- 1Department of Biological Engineering, Massachusetts Institute of Technology, Cambridge, MA, United States
- 2Department of Mechanical Engineering, University College London, London, United Kingdom
- 3199 Biotechnologies Ltd., London, United Kingdom
- 4Northeastern University London, London, United Kingdom
- 5Belfer Center for Applied Cancer Science, Dana-Farber Cancer Institute, Harvard Medical School, Boston, MA, United States
The interplay between genetic transformations, biochemical communications, and physical interactions is crucial in cancer progression. Metastasis, a leading cause of cancer-related deaths, involves a series of steps, including invasion, intravasation, circulation survival, and extravasation. Mechanical alterations, such as changes in stiffness and morphology, play a significant role in all stages of cancer initiation and dissemination. Accordingly, a better understanding of cancer mechanobiology can help in the development of novel therapeutic strategies. Targeting the physical properties of tumours and their microenvironment presents opportunities for intervention. Advancements in imaging techniques and lab-on-a-chip systems enable personalized investigations of tumor biomechanics and drug screening. Investigation of the interplay between genetic, biochemical, and mechanical factors, which is of crucial importance in cancer progression, offers insights for personalized medicine and innovative treatment strategies.
Introduction: mechanobiology and cancer
Genetic transformations, biochemical communications and physical interactions are interconnected processes involved from the initial steps of tumor formation until the latter phases of cancer metastasis, a major cause of cancer related deaths. Metastatic cascade begins when the primary tumor cells gain aggressive and migratory phenotypes resulting in leaving the primary tumor (Figure 1A), invading the local tissue (Figure 1B) (Craene and Berx, 2013) and transmigrating through the endothelial barrier into the blood or lymphatic microvasculature (a process known as intravasation, Figure 1C) (Van Zijl et al., 2011). The latter steps of disease involve survival of cancer cells in blood circulation (Figure 1D) (Aceto et al., 2015; Reymond et al., 2013) and exit from the vessels at distal tissues (a process known as extravasation) to ultimately invade and colonize in the secondary sites (Figure 1E) (Nguyen et al., 2009). In addition to a number of unique genetic and biochemical factors associated with metastasis (Suresh, 2007; Kumar and Weaver, 2009; Wirtz et al., 2011), irregular mechanical alterations such as structural, morphological and stiffness changes, in both cells and the extracellular environment, play a significant role during all stages of cancer initiation and dissemination. For example, the primary tumor is characterized by biochemically and mechanically altered environment that results from oncogenic mutations and epigenetic changes disrupting key physiological cellular processes such as cell cycle (Spill et al., 2016). Taking advantage of the abnormal mechanical properties of most tumors, palpation has been a conventional diagnostic method to assess the stiffness of tumor within the surrounding soft tissue. The disease stage is linked with tumor stiffness as monitored by in vivo MRI elastography or through ex vivo atomic force microscopy (Lopez et al., 2011). On the other hand, during tumor growth, the mechanical alterations in tumor environments trigger complex bio-mechanical signalling pathways, which may ultimately enhance the ability of tumor cells to acquire a malignant phenotype. The malignant evolution of cancer cells de-regulates cell-cell and cell-extracellular matrix (ECM) adhesions and cytoskeletal remodeling leading to abnormal tumor cell morphology, enhancement of metabolism (Torrino et al., 2021), and migratory behavior which facilitate invasion at the primary site and ultimately leads to intravasation. Following intravasation, circulating tumor cells (CTCs) must resist the mechanical forces in the bloodstream to survive and reach the secondary organ. Once at the secondary site, tumor cells must exert forces and undergo morphological changes to escape from the vasculature and invade the ECM of the distal organ.
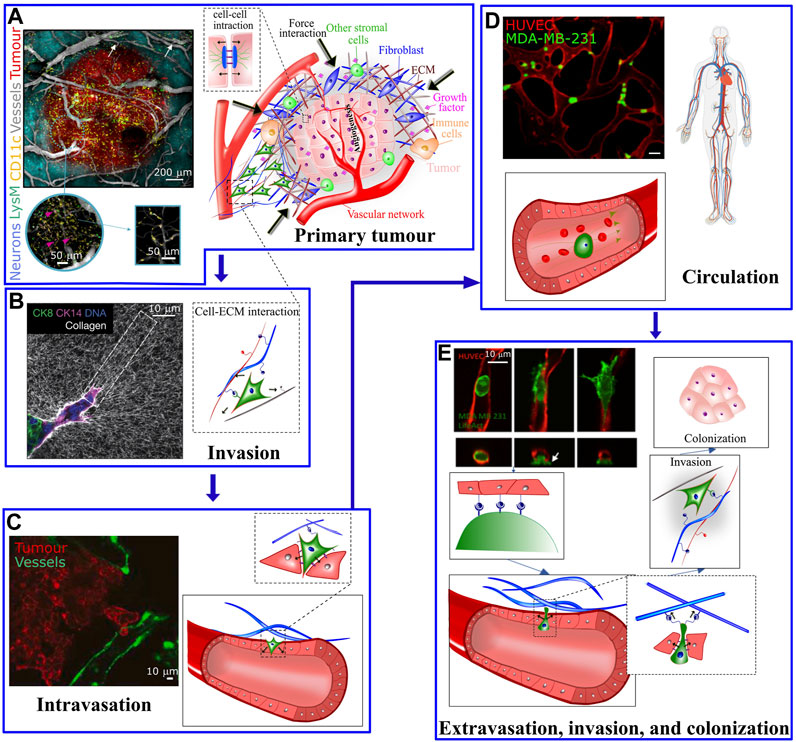
FIGURE 1. (A) The tumour microenvironment at the primary site is very complex; Hypoxic pathways and signaling with other supporting cells, including immune cells, mesenchymal stromal cells and fibroblasts activates endothelial cells to form vascular network in the tumor and surrounding areas. The left panel shows a five-color intravital two-photon image acquired from a bevacizumab-treated triple transgenic mouse. Arrows show the LysM-EGFP+ cells present in the vessels. The cells colored in pink refer to double-labelled cells (pink arrows) (CD11c-EYFP+/LysM-EGFP+) inside the tumor [taken from Soubéran et al. (2019)]. (B) At the primary tumor site, factors including matrix pore size, density, stiffness, and fiber orientation play a role in modulating the migratory and invasive capabilities of tumor cells. Left panel: Confocal reflection microscopy reveals that invasive organoids align the collagen network to facilitate tumor invasion (taken from Koorman et al. (2022)]. (C) Tumor cells cross the endothelial barrier to enter the circulation. (D) During circulation tumor cells are exposed to shear stress from the blood flow (left panel in C and D are taken from Agrawal et al. (2022) and Beyer et al. (2021), respectively). (E) To exit the circulation, tumor cells pass through the endothelial barrier. The process of transendothelial migration involves significant tumor cell deformations and generation of cellular forces that may activate downstream mechanosensitive pathways. Upper left image is taken from Chen et al. (2017). White arrow depicts actin-rich tumor cell protrusion passing through the endothelium.
These mechanical interactions during the metastatic journey of tumor cells highlight the importance of biomechanics in cancer dissemination (Kumar and Weaver, 2009; Moeendarbary and Harris, 2014; Malandrino et al., 2018a). Here we describe the crucial interplay between mechanics and biology during cancer initiation and progression together with recent conceptual and technological advances in mechanobiology which have led to a remarkable progress in leveraging cancer biomechanics to develop novel therapeutic strategies.
Mechanical forces during tumor initiation and growth
Even in a seemingly static tissue, the extracellular microenvironment exerts forces to the cells. Such forces are originated from adjacent cells, the interstitial fluid, or interaction with the ECM, and are sensed via cellular mechanosensitive receptors that regulate major cellular functions such as the cell cycle, morphogenesis, and migration (Wang et al., 1993). Indeed, the bio-mechanical interactions between intracellular biological machinery and the surrounding microenvironment, through mechanosensitive receptors, create a normal physiological condition. However, in the setting of cancer, genetic and epigenetic (Burdziak et al., 2023), including biochemical and physical, perturbations in intracellular or extracellular environments, disrupt the cellular homeostasis leading to dysplasia and in most cases to the formation of a solid tumor. During tumor growth, the aforementioned normal forces are disrupted as a result of mechanical stresses originated from aberrant homeostasis, excessive growth and tissue dysplasia (Jain et al., 2014). Tumor growth is known to generate compressional forces that perturb the interstitial space, the ECM and the flow in the vasculature. In turn, perturbations in the interstitial space may cause accumulation of growth factors and cytokines that facilitate tumor growth; whereas disruption in the mechanical properties of ECM and flow may alter cellular behaviour (Malandrino et al., 2018b).
It is now evident that the mechanical aspects such as ECM stiffness are critical in regulating a wide range of cellular behaviour. For example, in the context of stem cell differentiation, human mesenchymal stem cells preferentially differentiate into neurons or osteocytes when cultured on substrates with stiffnesses matching brain or bone tissues respectively (Saha et al., 2008). In the setting of cancer transformation, when epithelial cells are cultured on a compliant substrate, normal cells show a decrease in the rate of DNA synthesis and an increase in the rate of apoptosis while transformed cancer cells maintain their growth and apoptotic characteristics (Wang et al., 2000). Furthermore, transformed cells exert higher traction forces compared to non-transformed cells. Consequently, the increase in ECM stiffness and the extent of compression can lead to activation and increased expression of Rho GTPase and downstream effectors as well as high levels of extracellular signal-regulated kinases (ERK) activity that facilitate the process of epithelial-mesenchymal transformation (EMT) (Klein et al., 2009).
The impact of physical interactions in malignancy and invasion
Biochemical and biophysical characteristics of the ECM influences cell migration (Barenholz-Cohen et al., 2020) through variations in growth factors or chemokines (chemotaxis), stiffness (durotaxis), ligand density (haptotaxis), and topographical organization (contact guidance) to direct cells to target destinations (Wang et al., 2019). Recent advances in intravital imaging have revealed that cells can adopt a diverse set of migration strategies involving migration as single cells or collective strands, transitions between mesenchymal, epithelial, and amoeboid migration modes, deformation of the cell body and nucleus to squeeze through matrix pores, and remodeling of matrix structure to bypass the physical barriers presented by the ECM (Wang et al., 2019) (Figure 1B). Furthermore, heterogeneity of stiffness in tumor microenvironment, triggered by matrix remodeling can mechanically guide the tumor cells directional migration (Zhang et al., 2020).
