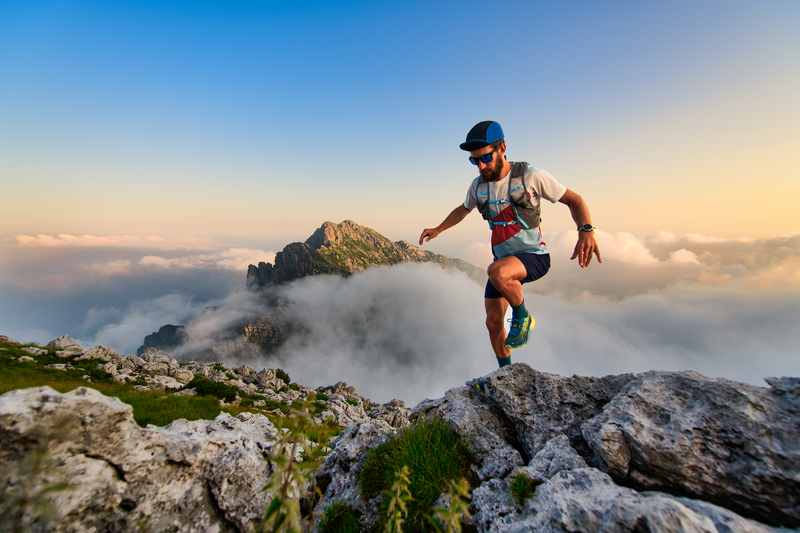
95% of researchers rate our articles as excellent or good
Learn more about the work of our research integrity team to safeguard the quality of each article we publish.
Find out more
REVIEW article
Front. Cell Dev. Biol. , 27 July 2023
Sec. Cell Death and Survival
Volume 11 - 2023 | https://doi.org/10.3389/fcell.2023.1219840
Nucleus pulposus, annulus fibrosus, and cartilage endplate constitute an avascular intervertebral disc (IVD), which is crucial for spinal and intervertebral joint mobility. As one of the most widespread health issues worldwide, intervertebral disc degeneration (IVDD) is recognized as a key contributor to back and neck discomfort. A number of degenerative disorders have a strong correlation with ferroptosis, a recently identified novel regulated cell death (RCD) characterized by an iron-dependent mechanism and a buildup of lipid reactive oxygen species (ROS). There is growing interest in the part ferroptosis plays in IVDD pathophysiology. Inhibiting ferroptosis has been shown to control IVDD development. Several studies have demonstrated that in TBHP-induced oxidative stress models, changes in ferroptosis marker protein levels and increased lipid peroxidation lead to the degeneration of intervertebral disc cells, which subsequently aggravates IVDD. Similarly, IVDD is significantly relieved with the use of ferroptosis inhibitors. The purpose of this review was threefold: 1) to discuss the occurrence of ferroptosis in IVDD; 2) to understand the mechanism of ferroptosis and its role in IVDD pathophysiology; and 3) to investigate the feasibility and prospect of ferroptosis in IVDD treatment.
Low back pain (LBP) is the primary global cause of absenteeism from work and disability affecting a total of 637 million people (Vos et al., 2017; GBD 2016 Disease and Injury Incidence and Prevalence Collaborators, 2017; James et al., 2018; GBD 2017 Disease and Injury Incidence and Prevalence Collaborators, 2018) In the United States, annual spending on managing patients with LBP is estimated to exceed $100 billion (Knezevic et al., 2021). Research has shown that intervertebral disc degeneration (IVDD) is a key factor leading to LBP (Francisco et al., 2022). However, current IVDD treatment can only relieve patient’s symptoms through drugs or surgery. Conservative treatment may result in the patient’s dependence on drugs while the pain relief is insufficient. Similarly, surgical treatment is highly invasive and usually requires a long postoperative recovery along with a high recurrence rate (Andersson, 1999). Therefore, it is necessary to find new guidance to promote IVD regeneration.
The related pathological changes that cause IVDD include extracellular matrix (ECM) degradation, apoptosis, senescence, and inflammation (Cazzanelli and Wuertz-Kozak, 2020) (Figure 1). During IVDD, the balance of ECM metabolism is critical for maintaining the physiological function of the intervertebral disc. Intervertebral disc cells secrete a large number of proinflammatory factors, which trigger a series of pathological reactions, leading to autophagy, senescence and apoptosis of intervertebral disc cells, resulting in decreased synthesis of aggrecan and type II collagen (Col II), and increased expression of type I collagen (Col I) and matrix metalloproteinases (MMPs). Moreover, these cytokines recruit immune cells (T cells, B cells, neutrophils, and macrophages) to further enhance the inflammatory response and accelerate disc degeneration (Phillips et al., 2015; Risbud and Shapiro, 2014). Recently, a new kind of cell death has been identified, which was named ferroptosis. Ferroptosis, described by Dixon et al., in 2012 (Figure 2), is a kind of cell death that depends on iron and reactive oxygen species (ROS) (Dixon et al., 2012; Mou et al., 2019). Lipid peroxidation, iron accumulation, ROS formation, and glutathione depletion are some of the processes involved. Ferroptosis is linked to a range of degenerative disorders, including cancer (Zhang et al., 2022), cardiovascular disease (Wu et al., 2021), orthopedic diseases (Sun K. et al., 2021), and neurological diseases (Mahoney-Sánchez et al., 2021).
FIGURE 1. Pathological changes in healthy and degenerative IVD. Degenerative IVD will produce metabolic disorders, extracellular matrix degeneration, inflammation, and a series of programmed cell death (including necrosis, apoptosis, pyroptosis, ferroptosis, etc.).
In this study, we concentrated on the pathophysiology of ferroptosis and its use in IVDD in this review to offer fresh perspectives and a frame of reference for subsequent research.
Cell death is a natural event that occurs in all living organisms (Green, 2019). In most previous studies, IVDD treatments were based on the prevention of cell loss due to programmed or regulated cell death (RCD). Increasing evidence shows that different forms of cell death, including apoptosis (Mirzayans and Murray, 2020), pyroptosis (Liu et al., 2021), necroptosis (Wu et al., 2020), autophagy (Galluzzi et al., 2017), ferroptosis (Chen J. et al., 2022) and senescence (Du et al., 2022), are associated with IVDD (Table 1).
TABLE 1. The prominent characteristics of ferroptosis, apoptosis, autophagy, necroptosis, senescence and pyroptosis in IVDD.
The most well-known RCD is apoptosis, which is often initiated by binding of the death ligand receptor, endoplasmic reticulum (ER) stress, and malfunction of the mitochondrial respiratory chain as a result of inflammation, mechanical stress, and oxidative stress (Kim and Kim, 2018). Degenerative disc expresses more Fas-L than healthy discs, indicating that degeneration may cause Fas-L-Fas binding and activate apoptosis. Pyroptosis is a form of inflammatory reaction. By recognizing specific pathogen-related molecular patterns (PRMPs) induced by exogenous pathogens and damage-related molecular patterns (DAMPs) derived from endogenous pathogens, caspase-1 is activated by a pattern recognition receptor (PRR), represented by NLRP3, which then stimulates the production of interleukin (IL)-1β, IL-18, and gasdermin-D (GSDMD), causing an extracellular IL excess and intracellular water flux leading to cell swelling and lysis (Kamali et al., 2021). ASIC1 and ASIC3 cause pyroptosis in nucleus pulposus cells (NPCs) in addition to activating the NLRP3 inflammasome by up-regulating the ROS/NF-κB signaling pathway and increasing the expression of inflammasome components (Zhao et al., 2021). Autophagy, the process by which cells self-digest and recycle damaged components, is an important mechanism for cell survival under stress conditions, especially under nutrient deprivation (Yurube et al., 2020). Autophagy is a double-edged sword for IVDD. On the one hand, autophagy can reduce the proliferation and metabolic activity of intervertebral disc cells, increase cell apoptosis and senescence, and promote IVDD. On the other hand, cartilaginous endplate stem cells can activate autophagy and inhibit IVDD by releasing exosomes to nucleus pulposus cells (Luo et al., 2021). Therefore, the role of autophagy in IVDD remains unclear. Cellular senescence is caused by DNA replication mistakes that are a byproduct of the DNA damage response. Senescent cell aggregation results in a secretory phenotype associated with aging, characterized by persistent inflammation that induces disc matrix degradation (Cherif et al., 2020). When cells lack the capability to go through apoptosis, as death ligands cannot connect to receptors, pro-survival complex I, which is composed of receptor interacting protein (RIP) 1 and others, is formed. Complex IIa or IIb is created as a result of RIP1 deubiquitination, and complex IIb formation leads to necrosis. Compression-induced NPC death in rats and herniated human NP tissues and cells have both been linked to RIP1/RIP3/MLKL-mediated necrosis in various studies.
A unique kind of cell death known as ferroptosis, which occurs in IVDD, is marked by lipid peroxidation and free iron-mediated Fenton reactions (Yang R. Z. et al., 2021). Because ferroptotic cells do not have the necessary defensive mechanisms to remove lipid peroxides, these peroxides accumulate in great numbers (Stockwell et al., 2020). Various morphological features set ferroptosis apart from other RCD processes. We have discovered that IVDD cells subjected to ferroptosis often exhibited mitochondrial shrinkage, reduced number, increased membrane density, disruption of the normal structure of the mitochondrial crest, regular nuclear size, and membrane foaming without rupture (Dixon et al., 2012; Xie et al., 2016; Yu et al., 2017; Han et al., 2020).
Ferroptosis is driven by the imbalance between oxidation and antioxidant systems, involving ROS accumulation, lipid peroxidation, and various metabolic pathways (Figure 3). Ferroptosis is accompanied by an increase in ROS generation due to the accumulation of harmful lipid peroxides. The selective permeability of the plasma membrane is lost, and the membrane lipids become peroxidized. Studies of ferroptosis have shown various mechanisms that might initiate or influence the process. This paper reviews the important roles of iron metabolism, lipid metabolism, amino acid metabolism, and oxidative stress in ferroptosis pathogenesis.
FIGURE 3. The latest reported mechanism of ferroptosis. The essential feature of ferroptosis is the imbalance between oxidative stress and anti-oxidative stress system. This article reviews the pathogenesis of ferroptosis, including its occurrence mechanism, regulation mechanism and typical inhibitors (Table 2).
Iron, which is involved in many critical biological activities, such as oxygen transport, DNA biosynthesis, and ATP creation, is a trace element vital to cell function (Bogdan et al., 2016). Therefore, maintaining iron homeostasis is crucial. The body obtains more than two-thirds of its iron from hemoglobin in red blood cells, with the majority of the remaining portion being stored in macrophages and liver cells (Puntarulo, 2005). Iron metabolism is divided into four components: uptake, storage, utilization, and export (Chen et al., 2021). TF/TFR binds iron very firmly and has been linked to iron intake. In most mammalian cells, iron uptake occurs through endocytosis of transferrin by specific receptors. After binding to the transferrin receptor, iron bound to transferrin in plasma can be taken up by cells via receptor-mediated endocytosis (Anderson and Frazer, 2017). The resultant complex is then transported to the endosomes, where Fe3+ is released in an acidic environment. Afterward, Fe3+ is converted to Fe2+ by STEAP3 (six-transmembrane epithelial antigen of prostate family member 3), which is an endosomal iron reductase (Li et al., 2020). With the help of SLC11A2/DMT1 (member 2 of solute carrier family 11), Fe2+ is further transported into the cytoplasm. There are three fates of iron after its intake. First, it is fed to the labile iron pool (LIP) for use (Philpott et al., 2017). Second, it can be saved in ferritin for future use. About 70%–80% of the freshly imported iron is stored in ferritin, which is the primary iron storage protein. Ferritin is divided into two subtypes, H and L, also known as FTH1 (ferritin heavy chain 1) and FTL (ferritin light chain). With its iron oxidase activity, FTH1 converts Fe2+ to Fe3+. FTL contributes to iron nucleation and mineralization (Knovich et al., 2009). Through holes in the ferritin shell, iron enters the ferritin lumen and travels to the catalytic ferroxidase core, where it is internally deposited as an iron hydride. Finally, cells can also remove iron from themselves via the output of FPN/SLC40A1. If large amounts of iron build up inside the cell, this output pathway can act as a safety valve.
Iron is absorbed primarily through the duodenum, and unabsorbed iron enters the colonic cavity, where many intestinal flora reside. Iron is essential for these bacteria, and taking iron orally can promote the growth and virulence of bacterial pathogens in the gut, leading to intestinal inflammation. In addition, gut bacteria also have the ability to transport iron, affecting iron metabolism (Seyoum et al., 2021). Iron metabolism is closely related to ferroptosis, therefore, intestinal flora affects ferroptosis. Lou et al. found that urinary phospholipid A, an anti-inflammatory metabolite of gut microbiota, attenuated ferroptosis in LPS-induced acute lung injury in mice by up-regulating the Keap1-Nrf2/HO-1 signaling pathway (Lou et al., 2023). Deng et al. found that the intestinal microbial metabolite capsiate inhibits intestinal ischi-reperfusion induced ferroptosis by activating TRPV1 (Deng et al., 2021). However, the relationship between intestinal flora and ferroptosis in IVDD has not been reported.
Atherosclerosis, hemochromatosis, anemia, and joint disease have all been linked to iron metabolism abnormalities (Dabbagh et al., 1993; Weiss and Goodnough, 2005; Vinchi et al., 2020). Low iron levels stimulate TFR1 production and suppress the expression of ferritin and FPN1, whereas high iron levels within the cells may suppress DMT1 expression in an IRE-IRP-mediated way, which is associated with ferroptosis (Rouault, 2006). Cellular susceptibility to ferroptosis is impacted by modifications in iron homeostasis regulators. It has been reported that TF knockout can reduce ferroptosis induced by siramesine and lapatinib in MDA-MB-231 and SKBR3 cancer cell lines (Ma et al., 2016). Loss of TFRC also prevents ferroptosis triggered by cystine deprivation or erastin, suggesting that ferroptosis is closely related to iron uptake (Yang and Stockwell, 2008; Gao et al., 2015). FTH1-mutated flies develop significant growth defects due to, in part, ferroptosis induction (Mumbauer et al., 2019). Knocking down SLC40A1 enhances ferroptosis, whereas its overexpression inhibits ferroptosis (Geng et al., 2018).
Many enzymes that generate ROS need iron or iron derivatives as their active centers (e.g., lipoxygenase, cytochrome P450, and NADPH oxidase) (Doll and Conrad, 2017). Although iron has a vital function in maintaining life, iron overload is toxic, generating ROS and triggering cell death. Currently, iron chelators are the standard treatment for iron excess sickness. As classical iron chelating agents, DFO, DFP, and DFX bind to Fe3+ in vivo to form stable complexes, which can be cleared by urine or bile (Rodrigues de Morais and Gambero, 2019; Chen X. et al., 2020). Additionally, lactoferrin possesses anti-inflammatory and antioxidant properties. To some degree, human lactoferrin may function as an iron chelator by neutralizing free iron in synovial fluid (Guillén et al., 1998).
