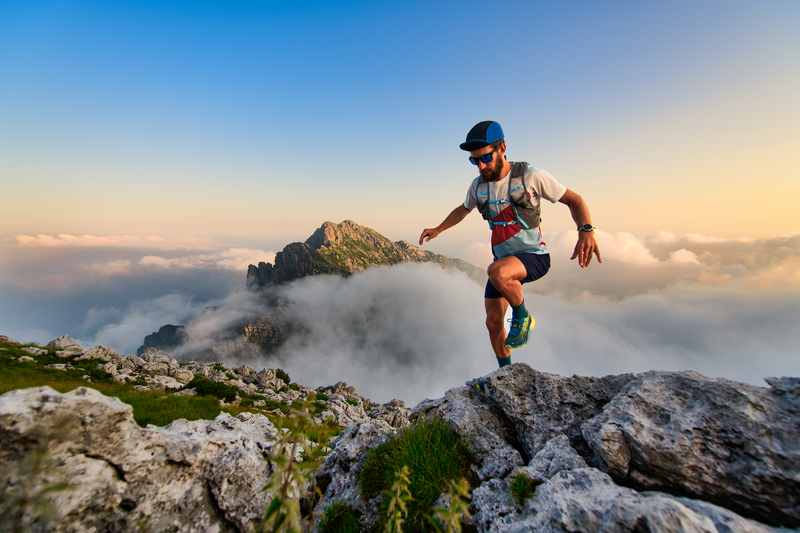
95% of researchers rate our articles as excellent or good
Learn more about the work of our research integrity team to safeguard the quality of each article we publish.
Find out more
REVIEW article
Front. Cell Dev. Biol. , 10 July 2023
Sec. Signaling
Volume 11 - 2023 | https://doi.org/10.3389/fcell.2023.1220405
This article is part of the Research Topic Pannexin and Connexin Signaling in Cell Death and Tissue Health and Disease View all 8 articles
Pannexin1 proteins form communication channels at the cell plasma membrane surface, which allow the transfer of small molecules and ions between the intracellular compartment and extracellular environment. In this way, pannexin1 channels play an important role in various cellular processes and diseases. Indeed, a plethora of human pathologies is associated with the activation of pannexin1 channels. The present paper reviews and summarizes the structure, life cycle, regulation and (patho)physiological roles of pannexin1 channels, with a particular focus on the relevance of pannexin1 channels in liver diseases.
Pannexin (Panx) proteins were first described in 2000. In the human body, 3 different types of Panx proteins are expressed, namely, Panx1, Panx2 and Panx3 (Vinken, 2022). Panx1 is undoubtedly the most abundantly studied Panx family member. Human Panx1 proteins are widely expressed and can be found in the brain, skin, lung, eye, breast, gastrointestinal tract, urinary system, reproductive system and blood cells (Table 1). These Panx1 proteins can assemble into oligomers to form communication channels (Deng et al., 2020; Qu et al., 2020; Zhang S. et al., 2021; Vinken, 2022). Over the past years, a large body of evidence has been generated showing that Panx1 channels are involved in physiological and pathological processes (Penuela et al., 2013; Vinken, 2022). Consequently, Panx1 channels are regarded as key determinants in the induction and propagation of inflammation and cell death processes, being hallmarks of a plethora of liver diseases (Cooreman et al., 2019; Vinken, 2022). Because of its unique anatomic location and multidimensional functions, the liver is a frequent target for disease (Vinken, 2020). Liver disease is one of the top 10 most common causes of death worldwide (Blachier et al., 2013; Ritchie et al., 2018). Liver disease is considered as a major public health concern affecting billions of people (Cheemerla and Balakrishnan, 2021; Riazi et al., 2022) and imposing a significant economic burden (Younossi et al., 2016). Moreover, it has become clear that the acting of Panx1 channels as so-called pathological pores equally holds true for hepatic Panx1 channels. In this review paper, we describe the structure, life cycle, regulation and (patho)physiological role of Panx1 channels. Critical attention is paid to the relevance of Panx1 channels in pathophysiology, in the specific context of acute and chronic liver disease.
TABLE 1. Human pannexin1 (Panx1) proteins are widely expressed. Panx1 proteins can be found in the brain, skin, lung, eye, breast, gastrointestinal tract, urinary system, reproductive system and blood cells.
Panx1 proteins are multi-pass transmembrane proteins consisting of 4 transmembrane domains, 2 extracellular loops, 1 cytoplasmic loop, 1 cytoplasmic N-terminal and 1 cytoplasmic C-terminal tail (Laird and Penuela, 2021; Vinken, 2022) (Figure 1). These Panx1 proteins can build-up heptameric channels, which facilitate paracrine communication by mediating the transfer of small molecules, including adenosine triphosphate (ATP), cyclic adenosine monophosphate and inositol triphosphate, and ions, such as calcium, chloride and potassium. By doing so, Panx1 channels control cellular communication between the cytosol and extracellular environment (Figure 1) (Deng et al., 2020; Qu et al., 2020; Zhang S. et al., 2021; Vinken, 2022). Concerning the liver, the presence of Panx1 protein has been demonstrated in both human and mouse liver tissue (Penuela et al., 2007; Leroy et al., 2022). Analysis of hepatic Panx1 mRNA levels in mice revealed that Panx1 is expressed by both parenchymal and non-parenchymal cells. In particular, Panx1 RNA was found in hepatic stellate cells, hepatocytes and Kupffer cells but not in liver sinusoidal endothelial cells (Willebrords et al., 2018).
FIGURE 1. Structure of pannexin1 (Panx1) proteins and Panx1 channels. Panx1 proteins consist of 4 transmembrane domains (TM1-4), 2 extracellular loops (ECL1-2), 1 cytoplasmic loop (CLP), 1 cytoplasmic N-terminal (NT) and 1 cytoplasmic C-terminal tail (CT). Panx1 proteins form heptameric channels at the cell plasma membrane surface to mediate the transfer of small molecules and ions between the intracellular and extracellular space.
The PANX1 gene is located on human chromosome 11q14.3, consisting of 5 exons and 4 introns (Baranova et al., 2004). As exon 5 is present in 2 variants, the human PANX1 gene drives the generation of 2 isoforms, PANX1a and PANX1b. PANX1a mRNA corresponds to a 426 amino acid protein with a molecular weight of 47.6 kDa, whereas the Panx1b mRNA encodes for a variant with an insertion of a 4 amino acid sequence 22 amino acids upstream of the C-terminal tail (Baranova et al., 2004). Furthermore, 2 shorter Panx1 isoforms, Panx1c and Panx1d, have been characterized in rat pituitary cells. Both are generated from alternative splicing at exons 2 and 4, respectively. Panx1c and Panx1d show a different cellular distribution pattern. Both splice variants are predominantly intracellular localized and they negatively modulate the functions of Panx1a through formation of heteromeric channels (Li et al., 2011). The presence of Panx1 splice variants might be explained by the notion that the Panx1 promotor is regulated via different mechanisms. The Panx1 promotor, which is highly homologous between rat, mouse and human sequences, possesses multiple transcriptional starting sites. Transcription factors E26 transformation-specific translocation variant 4 (ETV4) and 3′,5′-cyclic adenosine monophosphate response element-binding protein (CREB) bind to the rat Panx1 gene promoter to start transcription (Dufresne and Cyr, 2014). Next to cis/trans regulation, Panx1 gene expression is controlled by epigenetic mechanisms. In particular, methylation of DNA (Dufresne and Cyr, 2014) and changes in histone modifications (Zhang et al., 2015) contribute to increased Panx1 expression levels.
Panx1 proteins follow the classical endoplasmic reticulum-Golgi secretory pathway to form Panx1 channels at the cell plasma membrane surface (Bhalla-Gehi et al., 2010). While possibly serving as a reservoir of precursor Panx1 proteins, endoplasmic reticulum-bound Panx1 could equally reflect a role as a calcium channel (Vanden Abeele et al., 2006). Differences in subcellular localization are due to post-translational modifications. Unglycosylated Panx1 (Gly0) becomes glycosylated in the endoplasmic reticulum resulting in the high-mannose status (Gly1) (Penuela et al., 2007). Subsequently, Panx1 moves to the Golgi apparatus, where it is subjected to another round of glycosylation, yielding the Gly2 variant. This glycosylation event is involved in the trafficking to the cell plasma membrane surface (Penuela et al., 2007; Bhalla-Gehi et al., 2010). Accordingly, the Gly2 form is more abundant at the cell plasma membrane, while the Gly0 and Gly1 variants are preferentially found in the endoplasmic reticulum (Penuela et al., 2007). Cell plasma membrane-associated Panx1 proteins exhibit a fixed expression profile, i.e., their cell surface localization is steady for at least 8 h, after which they are internalized and subjected to lysosomal degradation (Penuela et al., 2007; Boassa et al., 2008; Bhalla-Gehi et al., 2010; Gehi et al., 2011). At the cell plasma membrane surface, Panx1 channels are activated by various triggers, including mechanical stress, intracellular calcium ions, extracellular ATP molecules and potassium ions (Qiu and Dahl, 2009; Suadicani et al., 2012; Diem et al., 2020; Lee et al., 2022). Furthermore, Panx1 channels are modulated by post-translational modifications of Panx1 proteins, including phosphorylation, deacetylation and proteolysis (Figure 2). First, the phosphorylation-induced channel activity is evidenced by conformational changes in the Panx1 channel structure, which increasing channel permeability in vitro (López et al., 2020). Moreover, kinase inhibitors are able to block Panx1 channel-mediated ATP release, indicating the involvement of tyrosine kinases in channel activity (Weilinger et al., 2012; Weilinger et al., 2016; DeLalio et al., 2019; Lohman et al., 2019). Second, acetylation/deacetylation reactions regulate Panx1 channel activity. Thus, histone deacetylase 6 evokes the opening of Panx1 channels in vitro (Chiu et al., 2021). Third, Panx1 channels are persistently activated by caspase-mediated cleavage of the C-terminal region of Panx1 proteins (Sandilos et al., 2012). Such caspase-driven channel activation can be mediated by both caspase 3 and caspase 11 (Yang et al., 2015; Narahari et al., 2021).
FIGURE 2. Pannexin1 (Panx1) channels are activated by various triggers. The amount of intracellular calcium ions (Ca2+), extracellular adenosine triphosphate (ATP) molecules and potassium (K+) ions, phosphorylation (P), deacetylation and cleavage of Panx1 proteins and mechanical stimulation are underlying mechanisms triggering Panx1 channel activity (+ = induction).