EMT is a critical process in metastasis and involves loss of epithelial characteristics (Bocci et al., 2019), resulting from downregulation of cell-cell adhesion strength (for example, through loss of E-cadherin and cytokeratin) and acquisition of a mesenchymal phenotype via activation of migratory processes (for example, through upregulation of vimentin and N-cadherin). Taken together, the EMT process disrupts cellular force balances and polarity leading to morphological changes and detachment of tumor cells from the tumor epithelium (Thiery and Sleeman, 2006; Kalluri and Weinberg, 2009; Chaffer and Weinberg, 2011). By developing a high-throughput screening assay to track displacements generated by 3D cultured multicellular clusters, Leggett et al. (2020) showed a successive reduction in protrusive and circumferential tractions during EMT. Subsequently, the modulation of cellular shape and forces in combination with mechanisms favouring migration including proteolytic (matrix metalloproteinase), adhesive, protrusive (invadopodia) and contractile processes, promote invasion of cancer cells (Wolf and Friedl, 2009). Therefore, to facilitate their three-dimensional motility, cancer cells navigate through the ECM via invadopodial protrusions, balance cell-ECM adhesion, and apply contractile forces to squeeze through ECM pores and ultimately digest and remodel the ECM via force application and matrix metalloproteinase secretion.
The generation of a new tumor-specific vasculature that aids tumor growth, is concomitant with tumor development and transformation to malignancy and facilitates the escape of tumor cells into the circulation. In addition to biochemical signals (Chen et al., 2021), physical factors such as mechanical, hydrodynamical, and collective processes (Rieger and Welter, 2015) influence the generation and architecture of tumor-specific vasculature. Indeed, the growth of an avascular tumor is limited to a critical size (<1 mm) because of the inability of diffusion mechanisms to supply oxygen and soluble factors into the tumor core. This phenomenon results in the development of a necrotic/hypoxic region at the tumor core, which is surrounded by a highly proliferative outer rim. Therefore, robust vascularization mechanisms are recruited to boost tumor growth in order to enhance delivery of different factors, such as oxygen. Vascularization of the tumor and surrounding areas is initiated and maintained through the recruitment and activation of endothelial cells, mainly triggered by hypoxic pathways (Madsen et al., 2015), and signaling with other supporting cells in the tumor microenvironment. These include, immune cells, mesenchymal stromal cells and fibroblasts (Figure 1A) (Weis and Cheresh, 2011). The newly developed vessels perturb the normal architecture of blood and lymphatic networks and induce an aberrant interaction between the fluid and solid phases within the tumor leading to high levels of the interstitial fluid pressure and the lack of gas and nutrients (Figure 1A) (Koumoutsakos et al., 2013). Due to such a chemically and mechanically disordered tumor environment, direct drug delivery to solid tumors is often inefficient (Minchinton and Tannock, 2006). Following tumor vascularization, the combination of protracted tumor cell proliferation, continuous genetic transformations, angiogenesis and activation of bio-mechanical signaling pathways promote malignancy, invasion, and metastasis (Polyak and Weinberg, 2009; Wirtz et al., 2011; Mierke, 2019; Yang et al., 2020; Dou et al., 2022).
Biomechanics of cancer cell during intravasation, circulation and extravasation
Intravasation and extravasation
Intravasation describes the process by which individual or multiple tumor cells migrate away from the primary tumor site, cross the endothelial barrier to gain entry into the circulation (Figure 1C). Similarly, extravasation is the sequences of events where CTCs exit the bloodstream and invade the parenchyma of a secondary metastatic site. The efficiency of these events may be modulated by the external physical microenvironment that drives intracellular signaling, as well as the cell’s ability to perturb its inherent mechanical properties.
External environment
To enter or leave the circulation, tumor cells must migrate across dense parenchymal tissue, which is composed of a network of highly cross-linked extracellular matrix and stromal cells. On average, pore sizes are on the scale of nanometers (<1 micron) (Laudani et al., 2020) while tumor cell size ranges 5–30 microns in diameter, suggesting that both matrix alterations and extreme cell deformation are required. One of the rate limiting steps of migration is the deformation of the tumor cell nucleus, which is approximately 5–10 times stiffer than the cytoplasm (Lammerding, 2011). As such, the matrix pore size and the deformability of the interstitial spaces are the factors that might dictate migration. Tumor cells are known to secrete matrix metalloproteinase (MMPs) like MMP2 to enable collagen proteolysis (Leong et al., 2014; Deryugina and Quigley, 2015; Micalet et al., 2022) and are shown to localize MT1-MMP at the leading edge of protrusion during migration, indicating an active role of degradation. Notably, the majority of vasculature is surrounded by a dense network of basement membrane (BM) proteins such as collagen IV and laminin, requiring further degradation processes like the secretion of gelatinases (e.g., MMP-9) prior to entering circulation (Maity et al., 2011; Sounni et al., 2011). Sikic et al. (2022) by tracking force-induced displacements and measuring local viscoelastic properties of Matrigel via magnetic micro-rheology, quantified tumor cell generated forces during invasion towards basement membrane in a 3D culture environment. They showed that protrusions extension involves stepwise increases in forces ranging from piconewtons to nanonewtons being exerted every few minutes. While matrix degradation could decrease the burden for the cell to undergo severe deformation, recent work has also shown the ability of tumor cells to exhibit substantial morphological changes in the absence of matrix loss (Wolf et al., 2003; Voura et al., 2013). This may involve large deformations of the tumor cell nucleus, which depends on mechanical properties of the nuclear lamina and organization of chromatin (Cao et al., 2016). In this regard, it is known that linker proteins (such as SUN domain-containing proteins) between nuclear and cytoplasmic (LINC) complexes can facilitate the proper positioning of the nucleus relative to the cell body to allow motility through narrow constrictions (Kraning-Rush et al., 2012; Denais et al., 2016).
The endothelium presents yet another barrier for tumor cell migration. Microvessels are lined with a single layer of endothelial cells connected to each other through junctional proteins such as VE-cadherin and CD31 (PECAM 1) that are responsible for the tight regulation of soluble factor transport between the blood and the surrounding tissue. The open gaps between endothelial cells are typically less than a few microns (McEvoy et al., 2022), suggesting that the transmigration of tumor cells may involve deformation of both tumor and endothelial cells. It has been shown that tumor cells can secrete inflammatory factors such as TNF-α which mediate endothelial junctional permeability and create discontinuities in the barrier to facilitate transmigration (Zervantonakis et al., 2012). Furthermore, the ability of tumor cells to anchor onto the endothelium through tumor-EC adhesion proteins such as integrins is critical for the generation of forces that allow translocation. Since integrins provide a connection between the ECM and the actin cytoskeleton, this mechanosensitive protein coupling and activation may lead to downstream intracellular signaling, thus determining the extent of intracellular forces required to maintain or obtain a certain cellular morphology. Also, BM mechanics at the primary and secondary tumour site plays a critical role in cancer progression, independent of tumour-mediated alterations; Reuten et al. showed that the BM stiffness is regulated through Netrin-4 in a laminin-binding-dependent manner by diluting laminin ternary node complexes. The more Netrin-4 molecules are present, the softer the laminin network and the more resistant it is to metastases formation (Reuten et al., 2021).
Influence of extracellular physical signals on intracellular environment
Interestingly, it has been found that cancer cells are consistently softer than their non-cancerous counterparts (Rianna et al., 2020), and that the softening correlates positively with metastatic potential (Xu et al., 2012). The softer cytoplasm of more aggressive tumor cell lines is often correlated with a loss of cytoskeletal organization. Since the ability to migrate through dense matrix and endothelial barriers likely depends on the intrinsic mechanical properties of the cells, an increase in tumor cell compliance may act in its favor. Additionally, external stimuli including the presence of interstitial flow, ECM stiffness, 2D or 3D dimensionality (Galarza et al., 2020), and availability of binding sites for cell surface receptors (Wei et al., 2015) may influence the nature and deformability of the cell membrane and cytoskeleton. For instance, features that appear to be important for 2D motility—focal adhesion, stress fibres, broad lamellipodia—are largely absent for models of 3D invasion, particularly in invasive cancer cells (Shibue and Weinberg, 2009; Mierke, 2013). On the other hand, several mechanosensory proteins, such as vinculin, play an important role in tumor cell migration within reconstituted 3D matrices, but not in 2D motility (e.g., on plastic culture dishes coated with the same ECM proteins).
Circulating tumor cells and shear stress
After entering the circulation, tumor cells are exposed to a variety of hemodynamic forces of flowing blood and collision with other cell types (Marrella et al., 2021). For example, shear flow can influence the motility of tumor cells and determine the likelihood that intercellular adhesions with the endothelium or circulating immune cells can occur. The average shear stresses a CTC experiences is estimated to be around 1–4 dyn/cm2 in the venous circulation, and 4–30 dyn/cm2 in arterial circulation. This is comparable to the levels of shear stress that cartilage and bone cells are subjected to on a daily basis from normal interstitial fluid movement (∼30 dyn/cm2), and to the level of renal epithelial cells undergo during hypertension (∼1 dyne/cm2) (Nagrath et al., 2007; Aceto et al., 2015; Au et al., 2016). Thus, it is highly possible that shear stress levels experienced by CTCs are significant enough to induce mechanotransductive cellular responses.
Additionally, shear flow can induce the deformation of tumor cells and influence their viability. For instance, CTCs migration in groups exhibit higher survival rates due to protection from deleterious shear stresses (Au et al., 2016). Thus, only tumor cells that overcome the effects of fluid shear stress and escape immunosurveillance can adhere to the vasculature and enter the tissues of the secondary site.
Tumor cell extravasation is thought to first require the firm adhesion and arrest of tumor cells on the endothelium. There are two mechanisms of tumor cell arrest (Craene and Berx, 2013): physical occlusion in capillaries narrower than the diameters of the CTC, and (Van Zijl et al., 2011) active adhesion between endothelial-tumor cell ligands/receptors. In vivo, tumor cells have mostly been observed to arrest in small capillaries of the brain and lung, suggesting the possibility of physical occlusion. Integrins and selectins are critical to determine tumor cell retention in several organs such as the lung and the liver, indicating that active cell adhesion may be involved in addition to pure physical occlusion. The increased ability of tumor cells to arrest on the endothelium may offer higher probability of exiting the bloodstream.
Adhesion to blood vessels is followed by cancer cell transendothelial migration (TEM). Depending on the vascular bed and tumor cell types, two mechanistically different routes are possible in vivo: transcellular (migration of CTCs through the EC body) and paracellular (moving between ECs junctions) (Herman et al., 2019). The latter is the most frequent accessed way for cancer cells to penetrate the vascular wall in vitro. This process involves many chemokines, receptors and intracellular signaling molecules leading to significant cytoskeletal changes of endothelial and cancer cells (Reymond et al., 2013). Additionally, Javanmardi et al. showed that mechanical properties of ECM, such as stiffness and porosity, regulate cell generated forces through mediating RhoA activity (Javanmardi et al., 2023). Furthermore, complex push–pull forces generated by cancer cell actin-rich protrusions are essential to initiate and drive transendothelial migration (Javanmardi et al., 2023).