Ferroptosis may be prevented both in vivo and in vitro by using iron chelators. First discovered to counteract erastin and RSL3-induced ferroptosis, DFO is now the most extensive medication for preventing lipid peroxidation-mediated ferroptosis (Dixon et al., 2012). Deferasirox inhibits heme-induced human monocyte ferroptosis and ROS production (Imoto et al., 2018). Baicalein is a flavonoid that reverses ferroptosis in human pancreatic cancer cell lines through its free 5,6,7-hydroxyl complex with iron in a 1:1 ratio (Xie et al., 2016). Particularly, 2,2′-bipyridine isolates iron from unstable iron pools (LIPs) through its membrane permeability, chelates mitochondrial iron in mitochondria, and reduces ROS production (Chang et al., 2016).
Fatty acids (FAs) are vital building blocks of biofilm lipids and substrates for energy metabolism. FAs are primarily synthesized by acetyl-CoA carboxylase (ACAC), acetyl-CoA, malonyl-CoA, FASN (FA synthetase), ELOVL (ELOVL FA elongase), and FA desaturase. Lipid droplets, which are composed of triglycerides and cholesterol esters, store FAs that protect against palmitic acid-induced lipotoxicity by partitioning off damaged cell membranes. Oxidative pathway reactions, including FA activation, acyl-CoA transfer, and oxidation of acyl-CoA, mostly take place in mitochondria and are essential for FA use.
A major cause of ferroptosis is lipid peroxidation. Lipid hydroperoxides (LOOHs) and then reactive aldehydes, such as malondialdehyde (MDA) and 4-hydroxynonenal (4HNE), are produced during the free radical-driven lipid peroxidation, which primarily targets unsaturated FAs on the cell membrane. Lipid peroxides cause membrane disintegration by destabilizing the lipid bilayer. Toxic lipid ROS are produced when an abundance of Fe2+ accumulates in the cytoplasm, damaging the cells they reside in and leading to a series of morphological features of ferroptosis. As a result of the presence of highly active hydrogen atoms in methylene bridges, polyunsaturated FAs (PUFAs) are more susceptible to lipid peroxidation. The PUFAs in membrane phospholipids may combine with hydroxyl radicals to create lipid peroxides, which, in turn, promote ferroptosis (Doll et al., 2017; Yan et al., 2021). As a result of their work, ACSL4 and LPCTA3 enrich the cell membrane with sensitive PUFAs, which are necessary for the oxidation of arachidonic acid (AA) and adrenalic acid to phospholipids (PLs). Furthermore, Fe2+ may work with lipoxygenase (LOX) as a cofactor to accelerate the lipid peroxidation of PUFA (Yang et al., 2016). Increased LOX15 and PUFA binding in the cell membrane, caused by phosphatidylethanolamine (PE) binding protein 1 (PEBP1), is necessary for ferroptosis to occur (Wenzel et al., 2017).
LOX is a non-heme iron dioxygenase in the acyl oxidation of PUFAs to lipid hydroperoxides (Mashima and Okuyama, 2015; Shintoku et al., 2017). LOX is one of the key factors driving lipid peroxidation during ferroptosis, especially at the initial stage (Yang et al., 2016). When undergoing ferroptosis, LOX promotes ROS accumulation, lipid peroxidation, and cell death. LOX inhibitors, which thus inhibit ferroptosis (Probst et al., 2017), include selective 12/15-LOX inhibitors (Deschamps et al., 2006), pan-LOX inhibitors, selective 5-LOX inhibitors (Rossi et al., 2010), and selective 15-LOX inhibitor (Sendobry et al., 1997) (Table 2). Additionally, many LOX inhibitors can also be used as free radical catchers and terminators of lipid autoxidation free radical chain reactions to inhibit ferroptosis (Hanthorn et al., 2012; Shah et al., 2018). The absence of free radical capture in LOX inhibitors, such as ML351, may also be able to demonstrate antiferroptotic activity (Kagan et al., 2017).
Fatty acyl-CoA esters are formed from free long-chain FAs by ACSL proteins, which are mostly found in the ER and mitochondrial outer membrane (OMM). Acyl-CoA synthetase long-chain family 4 (ACSL4), as a member of the ACSL family, is considered a marker of ferroptosis. AA and other lipid peroxides with PE omega-6 FAs are produced by ACSL4, which also catalyzes AA-CoA synthesis (Capelletti et al., 2020). By itself, GPX4 knockout triggers ferroptosis; however, GPX4 and ACSL4 cells could develop normally, indicating that ACSL4 inhibitors suppress ferroptosis (Doll et al., 2017). ACSL4 is selectively inhibited by thiazolidinediones (TZD) (Kim et al., 2001), such as rosiglitazone (ROSI). ROSI improves intestinal barrier failure due to ischemia-reperfusion by preventing ACSL4 activation and decreasing lipid peroxidation and ferroptotic cell death (Li Y. et al., 2019). Triacsin C also has the potential to inhibit ACSL4 (Zhu et al., 2022).
Ferroptosis is triggered by monounsaturated FAs (MUFAs), as shown by Magtanong et al. (Magtanong et al., 2019), and relies on ACSL3 (acyl-CoA synthase long-chain family member 3). MUFAs do not increase GPX4 expression but prevent the accumulation of plasma membrane lipid ROS and reduce the incorporation of PUFAs into phospholipids. Lactate is a nutrient that has many regulatory effects and is associated with oxidative stress resistance and lipid biosynthesis (Chen et al., 2016). Hepatocellular carcinoma cells have a lactate-mediated ferroptosis regulation system, as discovered by Zhao et al. Via this pathway, lactic acid can enhance the anti-ferroptosis ability of HCC cells by up-regulating the production of anti-ferroptotic MUFAs (Zhao et al., 2020).
Ferroptosis occurs when the body antioxidant and oxidation defenses cannot function properly. Lipid peroxidation, membrane damage, and ferroptosis are all results of an unbalanced environment, such as increased ROS generation or reduced antioxidant system activity (Hassannia et al., 2019). Along with the Xc− system and GPX4, glutathione (GSH) serves as the major antioxidant in mammalian cells, preventing the buildup of lipid hydrogen peroxide. Solute carrier family 7 member 11 (SLC7A11) and solute carrier family 3 member 2 (SLC3A2) form the heterodimer protein complex glutamate/cystine reverse transporter system Xc−. While SLC7A11—a multichannel transmembrane protein—is responsible for regulating the action of the glutamate/cystine reverse transporter in the Xc− system, SLC3A2 keeps SLC7A11 stable and in the right place on the membrane (Nakamura et al., 1999; Koppula et al., 2018). Besides encouraging the transmembrane interchange of external cysteine and internal glutamate, the transporter also stimulates the uptake of cysteine into the cell for its production. Glutathione consists of three amino acids: glutamate, cysteine, and glycine, which are arranged in a tripeptide structure. Cysteine is often cited as the limiting factor in glutathione formation due to its limited intracellular content. Three precursor substances (glutamate, cysteine, and glycine) form GSH by glutamate–cysteine ligase (GCL). For GPX4 to continue functioning and being expressed, GSH is required as a cofactor. GPX4 is a selenoprotein that converts active PLOOH to inactive phosphatidylcholine (PLalchol, PLOH), hence, blocking the free radical chain reaction responsible for lipid peroxidation (Hassannia et al., 2019). By employing glutathione as a substrate, GPX4 converts hazardous membrane lipid hydroperoxides into lipid alcohols, which are non-toxic. In addition to neutralizing active Fe2+, GPX4 may also convert H2O2 into H2O, preventing ferroptosis.
The destruction of the antioxidant barrier induces ferroptosis. Xc− is a sodium-dependent reverse transporter that absorbs extracellular cystine in exchange for an equal amount of intracellular glutamate (Bannai, 1986; Conrad and Sato, 2012). Many cancer cells may be effectively induced to undergo ferroptosis by eliminating cystine or preventing SLC7A11 from functioning via gene ablation or pharmacological suppression. In contrast, SLC7A11 overexpression stimulates GSH production and suppresses ferroptosis in cancer cells. GSH is essential in ferroptosis because of its antioxidant properties. Many studies have demonstrated that cells are more vulnerable to oxidative stress when intracellular GSH levels are low. Evidence suggests that reduced glutathione levels promote ferroptosis (Niu et al., 2021). Inhibiting glutathione production, whether chemically or genetically, stunts tumor cell development and triggers iron-like cell death (Liu et al., 2017). In ferroptosis, GPX4 alterations have regulatory effects. In contrast to mercaptoethanol, which prevents ferroptosis by boosting GPX4 activity via increasing cystine transport into cells, heat shock protein 90 prevents ferroptosis by blocking GPX4 breakdown (Dixon et al., 2012; Wu et al., 2019).
Temozolomide (TMZ) is a lipophilic 194-Da soluble molecule and an oral imidazolotetrazine DNA-alkylating agent. TMZ is thought to be an inhibitor of ferroptosis, which acts by inducing Xc− expression. In glioblastoma multiform cells induced by TMZ, Xc− ubunit expression is significantly increased, GSH synthesis is increased, and ROS levels are decreased (Sehm et al., 2016). In the presence of erastin, glutamine can be broken down into glutamic acid and ammonia in vivo through the action of glutaminase, inducing ferroptosis. Aminooxyacetic acid, a glutaminase inhibitor, heals heart tissue injury in mice by preventing ferroptosis via blocking glutamine metabolism (Gao et al., 2015). N-Acetylcysteine (NAC) itself cannot protect GPX-deficient cells but serves as a precursor to glutathione, which then enhances the activity of the GPX4-GSH-cysteine axis and has an antiferroptotic effect (Seiler et al., 2008). Furthermore, selenium is a crucial trace element involved in mammalian life, primarily as a selenoprotein. GPX4 is an extremely significant selenoprotein. Selenium protects GPX4 from irreversible inactivation while driving GPX4 transcriptional expression, thereby inhibiting ferroptosis (Friedmann Angeli and Conrad, 2018).
Coenzyme Q (CoQ) is an endogenous isovalentyl benzoquinone molecule that transports electrons between complexes I and II and complex III in the mitochondrial electron transport chain. Eukaryotes use the mevalonate pathway to generate CoQ10. The mevalonate (MVA) route and its intermediate isopentenyl pyrophosphate (IPP) are crucial in cellular metabolism. Under the influence of pyrophosphate synthase (GGPS), it may create CoQ10 in addition to cholesterol, vitamin K, and heme (Holstein and Hohl, 2004). Later, CoQ10 is converted to CoQ10H2 through FSP1-catalyzed reactions. As a result of its ability to eliminate PLOOH, CoQ10H2 may halt the lipid oxidation chain reaction and prevent ferroptosis. FSP1 is a scavenger protein that resides in lipid droplets and plasma membrane, where it helps remove dangerous lipid hydroperoxides (Bersuker et al., 2018; Doll et al., 2019). According to available research, the FSP1/CoQ10/NAD(P)H route can prevent ferroptosis without involving the GSH/GPX4 pathway (Hadian, 2020).
FIN56 may function as a ferroptosis agent by activating squalene synthase (SQS) to promote the MVA pathway and, thus, cholesterol synthesis, thereby decreasing the formation of CoQ10 (Ingold et al., 2018).
In addition, idebenone, a water-soluble CoQ10 analog, mimics the antioxidant effects of CoQ10 and inhibits FIN56-induced ferroptosis without changing the level of basal lipid ROS (Shimada et al., 2016). Evidence suggests that iFSP1 prevents ferroptosis by counteracting FSP1 pro-lipid-peroxidation effects. Conversely, it may also sensitize cancer cells that were previously resistant to RSL3-induced ferroptosis by promoting ferroptosis in GPX4-KO cancer cells that overexpress FSP1 (Doll et al., 2019).
As a channel protein on the mitochondrial outer membrane, voltage-dependent anion channel 2/3(VDAC2/3) allows a large number of ions and metabolites to pass in and out between cytoplasm and mitochondria, which has a crucial function in preserving homeostasis maintenance in vivo (Wang Z. et al., 2020). Reportedly, erastin-induced ferroptosis in melanoma is suppressed by Nedd4 ubiquitination of VDAC2/3 (Yang Y. et al., 2020). The precise methods by which erastin and VDAC2/3 work to reduce NADH oxidation and induce ferroptosis need to be discovered. However, it is known that erastin does this by directly binding VDAC2/3, which, in turn, affects the permeability of the OMM (Yagoda et al., 2007).
Inhibition of ferroptosis through the guanosine triphosphate (GTP) cyclic hydrolase 1/tetrahydrobiotrexate (GCH1/BH4) pathway occurs independently of the GPX4 protein (Kraft et al., 2020; Soula et al., 2020). GCH1 is a rate-limiting enzyme for the production of BH4 from GTP (Thöny et al., 2000). BH4 is a powerful antioxidant that prevents lipid peroxidation from causing ferroptosis, but it has to be replenished by dihydrofolate reductase (DHFR). Inhibiting DHFR and GPX4 at the same time lowers BH4 synthesis and increases ferroptosis sensitivity. By encouraging the conversion of phenylalanine to tyrosine, BH4 plays a role in the production of coenzyme Q10, which, in turn, regulates ferroptosis.
Transfer of electrons by NADPH oxidases (NOX) in biofilms leads to the production of superoxides. NADPH is required for ferroptosis because it serves as a source of ROS and is used to produce DPP4-NOX compounds (Kang et al., 2019). After knocking down NOX4, there is a dramatic reversal of ventricular remodeling due to an improvement in iron overload (Chen et al., 2019). P53 deletion hinders the nuclear accumulation of dipeptidyl-peptidase 4 (DPP4), which consequently promotes membrane-associated DPP4-mediated lipid peroxidation by interacting with NOX1, resulting in ferroptosis in colorectal cancer. Through the reduction in ROS production by preventing DPP4 from attaching to NOXs, DPP4 inhibitors, such as linagliptin, suppress ferroptosis (Xie et al., 2017).
Hydrogen groups and peroxyl radicals are formed when unstable cellular iron combines with H2O2, which further cause PUFAs to lose hydrogen atoms (PUFAs are more prone to lose hydrogen due to their pentadiene structure), forming lipid radicals L·. The resulting lipid peroxyl radical, LOO, is a byproduct of L reaction with oxygen. Since radical-trapping antioxidants (RTAs) have a high concentration of the relatively unstable O-H and N-H bonds, they can form free radicals, which may then interact with peroxyl radicals during the propagation stage to generate non-radical products that effectively stop ferroptosis (Ingold and Pratt, 2014). RTAs include ferrostatin-1 (Fer-1), liproxstatin-1 (Lip-1), alpha-tocopherol, and tetrahydrobiopterin. Fer-1 has found widespread use both in vitro and in vivo. Researchers have shown that blocking ferroptosis with Fer-1 reduces acute lung injury caused by lipopolysaccharide (Liu et al., 2020). LIP-1 has been proven to be a more durable inhibitor of ferroptosis than previous compounds, and it does not influence other forms of RCD (Li X. et al., 2019). Surprisingly, alpha-tocopherol (α-TOH) is a modest inhibitor of ferroptosis in comparison to Fer-1 or LIP-1 (Skouta et al., 2014). Tetrahydrobiotrexate, a natural free radical catcher, can also inhibit ferroptosis by increasing the CoQ10 reduction level.