Panx1 channels support the transfer of biomolecules and ions that play essential roles in many physiological processes (Table 2). Therefore, the ability of Panx1 channels to support the export of ATP molecules is critical to control hemodynamic responses. Panx1 channels modulate the vascular tone as evidenced by impairment of vasodilatory responses to hypoxia for Panx1 knock-out mice. Panx1 channel-regulated export of ATP from red blood cells to plasma is essential to support changes in mean arterial pressure and hindlimb blood flow during hypoxia (Kirby et al., 2021). Panx1 channel-mediated ATP release also contributes to metabolic processes. Adipocytes indeed express functional Panx1 channels that regulate insulin-stimulated glucose uptake through the liberation of ATP (Adamson et al., 2015). In the central nervous system, ATP release through Panx1 channels is critical for hippocampus-dependent memory. Adult mice bearing loss of Panx1 channel activity indeed have increased postsynaptic excitability in the hippocampal region (Prochnow et al., 2012; Ardiles et al., 2014; Südkamp et al., 2021). Ablation of Panx1 proteins gives rise to comprised synaptic plasticity, substantiated by impairment of spatial and object recognition memory in Panx1 knock-out mice (Prochnow et al., 2012). Panx1 channel-mediated ATP transport is also required for hearing (Chen et al., 2015). Thus, Panx1 channels located in the cochlear wall of mice release ATP to ensure endocochlear and auditory receptor potential generation (Chen et al., 2015). Furthermore, physiological functions for Panx1 proteins have been proposed in the eye. Panx1 channel activity influences retinal signal transmission mechanisms, regulates microglial morphology and controls dynamic behavior (Fontainhas et al., 2011; Kranz et al., 2013; Kurtenbach et al., 2014). Genetic ablation of Panx1 in mice revealed a prominent function for Panx1 channels in skeletal muscle regeneration. Myoblasts derived from Panx1 knock-out animals display low migratory characteristics, morphological changes and more prominent surface blebs compared to wild-type counterparts. This deletion of Panx1 goes hand in hand with disturbed myoblast functioning, which is reflected by deficits in muscle regeneration (Suarez-Berumen et al., 2021). Moreover, Panx1 channel activity promotes mucociliary lung clearance (Seminario-Vidal et al., 2011; van Heusden et al., 2021). Panx1 channel-mediated ATP release contributes to normal lung physiology, since ciliary beat frequencies and mucociliary clearance activities are lower upon deletion of Panx1 in mice (van Heusden et al., 2021). Collectively, these data show that Panx1 channel activity underlies various physiological functions. The clinical relevance of these findings is demonstrated by a case of a 17-year-old female bearing a homozygous germline variant in the PANX1 gene. Expression of a R217H Panx1 variant is associated with loss of channel functionality, and provokes dysfunctionalities in multiple organs, including primary ovarian failure, intellectual disability, sensorineural hearing loss and skeletal defects (Shao et al., 2016).
Inflammation is a complex, yet well-organized body response that can be mediated by inflammasomes, which are multiprotein complexes (Guo et al., 2015; Wang and Hauenstein, 2020). To activate the innate immune system, pathogen-associated molecular patterns (PAMPs) and damage-associated molecular patterns (DAMPs) bind to Toll-like receptors (TLRs) (Guo et al., 2015; Shao et al., 2015; Wang and Hauenstein, 2020; Unterberger et al., 2021). Upon TLR activation, inflammasomes regulate the maturation and production of interleukins (ILs). The NOD-like receptor family pyrin domain containing 3 (NLRP3) inflammasome, the most intensively studied inflammasome, is involved in many events related to activation and regulation of inflammation (Kelley et al., 2019; Zheng et al., 2020). Canonical activation of NLRP3 activity evokes the release of IL-1β and IL-18 and generally requires 2 signals (Figure 3). The first signal is denoted the priming step. During this step, DAMPs, PAMPs and cytokines, including tumor necrosis factor (TNF) and IL-1β, activate the nuclear factor (NF)-κβ transcription factor. Consequently, NF-κβ-dependent gene transcription induces the expression of NLRP3, pro-IL-1β and pro-IL-18 (Guo et al., 2015; Shao et al., 2015; Gritsenko et al., 2020; Wang and Hauenstein, 2020). The second signal requires the presence of NLRP3 agonists. Extracellular ATP molecules, uric acid crystals and viral RNAs drive changes in the intracellular environment, including efflux of potassium ions, reactive oxygen species (ROS) formation and mobilization of calcium ions to stimulate the oligomerization of NLRP3 with an apoptosis-associated speck-like protein containing caspase (ASC)-like protein and pro-caspase 1. Following assemblage, the NLRP3 inflammasome becomes functional and activates caspase 1, which drives the secretion of IL-1β and IL-18 (Guo et al., 2015; Shao et al., 2015; Kelley et al., 2019; Wang and Hauenstein, 2020). Panx1 channels act as key players in this NLRP3 inflammasome cascade. In particular, Panx1 channel-mediated ATP release triggers P2X7 receptors (Pelegrin and Surprenant, 2006; Kanneganti et al., 2007; Albalawi et al., 2017; Parzych et al., 2017). This exacerbates the efflux of potassium ions and perpetuates the opening of Panx1 channels (Parzych et al., 2017). Interplay between Panx1 channel and P2X7 receptor activity drives NLRP3 oligomerization, caspase 1 activation and secretion of ILs. This interaction is elicited by TLR stimulation (Parzych et al., 2017). Experiments with human acute monocytic leukemia THP-1 cells show that both TLR2 and TLR4 signaling induce activation of the NLRP3 inflammasome and subsequent IL-1β release. However, a differential role for Panx1 proteins is observed. While TLR2-mediated IL-1β release is induced through Panx1 channel and P2X7 receptor activation, TLR4 signaling is independent of Panx1 and P2X7 proteins (Parzych et al., 2017). Furthermore, a mechanism for cleavage-based activation of Panx1 channels underlying NLRP3 signaling has been identified in rat renal tubular epithelial cells. Thus, caspase 11 promotes Panx1 cleavage to stimulate channel activity and maturation of IL-1β (Yin et al., 2022). Although Panx1 channels are generally recognized as essential actors in NLRP3 inflammasome activation, NLRP3 signaling can be Panx1-independent. In particular, knockdown and overexpression of Panx1 proteins does not affect NLRP3 inflammasome activation in THP-1 cells upon lipopolysaccharides (LPS) stimulation (Wang et al., 2013). Further experiments with Panx1 knock-out mice showed that Panx1 proteins are not required for NLRP3 inflammasome activation (Wang et al., 2013; Chen et al., 2020). In addition, Panx1 channels associate with NLRP1 signaling to trigger caspase 1. High extracellular potassium concentrations induce Panx1 channel opening and caspase 1 activation in rat cortical neurons and astrocytes (Silverman et al., 2009). Panx1 channel opening is equally triggered by the AIM2 inflammasome pathway. Blocking Panx1 channel-mediated communication reduces the secretion of pro-inflammatory cytokines IL-1β and IL-18 in rats following AIM2 inflammasome activation (Zheng et al., 2022).
FIGURE 3. Open pannexin1 (Panx1) channels stimulate NOD-like receptor family pyrin domain containing 3 (NLRP3) inflammasome activation. Canonical activation of the NLRP3 inflammasome generally requires 2 signals. During the priming step, damage-associated molecular patterns (DAMPs), pathogen-associated molecular patterns (PAMPs) and cytokines, including, tumor necrosis factor (TNF) and interleukin (IL)-1β activate the nuclear factor (NF)-κβ transcription factor. Consequently, NF-κβ-dependent gene transcription induces the expression of NLRP3, pro-IL-1β and pro-IL-18. The second signal requires the presence of NLRP3 agonists. Extracellular adenosine triphosphate (ATP) molecules, uric acid crystals and viral RNAs drive changes in the intracellular environment, including efflux of potassium, reactive oxygen species (ROS) formation and mobilization of calcium ions to stimulate oligomerization of NLRP3 with an apoptosis-associated speck-like protein containing caspase (ASC) and pro-caspase 1. Following assemblage, the NLRP3 inflammasome becomes functional and activates caspase 1, which drives the secretion of IL-1β and IL-18.
The pro-inflammatory role of Panx1 channels is underscored by their ability to modulate inflammatory responses through immune cell stimulation. Murine adipocytes and aortic endothelial cells express functional Panx1 channels that facilitate extracellular release of ATP molecules (Tozzi et al., 2020; Filiberto et al., 2022). Functional Panx1 channels promote macrophage migration (Tozzi et al., 2020) and activation, by triggering P2X7 receptors, and increasing the secretion of IL-1β (Filiberto et al., 2022). Likewise, activated Panx1 channels in murine T lymphocytes stimulate P2X receptors in an autocrine way to mediate the production of IL-2 and the proliferation of T lymphocytes (Schenk et al., 2008). P2X1/P2X4 receptor-mediated activation of IL2 gene transcription is also observed in human peripheral blood mononuclear cells and mouse splenocytes upon Panx1 channel-induced ATP release (Woehrle et al., 2010). Additionally, the interplay between purinergic receptors and Panx1 proteins underlies macrophage maturation. In order to form multinucleated macrophages, P2X7 receptor-mediated ATP release and Panx1 protein expression are essential, as shown in murine peritoneal macrophages stimulated with the inflammatory cytokine macrophage colony-stimulating factor (Lemaire et al., 2011). Furthermore, leukocyte activation is associated with Panx1 channel signaling. Panx1 channel-mediated ATP release evokes adhesion and migration of leukocytes towards inflamed tissue in mice (Lohman et al., 2015). Moreover, Panx1 channel activity is important for chemokinesis processes. The opening of Panx1 channels in epithelial cells is required to control ATP-induced chemokinesis in rat epiplexus cells (Maslieieva and Thompson, 2014).
Apoptosis is an energy-dependent process of programmed cell death characterized by various morphological changes, such as chromatin condensation, nuclei fragmentation and cell plasma membrane blebbing. During apoptosis, the cellular content is sealed in apoptotic bodies, which are removed by macrophages (Battistelli and Falcieri, 2020). Panx1 proteins are instrumental in this process, in particular in the activation of macrophages (Qu et al., 2011). Furthermore, Panx1 channels play an important role in apoptosis by releasing “find-me” signals (Figure 4). Panx1 channel-mediated signaling controls the recruitment of monocytes in apoptotic human T lymphocytes. Panx1 channel activity is hereby triggered by caspase cleavage (Chekeni et al., 2010). On the other hand, Panx1 channels release metabolites as “goodbye” signals (Figure 4) that modulate multiple gene programs in neighboring cells. By doing so, the opening of Panx1 channels in apoptosis may influence surrounding tissue (Medina et al., 2020). In this way, Panx1 channels establish neutrophil extracellular traps in apoptotic human breast cancer cells (Mukai et al., 2022). A function for caspase-stimulated Panx1 channel activity in apoptosis has been further demonstrated in murine bone marrow-derived macrophages and apoptotic cervical cancer cells (Chen et al., 2020; Imamura et al., 2020). Furthermore, pharmacological blocking of Panx1 channels increases the formation of apoptotic bodies in human T lymphocytes undergoing apoptosis. This effect is only observed in apoptotic cells. Without an apoptotic stimulus, the inhibition of Panx1 channels does not induce apoptosis nor the formation of apoptotic bodies (Poon et al., 2014). The observation that Panx1 channels regulate fragmentation of apoptotic bodies in murine thymocytes demonstrate their involvement as regulators of cellular disassembly during apoptosis (Poon et al., 2014). Panx1 channel opening provokes the accumulation of intracellular calcium ions in rat spinal neurocytes. The overexpression of Panx1 proteins is associated with enhanced expression levels of proapoptotic markers and induction of apoptosis (Huang et al., 2021). In addition, silencing Panx1 protein expression and blocking Panx1 channel activity limit apoptosis and production of apoptosis-related proteins in cultured human renal tubular epithelial cells and mice, respectively (Huang et al., 2020). Panx1 channels also participate in chemotherapeutic drug-induced apoptosis (Wu D. et al., 2017; Boyd-Tressler et al., 2017). In particular, Panx1 channels mediate ATP release to regulate the apoptosis rate in cisplatin-treated mouse Leydig cells (Wu D. et al., 2017). On the other hand, the opening of Panx1 channels has been identified as an underlying cause of apoptosis. Inhibition of Panx1 channels prevents subsequent responses that lead to apoptosis. In this way, blocking Panx1 channels prevents apoptosis through antagonism of p38 mitogen-activated protein kinase and TLR2/4/NF-κβ signaling pathways (Wu L. Y. et al., 2017; Luo et al., 2017).
