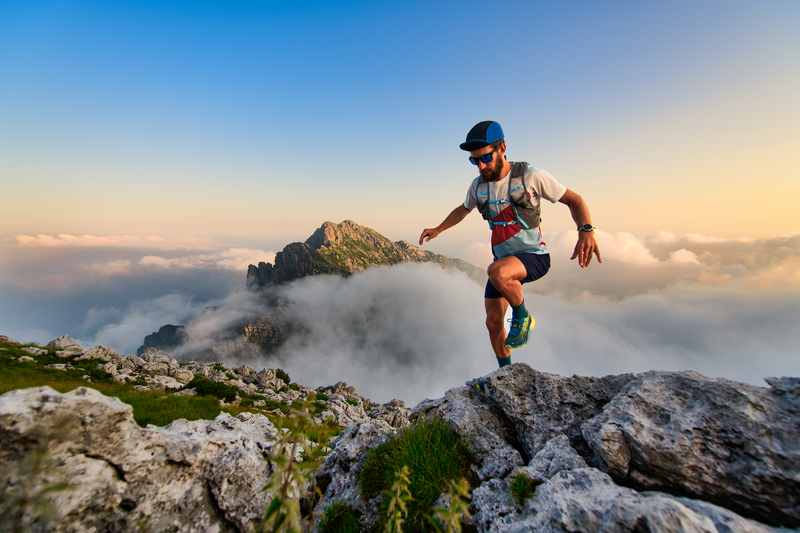
95% of researchers rate our articles as excellent or good
Learn more about the work of our research integrity team to safeguard the quality of each article we publish.
Find out more
REVIEW article
Front. Cell Dev. Biol. , 30 October 2023
Sec. Cellular Biochemistry
Volume 11 - 2023 | https://doi.org/10.3389/fcell.2023.1247339
This article is part of the Research Topic Mitochondrial Plasticity and Quality Control in Health and Disease View all 12 articles
Bronchopulmonary dysplasia (BPD) is a common complication in preterm infants, leading to chronic respiratory disease. There has been an improvement in perinatal care, but many infants still suffer from impaired branching morphogenesis, alveolarization, and pulmonary capillary formation, causing lung function impairments and BPD. There is an increased risk of respiratory infections, pulmonary hypertension, and neurodevelopmental delays in infants with BPD, all of which can lead to long-term morbidity and mortality. Unfortunately, treatment options for Bronchopulmonary dysplasia are limited. A growing body of evidence indicates that mesenchymal stromal/stem cells (MSCs) can treat various lung diseases in regenerative medicine. MSCs are multipotent cells that can differentiate into multiple cell types, including lung cells, and possess immunomodulatory, anti-inflammatory, antioxidative stress, and regenerative properties. MSCs are regulated by mitochondrial function, as well as oxidant stress responses. Maintaining mitochondrial homeostasis will likely be key for MSCs to stimulate proper lung development and regeneration in Bronchopulmonary dysplasia. In recent years, MSCs have demonstrated promising results in treating and preventing bronchopulmonary dysplasia. Studies have shown that MSC therapy can reduce inflammation, mitochondrial impairment, lung injury, and fibrosis. In light of this, MSCs have emerged as a potential therapeutic option for treating Bronchopulmonary dysplasia. The article explores the role of MSCs in lung development and disease, summarizes MSC therapy’s effectiveness in treating Bronchopulmonary dysplasia, and delves into the mechanisms behind this treatment.
Bronchopulmonary dysplasia (BPD) is a chronic lung disease of preterm infants characterized by impaired alveolar and vascular development, resulting in poor gas exchange and significant respiratory morbidity (Jobe, 2011; Gilfillan et al., 2021; Schmidt and Ramamoorthy, 2022). The primary drivers of BPD pathology are inflammation, oxidative stress, and parenchymal fibrosis (Higano et al., 2018; Benjamin et al., 2021; Holzfurtner et al., 2022; Kimble et al., 2022). BPD causes long-term issues like emphysema and pulmonary hypertension (Gough et al., 2014; Vom Hove et al., 2014).
MSCs have emerged as a promising candidate for BPD therapy due to their capacity for self-renewal, high proliferative potential, and ability to differentiate into cell types of mesodermal lineage (Thebaud et al., 2021). They also have immunomodulatory properties that allow allogeneic transplantation. Several clinical trials have evaluated MSC therapy in BPD patients. Intratracheal or intravenous MSC administration has decreased pro-inflammatory cytokines in tracheal aspirates, improved alveolarization, and reduced BPD severity (Alvarez-Fuente et al., 2018). While initially, it was thought that MSCs engrafted and differentiated directly into damaged lung tissue, recent evidence indicates paracrine signaling is the primary mechanism of action. Several recent studies have shown that MSCs communicate with other cells through paracrine signaling (Di Bernardo et al., 2014; Obendorf et al., 2020). MSC paracrine signaling of anti-apoptotic, anti-inflammatory, and pro-angiogenic bioactive substances to the microenvironment through extracellular vesicles (EVs) is thought to be the primary means of communication between MSCs and injured lung tissue (Lai et al., 2010; Aliotta et al., 2016; Reiter et al., 2017; Varkouhi et al., 2019; Varderidou-Minasian and Lorenowicz, 2020; Hao et al., 2022; Xiong et al., 2023). In particular, MSC-derived EVs like exosomes and endosomes have emerged as critical intercellular communicators that can reprogram damaged cells through the transfer of bioactive cargo (Jadli et al., 2020). MSC-EVs have been found to enhance cell proliferation, inhibit apoptosis, stimulate cell migration, modulate differentiation, promote angiogenesis, regulate immune responses, suppress inflammation, improve mitochondrial function, and attenuate oxidative stress. By orchestrating these diverse restorative effects in the damaged neonatal lung, MSC-EV therapies may alleviate structural and vascular defects associated with arrested alveolarization and dysregulated vascular development in BPD (Mansouri et al., 2019; Abele et al., 2022; Sharma et al., 2022).
The mitochondria are essential to the normal development and function of lung cells, including the regulation of metabolism, growth, differentiation, and injury responses (Zhang Y. et al., 2022). Bronchopulmonary dysplasia is associated with defects in mitochondrial structure, dynamics, DNA integrity, and oxidative metabolism (Xuefei et al., 2021). MSC therapy has been shown to alleviate mitochondrial dysfunction in BPD animal models, leading to improved alveolar and lung vascular growth (Islam et al., 2012; Dutra Silva et al., 2021). There is emerging evidence that mesenchymal stem cells (MSCs) can directly donate healthy mitochondria to damaged lung cells to restore mitochondrial function (Willis et al., 2018). In addition, MSCs could stimulate mitochondrial replication in injured lung cells through paracrine signaling molecules and extracellular vesicles (Phinney et al., 2015; Willis et al., 2018). Thus, MSCs may help resolve detrimental inflammation that can further impair mitochondrial and cellular function in lung cells injured by disease. By understanding how MSCs transfer mitochondria, promote mitochondrial biogenesis, and reduce mitochondrial inflammation, new therapeutic approaches may be developed to treat respiratory diseases and injuries caused by mitochondrial dysfunction.
This article explores the multifaceted role that MSCs play in lung development and disease. Additionally, we will provide an overview of current knowledge on the effectiveness of MSC therapy in treating BPD and shed light on the mechanisms driving this approach.
Mammalian lung development is precisely controlled by dynamic epithelial-mesenchymal interactions (Morrisey and Hogan, 2010). By controlling epithelial differentiation and function, MSCs play an essential role in lung morphogenesis and homeostasis (Driscoll et al., 2012). Through paracrine signaling, MSCs also secrete growth factors that influence lung development.
During embryogenesis, lung MSCs originate from the splanchnic mesoderm and undergo proliferation and differentiation into specialized mesenchymal cells (Cardoso and Lu, 2006). These lung MSCs exhibit clonogenicity, self-renewal, and multidifferentiation potential into mesodermal lineages (Jarvinen et al., 2008). Several studies have highlighted the intimate relationship between MSCs and their mitochondria. A healthy and efficient mitochondrial system is critical for optimizing MSCs (Forni et al., 2016). MSCs undergo rapid proliferation and differentiation during lung development, requiring mitochondrial energy. As differentiation proceeds, mitochondria undergo a metabolic shift toward OXPHOS, indicating their essential role in MSC maturation (Pattappa et al., 2011). For instance, during the differentiation of bone marrow mesenchymal stromal cells (BMSCs), mitochondrial length increased along with decreased DLP1 protein levels and increased OPA1 protein levels; this indicates a shift toward mitochondrial fusion, which maintains BMSC stemness (Feng et al., 2019). MSC differentiation can be hampered by dysfunctional mitochondria, characterized by decreased membrane potential, impaired OXPHOS, and increased ROS (Chen et al., 2008). Another fascinating aspect is MSCs’ ability to transfer mitochondria. As a result of mitochondrial transfer from MSCs to damaged cells, the recipient cells are more likely to be repaired and recovered (Islam et al., 2012). The mechanism may be instrumental in repairing lung injuries and preserving lung health.
