- 1French Armed-forces Biomedical Research Institute (IRBA), Clamart, France
- 2UMR-MD-U1197, Inserm, Villejuif, France
Despite several advances in the field of regenerative medicine, clinical management of extensive skin wounds or burns remains a major therapeutic issue. During the past few years, Mesenchymal Stromal Cells (MSCs) have emerged as a novel therapeutic tool to promote tissue repair through their anti-inflammatory, pro-trophic and pro-remodeling effects. They exert their biological activity mainly via the secretion of soluble bioactive molecules such as cytokines, growth factors, proteins and microRNAs which can be encapsulated within extracellular vesicles (EV). The recent discovery of their high plasticity to external stimuli has fostered the development of new targeted therapies known as priming strategies, to enhance their potential. Our team recently showed that Interleukin-1β (IL-1β)-primed gingival MSCs promote wound healing and epidermal engraftment in vitro, and in vivo through their secreted products that contain extracellular vesicles. In the present work, we investigated whether two common sources of MSCs, gingiva and bone marrow, could respond similarly to IL-1β to favor pro-healing capabilities of their secretome. We showed that both primed-MSC sources, or their related secreted products, are able to reduce inflammation in LPS-challenged human monocytic THP-1 cell line. IL-1β priming enhanced MSC secretion of wound healing-related growth factors, cytokines and miRNAs in both sources. Among them, interleukin 6 was shown to be involved in the anti-inflammatory effect of MSC secreted products. Overall, these results underline the pro-healing properties of both MSC sources and their secretome upon IL-1β priming and their potential to improve the current medical treatment of severe wounds.
1 Introduction
Restoration of the integrity and function of wounded skin is finely orchestrated in inflammation, proliferation and remodeling phases involving specialized cell types, growth factors and cytokines (Gurtner et al., 2008). For example, macrophages play key roles in the inflammatory-proliferative phase transition, but these versatile cells also assist in all other stages of wound healing, to promote successful repair (Koh and DiPietro, 2011). Abnormal macrophage function and excessive inflammation, seen in chronic diseases or important acute traumas, may result in delayed healing or excessive scarring (Landen et al., 2016; Xu et al., 2020).
In recent years, many studies have highlighted the interesting repair properties of mesenchymal stromal cells (MSC) (Cerqueira et al., 2016; Nourian Dehkordi et al., 2019). Preclinical studies have shown that MSCs influence distinct phases of wound healing, including macrophage polarization, acceleration of reepithelialization and matrix remodeling (Jackson et al., 2012; Chen et al., 2016). Early clinical data have also reported their safety and efficacy for wound repair (Bey et al., 2010; Huang et al., 2020). Eventually, numerous preclinical studies have emphasized the growing interest of their secretory products, especially their extracellular vesicles (EV), for skin repair, which paved the way for future acellular therapies (Chen et al., 2008; Li et al., 2016; Kucharzewski et al., 2019).
Since their discovery in the bone marrow, MSCs have been isolated from numerous tissues such as perinatal tissues, dental pulp or adipose tissues. However, MSC phenotype, biological characteristics, and secretory activities differ according to their tissue sources (Macrin et al., 2017). Indeed, it has been shown that gingiva-derived MSCs (G-MSCs) possess better proliferation and migration capacity than adipose-derived MSCs (Boink et al., 2015), and that bone marrow-derived MSCs (BM-MSCs) display a higher immunomodulatory activity compared to adipose or Wharton’s jelly-derived MSCs (Petrenko et al., 2020). There is currently no source commonly accepted for wound healing therapy. G-MSCs and BM-MSCs are two sources with interesting wound healing-related properties. On the one hand, G-MSCs have been shown to enhance wound healing through anti-inflammatory and pro-remodeling properties in a burn irradiation model (Linard et al., 2015). On the other hand, BM-MSCs have been shown to increase re-epithelialization and thickness of the regenerated epidermis (Fu et al., 2006), and to accelerate wound closure through induction of macrophage polarization toward a pro-healing phenotype (Alapure et al., 2018). Moreover, both sources are known to produce EVs and growth factors with potent repair activities (Pires et al., 2016; Yamada et al., 2019; Magne et al., 2020; Nakao et al., 2021; Lorenzini et al., 2023).
MSCs are also known for their ability to sense their environment and adapt accordingly to molecular, cellular, and physical environments (Kusuma et al., 2017). This property has led to the use of priming cues as stimuli to enhance MSC activity (Madrigal et al., 2014). Upon injury, resident and remote cells are both recruited to the wound area and activated by inflammatory cytokines. Therefore, interferon gamma (IFN-γ), tumor necrosis factor alpha (TNF-α) and interleukin 1 beta (IL-1β) have been widely used to prime MSCs to a wounding environment (Noronha et al., 2019). In our previous work, we showed that IL-1β-primed G-MSCs accelerate wound healing through the modification of their secretory profile (Magne et al., 2020). Several studies indicate that pure EVs are not sufficient to recapitulate MSC properties (Mitchell et al., 2019; Papait et al., 2022; Wolf et al., 2022). Soluble molecules can non-covalently bind around EVs forming a corona which itself can participate in the biological effects of MSC-secreted products (Wolf et al., 2022). However, the impact of an inflammatory priming on the secretome of different MSC sources and their efficacy to treat skin wound healing has not been explored.
Here, we aimed to compare the impact of IL-1β priming on the anti-inflammatory properties of two different MSC sources and their secretomes. Our results demonstrated that both sources can decrease inflammation. We also found that IL-1β priming modify the secretome (proteins and miRNAs) of both cell types. In particular, interleukin 6 (IL-6) is significantly highly secreted upon priming and plays a substantial part in the reduction of inflammation induced by MSC secretions.
2 Materials and methods
2.1 Cell isolation, culture and characterization
This study was conducted in accordance with ethical principles stated in the declaration of Helsinki. All the human cells were isolated from surgical residues collected from healthy subjects (surgical stomatology for G-MSC, hip replacement surgery for BM-MSC). An informed, written consent was obtained from donors. According to French law, a declaration but no ethical committee approval was required for using these samples.
