- 1Division of Cardiology, Department of Medicine, Emory University, Atlanta, GA, United States
- 2Instituto de Ciencias Biomédicas, Facultad de Ciencias de la Salud, Universidad Autónoma de Chile, Santiago, Chile
- 3Cellular Communication Laboratory, Programa de Biología Celular y Molecular, Center for Studies on Exercise, Metabolism and Cancer (CEMC), Instituto de Ciencias Biomédicas (ICBM), Facultad de Medicina, Universidad de Chile, Santiago, Chile
- 4Advanced Center for Chronic Diseases (ACCDiS), Faculty of Chemical and Pharmaceutical Sciences & Faculty of Medicine, Universidad de Chile, Santiago, Chile
Cell adhesion and migration depend on the assembly and disassembly of adhesive structures known as focal adhesions. Cells adhere to the extracellular matrix (ECM) and form these structures via receptors, such as integrins and syndecans, which initiate signal transduction pathways that bridge the ECM to the cytoskeleton, thus governing adhesion and migration processes. Integrins bind to the ECM and soluble or cell surface ligands to form integrin adhesion complexes (IAC), whose composition depends on the cellular context and cell type. Proteomic analyses of these IACs led to the curation of the term adhesome, which is a complex molecular network containing hundreds of proteins involved in signaling, adhesion, and cell movement. One of the hallmarks of these IACs is to sense mechanical cues that arise due to ECM rigidity, as well as the tension exerted by cell-cell interactions, and transduce this force by modifying the actin cytoskeleton to regulate cell migration. Among the integrin/syndecan cell surface ligands, we have described Thy-1 (CD90), a GPI-anchored protein that possesses binding domains for each of these receptors and, upon engaging them, stimulates cell adhesion and migration. In this review, we examine what is currently known about adhesomes, revise how mechanical forces have changed our view on the regulation of cell migration, and, in this context, discuss how we have contributed to the understanding of signaling mechanisms that control cell adhesion and migration.
1 Introduction
Cell migration is essential during homeostatic and pathological processes in multicellular organisms. Cells can migrate individually or collectively but their movement is rarely random; thus, migration is directional and cells move commanded by the myriads of cues that surround them (Shellard and Mayor, 2020). In addition, cellular events occurring during migration, such as cell adhesion, polarity, and mechanical strain, are tightly regulated in a spatiotemporal fashion by these environmental cues, which include the type, amount, and properties of the extracellular matrix (ECM) surrounding the cells (Yamada et al., 2019; Yamada and Sixt, 2019; Doyle et al., 2022). Cells interact with the ECM through integrin receptors to promote cell migration (Wu et al., 2017).
Integrins are α and β transmembrane heterodimers that bind ECM proteins, as well as cell receptors and soluble ligands. In humans, at least 18 α and 8 β subunits have been described, generating 24 heterodimers, which recognize and interact with specific ligands. Integrin activation relies on conformational changes triggered after interacting with their ligands (outside-in) and cytoplasmic tail-binding partners (inside-out) (Huttenlocher and Horwitz, 2011).
The initial engagement of integrins with the ECM forms Nascent Adhesions (NAs), which are small dot-like structures (∼0.25 μm diameter) composed of around 50 integrin dimers that serve as platforms for the recruitment and activation of numerous proteins (Changede et al., 2015; Henning Stumpf et al., 2020). NAs are transient structures with a short lifetime (∼1 min) that primarily form in the lamellipodium of migrating cells. Even though actin polymerization is necessary for the formation of NAs, they form and persist independently of the activity of non-muscle Myosin IIA. NAs that remain assembled grow to form Focal Contacts (FCs). These FCs are larger in size (1 μm), localize in the lamellipodium-lamella interface, and their formation depends on myosin activity. FCs are transient structures that display a 1–2 min lifetime and then mature into larger and elongated structures known as Focal Adhesions (FAs) (Figure 1). FAs exhibit lifetimes of several minutes and a broad spectrum of sizes (1 μm W x 3–5 μm L) due to their dynamic maturation process. Actin crosslinking mediated by α-actinin and Myosin IIA mediates the initial maturation of FAs, which directly bind to the actomyosin cytoskeleton to transduce cellular strain. Contractile actin microfilament bundles and Myosin crosslinked by several proteins form Stress Fibers (SFs) (red staining, Figure 1), which are involved in cell adhesion, migration, and mechanotransduction. SF contractility can either help FA maturation or promote their disassembly (Echtermeyer et al., 2001; Oakes et al., 2012; Burridge and Guilluy, 2016; Livne and Geiger, 2016).
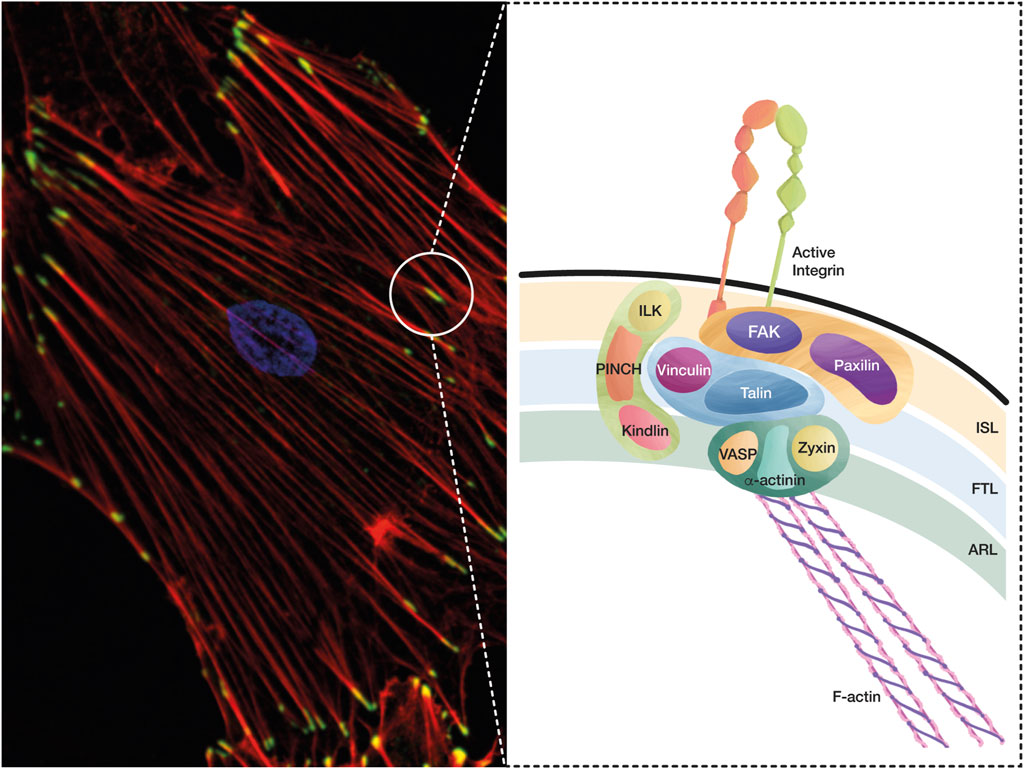
FIGURE 1. A conceptual map of the adhesome. The integrin adhesome is a protein complex that has been defined through careful evaluation of several proteomic analyses of IACs. These complexes formed by integrin-ligand interaction lead to FA and SF formation, which are essential structures in cell adhesion and migration, and play a key role during mechanotransduction. (A) The microphotography shows FAs in green (Vinculin staining, Alexa488), SFs in red (Phalloidin, Alexa594), and the nucleus in blue (DAPI). (B) Based on the interactions and functions of IACs during integrin adhesion and signaling, a consensus integrin adhesome has been curated into four nodes, which contain: i) ILK–PINCH–Kindlin, ii) FAK–Paxillin, iii) Talin–Vinculin, and iv) α-actinin–Zyxin–VASP. These protein nodes organize in three interconnected layers: i) the integrin signaling layer (ISL) that contains integrin tails, Paxillin, and FAK; ii) the force transducing layer (FTL) that includes the mechanotransducer proteins Talin and Vinculin, which are the physical link between integrins and the filamentous actin (F-Actin); and iii) the actin regulatory layer (ARL) that contains actin-regulatory proteins, such as VASP, Zyxin, and α-actinin.
Cell migration requires the integration and coordination of specific FA dynamics at the front, center, and rear of the cells. Migrating cells continuously form and disassemble their adhesions at the leading edge. FAs mature at the front edge and towards the side and back of the cells. Then, actin SFs attached to FAs contract and retract the rear end, and lastly, adhesions at the tail disassemble, resulting in forward movement. This process of constant assembly/disassembly of FAs is termed adhesion turnover (Webb et al., 2002). FA turnover is tightly regulated in a spatiotemporal manner by actin depolymerization and reorganization (Alexandrova et al., 2008; Shemesh et al., 2009), microtubule dynamics (Small et al., 2002), Calpain proteolysis (Franco and Huttenlocher, 2005), integrin endocytosis (Laukaitis et al., 2001; Broussard et al., 2008; Ezratty et al., 2009), and mechanical tension (Crowley and Horwitz, 1995; Wolfenson et al., 2011), among others. Thus, the front-to-rear polarity that governs cell migration is tightly regulated by many signaling pathways and feedback mechanisms that control cytoskeletal and FA dynamics.
Hundreds of proteins have been described within mature FAs. These proteins constitute “The Cell Adhesome”, composed of many types of proteins: adhesion receptors, signaling proteins, enzymes (protein kinases, phosphatases, and proteases), and cytoskeletal, adapter, and scaffold proteins, which interact with many others, thus forming large and complex networks (examples and references in Table 1) (Zaidel-Bar et al., 2007a). When multiple adhesome components are mutated or dysregulated, these adhesive structures mediating cell–ECM interactions lead to abnormal migration and invasion, which have been implicated in pathological conditions, such as cancer and cardiovascular diseases (Winograd-Katz et al., 2014; Byron et al., 2015).
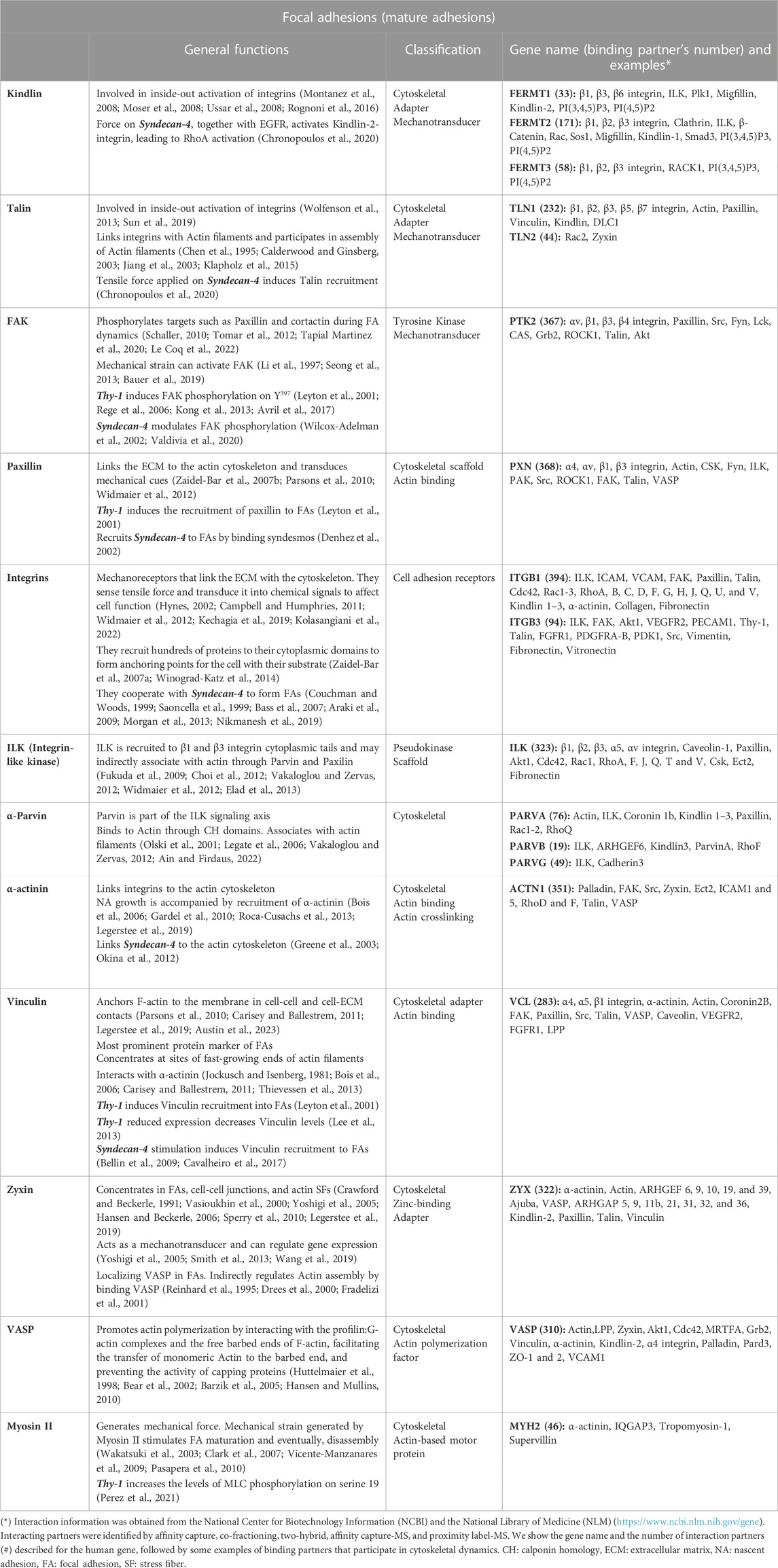
TABLE 1. General functions, classification, gene name, and binding partner’s examples of proteins forming mature focal adhesions.
Cells migrate in response to an enormous amount of cues, which have been separated in diverse categories according to the type of signal [reviewed in (Shellard and Mayor, 2020; SenGupta et al., 2021)]. The best described modes of cell migration are chemotaxis (soluble chemical cues), haptotaxis (immobilized cues), and durotaxis (mechanical cues). In chemotaxis, soluble chemical ligands bind to membrane receptors that concentrate at the cell front, thus leading to polarization of signaling molecules (SenGupta et al., 2021). During haptotaxis, gradients of immobilized ligands in extracellular sites are detected by molecules in FAs, promoting signals that are like those triggered by chemical signals (King et al., 2016). In durotaxis, mechanical forces exerted by ECM rigidity (Stephens et al., 2008; SenGupta et al., 2021), also known as stiffness, or pulling forces exerted by cell-cell interaction, transduce mechanical signals in a process known as mechanotransduction (Rens and Merks, 2020). Overall, all these signaling pathways, induced by different cues, translate into modifications of the actin cytoskeleton that regulate directional cell migration.
ECM proteins are the most typical integrin ligands but not the only ones. Other ligands include growth factor receptors that regulate cell proliferation, differentiation, and migration; as well as other cell surface receptors that mediate cell-cell communication (Brizzi et al., 2012; LaFoya et al., 2018). The latter category includes the integrin receptor (ligand) identified by our group, Thy-1, also known as CD90 (Leyton et al., 2001). Thy-1 is a cell adhesion molecule that contains a single Arg-Gly-Asp (RGD)-like peptide, which binds and activates different integrins, thus favoring communication between cells. Thy-1 also possesses a heparin-binding domain (HBD), which activates Syndecan-4, an equally important player of the cell migration process (Valdivia et al., 2020). Importantly, evidence reported by our group has contributed to identifying two participants of the integrin adhesome: Thy-1 as a cellular receptor for integrins (Leyton et al., 2001; Zaidel-Bar et al., 2007a), and the cell polarization protein Partition-defective 3 (PAR-3) (Valdivia et al., 2020). We initially described Thy-1 as the binding partner of integrin β3 (Leyton et al., 2001), and subsequently, Thy-1 was categorized by Geiger and collaborators as an associate component of the avb3 integrin adhesome (Zaidel-Bar et al., 2007a), whereas PAR-3 was reported to interact with Syndecan-4 to control FAK phosphorylation (Valdivia et al., 2020). Additionally, the interactions between Thy-1 and the mechanoreceptors integrin and Syndecan-4 led us to explore the effect of force on Thy-1-induced effects (Burgos-Bravo et al., 2020; Perez et al., 2021), which we discuss in this review.
In this article, we also describe the general structure and organization of adhesomes, we portray mechanobiology as an important regulator of adhesion and migration, and finally, we contrast and discuss how 20 years of our research converged and helped to elucidate new mechanisms in the context of cell adhesion and migration mediated by the interaction of Thy-1 with αvβ3 integrin and Syndecan-4.
2 Adhesomes: a growing list of proteins participating in adhesion and migration
2.1 The integrin adhesome
The term adhesome was first used by Richard Hynes in 2006 to describe the cluster of cell-cell and cell-matrix adhesion receptors in an organism (Whittaker et al., 2006). Benny Geiger and coworkers then expanded this concept to include the entire network of structural and signaling proteins that regulate the adhesion of cells to the ECM (Zaidel-Bar et al., 2007a; Zaidel-Bar and Geiger, 2010; Winograd-Katz et al., 2014). Since the major cell-matrix adhesion receptors are integrins, the adhesome containing this type of receptor is currently known as the “integrin adhesome” and contains cytoskeletal proteins recruited to integrin cytoplasmatic tails, which mediate integrin signaling functions (Winograd-Katz et al., 2014; Horton, 2021). Therefore, integrins and the signaling emanating from these receptors are the central components of the adhesome.
An integrin adhesome composed of 2,412 proteins was generated by integrating several integrin adhesion complex (IAC) proteomes (Chastney et al., 2021). Different biochemical approaches and rigorous curation of seven available data sets helped define a consensus integrin adhesome composed of 60 core proteins, such as Palladin, Vinculin, Paxillin, FAK, Tensin, and VASP (Horton et al., 2015). Of these 60 proteins, 42 were clustered into four nodes based on their interactions and previous functional implications in integrin adhesion and signaling: i) ILK–PINCH–Kindlin, which links signaling of receptor tyrosine kinases to integrins, ii) FAK–Paxillin, a node that facilitates FA turnover, iii) Talin–Vinculin, which provides a link between the FA complex and the cytoskeleton, and iv) α-actinin–Zyxin–VASP, which connects SFs to integrins through Vinculin (Horton et al., 2015) (Figure 1). This core integrin adhesome fulfills central functions of integrin adhesions: linkage of the ECM to F-actin, regulation of F-actin organization and dynamics, and signal transduction. The other 18 proteins did not have reported functions at FAs or interactions with other adhesome members (Han and de Rooij, 2016).
Decades of investigations have revealed that the large repertoire of FA proteins found in integrin adhesomes organizes at the nanoscale level in three interconnected layers or modules (Kanchanawong et al., 2010) running parallel to the cell membrane, rather than in three independent layers. Advances in three-dimensional super-resolution fluorescence microscopy techniques revealed these three distinct nanolayers, where integrins and actin are vertically separated by a ∼40 nm FA core region consisting of multiple protein-specific strata: i) the integrin signaling layer (ISL) lies closest to the adherent membrane (within ∼10–20 nm) and contains the cytoplasmic tails of integrins that interact with the signaling proteins Paxillin and FAK; ii) the actin-regulatory layer (ARL) is located approximately 50 nm below the ISL, associates with actin SFs, and contains actin-modulating proteins, such as Zyxin, VASP, and α-actinin; and iii) the force transducing layer (FTL) lies in between the ISL and ARL, and contains Talin and Vinculin (and possible interactors), which form a physical link that transmits force between integrins and the actin cytoskeleton (Kanchanawong et al., 2010; Case and Waterman, 2015; Xia and Kanchanawong, 2017) (Figure 1). Each of these three nanolayers contains the main proteins found in three of the four integrin adhesome interaction nodes mentioned above. The head domain of Talin interacts with the cytoplasmic tail of integrins, while its C-terminal rod domain binds F-actin, and these interactions physically link the ISL and ARL (Calderwood and Ginsberg, 2003; Yao et al., 2014). On the other hand, integrins and associated complexes segregate laterally into nanoclusters with distinct integrin activities and mechanical properties (Shroff et al., 2007; Spiess et al., 2018). As reported, the binding of proteins, such as Paxillin, VASP, Zyxin, and Vinculin, to FAs is dynamically regulated according to FA orientation (FAs aligned to the long-axis or perpendicular to the cell edge) and location (FAs close to or away from the cell edge) (Legerstee et al., 2019). Additionally, the composition of the nanolayers in FAs is very dynamic, and could constantly change depending on various factors, such as the cell microenvironment, ECM stiffness, cell contractility, or signaling due to phosphorylation/dephosphorylation of many proteins (Manninen and Varjosalo, 2017; Tang et al., 2018). These dynamic changes in the organization of FAs and their components can affect different cellular responses, including cell adhesion and migration.