In the surrounding mesenchyme of the bronchi, mesenchymal progenitors contribute to the differentiation of smooth muscle and endothelial cells. Mitochondrial capacity and ATP production in the mesenchyme were disrupted, resulting in MSC absence and impaired airway branching (Zhang K. et al., 2022). Conducting airways are formed by dichotomous branching morphogenesis triggered by inductive signals from the distal lung mesenchyme, such as fibroblast growth factor 10 (FGF10) (Bellusci et al., 1997). MSCs provide key signals regulating this process. Deletion of β-catenin in lung MSCs disrupted airway branching (De Langhe et al., 2008). Also, Wnt signaling in MSCs regulated FGF10 expression and epithelial branching (Shu et al., 2005). Sonic hedgehog (SHH) signaling in MSCs similarly regulates airway patterning (Ikonomou et al., 2020). Intriguingly, disrupting mitochondrial dynamics in the mesenchyme impaired airway branching (Liu et al., 2021). Crosstalk between mitochondrial networks and other cell signaling pathways that regulate MSC growth, migration, and paracrine signaling during branching morphogenesis is of particular interest.
Mesenchymal cells secrete VEGF, TGF-β, and FGFs, influencing alveolar differentiation, proliferation, and formation. The ablation of FGF10 from MSCs impaired alveolar formation (Volckaert et al., 2013). Paracrine signals such as SHH and VEGF regulate MSC differentiation of alveolar epithelial cells (Liu J. et al., 2022). Depleting lung MSCs post-pneumonectomy severely compromised compensatory alveolar regeneration (Zhen et al., 2008). A key role in alveolar formation is played by mesenchymal mitochondrial activity and distribution regulated by mTORC1 pathway. Therefore, MSCs are essential for alveolar development and regeneration after injury. Much is still to be learned about how modulating mitochondrial biogenesis, dynamics, and mitophagy in lung MSCs impacts alveolar epithelial cell growth, differentiation, and matrix production during alveolarization.
The mesenchyme layer and cells exhibit high VEGF, stimulating hemangioblasts to form blood pools (Greenberg et al., 2002). Deleting β-catenin in lung MSCs disrupted vascular patterning (De Langhe et al., 2008). Consequently, MSCs provide the trophic signals necessary for coordinated lung vascular development.
As a result, lung MSCs are crucial for airway branching, alveolarization, and vascular development throughout lung morphogenesis. Lung development is orchestrated by the intricate dance between mitochondria and MSCs (Figure 1). Despite our growing understanding over the years, much remains to be explored. Future studies elucidating the molecular and cellular mechanisms underlying their interaction can pave the way for innovative therapeutic strategies in lung medicine.
FIGURE 1. The role of mesenchymal stem cells in pulmonary development. During embryonic development, lung mesenchymal stem cells (MSCs) arise from the splanchnic mesoderm and subsequently differentiate into specialized mesenchymal lineages through paracrine signaling and mitochondrial regulation to coordinate key processes in lung morphogenesis, including airway branching, alveolarization, and vascular development.
Several prenatal risk factors for BPD have been identified, including maternal smoking, hypertension, placental insufficiency, and chorioamnionitis (Klinger et al., 2013; Mizikova and Thebaud, 2023). Placental abnormalities leading to imbalanced pro- and anti-angiogenic signaling may disrupt fetal lung vascular development, significantly impacting BPD susceptibility and severity (Hansen et al., 2010; Ozkan et al., 2012; Torchin et al., 2016; Sucre et al., 2021). Premature infants born during the canalicular and saccular stages of lung development are at highest risk for developing BPD (Jobe, 2011). Endogenous MSCs play a critical role in alveolarization through the tightly regulated processes of alveolar septation and vascularization (Morrisey and Hogan, 2010; Klein, 2021). The decrease or functional impairment in resident stem cells within the lung tissue of infants leads to simplified alveolar architecture and abnormal pulmonary vasculature contributing to BPD (Alphonse et al., 2012; Collins and Thebaud, 2014; Simones et al., 2018).
Extensive research has been conducted on how interference with MSCs and their signaling pathways can result in the onset of BPD. MSCs, similar to fibroblast cells, are found in the mesodermal tissue in adults and fetuses (Anker et al., 2003; Fang et al., 2019). Their presence has been noted in a variety of locations including bone marrow, adipose tissue, lung tissue, the placenta, and the stroma of the umbilical cord (Anker et al., 2003; Igura et al., 2004; Nguyen et al., 2022). BPD treatment methods such as hyperoxia and mechanical ventilation can negatively impact the functionality of the MSCs residing in the lung. Interestingly, these cells are copious in fetal lungs and can be a biomarker for predicting BPD onset when retrieved from tracheal aspirates of prematurely born infants. Growth factor expression involved in alveolar development and repair, such as VEGF and FGF10, is reduced in these MSCs (Mobius et al., 2019).
The precise mechanisms through which endogenous MSCs switch from protecting against BPD to contributing to BPD remain incompletely understood. The MSCs can repair damaged cells by promoting growth and differentiation. For instance, a subset of Dermo1+ endogenous MSCs has been recognized as having the capacity to function as stem cells in regenerating airway epithelial cells during the repair process following LPS-induced acute lung injury (Fang et al., 2019). Current data indicates that paracrine signaling and immune modulation are likely key players in mitigating hyperoxia-induced lung injury. EVs often facilitate paracrine signaling, carrying growth factors such as TGF-β. In a BPD model triggered by hyperoxia, an escalation in TGF-β expression was observed in the lungs, leading to structural changes; this included the multiplication of α-actin-positive myofibroblasts within the alveolar septal wall and the emergence of abnormal alveolar and vascular structures (Luan et al., 2015). Interestingly, when bone marrow stem cells were administered, a considerable decline in TGF-β levels was observed (Luan et al., 2015). These outcomes suggest that TGF-β could stimulate abnormal differentiation of alveolar mesenchymal progenitor cells into myofibroblasts, eventually playing a role in BPD progression. Furthermore, under intrauterine circumstances, human fetal lung MSCs were observed to generate considerable quantities of elastin and sulfated glycosaminoglycans, key constituents required for typical lung development. Examination of the secretome revealed that human fetal lung MSCs could be responsible for secreting molecules essential for angiogenic and inflammatory signaling, such as IL-8, VEGF, angiogenin and angiopoietin-1 (Mobius et al., 2019). Secretome analysis also revealed the existence of molecules that promote epithelial cell maturation and protection, such as keratinocyte growth factor/FGF7, FGF10 and the antioxidant known as stanniocalcin-1. In addition, inflammatory mediators such as monocyte chemotactic protein-1, stromal cell–derived factor-1, IL-6, and the tissue inhibitor of metalloproteinases 1 were also detected (Mobius et al., 2019). Exposing lung MSCs to hyperoxia recapitulated some harmful events associated with post-premature birth, such as cell apoptosis, decreased colony-forming capabilities, and alterations in MSCs’ surface markers. It hindered the secretion of factors crucial for lung growth. These effects may play a part in the development of BPD (Mobius et al., 2019).
In the following section, we delve into the regulatory role of MSCs in the complex pathogenesis of BPD (Table 1), intending to identify potential targets for MSC-based BPD treatments.
TABLE 1. Effects of decreased and dysfunctional endogenous mesenchymal stem cells on BPD in preclinical experiments.
While fetal lungs usually develop in a hypoxic intrauterine environment, BPD is a condition precipitated by premature exposure to hyperoxic conditions at birth, due either to oxygen treatment in efforts to oxygenate the infants sufficiently or simply by exposure to the relative hyperoxic conditions of the atmosphere. This condition subjects infants with extremely low birth weights to oxidative stress (Kimble et al., 2022). Cellular components such as the mitochondria: its DNA, the electron transport chain, and reactive oxygen generation machinery are particularly susceptible to oxidant damage. The function of mitochondria is pivotal in moderating the response to oxidative stress and affects the pluripotency and regenerative potential of MSCs (Paliwal et al., 2018). In a study exploring the correlation between mitochondrial function and the MSC function, electron transport chain complex-IV activity, basal and maximal oxygen consumption rates, spare respiratory capacity, and ATP-linked oxygen consumption rate were reduced. Proton leak was increased in MSCs from extremely low birth weight infants who either perished or developed moderate/severe BPD, compared to MSCs from infants who survived with none to mild BPD (Hazra et al., 2022).