G- and BM-MSC were harvested as described previously (Doucet et al., 2005; Magne et al., 2020) and were cultivated in MEMα (Sartorius) culture medium supplemented with 5% human platelet lysate [French Armed-forces Blood Transfusion Center (Doucet et al., 2005)], 2 IU/mL heparin (Sanofi) and 100 IU/mL penicillin (Panpharma), 50 μg/mL gentamicin (Panpharma), and 1 μg/mL amphotericin B (Cheplapharm Arzneimittel GmbH) or 10 μg/mL Ciprofloxacin (Bayer) for G- or BM-MSC respectively, at 37°C in a humid atmosphere under 5% CO2. G- and BM-MSCs were characterized by flow cytometry and differentiation assays as recommended by ISCT guidelines (Doucet et al., 2005; Dominici et al., 2006; Magne et al., 2020).
The human monocytic THP-1 cell line (ATCC) was cultured in RPMI (Gibco) medium supplemented with 10% heat-inactivated fetal bovine serum (Cytiva), 50 µM β-mercapto-ethanol (Sigma) and penicillin-streptomycin (100 U/mL and 100 μg/mL respectively, Gibco).
2.2 MSC priming and conditioned medium preparation
Following a previously-described method (Magne et al., 2020), 7 individual cell populations of either G-MSCs or BM-MSCs were grown at passage 4 until 60% confluence and primed for 24 h with 1 ng/mL human recombinant IL-1β (Peprotech) (MSCIL) or no treatment (MSCNV). All seven populations of either G-MSCs or BM-MSCs were grown and primed separately, but were used together in functional assays after pooling them in equal cell quantities. For conditioned media (CM) preparation, all seven populations of G-MSCs or BM-MSCs were grown and primed separately, washed three times in phosphate-buffered saline (PBS, Thermo fisher) and incubated in human platelet lysate- and antibiotic-free medium for 48 h. Conditioned media from G-MSCs (G-CM) and BM-MSCs (BM-CM), either untreated (CMNV) or after priming (CMIL) were separately collected and concentrated 40 times using Amicon ultra centrifugal filter units with 3-kDa cutoff (Millipore). In functional assays, all individual CMNV and CMIL for both G-MSCs and BM-MSCs were pooled together in equal volumes. Pooled CM total protein amount was determined using the Bio-Rad Protein Assay kit. The contents of each individual CM were analyzed using enzyme-linked immunosorbant assays (ELISAs) according to manufacturer’s instructions (Biotechne) and real time quantitative polymerase chain reaction (RT-qPCR, Section 2.4).
2.3 Inflammation assay
Human monocytic THP-1 cells were seeded in 24-well plates at 170,000 cells/mL, exposed to 1 μg/mL Lipopolysaccharide (LPS; Escherichia coli O55:B5, L6529; Sigma), and cultured with either pooled MSCNV or MSCIL at a 1:10 MSC-to-THP-1 ratio, pooled CMNV or CMIL at 10 μg/mL of total proteins, or human recombinant IL-6 (Biotechne) at 695 pg/mL (corresponds to the concentration measured in BM-CMIL). In some experiments, the THP-1 cells were also treated either with an IL-6 receptor blocking antibody (Tocilizumab, Selleckchem) or its IgG1 isotypic control (Biotechne) at 100 μg/mL. THP-1 supernatants were collected after 24 h and assayed for TNF-α and interleukin 1 receptor antagonist (IL-1RA) levels by ELISAs (Biotechne).
2.4 RT-qPCR
A volume of 100 µL of individual CM was mixed first with 300 µL Trizol LS Reagent (Sigma) and then with 80 µL chloroform (Sigma) according to the manufacturer’s protocol (Sigma). Next, aqueous phases were mixed with 200 µL isopropanol (Sigma) and 1 µL of glycoblue (Ambion AM9515). After 10 min of incubation, the preparations were centrifuged at 12,000 g for 10 min. The pellets were washed with 75% ethanol (Sigma), air-dried, and resuspended in 20 µL of RNAse free water (Qiagen). The samples were incubated at 55°C during 15 min and frozen for future experiments.
Total RNA (10 ng per sample) was transcribed into cDNA using miRCURY LNA RT Kit for miRNA (Qiagen). RT-qPCRs were carried out using miRCURY LNA miRNA PCR Assays according to manufacturer’s protocol (Qiagen) with LightCycler 480 II (Roche Diagnostics), and analyzed with the LightCycler software (Roche Diagnostics). The expressions of the target miRNAs were normalized with the geometric mean of 4 reference miRNAs (miR-191-5p, miR-23a-3p, miR-100-5p, miR-199a-3p) selected using GeNorm software V3.5 (Vandesompele et al., 2002). Final quantification is expressed as arbitrary units (AU) and consists of the geometrical mean of the relative quantification (2−ΔΔCT) performed with each reference miRNA.
2.5 Statistics
All statistical analyses were conducted on R software (v. 4.0.2). Wilcoxon or Mann-Whitney tests were performed to compared two conditions. For the variables having more than 2 modalities, we performed Kruskal–Wallis test and Wilcoxon post hoc test with p values adjusted by the FDR method. Significance level was set to p < 0.05. All charts were plotted as mean ± standard error of the mean on GraphPad Prism 6 software.
3 Results
3.1 Both MSC sources have comparable anti-inflammatory effects in vitro
We investigated the immunomodulation response of BM-MSCs and G-MSCs after IL-1β-priming, using an in vitro inflammation assay. First, MSCNV and MSCIL from bone marrow and gingiva both reduced the inflammatory response of LPS-challenged human monocytic THP-1 cell line as demonstrated by a strong decrease in TNF-α and increase in IL-1RA levels in supernatants (p < 0.001, Figure 1A). MSCIL tended to be superior to MSCNV in decreasing TNF-α levels in THP-1 supernatants, for the gingiva origin (p = 0.105, Figure 1A) compared to bone marrow (p = 0.357, Figure 1A). We next investigated whether similar effects would be observed using concentrated CM of MSCs from both sources. CM concentration was performed to increase the concentration of the soluble factors and EVs in CM (Magne et al., 2020). We showed that CMIL were more efficient to decrease TNF-α and increase IL-1RA compared to CMNV in both sources in LPS-challenged THP-1 supernatants (p < 0.001, Figure 1B). We also noted that BM-CMIL induced a higher increase of IL-1RA compared to G-CMIL (p < 0.05, Figure 1B). Thus, these results indicate a similar anti-inflammatory response for both MSC sources and their respective secretome upon IL-1β priming.