2.2 Regulation of adhesomes by protein domains
Adhesomes are enriched in various protein domains, such as the Pleckstrin homology (PH) and FERM domains, which target proteins to the plasma membrane; the Calponin homology (CH) domain, which binds to F-actin and plays essential roles in cytoskeletal dynamics and signaling; the Src homology 2 (SH2) domain, which interacts with phosphorylated tyrosine residues; and the armadillo (ARM) and LIM domains, which mediate specific protein-protein binding (Liu and Lauffenburger, 2009). One of the most important domains found in FA proteins is the SH2 domain. Tyrosine-specific kinases and phosphatases within FAs regulate the phosphorylation status of their substrates, creating docking sites for proteins with SH2 domains (Pawson et al., 2001). The transient localization of tyrosine-specific kinases and phosphatases within FAs probably plays a key role in dynamically controlling the association of other SH2 domain-containing FA proteins within FAs and thus, regulates the composition of FAs and FA-mediated signaling. For instance, FAK signaling results from its autophosphorylation on Tyr-397 (pY397) in response to integrin-mediated adhesion, allowing interactions with multiple SH2-containing signaling proteins that bind to this site, including Src, PI3K, Grb7, PLCγ1, and Nck-2 (Wu et al., 2015). Src is a non-receptor protein tyrosine kinase that contains an N-myristoylation site, as well as SH3, SH2, and kinase domains (Kaplan et al., 1994). Once recruited, Src phosphorylates two FAK-interacting proteins, Crk-associated substrate (CAS) and Paxillin, which regulate the activation of Rho-family GTPases controlling cell motility (Hanks et al., 2003). Among the phosphatases within FAs, the SH2-domain-containing inositol 5′-phosphatases (SHIPs), SH2-domain-containing PTP2 (SHP2), and SH2-domain-containing protein tyrosine phosphatase substrate 1 (SHPS1) have been found to bear SH2 domains that are required for locating in proximity to their target proteins. These phosphatases decrease the number of docking sites for proteins recruited to FAs and inactivate kinases (Saxton et al., 2000; Sattler et al., 2001). Of note, many other phosphatases, such as PP2A, SAP1, PTP-PEST, and PTP1B, are targeted to FAs to regulate adhesion and migration (Larsen et al., 2003; Frijhoff et al., 2014). In the case of LIM domains, the biochemical and structural nature of most of the proposed LIM domain-mediated interactions has not been precisely defined yet. However, many proteins containing multiple LIM domains are recruited to FAs under mechanical tension, such as LIMK1 and LIMK2, which stabilize the actin cytoskeleton by phosphorylating and inactivating Cofilin (Schiller and Fässler, 2013; Sun and Alushin, 2022). Therefore, LIM domains were postulated as tension sensors and localizers, targeting proteins to specific subcellular locations, such as tensioned or injured F-actin networks (Schiller et al., 2011; Smith et al., 2013).
These examples of regulation by protein domains present in many components of the adhesome are helping us to understand the structural interactions required for the physiological functioning of FAs at the level of their assembly, mechanosensing, and signal transduction and how these processes control cell migration (Ripamonti et al., 2021).
2.3 Regulation of adhesomes by molecular switches
The interactions between the various adhesome components regulate two major functions of adhesion sites: first, their role as “scaffolds”, which allows the physical interaction between the ECM-bound cell and the cytoskeleton, leading to the assembly of tissues with a particular structure and mechanical properties; and second, their “signaling” activity, through which cells can sense the chemical and mechanical properties of the external environment and respond accordingly by activating signaling pathways that regulate cell structure, dynamics, behavior, and fate (Winograd-Katz et al., 2014). More than half of the links interconnecting different adhesome components can be switched on or off by signaling elements, and some of these regulated interaction switches are conformational switches, GTPase switches, lipid switches, proteolytic switches, and phosphorylation switches (kinase/phosphatase balance). During conformational switches, adhesion proteins can be found in a folded, inactive state, and after binding to a lipid or a GTPase, being phosphorylated/dephosphorylated, or suffering mechanical strain, they change conformation into an open, active state that exposes new binding sites (Zaidel-Bar et al., 2007a). Integrins are a well-known example of a conformational switch because they thermodynamically fluctuate between different conformations. They are kept latent in a low-affinity conformation by inhibitory molecules. Endogenous inhibitory proteins like shank-associated RH domain-interacting protein (SHARPIN) and mammary-derived growth inhibitor (MDGI) maintain the bent conformation of integrins by binding to the cytoplasmic tail of the α integrin subunit to inhibit β1 integrin (Bouvard et al., 2013). Other inhibitory proteins are integrin cytoplasmic-associated protein-1 (ICAP-1) and Filamin A. ICAP-1 inactivates β1 integrins, while Filamin A binds to β1, β3, and β7 integrin cytoplasmic tails, stabilizing their bent conformation [reviewed by (Bouvard et al., 2013; Pang et al., 2023)]. Integrins then switch to a high-affinity conformation after interacting with the ECM. This high-affinity conformation is stabilized by mechanical forces transduced via Talin and Kindlin. Tensional forces induce integrin conformational changes both outside (headpiece extension) and inside the cell (leg opening) (Puklin-Faucher and Vogel, 2009; Thinn et al., 2018) and thereby, promote ligand binding and recruitment of adapter proteins in the cytoplasm to strengthen the integrin and actomyosin linkage. Therefore, adhesion is further stabilized by the formation of catch bonds (characterized by an increase in the lifetime of the bond after increasing the force applied) and integrin clusters (Askari et al., 2009; Sun et al., 2019; Kolasangiani et al., 2022). Each of the three adhesome vertical layers or modules mentioned in Section 2.1 contains mechanosensitive proteins that can undergo conformational changes or post-translational modifications under tensile forces, thus leading to changes in force transduction and/or induction of biochemical signaling, such as activation of FAK, Src, and Rho family GTPases (Sun et al., 2016).
The Rho family of small GTPases are also considered molecular switches that cycle between an “off” state (GDP-bound) and an “on” state (GTP-bound). Moreover, their activity is regulated by GTPase-activating proteins (GAPs) that accelerate their intrinsic GTPase activity through guanine nucleotide exchange factors (GEFs) that induce the release of GDP to allow their activation, or by guanine nucleotide dissociation inhibitors (GDIs), which stabilize the inactive GTPases (Arthur et al., 2002; Burridge and Wennerberg, 2004).
Among the lipid switches, the most studied is the PI(4,5)P2 to PI(3,4,5)P3 switch catalyzed by PI3K. Phosphoinositides differentially distribute in migrating cells, where PI(4,5)P2 localizes at the leading edge and PI(3,4,5)P3 at the rear end. In particular, PI(4,5)P2 has been related to FA turnover at the leading edge (Ijuin, 2019; Posor et al., 2022). Additionally, phosphoinositides participate in the oligomerization of receptors, such as the heparan sulfate proteoglycan Syndecan-4, an event necessary for the activation of its downstream signaling (Oh et al., 1997).
Lastly, one of the best-studied examples of a proteolytic switch is the cysteine protease Calpain. Calpain is activated by increased calcium concentration and cleaves various proteins of the integrin adhesome. Calpain is involved in FA turnover at the rear end by inducing proteolysis of FA proteins, such as Talin, FAK, Paxillin, and Vinculin (Chan et al., 2010; Cortesio et al., 2011; Ono and Sorimachi, 2012). Therefore, Calpain could represent a mechanism for regulating FA turnover, which is important in processes like cell adhesion, spreading, and migration (Franco et al., 2004; Wells et al., 2005; Rodriguez-Fernandez et al., 2021).
Overall, processes such as cell adhesion and migration are cyclically regulated and tightly coordinated by the linked interconnections occurring within the adhesome, where several of the proteins involved are switched on and off to maintain the dynamics of the process.
2.4 Regulation of cell migration by FA dynamics
FA dynamics or turnover refers to the cyclic assembly and disassembly of FAs. In migrating cells, FA turnover is precisely controlled by the coordination of several signaling pathways and the modulation of tensile forces (Broussard et al., 2008). The initial assembly of FAs is mediated by actin polymerization and treadmilling, which occurs either at the edge of the lamellipodium or at the tip of the invading protrusion. Application of mechanical stress by the treadmilling of actin to nearby adhesion molecules (mostly integrins and associated components, such as Talin, Vinculin, and FAK/PYK2) then triggers the assembly of functional adhesion structures (Revach et al., 2020). Integrin engagement, combined with the local tensile force, is essential and sufficient to activate these mechanosensitive adhesome components, resulting in the development and growth of FCs into FAs. The subsequent assembly and growth of an “adhesion plaque” is enriched with actin-binding adhesome components (including Talin and Vinculin) and also augments outward pressure by acting on the leading edge of the lamellipodium, thus driving cell migration (Revach et al., 2020). These specific events in FA dynamics occurring in different subcellular locations create a complex adhesion and migration machinery that is essential for cellular function.
Evidence has shown that FA disassembly is promoted by the cleavage of FA proteins in the rear end, protein phosphorylation, and the dissociation of adapter proteins (Parsons et al., 2000; Legerstee and Houtsmuller, 2021; Mishra and Manavathi, 2021). Other modes of FA disassembly involve microtubules, which traditionally have been considered key to triggering FA turnover, due to the crosstalk between microtubules and actin. Forces within FAs may exert feedback on microtubules to complete FA turnover, and vice versa (Burridge and Guilluy, 2016; Gupta et al., 2016). The evidence indicates that polymerizing microtubules have a role in the activation of the Rho family member Rac, which in turn promotes actin polymerization, membrane protrusion, FA turnover, and migration (Seetharaman and Etienne-Manneville, 2019). As reported in astrocytes, acetylated microtubules control the distribution and dynamics of FAs and the release of GEF-H1 from microtubules to the cytosol, where it activates RhoA-actomyosin contractility and generates forces to promote collective migration of astrocytes (Chang et al., 2008; Seetharaman et al., 2022). Therefore, as for the actin cytoskeleton, microtubules also play a crucial role in FA dynamics.
Indeed, pioneering live imaging experiments showed that microtubules use actin SFs as tracks to direct themselves to the vicinity of FAs (Kaverina et al., 1998). This microtubule guidance along SFs requires the crosslink protein MACF1/ACF7 to reach FAs (Wu et al., 2008). Microtubules are then captured and stabilized around FAs through their interaction with KANK1 and Talin, which in turn, bind to actin (Rafiq et al., 2019). Microtubules retract and regrow to contact FAs several times until FAs reach full maturation (Seetharaman and Etienne-Manneville, 2020). Fully mature FAs are then “sensed” by microtubules, which deliver autophagosomes to the FAs, and concomitantly retract (Kenific and Debnath, 2016). Autophagosomes contain LC3, which recognizes the cargo receptors NBR1 and c-Cbl that interact with Paxillin when phosphorylated by Src (Sharifi et al., 2016; Chang et al., 2017). These interactions activate signaling events involved in the removal of phosphorylated FA components that may lead to FA disintegration (Sandilands et al., 2011; Lu et al., 2021). Although the process of autophagy to selectively engulf FAs is poorly understood, it represents another mode of FA dynamics regulation requiring the communication of FAs with microtubules.
Another study in lung microvascular endothelial cells revealed that manipulation of β3 integrin expression leads to β3 integrin-dependent changes in microtubule behavior (Atkinson et al., 2018). According to these authors, engagement of αvβ3 integrin with fibronectin (FN) at mature FAs localizes a Rcc2/Anxa2/Rac1 complex to these sites to actively destabilize microtubules (perhaps by controlling its exposure to GEFs). Interestingly, when αvβ3 integrin was not present, the complex associated instead with α5β1 integrin and exerted the opposite effect on microtubules. This re-positioning of Rac1 activity means that this small GTPase plays a role in microtubule-linked endothelial cell migration only when αvβ3 integrin is not present in mature FAs (Atkinson et al., 2018).
Another group of cytoskeletal elements that interplay with actin to control FA maturation is septins [see review in (Cavini et al., 2021)]. Septins are GTP-binding proteins that form oligomeric complexes, which further assemble into filaments, gauzes, and rings that interact with the membrane and the cytoskeleton. Knockdown of SEPT2 or SEPT9 in migrating MDCK cells led to a higher number of smaller FAs closer to the cell edge (Dolat et al., 2014). Although assembly and disassembly rates of NAs were the same as in control cells, the stabilization phase was abolished in SEPT2 knockdown cells, resulting in short-lived FAs that failed to mature (Dolat et al., 2014). SEPT2 knockdown in cancer-associated fibroblasts was also reported to lead to fewer FAs, whereas SEPT2 or SEPT9 knockdown in murine and human melanoma cells led to a decrease in FA size (Calvo et al., 2015; Farrugia et al., 2020). How septins affect FA maturation and, thus the lifetime of FAs is still not clear since septins are largely excluded from FAs, as shown by immunostainings in NRK, MDCK, and U2OS cells. The role of septins in FA stabilization is most likely due to their association with FA-anchored SFs. The current hypothesis is that septins, through their actin filament cross-linking activity, maintain the integrity and organization of SFs, which in turn are required for the stabilization and maturation of FAs (Livne and Geiger, 2016).
Therefore, the evidence revealed that timely FA turnover and directed cell migration depend on the interplay between many molecular factors beyond microtubules (Mavrakis and Juanes, 2023).
2.5 Advances in the understanding of how integrin adhesomes regulate migration
The main challenges in studying the molecular functionality of adhesomes have been: i) the molecular heterogeneity of integrin adhesions within and between different cellular systems, ii) the large number of adhesome components, iii) the participation of multiple “functional switches” that can turn adhesome components “on” or “off”, and iv) the overall dynamic nature and plasticity of the adhesion system as a whole (Winograd-Katz et al., 2014). To add to this complexity, the repertoire of ECM components (collectively known as the “matrisome”), is rather wide and includes over 1,000 “core” and “associated” components (Naba et al., 2016). Many integrin-associated proteins and their differential interaction with the plasma membrane form a puzzle with 200 to 1,000 different pieces, for which there is only limited structural and biochemical information. Furthermore, many of these adhesome proteins undergo conformational changes under tensional stress, thus increasing the intricacy of adhesion sites (Bachmann et al., 2019). Due to the underlying complexity of the matrisome and adhesome, it has been historically challenging to undertake mass spectrometry-based profiling for the characterization of the ECM and IACs because of the low affinity and transient nature of the molecular interactions occurring at these sites. Despite these difficulties, the use of cell-permeant chemical cross-linkers improved the recovery of IAC proteins bound to either FN-coated microbeads or plastic dishes (Schiller et al., 2011; Horton et al., 2015). These advances led to the characterization of IACs from several cell types. Even though there is a consensus adhesome, the composition and stoichiometry of the meta-adhesome depend on the cell type being analyzed, the integrin-receptor repertoire expressed by that cell type, the turnover of FAs, and the experimental conditions that are used. Recent technical, methodological, and bioinformatic advances in proteomics have shown promise in characterizing the matrisome and topology of adhesome networks in both health and disease (Krasny and Huang, 2021). In addition, tight communication between the matrisome and adhesome controls the cytoskeleton and thus, cell migration [for more details, see (Krasny and Huang, 2021)].
Cell adhesion receptors like Thy-1 form part of the associated components of the adhesome due to their interaction with α and β integrin subunits, which are intrinsic adhesome components (Leyton et al., 2001; Zaidel-Bar et al., 2007a). Our group was the first to report the presence of a single RGD-like peptide in a conserved sequence of the human, rat, and mouse Thy-1 polypeptide (Leyton et al., 2001). The tripeptide found in Thy-1 was an RGD-like peptide (RLD), which recognizes the αvβ3 integrin present in astrocytes (Leyton et al., 2001; Hermosilla et al., 2008). This association, which reportedly occurs with many other integrins (Leyton et al., 2019), was recognized as part of the dense connectivity of the adhesome network (Zaidel-Bar et al., 2007a), and was also backed up by our findings, reporting that FAs formed due to Thy-1/integrin binding contain many proteins described in IACs [(see Section 2.1, and (Leyton et al., 2001)].
We additionally described that Thy-1 induces cell migration in astrocytes by interacting not only with integrins but also with Syndecan-4, which plays a known role in cell movement (Avalos et al., 2009). Using mass spectrometry to identify potential Syndecan-4-binding partners, we discovered that PAR-3 was one of the binding proteins, through an interaction dependent on the carboxy-terminal EFYA sequence present on Syndecan-4. When PAR-3 expression was reduced, Thy-1-induced cell migration and FA disassembly were impaired (Valdivia et al., 2020). PAR-3, a protein that plays a central role in establishing and maintaining cell polarity in various cell types (Harris, 2017), was thus defined as a novel adhesome-associated component, with an essential role in FA disassembly during polarized cell migration.
3 How mechanobiology has changed our view on cell migration
Cell migration aids in relevant physiological activities, such as embryonic development, morphogenesis, and wound healing. Since living cells are constantly exposed to mechanical stimulation, cell migration is influenced not only by chemical but also by mechanical cues (Mierke, 2020; SenGupta et al., 2021). Thus, forces coming from the extracellular (outside-in) and intracellular (inside-out) environment can control many cellular responses, including migration.
Mechanosensitive receptors expressed at the cell surface interact with the surrounding ECM or receptors on other cells to transduce force toward the inside of the cell. Integrins are considered the classical mechanoreceptors. The engagement of integrins with their ligands induces the formation of FAs and SFs to generate, transmit, and sense mechanical tension (Baker and Zaman, 2010; Schwartz, 2010; Sun et al., 2016). As previously mentioned, 3D super-resolution microscopy has defined FA nanoarchitecture, emphasizing a central force-transduction layer (FTL; see Section 2.1) containing the proteins Talin and Vinculin, which are essential during mechanotransduction because they undergo conformational changes after tension [reviewed in (Kanchanawong et al., 2010; Yan et al., 2015; Haining et al., 2016; Legerstee and Houtsmuller, 2021)]. This intricate FA architecture is tightly and timely controlled. The initial clustering of integrins sequentially recruits proteins such as Talin, Paxillin, and lower levels of Vinculin and FAK, to form NAs that grow into FCs. These FCs are small (< 1 μm2; e.g., 0.11 μm2 in NIH3T3 cells growing on FN) and unstable since they cannot sustain the tension of the cytoskeleton. Paradoxically, using a tension gauge tether probe that binds αvβ3 integrin, Wang and Ha showed that in epithelial-like cells derived from the ovary (CHO-K1), a tension of 43 pN was required for the initial cell adhesion mediated by FCs (< 15 min after seeding), and that 56 pN was enough to mediate FA and SF formation (∼1 h after seeding) (Wang and Ha, 2013; Austin et al., 2023), supporting the idea that tension is also necessary for FCs to mature into FAs (< 1 μm2).
SFs mediate contractility mainly by Myosin II and α-actinin activity. The tension generated by SFs can directly act on FA proteins, regulating their function. Some of these proteins form catch bonds (bond lifetime increases with force), others can form slip bonds, where the bond lifetime decreases with increasing force, and a third group can form an ideal bond that is insensitive to mechanical stress. For instance, selectins and integrins can have a combined catch-slip bond behavior depending on the intensity of the strain that they sense. Experiments using force spectroscopy demonstrated that increasing force first prolonged and then shortened the lifetime of the bond between P-selectin and its ligand, indicating that a catch-to-slip bond switch occurs under force application. The mechanical properties of selectins when binding its ligands are essential for the adhesion of immune cells to the endothelium, and their subsequent detachment from the vessel wall when forces become too high due to blood flow (Sundd et al., 2013; Morikis et al., 2017). Studies using atomic force microscopy and purified α5β1 integrin showed that a tensional force between 10 and 30 pN stabilized the α5β1 integrin-FN interaction lifetime (catch bond); then, when the force was higher than 30 pN, the lifetime of the interaction decreased (slip bond) (Kong et al., 2009). Moreover, Daniel Müller and colleagues also observed a catch-slip transition using live fibroblasts seeded on a FN fragment; however, this catch-slip switch was observed after applying a 40 pN force (Strohmeyer et al., 2017). These observations support the idea that mechanical strain plays a pivotal role in regulating the binding of cell adhesion molecules, such as integrins, with their ligands. Most FA proteins switch their bond behavior when exposed to mechanical forces; therefore, to our knowledge, no examples of ideal bond formation have been described so far for FA proteins.
An asymmetrical strain distribution between the front and rear ends of the cell is crucial for cell migration. Consequently, two tension levels can be identified during migration: those corresponding to non-clustered integrins (FCs, 40 pN), and clustered integrins (FAs, 54 pN). The latter depends on actomyosin and corresponds to high-tension FAs connected to SFs (Wang X. et al., 2015). The disassembly of adhesions occurs at the leading edge on the protrusions during FA turnover and at the rear end after tail retraction. As discussed in the previous Section 2.4, FA disassembly is regulated by various factors, including mechanical tension (Crowley and Horwitz, 1995; Wolfenson et al., 2011). In this context, mechanical tension generated by Myosin II contraction of SFs promotes adhesion maturation, but if this tension is released, FAs disassemble. On the other hand, excessive tension leads to an abrupt loss of adhesion and cell detachment (Sawada et al., 2006; Peacock et al., 2007; Vicente-Manzanares et al., 2007; del Rio et al., 2009; Parsons et al., 2010). Thus, tensional forces are also essential in regulating the dynamic balance between FA formation and disassembly required for cell movement.