Increasing evidence indicates the role of small molecules in ferroptosis regulation. These proteins act as promoters and blockers by regulating the expression of target genes in metabolic and antioxidant pathways. In the description below, we summarize some small molecules associated with IVDD and present their potential applications in IVDD (Figure 4).
Ferroportin (FPN), also known as solute carrier family 40 member (SLC40A1), is the sole iron export protein discovered to transport iron from cytoplasm to extracellular space (Drakesmith et al., 2015). FPN is strongly expressed in cells that store iron, including hepatocytes, macrophages, neurons, and duodenal gut cells, which deliver iron to the blood, to maintain the homeostasis of total iron (Donovan et al., 2005; Zhang et al., 2018). Changing the expression of FPN might result in either an iron surplus or a deficit, and the change of iron content will affect the expression of FPN (Nemeth et al., 2004; Schimanski et al., 2005; Hentze et al., 2010). Mice rely on FPN to maintain normal amounts of intracellular iron, which is essential for mitochondrial metabolism, osteoclast formation, and bone homeostasis. Increasing osteoclast genesis and reducing bone density in vivo, FPN depletion marginally raises iron levels in precursors and mature osteoclasts, whereas iron content decreases in precursors but not in mature cells (Wang et al., 2018b). FPN also exists in IVDD. Overproduction of iron inside cells occurs during oxidative stress due to FPN dysregulation. Iron overload leads to the generation of ferroptosis, which results in an imbalance of the extracellular matrix of nucleus pulposus cells, thereby aggravating IVDD. Rescuing FPN activity, reducing intercellular iron overload, and shielding cells from ferroptosis are all accomplished through the nuclear translocation of metal-regulated transcription factor 1 (MTF1). Bioactive substance Hinokitiol promotes nuclear translocation of MTF1 and blocks the JNK pathway, which is known to inhibit oxidative stress and restore FPN function. Lu et al. have used TBHP to establish an oxidative stress model and downregulated the expression of FPN via lowering MTF1 nuclear translocation, which leads to intracellular iron overload and ferroptosis in NPCs; thus, it is a promising therapeutic for treating IVDD associated with oxidative stress (Lu et al., 2021a).
Homocysteine (Hcy) is a sulfhydryl amino acid produced during the normal biosynthesis of methionine and cysteine. It is essential for many cellular processes, including the metabolism of methionine, nucleic acid synthesis, gene methylation, neurotransmission, and phospholipid synthesis (Mudd et al., 1985; Faeh et al., 2006; Shen et al., 2020). Evidence suggests that Hcy is associated with ferroptosis. Cao et al. demonstrated that DJ-1 preserves S-adenosine homocysteine hydrolase activity to promote the transsulfuration pathway, increasing Hcy generation and inhibiting ferroptosis (Cao et al., 2020). Cystathionine β synthase (CBS) is essential to Hcy transsulfuration. In hepatocellular carcinoma, upregulation of Hcy and subsequent ferroptosis may result from CBS inhibition (Wang et al., 2018a). Importantly, Hcy is overexpressed in musculoskeletal disorders, such as osteoarthritis, osteoporosis, and fractures (Koh et al., 2006; Fayfman et al., 2009). It has been reported that hyperhomocysteinemia promotes chondrocyte degeneration and decreases chondrocyte-mediated mineralization by boosting MMP production and the degradation of ECM associated with oxidative stress. Hyperhomocysteinemia is a major contributor to IVDD onset, according to clinical epidemiological investigations. Recently, Zhang et al. have established a new link between homocysteine and IVDD. Both the anabolic and catabolic changes of Hcy treatment are dosage-dependent. Induction of Hcy by a high-methionine diet in vivo can accelerate IVDD caused by a needle prick. Specifically, the researchers attribute this to homocysteine-induced oxidative stress and ferroptosis. Folic acid is a key cofactor in the methionine cycle, which reduces GPX4 methylation and protects against Hcy-induced oxidative stress and ferroptosis. Folic acid supplementation prevents the degeneration of NPCs and rescues the imbalance between anabolism and catabolism of ECM in IVDD.
Activation transcription factor 3 (ATF3) is a member of the ATF/CREB transcription factor family (Zhou et al., 2018). Physiologically, its functions are widespread, including the regulation of intracellular signaling pathways and cellular metabolism (Rohini et al., 2018). In several pathologies, including gastric cancer and glioma, ATF3 triggers ferroptosis (Fu D. et al., 2021; Lu et al., 2021b). ATF3 is a positive regulator of ferroptosis since it directly suppresses SLC7A11 expression in erastin-induced HT1080 cells (Wang L. et al., 2020). According to the report, ATF3 can restrain the Xc− system associated with the consumption of glutathione in cells, then inhibit GPX4, and induce ferroptosis (Wang et al., 2021a). Ferroptosis significantly contributes to the worsening of spinal cord injury (SCI) development despite the fact that the underlying process through which this happens is still poorly understood. Comparing the SCI group with the sham group, bioinformatics research performed by Li et al. has revealed the top 10 hub ferroptosis genes in the subgroup, with ATF3 ranking fourth among the differential genes of ferroptosis, which provides an idea for targeting ferroptosis to repair SCI (Li J. Z. et al., 2023). In rat NP degenerative tissues, ATF3 expression has been markedly elevated in prior research. It is possible that ATF3 is translocated into the nucleus, and its level in the nucleus is elevated in response to TBHP induction. As an important target for regulating ferroptosis, ATF3 regulates TBHP-induced ROS generation and ECM degradation in NPCs by inhibiting SLC7A11 and SOD2. Therefore, silencing ATF3 may be a potential treatment for preventing IVDD progression (Li et al., 2022).
Heme oxygenase-1 (HO-1) is highly expressed in lung and intestinal mucosa (Fan et al., 2019; Puentes-Pardo et al., 2020), which has become a central effector of the mammalian stress response (Ryter et al., 2006). Free heme is broken down by the vital cell-protective enzyme HO-1 into free iron, carbon monoxide (CO), and biliverdin (BV), which then can be changed into bilirubin (BR) (Wilks, 2002). The function of HO-1 in ferroptosis is contradictory. HO-1 is an antioxidant that prevents oxidative damage and inhibits ferroptosis (Adedoyin et al., 2018); when the HO-1 activity level is low to moderate, it offers cellular protection as the ROS is neutralized, and the resulting iron can enter a non-oxidophilic state. However, excessive HO-1 activation can cause ferroptosis because of elevated intracellular iron levels (Fang et al., 2019). The antioxidant response is impacted by the lowered NADPH level caused by highly activated HO-1, along with the higher iron levels that create overpowering ferritin-neutralizing effects on labile iron (Chang et al., 2018; Hassannia et al., 2018). A high oxidative iron pool promotes ferroptosis by increasing ROS and HO-1 activity and decreasing antioxidants, such as GSH (Dixon et al., 2012). HO-1 is closely related to IVDD. IVDD tissue and replicative senescent NPCs show downregulation of HO-1 expression. With HO-1 overexpression driven by a lentivirus, NPC senescence decreases, mitochondrial function is preserved, and autophagy becomes induced via a mitochondrial route (Yi et al., 2020). Chen et al. revealed that oxidative stress can inhibit the cell viability of endogenous NP-derived mesenchymal stem cells (NPMSCs), induce apoptosis, and increase ROS production. There was an uptick in HO-1 expression in the preliminary stage, but it decreased in the later stage, which could partially reverse the oxidative damage of NPMSCs by up-regulating HO-1 expression (Chen S. et al., 2020). HO-1 can inhibit the oxidative stress of IVD, thereby inhibiting inflammation, ECM metabolism, and other factors that eventually cause IVDD. Additionally, increased HO-1 expression and iron accumulation have been observed in rat IVDD models, but the specific mechanisms need to be further studied and elucidated (Zhang et al., 2021).
Ras GTPase-activating protein-binding proteins 1 (G3BP1) is a multifunctional binding protein with various biological functions, which plays an important role in the regulation of cell senescence, immune response and RNA metabolism (Mao et al., 2018; Omer et al., 2020). G3BP1 is able to regulate ferroptosis. Raised G3BP1 blocking the combination of P53 and SLC7A11 gene promoter regions, less influence on SLC7A11 transcription, promote SLC7A11-GSH-GPX4 signaling pathways, inhibit ferroptosis, relieve acute liver failure (Li W. et al., 2023). Under compression pressure, G3BP1 is mainly present in lysosomes, and its dysfunction promotes the inactivation of lysophagy. Li et al. found that in nucleus pulposus cells, G3BP1 can initiate lysosomal phagocytosis to remove damaged lysosomes by regulating TSC/mTOR signaling, thereby reversing the rise of free iron and lipid peroxidation and alleviating ferroptosis (Li S. et al., 2023).
BTB and CNC homology 1 (BACH1) is a heme-regulated transcription factor that inhibits genes involved in iron and heme metabolism in normal cells and has the potential to form the metabolism and metastasis of cancer cells (Igarashi et al., 2021). BACH1 is involved in the regulation of various physiological processes, including oxidative stress, aging, cell cycle and mitosis (Padilla and Lee, 2021). BACH1 is an important gene in the process of ferroptosis. BACH1 has been reported to promote ferroptosis after cerebral ischemia-reperfusion injury by activating KDM4C-mediated COX2 demethylation (Zhang et al., 2023). In addition, in the acute lung injury model established by Wang et al., the deletion of BACH1 gene was found to activate the Nrf2/HO-1 signaling pathway, thereby alleviating ferroptosis (Wang et al., 2023). Surprisingly, BACH1 also plays a regulatory role in IVDD. Transcription factor BACH1 targeting GPX4 inhibits oxidative stress-induced ferroptosis to promote nucleus pulposus cell degeneration. Moreover, in vivo experiments showed that knockdown of BACH1 reversed the increase of ACSL4 levels and the decrease of FTH, FTL, SLC7A11 and GPX4 levels in the IVDD group. This provides a new therapeutic strategy for the treatment of IVDD (Yao et al., 2023).
Ubiquitin specific protease 11 (USP11), located at Xp11.23, stabilizes other proteins through deubiquitination and plays an important role in regulating multiple biological processes such as cell proliferation, cancer growth and metastasis, and cancer drug resistance (Sun et al., 2019). USP11 plays an important role in the occurrence and development of ferroptosis. It has been reported that USP11 promotes autophagy activation and induces ferroptosis by deubiquitinating and stabilizing Beclin 1, thereby regulating spinal cord ischemia-reperfusion injury (Rong et al., 2022). In addition, Duan et al. found that USP11 can mediate LSH deubiquitination and activate CYP24A1 to inhibit ferroptosis in colorectal cancer (Duan et al., 2023). Zhu et al. found that Sirt3 decreased and ferroptosis occurred after the development of IVDD. USP11 can directly deubiquitinate and stabilize Sirt3. Inhibition of USP11 disrupts Sirt3 to promote oxidative stress-induced ferroptosis and aggravate IVDD (Zhu et al., 2023a).
Epigenetic regulation involves DNA methylation, non-coding RNA and exosomes. Epigenetic regulation determines gene transcription, cell fate, and developmental processes in an organism. These mechanisms regulate ferroptosis in IVDD (Figure 3). We outline the current epigenetic regulatory mechanisms of ferroptosis in IVDD.
Non-coding RNAs (ncRNAs) account for nearly 60% of transcription products in human cells, including microRNAs (miRNAs), long non-coding RNAs (lncRNAs), and circular RNAs (circRNAs), which modulate cellular processes and pathways in developmental and pathological environments (Djebali et al., 2012). ncRNAs may control ferroptosis by either directly influencing important regulatory variables or indirectly influencing upstream targets, and the specific mechanism has been partially identified. According to research by Luo et al., miR-137 overexpression suppresses ferroptosis in melanoma cells by binding SLC1A5 at its 3′-UTR (Luo et al., 2018). In IVDD, ncRNA plays a similar role. Current evidence indicates that degenerative IVD demonstrates upregulation of the proinflammatory cytokine IL-6 in its cartilage tissue. Increased oxidative stress and iron retention, as well as induction of ferroptosis, in chondrocytes are results of the miR-10A-5p-mediated release of IL-6R, which acts as an IL-6 receptor (Bin et al., 2021). Because ATF3 is a direct target of miR-874-3p, as determined by bioinformatics analysis and molecular tests, this observation raises the possibility that the downregulation of miR-874-3p in IVDD may be responsible for the upregulation of ATF3 (Li et al., 2022). Circ_0072464 downregulation and miR-431 upregulation have been observed in IVDD. Circ-0072464 upregulates NRF2 expression by competitive binding of miR-431 and induces matrix synthesis and NPC proliferation, causing a reduction in IVDD and the suppression of NPC ferroptosis (Yu et al., 2022). This finding opens the door to future treatment efforts aimed at reducing IVDD by inhibiting NPC ferroptosis. Additionally, when it comes to SCI, ncRNA is also commonly employed. MiR-672-3p inhibits ferroptosis through the FSP1 pathway, plays a neurorestorative role in vivo and in vitro, and improves motor function in SCI rats (Wang et al., 2022). Furthermore, lncGm36569, as a competitive RNA of miR-5627-5p, induces the upregulation of FSP1 and inhibits ferroptosis in neuronal cells, thereby attenuating neuronal dysfunction (Shao et al., 2022).
DNA methylation, put forward in 1944 by Avery (Avery et al., 1944)., is an epigenetic mechanism. The DNA methyltransferase (Dnmts) family is responsible for catalyzing DNA methylation, which occurs when S-adenosine methionine (SAM) methyl groups are transferred to the fifth carbon of cytosine residues to produce 5 mC. DNA methylation patterns in the genome undergo dynamic changes throughout development as a consequence of de novo DNA methylation and demethylation. Gene expression may be controlled by DNA methylation, which either recruits proteins participating in gene repression or blocks transcription factors connecting to DNA (Moore et al., 2013).
DNA methylation is widely used in orthopedics. According to research by Yang et al., THAP9-AS1 is highly expressed in osteosarcoma, where it increases methylation of the SOCS3 promoter, thereby promoting carcinogenesis while, at the same time, reducing ROS generation through the JAK2/STAT3 signaling pathway (Yang S. et al., 2021). Additionally, in SCI rats after treadmill exercise, DNA methylation is enhanced, and functional recovery is promoted. Consequently, we draw the conclusion that epigenetic modifications in the motor cortex may play a role in the functional benefits obtained by exercise (Davaa et al., 2021). DNA methylation has been significantly associated with ferroptosis. Head and neck cancer cells with DNA hypermethylation at CDH1 gene promoter reduce the production of e-cadherin (CDH1-encoded) and are more sensitive to iron (Lee J. et al., 2020). Gene expression can be inhibited by methylation transfer, which can also cause methylation. Methylase expression of DMNT1, DMNT3a, and DMNT3b was increased by Hcy in a study by Zhang et al. NPCs treated with Hcy have extensive methylation of GPX4 promoter. Enhanced GPX4 methylation upregulates oxidative stress and ferroptosis, thereby promoting ECM metabolic disorder in NPCs. Folic acid is a crucial coenzyme that inhibits the elevated methylase stimulated by Hcy. Gene and protein expression of GPX4 are both suppressed by methylation and recovered by methylase inhibitors, such as 5-AZA and folic acid (Zhang X. et al., 2020), suggesting a potential application of folic acid in IVDD. More research is necessary to determine whether DNA methylation also impacts additional genes involved in ferroptosis.