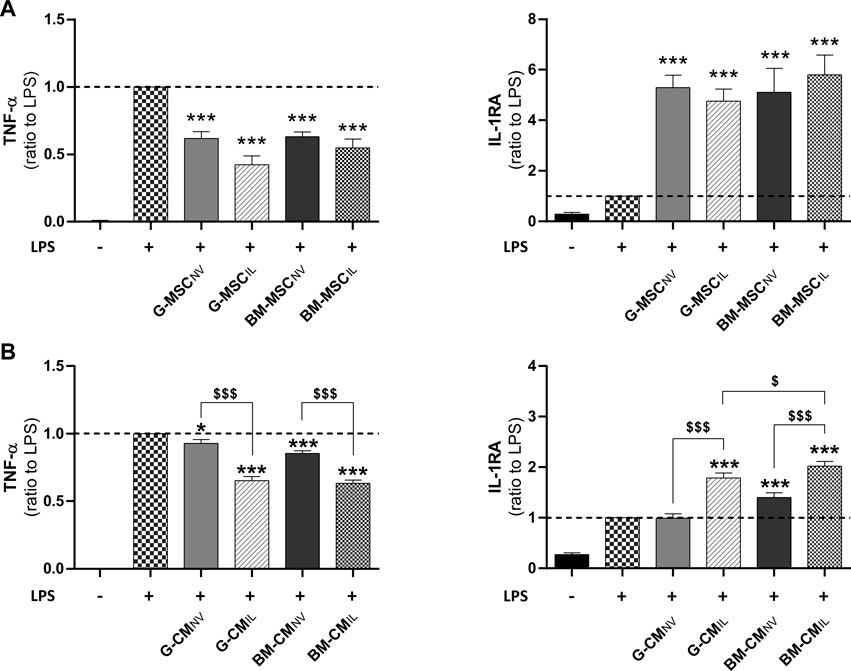
FIGURE 1. Bone marrow and gingiva-derived MSCs display comparable anti-inflammatory effects in vitro. (A) Dosage of TNF-α and IL-1RA in the supernatants of LPS-challenged THP-1 cells co-cultured for 24 h with BM or G-MSCNV or MSCIL (ratio to LPS, n = 5–10). (B) Dosage of TNF-α and IL-1RA in the supernatants of LPS-challenged THP-1 cells cultured with BM or G-CMNV or CMIL at 10 μg/mL of total proteins for 24 h (ratio to LPS, n = 9–19). Values are expressed as mean ± SEM. *p < 0.05, ***p < 0.001 (comparison with LPS alone condition); $ p < 0.05, $$$ p < 0.001 (comparison between indicated conditions); SEM, standard error of the mean; MSCNV, naive mesenchymal stromal cells; MSCIL, IL-1β-primed MSC; BM, bone marrow, G, gingiva; CM, conditioned medium; IL-1RA, IL-1 receptor antagonist; LPS, lipopolysaccharide; TNF-α, tumor necrosis factor-α.
3.2 IL-1β priming impacts MSC secretome of both sources
As MSC efficiency relies on release of secretory products (Nourian Dehkordi et al., 2019), we next sought to better understand their mechanisms of action. Therefore, we quantified by ELISA a selection of wound healing-related proteins known to be key paracrine actors of the MSC effects in our CM, to determine if both sources respond similarly to IL-1β. We found that IGFBP-7, STC-1, FGF-7, and IL-6 were significantly upregulated in CMIL compared to CMNV in both sources (p < 0.05, Figure 2A). The IL-1β priming significantly increased TGF-β1, VEGF and FGF-2 secretion (p < 0.05, Figure 2A) only in the G-CM whereas QSOX1 secretion was significantly increased only in the BM-CM (p < 0.05, Figure 2A). Priming induced different HGF secretion responses in both sources, with an increase in HGF expression in the G-CMIL and a decrease in HGF levels in the BM-CMIL (p < 0.05, Figure 2A). Molecules involved in extracellular matrix remodeling were also affected by the priming in both sources, with a significant increase in matrix metallopeptidase 1 (MMP-1) and MMP-9 secretion (p < 0.05, Figure 2A). However, IL-1β priming had no impact on tissue inhibitor metallopeptidase 1 (TIMP-1) secretion (Figure 2A). When comparing CMIL from both sources, our results indicated that BM-CM contained a significantly higher concentration of QSOX1 and FGF-2 compared to G-CM (p < 0.05, Figure 2A). We also studied the secretion of a selection of miRNAs specifically related to wound healing functions (Fang et al., 2016; Hu et al., 2018). Our results indicated a differential regulation of macrophage polarization-related miRNAs (Li H. et al., 2018) between both MSC sources after IL-1β priming. Concerning M2-polarizing miRNAs, we showed an upregulation of miR-146a-5p in CMIL compared to CMNV, in both sources (p < 0.05, Figure 2B). The secretion of miR-24-3p and miR-210a-3p were only upregulated in CMIL for BM source (p < 0.05, Figure 2B). Concerning M1-related miRNA, we found a decrease of miR-199a-3p in CMIL compared to CMNV in both sources (p < 0.05, Figure 2B). MiR-21-5p was decreased only in G-CMIL whereas miR-125b-5p secretion was decreased only in BM-CMIL (p < 0.05, Figure 2B). When comparing CMIL from both sources, our results indicated that G-CM contained a significantly higher concentration of miR-146a-5p (p < 0.001, Figure 2B) whereas BM-CM contained a significantly higher concentration of miR-24-3p (p < 0.01), miR-210-3p (p < 0.01), and miR-21-5p (p < 0.001, Figure 2B). Thus, these results indicate slight differences between the two secretory profiles among both sources with higher secretion of wound healing-related molecules in BM-MSCIL.