Tension can also be sensed and transduced by non-integrin receptors. Some of these receptors can sense shear stress (tangential force of a fluid on a cell layer), as is the case of mechanosensors expressed on the surface of endothelial cells in blood vessels (e.g., Heg-1 and PECAM-1), and other receptors can bind soluble ligands to control integrin activity (e.g., CXCR1 and CXCR2) or bind to the ECM and transduce force. Syndecan-4 has been considered a critical mechanoreceptor for its capacity to interact with the ECM and the actin cytoskeleton. Indeed, the interaction of Syndecan-4 with the HBD of FN induces FA formation (Saoncella et al., 1999; Woods et al., 2000). Consequently, Syndecan-4 deficiency impairs contractility and the formation of mature FAs (Longley et al., 1999; Okina et al., 2012; Cavalheiro et al., 2017). As previously reported, Syndecan-4 clusters in FAs and indirectly binds to FA proteins, such as Paxillin and Hic-5 (Denhez et al., 2002). Moreover, Syndecan-4 has been related to mechanotransduction during shear stress in endothelial cells by mediating diverse physiological effects, such as nitric oxide production, cell adhesion, sensing of the direction of flow, and endothelial cell alignment (Florian et al., 2003; Moon et al., 2005; Baeyens et al., 2014; Nikmanesh et al., 2019). Further studies, such as assays performed with electromagnetic tweezers and elastomeric membranes showed that Syndecan-4 activates mechanotransduction pathways involving ERK phosphorylation and MAPK signaling (Bellin et al., 2009). Similarly, the use of magnetic beads to apply 1 nN of tensional force pulses induced local stiffness, like that produced by integrins (Guilluy et al., 2011; Chronopoulos et al., 2020). Moreover, a constant tension of ∼200 pN applied to Syndecan-4 for 5 min induced the recruitment of Talin-1 and Kindlin-2 in an EGFR-PI3K-dependent manner. RhoA activation timely increased after constant tension, and this effect was blunted after β1 integrin signaling was inhibited by blocking the RGD domains of FN (Chronopoulos et al., 2020). Additional evidence has shown that Syndecan-4 ectodomains could physically interact with integrins (e.g., α3β1, α4β1, α6β4, αvβ3, and αvβ5) and receptor tyrosine kinases (e.g., EGFR, VEGFR2, IGF, and HER2), localize them in FAs and activate signaling pathways related to cell adhesion and migration (Wang H. et al., 2015; Rapraeger, 2021). These results highlight the fact that Syndecan-4 exerts a regulatory and cooperative effect during integrin-based mechanotransduction. Therefore, Syndecan-4 acts as a mechanosensor and regulates cell adhesion and migration. However, even though Syndecan-4 cooperates with integrins to mediate such effects, it is still unknown how the signaling pathways downstream of each receptor converge to potentiate each other.
Although the individual roles of integrins or Syndecan-4 during ECM-driven mechanotransduction have been broadly studied, much less is known about how these receptors sense mechanical cues during cell-cell interactions.
3.1 Integrins and Syndecan-4 mechanotransduction during cell-cell interactions
Integrins can mediate mechanotransduction during cell-cell interactions. αLβ2, α4β1, and αvβ3 integrin on the surface of leukocytes can interact with ICAM, VCAM, and PECAM-1 expressed on endothelial cells, respectively (Staunton et al., 1988; Elices et al., 1990; Buckley et al., 1996). For instance, ICAM-1 activation through leukocyte binding promotes cytosolic calcium-mediated myosin activity, leading to endothelial cell contractility. The resulting tension pulls VE-Cadherin in adherens junctions, causing gaps that allow leukocyte transmigration [reviewed in (Schwartz et al., 2021)]. Similarly, αLβ2 (LFA) and αMβ2 integrin in leukocytes can interact with the endothelial junctional adhesion proteins JAM-A and JAM-C, respectively, to allow leukocytes to migrate across the endothelial barrier (Ostermann et al., 2002; Imhof and Aurrand-Lions, 2004; Wojcikiewicz et al., 2009). The interaction force between LFA-1 and Mac-1, and their counterreceptor ligands [ICAMs, JAMs, and receptors for advanced glycation end products (RAGE)] have been extensively studied using single-molecule atomic force microscopy (Zhang et al., 2002; Wojcikiewicz et al., 2006; Yang et al., 2007; Li et al., 2018). In general terms, the strength of the bond depends on the integrin-ligand pair and correlates with the effectiveness of leukocyte adhesion to the endothelium. After attachment, the force is also transduced into the endothelial cells, affecting their contractility and disturbing the stability of tight and adherens junctions to mediate the transmigration of leukocytes through the endothelial layer. Another example is the interaction of leukocyte MAdCAM-1 expressed in high-endothelial venules and endothelial cells in the intestine, with α4β7 (LPAM-1) and α4β1 (VLA-4) integrin in myeloid cells. In this case, the role of MAdCAM-1 is to direct lymphocyte homing by interacting with integrins (Sun et al., 2014; Murakami et al., 2016). Integrins can also bind to receptors on the surface of cancer cells to mediate adhesion to the endothelium. For example, L1CAM on the surface of cancer-stem cell-like cells interacts with αvβ3 integrin in endothelial cells. This interaction promotes the activation of αvβ3 integrin and the phosphorylation of p130Cas and FAK, leading to enhanced migration towards bFGF (Burgett et al., 2016). Overall, these results confirm that integrins need to interact with their counterreceptors in other cells in order to transmit the force that leads to cell migration and invasion.
Less is known about Syndecan-4 mechanotransduction during cell-cell interactions. Although there is evidence that syndecans can interact with receptor tyrosine kinases, the corresponding ligands of these receptors are believed to promote this interaction. For example, VEGFA possesses a highly acidic HBD that allows its binding to the GAG chains of syndecans with different affinities. The strength of the VEGFA-syndecan interaction depends on the nature of the 6-O heparan sulfate chain sulfation, being optimal for Syndecan-2 and very low for Syndecan-1, -3, and -4. Most importantly, VEFGR2 exclusively co-precipitates with syndecans when stimulated with VEGFA. This indirect interaction positively regulates VEGFR2 activity and affects neovascularization and angiogenesis (Echtermeyer et al., 2001; De Rossi et al., 2014; Corti et al., 2019; De Rossi et al., 2021).
Similarly, Syndecan-4 has been involved more recently in regulating VE-cadherin function in the endothelium. In this case, Syndecan-4 interaction with VEGFA triggers the internalization of VE-cadherin, increasing vascular permeability and angiogenesis (De Rossi et al., 2021). Of note, VEGFR2 can also interact with VE-cadherin to regulate mechanotransduction (Coon et al., 2015). Surprisingly, the modulatory effect of Syndecan-4 on this signaling axis has not been studied in the context of mechanobiology, even though VEGFR2 and VE-cadherin both exert an individual and combined role during mechanotransduction in the endothelium (Barry et al., 2015; Coon et al., 2015; de Castro et al., 2015; Dorland and Huveneers, 2017; Mahajan et al., 2017; Miller and Sewell-Loftin, 2021).
Another Syndecan-4 receptor is Thy-1. Thy-1 is a unique counterreceptor possessing an RLD peptide that binds to integrin receptors, and an HBD, which interacts with Syndecan-4 (Leyton et al., 2019). Thus, Thy-1 is capable of simultaneously binding and activating integrins and Syndecan-4 to mediate mechanotransduction. This multi-receptor interaction can occur during cell-to-cell interactions (in trans) or within the same cells (in cis) (Figure 2). We now discuss how mechanobiology has increased the complexity of Thy-1-driven migration.
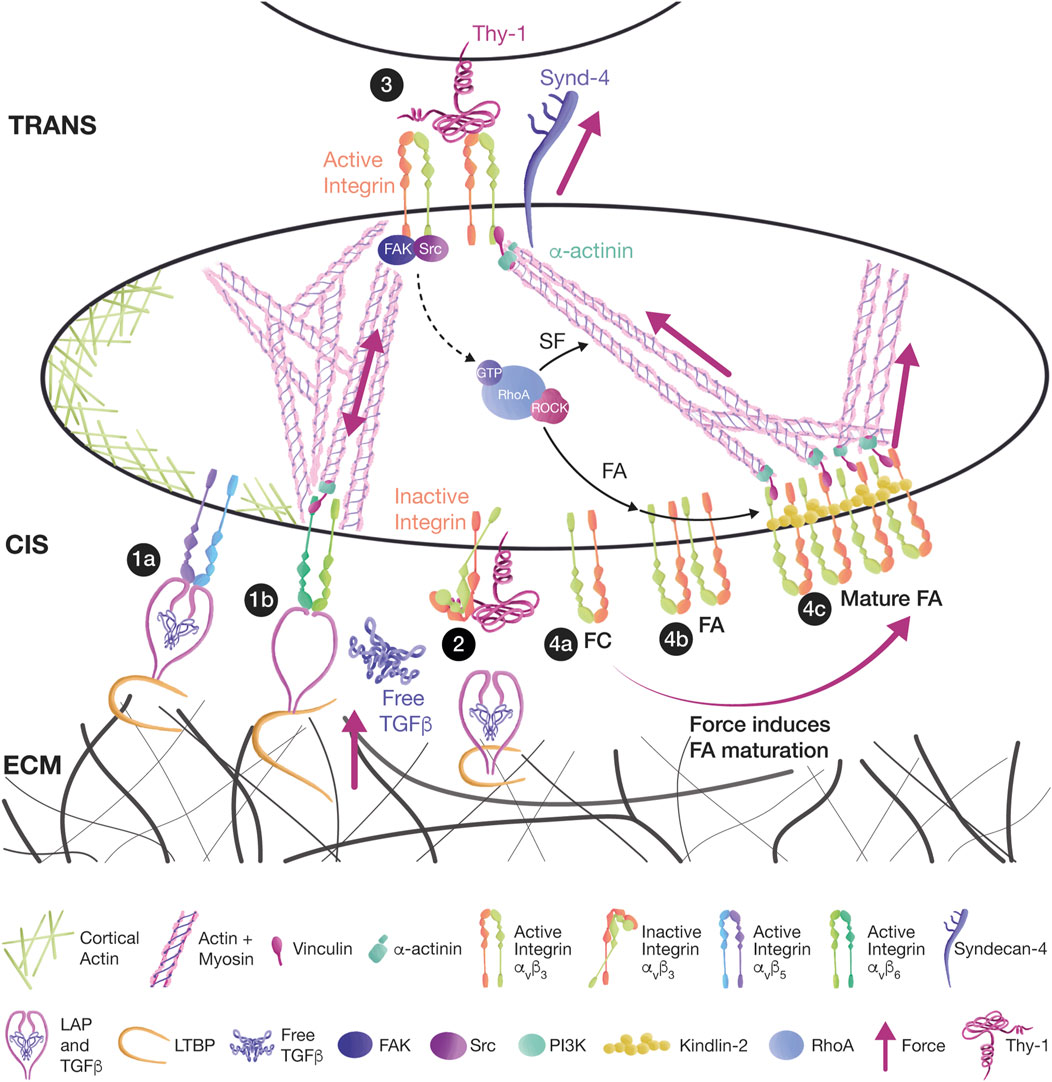
FIGURE 2. Thy-1 mediates mechanotransduction in cis and trans, affecting signaling. (1a) Thy-1 binding to αvβ5 and αvβ6 integrins in cis has an inhibitory effect during the mechanical activation of TGFβ in myofibroblasts. TGFβ remains dormant by interacting with the latency-associated peptide (LAP) and the latent TGFβ binding proteins (LTBPs). The TGFβ/LAP complex binds to integrins, and LTBPs link TGFβ/LAP to the ECM. (1b) Mechanical strain is transduced either from ECM-bound LTBPs or from integrin-bound LAP. Tension releases TGFβ and allows the activation of TGF receptors. (2) Thy-1 competes with LAP and ECM for integrin binding, leaving the integrin bent in a low-affinity inactive state in which TGFβ cannot be activated. (3) Thy-1 interaction with αvβ3 integrin and Syndecan-4 in trans induces the activation of the FAK/Src/RhoA/ROCK signaling pathway, leading to FA and SF formation in astrocytes. (4a) FCs are small clusters of integrins. (4b) FCs can grow into FAs because of RhoA signaling. (4c) Further tension transmitted by SFs leads to FA maturation and Kindlin-2 association.
3.2 The Thy-1/integrins/Syndecan-4 interaction in trans triggers mechanotransduction
Through its integrin-binding domain (RLD), Thy-1 binds and activates specific integrin heterodimers, including αvβ3, αxβ2, αMβ2, α5β1, and αvβ5 (Saalbach et al., 1999; Leyton et al., 2001; Avalos et al., 2004; Wetzel et al., 2004; Hermosilla et al., 2008; Fiore et al., 2015; Zhu et al., 2018). The engagement of Thy-1 with these integrins has been shown to carry out diverse tension-driven cell functions, such as adhesion, migration, and cell differentiation. For example, Thy-1 expressed on activated microvascular endothelial cells interacts with αvβ3 integrin expressed on melanoma cells to mediate cancer cell invasion. Consequently, Thy-1 knockout mice showed reduced metastasis (Saalbach et al., 2002; Saalbach et al., 2005; Schubert et al., 2013). Moreover, β3 integrin-silenced melanoma cells injected via the tail vein into a syngeneic mouse model exhibited almost undetectable lung tumor mass compared to the wild type cells, in which the tumor mass was 40% of the lung mass (Brenet et al., 2020). Similarly, Thy-1 in endothelial cells also mediated the adhesion and extravasation of leukocytes through αMβ2 (Mac-1) integrin (Wetzel et al., 2004).
Pioneer experiments using a biomembrane force probe to study the mechanical properties of the Thy-1/integrin/Syndecan-4 trimolecular complex revealed that when Thy-1 binds either an integrin (Thy-1/α5β1) or Syndecan-4 (Thy-1/Syndecan-4), the interaction is a classic slip bond. However, the trimolecular bond showed unique mechanical properties: a slip bond with forces < 20 pN, a catch bond from 20 to 35 pN, and then a second slip bond after reaching a tension > 35 pN. This mechanical behavior was described as a “dynamic catch” by the authors, and the proposed mechanism includes an initial α5β1 integrin/Thy-1 interaction that bears most of the force, which after reaching a threshold (∼15 pN), produces bond stiffening, shifting force to the already engaged, but unstretched Syndecan-4. When force induces the extension of the Syndecan-4 GAG chains, both α5β1 integrin and Syndecan-4 can bear a full-force load (Fiore et al., 2014). These results show synergism between integrins and Syndecan-4 during mechanotransduction in a model of melanoma-endothelial cell interaction.
Conversely, in the context of neuron-astrocyte interaction, our group showed a different bond behavior when the trimolecular complex contained αvβ3 integrin. Using molecular tweezers, we found that the Thy-1/αvβ3 integrin and Thy-1/Syndecan-4 interactions showed a slip bond, with forces between 10 and 50 pN. In contrast with Fiore’s findings (Fiore et al., 2014), the Thy-1/αvβ3 integrin/Syndecan-4 complex dissociated faster under tension, suggesting that the slip bond was maintained (Burgos-Bravo et al., 2018). Considering that α5β1 integrin was described to participate in FA maturation and strength, and that αvβ3 integrin can act as a mechanotransductor to regulate migration (Roca-Cusachs et al., 2009; Roca-Cusachs et al., 2012; Fiore et al., 2014; Burgos-Bravo et al., 2018), these reported results allow us to posit a differential role for Syndecan-4 during Thy-1/integrin bond formation. When Syndecan-4 is present, the Thy-1/α5β1 integrin catch bond resists tension to form strong and stable FAs, while the Thy-1/αvβ3 integrin slip bond is less resistant to tension, allowing the dynamic regulation of force sensing and mechanotransduction to facilitate cell migration.
The presence of Syndecan-4 also increased the lifetime of the Thy-1/αvβ3 integrin binary complex both in the absence of force and when force was applied. Remarkably, the lifetime of the Thy-1/αvβ3 integrin interaction was higher in comparison with that of Thy-1/Syndecan-4 after constant force, suggesting that the interaction between Thy-1/Syndecan-4 is weaker than that of the Thy-1/αvβ3 integrin interaction. This can be related to the nature of the interaction, which is protein-protein for Thy-1/αvβ3 integrin and protein-GAG for Thy-1/Syndecan-4 (Burgos-Bravo et al., 2020). However, since in the latter case protein-protein interactions can also occur, this assumption remains to be assessed.
Single-molecule experiments have added substantial evidence of the mechanical properties of the Thy-1/integrin/Syndecan-4 trimolecular complex. However, they may not accurately represent the full view since receptor clustering is important for FA and SF formation in astrocytes. For instance, although Thy-1 immobilized on a plate acts as a cell substrate or soluble recombinant Thy-1-Fc protein can induce weak cell adhesion and signaling, stimulation with Thy-1-Fc conjugated to Protein A-sepharose beads successfully stimulates strong cell adhesion, RhoA activation, SF formation, and migration in astrocytes (Avalos et al., 2004; Avalos et al., 2009; Kong et al., 2013). Similarly, co-culture experiments using neuronal cells expressing Thy-1 on their surface can also stimulate cytoskeletal changes that lead to astrocyte migration (Kong et al., 2013), which were also observed after stimulating astrocytes with EL4 cells, a lymphoma-derived cell line that expresses high levels of Thy-1 (Leyton et al., 2001). These observations sustain the idea that multivalent Thy-1 conjugated to beads or Thy-1 present on the surface of other cells can successfully stimulate strong adhesion, SF formation, and migration of cells, suggesting that clustering of Thy-1 with its co-receptors is necessary for an efficient cellular response.
Certainly, as with all GPI-anchored proteins, Thy-1 localizes in lipid rafts, and its interactions in cis could relocate integrins in lipid rafts (Barker et al., 2004; Hudon-David et al., 2007; Wang et al., 2010). Similarly, the aggregation of integrins in nanodomains has been linked to their activity (Lietha and Izard, 2020). However, it is still not entirely understood whether integrin clustering is triggered after ligand binding, leading to outside-in integrin activation, or whether clustering occurs due to integrin activation. Nevertheless, experiments performed with RGD peptides suggest that both possibilities are feasible (Ginsberg et al., 2005; Yu et al., 2011) and propose that integrin clustering occurs in phases. First, the initial binding of RGD to integrins leads to the recruitment of more integrins, Talin, Paxillin, and FAK, by lateral diffusion. Subsequently, actin polymerization and contractility mediated by Myosin generate an inward force associated with distant integrin clusters, resulting in larger FAs and the stimulation of lamellipodium extension (Yu et al., 2011). Accordingly, our group showed in primary astrocytes that αvβ3 Integrin overexpression by transfection or TNFα treatment induces integrin microclusters without triggering integrin activation, but priming the cells to respond to Thy-1 stimulation (Lagos-Cabre et al., 2018).
Moreover, in vitro assays using magnets to pull magnetic beads coated with recombinant Thy-1-Fc have shown that mechanical strain increases integrin levels at the cell surface, an effect that is followed by an increase in contractility reflected by elevated phosphorylation of MLC and an increase in the number and thickness of SFs (Perez et al., 2021) (Figure 3A).
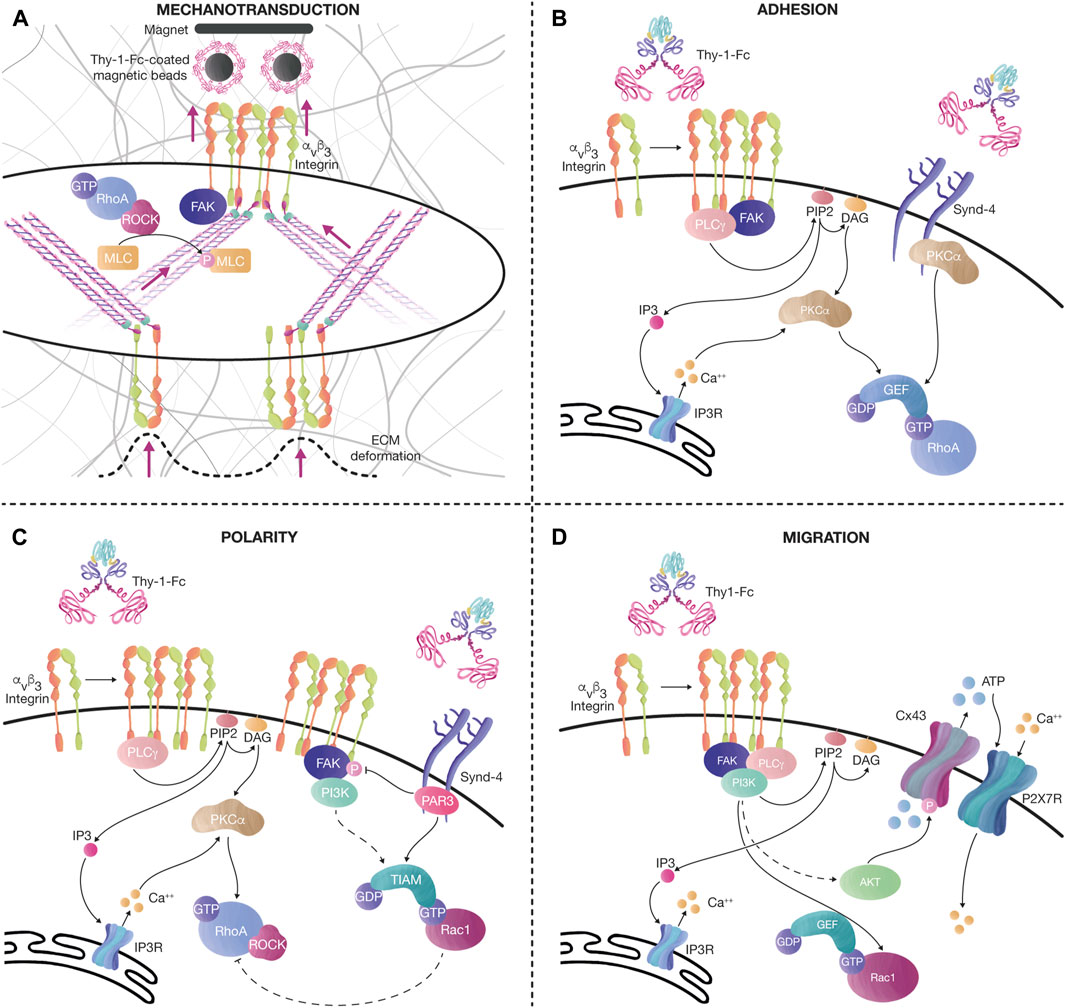
FIGURE 3. Thy-1 effects on mechanotransduction, cell adhesion, polarity, and migration. (A) Tension increases the levels of αvβ3 integrin at the cell surface after pulling magnetic beads coated with recombinant Thy-1-Fc. This effect is followed by an increase in cell contractility, evidenced by the augmented number and thickness of SFs, and elevated phosphorylation of MLC, probably due to the activation of the FAK/RhoA/ROCK signaling axis, as previously described. The tension transduced through SFs also induces ECM deformation. (B) Thy-1 interacts with αvβ3 integrin and Syndecan-4 to stimulate FA formation (< 15 min). Integrin clustering recruits signaling molecules, such as FAK and PLCγ. PLCγ activity on PIP2 produces DAG and IP3. IP3 activates Ca++ release from the IP3R in the endoplasmic reticulum. Ca++, together with DAG, activates PKCα, leading to RhoA activation. Alternatively, PKCα can also be activated by interacting with the variable region of the Syndecan-4 cytoplasmic tail, synergizing RhoA activity. (C) Longer stimulation of αvβ3 integrin and Syndecan-4 with Thy-1-Fc (< 60 min), induces changes in cell polarity, FA disassembly, and migration. The polarity complex protein Partitioning defective 3 (PAR-3) binds to the Syndecan-4 cytoplasmic tail to inhibit FAK phosphorylation and activate the RacGEF, Tiam, which further mediates Rac1 activation. PI3K is also activated after sustained stimulation with Thy-1-Fc in astrocytes, stimulating the activation of Rac1. Moreover, once Rac1 is active, an inhibitory axis can decrease RhoA activity through p190RhoAGAP. Altogether, this signaling pathway helps the cell disassemble FAs and migrate in a directional manner. (D) Prolonged Thy-1-Fc stimulation (∼16 h) allows the cell to migrate. Ca++ is released from the IP3R at the endoplasmic reticulum by IP3 produced by PLCγ. FAK/PI3K/Akt activation downstream of integrins induces Rac1 activation and phosphorylation of Connexin43 (Cx43) channels, which release ATP to the extracellular compartment. ATP activates the P2X7 receptor to mediate Ca++ influx. Bursts of cytoplasmic Ca++ transients, together with active Rac1, stimulate cell migration.
