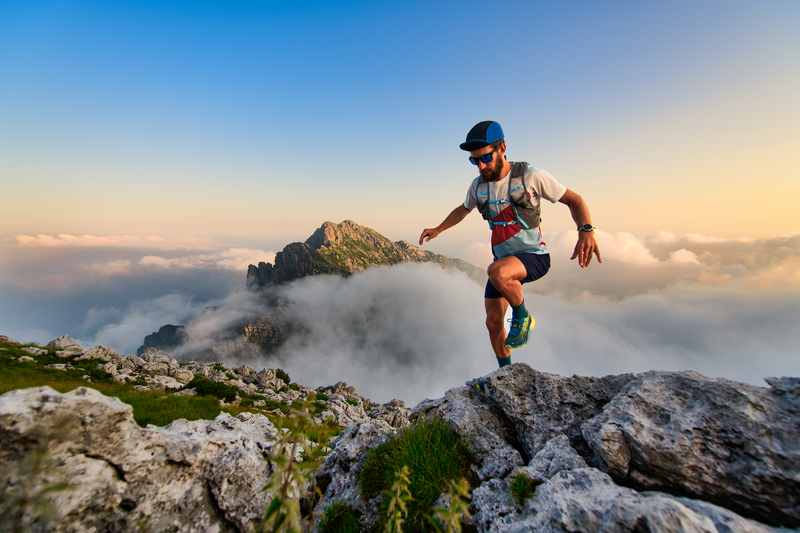
95% of researchers rate our articles as excellent or good
Learn more about the work of our research integrity team to safeguard the quality of each article we publish.
Find out more
ORIGINAL RESEARCH article
Front. Cell Dev. Biol. , 19 October 2023
Sec. Molecular and Cellular Pathology
Volume 11 - 2023 | https://doi.org/10.3389/fcell.2023.1241008
This article is part of the Research Topic Epithelial Barrier Dysfunction in Disease View all 11 articles
Introduction: Asthma is the most common chronic inflammatory disease of the airways. The airway epithelium is a key driver of the disease, and numerous studies have established genome-wide differences in mRNA expression between health and asthma. However, the underlying molecular mechanisms for such differences remain poorly understood. The human TTP family is comprised of ZFP36, ZFP36L1 and ZFP36L2, and has essential roles in immune regulation by determining the stability and translation of myriad mRNAs encoding for inflammatory mediators. We investigated the expression and possible role of the tristetraprolin (TTP) family of RNA binding proteins (RBPs), poorly understood in asthma.
Methods: We analysed the levels of ZFP36, ZFP36L1 and ZFP36L2 mRNA in several publicly available asthma datasets, including single cell RNA-sequencing. We also interrogated the expression of known targets of these RBPs in asthma. We assessed the lung mRNA expression and cellular localization of Zfp36l1 and Zfp36l2 in precision cut lung slices in murine asthma models. Finally, we determined the expression in airway epithelium of ZFP36L1 and ZFP36L2 in human bronchial biopsies and performed rescue experiments in primary bronchial epithelium from patients with severe asthma.
Results: We found ZFP36L1 and ZFP36L2 mRNA levels significantly downregulated in the airway epithelium of patients with very severe asthma in different cohorts (5 healthy vs. 8 severe asthma; 36 moderate asthma vs. 37 severe asthma on inhaled steroids vs. 26 severe asthma on oral corticoids). Integrating several datasets allowed us to infer that mRNAs potentially targeted by these RBPs are increased in severe asthma. Zfp36l1 was downregulated in the lung of a mouse model of asthma, and immunostaining of ex vivo lung slices with a dual antibody demonstrated that Zfp36l1/l2 nuclear localization was increased in the airway epithelium of an acute asthma mouse model, which was further enhanced in a chronic model. Immunostaining of human bronchial biopsies showed that airway epithelial cell staining of ZFP36L1 was decreased in severe asthma as compared with mild, while ZFP36L2 was upregulated. Restoring the levels of ZFP36L1 and ZFP36L2 in primary bronchial epithelial cells from patients with severe asthma decreased the mRNA expression of IL6, IL8 and CSF2.
Discussion: We propose that the dysregulation of ZFP36L1/L2 levels as well as their subcellular mislocalization contributes to changes in mRNA expression and cytoplasmic fate in asthma.
Asthma is a common chronic respiratory disease affecting between 1%–29% of the population in different countries (Asher et al., 2021; Mortimer et al., 2022; GINA, 2023). It is characterised by variable symptoms of wheeze, chest tightness, shortness of breath and variable expiratory airflow limitation. Asthma is often defined as an inflammatory disease, implying that asthma has a major immune-related component. However, it is well established that structural cells including smooth muscle and airway epithelium play a major role in the disease.
Airway epithelial cells lie at the interface between the lung and the external environment, primarily acting as a protective barrier but also as immune modulators (Holgate, 2008; Frey et al., 2020). The importance of airway epithelial cells is well described in the pathophysiology of asthma. Patients with asthma present altered barrier function, mucus overproduction by goblet cells, epithelial cell damage and impaired epithelial repair. All these features contribute to airway remodelling, which is a broad term to define the airway structural changes that are always present in asthma (Holgate et al., 2015). Multiple genome-wide approaches have been implemented to further our understanding of asthma and the role and profile of the bronchial epithelium in patients with this disease. These include RNA expression analysis (Kuo et al., 2017; Hekking et al., 2018), breathomics (Rufo et al., 2016), metabolomics (Kelly et al., 2017) or sputum proteomics (Schofield et al., 2019). However, there is poor insight into the underlying mechanisms regulating gene expression or driving their phenotype at the molecular level.
From transcription to translation into protein, RNA undergoes multiple steps such as splicing, transport and stability, encompassed under ‘post transcriptional regulation’. Post transcriptional regulation is mainly undertaken by microRNAs and RNA binding proteins (RBPs). Most omics approaches overlook these regulatory mechanisms and consider that mRNA expression is synonymous with corresponding protein levels. However, not all RNAs encode for proteins and there is little mRNA-to-protein correlation in many coding genes (Liu et al., 2016; Brion et al., 2020). To further our understanding of mRNA cytoplasmic fate, we developed subcellular fractionation and RNA-sequencing (Frac-seq) (Sterne-Weiler et al., 2013). Frac-seq enables analysing mRNA steady levels (transcriptional) and those of transcripts bound to the translation machinery (ribosomes), the latter a better proxy for protein levels (King and Gerber, 2016). Our previous work showed the disconnection between steady and ribosome-bound mRNA levels in bronchial epithelial cells from patients with asthma (Martinez-Nunez et al., 2018). We identified a network of six microRNAs that accounted for roughly 50% of the changes we observed in mRNA dysregulation (Martinez-Nunez et al., 2018). Thus, the remaining 50% levels must be mostly driven by RBPs. There is some evidence of RBP levels being dysregulated in airway epithelium in other respiratory diseases such as chronic obstructive pulmonary disease (Ricciardi et al., 2018), however, their role in airway epithelium in asthma remains poorly understood.