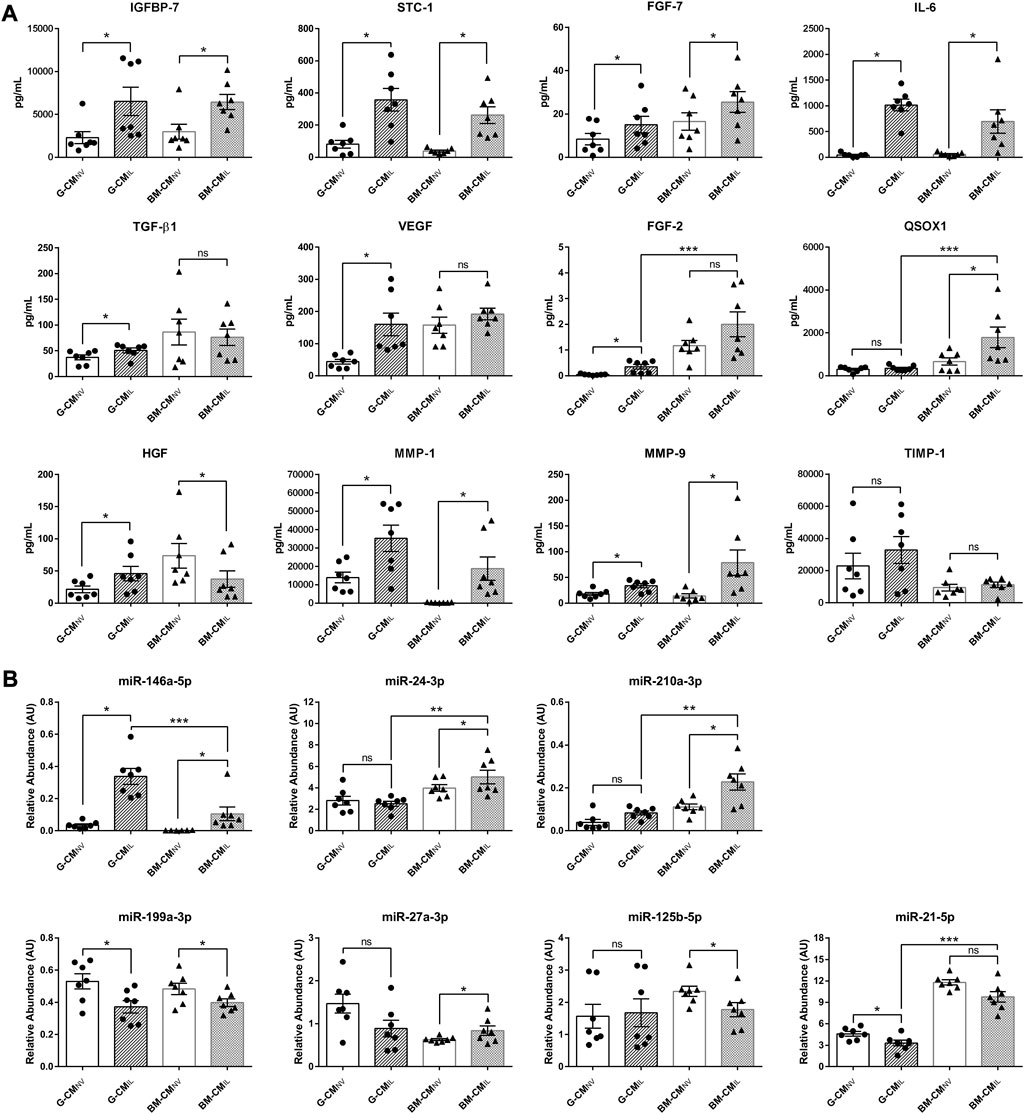
FIGURE 2. IL-1β priming remodel the secretome of bone marrow and gingiva-derived MSCs. (A) ELISA dosages of selected wound healing-related proteins in CMNV or CMIL of both sources G or BM used at 10 μg/mL of total proteins (n = 7 MSC donors). (B) Relative abundance of selected miRNA in CMNV or CMIL of both sources of MSCs normalized with four internal reference miRNA (n = 7 MSC donors). Values are expressed as mean ± SEM. *p < 0.05; **p < 0.01; ***p < 0.001; ns, non-significant; CM, conditioned medium; G, gingival; BM, bone marrow; NV, naive; IL, primed with IL-1β; AU, arbitrary units; IGFBP-7, insulin growth factor protein 7; STC-1, stanniocalcin-1; FGF-7, fibroblast growth factor-7; IL-6, interleukin-6; TGF-β1; transforming growth factor β1; VEGF, vascular endothelial growth factor; FGF-2, fibroblast growth factor 2; QSOX1, quiescin sulfhydryl oxidase 1; HGF, hepatocyte growth factor; TIMP-1, tissue inhibitor of metalloproteinases 1; MMP-1, matrix metalloproteinase 1; MMP-9, matrix metalloproteinase 9.
3.3 IL-6 plays key roles in the anti-inflammatory effect of CM from both MSC sources
In our secretome analysis (Figure 2), IL-6 appears to be one of the factors whose concentration is most increased during IL-1β priming. According to the literature, IL-6 plays important roles in wound healing (McFarland-Mancini et al., 2010). We thus investigated whether IL-6 could be responsible for the anti-inflammatory effect elicited by the CMIL in our inflammation assay. Similarly to BM-CMIL, recombinant human IL-6 (rhIL-6), used alone at the concentration measured in BM-CMIL, significantly decreased TNF-α levels in LPS-challenged THP-1 cell cultures compared to LPS (p < 0.001, Figure 3) and increased IL-1RA (p < 0.001, Figure 3). Similar results were obtained when using rhIL-6 alone at the concentration measured in G-CMIL (data not shown). The use of IL-6 receptor blocking antibody partly counteracted the effect of rhIL-6, BM-CMIL and BM-CMNV on TNF-α secretion (p = 0.069 for rhIL-6, p < 0.01 for BM-CMIL and BM-CMNV, Figure 3) and also on IL-1 RA secretion (p < 0.05 for rhIL-6, p < 0.001 for BM-CMIL and BM-CMNV, Figure 3). Taken together, these results suggest that BM-CMIL promote reduction of inflammation in part through IL-6 pathway.
