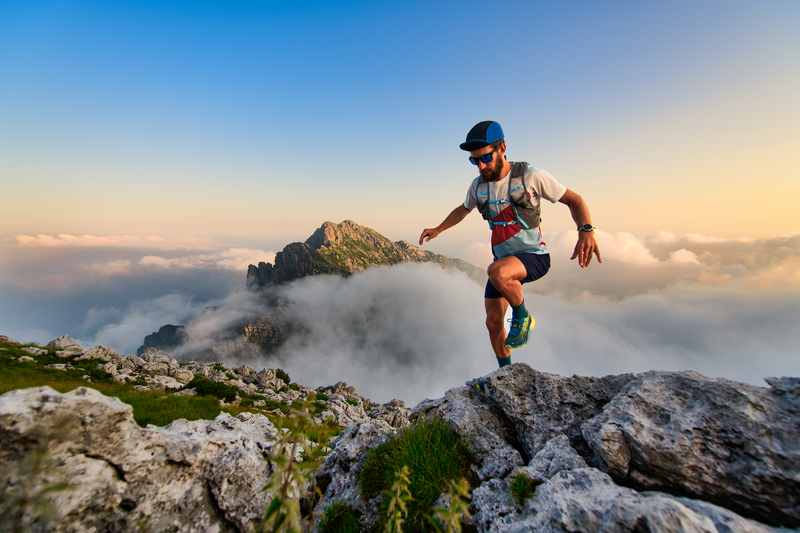
94% of researchers rate our articles as excellent or good
Learn more about the work of our research integrity team to safeguard the quality of each article we publish.
Find out more
REVIEW article
Front. Cell Dev. Biol. , 19 December 2023
Sec. Stem Cell Research
Volume 11 - 2023 | https://doi.org/10.3389/fcell.2023.1209047
This article is part of the Research Topic Recent Advancements in Musculoskeletal Regenerative Medicine View all 5 articles
The overall burden associated with musculoskeletal (MSK) conditions surpasses that of tobacco-related health effects, cancer, and diabetes (Barbour et al., 2017; United States Bone and Joint Initiative, 2020). Osteoarthritis (OA) is the most common degenerative joint disorder and a major worldwide challenge for health systems. OA is the leading cause of chronic disability among older adults (Anderson et al., 2011; Oo et al., 2021). It is a heterogenous condition with the pathogenesis differing across different joints. For example, obesity and meta-inflammatory processes have a significant role in knee OA while the shape of the joint and determinants of this are important in hip OA. Targeting the underlying pathological processes will be important in order to develop effective therapies. Post-traumatic OA (PTOA) develops after joint injury and is responsible for 12% of the cases of OA in the US. According to the US Bone and Joint Initiative (https://www.usbji.org), 62% of individuals with OA are women, being more prone to peripheral OA (hand, foot, knee) and experiencing more severe pain and disabling than men (Hawker, 2019; Leifer et al., 2022; Peshkova et al., 2022). Available pharmacological treatments only provide symptomatic pain relief and fail to arrest the progressive degeneration of cartilage associated with PTOA or idiopathic OA (Anderson et al., 2011; de Girolamo et al., 2016; de Girola et al., 2019). Surgical procedures that promote cartilage repair are expensive, require long rehabilitation, and might expose patients to the risk of complications. Thus, there is an imperative need to develop prophylactic novel biologic means that either prevent or treat early onset of the disease (Vincent, 2019; Vincent, 2020; Vincent et al., 2022).
The current management of OA is gradual and depends on the symptomatic evolution of the disease. Initially, a non-pharmacological approach can be proposed, mainly based on weight loss and the introduction of an adapted physical activity, accompanied by a general awareness of the patient to a healthy lifestyle (Skou and Roos, 2019). If insufficient symptom improvement is achieved with non-pharmacological approaches, pharmacological treatments using non-steroidal anti-inflammatory drugs (NSAIDs) are proposed to reduce pain and inflammation (Bannuru et al., 2019). Although their long-term efficacy and safety are still controversial, intra-articular injections of corticosteroids or hyaluronic acid, or a combination of both, are also being performed to improve the symptoms in patients who did not respond to conventional routes of analgesic administration (Fusco et al., 2021; Peck et al., 2021). Moreover, with the advent of orthobiologics, new potential therapeutic approaches are being exploited to preserve and, potentially restore, the articular surface (Kon et al., 2020; Kon and Di Matteo, 2021; Anzillotti et al., 2022). The group of S. Chubinskaya* has extensively studied the mechanisms of PTOA and the role of orthobiologics in cartilage repair and regeneration and successfully contributed to the clinical approval of the Agili-C implant (Anderson et al., 2011; de Girola et al., 2019). However, no drug can restore joint integrity or even stop the mechanisms leading to the development of OA and partial or total joint replacement by a prosthesis is considered for end-stage OA. Currently, 2,127 clinical trials related to OA (https://clinicaltrials.gov, accessed on 15 April 2023 under “OA+ drugs + interventional + female”) are underway to identify disease-modifying OA drugs (DMOADs) (Dietz et al., 2021; Kennedy et al., 2022). Of those expected to have clinical value, sprifermin and lorecivivint (SM04690) recently entered phase II clinical trials. Still, despite encouraging preliminary results in preclinical models (Deshmukh et al., 2018; Deshmukh et al., 2019), these clinical trials showed mitigated results on the global population studied, with encouraging results obtained in one subtype of patients (Yazici et al., 2020; Li et al., 2021).
Women have had a significant role in the translation of stem cell studies to clinical trials including for OA. This required the development of measurement tools to assess progression of structural disease in OA. That cartilage loss can be detected in a valid and reproducible way over 2 years was first shown using magnetic resonance imaging (MRI) by Wluka et al. (Wluka et al., 2004). This method was shown to detect the clinically important outcomes of pain (Wluka et al., 2004) and knee replacement (Cicuttini et al., 2004), meaning that it was now possible to use this method for proof of concept trials in OA. This has led to the testing of stem cells in those at high risk of OA, such as knee PTOA, with favorable results (Wang et al., 2017). The trials in this area have been summarized in a number of systematic reviews (Gong et al., 2021). These have tended to find consistent evidence for a beneficial effect of intra-articular injections of stem cells on articular cartilage and subchondral bone, irrespective of the source or contents of the stem cells. However, among the trials there remains significant heterogeneity in the source and composition of the injected cells, small to modest sample sizes, and the potential for publication bias. The use of stem cells remains an exciting area of endeavor (Fortier, 2005), including for OA where there is a lack of effective therapies. However, more work is needed before such therapies can be recommended in the management of the pathology.
In parallel with ongoing human studies, stem cells have been employed in veterinary medicine (Fortier and Travis, 2011; Ferris et al., 2014; Colbath et al., 2020) and the European Drugs Regulations Agency already authorized the use of two products based on stem cells (Arti-Cell Forte® and HorStem®) to treat mild to moderate signs of OA associated with lameness in horses, following various safety and efficacy studies of intra-articular injections (Bertoni et al., 2019; Broeckx et al., 2019; Be et al., 2021). As for humans, MSK injuries are the most prevalent and debilitating condition responsible for pain (in the form of lameness) and loss of performance associated with highly negative economic impact on the equine industry (IVIS, 2010). A wider range of therapies are used in equine practice compared with human practice to reduce the clinical signs and progression of OA (Velloso Alvarez et al., 2020), although there is still no curative treatment. In a “One Health, One Medicine” concept (Kahn, 2017), preclinical studies in horses led to applications in humans and vice versa, as recently illustrated by the marketing of the intra-articular polyacrylamide hydrogel (Arthrosamid®) or of autologous conditioned serum (ACS) (Camargo Garbin and Morris, 2021). Large animals including livestock, dogs or horses have similar biomechanical constraints to humans, leading to the development of MSK diseases in a similar pathogenesis (Yazici et al., 2020). Therefore, they are considered suitable target species and translational models for researching new therapeutic approaches, while small animal models are typically employed for investigating pathophysiological processes (McCoy, 2015; Manivong et al., 2023). Horses in particular demonstrated many similarities to human joints regarding articular cartilage thickness, cellular structure, biochemical composition, and mechanical properties. It is however interesting to note that in major international equestrian competitions and races, all genders compete in the same category and females achieve great victories (Hanousek et al., 2018).
This opinion paper focuses on the manipulation of mesenchymal progenitor cells as a promising therapeutic source of cells to treat MSK disorders with a focus on OA. This initiative was established by women researchers, with the purpose of highlighting some of the critical women’s contributions in the field of MSK tissue regeneration (including those of the current authors) as examples (Figure 1) with particular attention drawn in the text on outstanding women and on women (*) involved in the current opinion paper, and was performed under the umbrella of the International Cartilage Regeneration and Joint Preservation Society (ICRS; https://cartilage.org).
FIGURE 1. Women Input on Stem Cells for MSK Regeneration (initials in pink indicate the research contributions of the current authors).
The limb forms during embryogenesis as a limb bud, from the condensation of mesenchymal progenitor cells that chondrogenically differentiate into and form a cartilage anlage. This cartilage anlage will undergo endochondral ossification to form long bones, except at specific sites where an interzone is formed to give rise to the joint structures (Decker et al., 2014). The initial interzone consisting of growth and differentiation factor (Gdf)5+ cells generated from descendants of chondrocytes which after cavitation will form the articular cartilage and intra-articular ligaments. Flanking cells recruited into the interzone from surrounding tissues also express Gdf5 and will largely form the synovial lining and joint capsule. In adult mouse synovium, cells that originate from Gdf5-expressing joint interzone cells appear largely negative for the skeletal stem cell markers Nestin, Leptin receptor, and Gremlin1 (Roelofs et al., 2017), confirming a separate origin of these progenitor cells in synovium and skeletal progenitor cells. With different approaches, Decker et al. and Roelofs et al. demonstrated that upon osteochondral injury in adult mice, progenitor cells in the synovium proliferate and are recruited to the defect to contribute to tissue repair and articular chondrocytes have minimal contribution to tissue repair (Decker et al., 2017; Roelofs et al., 2017).
Although initially referred to as stem cells, the definition of MSCs has been under discussion over the last decades (Viswanathan et al., 2019). The term “MSCs” is interchangeably used for Mesenchymal Stem Cells, Mesenchymal Stromal Cells, Medicinal Signaling Cells, or Multipotent Stromal Cells, and for the most part in connection to a potential clinical use. In this review, we use the term “MSCs” as such and the exact meaning refers to the context of their potential clinical use: either to generate tissue or to modulate tissue repair (Viswanathan et al., 2019). Distinct progenitor cells are present in the different joint tissues but it became clear that also within one tissue, different populations of progenitor cells exist. Generally, one can discriminate perivascular progenitor cell populations and populations located in the stroma or the lining. In the human synovium, the group of G. J. V. M. van Osch* (Sivasubramaniyan et al., 2019) described progenitor cells that are CD45−CD31−CD73+ and discriminated a CD90− population in the intimal, synovial lining layer and a CD90+ population in the subintimal, perivascular area with distinct chondrogenic capacities. Similarly, synovial-derived MSCs have been reported in animal species by this group* (Teunissen et al., 2022). Moreover, as reported by this group in collaboration with the groups of E. Jones* (Sivasubramaniyan et al., 2018), in the human bone marrow, two different populations of CD45−CD271+ mesenchymal progenitor cells were found: a perivascular CD56− population with poor chondrogenic capacity and a CD56+ bone lining population with good chondrogenic capacity. In bone marrow aspirations, the less chondrogenic perivascular population is more abundant than the bone lining population. A higher abundance of the more chondrogenic bone lining populations was found when the bone marrow was collected after rasping the marrow canal before implanting a prosthesis (Sivasubramaniyan et al., 2018). Bone marrow-derived (BM) MSCs have also been isolated from animals (Fortier et al., 1998; Kisiday et al., 2020). Thus, the location and way of collecting MSCs is an important determinant for their capacity to generate tissues.
Subcutaneous adipose tissue has gained popularity as a source of MSCs more recently compared with bone marrow. Nevertheless, many research efforts have addressed it as an optimal tissue source for a variety of clinical applications, as well as for an impressive number of basic studies to clarify MSC properties. Adipose tissue is indisputably a convenient tissue to harvest, with potentially no limit in terms of quantity and it contains a higher number of MSCs (adipose-derived stem cells, i.e., ASCs) with a similar immunophenotype to BM MSCs, bar the higher expression of CD34 seen in freshly isolated ASCs as shown by the groups of F. J. van Milligen and of D. Noël* (Varma et al., 2007; Maumus et al., 2013). Also, adipose tissue-derived MSCs have been reported by the group of H. Roelofs (Melie et al., 2013) to possess a higher immunomodulatory capacity than their bone marrow counterparts, making ASCs a more appealing cell type compared with BM MSCs in some clinical applications. ASCs are associated with the so-called stromal vascular fraction (SVF), a heterogeneous collection of cells composed of pre-adipocytes, endothelial precursor cells, T regulatory cells, macrophages, smooth muscle cells, and MSCs/pericytes indeed. Nevertheless, the SVF is a versatile cellular system and the degree of heterogeneity depends on a variety of factors, such as the adipose tissue harvest site, the digestion protocol, and the patient’s own pathological status. For this reason, there is no consensus on the exact definition and proportion of these cell types within the SVF. Also, culturing SVF cells for even one passage can profoundly alter their cellular composition as reported by Nunes et al. (Nunes et al., 2013) and observed by the group of D. Noël* (Domergue et al., 2016). From a therapeutic perspective, beyond exploiting the adipose tissue for the isolation and in vitro expansion of ASCs, the relatively higher abundance of MSCs within the adipose tissue has driven the development of several medical devices to isolate the SVF intra-operatively without the need for further cell expansion. These devices can either provide mechanical digestion of the adipose tissue resulting in a mechanical-SVF as reported by the group of Y. Kul (Tiryaki et al., 2022) and observed by the group of D. Noël* (Bony et al., 2016) or resize the adipose tissue into microfragments while removing oil and blood contaminants and resulting into microfragmented adipose tissue as shown by the group of L. de Girolamo* (Ulivi et al., 2022). Notably, in addition to the subcutaneous adipose tissue, other adipose sites have been investigated as MSC sources. In particular, in the MSK field ASCs isolated from the infrapatellar fat pad (or Hoffa’s body) have been demonstrated to possess a differentiation ability equal or even superior to subcutaneous ASCs (Lopa et al., 2014; Sun et al., 2018).
MSCs have additionally been found in healthy and OA synovial fluid (SF) by the group of E. Jones* (Jones et al., 2004; Jones et al., 2008) and in patients with chondral defects by G. J. V. M. van Osch* and K. Wright (Garcia et al., 2020). Compared with BM MSCs, SF MSCs are highly proliferative and consistently chondrogenic (Jones et al., 2008). In OA human knees, as shown in the work of the group of E. Jones* (Ilas et al., 2019; Sanjurjo-Rodriguez et al., 2019), subchondral BM MSCs are driven towards osteogenesis in order to rapidly compensate for load distribution alterations following the loss of cartilage. In contrast, they showed that SF MSCs from the same knees express higher levels of cartilage formation and turnover genes, and lower levels of ossification molecules (Sanjurjo-Rodriguez et al., 2020). Furthermore, SF MSCs display a pro-chondrogenic and immunomodulatory response following a joint-sparing, knee joint distraction procedure (Sanjurjo-Rodriguez et al., 2020). Owing to their apparent ability to respond to biomechanical cues, both types of joint-resident MSCs can be manipulated towards cartilage regeneration using mechanically-competent scaffolds and smart biomaterials.
MSCs have also been isolated from the articular cartilage by the group of L. A. Vonk (Rikkers et al., 2021; Rikkers et al., 2022) and peripheral blood (PB-MSCs), both showing a certain ability for osteochondrogenic differentiation (Mödder and Khosla, 2008; Beane and Darling, 2012; Martinello et al., 2013; Orth et al., 2014; Frisch et al., 2015a; Rikkers et al., 2021; Rikkers et al., 2022). Other sources of MSCs include perinatal MSCs from the umbilical cord blood or connective tissue or from the amniotic fluid and membrane (El Omar et al., 2014; Gómez-Leduc et al., 2016; Borghesi et al., 2019; Lepage et al., 2019; Hyland et al., 2020; Perry et al., 2021) as well as embryonic stem cells (ESCs) (Hwang et al., 2008), all with chondrogenic abilities and showing a higher potential for self-renewal and lower immunogenic and tumorigenic activities relative to adult MSCs. Of further note, as shown by the groups of É. Velot*, of J. Elisseeff, and of S. J. Kimber and by Lepage et al. and Borghesi et al. (Hwang et al., 2006; Oldershaw et al., 2010; El Omar et al., 2014; Dostert et al., 2017; Borghesi et al., 2019; Lepage et al., 2019; Mesure et al., 2019; Velot et al., 2021), MSCs from birth tissues have a better accessibility and can be non-invasively isolated in large amounts relative to adult MSCs while showing fewer ethical issues compared with ESCs.
The number of MSCs that can be obtained from a donor is limited. ESCs and induced pluripotent stem cells (iPSCs) represent a potentially unlimited source of chondrocytes. Protocols from ESCs have been adapted for iPSCs with slight variations. These stem cells can be used to specifically generate hypertrophic or non-hypertrophic chondrocytes as shown in particular by the groups of S. J. Kimber, of R. A. Kandel, of S. Diederichs, and of W. Richter (Oldershaw et al., 2010; Craft et al., 2013; Cheng et al., 2014; Diederichs and Tuan, 2014; Diederichs et al., 2019a). Non-hypertrophic chondrocytes generated from human ESCs have been reported to promote the repair of focal cartilage defects in rats (Cheng et al., 2014). Hypertrophic chondrocytes are generally generated from iPSCs by first differentiating them into iPSC-derived MSC-like progenitor cells (iMPCs). iMPCs generated from human BM MSCs were comparable to the parental MSCs, although iMPCs appear less responsive to traditional MSC differentiation protocols (Diederichs and Tuan, 2014). The considerable heterogeneity in chondrogenesis found using iMPCs has been associated with variable SRY-related high-mobility-group-box gene 9 (SOX9) protein expression, with low SOX9 levels correlating to high levels of the SOX9-antagonizing hsa-miR-145 (Diederichs et al., 2016). Still, the clinical use of iPSCs remains controversial since these cells are potentially tumorigenic and immunogenic, with an instability of their genome (Hackett and Fortier, 2011; Guzzo et al., 2013; Schnabel et al., 2014; Vonk et al., 2015; Driessen et al., 2017; Xu et al., 2019; Velot et al., 2021). However, the secretome of iPSC-derived MSCs (iMSCs) primed with tumor necrosis alpha (TNF-α) and interferon gamma (IFN-γ) has a high resemblance to BM MSCs, with iMSC-derived extracellular vesicles (EVs) showing similar in vitro immunomodulation (Ramos et al., 2022).
Murphy* et al. were the first to intra-articularly inject BM MSCs and study their potential for the treatment of OA using a caprine model (Murphy et al., 2003). Many studies in animals and in human patients followed. Since cell tracking studies showed only limited cartilage formation by these cells (Murphy et al., 2003; Hamilton et al., 2019; Markides et al., 2019; Perry et al., 2021), the mechanism of action was hypothesized to be related to factors secreted by the MSCs (Hamilton et al., 2019; Markides et al., 2019; Perry et al., 2021). Given their established immunomodulatory and trophic properties, MSCs isolated from different sources have been proposed as a viable therapeutic option to treat cartilage damage and early OA (Lopa et al., 2019). The group of D. Noël* noted the therapeutic efficiency of human and equine ASCs in the inflammatory model of collagenase-induced OA (Ter Huurne et al., 2012; Maumus et al., 2016). Intra-articular injection of immune-selected allogeneic human mesenchymal precursor cells were reported by the group of F. Cicuttini* to improve symptoms and structure in PTOA, suggesting that they modulate some of the pathological processes responsible for the onset and progression of this phenotype (Wang et al., 2017). These cells help to establish a “regenerative microenvironment” through the paracrine secretion of bioactive molecules and promote tissue-specific progenitor proliferation while inhibiting cell apoptosis and tissue fibrosis as reported by the group of L. de Girolamo* (Colombini et al., 2019). Among the molecules that play a role in the chondroprotective effect of MSCs in OA, Fra-1, THBS1 and TGFβi have been noted by the group of D. Noël* (Schwabe et al., 2016; Maumus et al., 2017; Ruiz et al., 2020). Alternatively to MSCs, tissue-specific cells have been also proposed as a valuable regenerative alternative for decades. Autologous Chondrocyte Implantation or Transplantation (ACI/ACT) was first developed in the 90s and since then has been used to treat thousands of patients with satisfactory results compared with other available techniques (Kon et al., 2013). Over time, a number of implementations of the original techniques have been developed, including the association of suitable biomaterials acting as scaffolds for cell growth and colonization (MACI, matrix-assisted autologous chondrocyte implantation) as shown by the large case series published by the group of E. Kon* (Kon et al., 2012). Nevertheless, while ACI/MACI resulted in the successful treatment of focal isolated chondral injuries, the clinical outcomes in patients with diffuse chondral damages are still under debate (Colombini et al., 2022a). One of the possibilities to make these techniques more effective in patients with diffuse cartilage defects (i.e., early OA patients) is to focus on chondroprogenitors. Chondroprogenitors are represented within cartilage as cells with migratory, clonogenic ability, and differentiation potential, found both in healthy and damaged cartilage as reported by Vinod et al. (Vinod et al., 2023). In addition to their direct stimulation within the cartilage, cartilage progenitors, likewise MSCs, could indeed be exploited to improve the existing cell-based therapies for the treatment of cartilage defects. In this regard, the possibility to enrich the amount of cartilage progenitors throughout in vitro expansion of the whole cartilage cell population seems a valuable option to improve the current results in early OA patients. It was observed that, with increasing passages in culture, cartilage cell populations are characterized by a progressive enhancement of clonogenic ability and sustained expression of stemness markers. Moreover, these expanded cells revealed a noteworthy chondrogenic potential, an enhanced secretory response to inflammation compared with MSCs, and strong immunomodulatory functions after inflammatory priming as seen by De Luca et al. (De Luca et al., 2019). Although data derived from animal models also show that chondroprogenitors have the ability to attenuate OA, repair, chondral defects, and form stable cartilage, displaying better outcomes than BM MSCs, these preclinical data would require further studies to optimize their use before clinical translation (Vinod et al., 2023).