Interactions with other cells in the blood have also been shown to be critical. For example, adhesion to platelets through tumor integrin αvβ3 can promote tumor-platelet aggregation, which leads to the protection of tumor cells from shear flow. Additionally, platelets can secrete pro-extravasation factors such as platelet-derived nucleotides, which act to increase endothelial permeability and facilitate the transmigration of tumor cells (Labelle et al., 2011; Schumacher et al., 2013). Interactions with circulating neutrophils can increase tumor cell retention and extravasation via neutrophil CD11b, endothelial ICAM-1; and the success of these interactions decline with increasing shear rates resulting from blood flow (Peng et al., 2007; Huh et al., 2010).
Probing cancer biomechanics and emerging technologies
Recent technological advances provide a better insight on the biomechanical phenomena during cancer cell dissemination by probing morphological changes, mechanical properties and force interactions between cells and the extracellular environment. While advanced light microscopy techniques such as optical super-resolution imaging offer unprecedented information about the nano-scale molecular organisation of the cell (Colin-York et al., 2019a) and its link to cellular morphology and function, they are mostly limited to isolated two-dimensional (2D) cultures in which a monolayer of cells are grown on flat plastic or glass substrates. To access high resolution tumor pathophysiology in vivo, intravital imaging has revealed some fascinating morphological changes and cell migration processes associated with invasion and intravasation (Jain et al., 2002). Three dimensional (3D) interactions among cells and the extracellular environment are unique at all stages of metastasis and cannot be recapitulated in conventional 2D cultures. On the other hand, under in vivo conditions, it is extremely difficult to fully follow the temporal evolution of 3D interactions and run parametric studies to dissect the role of different factors. Therefore, advanced 3D engineered models such as microfluidic based approaches, have been used in recapitulating key biomechanical features that are specific to each step of metastasis (Chen et al., 2017; Agrawal et al., 2022; Straehla et al., 2022; Watson et al., 2022) and to quantify tumor cell secretions at the single cell resolution over a long period of culture (Hassanzadeh-Barforoushi et al., 2020). Together, they have allowed the investigation of a variety of cellular events with high spatiotemporal resolution and under tunable environments (Malandrino et al., 2018a).
The measurement of mechanical properties is critically important in cancer biomechanics. Mechanical changes and alternations in the composition, architecture and stiffness of tumor microenvironment regulate tumor growth, transformation to malignancy, and invasion (Pickup et al., 2014) being therefore critical aspects in cancer progression (Cox and Erler, 2011; Lu et al., 2012; Popova and Jücker, 2022). For instance, tumor-associated collagen exhibits specific features due to variation in fiber orientation and collagen deposition. Notably, three distinct tumor-associated collagen signatures (TACS) have been identified in relation to human breast cancers: the accumulation of collagen fibers around small tumors (TACS-1), the straighten fibers in the vicinity of non-invasive tumors (TACS-2) and the perpendicular alignment of collagen fibers at the tumor periphery (TACS-3) (Warli et al., 2023). Such irregularities have been visualized through microscopy techniques, such as second harmonic generation, which highlights the structural transformations in collagen fibers (Figure 2A). Furthermore, aberrant mechanical properties of the tumor environment can be measured as a diagnostic tool (Plodinec et al., 2012). The mechanical properties of the tumor per se, as well as the micro-mechanical features of tumor environment have been characterized through a number of in vivo, ex vivo, and in vitro based assays at micro-nano- scale resolutions. As an alternative to conventional palpation methods, in vivo elastography measurements, such as ultrasonography (Wells and Liang, 2011), optical coherence tomography (Kennedy et al., 2015), and magnetic resonance imaging (Venkatesh et al., 2008), revealed significant stiffening of tumor tissues particularly in malignant tumors (Ramião et al., 2016; Ishihara and Haga, 2022). For example, pancreatic cancer tissue exhibits greater stiffness of ∼6 kPa compared to the 1–3 kPa range observed in normal pancreatic tissue (Itoh et al., 2016). Lung solid tumors register stiffness levels of ∼20–30 kPa, whereas normal lung parenchyma stiffness typically ranges from 0.5 to 5 kPa (Miyazawa et al., 2018). For mammary tissues, cancerous tissue displays a significantly higher stiffness of around 4 kPa, in stark contrast to the ∼0.2 kPa stiffness found in normal mammary tissue (Paszek et al., 2005). Elastography-based ultrasound techniques offer a non-invasive and real-time approach to assessing tissue stiffness, enhancing the diagnostic accuracy, and providing valuable information for personalized cancer treatment (Figure 2B). Interestingly, Golatta et al. showed that adding combined shear wave elastography and strain elastography to routine B-mode breast ultrasound could help reduce the number of unnecessary biopsies in breast diagnostics by ∼35% (Golatta et al., 2022). However, increased stiffness signature, that has been a well-known characteristic of solid tumors, alone has a limited prognostic power. The prognostic potential enhances when examining other rheological properties of the tumor microenvironment through the application of in vivo multifrequency magnetic resonance elastography (MRE). Employing this technique, Sauer et al. (2023) outlined a roadmap for prognosis of a tumor’s aggressiveness and metastatic potential based on stiffness, fluidity, spatial heterogeneity, and texture of the tumor (Figure 2C). They showed that cancer progression is accompanied by tissue fluidization, where portions of the tissue can change position across different length scales.”
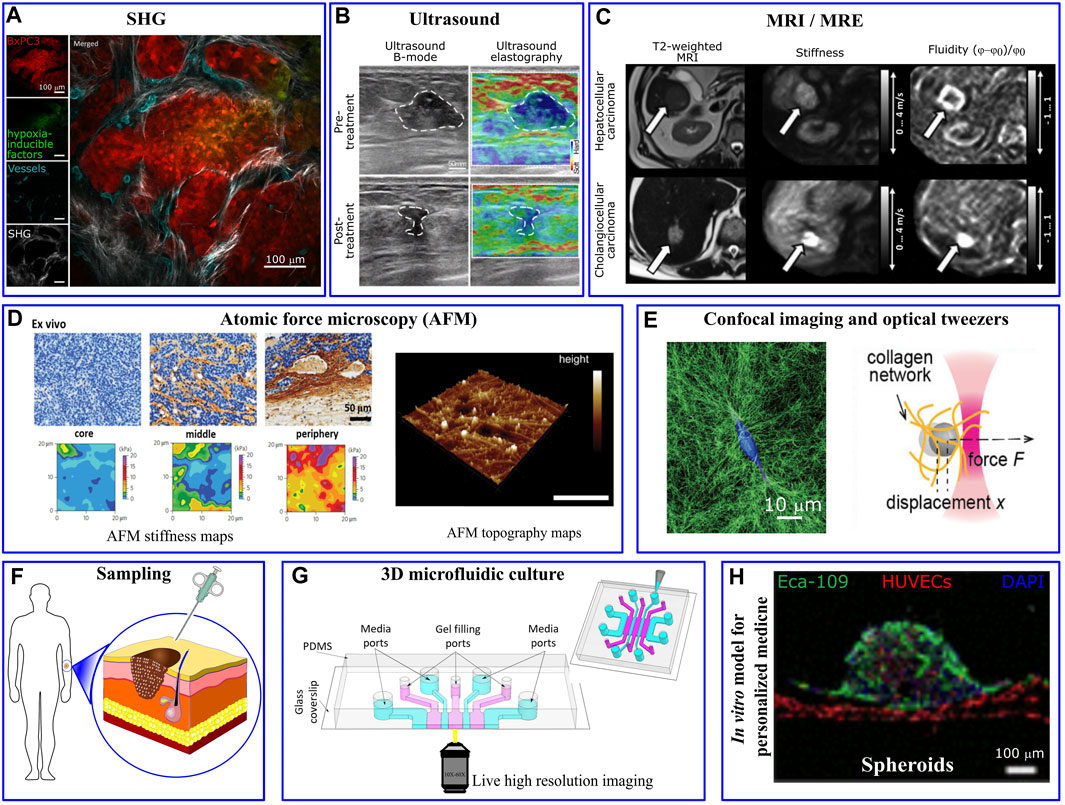
FIGURE 2. Mechanical/structural properties of the tumor microenvironment and microfluidic platform in personalized medicine. (A) Different tumor associated collagen signatures have been captured via in vivo second harmonic generation (SHG) imaging of mouse pancreatic tumor. Irregularly shaped and newly formed vessels are observed in the inner regions of the tumor, taken from Samuel et al. (2023). (B) Ultrasound and (C) Magnetic resonance elastography show various levels of tumor stiffness that can inform the planning of resection procedures, taken from Liu et al. (2023) and Sauer et al. (2023), respectively. (D) High resolution AFM stiffness maps of mouse mammary tumor shows highly heterogeneous tumor mechanical properties with a soft signature at the tumor core and significantly stiffer periphery. Taken from Plodinec et al. (2012). AFM topographic maps provides helpful information about matrix architecture (taken from Budden et al. (2021)). (E) Single MDA-MB-231 cancer cell embedded within collagen-I gel significantly deformed and remodeled collagen fibres imaged via confocal reflection microscopy and changed the gel stiffness quantified via optical tweezers. Taken from Han et al. (2018). (F) Cells that are derived directly from a patient are usually scarce. (G) The few isolated cells can be 3D-cultured in a biomimetic microfluidics device for drug discovery, personalized drug screening, or to investigate the impact of biomechanical/biochemical factors on cellular behaviour (taken from Whisler et al. (2023)). (H) Perfusable vascularized tumor spheroid-on-a-chip model incorporates patient’s isolated cells as a personalized medicine approach (taken from Hu et al. (2022)).
Alterations in cellular and extracellular composition/structures contribute to the increased tumor stiffness. For example, excessive proliferation of cancer cells and activation of stromal cells such as cancer associated fibroblasts (CAFs) (Calvo et al., 2013), induce ECM remodeling (Bertero et al., 2019); whereas tumor growth induces solid stress within the tumor itself (Nia et al., 2017; Nia et al., 2020). The presence of growth-induced solid stresses in tumors had been under suspicion for some time, as these stresses were largely estimated using mathematical models (Stylianopoulos et al., 2012). However, in the past decade various experimental techniques have emerged to directly measure such stresses. For instance, Campàs et al. (2014) introduced incompressible oil microdroplets into 3D cell aggregates and live embryonic tissue to assess the local anisotropic forces. Dolega et al. (2017) and Lee et al. (2019) employed compressible polyacrylamide microdroplets to quantify both radial and circumferential components of solid stress within spheroids. Additionally, L et al. (2017) showed that CAFs promote tumor invasion and metastasis through exerting mechanical forces on cancer cells which are mediated via adhesion proteins involving N-cadherin at the CAF membrane and E-cadherin at the cancer cell membrane. On the other hand, tumor vascularization leads to aberrant interactions between the blood flow that infiltrates and surrounds the tumor and the increased interstitial fluid pressure (Jain et al., 2014). To dissect the contribution of the different components of tumor microenvironment, high-resolution mechanical measurement techniques, such as AFM (atomic force microscopy), have been widely applied. AFM nanomechanical indentation tests on tumor slices showed a soft mechanical signature within the tumor core, where cancer cells are abundant, while the adjacent peripheral regions are stiffened mostly due to collagen alignment (Figure 2D) (Laklai et al., 2016; Stylianou and Stylianopoulos, 2016).
