:10.1038/s41419-018-0416-1
Riley, J., AL, E., Cloix, C., Lopez, J., O'Prey, J., Pearson, M., et al. (2018). Mitochondrial inner membrane permeabilisation enables mtDNA release during apoptosis. EMBO J. 37, e99238. doi:10.15252/embj.201899238
Rizk, J., AL, E., Agerholm, R., Kadekar, D., Ivars, F., Agace, W. W., et al. (2019). SMAC mimetics promote NIK-dependent inhibition of CD4 + T H 17 cell differentiation. Sci. Signal 12, eaaw3469. doi:10.1126/scisignal.aaw3469
Rodriguez, P., Felix, F., Woodley, D., and Shim, E. (2008). The role of oxygen in wound healing: A review of the literature. Dermatol Surg. 34, 1159–1169. doi:10.1111/j.1524-4725.2008.34254.x
Rohrig, F., and Schulze, A. (2016). The multifaceted roles of fatty acid synthesis in cancer. Nat. Rev. Cancer 16, 732–749. doi:10.1038/nrc.2016.89
Ron-Harel, N., Santos, D., Ghergurovich, J. M., Sage, P. T., Reddy, A., Lovitch, S. B., et al. (2016). Mitochondrial biogenesis and proteome remodeling promote one-carbon metabolism for T cell activation. Cell Metab. 24, 104–117. doi:10.1016/j.cmet.2016.06.007
Rosenberger, F., Thielert, M., and Mann, M. (2023). Making single-cell proteomics biologically relevant. Nat. Methods 20 (3), 320–323. doi:10.1038/s41592-023-01771-9
Ross, R., and Odland, G. (1968). Human wound repair. II. Inflammatory cells, epithelial-mesenchymal interrelations, and fibrogenesis. J. Cell Biol. 39, 152–168. doi:10.1083/jcb.39.1.152
Rousselle, P., Braye, F., and Dayan, G. (2019). Re-Epithelialization of adult skin wounds: cellular mechanisms and therapeutic strategies. Adv. Drug Deliv. Rev. 146, 344–365. doi:10.1016/j.addr.2018.06.019
Roy, M., Reddy, P., Iijima, M., and Sesaki, H. (2015). Mitochondrial division and fusion in metabolism. Curr. Opin. Cell Biol. 33, 111–118. doi:10.1016/j.ceb.2015.02.001
Ryu, S.-Y., Peixoto, P., Won, J., Yule, D., and Kinnally, K. (2010). Extracellular ATP and P2Y2 receptors mediate intercellular Ca2+ waves induced by mechanical stimulation in submandibular gland cells: role of mitochondrial regulation of store operated Ca2+ entry. Cell Calcium 47 (1), 65–76. doi:10.1016/j.ceca.2009.11.006
Sabour Alaoui, S., Dessirier, V., De Araujo, E., Tsapis, A., Pelekanou, V., Lkhider, M., et al. (2012). TWEAK affects keratinocyte G2/M growth arrest and induces apoptosis through the translocation of the AIF protein to the nucleus. PLoS One 7 (3), e33609. doi:10.1371/journal.pone.0033609
Sainero-Alcolado, L., Liaño-Pons, J., Ruiz-Pérez, M., and Arsenian-Henriksson, M. (2022). Targeting mitochondrial metabolism for precision medicine in cancer. Cell Death Differ. 29, 1304–1317. doi:10.1038/s41418-022-01022-y
Salzer, M., Lafzi, A., Berenguer-Llergo, A., Youssif, C., Castellanos, A., Solanas, G., et al. (2018). Identity noise and adipogenic traits characterize dermal fibroblast aging. Cell 175 (6), 1575–1590.e22. doi:10.1016/j.cell.2018.10.012
Scaffidi, C., Fulda, S., Srinivasan, A., Friesen, C., Li, F., Tomaselli, K., et al. (1998). Two CD95 (APO-1/Fas) signaling pathways. Embo J. 17 (6), 1675–1687. doi:10.1093/emboj/17.6.1675
Scheibye-Knudsen, M., Croteau, D., and Bohr, V. (2013). Mitochondrial deficiency in Cockayne syndrome. Mech. Ageing Dev. 134 (5-6), 275–283. doi:10.1016/j.mad.2013.02.007