PTEN-induced putative kinase 1 (PINK1) mediated mitophagy is one mechanism cells use to eliminate dysfunctional mitochondria. As a result, oxidative stress-induced mitochondrial dysfunction could lead to MSC depletion, thus obstructing lung development. Another finding described that MSCs from infants who died or developed moderate/severe BPD after O2 treatment showed lower PINK1 expression, implying decreased mitophagy (Hazra et al., 2022). The antioxidant stanniocalcin-1 was also reduced in fetal lung MSCs exposed to hyperoxia (Mobius et al., 2019).
In conclusion, prenatal risk factors for BPD, such as hyperoxic conditions, lead to oxidative stress and MSC dysfunction, which can contribute to BPD’s development and advancement.
Pulmonary microvascular dysplasia is a component of BPD pathogenesis; therefore, fostering pulmonary angiogenesis and enhancing vasculogenesis are crucial objectives to mitigate this dysplasia. Under hyperoxic conditions, it was found that MSCs in the tracheal aspirates of preterm infants’ lungs exhibited reduced expression of growth factors crucial for alveolar development and repair, such as VEGF, FGF10, and angiogenin, through the decrease in SOX-2 and OCT-4 (Mobius et al., 2019). Moreover, the CD146+ MSCs in the lungs exposed to hyperoxia displayed decreased gene expression of notable proangiogenic genes, including FGF9 and IL-6, through SHH signaling (White et al., 2007; Kwon et al., 2014). In a rat model of BPD induced by hyperoxia, the resident lung CD146+ MSCs were found to inhibit rather than promote angiogenesis through the axon guidance signaling (Semaphorins 3A and 3E) (Collins et al., 2018). Consequently, BPD insults prompt alterations in angiogenic pathways, which result in an MSC phenotype with aberrant microvascular development, a key characteristic of BPD pathogenesis (Alvira, 2016).
An investigation involving hyperoxia-induced BPD in rodent models reported decreased endothelial progenitor cells within the bloodstream, lungs, and bone marrow (Balasubramaniam et al., 2007). Endothelial progenitor cells, also called endothelial colony-forming cells, are required for vasculogenesis in the developing lung and are associated with the support and maturation of endothelial progenitor cells. Crucial to the regional specialization of embryonic lung tissue, MSCs at the outer edge of branching epithelium are noted for producing FGF10, a vital element in the signaling interplay involving BMP, Wnt, and sonic hedgehog pathways that direct the differentiation of epithelial stem/progenitors during lung development (Morrisey and Hogan, 2010). In vitro studies have shown that MSCs substantially support the growth and differentiation of epithelial stem cells (McQualter et al., 2010). Further highlighting this critical microenvironmental association, recent in vivo research employing the naphthalene injury model showed that parabronchial mesenchymal cells discharge FGF10 to stimulate epithelial regeneration in the remaining progenitor cells (McQualter et al., 2010). These findings support the theory that a reduction in MSCs or malfunctioning MSCs might cause the hindered growth of endothelial progenitor cells and affect their angiogenic supportive capacity, thereby contributing to the onset of BPD.
Inflammatory imbalance plays a significant role in the development of BPD. Various immune regulatory attributes have been credited to MSCs from different tissue origins, contributing to tissue regeneration, cell death prevention, tissue fibrosis inhibition, and the reduction of tissue damage (Li et al., 2019). MSCs isolated from the tracheal aspirates of preterm infants show a propensity towards inflammation, secreting increased levels of pro-inflammatory cytokines like CXCL-1, IL-6, and IL-8 and decreased anti-fibrotic factor levels of CXCL1/GRO-α and HGF, possibly through dysregulated FoxF1, Wnt5a, and Tbx3 signaling (Bozyk et al., 2011). Traditional pro-inflammatory cytokines such as TNF-α, IL-1β, IL-6, and IL-8 trigger comparable changes in MSCs derived from preterm infants with severe BPD. NFκB targeting was observed to reverse this pro-inflammatory tendency (Reicherzer et al., 2018). In the lungs of infants suffering from BPD, it was discovered that thicker alveolar walls were linked with a scarcity of PDGFR-α-positive cells in the malformed alveolar septa (Popova et al., 2014). A significant decrease in the volume of PDGFR-α-positive alveolar tips was observed in the lungs of neonatal mice exposed to excess oxygen in another study (Popova et al., 2014). Another study demonstrated enhanced apoptosis in PDGFR-α-positive mesenchymal cells through abrogation of TNF-α-mediated NFκB signaling and primarily influenced by TGF-β1 signaling (Ehrhardt et al., 2016). The authors concluded that this phenomenon explained much of the amplified lung damage during mechanical ventilation (Ehrhardt et al., 2016).
Alveolar macrophages, responsible for maintaining a balanced immune response in the lung, react to changing environmental conditions in various ways, often simplified into anti-inflammatory and pro-inflammatory effects. Hyperoxia, a prevalent condition in BPD supportive care, can damage alveolar macrophages. PGE2, a substance secreted by MSCs, plays a pivotal role in the bioenergetic shift in macrophages, increasing anti-inflammatory activation and decreasing pro-inflammatory activation through AMPK activity/SIRTUIN 1 signaling (Vasandan et al., 2016). Levels of prostaglandin E2 were found to be reduced in hyperoxia–exposed fetal lung MSCs (Mobius et al., 2019). Consequently, the decrease in anti-inflammatory MSCs or the impairment of their anti-inflammatory function contributes to the pathogenesis of BPD.
Studies using animal models continue to refine the use of MSCs in therapeutic strategies. The potential of MSCs to restore normal lung function has sparked a desire to understand their interplay with damaged lung tissue. As discussed, MSCs act through paracrine signaling, with EVs playing an essential role in this mechanism (Figure 2). The EVs are between 30 and 1000 nm in size and are secreted by most cell types. After binding and internalization, they initiate signal transduction in target cells (Omar et al., 2022). Typically, they arise from multivesicular bodies whose plasma membranes are budding inward. The EVs contain cytokines, proteins, mRNA, microRNAs, DNA, lipids, metabolites and even mitochondria from the parent cells. Several mechanisms can enable EVs to transfer their molecular cargo when released, including fusing with target cell membranes or being endocytosed (Jadli et al., 2020). EVs can modulate recipient cells, affecting immunity, development, homeostasis, and disease pathogenesis. Preconditioning MSCs in vitro can enhance the regenerative capacity of secreted EVs. MSC-EVs can also be enhanced therapeutically by bioengineering or genetic modification (Figure 2). By optimizing EV production protocols and delivery strategies, MSC-EV therapy for BPD may be translated into clinical practice. A better understanding of MSC-EV cargo and underlying mechanisms of lung repair will facilitate the development of more potent acellular treatments. Several studies have found that MSC-EVs have protective effects on the lung by blocking inflammation, improving lung function, and reducing pulmonary hypertension (Mansouri et al., 2019; Abele et al., 2022; Sharma et al., 2022). A recent study discovered that MSCs release several growth factors linked to angiogenesis, predominantly VEGF, stored in EVs, particularly exosomes (Xi et al., 2022); this suggests a potential method for MSC-exosomes to increase VEGF-driven angiogenesis in BPD treatment (Xi et al., 2022). An experimental study evaluating a hyperoxia-induced BPD rat model compared the protective effects of intratracheally administered MSCs versus EVs to ascertain the differences between MSC and MSC-derived extracellular vesicle administration. The study found that both MSCs and MSC-EVs alleviated hyperoxia-induced damage, but MSC-EVs achieved superior outcomes regarding alveolarization and lung vascularization (Braun et al., 2018; Moreira et al., 2020). The benefits of using EVs over MSCs for treatment include easier intratracheal administration, the potential for modifications to enhance the benefits of extracellular vesicle treatment, and the option to freeze-dry (lyophilize) them to preserve their biological activity.
FIGURE 2. MSC-EVs mediate therapeutic effects in BPD. MSCs predominantly promote lung repair in BPD through paracrine. MSCs release EVs containing bioactive molecules and mitochondria that mitigate injury. In addition, EV isolation offers the potential for cell-free treatment. MSC-EVs are enhanced in their regenerative capacity by pre-conditioning, bioengineering, and genetic modification in vitro. Optimizing EV production and delivery to maximize lung localization may enable clinical translation for treating BPD.