At the single cell level, several experimental methods such as magnetic twisting cytometry, magnetic and optical tweezers (Figure 2E) and AFM have been utilized to perturb small regions of the cell and characterize mechanical properties, such as stiffness, of isolated cancer cells. Furthermore, Kim et al. (2018) developed a multi-parametric single-cell-analysis method in which different cell lines were transported through a microfluidics channel to measure their mechanical properties. By defining a whole-cell deformability index, they showed that malignant and non-malignant cell lines have different mechanical signatures. Traction Force Microscopy has been widely utilized to observe force interaction of cells and measure cell-generated forces (Colin-York et al., 2019b; Javanmardi et al., 2021; Li et al., 2021). This force probing technique is based on imaging the cell-induced displacement of fiducial markers, embedded/targeted within extracellular environment, and use the computational procedure to back-calculate the cellular forces that generated the displacements. To streamline and simplify the computation procedures involved in TFM, Barrasa-Fano et al. (2021) developed TFMLAB, a MATLAB software package for 4D TFM. Interestingly, while highly metastatic cancer cells exhibited a softer phenotype (∼0.5 kPa) compared to non-metastatic cells (∼2 kPa), they generate stronger forces (Kraning-Rush et al., 2012; Kristal-Muscal et al., 2013) (∼300 nN for MDA-MB231 vs ∼150 nN for MCF10A) (Cross et al., 2007; Gal and Weihs, 2012)”, allowing them to squeeze through 3D ECM and metastasize more readily (Coughlin et al., 2013). Also, in the cluster level, contractile forces generated by tumor spheroid have been measured and normalized in a scale-independent manner (Mark et al., 2020).
The mechanical behavior of ECM, cancer cells, and tissues are often assumed to be elastic solids for simplicity and their time-dependent mechanical responses are frequently overlooked. However, it has been demonstrated that ECM exhibits a more complex mechanical behavior, including viscoelasticity, mechanical plasticity, and nonlinear elasticity (Chaudhuri et al., 2020) and indeed, the viscoelasticity of ECM plays a fundamental role in the progression of cancer (Mierke, 2021). Additionally, cellular behavior is not affected by only the mechanics of solid compartment of the ECM; viscosity of the extracellular fluid has also been shown to facilitate tumor cell migration and dissemination on 2D surfaces and in 3D spheroids (Bera et al., 2022). In addition to ECM, cancer cells themselves typically show a lower levels of viscosity and membrane tension compared to healthy cells (Ren et al., 2023). Moreover, cytoplasm of the living cells also has been shown to behave as a poroelastic material (Moeendarbary et al., 2013) with an enhanced diffusion coefficient for cancer cells (Ren et al., 2023). At the tissue level, higher levels of viscos (loss) modulus in cancerous tissues (Deptuła et al., 2020) has improved the diagnostic accuracy and capabilities in ultrasound (Nabavizadeh et al., 2019) and MRE techniques (Reiter et al., 2022).
Mechanobiology and challenges with translation
Modulation of physical properties as a path of pharmacological intervention
It is now accepted that the ability of a cancer cell to successfully invade the surrounding ECM and cross endothelial barriers during metastasis requires a finely regulated set of mechanical properties. Thus, any alterations to either the surrounding physical environment or the cell cytoskeletal organization could potentially become a target of therapeutic intervention.
For example, neutralization of matrix degrading MMPs may increase steric hindrance and decrease the ability of tumor cells to cross ECM during the invasion phase. While some tumor cells appear to be resistant to these perturbations, being able to migrate in an amoeboid manner (without degradation), inhibition of the matrix-degradation may prove to be effective in other types of cancers. Nuclear deformation is also seen to be a rate-limiting factor in migration through narrow constrictions (Cao et al., 2016). Thus, overexpression of proteins such as lamins that maintain nuclear stiffness, could hinder large nuclear deformations and delay the rate at which tumor cells invade (Wirtz et al., 2011; Díaz de la Loza et al., 2017).
Once tumor cells have spread into the circulation, it might still be possible to intervene at the stage of intravascular adhesion and arrest. For instance, perturbation of tumor-endothelial adhesion molecules such as E-selectin, CD44, PODXL, VCAM1 or ICAM1 could decrease the rate of heterotypic interaction under shear flow, resulting in lower tumor cell retention rates in the circulation and limitation of metastatic seeding at the distant site (Rosette et al., 2005; Zen et al., 2008). Similarly, targeting tumor-interacting adhesion proteins on immune cells like platelets and leukocytes could yield comparable anti-metastatic effects. Care, however, must be taken in all cases to minimize the perturbation to the normal homeostatic functions of the non-cancer cells involved.
It is still a matter of debate whether the physical characteristics of cancer cells, such as deformability and stiffness, are conserved through generations, or whether these are developed in response to heterogeneous extracellular mechanical and biochemical cues, spread over time and space. Whether these physical properties are inherited or acquired throughout different stages of metastasis, it might be possible to alter them, either through pharmacological inhibition or through the activation of proteins affecting cell mechanics. Together, it may be possible to exogenously achieve a set of optimal mechanical microenvironmental conditions (i.e., cell stiffness, matrix density and pore size, interstitial fluid forces) such that the likelihood of proceeding through the metastatic cascade is lowered.
Lastly, applicability of targeting mechanics is a relatively new approach compared to genes or molecular biomarkers methods and therefore most of the clinical interventions leading to mechano-therapeutics are still in the trial phase. Jain et al. (2014), Huang et al. (2021), Jiang et al. (2022), and Di et al. (2023) compiled a list of such drugs; among which it is worth mentioning: Cilengitide, a selective αvβ3/αvβ5 integrin inhibitor that has been assessed in phase III clinical trial for treating glioblastoma or GB 2064 (formerly PAT-1251) and a LOXL2 inhibitor that lowers collagen accumulation and ECM stiffness and currently being evaluated in phase II clinical trial for treating Myelofibrosis. Additionally, pamrevlumab is being tested in phase III in patients with locally advanced, unresectable pancreatic cancer. Pamrevlumab is a monoclonal antibody that targets connective tissue growth factor, thereby reducing the fibrotic tissue and making unresectable tumors amenable to surgical excision (Sheridan, 2019).
Mechanobiology in personalized medicine
It is well known that tumor cells can vary widely according to the oncogenic background. Often, further heterogeneity occurs within a metastatic tumor when different patients are considered. Lab-on-a-chip systems are particularly well poised to address such questions related to patient -specificity with key advantages such as the requirement of low sample and reagent inputs, which are often scarce when derived directly from a patient (Figure 2F). Further, the ability to multiplex and perform high-throughput experiments is particularly amenable in lab-on-a-chip assays, enabling clinical level drug screening to be done on a person-to-person basis. For instance, microfluidic assays that recapitulate the surrounding mechanical environment of tumor cells (matrix stiffness, composition, pore size) can be used to understand the migratory phenotype of individual tumor cells as a typical indicator of potential malignancy, and how different pharmacological perturbations modulate this behavior (Pathak and Kumar, 2012; Polacheck et al., 2013; Polacheck et al., 2014) (Figure 2G). More complex in vitro models that mirror not only the surrounding ECM but also other physical cues (such as vasculature and stromal cells and fluid flows (Achilli et al., 2012; Kim et al., 2015; Whelan et al., 2023) can be employed in a multiplexed and high throughput manner to understand how these variables influence tumor cell migration in a patient-specific manner. This is particularly important as the recent FDA modernization act aims to integrate complex in vitro models of different diseases into the drug development process. For instance, Prolyl hydroxylases (PHDs) inhibitors have been shown to improve drug distribution in mice tumors and increase the effectiveness of chemotherapy. To demonstrate such effects in human cell models, Hu et al. (2022) employed a perfusable vascularized spheroid-on-a-chip model to simulate tumor microenvironment in vivo. They showed that dimethylallyl glycine improves the efficacy of the anticancer drugs paclitaxel and cisplatin in human esophageal carcinoma (Eca-109) spheroids (Figure 2F). Additionally, this could allow further understanding of the effects of external physical features such as matrix composition, stiffness, pore size, tumor cell adhesion molecules, and MMPs, on tumor migratory abilities and potentially tumor type characterization.
In addition to personalized drug screening under relevant biomechanical condition, it may also be possible phenotyping tumor cells and assess for instance their degree of malignancy through mechanical measurements on patient derived single tumour cells. Stiffness values of tumor cells or even aggregates of cells like tumor spheroids can now be measured using various contact-based (e.g., AFM) and non-contact-based methods (e.g., Brillouin imaging (Scarcelli et al., 2015; Roberts et al., 2021)); correlations can be made between these stiffness values and the invasive properties of these cells, through parallel in vitro screening experiments, and even using clinical tumor phenotyping data. This could allow extrapolating the metastatic potential from a small number of patient-derived samples and would prove to be a useful predictor when used in conjunction with traditional diagnostic methods.
Lastly, a deep understanding of the physical and structural properties of the surrounding tumor matrix might be useful for optimizing drug delivery to tumor sites. As an example, second harmonic imaging is now widely used to quantify the structural composition of collagens in the tumor microenvironment (Condeelis and Weissleder, 2010), and allow the characterization of pore size and fiber thickness, which may have a large influence on the effectiveness of drug transport. Obtaining this data for patient-specific tumors and subsequent computational modelling of drug transport based on these parameters may aid in the construction of a more optimized drug delivery system in the clinic.
Discussion
Accumulated evidence has demonstrated the fundamental role of mechanobiology in cancer research as the physical properties of both cells and their microenvironment play a crucial role in the development and progression of cancer. In this short review we summarized the role of cellular biomechanical properties, e.g., stiffness, adhesion and motility, morphology, deformability, and contractility, as well as microenvironment properties such as ECM stiffness, tissue architecture, blood vessel permeability, and interstitial flow, in different steps of the tumor dissemination. Indeed, mechanobiological insight could benefit researchers and clinicians in various ways; For instance, mechanical quantification at the tissue level has been used as a diagnosis or prognosis tool for various types of cancer. Advancements in the in vivo non-invasive techniques such as MRE and ultrasound elastography as well as ex vivo techniques such as AFM have improved our diagnosis abilities in the past decade. Furthermore, at the cellular level, mechanobiology can contribute significantly to our comprehension of molecular and genetic alterations in cancer. The interplay between mechanical forces and cellular behaviors can influence how genes are expressed, proteins are synthesized, and signaling pathways are activated. Such insights are of crucial importance in the development of new therapeutic strategies, as many ongoing clinical trials target components of the extracellular matrix (Lampi and Reinhart-King, 2018; Huang et al., 2021) and proteins associated with mechanosignaling pathways (Jiang et al., 2022). Nonetheless, owing to the diversity in oncogenic backgrounds and the inherent variability within metastatic tumors, distinct therapeutic strategies are required for individual patients; In this regard, microfluidics technology holds substantial promise in advancing personalized medicine through its ability to create controlled environment for studying individualized patient samples and responses. Nevertheless, there are intriguing avenues for further research to enhance the translatability of mechanobiological knowledge derived from these platforms into clinical practice. This includes integration of the cancer mechanobiology insight with the data from genomics, transcriptomics, and proteomics approaches; this, in combination with single cell data analysis (obtained from limited number of tumor cells harvested from patient’s blood or tissue) could augment our understanding of mechanotransducive pathways involved in cancer. Moreover, by introducing immune cells along with other supporting cell types into the 3D tumor-mimicking microenvironment established within microfluidic chips, we can investigate effects of mechanical signals on immune cell behavior, as well as their implications for immunotherapy responses and the emergence of resistance.