Scheibye-Knudsen, M., Ramamoorthy, M., Sykora, P., Maynard, S., Lin, P., Minor, R., et al. (2012). Cockayne syndrome group B protein prevents the accumulation of damaged mitochondria by promoting mitochondrial autophagy. J. Exp. Med. 209 (4), 855–869. doi:10.1084/jem.20111721
Schell, J., Olson, K. A., Jiang, L., Hawkins, A. J., Van Vranken, J. G., Xie, J., et al. (2014). A role for the mitochondrial pyruvate carrier as a repressor of the Warburg effect and colon cancer cell growth. Mol. Cell 56, 400–413. doi:10.1016/j.molcel.2014.09.026
Schiffmann, L., Werthenbach, J., Heintges-Kleinhofer, F., Seeger, J. M., Fritsch, M., Günther, S. D., et al. (2020). Mitochondrial respiration controls neoangiogenesis during wound healing and tumour growth. Nat. Commun. 11 (1), 3653. doi:10.1038/s41467-020-17472-2
Schoors, S., Bruning, U., Missiaen, R., Queiroz, K. C., Borgers, G., Elia, I., et al. (2015). Fatty acid carbon is essential for dNTP synthesis in endothelial cells. Nature 520, 192–197. doi:10.1038/nature14362
Schreml, S., Szeimies, R., Prantl, L., Karrer, S., Landthaler, M., and Babilas, P. (2010). Oxygen in acute and chronic wound healing. Br. J. Derm. 163, 257–268. doi:10.1111/j.1365-2133.2010.09804.x
Schröder, R., Kunz, W., Rouan, F., Pfendner, E., Rolfs, A., Kappes-Horn, K., et al. (2002). Disorganization of the desmin cytoskeleton and mitochondrial dysfunction in plectin-related epidermolysis bullosa simplex with muscular dystrophy. J. Neuropathol. Exp. Neurol. 61 (6), 520–530. doi:10.1093/jnen/61.6.520
Schroeder, P., Gremmel, T., Berneburg, M., and Krutmann, J. (2008). Partial depletion of mitochondrial DNA from human skin fibroblasts induces a gene expression profile reminiscent of photoaged skin. J. Invest. Dermatol. 128, 2297–2303. doi:10.1038/jid.2008.57
Schultz, G., and Wysocki, A. (2009). Interactions between extracellular matrix and growth factors in wound healing. Wound Repair Regen. 17 (2), 153–162. doi:10.1111/j.1524-475X.2009.00466.x
Seaton, M., Hocking, A., and Gibran, N. (2015). Porcine models of cutaneous wound healing. ILAR J. 56, 127–138. doi:10.1093/ilar/ilv016
Segal, B., Grimm, M., Khan, A., Han, W., and Blackwell, T. (2012). Regulation of innate immunity by NADPH oxidase. Free Radic. Biol. Med. 53, 72–80. doi:10.1016/j.freeradbiomed.2012.04.022
Sen, C., and Roy, S. (2010). Oxygenation state as a driver of myofibroblast differentiation and wound contraction: hypoxia impairs wound closure. J. Invest Dermatol 130, 2701–2703. doi:10.1038/jid.2010.316
Seo, B., Yoon, S., and Do, J. (2018). Mitochondrial dynamics in stem cells and differentiation. Int. J. Mol. Sci. 19, 3893. doi:10.3390/ijms19123893
Shadel, G., and Horvath, T. (2015). Mitochondrial ROS signaling in organismal homeostasis. Cell 163 (3), 560–569. doi:10.1016/j.cell.2015.10.001
Sharifi, M., Mowers, E. E., Drake, L. E., Collier, C., Chen, H., Zamora, M., et al. (2016). Autophagy promotes focal adhesion disassembly and cell motility of metastatic tumor cells through the direct interaction of paxillin with LC3. Cell Rep. 15, 1660–1672. doi:10.1016/j.celrep.2016.04.065
Shen, K., Pender, C., Bar-Ziv, R., Zhang, H., Wickham, K., Willey, E., et al. (2022). Mitochondria as cellular and organismal signaling hubs. Annu. Rev. Cell Dev. Biol. 38, 179–218. doi:10.1146/annurev-cellbio-120420-015303