FIGURE 4. Role of pannexin1 (Panx1) channels on different cell death mechanisms. Panx1 channel activity controls the regulation of cell death processes, including apoptosis, pyroptosis, ferroptosis and autophagy (adenosine triphosphate (ATP) molecules, gasdermin D (GSMD), glutathione peroxidase 4 (GPX4), oxidized glutathione (GSSH), purinergic receptor 7 (P2X7), reactive oxygen species (ROS), reduced glutathione (GSH)) (+ = induction, - = inhibition, ? = not yet fully understood).
Pyroptosis is an inflammatory form of lytic cell death, which is linked with inflammasome signaling. Activation of the inflammatory pathway triggers caspases that produce pro-inflammatory cytokines and pore-forming proteins. These pores allow the influx of water, leading to cell swelling and rupture of the cell plasma membrane (Yu et al., 2021). Panx1 channel activity induces pyroptosis via a P2X7-dependent mechanism (Figure 4). As such, caspase 11 cleaves Panx1 to stimulate Panx1 channel-dependent ATP release. This activates P2X7 receptors, leading to pyroptosis in murine bone marrow-derived macrophages (Yang et al., 2015). Similarly, Panx1 expression is required for pyroptosis processes in THP-1-derived macrophages (Zhang S. et al., 2021). Furthermore, experiments using the D379A human PANX1 mutant show that the caspase cleavage site controls pyroptosis. In addition, S424A mutation in the C-terminus of the PANX1 channel promotes pyroptosis when human macrophages are stimulated with LPS and ATP (Zhang S. et al., 2021). Moreover, liver X receptor β activation induces pyroptosis of human and murine colon cancer cells through Panx1 channels. The liver X receptor β interacts with Panx1 to trigger ATP release, in turn inducing pyroptosis by mediating NLRP3-inflammasome-dependent activation of caspase 1 (Derangère et al., 2014). In THP-1 cells, Panx1 channels also drive NLRP3 inflammasome signaling to trigger pyroptosis (Zhou et al., 2022).
Ferroptosis, an iron-dependent type of programmed cell death, is characterized by typical morphological changes, including the loss of structural integrity, shrinkage of mitochondria, a reduction or disappearance of mitochondrial cristae and rupture of the cell plasma membrane (Li et al., 2020; Jiang et al., 2021). Ferroptosis is induced through inhibition of the glutathione/glutamine antiporter system Xc−, inactivation of glutathione peroxidase 4, lipid peroxidation or iron-driven generation of ROS (Figure 4) (Yang and Stockwell, 2016; Li et al., 2020). Although the link between Panx1 channel activity and ferroptosis is not yet fully understood, experiments with cultured human tubular cells and mice show that Panx1 proteins are implicated in the mediation of ferroptosis in a renal ischemia/reperfusion-related injury. PANX1 gene transcription silencing reduces ferroptotic cell death in vitro and in vivo (Su et al., 2019). Furthermore, Panx1 expression regulates the production of ferroptosis-related proteins in human tubular cells upon exposure to erastin, an inhibitor of glutathione peroxidase 4 activity (Su et al., 2019). A similar correlation between Panx1 mRNA expression and ferroptosis-associated markers is seen in placental tissues. In preeclampsia patients, increased expression of Panx1 and pro-ferroptosis mediators has been described, while anti-ferroptosis regulators are negatively correlated (El-Khalik et al., 2022). A gene signature study on lung adenocarcinoma also identified the PANX1 gene as a ferroptosis-related prognosis gene. The established ferroptosis-related gene signature associates the overexpression of PANX1 with unfavorable impacts on lung adenocarcinoma prognosis (Zhang A. et al., 2021).
Autophagy entails the process of clearing old, damaged or abnormal cellular components via lysosomal degradation. Although autophagy is generally considered a cell protection mechanism, it is also a decisive process for cell death. Autophagic cell death features a state of cell death utilizing the autophagy machinery (Denton and Kumar, 2019; Jung et al., 2020). Panx1 channel-mediated ATP release suppresses autophagy in murine testicular cancer cells (Figure 4). While the knockdown of Panx1 expression and inhibition of Panx1 channels promotes autophagy, overexpression of Panx1 leads to the release of ATP, in turn hampering the autophagy process (Yuan et al., 2022). However, Panx1 channel activity also limits autophagy (Figure 4). In a sepsis mouse model, the inhibition of Panx1 channels alleviates pyroptosis and activates autophagy to establish a neuroprotective effect (Lei et al., 2021). On the other hand, Panx1 channels introduce an inflammatory feature in autophagic cells. Mouse bone-marrow-derived B lymphocytes undergoing autophagy release ATP in a Panx1 channel-dependent manner. Phagocytosis of such autophagic cells causes inflammasome activation in mouse macrophages, as evidenced by an increase in NLRP3 signaling and secretion of IL-1β (Ayna et al., 2012).
Acute liver failure (ALF) encompasses a rapid decline in liver function, i.e., within days or weeks, in a patient without preexisting liver disease. ALF is characterized by jaundice, coagulopathy and hepatic encephalopathy (Weiler et al., 2020). In addition, liver function tests of ALF-patients often display a prolonged prothrombin time, elevated serum levels of bilirubin, alanine aminotransferase (ALT) and aspartate aminotransferase (AST) (Björnsson and Olsson, 2005; Weiler et al., 2020). Impairment of liver function can be evoked by many triggers, such as viruses and drugs (Fontana, 2008; Weiler et al., 2020). Although reports on the etiology and incidence of ALF vary by region, acetaminophen (APAP)-induced liver disease is the leading cause of drug-induced liver failure in Western countries (Jaeschke et al., 2020). In overdose, APAP induces hepatotoxicity through the accumulation of N-acetyl-p-benzoquinone imine (NAPQI), a metabolite of APAP. Consequently, NAPQI provokes an inflammatory response in the liver (Jaeschke et al., 2020). Panx1 channels play an essential role in the pathogenesis of liver injury triggered by APAP. Genetic deletion of Panx1 partially prevents mice from developing APAP-induced liver damage as indicated by a decrease in serum ALT and AST activities. Application of a Panx1 channel blocker diminishes APAP-mediated liver injury as evidenced by a decrease in serum and liver levels of TNF-α, IL-6 and IL-10, reducing recruitment of neutrophils, altering the oxidative liver status and promoting liver regeneration in mice (Maes et al., 2017; Willebrords et al., 2018). Furthermore, APAP overdosing changes hepatic RNA and protein expression of Panx1 in mice. After APAP treatment, enhanced Panx1 gene transcription and Panx1 protein levels are measured in liver tissue (Maes et al., 2017). Panx1 RNA and protein expression levels are also increased during hepatic ischemia-reperfusion in rats and mice, respectively (Kim et al., 2015; Musavi et al., 2022). Ischemia-reperfusion injury is a major cause of ALF and hampers the overall success of clinical liver transplantation. This pathological process involves hepatic cell death and inflammation-mediated liver injury. Ischemic conditions expose hepatic cells to oxygen deprivation, pH changes and ATP depletion, heightening their glycogen-dependency and leading to parenchymal cell death. Subsequently, hepatic reperfusion triggers inflammation reactions promoting hepatocellular injury in the acute phase of liver ischemia-reperfusion injury (Zhai et al., 2013; Lu et al., 2016; Hirao et al., 2022). In this regard, an NLRP3 inflammasome-mediated response is triggered during hepatic ischemia-reperfusion injury in mice. This reaction is attenuated by blocking Panx1 channel activity or through Panx1 gene silencing and leads to a decrease in serum levels of IL-1β (Kim et al., 2015).
The most prevalent chronic liver disease worldwide is a non-alcoholic fatty liver disease (NAFLD), characterized by fat accumulation in hepatocytes that is not caused by alcohol consumption (Cheemerla and Balakrishnan, 2021; Pouwels et al., 2022). The progression of NAFLD towards non-alcoholic steatohepatitis (NASH) is driven by multiple insults, including insulin resistance, hormones secreted from the adipose tissue, dietary components, gut microbiota and genetic pathways (Buzzetti et al., 2016; Caturano et al., 2021). These mechanisms trigger intense inflammatory activity in the liver, which is a hallmark of NASH. NASH-related hepatotoxicity can be experimentally induced by feeding mice a specific diet, administration of drugs or through genetic modification (Willebrords et al., 2015; Lee et al., 2019; Matthews et al., 2021). A role for Panx1 proteins in NASH has been demonstrated by feeding Panx1 knock-out mice a methionine-choline-deficient diet. Panx1 knock-out mice show less liver damage compared to wild-type animals, evidenced by a reduction in ALT and AST activities and decrease in expression levels of liver inflammatory markers (Willebrords et al., 2018). Upon stimulation with free fatty acids, rat liver hepatoma-derived cells undergo lipoapoptosis, which goes along with Panx1 channel-mediated ATP release. This pathophysiological ATP release may act as a proinflammatory signal and contributes to hepatic inflammation in lipotoxic liver injury (Xiao et al., 2012, 2015). Similar to NASH, enhanced hepatic Panx1 RNA expression is also seen during liver fibrosis in mice (Crespo Yanguas et al., 2018; Willebrords et al., 2018). This chronic liver pathology is featured by abnormal wound healing, and is accompanied by injury and death of hepatocytes, scar formation, oxidative stress and inflammation. Liver fibrosis is typically induced in rodents through the repeated administration of carbon tetrachloride (CCl4) or thioacetamide (TAA) (Crespo Yanguas et al., 2016; Bao et al., 2021). Panx1 knock-out mice are found to be less sensitive to the effects of repeated administration of CCl4 (Crespo Yanguas et al., 2018). Moreover, Panx1 knock-out animals display reduced collagen content, hepatic stellate cell activation, inflammation and hepatic regeneration. The latter might comply with an underlying mechanism of NLRP3 inflammasome activation. Mice lacking ASC and NLRP3 show less fibrosis than wild-type littermates after treatment with CCl4 and TAA (Watanabe et al., 2009). The direct role of NLRP3 inflammasome in liver fibrosis has also been demonstrated in other experimental models of liver fibrosis. The absence and inhibition of NLRP3 attenuate hepatic fibrosis in murine models of aging and Schistosoma japonicum infection, respectively (Zhang et al., 2019; Gallego et al., 2020). Moreover, Panx1 channel-directed ATP release contributes to fibrosis in mice. Thus, blocking Panx1 channels prevents the development of hepatic fibrosis in a murine model of TAA-induced fibrosis (Feig et al., 2017). In contrast, ablation of Panx1 proteins even exacerbates the inflammatory profile in a surgical-based bile duct ligation (BDL) mouse model (Crespo Yanguas et al., 2018). BDL elicits a cholestatic injury and periportal biliary liver fibrosis (Crespo Yanguas et al., 2016), but this might be independent of NLRP3-independent mechanisms as a BDL-surgery increases liver fibrosis in the absence of caspase 1 in mice (Cai et al., 2020). This is, however, still surrounded by some controversy, since it has been documented that NLRP3 inflammasome inhibition suppresses cholestatic liver fibrosis in BDL-mice (Gong et al., 2016; Qu et al., 2019; Wang and Hauenstein, 2020; Frissen et al., 2021). Furthermore, a putative role for Panx1 channels is also seen during hepatitis C-virus (HCV) infection. In human hepatoma-derived cells, Panx1 channels control the release of exosomes and microRNAs contributing to liver disease development caused by HCV infection (Kim et al., 2021). On the other hand, hepatic Panx1 channels convey protective roles in donor livers. Panx1 channel activity in the liver controls the production of IL-33 and stimulates recruitment of macrophages and neutrophils. Thus, low Panx1 expression profiles are associated with an increased risk for methicillin-resistant Staphylococcus aureus infection in human liver transplantation recipients (Li et al., 2021). Moreover, this Panx1-IL33 axis plays a protective role in LPS-induced endotoxemia in mice by promoting the resolution of inflammation. Hepatic Panx1 expression and associated Panx1 channel activity regulates a defense mechanism by increasing the number of liver-infiltrating regulatory T lymphocytes and attenuating the cytokine storm (Wang et al., 2022).