Our previous work using Thy-1-Fc beads was based on stimulating cells on the dorsal surface to effectively form FAs and SFs on the ventral part of the cells (Perez et al., 2021). However, how exactly does dorsal stimulation transduce the signals to the interior of the cell to generate an effect on the ventral surface? We speculate that dorsal engagement of integrins triggers signaling pathways that involve the activation of small Rho GTPases. These pathways would then crosstalk with those engaged by the ventral integrins stimulated by the ECM-cell contacts, thus enhancing/synergizing the effect, and producing a more robust cellular response (Figure 3A).
3.3 The Thy-1/integrin/Syndecan-4 interaction in cis regulates mechanotransduction
Primary fibroblasts expressing Thy-1 (Thy-1+/+) form strong FAs and SFs and, consequently, are less migratory than fibroblasts not expressing this protein (Thy-1−/−). Thy-1 expressed on the surface of lung fibroblasts interacts in cis with αvβ5 integrin, keeps it in a low-affinity bent conformation, and decreases its binding to ECM proteins (Zhou et al., 2010). Moreover, the Thy-1/αvβ3 integrin interaction occurs in lipids rafts, where Fyn and Src are recruited to FAs. Fyn itself has been described as critical during mechanosignaling since it activates the RhoA pathway by reducing Src-induced phosphorylation of p190RhoGAP through the recruitment of CBP and by directly phosphorylating RhoGEFs (Guilluy et al., 2011; Fiore et al., 2015).
Additionally, Thy-1 can also regulate mechanotransduction by modulating the avidity of the mechanoreceptors and modifying the composition of the ECM. The mechanics and composition of the ECM are particularly important during idiopathic pulmonary fibrosis, where a stiffer ECM is associated with mature fibrosis. Here, fibroblasts sense mechanical cues and undergo aberrant activation (Guo et al., 2022). In this context, a stiff FN-rich provisional ECM promotes FA formation and actomyosin contractility, leading to fibroblast-induced remodeling of the ECM, thereby amplifying the profibrotic phenotype. Consequently, pulmonary fibroblasts secrete FN independently of Thy-1 expression; however, Thy-1+/+ fibroblasts produce two-to three-fold more collagen than Thy-1−/− cells, contributing to the deposition of a softer ECM (Derdak et al., 1992; Abeysinghe et al., 2005). Moreover, in a model of fibrosis, Fiore et al. showed that fibrosis-associated fibroblasts lacking Thy-1 expression displayed elevated activation of αvβ3 integrin, thus leading to enhanced fibroblast activation, stiffer provisional ECM, and progression of fibrosis (Fiore et al., 2018).
The inhibitory effect of Thy-1 binding to integrins in cis has also been associated with the mechanical activation of TGFβ. The latency-associated peptide (LAP) binds TGFβ, and latent TGFβ binding proteins (LTBPs) link the TGFβ/LAP complex to the ECM, keeping a dormant TGFβ pool that can be activated by mechanical force transduced by αvβ5 and αvβ6 integrins expressed in myofibroblasts. Tension transmitted either from ECM-bound LTBPs or from myofibroblast contractility can pull LAP, release TGFβ, and allow the activation of TGF receptors (Munger et al., 1998; Annes et al., 2002; Annes et al., 2004; Wipff et al., 2007). Thy-1 competes with LAP and the ECM for integrin binding; thus, Thy-1 interaction with αvβ5/αvβ6 integrins in cis maintains these integrins inactive, and TGFβ cannot be activated, thus affecting myofibroblast differentiation and fibrosis progression (Figure 2) (Zhou et al., 2004; Zhou et al., 2010).
3.4 What we still do not know about Thy-1/integrin/Syndecan-4 mechanotransduction
Syndecan-4 mechanical properties have been broadly studied in the context of shear stress and ECM-driven mechanotransduction. To date, only a few studies have shown the mechanical properties of the Thy-1/Syndecan-4 and Thy-1/integrins/Syndecan-4 interactions (Fiore et al., 2015; Burgos-Bravo et al., 2018; Burgos-Bravo et al., 2020; Perez et al., 2021). Studying the effects of the Thy-1/integrin/Syndecan-4 trimolecular complex in the context of mechanotransduction is important since mechanical strain controls many physiological and pathological functions, such as embryonic development, morphogenesis, wound healing, cancer, and atherosclerosis.
Even though Syndecan-4 cooperates with integrins during mechanotransduction, it is still not clear how the signaling between both receptors synergizes. We posit the possibility of downstream signaling crosstalk between integrins and Syndecan-4, in which integrin activation would induce the activation of RhoA and several kinases, including FAK and PI3K. Similarly, the activation of Syndecan-4 would also recruit FAK and PKC and further activate RhoA, increasing the overall response (Figure 3B). Optimal signaling would occur when both receptors are active since the loss of either receptor has shown defects in contractility and FA formation (Saoncella et al., 1999; Bass et al., 2007). Another possibility is the direct cis interaction between integrins and Syndecan-4. It is plausible that they influence each other’s membrane location; therefore, external engagement of either integrins or Syndecan-4 could favor receptor clustering and potentiate the initial signaling.
Syndecan-4 could also antagonize integrin signaling. After sustained Thy-1 stimulation (∼30 min), PAR-3 is recruited to the Syndecan-4 cytoplasmic tail, leading to Rac1 activation through its GEF, Tiam (Valdivia et al., 2020). Rac1 signaling antagonizes RhoA activity, prompting the cell to polarize, disassemble FAs, decrease contractility, and migrate (Figure 3C). In this case, it is still unknown how Syndecan-4 signaling turns off integrin signaling. However, it is likely that Rac1 activates p190RhoGAP and induces RhoA inactivation, as previously described [reviewed in (Lawson and Burridge, 2014)] (Figure 3C). Thus, the crosstalk between integrin and syndecan receptors can tightly regulate FA dynamics and the cytoskeleton changes occurring during cell adhesion and migration.
Alternatively, Syndecan-4 probably acts as a regulator of the inside-out activation of integrins. In this scenario, we can hypothesize that Syndecan-4 engagement could activate certain signaling pathways, such as PI3K or PKCα, which would then transduce signals to activate integrins and enhance cellular contractility. A similar mechanism has already been proposed for the mechanoreceptor PECAM-1. In this case, shear stress activates PECAM-1, leading to the PI3K signaling necessary for β1 integrin activation (Collins et al., 2012). A different scenario has been described in fibroblasts, where Src-mediated Syndecan-4 phosphorylation on Y180 inactivates Arf6, which regulates integrin recycling (Morgan et al., 2013). In this case, Syndecan-4 phosphorylation lowers α5β1 integrin recycling to the membrane, while favoring the passage of αvβ3 integrin from endosomes to the plasma membrane. This is another type of integrin switch but mediated by Syndecan-4 signaling, which is crucial for controlling integrin trafficking and FA dynamics and inducing FA stabilization and more directional cell migration by inactivating Arf6 (Morgan et al., 2013).
4 What we have learned from our studies on cell adhesion and migration
4.1 The discovery of the integrin/Thy-1 interaction in cell adhesion and migration
Our interest in this topic started a little over 20 years ago while searching for the ligand of the cell adhesion molecule Thy-1. Research on Thy-1 led to the important discovery that GPI-anchored glycoproteins could signal intracellularly despite only crossing the outer leaflet of the plasma membrane and lacking a transmembrane-spanning domain (Morris, 1992). In addition, Thy-1 was known to modulate neurite outgrowth (Tiveron et al., 1992); however, this and other potential functions of Thy-1 were uncertain due to its unidentified ligand.
We were the first group to describe that the RLD tripeptide found in the Thy-1 sequence recognized the αvβ3 integrin present in astrocytes (Leyton et al., 2001; Hermosilla et al., 2008). Integrin ligation by Thy-1 was functional and induced the formation of FAs in astrocytes, an effect that was precluded when the cells were stimulated with a Thy-1 fusion protein in which the RLD tripeptide was mutated to RLE (D18E). Other features of this interaction that supported a role for the αvβ3 integrin were that the adhesion of astrocytes to Thy-1 required Mn2+ or Mg2+ but neither Ca2+ nor a combination of both Ca2+/Mg2+, and that the interaction was inhibited by anti-β3 but not by anti-β1 integrin antibodies (Hermosilla et al., 2008). The FAs formed because the Thy-1-engaged integrin interaction possessed many proteins described in IACs (see Section 2.1), including Vinculin, Paxillin, p130Cas, and FAK (Leyton et al., 2001). Our initial findings reporting αvβ3 integrin as a Thy-1 receptor were later confirmed in other cell types, including melanomas and breast cancer cells (Brenet et al., 2020), fibroblasts (Valdivia et al., 2020), and leukocytes (Wetzel et al., 2004). The αvβ3 integrin/Thy-1 interaction was additionally extended to other integrins, such as αxβ2 and αMβ2 in leukocytes (Choi et al., 2005), and α5β1 in melanoma cells (Fiore et al., 2015)[reviewed in (Leyton et al., 2019)].
4.2 Focal adhesions induced by the direct interaction of Thy-1 with integrins/Syndecan-4
As previously mentioned, FAs are formed and maintained by the activation of RhoA, whose activity is also required to form SFs (Ridley and Hall, 1992). In astrocytes stimulated with Thy-1, we have shown that integrin clustering, RhoA activation, and enhanced activity of the RhoA effector, ROCK, are required to promote FA and SF formation in cells bound to their own secreted matrix (Avalos et al., 2002; Avalos et al., 2004) (Figure 3B). Thus, this mechanism for cell contraction seems to be conserved whether generated by cell-cell [Thy-1/integrin (Avalos et al., 2004; Hermosilla et al., 2008)] or cell-matrix [integrin/ECM (Berrier and Yamada, 2007)] interactions.
In our original study, results obtained by competing the Thy-1/integrin interaction with antibodies, recombinant proteins, or peptides suggested that a third molecule was involved, since complete inhibition was never attained (Leyton et al., 2001). Considering the available information, we hypothesized that Syndecan-4 could act as a co-receptor for αvβ3 integrin to respond to Thy-1. This hypothesis was additionally supported by studies indicating the existence of a direct interaction between Thy-1 and sulfated glycans (Hueber et al., 1992). By using different heparin dilutions, we demonstrated that Thy-1-stimulated adhesion of astrocytes involved a Thy-1-HBD interaction, which was required for Syndecan-4 binding, as demonstrated by silencing Syndecan-4 or expressing a mutant that lacked its cytoplasmic domain (Avalos et al., 2009). In addition, treatment of astrocytes with heparitinase decreased the number of FAs formed, and the observed contacts were of smaller size (Avalos et al., 2009). Moreover, mutation of the basic amino acids composing the HBD (REKRK→AEAAA) led to the production of a recombinant protein that could no longer stimulate FA formation. Thus, both the integrin-binding domain (RLD) and the HBD (REKRK) of Thy-1 were necessary to trigger astrocyte adhesion (Avalos et al., 2009). We additionally demonstrated that Thy-1-mediated integrin/Syndecan-4 engagement involved the activation of PKCα upstream of the loading of RhoA with GTP (active state) (Avalos et al., 2009) (Figure 3B). Because RhoA is associated with cell contraction and strong cell adhesion, we proposed that Thy-1/integrin interaction would prevent cell migration. However, we then discovered that upon sustained stimulation with Thy-1 (24 h), RhoA activity decreased, whereas that of Rac1 increased, thus leading to astrocyte migration (Kong et al., 2013).
4.3 Signaling mechanisms involved in Thy-1-induced astrocyte migration
PKCα is a calcium- and DAG-dependent enzyme; therefore, we next studied signaling mechanisms that could account for changes in these second messengers. Over the years, we have been able to decipher, in part, the puzzle of the signal transduction pathways involved in Thy-1/integrin-induced astrocyte adhesion and migration. The elucidated sequence of events includes the activation of FAK/Src/PI3K/PLCγ and hydrolysis of PIP2 to generate DAG and IP3, which activates the IP3R and induces intracellular calcium increase (Kong et al., 2013). Most recently, we have shown that AKT, activated downstream of PI3K, phosphorylates and opens the hemichannel Connexin43, releasing ATP (Perez-Nunez et al., 2023) (Figure 3D). The opening of Connexin43 is accompanied by Pannexin1 hemichannel activation, and both hemichannels release ATP to the extracellular compartment, thereby leading to the activation of the purinergic receptor P2X7R and allowing calcium entry (Henriquez et al., 2011; Alvarez et al., 2016) (Figure 3D). This functional link between integrins and P2X7R in the context of cell migration was a novel finding exhibited by the DITNC1 astrocyte cell line but later, was also found in breast cancer and melanoma cells (Brenet et al., 2020).
Remarkably, the signaling events described in the DITNC1 astrocyte cell line in response to Thy-1 were not observed in primary astrocytes unless these cells were treated with inflammatory cytokines (Lagos-Cabre et al., 2017). TNF-treated primary astrocytes showed increased levels of reactivity markers, such as GFAP, iNOS, and Connexin43. To our surprise, astrocytes responded to Thy-1 stimulation only when they were reactive (TNF-treated). The response comprised morphological features associated with in vivo reactivity, such as hypertrophy, a fibroblast-like phenotype, and increased cell migration (Lagos-Cabre et al., 2017). The molecular mechanisms triggered by Thy-1 in these reactive astrocytes were similar to those reported for the DITNC1 cell line, and the inflammatory signals were necessary to increase the levels of both αvβ3 integrin and Syndecan-4 (Lagos-Cabre et al., 2017; Palacios et al., 2023). Importantly, the increase in αvβ3 integrin levels occurred in a NFkB-dependent manner (Lagos-Cabre et al., 2017; Palacios et al., 2023).
Most importantly, αvβ3 integrin appeared in large membrane patches in immunofluorescence microscopy assays, suggesting that TNF prompted the cells to respond more effectively to Thy-1 by increasing the surface levels of this integrin and inducing the formation of αvβ3 integrin microclusters (Lagos-Cabre et al., 2018). Astrocytes overexpressing β3 integrin exhibited spontaneous microclusters in the absence of TNF treatment and responded to Thy-1 without any prior pro-inflammatory exposure (Lagos-Cabre et al., 2017). These results were intriguing, and further analysis indicated that the sole overexpression of this integrin was sufficient to turn the astrocytes into reactive ones and prompt their migration in response to Thy-1 stimulation (Lagos-Cabre et al., 2017; Lagos-Cabre et al., 2018).
Another central question that interested us was: How does the ATP that travels through open hemichannels reach the juxtamembrane localization to be released by the cells? Based on the reported evidence and our results indicating that exocytosis was not involved in ATP release [Brefeldin A did not affect Thy-1-induced ATP release (Alvarez et al., 2016)], we hypothesized that the release of ATP required calcium uptake by mitochondria and re-localization of these organelles to the cell cortex via microtubule-dependent transport. Before we were able to test this assumption with our astrocyte cell line, different researchers showed that migrating cells transfer the mitochondria to the leading edge over the microtubules in order to drive ATP production and provide energy demands where needed (Cunniff et al., 2016). These results were confirmed in cancer and mesothelial cells, as well as fibroblasts, and therefore, we anticipated that astrocytes probably use a similar mechanism to provide the local ATP production required for its release, although this has not been demonstrated yet using astrocytes. ATP could then be released through hemichannels, for example, via the opening of Connexin43 phosphorylated by active AKT (Perez-Nunez et al., 2023).
The role of Syndecan-4 on astrocyte migration was also intriguing, and therefore, we studied its role by generating a Thy-1 fusion protein mutated in the HBD (Thy-1(AEAAA)-Fc). We learned that this mutated protein does not promote astrocyte migration (24 h), despite inducing ATP release, although in lower amounts (40%), and in a delayed manner (15 min instead of 10 min), compared to ATP release induced by the wild type protein Thy-1(RLD)-Fc (Alvarez et al., 2016). On the contrary, the Thy-1 fusion protein mutated in its integrin-binding domain (Thy-1(RLE)-Fc) did not induce cell migration, nor elicited any ATP release. However, Thy-1(RLE)-Fc induced astrocyte migration when added with a threshold amount of BzATP (a non-hydrolyzable ATP analogue added at a concentration three orders of magnitude lower than that required to induce migration). Our results suggested that Thy-1-engaged αvβ3 integrin led to ATP release, whereas Thy-1-ligated Syndecan-4 was likely involved in the regulation of the kinetics, as well as the threshold amount of ATP released by astrocytes required to induce cell migration (Alvarez et al., 2016). These results led us to propose a sequential activation: integrins first, followed by Syndecan-4, which would then be in charge of inducing a feed-forward loop to maintain the cycle of protein activation required for cells to move.
In agreement with the idea of Syndecan-4 being a regulator of the αvβ3 integrin/Thy-1 interaction, we then reported that Syndecan-4 forms a ternary complex with Thy-1 and αvβ3 integrin, increasing the lifetime of the Thy-1/αvβ3 integrin bond even when exposed to force, as would occur with mechanical signals coming from the ECM or cell-to-cell contacts (Burgos-Bravo et al., 2018). Importantly, the use of optical tweezers allowed us to demonstrate that at a single molecule level, the Thy-1/αvβ3 integrin bond dissociated faster by force application (Burgos-Bravo et al., 2018). However, when Syndecan-4 was present, bond stabilization of the bimolecular Thy-1/integrin interaction accelerated the biological functions exerted by αvβ3 integrin (Burgos-Bravo et al., 2018). We provided additional validation of these findings by simultaneously applying chemical and mechanical stimulation to astrocytes and found that the kinetics of astrocyte adhesion and contraction were accelerated by adding a mechanical force to Thy-1 stimulation (Perez et al., 2021). Considering these results, we proposed that Syndecan-4 leads to structural changes in αvβ3 integrin, allowing it to interact more efficiently with Thy-1, thus inducing integrin clustering and activation, and a faster cellular response.
An additional piece of the signaling puzzle was added after we discovered that upon Thy-1 binding, Syndecan-4 interacted with PAR-3, a protein that had not been associated with the pathways that determine whether a cell migrates or not. In one of our recent reports (Valdivia et al., 2020), we described that after Thy-1 stimulation, PAR-3 interaction with the Syndecan-4 intracellular tail is required for the disassembly of FAs through FAK dephosphorylation and the activation of Tiam1 and Rac1 (Figure 3C). This report is relevant because it is the first one to describe PAR-3 as an adhesome-associated component involved in the polarized migration of astrocytes and fibroblasts through the regulation of FA dynamics (Valdivia et al., 2020).
Therefore, Thy-1 signaling triggered in astrocytes by binding to its partners, αvβ3 integrin and Syndecan-4, is an intricate network regulated by chemical (Thy-1) and mechanical (force) cues (Figure 3A) that requires a threshold level of receptors, which are controlled in part, by inflammatory signals.
5 Concluding remarks and clinical relevance
In this review, we summarized studies related to adhesion receptors, their signaling mechanisms and how the complex networks they form are regulated by the ECM; we also described the mechanical forces generated by the ECM, and the interactions with other cells. The main adhesion receptors are integrins and Syndecan-4, which are also mechanosensors and thus, translate the mechanical cues to the cytoskeleton and change the cellular responses. Integrins and the hundreds of proteins that are recruited to cell adhesion points, which change from NAs to FCs, and then to FAs and mature FAs, are the main components of the adhesome, a structure that has become relevant due to the control it exerts on cell adhesion and migration.