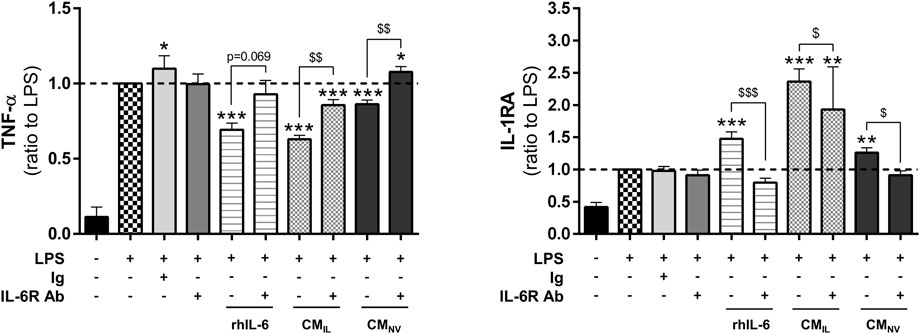
FIGURE 3. IL-6 plays a key role in the anti-inflammatory effect of CM from bone marrow and gingiva-derived MSCs. Dosage of TNF-α and IL-1RA in the supernatants of LPS-challenged THP-1 cells with rhIL-6 at the concentration found in BM-CMIL, BM-CMIL or BM-CMNV with or without IL-6 antibody or control antibody (ratio to LPS, n = 5–14) for 24 h. Values are expressed as mean ± SEM. *p < 0.05; **p < 0,01; ***p < 0.001 (comparison with LPS alone condition); $ p < 0.05; $$ p < 0,01; $$$ p < 0.001 (comparison between indicated conditions); SEM, standard error of the mean; IL-1RA, IL-1 receptor antagonist; LPS, lipopolysaccharide; TNF-α, tumor necrosis factor-α; CMNV, conditioned medium from naive MSC; CMIL conditioned medium from IL-1β-primed MSC; IL-6R Ab, IL-6 receptor antibody; Ig, control antibody; rhIL-6, recombinant IL-6.
4 Discussion
MSCs hold great potential in cell therapy for skin wound healing due to their immunomodulatory properties, their pleiotropic activities to support other cell types and their capacity to respond to their environment. In this study, we tested whether MSCs from different tissue sources (including bone marrow and gingiva) can display similar anti-inflammatory potency and respond comparably to IL-1β priming. Our results indicate that both sources are comparable after IL-1β priming in our in vitro model of inflammation (Figures 1A, B). These findings correlate with previous in vitro studies showing no difference between both sources for their immunomodulatory ability in a T lymphocyte proliferation test (Li J. et al., 2018), or with in vivo study indicating similar effects of BM and G-MSCs to reduce necrosis in the stasis zone around the burn in a rat model (Abbas et al., 2019).
We also showed that IL-1β priming improves the anti-inflammatory effect of MSC secretome from both sources (Figure 1B and data not shown). This beneficial effect correlates with other studies evidencing that IL-1β-primed MSC-derived secretory products ameliorate sepsis through macrophage M2 polarization (Song et al., 2017; Yao et al., 2021) and favor wound healing of full-thickness excisional skin wounds (Park et al., 2018). Our study, along with others (Maffioli et al., 2017; Redondo-Castro et al., 2018), evidence that inflammatory priming leads to major secretome changes in terms of growth factors, interleukins, inflammatory mediators and extracellular matrix remodeling components. Moreover, studies comparing different inflammatory primings (Burja et al., 2020; Wedzinska et al., 2021), indicated that IL-1β most profoundly increases the expression of cytokines and chemokines with anti-inflammatory properties. Our results indicated that basal level in CMNV of some factors (VEGF, HGF, MMP-1) were significantly different between both sources but became similar in CMIL. While some factors were differentially secreted upon IL-1β priming between the two sources, anti-inflammatory factors such as IGFBP-7 or IL-6 were strongly secreted in both sources (Figure 2A). As shown in several studies (Peltzer et al., 2020; Giunti et al., 2021), inflammatory priming impacts also the composition of EV cargoes such as miRNAs. Several mi-RNAs as miR-199a-3p, miR-125b-5p, miR-21-5p found in our study are also reported in miRNAs landscape of MSC-Extracellular Vesicles described by Ferguson (Ferguson et al., 2018), comforting that miRNA found in our secretome may be carried out by EVs. Several studies reported that miR-146a-5p, which is also upregulated after IL-1β priming, is capable to decrease inflammation and fibrosis formation (Song et al., 2017; Liang et al., 2019; Wu et al., 2019). Our study showed variations in the factors and miRNAs evaluated between both sources. For example, some factors with favorable ant-inflammatory activity reported in literature such as miR-146a-5p or FGF-2, QSOX-1 and miR-24-3p were found in higher amount in G-CMIL or BM-CMIL respectively. However, CMIL from both sources displayed anti-inflammatory activity (Figure 1B) suggesting a balance provided by the amounts of the different factors. As other investigators, we showed that IL-1β induces the secretion of IL-6 in both MSC tissue sources (Burja et al., 2020). In our study, we demonstrated with a blocking antibody that IL-6 plays a key role in the anti-inflammatory properties of the CM (Figure 3). These results correlate with different studies shedding light on the potential role of IL-6 in wound healing. Heo et al. (2011) showed that a conditioned medium from TNF-α-primed MSCs stimulates cutaneous wound healing through IL-6- and IL-8-dependent mechanisms. Another team provided evidence that BM-MSC inflammatory preconditioning highly favors their potential to promote IL-6-dependent M2b polarization (Philipp et al., 2018). A cyto-protective effect of MSC-EV on liver failure has also been evidenced involving the IL-6/STAT3 pathway (Tan et al., 2014). Thus, the reported effects of CMIL in our study could be attributable to the presence of proteins and EVs carrying growth factors, cytokines, and miRNAs.
In conclusion, in this article, both sources of primed-MSCs exhibit similar pro-healing properties. The priming strategy represents an interesting option to optimize the properties of MSC secretory products and possibly extracellular vesicles that could be used as a promising skin wound healing therapy.
Data availability statement
The original contributions presented in the study are included in the article/supplementary material, further inquiries can be directed to the corresponding author.
Author contributions
Conceptualization: MD, BM, and MT; data curation: MD and MT; formal analysis: MD, BM, and MT; funding acquisition: MT and SB; investigation: MD, BM, MN, and MT; methodology: MD, BM, and MT; project administration: MT; supervision: SB; validation: MT; visualization: MD and MT; writing—original draft preparation: MD, BM, and MT; writing—review and editing: MT. All authors contributed to the article and approved the submitted version.
Funding
This work was supported by grants SAN-1-0225 from DGA (Direction Générale de l’Armement). The PhD of the first two authors was supported by “Agence Innovation Defense”.
Acknowledgments
We acknowledge the Agence Nationale pour la Recherche for support of the national infrastructure: “ECELLFRANCE: Development of a national adult mesenchymal stem cell based therapy platform” (PIA/ANR-11-INSB-005).
Conflict of interest
The authors declare that the research was conducted in the absence of any commercial or financial relationships that could be construed as a potential conflict of interest.