Although Panx proteins have only been discovered about 2 decades ago, there is a general consensus regarding their role in physiological and, in particular, pathological processes. Panx1 channel activation is indeed considered as a key event in inflammation and cell death. A generic mechanism for the onset of inflammation and cell death could involve Panx1 channel-mediated extracellular ATP release. This trigger is involved in a plethora of diseases, including liver pathologies. However, few reports describe a protective role for Panx1 proteins. Indeed, genetic deletion of Panx1 is associated with dysfunctionalities in multiple organs. Future studies should pinpoint whether Panx1 protein expression or Panx1 channel activity controls the regulation of inflammatory reactions and cell death processes. The Panx research domain strongly relies on Panx1 channel blockers to elucidate (patho) physiological functions of Panx1 channels. However, established Panx1 channel inhibitors lack selectivity, specificity, potency and/or stability (Willebrords et al., 2017; Caufriez et al., 2020; Van Campenhout et al., 2021; Koval et al., 2023). New quinoline-based Panx1 channel inhibitors show great promise with their efficiency and structural relevance for channel blocking. However, more in vivo studies must confirm their efficiency and safety (Crocetti et al., 2022; Crocetti et al., 2023). Nevertheless, Panx1 channel blockers show great potential as anti-inflammatory drugs (Willebrords et al., 2017; Caufriez et al., 2020; Van Campenhout et al., 2021). Several groups are therefore focusing on the development of new Panx1 channel inhibitors. In line with this, the artificial intelligence (AI)-assisted drug discovery industry is gaining more attention. To date, numerous AI-based computer programs are applied for de novo drug design (Paul et al., 2021). Although this AI-driven approach is promising, it should be emphasized that the classic research path for drug discovery remains relevant. Thus, existing compound libraries show tremendous potential. High-throughput screening of natural product libraries should be embraced in the search for novel Panx1 channel blockers. Silibinin, a plant-derived polyphenol, protects hepatocytes against ischemia-reperfusion injury by suppressing mRNA expression of Panx1 in the liver (Musavi et al., 2022). In line with this, various screening strategies and functional assays can be developed to select for specific compound characteristics, such as affinity, specificity or blocking potential. Indeed, the identification of Panx1 channel inhibitors is a technical challenge. A number of tools exist to study the functionality of Panx1 channels, yet they are based on the measurement of variable and non-specific outputs, such as conductance states, extracellular release of ATP or cytosolic uptake of tracer dyes (Koval et al., 2023). In addition, progress has been made over the last few years with the establishment of Panx1 cryo-electron microscopy data (Deng et al., 2020; Qu et al., 2020; Zhang S. et al., 2021). This data suggest Panx1 channels be heptameric (Qu et al., 2020) instead of hexameric (Boassa et al., 2007), as initially predicted. However, the structure of the ATP-release channel is unknown. Encouraging research in this direction will open perspectives in the search for (hepatic) Panx1 channel blockers as it allows to determine how a Panx1 channel inhibitor interacts with its target, i.e., whether it sterically blocks the channel or induces a conformational rearrangement leading to channel closure.
RV, AC, AT, AM, SD, JS-S, PK, and MV drafted the manuscript. RV, PK, and MV critically revised the manuscript. MV secured the funding. All authors contributed to the article and approved the submitted version.
This work was financially supported by the European Future and Emerging Technologies program (grant 858014 “PANACHE”), the Fund for Scientific Research Research-Flanders, the University Hospital of the Vrije Universiteit Brussel-Belgium (“Willy Gepts Fonds” UZ-VUB) and the Methusalem program of the Flemish government-Belgium.
The authors declare that the research was conducted in the absence of any commercial or financial relationships that could be construed as a potential conflict of interest.
All claims expressed in this article are solely those of the authors and do not necessarily represent those of their affiliated organizations, or those of the publisher, the editors and the reviewers. Any product that may be evaluated in this article, or claim that may be made by its manufacturer, is not guaranteed or endorsed by the publisher.
AI, artificial intelligence; ALF, acute liver failure; ALT, alanine aminotransferase; APAP, acetaminophen; ASC, apoptosis-associated speck-like protein containing caspase; AST, aspartate aminotransferase; ATP, adenosine triphosphate; BDL, bile duct ligation; CCl4, carbon tetrachloride; CREB, 3′,5′-cyclic adenosine monophosphate response element-binding protein; DAMPs, damage-associated molecular patterns; ETV4, E26 transformation-specific translocation variant 4; HCV, hepatitis C-virus; ILs, interleukins; LPS, lipopolysaccharides; NAFLD, non-alcoholic fatty liver disease; NAPQI, N-acetyl-p-benzoquinone imine; NASH, non-alcoholic steatohepatitis; NF, nuclear factor; NLRP3, NOD-like receptor family pyrin domain containing 3; PAMPs, pathogen-associated molecular patterns; Panx, pannexin; TAA, thioacetamide; TLRs, Toll-like receptors; TNF, tumor necrosis factor.
Adamson, S. E., Meher, A. K., Chiu, Y. H., Sandilos, J. K., Oberholtzer, N. P., Walker, N. N., et al. (2015). Pannexin 1 is required for full activation of insulin-stimulated glucose uptake in adipocytes. Mol. Metab. 4, 610–618. doi:10.1016/j.molmet.2015.06.009
Albalawi, F., Lu, W., Beckel, J. M., Lim, J. C., McCaughey, S. A., and Mitchell, C. H. (2017). The P2X7 receptor primes IL-1β and the NLRP3 inflammasome in astrocytes exposed to mechanical strain. Front. Cell. Neurosci. 11, 227. doi:10.3389/fncel.2017.00227
Alhouayek, M., Sorti, R., Gilthorpe, J. D., and Fowler, C. J. (2019). Role of pannexin-1 in the cellular uptake, release and hydrolysis of anandamide by T84 colon cancer cells. Sci. Rep. 9, 1–12. doi:10.1038/s41598-019-44057-x
Ardiles, A. O., Flores-Muñoz, C., Toro-Ayala, G., Cárdenas, A. M., Palacios, A. G., Muñoz, P., et al. (2014). Pannexin 1 regulates bidirectional hippocampal synaptic plasticity in adult mice. Front. Cell. Neurosci. 8, 1–11. doi:10.3389/fncel.2014.00326
Ayna, G., Krysko, D. V., Kaczmarek, A., Petrovski, G., Vandenabeele, P., and Fésüs, L. (2012). ATP release from dying autophagic cells and their phagocytosis are crucial for inflammasome activation in macrophages. PLoS One 7, e40069. doi:10.1371/journal.pone.0040069
Bao, Y. L., Wang, L., Pan, H. T., Zhang, T. R., Chen, Y. H., Xu, S. J., et al. (2021). Animal and organoid models of liver fibrosis. Front. Physiol. 12, 666138. doi:10.3389/fphys.2021.666138
Baranova, A., Ivanov, D., Petrash, N., Pestova, A., Skoblov, M., Kelmanson, I., et al. (2004). The mammalian pannexin family is homologous to the invertebrate innexin gap junction proteins. Genomics 83, 706–716. doi:10.1016/j.ygeno.2003.09.025
Bartley, C., Brun, T., Oberhauser, L., Grimaldi, M., Molica, F., Kwak, B. R., et al. (2019). Chronic fructose renders pancreatic β-cells hyper-responsive to glucose-stimulated insulin secretion through extracellular ATP signaling. Am. J. Physiol. - Endocrinol. Metab. 317, 25–41. doi:10.1152/ajpendo.00456.2018
Battistelli, M., and Falcieri, E. (2020). Apoptotic bodies: Particular extracellular vesicles involved in intercellular communication. Biol. (Basel). 9, 21. doi:10.3390/biology9010021
Bhalla-Gehi, R., Penuela, S., Churko, J. M., Shao, Q., and Laird, D. W. (2010). Pannexin1 and pannexin3 delivery, cell surface dynamics, and cytoskeletal interactions. J. Biol. Chem. 285, 9147–9160. doi:10.1074/jbc.M109.082008
Björnsson, E., and Olsson, R. (2005). Outcome and prognostic markers in severe drug-induced liver disease. Hepatology 42, 481–489. doi:10.1002/hep.20800
Blachier, M., Leleu, H., Peck-Radosavljevic, M., Valla, D. C., and Roudot-Thoraval, F. (2013). The burden of liver disease in europe: A review of available epidemiological data. J. Hepatol. 58, 593–608. doi:10.1016/j.jhep.2012.12.005
Boassa, D., Ambrosi, C., Qiu, F., Dahl, G., Gaietta, G., and Sosinsky, G. (2007). Pannexin1 channels contain a glycosylation site that targets the hexamer to the plasma membrane. J. Biol. Chem. 282, 31733–31743. doi:10.1074/jbc.M702422200
Boassa, D., Qiu, F., Dahl, G., and Sosinsky, G. (2008). Trafficking dynamics of glycosylated pannexin1 proteins. Cell Commun. Adhes. 15, 119–132. doi:10.1080/15419060802013885
Boyd-Tressler, A. M., Lane, G. S., and Dubyak, G. R. (2017). Up-regulated ectonucleotidases in fas-associated death domain protein- and receptor-interacting protein kinase 1-deficient jurkat leukemia cells counteract extracellular ATP/AMP accumulation via pannexin-1 channels during chemotherapeutic drug-induced apoptosis. Mol. Pharmacol. 92, 30–47. doi:10.1124/mol.116.104000