Another possibility would be to combine the favorable properties of chondrocytes and MSC into a combined treatment. Apart from anti-inflammatory or anti-catabolic effects, the secretome of MSCs can also have trophic effects and in co-culture with chondrocytes, both adipose tissue-derived and BM MSCs demonstrated to enhance cartilage generation by the chondrocytes as reported by the group of G. J. V. M. van Osch* (Pleumeekers et al., 2018). This property of MSCs might explain the potential of a proposed one-stage cell therapy for cartilage defects where 75% of the chondrocytes can be replaced by MSCs (US20140329316A1, US20100144036A1), although the initial claim referring to an effect of the chondrocytes on the differentiation of the MSCs cannot be fully excluded.
MSCs can be differentiated into cartilage-like cells in vitro. A large body of research from the groups of W. Richter and of G. J. V. M. van Osch* have investigated the stability of the cartilage formed by these cells and ways to improve this. They demonstrated that chondrogenically differentiated MSCs, though, were shown to be prone to endochondral ossification when implanted in vivo (Pelttari et al., 2006; Farrell et al., 2009). This finding redirected the bone tissue engineering field since more bone could be generated by pre-differentiating MSCs chondrogenically than the original attempts of pre-differentiating them towards the osteogenic lineage. Whereas research mostly focused on stimulating the chondrogenic capacity of these cells, such as, for example, by addition of parathyroid hormone-related protein (PTHrP) (Fischer et al., 2014; Fischer et al., 2016), TGFβi, NMB, or miR-29a as noted by the group of D. Noël* (Ruiz et al., 2020; Maumus et al., 2021; Guérit et al., 2014), inhibition of the anti-chondrogenic regulators became an emerging area (Lolli et al., 2019a). Inhibition of SMAD1/5/9 (Helli et al., 2011), WNT (Narcisi et al., 2015; Diederichs et al., 2019b), or anti-chondrogenic miRNAs, such as, for example, miRNA221 (Lolli et al., 2016) and miR-574–3p as observed by the group of D. Noël* (Guérit et al., 2013), has shown promise to inhibit hypertrophic differentiation. In addition to modulating chondrogenesis during the differentiation phase, modulation of the MSC expansion medium, for example, by the addition of WNT (Narcisi et al., 2015) or of TNF-α (Voskamp et al., 2020) resulted in improvement of chondrogenic capacity. The fact that these factors have demonstrated anti-chondrogenic properties during the differentiation phase demonstrates the complexity and importance of paying to the different stages of cell differentiation.
The immunomodulatory capacity of MSCs and ASCs can be influenced by disease status of the joint as noted by the groups of G. J. V. M. van Osch* and of G. Lisignoli* (Leijs et al., 2012; Manferdini et al., 2020). The immunomodulatory ability of ASCs was not affected by hypoxia as reported by the group of G. J. V. M. van Osch* (Roemeling-van Rhijn et al., 2013), whereas it was positively affected by an inflammatory stimulus such as interleukin 1 beta (IL-1β) as observed by the groups of L. de Girolamo* and of D. Noël* (Colombini et al., 2022b; Maumus et al., 2016). Similarly, the protein cargo of umbilical cord EVs was affected by their pro-inflammatory priming, but not in hypoxic conditions (Hyland et al., 2020).
Stem cells are amenable to genetic modification to enhance their reparative potential in a variety of MSK disorders. Gene therapy is the transfer of candidate nucleic acid sequences as direct molecules or using a gene vector derived from nonviral material (most commonly a plasmid) or from viral material (adenoviral [AV], herpes simplex viral, retro-/lentiviral [LV], or recombinant adeno-associated viral [rAAV] vectors) to prolong the therapeutic effects of a gene product compared with recombinant agents with short pharmacological half-lives (Jorgensen et al., 2001; Noël et al., 2002; Jorgensen et al., 2003; Djouad et al., 2009; van Osch et al., 2009; Vinatier et al., 2009; Coleman et al., 2010; Ansboro et al., 2012; Cucchiarini et al., 2012; Demoor et al., 2014; Frisch et al., 2015a; Docheva et al., 2015; Balmayor, 2015; Cucchiarini, 2016; Fris et al., 2016; Jones et al., 2016; Rey-Rico and Cucchiarini, 2017; Poh et al., 2018; Mesure et al., 2019; Roseti et al., 2019; De la Vega et al., 2021; Amini et al., 2022). Gene therapy is performed via classical gene transfer or using genome editing methodologies such as use of the clustered regularly interspaced short palindromic repeats (CRIPSR)-associated 9 (CRISPR/Cas9) system (Tanikella et al., 2020). The contribution of women researchers in the field of gene therapy to target stem cells is broadly illustrated by reports showing the improved commitment of stem cell to MSK profiles using various classes of gene transfer vectors. Nonviral vectors carrying the SOX9 transcription factor were reported by the group of A. Rey-Rico to activate MSC chondrogenesis (Carball et al., 2022) (or the vascular endothelial growth factor - VEGF - to tackle MSC osteogenesis and bone healing as noted by the group of W. Richter (Geiger et al., 2007)). Adenoviral vectors carrying IL-10 were successfully used by the group of M. Murphy* to prevent OA (Farrell et al., 2016) (or bone morphogenetic proteins - BMP-2, BMP-6 - to modulate MSC osteogenesis and bone healing as reported by the group of A. L. Bertone (Zachos et al., 2006; Zachos et al., 2007; Murray et al., 2010; Ishihara et al., 2015)). rAAV vectors were manipulated by the groups of C. R. Chu, of L. R. Goodrich, and of M. Cucchiarini* to deliver the basic fibroblast growth factor (FGF-2) (Cucchiarini et al., 2005; Cucchiarini et al., 2011), transforming growth factor beta (TGF-β) (Pagnotto et al., 2007; Lee et al., 2011; Frisch et al., 2014a; Frisch et al., 2016; Frisch et al., 2017a; Cucchiarini et al., 2018), insulin-like growth factor I (IGF-I) alone (Frisch et al., 2014b; Cucchiarini and Madry, 2014; Frisch et al., 2015b; Frisch et al., 2017b) or with TGF-β (Morscheid et al., 2019a; Morscheid et al., 2019b), BMP-3 (Venkatesan et al., 2022), SOX9 (Cucchiarini et al., 2013), and chondromodulin (Klinger et al., 2011) to stimulate MSC chondrogenesis and cartilage repair (or BMP-2 for MSC osteogenesis (Ball et al., 2019)). Gene silencing in MSCs based on gene knockdown strategies using RNA interference (RNAi) technology is also a potent approach to modulate the reparative potential of these cells for MSK regeneration (Lolli et al., 2017; Demoor et al., 2014). For instance, silencing the antichondrogenic regulator microRNA 221 (miR-221) via specific antagomiR-221 or antimiR-221 oligonucleotides is capable of enhancing chondrogenesis and cartilage repair as seen in work from the group of G. J. V. M. van Osch* (Lolli et al., 2016; Lolli et al., 2019b).
Adenoviral- and rAAV-based vectors, while considered the most promising systems for OA, still have two main limitations: potential anti-viral host immune responses and the levels of efficiency and specificity of transgene expression in joint tissues. Targeting the joint cells of interest is still arduous even using intra-articular injections. Indeed, chondrocytes embedded in a dense extracellular matrix (ECM) are less accessible than synovial, ligament, or fat pad cells. In addition, disturbances in intracellular trafficking may decrease the efficiency of viral vector transduction. To address these issues, optimization of the carrier nucleic acid sequence and a modification of viral vectors have been considered with increasing interest. The capsids of adenoviral and rAAV vectors promote tropism but can also be recognized by neutralizing antibodies present in the circulation and synovial fluid as evidenced by the group of N. Bessis (Cottard et al., 2004) due to a pre-existing immunity, overall reducing the transduction efficiency. Yet, capsids may be engineered to escape such neutralization, and in this context, several improved hybrid AAV serotypes have emerged. Among them, the rAAV 2.5, a chimera of AAV1 and AAV2, evaluated in preclinical models of equine OA by Watson-Levings et al. (Watson Levings et al., 2018a) is currently being used in a clinical trial for OA (NCT05454566) and rAAV-DJ, which has been successfully used in a preclinical rat model of OA by Martinez-Redondo et al. (Martinez-Redondo et al., 2020). Also of interest in OA, Eichhoff et al. (Eichhoff et al., 2019) designed an rAAV preferentially targeting cells expressing the purinergic receptor (P2X7R) overexpressed in OA chondrocytes. Chemical modification allowing a large panel of chemical compounds or peptides to be coupled may also improve capsids to limit off-target effects, overcome undesirable properties, and permit viral escape from neutralizing antibodies. For instance, the chondrocyte-affinity peptide (CAP) has been used by the group of P. Pothacharoen (Chongchai et al., 2023) to enhance rAAV targeting of chondrocytes. Finally, self-complementary AAV (scAAV) composed of a double-stranded DNA genome have been successfully used by Watson-Levings et al. (Watson Levings et al., 2018a; Watson Levings et al., 2018b) to target chondrocytes, bypassing their step-limiting, low rates of cell replication/proliferation.
Despite the clear advantages of gene therapy, safety concerns related to viral vectors and affordability issues have restricted their clinical use as noted by the group of E. R. Balmayor* among others (Evans, 2019; De la Vega et al., 2021) In fact, affordability remains a pressuring issue, with gene therapy products tagged at $2 million a dose (Dyer, 2020) related to the manufacturing process under Good Manufacturing Practice (GMP). In addition, achieving efficient gene transfer and appropriate levels of transgene expression proved to be cumbersome for some applications as described by the group of E. R. Balmayor* (De la Vega et al., 2021), motivating researchers to explore alternatives to traditional MSK gene therapy. Applied to tissue regeneration, gene therapies commonly deliver protein-coding DNA. However, messenger RNA (mRNA), offers a more efficient way to achieve protein translation while accommodating numerous advantages as explained by Balmayor* et al. (Balmayor, 2022). Like traditional gene therapy, mRNA transcript therapy offers control over the production of therapeutic proteins. In addition, since mRNA function is conducted in the cell cytoplasm, it results in an immediate initiation of translation. Unlike viral gene therapy, mRNA transcript therapy is safe as it does not carry the risk of insertional mutagenesis and oncogenesis. Also, mRNA’s transient nature is valuable in many regenerative medicine applications when long-term effects are not necessary as stated by others and by Balmayor* et al. (Sahin et al., 2014; Balmayor and Evans, 2019). mRNA is produced by in vitro transcription (IVT), a relatively simple, single-reaction procedure easily controllable and scalable and identical for all mRNAs, regardless of the sequence. Importantly, no cell or bacterial culture is required, reducing the risk of contamination. IVT allows the introduction of numerous chemical modifications and the use of modified nucleosides to produce defined mRNAs with reduced immunogenicity and low stability, with costs described as up to 10-fold lower that their protein therapeutic counterparts as demonstrated by K. Karikó among others (Karikó et al., 2005; Weissman, 2015). The recent use of mRNA in COVID-19 vaccines receiving approval in various countries demonstrates that this technology can be safe and efficient. Regarding MSK regeneration, local mRNA delivery has been investigated mostly for bone by the group of E. R. Balmayor* and by Badieyan et al. and Khorsand et al. (Elangovan et al., 2015; Badieyan et al., 2016; Balmayor et al., 2016; Balmayor et al., 2017; Khorsand et al., 2017; Zhang et al., 2019; Fayed et al., 2021; Geng et al., 2021; De La Vega et al., 2022; European Scoiety of Gene and Cell Therapy, 2022), with a strong focus on effective BMP-2 mRNAs but also with an emerging interest for BMP-9 (Khorsand et al., 2017), BMP-7 (European Scoiety of Gene and Cell Therapy, 2022), and VEGF mRNAs by Geng et al. (Geng et al., 2021). In one of the most translatable studies performed to date, a BMP-2 mRNA administered to rat femoral critical-size defects via a collagen carrier was reported by the group of E. R. Balmayor* (De La Vega et al., 2022) to remain local in a safe manner and to efficiently induce bone healing in a dose-dependent manner. For cartilage repair, mRNAs coding for the runt-related transcription factor 1 (Runx1) (Aini et al., 2016), Link N by the group of M. Avci-Adali (Tendulkar et al., 2019), and IGF-I (Wu et al., 2022) have been investigated as OA treatments. Direct injections of Runx1 mRNA in mouse OA knee joints significantly suppressed OA progression (Aini et al., 2016) while injection of stem cells transfected with an IGF-I mRNA in mouse knee joints safely enhanced cell survival and engraftment in the cartilage tissue (Wu et al., 2022). Overall, all these findings support the safety and efficacy of this emerging technology for MSK regeneration.
New tissue engineering therapeutic strategies mainly based on the use of biomaterials combined with stem cells showed good potential both in preclinical and clinical studies as reported by the groups of G. Lisignoli*, of D. Noel* and of L. R. Goodrich and by Nooeaid et al., Moradi et al., Taraballi et al., Armiento et al., and Kwon et al. (Nooeaid et al., 2012; Moradi et al., 2017; Taraballi et al., 2017; Yang et al., 2017; Armiento et al., 2018; Kwon et al., 2019; Pascual-Garrido et al., 2019; Trucco et al., 2021, Valot et al., 2021; Manferdini et al., 2022). Regenerative scaffold-based methods are emerging as possible therapeutic alternatives for the treatment of various types of cartilage lesions as noted by the group of E. Kon* (Kon et al., 2015). In order to support the proliferation of live cells, a scaffold is a temporary three-dimensional (3D) framework made of biodegradable polymers that may replicate the highly structured functional architecture of the articular cartilage. Chondrogenic differentiation of MSCs can be achieved on different types of scaffolds provided that a growth factor can be released to induce the process as observed by the group of D. Noël* (Morille et al., 2013; Morille et al., 2016; Mathieu et al., 2014). Different types of chondral and osteochondral scaffolds have been used in the past, but the most promising results have being obtained with biodegradable scaffolds as observed by the group of E. Kon* (Kon et al., 2014; Kon et al., 2018; Kon et al., 2021; Altschuler et al., 2023). The use of scaffolds is often integrated with cells seeded on the scaffold itself. The group of E. Kon* (Kon et al., 2008; Kon et al., 2012) was the first to use articular chondrocytes in association with biomaterials for cartilage regeneration in clinical practice with discrete success in treating focal defects. Although some products containing chondrocytes and biomaterials are still on the market, the necessity of addressing different tissues and the need of modulating the joint environment pushed towards the use of stem cells instead of chondrocytes.
Both natural and synthetic biomaterials have been used to regenerate different joint tissues (cartilage, bone, meniscus) and cells have been mainly seeded or encapsulated into these structures for instance by Sanchez-Tellez et al., Yang et al., and Critchley et al. (Sánchez-Téllez et al., 2017; Li et al., 2019; Yang et al., 2019; Critchley et al., 2020; Rahman et al., 2022). However, the main limitation of this approach is the retention of stem cells in the target joint tissue that may be influenced by the presence of synovial fluid, inflammation, load, and joint movement as noted by the groups of E. Kon* and of G. J. V. M. van Osch* and by Bakhshandeh et al. (Fahy et al., 2014; Perdisa et al., 2014; Bakhshandeh et al., 2017; Dong et al., 2017). To overcome this issue, it is necessary to design advanced biomaterials capable of retaining cells in situ, with immunomodulating properties while at the same time, retaining the capacity to stably adhere to damaged joint tissues and boost their regeneration. Biomaterial stiffness and viscoelasticity, as other critical parameters, are selected depending on the anatomical characteristics of the regenerated joint tissues as reported for instance by Sarem et al. (Sarem et al., 2018; Zhang et al., 2020; Huang et al., 2023). All biomaterial parameters directly control and influence the characteristics of the cells by acting through specific receptors directing their migration, proliferation, matrix synthesis, and differentiation as observed by the group of G. Lisignoli* and by Yang et al., Sarem et al., and Hafezi et al. (Sarem et al., 2018; Yang et al., 2019; Hafezi et al., 2021; Sartore et al., 2021). These strategies contribute to joint tissue regeneration by showing effects on different physiological processes including inflammation, metabolic processes, aging, apoptosis, and autophagy as shown by the groups of E. R. Balmayor* and of G. Lisignoli* and by Koh et al. (Amann et al., 2017; Chen et al., 2020; Koh et al., 2020; Gabusi et al., 2022).
Stem cells have also been reported to secrete an ECM that may be valuable for MSK regeneration. For instance, MSCs from the connective tissue of the umbilical cord (Wharton’s jelly) produce ECM components similar to those of articular cartilage as reported by Russo et al. (Russo et al., 2022) and participate in MSC chondrogenic differentiation, offering a potential scaffold that may be used as an alternative to improve cartilage regeneration as noted by Ramzan et al. (Ramzan et al., 2022).
Emerging technologies like additive manufacturing (three-dimensional [3D] printing) will lead future directions for tissue engineering therapeutic strategies. 3D printing replicates the damaged tissue shape starting from the patient medical image. It consists of the fabrication of living tissue/organ-like structures throughout the bottom-up deposition of either cell-laden droplets or cells embedded in a hydrogel, in both cases termed as “bioink” as described for instance by Abdollahiyan et al. (Abdollahiyan et al., 2020). Such a technology makes is possible to overcome issues associated with more conventional methods like static (manual) seeding onto scaffolds or dynamic seeding using bioreactors as shown by the group of B. Grigolo* (Roseti et al., 2018). In these cases, problems are due to cell accumulation at the surface of scaffold and to the low density in the inner part where cells tend to die because of the scarcity of nutrients. This may lead to inaccurate experimental results and consequent speculation. Differently, 3D bioprinting offers the advantage of fine control of cell spatial distribution in terms of homogeneity. When scaffolds were cultured with chondrogenic or osteogenic medium, cartilage and bone tissues were produced, respectively, as determined by specific gene and protein expression (Gao et al., 2015). Further benefits of 3D bioprinting techniques include reduced production times, an increased versatility, and the possibility to work at room temperature and “solvent-free” conditions, taking advantage of the features of water-based gels such as bioinks as reviewed by the groups of B. Grigolo* and of D. Noël* (Roseti et al., 2017; Montheil et al., 2022). 3D bioprinting also enables the fabrication of custom-made products based on patient’s medical images. Such options improve the match between implant and defect size, thus shortening the time required for surgery and for patient recovery, and positively affecting the success of treatment.
Development and use of bio-inspired, fabricated bio-printed constructs will require validated and relevant cell sources and/or use of appropriate factors to attract endogenous stem cells to the constructs. The cost of producing GMP-grade MSCs, or even iMSCs, using manual processes is such that translation to patient use at scale to enable widespread use is prohibitive and a critical impediment in the field as noted by the group of M. Murphy* (Ochs et al., 2022). The automation of production of advanced therapy medicinal products (ATMPs), state-of-the-art medicines for human use based on genes, tissues or cell-derived EVs can address this hurdle to clinical translation as reported by the group of M. Murphy* (Ochs et al., 2022).