The tristetraprolin (TTP) family of RBPs, consisting of ZFP36, ZFP36L1 and ZFP36L2 in humans, has been implicated in the regulation of immune responses (Makita et al., 2021), but little is known about their role in epithelium or asthma. These RBPs inhibit mRNA expression by binding to AU-rich elements (ARE) present in the 3′UnTranslated Region (UTR) of their target RNAs (Blackshear, 2002; Cassandri et al., 2017). TTP is known to modulate the effect of glucocorticoids (Ishmael et al., 2008), and we have recently discovered that ZFP36L1/L2 modulate the effect of glucocorticoids and the expression levels of mRNAs encoding epithelial-related functions (Rynne et al., 2022). Considering that glucocorticoids are the mainstay treatment of patients with asthma (Chung et al., 2014), we hypothesised that the TTP family may be dysregulated in asthma and set out to investigate their expression and potential roles in airway epithelium of human samples and asthma murine models.
We initially mined our previous Frac-seq dataset to determine the levels of all TTP members. Patient details were reported previously (Martinez-Nunez et al., 2018). Changes in mRNA levels were determined by comparing total (Total) and polyribosome bound (Polyribosome) mRNA in health vs. asthma. Polyribosome fractions excluded the monosomal (80 S or one ribosome) fraction. We observed significantly decreased binding of ZFP36L1 and ZFP36L2 mRNAs to polyribosomes in primary bronchial epithelial cells from severe asthma patients compared to age- and sex-matched healthy controls (both p < 0.05, Figure 1A). Comparing total mRNA from healthy controls to severe asthma patients showed no difference in the expression of ZFP36, ZFP36L1 or ZFP36L2 mRNA (Figure 1A).
FIGURE 1. Analysis of the expression of the TTP family in human bronchial epithelial samples. (A) Frac-seq data showing the log counts for ZFP36, ZFP36L1 and ZFP36L2 in bronchial epithelial cells from severe asthma (SA, n = 8) vs. healthy control (HC, n = 5) donors from (Martinez-Nunez et al., 2018) in total mRNA (Total) or polyribosome-bound mRNA (Polyribosome). (B) Analysis of GSE76227 dataset from U-BIOPRED showing the log intensity values of microarrays in bronchial brushings from patients with moderate (MO) and severe asthma (SA). (C) Analysis of GSE76227 dataset from U-BIOPRED showing the log intensity values of microarrays in bronchial brushing from patients with moderate (MO, n = 36), severe asthma on oral corticoids (SA (OC), n = 26) and severe asthma on inhaled GCs (SA (no OC)), n = 37). Statistical significance was assessed by two-tailed t-tests on log transformed data. *p < 0.05.
These data prompted us to further interrogate the levels and role of these RBPs in asthma in a larger cohort. We analysed datasets from U-BIOPRED, the largest European consortium of severe asthma patients. The U-BIOPRED dataset (GSE76227) consists of transcriptomic arrays from bronchial brushings, which are enriched in bronchial epithelium (Perotin et al., 2019). The authors compared moderate asthma patients on inhaled glucocorticoids (MO), representing patients with disease control, to severe asthma patients (SA), without differentiating between corticosteroid use. SA patients taking oral corticosteroids represent patients with inadequate control of disease. Figure 1B depicts graphs displaying the log intensity values from the microarray probes showing that there was no difference in ZFP36, ZFP36L1 or ZFP36L2 mRNA expression. Upon stratification of severe asthma patients into those on inhaled glucocorticoids (SA-no OC) or taking oral glucocorticoids (SA-OC), we observed downregulation of ZFP36L1 mRNA expression in SA-no OC patients compared to MO patients (p < 0.05), and a further downregulation in SA-OC versus SA-no OC patients (p < 0.05, Figure 1C). We also found that the expression of ZFP36L2 mRNA was downregulated in SA-OC patients compared to SA-no OC (Figure 1C). We did not observe differential expression of ZFP36 mRNA levels.
We further explored the potential role of ZFP36L1 and ZFP36L2 in bronchial epithelial cells in asthma. Our Frac-seq dataset consisted of mainly basal epithelial cells, while bronchial brushings from U-BIOPRED will contain a mixture of different airway epithelial cells. We interrogated the Lung Cell Atlas datasets comparing very mild patients with asthma not on inhaled corticosteroid therapy vs. healthy controls (Vieira Braga et al., 2019). In health, ZFP36L1 mRNA was expressed quite broadly in most epithelial cell types while ZFP36L2 mRNAs appeared more present in Basal 1 and Basal 2 cells (Supplementary Figure S1). In asthma samples, ZFP36L1 mRNA was decreased in mucous ciliated cells while it appeared to increase in ionocytes, ciliated and basal cycling cells (Figures 2A,B). ZFP36L2 mRNA showed a trend towards downregulation in most airway cell types, with only submucosal and Club cells showing an increase in expression. Thus, single cell data support the concept that RBP mRNA expression is decreased in specific epithelial cell subsets in asthma.
FIGURE 2. Airway epithelial cell-specific patterns of ZFP36L1 and ZFP36L2 mRNA expression and ZFP36L2 targets’ behaviour in severe asthma. (A) t-Distributed Stochastic Neighbor Embedding (t-SNE) plots representing the different airway epithelial cell types present in very mild asthma as per (Vieira Braga et al., 2019) (left), with ZFP36L1 and ZFP36L2 mRNA expression patterns represented on the tSNE’s on the right. (B) Relative expression ZFP36L1 and ZFP36L2 mRNA levels between health and asthma per airway epithelial cell type. IO: Ionocytes, SM: Submucosal, MC: Mucous ciliated, GO: Goblet, CI: Ciliated, BA: Basal activated, BC: Basal Cycling, B1: Basal 1, CL: Club, B2: Basal 2. (C) Left: Venn diagram representing the overlap of differentially expressed genes (DEGs in Total, red) or differentially bound genes (DBGs in Polyribosome, green) containing AU-rich element (ARE, blue) (Bakheet et al., 2018) when comparing health vs. severe asthma. Right: bar plot showing the proportion and direction of changes (Up or Downregulated) of ARE-containing mRNAs in DEGs and DBGs when comparing health vs. severe asthma. (D) Bar plot showing the proportion of ZFP36L2 targets as per (Zhang et al., 2013) present per subcellular fraction in our Frac-seq health vs. severe asthma dataset. Statistics were done employing a two-sided Chi-square test. ****: p < 0.0001.
We next analyzed if mRNAs containing AREs (Bakheet et al., 2018) were dysregulated in our Frac-seq dataset comparing health vs. severe asthma (Martinez-Nunez et al., 2018). ARE-containing transcripts are targets of ZFP36L1 and ZFP36L2. Although we observed that a proportion of differentially expressed genes (DEGs) in total mRNA and differentially bound genes to polyribosome (DBGs) contain ARE in their 3′UTR (Figure 2C), these did not appear to be significantly enriched amongst DEGs or DBGs. To determine if ZFP36L2, specifically, may target DEGs and DBGs, we extracted known targets to be bound by ZFP36L2 from (Zhang et al., 2013) and analyzed their presence in our Frac-seq DEGs and DBGs. Figure 2D shows that direct targets of ZFP36L2 were predominantly upregulated in polyribosome-bound genes in severe asthma. This enrichment was absent in total DEGs between healthy controls and severe asthma patients.