Boyd-Tressler, A., Penuela, S., Laird, D. W., and Dubyak, G. R. (2014). Chemotherapeutic drugs induce ATP release via caspase-gated pannexin-1 channels and a caspase/pannexin-1-independent mechanism. J. Biol. Chem. 289, 27246–27263. doi:10.1074/jbc.M114.590240
Buzzetti, E., Pinzani, M., and Tsochatzis, E. A. (2016). The multiple-hit pathogenesis of non-alcoholic fatty liver disease (NAFLD). Metabolism 65, 1038–1048. doi:10.1016/j.metabol.2015.12.012
Cai, S. Y., Ge, M., Mennone, A., Hoque, R., Ouyang, X., and Boyer, J. L. (2020). Inflammasome is activated in the liver of cholestatic patients and aggravates hepatic injury in bile duct–ligated mouse. Cell Mol. Gastroenterol. Hepatol. 9, 679–688. doi:10.1016/j.jcmgh.2019.12.008
Cárcaba, L., García-Piqueras, J., García-Mesa, Y., Cobo, R., García-Suárez, O., Feito, J., et al. (2022). Human digital merkel cells display pannexin1 immunoreactivity. Ann. Anat. 239, 151813. doi:10.1016/j.aanat.2021.151813
Caturano, A., Acierno, C., Nevola, R., Pafundi, P. C., Galiero, R., Rinaldi, L., et al. (2021). Non-alcoholic fatty liver disease: From pathogenesis to clinical impact. Processes 9, 135. doi:10.3390/pr9010135
Caufriez, A., Böck, D., Martin, C., Ballet, S., and Vinken, M. (2020). Peptide-based targeting of connexins and pannexins for therapeutic purposes. Expert Opin. Drug Discov. 15, 1213–1222. doi:10.1080/17460441.2020.1773787
Cheemerla, S., and Balakrishnan, M. (2021). Global epidemiology of chronic liver disease. Clin. Liver Dis. 17, 365–370. doi:10.1002/cld.1061
Chekeni, F. B., Elliott, M. R., Sandilos, J. K., Walk, S. F., Kinchen, J. M., Lazarowski, E. R., et al. (2010). Pannexin 1 channels mediate “find-me” signal release and membrane permeability during apoptosis. Nature 467, 863–867. doi:10.1038/nature09413
Chen, J., Zhu, Y., Liang, C., Chen, J., and Zhao, H. B. (2015). Pannexin1 channels dominate ATP release in the cochlea ensuring endocochlear potential and auditory receptor potential generation and hearing. Sci. Rep. 5, 10762. doi:10.1038/srep10762
Chen, K. W., Demarco, B., and Broz, P. (2020). Pannexin-1 promotes NLRP3 activation during apoptosis but is dispensable for canonical or noncanonical inflammasome activation. Eur. J. Immunol. 50, 170–177. doi:10.1002/eji.201948254
Chen, W., Zhu, S., Wang, Y., Li, J., Qiang, X., Zhao, X., et al. (2019). Enhanced macrophage pannexin 1 expression and hemichannel activation exacerbates lethal experimental Sepsis. Sci. Rep. 9, 160. doi:10.1038/s41598-018-37232-z
Chiu, Y. H., Medina, C. B., Doyle, C. A., Zhou, M., Narahari, A. K., Sandilos, J. K., et al. (2021). Deacetylation as a receptor-regulated direct activation switch for pannexin channels. Nat. Commun. 12, 1–14. doi:10.1038/s41467-021-24825-y
Cooreman, A., Van Campenhout, R., Ballet, S., Annaert, P., Van Den Bossche, B., Colle, I., et al. (2019). Connexin and pannexin (hemi)channels: Emerging targets in the treatment of liver disease. Hepatology 69, 1317–1323. doi:10.1002/hep.30306
Cowan, K. N., Langlois, S., Penuela, S., Cowan, B. J., and Laird, D. W. (2012). Pannexin1 and pannexin3 exhibit distinct localization patterns in human skin appendages and are regulated during keratinocyte differentiation and carcinogenesis. Cell Commun. Adhes. 19, 45–53. doi:10.3109/15419061.2012.712575
Crespo Yanguas, S., Cogliati, B., Willebrords, J., Maes, M., Colle, I., Van Den Bossche, B., et al. (2016). Experimental models of liver fibrosis. Arch. Toxicol. 90, 1025–1048. doi:10.1007/s00204-015-1543-4
Crespo Yanguas, S., da Silva, T. C., Pereira, I. V. A., Maes, M., Willebrords, J., Shestopalov, V. I., et al. (2018). Genetic ablation of pannexin1 counteracts liver fibrosis in a chemical, but not in a surgical mouse model. Arch. Toxicol. 92, 2607–2627. doi:10.1007/s00204-018-2255-3
Crocetti, L., Giovannoni, M. P., Guerrini, G., Lamanna, S., Melani, F., Bartolucci, G., et al. (2023). Design, synthesis and pharmacological evaluation of new quinoline-based panx-1 channel blockers. Int. J. Mol. Sci. 24, 2022. doi:10.3390/ijms24032022
Crocetti, L., Guerrini, G., Giovannoni, M. P., Melani, F., Lamanna, S., Di Cesare, M. L., et al. (2022). New panx-1 blockers: Synthesis, biological evaluation and molecular dynamic studies. Int. J. Mol. Sci. 23, 4827. doi:10.3390/ijms23094827
Cui, H., Liu, Y., Qin, L., Wang, L., and Huang, Y. (2016). Increased membrane localization of pannexin1 in human corneal synaptosomes causes enhanced stimulated ATP release in chronic diabetes mellitus. Med. (United States) 95, e5084. doi:10.1097/MD.0000000000005084
DeLalio, L. J., Billaud, M., Ruddiman, C. A., Johnstone, S. R., Butcher, J. T., Wolpe, A. G., et al. (2019). Constitutive SRC-mediated phosphorylation of pannexin 1 at tyrosine 198 occurs at the plasma membrane. J. Biol. Chem. 294, 6940–6956. doi:10.1074/jbc.RA118.006982
Deng, Z., He, Z., Maksaev, G., Bitter, R. M., Rau, M., Fitzpatrick, J. A. J., et al. (2020). Cryo-EM structures of the ATP release channel pannexin 1. Nat. Struct. Mol. Biol. 27, 373–381. doi:10.1038/s41594-020-0401-0
Denton, D., and Kumar, S. (2019). Autophagy-dependent cell death. Cell Death Differ. 26, 605–616. doi:10.1038/s41418-018-0252-y
Derangère, V., Chevriaux, A., Courtaut, F., Bruchard, M., Berger, H., Chalmin, F., et al. (2014). Liver X receptor β activation induces pyroptosis of human and murine colon cancer cells. Cell Death Differ. 21, 1914–1924. doi:10.1038/cdd.2014.117
Diem, K., Fauler, M., Fois, G., Hellmann, A., Winokurow, N., Schumacher, S., et al. (2020). Mechanical stretch activates piezo1 in caveolae of alveolar type I cells to trigger ATP release and paracrine stimulation of surfactant secretion from alveolar type II cells. FASEB J. 34, 12785–12804. doi:10.1096/fj.202000613RRR
Diezmos, E. F., Sandow, S. L., Markus, I., Shevy Perera, D., Lubowski, D. Z., King, D. W., et al. (2013). Expression and localization of pannexin-1 hemichannels in human colon in health and disease. Neurogastroenterol. Motil. 25, 395–405. doi:10.1111/nmo.12130
Dossi, E., Blauwblomme, T., Moulard, J., Chever, O., Vasile, F., Guinard, E., et al. (2018). Pannexin-1 channels contribute to seizure generation in human epileptic brain tissue and in a mouse model of epilepsy. Sci. Transl. Med. 10, eaar3796. doi:10.1126/scitranslmed.aar3796
Dufresne, J., and Cyr, D. G. (2014). Regulation of the pannexin-1 promoter in the rat epididymis. Biol. Reprod. 91, 1–13. doi:10.1095/biolreprod.114.122168
El-Khalik, S. R. A., Ibrahim, R. R., Ghafar, M. T. A., Shatat, D., and El-Deeb, O. S. (2022). Novel insights into the slc7a11-mediated ferroptosis signaling pathways in preeclampsia patients: Identifying pannexin 1 and toll-like receptor 4 as innovative prospective diagnostic biomarkers. J. Assist. Reprod. Genet. 39, 1115–1124. doi:10.1007/s10815-022-02443-x
Feig, J. L., Mediero, A., Corciulo, C., Liu, H., Zhang, J., Perez-Aso, M., et al. (2017). The antiviral drug tenofovir, an inhibitor of Pannexin-1-mediated ATP release, prevents liver and skin fibrosis by downregulating adenosine levels in the liver and skin. PLoS One 12, 1–22. doi:10.1371/journal.pone.0188135
Filiberto, A. C., Ladd, Z., Leroy, V., Su, G., Elder, C. T., Pruitt, E. Y., et al. (2022). Resolution of inflammation via RvD1/FPR2 signaling mitigates Nox2 activation and ferroptosis of macrophages in experimental abdominal aortic aneurysms. FASEB J. 36, e22579. doi:10.1096/fj.202201114R
Fontainhas, A. M., Wang, M., Liang, K. J., Chen, S., Mettu, P., Damani, M., et al. (2011). Microglial morphology and dynamic behavior is regulated by ionotropic glutamatergic and GABAergic neurotransmission. PLoS One 6, e15973. doi:10.1371/journal.pone.0015973
Fontana, R. J. (2008). Acute liver failure including acetaminophen overdose. Med. Clin. North Am. 92, 761–794. doi:10.1016/j.mcna.2008.03.005
Frissen, M., Liao, L., Schneider, K. M., Djudjaj, S., Haybaeck, J., Wree, A., et al. (2021). Bidirectional role of NLRP3 during acute and chronic cholestatic liver injury. Hepatology 73, 1836–1854. doi:10.1002/hep.31494
Gallego, P., Castejón-Vega, B., Del Campo, J. A., and Cordero, M. D. (2020). The absence of NLRP3-inflammasome modulates hepatic fibrosis progression, lipid metabolism, and inflammation in KO NLRP3 mice during aging. Cells 9, 2148. doi:10.3390/cells9102148
Gehi, R., Shao, Q., and Laird, D. W. (2011). Pathways regulating the trafficking and turnover of Pannexin1 protein and the role of the C-terminal domain. J. Biol. Chem. 286, 27639–27653. doi:10.1074/jbc.M111.260711
Gong, Z., Zhou, J., Zhao, S., Tian, C., Wang, P., Xu, C., et al. (2016). Chenodeoxycholic acid activates NLRP3 inflammasome and contributes to cholestatic liver fibrosis. Oncotarget 7, 83951–83963. doi:10.18632/oncotarget.13796