Exosomes and microvesicles are released from cells, play important roles in cell-to-cell communication and disease development, and serve as biomarkers due to mRNAs, miRNAs, lipids, and proteins they carry (Mead and Tomarev, 2020). Recently, extracellular vesicles have been increasingly used in ferroptosis for their therapeutic value. Lin et al. found that endothelial progenitor cells-secreted extracellular vesicles (EPC-EVs) inhibited FSP1 by delivering miR-199a-3p. As a result, endothelial ferroptosis was suppressed, and atherosclerosis development was slowed (Li L. et al., 2021). Recently, the use of EVs released by MSC as a treatment for IVDD has gained popularity (Sun Y. et al., 2021). BMSC-EV-loaded CIRC 0072464 suppresses NPC ferroptosis via up-regulating miR-431-mediated NRF2 to ameliorate IVDD, offering a possible therapeutic target for IVDD (Yu et al., 2022).
Ferroptosis is a promising future research topic and might have important therapeutic applications. Ferroptosis has a connection to the occurrence and prognosis of numerous illnesses, such as cancer, hematologic diseases, neurodegenerative diseases, and heart diseases. Additionally, many published studies now point in the direction of ferroptosis as a viable option for treating a wide range of orthopedic disorders. The prognosis for osteosarcoma, the most frequent primary malignant bone cancer, is not optimistic. The major cause of osteosarcoma not responding to therapy and subsequent relapse is drug resistance. By blocking the STAT3/Nrf2/GPX4 signaling pathway, osteosarcoma cells become more susceptible to cisplatin. This finding demonstrates a unique strategy for increasing drug sensitivity in osteosarcoma by either ferroptosis inducers or STAT3 inhibitors (Liu and Wang, 2019). Furthermore, synergistic growth inhibition in hypoxic osteosarcoma has been achieved by the induction of ferroptosis and substantial GPX4 downregulation using ultrasound-activated doxorubicin (DOX)-Fe(VI)@HMS-HE-PEG (DFHHP) nanoparticles (Fu J. et al., 2021). SCI is a disorder of the neurological system caused by a sudden injury, resulting in varying degrees of motor, sensory, and autonomic dysfunction. Ferroptosis inhibitor U0126 blocks the RAS/RAF/ERK pathway, which restores SCI and decreases glial scar formation in injured regions. This has also been achieved by lowering astrocyte proliferation, protecting neurons, and promoting axonal regeneration (Table 3).
Moreover, ferroptosis points the way to the clinical treatment of IVDD. The formation of new blood vessels is a characteristic of IVDD. Yasuma et al. first observed neovascularization in the herniated NP of LDH patients by histological staining in 1993 (Yasuma et al., 1993). It has been recently proposed that angiogenesis promotes tissue degradation following disc damage and may be a healing mechanism (Xiao et al., 2020). Vascularized granulation tissue produces inflammatory cytokines that stimulate nociceptors in the protruding NP, leading to further exacerbation of clinical symptoms (Yasuma et al., 1993). Previous studies have shown that hemoglobin is released from red blood cells oozing from immature capillaries in atherosclerotic lesions and human tumors (Nagy et al., 2010). The oxidation of hemoglobin to ferriyl hemoglobin then results in the emission of iron porphyrins, leading to an increase in intracellular iron content (Chifman et al., 2014). Matrix-assisted laser desorption-ionization time of flight mass spectrometry (MALDI-TOF MS) was used to analyze clinically herniated and nonherniated NP; the study found a significant increase in hemoglobin and heme signaling and a decrease in ferroptosis markers GPX4 and glutathione in herniated NP. Therefore, herniated NP with neovascularization may expose tissue to high amounts of heme, which may trigger cytotoxicity and ferroptosis and accelerate progressive degeneration. These findings aid in the study of degenerative illnesses and will lead to novel approaches for the conservative treatment of patients with LDH, for instance, angiogenesis intervention (Shan et al., 2021).
Ferritin autophagy is a property of intracellular iron concentration and selective ferritin degradation. Serum iron metabolism parameters, particularly serum ferritin (SF), have been the subject of research on IVDD by Guo et al. Correlation analysis has shown that intervertebral disc degeneration was significantly associated with SF but not with serum iron (SI), transferrin saturation (TS), unsaturated iron binding capacity (UIBC), and total iron binding capacity (TIBC). The concentrations of SF were surprisingly greater in patients with low-severe IVDD compared to those with high-severe IVDD in the single-disc grade. According to the receiver operating characteristic curve, patients with SF levels below 170.5 ng/mL exhibited advanced disc degeneration. Therefore, SF is inversely associated with IVDD severity, suggesting a novel approach to the clinical prediction of IVDD severity.
With the help of ongoing studies, IVDD has transformed from a debilitating condition to a treatable one. While current IVDD treatments, such as medication and surgery, are effective, they also come with drawbacks, such as a high recurrence rate, excessive bleeding, and other complications. Therefore, improving IVDD is a difficult problem for clinicians.
Ferroptosis has received a lot of attention, and great progress has been recently achieved in its research. In this article, we provided a summary of the cellular morphology, pathogenesis, and regulation of ferroptosis. Iron metabolism, lipid peroxidation, ROS accumulation, GPX4 regulation, and FSP1-mediated pathway are a few of the processes involved in ferroptosis. However, the study of ferroptosis in IVDD is just beginning and there are still many unanswered questions. First, current studies of ferroptosis in IVDD are only scratching the surface of the results, and it is unclear exactly what causes it and what the target molecules and associated signaling pathways are. Second, many researchers have only studied ferroptosis in cell and animal models, and there is a lack of effective clinical evidence. Subsequently, ferroptosis in IVDD have been studied in nucleus pulposus cells, while other intervertebral disc cells, such as annulus fibrosus cells and endplate chondrocytes, have been poorly studied. In addition, many long non-coding RNAs and circular RNAs have been found to affect ferroptosis in IVDD, indicating that molecular mechanisms at the post-recording level are important in regulating cellular ferroptosis, and more small RNAs related to ferroptosis need to be explored in the future. Additionally, current models of ferroptosis in IVDD are primarily caused by oxidative stress and inflammation. Factors such as hypoxia and acidic microenvironment need to be confirmed whether they can be used as ferroptosis models in IVDD. Finally, as we all know, mixed cell death has become a hot topic. At present, autophagy is closely related to ferroptosis. A large number of literatures have proved that ferroptosis is an autophagy-dependent cell death (Zhou et al., 2020). It is also involved in the intervertebral disc field. Li et al. found that G3BP1 coordinates TSC/mTOR complex activation of lysophagy, clearance of damaged lysosomes, and inhibition of ferroptosis. However, there are few published literatures, and the relationship between autophagy and ferroptosis in intervertebral disc has not been clarified. Furthermore, whether other forms of cell death, such as apoptosis, interact with ferroptosis also needs to be further explored.
CF and HM contributed to the conception and design of this review article. CF, GC, and ZY drafted the manuscript. DG and XW performed searches, analyses, and interpretations. ZJ, FK, LY, and JW searched for some manuscripts and figures and contributed to the final version of the manuscript. DG, XW, and HM substantially revised the paper. HM gave final approval for the version to be submitted. All authors contributed to the article and approved the submitted version.
This work was supported by the National Natural Science Foundation of China (81972104), the Natural Science Foundation of Jiangsu Province (BK20200199), Suzhou Youth Science and Technology Fund Project (KJXW2021006), the Natural Science Foundation of The First Affiliated Hospital of Soochow University (BXQN202112) and the Priority Academic Program Development of Jiangsu Higher Education Institutions (PAPD).
The authors declare that the research was conducted in the absence of any commercial or financial relationships that could be construed as a potential conflict of interest.
All claims expressed in this article are solely those of the authors and do not necessarily represent those of their affiliated organizations, or those of the publisher, the editors and the reviewers. Any product that may be evaluated in this article, or claim that may be made by its manufacturer, is not guaranteed or endorsed by the publisher.
Abdul, Y., Li, W., Ward, R., Abdelsaid, M., Hafez, S., Dong, G., et al. (2021). Deferoxamine treatment prevents post-stroke vasoregression and neurovascular unit remodeling leading to improved functional outcomes in type 2 male diabetic rats: Role of endothelial ferroptosis. Transl. Stroke Res. 12 (4), 615–630. doi:10.1007/s12975-020-00844-7
Abou Daher, A., Francis, M., Azzam, P., Ahmad, A., Eid, A. A., Fornoni, A., et al. (2020). Modulation of radiation-induced damage of human glomerular endothelial cells by SMPDL3B. Faseb J. 34 (6), 7915–7926. doi:10.1096/fj.201902179R
Adedoyin, O., Boddu, R., Traylor, A., Lever, J. M., Bolisetty, S., George, J. F., et al. (2018). Heme oxygenase-1 mitigates ferroptosis in renal proximal tubule cells. Am. J. Physiol. Ren. Physiol. 314 (5), F702–f714. doi:10.1152/ajprenal.00044.2017
Alborzinia, H., Ignashkova, T. I., Dejure, F. R., Gendarme, M., Theobald, J., Wölfl, S., et al. (2018). Golgi stress mediates redox imbalance and ferroptosis in human cells. Commun. Biol. 1, 210. doi:10.1038/s42003-018-0212-6
Alim, I., Caulfield, J. T., Chen, Y., Swarup, V., Geschwind, D. H., Ivanova, E., et al. (2019). Selenium drives a transcriptional adaptive Program to block ferroptosis and treat stroke. Cell 177 (5), 1262–1279. doi:10.1016/j.cell.2019.03.032
Anderson, G. J., and Frazer, D. M. (2017). Current understanding of iron homeostasis. Am. J. Clin. Nutr. 106 (6), 1559S–1566s. doi:10.3945/ajcn.117.155804
Andersson, G. B. (1999). Epidemiological features of chronic low-back pain. Lancet 354 (9178), 581–585. doi:10.1016/s0140-6736(99)01312-4
Avery, O. T., Macleod, C. M., and McCarty, M. (1944). Studies on the chemical nature of the substance inducing transformation of pneumococcal types: Induction of transformation by A desoxyribonucleic acid fraction isolated from pneumococcus type III. J. Exp. Med. 79 (2), 137–158. doi:10.1084/jem.79.2.137
Bannai, S. (1986). Exchange of cystine and glutamate across plasma membrane of human fibroblasts. J. Biol. Chem. 261 (5), 2256–2263. doi:10.1016/s0021-9258(17)35926-4
Bersuker, K., Peterson, C. W. H., To, M., Sahl, S. J., Savikhin, V., Grossman, E. A., et al. (2018). A proximity labeling strategy provides insights into the composition and dynamics of lipid droplet proteomes. Dev. Cell 44 (1), 97–112. doi:10.1016/j.devcel.2017.11.020
Bin, S., Xin, L., Lin, Z., Jinhua, Z., Rui, G., and Xiang, Z. (2021). Targeting miR-10a-5p/IL-6R axis for reducing IL-6-induced cartilage cell ferroptosis. Exp. Mol. Pathol. 118, 104570. doi:10.1016/j.yexmp.2020.104570
Bogdan, A. R., Miyazawa, M., Hashimoto, K., and Tsuji, Y. (2016). Regulators of iron homeostasis: New players in metabolism, cell death, and disease. Trends Biochem. Sci. 41 (3), 274–286. doi:10.1016/j.tibs.2015.11.012