Publisher’s note
All claims expressed in this article are solely those of the authors and do not necessarily represent those of their affiliated organizations, or those of the publisher, the editors and the reviewers. Any product that may be evaluated in this article, or claim that may be made by its manufacturer, is not guaranteed or endorsed by the publisher.
References
Abbas, O. L., Ozatik, O., Gonen, Z. B., Ogut, S., Ozatik, F. Y., Salkin, H., et al. (2019). Comparative analysis of mesenchymal stem cells from bone marrow, adipose tissue, and dental pulp as sources of cell therapy for zone of stasis burns. J. Invest. Surg. 32 (6), 477–490. doi:10.1080/08941939.2018.1433254
Alapure, B. V., Lu, Y., He, M., Chu, C. C., Peng, H., Muhale, F., et al. (2018). Accelerate healing of severe burn wounds by mouse bone marrow mesenchymal stem cell-seeded biodegradable hydrogel scaffold synthesized from arginine-based poly(ester amide) and chitosan. Stem Cells Dev. 27 (23), 1605–1620. doi:10.1089/scd.2018.0106
Bey, E., Prat, M., Duhamel, P., Benderitter, M., Brachet, M., Trompier, F., et al. (2010). Emerging therapy for improving wound repair of severe radiation burns using local bone marrow-derived stem cell administrations. Wound Repair Regen. 18 (1), 50–58. doi:10.1111/j.1524-475X.2009.00562.x
Boink, M. A., van den Broek, L. J., Roffel, S., Nazmi, K., Bolscher, J. G. M., Gefen, A., et al. (2015). Different wound healing properties of dermis, adipose, and gingiva mesenchymal stromal cells. Wound Repair Regen. 24, 100–109. doi:10.1111/wrr.12380
Burja, B., Barlic, A., Erman, A., Mrak-Poljsak, K., Tomsic, M., Sodin-Semrl, S., et al. (2020). Human mesenchymal stromal cells from different tissues exhibit unique responses to different inflammatory stimuli. Curr. Res. Transl. Med. 68 (4), 217–224. doi:10.1016/j.retram.2020.05.006
Cerqueira, M. T., Pirraco, R. P., and Marques, A. P. (2016). Stem cells in skin wound healing: are we there yet? Adv. Wound Care (New Rochelle) 5 (4), 164–175. doi:10.1089/wound.2014.0607
Chen, D., Hao, H., Fu, X., and Han, W. (2016). Insight into reepithelialization: how do mesenchymal stem cells perform? Stem Cells Int. 2016, 6120173. doi:10.1155/2016/6120173
Chen, L., Tredget, E. E., Wu, P. Y., and Wu, Y. (2008). Paracrine factors of mesenchymal stem cells recruit macrophages and endothelial lineage cells and enhance wound healing. PLoS One 3 (4), e1886. doi:10.1371/journal.pone.0001886
Dominici, M., Le Blanc, K., Mueller, I., Slaper-Cortenbach, I., Marini, F., Krause, D., et al. (2006). Minimal criteria for defining multipotent mesenchymal stromal cells. The International Society for Cellular Therapy position statement. Cytotherapy 8 (4), 315–317. doi:10.1080/14653240600855905
Doucet, C., Ernou, I., Zhang, Y., Llense, J. R., Begot, L., Holy, X., et al. (2005). Platelet lysates promote mesenchymal stem cell expansion: A safety substitute for animal serum in cell-based therapy applications. J. Cell Physiol. 205 (2), 228–236. doi:10.1002/jcp.20391
Fang, S., Xu, C., Zhang, Y., Xue, C., Yang, C., Bi, H., et al. (2016). Umbilical cord-derived mesenchymal stem cell-derived exosomal MicroRNAs suppress myofibroblast differentiation by inhibiting the transforming growth factor-β/SMAD2 pathway during wound healing. Stem Cells Transl. Med. 5 (10), 1425–1439. doi:10.5966/sctm.2015-0367
Ferguson, S. W., Wang, J., Lee, C. J., Liu, M., Neelamegham, S., Canty, J. M., et al. (2018). The microRNA regulatory landscape of MSC-derived exosomes: A systems view. Sci. Rep. 8 (1), 1419. doi:10.1038/s41598-018-19581-x
Fu, X., Fang, L., Li, X., Cheng, B., and Sheng, Z. (2006). Enhanced wound-healing quality with bone marrow mesenchymal stem cells autografting after skin injury. Wound Repair Regen. 14 (3), 325–335. doi:10.1111/j.1743-6109.2006.00128.x
Giunti, D., Marini, C., Parodi, B., Usai, C., Milanese, M., Bonanno, G., et al. (2021). Role of miRNAs shuttled by mesenchymal stem cell-derived small extracellular vesicles in modulating neuroinflammation. Sci. Rep. 11 (1), 1740. doi:10.1038/s41598-021-81039-4
Gurtner, G. C., Werner, S., Barrandon, Y., and Longaker, M. T. (2008). Wound repair and regeneration. Nature 453 (7193), 314–321. doi:10.1038/nature07039
Heo, S. C., Jeon, E. S., Lee, I. H., Kim, H. S., Kim, M. B., and Kim, J. H. (2011). Tumor necrosis factor-alpha-activated human adipose tissue-derived mesenchymal stem cells accelerate cutaneous wound healing through paracrine mechanisms. J. Invest. Dermatol 131 (7), 1559–1567. doi:10.1038/jid.2011.64
Hu, Y., Rao, S. S., Wang, Z. X., Cao, J., Tan, Y. J., Luo, J., et al. (2018). Exosomes from human umbilical cord blood accelerate cutaneous wound healing through miR-21-3p-mediated promotion of angiogenesis and fibroblast function. Theranostics 8 (1), 169–184. doi:10.7150/thno.21234
Huang, Y. Z., Gou, M., Da, L. C., Zhang, W. Q., and Xie, H. Q. (2020). Mesenchymal stem cells for chronic wound healing: current status of preclinical and clinical studies. Tissue Eng. Part B Rev. 26 (6), 555–570. doi:10.1089/ten.TEB.2019.0351
Jackson, W. M., Nesti, L. J., and Tuan, R. S. (2012). Concise review: clinical translation of wound healing therapies based on mesenchymal stem cells. Stem Cells Transl. Med. 1 (1), 44–50. doi:10.5966/sctm.2011-0024