Gritsenko, A., Green, J. P., Brough, D., and Lopez-Castejon, G. (2020). Mechanisms of NLRP3 priming in inflammaging and age related diseases. Cytokine Growth Factor Rev. 55, 15–25. doi:10.1016/j.cytogfr.2020.08.003
Guo, S., Yang, C., Diao, B., Huang, X., Jin, M., Chen, L., et al. (2015). The NLRP3 inflammasome and IL-1β accelerate immunologically mediated pathology in experimental viral fulminant hepatitis. PLoS Pathog. 11, e1005155. doi:10.1371/journal.ppat.1005155
Hirao, H., Nakamura, K., and Kupiec-Weglinski, J. W. (2022). Liver ischaemia–reperfusion injury: A new understanding of the role of innate immunity. Nat. Rev. Gastroenterol. Hepatol. 19, 239–256. doi:10.1038/s41575-021-00549-8
Huang, G., Bao, J., Shao, X., Zhou, W., Wu, B., Ni, Z., et al. (2020). Inhibiting pannexin-1 alleviates sepsis-induced acute kidney injury via decreasing NLRP3 inflammasome activation and cell apoptosis. Life Sci. 254, 117791. doi:10.1016/j.lfs.2020.117791
Huang, Y., Lin, J., Chen, X., and Lin, J. (2021). Pannexin-1 contributes to the apoptosis of spinal neurocytes in spinal cord injury. Front. Physiol. 12, 656647–656648. doi:10.3389/fphys.2021.656647
Imamura, H., Sakamoto, S., Yoshida, T., Matsui, Y., Penuela, S., Laird, D. W., et al. (2020). Single-cell dynamics of pannexin-1-facilitated programmed ATP loss during apoptosis. Elife 9, 1–20. doi:10.7554/eLife.61960
Jaeschke, H., Akakpo, J. Y., Umbaugh, D. S., and Ramachandran, A. (2020). Novel therapeutic approaches against acetaminophen-induced liver injury and acute liver failure. Toxicol. Sci. 174, 159–167. doi:10.1093/toxsci/kfaa002
Jalaleddine, N., El-Hajjar, L., Dakik, H., Shaito, A., Saliba, J., Safi, R., et al. (2019). Pannexin1 is associated with enhanced epithelial-to-mesenchymal transition in human patient breast cancer tissues and in breast cancer cell lines. Cancers (Basel). 11, 11121967. doi:10.3390/cancers11121967
Jeličić, I., Vukojević, K., Racetin, A., Čarić, D., Durdov, M. G., Saraga-Babić, M., et al. (2022). Expression of pannexin 1 in the human kidney during embryonal, early fetal and postnatal development and its prognostic significance in diabetic nephropathy. Biomedicines 10, 944. doi:10.3390/biomedicines10050944
Jiang, X., Stockwell, B. R., and Conrad, M. (2021). Ferroptosis: Mechanisms, biology and role in disease. Nat. Rev. Mol. Cell Biol. 22, 266–282. doi:10.1038/s41580-020-00324-8
Jung, S., Jeong, H., and Yu, S. W. (2020). Autophagy as a decisive process for cell death. Exp. Mol. Med. 52, 921–930. doi:10.1038/s12276-020-0455-4
Kanneganti, T. D., Lamkanfi, M., Kim, Y. G., Chen, G., Park, J. H., Franchi, L., et al. (2007). Pannexin-1-mediated recognition of bacterial molecules activates the cryopyrin inflammasome independent of Toll-like receptor signaling. Immunity 26, 433–443. doi:10.1016/j.immuni.2007.03.008
Kelley, N., Jeltema, D., Duan, Y., and He, Y. (2019). The NLRP3 inflammasome: An overview of mechanisms of activation and regulation. Int. J. Mol. Sci. 20, 3328. doi:10.3390/ijms20133328
Kim, H. Y., Kim, S. J., and Lee, S. M. (2015). Activation of NLRP3 and AIM2 inflammasomes in Kupffer cells in hepatic ischemia/reperfusion. FEBS J. 282, 259–270. doi:10.1111/febs.13123
Kim, O. K., Nam, D., and Hahn, Y. S. (2021). The pannexin 1/purinergic receptor P2X4 pathway controls the secretion of microRNA-containing exosomes by HCV-infected hepatocytes. Hepatology 74, 3409–3426. doi:10.1002/hep.32042
Kirby, B. S., Sparks, M. A., Lazarowski, E. R., Lopez Domowicz, D. A., Zhu, H., and McMahon, T. J. (2021). Pannexin 1 channels control the hemodynamic response to hypoxia by regulating O2-sensitive extracellular ATP in blood. Am. J. Physiol. - Hear. Circ. Physiol. 320, 1055–1065. doi:10.1152/AJPHEART.00651.2020
Koval, M., Schug, W. J., and Isakson, B. E. (2023). Pharmacology of pannexin channels. Curr. Opin. Pharmacol. 69, 102359. doi:10.1016/j.coph.2023.102359
Kranz, K., Dorgau, B., Pottek, M., Herrling, R., Schultz, K., Bolte, P., et al. (2013). Expression of Pannexin1 in the outer plexiform layer of the mouse retina and physiological impact of its knockout. J. Comp. Neurol. 521, 1119–1135. doi:10.1002/cne.23223
Kurtenbach, S., Kurtenbach, S., and Zoidl, G. (2014). Emerging functions of pannexin 1 in the eye. Front. Cell. Neurosci. 8, 263. doi:10.3389/fncel.2014.00263
Laird, D. W., and Penuela, S. (2021). Pannexin biology and emerging linkages to cancer. Trends Cancer 7, 1119–1131. doi:10.1016/j.trecan.2021.07.002
Lee, S., Kwak, J.-H., Kim, S. H., Jeong, T. B., Son, S. W., Kim, J.-H., et al. (2019). Comparative study of liver injury induced by high-fat methionine- and choline-deficient diet in ICR mice originating from three different sources. Lab. Anim. Res. 35, 15. doi:10.1186/s42826-019-0016-y
Lee, S. T., Chang, Y., and Venton, B. J. (2022). Pannexin1 channels regulate mechanically stimulated but not spontaneous adenosine release. Anal. Bioanal. Chem. 414, 3781–3789. doi:10.1007/s00216-022-04047-x
Lei, Y., Zhou, R., Sun, X., Tang, F., Gao, H., Chen, L., et al. (2021). The pannexin-1 channel regulates pyroptosis through autophagy in a mouse model of sepsis-associated encephalopathy. Ann. Transl. Med. 9, 1802. doi:10.21037/atm-21-6579
Lemaire, I., Falzoni, S., Zhang, B., Pellegatti, P., and Di Virgilio, F. (2011). The P2X7 receptor and pannexin-1 are both required for the promotion of multinucleated macrophages by the inflammatory cytokine GM-CSF. J. Immunol. 187, 3878–3887. doi:10.4049/jimmunol.1002780
Leroy, K., Vilas-Boas, V., Gijbels, E., Vanderborght, B., Devisscher, L., Cogliati, B., et al. (2022). Expression of connexins and pannexins in diseased human liver. EXCLI J. 21, 1111–1129. doi:10.17179/excli2022-5163
Li, H., Jiang, W., Zhang, S.-R., Li, P.-C., Li, T.-J., Jin, W., et al. (2023). The platelet pannexin 1-IL-1β axis orchestrates pancreatic ductal adenocarcinoma invasion and metastasis. Oncogene 15, 1453–1465. doi:10.1038/s41388-023-02647-0
Li, H., Yu, X., Shi, B., Zhang, K., Yuan, L., Liu, X., et al. (2021). Reduced pannexin 1-IL-33 axis function in donor livers increases risk of MRSA infection in liver transplant recipients. Sci. Transl. Med. 13, eaaz6169–15. doi:10.1126/scitranslmed.aaz6169
Li, J., Cao, F., Yin, H., Huang, Z., Lin, Z., Mao, N., et al. (2020). Ferroptosis: Past, present and future. Cell Death Dis. 11, 88. doi:10.1038/s41419-020-2298-2
Li, S., Tomić, M., and Stojilkovic, S. S. (2011). Characterization of novel Pannexin 1 isoforms from rat pituitary cells and their association with ATP-gated P2X channels. Gen. Comp. Endocrinol. 174, 202–210. doi:10.1016/j.ygcen.2011.08.019
Lohman, A. W., Leskov, I. L., Butcher, J. T., Johnstone, S. R., Stokes, T. A., Begandt, D., et al. (2015). Pannexin 1 channels regulate leukocyte emigration through the venous endothelium during acute inflammation. Nat. Commun. 6, 7965. doi:10.1038/ncomms8965
Lohman, A. W., Weilinger, N. L., Santos, S. M., Bialecki, J., Werner, A. C., Anderson, C. L., et al. (2019). Regulation of pannexin channels in the central nervous system by Src family kinases. Neurosci. Lett. 16, 65–70. doi:10.1016/j.neulet.2017.09.019
López, X., Escamilla, R., Fernández, P., Duarte, Y., González-Nilo, F., Palacios-Prado, N., et al. (2020). Stretch-induced activation of pannexin 1 channels can be prevented by PKA-dependent phosphorylation. Int. J. Mol. Sci. 21, 9180. doi:10.3390/ijms21239180
Lu, L., Zhou, H., Ni, M., Wang, X., Busuttil, R., Kupiec-Weglinski, J., et al. (2016). Innate immune regulations and liver ischemia-reperfusion injury. Transplantation 100, 2601–2610. doi:10.1097/TP.0000000000001411
Luo, W., Feldman, D., McCallister, R., Brophy, C., and Cheung-Flynn, J. (2017). P2X7R antagonism after subfailure overstretch injury of blood vessels reverses vasomotor dysfunction and prevents apoptosis. Purinergic Signal 13, 579–590. doi:10.1007/s11302-017-9585-0
Luu, R., Valdebenito, S., Scemes, E., Cibelli, A., Spray, D. C., Rovegno, M., et al. (2021). Pannexin-1 channel opening is critical for COVID-19 pathogenesis. iScience 24, 103478. doi:10.1016/j.isci.2021.103478
Maes, M., Mcgill, M. R., Cristina, T., Abels, C., Weemhoff, J. L., Tiburcio, T., et al. (2017). Inhibition of pannexin1 channels alleviates acetaminophen-induced hepatotoxicity. Arch. Toxicol. 91, 2245–2261. doi:10.1007/s00204-016-1885-6
Maslieieva, V., and Thompson, R. J. (2014). A critical role for pannexin-1 in activation of innate immune cells of the choroid plexus. Channels 8, 131–141. doi:10.4161/chan.27653
Matthews, D. R., Li, H., Zhou, J., Li, Q., Glaser, S., Francis, H., et al. (2021). Methionine- and choline-deficient diet–induced nonalcoholic steatohepatitis is associated with increased intestinal inflammation. Am. J. Pathol. 191, 1743–1753. doi:10.1016/j.ajpath.2021.06.010
Medina, C. B., Mehrotra, P., Arandjelovic, S., Perry, J. S. A., Guo, Y., Morioka, S., et al. (2020). Metabolites released from apoptotic cells act as tissue messengers. Nature 580, 130–135. doi:10.1038/s41586-020-2121-3