Considering that patients with severe asthma are treated with high doses of inhaled and/or oral corticosteroids, we also investigated if the changes seen in the DEGs and DBGs between health and SA were due to glucocorticoid (GC) exposure. We cross referenced our health vs. severe asthma Frac-seq dataset with our recent Frac-seq data investigating post-transcriptional changes induced by GCs in primary bronchial epithelial cells (Rynne et al., 2022). To that end, we compared upregulated and downregulated DEGs and DBGs between health and severe asthma and upregulated and downregulated DEGs and DBGs upon GC exposure. We only found 5 DEGs and 1 DBG between healthy controls and severe asthma that followed the same trend (up- or downregulated in a fraction-dependent manner) upon GC treatment). We also investigated the expression of these mRNAs in the U-BIOPRED dataset and found only 3 were present, namely, DUSP1, SRM, and PTK2B. These genes were not altered between moderate and severe asthma patients, or between severe asthma patients stratified by OCS usage (Supplementary Figure S2). These data suggest that decreased RBP expression in severe asthma is unlikely to be due simply to oral corticosteroid use.
In summary, our analysis inferred that ZFP36L1 and ZFP36L2 encoding mRNAs are present in distinct airway epithelial cells and that ZFP36L2 preferentially targets mRNAs that are bound to polyribosomes in bronchial epithelial cells from patients with severe asthma.
After having analysed the levels of ZFP36L1 and ZFP36L2 in omics mRNA datasets and inferring their role in ARE regulation and epithelial cell biology, we interrogated their expression levels in an in vivo model of asthma. The House Dust Mite (HDM) model is an established model of allergen-induced inflammation and one of the most used in vivo models of asthma (McMillan and Lloyd, 2004). C57BL/6J mice were administered intranasally 25 μL (1 mg/mL) protein weight solution dissolved in PBS of HDM extract (Citeq) or equal volumes of PBS five times a week for 1 week or 5 weeks, to produce acute or chronic inflammation. This model has already been published elsewhere (Johnson et al., 2004; Ortiz-Zapater et al., 2022) but we tested the effect of the HDM at different times using both H&E staining from formalin-fixed paraffin embedded (FFPE) sections of mice from the different groups (Figure 3A). Supplementary Figure S3 shows a diagram of the different lung reagions employed in each experiment. We can observe immune infiltration in the section of HDM mice, increased after chronic exposure of HDM. After 5 weeks, the increase in the epithelium height is also evident (see arrows in Figure 3A). Total RNA from whole lung tissue from the different four groups was also analysed for the expression of two asthma-associated inflammatory cytokines, Ccl20 and Il13. As expected, there was a clear increase in the expression of both transcripts after 1 week of HDM treatment (Figure 3B) and 5 weeks of HDM treatment (Figure 3C). We then assessed the mRNA levels of both Zfp36l1 and Zfp36l2 after both acute and chronic HDM treatment. As shown in Figure 3D, the expression of both Zfp36l1 and Zfp36l2 was not modified between PBS and acutely HDM treated mice. However, the mRNA expression of Zfp36l1 in total lung tissue was significantly downregulated after chronic HDM exposure (Figure 3E, left panel). This was not seen for Zfp36l2, although there was lower expression compared to PBS mice in around half of the mice (Figure 3E, right panel).
FIGURE 3. Zfp36l1 and Zfp36l2 mRNA expression in the lungs different mouse models of asthma. (A) Representative images of Hematoxilyn and Eosin (H and E) staining of FFPE lung sections from mice treated with PBS as a control or with HDM for different times as indicated in the figure. Scale bars 125 μm. (B). The expression of two cytokines (Ccl20 and Il13) in whole lung mRNA was analyzed by quantitative qPCR for 1 week and in (C) for 5 weeks. Bar graphs represent mean fold change (±SEM) over the average PBS-treated control (n = 3 mice per group at PBS/HDM for 1 week and n = 5 mice per group in 3 independent experiments for the 5 weeks treatment). (D) Expression of both Zfp36l1 and Zfp26l2 in whole lung mRNA analysed by quantitative PCR for 1 week and in (E) for 5 weeks. Bar graphs represent mean fold change (±SEM) over the average PBS-treated control (n = 3 mice per group at PBS/HDM for 1 week and n = 5 mice per group in 3 independent experiments for the 5 weeks treatment). Statistical significance was assessed by multiple two-tailed parametric t-tests on log transformed data. p < 0.05*, p < 0.01**, p < 0.001*** and p ≤ 0.0001****. Black arrows point towards increased epithelium height.
To further investigate our findings in vivo, we assessed the localization of Zfp36l1 and Zfp36l2 in the airways of HDM exposed mice. We employed mice exposed to HDM over three and 5 weeks; these mice produced a robust T2-driven inflammatory response and asthma-like phenotype including airway hyperresponsiveness, mucus hyperproduction, and immune cell infiltration by 3 weeks. PBS- and HDM-treated mice were sacrificed and lungs harvested and processed to obtain precision-cut ex vivo lung slices (PCLS) that were fixed and analyzed by immunofluorescence and confocal imaging. Indeed, in healthy airways and bronchioles there was little to no Muc5ac (a pathological mucin highly upregulated in asthma) expression in the epithelial monolayer, nor immune cell infiltrate near airways in the surrounding alveolar space (Figure 4A, top left panels). Unsurprisingly, HDM-treated airways had drastically increased expression of Muc5ac in the epithelium and significant immune cell infiltration near airways as shown by Ly-6G + neutrophils (immune cell type notoriously increased in many patients with asthma) (Figure 4A, top right panels). We found that Zfp36l1/Zfp36l2 (using a dual-staining antibody for both Zfp36l1 and Zfp36l2 proteins) were expressed in airway epithelial cells and nearly evenly distributed between the nucleus and cytoplasm in healthy mice. However, in airways exposed to HDM, Zfp36l1/Zfp36l2 became robustly recruited into the nucleus of airway epithelial cells (Figures 4B,C). Notably, nuclear accumulation of Zfp36l1/l2 at 5-week HDM treatment was even more dramatic, demonstrating a positive correlation between nuclear Zfp36l1/l2 and asthma severity (Figures 4B,C). To confirm this, measurements of fluorescence intensity were made for nuclear and cytoplasmic Zfp36l1/Zfp36l2 and ratios calculated from healthy and HDM treated airways at three and 5 weeks, demonstrating a dramatic recruitment of Zfp36l1/l2 to the nucleus with HDM treatment that increased over time and disease severity (Figure 4D).
FIGURE 4. Intracellular mislocalization of Zfp36l1/Zfp36l2 in airway epithelial cells of mice with asthma-like characteristics. (A) Representative spinning disc confocal images of ex vivo lung slices from healthy controls and HDM-treated (3 weeks) mice were fixed and immune-stained for asthmatic markers of mucus (Muc5ac) and neutrophils (Ly-6G) to demonstrate an asthma phenotype (scale bars are 100 and 50 microns, respectively). (B) Confocal projections of healthy and HDM-treated airway epithelial cells immune-stained for Zfp36l1/l2, actin and nuclei (scale bar 25 microns), with insets highlighting Zfp36l1/Zfp36l2 intracellular localization in epithelial cells in health (Control), HDM after 3 weeks of exposure (HDM 3 weeks) and after 5 weeks of exposure (HDM 5 weeks). (C): Insets highlighting its nuclear accumulation of Zfp36l1/l2 in epithelial cells in HDM. (D) Quantification of nuclear and cytoplasmic mean fluorescence intensity of Zfp36l1/Zfp36l2 in control and HDM-treated airways (3 weeks and 5 weeks of exposure), using regions of interest (ROIs) defined by the DAPI (nuclei) and actin (cytoplasm) channels. Mann-Whitney test was performed on 100 cells from 3 mice per group. ****: p < 0.0001. Ctrl: Control. HDM: House dust mite.