Shi, X., Zhao, M., Fu, C., and Fu, A. (2017). Intravenous administration of mitochondria for treating experimental Parkinson's disease. Mitochondrion 34, 91–100. doi:10.1016/j.mito.2017.02.005
Shimada, K., AL, E., Karlin, J., Dagvadorj, J., Chiba, N., Chen, S., et al. (2012). Oxidized mitochondrial DNA activates the NLRP3 inflammasome during apoptosis. Immunity 36, 401–414. doi:10.1016/j.immuni.2012.01.009
Shimomura, Y., Murakami, T., Nakai, N., Nagasaki, M., and Harris, R. (2023). Exercise promotes BCAA catabolism: effects of BCAA supplementation on skeletal muscle during exercise. J. Nutr. 134, 1583S-1587S–1587S. doi:10.1093/jn/134.6.1583S
Shook, B., Wasko, R. R., Rivera-Gonzalez, G. C., Salazar-Gatzimas, E., López-Giráldez, F., Dash, B. C., et al. (2018). Myofibroblast proliferation and heterogeneity are supported by macrophages during skin repair. Science 362, eaar2971. doi:10.1126/science.aar2971
Shook, B., Xiao, E., Kumamoto, Y., Iwasaki, A., and Horsley, V. (2016). CD301b+ macrophages are essential for effective skin wound healing. J. Investig. Dermatol. 136, 1885–1891. doi:10.1016/j.jid.2016.05.107
Shpilka, T., and Haynes, C. (2018). The mitochondrial UPR: mechanisms, physiological functions and implications in ageing. Nat. Rev. Mol. Cell Biol. 19, 109–120. doi:10.1038/nrm.2017.110
Sicheritz-Pontén T, K. C., and Andersson, S. G. (1998). A phylogenetic analysis of the cytochrome b and cytochrome c oxidase I genes supports an origin of mitochondria from within the Rickettsiaceae. Biochim. Biophys. Acta 1365 (3), 545–551. doi:10.1016/s0005-2728(98)00099-1
Sil, P., Wong, S., and Martinez, J. (2018). More than skin deep: autophagy is vital for skin barrier function. Front. Immunol. 9, 1376. doi:10.3389/fimmu.2018.01376
Simpson, C., Tokito, M., Uppala, R., Sarkar, M., Gudjonsson, J., and Holzbaur, E. (2021). NIX initiates mitochondrial fragmentation via DRP1 to drive epidermal differentiation. Cell Rep. 34, 108689. doi:10.1016/j.celrep.2021.108689
Sindrilaru, A., Peters, T., Schymeinsky, J., Oreshkova, T., Wang, H., Gompf, A., et al. (2009). Wound healing defect of Vav3-/- mice due to impaired {beta}2-integrin-dependent macrophage phagocytosis of apoptotic neutrophils. Blood 113 (21), 5266–5276. doi:10.1182/blood-2008-07-166702
Sinno, H., and Prakash, S. (2013). Complements and the wound healing cascade: an updated review. Plast. Surg. Int. 2013, 146764. doi:10.1155/2013/146764
Soares, M., Cohen, O., Low, Y., Sartor, R., Ellison, T., Anil, U., et al. (2016). Restoration of Nrf2 signaling normalizes the regenerative niche. Diabetes 65, 633–646. doi:10.2337/db15-0453
Song, X., Narzt, M., Nagelreiter, I., Hohensinner, P., Terlecki-Zaniewicz, L., Tschachler, E., et al. (2017). Autophagy deficient keratinocytes display increased DNA damage, senescence and aberrant lipid composition after oxidative stress in vitro and in vivo. Redox Biol. 11, 219–230. doi:10.1016/j.redox.2016.12.015
Sowter, H., Ratcliffe, P., Watson, P., Greenberg, A., and Harris, A. (2001). HIF-1-dependent regulation of hypoxic induction of the cell death factors BNIP3 and NIX in human tumors. Cancer Res. 61, 6669–6673.