Metz, L. M., Feige, T., de Biasi, L., Ehrenberg, A., Mulorz, J., Toska, L. M., et al. (2023). Platelet pannexin-1 channels modulate neutrophil activation and migration but not the progression of abdominal aortic aneurysm. Front. Mol. Biosci. 10, 1111108. doi:10.3389/fmolb.2023.1111108
Mukai, H., Miki, N., Yamada, H., Goto, H., Kawakami, T., Suzuki, A., et al. (2022). Pannexin1 channel-dependent secretome from apoptotic tumor cells shapes immune-escape microenvironment. Biochem. Biophys. Res. Commun. 628, 116–122. doi:10.1016/j.bbrc.2022.08.062
Musavi, H., Safaee, M. S., Nasiri, Z., Ghorbani, F., and Mohamadi, P. (2022). Evaluating the effect of silibinin on the expression of pannexin1 gene during hepatic ischemia-reperfusion. x. doi:10.34172/cjmb.2022.xxx
Narahari, A. K., Kreutzberger, A. J. B., Gaete, P. S., Chiu, Y. H., Leonhardt, S. A., Medina, C. B., et al. (2021). ATP and large signaling metabolites flux through caspase-activated pannexin 1 channels. Elife 10, 1–21. doi:10.7554/ELIFE.64787
Negoro, H., Lutz, S. E., Liou, L. S., Kanematsu, A., Ogawa, O., Scemes, E., et al. (2013). Pannexin 1 involvement in bladder dysfunction in a multiple sclerosis model. Sci. Rep. 3, 2152. doi:10.1038/srep02152
Parzych, K., Zetterqvist, A. V., Wright, W. R., Kirkby, N. S., Mitchell, J. A., and Paul-Clark, M. J. (2017). Differential role of pannexin-1/ATP/P2X7 axis in IL-1β release by human monocytes. FASEB J. 31, 2439–2445. doi:10.1096/fj.201600256
Paul, D., Sanap, G., Shenoy, S., Kalyane, D., Kalia, K., and Tekade, R. K. (2021). Artificial intelligence in drug discovery and development. Drug Discov. Today 26, 80–93. doi:10.1016/j.drudis.2020.10.010
Pelegrin, P., and Surprenant, A. (2006). Pannexin-1 mediates large pore formation and interleukin-1beta release by the ATP-gated P2X7 receptor. EMBO J. 25, 5071–5082. doi:10.1038/sj.emboj.7601378
Penuela, S., Bhalla, R., Gong, X. Q., Cowan, K. N., Celetti, S. J., Cowan, B. J., et al. (2007). Pannexin 1 and pannexin 3 are glycoproteins that exhibit many distinct characteristics from the connexin family of gap junction proteins. J. Cell Sci. 120, 3772–3783. doi:10.1242/jcs.009514
Penuela, S., Gehi, R., and Laird, D. W. (2013). The biochemistry and function of pannexin channels. Biochim. Biophys. Acta - Biomembr. 1828, 15–22. doi:10.1016/j.bbamem.2012.01.017
Poon, I. K. H., Chiu, Y. H., Armstrong, A. J., Kinchen, J. M., Juncadella, I. J., Bayliss, D. A., et al. (2014). Unexpected link between an antibiotic, pannexin channels and apoptosis. Nature 507, 329–334. doi:10.1038/nature13147
Pouwels, S., Sakran, N., Graham, Y., Leal, A., Pintar, T., Yang, W., et al. (2022). Non-alcoholic fatty liver disease (NAFLD): A review of pathophysiology, clinical management and effects of weight loss. BMC Endocr. Disord. 22, 63. doi:10.1186/s12902-022-00980-1
Prochnow, N., Abdulazim, A., Kurtenbach, S., Wildförster, V., Dvoriantchikova, G., Hanske, J., et al. (2012). Pannexin1 stabilizes synaptic plasticity and is needed for learning. PLoS. One 7, e51767. doi:10.1371/journal.pone.0051767
Qiu, F., and Dahl, G. (2009). A permeant regulating its permeation pore: Inhibition of pannexin 1 channels by ATP. Am. J. Physiol. - Cell Physiol. 296, 250–255. doi:10.1152/ajpcell.00433.2008
Qu, J., Yuan, Z., Wang, G., Wang, X., and Li, K. (2019). The selective NLRP3 inflammasome inhibitor MCC950 alleviates cholestatic liver injury and fibrosis in mice. Int. Immunopharmacol. 70, 147–155. doi:10.1016/j.intimp.2019.02.016
Qu, R., Dong, L., Zhang, J., Yu, X., Wang, L., and Zhu, S. (2020). Cryo-EM structure of human heptameric Pannexin 1 channel. Cell Res. 30, 446–448. doi:10.1038/s41422-020-0298-5
Qu, Y., Misaghi, S., Newton, K., Gilmour, L. L., Louie, S., Cupp, J. E., et al. (2011). Pannexin-1 is required for ATP release during apoptosis but not for inflammasome activation. J. Immunol. 186, 6553–6561. doi:10.4049/jimmunol.1100478
Ransford, G. A., Fregien, N., Qiu, F., Dahl, G., Conner, G. E., and Salathe, M. (2009). Pannexin 1 contributes to ATP release in airway epithelia. Am. J. Respir. Cell Mol. Biol. 41, 525–534. doi:10.1165/rcmb.2008-0367OC
Riazi, K., Azhari, H., Charette, J. H., Underwood, F. E., King, J. A., Afshar, E. E., et al. (2022). The prevalence and incidence of NAFLD worldwide: A systematic review and meta-analysis. Lancet Gastroenterol. Hepatol. 7, 851–861. doi:10.1016/S2468-1253(22)00165-0
Riquelme, M. A., Cea, L. A., Vega, J. L., Boric, M. P., Monyer, H., Bennett, M. V., et al. (2013). The ATP required for potentiation of skeletal muscle contraction is released via pannexin hemichannels. Neuropharmacology 75, 594–603. doi:10.1016/j.neuropharm.2013.03.022
Ritchie, H., Spooner, F., and Rose, M. (2018). Causes of death. Our World Data. Available at: https://ourworldindata.org/causes-of-death#citation.
Rougé, S., Genetet, S., Leal Denis, M. F., Dussiot, M., Schwarzbaum, P. J., Ostuni, M. A., et al. (2022). Mechanosensitive pannexin 1 activity is modulated by stomatin in human red blood cells. Int. J. Mol. Sci. 23, 9401. doi:10.3390/ijms23169401
Sandilos, J. K., Chiu, Y. H., Chekeni, F. B., Armstrong, A. J., Walk, S. F., Ravichandran, K. S., et al. (2012). Pannexin 1, an ATP release channel, is activated by caspase cleavage of its pore-associated C-terminal autoinhibitory region. J. Biol. Chem. 287, 11303–11311. doi:10.1074/jbc.M111.323378
Schenk, U., Westendorf, A. M., Radaelli, E., Casati, A., Ferro, M., Fumagalli, M., et al. (2008). Purinergic control of T cell activation by ATP released through pannexin-1 hemichannels. Sci. Signal. 1, ra6–14. doi:10.1126/scisignal.1160583
Seminario-Vidal, L., Okada, S. F., Sesma, J. I., Kreda, S. M., Van Heusden, C. A., Zhu, Y., et al. (2011). Rho signaling regulates pannexin 1-mediated ATP release from airway epithelia. J. Biol. Chem. 286, 26277–26286. doi:10.1074/jbc.M111.260562
Shao, B. Z., Xu, Z. Q., Han, B. Z., Su, D. F., and Liu, C. (2015). NLRP3 inflammasome and its inhibitors: A review. Front. Pharmacol. 6, 262. doi:10.3389/fphar.2015.00262
Shao, Q., Lindstrom, K., Shi, R., Kelly, J., Schroeder, A., Juusola, J., et al. (2016). A germline variant in the PANX1 gene has reduced channel function and is associated with multisystem dysfunction. J. Biol. Chem. 291, 12432–12443. doi:10.1074/jbc.M116.717934
Silverman, W. R., de Rivero Vaccari, J. P., Locovei, S., Qiu, F., Carlsson, S. K., Scemes, E., et al. (2009). The pannexin 1 channel activates the inflammasome in neurons and astrocytes. J. Biol. Chem. 284, 18143–18151. doi:10.1074/jbc.M109.004804
Su, L., Jiang, X., Yang, C., Zhang, J., Chen, B., Li, Y., et al. (2019). Pannexin 1 mediates ferroptosis that contributes to renal ischemia/reperfusion injury. J. Biol. Chem. 294, 19395–19404. doi:10.1074/jbc.RA119.010949
Suadicani, S. O., Iglesias, R., Wang, J., Dahl, G., Spray, D. C., and Scemes, E. (2012). ATP signaling is deficient in cultured pannexin1-null mouse astrocytes. Glia 60, 1106–1116. doi:10.1002/glia.22338
Suarez-Berumen, K., Collins-Hooper, H., Gromova, A., Meech, R., Sacco, A., Dash, P. R., et al. (2021). Pannexin 1 regulates skeletal muscle regeneration by promoting bleb-based myoblast migration and fusion through a novel lipid based signaling mechanism. Front. Cell Dev. Biol. 9, 736813. doi:10.3389/fcell.2021.736813
Südkamp, N., Shchyglo, O., and Manahan-Vaughan, D. (2021). Absence of pannexin 1 stabilizes hippocampal excitability after intracerebral treatment with Aβ (1-42) and prevents LTP deficits in middle-aged mice. Front. Aging Neurosci. 13, 591735. doi:10.3389/fnagi.2021.591735
Tozzi, M., Hansen, J. B., and Novak, I. (2020). Pannexin-1 mediated ATP release in adipocytes is sensitive to glucose and insulin and modulates lipolysis and macrophage migration. Acta Physiol. 228, e13360. doi:10.1111/apha.13360
Unterberger, S., Davies, K. A., Rambhatla, S. B., and Sacre, S. (2021). Contribution of Toll-like receptors and the NLRP3 inflammasome in rheumatoid arthritis pathophysiology. Immunotargets Ther. 10, 285–298. doi:10.2147/itt.s288547
Van Campenhout, R., Muyldermans, S., Vinken, M., Devoogdt, N., and De Groof, T. W. M. (2021). Therapeutic nanobodies targeting cell plasma membrane transport proteins: A high-risk/high-gain endeavor. Biomolecules 11, 63. doi:10.3390/biom11010063
Van Heusden, C., Grubb, B., Button, B., and Lazarowski, E. (2021). Airway epithelial nucleotide release contributes to mucociliary clearance. Life 11, 430. doi:10.3390/life11050430
Vanden Abeele, F., Bidaux, G., Gordienko, D., Beck, B., Panchin, Y. V., Baranova, A. V., et al. (2006). Functional implications of calcium permeability of the channel formed by pannexin 1. J. Cell Biol. 174, 535–546. doi:10.1083/jcb.200601115
Vinken, M. (2020). A putative AOP for pneumonia related to COVID-19. Arch. Toxicol. 94, 3343–3345. doi:10.1007/s00204-020-02860-w
Vinken, M. (2022). Toxic talk: pannexin1 channel communication as an emerging mechanism of toxicity. Toxicology 478, 153295. doi:10.1016/j.tox.2022.153295