To further investigate the dysregulation of both ZFP36L1 and ZFP36L2 mRNA levels in human (Figure 1) and mouse asthma (Figure 3; Figure 4) we performed immunostaining of ZFP36L1 and ZFP36L2 in bronchial biopsies from patients with different asthma severities and non-asthma controls. Our results show that immunostaining with both ZFP36L1 and ZFP36L2 was predominant in bronchial epithelium (Figures 5A,B) while also present in other cell types. Analysis of the positively stained airway epithelial cells showed that patients with mild asthma had increased staining of ZFP36L1 in epithelial cells as compared with healthy controls and severe asthma (Figure 5C). Patients with severe asthma showed low ZFP36L1 staining, significantly decreased as compared with mild but not healthy controls. ZFP36L2 staining was increased in patients with severe asthma as compared to both healthy controls and mild patients (Figure 5D), but there was no difference in the ZFP36L2 staining between healthy controls and mild asthmatic patients. To determine the effects of restoring ZFP36L1 and ZFP36L2 levels, we employed a vector to overexpress Zfp36l1 and siRNAs to deplete ZFP36L2 in primary bronchial epithelial cells from patients with severe asthma. Transfection efficiency showed increased ZFP36L1 and decreased ZFP36L2 mRNA levels (Figure 5E) and led to decreased levels of pro-inflammatory IL6, IL8 and CSF2 mRNAs.
FIGURE 5. Immunohistochemistry staining of ZFP36L1 and ZFP36L2 in from bronchial biopsies from healthy controls and patients with mild and severe asthma. (A) Representative images of sections from patients classified as healthy controls (A, D), mild asthmatics (B, E) or patients with severe asthma (C, F). Staining was performed for ZFP36L1 and ZFP36L2 as indicated in the figure. Scale bar represents 100 μm. (B) Insets of the different sections shown in (A). The scale bar represents 50 μm. (C) and (D). Quantification of ZFP36L1 and ZFP36L2 staining in FFPE sections from each experimental group. In (C) Healthy controls n = 7, Mild asthmatics n = 9 and severe asthmatics n = 6. In D, Healthy controls n = 6, mild asthmatics n = 6 and severe asthmatics n = 7. Statistical significance was assessed by multiple two-tailed t-tests on log-transformed data. (E): transfection efficiency of primary bronchial epithelial cells from patients with severe asthma (2 donors, 1 donor at two different passages, n = 3). Co-transfection of an overexpression vector for Zfp36l1 and siRNAs against ZFP36L2 led to upregulation of ZFP36L1 and downregulation of ZFP36L2 mRNAs. (F): IL6, IL8 and CSF2 mRNA expression was decreased upon upregulation of ZFP36L1 and downregulation of ZFP36L2. One-tailed t-test of log transformed data. *: p < 0.05, **: p < 0.01. oeC, overexpression control; oeL1, overexpression plasmid for Zfp36l1; siC, siRNA control; siL2, siRNA targeting ZFP36L2.
Taken together, our data show that ZFP36L1 and ZFP36L2 are expressed in airway epithelial cells and that their levels and subcellular localization are modified in asthma, in a severity-dependent manner. Restoring ZFP36L1 and ZFP36L2 levels in primary bronchial epithelial cells from patients with severe asthma led to decreased expression of mRNAs encoding pro-inflammatory factors. ZFP36L1 and ZFP36L2 alter post-transcriptional gene expression in bronchial epithelial cells in severe asthma, granting further investigation into their role in chronic airway inflammation.
Our data demonstrate that ZFP36L1 and ZFP36L2 levels and subcellular localization are dysregulated in primary samples from both human and murine-like asthma. We have also performed in silico analysis of publicly available datasets of Zfp36l2 targets and observed that these are particularly enriched in genes that present increased binding to polyribosomes in severe asthma human primary bronchial epithelium. Modulating ZFP36L1 and ZFP36L2 in primary bronchial epithelial cells from patients with asthma decreased the expression of known pro-inflammatory mediators. Together, our data strongly suggest that ZFP36L1 and ZFP36L2 drive changes in gene expression in primary epithelial cells in asthma.
Airway epithelial cells contribute to disease pathobiology in asthma through immune modulation, defective barrier function, and airway remodelling. All asthma phenotypes present epithelial cell damage (Holgate, 2008; Xiao et al., 2011; Hardyman et al., 2013; Calven et al., 2020), and airway structural changes can be present before the onset of inflammation (Pohunek et al., 2005; Barbato et al., 2006). These observations are contrary to the old paradigm of repeated inflammation driving epithelial damage and deficient repair resulting in airway remodelling (Bush, 2008). Thus, epithelial changes appear to occur early on and be central to disease progression. It is possible that early epithelial cell gene expression reprogramming occurs leading to these cells behaving differently in asthma. This is indeed supported by studies that have determined a differential epigenetic signature in primary airway epithelium in asthma (McErlean et al., 2020). It is also possible that relatively small changes in master-regulators, such as RBPs, cause a sustained effect that leads to a different profile and cellular behaviour.
RBPs can modulate mRNA transcript levels and fate by binding to their targets and controlling most post-transcriptional steps, including alternative splicing, export, stability, storage, decay or translation into protein. The role of post-transcriptional gene expression regulation in inflammation is well established (Piccirillo et al., 2014; Makita et al., 2021) and defective RNA-RBP interactions can contribute to dysregulated immune responses (Liu and Cao, 2023). Additionally, RBPs have been found dysregulated in the epithelium of patients with chronic obstructive pulmonary disease (Ricciardi et al., 2018). There is no literature, to our knowledge, about the role of RBPs in asthma, where research in post-transcriptional regulation has focused on microRNAs (Solberg et al., 2012).
Amongst RBPs, the TTP family has well-established roles in modulating the immune system (Galloway et al., 2016; Vogel et al., 2016; Salerno et al., 2018), as well as the response to glucocorticoids (Ishmael et al., 2008; Rynne et al., 2022). Our data in Figure 1 showed a decreased expression of ZFP36L1 and ZFP36L2 mRNA in airway epithelial cells from patients with severe asthma, particularly of those that are undergoing oral corticosteroid treatment. We also found decreased of Zfp36l1 and Zfp36l2 mRNA in chronic asthma-like inflammation (akin to human severe asthma) in mice (Figure 3). At the protein level, in human bronchial biopsies (Figure 5), ZFP36L1 showed an increase in expression in mild patients, i.e., not taking glucocorticoids, as compared to healthy controls, and a decrease in patients with severe asthma (Figure 5). Contrary to mRNA expression, ZFP36L2 protein appeared upregulated in patients with severe asthma, i.e., on high doses of inhaled and/or oral glucocorticoids. These data highlight the importance of performing validations at the protein level, and the poor correlation existing between mRNA and protein levels (Gygi et al., 1999). It is also important to highlight that immunohistochemistry is a good methodology to indicate where a protein is expressed, but it is semi-quantitative. Further analysis of protein levels in specific airway epithelial types employing other methodologies such as fluorescent western blotting could offer further insight into total protein levels.