Spinelli, J., and Haigis, M. (2018). The multifaceted contributions of mitochondria to cellular metabolism. Nat. Cell Biol. 20, 745–754. doi:10.1038/s41556-018-0124-1
Sreedhar, A., Aguilera-Aguirre, L., and Singh, K. (2020). Mitochondria in skin health, aging, and disease. Cell Death Dis. 9 (6), 444. doi:10.1038/s41419-020-2649-z
Stetson, D., and Medzhitov, R. (2006). Recognition of cytosolic DNA activates an IRF3-dependent innate immune response. Immunity 24, 93–103. doi:10.1016/j.immuni.2005.12.003
Stewart, J., and Chinnery, P. (2021). Extreme heterogeneity of human mitochondrial DNA from organelles to populations. Nat. Rev. Genet. 22, 106–118. doi:10.1038/s41576-020-00284-x
Stout, R., and Birch-Machin, M. (2019). Mitochondria's role in skin ageing. Biol. (Basel) 8 (2), 29. doi:10.3390/biology8020029
Subramaniam, T., Fauzi, M., Lokanathan, Y., and Jx, L. (2021). The role of calcium in wound healing. Int. J. Mol. Sci. 22 (12), 6486. doi:10.3390/ijms22126486
Sumitra, M., Manikandan, P., Gayathri, V., and Suguna, L. (2009). Influence of honey on energy metabolism during wound healing in rats. Sch. Res. Exch. 2009, 1–6. doi:10.3814/2009/715320
Sun, T., Jiao, L., Wang, Y., Yu, Y., and Ming, L. (2018). SIRT1 induces epithelialmesenchymal transition by promoting autophagic degradation of E-cadherin in melanoma cells. Cell death Dis. 9, 136. doi:10.1038/s41419-017-0167-4
Swift, M., Burns, A., Gray, K., and Dipietro, L. (2001). Age-related alterations in the inflammatory response to dermal injury. J. Invest. Dermatol. 117, 1027–1035. doi:10.1046/j.0022-202x.2001.01539.x
Tao, Y., Wang, M., Chen, E., and Tang, H. (2017). Liver regeneration: analysis of the main relevant signaling molecules. Mediat. Inflamm. 2017, 4256352. doi:10.1155/2017/4256352
Taylor, R., Cullen, S., and Martin, S. (2008). Apoptosis: controlled demolition at the cellular level. Nat. Rev. Mol. Cell Bio 9 (3), 231–241. doi:10.1038/nrm2312
Thankam, F., Chandra, I., Kovilam, A., Diaz, C. G., Volberding, B. T., Dilisio, M. F., et al. (2018). Amplification of mitochondrial activity in the healing response following rotator cuff tendon injury. Sci. Rep. 8, 17027. doi:10.1038/s41598-018-35391-7
Theocharidis, G., Thomas, B. E., Sarkar, D., Mumme, H. L., Pilcher, W. J. R., Dwivedi, B., et al. (2022). Single cell transcriptomic landscape of diabetic foot ulcers. Nat. Commun. 13, 181. doi:10.1038/s41467-021-27801-8
Tietzova, I., Twaroski, K., Eide, C., Ostrander, J., Crawford, P., and Tolar, J. (2020). Impaired mitochondrial and metabolic function of fibroblasts derived from patients with recessive dystrophic and junctional epidermolysis bullosa. EMJ Dermatol. 8 (1), 75–83. doi:10.33590/emjdermatol/20-00007
Toda, C., Kim, J. D., Impellizzeri, D., Cuzzocrea, S., Liu, Z. W., and Diano, S. (2016). UCP2 regulates mitochondrial fission and ventromedial nucleus control of glucose responsiveness. Cell 164, 872–883. doi:10.1016/j.cell.2016.02.010
Tonnesen, M., Feng, X., and Clark, R. (2000). Angiogenesis in wound healing. J. Investig. Dermatol Symp. Proc. 5, 40–46. doi:10.1046/j.1087-0024.2000.00014.x
Trachootham, D., Lu, W., Ogasawara, M., Rivera-Del Valle, N., and Huang, P. (2008). Redox regulation of cell survival. Antioxid. Redox Signal. 10 (8), 1343–1374. doi:10.1089/ars.2007.1957
Trotta, A., and Chipuk, J. (2017). Mitochondrial dynamics as regulators of cancer biology. Cell Mol. Life Sci. 74, 1999–2017. doi:10.1007/s00018-016-2451-3
Tuloup-Minguez, V., Hamaï, A., Greffard, A., Nicolas, V., Codogno, P., and Botti, J. (2013). Autophagy modulates cell migration and β1 integrin membrane recycling. Cell Cycle 12, 3317–3328. doi:10.4161/cc.26298