As highlighted earlier, decreased VEGF results in compromised angiogenesis, significantly contributing to BPD development. Extended hyperoxia interrupts normal lung development, a phenomenon linked with abnormal expression of Akt and VEGF (You et al., 2020). A study on a hyperoxia-induced rat model of BPD found that the expressions of phosphorylated Akt and VEGF-A significantly increased following treatment with small EVs derived from human umbilical cord mesenchymal stem cells. At the same time, PTEN and cleaved caspase-3 expression were found to negatively correlate with phosphorylated AKT in the lungs after treatment with these small EVs (You et al., 2020). PTEN functions as the primary regulator of Akt, and it has been documented that the signaling pathway involving PTEN and Akt governs cellular growth, programmed cell death (apoptosis), and the formation of new blood vessels (angiogenesis). Therefore, these findings suggest that small EVs, derived from human umbilical cord mesenchymal stem cells, boost pulmonary alveolarization and angiogenesis to counteract BPD through suppressing PTEN and activating Akt/VEGF pathway.
Research into the paracrine signaling of MSCs via EVs has been paralleled by studies delving into microRNA. One study examined the effect of bone marrow-derived MSCs on microRNA (miR) in a trial involving newborn mice. MiRs, non-coding RNAs of 21–25 nucleotides in length, have roles in several biological processes, including cell proliferation, cell death, resistance to stress, and tumorigenesis. MiR-206 is known to be downregulated in both patients with BPD and newborn mice. Previous research has highlighted the role of miR-206 in BPD by demonstrating its impact on the expression of fibronectin 1. This glycoprotein, a vital component of the extracellular matrix (ECM), plays a crucial role in various biological processes such as cell adhesion and migration, embryonic development, wound repair, cancer metastasis, and immune responses (Zhang et al., 2013). Bone marrow-derived MSCs, when transfected with miR-206, showed functional regulation by enhancing the expression of surfactant protein C and reducing the levels of inflammatory cytokines such as fibronectin 1, TGF-β1, and IL-6. These changes led to a reduction in lung fluid accumulation (pulmonary edema) and an improvement in the formation of alveoli. This finding hints at the potential therapeutic benefits these MSCs might offer in treating BPD (Zhang et al., 2013).
Porzionato et al. (Porzionato et al., 2021) evaluated the protective effects of MSC-derived EVs administered intratracheally using a newborn rat model exposed to hyperoxia for 2 weeks and 4 weeks of recovery. The rats exposed to hyperoxia displayed decreased and expanded distal air spaces, along with sporadic areas of interstitial thickening. The study showed that the treatment with MSC-derived EVs prevented the accumulation of connective or fibrous tissue in the lung parenchyma and sustained the presence of CD163-positive macrophages in hyperoxia-exposed rats, compared to those maintained in normoxic conditions (Porzionato et al., 2021). The treatment did not decrease the total macrophage population but influenced the balance between pro-inflammatory and anti-inflammatory macrophages. Anti-inflammatory macrophages, identified by CD163, have been shown to facilitate alveolarization during normal lung development. The study disclosed that extracellular vesicle treatment averted a decline in the density of interstitial alveolar CD163+ macrophages in rats exposed to hyperoxia (Porzionato et al., 2021). Despite the theoretical benefit of preventing a decrease in CD163+ cells through extracellular vesicle treatment, ongoing damage might still cause these macrophages to stimulate excessive ECM deposition by activating resident fibroblasts via the release of pro-fibrotic factors (Porzionato et al., 2021); this implies additional mechanisms play a part in the anti-fibrotic effect of MSC-EVs, necessitating further research.
A recent study shows that MSC-derived extracellular vesicles can reshape the lung macrophage profile, reducing inflammation and immune reactions to counteract BPD caused by hyperoxia (Willis et al., 2018). The role of MSCs extends beyond paracrine processes (such as carriers of 150-nm exosomes). Alternatively, more giant vesicles (over 500 nm wide) or direct connections between cells might carry more intricate structures, such as mitochondria (Willis et al., 2018). Mitochondria’s potential role in MSC action could pave the way for more refined, effective MSC therapies for BPD.
The study of placental mesenchymal stem cells (PMSCs) has grown in recent years due to their application in various physiological and pathological conditions. Numerous benefits can be gained from PMSCs, including their multipotency and immunomodulatory capabilities. In contrast to embryonic stem cells, PMSCs can be harvested from young donors in large quantities, overcoming ethical concerns associated with embryonic stem cells (Mathew et al., 2020; Zhang Y. et al., 2022). In an ex-vivo fetal lung culture model, PMSCs exert more effective stimulation on perinatal lung morphogenesis than BMSCs (Di Bernardo et al., 2014); this suggests PMSCs are excellent candidates for regenerative medicine, enabling their extensive use in various stem cell therapy studies. PMSCs have shown the capacity to preserve expanded umbilical cord blood CD34+ cells during cryopreservation, enhancing the recovery, survival, and functionality of these cells upon revival; this is achieved through the reduction of oxidative stress. Interestingly, PMSCs have outperformed cord MSCs in providing cryoprotection to the umbilical cord blood cells (Kadekar et al., 2016). A recent study found that co-cultivation with PMSCs boosted ATP production in trophoblasts by regulating calcium channel expression and inducing mild oxidative stress (Seok et al., 2020). Moreover, exosomes derived from human placenta choriodecidual membrane-derived mesenchymal stem cells significantly mitigated LPS-induced lipid peroxidation and apoptosis in the lung (Seok et al., 2020). These studies suggest that PMSC may help treat the cellular metabolic alterations associated with BPD. However, further research is required to explore the regulatory role of PMSCs in oxidative stress and mitochondrial function in the context of BPD development and treatment. Mass augmenting mitochondrial function and electron transport chain complexes could contribute to the therapeutic efficacy of MSCs, a concept currently under evaluation in clinical trials.
Another provocative finding is that the conditioned medium from PMSCs has been found to contain both factors that stimulate and inhibit angiogenesis, aiding the enhancement of endothelial tube formation. It was observed that endothelial cells take in exosomes from PMSCs, which promotes the formation and migration of tubes and enhances the expression of genes linked to angiogenesis, leading to better angiogenesis in vivo (Komaki et al., 2017). Growing attention is being focused on the possible role of PMSCs in influencing angiogenesis in the progression and treatment of BPD. Notably, PMSCs display ease of propagation and superior immunoregulatory attributes, rendering them an appealing treatment option for diseases linked with inflammation in the future (Wu et al., 2017). Studies have shown that human PMSCs can counteract the polarization of pro-inflammatory macrophages and release pro-inflammatory cytokines triggered by LPS by modulating TLR4 expression and the NF-κB signaling pathway (Liu Y. et al., 2022). Therefore, in the landscape of BPD, immune cells may represent promising targets for therapeutic interventions employing PMSC-derived EVs.
Numerous preclinical studies have conducted comprehensive experimental studies, leading to a handful of clinical trials currently underway to examine the impact of MSC therapy (Table 2). A pioneering phase I clinical trial was conducted on nine preterm infants, with gestational ages ranging from 23 to 29 weeks, who needed mechanical ventilation within 5–14 days after birth (Ahn et al., 2017). These infants received an intratracheal delivery of either 107 or 2 × 107 MSCs derived from the umbilical cord. This group of infants did not experience any unfavorable events, thus affirming the safety of the MSC treatment administered intratracheally (Ahn et al., 2017). Following this, the same research team reported that the treated infants displayed no deficits in neurological, respiratory, or growth parameters even after 2 years (Ahn et al., 2017). A noteworthy aspect of this phase I clinical trial is its establishment of the safety of intratracheal MSC administration, opening doors for future clinical trials. A different clinical trial investigates the safety and effectiveness of allogeneic human umbilical cord-derived MSCs (hUC-MSCs) delivered intravenously. The dosage was incrementally increased to gather supportive data for the safety of intravenously delivered hUC-MSCs in treating patients with severe BPD. Importantly, this trial is the first to focus on the therapeutic effects of hUC-MSCs in children suffering from severe BPD rather than merely the preventative effects (Wu et al., 2020).
A case study recounted the treatment of an extremely premature baby suffering from severe BPD who received MSC therapy (1 × 107 cells/kg/dose) both intratracheally and intravenously on the 78th day after birth. Before the treatment, the baby had been dependent on mechanical ventilation, initially with volume-targeted conventional therapy and later with high-frequency oscillatory ventilation. Despite receiving four doses of surfactant and undergoing two cycles of steroid therapy, the infant showed no improvement (Yilmaz et al., 2021). Following the MSC treatment, no deterioration in blood gas or indications of sepsis were noted, and there were no reported adverse events. The treatment was initiated after detecting chronic changes in the infant’s chest X-ray on the 78th day after birth. It yielded positive results of decreased emphysema and alveolar damage (Yilmaz et al., 2021). This encouraging case study indicates that MSC therapy may offer a promising therapeutic avenue for managing severe BPD in premature babies in the future.