In accordance with the 3Rs concept (replacement, reduction, refinement) and with the strategic directions of the European Medicines Agency (EMA) (announcement of 29 September 2021) to promote alternative approaches to animal models, the development and use of effective alternative in vitro methods before formal animal testing are now mandatory. Advanced techniques such as biomanufacturing (3D bioprinting and bioassembly) and organotypic models (organoids, organ-on-a-chip) are gaining interest due to their ability to mimic OA conditions. Although 2D and 3D cellular cultures have limitations, they are still useful in answering fundamental questions in a molecular, cellular, and tissue continuum and provide a cost-effective platform for rapid, high-throughput screening of new drugs, delivery systems, or biolubricants. This provides an efficient standardized functional qualification without the use of experimental animals for more ethical research (Manivong et al., 2023). Tissue engineering studies conducted since the emergence of ACT in 1994 by Brittberg (Brittberg et al., 1994) led to the development of suitable biological substitutes and refinement of organotypic models by the group of M. Demoor* (Demoor et al., 2014). These mini-tissues/organoids have been incorporated in some translational research studies by the same group* (Desancé et al., 2018; Cullier et al., 2022) to develop cell-based and cell-free orthobiological therapeutic approaches. Therefore, some in vitro studies have been transposed from humans (Legendre et al., 2013; Gómez-Leduc et al., 2016; Gómez-Leduc et al., 2017; Legendre et al., 2017) to horses (Desancé et al., 2018) again by this group* to demonstrate the therapeutic potential of chondrocytes and stem cells for cartilage engineering in both species and to have the possibility to carry out preparatory work in horses before transposing it to humans.
MSC capacity to induce tissue regeneration is due to its secretome that comprises all cell secretions (growth factors, cytokines, EVs, etc.). Among regenerative medicine strategies, acellular or cell-free therapies requires the delivery of exogenous active molecules, such as synthetic molecules or MSC secretome, as therapeutic agents into the joint as reported by Velot* et al. (Dostert et al., 2017; Velot et al., 2021).
Indeed, the secretome of BM MSCs that were primed with inflammatory factors were shown to inhibit inflammatory processes in human OA synovial explants in vitro as observed by the group of G. J. V. M. van Osch* (van Buul et al., 2012). The activity of the secretome of MSCs is not specific for BM MSCs (Manferdini et al., 2020). The groups of G. Lisignoli* and of D. Noël* showed that adipose tissue-derived MSCs (ASCs) have similar effects in vitro, and their anti-inflammatory and anti-catabolic effects were indicated to be dependent on the inflammatory status of the chondrocytes or synoviocytes (Maumus et al., 2016). The secretome of human BM MSCs reduced pain and joint degeneration in a mouse model of OA (Khatab et al., 2018).
Most animal studies aimed at improving acellular MSC-based therapies, especially in horses, are still predominantly conducted in vitro as reported by Jammes et al. (Jammes et al., 2023). The same author participated in a study (Contentin et al., 2022) that specifically highlighted the pro-anabolic potential of equine MSC-conditioned media containing exosomes on a cartilage organoid model in vitro. Kearney et al. (Kearney et al., 2022) performed a recent study in acute inflammatory arthritis by injection of lipopolysaccharide (LPS) in horses, showing no difference between the injection of allogenic BM MSCs and of their secretome. Additionally, proofs of concept for the manufacturing of clinical-grade equine and canine freeze-dried secretome which present many advantages in terms of practical use (stability, easy to store and use) have been published by Mocchi et al. (Mocchi et al., 2021a; Mocchi et al., 2021b). These authors showed in a canine study that an intra-articular injection of secretome in five dogs with OA was safe, without significant adverse effects. These preliminary data suggest that clinical trials may be established to evaluate the potential of these therapies in the treatment of spontaneous OA in animals.
Research is also well underway in animals to characterize the MSC secretome in order to identify the most promising factors for treating OA and to explore the EVs as potential biomarkers for monitoring its progression (Anderson et al., 2022). In particular, techniques for isolating and characterizing EVs have been described for several types of MSCs and work on chondrocytes indicated that MSC-EVs reduce gene expression of inflammatory markers (Hotham et al., 2021; Arévalo-Turrubiarte et al., 2022).
MSCs produce large and small size EV subtypes that exert similar protective effects on chondrocytes and anti-inflammatory effects on macrophages in vitro as reported by Vonk et al. and in vivo in the collagenase-induced OA model of the group of D. Noël* (Cosenza et al., 2017; Vonk et al., 2018). However, small size EVs were more potent to suppress the clinical signs of inflammatory arthritis in the collagen-induced model of the group of D. Noël* (Cosenza et al., 2018). This observation was attributed to the induction of a regulatory response by small size EVs as shown by the upregulation of CD19+IL10+ Breg-like cells and the decrease in plasmablast cells in the lymph nodes of mice. Down-regulating the TGF-β-induced gene product-h3 (TGFBI/BIGH3) in MSCs partly inhibited their anabolic effects on chondrocytes and did not protect mice from developing OA. Although not demonstrated to be directly related, the detection of TGFBI/BIGH3 in the cargo of both small and large size EVs might explain the protective role of EVs in OA as seen by the group of D. Noël* (Ruiz et al., 2020). Priming MSCs was shown to modify their proteome and secretome as well as their content in miRNAs, thereby modulating their functional properties, in particular their immunoregulatory function as described by the group of D. Noël* (Pers et al., 2021). Parental cell priming also impacts the cargo of EVs and inflammatory priming was reported to modulate cytokine and miRNA levels in EVs and enhance their anti-inflammatory potency (Ragni et al., 2020). Another strategy is based on genetic engineering of MSCs to overexpress the hypoxia-inducible factor 1 alpha (HIF-1α) and telomerase to generate large-scale production of reproducible batches of MSC-derived EVs with higher immunosuppressive activity (Gómez-Ferrer et al., 2021). In addition, overexpression of several miRNAs in MSCs or in synovial fibroblasts, as shown with miR-126–3p, may suppress apoptosis and inflammation in chondrocytes and prevent cartilage degradation in a OA model (Zhou et al., 2021).
Indeed, miRNAs were shown to be a crucial player for MSC-EVs function. This paradigm has been confirmed for EVs released by MSCs obtained from several sources such as adipose tissue, bone marrow, and amniotic membrane as studied by the group of L. de Girolamo* (Ragni et al., 2020; Ragni et al., 2021a; Ragni et al., 2022a). With respect to EV-miRNA activity for orthopedic conditions, a complex scenario has emerged with respect to how they can function/contribute to MSC therapeutic potential. MSC-EVs are enriched in miRNAs, predicted to suppress the activation of immune cells and the production of OA-related inflammatory mediators, as well as to promote cartilage protection by acting on both chondrocyte homeostasis and extracellular matrix-degrading enzymes. Most importantly, pre-activation of MSCs with pro-inflammatory mediators allows for the release of EVs with increased amounts of protective miRNAs, as clearly shown for adipose MSCs (Ragni et al., 2020). These results were confirmed by the group of L. de Girolamo* (Ragni et al., 2021b; Ragni et al., 2022b) for EVs released from MSCs in the presence of synovial fluid from OA patients that is rich in pro-inflammatory molecules. Consistently, when envisioned as a cell-free therapy, MSC-EVs protected mice from developing OA, suggesting that these nanoparticles can reproduce the main therapeutic effects of secreting cells by reducing OA symptoms (Cosenza et al., 2017). Notably, inhibition of inflammation (Cosenza et al., 2018) and reduction of cartilage degradation (Cosenza et al., 2017) in vivo confirmed in silico molecular data on EV-miRNAs fingerprints, leading to the potential for new strategies using single miRNA modulation (Xi et al., 2021) as a means to improve therapeutic potential for both MSK disorders and other diseases where cutting-edge treatments are actively needed.
Native EVs or EVs from primed cells do not necessarily contain appropriate mediators to improve OA. The use of synthetic vesicles such as liposomes may be a means to encapsulate and deliver exogenous active molecules of interest to joint tissues via intra-articular injection. This drug delivery system may counteract the degradation of selected mediators and allow for their sustained release in the joint (Velot et al., 2022). However, liposomes can be silenced by immune cell phagocytosis. As the composition of EV membrane prevents phagocytosis, new delivery systems to carry selected active molecules may be developed by merging MSC-derived EVs and liposomes to engineer a hybrid vesicle designed for joint healing as reported by Velot* et al. and by others (Velot et al., 2021; Elkhoury et al., 2022).
MSK gene therapy has been further expanded by combining gene transfer technologies with tissue engineering to generate gene-activated matrices (GAMs), a powerful tool also referred to as biomaterial-guided gene therapy, improving the spatiotemporal, controlled delivery of therapeutic genes to their targets (Borghesi et al., 2019; Perry et al., 2021; Hyland et al., 2020; Hwang et al., 2008; Karikó et al., 2005; Weissman, 2015; Elangovan et al., 2015; Balmayor et al., 2016; Badieyan et al., 2016; Balmayor et al., 2017; Khorsand et al., 2017; Zhang et al., 2019; Geng et al., 2021; Fayed et al., 2021; De La Vega et al., 2022; European Scoiety of Gene and Cell Therapy, 2022; Aini et al., 2016; Tendulkar et al., 2019; Liu et al., 2022; Madry et al., 2020a; Venkatesan et al., 2019; Cucchiarini and Madry, 2019; Atasoy-Zeybek and Kose, 2018; Shapiro et al., 2018; Venkatesan et al., 2018; Rey-Rico et al., 2017a; D’Mello et al., 2017; Rey-Rico et al., 2016; Raisin et al., 2016; Cucchiarini et al., 2016; Raftery et al., 2016; Rey-Rico and Cucchiarini, 2016). Different systems (micelles, hydrogels, solid scaffolds) have been used to generate GAMs capable of delivering a variety of gene transfer vectors. Nonviral vectors were incorporated in GAMs by the groups of A. L. Bertone, of W. Richter, and of M. Murphy* and also by Curtin et al., Raftery et al., and Tierney et al. to carry PTH(1–34) (collagen) (Backstrom et al., 2004), VEGF (collagen) (Geiger et al., 2005), BMP-2 (collagen, nano-hydroxyapatite - nHA, alginate, chondroitin sulfate) (Curtin et al., 2012; Loozen et al., 2013; Nedorubova et al., 2022; Husteden et al., 2023), BMP-2/VEGF (collagen, nHA, chitosan) (Curtin et al., 2015; Raftery et al., 2017; Raftery et al., 2019; Walsh et al., 2021), BMP-2/BMP-7 (collagen, nHA, chitosan) (Raftery et al., 2018), SOX9 (collagen, alginate) (Ledo et al., 2020), ephrinB2 (collagen, nHA) (Tierney et al., 2013), and the stromal-derived factor 1 alpha (SDF-1α) (collagen, nHA) (Power et al., 2022) to stimulate MSC chondro-/osteogenesis and bone healing. GAMs formulating rAAV vectors carrying TGF-β (pluronics, carbon dots - CDs, poly (ε-caprolactone) - PCL) (Rey-Rico et al., 2017b; Meng et al., 2020; Venkatesan et al., 2021), IGF-I (alginate) (Maihöfer et al., 2021), and SOX9 (pluronics, PCL, CDs) (Rey-Rico et al., 2018; Madry et al., 2020b; Urich et al., 2020; Venkatesan et al., 2020) were also used by the group of M. Cucchiarini* to stimulate MSC chondrogenesis, cartilage repair, and prevent OA. Interestingly, GAM have been also tested by the groups of E. R. Balmayor*, of M. Murphy*, and of D. Noël* to deliver RNAs, like for instance an mRNA for BMP-2 (micro-macro biphasic calcium phosphate - MBCP - granules, fibrin gel, collagen sponge, titanium implants) (Tierney et al., 2013; Morille et al., 2016; Balmayor et al., 2017; Zhang et al., 2019; Fayed et al., 2021; De La Vega et al., 2022) to stimulate MSC osteogenesis and bone healing or siRNAs against Runx2 (pluronics, collagen) (Raisin et al., 2017; Salvador et al., 2022) to reduce MSC osteogenesis.
The use of MSC-derived EVs in combination with biomaterials represent a future MSK tissue engineering strategy to influence and facilitate local therapeutic release of EVs by means of their design and chemical characteristics (Yan et al., 2020; Casanova et al., 2021; Zhang et al., 2021). Nanofibrous substrates designed to immobilize EVs induce the chondrogenic differentiation of BM MSCs as seen by Casanova et al. (Casanova et al., 2021). Hydrogels containing MSC-derived EVs have the ability to integrate cartilage ECM and to promote MSC recruitment, resulting in cartilage defect repair (Liu et al., 2017; Zhang et al., 2021). The association of scaffolds with EVs and MSCs is also an alternative to improve cartilage regeneration as reported by Heirani-Tabasi et al. and by others (Heirani-Tabasi et al., 2021; Cho et al., 2023).
The last 30 years of research in cartilage regeneration and its biology have been marked by numerous and essential steps forward in the technologies available to study and apply research aimed at joint regeneration. The fact that women have been a vital part of this effort, especially via work performed in interdisciplinary teams, is to be noted, as evidenced by the large (although non-exhaustive) body of literature cited here. It is critical for us to be at the forefront of research in a field of particular interest to us as doctors, researchers, and women (Alliston et al., 2020), but also as potential patients. If medicine has taught us anything, one must not only view one component of what we are trying to treat, but related components and how they all interact.
In the future, patients will benefit from a continuously maturing collaborative approach, combining the best biological research with robotics for biomaterial and scaffold development and printing. For example, 3D printing has already shown its relevance in creating patient-specific scaffolds based on defect evaluation via radiological evaluation, allowing for near-perfect replication and correction. Automated production systems for cells and cell-derived products synergize to provide relevant cells for these scaffolds in a cost effective manner. This personalized approach allows for a more precise correction and treatment, and better outcomes. Additionally, with potential for the continuous growth of the industry due to the increased incidence of OA in the general population, therapy production will be further facilitated and standardized; therefore, more patients will be able to benefit from a better access to improved technologies to treat OA.
We have also seen that OA and MSK research has diverged to genetic engineering, secretomes, and viral vectors. These approaches based on basic biological principles common to cells show promise, notably when combined with the application of stem cells. In vitro studies on MSC secretomes and EVs by the groups of G. Lisignoli*, of D. Noël*, and of G. J. V. M. van Osch* (van Buul et al., 2012; Manferdini et al., 2020; Ruiz et al., 2020) show promise in terms of anti-inflammatory properties and anti-catabolic effects dependent on the inflammatory status of chondrocytes and synoviocytes. These approaches allow for a more direct cellular-based approach to managing the degeneration leading to OA and the potential for slowing down the catabolic mechanisms leading to its advancement.
The future of MSK regeneration is based on a patient-centered approach. OA is a multimodal disease, with multiple factors leading to its development and progression that can be targeted to delay or even prevent its progression. The ideal scenario for future patients is a multimodal approach from personalized lifestyle modifications informed by polygenic risk scores that characterize an individual genetic risk profile as reported by the group of F. Cicuttini* (Lacaze et al., 2022) to the study of the degenerative and inflammatory state of the joint down to a cellular level to counteract destructive processes and even stimulate regeneration. Combining these different levels of therapeutic impact, one could potentially modulate the disease progression by targeting therapies to different patient phenotype in OA. There remain some challenges regarding how to make such an approach applicable, but once a multimodal method can be applied, the results may be revolutionary by targeting therapies to those most likely to benefit.
Research in the MSK field has seen many breakthroughs in the last decades, with women being at the forefront of several, especially in the field of biology. Yet, a significant disparity remains due to the generally imbalanced ratio of women to men in the field as can be noted on the representation of women in the membership (Figure 2A), in the Board of Directors (Figure 2B), and as recipients of Lifetime/Career Awards (Figure 2C) of various MSK and cell therapy/tissue regeneration societies (data generously provided by the European Society for Sports Traumatology, Knee Surgery and Arthroscopy - ESSKA - https://www.esska.org, the ICRS with a woman current President - EK*, the International Society for Cell and Gene Therapy - ISCT - https://www.isctglobal.org/home, the Osteoarthritis Research Society International - OARSI - https://www.oarsi.org, the Orthopaedic Research Society - ORS - https://www.ors.org with a woman as Past President and a woman as Incoming President, and the Tissue Engineering and Regenerative Medicine International Society - TERMIS - https://www.termis.org with a woman as Incoming President). In particular, the societies associated to clinical orthopedics (i.e., ESSKA and ICRS) are still male-dominated. Since a majority of the 2023 Board of Directors of the societies presented here are well balanced, we can speculate that this token of involvement and recognition of women in the field will translate in a better gender balance of Lifetime/Career awards in the future. The current under-representation of women in MSK research, in particular when it involves orthopedics, has a number of reasons. These include the lack of female role models and mentors in the academic world and in industry is one aspect that makes it more challenging for women to see themselves pursuing careers in MSK research. Moreover, gender prejudice and discrimination might make it more difficult for women to excel in both academia and industry, particularly in male-dominated specialties such as orthopedic surgery.
FIGURE 2. Representation of Women in MSK Societies. (A): Society Membership; (B) Board of Directors; (C) Recipients of Lifetime/Career Awards; n/a: not available).
Orthopedics remains the least diversified medical specialty, with the highest prevalence of men. In fact, only 7.4% and 7% of orthopedic surgeons in the United States and UK, respectively, are female (Ahmed and Hamilton, 2021; Peterman et al., 2022). This disparity also applies in academic and research fields, with only 20% of women assistant professors, 15% of women associate professors, and 9% of women full professors in orthopedics for instance (Gerull et al., 2020), although their impact should not be overlooked. Women are also under-represented in the biomedical engineering field (Barabino et al., 2020) which is key for MSK research and translation. It is therefore crucial to take action to address these underlying problems. This can include campaigns to eliminate gender bias and discrimination as well as initiatives to raise the profile of female researchers and clinicians in academics and associated industries, such as the “Women in ESSKA” initiative that (sic) “wants to promote ESSKA’s educational and research activities among female orthopedic surgeons during and after their specialization” (https://www.esska.org/page/WomeninESSKA) or the “ORS Women’s Leadership Forum” that (sic) “will mentor, foster, encourage and inspire women at the start and throughout their careers in orthopedic research, and will assist women in obtaining leadership roles in orthopedic-related organizations” (https://www.ors.org/womens-leadership-forum).
Hope now lies in the upcoming generation of doctors and researchers, with medical and scientific Universities today having a higher prevalence of female students than males (Barabino et al., 2020; Ahmed and Hamilton, 2021). Moreover, gender should no longer be considered as binary. Hopefully, these developments will have a “butterfly” effect to increase diversity and to continue to open doors for women and minorities in the field of MSK research, including stem cell research. These new and upcoming colleagues also have the advantage of having grown up in parallel to all the new technologies of today. They have a different approach and mindset to search for opportunities, bringing a breath of fresh air and innovation to biomedical research in general. Although these newcomers may have suffered from a lack of mentorship and role models, we hope that this paper will have highlighted women who can illustrate the part and that it will inspire them to become the leaders of tomorrow in this disciplinary field (Barabino et al., 2020).
All authors participated in writing the Introduction and Discussion sections and in preparing and editing Figure 1 and Figure 2. ÉV wrote “EV and biomaterials” and parts of “Joint MSC sources”, of “Tissue engineering”, of “Secretome”, and of “Extracellular vesicles”; EB wrote “Transcript therapy”; LB wrote parts of “Secretome”; SC wrote parts of the “Introduction”; FC wrote parts of the “Introduction”; LdG wrote parts of “Joint MSC sources”, of “MSCs versus tissue-specific cells for joint regeneration”, of “Differentiation and culture conditions for appropriate MSK differentiation of MSCs, priming, immunomodulation”, and of “Extracellular vesicles”; MD wrote “From biologic substitutes to organoids, 3D models, and organ-on-a-chip” and parts of “Secretome”; BG wrote parts of “Bioprinting, sustainable automated manufacturing for therapeutic cells”; EJ wrote parts of “Joint MSC sources”, of “Differentiation and culture conditions for appropriate MSK differentiation of MSCs, priming, immunomodulation”, and of “Secretome”; EK wrote parts of “Tissue engineering”; GL wrote parts of “Tissue engineering”; MM wrote parts of “Joint MSC sources” and of “Bioprinting, sustainable automated manufacturing for therapeutic cells”; EJ wrote parts of “Joint MSC sources”; DN wrote parts of “MSCs versus tissue-specific cells for joint regeneration”, “Joint MSC sources”, “Differentiation and culture conditions for appropriate MSK differentiation of MSCs, priming, immunomodulation”, “Tissue engineering”, “Bioprinting, sustainable automated manufacturing for therapeutic cells” and “Extracellular vesicles”; CV wrote “Gene therapy modifications”; GJVMvO wrote “Embryology and joint development, progenitor cells in the joint” and parts of “Joint MSC sources”, of “MSCs versus tissue-specific cells for joint regeneration”, of “Differentiation and culture conditions for appropriate MSK differentiation of MSCs, priming, immunomodulation”, of “Secretome”, and of “Extracellular vesicles”; MC wrote “Gene therapy”, “Gene-activated matrices”, and parts of “Joint MSC sources” and edited all the sections and the complete manuscript. All authors contributed to the article and approved the submitted version.