Author contributions
AA and EM conceived the idea of the manuscript. MC, YJ, NS, BS, and EM performed the literature database and drafted the manuscript. YJ sketched and implemented figures. EM supervised the study. All authors contributed to the article and approved the submitted version.
Funding
YJ and EM acknowledge financial support by Leverhulme Trust Research Project Grant (RPG-2018-443), the Cancer Research United Kingdom Multidisciplinary Award (C57744/A22057), and Biotechnology and Biological Sciences Research Council Grant (BB/V001418/1) supports.
Acknowledgments
EM is grateful for Wellcome Trust-MIT Fellowship (WT103883).
Conflict of interest
Authors BS and BD were employed by 199 Biotechnologies Ltd.
The remaining authors declare that the research was conducted in the absence of any commercial or financial relationships that could be construed as a potential conflict of interest.
Publisher’s note
All claims expressed in this article are solely those of the authors and do not necessarily represent those of their affiliated organizations, or those of the publisher, the editors and the reviewers. Any product that may be evaluated in this article, or claim that may be made by its manufacturer, is not guaranteed or endorsed by the publisher.
References
Aceto, N., Toner, M., Maheswaran, S., and Haber, D. A. (2015). En route to metastasis: circulating tumor cell clusters and epithelial-to-mesenchymal transition. Trends Cancer 1, 44–52. doi:10.1016/j.trecan.2015.07.006
Achilli, T.-M., Meyer, J., and Morgan, J. R. (2012). Advances in the formation, use and understanding of multi-cellular spheroids. Expert Opin. Biol. Ther. 12, 1347–1360. doi:10.1517/14712598.2012.707181
Agrawal, A., Shahreza, S., Javanmardi, Y., Szita, N., and Moeendarbary, E. (2022). The tumour microenvironment modulates cancer cell intravasation. Organs-on-a-Chip 4, 100024. doi:10.1016/j.ooc.2022.100024
Au, S. H., Storey, B. D., Moore, J. C., Tang, Q., Chen, Y.-L., Javaid, S., et al. (2016). Clusters of circulating tumor cells traverse capillary-sized vessels. Proc. Natl. Acad. Sci. 113, 4947–4952. doi:10.1073/pnas.1524448113
Barenholz-Cohen, T., Merkher, Y., Haj, J., Shechter, D., Kirchmeier, D., Shaked, Y., et al. (2020). Lung mechanics modifications facilitating metastasis are mediated in part by breast cancer-derived extracellular vesicles. Int. J. Cancer 147, 2924–2933. doi:10.1002/ijc.33229
Barrasa-Fano, J., Shapeti, A., Jorge-Peñas, Á., Barzegari, M., Sanz-Herrera, J. A., and Van Oosterwyck, H. (2021). TFMLAB: a MATLAB toolbox for 4D traction force microscopy. SoftwareX 15, 100723. doi:10.1016/j.softx.2021.100723
Bera, K., Kiepas, A., Godet, I., Li, Y., Mehta, P., Ifemembi, B., et al. (2022). Extracellular fluid viscosity enhances cell migration and cancer dissemination. Nature 611, 365–373. doi:10.1038/s41586-022-05394-6
Bertero, T., Oldham, W. M., Grasset, E. M., Bourget, I., Boulter, E., Pisano, S., et al. (2019). Tumor-stroma mechanics coordinate amino acid availability to sustain tumor growth and malignancy. Cell Metab. 29, 124–140. doi:10.1016/j.cmet.2018.09.012
Beyer, S., Blocki, A., Cheung, M. C. Y., Wan, Z. H. Y., Mehrjou, B., and Kamm, R. D. (2021). Lectin staining of microvascular Glycocalyx in microfluidic cancer cell extravasation assays. Life 11, 179. doi:10.3390/life11030179
Bocci, F., Gearhart-Serna, L., Boareto, M., Ribeiro, M., Ben-Jacob, E., Devi, G. R., et al. (2019). Toward understanding cancer stem cell heterogeneity in the tumor microenvironment. Proc. Natl. Acad. Sci. 116, 148–157. doi:10.1073/pnas.1815345116
Budden, T., Gaudy-Marqueste, C., Porter, A., Kay, E., Gurung, S., Earnshaw, C. H., et al. (2021). Ultraviolet light-induced collagen degradation inhibits melanoma invasion. Nat. Commun. 12, 2742. doi:10.1038/s41467-021-22953-z
Burdziak, C., Alonso-Curbelo, D., Walle, T., Reyes, J., Barriga, F. M., Haviv, D., et al. (2023). Epigenetic plasticity cooperates with cell-cell interactions to direct pancreatic tumorigenesis. Sci. (80-) 380, eadd5327. doi:10.1126/science.add5327
Calvo, F., Ege, N., Grande-Garcia, A., Hooper, S., Jenkins, R. P., Chaudhry, S. I., et al. (2013). Mechanotransduction and YAP-dependent matrix remodelling is required for the generation and maintenance of cancer-associated fibroblasts. Nat. Cell Biol. 15, 637–646. doi:10.1038/ncb2756
Campàs, O., Mammoto, T., Hasso, S., Sperling, R. A., O’Connell, D., Bischof, A. G., et al. (2014). Quantifying cell-generated mechanical forces within living embryonic tissues. Nat. Methods 11, 183–189. doi:10.1038/nmeth.2761
Cao, X., Moeendarbary, E., Isermann, P., Davidson, P. M., Wang, X., Chen, M. B., et al. (2016). A chemomechanical model for nuclear morphology and stresses during cell transendothelial migration. Biophys. J. 111, 1541–1552. doi:10.1016/J.BPJ.2016.08.011
Chaffer, C. L., and Weinberg, R. (2011). A perspective on cancer cell metastasis. Science 331, 1559–1564. doi:10.1126/science.1203543
Chaudhuri, O., Cooper-White, J., Janmey, P. A., Mooney, D. J., and Shenoy, V. B. (2020). Effects of extracellular matrix viscoelasticity on cellular behaviour. Nature 584, 535–546. doi:10.1038/s41586-020-2612-2
Chen, M. B., Whisler, J. A., Fröse, J., Yu, C., Shin, Y., and Kamm, R. D. (2017). On-chip human microvasculature assay for visualization and quantification of tumor cell extravasation dynamics. Nat. Protoc. 12, 865–880. doi:10.1038/nprot.2017.018
Chen, Y., Pan, C., Wang, X., Xu, D., Ma, Y., Hu, J., et al. (2021). Silencing of METTL3 effectively hinders invasion and metastasis of prostate cancer cells. Theranostics 11, 7640–7657. doi:10.7150/thno.61178
Colin-York, H., Javanmardi, Y., Barbieri, L., Li, D., Korobchevskaya, K., Guo, Y., et al. (2019a). Spatiotemporally super-resolved volumetric traction force microscopy. Nano Lett. 19, 4427–4434. doi:10.1021/acs.nanolett.9b01196
Colin-York, H., Javanmardi, Y., Skamrahl, M., Kumari, S., Chang, V. T., Khuon, S., et al. (2019b). Cytoskeletal control of antigen-dependent T cell activation. Cell Rep. 26, 3369–3379. doi:10.1016/j.celrep.2019.02.074
Condeelis, J., and Weissleder, R. (2010). In vivo imaging in cancer. Cold Spring Harb. Perspect. Biol. 2, a003848. doi:10.1101/cshperspect.a003848
Coughlin, M. F., Bielenberg, D. R., Lenormand, G., Marinkovic, M., Waghorne, C. G., Zetter, B. R., et al. (2013). Cytoskeletal stiffness, friction, and fluidity of cancer cell lines with different metastatic potential. Clin. Exp. Metastasis 30, 237–250. doi:10.1007/s10585-012-9531-z
Cox, T. R., and Erler, J. T. (2011). Remodeling and homeostasis of the extracellular matrix: implications for fibrotic diseases and cancer. Dis. Model Mech. 4, 165–178. doi:10.1242/dmm.004077
Craene, B. D, and Berx, G. (2013). Regulatory networks defining EMT during cancer initiation and progression. Nat. Rev. Cancer 13, 97–110. doi:10.1038/nrc3447
Cross, S. E., Jin, Y.-S., Rao, J., and Gimzewski, J. K. (2007). Nanomechanical analysis of cells from cancer patients. Nat. Nanotechnol. 2, 780–783. doi:10.1038/nnano.2007.388
Denais, C. M., Gilbert, R. M., Isermann, P., McGregor, A. L., te Lindert, M., Weigelin, B., et al. (2016). Nuclear envelope rupture and repair during cancer cell migration. Sci. (80-) 352, 353–358. doi:10.1126/science.aad7297
Deptuła, P., Łysik, D., Pogoda, K., Cieśluk, M., Namiot, A., Mystkowska, J., et al. (2020). Tissue rheology as a possible complementary procedure to advance histological diagnosis of Colon cancer. ACS Biomater. Sci. Eng. 6, 5620–5631. doi:10.1021/acsbiomaterials.0c00975
Deryugina, E. I., and Quigley, J. P. (2015). Tumor angiogenesis: MMP-mediated induction of intravasation- and metastasis-sustaining neovasculature. Matrix Biol. 44–46, 94–112. doi:10.1016/j.matbio.2015.04.004
Di, X., Gao, X., Peng, L., Ai, J., Jin, X., Qi, S., et al. (2023). Cellular mechanotransduction in health and diseases: from molecular mechanism to therapeutic targets. Signal Transduct. Target Ther. 8, 282. doi:10.1038/s41392-023-01501-9
Díaz de la Loza, M. C., Díaz-Torres, A., Zurita, F., Rosales-Nieves, A. E., Moeendarbary, E., Franze, K., et al. (2017). Laminin levels regulate tissue migration and anterior-posterior polarity during egg morphogenesis in Drosophila. Cell Rep. 20, 211–223. doi:10.1016/j.celrep.2017.06.031
Dolega, M. E., Delarue, M., Ingremeau, F., Prost, J., Delon, A., and Cappello, G. (2017). Cell-like pressure sensors reveal increase of mechanical stress towards the core of multicellular spheroids under compression. Nat. Commun. 8, 14056. doi:10.1038/ncomms14056
Dou, C., Zhou, Z., Xu, Q., Liu, Z., Zeng, Y., Wang, Y., et al. (2022). Correction: hypoxia-induced TUFT1 promotes the growth and metastasis of hepatocellular carcinoma by activating the Ca2+/PI3K/AKT pathway. Oncogene 41, 4330–4332. doi:10.1038/s41388-022-02421-8