Ulger, O., and Kubat, G. (2022). Therapeutic applications of mitochondrial transplantation. Biochimie 195, 1–15. doi:10.1016/j.biochi.2022.01.002
Van Der Vliet, A., and Janssen-Heininger, Y. (2014). Hydrogen peroxide as a damage signal in tissue injury and inflammation: murderer, mediator, or messenger? J. Cell Biochem. 115 (3), 427–435. doi:10.1002/jcb.24683
Vandekeere, S., Dubois, C., Kalucka, J., Sullivan, M. R., García-Caballero, M., Goveia, J., et al. (2018). Serine synthesis via PHGDH is essential for heme production in endothelial cells. Cell Metab. 28, 573–587. doi:10.1016/j.cmet.2018.06.009
Vanpouille-Box, C., Hoffmann, J., and Galluzzi, L. (2019). Pharmacological modulation of nucleic acid sensors - therapeutic potential and persisting obstacles. Nat. Rev. Drug Discov. 18, 845–867. doi:10.1038/s41573-019-0043-2
Vasan, K., Werner, M., and Chandel, N. (2020). Mitochondrial metabolism as a target for cancer therapy. Cell Metab. 32 (3), 341–352. doi:10.1016/j.cmet.2020.06.019
Ved, R., Saha, S., Westlund, B., Perier, C., Burnam, L., Sluder, A., et al. (2005). Similar patterns of mitochondrial vulnerability and rescue induced by genetic modification of alpha-synuclein, parkin, and DJ-1 in Caenorhabditis elegans. J. Biol. Chem. 280, 42655–42668. doi:10.1074/jbc.M505910200
Velnar, T., Bailey, T. V. S., and Smrkolj, V. (2009). The wound healing process: an overview of the cellular and molecular mechanisms. J. Int. Med. Res. 37 (5), 1528–1542. doi:10.1177/147323000903700531
Vetter, A., Jahn, K., Bouameur, J., Kiritsi, D., and Magin, T. (2020). Epidermolysis bullosa simplex keratinocytes show disturbed mitochondrial Positioning and activity. J. Invest Dermatol 140 (7), 1438–1442. doi:10.1016/j.jid.2019.10.023
Villarreal-Martinez, A., Martinez-DE-Villarreal, L., Gomez-Flores, M., Calvo-Anguiano, G., Cerda-Flores, R., Ocampo-Candiani, J., et al. (2023). Mitochondrial dysfunction: the pathological link between psoriasis and insulin resistance? J. Eur. Acad. Dermatol Venereol. 37 (2), 340–347. doi:10.1111/jdv.18631
Vinaik, R. B. D., Auger, C., Abdullahi, A., and And Jeschke, M. G. (2020). Regulation of glycolysis and the Warburg effect in wound healing. JCI Insight 5 (17), e138949. doi:10.1172/jci.insight.138949
VON Zglinicki, T., Wan, T., and Miwa, S. (2021). Senescence in postmitotic cells: A driver of aging? Antioxid. Redox Signal 34, 308–323. doi:10.1089/ars.2020.8048
Vu, R., Jin, S., Sun, P., Haensel, D., Nguyen, Q., Dragan, M., et al. (2022). Wound healing in aged skin exhibits systems-level alterations in cellular composition and cell-cell communication. Cell Rep. 40 (5), 111155. doi:10.1016/j.celrep.2022.111155
Wall, I., Moseley, R., Baird, D., Kipling, D., Giles, P., Laffafian, I., et al. (2008). Fibroblast dysfunction is a key factor in the non-healing of chronic venous leg ulcers. J. Invest. Dermatol. 128, 2526–2540. doi:10.1038/jid.2008.114
Walsh, C., Tu, B., and Tang, Y. (2017). Eight kinetically stable but thermodynamically activated molecules that power cell metabolism. Chem. Rev. 118, 1460–1494. doi:10.1021/acs.chemrev.7b00510
Wang, R., Dillon, C. P., Shi, L. Z., Milasta, S., Carter, R., Finkelstein, D., et al. (2011a). The transcription factor Myc controls metabolic reprogramming upon T lymphocyte activation. Immunity 35, 871–882. doi:10.1016/j.immuni.2011.09.021
Wang, W., Fang, H., Groom, L., Cheng, A., Zhang, W., Liu, J., et al. (2008). Superoxide flashes in single mitochondria. Cell 134 (2), 279–290. doi:10.1016/j.cell.2008.06.017