Mesenchymal stem cells (MSCs) have shown promising potential as a treatment for bronchopulmonary dysplasia (BPD), a chronic lung disease affecting premature infants. MSCs possess immunomodulatory and regenerative capacities that may mitigate lung injury and facilitate repair. However, several challenges must be addressed before MSC therapy can be widely adopted for BPD.
A significant hurdle is the inherent variability of MSCs depending on tissue source. MSCs derived from different donors and adult tissues exhibit differences in proliferative capacity, migratory abilities, cytokine secretion, and other functions relevant to BPD treatment. Standardizing and optimizing MSC-based therapies will require identifying the ideal MSC populations and culture methods tailored to BPD pathology. Factors influencing the localization, survival, and bioactivity of administered MSCs in the injured neonatal lung remain unclear.
Another concern is the potential influence of MSCs on cancer development. While MSCs appear less tumorigenic than other stem cells, their long-term safety requires further investigation, especially in vulnerable neonate populations. Animal studies have not detected tumor growth, but clinical evidence is still lacking.
It is imperative to note that MSCs are not intrinsically immune-privileged, and both autologous and allogeneic ways can stimulate anti-MSC immune responses, which can negatively impact their survival and efficacy. It is crucial to understand these complex dynamics to improve MSC-based therapies.
Additionally, guidelines are needed for identifying patients most likely to benefit from MSC treatment and the optimal administration timeline. Recent trials in ventilated preterm infants suggest efficacy, but more extensive studies are necessary to evaluate long-term outcomes and standardize cell production. Variations in manufacturing processes can alter MSC potency.
Future research should clarify mechanisms underlying MSC-mediated lung repair in BPD, such as paracrine signaling via EVs mitochondria through more giant vesicles (over 500 nm wide) or direct connections between cells. Strategies to enhance MSC engraftment and survival post-transplantation warrant exploration. As MSC therapy for BPD advances, international consensus on cell preparation, characterization, and delivery protocols will enable meaningful comparisons across clinical trials. A precision medicine approach adapting MSC therapy to each patient’s disease phenotype may prove optimal.
Bronchopulmonary dysplasia (BPD) is a chronic pulmonary disease that affects preterm infants, and its prevalence is increasing due to improved survival rates among this at-risk group. This condition arises from various factors, including maternal health, prenatal environment, and modern treatments for preterm babies, such as mechanical ventilation. Recent experimental studies have increased our understanding of the underlying pathogenetic mechanisms of BPD and revealed potential therapeutic targets. As BPD primarily manifests as a functional decline and reduction in lung stem cell populations, the prospect of replenishing and regenerating these cells could be crucial to its treatment. Recent clinical trials have validated the safety and effectiveness of MSC transplantation, with outcomes including a reduction in inflammation, mitochondrial oxidative stress, lung damage, and fibrosis. MSC-based therapy holds significant promise for promoting lung repair and regeneration in BPD. Realizing the full potential of MSCs will require systematic preclinical studies to elucidate mechanisms, optimize treatment protocols, and assess long-term safety. Well-designed clinical trials are needed to evaluate different MSC sources, combination therapies, and cell-free approaches. If critical challenges are addressed, MSCs could become an effective treatment for this debilitating developmental lung disease.
DY conceptualization; SZ and CM writing–original draft preparation; SR, RS, and DY writing–review and editing. All authors contributed to the article and approved the submitted version.
This work was supported by funding from Science and Technology Plan Project of Liaoning Province (No. 2020JH2/10300128) and Natural Science Foundation of Liaoning Province (No. 201602873).
The authors declare that the research was conducted in the absence of any commercial or financial relationships that could be construed as a potential conflict of interest.
All claims expressed in this article are solely those of the authors and do not necessarily represent those of their affiliated organizations, or those of the publisher, the editors and the reviewers. Any product that may be evaluated in this article, or claim that may be made by its manufacturer, is not guaranteed or endorsed by the publisher.
Abele, A. N., Taglauer, E. S., Almeda, M., Wilson, N., Abikoye, A., Seedorf, G. J., et al. (2022). Antenatal mesenchymal stromal cell extracellular vesicle treatment preserves lung development in a model of bronchopulmonary dysplasia due to chorioamnionitis. Am. J. Physiol. Lung Cell Mol. Physiol. 322, L179–L190. doi:10.1152/ajplung.00329.2021
Ahn, S. Y., Chang, Y. S., Kim, J. H., Sung, S. I., and Park, W. S. (2017). Two-year follow-up outcomes of premature infants enrolled in the phase I trial of mesenchymal stem cells transplantation for bronchopulmonary dysplasia. J. Pediatr. 185, 49–54. doi:10.1016/j.jpeds.2017.02.061
Aliotta, J. M., Pereira, M., Wen, S., Dooner, M. S., Del Tatto, M., Papa, E., et al. (2016). Exosomes induce and reverse monocrotaline-induced pulmonary hypertension in mice. Cardiovasc Res. 110, 319–330. doi:10.1093/cvr/cvw054
Alphonse, R. S., Rajabali, S., and Thebaud, B. (2012). Lung injury in preterm neonates: the role and therapeutic potential of stem cells. Antioxid. Redox Signal 17, 1013–1040. doi:10.1089/ars.2011.4267
Alvarez-Fuente, M., Arruza, L., Lopez-Ortego, P., Moreno, L., Ramirez-Orellana, M., Labrandero, C., et al. (2018). Off-label mesenchymal stromal cell treatment in two infants with severe bronchopulmonary dysplasia: clinical course and biomarkers profile. Cytotherapy 20, 1337–1344. doi:10.1016/j.jcyt.2018.09.003
Alvira, C. M. (2016). Aberrant pulmonary vascular growth and remodeling in bronchopulmonary dysplasia. Front. Med. (Lausanne) 3, 21. doi:10.3389/fmed.2016.00021
Anker, P. S., Noort, W. A., Scherjon, S. A., Kleijburg-Van Der Keur, C., Kruisselbrink, A. B., Van Bezooijen, R. L., et al. (2003). Mesenchymal stem cells in human second-trimester bone marrow, liver, lung, and spleen exhibit a similar immunophenotype but a heterogeneous multilineage differentiation potential. Haematologica 88, 845–852.