Cao, J., Chen, X., Jiang, L., Lu, B., Yuan, M., Zhu, D., et al. (2020). DJ-1 suppresses ferroptosis through preserving the activity of S-adenosyl homocysteine hydrolase. Nat. Commun. 11 (1), 1251. doi:10.1038/s41467-020-15109-y
Capelletti, M. M., Manceau, H., Puy, H., and Peoc'h, K. (2020). Ferroptosis in liver diseases: An overview. Int. J. Mol. Sci. 21 (14), 4908. doi:10.3390/ijms21144908
Cazzanelli, P., and Wuertz-Kozak, K. (2020). MicroRNAs in intervertebral disc degeneration, apoptosis, inflammation, and mechanobiology. Int. J. Mol. Sci. 21 (10), 3601. doi:10.3390/ijms21103601
Chang, H. C., Wu, R., Shang, M., Sato, T., Chen, C., Shapiro, J. S., et al. (2016). Reduction in mitochondrial iron alleviates cardiac damage during injury. EMBO Mol. Med. 8 (3), 247–267. doi:10.15252/emmm.201505748
Chang, L. C., Chiang, S. K., Chen, S. E., Yu, Y. L., Chou, R. H., and Chang, W. C. (2018). Heme oxygenase-1 mediates BAY 11-7085 induced ferroptosis. Cancer Lett. 416, 124–137. doi:10.1016/j.canlet.2017.12.025
Chen, J., Yang, X., Feng, Y., Li, Q., Ma, J., Wang, L., et al. (2022a). Targeting ferroptosis holds potential for intervertebral disc degeneration therapy. Cells 11 (21), 3508. doi:10.3390/cells11213508
Chen, S., Lei, L., Li, Z., Chen, F., Huang, Y., Jiang, G., et al. (2022b). Grem1 accelerates nucleus pulposus cell apoptosis and intervertebral disc degeneration by inhibiting TGF-β-mediated Smad2/3 phosphorylation. Exp. Mol. Med. 54 (4), 518–530. doi:10.1038/s12276-022-00753-9
Chen, S., Liu, S., Zhao, L., Lin, H., Ma, K., and Shao, Z. (2020a). Heme oxygenase-1-mediated autophagy protects against oxidative damage in rat nucleus pulposus-derived mesenchymal stem cells. Oxid. Med. Cell Longev. 2020, 9349762. doi:10.1155/2020/9349762
Chen, X., Li, J., Kang, R., Klionsky, D. J., and Tang, D. (2021). Ferroptosis: Machinery and regulation. Autophagy 17 (9), 2054–2081. doi:10.1080/15548627.2020.1810918
Chen, X., Xu, S., Zhao, C., and Liu, B. (2019). Role of TLR4/NADPH oxidase 4 pathway in promoting cell death through autophagy and ferroptosis during heart failure. Biochem. Biophys. Res. Commun. 516 (1), 37–43. doi:10.1016/j.bbrc.2019.06.015
Chen, X., Yu, C., Kang, R., and Tang, D. (2020b). Iron metabolism in ferroptosis. Front. Cell Dev. Biol. 8, 590226. doi:10.3389/fcell.2020.590226
Chen, Y. J., Mahieu, N. G., Huang, X., Singh, M., Crawford, P. A., Johnson, S. L., et al. (2016). Lactate metabolism is associated with mammalian mitochondria. Nat. Chem. Biol. 12 (11), 937–943. doi:10.1038/nchembio.2172
Cherif, H., Bisson, D. G., Mannarino, M., Rabau, O., Ouellet, J. A., and Haglund, L. (2020). Senotherapeutic drugs for human intervertebral disc degeneration and low back pain. Elife 9, e54693. doi:10.7554/eLife.54693
Chifman, J., Laubenbacher, R., and Torti, S. V. (2014). A systems biology approach to iron metabolism. Adv. Exp. Med. Biol. 844, 201–225. doi:10.1007/978-1-4939-2095-2_10
Conrad, M., and Pratt, D. A. (2019). The chemical basis of ferroptosis. Nat. Chem. Biol. 15 (12), 1137–1147. doi:10.1038/s41589-019-0408-1
Conrad, M., and Sato, H. (2012). The oxidative stress-inducible cystine/glutamate antiporter, system x (c) (-): Cystine supplier and beyond. Amino Acids 42 (1), 231–246. doi:10.1007/s00726-011-0867-5
Dabbagh, A. J., Trenam, C. W., Morris, C. J., and Blake, D. R. (1993). Iron in joint inflammation. Ann. Rheum. Dis. 52 (1), 67–73. doi:10.1136/ard.52.1.67
Davaa, G., Hong, J. Y., Kim, T. U., Lee, S. J., Kim, S. Y., Hong, K., et al. (2021). Exercise ameliorates spinal cord injury by changing DNA methylation. Cells 10 (1), 143. doi:10.3390/cells10010143
Deng, F., Zhao, B. C., Yang, X., Lin, Z. B., Sun, Q. S., Wang, Y. F., et al. (2021). The gut microbiota metabolite capsiate promotes Gpx4 expression by activating TRPV1 to inhibit intestinal ischemia reperfusion-induced ferroptosis. Gut Microbes 13 (1), 1–21. doi:10.1080/19490976.2021.1902719
Deschamps, J. D., Kenyon, V. A., and Holman, T. R. (2006). Baicalein is a potent in vitro inhibitor against both reticulocyte 15-human and platelet 12-human lipoxygenases. Bioorg Med. Chem. 14 (12), 4295–4301. doi:10.1016/j.bmc.2006.01.057
Dixon, S. J., Lemberg, K. M., Lamprecht, M. R., Skouta, R., Zaitsev, E. M., Gleason, C. E., et al. (2012). Ferroptosis: An iron-dependent form of nonapoptotic cell death. Cell 149 (5), 1060–1072. doi:10.1016/j.cell.2012.03.042
Djebali, S., Davis, C. A., Merkel, A., Dobin, A., Lassmann, T., Mortazavi, A., et al. (2012). Landscape of transcription in human cells. Nature 489 (7414), 101–108. doi:10.1038/nature11233
Doll, S., and Conrad, M. (2017). Iron and ferroptosis: A still ill-defined liaison. IUBMB Life 69 (6), 423–434. doi:10.1002/iub.1616
Doll, S., Freitas, F. P., Shah, R., Aldrovandi, M., da Silva, M. C., Ingold, I., et al. (2019). FSP1 is a glutathione-independent ferroptosis suppressor. Nature 575 (7784), 693–698. doi:10.1038/s41586-019-1707-0
Doll, S., Proneth, B., Tyurina, Y. Y., Panzilius, E., Kobayashi, S., Ingold, I., et al. (2017). ACSL4 dictates ferroptosis sensitivity by shaping cellular lipid composition. Nat. Chem. Biol. 13 (1), 91–98. doi:10.1038/nchembio.2239
Donovan, A., Lima, C. A., Pinkus, J. L., Pinkus, G. S., Zon, L. I., Robine, S., et al. (2005). The iron exporter ferroportin/Slc40a1 is essential for iron homeostasis. Cell Metab. 1 (3), 191–200. doi:10.1016/j.cmet.2005.01.003
Drakesmith, H., Nemeth, E., and Ganz, T. (2015). Ironing out ferroportin. Cell Metab. 22 (5), 777–787. doi:10.1016/j.cmet.2015.09.006
Du, J., Xu, M., Kong, F., Zhu, P., Mao, Y., Liu, Y., et al. (2022). CB2R attenuates intervertebral disc degeneration by delaying nucleus pulposus cell senescence through AMPK/GSK3β pathway. Aging Dis. 13 (2), 552–567. doi:10.14336/ad.2021.1025
Duan, J., Huang, D., Liu, C., Lv, Y., Zhang, L., Chang, F., et al. (2023). USP11-mediated LSH deubiquitination inhibits ferroptosis in colorectal cancer through epigenetic activation of CYP24A1. Cell Death Dis. 14 (7), 402. doi:10.1038/s41419-023-05915-9
Faeh, D., Chiolero, A., and Paccaud, F. (2006). Homocysteine as a risk factor for cardiovascular disease: Should we (still) worry about? Swiss Med. Wkly. 136 (47-48), 745–756. doi:10.4414/smw.2006.11283
Fan, J., Lv, H., Li, J., Che, Y., Xu, B., Tao, Z., et al. (2019). Roles of Nrf2/HO-1 and HIF-1α/VEGF in lung tissue injury and repair following cerebral ischemia/reperfusion injury. J. Cell Physiol. 234 (6), 7695–7707. doi:10.1002/jcp.27767
Fang, X., Wang, H., Han, D., Xie, E., Yang, X., Wei, J., et al. (2019). Ferroptosis as a target for protection against cardiomyopathy. Proc. Natl. Acad. Sci. U. S. A. 116 (7), 2672–2680. doi:10.1073/pnas.1821022116
Fayfman, M., Niu, J., Zhang, Y. Q., Felson, D. T., Sack, B., Aliabadi, P., et al. (2009). The relation of plasma homocysteine to radiographic knee osteoarthritis. Osteoarthr. Cartil. 17 (6), 766–771. doi:10.1016/j.joca.2008.11.015
Francisco, V., Pino, J., González-Gay, M., Lago, F., Karppinen, J., Tervonen, O., et al. (2022). A new immunometabolic perspective of intervertebral disc degeneration. Nat. Rev. Rheumatol. 18 (1), 47–60. doi:10.1038/s41584-021-00713-z
Friedmann Angeli, J. P., and Conrad, M. (2018). Selenium and GPX4, a vital symbiosis. Free Radic. Biol. Med. 127, 153–159. doi:10.1016/j.freeradbiomed.2018.03.001
Friedmann Angeli, J. P., Schneider, M., Proneth, B., Tyurina, Y. Y., Tyurin, V. A., Hammond, V. J., et al. (2014). Inactivation of the ferroptosis regulator Gpx4 triggers acute renal failure in mice. Nat. Cell Biol. 16 (12), 1180–1191. doi:10.1038/ncb3064
Fu, D., Wang, C., Yu, L., and Yu, R. (2021a). Induction of ferroptosis by ATF3 elevation alleviates cisplatin resistance in gastric cancer by restraining Nrf2/Keap1/xCT signaling. Cell Mol. Biol. Lett. 26 (1), 26. doi:10.1186/s11658-021-00271-y
Fu, J., Li, T., Yang, Y., Jiang, L., Wang, W., Fu, L., et al. (2021b). Activatable nanomedicine for overcoming hypoxia-induced resistance to chemotherapy and inhibiting tumor growth by inducing collaborative apoptosis and ferroptosis in solid tumors. Biomaterials 268, 120537. doi:10.1016/j.biomaterials.2020.120537
Galluzzi, L., Baehrecke, E. H., Ballabio, A., Boya, P., Bravo-San Pedro, J. M., Cecconi, F., et al. (2017). Molecular definitions of autophagy and related processes. Embo J. 36 (13), 1811–1836. doi:10.15252/embj.201796697
Gao, M., Monian, P., Quadri, N., Ramasamy, R., and Jiang, X. (2015). Glutaminolysis and transferrin regulate ferroptosis. Mol. Cell 59 (2), 298–308. doi:10.1016/j.molcel.2015.06.011
GBD 2016 Disease and Injury Incidence and Prevalence Collaborators (2017). Global, regional, and national incidence, prevalence, and years lived with disability for 328 diseases and injuries for 195 countries, 1990-2016: A systematic analysis for the global burden of disease study 2016. Lancet 390 (10100), 1211–1259. doi:10.1016/s0140-6736(17)32154-2
GBD 2017 Disease and Injury Incidence and Prevalence Collaborators (2018). Global, regional, and national incidence, prevalence, and years lived with disability for 354 diseases and injuries for 195 countries and territories, 1990-2017: A systematic analysis for the global burden of disease study 2017. Lancet 392 (10159), 1789–1858. doi:10.1016/s0140-6736(18)32279-7
Geng, N., Shi, B. J., Li, S. L., Zhong, Z. Y., Li, Y. C., Xua, W. L., et al. (2018). Knockdown of ferroportin accelerates erastin-induced ferroptosis in neuroblastoma cells. Eur. Rev. Med. Pharmacol. Sci. 22 (12), 3826–3836. doi:10.26355/eurrev_201806_15267
Green, D. R. (2019). The coming decade of cell death research: Five riddles. Cell 177 (5), 1094–1107. doi:10.1016/j.cell.2019.04.024
Guillén, C., McInnes, I. B., Kruger, H., and Brock, J. H. (1998). Iron, lactoferrin and iron regulatory protein activity in the synovium; relative importance of iron loading and the inflammatory response. Ann. Rheum. Dis. 57 (5), 309–314. doi:10.1136/ard.57.5.309
Hadian, K. (2020). Ferroptosis suppressor protein 1 (FSP1) and coenzyme Q(10) cooperatively suppress ferroptosis. Biochemistry 59 (5), 637–638. doi:10.1021/acs.biochem.0c00030
Han, C., Liu, Y., Dai, R., Ismail, N., Su, W., and Li, B. (2020). Ferroptosis and its potential role in human diseases. Front. Pharmacol. 11, 239. doi:10.3389/fphar.2020.00239
Hanthorn, J. J., Valgimigli, L., and Pratt, D. A. (2012). Incorporation of ring nitrogens into diphenylamine antioxidants: Striking a balance between reactivity and stability. J. Am. Chem. Soc. 134 (20), 8306–8309. doi:10.1021/ja300086z
Hassannia, B., Vandenabeele, P., and Vanden Berghe, T. (2019). Targeting ferroptosis to iron out cancer. Cancer Cell 35 (6), 830–849. doi:10.1016/j.ccell.2019.04.002
Hassannia, B., Wiernicki, B., Ingold, I., Qu, F., Van Herck, S., Tyurina, Y. Y., et al. (2018). Nano-targeted induction of dual ferroptotic mechanisms eradicates high-risk neuroblastoma. J. Clin. Invest. 128 (8), 3341–3355. doi:10.1172/jci99032
Hentze, M. W., Muckenthaler, M. U., Galy, B., and Camaschella, C. (2010). Two to tango: Regulation of mammalian iron metabolism. Cell 142 (1), 24–38. doi:10.1016/j.cell.2010.06.028
Holstein, S. A., and Hohl, R. J. (2004). Isoprenoids: Remarkable diversity of form and function. Lipids 39 (4), 293–309. doi:10.1007/s11745-004-1233-3
Hwang, J. S., Cha, E. H., Ha, E., Park, B., and Seo, J. H. (2020). GKT136901 protects primary human brain microvascular endothelial cells against methamphetamine-induced blood-brain barrier dysfunction. Life Sci. 256, 117917. doi:10.1016/j.lfs.2020.117917
Igarashi, K., Nishizawa, H., Saiki, Y., and Matsumoto, M. (2021). The transcription factor BACH1 at the crossroads of cancer biology: From epithelial-mesenchymal transition to ferroptosis. J. Biol. Chem. 297 (3), 101032. doi:10.1016/j.jbc.2021.101032
Imoto, S., Kono, M., Suzuki, T., Shibuya, Y., Sawamura, T., Mizokoshi, Y., et al. (2018). Haemin-induced cell death in human monocytic cells is consistent with ferroptosis. Transfus. Apher. Sci. 57 (4), 524–531. doi:10.1016/j.transci.2018.05.028