Wang, H., Xing, Y., Mao, L., Luo, Y., Kang, L., and Meng, G. (2013). Pannexin-1 influences peritoneal cavity cell population but is not involved in NLRP3 inflammasome activation. Protein Cell 4, 259–265. doi:10.1007/s13238-013-2114-1
Wang, L., and Hauenstein, A. V. (2020). The NLRP3 inflammasome: Mechanism of action, role in disease and therapies. Mol. Asp. Med. 76, 100889. doi:10.1016/j.mam.2020.100889
Wang, P., Shi, B., Wang, C., Wang, Y., Que, W., Jiang, Z., et al. (2022). Hepatic pannexin-1 mediates ST2 + regulatory T cells promoting resolution of inflammation in lipopolysaccharide-induced endotoxemia. Clin. Transl. Med. 12, e849. doi:10.1002/ctm2.849
Watanabe, A., Sohail, M. A., Gomes, D. A., Hashmi, A., Nagata, J., Sutterwala, F. S., et al. (2009). Inflammasome-mediated regulation of hepatic stellate cells. Am. J. Physiol. - Gastrointest. Liver Physiol. 296, 1248–1257. doi:10.1152/ajpgi.90223.2008
Wei, L., Yang, X., Shi, X., and Chen, Y. (2015). Pannexin-1 silencing inhibits the proliferation of U87-MG cells. Mol. Med. Rep. 11, 3487–3492. doi:10.3892/mmr.2015.3169
Weiler, N., Schlotmann, A., Schnitzbauer, A. A., Zeuzem, S., and Welker, M. W. (2020). The epidemiology of acute liver failure: Results of a population-based study including 25 million state-insured individuals. Dtsch. Arztebl. Int. 117, 43–50. doi:10.3238/arztebl.2020.0043
Weilinger, N. L., Lohman, A. W., Rakai, B. D., Ma, E. M., Bialecki, J., Maslieieva, V., et al. (2016). Metabotropic NMDA receptor signaling couples Src family kinases to pannexin-1 during excitotoxicity. Nat. Neurosci. 19, 432–442. doi:10.1038/nn.4236
Weilinger, N. L., Tang, P. L., and Thompson, R. J. (2012). Anoxia-induced NMDA receptor activation opens pannexin channels via Src family kinases. J. Neurosci. 32, 12579–12588. doi:10.1523/jneurosci.1267-12.2012
Willebrords, J., Maes, M., Crespo Yanguas, S., and Vinken, M. (2017). Inhibitors of connexin and pannexin channels as potential therapeutics. Pharmacol. Ther. 180, 144–160. doi:10.1016/j.pharmthera.2017.07.001
Willebrords, J., Maes, M., Pereira, I. V. A., da Silva, T. C., Govoni, V. M., Lopes, V. V., et al. (2018). Protective effect of genetic deletion of pannexin1 in experimental mouse models of acute and chronic liver disease. Biochim. Biophys. Acta - Mol. Basis Dis. 1864, 819–830. doi:10.1016/j.bbadis.2017.12.013
Willebrords, J., Pereira, I. V. A., Maes, M., Crespo Yanguas, S., Colle, I., Van Den Bossche, B., et al. (2015). Strategies, models and biomarkers in experimental non-alcoholic fatty liver disease research. Prog. Lipid Res. 59, 106–125. doi:10.1016/j.plipres.2015.05.002
Woehrle, T., Yip, L., Elkhal, A., Sumi, Y., Chen, Y., Yao, Y., et al. (2010). Pannexin-1 hemichannel-mediated ATP release together with P2X1 and P2X4 receptors regulate T-cell activation at the immune synapse. Blood 116, 3475–3484. doi:10.1182/blood-2010-04-277707
Wu, D., Wu, J., Liu, H., Yu, M., Tao, L., Dong, S., et al. (2017a). Role of Pannexin1 channels in the resistance of I-10 testicular cancer cells to cisplatin mediated by ATP/IP3 pathway. Biomed. Pharmacother. 94, 514–522. doi:10.1016/j.biopha.2017.07.144
Wu, L. Y., Ye, Z. N., Zhou, C. H., Wang, C. X., Xie, G. Bin, Zhang, X. S., et al. (2017b). Roles of pannexin-1 channels in inflammatory response through the TLRs/NF-Kappa B signaling pathway following experimental subarachnoid hemorrhage in rats. Front. Mol. Neurosci. 10, 1–15. doi:10.3389/fnmol.2017.00175
Xiao, F., Waldrop, S. L., Bronk, S. F., Gores, G. J., Davis, L. S., and Kilic, G. (2015). Lipoapoptosis induced by saturated free fatty acids stimulates monocyte migration: A novel role for Pannexin1 in liver cells. Purinergic Signal 11, 347–359. doi:10.1007/s11302-015-9456-5
Xiao, F., Waldrop, S. L., Khimji, A., and Kilic, G. (2012). Pannexin1 contributes to pathophysiological ATP release in lipoapoptosis induced by saturated free fatty acids in liver cells. Am. J. Physiol. Physiol. 303, C1034–C1044. doi:10.1152/ajpcell.00175.2012
Yang, D., He, Y., Muñoz-Planillo, R., Liu, Q., and Núñez, G. (2015). Caspase-11 requires the pannexin-1 channel and the purinergic P2X7 pore to mediate pyroptosis and endotoxic Shock. Immunity 43, 923–932. doi:10.1016/j.immuni.2015.10.009
Yang, W. S., and Stockwell, B. R. (2016). Ferroptosis: Death by lipid peroxidation. Trends Cell Biol. 26, 165–176. doi:10.1016/j.tcb.2015.10.014
Yin, F., Zheng, P., Zhao, L., Wang, Y., Miao, N., Zhou, Z., et al. (2022). Caspase-11 promotes NLRP3 inflammasome activation via the cleavage of pannexin1 in acute kidney disease. Acta Pharmacol. Sin. 43, 86–95. doi:10.1038/s41401-021-00619-2
Ying, W., Zheng, K., Wu, Y., and Wang, O. (2021). Pannexin 1 mediates gastric cancer cell epithelial–mesenchymal transition via aquaporin 5. Biol. Pharm. Bull. 44, 1111–1119. doi:10.1248/bpb.b21-00292
Younossi, Z. M., Blissett, D., Blissett, R., Henry, L., Stepanova, M., Younossi, Y., et al. (2016). The economic and clinical burden of nonalcoholic fatty liver disease in the United States and Europe. Hepatology 64, 1577–1586. doi:10.1002/hep.28785
Yu, P., Zhang, X., Liu, N., Tang, L., Peng, C., and Chen, X. (2021). Pyroptosis: Mechanisms and diseases. Signal Transduct. Target. Ther. 6, 128. doi:10.1038/s41392-021-00507-5
Yuan, M., Yao, Y., Wu, D., Zhu, C., Dong, S., and Tong, X. (2022). Pannexin1 inhibits autophagy of cisplatin-resistant testicular cancer cells by mediating ATP release. Cell Cycle 21, 1651–1661. doi:10.1080/15384101.2022.2060655
Zhai, Y., Petrowsky, H., Hong, J. C., Busuttil, R. W., and Kupiec-Weglinski, J. W. (2013). Ischaemia-reperfusion injury in liver transplantation-from bench to bedside. Nat. Rev. Gastroenterol. Hepatol. 10, 79–89. doi:10.1038/nrgastro.2012.225
Zhang, A., Yang, J., Ma, C., Li, F., and Luo, H. (2021a). Development and validation of a robust ferroptosis-related prognostic signature in lung adenocarcinoma. Front. Cell Dev. Biol. 9, 616271–616321. doi:10.3389/fcell.2021.616271
Zhang, S., Yuan, B., Lam, J. H., Zhou, J., Zhou, X., Ramos-Mandujano, G., et al. (2021b). Structure of the full-length human pannexin1 channel and insights into its role in pyroptosis. Cell Discov. 7, 30. doi:10.1038/s41421-021-00259-0
Zhang, W. J., Fang, Z. M., and Liu, W. Q. (2019). NLRP3 inflammasome activation from Kupffer cells is involved in liver fibrosis of Schistosoma japonicum-infected mice via NF-κB. Parasites Vectors 12, 29–18. doi:10.1186/s13071-018-3223-8
Zhang, Y., Laumet, G., Chen, S. R., Hittelman, W. N., and Pan, H. L. (2015). Pannexin-1 up-regulation in the dorsal root ganglion contributes to neuropathic pain development. J. Biol. Chem. 290, 14647–14655. doi:10.1074/jbc.M115.650218
Zheng, D., Liwinski, T., and Elinav, E. (2020). Inflammasome activation and regulation: Toward a better understanding of complex mechanisms. Cell Discov. 6, 36. doi:10.1038/s41421-020-0167-x
Zheng, Y., Tang, W., Zeng, H., Peng, Y., Yu, X., Yan, F., et al. (2022). Probenecid-blocked pannexin-1 channel protects against early brain injury via inhibiting neuronal AIM2 inflammasome activation after subarachnoid hemorrhage. Front. Neurol. 13, 854671. doi:10.3389/fneur.2022.854671
Zhou, B., Ryder, C. B., Dubyak, G. R., and Abbott, D. W. (2022). Gasdermins and pannexin-1 mediate pathways of chemotherapy-induced cell lysis in hematopoietic malignancies. Sci. Signal. 15, eabl6781. doi:10.1126/scisignal.abl6781
Keywords: pannexin1, physiology, pathology, inflammation, cell death, liver disease
Citation: Van Campenhout R, Caufriez A, Tabernilla A, Maerten A, De Boever S, Sanz-Serrano J, Kadam P and Vinken M (2023) Pannexin1 channels in the liver: an open enemy. Front. Cell Dev. Biol. 11:1220405. doi: 10.3389/fcell.2023.1220405
Received: 10 May 2023; Accepted: 23 June 2023;
Published: 10 July 2023.
Edited by:
Catherine Wright, Glasgow Caledonian University, United KingdomCopyright © 2023 Van Campenhout, Caufriez, Tabernilla, Maerten, De Boever, Sanz-Serrano, Kadam and Vinken. This is an open-access article distributed under the terms of the Creative Commons Attribution License (CC BY). The use, distribution or reproduction in other forums is permitted, provided the original author(s) and the copyright owner(s) are credited and that the original publication in this journal is cited, in accordance with accepted academic practice. No use, distribution or reproduction is permitted which does not comply with these terms.
*Correspondence: Mathieu Vinken, bWF0aGlldS52aW5rZW5AdnViLmJl
†These authors share last authorship
Disclaimer: All claims expressed in this article are solely those of the authors and do not necessarily represent those of their affiliated organizations, or those of the publisher, the editors and the reviewers. Any product that may be evaluated in this article or claim that may be made by its manufacturer is not guaranteed or endorsed by the publisher.
Research integrity at Frontiers
Learn more about the work of our research integrity team to safeguard the quality of each article we publish.