Balasubramaniam, V., Mervis, C. F., Maxey, A. M., Markham, N. E., and Abman, S. H. (2007). Hyperoxia reduces bone marrow, circulating, and lung endothelial progenitor cells in the developing lung: implications for the pathogenesis of bronchopulmonary dysplasia. Am. J. Physiol. Lung Cell Mol. Physiol. 292, L1073–L1084. doi:10.1152/ajplung.00347.2006
Bellusci, S., Grindley, J., Emoto, H., Itoh, N., and Hogan, B. L. (1997). Fibroblast growth factor 10 (FGF10) and branching morphogenesis in the embryonic mouse lung. Development 124, 4867–4878. doi:10.1242/dev.124.23.4867
Benjamin, J. T., Plosa, E. J., Sucre, J. M., Van Der Meer, R., Dave, S., Gutor, S., et al. (2021). Neutrophilic inflammation during lung development disrupts elastin assembly and predisposes adult mice to COPD. J. Clin. Invest. 131, e139481. doi:10.1172/JCI139481
Bozyk, P. D., Popova, A. P., Bentley, J. K., Goldsmith, A. M., Linn, M. J., Weiss, D. J., et al. (2011). Mesenchymal stromal cells from neonatal tracheal aspirates demonstrate a pattern of lung-specific gene expression. Stem Cells Dev. 20, 1995–2007. doi:10.1089/scd.2010.0494
Braun, R. K., Chetty, C., Balasubramaniam, V., Centanni, R., Haraldsdottir, K., Hematti, P., et al. (2018). Intraperitoneal injection of MSC-derived exosomes prevent experimental bronchopulmonary dysplasia. Biochem. Biophys. Res. Commun. 503, 2653–2658. doi:10.1016/j.bbrc.2018.08.019
Cardoso, W. V., and Lu, J. (2006). Regulation of early lung morphogenesis: questions, facts and controversies. Development 133, 1611–1624. doi:10.1242/dev.02310
Chen, C. T., Shih, Y. R., Kuo, T. K., Lee, O. K., and Wei, Y. H. (2008). Coordinated changes of mitochondrial biogenesis and antioxidant enzymes during osteogenic differentiation of human mesenchymal stem cells. Stem Cells 26, 960–968. doi:10.1634/stemcells.2007-0509
Collins, J. J., and Thebaud, B. (2014). Lung mesenchymal stromal cells in development and disease: to serve and protect? Antioxid. Redox Signal 21, 1849–1862. doi:10.1089/ars.2013.5781
Collins, J. J. P., Lithopoulos, M. A., Dos Santos, C. C., Issa, N., Mobius, M. A., Ito, C., et al. (2018). Impaired angiogenic supportive capacity and altered gene expression profile of resident CD146(+) mesenchymal stromal cells isolated from hyperoxia-injured neonatal rat lungs. Stem Cells Dev. 27, 1109–1124. doi:10.1089/scd.2017.0145
De Langhe, S. P., Carraro, G., Tefft, D., Li, C., Xu, X., Chai, Y., et al. (2008). Formation and differentiation of multiple mesenchymal lineages during lung development is regulated by beta-catenin signaling. PLoS One 3, e1516. doi:10.1371/journal.pone.0001516
Di Bernardo, J., Maiden, M. M., Jiang, G., Hershenson, M. B., and Kunisaki, S. M. (2014). Paracrine regulation of fetal lung morphogenesis using human placenta-derived mesenchymal stromal cells. J. Surg. Res. 190, 255–263. doi:10.1016/j.jss.2014.04.013
Driscoll, B., Kikuchi, A., Lau, A. N., Lee, J., Reddy, R., Jesudason, E., et al. (2012). Isolation and characterization of distal lung progenitor cells. Methods Mol. Biol. 879, 109–122. doi:10.1007/978-1-61779-815-3_7
Dutra Silva, J., Su, Y., Calfee, C. S., Delucchi, K. L., Weiss, D., Mcauley, D. F., et al. (2021). Mesenchymal stromal cell extracellular vesicles rescue mitochondrial dysfunction and improve barrier integrity in clinically relevant models of ARDS. Eur. Respir. J. 58, 2002978. doi:10.1183/13993003.02978-2020
Ehrhardt, H., Pritzke, T., Oak, P., Kossert, M., Biebach, L., Forster, K., et al. (2016). Absence of TNF-alpha enhances inflammatory response in the newborn lung undergoing mechanical ventilation. Am. J. Physiol. Lung Cell Mol. Physiol. 310, L909–L918. doi:10.1152/ajplung.00367.2015
Fang, S., Zhang, S., Dai, H., Hu, X., Li, C., and Xing, Y. (2019). The role of pulmonary mesenchymal cells in airway epithelium regeneration during injury repair. Stem Cell Res. Ther. 10, 366. doi:10.1186/s13287-019-1452-1
Feng, X., Zhang, W., Yin, W., and Kang, Y. J. (2019). Feature Article: the involvement of mitochondrial fission in maintenance of the stemness of bone marrow mesenchymal stem cells. Exp. Biol. Med. (Maywood) 244, 64–72. doi:10.1177/1535370218821063
Forni, M. F., Peloggia, J., Trudeau, K., Shirihai, O., and Kowaltowski, A. J. (2016). Murine mesenchymal stem cell commitment to differentiation is regulated by mitochondrial dynamics. Stem Cells 34, 743–755. doi:10.1002/stem.2248
Gilfillan, M., Bhandari, A., and Bhandari, V. (2021). Diagnosis and management of bronchopulmonary dysplasia. BMJ 375, n1974. doi:10.1136/bmj.n1974
Gough, A., Linden, M., Spence, D., Patterson, C. C., Halliday, H. L., and Mcgarvey, L. P. (2014). Impaired lung function and health status in adult survivors of bronchopulmonary dysplasia. Eur. Respir. J. 43, 808–816. doi:10.1183/09031936.00039513
Greenberg, J. M., Thompson, F. Y., Brooks, S. K., Shannon, J. M., Mccormick-Shannon, K., Cameron, J. E., et al. (2002). Mesenchymal expression of vascular endothelial growth factors D and A defines vascular patterning in developing lung. Dev. Dyn. 224, 144–153. doi:10.1002/dvdy.10095
Hansen, A. R., Barnes, C. M., Folkman, J., and Mcelrath, T. F. (2010). Maternal preeclampsia predicts the development of bronchopulmonary dysplasia. J. Pediatr. 156, 532–536. doi:10.1016/j.jpeds.2009.10.018
Hao, C., You, J., Qiu, H., Zhou, O., Liu, J., Zou, W., et al. (2022). Hypoxic preconditioning improves the survival and pro-angiogenic capacity of transplanted human umbilical cord mesenchymal stem cells via HIF-1α signaling in a rat model of bronchopulmonary dysplasia. Biochem. Biophys. Res. Commun. 605, 111–118. doi:10.1016/j.bbrc.2022.03.044
Hazra, S., Li, R., Vamesu, B. M., Jilling, T., Ballinger, S. W., Ambalavanan, N., et al. (2022). Mesenchymal stem cell bioenergetics and apoptosis are associated with risk for bronchopulmonary dysplasia in extremely low birth weight infants. Sci. Rep. 12, 17484. doi:10.1038/s41598-022-22478-5
Higano, N. S., Spielberg, D. R., Fleck, R. J., Schapiro, A. H., Walkup, L. L., Hahn, A. D., et al. (2018). Neonatal pulmonary magnetic resonance imaging of bronchopulmonary dysplasia predicts short-term clinical outcomes. Am. J. Respir. Crit. Care Med. 198, 1302–1311. doi:10.1164/rccm.201711-2287OC
Holzfurtner, L., Shahzad, T., Dong, Y., Rekers, L., Selting, A., Staude, B., et al. (2022). When inflammation meets lung development-an update on the pathogenesis of bronchopulmonary dysplasia. Mol. Cell Pediatr. 9, 7. doi:10.1186/s40348-022-00137-z
Igura, K., Zhang, X., Takahashi, K., Mitsuru, A., Yamaguchi, S., and Takashi, T. A. (2004). Isolation and characterization of mesenchymal progenitor cells from chorionic villi of human placenta. Cytotherapy 6, 543–553. doi:10.1080/14653240410005366-1
Ikonomou, L., Herriges, M. J., Lewandowski, S. L., Marsland, R., Villacorta-Martin, C., Caballero, I. S., et al. (2020). The in vivo genetic program of murine primordial lung epithelial progenitors. Nat. Commun. 11, 635. doi:10.1038/s41467-020-14348-3
Islam, M. N., Das, S. R., Emin, M. T., Wei, M., Sun, L., Westphalen, K., et al. (2012). Mitochondrial transfer from bone-marrow-derived stromal cells to pulmonary alveoli protects against acute lung injury. Nat. Med. 18, 759–765. doi:10.1038/nm.2736
Jadli, A. S., Ballasy, N., Edalat, P., and Patel, V. B. (2020). Inside(sight) of tiny communicator: exosome biogenesis, secretion, and uptake. Mol. Cell Biochem. 467, 77–94. doi:10.1007/s11010-020-03703-z
Jarvinen, L., Badri, L., Wettlaufer, S., Ohtsuka, T., Standiford, T. J., Toews, G. B., et al. (2008). Lung resident mesenchymal stem cells isolated from human lung allografts inhibit T cell proliferation via a soluble mediator. J. Immunol. 181, 4389–4396. doi:10.4049/jimmunol.181.6.4389