Gal, N., and Weihs, D. (2012). Intracellular mechanics and activity of breast cancer cells correlate with metastatic potential. Cell Biochem. Biophys. 63, 199–209. doi:10.1007/s12013-012-9356-z
Galarza, S., Kim, H., Atay, N., Peyton, S. R., and Munson, J. M. (2020). 2D or 3D? How cell motility measurements are conserved across dimensions in vitro and translate in vivo. Bioeng. Transl. Med. 5, e10148. doi:10.1002/btm2.10148
Golatta, M., Pfob, A., Büsch, C., Bruckner, T., Alwafai, Z., Balleyguier, C., et al. (2022). The potential of combined shear wave and strain elastography to reduce unnecessary biopsies in breast cancer diagnostics – an international, multicentre trial. Eur. J. Cancer 161, 1–9. doi:10.1016/j.ejca.2021.11.005
Han, Y. L., Ronceray, P., Xu, G., Malandrino, A., Kamm, R. D., Lenz, M., et al. (2018). Cell contraction induces long-ranged stress stiffening in the extracellular matrix. Proc. Natl. Acad. Sci. U. S. A. 115, 4075–4080. doi:10.1073/pnas.1722619115
Hassanzadeh-Barforoushi, A., Warkiani, M. E., Gallego-Ortega, D., Liu, G., and Barber, T. (2020). Capillary-assisted microfluidic biosensing platform captures single cell secretion dynamics in nanoliter compartments. Biosens. Bioelectron. 155, 112113. doi:10.1016/j.bios.2020.112113
Herman, H., Fazakas, C., Haskó, J., Molnár, K., Mészáros, Á., Nyúl-Tóth, Á., et al. (2019). Paracellular and transcellular migration of metastatic cells through the cerebral endothelium. J. Cell Mol. Med. 23, 2619–2631. doi:10.1111/jcmm.14156
Hu, Z., Cao, Y., Galan, E. A., Hao, L., Zhao, H., Tang, J., et al. (2022). Vascularized tumor spheroid-on-a-chip model Verifies synergistic vasoprotective and chemotherapeutic effects. ACS Biomater. Sci. Eng. 8, 1215–1225. doi:10.1021/acsbiomaterials.1c01099
Huang, J., Zhang, L., Wan, D., Zhou, L., Zheng, S., Lin, S., et al. (2021). Extracellular matrix and its therapeutic potential for cancer treatment. Signal Transduct. Target Ther. 6, 153. doi:10.1038/s41392-021-00544-0
Huh, S. J., Liang, S., Sharma, A., Dong, C., and Robertson, G. P. (2010). Transiently entrapped circulating tumor cells interact with neutrophils to facilitate lung metastasis development. Cancer Res. 70, 6071–6082. doi:10.1158/0008-5472.CAN-09-4442
Ishihara, S., and Haga, H. (2022). Matrix stiffness contributes to cancer progression by regulating transcription factors. Cancers (Basel) 14, 1049. doi:10.3390/cancers14041049
Itoh, Y., Takehara, Y., Kawase, T., Terashima, K., Ohkawa, Y., Hirose, Y., et al. (2016). Feasibility of magnetic resonance elastography for the pancreas at 3T. J. Magn. Reson Imaging 43, 384–390. doi:10.1002/jmri.24995
Jain, R. K., Martin, J. D., and Stylianopoulos, T. (2014). The role of mechanical forces in tumor growth and therapy. Annu. Rev. Biomed. Eng. 16, 321–346. doi:10.1146/annurev-bioeng-071813-105259
Jain, R. K., Munn, L. L., and Fukumura, D. (2002). Dissecting tumour pathophysiology using intravital microscopy. Nat. Rev. Cancer 2, 266–276. doi:10.1038/nrc778
Javanmardi, Y., Agrawal, A., Malandrino, A., Lasli, S., Chen, M., Shahreza, S., et al. (2023). Endothelium and subendothelial matrix mechanics modulate cancer cell transendothelial migration. Adv. Sci. 10, e2206554. doi:10.1002/advs.202206554
Javanmardi, Y., Colin-York, H., Szita, N., Fritzsche, M., and Moeendarbary, E. (2021). Quantifying cell-generated forces: poisson’s ratio matters. Commun. Phys. 41 (4), 237–310. doi:10.1038/s42005-021-00740-y
Jiang, Y., Zhang, H., Wang, J., Liu, Y., Luo, T., and Hua, H. (2022). Targeting extracellular matrix stiffness and mechanotransducers to improve cancer therapy. J. Hematol. Oncol. 15, 34. doi:10.1186/s13045-022-01252-0
Kalluri, R., and Weinberg, R. a. (2009). The basics of epithelial-mesenchymal transition. J. Clin. Invest. 119, 1420–1428. doi:10.1172/JCI39104
Kennedy, K. M., Chin, L., McLaughlin, R. A., Latham, B., Saunders, C. M., Sampson, D. D., et al. (2015). Quantitative micro-elastography: imaging of tissue elasticity using compression optical coherence elastography. Sci. Rep. 5, 15538. doi:10.1038/srep15538
Kim, J., Chung, M., Kim, S., Jo, D. H., Kim, J. H., and Jeon, N. L. (2015). Engineering of a biomimetic pericyte-covered 3D microvascular network. PLoS One 10, e0133880. doi:10.1371/journal.pone.0133880
Kim, J., Han, S., Lei, A., Miyano, M., Bloom, J., Srivastava, V., et al. (2018). Characterizing cellular mechanical phenotypes with mechano-node-pore sensing. Microsystems Nanoeng. 4, 17091. doi:10.1038/micronano.2017.91
Klein, E. A., Yin, L., Kothapalli, D., Castagnino, P., Byfield, F. J., Xu, T., et al. (2009). Cell-cycle control by physiological matrix elasticity and in vivo tissue stiffening. Curr. Biol. 19, 1511–1518. doi:10.1016/j.cub.2009.07.069
Koorman, T., Jansen, K. A., Khalil, A., Haughton, P. D., Visser, D., Rätze, M. A. K., et al. (2022). Spatial collagen stiffening promotes collective breast cancer cell invasion by reinforcing extracellular matrix alignment. Oncogene 41, 2458–2469. doi:10.1038/s41388-022-02258-1
Koumoutsakos, P., Pivkin, I., and Milde, F. (2013). The fluid mechanics of cancer and its therapy. Annu. Rev. Fluid Mech. 45, 325–355. doi:10.1146/annurev-fluid-120710-101102
Kraning-Rush, C. M., Califano, J. P., and Reinhart-King, C. A. (2012). Cellular traction stresses increase with increasing metastatic potential. PLoS One 7, e32572. doi:10.1371/journal.pone.0032572
Kristal-Muscal, R., Dvir, L., and Weihs, D. (2013). Metastatic cancer cells tenaciously indent impenetrable, soft substrates. New J. Phys. 15, 035022. doi:10.1088/1367-2630/15/3/035022
Kumar, S., and Weaver, V. M. (2009). Mechanics, malignancy, and metastasis: the force journey of a tumor cell. Cancer Metastasis Rev. 28, 113–127. doi:10.1007/s10555-008-9173-4
Labernadie, A., Kato, T., Brugués, A., Serra-Picamal, X., Derzsi, S., Arwert, E., et al. (2017). A mechanically active heterotypic E-cadherin/N-cadherin adhesion enables fibroblasts to drive cancer cell invasion. Nat. Cell Biol. 19, 224–237. doi:10.1038/ncb3478
Labelle, M., Begum, S., and Hynes, R. O. (2011). Direct signaling between platelets and cancer cells induces an epithelial-mesenchymal-like transition and promotes metastasis. Cancer Cell 20, 576–590. doi:10.1016/j.ccr.2011.09.009
Laklai, H., Miroshnikova, Y. A., Pickup, M. W., Collisson, E. A., Kim, G. E., Barrett, A. S., et al. (2016). Genotype tunes pancreatic ductal adenocarcinoma tissue tension to induce matricellular fibrosis and tumor progression. Nat. Med. 22, 497–505. doi:10.1038/nm.4082
Lammerding, J. (2011). Mechanics of the nucleus. Compr. Physiol. 1, 783–807. doi:10.1002/cphy.c100038
Lampi, M. C., and Reinhart-King, C. A. (2018). Targeting extracellular matrix stiffness to attenuate disease: from molecular mechanisms to clinical trials. Sci. Transl. Med. 10, eaao0475. doi:10.1126/scitranslmed.aao0475
Laudani, S., La Cognata, V., Iemmolo, R., Bonaventura, G., Villaggio, G., Saccone, S., et al. (2020). Effect of a bone marrow-derived extracellular matrix on cell adhesion and neural induction of dental pulp stem cells. Front. Cell Dev. Biol. 8, 100. doi:10.3389/fcell.2020.00100
Lee, W., Kalashnikov, N., Mok, S., Halaoui, R., Kuzmin, E., Putnam, A. J., et al. (2019). Dispersible hydrogel force sensors reveal patterns of solid mechanical stress in multicellular spheroid cultures. Nat. Commun. 10, 144. doi:10.1038/s41467-018-07967-4
Leggett, S. E., Patel, M., Valentin, T. M., Gamboa, L., Khoo, A. S., Williams, E. K., et al. (2020). Mechanophenotyping of 3D multicellular clusters using displacement arrays of rendered tractions. Proc. Natl. Acad. Sci. 117, 5655–5663. doi:10.1073/pnas.1918296117
Leong, H. S., Robertson, A. E., Stoletov, K., Leith, S. J., Chin, C. A., Chien, A. E., et al. (2014). Invadopodia are required for cancer cell extravasation and are a therapeutic target for metastasis. Cell Rep. 8, 1558–1570. doi:10.1016/j.celrep.2014.07.050
Li, D., Colin-York, H., Barbieri, L., Javanmardi, Y., Guo, Y., Korobchevskaya, K., et al. (2021). Astigmatic traction force microscopy (aTFM). Nat. Commun. 12, 2168. doi:10.1038/s41467-021-22376-w
Liu, X., Ye, Y., Zhu, L., Xiao, X., Zhou, B., Gu, Y., et al. (2023). Niche stiffness sustains cancer stemness via TAZ and NANOG phase separation. Nat. Commun. 14, 238. doi:10.1038/s41467-023-35856-y
Lopez, J. I., Kang, I., You, W.-K., McDonald, D. M., and Weaver, V. M. (2011). In situ force mapping of mammary gland transformation. Integr. Biol. 3, 910–921. doi:10.1039/c1ib00043h
Lu, P., Weaver, V. M., and Werb, Z. (2012). The extracellular matrix: a dynamic niche in cancer progression. J. Cell Biol. 196, 395–406. doi:10.1083/jcb.201102147