Mechanistically, it possible that expression levels of ZFP36L1 and ZFP36L2 are driven by different mechanisms in, particularly, severe/chronic asthma. Our data on murine models of acute and chronic asthma-like inflammation (Figure 3) showed decreased of Zfp36l1 mRNA in the lung of chronic asthma-like inflammation (akin to human severe asthma), although these data correspond to whole lung. More detailed time courses of HDM exposure and analysis of specific cell types may unravel the dynamics of expression of these RBPs. Zfp36l1/l2 nuclear localization was increased in the airways of mice with asthma-like characteristics (Figure 4). ZFP36L1 and predominantly ZFP36L2 are increased in bronchial epithelial cells exposed to glucocorticoids, while their nuclear localization is also enhanced upon glucocorticoid exposure (Rynne et al., 2022). Noteworthy, our antibody staining for immunofluorescence (in both (Rynne et al., 2022) and Figure 4 PCLS) did not allow us to distinguish between ZFP36L1/Zfp36l1 and ZFP36L2/Zfp36l2, which is a caveat in our study. Further determination of the localization of each protein separately is warranted. In bronchial biopsies, we observed a trend towards increased nuclear localization for ZFP36L2 in patients with severe asthma (Supplementary Figure S4) while ZFP36L1 appeared more localised in the cytosol. Together, these data point towards chronic inflammation driving changes in ZFP36L1 expression in asthma, while glucocorticoids may be the main drivers of ZFP36L2 protein expression in severe asthma. In either case, it appears that there is an increase in nuclear localization for these proteins in asthma. While this may infer an increase in their nuclear role, our integration of Zfp36l2 targets showed that ZFP36L2 exerts its effects mainly by modulating polyribosome association of mRNAs in severe asthma (Figure 2C). Thus, it is possible that the changes exerted by ZFP36L2 in the cytosol are driven by is nuclear kidnapping.
ZFP36L1 and ZFP36L2 encoding mRNAs also contain ARE in their 3′UTRs and can therefore regulate the expression of one another. Although our ARE analysis did not show an enrichment in ARE-containing mRNAs in patients with severe asthma, ARE-containing mRNAs accounted for 20%–28% of the genes differentially expressed and differentially bound to polyribosomes between health and severe asthma (Figure 2C). Our co-transfections also demonstrated that restoring the levels of ZFP36L1 and ZFP36L2 decreased the levels of IL6, IL8 and CSF2 (Figures 5E,F) mRNAs, which are known to contain AREs. However, TNFA and VEGF were not modulated (Supplementary Figure S5), suggesting a more complex interplay of these RBPs. Further investigation into the specific dynamics of these two RBPs in primary epithelium will enable elucidating their potential influence on each other’s expression and/or targets. Determining the levels of ZFP36L1 and ZFP36L2 in bronchial biopsies and those of their direct targets employing methodologies such as spatial omics would enable dissecting their complementary, separate or synergistic roles in a cell-type dependent manner. It is also possible that these proteins exert distinct effects in an airway cell-type manner. ZFP36L1 and ZFP36L2 mRNAs appeared differentially expressed in individual airway epithelial cell types, with these expression patterns varying between health and asthma (Figures 2A,B). This granularity is lost in bulk RNA-sequencing and these data suggest that these RBPs are indeed not redundant.
In summary, we provide, to our knowledge, the first evidence that RBPs are dysregulated in airway epithelial cells in human asthma and murine models of asthma in a severity-dependent manner. ZFP36L1/L2 appear to be modulated post-transcriptionally and post-translationally, with their subcellular localization influenced by chronic inflammation and possibly glucocorticoids. Recent evidence shows that ZFP36L1/L2 modulate epithelial-encoding mRNAs (Rynne et al., 2022). We propose RBPs as novel modulators of inflammation and epithelial structure in asthma and their further investigation in chronic airway disease.
Our Frac-seq cohort (n = 5 healthy and n = 8 severe asthma) and those utilized in biopsy staining were part of the Wessex severe asthma cohort (Azim et al., 2019), in which patients with severe asthma were defined as those fulfilling the European Respiratory Society/American Thoracic Society (ERS/ATS) criteria for severe asthma (Chung et al., 2014) and thus treated with high doses of inhaled and/or oral corticosteroids. Bronchial biopsies from Figure 5 were also part of the same cohort. Primary bronchial epithelial cells from Figures 5E,F and Supplementary Figure S5 were collected under REC 23/YH/0018. For all the rest of data we are using previously collected data that is publicly available. REC Numbers 06/Q0505/12 and 05/Q1702/165.
All the research in this manuscript complies with ethical regulations. The use of animals for this study was approved by the Ethical Review Committee at King’s College London and the Home Office, United Kingdom. All animals were housed in the Biological Support Unit (BSU) located in New Hunt’s House at King’s College London. All experiments were carried out under project license number P9672569A and personal license number I0F9CA46A. For House Dust Mite (HDM) immune-sensitised protocol, 6–8 weeks female C57BL/6 mice, were anaesthetised with isofluorane and administered either 25 μg (total protein) of HDM extract (Citeq Biologics; 1 mg/mL protein weight solution dissolved in PBS) or 25 μL of PBS intranasally 5 times per week for 5 consecutive weeks. Control mice received 25 μL of PBS. Mice were culled 24 h after the final HDM or PBS dose. Mice were sacrificed in a CO2 gas chamber for lung dissection.
RNA was isolated from cells or lung tissues using the RNeasy Qiagen kit (Qiagen; cat no 74004) following the manufacturer’s instructions. In the case of isolating RNA from lung tissue, lung lobes were chopped and stored in TRIzol. The tissue was then disaggregated using a Bullet Blender. RNA was used for cDNA synthesis using the LunaScript RT SuperMix Kit (NEB; cat no. E3010). cDNA synthesis reaction, consisting of 4 µL of 5X LunaScript RT SuperMix, 1 µg of RNA and Nuclease-free water, was prepared. Using a thermal cycler, the reaction was initialised by a primer annealing step of 25 °C for 2 min, followed by a cDNA synthesis step of 55 °C for 10 min, and a heat inactivation step of 95 °C for 1 min. Quantitative real-time PCR (qPCR) was performed using a QuantStudio 5 (Applied Biosystems/ThermoFisher) thermal cycler, the reaction was first heated to 95 °C for 1 min, followed by 40 cycles of 95 °C for 10 s. This was followed by an extension time of 30 s at 60 °C. We employed TaqMan assays (ThermoFisher Scientific).