Cell adhesion and migration are cyclic and dynamic processes highly regulated by the intricate interconnections that occur within the adhesome. Many proteins that form part of the adhesome are activated/inactivated, switched on/off, or phosphorylated/dephosphorylated, and these events are constantly occurring within the complex to maintain the dynamics and cyclicity of these processes. During FA dynamics, the cytoskeleton also plays a key role; both actin filaments and microtubules contribute to regulate FA maturation and disassembly to maintain the cyclic machinery that controls cell migration. In the past years, much has been learned about the composition of the adhesome and the turnover of FAs; however, how much of this knowledge could be applied to living organisms is still a matter of debate. The current availability of 3D culturing techniques, organoids, organs on a chip, etc., will be key to solving this discussion. Similarly, the comparative analysis of adhesomes present in normal versus tumoral and metastatic cells can help identify the components of the adhesome that are differentially expressed in diverse stages of cancer, which could lead to the development of targeted therapies (Sauzay et al., 2019; Brenet et al., 2020; Keller-Pinter et al., 2021; Bergonzini et al., 2022).
Mechanobiology has certainly added complexity to how we initially pictured cell migration. Mechanical strain is tightly regulated at the level of substrate stiffness, mechanoreceptor abundance, the readiness of mechanotransducers to signal, and ECM remodeling. Our results have shown that the interaction between Thy-1 and integrins/Syndecan-4 in trans can regulate FA and SF formation, enhancing the complexity of integrin-mediated mechanotransduction.
Acute or chronic inflammation is a hallmark of many pathological conditions, such as cancer, wound healing, autoimmune diseases, and arteriosclerosis. Different pathophysiological processes are positively or negatively affected by mechanical forces. Of relevance, Thy-1, integrins, and Syndecan-4 expression can be stimulated by inflammatory molecules, which may play an important role in regulating conditions of clinical relevance, such as wound healing [reviewed in (Perez et al., 2022b)]. Accordingly, our group and others have shown that Thy-1 and Syndecan-4 promote skin wound healing (Echtermeyer et al., 2001; Araki et al., 2009; Bass et al., 2011; Brooks et al., 2012; Vuong et al., 2015; Das et al., 2016; Perez et al., 2022a). In particular, we demonstrated that Thy-1 increases the healing rate by increasing skin perfusion. However, further experiments are necessary to determine if the interaction between Thy-1, Syndecan-4, and integrins is relevant during wound remodeling and scarring, where mechanical forces play a pivotal role.
Cell migration is necessary but requires to be tightly regulated. If occurring in an uncontrolled manner, cell migration is detrimental, as occurs during cancer metastasis, autoimmune diseases, or fibrosis. The interaction of Thy-1 with integrins in cis plays an essential role in inhibiting TGFβ signaling and fibrosis. Indeed, in an in vivo model of lung fibrosis, the administration of soluble Thy-1 therapeutically inhibits integrin-mediated fibrosis (Phuan et al., 2019). Our discoveries have contributed to developing transcendental pre-clinical research, and we expect that these studies can soon translate into therapeutics for curing or improving the outcome of many clinical conditions related to cell adhesion and migration.
Author contributions
LL contributed to the conception and design of the work; conceptualization, writing–original draft preparation, writing–review and editing: AV, AA, and LL; funding acquisition: LL. All authors have read and agreed to the published version of the manuscript.
Funding
LL was supported by Fondo Nacional de Desarrollo Científico y Tecnológico #1200836 and Agencia Nacional de Investigación y Desarrollo (ANID)-FONDAP #15130011.
Acknowledgments
LL would like to thank the professional drawings performed by Sylk Illustrations.
Conflict of interest
The authors declare that the research was conducted in the absence of any commercial or financial relationships that could be construed as a potential conflict of interest.
Publisher’s note
All claims expressed in this article are solely those of the authors and do not necessarily represent those of their affiliated organizations, or those of the publisher, the editors and the reviewers. Any product that may be evaluated in this article, or claim that may be made by its manufacturer, is not guaranteed or endorsed by the publisher.
Abbreviations
bFGF, Basic fibroblast growth factor; CD90, Cluster of differentiation 90; ECM, Extracellular matrix; EGF, Epidermal growth factor; FA, Focal adhesion; FAK, Focal adhesion kinase; FC, Focal contact; FGFR, Fibroblast growth factor receptor; FN, Fibronectin; GAGs, Glycosaminoglycans; GAP, GTPase-activating protein; GEF, Guanine nucleotide exchange factor; GDI, Guanine nucleotide dissociation inhibitor; HBD, Heparin-binding domain; IAC, Integrin-associated complex; IGF, Insulin growth factor; LAP, Latency-associated peptide; LTBP, Latent TGFβ binding protein; MAPK, Mitogen-activated protein (MAP) kinase; NA, Nascent adhesion; PAR-3, Partition-defective 3; PDGF, Platelet-derived growth factor; PLC, Phospholipase C; SF, Stress fiber; TGFβ, Transforming growth factor β; Thy-1, Thymus cell antigen 1; VEGF, Vascular endothelial growth factor.
References
Abeysinghe, H. R., Li, L. Q., Guckert, N. L., Reeder, J., and Wang, N. (2005). THY-1 induction is associated with up-regulation of fibronectin and thrombospondin-1 in human ovarian cancer. Cancer Genet. Cytogenet 161 (2), 151–158. doi:10.1016/j.cancergencyto.2005.02.014
Ain, U., and Firdaus, H. (2022). Parvin: a hub of intracellular signalling pathways regulating cellular behaviour and disease progression. Acta histochem. 124 (7), 151935. doi:10.1016/j.acthis.2022.151935
Alexandrova, A. Y., Arnold, K., Schaub, S., Vasiliev, J. M., Meister, J. J., Bershadsky, A. D., et al. (2008). Comparative dynamics of retrograde actin flow and focal adhesions: formation of nascent adhesions triggers transition from fast to slow flow. PLoS One 3 (9), e3234. doi:10.1371/journal.pone.0003234
Alvarez, A., Lagos-Cabre, R., Kong, M., Cardenas, A., Burgos-Bravo, F., Schneider, P., et al. (2016). Integrin-mediated transactivation of P2X7R via hemichannel-dependent ATP release stimulates astrocyte migration. Biochim. Biophys. Acta 1863 (9), 2175–2188. doi:10.1016/j.bbamcr.2016.05.018
Annes, J. P., Chen, Y., Munger, J. S., and Rifkin, D. B. (2004). Integrin alphaVbeta6-mediated activation of latent TGF-beta requires the latent TGF-beta binding protein-1. J. Cell Biol. 165 (5), 723–734. doi:10.1083/jcb.200312172
Annes, J. P., Rifkin, D. B., and Munger, J. S. (2002). The integrin alphaVbeta6 binds and activates latent TGFbeta3. FEBS Lett. 511 (1-3), 65–68. doi:10.1016/s0014-5793(01)03280-x
Araki, E., Momota, Y., Togo, T., Tanioka, M., Hozumi, K., Nomizu, M., et al. (2009). Clustering of Syndecan-4 and integrin β1 by laminin α3 Chain-derived peptide promotes keratinocyte migration. Mol. Biol. Cell 20 (13), 3012–3024. doi:10.1091/mbc.e08-09-0977
Arthur, W. T., Noren, N. K., and Burridge, K. (2002). Regulation of Rho family GTPases by cell-cell and cell-matrix adhesion. Biol. Res. 35 (2), 239–246. doi:10.4067/s0716-97602002000200016
Askari, J. A., Buckley, P. A., Mould, A. P., and Humphries, M. J. (2009). Linking integrin conformation to function. J. Cell Sci. 122 (Pt 2), 165–170. doi:10.1242/jcs.018556
Atkinson, S. J., Gontarczyk, A. M., Alghamdi, A. A., Ellison, T. S., Johnson, R. T., Fowler, W. J., et al. (2018). The β3-integrin endothelial adhesome regulates microtubule-dependent cell migration. EMBO Rep. 19 (7), e44578. doi:10.15252/embr.201744578
Austin, J., Tu, Y., Pal, K., and Wang, X. (2023). Vinculin transmits high-level integrin tensions that are dispensable for focal adhesion formation. Biophys. J. 122 (1), 156–167. doi:10.1016/j.bpj.2022.11.013
Avalos, A. M., Arthur, W. T., Schneider, P., Quest, A. F., Burridge, K., and Leyton, L. (2004). Aggregation of integrins and RhoA activation are required for Thy-1-induced morphological changes in astrocytes. J. Biol. Chem. 279 (37), 39139–39145. doi:10.1074/jbc.M403439200
Avalos, A. M., Labra, C. V., Quest, A. F., and Leyton, L. (2002). Signaling triggered by Thy-1 interaction with beta 3 integrin on astrocytes is an essential step towards unraveling neuronal Thy-1 function. Biol. Res. 35 (2), 231–238. doi:10.4067/s0716-97602002000200015
Avalos, A. M., Valdivia, A. D., Munoz, N., Herrera-Molina, R., Tapia, J. C., Lavandero, S., et al. (2009). Neuronal Thy-1 induces astrocyte adhesion by engaging syndecan-4 in a cooperative interaction with alphavbeta3 integrin that activates PKCalpha and RhoA. J. Cell Sci. 122 (Pt 19), 3462–3471. doi:10.1242/jcs.034827
Avril, T., Etcheverry, A., Pineau, R., Obacz, J., Jegou, G., Jouan, F., et al. (2017). CD90 expression controls migration and predicts dasatinib response in glioblastoma. Clin. Cancer Res. 23 (23), 7360–7374. doi:10.1158/1078-0432.CCR-17-1549
Bachmann, M., Kukkurainen, S., Hytonen, V. P., and Wehrle-Haller, B. (2019). Cell adhesion by integrins. Physiol. Rev. 99 (4), 1655–1699. doi:10.1152/physrev.00036.2018
Baeyens, N., Mulligan-Kehoe, M. J., Corti, F., Simon, D. D., Ross, T. D., Rhodes, J. M., et al. (2014). Syndecan 4 is required for endothelial alignment in flow and atheroprotective signaling. Proc. Natl. Acad. Sci. 111 (48), 17308–17313. doi:10.1073/pnas.1413725111
Baker, E. L., and Zaman, M. H. (2010). The biomechanical integrin. J. Biomech. 43 (1), 38–44. doi:10.1016/j.jbiomech.2009.09.007
Barker, T. H., Pallero, M. A., MacEwen, M. W., Tilden, S. G., Woods, A., Murphy-Ullrich, J. E., et al. (2004). Thrombospondin-1-induced focal adhesion disassembly in fibroblasts requires Thy-1 surface expression, lipid raft integrity, and Src activation. J. Biol. Chem. 279 (22), 23510–23516. doi:10.1074/jbc.M402169200
Barry, A. K., Wang, N., and Leckband, D. E. (2015). Local VE-cadherin mechanotransduction triggers long-ranged remodeling of endothelial monolayers. J. Cell Sci. 128 (7), 1341–1351. doi:10.1242/jcs.159954
Barzik, M., Kotova, T. I., Higgs, H. N., Hazelwood, L., Hanein, D., Gertler, F. B., et al. (2005). Ena/VASP proteins enhance actin polymerization in the presence of barbed end capping proteins. J. Biol. Chem. 280 (31), 28653–28662. doi:10.1074/jbc.M503957200
Bass, M. D., Morgan, M. R., and Humphries, M. J. (2007). Integrins and syndecan-4 make distinct, but critical, contributions to adhesion contact formation. Soft Matter 3 (3), 372–376. doi:10.1039/b614610d
Bass, M. D., Williamson, R. C., Nunan, R. D., Humphries, J. D., Byron, A., Morgan, M. R., et al. (2011). A syndecan-4 hair trigger initiates wound healing through caveolin- and RhoG-regulated integrin endocytosis. Dev. Cell 21 (4), 681–693. doi:10.1016/j.devcel.2011.08.007
Bauer, M. S., Baumann, F., Daday, C., Redondo, P., Durner, E., Jobst, M. A., et al. (2019). Structural and mechanistic insights into mechanoactivation of focal adhesion kinase. Proc. Natl. Acad. Sci. U. S. A. 116 (14), 6766–6774. doi:10.1073/pnas.1820567116
Bear, J. E., Svitkina, T. M., Krause, M., Schafer, D. A., Loureiro, J. J., Strasser, G. A., et al. (2002). Antagonism between Ena/VASP proteins and actin filament capping regulates fibroblast motility. Cell 109 (4), 509–521. doi:10.1016/s0092-8674(02)00731-6
Bellin, R. M., Kubicek, J. D., Frigault, M. J., Kamien, A. J., Steward, R. L., Barnes, H. M., et al. (2009). Defining the role of syndecan-4 in mechanotransduction using surface-modification approaches. Proc. Natl. Acad. Sci. U. S. A. 106 (52), 22102–22107. doi:10.1073/pnas.0902639106
Bergonzini, C., Kroese, K., Zweemer, A. J. M., and Danen, E. H. J. (2022). Targeting integrins for cancer therapy - disappointments and opportunities. Front. Cell Dev. Biol. 10, 863850. doi:10.3389/fcell.2022.863850
Berrier, A. L., and Yamada, K. M. (2007). Cell-matrix adhesion. J. Cell Physiol. 213 (3), 565–573. doi:10.1002/jcp.21237
Bois, P. R., O'Hara, B. P., Nietlispach, D., Kirkpatrick, J., and Izard, T. (2006). The vinculin binding sites of talin and alpha-actinin are sufficient to activate vinculin. J. Biol. Chem. 281 (11), 7228–7236. doi:10.1074/jbc.M510397200
Bouvard, D., Pouwels, J., De Franceschi, N., and Ivaska, J. (2013). Integrin inactivators: balancing cellular functions in vitro and in vivo. Nat. Rev. Mol. Cell Biol. 14 (7), 430–442. doi:10.1038/nrm3599
Brenet, M., Martinez, S., Perez-Nunez, R., Perez, L. A., Contreras, P., Diaz, J., et al. (2020). Thy-1 (CD90)-Induced metastatic cancer cell migration and invasion are β3 integrin-dependent and involve a Ca2+/P2X7 receptor signaling Axis. Front. Cell Dev. Biol. 8, 592442. doi:10.3389/fcell.2020.592442
Brizzi, M. F., Tarone, G., and Defilippi, P. (2012). Extracellular matrix, integrins, and growth factors as tailors of the stem cell niche. Curr. Opin. Cell Biol. 24 (5), 645–651. doi:10.1016/j.ceb.2012.07.001
Brooks, R., Williamson, R., and Bass, M. (2012). Syndecan-4 independently regulates multiple small GTPases to promote fibroblast migration during wound healing. Small GTPases 3 (2), 73–79. doi:10.4161/sgtp.19301
Broussard, J. A., Webb, D. J., and Kaverina, I. (2008). Asymmetric focal adhesion disassembly in motile cells. Curr. Opin. Cell Biol. 20 (1), 85–90. doi:10.1016/j.ceb.2007.10.009
Buckley, C., Doyonnas, R., Newton, J., Blystone, S., Brown, E., Watt, S., et al. (1996). Identification of alpha v beta 3 as a heterotypic ligand for CD31/PECAM-1. J. Cell Sci. 109 (2), 437–445. doi:10.1242/jcs.109.2.437
Burgett, M. E., Lathia, J. D., Roth, P., Nowacki, A. S., Galileo, D. S., Pugacheva, E., et al. (2016). Direct contact with perivascular tumor cells enhances integrin αvβ3 signaling and migration of endothelial cells. Oncotarget 7 (28), 43852–43867. doi:10.18632/oncotarget.9700
Burgos-Bravo, F., Figueroa, N. L., Casanova-Morales, N., Quest, A. F. G., Wilson, C. A. M., and Leyton, L. (2018). Single-molecule measurements of the effect of force on Thy-1/αvβ3-integrin interaction using nonpurified proteins. Mol. Biol. Cell 29 (3), 326–338. doi:10.1091/mbc.E17-03-0133
Burgos-Bravo, F., Martinez-Meza, S., Quest, A. F. G., Wilson, C. A. M., and Leyton, L. (2020). Application of force to a syndecan-4 containing complex with thy-1-αvβ3 integrin accelerates neurite retraction. Front. Mol. Biosci. 7, 582257. doi:10.3389/fmolb.2020.582257
Burridge, K., and Guilluy, C. (2016). Focal adhesions, stress fibers and mechanical tension. Exp. Cell Res. 343 (1), 14–20. doi:10.1016/j.yexcr.2015.10.029
Burridge, K., and Wennerberg, K. (2004). Rho and Rac take center stage. Cell 116 (2), 167–179. doi:10.1016/s0092-8674(04)00003-0
Byron, A., Askari, J. A., Humphries, J. D., Jacquemet, G., Koper, E. J., Warwood, S., et al. (2015). A proteomic approach reveals integrin activation state-dependent control of microtubule cortical targeting. Nat. Commun. 6, 6135–6149. doi:10.1038/ncomms7135
Calderwood, D. A., and Ginsberg, M. H. (2003). Talin forges the links between integrins and actin. Nat. Cell Biol. 5 (8), 694–697. doi:10.1038/ncb0803-694
Calvo, F., Ranftl, R., Hooper, S., Farrugia, A. J., Moeendarbary, E., Bruckbauer, A., et al. (2015). Cdc42EP3/BORG2 and septin network enables mechano-transduction and the emergence of cancer-associated fibroblasts. Cell Rep. 13 (12), 2699–2714. doi:10.1016/j.celrep.2015.11.052
Campbell, I. D., and Humphries, M. J. (2011). Integrin structure, activation, and interactions. Cold Spring Harb. Perspect. Biol. 3 (3), a004994–a005010. doi:10.1101/cshperspect.a004994
Carisey, A., and Ballestrem, C. (2011). Vinculin, an adapter protein in control of cell adhesion signalling. Eur. J. Cell Biol. 90 (2-3), 157–163. doi:10.1016/j.ejcb.2010.06.007
Case, L. B., and Waterman, C. M. (2015). Integration of actin dynamics and cell adhesion by a three-dimensional, mechanosensitive molecular clutch. Nat. Cell Biol. 17 (8), 955–963. doi:10.1038/ncb3191
Cavalheiro, R. P., Lima, M. A., Jarrouge-Boucas, T. R., Viana, G. M., Lopes, C. C., Coulson-Thomas, V. J., et al. (2017). Coupling of vinculin to F-actin demands Syndecan-4 proteoglycan. Matrix Biol. 63, 23–37. doi:10.1016/j.matbio.2016.12.006
Cavini, I. A., Leonardo, D. A., Rosa, H. V. D., Castro, D., D'Muniz Pereira, H., Valadares, N. F., et al. (2021). The structural biology of septins and their filaments: an update. Front. Cell Dev. Biol. 9, 765085–765110. doi:10.3389/fcell.2021.765085
Chan, K. T., Bennin, D. A., and Huttenlocher, A. (2010). Regulation of adhesion dynamics by calpain-mediated proteolysis of focal adhesion kinase (FAK). J. Biol. Chem. 285 (15), 11418–11426. doi:10.1074/jbc.M109.090746
Chang, T. Y., Chen, C., Lee, M., Chang, Y. C., Lu, C. H., Lu, S. T., et al. (2017). Paxillin facilitates timely neurite initiation on soft-substrate environments by interacting with the endocytic machinery. Elife 6, e31101–e31142. doi:10.7554/eLife.31101
Chang, Y. C., Nalbant, P., Birkenfeld, J., Chang, Z. F., and Bokoch, G. M. (2008). GEF-H1 couples nocodazole-induced microtubule disassembly to cell contractility via RhoA. Mol. Biol. Cell 19 (5), 2147–2153. doi:10.1091/mbc.e07-12-1269
Changede, R., Xu, X., Margadant, F., and Sheetz, M. P. (2015). Nascent integrin adhesions form on all matrix rigidities after integrin activation. Dev. Cell 35 (5), 614–621. doi:10.1016/j.devcel.2015.11.001
Chastney, M. R., Conway, J. R. W., and Ivaska, J. (2021). Integrin adhesion complexes. Curr. Biol. 31 (10), R536–R542. doi:10.1016/j.cub.2021.01.038
Chen, H. C., Appeddu, P. A., Parsons, J. T., Hildebrand, J. D., Schaller, M. D., and Guan, J. L. (1995). Interaction of focal adhesion kinase with cytoskeletal protein talin. J. Biol. Chem. 270 (28), 16995–16999. doi:10.1074/jbc.270.28.16995
Choi, J., Leyton, L., and Nham, S. U. (2005). Characterization of alphaX I-domain binding to Thy-1. Biochem. Biophys. Res. Commun. 331 (2), 557–561. doi:10.1016/j.bbrc.2005.04.006
Choi, Y. P., Kim, B. G., Gao, M. Q., Kang, S., and Cho, N. H. (2012). Targeting ILK and β4 integrin abrogates the invasive potential of ovarian cancer. Biochem. Biophys. Res. Commun. 427 (3), 642–648. doi:10.1016/j.bbrc.2012.09.114
Chronopoulos, A., Thorpe, S. D., Cortes, E., Lachowski, D., Rice, A. J., Mykuliak, V. V., et al. (2020). Syndecan-4 tunes cell mechanics by activating the kindlin-integrin-RhoA pathway. Nat. Mater 19 (6), 669–678. doi:10.1038/s41563-019-0567-1
Clark, K., Langeslag, M., Figdor, C. G., and van Leeuwen, F. N. (2007). Myosin II and mechanotransduction: a balancing act. Trends Cell Biol. 17 (4), 178–186. doi:10.1016/j.tcb.2007.02.002
Collins, C., Guilluy, C., Welch, C., O'Brien, E. T., Hahn, K., Superfine, R., et al. (2012). Localized tensional forces on PECAM-1 elicit a global mechanotransduction response via the integrin-RhoA pathway. Curr. Biol. 22 (22), 2087–2094. doi:10.1016/j.cub.2012.08.051