Madsen, C. D., Pedersen, J. T., Venning, F. A., Singh, L. B., Moeendarbary, E., Charras, G., et al. (2015). Hypoxia and loss of PHD2 inactivate stromal fibroblasts to decrease tumour stiffness and metastasis. EMBO Rep. 16, 1394–1408. doi:10.15252/embr.201540107
Maity, G., Sen, T., and Chatterjee, A. (2011). Laminin induces matrix metalloproteinase-9 expression and activation in human cervical cancer cell line (SiHa). J. Cancer Res. Clin. Oncol. 137, 347–357. doi:10.1007/s00432-010-0892-x
Malandrino, A., Kamm, R. D., and Moeendarbary, E. (2018a). In vitro modeling of mechanics in cancer metastasis. ACS Biomater. Sci. Eng. 4, 294–301. doi:10.1021/acsbiomaterials.7b00041
Malandrino, A., Mak, M., Kamm, R. D., and Moeendarbary, E. (2018b). Complex mechanics of the heterogeneous extracellular matrix in cancer. Extrem Mech. Lett. 21, 25–34. doi:10.1016/j.eml.2018.02.003
Mark, C., Grundy, T. J., Strissel, P. L., Böhringer, D., Grummel, N., Gerum, R., et al. (2020). Collective forces of tumor spheroids in three-dimensional biopolymer networks. Elife 9, e51912. doi:10.7554/eLife.51912
Marrella, A., Fedi, A., Varani, G., Vaccari, I., Fato, M., Firpo, G., et al. (2021). High blood flow shear stress values are associated with circulating tumor cells cluster disaggregation in a multi-channel microfluidic device. PLoS One 16, e0245536. doi:10.1371/journal.pone.0245536
McEvoy, E., Sneh, T., Moeendarbary, E., Javanmardi, Y., Efimova, N., Yang, C., et al. (2022). Feedback between mechanosensitive signaling and active forces governs endothelial junction integrity. Nat. Commun. 13, 7089. doi:10.1038/s41467-022-34701-y
Micalet, A., Pape, J., Bakkalci, D., Javanmardi, Y., Hall, C., Cheema, U., et al. (2022). Evaluating the impact of a biomimetic mechanical environment on cancer invasion and matrix remodeling. Adv. Healthc. Mater 12, 2201749. doi:10.1002/adhm.202201749
Mierke, C. T. (2019). The matrix environmental and cell mechanical properties regulate cell migration and contribute to the invasive phenotype of cancer cells. Rep. Prog. Phys. 82, 064602. doi:10.1088/1361-6633/ab1628
Mierke, C. T. (2013). The role of focal adhesion kinase in the regulation of cellular mechanical properties. Phys. Biol. 10, 065005. doi:10.1088/1478-3975/10/6/065005
Mierke, C. T. (2021). Viscoelasticity acts as a marker for tumor extracellular matrix characteristics. Front. Cell Dev. Biol. 9, 785138. doi:10.3389/fcell.2021.785138
Minchinton, A. I., and Tannock, I. F. (2006). Drug penetration in solid tumours. Nat. Rev. Cancer 6, 583–592. doi:10.1038/nrc1893
Miyazawa, A., Ito, S., Asano, S., Tanaka, I., Sato, M., Kondo, M., et al. (2018). Regulation of PD-L1 expression by matrix stiffness in lung cancer cells. Biochem. Biophys. Res. Commun. 495, 2344–2349. doi:10.1016/j.bbrc.2017.12.115
MJMJJ, Paszek, Zahir, N., Johnson, K. R., Lakins, JNJN, Rozenberg, GIGI, Gefen, A., et al. (2005). Tensional homeostasis and the malignant phenotype. Cancer Cell 8, 241–254. doi:10.1016/j.ccr.2005.08.010
Moeendarbary, E., and Harris, A. R. (2014). Cell mechanics: principles, practices, and prospects. Wiley Interdiscip. Rev. Syst. Biol. Med. 6, 371–388. doi:10.1002/wsbm.1275
Moeendarbary, E., Valon, L., Fritzsche, M., Harris, A. R., Moulding, D. A., Thrasher, A. J., et al. (2013). The cytoplasm of living cells behaves as a poroelastic material. Nat. Mater 12, 253–261. doi:10.1038/nmat3517
Nabavizadeh, A., Bayat, M., Kumar, V., Gregory, A., Webb, J., Alizad, A., et al. (2019). Viscoelastic biomarker for differentiation of benign and malignant breast lesion in ultra-low frequency range. Sci. Rep. 9, 5737. doi:10.1038/s41598-019-41885-9
Nagrath, S., Sequist, L. V., Maheswaran, S., Bell, D. W., Irimia, D., Ulkus, L., et al. (2007). Isolation of rare circulating tumour cells in cancer patients by microchip technology. Nature 450, 1235–1239. doi:10.1038/nature06385
Nguyen, D. X., Bos, P. D., and Massagué, J. (2009). Metastasis: from dissemination to organ-specific colonization. Nat. Rev. Cancer 9, 274–284. doi:10.1038/nrc2622
Nia, H. T., Liu, H., Seano, G., Datta, M., Jones, D., Rahbari, N., et al. (2017). Solid stress and elastic energy as measures of tumour mechanopathology. Nat. Biomed. Eng. 1, 0004–0011. doi:10.1038/s41551-016-0004
Nia, H. T., Munn, L. L., and Jain, R. K. (2020). Physical traits of cancer. Sci. (80-) 370, eaaz0868. doi:10.1126/science.aaz0868
Pathak, A., and Kumar, S. (2012). Independent regulation of tumor cell migration by matrix stiffness and confinement. Proc. Natl. Acad. Sci. U. S. A. 109, 10334–10339. doi:10.1073/pnas.1118073109
Peng, H.-H., Liang, S., Henderson, A. J., and Dong, C. (2007). Regulation of interleukin-8 expression in melanoma-stimulated neutrophil inflammatory response. Exp. Cell Res. 313, 551–559. doi:10.1016/j.yexcr.2006.10.030
Pickup, M. W., Mouw, J. K., and Weaver, V. M. (2014). The extracellular matrix modulates the hallmarks of cancer. EMBO Rep. 15, 1243–1253. doi:10.15252/embr.201439246
Plodinec, M., Loparic, M., Monnier, C. A., Obermann, E. C., Zanetti-Dallenbach, R., Oertle, P., et al. (2012). The nanomechanical signature of breast cancer. Nat. Nanotechnol. 7, 757–765. doi:10.1038/nnano.2012.167
Polacheck, W. J., German, A. E., Mammoto, A., Ingber, D. E., and Kamm, R. D. (2014). Mechanotransduction of fluid stresses governs 3D cell migration. Proc. Natl. Acad. Sci. U. S. A. 111, 2447–2452. doi:10.1073/pnas.1316848111
Polacheck, W. J., Li, R., Uzel, S. G. M., and Kamm, R. D. (2013). Microfluidic platforms for mechanobiology. Lab. Chip 13, 2252–2267. doi:10.1039/c3lc41393d
Polyak, K., and Weinberg, R. A. (2009). Transitions between epithelial and mesenchymal states: acquisition of malignant and stem cell traits. Nat. Rev. Cancer 9, 265–273. doi:10.1038/nrc2620
Popova, N. V., and Jücker, M. (2022). The functional role of extracellular matrix proteins in cancer. Cancers (Basel) 14, 238. doi:10.3390/cancers14010238
Ramião, N. G., Martins, P. S., Rynkevic, R., Fernandes, A. A., Barroso, M., and Santos, D. C. (2016). Biomechanical properties of breast tissue, a state-of-the-art review. Biomech. Model Mechanobiol. 15, 1307–1323. doi:10.1007/s10237-016-0763-8
Reiter, R., Majumdar, S., Kearney, S., Kajdacsy-Balla, A., Macias, V., Crivellaro, S., et al. (2022). Investigating the heterogeneity of viscoelastic properties in prostate cancer using MR elastography at 9.4T in fresh prostatectomy specimens. Magn. Reson Imaging 87, 113–118. doi:10.1016/j.mri.2022.01.005
Ren, K., Feng, J., Bi, H., Sun, Q., Li, X., and Han, D. (2023). AFM-based Poroelastic@Membrane analysis of cells and its opportunities for translational medicine. Small 2023, e2303610. doi:10.1002/smll.202303610
Reuten, R., Zendehroud, S., Nicolau, M., Fleischhauer, L., Laitala, A., Kiderlen, S., et al. (2021). Basement membrane stiffness determines metastases formation. Nat. Mater 20, 892–903. doi:10.1038/s41563-020-00894-0
Reymond, N., d’Água, B. B., and Ridley, A. J. (2013). Crossing the endothelial barrier during metastasis. Nat. Rev. Cancer 13, 858–870. doi:10.1038/nrc3628
Rianna, C., Radmacher, M., and Kumar, S. (2020). Direct evidence that tumor cells soften when navigating confined spaces. Mol. Biol. Cell 31, 1726–1734. doi:10.1091/mbc.E19-10-0588
Rieger, H., and Welter, M. (2015). Integrative models of vascular remodeling during tumor growth. Wiley Interdiscip. Rev. Syst. Biol. Med. 7, 113–129. doi:10.1002/wsbm.1295
Roberts, A. B., Zhang, J., Raj Singh, V., Nikolić, M., Moeendarbary, E., Kamm, R. D., et al. (2021). Tumor cell nuclei soften during transendothelial migration. J. Biomech. 121, 110400. doi:10.1016/j.jbiomech.2021.110400
Rosette, C., Roth, R. B., Oeth, P., Braun, A., Kammerer, S., Ekblom, J., et al. (2005). Role of ICAM1 in invasion of human breast cancer cells. Carcinogenesis 26, 943–950. doi:10.1093/carcin/bgi070
Saha, K., Keung, A. J., Irwin, E. F., Li, Y., Little, L., Schaffer, D. V., et al. (2008). Substrate modulus directs neural stem cell behavior. Biophys. J. 95, 4426–4438. doi:10.1529/biophysj.108.132217
Samuel, T., Rapic, S., O’Brien, C., Edson, M., Zhong, Y., and DaCosta, R. S. (2023). Quantitative intravital imaging for real-time monitoring of pancreatic tumor cell hypoxia and stroma in an orthotopic mouse model. Sci. Adv. 9, eade8672. doi:10.1126/sciadv.ade8672
Sauer, F., Grosser, S., Shahryari, M., Hayn, A., Guo, J., Braun, J., et al. (2023). Changes in tissue fluidity Predict tumor aggressiveness in vivo. Adv. Sci. 10, e2303523. doi:10.1002/advs.202303523
Scarcelli, G., Polacheck, W. J., Nia, H. T., Patel, K., Grodzinsky, A. J., Kamm, R. D., et al. (2015). Noncontact three-dimensional mapping of intracellular hydromechanical properties by Brillouin microscopy. Nat. Methods 12, 1132–1134. doi:10.1038/nmeth.3616