This work was supported by the NIH (grant number R01AR074395, NIAMS) and the European Union’s Horizon 2020 research and innovation program (grant “cmRNAbone”, agreement number 874790) (EB), the NHMRC Investigator Grant (APP1194829) (FC), the European Union-funded project “OA-ACTIVE” within the framework of the Operational Program ERDF (European Regional Development Funds)/ESF 2014–2020, also supported by ERDF grants “EQUISTEM” 917CB210), by Fonds Eperon (“EQUISTEM” N80-2014) (MD, LB), the GIS CENTAURE-equine research, the ERDF and Regional Council of Normandy (France) grant in the CPER Centaure program (N◦917CB213, 917CB224), the French National Research Agency (ANR/EuroNanoMedIII, ANR-20-ANM3-0006-02) (MD), the Italian Ministry of Health “Ricerca Corrente” (BG), the Centre of Excellence in Medical Engineering funded by the Wellcome Trust and EPSRC (WELMEC; grant number WT 088908/Z/09/Z) and the Foundation for Research in Rheumatology (FOREUM, grant number 104929) (EJ), the Italian Ministry of Health (BANDO RICERCA FINALIZZATA 2019 esercizio finanziario anni 2018–2019, “PATTERN” grant CO-2019–12370720) (EK), the Italian Ministry of Health “Ricerca Corrente” (GL), the European Union’s Horizon 2020 research and innovation programmes (grant numbers 874671 and 667932), the Higher Education Authority Ireland (EpiHyper), the INTERREG Ireland Wales Programme (80885), the Science Foundation Ireland (2021/01/29FIDEC), and the European Union’s Horizon 2020 research and innovation programme (grant agreements “AutoCRAT” 874671 and “AutoSTEM” 667932) (MM), the French National Research Agency (ANR) (“ECELLFRANCE " grant PIA/ANR-11-INSB-005) (DN), the French National Research Agency (ANR) through the ANR JCJC “KLOTHOA” (ANR-18-CE14-0024-01), the region Pays de la Loire (“RFI Bioregate”, “GenOA”), the Arthritis Foundation, and the Société Française de Rhumatologie (CV), and the World Arthrosis Foundation (MC).
The authors declare that the research was conducted in the absence of any commercial or financial relationships that could be construed as a potential conflict of interest.
All claims expressed in this article are solely those of the authors and do not necessarily represent those of their affiliated organizations, or those of the publisher, the editors and the reviewers. Any product that may be evaluated in this article, or claim that may be made by its manufacturer, is not guaranteed or endorsed by the publisher.
Abdollahiyan, P., Oroojalian, F., Mokhtarzadeh, A., and de la Guardia, M. (2020). Hydrogel-based 3D bioprinting for bone and cartilage tissue engineering. Biotechnol. J. 15, e2000095. doi:10.1002/biot.202000095
Ahmed, M., and Hamilton, L. C. (2021). Current challenges for women in orthopaedics. Bone Jt. Open 2, 893–899. doi:10.1302/2633-1462.210.BJO-2021-0089.R1
Aini, H., Itaka, K., Fujisawa, A., Uchida, H., Uchida, S., Fukushima, S., et al. (2016). Messenger RNA delivery of a cartilage-anabolic transcription factor as a disease-modifying strategy for osteoarthritis treatment. Sci. Rep. 6, 18743. doi:10.1038/srep18743
Alliston, T., Foucher, K. C., Frederick, B., Hernandez, C. J., Iatridis, J. C., Kozloff, K. M., et al. (2020). The importance of diversity, equity, and inclusion in orthopedic research. J. Orthop. Res. 38, 1661–1665. doi:10.1002/jor.24685
Altschuler, N., Zaslav, K. R., Di Matteo, B., Sherman, S. L., Gomoll, A. H., Hacker, S. A., et al. (2023). Aragonite-based scaffold versus microfracture and debridement for the treatment of knee chondral and osteochondral lesions: results of a multicenter randomized controlled trial. Am. J. Sports Med. 51, 957–967. doi:10.1177/03635465231151252
Amann, E., Wolff, P., Breel, E., van Griensven, M., and Balmayor, E. R. (2017). Hyaluronic acid facilitates chondrogenesis and matrix deposition of human adipose derived mesenchymal stem cells and human chondrocytes Co-cultures. Acta Biomater. 52, 130–144. doi:10.1016/j.actbio.2017.01.064
Amini, M., Venkatesan, J. K., Liu, W., Leroux, A., Nguyen, T. N., Madry, H., et al. (2022). Advanced gene therapy strategies for the repair of ACL injuries. Int. J. Mol. Sci. 23, 14467. doi:10.3390/ijms232214467
Anderson, D. D., Chubinskaya, S., Guilak, F., Martin, J. A., Oegema, T. R., Olson, S. A., et al. (2011). Post-traumatic osteoarthritis: improved understanding and opportunities for early intervention. J. Orthop. Res. 29, 802–809. doi:10.1002/jor.21359
Anderson, J. R., Jacobsen, S., Walters, M., Bundgaard, L., Diendorfer, A., Hackl, M., et al. (2022). Small non-coding RNA landscape of extracellular vesicles from a post-traumatic model of equine osteoarthritis. Front. Vet. Sci. 9, 901269. doi:10.3389/fvets.2022.901269
Ansboro, S., Greiser, U., Barry, F., and Murphy, M. (2012). Strategies for improved targeting of therapeutic cells: implications for tissue repair. Eur. Cell Mater 23, 310–318. doi:10.22203/ecm.v023a24
Anzillotti, G., Conte, P., Di Matteo, B., Bertolino, E. M., Marcacci, M., and Kon, E. (2022). Injection of biologic agents for treating severe knee osteoarthritis: is there a chance for a good outcome? a systematic review of clinical evidence. Eur. Rev. Med. Pharmacol. Sci. 26, 5447–5459. doi:10.26355/eurrev_202208_29413
Arévalo-Turrubiarte, M., Baratta, M., Ponti, G., Chiaradia, E., and Martignani, E. (2022). Extracellular vesicles from equine mesenchymal stem cells decrease inflammation markers in chondrocytes in vitro. Equine Vet. J. 54, 1133–1143. doi:10.1111/evj.13537
Armiento, A. R., Stoddart, M. J., Alini, M., and Eglin, D. (2018). Biomaterials for articular cartilage tissue engineering: learning from biology. Acta Biomater. 65, 1–20. doi:10.1016/j.actbio.2017.11.021
Atasoy-Zeybek, A., and Kose, G. T. (2018). Gene therapy strategies in bone tissue engineering and current clinical applications. Adv. Exp. Med. Biol. 1119, 85–101. doi:10.1007/5584_2018_253
Backstrom, K. C., Bertone, A. L., Wisner, E. R., and Weisbrode, S. E. (2004). Response of induced bone defects in horses to collagen matrix containing the human parathyroid hormone gene. Am. J. Vet. Res. 65, 1223–1232. doi:10.2460/ajvr.2004.65.1223
Badieyan, Z. S., Berezhanskyy, T., Utzinger, M., Aneja, M. K., Emrich, D., Erben, R., et al. (2016). Transcript-activated collagen matrix as sustained MRNA delivery system for bone regeneration. J. Control Release 239, 137–148. doi:10.1016/j.jconrel.2016.08.037
Bakhshandeh, B., Zarrintaj, P., Oftadeh, M. O., Keramati, F., Fouladiha, H., Sohrabi-Jahromi, S., et al. (2017). Tissue engineering; strategies, tissues, and biomaterials. Biotechnol. Genet. Eng. Rev. 33, 144–172. doi:10.1080/02648725.2018.1430464
Ball, A. N., Phillips, J. N., McIlwraith, C. W., Kawcak, C. E., Samulski, R. J., and Goodrich, L. R. (2019). Genetic modification of ScAAV-equine-BMP-2 transduced bone-marrow-derived mesenchymal stem cells before and after cryopreservation: an “off-the-shelf” option for fracture repair. J. Orthop. Res. 37, 1310–1317. doi:10.1002/jor.24209
Balmayor, E. R., and Evans, C. H. (2019). RNA therapeutics for tissue engineering. Tissue Eng. Part A 25, 9–11. doi:10.1089/ten.tea.2018.0315
Balmayor, E. R., Geiger, J. P., Aneja, M. K., Berezhanskyy, T., Utzinger, M., Mykhaylyk, O., et al. (2016). Chemically modified RNA induces osteogenesis of stem cells and human tissue explants as well as accelerates bone healing in rats. Biomaterials 87, 131–146. doi:10.1016/j.biomaterials.2016.02.018
Balmayor, E. R., Geiger, J. P., Koch, C., Aneja, M. K., van Griensven, M., Rudolph, C., et al. (2017). Modified MRNA for BMP-2 in combination with biomaterials serves as a transcript-activated matrix for effectively inducing osteogenic pathways in stem cells. Stem Cells Dev. 26, 25–34. doi:10.1089/scd.2016.0171
Balmayor, E. R. (2022). Synthetic MRNA - emerging new class of drug for tissue regeneration. Curr. Opin. Biotechnol. 74, 8–14. doi:10.1016/j.copbio.2021.10.015
Balmayor, E. R. (2015). Targeted delivery as key for the success of small osteoinductive molecules. Adv. Drug Deliv. Rev. 94, 13–27. doi:10.1016/j.addr.2015.04.022
Bannuru, R. R., Osani, M. C., Vaysbrot, E. E., Arden, N. K., Bennell, K., Bierma-Zeinstra, S. M. A., et al. (2019). OARSI guidelines for the non-surgical management of knee, hip, and polyarticular osteoarthritis. Osteoarthr. Cartil. 27, 1578–1589. doi:10.1016/j.joca.2019.06.011
Barabino, G., Frize, M., Ibrahim, F., Kaldoudi, E., Lhotska, L., Marcu, L., et al. (2020). Solutions to gender balance in STEM fields through support, training, education and mentoring: report of the international women in medical physics and biomedical engineering task group. Sci. Eng. Ethics 26, 275–292. doi:10.1007/s11948-019-00097-0
Barbour, K. E., Helmick, C. G., Boring, M., and Brady, T. J. (2017). Vital signs: prevalence of doctor-diagnosed arthritis and arthritis-attributable activity limitation - united states, 2013-2015. MMWR Morb. Mortal. Wkly. Rep. 66, 246–253. doi:10.15585/mmwr.mm6609e1
Bertoni, L., Jacquet-Guibon, S., Branly, T., Desancé, M., Legendre, F., Melin, M., et al. (2021). Evaluation of allogeneic bone-marrow-derived and umbilical cord blood-derived mesenchymal stem cells to prevent the development of osteoarthritis in an equine model. Int. J. Mol. Sci. 22, 2499. doi:10.3390/ijms22052499
Beane, O. S., and Darling, E. M. (2012). Isolation, characterization, and differentiation of stem cells for cartilage regeneration. Ann. Biomed. Eng. 40, 2079–2097. doi:10.1007/s10439-012-0639-8
Bertoni, L., Branly, T., Jacquet, S., Desancé, M., Desquilbet, L., Rivory, P., et al. (2019). Intra-articular injection of 2 different dosages of autologous and allogeneic bone marrow- and umbilical cord-derived mesenchymal stem cells triggers a variable inflammatory response of the fetlock joint on 12 sound experimental horses. Stem Cells Int. 2019, 9431894–9431917. doi:10.1155/2019/9431894
Borghesi, J., Ferreira Lima, M., Mario, L. C., de Almeida da Anunciação, A. R., Silveira Rabelo, A. C., Giancoli Kato Cano da Silva, M., et al. (2019). Canine amniotic membrane mesenchymal stromal/stem cells: isolation, characterization and differentiation. Tissue Cell 58, 99–106. doi:10.1016/j.tice.2019.04.007
Bony, C., Cren, M., Domergue, S., Toupet, K., Jorgensen, C., and Noël, D. (2016). Adipose mesenchymal stem cells isolated after manual or water-jet-assisted liposuction display similar properties. Front. Immunol. 6, 655. doi:10.3389/fimmu.2015.00655
Brittberg, M., Lindahl, A., Nilsson, A., Ohlsson, C., Isaksson, O., and Peterson, L. (1994). Treatment of deep cartilage defects in the knee with autologous chondrocyte transplantation. N. Engl. J. Med. 331, 889–895. doi:10.1056/NEJM199410063311401
Broeckx, S. Y., Seys, B., Suls, M., Vandenberghe, A., Mariën, T., Adriaensen, E., et al. (2019). Equine allogeneic chondrogenic induced mesenchymal stem cells are an effective treatment for degenerative joint disease in horses. Stem Cells Dev. 28, 410–422. doi:10.1089/scd.2018.0061
Camargo Garbin, L., and Morris, M. J. (2021). A comparative review of autologous conditioned serum and autologous protein solution for treatment of osteoarthritis in horses. Front. Vet. Sci. 8, 602978. doi:10.3389/fvets.2021.602978
Carballo-Pedrares, N., Sanjurjo-Rodriguez, C., Señarís, J., Díaz-Prado, S., and Rey-Rico, A. (2022). Chondrogenic differentiation of human mesenchymal stem cells via SOX9 delivery in cationic niosomes. Pharmaceutics 14, 2327. doi:10.3390/pharmaceutics14112327
Casanova, M. R., Osório, H., Reis, R. L., Martins, A., and Neves, N. M. (2021). Chondrogenic differentiation induced by extracellular vesicles bound to a nanofibrous substrate. NPJ Regen. Med. 6, 79. doi:10.1038/s41536-021-00190-8
Chen, M., Hu, Y., Hou, Y., Li, M., Chen, M., Tan, L., et al. (2020). Osteogenesis regulation of mesenchymal stem cells via autophagy induced by silica-titanium composite surfaces with different mechanical moduli. J. Mater Chem. B 8, 9314–9324. doi:10.1039/d0tb01412e
Cheng, A., Kapacee, Z., Peng, J., Lu, S., Lucas, R. J., Hardingham, T. E., et al. (2014). Cartilage repair using human embryonic stem cell-derived chondroprogenitors. Stem Cells Transl. Med. 3, 1287–1294. doi:10.5966/sctm.2014-0101
Cho, W. J., Ahn, J., Lee, M., Choi, H., Park, S., Cha, K.-Y., et al. (2023). Combinatorial effect of mesenchymal stem cells and extracellular vesicles in a hydrogel on cartilage regeneration. Tissue Eng. Regen. Med. 20, 143–154. doi:10.1007/s13770-022-00509-6
Chongchai, A., Waramit, S., Wongwichai, T., Kampangtip, J., Phitak, T., Kongtawelert, P., et al. (2023). Targeting human osteoarthritic chondrocytes with ligand directed bacteriophage-based particles. Viruses 221 (13), 2343. doi:10.3390/v13122343
Cicuttini, F. M., Jones, G., Forbes, A., and Wluka, A. E. (2004). Rate of cartilage loss at two years predicts subsequent total knee arthroplasty: A prospective study. Ann. Rheum. Dis. 63, 1124–1127. doi:10.1136/ard.2004.021253
Colbath, A. C., Dow, S. W., McIlwraith, C. W., and Goodrich, L. R. (2020). Mesenchymal stem cells for treatment of musculoskeletal disease in horses: relative merits of allogeneic versus autologous stem cells. Equine Vet. J. 52, 654–663. doi:10.1111/evj.13233
Coleman, C. M., Curtin, C., Barry, F. P., O’Flatharta, C., and Murphy, J. M. (2010). Mesenchymal stem cells and osteoarthritis: remedy or accomplice? Hum. Gene Ther. 21, 1239–1250. doi:10.1089/hum.2010.138
Colombini, A., Libonati, F., Cangelosi, D., Lopa, S., De Luca, P., Coviello, D. A., et al. (2022b). Inflammatory priming with IL-1β promotes the immunomodulatory behavior of adipose derived stem cells. Front. Bioeng. Biotechnol. 10, 1000879. doi:10.3389/fbioe.2022.1000879
Colombini, A., Libonati, F., Lopa, S., Peretti, G. M., Moretti, M., and de Girolamo, L. (2022a). Autologous chondrocyte implantation provides good long-term clinical results in the treatment of knee osteoarthritis: A systematic review. Knee Surg. Sports Traumatol. Arthrosc. 31, 2338–2348. doi:10.1007/s00167-022-07030-2
Colombini, A., Perucca Orfei, C., Kouroupis, D., Ragni, E., De Luca, P., ViganÒ, M., et al. (2019). Mesenchymal stem cells in the treatment of articular cartilage degeneration: new biological insights for an old-timer cell. Cytotherapy 21, 1179–1197. doi:10.1016/j.jcyt.2019.10.004
Contentin, R., Jammes, M., Bourdon, B., Cassé, F., Bianchi, A., Audigié, F., et al. (2022). Bone marrow MSC secretome increases equine articular chondrocyte collagen accumulation and their migratory capacities. IJMS 23, 5795. doi:10.3390/ijms23105795
Cosenza, S., Ruiz, M., Toupet, K., Jorgensen, C., and Noël, D. (2017). Mesenchymal stem cells derived exosomes and microparticles protect cartilage and bone from degradation in osteoarthritis. Sci. Rep. 7, 16214. doi:10.1038/s41598-017-15376-8
Cosenza, S., Toupet, K., Maumus, M., Luz-Crawford, P., Blanc-Brude, O., Jorgensen, C., et al. (2018). Mesenchymal stem cells-derived exosomes are more immunosuppressive than microparticles in inflammatory arthritis. Theranostics 8, 1399–1410. doi:10.7150/thno.21072
Cottard, V., Valvason, C., Falgarone, G., Lutomski, D., Boissier, M.-C., and Bessis, N. (2004). Immune response against gene therapy vectors: influence of synovial fluid on adeno-associated virus mediated gene transfer to chondrocytes. J. Clin. Immunol. 24, 162–169. doi:10.1023/B:JOCI.0000019781.64421.5c
Craft, A. M., Ahmed, N., Rockel, J. S., Baht, G. S., Alman, B. A., Kandel, R. A., et al. (2013). Specification of chondrocytes and cartilage tissues from embryonic stem cells. Development 140, 2597–2610. doi:10.1242/dev.087890
Critchley, S., Sheehy, E. J., Cunniffe, G., Diaz-Payno, P., Carroll, S. F., Jeon, O., et al. (2020). 3D printing of fibre-reinforced cartilaginous templates for the regeneration of osteochondral defects. Acta Biomater. 113, 130–143. doi:10.1016/j.actbio.2020.05.040
Cucchiarini, M., Asen, A.-K., Goebel, L., Venkatesan, J. K., Schmitt, G., Zurakowski, D., et al. (2018). Effects of TGF-β overexpression via RAAV gene transfer on the early repair processes in an osteochondral defect model in minipigs. Am. J. Sports Med. 46, 1987–1996. doi:10.1177/0363546518773709
Cucchiarini, M., Ekici, M., Schetting, S., Kohn, D., and Madry, H. (2011). Metabolic activities and chondrogenic differentiation of human mesenchymal stem cells following recombinant adeno-associated virus-mediated gene transfer and overexpression of fibroblast growth factor 2. Tissue Eng. Part A 17, 1921–1933. doi:10.1089/ten.TEA.2011.0018
Cucchiarini, M. (2016). Human gene therapy: novel approaches to improve the current gene delivery systems. Discov. Med. 21, 495–506.