Coon, B. G., Baeyens, N., Han, J., Budatha, M., Ross, T. D., Fang, J. S., et al. (2015). Intramembrane binding of VE-cadherin to VEGFR2 and VEGFR3 assembles the endothelial mechanosensory complex. J. Cell Biol. 208 (7), 975–986. doi:10.1083/jcb.201408103
Cortesio, C. L., Boateng, L. R., Piazza, T. M., Bennin, D. A., and Huttenlocher, A. (2011). Calpain-mediated proteolysis of paxillin negatively regulates focal adhesion dynamics and cell migration. J. Biol. Chem. 286 (12), 9998–10006. doi:10.1074/jbc.M110.187294
Corti, F., Wang, Y., Rhodes, J. M., Atri, D., Archer-Hartmann, S., Zhang, J., et al. (2019). N-terminal syndecan-2 domain selectively enhances 6-O heparan sulfate chains sulfation and promotes VEGFA(165)-dependent neovascularization. Nat. Commun. 10 (1), 1562. doi:10.1038/s41467-019-09605-z
Couchman, J. R., and Woods, A. (1999). Syndecan-4 and integrins: combinatorial signaling in cell adhesion. J. Cell Sci. 112 (Pt 20), 3415–3420. doi:10.1242/jcs.112.20.3415
Crawford, A. W., and Beckerle, M. C. (1991). Purification and characterization of zyxin, an 82,000-dalton component of adherens junctions. J. Biol. Chem. 266 (9), 5847–5853. doi:10.1016/s0021-9258(19)67675-1
Crowley, E., and Horwitz, A. F. (1995). Tyrosine phosphorylation and cytoskeletal tension regulate the release of fibroblast adhesions. J. Cell Biol. 131 (2), 525–537. doi:10.1083/jcb.131.2.525
Cunniff, B., McKenzie, A. J., Heintz, N. H., and Howe, A. K. (2016). AMPK activity regulates trafficking of mitochondria to the leading edge during cell migration and matrix invasion. Mol. Biol. Cell 27 (17), 2662–2674. doi:10.1091/mbc.E16-05-0286
Das, S., Majid, M., and Baker, A. B. (2016). Syndecan-4 enhances PDGF-BB activity in diabetic wound healing. Acta Biomater. 42, 56–65. doi:10.1016/j.actbio.2016.07.001
de Castro, L. F., Maycas, M., Bravo, B., Esbrit, P., and Gortazar, A. (2015). VEGF receptor 2 (VEGFR2) activation is essential for osteocyte survival induced by mechanotransduction. J. Cell Physiol. 230 (2), 278–285. doi:10.1002/jcp.24734
del Rio, A., Perez-Jimenez, R., Liu, R., Roca-Cusachs, P., Fernandez, J. M., and Sheetz, M. P. (2009). Stretching single talin rod molecules activates vinculin binding. Science 323 (5914), 638–641. doi:10.1126/science.1162912
Denhez, F., Wilcox-Adelman, S. A., Baciu, P. C., Saoncella, S., Lee, S., French, B., et al. (2002). Syndesmos, a syndecan-4 cytoplasmic domain interactor, binds to the focal adhesion adaptor proteins paxillin and Hic-5. J. Biol. Chem. 277 (14), 12270–12274. doi:10.1074/jbc.M110291200
Derdak, S., Penney, D. P., Keng, P., Felch, M. E., Brown, D., and Phipps, R. P. (1992). Differential collagen and fibronectin production by Thy 1+ and Thy 1- lung fibroblast subpopulations. Am. J. Physiol. 263 (2 Pt 1), L283–L290. doi:10.1152/ajplung.1992.263.2.L283
De Rossi, G., Vahatupa, M., Cristante, E., Arokiasamy, S., Liyanage, S. E., May, U., et al. (2021). Pathological angiogenesis requires syndecan-4 for efficient VEGFA-induced VE-cadherin internalization. Arterioscler. Thromb. Vasc. Biol. 41 (4), 1374–1389. doi:10.1161/ATVBAHA.121.315941
De Rossi, G., and Whiteford, J. R. (2014). Syndecans in angiogenesis and endothelial cell biology. Biochem. Soc. Trans. 42 (6), 1643–1646. doi:10.1042/BST20140232
Dolat, L., Hunyara, J. L., Bowen, J. R., Karasmanis, E. P., Elgawly, M., Galkin, V. E., et al. (2014). Septins promote stress fiber-mediated maturation of focal adhesions and renal epithelial motility. J. Cell Biol. 207 (2), 225–235. doi:10.1083/jcb.201405050
Dorland, Y. L., and Huveneers, S. (2017). Cell-cell junctional mechanotransduction in endothelial remodeling. Cell Mol. Life Sci. 74 (2), 279–292. doi:10.1007/s00018-016-2325-8
Doyle, A. D., Nazari, S. S., and Yamada, K. M. (2022). Cell-extracellular matrix dynamics. Phys. Biol. 19 (2), 021002. doi:10.1088/1478-3975/ac4390
Drees, B., Friederich, E., Fradelizi, J., Louvard, D., Beckerle, M. C., and Golsteyn, R. M. (2000). Characterization of the interaction between zyxin and members of the Ena/vasodilator-stimulated phosphoprotein family of proteins. J. Biol. Chem. 275 (29), 22503–22511. doi:10.1074/jbc.M001698200
Echtermeyer, F., Streit, M., Wilcox-Adelman, S., Saoncella, S., Denhez, F., Detmar, M., et al. (2001). Delayed wound repair and impaired angiogenesis in mice lacking syndecan-4. J. Clin. Invest. 107 (2), R9-R14–R14. doi:10.1172/JCI10559
Elad, N., Volberg, T., Patla, I., Hirschfeld-Warneken, V., Grashoff, C., Spatz, J. P., et al. (2013). The role of integrin-linked kinase in the molecular architecture of focal adhesions. J. Cell Sci. 126 (Pt 18), 4099–4107. doi:10.1242/jcs.120295
Elices, M. J., Osborn, L., Takada, Y., Crouse, C., Luhowskyj, S., Hemler, M. E., et al. (1990). VCAM-1 on activated endothelium interacts with the leukocyte integrin VLA-4 at a site distinct from the VLA-4/fibronectin binding site. Cell 60 (4), 577–584. doi:10.1016/0092-8674(90)90661-w
Evans, E., Leung, A., Heinrich, V., and Zhu, C. (2004). Mechanical switching and coupling between two dissociation pathways in a P-selectin adhesion bond. Proc. Natl. Acad. Sci. U. S. A. 101 (31), 11281–11286. doi:10.1073/pnas.0401870101
Ezratty, E. J., Bertaux, C., Marcantonio, E. E., and Gundersen, G. G. (2009). Clathrin mediates integrin endocytosis for focal adhesion disassembly in migrating cells. J. Cell Biol. 187 (5), 733–747. doi:10.1083/jcb.200904054
Farrugia, A. J., Rodriguez, J., Orgaz, J. L., Lucas, M., Sanz-Moreno, V., and Calvo, F. (2020). CDC42EP5/BORG3 modulates SEPT9 to promote actomyosin function, migration, and invasion. J. Cell Biol. 219 (9), 570747–570772. doi:10.1083/jcb.201912159
Fiore, V. F., Ju, L., Chen, Y., Zhu, C., and Barker, T. H. (2014). Dynamic catch of a Thy-1-α5β1+syndecan-4 trimolecular complex. Nat. Commun. 5, 4886–4898. doi:10.1038/ncomms5886
Fiore, V. F., Strane, P. W., Bryksin, A. V., White, E. S., Hagood, J. S., and Barker, T. H. (2015). Conformational coupling of integrin and Thy-1 regulates Fyn priming and fibroblast mechanotransduction. J. Cell Biol. 211 (1), 173–190. doi:10.1083/jcb.201505007
Fiore, V. F., Wong, S. S., Tran, C., Tan, C., Xu, W., Sulchek, T., et al. (2018). αvβ3 Integrin drives fibroblast contraction and strain stiffening of soft provisional matrix during progressive fibrosis. JCI Insight 3 (20), 97597–97612. doi:10.1172/jci.insight.97597
Florian, J. A., Kosky, J. R., Ainslie, K., Pang, Z., Dull, R. O., and Tarbell, J. M. (2003). Heparan sulfate proteoglycan is a mechanosensor on endothelial cells. Circulation Res. 93 (10), e136–e142. doi:10.1161/01.RES.0000101744.47866.D5
Fradelizi, J., Noireaux, V., Plastino, J., Menichi, B., Louvard, D., Sykes, C., et al. (2001). ActA and human zyxin harbour Arp2/3-independent actin-polymerization activity. Nat. Cell Biol. 3 (8), 699–707. doi:10.1038/35087009
Franco, S. J., and Huttenlocher, A. (2005). Regulating cell migration: calpains make the cut. J. Cell Sci. 118 (Pt 17), 3829–3838. doi:10.1242/jcs.02562
Franco, S. J., Rodgers, M. A., Perrin, B. J., Han, J., Bennin, D. A., Critchley, D. R., et al. (2004). Calpain-mediated proteolysis of talin regulates adhesion dynamics. Nat. Cell Biol. 6 (10), 977–983. doi:10.1038/ncb1175
Frijhoff, J., Dagnell, M., Godfrey, R., and Ostman, A. (2014). Regulation of protein tyrosine phosphatase oxidation in cell adhesion and migration. Antioxid. Redox Signal 20 (13), 1994–2010. doi:10.1089/ars.2013.5643
Fukuda, K., Gupta, S., Chen, K., Wu, C., and Qin, J. (2009). The pseudoactive site of ILK is essential for its binding to alpha-Parvin and localization to focal adhesions. Mol. Cell 36 (5), 819–830. doi:10.1016/j.molcel.2009.11.028
Gardel, M. L., Schneider, I. C., Aratyn-Schaus, Y., and Waterman, C. M. (2010). Mechanical integration of actin and adhesion dynamics in cell migration. Annu. Rev. Cell Dev. Biol. 26, 315–333. doi:10.1146/annurev.cellbio.011209.122036
Ginsberg, M. H., Partridge, A., and Shattil, S. J. (2005). Integrin regulation. Curr. Opin. Cell Biol. 17 (5), 509–516. doi:10.1016/j.ceb.2005.08.010
Greene, D. K., Tumova, S., Couchman, J. R., and Woods, A. (2003). Syndecan-4 associates with alpha-actinin. J. Biol. Chem. 278 (9), 7617–7623. doi:10.1074/jbc.M207123200
Guilluy, C., Swaminathan, V., Garcia-Mata, R., O'Brien, E. T., Superfine, R., and Burridge, K. (2011). The Rho GEFs LARG and GEF-H1 regulate the mechanical response to force on integrins. Nat. Cell Biol. 13 (6), 722–727. doi:10.1038/ncb2254
Guo, T., He, C., Venado, A., and Zhou, Y. (2022). Extracellular matrix stiffness in lung health and disease. Compr. Physiol. 12 (3), 3523–3558. doi:10.1002/cphy.c210032
Gupta, M., Doss, B., Lim, C. T., Voituriez, R., and Ladoux, B. (2016). Single cell rigidity sensing: a complex relationship between focal adhesion dynamics and large-scale actin cytoskeleton remodeling. Cell Adh Migr. 10 (5), 554–567. doi:10.1080/19336918.2016.1173800
Haining, A. W., Lieberthal, T. J., and Del Rio Hernandez, A. (2016). Talin: a mechanosensitive molecule in health and disease. FASEB J. 30 (6), 2073–2085. doi:10.1096/fj.201500080R
Han, M. K. L., and de Rooij, J. (2016). Converging and unique mechanisms of mechanotransduction at adhesion sites. Trends Cell Biol. 26 (8), 612–623. doi:10.1016/j.tcb.2016.03.005
Hanks, S. K., Ryzhova, L., Shin, N. Y., and Brabek, J. (2003). Focal adhesion kinase signaling activities and their implications in the control of cell survival and motility. Front. Biosci. 8, d982–d996. doi:10.2741/1114
Hansen, M. D., and Beckerle, M. C. (2006). Opposing roles of zyxin/LPP ACTA repeats and the LIM domain region in cell-cell adhesion. J. Biol. Chem. 281 (23), 16178–16188. doi:10.1074/jbc.M512771200
Hansen, S. D., and Mullins, R. D. (2010). VASP is a processive actin polymerase that requires monomeric actin for barbed end association. J. Cell Biol. 191 (3), 571–584. doi:10.1083/jcb.201003014
Harris, T. J. C. (2017). Protein clustering for cell polarity: par-3 as a paradigm. F1000Res 6, 1620. doi:10.12688/f1000research.11976.1
Henning Stumpf, B., Ambriovic-Ristov, A., Radenovic, A., and Smith, A. S. (2020). Recent advances and prospects in the research of nascent adhesions. Front. Physiol. 11, 574371. doi:10.3389/fphys.2020.574371
Henriquez, M., Herrera-Molina, R., Valdivia, A., Alvarez, A., Kong, M., Munoz, N., et al. (2011). ATP release due to Thy-1-integrin binding induces P2X7-mediated calcium entry required for focal adhesion formation. J. Cell Sci. 124 (Pt 9), 1581–1588. doi:10.1242/jcs.073171
Hermosilla, T., Munoz, D., Herrera-Molina, R., Valdivia, A., Munoz, N., Nham, S. U., et al. (2008). Direct Thy-1/alphaVbeta3 integrin interaction mediates neuron to astrocyte communication. Biochim. Biophys. Acta 1783 (6), 1111–1120. doi:10.1016/j.bbamcr.2008.01.034
Horton, E. R. (2021). Functional bioinformatics analyses of the matrisome and integrin adhesome. Methods Mol. Biol. 2217, 285–300. doi:10.1007/978-1-0716-0962-0_16
Horton, E. R., Byron, A., Askari, J. A., Ng, D. H. J., Millon-Fremillon, A., Robertson, J., et al. (2015). Definition of a consensus integrin adhesome and its dynamics during adhesion complex assembly and disassembly. Nat. Cell Biol. 17 (12), 1577–1587. doi:10.1038/ncb3257
Hudon-David, F., Bouzeghrane, F., Couture, P., and Thibault, G. (2007). Thy-1 expression by cardiac fibroblasts: lack of association with myofibroblast contractile markers. J. Mol. Cell Cardiol. 42 (5), 991–1000. doi:10.1016/j.yjmcc.2007.02.009
Hueber, A. O., Pierres, M., and He, H. T. (1992). Sulfated glycans directly interact with mouse Thy-1 and negatively regulate Thy-1-mediated adhesion of thymocytes to thymic epithelial cells. J. Immunol. 148 (12), 3692–3699. doi:10.4049/jimmunol.148.12.3692
Huttelmaier, S., Mayboroda, O., Harbeck, B., Jarchau, T., Jockusch, B. M., and Rudiger, M. (1998). The interaction of the cell-contact proteins VASP and vinculin is regulated by phosphatidylinositol-4,5-bisphosphate. Curr. Biol. 8 (9), 479–488. doi:10.1016/s0960-9822(98)70199-x
Huttenlocher, A., and Horwitz, A. R. (2011). Integrins in cell migration. Cold Spring Harb. Perspect. Biol. 3 (9), a005074–a005091. doi:10.1101/cshperspect.a005074
Hynes, R. O. (2002). Integrins: bidirectional, allosteric signaling machines. Cell 110 (6), 673–687. doi:10.1016/s0092-8674(02)00971-6
Ijuin, T. (2019). Phosphoinositide phosphatases in cancer cell dynamics-Beyond PI3K and PTEN. Semin. Cancer Biol. 59, 50–65. doi:10.1016/j.semcancer.2019.03.003
Imhof, B. A., and Aurrand-Lions, M. (2004). Adhesion mechanisms regulating the migration of monocytes. Nat. Rev. Immunol. 4 (6), 432–444. doi:10.1038/nri1375
Jiang, G., Giannone, G., Critchley, D. R., Fukumoto, E., and Sheetz, M. P. (2003). Two-piconewton slip bond between fibronectin and the cytoskeleton depends on talin. Nature 424 (6946), 334–337. doi:10.1038/nature01805
Jockusch, B. M., and Isenberg, G. (1981). Interaction of alpha-actinin and vinculin with actin: opposite effects on filament network formation. Proc. Natl. Acad. Sci. U. S. A. 78 (5), 3005–3009. doi:10.1073/pnas.78.5.3005
Kanchanawong, P., Shtengel, G., Pasapera, A. M., Ramko, E. B., Davidson, M. W., Hess, H. F., et al. (2010). Nanoscale architecture of integrin-based cell adhesions. Nature 468 (7323), 580–584. doi:10.1038/nature09621
Kaplan, K. B., Bibbins, K. B., Swedlow, J. R., Arnaud, M., Morgan, D. O., and Varmus, H. E. (1994). Association of the amino-terminal half of c-Src with focal adhesions alters their properties and is regulated by phosphorylation of tyrosine 527. EMBO J. 13 (20), 4745–4756. doi:10.1002/j.1460-2075.1994.tb06800.x
Kaverina, I., Rottner, K., and Small, J. V. (1998). Targeting, capture, and stabilization of microtubules at early focal adhesions. J. Cell Biol. 142 (1), 181–190. doi:10.1083/jcb.142.1.181
Kechagia, J. Z., Ivaska, J., and Roca-Cusachs, P. (2019). Integrins as biomechanical sensors of the microenvironment. Nat. Rev. Mol. Cell Biol. 20 (8), 457–473. doi:10.1038/s41580-019-0134-2
Keller-Pinter, A., Gyulai-Nagy, S., Becsky, D., Dux, L., and Rovo, L. (2021). Syndecan-4 in tumor cell motility. Cancers (Basel) 13 (13), 3322. doi:10.3390/cancers13133322
Kenific, C. M., and Debnath, J. (2016). NBR1-dependent selective autophagy is required for efficient cell-matrix adhesion site disassembly. Autophagy 12 (10), 1958–1959. doi:10.1080/15548627.2016.1212789
King, S. J., Asokan, S. B., Haynes, E. M., Zimmerman, S. P., Rotty, J. D., Alb, J. G., et al. (2016). Lamellipodia are crucial for haptotactic sensing and response. J. Cell Sci. 129 (12), 2329–2342. doi:10.1242/jcs.184507
Klapholz, B., Herbert, S. L., Wellmann, J., Johnson, R., Parsons, M., and Brown, N. H. (2015). Alternative mechanisms for talin to mediate integrin function. Curr. Biol. 25 (7), 847–857. doi:10.1016/j.cub.2015.01.043
Kolasangiani, R., Bidone, T. C., and Schwartz, M. A. (2022). Integrin conformational dynamics and mechanotransduction. Cells 11 (22), 3584. doi:10.3390/cells11223584
Kong, F., Garcia, A. J., Mould, A. P., Humphries, M. J., and Zhu, C. (2009). Demonstration of catch bonds between an integrin and its ligand. J. Cell Biol. 185 (7), 1275–1284. doi:10.1083/jcb.200810002
Kong, M., Munoz, N., Valdivia, A., Alvarez, A., Herrera-Molina, R., Cardenas, A., et al. (2013). Thy-1-mediated cell-cell contact induces astrocyte migration through the engagement of αVβ3 integrin and syndecan-4. Biochim. Biophys. Acta 1833 (6), 1409–1420. doi:10.1016/j.bbamcr.2013.02.013
Krasny, L., and Huang, P. H. (2021). Advances in the proteomic profiling of the matrisome and adhesome. Expert Rev. Proteomics 18 (9), 781–794. doi:10.1080/14789450.2021.1984885
LaFoya, B., Munroe, J. A., Miyamoto, A., Detweiler, M. A., Crow, J. J., Gazdik, T., et al. (2018). Beyond the matrix: the many non-ECM ligands for integrins. Int. J. Mol. Sci. 19 (2), 449. doi:10.3390/ijms19020449
Lagos-Cabre, R., Alvarez, A., Kong, M., Burgos-Bravo, F., Cardenas, A., Rojas-Mancilla, E., et al. (2017). αVβ3 Integrin regulates astrocyte reactivity. J. Neuroinflammation 14 (1), 194. doi:10.1186/s12974-017-0968-5
Lagos-Cabre, R., Brenet, M., Diaz, J., Perez, R. D., Perez, L. A., Herrera-Molina, R., et al. (2018). Intracellular Ca(2+) increases and Connexin 43 hemichannel opening are necessary but not sufficient for thy-1-induced astrocyte migration. Int. J. Mol. Sci. 19 (8), 2179–2195. doi:10.3390/ijms19082179
Larsen, M., Tremblay, M. L., and Yamada, K. M. (2003). Phosphatases in cell–matrix adhesion and migration. Nat. Rev. Mol. Cell Biol. 4 (9), 700–711. doi:10.1038/nrm1199
Laukaitis, C. M., Webb, D. J., Donais, K., and Horwitz, A. F. (2001). Differential dynamics of alpha 5 integrin, paxillin, and alpha-actinin during formation and disassembly of adhesions in migrating cells. J. Cell Biol. 153 (7), 1427–1440. doi:10.1083/jcb.153.7.1427
Lawson, C. D., and Burridge, K. (2014). The on-off relationship of Rho and Rac during integrin-mediated adhesion and cell migration. Small GTPases 5, e27958–e27960. doi:10.4161/sgtp.27958
Le Coq, J., Acebron, I., Rodrigo Martin, B., Lopez Navajas, P., and Lietha, D. (2022). New insights into FAK structure and function in focal adhesions. J. Cell Sci. 135 (20), jcs259089. doi:10.1242/jcs.259089
Lee, M. J., Shin, J. O., and Jung, H. S. (2013). Thy-1 knockdown retards wound repair in mouse skin. J. Dermatol Sci. 69 (2), 95–104. doi:10.1016/j.jdermsci.2012.11.009
Legate, K. R., Montanez, E., Kudlacek, O., and Fassler, R. (2006). ILK, PINCH and parvin: the tIPP of integrin signalling. Nat. Rev. Mol. Cell Biol. 7 (1), 20–31. doi:10.1038/nrm1789
Legerstee, K., Geverts, B., Slotman, J. A., and Houtsmuller, A. B. (2019). Dynamics and distribution of paxillin, vinculin, zyxin and VASP depend on focal adhesion location and orientation. Sci. Rep. 9 (1), 10460–10478. doi:10.1038/s41598-019-46905-2
Legerstee, K., and Houtsmuller, A. B. (2021). A layered view on focal adhesions. Biol. (Basel) 10 (11), 1189–1220. doi:10.3390/biology10111189
Leyton, L., Diaz, J., Martinez, S., Palacios, E., Perez, L. A., and Perez, R. D. (2019). Thy-1/CD90 a bidirectional and lateral signaling scaffold. Front. Cell Dev. Biol. 7, 132–143. doi:10.3389/fcell.2019.00132
Leyton, L., Schneider, P., Labra, C. V., Ruegg, C., Hetz, C. A., Quest, A. F., et al. (2001). Thy-1 binds to integrin beta(3) on astrocytes and triggers formation of focal contact sites. Curr. Biol. 11 (13), 1028–1038. doi:10.1016/s0960-9822(01)00262-7
Li, N., Yang, H., Wang, M., Lu, S., Zhang, Y., and Long, M. (2018). Ligand-specific binding forces of LFA-1 and Mac-1 in neutrophil adhesion and crawling. Mol. Biol. Cell 29 (4), 408–418. doi:10.1091/mbc.E16-12-0827
Li, S., Kim, M., Hu, Y. L., Jalali, S., Schlaepfer, D. D., Hunter, T., et al. (1997). Fluid shear stress activation of focal adhesion kinase. Linking to mitogen-activated protein kinases. J. Biol. Chem. 272 (48), 30455–30462. doi:10.1074/jbc.272.48.30455
Lietha, D., and Izard, T. (2020). Roles of membrane domains in integrin-mediated cell adhesion. Int. J. Mol. Sci. 21 (15), 5531–5550. doi:10.3390/ijms21155531
Liu, E. T., and Lauffenburger, D. A. (2009). Systems biomedicine: concepts and perspectives. Academic Press.