Schumacher, D., Strilic, B., Sivaraj, K. K., Wettschureck, N., and Offermanns, S. (2013). Platelet-derived nucleotides promote tumor-cell transendothelial migration and metastasis via P2Y2 receptor. Cancer Cell 24, 130–137. doi:10.1016/j.ccr.2013.05.008
Sheridan, C. (2019). Pancreatic cancer provides testbed for first mechanotherapeutics. Nat. Biotechnol. 37, 829–831. doi:10.1038/d41587-019-00019-2
Shibue, T., and Weinberg, R. A. (2009). Integrin beta1-focal adhesion kinase signaling directs the proliferation of metastatic cancer cells disseminated in the lungs. Proc. Natl. Acad. Sci. 106, 10290–10295. doi:10.1073/pnas.0904227106
Sikic, L., Schulman, E., Kosklin, A., Saraswathibhatla, A., Chaudhuri, O., and Pokki, J. (2022). Nanoscale tracking combined with cell-scale Microrheology reveals stepwise increases in force generated by cancer cell protrusions. Nano Lett. 22, 7742–7750. doi:10.1021/acs.nanolett.2c01327
Soubéran, A., Brustlein, S., Gouarné, C., Chasson, L., Tchoghandjian, A., Malissen, M., et al. (2019). Effects of VEGF blockade on the dynamics of the inflammatory landscape in glioblastoma-bearing mice. J. Neuroinflammation 16, 191. doi:10.1186/s12974-019-1563-8
Sounni, N. E., Paye, A., Host, L., and Noël, A. (2011). MT-MMPS as regulators of vessel stability associated with angiogenesis. Front. Pharmacol. 2, 111. doi:10.3389/fphar.2011.00111
Spill, F., Reynolds, D. S., Kamm, R. D., and Zaman, M. H. (2016). Impact of the physical microenvironment on tumor progression and metastasis. Curr. Opin. Biotechnol. 40, 41–48. doi:10.1016/j.copbio.2016.02.007
Straehla, J. P., Hajal, C., Safford, H. C., Offeddu, G. S., Boehnke, N., Dacoba, T. G., et al. (2022). A predictive microfluidic model of human glioblastoma to assess trafficking of blood–brain barrier-penetrant nanoparticles. Proc. Natl. Acad. Sci. 119, e2118697119. doi:10.1073/pnas.2118697119
Stylianopoulos, T., Martin, J. D., Chauhan, V. P., Jain, S. R., Diop-Frimpong, B., Bardeesy, N., et al. (2012). Causes, consequences, and remedies for growth-induced solid stress in murine and human tumors. Proc. Natl. Acad. Sci. 109, 15101–15108. doi:10.1073/pnas.1213353109
Stylianou, A., and Stylianopoulos, T. (2016). Atomic force microscopy probing of cancer cells and tumor microenvironment components. Bionanoscience 6, 33–46. doi:10.1007/s12668-015-0187-4
Suresh, S. (2007). Biomechanics and biophysics of cancer cells. Acta Mater 55, 3989–4014. doi:10.1016/j.actamat.2007.04.022
Thiery, J. P., and Sleeman, J. P. (2006). Complex networks orchestrate epithelial-mesenchymal transitions. Nat. Rev. Mol. Cell Biol. 7, 131–142. doi:10.1038/nrm1835
Torrino, S., Grasset, E. M., Audebert, S., Belhadj, I., Lacoux, C., Haynes, M., et al. (2021). Mechano-induced cell metabolism promotes microtubule glutamylation to force metastasis. Cell Metab. 33, 1342–1357.e10. doi:10.1016/j.cmet.2021.05.009
Van Zijl, F., Krupitza, G., and Mikulits, W. (2011). Initial steps of metastasis: cell invasion and endothelial transmigration. Mutat. Res. - Rev. Mutat. Res. 728, 23–34. doi:10.1016/j.mrrev.2011.05.002
Venkatesh, S. K., Yin, M., Glockner, J. F., Takahashi, N., Araoz, P. A., Talwalkar, J. A., et al. (2008). MR elastography of liver tumors: preliminary results. Am. J. Roentgenol. 190, 1534–1540. doi:10.2214/AJR.07.3123
Voura, E. B., English, J. L., Yu, H.-Y. E., Ho, A. T., Subarsky, P., Hill, R. P., et al. (2013). Proteolysis during tumor cell extravasation in vitro: metalloproteinase involvement across tumor cell types. PLoS One 8, e78413. doi:10.1371/journal.pone.0078413
Wang, H. B., Dembo, M., and Wang, Y. L. (2000). Substrate flexibility regulates growth and apoptosis of normal but not transformed cells. Am. J. Physiol. Cell Physiol. 279, C1345–C1350. doi:10.1152/ajpcell.2000.279.5.C1345
Wang, N., Butler, J., and Ingber, D. (1993). Mechanotransduction across the cell surface and through the cytoskeleton. Sci. (80-) 260, 1124–1127. doi:10.1126/science.7684161
Wang, W. Y., Davidson, C. D., Lin, D., and Baker, B. M. (2019). Actomyosin contractility-dependent matrix stretch and recoil induces rapid cell migration. Nat. Commun. 10, 1186. doi:10.1038/s41467-019-09121-0
Warli, S. M., Putrantyo, , and Laksmi, L. I. (2023). Correlation between tumor-associated collagen signature and fibroblast activation protein expression with prognosis of clear cell renal cell carcinoma patient. World J. Oncol. 14, 145–149. doi:10.14740/wjon1564
Watson, S. A., Javanmardi, Y., Zanieri, L., Shahreza, S., Ragazzini, R., Bonfanti, P., et al. (2022). Integrated role of human thymic stromal cells in hematopoietic stem cell extravasation. Bioeng. Transl. Med. 8, e10454. doi:10.1002/btm2.10454
Wei, S. C., Fattet, L., Tsai, J. H., Guo, Y., Pai, V. H., Majeski, H. E., et al. (2015). Matrix stiffness drives epithelial – mesenchymal transition and tumour metastasis through a TWIST1 – G3BP2 mechanotransduction pathway. Nat. Cell Biol. 17, 678–688. doi:10.1038/ncb3157
Weis, S., and Cheresh, D. (2011). Tumor angiogenesis: molecular pathways and therapeutic targets. Nat. Med. 17, 1359–1370. doi:10.1038/nm.2537
Wells, P. N. T., and Liang, H.-D. (2011). Medical ultrasound: imaging of soft tissue strain and elasticity. J. R. Soc. Interface 8, 1521–1549. doi:10.1098/rsif.2011.0054
Whelan, I. T., Burdis, R., Shahreza, S., Moeendarbary, E., Hoey, D. A., and Kelly, D. J. (2023). A microphysiological model of bone development and regeneration. Biofabrication 15, 034103. doi:10.1088/1758-5090/acd6be
Whisler, J., Shahreza, S., Schlegelmilch, K., Ege, N., Javanmardi, Y., Malandrino, A., et al. (2023). Emergent mechanical control of vascular morphogenesis. Sci. Adv. 9, eadg9781. doi:10.1126/sciadv.adg9781
Wirtz, D., Konstantopoulos, K., and Searson, P. C. (2011). The physics of cancer: the role of physical interactions and mechanical forces in metastasis. Nat. Rev. Cancer 11, 512–522. doi:10.1038/nrc3080
Wolf, K., and Friedl, P. (2009). Mapping proteolytic cancer cell-extracellular matrix interfaces. Clin. Exp. Metastasis 26, 289–298. doi:10.1007/s10585-008-9190-2
Wolf, K., Mazo, I., Leung, H., Engelke, K., von Andrian, U. H., Deryugina, E. I., et al. (2003). Compensation mechanism in tumor cell migration: mesenchymal-amoeboid transition after blocking of pericellular proteolysis. J. Cell Biol. 160, 267–277. doi:10.1083/jcb.200209006
Xu, W., Mezencev, R., Kim, B., Wang, L., Mcdonald, J., and Sulchek, T. (2012). Cell stiffness is a biomarker of the metastatic potential of ovarian cancer cells. PLoS One 7, e46609. doi:10.1371/journal.pone.0046609
Yang, H., Zhang, H., Yang, Y., Wang, X., Deng, T., Liu, R., et al. (2020). Hypoxia induced exosomal circRNA promotes metastasis of Colorectal Cancer via targeting GEF-H1/RhoA axis. Theranostics 10, 8211–8226. doi:10.7150/thno.44419
Zen, K., Liu, D.-Q., Guo, Y.-L., Wang, C., Shan, J., Fang, M., et al. (2008). CD44v4 is a major E-selectin ligand that mediates breast cancer cell transendothelial migration. PLoS One 3, e1826. doi:10.1371/journal.pone.0001826
Zervantonakis, I. K., Hughes-Alford, S. K., Charest, J. L., Condeelis, J. S., Gertler, F. B., and Kamm, R. D. (2012). Three-dimensional microfluidic model for tumor cell intravasation and endothelial barrier function. Proc. Natl. Acad. Sci. 109, 13515–13520. doi:10.1073/pnas.1210182109
Keywords: cancer, mechanobiolgy, extracellular matrix (ECM), metastasis (cancer metastasis), invasion, mechanotherapeutics
Citation: Chen MB, Javanmardi Y, Shahreza S, Serwinski B, Aref A, Djordjevic B and Moeendarbary E (2023) Mechanobiology in oncology: basic concepts and clinical prospects. Front. Cell Dev. Biol. 11:1239749. doi: 10.3389/fcell.2023.1239749
Received: 14 June 2023; Accepted: 10 October 2023;
Published: 31 October 2023.
Edited by:
Namrata Gundiah, Indian Institute of Science (IISc), IndiaReviewed by:
Susan E. Leggett, University of Illinois at Urbana-Champaign, United StatesCopyright © 2023 Chen, Javanmardi, Shahreza, Serwinski, Aref, Djordjevic and Moeendarbary. This is an open-access article distributed under the terms of the Creative Commons Attribution License (CC BY). The use, distribution or reproduction in other forums is permitted, provided the original author(s) and the copyright owner(s) are credited and that the original publication in this journal is cited, in accordance with accepted academic practice. No use, distribution or reproduction is permitted which does not comply with these terms.
*Correspondence: Emad Moeendarbary, ZS5tb2VlbmRhcmJhcnlAdWNsLmFjLnVr
†These authors have contributed equally to this work