Immunohistochemistry staining of ZFP36L1 and ZFP36L2 in lung tissue from bronchial biopsies in non-asthmatic patients, mild asthma, and severe asthma was carried out by Dr Jon Ward at the University of Southampton. Briefly, 10 µm thick sections paraffin-embedded sections were melted at 95°C for 2 h and de-waxed by dipping slides in xylene 2 × 10 min, 100% EtOh 2 × 5 min, 70% EtOH 1 × 5 min and 50% EtOH 1 × 5 min. Antigen retrieval was carried using sodium citrate buffer (0.0874 M sodium citrate, 0.0126 M citric acid pH 6) and incubating the slides for 20 min in a pressure cooker at 95°C. Endogenous peroxidase activity was blocked via 10 min incubation in hydrogen peroxide (3% in TBS) for DAB staining. Tissues were then washed 3 × with TBS and non-specific binding was blocked via incubation with TBS-1%BSA-1%FBS blocking solution for 1 h at room temperature. Primary antibody was added to the tissues, and these were left at 4°C overnight. After 3 × washes with TBS, DAB staining was visualised by adding DAB developing solution for up to 20 min (Dako). Tissues were then counterstained using haematoxylin for 1 s. Finally, tissues were dehydrated with graded alcohols and xylene before being mounted with DPX. The slides were stained for ZFP36L1 (abx124297, Abbexa, 1:3000 dilution) and for ZFP36L2 (PA5-30644, Invitrogen, 1:1500 dilution). Immunohistochemistry analysis was done employing Qupath (https://qupath.github.io/) for quantitative analysis. Positive epithelial cells were counted and expressed as a percentage of the total epithelium per slide. Mild asthma patients had controlled asthma, while severe asthma samples were obtained from the Wessex Severe Asthma Cohort and were classified as having inadequately controlled disease and fulfilled the ERS/ATS criteria for severe asthma.
Ex vivo lung slices were obtained from mice, within 48hrs of their last allergen priming challenge, adapted from the protocol of (Akram et al., 2019). Briefly, mice were humanely killed by CO2 inhalation followed by cervical dislocation. The lungs were inflated with 2% low melting agarose (Fisher–BP1360) prepared in HBSS+ (Gibco–14,025) before lungs, along with the heart and trachea, were excised, washed in PBS, and the lobes separated. Individual lobes were then embedded in 4% low melting agarose and solidified on ice. 200micron thick slices were cut on a Leica VT1200S vibratome and washed and incubated in DMEM/F-12 medium supplemented with 10% foetal bovine serum (FBS) and antibiotics.
PFA fixed ex vivo lung slices were incubated for 1 hour at room temperature in blocking solution: PBS containing 0.1% triton X-100, 0.1% sodium azide, and 2% bovine albumin (BSA), before incubating overnight a 4°C at 1:100 in blocking solution for all primary antibodies used: mouse anti-Muc5ac (Abcam ab3649), rat anti-Ly-6G (Abcam ab2557), rabbit anti-Zfp36l1/l2 (Cell Signaling Technologies). Ex vivo lung slices were washed 3 × 30 min in PBS+0.5% Triton X-100) before incubating overnight at 4°C overnight with: 1:100 Alexa Fluor 488 goat anti-rabbit (Thermo Scientific - A11008) or anti-mouse (A32723) IgG, Alexa Fluor 568 goat anti-rabbit (A11011) or anti-mouse (A11004) IgG, or Alexa Fluor 647 goat anit-rabbit (A32733) or anti-mouse (A21235) IgG +1:250 Alexa Fluor 488, 568, or 647 Phalloidin (Thermo Scientific–A12379, A12380, A22287, respectively). Slices were washed 3 × 30 min in PBS+0.5% Triton X-100, stained with 1:1000 DAPI in PBS for 20 min, mounted in ProLong Gold (Invitrogen P36930), and imaged on a Nikon Eclipse Ti2 spinning disc confocal microscope with a 20X or ×40 objective.
Primary bronchial epithelial cells were cultured on collagen-coated 24 well plates and transfected employing Interferin (Polyplus) with an over-expression vector for Zfp36l1 (courtesy of Prof Mayr, Memorial Sloan Kettering Cancer Center, United States) and siRNAs against ZFP36L2. RNA was extracted using TRIzol following manufacturer’s instructions. RT was performed employing H-minus RT (ThermoFisher Scientific) and qPCR performed using Luna Universal Probe qPCR Master Mix (New England Biolabs) using TaqMan primers (ThermoFisher Scientific).
GraphPad Prism and R studio software was used for the generation of graphs and analysis of data. All packages are available at CRAN. Two group analyses were done employing one or two-tailed tests. Enrichment analysis was performed using binomial tests. Differential proportions in Figure 2 were done employing a Chi-Square test. In all cases, p < 0.05 *, p < 0.01 **, p < 0.001 ***. RNA-seq analysis was evaluated using the DESeq2 package for differential gene expression, for details please see (Rynne et al., 2022). Microarray analysis was performed using the limma package.
The datasets presented in this study can be found in online repositories. The names of the repository/repositories and accession number(s) can be found below: https://www.ncbi.nlm.nih.gov/geo/, GSE213495 https://www.ncbi.nlm.nih.gov/geo/, GSE76227.
The studies involving humans were approved Primary bronchial epithelial cells from Figures 5E,F were collected under REC 23/YH/0018. For all the rest of data we are using previously collected data that is publicly available. Biopsies belonging to the Wessex Severe Asthma Cohort were collected under REC 14/WM/1226 and 05/Q1702/165. The studies were conducted in accordance with the local legislation and institutional requirements. The participants provided their written informed consent to participate in this study. The use of animals for this study was approved by the Ethical Review Committee at King’s College London and the Home Office, United Kingdom. All animals were housed in the Biological Support Unit (BSU) located in New Hunt’s House at King’s College London. All experiments were carried out under project license number P9672569A and personal license number I0F9CA46A. The study was conducted in accordance with the local legislation and institutional requirements.
JR, EO-Z, and DB acquired, analyzed and interpreted the data; OZ performed experiments and analyzed the data; GD and VK contributed experimentally; DJ, MP, and JR contributed intellectually and/or financially; IA provided critical intellectual input; RM-N conceived and designed the work and analyzed data. JW performed the titrations and immunostainings of human bronchial biopsies. All authors contributed to the article and approved the submitted version.
This work was supported by King’s Health Partners (Challenge Fund to RM-N); AAIR Charity to RM-N; Asthma United Kingdom Centre Grant (G1000758) to RM-N; Huo Family Foundation Grant to RM-N; Wellcome Trust (221908/Z/20/Z) to JR; Medical Research Council United Kingdom (MR/S009191/1 and R151002 to MP); GSK (RM-N) and AstraZeneca (DJ and RM-N). For the purpose of open access, the author has applied a CC BY public copyright licence to any Author Accepted Manuscript version arising from this submission. The funders had no role in study design, data collection and analysis, decision to publish, or preparation of the manuscript.
We thank all the participants of the Wessex Severe Asthma Cohort (Immunohistochemistry Unit, University of Southampton) for his work immunostaining human bronchial biopsies.
RM-N has received consultancy fees from Roche outside the scope of this work.
The remaining authors declare that the research was conducted in the absence of any commercial or financial relationships that could be construed as a potential conflict of interest.
All claims expressed in this article are solely those of the authors and do not necessarily represent those of their affiliated organizations, or those of the publisher, the editors and the reviewers. Any product that may be evaluated in this article, or claim that may be made by its manufacturer, is not guaranteed or endorsed by the publisher.