Wang, X., and Chen, X. (2015). A cytosolic network suppressing mitochondria-mediated proteostatic stress and cell death. Nature 524, 481–484. doi:10.1038/nature14859
Wang, Y., Subramanian, M., Yurdagul, A., Barbosa-Lorenzi, V. C., Cai, B., de Juan-Sanz, J., et al. (2017). Mitochondrial fission promotes the continued clearance of apoptotic cells by macrophages. Cell 171, 331–345.e22. doi:10.1016/j.cell.2017.08.041
Wang, Y., Zang, Q., Liu, Z., Wu, Q., Maass, D., Dulan, G., et al. (2011b). Regulation of VEGF-induced endothelial cell migration by mitochondrial reactive oxygen species. Am. J. Physiol. Cell Physiol. 301, C695–C704. doi:10.1152/ajpcell.00322.2010
Wassermann, R., Polo, M., Smith, P., Wang, X., Ko, F., and Robson, M. (1998). Differential production of apoptosis-modulating proteins in patients with hypertrophic burn scar. J. Surg. Res. 75 (1), 74–80. doi:10.1006/jsre.1998.5267
Watt, I., Montgomery, M., Runswick, M., Leslie, A., and Walker, J. (2010). Bioenergetic cost of making an adenosine triphosphate molecule in animal mitochondria. Proc. Natl. Acad. Sci. U. S. A. 107, 16823–16827. doi:10.1073/pnas.1011099107
Weidong, P., Xin, W., Xiaowei, W., Fei, L., Chunyan, H., and Qiming, Z. (2022). Mitochondrial superoxide is the key for promoting cell migration in wound healing. Thromb. Haemost. Res. 6. doi:10.26420/thrombhaemostres.2022.1072
Weinstein, A., Lalezarzadeh, F., Soares, M., Saadeh, P., and Ceradini, D. (2015). Normalizing dysfunctional purine metabolism accelerates diabetic wound healing. Wound Repair Regen. 23, 14–21. doi:10.1111/wrr.12249
West, A., Brodsky, I., Rahner, C., Woo, D., Erdjument-Bromage, H., Tempst, P., et al. (2011). TLR signalling augments macrophage bactericidal activity through mitochondrial ROS. Nature 472 (7344), 476–480. doi:10.1038/nature09973
West, A., Khoury-Hanold, W., Staron, M., Tal, M., Pineda, C., Lang, S., et al. (2015). Mitochondrial DNA stress primes the antiviral innate immune response. Nature 520 (7548), 553–557. doi:10.1038/nature14156
Westermann, B. (2010). Mitochondrial fusion and fission in cell life and death. Nat. Rev. Mol. Cell Biol. 11, 872–884. doi:10.1038/nrm3013
Wijesinghe, D., Brentnall, M., Mietla, J., Hoeferlin, L., Diegelmann, R., Boise, L., et al. (2014). Ceramide kinase is required for a normal eicosanoid response and the subsequent orderly migration of fibroblasts. J. Lipid Res. 55 (7), 1298–1309. doi:10.1194/jlr.M048207
Wijesinghe, D., Warncke, U., and Diegelmann, R. (2016). Human as the ultimate wound healing model: strategies for studies investigating the dermal lipidome. Curr. Dermatol Rep. 5 (4), 244–251. doi:10.1007/s13671-016-0156-3
Wilkinson, H., and Hardman, M. (2020). Senescence in wound repair: emerging strategies to target chronic healing wounds. Front. Cell Dev. Biol. 8, 773. doi:10.3389/fcell.2020.00773
Wilkinson, H., Upson, S., Banyard, K., Knight, R., Mace, K., and Hardman, M. (2019). Reduced iron in diabetic wounds: an oxidative stress-dependent role for STEAP3 in extracellular matrix deposition and remodeling. J. Invest. Dermatol 139, 2368–2377.e7. doi:10.1016/j.jid.2019.05.014
Willemsen, J., AL, E., Hoyler, T., Noir, E., Tessier, C., Sarret, S., et al. (2021). TNF leads to mtDNA release and cGAS/STING-dependent interferon responses that support inflammatory arthritis. Cell Rep. 37, 109977. doi:10.1016/j.celrep.2021.109977
Willenborg, S., Sanin, D., Jais, A., Ding, X., Ulas, T., Nuchel, J., et al. (2020). Mitochondrial metabolism coordinates stage-specific repair processes in macrophages during wound healing. Cell Metab. 33, 2398–2414.e9. doi:10.1016/j.cmet.2021.10.004