Jobe, A. H. (2011). The new bronchopulmonary dysplasia. Curr. Opin. Pediatr. 23, 167–172. doi:10.1097/MOP.0b013e3283423e6b
Kadekar, D., Rangole, S., Kale, V., and Limaye, L. (2016). Conditioned medium from placental mesenchymal stem cells reduces oxidative stress during the cryopreservation of ex vivo expanded umbilical cord blood cells. PLoS One 11, e0165466. doi:10.1371/journal.pone.0165466
Kimble, A., Robbins, M. E., and Perez, M. (2022). Pathogenesis of bronchopulmonary dysplasia: role of oxidative stress from 'omics' studies, Antioxidants (Basel) 11, 2380. doi:10.3390/antiox11122380
Klein, D. (2021). Lung multipotent stem cells of mesenchymal nature: cellular basis, clinical relevance, and implications for stem cell therapy. Antioxid. Redox Signal 35, 204–216. doi:10.1089/ars.2020.8190
Klinger, G., Sokolover, N., Boyko, V., Sirota, L., Lerner-Geva, L., Reichman, B., et al. (2013). Perinatal risk factors for bronchopulmonary dysplasia in a national cohort of very-low-birthweight infants. Am. J. Obstet. Gynecol. 208, 115.e1–115.e9. doi:10.1016/j.ajog.2012.11.026
Komaki, M., Numata, Y., Morioka, C., Honda, I., Tooi, M., Yokoyama, N., et al. (2017). Exosomes of human placenta-derived mesenchymal stem cells stimulate angiogenesis. Stem Cell Res. Ther. 8, 219. doi:10.1186/s13287-017-0660-9
Kwon, H. M., Hur, S. M., Park, K. Y., Kim, C. K., Kim, Y. M., Kim, H. S., et al. (2014). Multiple paracrine factors secreted by mesenchymal stem cells contribute to angiogenesis. Vasc. Pharmacol. 63, 19–28. doi:10.1016/j.vph.2014.06.004
Lai, R. C., Arslan, F., Lee, M. M., Sze, N. S., Choo, A., Chen, T. S., et al. (2010). Exosome secreted by MSC reduces myocardial ischemia/reperfusion injury. Stem Cell Res. 4, 214–222. doi:10.1016/j.scr.2009.12.003
Li, H., Shen, S., Fu, H., Wang, Z., Li, X., Sui, X., et al. (2019). Immunomodulatory functions of mesenchymal stem cells in tissue engineering. Stem Cells Int. 2019, 9671206. doi:10.1155/2019/9671206
Liu, D., Gao, Y., Liu, J., Huang, Y., Yin, J., Feng, Y., et al. (2021). Intercellular mitochondrial transfer as a means of tissue revitalization. Signal Transduct. Target Ther. 6, 65. doi:10.1038/s41392-020-00440-z
Liu, J., Xiao, Q., Xiao, J., Niu, C., Li, Y., Zhang, X., et al. (2022a). Wnt/β-catenin signalling: function, biological mechanisms, and therapeutic opportunities. Signal Transduct. Target Ther. 7, 3. doi:10.1038/s41392-021-00762-6
Liu, Y., Zhang, X., Hu, Y., Kang, M., Wu, Y., Wang, Y., et al. (2022b). Human placental mesenchymal stem cells regulate inflammation via the NF‑κB signaling pathway. Exp. Ther. Med. 24, 654. doi:10.3892/etm.2022.11591
Luan, Y., Ding, W., Ju, Z. Y., Zhang, Z. H., Zhang, X., and Kong, F. (2015). Bone marrow-derived mesenchymal stem cells protect against lung injury in a mouse model of bronchopulmonary dysplasia. Mol. Med. Rep. 11, 1945–1950. doi:10.3892/mmr.2014.2959
Mansouri, N., Willis, G. R., Fernandez-Gonzalez, A., Reis, M., Nassiri, S., Mitsialis, S. A., et al. (2019). Mesenchymal stromal cell exosomes prevent and revert experimental pulmonary fibrosis through modulation of monocyte phenotypes. JCI Insight 4, e128060. doi:10.1172/jci.insight.128060
Mathew, S. A., Naik, C., Cahill, P. A., and Bhonde, R. R. (2020). Placental mesenchymal stromal cells as an alternative tool for therapeutic angiogenesis. Cell Mol. Life Sci. 77, 253–265. doi:10.1007/s00018-019-03268-1
Mcqualter, J. L., Yuen, K., Williams, B., and Bertoncello, I. (2010). Evidence of an epithelial stem/progenitor cell hierarchy in the adult mouse lung. Proc. Natl. Acad. Sci. U. S. A. 107, 1414–1419. doi:10.1073/pnas.0909207107
Mizikova, I., and Thebaud, B. (2023). Perinatal origins of bronchopulmonary dysplasia-deciphering normal and impaired lung development cell by cell. Mol. Cell Pediatr. 10, 4. doi:10.1186/s40348-023-00158-2
Mobius, M. A., Freund, D., Vadivel, A., Koss, S., Mcconaghy, S., Ohls, R. K., et al. (2019). Oxygen disrupts human fetal lung mesenchymal cells. Implications for bronchopulmonary dysplasia. Am. J. Respir. Cell Mol. Biol. 60, 592–600. doi:10.1165/rcmb.2018-0358OC
Moreira, A., Winter, C., Joy, J., Winter, L., Jones, M., Noronha, M., et al. (2020). Intranasal delivery of human umbilical cord Wharton's jelly mesenchymal stromal cells restores lung alveolarization and vascularization in experimental bronchopulmonary dysplasia. Stem Cells Transl. Med. 9, 221–234. doi:10.1002/sctm.18-0273
Morrisey, E. E., and Hogan, B. L. (2010). Preparing for the first breath: genetic and cellular mechanisms in lung development. Dev. Cell 18, 8–23. doi:10.1016/j.devcel.2009.12.010
Nguyen, L. T., Tran, N. T., Than, U. T. T., Nguyen, M. Q., Tran, A. M., Do, P. T. X., et al. (2022). Optimization of human umbilical cord blood-derived mesenchymal stem cell isolation and culture methods in serum- and xeno-free conditions. Stem Cell Res. Ther. 13, 15. doi:10.1186/s13287-021-02694-y
Obendorf, J., Fabian, C., Thome, U. H., and Laube, M. (2020). Paracrine stimulation of perinatal lung functional and structural maturation by mesenchymal stem cells. Stem Cell Res. Ther. 11, 525. doi:10.1186/s13287-020-02028-4
Omar, S. A., Abdul-Hafez, A., Ibrahim, S., Pillai, N., Abdulmageed, M., Thiruvenkataramani, R. P., et al. (2022). Stem-cell therapy for bronchopulmonary dysplasia (BPD) in newborns. Cells 11, 1275. doi:10.3390/cells11081275
Ozkan, H., Cetinkaya, M., and Koksal, N. (2012). Increased incidence of bronchopulmonary dysplasia in preterm infants exposed to preeclampsia. J. Matern. Fetal Neonatal Med. 25, 2681–2685. doi:10.3109/14767058.2012.708371
Paliwal, S., Chaudhuri, R., Agrawal, A., and Mohanty, S. (2018). Regenerative abilities of mesenchymal stem cells through mitochondrial transfer. J. Biomed. Sci. 25, 31. doi:10.1186/s12929-018-0429-1
Pattappa, G., Heywood, H. K., De Bruijn, J. D., and Lee, D. A. (2011). The metabolism of human mesenchymal stem cells during proliferation and differentiation. J. Cell Physiol. 226, 2562–2570. doi:10.1002/jcp.22605
Phinney, D. G., Di Giuseppe, M., Njah, J., Sala, E., Shiva, S., St Croix, C. M., et al. (2015). Mesenchymal stem cells use extracellular vesicles to outsource mitophagy and shuttle microRNAs. Nat. Commun. 6, 8472. doi:10.1038/ncomms9472
Popova, A. P., Bentley, J. K., Cui, T. X., Richardson, M. N., Linn, M. J., Lei, J., et al. (2014). Reduced platelet-derived growth factor receptor expression is a primary feature of human bronchopulmonary dysplasia. Am. J. Physiol. Lung Cell Mol. Physiol. 307, L231–L239. doi:10.1152/ajplung.00342.2013
Porzionato, A., Zaramella, P., Dedja, A., Guidolin, D., Bonadies, L., Macchi, V., et al. (2021). Intratracheal administration of mesenchymal stem cell-derived extracellular vesicles reduces lung injuries in a chronic rat model of bronchopulmonary dysplasia. Am. J. Physiol. Lung Cell Mol. Physiol. 320, L688–L704. doi:10.1152/ajplung.00148.2020
Reicherzer, T., Haffner, S., Shahzad, T., Gronbach, J., Mysliwietz, J., Hubener, C., et al. (2018). Activation of the NF-κB pathway alters the phenotype of MSCs in the tracheal aspirates of preterm infants with severe BPD. Am. J. Physiol. Lung Cell Mol. Physiol. 315, L87–L101. doi:10.1152/ajplung.00505.2017
Reiter, J., Drummond, S., Sammour, I., Huang, J., Florea, V., Dornas, P., et al. (2017). Stromal derived factor-1 mediates the lung regenerative effects of mesenchymal stem cells in a rodent model of bronchopulmonary dysplasia. Respir. Res. 18, 137. doi:10.1186/s12931-017-0620-z
Schmidt, A. R., and Ramamoorthy, C. (2022). Bronchopulmonary dysplasia. Paediatr. Anaesth. 32, 174–180. doi:10.1111/pan.14365
Seok, J., Jun, S., Lee, J. O., and Kim, G. J. (2020). Mitochondrial dynamics in placenta-derived mesenchymal stem cells regulate the invasion activity of trophoblast. Int. J. Mol. Sci. 21, 8599. doi:10.3390/ijms21228599