Livne, A., and Geiger, B. (2016). The inner workings of stress fibers - from contractile machinery to focal adhesions and back. J. Cell Sci. 129 (7), 1293–1304. doi:10.1242/jcs.180927
Longley, R. L., Woods, A., Fleetwood, A., Cowling, G. J., Gallagher, J. T., and Couchman, J. R. (1999). Control of morphology, cytoskeleton and migration by syndecan-4. J. Cell Sci. 112 (Pt 20), 3421–3431. doi:10.1242/jcs.112.20.3421
Lu, J., Linares, B., Xu, Z., and Rui, Y. N. (2021). Mechanisms of FA-phagy, a new form of selective autophagy/organellophagy. Front. Cell Dev. Biol. 9, 799123. doi:10.3389/fcell.2021.799123
Mahajan, K. D., Nabar, G. M., Xue, W., Anghelina, M., Moldovan, N. I., Chalmers, J. J., et al. (2017). Mechanotransduction effects on endothelial cell proliferation via CD31 and VEGFR2: implications for immunomagnetic separation. Biotechnol. J. 12 (9). doi:10.1002/biot.201600750
Manninen, A., and Varjosalo, M. (2017). A proteomics view on integrin-mediated adhesions. Proteomics 17 (3)–4. doi:10.1002/pmic.201600022
Marshall, B. T., Long, M., Piper, J. W., Yago, T., McEver, R. P., and Zhu, C. (2003). Direct observation of catch bonds involving cell-adhesion molecules. Nature 423 (6936), 190–193. doi:10.1038/nature01605
Mavrakis, M., and Juanes, M. A. (2023). The compass to follow: focal adhesion turnover. Curr. Opin. Cell Biol. 80, 102152. doi:10.1016/j.ceb.2023.102152
Mierke, C. T. (2020). Mechanical cues affect migration and invasion of cells from three different directions. Front. Cell Dev. Biol. 8, 583226. doi:10.3389/fcell.2020.583226
Miller, B., and Sewell-Loftin, M. K. (2021). Mechanoregulation of vascular endothelial growth factor receptor 2 in angiogenesis. Front. Cardiovasc Med. 8, 804934. doi:10.3389/fcvm.2021.804934
Mishra, Y. G., and Manavathi, B. (2021). Focal adhesion dynamics in cellular function and disease. Cell Signal 85, 110046. doi:10.1016/j.cellsig.2021.110046
Montanez, E., Ussar, S., Schifferer, M., Bosl, M., Zent, R., Moser, M., et al. (2008). Kindlin-2 controls bidirectional signaling of integrins. Genes Dev. 22 (10), 1325–1330. doi:10.1101/gad.469408
Moon, J. J., Matsumoto, M., Patel, S., Lee, L., Guan, J. L., and Li, S. (2005). Role of cell surface heparan sulfate proteoglycans in endothelial cell migration and mechanotransduction. J. Cell Physiol. 203 (1), 166–176. doi:10.1002/jcp.20220
Morgan, M. R., Hamidi, H., Bass, M. D., Warwood, S., Ballestrem, C., and Humphries, M. J. (2013). Syndecan-4 phosphorylation is a control point for integrin recycling. Dev. Cell 24 (5), 472–485. doi:10.1016/j.devcel.2013.01.027
Morikis, V. A., Chase, S., Wun, T., Chaikof, E. L., Magnani, J. L., and Simon, S. I. (2017). Selectin catch-bonds mechanotransduce integrin activation and neutrophil arrest on inflamed endothelium under shear flow. Blood 130 (19), 2101–2110. doi:10.1182/blood-2017-05-783027
Morris, R. (1992). Thy-1, the enigmatic extrovert on the neuronal surface. Bioessays 14 (10), 715–722. doi:10.1002/bies.950141014
Moser, M., Nieswandt, B., Ussar, S., Pozgajova, M., and Fassler, R. (2008). Kindlin-3 is essential for integrin activation and platelet aggregation. Nat. Med. 14 (3), 325–330. doi:10.1038/nm1722
Munger, J. S., Harpel, J. G., Giancotti, F. G., and Rifkin, D. B. (1998). Interactions between growth factors and integrins: latent forms of transforming growth factor-beta are ligands for the integrin alphavbeta1. Mol. Biol. Cell 9 (9), 2627–2638. doi:10.1091/mbc.9.9.2627
Murakami, J. L., Xu, B., Franco, C. B., Hu, X., Galli, S. J., Weissman, I. L., et al. (2016). Evidence that β7 integrin regulates hematopoietic stem cell homing and engraftment through interaction with MAdCAM-1. Stem Cells Dev. 25 (1), 18–26. doi:10.1089/scd.2014.0551
Naba, A., Clauser, K. R., Ding, H., Whittaker, C. A., Carr, S. A., and Hynes, R. O. (2016). The extracellular matrix: tools and insights for the "omics" era. Matrix Biol. 49, 10–24. doi:10.1016/j.matbio.2015.06.003
Nikmanesh, M., Cancel, L. M., Shi, Z. D., and Tarbell, J. M. (2019). Heparan sulfate proteoglycan, integrin, and syndecan-4 are mechanosensors mediating cyclic strain-modulated endothelial gene expression in mouse embryonic stem cell-derived endothelial cells. Biotechnol. Bioeng. 116 (10), 2730–2741. doi:10.1002/bit.27104
Oakes, P. W., Beckham, Y., Stricker, J., and Gardel, M. L. (2012). Tension is required but not sufficient for focal adhesion maturation without a stress fiber template. J. Cell Biol. 196 (3), 363–374. doi:10.1083/jcb.201107042
Oh, E. S., Woods, A., and Couchman, J. R. (1997). Multimerization of the cytoplasmic domain of syndecan-4 is required for its ability to activate protein kinase C. J. Biol. Chem. 272 (18), 11805–11811. doi:10.1074/jbc.272.18.11805
Okina, E., Grossi, A., Gopal, S., Multhaupt, H. A., and Couchman, J. R. (2012). Alpha-actinin interactions with syndecan-4 are integral to fibroblast-matrix adhesion and regulate cytoskeletal architecture. Int. J. Biochem. Cell Biol. 44 (12), 2161–2174. doi:10.1016/j.biocel.2012.08.017
Olski, T. M., Noegel, A. A., and Korenbaum, E. (2001). Parvin, a 42 kDa focal adhesion protein, related to the alpha-actinin superfamily. J. Cell Sci. 114 (Pt 3), 525–538. doi:10.1242/jcs.114.3.525
Ono, Y., and Sorimachi, H. (2012). Calpains: an elaborate proteolitic system. BBA 1824 (1), 224–236. doi:10.1016/j.bbapap.2011.08.005
Ostermann, G., Weber, K. S., Zernecke, A., Schroder, A., and Weber, C. (2002). JAM-1 is a ligand of the beta(2) integrin LFA-1 involved in transendothelial migration of leukocytes. Nat. Immunol. 3 (2), 151–158. doi:10.1038/ni755
Palacios, E., Lobos-Gonzalez, L., Guerrero, S., Kogan, M. J., Shao, B., Heinecke, J. W., et al. (2023). Helicobacter pylori outer membrane vesicles induce astrocyte reactivity through nuclear factor-κappa B activation and cause neuronal damage in vivo in a murine model. J. Neuroinflammation 20 (1), 66. doi:10.1186/s12974-023-02728-7
Pang, X., He, X., Qiu, Z., Zhang, H., Xie, R., Liu, Z., et al. (2023). Targeting integrin pathways: mechanisms and advances in therapy. Signal Transduct. Target Ther. 8 (1), 1. doi:10.1038/s41392-022-01259-6
Parsons, J. T., Horwitz, A. R., and Schwartz, M. A. (2010). Cell adhesion: integrating cytoskeletal dynamics and cellular tension. Nat. Rev. Mol. Cell Biol. 11 (9), 633–643. doi:10.1038/nrm2957
Parsons, J. T., Martin, K. H., Slack, J. K., Taylor, J. M., and Weed, S. A. (2000). Focal adhesion kinase: a regulator of focal adhesion dynamics and cell movement. Oncogene 19 (49), 5606–5613. doi:10.1038/sj.onc.1203877
Pasapera, A. M., Schneider, I. C., Rericha, E., Schlaepfer, D. D., and Waterman, C. M. (2010). Myosin II activity regulates vinculin recruitment to focal adhesions through FAK-mediated paxillin phosphorylation. J. Cell Biol. 188 (6), 877–890. doi:10.1083/jcb.200906012
Pawson, T., Gish, G. D., and Nash, P. (2001). SH2 domains, interaction modules and cellular wiring. Trends Cell Biol. 11 (12), 504–511. doi:10.1016/s0962-8924(01)02154-7
Peacock, J. G., Miller, A. L., Bradley, W. D., Rodriguez, O. C., Webb, D. J., and Koleske, A. J. (2007). The Abl-related gene tyrosine kinase acts through p190RhoGAP to inhibit actomyosin contractility and regulate focal adhesion dynamics upon adhesion to fibronectin. Mol. Biol. Cell 18 (10), 3860–3872. doi:10.1091/mbc.e07-01-0075
Perez, L. A., Leon, J., Lopez, J., Rojas, D., Reyes, M., Contreras, P., et al. (2022a). The GPI-anchored protein thy-1/CD90 promotes wound healing upon injury to the skin by enhancing skin perfusion. Int. J. Mol. Sci. 23 (20), 12539. doi:10.3390/ijms232012539
Perez, L. A., Leyton, L., and Valdivia, A. (2022b). Thy-1 (CD90), integrins and syndecan 4 are key regulators of skin wound healing. Front. Cell Dev. Biol. 10, 810474. doi:10.3389/fcell.2022.810474
Perez, L. A., Rashid, A., Combs, J. D., Schneider, P., Rodriguez, A., Salaita, K., et al. (2021). An outside-in switch in integrin signaling caused by chemical and mechanical signals in reactive astrocytes. Front. Cell Dev. Biol. 9, 712627. doi:10.3389/fcell.2021.712627
Perez-Nunez, R., Chamorro, A., Gonzalez, M. F., Contreras, P., Artigas, R., Corvalan, A. H., et al. (2023). Protein kinase B (AKT) upregulation and Thy-1-αvβ3 integrin-induced phosphorylation of Connexin43 by activated AKT in astrogliosis. J. Neuroinflammation 20 (1), 5. doi:10.1186/s12974-022-02677-7
Phuan, P. W., Tan, J. A., Rivera, A. A., Zlock, L., Nielson, D. W., Finkbeiner, W. E., et al. (2019). Nanomolar-potency 'co-potentiator' therapy for cystic fibrosis caused by a defined subset of minimal function CFTR mutants. Sci. Rep. 9 (1), 17640. doi:10.1038/s41598-019-54158-2
Posor, Y., Kampyli, C., Bilanges, B., Ganguli, S., Koch, P. A., Wallroth, A., et al. (2022). Local synthesis of the phosphatidylinositol-3,4-bisphosphate lipid drives focal adhesion turnover. Dev. Cell 57 (14), 1694–1711.e7. doi:10.1016/j.devcel.2022.06.011
Puklin-Faucher, E., and Vogel, V. (2009). Integrin activation dynamics between the RGD-binding site and the headpiece hinge. J. Biol. Chem. 284 (52), 36557–36568. doi:10.1074/jbc.M109.041194
Rafiq, N. B. M., Nishimura, Y., Plotnikov, S. V., Thiagarajan, V., Zhang, Z., Shi, S., et al. (2019). A mechano-signalling network linking microtubules, myosin IIA filaments and integrin-based adhesions. Nat. Mater 18 (6), 638–649. doi:10.1038/s41563-019-0371-y
Rapraeger, A. C. (2021). Syndecans and their synstatins: targeting an organizer of receptor tyrosine kinase signaling at the cell-matrix interface. Front. Oncol. 11, 775349. doi:10.3389/fonc.2021.775349
Rege, T. A., Pallero, M. A., Gomez, C., Grenett, H. E., Murphy-Ullrich, J. E., and Hagood, J. S. (2006). Thy-1, via its GPI anchor, modulates Src family kinase and focal adhesion kinase phosphorylation and subcellular localization, and fibroblast migration, in response to thrombospondin-1/hep I. Exp. Cell Res. 312 (19), 3752–3767. doi:10.1016/j.yexcr.2006.07.029
Reinhard, M., Jouvenal, K., Tripier, D., and Walter, U. (1995). Identification, purification, and characterization of a zyxin-related protein that binds the focal adhesion and microfilament protein VASP (vasodilator-stimulated phosphoprotein). Proc. Natl. Acad. Sci. U. S. A. 92 (17), 7956–7960. doi:10.1073/pnas.92.17.7956
Rens, E. G., and Merks, R. M. H. (2020). Cell shape and durotaxis explained from cell-extracellular matrix forces and focal adhesion dynamics. iScience 23 (9), 101488. doi:10.1016/j.isci.2020.101488
Revach, O. Y., Grosheva, I., and Geiger, B. (2020). Biomechanical regulation of focal adhesion and invadopodia formation. J. Cell Sci. 133 (20), jcs244848. doi:10.1242/jcs.244848
Ridley, A. J., and Hall, A. (1992). The small GTP-binding protein rho regulates the assembly of focal adhesions and actin stress fibers in response to growth factors. Cell 70 (3), 389–399. doi:10.1016/0092-8674(92)90163-7
Ripamonti, M., Liaudet, N., Azizi, L., Bouvard, D., Hytonen, V. P., and Wehrle-Haller, B. (2021). Structural and functional analysis of LIM domain-dependent recruitment of paxillin to αvβ3 integrin-positive focal adhesions. Commun. Biol. 4 (1), 380. doi:10.1038/s42003-021-01886-9
Roca-Cusachs, P., del Rio, A., Puklin-Faucher, E., Gauthier, N. C., Biais, N., and Sheetz, M. P. (2013). Integrin-dependent force transmission to the extracellular matrix by alpha-actinin triggers adhesion maturation. Proc. Natl. Acad. Sci. U. S. A. 110 (15), E1361–E1370. doi:10.1073/pnas.1220723110
Roca-Cusachs, P., Gauthier, N. C., Del Rio, A., and Sheetz, M. P. (2009). Clustering of alpha(5)beta(1) integrins determines adhesion strength whereas alpha(v)beta(3) and talin enable mechanotransduction. Proc. Natl. Acad. Sci. U. S. A. 106 (38), 16245–16250. doi:10.1073/pnas.0902818106
Roca-Cusachs, P., Iskratsch, T., and Sheetz, M. P. (2012). Finding the weakest link: exploring integrin-mediated mechanical molecular pathways. J. Cell Sci. 125 (Pt 13), 3025–3038. doi:10.1242/jcs.095794
Rodriguez-Fernandez, L., Company, S., Zaragoza, R., Vina, J. R., and Garcia-Trevijano, E. R. (2021). Cleavage and activation of LIM kinase 1 as a novel mechanism for calpain 2-mediated regulation of nuclear dynamics. Sci. Rep. 11 (1), 16339. doi:10.1038/s41598-021-95797-8
Rognoni, E., Ruppert, R., and Fassler, R. (2016). The kindlin family: functions, signaling properties and implications for human disease. J. Cell Sci. 129 (1), 17–27. doi:10.1242/jcs.161190
Saalbach, A., Hildebrandt, G., Haustein, U. F., and Anderegg, U. (2002). The Thy-1/Thy-1 ligand interaction is involved in binding of melanoma cells to activated Thy-1- positive microvascular endothelial cells. Microvasc. Res. 64 (1), 86–93. doi:10.1006/mvre.2002.2401
Saalbach, A., Wetzel, A., Haustein, U. F., Sticherling, M., Simon, J. C., and Anderegg, U. (2005). Interaction of human Thy-1 (CD 90) with the integrin alphavbeta3 (CD51/CD61): an important mechanism mediating melanoma cell adhesion to activated endothelium. Oncogene 24 (29), 4710–4720. doi:10.1038/sj.onc.1208559
Saalbach, A., Wetzig, T., Haustein, U. F., and Anderegg, U. (1999). Detection of human soluble Thy-1 in serum by ELISA. Fibroblasts and activated endothelial cells are a possible source of soluble Thy-1 in serum. Cell Tissue Res. 298 (2), 307–315. doi:10.1007/s004419900079
Sandilands, E., Serrels, B., McEwan, D. G., Morton, J. P., Macagno, J. P., McLeod, K., et al. (2011). Autophagic targeting of Src promotes cancer cell survival following reduced FAK signalling. Nat. Cell Biol. 14 (1), 51–60. doi:10.1038/ncb2386
Saoncella, S., Echtermeyer, F., Denhez, F., Nowlen, J. K., Mosher, D. F., Robinson, S.D., et al. (1999). Syndecan-4 signals cooperatively with integrins in a Rho-dependent manner in the assembly of focal adhesions and actin stress fibers. Proc. Natl. Acad. Sci. U. S. A. 96 (6), 2805–2810. doi:10.1073/pnas.96.6.2805
Sattler, M., Verma, S., Pride, Y. B., Salgia, R., Rohrschneider, L. R., and Griffin, J. D. (2001). SHIP1, an SH2 domain containing polyinositol-5-phosphatase, regulates migration through two critical tyrosine residues and forms a novel signaling complex with DOK1 and CRKL. J. Biol. Chem. 276 (4), 2451–2458. doi:10.1074/jbc.M006250200
Sauzay, C., Voutetakis, K., Chatziioannou, A., Chevet, E., and Avril, T. (2019). CD90/Thy-1, a cancer-associated cell surface signaling molecule. Front. Cell Dev. Biol. 7, 66. doi:10.3389/fcell.2019.00066
Sawada, Y., Tamada, M., Dubin-Thaler, B. J., Cherniavskaya, O., Sakai, R., Tanaka, S., et al. (2006). Force sensing by mechanical extension of the Src family kinase substrate p130Cas. Cell 127 (5), 1015–1026. doi:10.1016/j.cell.2006.09.044
Saxton, T. M., Ciruna, B. G., Holmyard, D., Kulkarni, S., Harpal, K., Rossant, J., et al. (2000). The SH2 tyrosine phosphatase shp2 is required for mammalian limb development. Nat. Genet. 24 (4), 420–423. doi:10.1038/74279
Schaller, M. D. (2010). Cellular functions of FAK kinases: insight into molecular mechanisms and novel functions. J. Cell Sci. 123 (Pt 7), 1007–1013. doi:10.1242/jcs.045112
Schiller, H. B., and Fässler, R. (2013). Mechanosensitivity and compositional dynamics of cell–matrix adhesions. EMBO Rep. 14 (6), 509–519. doi:10.1038/embor.2013.49
Schiller, H. B., Friedel, C. C., Boulegue, C., and Fassler, R. (2011). Quantitative proteomics of the integrin adhesome show a myosin II-dependent recruitment of LIM domain proteins. EMBO Rep. 12 (3), 259–266. doi:10.1038/embor.2011.5
Schubert, K., Gutknecht, D., Koberle, M., Anderegg, U., and Saalbach, A. (2013). Melanoma cells use Thy-1 (CD90) on endothelial cells for metastasis formation. Am. J. Pathol. 182 (1), 266–276. doi:10.1016/j.ajpath.2012.10.003
Schwartz, A. B., Campos, O. A., Criado-Hidalgo, E., Chien, S., Del Alamo, J. C., Lasheras, J. C., et al. (2021). Elucidating the biomechanics of leukocyte transendothelial migration by quantitative imaging. Front. Cell Dev. Biol. 9, 635263. doi:10.3389/fcell.2021.635263
Schwartz, M. A. (2010). Integrins and extracellular matrix in mechanotransduction. Cold Spring Harb. Perspect. Biol. 2 (12), a005066. doi:10.1101/cshperspect.a005066
Seetharaman, S., and Etienne-Manneville, S. (2019). Microtubules at focal adhesions - a double-edged sword. J. Cell Sci. 132 (19), jcs232843. doi:10.1242/jcs.232843
Seetharaman, S., and Etienne-Manneville, S. (2020). Cytoskeletal crosstalk in cell migration. Trends Cell Biol. 30 (9), 720–735. doi:10.1016/j.tcb.2020.06.004