Koh, T. J., and DiPietro, L. A. (2011). Inflammation and wound healing: the role of the macrophage. Expert Rev. Mol. Med. 13, e23. doi:10.1017/S1462399411001943
Kucharzewski, M., Rojczyk, E., Wilemska-Kucharzewska, K., Wilk, R., Hudecki, J., and Los, M. J. (2019). Novel trends in application of stem cells in skin wound healing. Eur. J. Pharmacol. 843, 307–315. doi:10.1016/j.ejphar.2018.12.012
Kusuma, G. D., Carthew, J., Lim, R., and Frith, J. E. (2017). Effect of the microenvironment on mesenchymal stem cell paracrine signaling: opportunities to engineer the therapeutic effect. Stem Cells Dev. 26 (9), 617–631. doi:10.1089/scd.2016.0349
Landen, N. X., Li, D., and Stahle, M. (2016). Transition from inflammation to proliferation: A critical step during wound healing. Cell Mol. Life Sci. 73 (20), 3861–3885. doi:10.1007/s00018-016-2268-0
Li, H., Jiang, T., Li, M. Q., Zheng, X. L., and Zhao, G. J. (2018a). Transcriptional regulation of macrophages polarization by MicroRNAs. Front. Immunol. 9, 1175. doi:10.3389/fimmu.2018.01175
Li, J., Xu, S. Q., Zhao, Y. M., Yu, S., Ge, L. H., and Xu, B. H. (2018b). Comparison of the biological characteristics of human mesenchymal stem cells derived from exfoliated deciduous teeth, bone marrow, gingival tissue, and umbilical cord. Mol. Med. Rep. 18 (6), 4969–4977. doi:10.3892/mmr.2018.9501
Li, X., Liu, L., Yang, J., Yu, Y., Chai, J., Wang, L., et al. (2016). Exosome derived from human umbilical cord mesenchymal stem cell mediates MiR-181c attenuating burn-induced excessive inflammation. EBioMedicine 8, 72–82. doi:10.1016/j.ebiom.2016.04.030
Liang, Y. C., Wu, Y. P., Li, X. D., Chen, S. H., Ye, X. J., Xue, X. Y., et al. (2019). TNF-alpha-induced exosomal miR-146a mediates mesenchymal stem cell-dependent suppression of urethral stricture. J. Cell Physiol. 234 (12), 23243–23255. doi:10.1002/jcp.28891
Linard, C., Tissedre, F., Busson, E., Holler, V., Leclerc, T., Strup-Perrot, C., et al. (2015). Therapeutic potential of gingival fibroblasts for cutaneous radiation syndrome: comparison to bone marrow-mesenchymal stem cell grafts. Stem Cells Dev. 24 (10), 1182–1193. doi:10.1089/scd.2014.0486
Lorenzini, B., Peltzer, J., Goulinet, S., Rival, B., Lataillade, J. J., Uzan, G., et al. (2023). Producing vesicle-free cell culture additive for human cells extracellular vesicles manufacturing. J. Control Release 355, 501–514. doi:10.1016/j.jconrel.2023.01.073
Macrin, D., Joseph, J. P., Pillai, A. A., and Devi, A. (2017). Eminent sources of adult mesenchymal stem cells and their therapeutic imminence. Stem Cell Rev. 13 (6), 741–756. doi:10.1007/s12015-017-9759-8
Madrigal, M., Rao, K. S., and Riordan, N. H. (2014). A review of therapeutic effects of mesenchymal stem cell secretions and induction of secretory modification by different culture methods. J. Transl. Med. 12, 260. doi:10.1186/s12967-014-0260-8
Maffioli, E., Nonnis, S., Angioni, R., Santagata, F., Cali, B., Zanotti, L., et al. (2017). Proteomic analysis of the secretome of human bone marrow-derived mesenchymal stem cells primed by pro-inflammatory cytokines. J. Proteomics 166, 115–126. doi:10.1016/j.jprot.2017.07.012
Magne, B., Dedier, M., Nivet, M., Coulomb, B., Banzet, S., Lataillade, J. J., et al. (2020). IL-1β-Primed mesenchymal stromal cells improve epidermal substitute engraftment and wound healing via matrix metalloproteinases and transforming growth factor-β1. J. Invest. Dermatol 140 (3), 688–698. doi:10.1016/j.jid.2019.07.721
McFarland-Mancini, M. M., Funk, H. M., Paluch, A. M., Zhou, M., Giridhar, P. V., Mercer, C. A., et al. (2010). Differences in wound healing in mice with deficiency of IL-6 versus IL-6 receptor. J. Immunol. 184 (12), 7219–7228. doi:10.4049/jimmunol.0901929
Mitchell, R., Mellows, B., Sheard, J., Antonioli, M., Kretz, O., Chambers, D., et al. (2019). Secretome of adipose-derived mesenchymal stem cells promotes skeletal muscle regeneration through synergistic action of extracellular vesicle cargo and soluble proteins. Stem Cell Res. Ther. 10 (1), 116. doi:10.1186/s13287-019-1213-1
Nakao, Y., Fukuda, T., Zhang, Q., Sanui, T., Shinjo, T., Kou, X., et al. (2021). Exosomes from TNF-α-treated human gingiva-derived MSCs enhance M2 macrophage polarization and inhibit periodontal bone loss. Acta Biomater. 122, 306–324. doi:10.1016/j.actbio.2020.12.046
Noronha, N. C., Mizukami, A., Caliari-Oliveira, C., Cominal, J. G., Rocha, J. L. M., Covas, D. T., et al. (2019). Priming approaches to improve the efficacy of mesenchymal stromal cell-based therapies. Stem Cell Res. Ther. 10 (1), 131. doi:10.1186/s13287-019-1224-y
Nourian Dehkordi, A., Mirahmadi Babaheydari, F., Chehelgerdi, M., and Raeisi Dehkordi, S. (2019). Skin tissue engineering: wound healing based on stem-cell-based therapeutic strategies. Stem Cell Res. Ther. 10 (1), 111. doi:10.1186/s13287-019-1212-2
Papait, A., Ragni, E., Cargnoni, A., Vertua, E., Romele, P., Masserdotti, A., et al. (2022). Comparison of EV-free fraction, EVs, and total secretome of amniotic mesenchymal stromal cells for their immunomodulatory potential: A translational perspective. Front. Immunol. 13, 960909. doi:10.3389/fimmu.2022.960909