Ingold, I., Berndt, C., Schmitt, S., Doll, S., Poschmann, G., Buday, K., et al. (2018). Selenium utilization by GPX4 is required to prevent hydroperoxide-induced ferroptosis. Cell 172 (3), 409–422. doi:10.1016/j.cell.2017.11.048
Ingold, K. U., and Pratt, D. A. (2014). Advances in radical-trapping antioxidant chemistry in the 21st century: A kinetics and mechanisms perspective. Chem. Rev. 114 (18), 9022–9046. doi:10.1021/cr500226n
James, S. L., Abate, D., Abate, K. H., Abay, S. M., Abbafati, C., Abbasi, N., et al. (2018). Global, regional, and national incidence, prevalence, and years lived with disability for 354 diseases and injuries for 195 countries and territories, 1990-2017: a systematic analysis for the Global Burden of Disease Study 2017. Lancet 392 (10159), 1789–1858. doi:10.1016/s0140-6736(18)32279-7
Jelinek, A., Heyder, L., Daude, M., Plessner, M., Krippner, S., Grosse, R., et al. (2018). Mitochondrial rescue prevents glutathione peroxidase-dependent ferroptosis. Free Radic. Biol. Med. 117, 45–57. doi:10.1016/j.freeradbiomed.2018.01.019
Jo, A., Bae, J. H., Yoon, Y. J., Chung, T. H., Lee, E. W., Kim, Y. H., et al. (2022). Plasma-activated medium induces ferroptosis by depleting FSP1 in human lung cancer cells. Cell Death Dis. 13 (3), 212. doi:10.1038/s41419-022-04660-9
Jomen, W., Ohtake, T., Akita, T., Suto, D., Yagi, H., Osawa, Y., et al. (2022). Iron chelator deferasirox inhibits NF-κB activity in hepatoma cells and changes sorafenib-induced programmed cell deaths. Biomed. Pharmacother. 153, 113363. doi:10.1016/j.biopha.2022.113363
Kagan, V. E., Mao, G., Qu, F., Angeli, J. P., Doll, S., Croix, C. S., et al. (2017). Oxidized arachidonic and adrenic PEs navigate cells to ferroptosis. Nat. Chem. Biol. 13 (1), 81–90. doi:10.1038/nchembio.2238
Kamali, A., Ziadlou, R., Lang, G., Pfannkuche, J., Cui, S., Li, Z., et al. (2021). Small molecule-based treatment approaches for intervertebral disc degeneration: Current options and future directions. Theranostics 11 (1), 27–47. doi:10.7150/thno.48987
Kang, R., Kroemer, G., and Tang, D. (2019). The tumor suppressor protein p53 and the ferroptosis network. Free Radic. Biol. Med. 133, 162–168. doi:10.1016/j.freeradbiomed.2018.05.074
Kim, J. H., Lewin, T. M., and Coleman, R. A. (2001). Expression and characterization of recombinant rat Acyl-CoA synthetases 1, 4, and 5. Selective inhibition by triacsin C and thiazolidinediones. J. Biol. Chem. 276 (27), 24667–24673. doi:10.1074/jbc.M010793200
Kim, S. H., and Kim, H. (2018). Inhibitory effect of astaxanthin on oxidative stress-induced mitochondrial dysfunction-A mini-review. Nutrients 10 (9), 1137. doi:10.3390/nu10091137
Knezevic, N. N., Candido, K. D., Vlaeyen, J. W. S., Van Zundert, J., and Cohen, S. P. (2021). Low back pain. Lancet 398 (10294), 78–92. doi:10.1016/s0140-6736(21)00733-9
Knovich, M. A., Storey, J. A., Coffman, L. G., Torti, S. V., and Torti, F. M. (2009). Ferritin for the clinician. Blood Rev. 23 (3), 95–104. doi:10.1016/j.blre.2008.08.001
Koh, J. M., Lee, Y. S., Kim, Y. S., Kim, D. J., Kim, H. H., Park, J. Y., et al. (2006). Homocysteine enhances bone resorption by stimulation of osteoclast formation and activity through increased intracellular ROS generation. J. Bone Min. Res. 21 (7), 1003–1011. doi:10.1359/jbmr.060406
Koppula, P., Zhang, Y., Zhuang, L., and Gan, B. (2018). Amino acid transporter SLC7A11/xCT at the crossroads of regulating redox homeostasis and nutrient dependency of cancer. Cancer Commun. (Lond) 38 (1), 12. doi:10.1186/s40880-018-0288-x
Kraft, V. A. N., Bezjian, C. T., Pfeiffer, S., Ringelstetter, L., Müller, C., Zandkarimi, F., et al. (2020). GTP cyclohydrolase 1/tetrahydrobiopterin counteract ferroptosis through lipid remodeling. ACS Cent. Sci. 6 (1), 41–53. doi:10.1021/acscentsci.9b01063
Lee, H., Zandkarimi, F., Zhang, Y., Meena, J. K., Kim, J., Zhuang, L., et al. (2020a). Energy-stress-mediated AMPK activation inhibits ferroptosis. Nat. Cell Biol. 22 (2), 225–234. doi:10.1038/s41556-020-0461-8
Lee, J., You, J. H., Kim, M. S., and Roh, J. L. (2020b). Epigenetic reprogramming of epithelial-mesenchymal transition promotes ferroptosis of head and neck cancer. Redox Biol. 37, 101697. doi:10.1016/j.redox.2020.101697
Li, C., Deng, X., Zhang, W., Xie, X., Conrad, M., Liu, Y., et al. (2019a). Novel allosteric activators for ferroptosis regulator glutathione peroxidase 4. J. Med. Chem. 62 (1), 266–275. doi:10.1021/acs.jmedchem.8b00315
Li, J., Cao, F., Yin, H. L., Huang, Z. J., Lin, Z. T., Mao, N., et al. (2020). Ferroptosis: Past, present and future. Cell Death Dis. 11 (2), 88. doi:10.1038/s41419-020-2298-2
Li, J. Z., Fan, B. Y., Sun, T., Wang, X. X., Li, J. J., Zhang, J. P., et al. (2023a). Bioinformatics analysis of ferroptosis in spinal cord injury. Neural Regen. Res. 18 (3), 626–633. doi:10.4103/1673-5374.350209
Li, L., Liu, Y. R., Gao, S., Li, J. F., Li, S. S., Zhang, D. D., et al. (2014). Inhibition of 5-lipoxygenase pathway attenuates acute liver failure by inhibiting macrophage activation. J. Immunol. Res. 2014, 697560. doi:10.1155/2014/697560
Li, L., Wang, H., Zhang, J., Chen, X., Zhang, Z., and Li, Q. (2021a). Effect of endothelial progenitor cell-derived extracellular vesicles on endothelial cell ferroptosis and atherosclerotic vascular endothelial injury. Cell Death Discov. 7 (1), 235. doi:10.1038/s41420-021-00610-0
Li, S., Liao, Z., Yin, H., Liu, O., Hua, W., Wu, X., et al. (2023b). G3BP1 coordinates lysophagy activity to protect against compression-induced cell ferroptosis during intervertebral disc degeneration. Cell Prolif. 56 (3), e13368. doi:10.1111/cpr.13368
Li, W., Li, W., Li, X., Wang, L., and Wang, Y. (2023c). Effect of P53 nuclear localization mediated by G3BP1 on ferroptosis in acute liver failure. Apoptosis 28, 1226–1240. doi:10.1007/s10495-023-01856-y
Li, X., Duan, L., Yuan, S., Zhuang, X., Qiao, T., and He, J. (2019b). Ferroptosis inhibitor alleviates Radiation-induced lung fibrosis (RILF) via down-regulation of TGF-β1. J. Inflamm. (Lond) 16, 11. doi:10.1186/s12950-019-0216-0
Li, Y., Feng, D., Wang, Z., Zhao, Y., Sun, R., Tian, D., et al. (2019c). Ischemia-induced ACSL4 activation contributes to ferroptosis-mediated tissue injury in intestinal ischemia/reperfusion. Cell Death Differ. 26 (11), 2284–2299. doi:10.1038/s41418-019-0299-4
Li, Y., Pan, D., Wang, X., Huo, Z., Wu, X., Li, J., et al. (2022). Silencing ATF3 might delay TBHP-induced intervertebral disc degeneration by repressing NPC ferroptosis, apoptosis, and ECM degradation. Oxid. Med. Cell Longev. 2022, 4235126. doi:10.1155/2022/4235126
Li, Z., Wang, J., Deng, X., Huang, D., Shao, Z., and Ma, K. (2021b). Compression stress induces nucleus pulposus cell autophagy by inhibition of the PI3K/AKT/mTOR pathway and activation of the JNK pathway. Connect. Tissue Res. 62 (3), 337–349. doi:10.1080/03008207.2020.1736578
Lin, Y., Jiao, Y., Yuan, Y., Zhou, Z., Zheng, Y., Xiao, J., et al. (2018). Propionibacterium acnes induces intervertebral disc degeneration by promoting nucleus pulposus cell apoptosis via the TLR2/JNK/mitochondrial-mediated pathway. Emerg. Microbes Infect. 7 (1), 1. doi:10.1038/s41426-017-0002-0
Liu, D. S., Duong, C. P., Haupt, S., Montgomery, K. G., House, C. M., Azar, W. J., et al. (2017). Inhibiting the system x(C)(-)/glutathione axis selectively targets cancers with mutant-p53 accumulation. Nat. Commun. 8, 14844. doi:10.1038/ncomms14844
Liu, P., Feng, Y., Li, H., Chen, X., Wang, G., Xu, S., et al. (2020). Ferrostatin-1 alleviates lipopolysaccharide-induced acute lung injury via inhibiting ferroptosis. Cell Mol. Biol. Lett. 25, 10. doi:10.1186/s11658-020-00205-0
Liu, P., Zhang, Z., and Li, Y. (2021). Relevance of the pyroptosis-related inflammasome pathway in the pathogenesis of diabetic kidney disease. Front. Immunol. 12, 603416. doi:10.3389/fimmu.2021.603416
Liu, Q., and Wang, K. (2019). The induction of ferroptosis by impairing STAT3/Nrf2/GPx4 signaling enhances the sensitivity of osteosarcoma cells to cisplatin. Cell Biol. Int. 43 (11), 1245–1256. doi:10.1002/cbin.11121
Lou, L., Wang, M., He, J., Yang, S., Meng, F., Wang, S., et al. (2023). Urolithin A (UA) attenuates ferroptosis in LPS-induced acute lung injury in mice by upregulating Keap1-Nrf2/HO-1 signaling pathway. Front. Pharmacol. 14, 1067402. doi:10.3389/fphar.2023.1067402
Lu, S., Song, Y., Luo, R., Li, S., Li, G., Wang, K., et al. (2021a). Ferroportin-dependent iron homeostasis protects against oxidative stress-induced nucleus pulposus cell ferroptosis and ameliorates intervertebral disc degeneration in vivo. Oxid. Med. Cell Longev. 2021, 6670497. doi:10.1155/2021/6670497
Lu, S., Wang, X. Z., He, C., Wang, L., Liang, S. P., Wang, C. C., et al. (2021b). ATF3 contributes to brucine-triggered glioma cell ferroptosis via promotion of hydrogen peroxide and iron. Acta Pharmacol. Sin. 42 (10), 1690–1702. doi:10.1038/s41401-021-00700-w
Luo, L., Jian, X., Sun, H., Qin, J., Wang, Y., Zhang, J., et al. (2021). Cartilage endplate stem cells inhibit intervertebral disc degeneration by releasing exosomes to nucleus pulposus cells to activate Akt/autophagy. Stem Cells 39 (4), 467–481. doi:10.1002/stem.3322
Luo, M., Wu, L., Zhang, K., Wang, H., Zhang, T., Gutierrez, L., et al. (2018). miR-137 regulates ferroptosis by targeting glutamine transporter SLC1A5 in melanoma. Cell Death Differ. 25 (8), 1457–1472. doi:10.1038/s41418-017-0053-8
Ma, S., Henson, E. S., Chen, Y., and Gibson, S. B. (2016). Ferroptosis is induced following siramesine and lapatinib treatment of breast cancer cells. Cell Death Dis. 7 (7), e2307. doi:10.1038/cddis.2016.208
Magtanong, L., Ko, P. J., To, M., Cao, J. Y., Forcina, G. C., Tarangelo, A., et al. (2019). Exogenous monounsaturated fatty acids promote a ferroptosis-resistant cell state. Cell Chem. Biol. 26 (3), 420–432. doi:10.1016/j.chembiol.2018.11.016
Mahoney-Sánchez, L., Bouchaoui, H., Ayton, S., Devos, D., Duce, J. A., and Devedjian, J. C. (2021). Ferroptosis and its potential role in the physiopathology of Parkinson's Disease. Prog. Neurobiol. 196, 101890. doi:10.1016/j.pneurobio.2020.101890
Mao, C., Wang, X., Liu, Y., Wang, M., Yan, B., Jiang, Y., et al. (2018). A G3BP1-interacting lncRNA promotes ferroptosis and apoptosis in cancer via nuclear sequestration of p53. Cancer Res. 78 (13), 3484–3496. doi:10.1158/0008-5472.Can-17-3454
Mashima, R., and Okuyama, T. (2015). The role of lipoxygenases in pathophysiology; new insights and future perspectives. Redox Biol. 6, 297–310. doi:10.1016/j.redox.2015.08.006
Matsushita, M., Freigang, S., Schneider, C., Conrad, M., Bornkamm, G. W., and Kopf, M. (2015). T cell lipid peroxidation induces ferroptosis and prevents immunity to infection. J. Exp. Med. 212 (4), 555–568. doi:10.1084/jem.20140857
Mead, B., and Tomarev, S. (2020). Extracellular vesicle therapy for retinal diseases. Prog. Retin Eye Res. 79, 100849. doi:10.1016/j.preteyeres.2020.100849
Mirzayans, R., and Murray, D. (2020). Do TUNEL and other apoptosis assays detect cell death in preclinical studies? Int. J. Mol. Sci. 21 (23), 9090. doi:10.3390/ijms21239090
Mishima, E., Sato, E., Ito, J., Yamada, K. I., Suzuki, C., Oikawa, Y., et al. (2020). Drugs repurposed as antiferroptosis agents suppress organ damage, including AKI, by functioning as lipid peroxyl radical scavengers. J. Am. Soc. Nephrol. 31 (2), 280–296. doi:10.1681/asn.2019060570
Moore, L. D., Le, T., and Fan, G. (2013). DNA methylation and its basic function. Neuropsychopharmacology 38 (1), 23–38. doi:10.1038/npp.2012.112
Mou, Y., Wang, J., Wu, J., He, D., Zhang, C., Duan, C., et al. (2019). Ferroptosis, a new form of cell death: Opportunities and challenges in cancer. J. Hematol. Oncol. 12 (1), 34. doi:10.1186/s13045-019-0720-y
Mudd, S. H., Skovby, F., Levy, H. L., Pettigrew, K. D., Wilcken, B., Pyeritz, R. E., et al. (1985). The natural history of homocystinuria due to cystathionine beta-synthase deficiency. Am. J. Hum. Genet. 37 (1), 1–31.