Wise, D., DeBerardinis, R. J., Mancuso, A., Sayed, N., Zhang, X. Y., Pfeiffer, H. K., et al. (2008). Myc regulates a transcriptional program that stimulates mitochondrial glutaminolysis and leads to glutamine addiction. Proc. Natl. Acad. Sci. 105, 18782–18787. doi:10.1073/pnas.0810199105
Wrobel, L., AL, E., Bragoszewski, P., Wiese, S., Sztolsztener, M. E., Oeljeklaus, S., et al. (2015). Mistargeted mitochondrial proteins activate a proteostatic response in the cytosol. Nature 524, 485–488. doi:10.1038/nature14951
Wu, T., Teslaa, T., Kalim, S., French, C. T., Moghadam, S., Wall, R., et al. (2011). Photothermal nanoblade for large cargo delivery into mammalian cells. Anal. Chem. 83 (4), 1321–1327. doi:10.1021/ac102532w
Wu, W., Tian, W., Hu, Z., Chen, G., Huang, L., Li, W., et al. (2014). ULK1 translocates to mitochondria and phosphorylates FUNDC1 to regulate mitophagy. EMBO Rep. 15 (5), 566–575. doi:10.1002/embr.201438501
Xu, J., and Huang, X. (2020). Lipid metabolism at membrane contacts: dynamics and functions beyond lipid homeostasis. Front. Cell Dev. Biol. 8, 615856. doi:10.3389/fcell.2020.615856
Xu, S., and Chisholm, A. (2011). A Gαq-Ca2⁺ signaling pathway promotes actin-mediated epidermal wound closure in C. elegans. Curr. Biol. 21 (23), 1960–1967. doi:10.1016/j.cub.2011.10.050
Xu, S., and Chisholm, A. (2014). C. elegans epidermal wounding induces a mitochondrial ROS burst that promotes wound repair. Dev. Cell 31 (1), 48–60. doi:10.1016/j.devcel.2014.08.002
Xue, M., and Jackson, C. (2015). Extracellular matrix reorganization during wound healing and its impact on abnormal scarring. Adv. Wound Care (New Rochelle) 4 (3), 119–136. doi:10.1089/wound.2013.0485
Yamamoto, M., Sato, T., Beren, J., Verthelyi, D., and Dm, K. (2011). The acceleration of wound healing in primates by the local administration of immunostimulatory CpG oligonucleotides. Biomaterials 32, 4238–4242. doi:10.1016/j.biomaterials.2011.02.043
Yamazaki, T., AL, E., Sato, A., Buqué, A., Rybstein, M., Petroni, G., et al. (2020). Mitochondrial DNA drives abscopal responses to radiation that are inhibited by autophagy. Nat. Immunol. 21, 1160–1171. doi:10.1038/s41590-020-0751-0
Yang, J., Lee, H., and Wei, Y. (1995). Photoageing-associated mitochondrial DNA length mutations in human skin. Arch. Dermatol. Res. 287, 641–648. doi:10.1007/BF00371736
Yang, S., Zhang, H., Shi, S., Zhu, Y., Song, N., Dai, Q., et al. (2020). The role of mitochondria in systemic lupus erythematosus: A glimpse of various pathogenetic mechanisms. Curr. Med. Chem. 27 (20), 3346–3361. doi:10.2174/0929867326666181126165139
Yasukawa, K., Okuno, T., and Yokomizo, T. (2020). Eicosanoids in skin wound healing. Int. J. Mol. Sci. 21 (22), 8435. doi:10.3390/ijms21228435
Yoo, S., Starnes, T., Deng, Q., and Huttenlocher, A. (2011). Lyn is a redox sensor that mediates leukocyte wound attraction in vivo. Nature 480 (7375), 109–112. doi:10.1038/nature10632
Yoshihara, N., Ueno, T., Takagi, A., Oliva Trejo, J., Haruna, K., Suga, Y., et al. (2015). The significant role of autophagy in the granular layer in normal skin differentiation and hair growth. Arch. Dermatol. Res. 307, 159–169. doi:10.1007/s00403-014-1508-0
Yu, C., AL, E., Harapas, C. R., Hilton, J. B., Mlodzianoski, M. J., Laohamonthonkul, P., et al. (2020). TDP-43 triggers mitochondrial DNA release via mPTP to activate cGAS/STING in ALS. Cell 183, 636–649. doi:10.1016/j.cell.2020.09.020
Yu, T., Gao, M., Yang, P., Pei, Q., Liu, D., Wang, D., et al. (2017). Topical insulin accelerates cutaneous wound healing in insulin-resistant diabetic rats. Am. J. Transl. Res. 9 (10), 4682–4693.