Cucchiarini, M., and Madry, H. (2019). Biomaterial-guided delivery of gene vectors for targeted articular cartilage repair. Nat. Rev. Rheumatol. 15, 18–29. doi:10.1038/s41584-018-0125-2
Cucchiarini, M., Madry, H., Ma, C., Thurn, T., Zurakowski, D., Menger, M. D., et al. (2005). Improved tissue repair in articular cartilage defects in vivo by RAAV-mediated overexpression of human fibroblast growth factor 2. Mol. Ther. 12, 229–238. doi:10.1016/j.ymthe.2005.03.012
Cucchiarini, M., and Madry, H. (2014). Overexpression of human IGF-I via direct RAAV-mediated gene transfer improves the early repair of articular cartilage defects in vivo. Gene Ther. 21, 811–819. doi:10.1038/gt.2014.58
Cucchiarini, M., McNulty, A. L., Mauck, R. L., Setton, L. A., Guilak, F., and Madry, H. (2016). Advances in combining gene therapy with cell and tissue engineering-based approaches to enhance healing of the meniscus. Osteoarthr. Cartil. 24, 1330–1339. doi:10.1016/j.joca.2016.03.018
Cucchiarini, M., Orth, P., and Madry, H. (2013). Direct RAAV SOX9 administration for durable articular cartilage repair with delayed terminal differentiation and hypertrophy in vivo. J. Mol. Med. Berl. 91, 625–636. doi:10.1007/s00109-012-0978-9
Cucchiarini, M., Venkatesan, J. K., Ekici, M., Schmitt, G., and Madry, H. (2012). Human mesenchymal stem cells overexpressing therapeutic genes: from basic science to clinical applications for articular cartilage repair. Biomed. Mater Eng. 22, 197–208. doi:10.3233/BME-2012-0709
Cullier, A., Cassé, F., Manivong, S., Contentin, R., Legendre, F., Garcia Ac, A., et al. (2022). Functionalized nanogels with endothelin-1 and bradykinin receptor antagonist peptides decrease inflammatory and cartilage degradation markers of osteoarthritis in a horse organoid model of cartilage. Int. J. Mol. Sci. 23, 8949. doi:10.3390/ijms23168949
Curtin, C. M., Cunniffe, G. M., Lyons, F. G., Bessho, K., Dickson, G. R., Duffy, G. P., et al. (2012). Innovative collagen nano-hydroxyapatite scaffolds offer a highly efficient non-viral gene delivery platform for stem cell-mediated bone formation. Adv. Mater 24, 749–754. doi:10.1002/adma.201103828
Curtin, C. M., Tierney, E. G., McSorley, K., Cryan, S.-A., Duffy, G. P., and O’Brien, F. J. (2015). Combinatorial gene therapy accelerates bone regeneration: non-viral dual delivery of vegf and bmp2 in a collagen-nanohydroxyapatite scaffold. Adv. Healthc. Mater 4, 223–227. doi:10.1002/adhm.201400397
de Girolamo, L., Ragni, E., Cucchiarini, M., van Bergen, C. J. A., Hunziker, E. B., and Chubinskaya, S. (2019). Cells, soluble factors and matrix harmonically play the concert of allograft integration. Knee Surg. Sports Traumatol. Arthrosc. 27, 1717–1725. doi:10.1007/s00167-018-5182-1
de Girolamo, L., Kon, E., Filardo, G., Marmotti, A. G., Soler, F., Peretti, G. M., et al. (2016). Regenerative approaches for the treatment of early OA. Knee Surg. Sports Traumatol. Arthrosc. 24, 1826–1835. doi:10.1007/s00167-016-4125-y
De la Vega, R. E., Atasoy-Zeybek, A., Panos, J. A., Van Griensven, M., Evans, C. H., and Balmayor, E. R. (2021). Gene therapy for bone healing: lessons learned and new approaches. Transl. Res. 236, 1–16. doi:10.1016/j.trsl.2021.04.009
De La Vega, R. E., van Griensven, M., Zhang, W., Coenen, M. J., Nagelli, C. V., Panos, J. A., et al. (2022). Efficient healing of large osseous segmental defects using optimized chemically modified messenger RNA encoding BMP-2. Sci. Adv. 8, eabl6242. doi:10.1126/sciadv.abl6242
De Luca, P., Kouroupis, D., Viganò, M., Perucca-Orfei, C., Kaplan, L., Zagra, L., et al. (2019). Human diseased articular cartilage contains a mesenchymal stem cell-like population of chondroprogenitors with strong immunomodulatory responses. J. Clin. Med. 8, 423. doi:10.3390/jcm8040423
Decker, R. S., Koyama, E., and Pacifici, M. (2014). Genesis and morphogenesis of limb synovial joints and articular cartilage. Matrix Biol. 39, 5–10. doi:10.1016/j.matbio.2014.08.006
Decker, R. S., Um, H.-B., Dyment, N. A., Cottingham, N., Usami, Y., Enomoto-Iwamoto, M., et al. (2017). Cell origin, volume and arrangement are drivers of articular cartilage formation, morphogenesis and response to injury in mouse limbs. Dev. Biol. 426, 56–68. doi:10.1016/j.ydbio.2017.04.006
Demoor, M., Ollitrault, D., Gomez-Leduc, T., Bouyoucef, M., Hervieu, M., Fabre, H., et al. (2014). Cartilage tissue engineering: molecular control of chondrocyte differentiation for proper cartilage matrix reconstruction. Biochim. Biophys. Acta 1840, 2414–2440. doi:10.1016/j.bbagen.2014.02.030
Desancé, M., Contentin, R., Bertoni, L., Gomez-Leduc, T., Branly, T., Jacquet, S., et al. (2018). Chondrogenic differentiation of defined equine mesenchymal stem cells derived from umbilical cord blood for use in cartilage repair therapy. Int. J. Mol. Sci. 19, 537. doi:10.3390/ijms19020537
Deshmukh, V., Hu, H., Barroga, C., Bossard, C., Kc, S., Dellamary, L., et al. (2018). A small-molecule inhibitor of the wnt pathway (SM04690) as a potential disease modifying agent for the treatment of osteoarthritis of the knee. Osteoarthr. Cartil. 26, 18–27. doi:10.1016/j.joca.2017.08.015
Deshmukh, V., O’Green, A. L., Bossard, C., Seo, T., Lamangan, L., Ibanez, M., et al. (2019). Modulation of the wnt pathway through inhibition of CLK2 and DYRK1A by lorecivivint as a novel, potentially disease-modifying approach for knee osteoarthritis treatment. Osteoarthr. Cartil. 27, 1347–1360. doi:10.1016/j.joca.2019.05.006
Diederichs, S., Gabler, J., Autenrieth, J., Kynast, K. L., Merle, C., Walles, H., et al. (2016). Differential regulation of SOX9 protein during chondrogenesis of induced pluripotent stem cells versus mesenchymal stromal cells: a shortcoming for cartilage formation. Stem Cells Dev. 25, 598–609. doi:10.1089/scd.2015.0312
Diederichs, S., Klampfleuthner, F. A. M., Moradi, B., and Richter, W. (2019a). Chondral differentiation of induced pluripotent stem cells without progression into the endochondral pathway. Front. Cell Dev. Biol. 7, 270. doi:10.3389/fcell.2019.00270
Diederichs, S., Tonnier, V., März, M., Dreher, S. I., Geisbüsch, A., and Richter, W. (2019b). Regulation of WNT5A and WNT11 during MSC in vitro chondrogenesis: WNT inhibition lowers BMP and hedgehog activity, and reduces hypertrophy. Cell Mol. Life Sci. 76, 3875–3889. doi:10.1007/s00018-019-03099-0
Diederichs, S., and Tuan, R. S. (2014). Functional comparison of human-induced pluripotent stem cell-derived mesenchymal cells and bone marrow-derived mesenchymal stromal cells from the same donor. Stem Cells Dev. 23, 1594–1610. doi:10.1089/scd.2013.0477
Dietz, B. W., Nakamura, M. C., Bell, M. T., and Lane, N. E. (2021). Targeting nerve growth factor for pain management in osteoarthritis-clinical efficacy and safety. Rheum. Dis. Clin. North Am. 47, 181–195. doi:10.1016/j.rdc.2020.12.003
Djouad, F., Bouffi, C., Ghannam, S., Noël, D., and Jorgensen, C. (2009). Mesenchymal stem cells: innovative therapeutic tools for rheumatic diseases. Nat. Rev. Rheumatol. 5, 392–399. doi:10.1038/nrrheum.2009.104
D’Mello, S., Atluri, K., Geary, S. M., Hong, L., Elangovan, S., and Salem, A. K. (2017). Bone regeneration using gene-activated matrices. AAPS J. 19, 43–53. doi:10.1208/s12248-016-9982-2
Docheva, D., Müller, S. A., Majewski, M., and Evans, C. H. (2015). Biologics for tendon repair. Adv. Drug Deliv. Rev. 84, 222–239. doi:10.1016/j.addr.2014.11.015
Domergue, S., Bony, C., Maumus, M., Toupet, K., Frouin, E., Rigau, V., et al. (2016). Comparison between stromal vascular fraction and adipose mesenchymal stem cells in remodeling hypertrophic scars. PLoS ONE 11, e0156161. doi:10.1371/journal.pone.0156161
Dong, L., Wang, S.-J., Zhao, X.-R., Zhu, Y.-F., and Yu, J.-K. (2017). 3D- printed poly(ε-caprolactone) scaffold integrated with cell-laden chitosan hydrogels for bone tissue engineering. Sci. Rep. 7, 13412. doi:10.1038/s41598-017-13838-7
Dostert, G., Mesure, B., Menu, P., and Velot, É. (2017). How do mesenchymal stem cells influence or are influenced by microenvironment through extracellular vesicles communication? Front. Cell Dev. Biol. 5, 6. doi:10.3389/fcell.2017.00006
Driessen, B. J. H., Logie, C., and Vonk, L. A. (2017). Cellular reprogramming for clinical cartilage repair. Cell Biol. Toxicol. 33, 329–349. doi:10.1007/s10565-017-9382-0
Dyer, O. (2020). Health ministers condemn novartis lottery for zolgensma, the world’s most expensive drug. BMJ 368, m580. doi:10.1136/bmj.m580
Eichhoff, A. M., Börner, K., Albrecht, B., Schäfer, W., Baum, N., Haag, F., et al. (2019). Nanobody-enhanced targeting of AAV gene therapy vectors. Mol. Ther. Methods Clin. Dev. 15, 211–220. doi:10.1016/j.omtm.2019.09.003
El Omar, R., Beroud, J., Stoltz, J.-F., Menu, P., Velot, E., and Decot, V. (2014). Umbilical cord mesenchymal stem cells: the new gold standard for mesenchymal stem cell-based therapies? Tissue Eng. Part B Rev. 20, 523–544. doi:10.1089/ten.TEB.2013.0664
Elangovan, S., Khorsand, B., Do, A.-V., Hong, L., Dewerth, A., Kormann, M., et al. (2015). Chemically modified RNA activated matrices enhance bone regeneration. J. Control Release 218, 22–28. doi:10.1016/j.jconrel.2015.09.050
Elkhoury, K., Chen, M., Koçak, P., Enciso-Martínez, E., Bassous, N. J., Lee, M. C., et al. (2022). Hybrid extracellular vesicles-liposome incorporated advanced bioink to deliver MicroRNA. Biofabrication 14, 045008. doi:10.1088/1758-5090/ac8621
European Scoiety of Gene and Cell Therapy, Human gene therapy, Proceedings of the ESGCT 29th annual congress in collaboration with BSGCT edinburgh, UK October, 2022, doi:10.1089/hum.2022.29225.abstracts
Evans, C. H. (2019). The vicissitudes of gene therapy. Bone Jt. Res. 8, 469–471. doi:10.1302/2046-3758.810.BJR-2019-0265
Fahy, N., de Vries-van Melle, M. L., Lehmann, J., Wei, W., Grotenhuis, N., Farrell, E., et al. (2014). Human osteoarthritic synovium impacts chondrogenic differentiation of mesenchymal stem cells via macrophage polarisation state. Osteoarthr. Cartil. 22, 1167–1175. doi:10.1016/j.joca.2014.05.021
Farrell, E., Fahy, N., Ryan, A. E., Flatharta, C. O., O’Flynn, L., Ritter, T., et al. (2016). VIL-10-Overexpressing human MSCs modulate naïve and activated T lymphocytes following induction of collagenase-induced osteoarthritis. Stem Cell Res. Ther. 7, 74. doi:10.1186/s13287-016-0331-2
Farrell, E., van der Jagt, O. P., Koevoet, W., Kops, N., van Manen, C. J., Hellingman, C. A., et al. (2009). Chondrogenic priming of human bone marrow stromal cells: A better route to bone repair? Tissue Eng. Part C Methods 15, 285–295. doi:10.1089/ten.tec.2008.0297
Fayed, O., van Griensven, M., Tahmasebi Birgani, Z., Plank, C., and Balmayor, E. R. (2021). Transcript-activated coatings on titanium mediate cellular osteogenesis for enhanced osteointegration. Mol. Pharm. 18, 1121–1137. doi:10.1021/acs.molpharmaceut.0c01042
Ferris, D. J., Frisbie, D. D., Kisiday, J. D., McIlwraith, C. W., Hague, B. A., Major, M. D., et al. (2014). Clinical outcome after intra-articular administration of bone marrow derived mesenchymal stem cells in 33 horses with stifle injury. Vet. Surg. 43, 255–265. doi:10.1111/j.1532-950X.2014.12100.x
Fischer, J., Aulmann, A., Dexheimer, V., Grossner, T., and Richter, W. (2014). Intermittent PTHrP(1-34) exposure augments chondrogenesis and reduces hypertrophy of mesenchymal stromal cells. Stem Cells Dev. 23, 2513–2523. doi:10.1089/scd.2014.0101
Fischer, J., Ortel, M., Hagmann, S., Hoeflich, A., and Richter, W. (2016). Role of PTHrP(1-34) pulse frequency versus pulse duration to enhance mesenchymal stromal cell chondrogenesis. J. Cell Physiol. 231, 2673–2681. doi:10.1002/jcp.25369
Fortier, L. A., Nixon, A. J., Williams, J., and Cable, C. S. (1998). Isolation and chondrocytic differentiation of equine bone marrow-derived mesenchymal stem cells. Am. J. Vet. Res. 59, 1182–1187.
Fortier, L. A. (2005). Stem cells: classifications, controversies, and clinical applications. Vet. Surg. 34, 415–423. doi:10.1111/j.1532-950X.2005.00063.x
Fortier, L. A., and Travis, A. J. (2011). Stem cells in veterinary medicine. Stem Cell Res. Ther. 2, 9. doi:10.1186/scrt50
Frisch, J., and Cucchiarini, M. (2016). Gene- and stem cell-based approaches to regulate hypertrophic differentiation in articular cartilage disorders. Stem Cells Dev. 25, 1495–1512. doi:10.1089/scd.2016.0106
Frisch, J., Orth, P., Venkatesan, J. K., Rey-Rico, A., Schmitt, G., Kohn, D., et al. (2017a). Genetic modification of human peripheral blood aspirates using recombinant adeno-associated viral vectors for articular cartilage repair with a focus on chondrogenic transforming growth factor-β gene delivery. Stem Cells Transl. Med. 6, 249–260. doi:10.5966/sctm.2016-0149
Frisch, J., Orth, P., Rey-Rico, A., Venkatesan, J. K., Schmitt, G., Madry, H., et al. (2017b). Peripheral blood aspirates overexpressing IGF-I via RAAV gene transfer undergo enhanced chondrogenic differentiation processes. J. Cell Mol. Med. 21, 2748–2758. doi:10.1111/jcmm.13190
Frisch, J., Rey-Rico, A., Venkatesan, J. K., Schmitt, G., Madry, H., and Cucchiarini, M. (2015b). Chondrogenic differentiation processes in human bone marrow aspirates upon RAAV-mediated gene transfer and overexpression of the insulin-like growth factor I. Tissue Eng. Part A 21, 2460–2471. doi:10.1089/ten.TEA.2014.0679
Frisch, J., Rey-Rico, A., Venkatesan, J. K., Schmitt, G., Madry, H., and Cucchiarini, M. (2016). TGF-Β gene transfer and overexpression via RAAV vectors stimulates chondrogenic events in human bone marrow aspirates. J. Cell Mol. Med. 20, 430–440. doi:10.1111/jcmm.12774
Frisch, J., Venkatesan, J. K., Rey-Rico, A., Madry, H., and Cucchiarini, M. (2015a). Current progress in stem cell-based gene therapy for articular cartilage repair. Curr. Stem Cell Res. Ther. 10, 121–131. doi:10.2174/1574888x09666140922112326
Frisch, J., Venkatesan, J. K., Rey-Rico, A., Schmitt, G., Madry, H., and Cucchiarini, M. (2014a). Determination of the chondrogenic differentiation processes in human bone marrow-derived mesenchymal stem cells genetically modified to overexpress transforming growth factor-β via recombinant adeno-associated viral vectors. Hum. Gene Ther. 25, 1050–1060. doi:10.1089/hum.2014.091
Frisch, J., Venkatesan, J. K., Rey-Rico, A., Schmitt, G., Madry, H., and Cucchiarini, M. (2014b). Influence of insulin-like growth factor I overexpression via recombinant adeno-associated vector gene transfer upon the biological activities and differentiation potential of human bone marrow-derived mesenchymal stem cells. Stem Cell Res. Ther. 5, 103. doi:10.1186/scrt491
Fusco, G., Gambaro, F. M., Di Matteo, B., and Kon, E. (2021). Injections in the osteoarthritic knee: A review of current treatment options. EFORT Open Rev. 6, 501–509. doi:10.1302/2058-5241.6.210026
Gabusi, E., Lenzi, E., Manferdini, C., Dolzani, P., Columbaro, M., Saleh, Y., et al. (2022). Autophagy is a crucial path in chondrogenesis of adipose-derived mesenchymal stromal cells laden in hydrogel. Gels 8, 766. doi:10.3390/gels8120766
Gao, G., Schilling, A. F., Hubbell, K., Yonezawa, T., Truong, D., Hong, Y., et al. (2015). Improved properties of bone and cartilage tissue from 3D inkjet-bioprinted human mesenchymal stem cells by simultaneous deposition and photocrosslinking in PEG-GelMA. Biotechnol. Lett. 37, 2349–2355. doi:10.1007/s10529-015-1921-2
Garcia, J., Hulme, C., Mennan, C., Roberts, S., Bastiaansen-Jenniskens, Y. M., van Osch, G. J. V. M., et al. (2020). The synovial fluid from patients with focal cartilage defects contains mesenchymal stem/stromal cells and macrophages with pro- and anti-inflammatory phenotypes. Osteoarthr. Cartil. Open 2, 100039. doi:10.1016/j.ocarto.2020.100039
Geiger, F., Bertram, H., Berger, I., Lorenz, H., Wall, O., Eckhardt, C., et al. (2005). Vascular endothelial growth factor gene-activated matrix (VEGF165-GAM) enhances osteogenesis and angiogenesis in large segmental bone defects. J. Bone Min. Res. 20, 2028–2035. doi:10.1359/JBMR.050701
Geiger, F., Lorenz, H., Xu, W., Szalay, K., Kasten, P., Claes, L., et al. (2007). VEGF producing bone marrow stromal cells (BMSC) enhance vascularization and resorption of a natural coral bone substitute. Bone 41, 516–522. doi:10.1016/j.bone.2007.06.018
Geng, Y., Duan, H., Xu, L., Witman, N., Yan, B., Yu, Z., et al. (2021). BMP-2 and VEGF-A ModRNAs in collagen scaffold synergistically drive bone repair through osteogenic and angiogenic pathways. Commun. Biol. 4, 82. doi:10.1038/s42003-020-01606-9
Gerull, K. M., Kim, D. J., Cogsil, T., Rhea, L., and Cipriano, C. (2020). Are women proportionately represented as speakers at orthopaedic surgery annual meetings? A cross-sectional analysis. Clin. Orthop. Relat. Res. 478, 2729–2740. doi:10.1097/CORR.0000000000001359
Gómez-Ferrer, M., Villanueva-Badenas, E., Sánchez-Sánchez, R., Sánchez-López, C. M., Baquero, M. C., Sepúlveda, P., et al. (2021). HIF-1α and pro-inflammatory signaling improves the immunomodulatory activity of MSC-derived extracellular vesicles. Int. J. Mol. Sci. 22, 3416. doi:10.3390/ijms22073416
Gómez-Leduc, T., Desancé, M., Hervieu, M., Legendre, F., Ollitrault, D., de Vienne, C., et al. (2017). Hypoxia is a critical parameter for chondrogenic differentiation of human umbilical cord blood mesenchymal stem cells in type I/III collagen sponges. IJMS 18, 1933. doi:10.3390/ijms18091933
Gómez-Leduc, T., Hervieu, M., Legendre, F., Bouyoucef, M., Gruchy, N., Poulain, L., et al. (2016). Chondrogenic commitment of human umbilical cord blood-derived mesenchymal stem cells in collagen matrices for cartilage engineering. Sci. Rep. 6, 32786. doi:10.1038/srep32786
Gong, J., Fairley, J., Cicuttini, F. M., Hussain, S. M., Vashishtha, R., Chou, L., et al. (2021). Effect of stem cell injections on osteoarthritis-related structural outcomes: A systematic review. J. Rheumatol. 48, 585–597. doi:10.3899/jrheum.200021
Guzzo, R. M., Gibson, J., Xu, R.-H., Lee, F. Y., and Drissi, H. (2013). Efficient differentiation of human IPSC-derived mesenchymal stem cells to chondroprogenitor cells. J. Cell Biochem. 114, 480–490. doi:10.1002/jcb.24388
Guérit, D., Brondello, J.-M., Chuchana, P., Philipot, D., Toupet, K., Bony, C., et al. (2014). FOXO3A regulation by miRNA-29a controls chondrogenic differentiation of mesenchymal stem cells and cartilage formation. Stem Cells Dev. 23, 1195–1205. doi:10.1089/scd.2013.0463
Guérit, D., Philipot, D., Chuchana, P., Toupet, K., Brondello, J.-M., Mathieu, M., et al. (2013). Sox9-Regulated miRNA-574-3p inhibits chondrogenic differentiation of mesenchymal stem cells. PLoS ONE 8, e62582. doi:10.1371/journal.pone.0062582
Hackett, C. H., and Fortier, L. A. (2011). Embryonic stem cells and IPS cells: sources and characteristics. Vet. Clin. North Am. Equine Pract. 27, 233–242. doi:10.1016/j.cveq.2011.04.003
Hafezi, M., Nouri Khorasani, S., Zare, M., Esmaeely Neisiany, R., and Davoodi, P. (2021). Advanced hydrogels for cartilage tissue engineering: recent progress and future directions. Polym. (Basel) 13, 4199. doi:10.3390/polym13234199
Hamilton, A. M., Cheung, W.-Y., Gómez-Aristizábal, A., Sharma, A., Nakamura, S., Chaboureau, A., et al. (2019). Iron nanoparticle-labeled murine mesenchymal stromal cells in an osteoarthritic model persists and suggests anti-inflammatory mechanism of action. PLoS One 14, e0214107. doi:10.1371/journal.pone.0214107
Hanousek, K., Salavati, M., and Fouladi-Nashta, A. (2018). Effect of horse sex status on British eventing competition performance: an observational study between 1998 and 2016. Vet. Rec. 182, 666. doi:10.1136/vr.104719
Heirani-Tabasi, A., Hosseinzadeh, S., Rabbani, S., Ahmadi Tafti, S. H., Jamshidi, K., Soufizomorrod, M., et al. (2021). Cartilage tissue engineering by Co-transplantation of chondrocyte extracellular vesicles and mesenchymal stem cells, entrapped in chitosan-hyaluronic acid hydrogel. Biomed. Mater 16, 055003. doi:10.1088/1748-605X/ac0cbf
Hellingman, C. A., Davidson, E. N. B., Koevoet, W., Vitters, E. L., van den Berg, W. B., van Osch, G. J. V. M., et al. (2011). Smad signaling determines chondrogenic differentiation of bone-marrow-derived mesenchymal stem cells: inhibition of smad1/5/8p prevents terminal differentiation and calcification. Tissue Eng. Part A 17, 1157–1167. doi:10.1089/ten.TEA.2010.0043
Hotham, W. E., Thompson, C., Szu-Ting, L., and Henson, F. M. D. (2021). The anti-inflammatory effects of equine bone marrow stem cell-derived extracellular vesicles on autologous chondrocytes. Vet. Rec. Open 8, e22. doi:10.1002/vro2.22
Huang, D., Li, Y., Ma, Z., Lin, H., Zhu, X., Xiao, Y., et al. (2023). Collagen hydrogel viscoelasticity regulates MSC chondrogenesis in a ROCK-dependent manner. Sci. Adv. 9, eade9497. doi:10.1126/sciadv.ade9497
Husteden, C., Brito Barrera, Y. A., Tegtmeyer, S., Borges, J., Giselbrecht, J., Menzel, M., et al. (2023). Lipoplex-functionalized thin-film surface coating based on extracellular matrix components as local gene delivery system to control osteogenic stem cell differentiation. Adv. Healthc. Mater 12, e2201978. doi:10.1002/adhm.202201978
Hwang, N. S., Varghese, S., Lee, H. J., Zhang, Z., Ye, Z., Bae, J., et al. (2008). In vivo commitment and functional tissue regeneration using human embryonic stem cell-derived mesenchymal cells. Proc. Natl. Acad. Sci. U. S. A. 105, 20641–20646. doi:10.1073/pnas.0809680106
Hwang, N. S., Varghese, S., Zhang, Z., and Elisseeff, J. (2006). Chondrogenic differentiation of human embryonic stem cell-derived cells in arginine-glycine-aspartate-modified hydrogels. Tissue Eng. 12, 2695–2706. doi:10.1089/ten.2006.12.2695
Hyland, M., Mennan, C., Wilson, E., Clayton, A., and Kehoe, O. (2020). Pro-inflammatory priming of umbilical cord mesenchymal stromal cells alters the protein cargo of their extracellular vesicles. Cells 9, 726. doi:10.3390/cells9030726
Ilas, D. C., Churchman, S. M., Baboolal, T., Giannoudis, P. V., Aderinto, J., McGonagle, D., et al. (2019). The simultaneous analysis of mesenchymal stem cells and early osteocytes accumulation in osteoarthritic femoral head sclerotic bone. Rheumatol. Oxf. 58, 1777–1783. doi:10.1093/rheumatology/kez130
Ishihara, A., Weisbrode, S. E., and Bertone, A. L. (2015). Autologous implantation of BMP2-expressing dermal fibroblasts to improve bone mineral density and architecture in rabbit long bones. J. Orthop. Res. 33, 1455–1465. doi:10.1002/jor.22791
Ivis, (2010). AAEP annual convention - baltimore. Available online: https://www.ivis.org/library/aaep/aaep-annual-convention-baltimore-2010 (accessed on March 6, 2023).