Seetharaman, S., Vianay, B., Roca, V., Farrugia, A. J., De Pascalis, C., Boeda, B., et al. (2022). Microtubules tune mechanosensitive cell responses. Nat. Mater 21 (3), 366–377. doi:10.1038/s41563-021-01108-x
SenGupta, S., Parent, C. A., and Bear, J. E. (2021). The principles of directed cell migration. Nat. Rev. Mol. Cell Biol. 22 (8), 529–547. doi:10.1038/s41580-021-00366-6
Seong, J., Tajik, A., Sun, J., Guan, J. L., Humphries, M. J., Craig, S. E., et al. (2013). Distinct biophysical mechanisms of focal adhesion kinase mechanoactivation by different extracellular matrix proteins. Proc. Natl. Acad. Sci. U. S. A. 110 (48), 19372–19377. doi:10.1073/pnas.1307405110
Sharifi, M. N., Mowers, E. E., Drake, L. E., Collier, C., Chen, H., Zamora, M., et al. (2016). Autophagy promotes focal adhesion disassembly and cell motility of metastatic tumor cells through the direct interaction of paxillin with LC3. Cell Rep. 15 (8), 1660–1672. doi:10.1016/j.celrep.2016.04.065
Shellard, A., and Mayor, R. (2020). All roads lead to directional cell migration. Trends Cell Biol. 30 (11), 852–868. doi:10.1016/j.tcb.2020.08.002
Shemesh, T., Verkhovsky, A. B., Svitkina, T. M., Bershadsky, A. D., and Kozlov, M. M. (2009). Role of focal adhesions and mechanical stresses in the formation and progression of the lamellipodium-lamellum interface [corrected]. Biophys. J. 97 (5), 1254–1264. doi:10.1016/j.bpj.2009.05.065
Shroff, H., Galbraith, C. G., Galbraith, J. A., White, H., Gillette, J., Olenych, S., et al. (2007). Dual-color superresolution imaging of genetically expressed probes within individual adhesion complexes. Proc. Natl. Acad. Sci. U. S. A. 104 (51), 20308–20313. doi:10.1073/pnas.0710517105
Small, J. V., Geiger, B., Kaverina, I., and Bershadsky, A. (2002). How do microtubules guide migrating cells?. Nat. Rev. Mol. Cell Biol. 3 (12), 957–964. doi:10.1038/nrm971
Smith, M. A., Blankman, E., Deakin, N. O., Hoffman, L. M., Jensen, C. C., Turner, C. E., et al. (2013). LIM domains target actin regulators paxillin and zyxin to sites of stress fiber strain. PLoS One 8 (8), e69378. doi:10.1371/journal.pone.0069378
Sperry, R. B., Bishop, N. H., Bramwell, J. J., Brodeur, M. N., Carter, M. J., Fowler, B. T., et al. (2010). Zyxin controls migration in epithelial-mesenchymal transition by mediating actin-membrane linkages at cell-cell junctions. J. Cell Physiol. 222 (3), 612–624. doi:10.1002/jcp.21977
Spiess, M., Hernandez-Varas, P., Oddone, A., Olofsson, H., Blom, H., Waithe, D., et al. (2018). Active and inactive β1 integrins segregate into distinct nanoclusters in focal adhesions. J. Cell Biol. 217 (6), 1929–1940. doi:10.1083/jcb.201707075
Staunton, D. E., Marlin, S. D., Stratowa, C., Dustin, M. L., and Springer, T. A. (1988). Primary structure of ICAM-1 demonstrates interaction between members of the immunoglobulin and integrin supergene families. Cell 52 (6), 925–933. doi:10.1016/0092-8674(88)90434-5
Stephens, L., Milne, L., and Hawkins, P. (2008). Moving towards a better understanding of chemotaxis. Curr. Biol. 18 (11), R485–R494. doi:10.1016/j.cub.2008.04.048
Strohmeyer, N., Bharadwaj, M., Costell, M., Fässler, R., and Müller, D. J. (2017). Fibronectin-bound α5β1 integrins sense load and signal to reinforce adhesion in less than a second. Nat. Mater. 16 (12), 1262–1270. doi:10.1038/nmat5023
Sun, H., Liu, J., Zheng, Y., Pan, Y., Zhang, K., and Chen, J. (2014). Distinct chemokine signaling regulates integrin ligand specificity to dictate tissue-specific lymphocyte homing. Dev. Cell 30 (1), 61–70. doi:10.1016/j.devcel.2014.05.002
Sun, X., and Alushin, G. M. (2022). Cellular force-sensing through actin filaments. FEBS J. 290, 2576–2589. doi:10.1111/febs.16568
Sun, Z., Costell, M., and Fässler, R. (2019). Integrin activation by talin, kindlin and mechanical forces. Nat. Cell Biol. 21 (1), 25–31. doi:10.1038/s41556-018-0234-9
Sun, Z., Guo, S. S., and Fassler, R. (2016). Integrin-mediated mechanotransduction. J. Cell Biol. 215 (4), 445–456. doi:10.1083/jcb.201609037
Sundd, P., Pospieszalska, M. K., and Ley, K. (2013). Neutrophil rolling at high shear: flattening, catch bond behavior, tethers and slings. Mol. Immunol. 55 (1), 59–69. doi:10.1016/j.molimm.2012.10.025
Tang, K., Boudreau, C. G., Brown, C. M., and Khadra, A. (2018). Paxillin phosphorylation at serine 273 and its effects on Rac, Rho and adhesion dynamics. PLoS Comput. Biol. 14 (7), e1006303. doi:10.1371/journal.pcbi.1006303
Tapial Martinez, P., Lopez Navajas, P., and Lietha, D. (2020). FAK structure and regulation by membrane interactions and force in focal adhesions. Biomolecules 10 (2), 179. doi:10.3390/biom10020179
Thievessen, I., Thompson, P. M., Berlemont, S., Plevock, K. M., Plotnikov, S. V., Zemljic-Harpf, A., et al. (2013). Vinculin-actin interaction couples actin retrograde flow to focal adhesions, but is dispensable for focal adhesion growth. J. Cell Biol. 202 (1), 163–177. doi:10.1083/jcb.201303129
Thinn, A. M. M., Wang, Z., Zhou, D., Zhao, Y., Curtis, B. R., and Zhu, J. (2018). Autonomous conformational regulation of β3 integrin and the conformation-dependent property of HPA-1a alloantibodies. Proc. Natl. Acad. Sci. U. S. A. 115 (39), E9105–E9114. doi:10.1073/pnas.1806205115
Tiveron, M. C., Barboni, E., Pliego Rivero, F. B., Gormley, A. M., Seeley, P. J., Grosveld, F., et al. (1992). Selective inhibition of neurite outgrowth on mature astrocytes by Thy-1 glycoprotein. Nature 355 (6362), 745–748. doi:10.1038/355745a0
Tomar, A., Lawson, C., Ghassemian, M., and Schlaepfer, D. D. (2012). Cortactin as a target for FAK in the regulation of focal adhesion dynamics. PLoS One 7 (8), e44041. doi:10.1371/journal.pone.0044041
Ussar, S., Moser, M., Widmaier, M., Rognoni, E., Harrer, C., Genzel-Boroviczeny, O., et al. (2008). Loss of Kindlin-1 causes skin atrophy and lethal neonatal intestinal epithelial dysfunction. PLoS Genet. 4 (12), e1000289. doi:10.1371/journal.pgen.1000289
Vakaloglou, K., and Zervas, C. (2012). Parvin-ILK: an intimate relationship. Bioarchitecture 2 (3), 91–94. doi:10.4161/bioa.20700
Valdivia, A., Cardenas, A., Brenet, M., Maldonado, H., Kong, M., Diaz, J., et al. (2020). Syndecan-4/PAR-3 signaling regulates focal adhesion dynamics in mesenchymal cells. Cell Commun. Signal 18 (1), 129. doi:10.1186/s12964-020-00629-3
Vasioukhin, V., Bauer, C., Yin, M., and Fuchs, E. (2000). Directed actin polymerization is the driving force for epithelial cell-cell adhesion. Cell 100 (2), 209–219. doi:10.1016/s0092-8674(00)81559-7
Vicente-Manzanares, M., Ma, X., Adelstein, R. S., and Horwitz, A. R. (2009). Non-muscle myosin II takes centre stage in cell adhesion and migration. Nat. Rev. Mol. Cell Biol. 10 (11), 778–790. doi:10.1038/nrm2786
Vicente-Manzanares, M., Zareno, J., Whitmore, L., Choi, C. K., and Horwitz, A. F. (2007). Regulation of protrusion, adhesion dynamics, and polarity by myosins IIA and IIB in migrating cells. J. Cell Biol. 176 (5), 573–580. doi:10.1083/jcb.200612043
Vuong, T. T., Reine, T. M., Sudworth, A., Jenssen, T. G., and Kolset, S. O. (2015). Syndecan-4 is a major syndecan in primary human endothelial cells in vitro, modulated by inflammatory stimuli and involved in wound healing. J. Histochem Cytochem 63 (4), 280–292. doi:10.1369/0022155415568995
Wakatsuki, T., Wysolmerski, R. B., and Elson, E. L. (2003). Mechanics of cell spreading: role of myosin II. J. Cell Sci. 116 (Pt 8), 1617–1625. doi:10.1242/jcs.00340
Wang, C., Yoo, Y., Fan, H., Kim, E., Guan, K. L., and Guan, J. L. (2010). Regulation of Integrin beta 1 recycling to lipid rafts by Rab1a to promote cell migration. J. Biol. Chem. 285 (38), 29398–29405. doi:10.1074/jbc.M110.141440
Wang, H., Jin, H., and Rapraeger, A. C. (2015a). Syndecan-1 and syndecan-4 capture epidermal growth factor receptor family members and the α3β1 integrin via binding sites in their ectodomains: NOVEL synstatins prevent kinase capture and inhibit α6β4-INTEGRIN-DEPENDENT epithelial cell motility. J. Biol. Chem. 290 (43), 26103–26113. doi:10.1074/jbc.M115.679084
Wang, X., and Ha, T. (2013). Defining single molecular forces required to activate integrin and notch signaling. Science 340 (6135), 991–994. doi:10.1126/science.1231041
Wang, X., Sun, J., Xu, Q., Chowdhury, F., Roein-Peikar, M., Wang, Y., et al. (2015b). Integrin molecular tension within motile focal adhesions. Biophys. J. 109 (11), 2259–2267. doi:10.1016/j.bpj.2015.10.029
Wang, Y. X., Wang, D. Y., Guo, Y. C., and Guo, J. (2019). Zyxin: a mechanotransductor to regulate gene expression. Eur. Rev. Med. Pharmacol. Sci. 23 (1), 413–425. doi:10.26355/eurrev_201901_16790
Webb, D. J., Parsons, J. T., and Horwitz, A. F. (2002). Adhesion assembly, disassembly and turnover in migrating cells–over and over and over again. Nat. Cell Biol. 4 (4), E97–E100. doi:10.1038/ncb0402-e97
Wells, A., Huttenlocher, A., and Lauffenburger, D. A. (2005). Calpain proteases in cell adhesion and motility. Int. Rev. Cytol. 245, 1–16. doi:10.1016/S0074-7696(05)45001-9
Wetzel, A., Chavakis, T., Preissner, K. T., Sticherling, M., Haustein, U. F., Anderegg, U., et al. (2004). Human Thy-1 (CD90) on activated endothelial cells is a counterreceptor for the leukocyte integrin Mac-1 (CD11b/CD18). J. Immunol. 172 (6), 3850–3859. doi:10.4049/jimmunol.172.6.3850
Whittaker, C. A., Bergeron, K. F., Whittle, J., Brandhorst, B. P., Burke, R. D., and Hynes, R. O. (2006). The echinoderm adhesome. Dev. Biol. 300 (1), 252–266. doi:10.1016/j.ydbio.2006.07.044
Widmaier, M., Rognoni, E., Radovanac, K., Azimifar, S. B., and Fassler, R. (2012). Integrin-linked kinase at a glance. J. Cell Sci. 125 (Pt 8), 1839–1843. doi:10.1242/jcs.093864
Wilcox-Adelman, S. A., Denhez, F., and Goetinck, P. F. (2002). Syndecan-4 modulates focal adhesion kinase phosphorylation. J. Biol. Chem. 277 (36), 32970–32977. doi:10.1074/jbc.M201283200
Winograd-Katz, S. E., Fassler, R., Geiger, B., and Legate, K. R. (2014). The integrin adhesome: from genes and proteins to human disease. Nat. Rev. Mol. Cell Biol. 15 (4), 273–288. doi:10.1038/nrm3769
Wipff, P. J., Rifkin, D. B., Meister, J. J., and Hinz, B. (2007). Myofibroblast contraction activates latent TGF-beta1 from the extracellular matrix. J. Cell Biol. 179 (6), 1311–1323. doi:10.1083/jcb.200704042
Wojcikiewicz, E. P., Abdulreda, M. H., Zhang, X., and Moy, V. T. (2006). Force spectroscopy of LFA-1 and its ligands, ICAM-1 and ICAM-2. Biomacromolecules 7 (11), 3188–3195. doi:10.1021/bm060559c
Wojcikiewicz, E. P., Koenen, R. R., Fraemohs, L., Minkiewicz, J., Azad, H., Weber, C., et al. (2009). LFA-1 binding destabilizes the JAM-A homophilic interaction during leukocyte transmigration. Biophys. J. 96 (1), 285–293. doi:10.1529/biophysj.108.135491
Wolfenson, H., Bershadsky, A., Henis, Y. I., and Geiger, B. (2011). Actomyosin-generated tension controls the molecular kinetics of focal adhesions. J. Cell Sci. 124 (Pt 9), 1425–1432. doi:10.1242/jcs.077388
Wolfenson, H., Lavelin, I., and Geiger, B. (2013). Dynamic regulation of the structure and functions of integrin adhesions. Dev. Cell 24 (5), 447–458. doi:10.1016/j.devcel.2013.02.012
Woods, A., Longley, R. L., Tumova, S., and Couchman, J. R. (2000). Syndecan-4 binding to the high affinity heparin-binding domain of fibronectin drives focal adhesion formation in fibroblasts. Arch. Biochem. Biophys. 374 (1), 66–72. doi:10.1006/abbi.1999.1607
Wu, D., Xu, Y., Ding, T., Zu, Y., Yang, C., and Yu, L. (2017). Pairing of integrins with ECM proteins determines migrasome formation. Cell Res. 27 (11), 1397–1400. doi:10.1038/cr.2017.108
Wu, J.-C., Chen, Y.-C., Kuo, C.-T., Wenshin Yu, H., Chen, Y.-Q., Chiou, A., et al. (2015). Focal adhesion kinase-dependent focal adhesion recruitment of SH2 domains directs SRC into focal adhesions to regulate cell adhesion and migration. Sci. Rep. 5 (1), 18476–18513. doi:10.1038/srep18476
Wu, X., Kodama, A., and Fuchs, E. (2008). ACF7 regulates cytoskeletal-focal adhesion dynamics and migration and has ATPase activity. Cell 135 (1), 137–148. doi:10.1016/j.cell.2008.07.045
Xia, S., and Kanchanawong, P. (2017). Nanoscale mechanobiology of cell adhesions. Semin. Cell Dev. Biol. 71, 53–67. doi:10.1016/j.semcdb.2017.07.029
Yamada, K. M., Collins, J. W., Cruz Walma, D. A., Doyle, A. D., Morales, S. G., Lu, J., et al. (2019). Extracellular matrix dynamics in cell migration, invasion and tissue morphogenesis. Int. J. Exp. Pathol. 100 (3), 144–152. doi:10.1111/iep.12329
Yamada, K. M., and Sixt, M. (2019). Mechanisms of 3D cell migration. Nat. Rev. Mol. Cell Biol. 20 (12), 738–752. doi:10.1038/s41580-019-0172-9
Yan, J., Yao, M., Goult, B. T., and Sheetz, M. P. (2015). Talin dependent mechanosensitivity of cell focal adhesions. Cell Mol. Bioeng. 8 (1), 151–159. doi:10.1007/s12195-014-0364-5
Yang, H., Yu, J., Fu, G., Shi, X., Xiao, L., Chen, Y., et al. (2007). Interaction between single molecules of Mac-1 and ICAM-1 in living cells: an atomic force microscopy study. Exp. Cell Res. 313 (16), 3497–3504. doi:10.1016/j.yexcr.2007.08.001
Yao, M., Goult, B. T., Chen, H., Cong, P., Sheetz, M. P., and Yan, J. (2014). Mechanical activation of vinculin binding to talin locks talin in an unfolded conformation. Sci. Rep. 4, 4610–4617. doi:10.1038/srep04610
Yoshigi, M., Hoffman, L. M., Jensen, C. C., Yost, H. J., and Beckerle, M. C. (2005). Mechanical force mobilizes zyxin from focal adhesions to actin filaments and regulates cytoskeletal reinforcement. J. Cell Biol. 171 (2), 209–215. doi:10.1083/jcb.200505018
Yu, C. H., Law, J. B., Suryana, M., Low, H. Y., and Sheetz, M. P. (2011). Early integrin binding to Arg-Gly-Asp peptide activates actin polymerization and contractile movement that stimulates outward translocation. Proc. Natl. Acad. Sci. U. S. A. 108 (51), 20585–20590. doi:10.1073/pnas.1109485108
Zaidel-Bar, R., and Geiger, B. (2010). The switchable integrin adhesome. J. Cell Sci. 123 (Pt 9), 1385–1388. doi:10.1242/jcs.066183
Zaidel-Bar, R., Itzkovitz, S., Ma'ayan, A., Iyengar, R., and Geiger, B. (2007a). Functional atlas of the integrin adhesome. Nat. Cell Biol. 9 (8), 858–867. doi:10.1038/ncb0807-858
Zaidel-Bar, R., Milo, R., Kam, Z., and Geiger, B. (2007b). A paxillin tyrosine phosphorylation switch regulates the assembly and form of cell-matrix adhesions. J. Cell Sci. 120 (Pt 1), 137–148. doi:10.1242/jcs.03314
Zhang, X., Wojcikiewicz, E., and Moy, V. T. (2002). Force spectroscopy of the leukocyte function-associated antigen-1/intercellular adhesion molecule-1 interaction. Biophys. J. 83 (4), 2270–2279. doi:10.1016/S0006-3495(02)73987-8
Zhou, Y., Hagood, J. S., Lu, B., Merryman, W. D., and Murphy-Ullrich, J. E. (2010). Thy-1-integrin alphav beta5 interactions inhibit lung fibroblast contraction-induced latent transforming growth factor-beta1 activation and myofibroblast differentiation. J. Biol Chem. 285 (29), 22382–22393. doi:10.1074/jbc.M110.126227
Zhou, Y., Hagood, J. S., and Murphy-Ullrich, J. E. (2004). Thy-1 expression regulates the ability of rat lung fibroblasts to activate transforming growth factor-beta in response to fibrogenic stimuli. Am. J. Pathol. 165 (2), 659–669. doi:10.1016/s0002-9440(10)63330-5
Keywords: reactive astrocytes, cancer cells, fibroblasts, signal transduction, adhesome, mechanobiology, integrins, Thy-1 (CD90)
Citation: Valdivia A, Avalos AM and Leyton L (2023) Thy-1 (CD90)-regulated cell adhesion and migration of mesenchymal cells: insights into adhesomes, mechanical forces, and signaling pathways. Front. Cell Dev. Biol. 11:1221306. doi: 10.3389/fcell.2023.1221306
Received: 12 May 2023; Accepted: 25 September 2023;
Published: 30 November 2023.
Edited by:
Claudia Tanja Mierke, Leipzig University, GermanyReviewed by:
Ralph Böttcher, Max Planck Institute of Biochemistry, GermanyDaniel Bouvard, UMR5237 Centre de Recherche en Biologie cellulaire de Montpellier (CRBM), France
Copyright © 2023 Valdivia, Avalos and Leyton. This is an open-access article distributed under the terms of the Creative Commons Attribution License (CC BY). The use, distribution or reproduction in other forums is permitted, provided the original author(s) and the copyright owner(s) are credited and that the original publication in this journal is cited, in accordance with accepted academic practice. No use, distribution or reproduction is permitted which does not comply with these terms.
*Correspondence: Alejandra Valdivia, YWxlamFuZHJhLnZhbGRpdmlhQGVtb3J5LmVkdQ==; Lisette Leyton, bGxleXRvbkBtZWQudWNoaWxlLmNs