Mumbauer, S., Pascual, J., Kolotuev, I., and Hamaratoglu, F. (2019). Ferritin heavy chain protects the developing wing from reactive oxygen species and ferroptosis. PLoS Genet. 15 (9), e1008396. doi:10.1371/journal.pgen.1008396
Nagy, E., Eaton, J. W., Jeney, V., Soares, M. P., Varga, Z., Galajda, Z., et al. (2010). Red cells, hemoglobin, heme, iron, and atherogenesis. Arterioscler. Thromb. Vasc. Biol. 30 (7), 1347–1353. doi:10.1161/atvbaha.110.206433
Nakamura, E., Sato, M., Yang, H., Miyagawa, F., Harasaki, M., Tomita, K., et al. (1999). 4F2 (CD98) heavy chain is associated covalently with an amino acid transporter and controls intracellular trafficking and membrane topology of 4F2 heterodimer. J. Biol. Chem. 274 (5), 3009–3016. doi:10.1074/jbc.274.5.3009
Nemeth, E., Tuttle, M. S., Powelson, J., Vaughn, M. B., Donovan, A., Ward, D. M., et al. (2004). Hepcidin regulates cellular iron efflux by binding to ferroportin and inducing its internalization. Science 306 (5704), 2090–2093. doi:10.1126/science.1104742
Nieva-Echevarría, B., Goicoechea, E., and Guillén, M. D. (2017). Polyunsaturated lipids and vitamin A oxidation during cod liver oil in vitro gastrointestinal digestion. Antioxidant effect of added BHT. Food Chem. 232, 733–743. doi:10.1016/j.foodchem.2017.04.057
Niu, B., Liao, K., Zhou, Y., Wen, T., Quan, G., Pan, X., et al. (2021). Application of glutathione depletion in cancer therapy: Enhanced ROS-based therapy, ferroptosis, and chemotherapy. Biomaterials 277, 121110. doi:10.1016/j.biomaterials.2021.121110
Omer, A., Barrera, M. C., Moran, J. L., Lian, X. J., Di Marco, S., Beausejour, C., et al. (2020). G3BP1 controls the senescence-associated secretome and its impact on cancer progression. Nat. Commun. 11 (1), 4979. doi:10.1038/s41467-020-18734-9
Padilla, J., and Lee, J. (2021). A novel therapeutic target, BACH1, regulates cancer metabolism. Cells 10 (3), 634. doi:10.3390/cells10030634
Phillips, K. L., Cullen, K., Chiverton, N., Michael, A. L., Cole, A. A., Breakwell, L. M., et al. (2015). Potential roles of cytokines and chemokines in human intervertebral disc degeneration: interleukin-1 is a master regulator of catabolic processes. Osteoarthr. Cartil. 23 (7), 1165–1177. doi:10.1016/j.joca.2015.02.017
Philpott, C. C., Ryu, M. S., Frey, A., and Patel, S. (2017). Cytosolic iron chaperones: Proteins delivering iron cofactors in the cytosol of mammalian cells. J. Biol. Chem. 292 (31), 12764–12771. doi:10.1074/jbc.R117.791962
Poon, J. F., Zilka, O., and Pratt, D. A. (2020). Potent ferroptosis inhibitors can catalyze the cross-dismutation of phospholipid-derived peroxyl radicals and hydroperoxyl radicals. J. Am. Chem. Soc. 142 (33), 14331–14342. doi:10.1021/jacs.0c06379
Probst, L., Dächert, J., Schenk, B., and Fulda, S. (2017). Lipoxygenase inhibitors protect acute lymphoblastic leukemia cells from ferroptotic cell death. Biochem. Pharmacol. 140, 41–52. doi:10.1016/j.bcp.2017.06.112
Puentes-Pardo, J. D., Moreno-SanJuan, S., Carazo, Á., and León, J. (2020). Heme oxygenase-1 in gastrointestinal tract health and disease. Antioxidants (Basel) 9 (12), 1214. doi:10.3390/antiox9121214
Puntarulo, S. (2005). Iron, oxidative stress and human health. Mol. Asp. Med. 26 (4-5), 299–312. doi:10.1016/j.mam.2005.07.001
Risbud, M. V., and Shapiro, I. M. (2014). Role of cytokines in intervertebral disc degeneration: Pain and disc content. Nat. Rev. Rheumatol. 10 (1), 44–56. doi:10.1038/nrrheum.2013.160
Rodrigues de Morais, T., and Gambero, A. (2019). Iron chelators in obesity therapy - old drugs from a new perspective? Eur. J. Pharmacol. 861, 172614. doi:10.1016/j.ejphar.2019.172614
Rohini, M., Haritha Menon, A., and Selvamurugan, N. (2018). Role of activating transcription factor 3 and its interacting proteins under physiological and pathological conditions. Int. J. Biol. Macromol. 120, 310–317. doi:10.1016/j.ijbiomac.2018.08.107
Rong, Y., Fan, J., Ji, C., Wang, Z., Ge, X., Wang, J., et al. (2022). USP11 regulates autophagy-dependent ferroptosis after spinal cord ischemia-reperfusion injury by deubiquitinating Beclin 1. Cell Death Differ. 29 (6), 1164–1175. doi:10.1038/s41418-021-00907-8
Rossi, A., Pergola, C., Koeberle, A., Hoffmann, M., Dehm, F., Bramanti, P., et al. (2010). The 5-lipoxygenase inhibitor, zileuton, suppresses prostaglandin biosynthesis by inhibition of arachidonic acid release in macrophages. Br. J. Pharmacol. 161 (3), 555–570. doi:10.1111/j.1476-5381.2010.00930.x
Rouault, T. A. (2006). The role of iron regulatory proteins in mammalian iron homeostasis and disease. Nat. Chem. Biol. 2 (8), 406–414. doi:10.1038/nchembio807
Ryter, S. W., Alam, J., and Choi, A. M. (2006). Heme oxygenase-1/carbon monoxide: From basic science to therapeutic applications. Physiol. Rev. 86 (2), 583–650. doi:10.1152/physrev.00011.2005
Schimanski, L. M., Drakesmith, H., Merryweather-Clarke, A. T., Viprakasit, V., Edwards, J. P., Sweetland, E., et al. (2005). In vitro functional analysis of human ferroportin (FPN) and hemochromatosis-associated FPN mutations. Blood 105 (10), 4096–4102. doi:10.1182/blood-2004-11-4502
Sehm, T., Rauh, M., Wiendieck, K., Buchfelder, M., Eyüpoglu, I. Y., and Savaskan, N. E. (2016). Temozolomide toxicity operates in a xCT/SLC7a11 dependent manner and is fostered by ferroptosis. Oncotarget 7 (46), 74630–74647. doi:10.18632/oncotarget.11858
Seiler, A., Schneider, M., Förster, H., Roth, S., Wirth, E. K., Culmsee, C., et al. (2008). Glutathione peroxidase 4 senses and translates oxidative stress into 12/15-lipoxygenase dependent- and AIF-mediated cell death. Cell Metab. 8 (3), 237–248. doi:10.1016/j.cmet.2008.07.005
Sendobry, S. M., Cornicelli, J. A., Welch, K., Bocan, T., Tait, B., Trivedi, B. K., et al. (1997). Attenuation of diet-induced atherosclerosis in rabbits with a highly selective 15-lipoxygenase inhibitor lacking significant antioxidant properties. Br. J. Pharmacol. 120 (7), 1199–1206. doi:10.1038/sj.bjp.0701007
Seyoum, Y., Baye, K., and Humblot, C. (2021). Iron homeostasis in host and gut bacteria - a complex interrelationship. Gut Microbes 13 (1), 1–19. doi:10.1080/19490976.2021.1874855
Sha, L. K., Sha, W., Kuchler, L., Daiber, A., Giegerich, A. K., Weigert, A., et al. (2015). Loss of Nrf2 in bone marrow-derived macrophages impairs antigen-driven CD8(+) T cell function by limiting GSH and Cys availability. Free Radic. Biol. Med. 83, 77–88. doi:10.1016/j.freeradbiomed.2015.02.004
Shah, R., Margison, K., and Pratt, D. A. (2017). The potency of diarylamine radical-trapping antioxidants as inhibitors of ferroptosis underscores the role of autoxidation in the mechanism of cell death. ACS Chem. Biol. 12 (10), 2538–2545. doi:10.1021/acschembio.7b00730
Shah, R., Shchepinov, M. S., and Pratt, D. A. (2018). Resolving the role of lipoxygenases in the initiation and execution of ferroptosis. ACS Cent. Sci. 4 (3), 387–396. doi:10.1021/acscentsci.7b00589
Shan, L., Xu, X., Zhang, J., Cai, P., Gao, H., Lu, Y., et al. (2021). Increased hemoglobin and heme in MALDI-TOF MS analysis induce ferroptosis and promote degeneration of herniated human nucleus pulposus. Mol. Med. 27 (1), 103. doi:10.1186/s10020-021-00368-2
Shao, C., Chen, Y., Yang, T., Zhao, H., and Li, D. (2022). Mesenchymal stem cell derived exosomes suppress neuronal cell ferroptosis via lncGm36569/miR-5627-5p/FSP1 Axis in acute spinal cord injury. Stem Cell Rev. Rep. 18 (3), 1127–1142. doi:10.1007/s12015-022-10327-x
Shen, W., Gao, C., Cueto, R., Liu, L., Fu, H., Shao, Y., et al. (2020). Homocysteine-methionine cycle is a metabolic sensor system controlling methylation-regulated pathological signaling. Redox Biol. 28, 101322. doi:10.1016/j.redox.2019.101322
Shimada, K., Skouta, R., Kaplan, A., Yang, W. S., Hayano, M., Dixon, S. J., et al. (2016). Global survey of cell death mechanisms reveals metabolic regulation of ferroptosis. Nat. Chem. Biol. 12 (7), 497–503. doi:10.1038/nchembio.2079
Shintoku, R., Takigawa, Y., Yamada, K., Kubota, C., Yoshimoto, Y., Takeuchi, T., et al. (2017). Lipoxygenase-mediated generation of lipid peroxides enhances ferroptosis induced by erastin and RSL3. Cancer Sci. 108 (11), 2187–2194. doi:10.1111/cas.13380
Skouta, R., Dixon, S. J., Wang, J., Dunn, D. E., Orman, M., Shimada, K., et al. (2014). Ferrostatins inhibit oxidative lipid damage and cell death in diverse disease models. J. Am. Chem. Soc. 136 (12), 4551–4556. doi:10.1021/ja411006a
Soula, M., Weber, R. A., Zilka, O., Alwaseem, H., La, K., Yen, F., et al. (2020). Metabolic determinants of cancer cell sensitivity to canonical ferroptosis inducers. Nat. Chem. Biol. 16 (12), 1351–1360. doi:10.1038/s41589-020-0613-y
Southon, A., Szostak, K., Acevedo, K. M., Dent, K. A., Volitakis, I., Belaidi, A. A., et al. (2020). Cu(II) (atsm) inhibits ferroptosis: Implications for treatment of neurodegenerative disease. Br. J. Pharmacol. 177 (3), 656–667. doi:10.1111/bph.14881
Stockwell, B. R., Friedmann Angeli, J. P., Bayir, H., Bush, A. I., Conrad, M., Dixon, S. J., et al. (2017). Ferroptosis: A regulated cell death nexus linking metabolism, redox biology, and disease. Cell 171 (2), 273–285. doi:10.1016/j.cell.2017.09.021
Stockwell, B. R., Jiang, X., and Gu, W. (2020). Emerging mechanisms and disease relevance of ferroptosis. Trends Cell Biol. 30 (6), 478–490. doi:10.1016/j.tcb.2020.02.009
Sun, H., Ou, B., Zhao, S., Liu, X., Song, L., Liu, X., et al. (2019). USP11 promotes growth and metastasis of colorectal cancer via PPP1CA-mediated activation of ERK/MAPK signaling pathway. EBioMedicine 48, 236–247. doi:10.1016/j.ebiom.2019.08.061
Sun, K., Guo, Z., Hou, L., Xu, J., Du, T., Xu, T., et al. (2021a). Iron homeostasis in arthropathies: From pathogenesis to therapeutic potential. Ageing Res. Rev. 72, 101481. doi:10.1016/j.arr.2021.101481
Sun, Y., Zhang, W., and Li, X. (2021b). Induced pluripotent stem cell-derived mesenchymal stem cells deliver exogenous miR-105-5p via small extracellular vesicles to rejuvenate senescent nucleus pulposus cells and attenuate intervertebral disc degeneration. Stem Cell Res. Ther. 12 (1), 286. doi:10.1186/s13287-021-02362-1
Thöny, B., Auerbach, G., and Blau, N. (2000). Tetrahydrobiopterin biosynthesis, regeneration and functions. Biochem. J. 347, 1–16. doi:10.1042/bj3470001
Torii, S., Shintoku, R., Kubota, C., Yaegashi, M., Torii, R., Sasaki, M., et al. (2016). An essential role for functional lysosomes in ferroptosis of cancer cells. Biochem. J. 473 (6), 769–777. doi:10.1042/bj20150658
Vinchi, F., Porto, G., Simmelbauer, A., Altamura, S., Passos, S. T., Garbowski, M., et al. (2020). Atherosclerosis is aggravated by iron overload and ameliorated by dietary and pharmacological iron restriction. Eur. Heart J. 41 (28), 2681–2695. doi:10.1093/eurheartj/ehz112
Vos, T., Abajobir, A. A., Abate, K. H., Abbafati, C., Abbas, K. M., Abd Allah, M., et al. (2017). Global, regional, and national incidence, prevalence, and years lived with disability for 328 diseases and injuries for 195 countries, 1990-2016: a systematic analysis for the Global Burden of Disease Study 2016. Lancet 390 (10100), 1211–1259. doi:10.1016/s0140-6736(17)32154-2
Wang, D., Peng, Y., Xie, Y., Zhou, B., Sun, X., Kang, R., et al. (2016). Antiferroptotic activity of non-oxidative dopamine. Biochem. Biophys. Res. Commun. 480 (4), 602–607. doi:10.1016/j.bbrc.2016.10.099
Wang, F., Li, J., Zhao, Y., Guo, D., Liu, D., Chang, S., et al. (2022). miR-672-3p promotes functional recovery in rats with contusive spinal cord injury by inhibiting ferroptosis suppressor protein 1. Oxid. Med. Cell Longev. 2022, 6041612. doi:10.1155/2022/6041612
Wang, L., Cai, H., Hu, Y., Liu, F., Huang, S., Zhou, Y., et al. (2018a). A pharmacological probe identifies cystathionine β-synthase as a new negative regulator for ferroptosis. Cell Death Dis. 9 (10), 1005. doi:10.1038/s41419-018-1063-2
Wang, L., Fang, B., Fujiwara, T., Krager, K., Gorantla, A., Li, C., et al. (2018b). Deletion of ferroportin in murine myeloid cells increases iron accumulation and stimulates osteoclastogenesis in vitro and in vivo. J. Biol. Chem. 293 (24), 9248–9264. doi:10.1074/jbc.RA117.000834
Wang, L., Liu, Y., Du, T., Yang, H., Lei, L., Guo, M., et al. (2020a). ATF3 promotes erastin-induced ferroptosis by suppressing system Xc. Cell Death Differ. 27 (2), 662–675. doi:10.1038/s41418-019-0380-z
Wang, R. X., Gu, X., Zhang, S. X., Zhao, Y. J., Zhang, H. J., and Li, F. Y. (2023). Deletion of BACH1 alleviates ferroptosis and protects against LPS-triggered acute lung injury by activating Nrf2/HO-1 signaling pathway. Biochem. Biophys. Res. Commun. 644, 8–14. doi:10.1016/j.bbrc.2023.01.002
Wang, Y., Quan, F., Cao, Q., Lin, Y., Yue, C., Bi, R., et al. (2021a). Quercetin alleviates acute kidney injury by inhibiting ferroptosis. J. Adv. Res. 28, 231–243. doi:10.1016/j.jare.2020.07.007
Wang, Y., Yu, R., Wu, L., and Yang, G. (2021b). Hydrogen sulfide guards myoblasts from ferroptosis by inhibiting ALOX12 acetylation. Cell Signal 78, 109870. doi:10.1016/j.cellsig.2020.109870