The Supplementary Material for this article can be found online at: https://www.frontiersin.org/articles/10.3389/fcell.2023.1241008/full#supplementary-material
Akram, K. M., Yates, L. L., Mongey, R., Rothery, S., Gaboriau, D. C. A., Sanderson, J., et al. (2019). Live imaging of alveologenesis in precision-cut lung slices reveals dynamic epithelial cell behaviour. Nat. Commun. 10 (1), 1178. doi:10.1038/s41467-019-09067-3
Asher, M. I., Rutter, C. E., Bissell, K., Chiang, C. Y., El Sony, A., Ellwood, E., et al. (2021). Worldwide trends in the burden of asthma symptoms in school-aged children: global asthma network phase I cross-sectional study. Lancet 398 (10311), 1569–1580. doi:10.1016/S0140-6736(21)01450-1
Azim, A., Mistry, H., Freeman, A., Barber, C., Newell, C., Gove, K., et al. (2019). Protocol for the Wessex AsThma CoHort of difficult asthma (WATCH): a pragmatic real-life longitudinal study of difficult asthma in the clinic. BMC Pulm. Med. 19 (1), 99. doi:10.1186/s12890-019-0862-2
Bakheet, T., Hitti, E., and Khabar, K. S. A. (2018). ARED-plus: an updated and expanded database of AU-rich element-containing mRNAs and pre-mRNAs. Nucleic Acids Res. 46 (D1), D218–D20. doi:10.1093/nar/gkx975
Barbato, A., Turato, G., Baraldo, S., Bazzan, E., Calabrese, F., Panizzolo, C., et al. (2006). Epithelial damage and angiogenesis in the airways of children with asthma. Am. J. Respir. Crit. Care Med. 174 (9), 975–981. doi:10.1164/rccm.200602-189OC
Blackshear, P. J. (2002). Tristetraprolin and other CCCH tandem zinc-finger proteins in the regulation of mRNA turnover. Biochem. Soc. Trans. 30 (6), 945–952. doi:10.1042/bst0300945
Brion, C., Lutz, S. M., and Albert, F. W. (2020). Simultaneous quantification of mRNA and protein in single cells reveals post-transcriptional effects of genetic variation. Elife 9, e60645. doi:10.7554/eLife.60645
Bush, A. (2008). How early do airway inflammation and remodeling occur? Allergol. Int. 57 (1), 11–19. doi:10.2332/allergolint.R-07-155
Calven, J., Ax, E., and Radinger, M. (2020). The airway epithelium-A central player in asthma pathogenesis. Int. J. Mol. Sci. 21 (23), 8907. doi:10.3390/ijms21238907
Cassandri, M., Smirnov, A., Novelli, F., Pitolli, C., Agostini, M., Malewicz, M., et al. (2017). Zinc-finger proteins in health and disease. Cell Death Discov. 3, 17071. doi:10.1038/cddiscovery.2017.71
Chung, K. F., Wenzel, S. E., Brozek, J. L., Bush, A., Castro, M., Sterk, P. J., et al. (2014). International ERS/ATS guidelines on definition, evaluation and treatment of severe asthma. Eur. Respir. J. 43 (2), 343–373. doi:10.1183/09031936.00202013
Frey, A., Lunding, L. P., Ehlers, J. C., Weckmann, M., Zissler, U. M., and Wegmann, M. (2020). More than just a barrier: the immune functions of the airway epithelium in asthma pathogenesis. Front. Immunol. 11, 761. doi:10.3389/fimmu.2020.00761
Galloway, A., Saveliev, A., Lukasiak, S., Hodson, D. J., Bolland, D., Balmanno, K., et al. (2016). RNA-binding proteins ZFP36L1 and ZFP36L2 promote cell quiescence. Science 352 (6284), 453–459. doi:10.1126/science.aad5978
Gygi, S. P., Rochon, Y., Franza, B. R., and Aebersold, R. (1999). Correlation between protein and mRNA abundance in yeast. Mol. Cell Biol. 19 (3), 1720–1730. doi:10.1128/mcb.19.3.1720
Hardyman, M. A., Wilkinson, E., Martin, E., Jayasekera, N. P., Blume, C., Swindle, E. J., et al. (2013). TNF-alpha-mediated bronchial barrier disruption and regulation by src-family kinase activation. J. Allergy Clin. Immunol. 132 (3), 665–675. doi:10.1016/j.jaci.2013.03.005
Hekking, P. P., Loza, M. J., Pavlidis, S., de Meulder, B., Lefaudeux, D., Baribaud, F., et al. (2018). Pathway discovery using transcriptomic profiles in adult-onset severe asthma. J. Allergy Clin. Immunol. 141 (4), 1280–1290. doi:10.1016/j.jaci.2017.06.037
Holgate, S. T. (2008). The airway epithelium is central to the pathogenesis of asthma. Allergol. Int. 57 (1), 1–10. doi:10.2332/allergolint.R-07-154
Holgate, S. T., Wenzel, S., Postma, D. S., Weiss, S. T., Renz, H., and Sly, P. D. (2015). Asthma. Nat. Rev. Dis. Prim. 1 (1), 15025. doi:10.1038/nrdp.2015.25
Ishmael, F. T., Fang, X., Galdiero, M. R., Atasoy, U., Rigby, W. F., Gorospe, M., et al. (2008). Role of the RNA-binding protein tristetraprolin in glucocorticoid-mediated gene regulation. J. Immunol. 180 (12), 8342–8353. doi:10.4049/jimmunol.180.12.8342
Johnson, J. R., Wiley, R. E., Fattouh, R., Swirski, F. K., Gajewska, B. U., Coyle, A. J., et al. (2004). Continuous exposure to house dust mite elicits chronic airway inflammation and structural remodeling. Am. J. Respir. Crit. Care Med. 169 (3), 378–385. doi:10.1164/rccm.200308-1094OC
Kelly, R. S., Dahlin, A., McGeachie, M. J., Qiu, W., Sordillo, J., Wan, E. S., et al. (2017). Asthma metabolomics and the potential for integrative omics in research and the clinic. Chest 151 (2), 262–277. doi:10.1016/j.chest.2016.10.008
King, H. A., and Gerber, A. P. (2016). Translatome profiling: methods for genome-scale analysis of mRNA translation. Brief. Funct. Genomics 15 (1), 22–31. doi:10.1093/bfgp/elu045
Kuo, C. S., Pavlidis, S., Loza, M., Baribaud, F., Rowe, A., Pandis, I., et al. (2017). A transcriptome-driven analysis of epithelial brushings and bronchial biopsies to define asthma phenotypes in U-biopred. Am. J. Respir. Crit. Care Med. 195 (4), 443–455. doi:10.1164/rccm.201512-2452OC
Liu, J., and Cao, X. (2023). RBP-RNA interactions in the control of autoimmunity and autoinflammation. Cell Res. 33 (2), 97–115. doi:10.1038/s41422-022-00752-5
Liu, Y., Beyer, A., and Aebersold, R. (2016). On the dependency of cellular protein levels on mRNA abundance. Cell 165 (3), 535–550. doi:10.1016/j.cell.2016.03.014
Makita, S., Takatori, H., and Nakajima, H. (2021). Post-transcriptional regulation of immune responses and inflammatory diseases by RNA-binding ZFP36 family proteins. Front. Immunol. 12, 711633. doi:10.3389/fimmu.2021.711633
Martinez-Nunez, R. T., Rupani, H., Plate, M., Niranjan, M., Chambers, R. C., Howarth, P. H., et al. (2018). Genome-wide posttranscriptional dysregulation by MicroRNAs in human asthma as revealed by frac-seq. J. Immunol. 201 (1), 251–263. doi:10.4049/jimmunol.1701798
McErlean, P., Kelly, A., Dhariwal, J., Kirtland, M., Watson, J., Ranz, I., et al. (2020). Profiling of H3K27Ac reveals the influence of asthma on the epigenome of the airway epithelium. Front. Genet. 11, 585746. doi:10.3389/fgene.2020.585746