Park, S. R., Kim, J. W., Jun, H. S., Roh, J. Y., Lee, H. Y., and Hong, I. S. (2018). Stem cell secretome and its effect on cellular mechanisms relevant to wound healing. Mol. Ther. 26 (2), 606–617. doi:10.1016/j.ymthe.2017.09.023
Peltzer, J., Lund, K., Goriot, M. E., Grosbot, M., Lataillade, J. J., Mauduit, P., et al. (2020). Interferon-gamma and hypoxia priming have limited effect on the miRNA landscape of human mesenchymal stromal cells-derived extracellular vesicles. Front. Cell Dev. Biol. 8, 581436. doi:10.3389/fcell.2020.581436
Petrenko, Y., Vackova, I., Kekulova, K., Chudickova, M., Koci, Z., Turnovcova, K., et al. (2020). A comparative analysis of multipotent mesenchymal stromal cells derived from different sources, with a focus on neuroregenerative potential. Sci. Rep. 10 (1), 4290. doi:10.1038/s41598-020-61167-z
Philipp, D., Suhr, L., Wahlers, T., Choi, Y. H., and Paunel-Gorgulu, A. (2018). Preconditioning of bone marrow-derived mesenchymal stem cells highly strengthens their potential to promote IL-6-dependent M2b polarization. Stem Cell Res. Ther. 9 (1), 286. doi:10.1186/s13287-018-1039-2
Pires, A. O., Mendes-Pinheiro, B., Teixeira, F. G., Anjo, S. I., Ribeiro-Samy, S., Gomes, E. D., et al. (2016). Unveiling the differences of secretome of human bone marrow mesenchymal stem cells, adipose tissue-derived stem cells, and human umbilical cord perivascular cells: A proteomic analysis. Stem Cells Dev. 25 (14), 1073–1083. doi:10.1089/scd.2016.0048
Redondo-Castro, E., Cunningham, C. J., Miller, J., Brown, H., Allan, S. M., and Pinteaux, E. (2018). Changes in the secretome of tri-dimensional spheroid-cultured human mesenchymal stem cells in vitro by interleukin-1 priming. Stem Cell Res. Ther. 9 (1), 11. doi:10.1186/s13287-017-0753-5
Song, Y., Dou, H., Li, X., Zhao, X., Li, Y., Liu, D., et al. (2017). Exosomal miR-146a contributes to the enhanced therapeutic efficacy of interleukin-1β-primed mesenchymal stem cells against sepsis. Stem Cells 35 (5), 1208–1221. doi:10.1002/stem.2564
Tan, C. Y., Lai, R. C., Wong, W., Dan, Y. Y., Lim, S. K., and Ho, H. K. (2014). Mesenchymal stem cell-derived exosomes promote hepatic regeneration in drug-induced liver injury models. Stem Cell Res. Ther. 5 (3), 76. doi:10.1186/scrt465
Vandesompele, J., De Preter, K., Pattyn, F., Poppe, B., Van Roy, N., De Paepe, A., et al. (2002). Accurate normalization of real-time quantitative RT-PCR data by geometric averaging of multiple internal control genes. Genome Biol. 3 (7), RESEARCH0034. doi:10.1186/gb-2002-3-7-research0034
Wedzinska, A., Figiel-Dabrowska, A., Kozlowska, H., and Sarnowska, A. (2021). The effect of proinflammatory cytokines on the proliferation, migration and secretory activity of mesenchymal stem/stromal cells (WJ-MSCs) under 5% O2 and 21% O2 culture conditions. J. Clin. Med. 10 (9), 1813. doi:10.3390/jcm10091813
Wolf, M., Poupardin, R. W., Ebner-Peking, P., Andrade, A. C., Blochl, C., Obermayer, A., et al. (2022). A functional corona around extracellular vesicles enhances angiogenesis, skin regeneration and immunomodulation. J. Extracell. Vesicles 11 (4), e12207. doi:10.1002/jev2.12207
Wu, H., Fan, H., Shou, Z., Xu, M., Chen, Q., Ai, C., et al. (2019). Extracellular vesicles containing miR-146a attenuate experimental colitis by targeting TRAF6 and IRAK1. Int. Immunopharmacol. 68, 204–212. doi:10.1016/j.intimp.2018.12.043
Xu, X., Gu, S., Huang, X., Ren, J., Gu, Y., Wei, C., et al. (2020). The role of macrophages in the formation of hypertrophic scars and keloids. Burns Trauma 8, tkaa006. tkaa006. doi:10.1093/burnst/tkaa006
Yamada, Y., Nakamura-Yamada, S., Umemura-Kubota, E., and Baba, S. (2019). Diagnostic cytokines and comparative analysis secreted from exfoliated deciduous teeth, dental pulp, and bone marrow derived mesenchymal stem cells for functional cell-based therapy. Int. J. Mol. Sci. 20 (23), 5900. doi:10.3390/ijms20235900
Keywords: mesenchymal stromal cells, priming, extracellular vesicles, secretome, interleurkin-6, wound healing
Citation: Dedier M, Magne B, Nivet M, Banzet S and Trouillas M (2023) Anti-inflammatory effect of interleukin-6 highly enriched in secretome of two clinically relevant sources of mesenchymal stromal cells. Front. Cell Dev. Biol. 11:1244120. doi: 10.3389/fcell.2023.1244120
Received: 21 June 2023; Accepted: 28 August 2023;
Published: 07 September 2023.
Edited by:
Anna Meyfour, Shahid Beheshti University of Medical Sciences, IranReviewed by:
Jia Xian Law, National University of Malaysia, MalaysiaAnna Valeria Samarelli, University of Modena and Reggio Emilia, Italy
Copyright © 2023 Dedier, Magne, Nivet, Banzet and Trouillas. This is an open-access article distributed under the terms of the Creative Commons Attribution License (CC BY). The use, distribution or reproduction in other forums is permitted, provided the original author(s) and the copyright owner(s) are credited and that the original publication in this journal is cited, in accordance with accepted academic practice. No use, distribution or reproduction is permitted which does not comply with these terms.
*Correspondence: Marina Trouillas, bWFyaW5hLWxhdXJpZS50cm91aWxsYXNAaW5zZXJtLmZy
†Present address: Brice Magne, Department of Pathology and Laboratory Medicine, Children’s Hospital of Philadelphia Research Institute, Philadelphia, PA, United States