Zamzami, N., Larochette, N., and Kroemer, G. (2005). Mitochondrial permeability transition in apoptosis and necrosis. Cell Death Differ. 12 (2), 1478–1480. doi:10.1038/sj.cdd.4401682
Zapatero-Solana, E., García-Giménez, J., Guerrero-Aspizua, S., García, M., Toll, A., Baselga, E., et al. (2014). Oxidative stress and mitochondrial dysfunction in Kindler syndrome. Orphanet J. Rare Dis. 9, 211. doi:10.1186/s13023-014-0211-8
Zhan, R., Yang, S., He, W., Wang, F., Tan, J., Zhou, J., et al. (2015). Nitric oxide enhances keratinocyte cell migration by regulating Rho GTPase via cGMP-PKG signalling. PLoS One 10 (3), e0121551. doi:10.1371/journal.pone.0121551
Zhang, J., and Ney, P. (2009). Role of BNIP3 and NIX in cell death, autophagy, and mitophagy. Cell Death Differ. 16 (7), 939–946. doi:10.1038/cdd.2009.16
Zhang, J., Zhang, C., Jiang, X., Li, L., Zhang, D., Tang, D., et al. (2019). Involvement of autophagy in hypoxia-BNIP3 signaling to promote epidermal keratinocyte migration. Cell Death Dis. 10, 234. doi:10.1038/s41419-019-1473-9
Zhang, J., Zhang, D., Jiang, X., Zhang, C., Zhao, L., et al. (2017). BNIP3 promotes the motility and migration of keratinocyte under hypoxia. Exp. Dermatol 26, 416–422. doi:10.1111/exd.13248
Zhao, P., Sui, B., Liu, N., Lv, Y., Zheng, C., Lu, Y., et al. (2017). Anti-aging pharmacology in cutaneous wound healing: effects of metformin, resveratrol, and rapamycin by local application. Aging cell 16 (5), 1083–1093. doi:10.1111/acel.12635
Zhao, Q., AL, E., Levichkin, I. V., Stasinopoulos, S., Ryan, M. T., and Hoogenraad, N. J. (2002). A mitochondrial specific stress response in mammalian cells. EMBO J. 21, 4411–4419. doi:10.1093/emboj/cdf445
Zhao, Y., Zhang, C. F., Rossiter H, E. A., Eckhart, L., König, U., Karner, S., et al. (2013). Autophagy is induced by UVA and promotes removal of oxidized phospholipids and protein aggregates in epidermal keratinocytes. J. Invest Dermatol 133, 1629–1637. doi:10.1038/jid.2013.26
Zhong, Z., Umemura, A., Sanchez-Lopez, E., Liang, S., Shalapour, S., Wong, J., et al. (2016). NF-κB restricts inflammasome activation via elimination of damaged mitochondria. Cell 164 (5), 896–910. doi:10.1016/j.cell.2015.12.057
Zomer, H., and Trentin, A. (2018). Skin wound healing in humans and mice: challenges in translational research. J. Dermatol Sci. 90 (1), 3–12. doi:10.1016/j.jdermsci.2017.12.009
Keywords: mitochondria, metabolism, wound healing, chronic wounds, hypoxia
Citation: Hunt M, Torres M, Bachar-Wikström E and Wikström JD (2023) Multifaceted roles of mitochondria in wound healing and chronic wound pathogenesis. Front. Cell Dev. Biol. 11:1252318. doi: 10.3389/fcell.2023.1252318
Received: 03 July 2023; Accepted: 28 August 2023;
Published: 11 September 2023.
Edited by:
Anne Chiaramello, George Washington University, United StatesReviewed by:
Chiwei Xu, The Rockefeller University, United StatesCopyright © 2023 Hunt, Torres, Bachar-Wikström and Wikström. This is an open-access article distributed under the terms of the Creative Commons Attribution License (CC BY). The use, distribution or reproduction in other forums is permitted, provided the original author(s) and the copyright owner(s) are credited and that the original publication in this journal is cited, in accordance with accepted academic practice. No use, distribution or reproduction is permitted which does not comply with these terms.
*Correspondence: Jakob D. Wikström, SmFrb2Iud2lrc3Ryb21Aa2kuc2U=
Disclaimer: All claims expressed in this article are solely those of the authors and do not necessarily represent those of their affiliated organizations, or those of the publisher, the editors and the reviewers. Any product that may be evaluated in this article or claim that may be made by its manufacturer is not guaranteed or endorsed by the publisher.
Research integrity at Frontiers
Learn more about the work of our research integrity team to safeguard the quality of each article we publish.