Jammes, M., Contentin, R., Cassé, F., and Galéra, P. (2023). Equine osteoarthritis: strategies to enhance mesenchymal stromal cell-based acellular therapies. Front. Vet. Sci. 10, 1115774. doi:10.3389/fvets.2023.1115774
Jones, E. A., Crawford, A., English, A., Henshaw, K., Mundy, J., Corscadden, D., et al. (2008). Synovial fluid mesenchymal stem cells in health and early osteoarthritis: detection and functional evaluation at the single-cell level. Arthritis Rheum. 58, 1731–1740. doi:10.1002/art.23485
Jones, E. A., English, A., Henshaw, K., Kinsey, S. E., Markham, A. F., Emery, P., et al. (2004). Enumeration and phenotypic characterization of synovial fluid multipotential mesenchymal progenitor cells in inflammatory and degenerative arthritis. Arthritis Rheum. 50, 817–827. doi:10.1002/art.20203
Jones, E. A., Giannoudis, P. V., and Kouroupis, D. (2016). Bone repair with skeletal stem cells: rationale, progress to date and clinical application. Ther. Adv. Musculoskelet. Dis. 8, 57–71. doi:10.1177/1759720X16642372
Jorgensen, C., Djouad, F., Apparailly, F., and Noël, D. (2003). Engineering mesenchymal stem cells for immunotherapy. Gene Ther. 10, 928–931. doi:10.1038/sj.gt.3302019
Jorgensen, C., Noel, D., Apparailly, F., and Sany, J. (2001). Stem cells for repair of cartilage and bone: the next challenge in osteoarthritis and rheumatoid arthritis. Ann. Rheum. Dis. 60, 305–309. doi:10.1136/ard.60.4.305
Karikó, K., Buckstein, M., Ni, H., and Weissman, D. (2005). Suppression of RNA recognition by toll-like receptors: the impact of nucleoside modification and the evolutionary origin of RNA. Immunity 23, 165–175. doi:10.1016/j.immuni.2005.06.008
Kearney, C. M., Khatab, S., van Buul, G. M., Plomp, S. G. M., Korthagen, N. M., Labberté, M. C., et al. (2022). Treatment effects of intra-articular allogenic mesenchymal stem cell secretome in an equine model of joint inflammation. Front. Vet. Sci. 9, 907616. doi:10.3389/fvets.2022.907616
Kennedy, S., Tambiah, J. R. S., and Lane, N. E. (2022). Osteoarthritis today: lost in translation? Best. Pract. Res. Clin. Rheumatol. 2023, 101810. doi:10.1016/j.berh.2022.101810
Khatab, S., van Osch, G. J., Kops, N., Bastiaansen-Jenniskens, Y. M., Bos, P. K., Verhaar, J. A., et al. (2018). Mesenchymal stem cell secretome reduces pain and prevents cartilage damage in a murine osteoarthritis model. Eur. Cell Mater 36, 218–230. doi:10.22203/eCM.v036a16
Khorsand, B., Elangovan, S., Hong, L., Dewerth, A., Kormann, M. S. D., and Salem, A. K. (2017). A comparative study of the bone regenerative effect of chemically modified RNA encoding BMP-2 or BMP-9. AAPS J. 19, 438–446. doi:10.1208/s12248-016-0034-8
Kisiday, J. D., Liebig, B. E., and Goodrich, L. R. (2020). Adult ovine chondrocytes in expansion culture adopt progenitor cell properties that are favorable for cartilage tissue engineering. J. Orthop. Res. 38, 1996–2005. doi:10.1002/jor.24671
Klinger, P., Surmann-Schmitt, C., Brem, M., Swoboda, B., Distler, J. H., Carl, H.-D., et al. (2011). Chondromodulin 1 stabilizes the chondrocyte phenotype and inhibits endochondral ossification of porcine cartilage repair tissue. Arthritis Rheum. 63, 2721–2731. doi:10.1002/art.30335
Koh, R. H., Jin, Y., Kim, J., and Hwang, N. S. (2020). Inflammation-modulating hydrogels for osteoarthritis cartilage tissue engineering. Cells 9, 419. doi:10.3390/cells9020419
Kon, E., Delcogliano, M., Filardo, G., Montaperto, C., and Marcacci, M. (2008). Second generation issues in cartilage repair. Sports Med. Arthrosc. Rev. 16, 221–229. doi:10.1097/JSA.0b013e31818cdbc5
Kon, E., Di Matteo, B., Delgado, D., Cole, B. J., Dorotei, A., Dragoo, J. L., et al. (2020). Platelet-rich plasma for the treatment of knee osteoarthritis: an expert opinion and proposal for a novel classification and coding system. Expert Opin. Biol. Ther. 20, 1447–1460. doi:10.1080/14712598.2020.1798925
Kon, E., and Di Matteo, B. (2021). Editorial commentary: minimally invasive strategies for osteoarthritis: from platelets to mesenchymal stem cells. Arthroscopy 37, 2258–2261. doi:10.1016/j.arthro.2021.04.010
Kon, E., Di Matteo, B., Verdonk, P., Drobnic, M., Dulic, O., Gavrilovic, G., et al. (2021). Aragonite-based scaffold for the treatment of joint surface lesions in mild to moderate osteoarthritic knees: results of a 2-year multicenter prospective study. Am. J. Sports Med. 49, 588–598. doi:10.1177/0363546520981750
Kon, E., Filardo, G., Brittberg, M., Busacca, M., Condello, V., Engebretsen, L., et al. (2018). A multilayer biomaterial for osteochondral regeneration shows superiority vs microfractures for the treatment of osteochondral lesions in a multicentre randomized trial at 2 years. Knee Surg. Sports Traumatol. Arthrosc. 26, 2704–2715. doi:10.1007/s00167-017-4707-3
Kon, E., Filardo, G., Di Martino, A., Busacca, M., Moio, A., Perdisa, F., et al. (2014). Clinical results and MRI evolution of a nano-composite multilayered biomaterial for osteochondral regeneration at 5 years. Am. J. Sports Med. 42, 158–165. doi:10.1177/0363546513505434
Kon, E., Filardo, G., Di Martino, A., and Marcacci, M. (2012). ACI and MACI. J. Knee Surg. 25, 17–22. doi:10.1055/s-0031-1299651
Kon, E., Filardo, G., Di Matteo, B., Perdisa, F., and Marcacci, M. (2013). Matrix assisted autologous chondrocyte transplantation for cartilage treatment: A systematic review. Bone Jt. Res. 2, 18–25. doi:10.1302/2046-3758.22.2000092
Kon, E., Roffi, A., Filardo, G., Tesei, G., and Marcacci, M. (2015). Scaffold-based cartilage treatments: with or without cells? a systematic review of preclinical and clinical evidence. Arthroscopy 31, 767–775. doi:10.1016/j.arthro.2014.11.017
Kwon, H., Brown, W. E., Lee, C. A., Wang, D., Paschos, N., Hu, J. C., et al. (2019). Surgical and tissue engineering strategies for articular cartilage and meniscus repair. Nat. Rev. Rheumatol. 15, 550–570. doi:10.1038/s41584-019-0255-1
Lacaze, P., Wang, Y., Polekhina, G., Bakshi, A., Riaz, M., Owen, A., et al. (2022). Genomic risk score for advanced osteoarthritis in older adults. Arthritis Rheumatol. 74, 1480–1487. doi:10.1002/art.42156
Ledo, A. M., Vining, K. H., Alonso, M. J., Garcia-Fuentes, M., and Mooney, D. J. (2020). Extracellular matrix mechanics regulate transfection and SOX9-directed differentiation of mesenchymal stem cells. Acta Biomater. 110, 153–163. doi:10.1016/j.actbio.2020.04.027
Lee, H. H., Haleem, A. M., Yao, V., Li, J., Xiao, X., and Chu, C. R. (2011). Release of bioactive adeno-associated virus from fibrin scaffolds: effects of fibrin glue concentrations. Tissue Eng. Part A 17, 1969–1978. doi:10.1089/ten.TEA.2010.0586
Legendre, F., Ollitrault, D., Gomez-Leduc, T., Bouyoucef, M., Hervieu, M., Gruchy, N., et al. (2017). Enhanced chondrogenesis of bone marrow-derived stem cells by using a combinatory cell therapy strategy with BMP-2/TGF-Β1, hypoxia, and COL1A1/HtrA1 SiRNAs. Sci. Rep. 7, 3406. doi:10.1038/s41598-017-03579-y
Legendre, F., Ollitrault, D., Hervieu, M., Baugé, C., Maneix, L., Goux, D., et al. (2013). Enhanced hyaline cartilage matrix synthesis in collagen sponge scaffolds by using SiRNA to stabilize chondrocytes phenotype cultured with bone morphogenetic protein-2 under hypoxia. Tissue Eng. Part C Methods 19, 550–567. doi:10.1089/ten.TEC.2012.0508
Leifer, V. P., Katz, J. N., and Losina, E. (2022). The burden of OA-health services and economics. Osteoarthr. Cartil. 30, 10–16. doi:10.1016/j.joca.2021.05.007
Leijs, M. J. C., van Buul, G. M., Lubberts, E., Bos, P. K., Verhaar, J. A. N., Hoogduijn, M. J., et al. (2012). Effect of arthritic synovial fluids on the expression of immunomodulatory factors by mesenchymal stem cells: an explorative in vitro study. Front. Immunol. 3, 231. doi:10.3389/fimmu.2012.00231
Lepage, S. I. M., Lee, O. J., and Koch, T. G. (2019). Equine cord blood mesenchymal stromal cells have greater differentiation and similar immunosuppressive potential to cord tissue mesenchymal stromal cells. Stem Cells Dev. 28, 227–237. doi:10.1089/scd.2018.0135
Li, J., Wang, X., Ruan, G., Zhu, Z., and Ding, C. (2021). Sprifermin: A recombinant human fibroblast growth factor 18 for the treatment of knee osteoarthritis. Expert Opin. Investig. Drugs 30, 923–930. doi:10.1080/13543784.2021.1972970
Li, L., Yu, F., Zheng, L., Wang, R., Yan, W., Wang, Z., et al. (2019). Natural hydrogels for cartilage regeneration: modification, preparation and application. J. Orthop. Transl. 17, 26–41. doi:10.1016/j.jot.2018.09.003
Liu, W., Madry, H., and Cucchiarini, M. (2022). Application of alginate hydrogels for next-generation articular cartilage regeneration. Int. J. Mol. Sci. 23, 1147. doi:10.3390/ijms23031147
Liu, X., Yang, Y., Li, Y., Niu, X., Zhao, B., Wang, Y., et al. (2017). Integration of stem cell-derived exosomes with in situ hydrogel glue as a promising tissue patch for articular cartilage regeneration. Nanoscale 9, 4430–4438. doi:10.1039/c7nr00352h
Lolli, A., Colella, F., De Bari, C., and van Osch, G. J. V. M. (2019a). Targeting anti-chondrogenic factors for the stimulation of chondrogenesis: A new paradigm in cartilage repair. J. Orthop. Res. 37, 12–22. doi:10.1002/jor.24136
Lolli, A., Narcisi, R., Lambertini, E., Penolazzi, L., Angelozzi, M., Kops, N., et al. (2016). Silencing of antichondrogenic MicroRNA-221 in human mesenchymal stem cells promotes cartilage repair in vivo. Stem Cells 34, 1801–1811. doi:10.1002/stem.2350
Lolli, A., Penolazzi, L., Narcisi, R., van Osch, G. J. V. M., and Piva, R. (2017). Emerging potential of gene silencing approaches targeting anti-chondrogenic factors for cell-based cartilage repair. Cell Mol. Life Sci. 74, 3451–3465. doi:10.1007/s00018-017-2531-z
Lolli, A., Sivasubramaniyan, K., Vainieri, M. L., Oieni, J., Kops, N., Yayon, A., et al. (2019b). Hydrogel-based delivery of AntimiR-221 enhances cartilage regeneration by endogenous cells. J. Control Release 309, 220–230. doi:10.1016/j.jconrel.2019.07.040
Loozen, L. D., Wegman, F., Öner, F. C., Dhert, W. J. A., and Alblas, J. (2013). Porous bioprinted constructs in BMP-2 non-viral gene therapy for bone tissue engineering. J. Mater Chem. B 1, 6619–6626. doi:10.1039/c3tb21093f
Lopa, S., Colombini, A., Moretti, M., and de Girolamo, L. (2019). Injective mesenchymal stem cell-based treatments for knee osteoarthritis: from mechanisms of action to current clinical evidences. Knee Surg. Sports Traumatol. Arthrosc. 27, 2003–2020. doi:10.1007/s00167-018-5118-9
Lopa, S., Colombini, A., Stanco, D., de Girolamo, L., Sansone, V., and Moretti, M. (2014). Donor-matched mesenchymal stem cells from knee infrapatellar and subcutaneous adipose tissue of osteoarthritic donors display differential chondrogenic and osteogenic commitment. Eur. Cell Mater 27, 298–311. doi:10.22203/ecm.v027a21
Madry, H., Gao, L., Rey-Rico, A., Venkatesan, J. K., Müller-Brandt, K., Cai, X., et al. (2020b). Thermosensitive hydrogel based on PEO-PPO-PEO poloxamers for a controlled in situ release of recombinant adeno-associated viral vectors for effective gene therapy of cartilage defects. Adv. Mater 32, e1906508. doi:10.1002/adma.201906508
Madry, H., Venkatesan, J. K., Carballo-Pedrares, N., Rey-Rico, A., and Cucchiarini, M. (2020a). Scaffold-Mediated gene delivery for osteochondral repair. Pharmaceutics 12, 930. doi:10.3390/pharmaceutics12100930
Maihöfer, J., Madry, H., Rey-Rico, A., Venkatesan, J. K., Goebel, L., Schmitt, G., et al. (2021). Hydrogel-guided, RAAV-mediated IGF-I overexpression enables long-term cartilage repair and protection against perifocal osteoarthritis in a large-animal full-thickness chondral defect model at one year in vivo. Adv. Mater 33, e2008451. doi:10.1002/adma.202008451
Manferdini, C., Gabusi, E., Saleh, Y., Lenzi, E., D’Atri, G., Ricotti, L., et al. (2022). Mesenchymal stromal cells laden in hydrogels for osteoarthritis cartilage regeneration: A systematic review from in vitro studies to clinical applications. Cells 11, 3969. doi:10.3390/cells11243969
Manferdini, C., Paolella, F., Gabusi, E., Cattini, L., Rojewski, M., Schrezenmeier, H., et al. (2020). Osteoarthritic milieu affects adipose-derived mesenchymal stromal cells. J. Orthop. Res. 38, 336–347. doi:10.1002/jor.24446
Manivong, S., Cullier, A., Audigié, F., Banquy, X., Moldovan, F., Demoor, M., et al. (2023). New trends for osteoarthritis: biomaterials, models and modeling. Drug Discov. Today 28, 103488. doi:10.1016/j.drudis.2023.103488
Markides, H., Newell, K. J., Rudorf, H., Ferreras, L. B., Dixon, J. E., Morris, R. H., et al. (2019). Ex vivo MRI cell tracking of autologous mesenchymal stromal cells in an ovine osteochondral defect model. Stem Cell Res. Ther. 10, 25. doi:10.1186/s13287-018-1123-7
Martinello, T., Bronzini, I., Perazzi, A., Testoni, S., De Benedictis, G. M., Negro, A., et al. (2013). Effects of in vivo applications of peripheral blood-derived mesenchymal stromal cells (PB-MSCs) and platlet-rich plasma (PRP) on experimentally injured deep digital flexor tendons of sheep. J. Orthop. Res. 31, 306–314. doi:10.1002/jor.22205
Martinez-Redondo, P., Guillen-Guillen, I., Davidsohn, N., Wang, C., Prieto, J., Kurita, M., et al. (2020). αKLOTHO and sTGFβR2 treatment counteract the osteoarthritic phenotype developed in a rat model. Protein Cell 11, 219–226. doi:10.1007/s13238-019-00685-7
Mathieu, M., Vigier, S., Labour, M.-N., Jorgensen, C., Belamie, E., et al. (2014). Induction of mesenchymal stem cell differentiation and cartilage formation by cross-linker-free collagen microspheres. eCM 28, 82–97. doi:10.22203/eCM.v028a07
Maumus, M., Fonteneau, G., Ruiz, M., Assou, S., Boukhaddaoui, H., Pastoureau, P., et al. (2021). Neuromedin B promotes chondrocyte differentiation of mesenchymal stromal cells via calcineurin and calcium signaling. Cell Biosci. 11, 183. doi:10.1186/s13578-021-00695-1
Maumus, M., Manferdini, C., Toupet, K., Chuchana, P., Casteilla, L., Gachet, M., et al. (2017). Thrombospondin-1 partly mediates the cartilage protective effect of adipose-derived mesenchymal stem cells in osteoarthritis. Front. Immunol. 8, 1638. doi:10.3389/fimmu.2017.01638
Maumus, M., Manferdini, C., Toupet, K., Peyrafitte, J.-A., Ferreira, R., Facchini, A., et al. (2013). Adipose mesenchymal stem cells protect chondrocytes from degeneration associated with osteoarthritis. Stem Cell Res. 11, 834–844. doi:10.1016/j.scr.2013.05.008
Maumus, M., Roussignol, G., Toupet, K., Penarier, G., Bentz, I., Teixeira, S., et al. (2016). Utility of a mouse model of osteoarthritis to demonstrate cartilage protection by ifnγ-primed equine mesenchymal stem cells. Front. Immunol. 7, 392. doi:10.3389/fimmu.2016.00392
McCoy, A. M. (2015). Animal models of osteoarthritis: comparisons and key considerations. Vet. Pathol. 52, 803–818. doi:10.1177/0300985815588611
Melief, S. M., Zwaginga, J. J., Fibbe, W. E., and Roelofs, H. (2013). Adipose tissue-derived multipotent stromal cells have a higher immunomodulatory capacity than their bone marrow-derived counterparts. Stem Cells Transl. Med. 2, 455–463. doi:10.5966/sctm.2012-0184
Meng, W., Rey-Rico, A., Claudel, M., Schmitt, G., Speicher-Mentges, S., Pons, F., et al. (2020). RAAV-mediated overexpression of SOX9 and TGF-β via carbon dot-guided vector delivery enhances the biological activities in human bone marrow-derived mesenchymal stromal cells. Nanomater. (Basel) 10, 855. doi:10.3390/nano10050855
Mesure, B., Menu, P., Venkatesan, J. K., Cucchiarini, M., and Velot, É. (2019). Biomaterials and gene therapy: A smart combination for MSC musculoskeletal engineering. Curr. stem cell Res. Ther. 14, 337–343. doi:10.2174/1574888X14666181205121658
Mocchi, M., Bari, E., Dotti, S., Villa, R., Berni, P., Conti, V., et al. (2021b). Canine mesenchymal cell lyosecretome production and safety evaluation after allogenic intraarticular injection in osteoarthritic dogs. Anim. (Basel) 11, 3271. doi:10.3390/ani11113271