Wang, Z., Qin, J., Zhao, J., Li, J., Li, D., Popp, M., et al. (2020b). Inflammatory IFIT3 renders chemotherapy resistance by regulating post-translational modification of VDAC2 in pancreatic cancer. Theranostics 10 (16), 7178–7192. doi:10.7150/thno.43093
Weiss, G., and Goodnough, L. T. (2005). Anemia of chronic disease. N. Engl. J. Med. 352 (10), 1011–1023. doi:10.1056/NEJMra041809
Wenzel, S. E., Tyurina, Y. Y., Zhao, J., St Croix, C. M., Dar, H. H., Mao, G., et al. (2017). PEBP1 wardens ferroptosis by enabling lipoxygenase generation of lipid death signals. Cell 171 (3), 628–641. doi:10.1016/j.cell.2017.09.044
Wilks, A. (2002). Heme oxygenase: Evolution, structure, and mechanism. Antioxid. Redox Signal 4 (4), 603–614. doi:10.1089/15230860260220102
Wu, J., Ye, J., Kong, W., Zhang, S., and Zheng, Y. (2020). Programmed cell death pathways in hearing loss: A review of apoptosis, autophagy and programmed necrosis. Cell Prolif. 53 (11), e12915. doi:10.1111/cpr.12915
Wu, X., Li, Y., Zhang, S., and Zhou, X. (2021). Ferroptosis as a novel therapeutic target for cardiovascular disease. Theranostics 11 (7), 3052–3059. doi:10.7150/thno.54113
Wu, Z., Geng, Y., Lu, X., Shi, Y., Wu, G., Zhang, M., et al. (2019). Chaperone-mediated autophagy is involved in the execution of ferroptosis. Proc. Natl. Acad. Sci. U. S. A. 116 (8), 2996–3005. doi:10.1073/pnas.1819728116
Xiao, Z. F., Su, G. Y., Hou, Y., Chen, S. D., Zhao, B. D., He, J. B., et al. (2020). Mechanics and biology interact in intervertebral disc degeneration: A novel composite mouse model. Calcif. Tissue Int. 106 (4), 401–414. doi:10.1007/s00223-019-00644-8
Xie, Y., Song, X., Sun, X., Huang, J., Zhong, M., Lotze, M. T., et al. (2016). Identification of baicalein as a ferroptosis inhibitor by natural product library screening. Biochem. Biophys. Res. Commun. 473 (4), 775–780. doi:10.1016/j.bbrc.2016.03.052
Xie, Y., Zhu, S., Song, X., Sun, X., Fan, Y., Liu, J., et al. (2017). The tumor suppressor p53 limits ferroptosis by blocking DPP4 activity. Cell Rep. 20 (7), 1692–1704. doi:10.1016/j.celrep.2017.07.055
Yagoda, N., von Rechenberg, M., Zaganjor, E., Bauer, A. J., Yang, W. S., Fridman, D. J., et al. (2007). RAS-RAF-MEK-dependent oxidative cell death involving voltage-dependent anion channels. Nature 447 (7146), 864–868. doi:10.1038/nature05859
Yan, H. F., Zou, T., Tuo, Q. Z., Xu, S., Li, H., Belaidi, A. A., et al. (2021). Ferroptosis: Mechanisms and links with diseases. Signal Transduct. Target Ther. 6 (1), 49. doi:10.1038/s41392-020-00428-9
Yang, C. C., Hsiao, L. D., Tseng, H. C., Kuo, C. M., and Yang, C. M. (2020a). Pristimerin inhibits MMP-9 expression and cell migration through attenuating NOX/ROS-Dependent NF-κB activation in rat brain astrocytes challenged with LPS. J. Inflamm. Res. 13, 325–341. doi:10.2147/jir.S252659
Yang, C., Han, M., Li, R., Zhou, L., Zhang, Y., Duan, L., et al. (2021a). Curcumin nanoparticles inhibiting ferroptosis for the enhanced treatment of intracerebral hemorrhage. Int. J. Nanomedicine 16, 8049–8065. doi:10.2147/ijn.S334965
Yang, R. Z., Xu, W. N., Zheng, H. L., Zheng, X. F., Li, B., Jiang, L. S., et al. (2021b). Involvement of oxidative stress-induced annulus fibrosus cell and nucleus pulposus cell ferroptosis in intervertebral disc degeneration pathogenesis. J. Cell Physiol. 236 (4), 2725–2739. doi:10.1002/jcp.30039
Yang, S., Wang, B., Liu, C., Wang, Q., Wang, R., Su, W., et al. (2021c). THAP9-AS1 promotes tumorigenesis and reduces ROS generation through the JAK2/STAT3 signaling pathway by increasing SOCS3 promoter methylation in osteosarcoma. Oxid. Med. Cell Longev. 2021, 5620475. doi:10.1155/2021/5620475
Yang, W., Liu, X., Song, C., Ji, S., Yang, J., Liu, Y., et al. (2021d). Structure-activity relationship studies of phenothiazine derivatives as a new class of ferroptosis inhibitors together with the therapeutic effect in an ischemic stroke model. Eur. J. Med. Chem. 209, 112842. doi:10.1016/j.ejmech.2020.112842
Yang, W. S., Kim, K. J., Gaschler, M. M., Patel, M., Shchepinov, M. S., and Stockwell, B. R. (2016). Peroxidation of polyunsaturated fatty acids by lipoxygenases drives ferroptosis. Proc. Natl. Acad. Sci. U. S. A. 113 (34), E4966–E4975. doi:10.1073/pnas.1603244113
Yang, W. S., SriRamaratnam, R., Welsch, M. E., Shimada, K., Skouta, R., Viswanathan, V. S., et al. (2014). Regulation of ferroptotic cancer cell death by GPX4. Cell 156 (1-2), 317–331. doi:10.1016/j.cell.2013.12.010
Yang, W. S., and Stockwell, B. R. (2008). Synthetic lethal screening identifies compounds activating iron-dependent, nonapoptotic cell death in oncogenic-RAS-harboring cancer cells. Chem. Biol. 15 (3), 234–245. doi:10.1016/j.chembiol.2008.02.010
Yang, Y., Luo, M., Zhang, K., Zhang, J., Gao, T., Connell, D. O., et al. (2020b). Nedd4 ubiquitylates VDAC2/3 to suppress erastin-induced ferroptosis in melanoma. Nat. Commun. 11 (1), 433. doi:10.1038/s41467-020-14324-x
Yao, B., Cai, Y., Wan, L., Deng, J., Zhao, L., Wang, W., et al. (2023). BACH1 promotes intervertebral disc degeneration by regulating HMOX1/GPX4 to mediate oxidative stress, ferroptosis, and lipid metabolism in nucleus pulposus cells. J. Gene Med. 25 (6), e3488. doi:10.1002/jgm.3488
Yasuma, T., Arai, K., and Yamauchi, Y. (1993). The histology of lumbar intervertebral disc herniation. The significance of small blood vessels in the extruded tissue. Spine (Phila Pa) 18(13), 1761–1765. doi:10.1097/00007632-199310000-00008
Ye, R. R., Chen, B. C., Lu, J. J., Ma, X. R., and Li, R. T. (2021). Phosphorescent rhenium(I) complexes conjugated with artesunate: Mitochondrial targeting and apoptosis-ferroptosis dual induction. J. Inorg. Biochem. 223, 111537. doi:10.1016/j.jinorgbio.2021.111537
Yi, W., Lan, H., Wen, Y., Wang, Y., He, D., Bai, Z., et al. (2020). HO-1 overexpression alleviates senescence by inducing autophagy via the mitochondrial route in human nucleus pulposus cells. J. Cell Physiol. 235 (11), 8402–8415. doi:10.1002/jcp.29684
Yu, H., Guo, P., Xie, X., Wang, Y., and Chen, G. (2017). Ferroptosis, a new form of cell death, and its relationships with tumourous diseases. J. Cell Mol. Med. 21 (4), 648–657. doi:10.1111/jcmm.13008
Yu, X., Xu, H., Liu, Q., Wang, Y., Wang, S., Lu, R., et al. (2022). circ_0072464 shuttled by bone mesenchymal stem cell-secreted extracellular vesicles inhibits nucleus pulposus cell ferroptosis to relieve intervertebral disc degeneration. Oxid. Med. Cell Longev. 2022, 2948090. doi:10.1155/2022/2948090
Yu, Y., Xie, Y., Cao, L., Yang, L., Yang, M., Lotze, M. T., et al. (2015). The ferroptosis inducer erastin enhances sensitivity of acute myeloid leukemia cells to chemotherapeutic agents. Mol. Cell Oncol. 2 (4), e1054549. doi:10.1080/23723556.2015.1054549
Yuan, H., Li, X., Zhang, X., Kang, R., and Tang, D. (2016). Identification of ACSL4 as a biomarker and contributor of ferroptosis. Biochem. Biophys. Res. Commun. 478 (3), 1338–1343. doi:10.1016/j.bbrc.2016.08.124
Yurube, T., Ito, M., Kakiuchi, Y., Kuroda, R., and Kakutani, K. (2020). Autophagy and mTOR signaling during intervertebral disc aging and degeneration. JOR Spine 3 (1), e1082. doi:10.1002/jsp2.1082
Zalyte, E., and Cicenas, J. (2022). Starvation mediates pancreatic cancer cell sensitivity to ferroptosis via ERK1/2, JNK and changes in the cell mesenchymal state. Int. J. Mol. Med. 49 (6), 84. doi:10.3892/ijmm.2022.5140
Zhang, C., Liu, X., Jin, S., Chen, Y., and Guo, R. (2022). Ferroptosis in cancer therapy: A novel approach to reversing drug resistance. Mol. Cancer 21 (1), 47. doi:10.1186/s12943-022-01530-y
Zhang, D. L., Wu, J., Shah, B. N., Greutélaers, K. C., Ghosh, M. C., Ollivierre, H., et al. (2018). Erythrocytic ferroportin reduces intracellular iron accumulation, hemolysis, and malaria risk. Science 359 (6383), 1520–1523. doi:10.1126/science.aal2022
Zhang, Q. X., Guo, D., Wang, F. C., and Ding, W. Y. (2020a). Necrosulfonamide (NSA) protects intervertebral disc degeneration via necroptosis and apoptosis inhibition. Eur. Rev. Med. Pharmacol. Sci. 24 (5), 2683–2691. doi:10.26355/eurrev_202003_20538
Zhang, T., Yang, M., Ma, C., Wei, X., and Zhang, Z. (2023). BACH1 encourages ferroptosis by activating KDM4C-mediated COX2 demethylation after cerebral ischemia-reperfusion injury. Eur. J. Neurosci. 58 (1), 2194–2214. doi:10.1111/ejn.16035
Zhang, X., Huang, Z., Xie, Z., Chen, Y., Zheng, Z., Wei, X., et al. (2020b). Homocysteine induces oxidative stress and ferroptosis of nucleus pulposus via enhancing methylation of GPX4. Free Radic. Biol. Med. 160, 552–565. doi:10.1016/j.freeradbiomed.2020.08.029
Zhang, Y., Han, S., Kong, M., Tu, Q., Zhang, L., and Ma, X. (2021). Single-cell RNA-seq analysis identifies unique chondrocyte subsets and reveals involvement of ferroptosis in human intervertebral disc degeneration. Osteoarthr. Cartil. 29 (9), 1324–1334. doi:10.1016/j.joca.2021.06.010
Zhao, K., An, R., Xiang, Q., Li, G., Wang, K., Song, Y., et al. (2021). Acid-sensing ion channels regulate nucleus pulposus cell inflammation and pyroptosis via the NLRP3 inflammasome in intervertebral disc degeneration. Cell Prolif. 54 (1), e12941. doi:10.1111/cpr.12941
Zhao, Y., Li, M., Yao, X., Fei, Y., Lin, Z., Li, Z., et al. (2020). HCAR1/MCT1 regulates tumor ferroptosis through the lactate-mediated AMPK-SCD1 activity and its therapeutic implications. Cell Rep. 33 (10), 108487. doi:10.1016/j.celrep.2020.108487
Zhou, B., Liu, J., Kang, R., Klionsky, D. J., Kroemer, G., and Tang, D. (2020). Ferroptosis is a type of autophagy-dependent cell death. Semin. Cancer Biol. 66, 89–100. doi:10.1016/j.semcancer.2019.03.002
Zhou, H., Li, N., Yuan, Y., Jin, Y. G., Guo, H., Deng, W., et al. (2018). Activating transcription factor 3 in cardiovascular diseases: A potential therapeutic target. Basic Res. Cardiol. 113 (5), 37. doi:10.1007/s00395-018-0698-6
Zhou, L. P., Zhang, R. J., Jia, C. Y., Kang, L., Zhang, Z. G., Zhang, H. Q., et al. (2022). Ferroptosis: A potential target for the intervention of intervertebral disc degeneration. Front. Endocrinol. (Lausanne) 13, 1042060. doi:10.3389/fendo.2022.1042060
Zhu, J., Sun, R., Sun, K., Yan, C., Jiang, J., Kong, F., et al. (2023a). The deubiquitinase USP11 ameliorates intervertebral disc degeneration by regulating oxidative stress-induced ferroptosis via deubiquitinating and stabilizing Sirt3. Redox Biol. 62, 102707. doi:10.1016/j.redox.2023.102707
Zhu, J., Sun, R., Yan, C., Sun, K., Gao, L., Zheng, B., et al. (2023b). Hesperidin mitigates oxidative stress-induced ferroptosis in nucleus pulposus cells via Nrf2/NF-κB axis to protect intervertebral disc from degeneration. Cell Cycle 22 (10), 1196–1214. doi:10.1080/15384101.2023.2200291
Zhu, Z. Y., Liu, Y. D., Gong, Y., Jin, W., Topchiy, E., Turdi, S., et al. (2022). Mitochondrial aldehyde dehydrogenase (ALDH2) rescues cardiac contractile dysfunction in an APP/PS1 murine model of Alzheimer's disease via inhibition of ACSL4-dependent ferroptosis. Acta Pharmacol. Sin. 43 (1), 39–49. doi:10.1038/s41401-021-00635-2
Zilka, O., Shah, R., Li, B., Friedmann Angeli, J. P., Griesser, M., Conrad, M., et al. (2017). On the mechanism of cytoprotection by ferrostatin-1 and liproxstatin-1 and the role of lipid peroxidation in ferroptotic cell death. ACS Cent. Sci. 3 (3), 232–243. doi:10.1021/acscentsci.7b00028
Keywords: intervertebral disc degeneration, ferroptosis, lipid peroxidation, antioxidant system, reactive oxygen species, epigenetics
Citation: Fan C, Chu G, Yu Z, Ji Z, Kong F, Yao L, Wang J, Geng D, Wu X and Mao H (2023) The role of ferroptosis in intervertebral disc degeneration. Front. Cell Dev. Biol. 11:1219840. doi: 10.3389/fcell.2023.1219840
Received: 12 May 2023; Accepted: 17 July 2023;
Published: 27 July 2023.
Edited by:
Xianwei Wang, Xinxiang Medical University, ChinaReviewed by:
Lian Xiang Luo, Guangdong Medical University, ChinaCopyright © 2023 Fan, Chu, Yu, Ji, Kong, Yao, Wang, Geng, Wu and Mao. This is an open-access article distributed under the terms of the Creative Commons Attribution License (CC BY). The use, distribution or reproduction in other forums is permitted, provided the original author(s) and the copyright owner(s) are credited and that the original publication in this journal is cited, in accordance with accepted academic practice. No use, distribution or reproduction is permitted which does not comply with these terms.
*Correspondence: Haiqing Mao, bWFvaHFAc3VkYS5lZHUuY24=; Xiexing Wu, d3V4aWV4aW5nQDE2My5jb20=; Dechun Geng, c3pnZW5nZGNAc3VkYS5lZHUuY24=
†These authors have contributed equally to this work
Disclaimer: All claims expressed in this article are solely those of the authors and do not necessarily represent those of their affiliated organizations, or those of the publisher, the editors and the reviewers. Any product that may be evaluated in this article or claim that may be made by its manufacturer is not guaranteed or endorsed by the publisher.
Research integrity at Frontiers
Learn more about the work of our research integrity team to safeguard the quality of each article we publish.