Sharma, M., Bellio, M. A., Benny, M., Kulandavelu, S., Chen, P., Janjindamai, C., et al. (2022). Mesenchymal stem cell-derived extracellular vesicles prevent experimental bronchopulmonary dysplasia complicated by pulmonary hypertension. Stem Cells Transl. Med. 11, 828–840. doi:10.1093/stcltm/szac041
Shu, W., Guttentag, S., Wang, Z., Andl, T., Ballard, P., Lu, M. M., et al. (2005). Wnt/beta-catenin signaling acts upstream of N-myc, BMP4, and FGF signaling to regulate proximal-distal patterning in the lung. Dev. Biol. 283, 226–239. doi:10.1016/j.ydbio.2005.04.014
Simones, A. A., Beisang, D. J., Panoskaltsis-Mortari, A., and Roberts, K. D. (2018). Mesenchymal stem cells in the pathogenesis and treatment of bronchopulmonary dysplasia: a clinical review. Pediatr. Res. 83, 308–317. doi:10.1038/pr.2017.237
Sucre, J., Haist, L., Bolton, C. E., and Hilgendorff, A. (2021). Early changes and indicators characterizing lung aging in neonatal chronic lung disease. Front. Med. (Lausanne) 8, 665152. doi:10.3389/fmed.2021.665152
Thebaud, B., Lalu, M., Renesme, L., Van Katwyk, S., Presseau, J., Thavorn, K., et al. (2021). Benefits and obstacles to cell therapy in neonates: the INCuBAToR (innovative neonatal cellular therapy for bronchopulmonary dysplasia: accelerating translation of research). Stem Cells Transl. Med. 10, 968–975. doi:10.1002/sctm.20-0508
Torchin, H., Ancel, P. Y., Goffinet, F., Hascoet, J. M., Truffert, P., Tran, D., et al. (2016). Placental complications and bronchopulmonary dysplasia: EPIPAGE-2 cohort study. Pediatrics 137, e20152163. doi:10.1542/peds.2015-2163
Varderidou-Minasian, S., and Lorenowicz, M. J. (2020). Mesenchymal stromal/stem cell-derived extracellular vesicles in tissue repair: challenges and opportunities. Theranostics 10, 5979–5997. doi:10.7150/thno.40122
Varkouhi, A. K., Jerkic, M., Ormesher, L., Gagnon, S., Goyal, S., Rabani, R., et al. (2019). Extracellular vesicles from interferon-gamma-primed human umbilical cord mesenchymal stromal cells reduce Escherichia coli-induced acute lung injury in rats. Anesthesiology 130, 778–790. doi:10.1097/ALN.0000000000002655
Vasandan, A. B., Jahnavi, S., Shashank, C., Prasad, P., Kumar, A., and Prasanna, S. J. (2016). Human Mesenchymal stem cells program macrophage plasticity by altering their metabolic status via a PGE(2)-dependent mechanism. Sci. Rep. 6, 38308. doi:10.1038/srep38308
Volckaert, T., Campbell, A., Dill, E., Li, C., Minoo, P., and De Langhe, S. (2013). Localized Fgf10 expression is not required for lung branching morphogenesis but prevents differentiation of epithelial progenitors. Development 140, 3731–3742. doi:10.1242/dev.096560
Vom Hove, M., Prenzel, F., Uhlig, H. H., and Robel-Tillig, E. (2014). Pulmonary outcome in former preterm, very low birth weight children with bronchopulmonary dysplasia: a case-control follow-up at school age. J. Pediatr. 164, 40–45. doi:10.1016/j.jpeds.2013.07.045
White, A. C., Lavine, K. J., and Ornitz, D. M. (2007). FGF9 and SHH regulate mesenchymal Vegfa expression and development of the pulmonary capillary network. Development 134, 3743–3752. doi:10.1242/dev.004879
Willis, G. R., Fernandez-Gonzalez, A., Anastas, J., Vitali, S. H., Liu, X., Ericsson, M., et al. (2018). Mesenchymal stromal cell exosomes ameliorate experimental bronchopulmonary dysplasia and restore lung function through macrophage immunomodulation. Am. J. Respir. Crit. Care Med. 197, 104–116. doi:10.1164/rccm.201705-0925OC
Wu, Q., Fang, T., Lang, H., Chen, M., Shi, P., Pang, X., et al. (2017). Comparison of the proliferation, migration and angiogenic properties of human amniotic epithelial and mesenchymal stem cells and their effects on endothelial cells. Int. J. Mol. Med. 39, 918–926. doi:10.3892/ijmm.2017.2897
Wu, X., Xia, Y., Zhou, O., Song, Y., Zhang, X., Tian, D., et al. (2020). Allogeneic human umbilical cord-derived mesenchymal stem cells for severe bronchopulmonary dysplasia in children: study protocol for a randomized controlled trial (MSC-BPD trial). Trials 21, 125. doi:10.1186/s13063-019-3935-x
Xi, Y., Ju, R., and Wang, Y. (2022). Mesenchymal stem cell-derived extracellular vesicles for the treatment of bronchopulmonary dysplasia. Front. Pediatr. 10, 852034. doi:10.3389/fped.2022.852034
Xiong, J., Ai, Q., Bao, L., Gan, Y., Dai, X., Han, M., et al. (2023). Dose-dependent effects of human umbilical cord-derived mesenchymal stem cell treatment in hyperoxia-induced lung injury of neonatal rats. Front. Pediatr. 11, 1111829. doi:10.3389/fped.2023.1111829
Xuefei, Y., Xinyi, Z., Qing, C., Dan, Z., Ziyun, L., Hejuan, Z., et al. (2021). Effects of hyperoxia on mitochondrial homeostasis: are mitochondria the hub for bronchopulmonary dysplasia? Front. Cell Dev. Biol. 9, 642717. doi:10.3389/fcell.2021.642717
Yilmaz, A., Aslan, M. T., Ince, Z., and Vural, M. (2021). Mesenchymal stem cell therapy in a preterm infant with bronchopulmonary dysplasia. Indian J. Pediatr. 88, 1262. doi:10.1007/s12098-021-03946-8
You, J., Zhou, O., Liu, J., Zou, W., Zhang, L., Tian, D., et al. (2020). Human umbilical cord mesenchymal stem cell-derived small extracellular vesicles alleviate lung injury in rat model of bronchopulmonary dysplasia by affecting cell survival and angiogenesis. Stem Cells Dev. 29, 1520–1532. doi:10.1089/scd.2020.0156
Zhang, K., Yao, E., Chuang, E., Chen, B., Chuang, E. Y., and Chuang, P. T. (2022a). mTORC1 signaling facilitates differential stem cell differentiation to shape the developing murine lung and is associated with mitochondrial capacity. Nat. Commun. 13, 7252. doi:10.1038/s41467-022-34763-y
Zhang, X., Xu, J., Wang, J., Gortner, L., Zhang, S., Wei, X., et al. (2013). Reduction of microRNA-206 contributes to the development of bronchopulmonary dysplasia through up-regulation of fibronectin 1. PLoS One 8, e74750. doi:10.1371/journal.pone.0074750
Zhang, Y., Zhong, Y., Zou, L., and Liu, X. (2022b). Significance of placental mesenchymal stem cell in placenta development and implications for preeclampsia. Front. Pharmacol. 13, 896531. doi:10.3389/fphar.2022.896531
Keywords: stem cells, bronchopulmonary dysplasia, development, extracellular vesicles, mitochondria
Citation: Zhang S, Mulder C, Riddle S, Song R and Yue D (2023) Mesenchymal stromal/stem cells and bronchopulmonary dysplasia. Front. Cell Dev. Biol. 11:1247339. doi: 10.3389/fcell.2023.1247339
Received: 25 June 2023; Accepted: 17 October 2023;
Published: 30 October 2023.
Edited by:
Francesca Forini, National Research Council (CNR), ItalyReviewed by:
Mudasir Bashir Gugjoo, Sher-e-Kashmir University of Agricultural Sciences and Technology of Kashmir, IndiaCopyright © 2023 Zhang, Mulder, Riddle, Song and Yue. This is an open-access article distributed under the terms of the Creative Commons Attribution License (CC BY). The use, distribution or reproduction in other forums is permitted, provided the original author(s) and the copyright owner(s) are credited and that the original publication in this journal is cited, in accordance with accepted academic practice. No use, distribution or reproduction is permitted which does not comply with these terms.
*Correspondence: Dongmei Yue, eXVlZG1Ac2otaG9zcGl0YWwub3Jn
Disclaimer: All claims expressed in this article are solely those of the authors and do not necessarily represent those of their affiliated organizations, or those of the publisher, the editors and the reviewers. Any product that may be evaluated in this article or claim that may be made by its manufacturer is not guaranteed or endorsed by the publisher.
Research integrity at Frontiers
Learn more about the work of our research integrity team to safeguard the quality of each article we publish.