Mocchi, M., Grolli, S., Dotti, S., Di Silvestre, D., Villa, R., Berni, P., et al. (2021a). Equine mesenchymal stem/stromal cells freeze-dried secretome (lyosecretome) for the treatment of musculoskeletal diseases: production process validation and batch release test for clinical use. Pharm. (Basel) 14, 553. doi:10.3390/ph14060553
Mödder, U. I., and Khosla, S. (2008). Skeletal stem/osteoprogenitor cells: current concepts, alternate hypotheses, and relationship to the bone remodeling compartment. J. Cell Biochem. 103, 393–400. doi:10.1002/jcb.21423
Montheil, T., Simon, M., Noël, D., Mehdi, A., Subra, G., and Echalier, C. (2022). Silylated biomolecules: versatile components for bioinks. Front. Bioeng. Biotechnol. 10, 888437. doi:10.3389/fbioe.2022.888437
Moradi, L., Vasei, M., Dehghan, M. M., Majidi, M., Farzad Mohajeri, S., and Bonakdar, S. (2017). Regeneration of meniscus tissue using adipose mesenchymal stem cells-chondrocytes Co-culture on a hybrid scaffold: in vivo study. Biomaterials 126, 18–30. doi:10.1016/j.biomaterials.2017.02.022
Morille, M., Toupet, K., Montero-Menei, C. N., Jorgensen, C., and Noël, D. (2016). PLGA-based microcarriers induce mesenchymal stem cell chondrogenesis and stimulate cartilage repair in osteoarthritis. Biomaterials 88, 60–69. doi:10.1016/j.biomaterials.2016.02.022
Morille, M., Van-Thanh, T., Garric, X., Cayon, J., Coudane, J., Noël, D., et al. (2013). New PLGA–P188–PLGA matrix enhances TGF-β3 release from pharmacologically active microcarriers and promotes chondrogenesis of mesenchymal stem cells. J. Control. Release 170, 99–110. doi:10.1016/j.jconrel.2013.04.017
Morscheid, S., Rey-Rico, A., Schmitt, G., Madry, H., Cucchiarini, M., and Venkatesan, J. K. (2019a). Therapeutic effects of RAAV-mediated concomittant gene transfer and overexpression of TGF-β and IGF-I on the chondrogenesis of human bone-marrow-derived mesenchymal stem cells. Int. J. Mol. Sci. 20, 2591. doi:10.3390/ijms20102591
Morscheid, S., Venkatesan, J. K., Rey-Rico, A., Schmitt, G., and Cucchiarini, M. (2019b). Remodeling of human osteochondral defects via RAAV-mediated Co-overexpression of TGF-β and IGF-I from implanted human bone marrow-derived mesenchymal stromal cells. J. Clin. Med. 8, 1326. doi:10.3390/jcm8091326
Murphy, J. M., Fink, D. J., Hunziker, E. B., and Barry, F. P. (2003). Stem cell therapy in a caprine model of osteoarthritis. Arthritis Rheum. 48, 3464–3474. doi:10.1002/art.11365
Murray, S. J., Santangelo, K. S., and Bertone, A. L. (2010). Evaluation of early cellular influences of bone morphogenetic proteins 12 and 2 on equine superficial digital flexor tenocytes and bone marrow-derived mesenchymal stem cells in vitro. Am. J. Vet. Res. 71, 103–114. doi:10.2460/ajvr.71.1.103
Narcisi, R., Cleary, M. A., Brama, P. A. J., Hoogduijn, M. J., Tüysüz, N., ten Berge, D., et al. (2015). Long-term expansion, enhanced chondrogenic potential, and suppression of endochondral ossification of adult human MSCs via WNT signaling modulation. Stem Cell Rep. 4, 459–472. doi:10.1016/j.stemcr.2015.01.017
Nedorubova, I. A., Bukharova, T. B., Mokrousova, V. O., Khvorostina, M. A., Vasilyev, A. V., Nedorubov, A. A., et al. (2022). Comparative efficiency of gene-activated matrices based on chitosan hydrogel and PRP impregnated with BMP2 polyplexes for bone regeneration. Int. J. Mol. Sci. 23, 14720. doi:10.3390/ijms232314720
Noël, D., Djouad, F., and Jorgensen, C. (2002). Regenerative medicine through mesenchymal stem cells for bone and cartilage repair. Curr. Opin. Investig. Drugs 3, 1000–1004.
Nooeaid, P., Salih, V., Beier, J. P., and Boccaccini, A. R. (2012). Osteochondral tissue engineering: scaffolds, stem cells and applications. J. Cell Mol. Med. 16, 2247–2270. doi:10.1111/j.1582-4934.2012.01571.x
Nunes, S. S., Maijub, J. G., Krishnan, L., Ramakrishnan, V. M., Clayton, L. R., Williams, S. K., et al. (2013). Generation of a functional liver tissue mimic using adipose stromal vascular fraction cell-derived vasculatures. Sci. Rep. 3, 2141. doi:10.1038/srep02141
Ochs, J., Hanga, M. P., Shaw, G., Duffy, N., Kulik, M., Tissin, N., et al. (2022). Needle to needle ROBOT-ASSISTED manufacture of cell therapy products. Bioeng. Transl Med 7, e10387. doi:10.1002/btm2.10387
Oldershaw, R. A., Baxter, M. A., Lowe, E. T., Bates, N., Grady, L. M., Soncin, F., et al. (2010). Directed differentiation of human embryonic stem cells toward chondrocytes. Nat. Biotechnol. 28, 1187–1194. doi:10.1038/nbt.1683
Oo, W. M., Little, C., Duong, V., and Hunter, D. J. (2021). The development of disease-modifying therapies for osteoarthritis (DMOADs): the evidence to date. Drug Des. Devel Ther. 15, 2921–2945. doi:10.2147/DDDT.S295224
Orth, P., Rey-Rico, A., Venkatesan, J. K., Madry, H., and Cucchiarini, M. (2014). Current perspectives in stem cell research for knee cartilage repair. Stem Cells Cloning 7, 1–17. doi:10.2147/SCCAA.S42880
Pagnotto, M. R., Wang, Z., Karpie, J. C., Ferretti, M., Xiao, X., and Chu, C. R. (2007). Adeno-associated viral gene transfer of transforming growth factor-beta1 to human mesenchymal stem cells improves cartilage repair. Gene Ther. 14, 804–813. doi:10.1038/sj.gt.3302938
Pascual-Garrido, C., Aisenbrey, E. A., Rodriguez-Fontan, F., Payne, K. A., Bryant, S. J., and Goodrich, L. R. (2019). Photopolymerizable injectable cartilage mimetic hydrogel for the treatment of focal chondral lesions: A proof of concept study in a rabbit animal model. Am. J. Sports Med. 47, 212–221. doi:10.1177/0363546518808012
Peck, J., Slovek, A., Miro, P., Vij, N., Traube, B., Lee, C., et al. (2021). A comprehensive review of viscosupplementation in osteoarthritis of the knee. Orthop. Rev. (Pavia) 13, 25549. doi:10.52965/001c.25549
Pelttari, K., Winter, A., Steck, E., Goetzke, K., Hennig, T., Ochs, B. G., et al. (2006). Premature induction of hypertrophy during in vitro chondrogenesis of human mesenchymal stem cells correlates with calcification and vascular invasion after ectopic transplantation in SCID mice. Arthritis Rheum. 54, 3254–3266. doi:10.1002/art.22136
Perdisa, F., Filardo, G., Di Matteo, B., Marcacci, M., and Kon, E. (2014). Platelet rich plasma: A valid augmentation for cartilage scaffolds? A systematic review. Histol. Histopathol. 29, 805–814. doi:10.14670/HH-29.805
Perry, J., Roelofs, A. J., Mennan, C., McCarthy, H. S., Richmond, A., Clark, S. M., et al. (2021). Human mesenchymal stromal cells enhance cartilage healing in a murine joint surface injury model. Cells 10, 1999. doi:10.3390/cells10081999
Pers, Y.-M., Bony, C., Duroux-Richard, I., Bernard, L., Maumus, M., Assou, S., et al. (2021). MiR-155 contributes to the immunoregulatory function of human mesenchymal stem cells. Front. Immunol. 12, 624024. doi:10.3389/fimmu.2021.624024
Peshkova, M., Lychagin, A., Lipina, M., Di Matteo, B., Anzillotti, G., Ronzoni, F., et al. (2022). Gender-related aspects in osteoarthritis development and progression: A review. IJMS 23, 2767. doi:10.3390/ijms23052767
Peterman, N. J., Macinnis, B., Stauffer, K., Mann, R., Yeo, E. G., and Carpenter, K. (2022). Gender representation in orthopaedic surgery: A geospatial analysis from 2015 to 2022. Cureus 14, e27305. doi:10.7759/cureus.27305
Pleumeekers, M. M., Nimeskern, L., Koevoet, J. L. M., Karperien, M., Stok, K. S., and van Osch, G. J. V. M. (2018). Trophic effects of adipose-tissue-derived and bone-marrow-derived mesenchymal stem cells enhance cartilage generation by chondrocytes in Co-culture. PLoS One 13, e0190744. doi:10.1371/journal.pone.0190744
Poh, P. S. P., Seeliger, C., Unger, M., Falldorf, K., Balmayor, E. R., and van Griensven, M. (2018). Osteogenic effect and cell signaling activation of extremely low-frequency pulsed electromagnetic fields in adipose-derived mesenchymal stromal cells. Stem Cells Int. 2018, 5402853–5402911. doi:10.1155/2018/5402853
Power, R. N., Cavanagh, B. L., Dixon, J. E., Curtin, C. M., and O’Brien, F. J. (2022). Development of a gene-activated scaffold incorporating multifunctional cell-penetrating peptides for PSDF-1α delivery for enhanced angiogenesis in tissue engineering applications. Int. J. Mol. Sci. 23, 1460. doi:10.3390/ijms23031460
Raftery, R. M., Mencía Castaño, I., Chen, G., Cavanagh, B., Quinn, B., Curtin, C. M., et al. (2017). Translating the role of osteogenic-angiogenic coupling in bone formation: highly efficient chitosan-pdna activated scaffolds can accelerate bone regeneration in critical-sized bone defects. Biomaterials 149, 116–127. doi:10.1016/j.biomaterials.2017.09.036
Raftery, R. M., Mencía-Castaño, I., Sperger, S., Chen, G., Cavanagh, B., Feichtinger, G. A., et al. (2018). Delivery of the improved BMP-2-advanced plasmid DNA within a gene-activated scaffold accelerates mesenchymal stem cell osteogenesis and critical size defect repair. J. Control Release 283, 20–31. doi:10.1016/j.jconrel.2018.05.022
Raftery, R. M., Walsh, D. P., Blokpoel Ferreras, L., Mencía Castaño, I., Chen, G., LeMoine, M., et al. (2019). Highly versatile cell-penetrating peptide loaded scaffold for efficient and localised gene delivery to multiple cell types: from development to application in tissue engineering. Biomaterials 216, 119277. doi:10.1016/j.biomaterials.2019.119277
Raftery, R. M., Walsh, D. P., Castaño, I. M., Heise, A., Duffy, G. P., Cryan, S.-A., et al. (2016). Delivering nucleic-acid based nanomedicines on biomaterial scaffolds for orthopedic tissue repair: challenges, progress and future perspectives. Adv. Mater 28, 5447–5469. doi:10.1002/adma.201505088
Ragni, E., Colombini, A., Viganò, M., Libonati, F., Perucca Orfei, C., Zagra, L., et al. (2021b). Cartilage protective and immunomodulatory features of osteoarthritis synovial fluid-treated adipose-derived mesenchymal stem cells secreted factors and extracellular vesicles-embedded MiRNAs. Cells 10, 1072. doi:10.3390/cells10051072
Ragni, E., Papait, A., Perucca Orfei, C., Silini, A. R., Colombini, A., Viganò, M., et al. (2021a). Amniotic membrane-mesenchymal stromal cells secreted factors and extracellular vesicle-MiRNAs: anti-inflammatory and regenerative features for musculoskeletal tissues. Stem Cells Transl. Med. 10, 1044–1062. doi:10.1002/sctm.20-0390
Ragni, E., Perucca Orfei, C., and de Girolamo, L. (2022a). Secreted factors and extracellular vesicles account for the immunomodulatory and tissue regenerative properties of bone-marrow-derived mesenchymal stromal cells for osteoarthritis. Cells 11, 3501. doi:10.3390/cells11213501
Ragni, E., Perucca Orfei, C., De Luca, P., Mondadori, C., Viganò, M., Colombini, A., et al. (2020). Inflammatory priming enhances mesenchymal stromal cell secretome potential as a clinical product for regenerative medicine approaches through secreted factors and EV-MiRNAs: the example of joint disease. Stem Cell Res. Ther. 11, 165. doi:10.1186/s13287-020-01677-9
Ragni, E., Perucca Orfei, C., Valli, F., Zagra, L., and de Girolamo, L. (2022b). Molecular characterization of secreted factors and extracellular vesicles-embedded MiRNAs from bone marrow-derived mesenchymal stromal cells in presence of synovial fluid from osteoarthritis patients. Biol. (Basel) 11, 1632. doi:10.3390/biology11111632
Rahman, G., Frazier, T. P., Gimble, J. M., and Mohiuddin, O. A. (2022). The emerging use of ASC/scaffold composites for the regeneration of osteochondral defects. Front. Bioeng. Biotechnol. 10, 893992. doi:10.3389/fbioe.2022.893992
Raisin, S., Belamie, E., and Morille, M. (2016). Non-viral gene activated matrices for mesenchymal stem cells based tissue engineering of bone and cartilage. Biomaterials 104, 223–237. doi:10.1016/j.biomaterials.2016.07.017
Raisin, S., Morille, M., Bony, C., Noël, D., Devoisselle, J.-M., and Belamie, E. (2017). Tripartite polyionic complex (PIC) micelles as non-viral vectors for mesenchymal stem cell SiRNA transfection. Biomater. Sci. 5, 1910–1921. doi:10.1039/c7bm00384f
Ramos, Y. F. M., Tertel, T., Shaw, G., Staubach, S., de Almeida, R. C., Suchiman, E., et al. (2022). Characterizing the secretome of licensed HiPSC-derived MSCs. Stem Cell Res. Ther. 13, 434. doi:10.1186/s13287-022-03117-2
Ramzan, F., Ekram, S., Frazier, T., Salim, A., Mohiuddin, O. A., and Khan, I. (2022). Decellularized human umbilical tissue-derived hydrogels promote proliferation and chondrogenic differentiation of mesenchymal stem cells. Bioeng. (Basel) 9, 239. doi:10.3390/bioengineering9060239
Rey-Rico, A., and Cucchiarini, M. (2016). Controlled release strategies for RAAV-mediated gene delivery. Acta Biomater. 29, 1–10. doi:10.1016/j.actbio.2015.10.015
Rey-Rico, A., Cucchiarini, M., and Madry, H. (2017a). Hydrogels for precision meniscus tissue engineering: A comprehensive review. Connect. Tissue Res. 58, 317–328. doi:10.1080/03008207.2016.1276576
Rey-Rico, A., and Cucchiarini, M. (2017). Smart and controllable RAAV gene delivery carriers in progenitor cells for human musculoskeletal regenerative medicine with a focus on the articular cartilage. Curr. Gene Ther. 17, 127–138. doi:10.2174/1566523217666170510162459
Rey-Rico, A., Madry, H., and Cucchiarini, M. (2016). Hydrogel-based controlled delivery systems for articular cartilage repair. Biomed. Res. Int. 2016, 1215263. doi:10.1155/2016/1215263
Rey-Rico, A., Venkatesan, J. K., Schmitt, G., Concheiro, A., Madry, H., Alvarez-Lorenzo, C., et al. (2017b). RAAV-mediated overexpression of TGF-β via vector delivery in polymeric micelles stimulates the biological and reparative activities of human articular chondrocytes in vitro and in a human osteochondral defect model. Int. J. Nanomedicine 12, 6985–6996. doi:10.2147/IJN.S144579
Rey-Rico, A., Venkatesan, J. K., Schmitt, G., Speicher-Mentges, S., Madry, H., and Cucchiarini, M. (2018). Effective remodelling of human osteoarthritic cartilage by Sox9 gene transfer and overexpression upon delivery of RAAV vectors in polymeric micelles. Mol. Pharm. 15, 2816–2826. doi:10.1021/acs.molpharmaceut.8b00331
Rikkers, M., Korpershoek, J. V., Levato, R., Malda, J., and Vonk, L. A. (2021). Progenitor cells in healthy and osteoarthritic human cartilage have extensive culture expansion capacity while retaining chondrogenic properties. Cartilage 13, 129S–142S. doi:10.1177/19476035211059600
Rikkers, M., Korpershoek, J. V., Levato, R., Malda, J., and Vonk, L. A. (2022). The clinical potential of articular cartilage-derived progenitor cells: A systematic review. NPJ Regen. Med. 7, 2. doi:10.1038/s41536-021-00203-6
Roelofs, A. J., Zupan, J., Riemen, A. H. K., Kania, K., Ansboro, S., White, N., et al. (2017). Joint morphogenetic cells in the adult mammalian synovium. Nat. Commun. 8, 15040. doi:10.1038/ncomms15040
Roemeling-van Rhijn, M., Mensah, F. K. F., Korevaar, S. S., Leijs, M. J., van Osch, G. J. V. M., Ijzermans, J. N. M., et al. (2013). Effects of hypoxia on the immunomodulatory properties of adipose tissue-derived mesenchymal stem cells. Front. Immunol. 4, 203. doi:10.3389/fimmu.2013.00203
Roseti, L., Cavallo, C., Desando, G., Parisi, V., Petretta, M., Bartolotti, I., et al. (2018). Three-dimensional bioprinting of cartilage by the use of stem cells: A strategy to improve regeneration. Mater. (Basel) 11, 1749. doi:10.3390/ma11091749
Roseti, L., Desando, G., Cavallo, C., Petretta, M., and Grigolo, B. (2019). Articular cartilage regeneration in osteoarthritis. Cells 8, 1305. doi:10.3390/cells8111305
Roseti, L., Parisi, V., Petretta, M., Cavallo, C., Desando, G., Bartolotti, I., et al. (2017). Scaffolds for bone tissue engineering: state of the art and new perspectives. Mater Sci. Eng. C Mater Biol. Appl. 78, 1246–1262. doi:10.1016/j.msec.2017.05.017