McMillan, S. J., and Lloyd, C. M. (2004). Prolonged allergen challenge in mice leads to persistent airway remodelling. Clin. Exp. Allergy 34 (3), 497–507. doi:10.1111/j.1365-2222.2004.01895.x
Mortimer, K., Lesosky, M., Garcia-Marcos, L., Asher, M. I., Pearce, N., Ellwood, E., et al. (2022). The burden of asthma, hay fever and eczema in adults in 17 countries: GAN phase I study. Eur. Respir. J. 60 (3), 2102865. doi:10.1183/13993003.02865-2021
Ortiz-Zapater, E., Bagley, D. C., Hernandez, V. L., Roberts, L. B., Maguire, T. J. A., Voss, F., et al. (2022). Epithelial coxsackievirus adenovirus receptor promotes house dust mite-induced lung inflammation. Nat. Commun. 13 (1), 6407. doi:10.1038/s41467-022-33882-w
Perotin, J. M., Schofield, J. P. R., Wilson, S. J., Ward, J., Brandsma, J., Strazzeri, F., et al. (2019). Epithelial dysregulation in obese severe asthmatics with gastro-oesophageal reflux. Eur. Respir. J. 53 (6), 1900453. doi:10.1183/13993003.00453-2019
Piccirillo, C. A., Bjur, E., Topisirovic, I., Sonenberg, N., and Larsson, O. (2014). Translational control of immune responses: from transcripts to translatomes. Nat. Immunol. 15 (6), 503–511. doi:10.1038/ni.2891
Pohunek, P., Warner, J. O., Turzikova, J., Kudrmann, J., and Roche, W. R. (2005). Markers of eosinophilic inflammation and tissue re-modelling in children before clinically diagnosed bronchial asthma. Pediatr. Allergy Immunol. 16 (1), 43–51. doi:10.1111/j.1399-3038.2005.00239.x
Ricciardi, L., Col, J. D., Casolari, P., Memoli, D., Conti, V., Vatrella, A., et al. (2018). Differential expression of RNA-binding proteins in bronchial epithelium of stable COPD patients. Int. J. Chron. Obstruct Pulmon Dis. 13, 3173–3190. doi:10.2147/COPD.S166284
Rufo, J. C., Madureira, J., Fernandes, E. O., and Moreira, A. (2016). Volatile organic compounds in asthma diagnosis: a systematic review and meta-analysis. Allergy 71 (2), 175–188. doi:10.1111/all.12793
Rynne, J., Ortiz-Zapater, E., Khooshemehri, P., Ponde, N. O., Bucca, G., Hesketh, A., et al. (2022). The RNA binding proteins ZFP36L1 and ZFP36L2 modulate transcriptional and post-transcriptional genome-wide effects of glucocorticoids. bioRxiv.
Salerno, F., Engels, S., van den Biggelaar, M., van Alphen Fpj, , Guislain, A., Zhao, W., et al. (2018). Translational repression of pre-formed cytokine-encoding mRNA prevents chronic activation of memory T cells. Nat. Immunol. 19 (8), 828–837. doi:10.1038/s41590-018-0155-6
Schofield, J. P. R., Burg, D., Nicholas, B., Strazzeri, F., Brandsma, J., Staykova, D., et al. (2019). Stratification of asthma phenotypes by airway proteomic signatures. J. Allergy Clin. Immunol. 144 (1), 70–82. doi:10.1016/j.jaci.2019.03.013
Solberg, O. D., Ostrin, E. J., Love, M. I., Peng, J. C., Bhakta, N. R., Hou, L., et al. (2012). Airway epithelial miRNA expression is altered in asthma. Am. J. Respir. Crit. Care Med. 186 (10), 965–974. doi:10.1164/rccm.201201-0027OC
Sterne-Weiler, T., Martinez-Nunez, R. T., Howard, J. M., Cvitovik, I., Katzman, S., Tariq, M. A., et al. (2013). Frac-seq reveals isoform-specific recruitment to polyribosomes. Genome Res. 23 (10), 1615–1623. doi:10.1101/gr.148585.112
Vieira Braga, F. A., Kar, G., Berg, M., Carpaij, O. A., Polanski, K., Simon, L. M., et al. (2019). A cellular census of human lungs identifies novel cell states in health and in asthma. Nat. Med. 25 (7), 1153–1163. doi:10.1038/s41591-019-0468-5
Vogel, K. U., Bell, L. S., Galloway, A., Ahlfors, H., and Turner, M. (2016). The RNA-binding proteins Zfp36l1 and Zfp36l2 enforce the thymic beta-selection checkpoint by limiting DNA damage response signaling and cell cycle progression. J. Immunol. 197 (7), 2673–2685. doi:10.4049/jimmunol.1600854
Xiao, C., Puddicombe, S. M., Field, S., Haywood, J., Broughton-Head, V., Puxeddu, I., et al. (2011). Defective epithelial barrier function in asthma. J. Allergy Clin. Immunol. 128(3), 549–556. doi:10.1016/j.jaci.2011.05.038
Keywords: airway and lung cell biology, RNA binding protein, asthma, post-transcriptional control, tristetraprolin, ZFP36L1, ZFP36L2
Citation: Rynne J, Ortiz-Zapater E, Bagley DC, Zanin O, Doherty G, Kanabar V, Ward J, Jackson DJ, Parsons M, Rosenblatt J, Adcock IM and Martinez-Nunez RT (2023) The RNA binding proteins ZFP36L1 and ZFP36L2 are dysregulated in airway epithelium in human and a murine model of asthma. Front. Cell Dev. Biol. 11:1241008. doi: 10.3389/fcell.2023.1241008
Received: 15 June 2023; Accepted: 25 September 2023;
Published: 19 October 2023.
Edited by:
Chih-Yang Wang, Taipei Medical University, TaiwanReviewed by:
Feng Yuan, The University of Iowa, United StatesCopyright © 2023 Rynne, Ortiz-Zapater, Bagley, Zanin, Doherty, Kanabar, Ward, Jackson, Parsons, Rosenblatt, Adcock and Martinez-Nunez. This is an open-access article distributed under the terms of the Creative Commons Attribution License (CC BY). The use, distribution or reproduction in other forums is permitted, provided the original author(s) and the copyright owner(s) are credited and that the original publication in this journal is cited, in accordance with accepted academic practice. No use, distribution or reproduction is permitted which does not comply with these terms.
*Correspondence: Rocio T. Martinez-Nunez, cm9jaW8ubWFydGluZXpfbnVuZXpAa2NsLmFjLnVr
†Present Address: Jennifer Rynne, Centre for Inflammation Research, Institute for Regeneration and Repair, University of Edinburgh, Edinburgh, United Kingdom
Elena Ortiz-Zapater, Department of Biochemistry and Molecular Biology, Faculty of Medicine-IIS INCLIVA, University of Valencia, Valencia, Spain
‡These authors have contributed equally to this work and share first authorship
Disclaimer: All claims expressed in this article are solely those of the authors and do not necessarily represent those of their affiliated organizations, or those of the publisher, the editors and the reviewers. Any product that may be evaluated in this article or claim that may be made by its manufacturer is not guaranteed or endorsed by the publisher.
Research integrity at Frontiers
Learn more about the work of our research integrity team to safeguard the quality of each article we publish.