Ruiz, M., Toupet, K., Maumus, M., Rozier, P., Jorgensen, C., and Noël, D. (2020). TGFBI secreted by mesenchymal stromal cells ameliorates osteoarthritis and is detected in extracellular vesicles. Biomaterials 226, 119544. doi:10.1016/j.biomaterials.2019.119544
Russo, E., Caprnda, M., Kruzliak, P., Conaldi, P. G., Borlongan, C. V., and La Rocca, G. (2022). Umbilical cord mesenchymal stromal cells for cartilage regeneration applications. Stem Cells Int. 2022, 2454168. doi:10.1155/2022/2454168
Sahin, U., Karikó, K., and Türeci, Ö. (2014). MRNA-based therapeutics--developing a new class of drugs. Nat. Rev. Drug Discov. 13, 759–780. doi:10.1038/nrd4278
Salvador, J., Berthelot, J., Bony, C., Robin, B., Him, J. L. K., Noël, D., et al. (2022). Size-tunable lipid vectors for controlled local delivery of SiRNA from gene activated matrix. Acta Biomater. 153, 97–107. doi:10.1016/j.actbio.2022.09.016
Sánchez-Téllez, D. A., Téllez-Jurado, L., and Rodríguez-Lorenzo, L. M. (2017). Hydrogels for cartilage regeneration, from polysaccharides to hybrids. Polym. (Basel) 9, 671. doi:10.3390/polym9120671
Sanjurjo-Rodriguez, C., Altaie, A., Mastbergen, S., Baboolal, T., Welting, T., Lafeber, F., et al. (2020). Gene expression signatures of synovial fluid multipotent stromal cells in advanced knee osteoarthritis and following knee joint distraction. Front. Bioeng. Biotechnol. 8, 579751. doi:10.3389/fbioe.2020.579751
Sanjurjo-Rodriguez, C., Baboolal, T. G., Burska, A. N., Ponchel, F., El-Jawhari, J. J., Pandit, H., et al. (2019). Gene expression and functional comparison between multipotential stromal cells from lateral and medial condyles of knee osteoarthritis patients. Sci. Rep. 9, 9321. doi:10.1038/s41598-019-45820-w
Sarem, M., Arya, N., Heizmann, M., Neffe, A. T., Barbero, A., Gebauer, T. P., et al. (2018). Interplay between stiffness and degradation of architectured gelatin hydrogels leads to differential modulation of chondrogenesis in vitro and in vivo. Acta Biomater. 69, 83–94. doi:10.1016/j.actbio.2018.01.025
Sartore, L., Manferdini, C., Saleh, Y., Dey, K., Gabusi, E., Ramorino, G., et al. (2021). Polysaccharides on gelatin-based hydrogels differently affect chondrogenic differentiation of human mesenchymal stromal cells. Mater Sci. Eng. C Mater Biol. Appl. 126, 112175. doi:10.1016/j.msec.2021.112175
Schnabel, L. V., Abratte, C. M., Schimenti, J. C., Felippe, M. J. B., Cassano, J. M., Southard, T. L., et al. (2014). Induced pluripotent stem cells have similar immunogenic and more potent immunomodulatory properties compared with bone marrow-derived stromal cells in vitro. Regen. Med. 9, 621–635. doi:10.2217/rme.14.29
Schwabe, K., Garcia, M., Ubieta, K., Hannemann, N., Herbort, B., Luther, J., et al. (2016). Inhibition of osteoarthritis by adipose-derived stromal cells overexpressing fra-1 in mice. Arthritis & Rheumatology 68, 138–151. doi:10.1002/art.39425
Shapiro, G., Lieber, R., Gazit, D., and Pelled, G. (2018). Recent advances and future of gene therapy for bone regeneration. Curr. Osteoporos. Rep. 16, 504–511. doi:10.1007/s11914-018-0459-3
Sivasubramaniyan, K., Ilas, D. C., Harichandan, A., Bos, P. K., Santos, D. L., de Zwart, P., et al. (2018). Bone marrow-harvesting technique influences functional heterogeneity of mesenchymal stem/stromal cells and cartilage regeneration. Am. J. Sports Med. 46, 3521–3531. doi:10.1177/0363546518804807
Sivasubramaniyan, K., Koevoet, W. J. L. M., Hakimiyan, A. A., Sande, M., Farrell, E., Hoogduijn, M. J., et al. (2019). Cell-surface markers identify tissue resident multipotential stem/stromal cell subsets in synovial intimal and sub-intimal compartments with distinct chondrogenic properties. Osteoarthr. Cartil. 27, 1831–1840. doi:10.1016/j.joca.2019.08.006
Skou, S. T., and Roos, E. M. (2019). Physical therapy for patients with knee and hip osteoarthritis: supervised, active treatment is current best practice. Clin. Exp. Rheumatol. 37, 112–117.
Sun, Y., Chen, S., and Pei, M. (2018). Comparative advantages of infrapatellar fat pad: an emerging stem cell source for regenerative medicine. Rheumatol. Oxf. 57, 2072–2086. doi:10.1093/rheumatology/kex487
Tanikella, A. S., Hardy, M. J., Frahs, S. M., Cormier, A. G., Gibbons, K. D., Fitzpatrick, C. K., et al. (2020). Emerging gene-editing modalities for osteoarthritis. Int. J. Mol. Sci. 21, 6046. doi:10.3390/ijms21176046
Taraballi, F., Bauza, G., McCulloch, P., Harris, J., and Tasciotti, E. (2017). Concise review: biomimetic functionalization of biomaterials to stimulate the endogenous healing process of cartilage and bone tissue. Stem Cells Transl. Med. 6, 2186–2196. doi:10.1002/sctm.17-0181
Ter Huurne, M., Schelbergen, R., Blattes, R., Blom, A., De Munter, W., Grevers, L. C., et al. (2012). Antiinflammatory and chondroprotective effects of intraarticular injection of adipose-derived stem cells in experimental osteoarthritis. Arthritis & Rheumatism 64, 3604–3613. doi:10.1002/art.34626
Tendulkar, G., Ehnert, S., Sreekumar, V., Chen, T., Kaps, H.-P., Golombek, S., et al. (2019). Exogenous delivery of Link N MRNA into chondrocytes and MSCs-the potential role in increasing anabolic response. Int. J. Mol. Sci. 20, 1716. doi:10.3390/ijms20071716
Teunissen, M., Ahrens, N. S., Snel, L., Narcisi, R., Kamali, S. A., van Osch, G. J. V. M., et al. (2022). Synovial membrane-derived mesenchymal progenitor cells from osteoarthritic joints in dogs possess lower chondrogenic-and higher osteogenic capacity compared to normal joints. Stem Cell Res. Ther. 13, 457. doi:10.1186/s13287-022-03144-z
Tierney, E. G., McSorley, K., Hastings, C. L., Cryan, S.-A., O’Brien, T., Murphy, M. J., et al. (2013). High levels of EphrinB2 over-expression increases the osteogenic differentiation of human mesenchymal stem cells and promotes enhanced cell mediated mineralisation in a polyethyleneimine-EphrinB2 gene-activated matrix. J. Control Release 165, 173–182. doi:10.1016/j.jconrel.2012.11.013
Tiryaki, T., Cohen, S. R., Canikyan Turkay, S., Kocak, P., Sterodimas, A., Schlaudraff, K.-U., et al. (2022). Hybrid stromal vascular fraction (Hybrid-SVF): A new paradigm in mechanical regenerative cell processing. Plast. Reconstr. Surg. Glob. Open 10, e4702. doi:10.1097/GOX.0000000000004702
Trucco, D., Sharma, A., Manferdini, C., Gabusi, E., Petretta, M., Desando, G., et al. (2021). Modeling and fabrication of silk fibroin–gelatin-based constructs using extrusion-based three-dimensional bioprinting. ACS Biomater. Sci. Eng. 7, 3306–3320. doi:10.1021/acsbiomaterials.1c00410
Ulivi, M., Meroni, V., Viganò, M., Colombini, A., Lombardo, M. D. M., Rossi, N., et al. (2022). Micro-fragmented adipose tissue (MFAT) associated with arthroscopic debridement provides functional improvement in knee osteoarthritis: A randomized controlled trial. Knee Surg. Sports Traumatol. Arthrosc. 31, 3079–3090. doi:10.1007/s00167-022-07101-4
United States Bone and Joint Initiative, (2020). The burden of musculoskeletal diseases in the United States (BMUS). Available online: https://www.boneandjointburden.org (accessed on March 6, 2023).
Urich, J., Cucchiarini, M., and Rey-Rico, A. (2020). Therapeutic delivery of RAAV Sox9 via polymeric micelles counteracts the effects of osteoarthritis-associated inflammatory cytokines in human articular chondrocytes. Nanomater. (Basel) 10, 1238. doi:10.3390/nano10061238
van Buul, G. M., Villafuertes, E., Bos, P. K., Waarsing, J. H., Kops, N., Narcisi, R., et al. (2012). Mesenchymal stem cells secrete factors that inhibit inflammatory processes in short-term osteoarthritic synovium and cartilage explant culture. Osteoarthr. Cartil. 20, 1186–1196. doi:10.1016/j.joca.2012.06.003
van Osch, G. J. V. M., Brittberg, M., Dennis, J. E., Bastiaansen-Jenniskens, Y. M., Erben, R. G., Konttinen, Y. T., et al. (2009). Cartilage repair: past and future--lessons for regenerative medicine. J. Cell Mol. Med. 13, 792–810. doi:10.1111/j.1582-4934.2009.00789.x
Valot, L., Maumus, M., Brunel, L., Martinez, J., Amblard, M., Noël, D., et al. (2021). A collagen-mimetic organic-inorganic hydrogel for cartilage engineering. Gels 7, 73. doi:10.3390/gels7020073
Varma, M. J. O., Breuls, R. G. M., Schouten, T. E., Jurgens, W. J. F. M., Bontkes, H. J., Schuurhuis, G. J., et al. (2007). Phenotypical and functional characterization of freshly isolated adipose tissue-derived stem cells. Stem Cells Dev. 16, 91–104. doi:10.1089/scd.2006.0026
Velloso Alvarez, A., Boone, L. H., Braim, A. P., Taintor, J. S., Caldwell, F., Wright, J. C., et al. (2020). A survey of clinical usage of non-steroidal intra-articular therapeutics by equine practitioners. Front. Vet. Sci. 7, 579967. doi:10.3389/fvets.2020.579967
Velot, É., Elkhoury, K., Kahn, C., Kempf, H., Linder, M., Arab-Tehrany, E., et al. (2022). Efficient TGF-B1 delivery to articular chondrocytes in vitro using agro-based liposomes. IJMS 23, 2864. doi:10.3390/ijms23052864
Velot, É., Madry, H., Venkatesan, J. K., Bianchi, A., and Cucchiarini, M. (2021). Is extracellular vesicle-based therapy the next answer for cartilage regeneration? Front. Bioeng. Biotechnol. 9, 645039. doi:10.3389/fbioe.2021.645039
Venkatesan, J. K., Cai, X., Meng, W., Rey-Rico, A., Schmitt, G., Speicher-Mentges, S., et al. (2021). PNaSS-grafted PCL film-guided RAAV TGF-β gene therapy activates the chondrogenic activities in human bone marrow aspirates. Hum. Gene Ther. 32, 895–906. doi:10.1089/hum.2020.329
Venkatesan, J. K., Falentin-Daudré, C., Leroux, A., Migonney, V., and Cucchiarini, M. (2018). Controlled release of gene therapy constructs from solid scaffolds for therapeutic applications in orthopedics. Discov. Med. 25, 195–203.
Venkatesan, J. K., Meng, W., Rey-Rico, A., Schmitt, G., Speicher-Mentges, S., Falentin-Daudré, C., et al. (2020). Enhanced chondrogenic differentiation activities in human bone marrow aspirates via Sox9 overexpression mediated by PNaSS-grafted PCL film-guided RAAV gene transfer. Pharmaceutics 12, 280. doi:10.3390/pharmaceutics12030280
Venkatesan, J. K., Rey-Rico, A., and Cucchiarini, M. (2019). Current trends in viral gene therapy for human orthopaedic regenerative medicine. Tissue Eng. Regen. Med. 16, 345–355. doi:10.1007/s13770-019-00179-x
Venkatesan, J. K., Schmitt, G., Speicher-Mentges, S., Orth, P., Madry, H., and Cucchiarini, M. (2022). Effects of recombinant adeno-associated virus-mediated overexpression of bone morphogenetic *protein 3 on the chondrogenic fate of human bone marrow-derived mesenchymal stromal cells. Hum. Gene Ther. 33, 950–958. doi:10.1089/hum.2022.004
Vinatier, C., Bouffi, C., Merceron, C., Gordeladze, J., Brondello, J.-M., Jorgensen, C., et al. (2009). Cartilage tissue engineering: towards a biomaterial-assisted mesenchymal stem cell therapy. Curr. Stem Cell Res. Ther. 4, 318–329. doi:10.2174/157488809789649205
Vincent, T. L., Alliston, T., Kapoor, M., Loeser, R. F., Troeberg, L., and Little, C. B. (2022). Osteoarthritis pathophysiology: therapeutic target discovery may require a multifaceted approach. Clin. Geriatr. Med. 38, 193–219. doi:10.1016/j.cger.2021.11.015
Vincent, T. L. (2019). Mechanoflammation in osteoarthritis pathogenesis. Semin. Arthritis Rheum. 49, S36–S38. doi:10.1016/j.semarthrit.2019.09.018
Vincent, T. L. (2020). Of mice and men: converging on a common molecular understanding of osteoarthritis. Lancet Rheumatol. 2, e633–e645. doi:10.1016/S2665-9913(20)30279-4
Vinod, E., Padmaja, K., Ramasamy, B., and Sathishkumar, S. (2023). Systematic review of articular cartilage derived chondroprogenitors for cartilage repair in animal models. J. Orthop. 35, 43–53. doi:10.1016/j.jor.2022.10.012
Viswanathan, S., Shi, Y., Galipeau, J., Krampera, M., Leblanc, K., Martin, I., et al. (2019). Mesenchymal stem versus stromal cells: international society for cell and gene therapy (isct®) mesenchymal stromal cell committee position statement on nomenclature. Cytotherapy 21, 1019–1024. doi:10.1016/j.jcyt.2019.08.002
Vonk, L. A., de Windt, T. S., Slaper-Cortenbach, I. C. M., and Saris, D. B. F. (2015). Autologous, allogeneic, induced pluripotent stem cell or a combination stem cell therapy? Where are we headed in cartilage repair and why: A concise review. Stem Cell Res. Ther. 6, 94. doi:10.1186/s13287-015-0086-1
Vonk, L. A., van Dooremalen, S. F. J., Liv, N., Klumperman, J., Coffer, P. J., Saris, D. B. F., et al. (2018). Mesenchymal stromal/stem cell-derived extracellular vesicles promote human cartilage regeneration in vitro. Theranostics 8, 906–920. doi:10.7150/thno.20746
Voskamp, C., Koevoet, W. J. L. M., Somoza, R. A., Caplan, A. I., Lefebvre, V., van Osch, G. J. V. M., et al. (2020). Enhanced chondrogenic capacity of mesenchymal stem cells after TNFα pre-treatment. Front. Bioeng. Biotechnol. 8, 658. doi:10.3389/fbioe.2020.00658
Walsh, D. P., Raftery, R. M., Murphy, R., Chen, G., Heise, A., O’Brien, F. J., et al. (2021). Gene activated scaffolds incorporating star-shaped polypeptide-PDNA nanomedicines accelerate bone tissue regeneration in vivo. Biomater. Sci. 9, 4984–4999. doi:10.1039/d1bm00094b
Wang, Y., Shimmin, A., Ghosh, P., Marks, P., Linklater, J., Connell, D., et al. (2017). Safety, tolerability, clinical, and joint structural outcomes of a single intra-articular injection of allogeneic mesenchymal precursor cells in patients following anterior cruciate ligament reconstruction: A controlled double-blind randomised trial. Arthritis Res. Ther. 19, 180. doi:10.1186/s13075-017-1391-0
Watson Levings, R. S., Broome, T. A., Smith, A. D., Rice, B. L., Gibbs, E. P., Myara, D. A., et al. (2018b). Gene therapy for osteoarthritis: pharmacokinetics of intra-articular self-complementary adeno-associated virus interleukin-1 receptor antagonist delivery in an equine model. Hum. Gene Ther. Clin. Dev. 29, 90–100. doi:10.1089/humc.2017.142
Watson Levings, R. S., Smith, A. D., Broome, T. A., Rice, B. L., Gibbs, E. P., Myara, D. A., et al. (2018a). Self-complementary adeno-associated virus-mediated interleukin-1 receptor antagonist gene delivery for the treatment of osteoarthritis: test of efficacy in an equine model. Hum. Gene Ther. Clin. Dev. 29, 101–112. doi:10.1089/humc.2017.143
Weissman, D. (2015). MRNA transcript therapy. Expert Rev. Vaccines 14, 265–281. doi:10.1586/14760584.2015.973859
Wluka, A. E., Wolfe, R., Stuckey, S., and Cicuttini, F. M. (2004). How does tibial cartilage volume relate to symptoms in subjects with knee osteoarthritis? Ann. Rheum. Dis. 63, 264–268. doi:10.1136/ard/2003.007666
Wu, H., Peng, Z., Xu, Y., Sheng, Z., Liu, Y., Liao, Y., et al. (2022). Engineered adipose-derived stem cells with IGF-1-modified MRNA ameliorates osteoarthritis development. Stem Cell Res. Ther. 13, 19. doi:10.1186/s13287-021-02695-x
Xi, X.-M., Xia, S.-J., and Lu, R. (2021). Drug loading techniques for exosome-based drug delivery systems. Pharmazie 76, 61–67. doi:10.1691/ph.2021.0128
Xu, M., Shaw, G., Murphy, M., and Barry, F. (2019). Induced pluripotent stem cell-derived mesenchymal stromal cells are functionally and genetically different from bone marrow-derived mesenchymal stromal cells. Stem Cells 37, 754–765. doi:10.1002/stem.2993
Yan, H.-C., Yu, T.-T., Li, J., Qiao, Y.-Q., Wang, L.-C., Zhang, T., et al. (2020). The delivery of extracellular vesicles loaded in biomaterial scaffolds for bone regeneration. Front. Bioeng. Biotechnol. 8, 1015. doi:10.3389/fbioe.2020.01015
Yang, J., Li, Y., Liu, Y., Li, D., Zhang, L., Wang, Q., et al. (2019). Influence of hydrogel network microstructures on mesenchymal stem cell chondrogenesis in vitro and in vivo. Acta Biomater. 91, 159–172. doi:10.1016/j.actbio.2019.04.054
Yang, J., Zhang, Y. S., Yue, K., and Khademhosseini, A. (2017). Cell-laden hydrogels for osteochondral and cartilage tissue engineering. Acta Biomater. 57, 1–25. doi:10.1016/j.actbio.2017.01.036
Yazici, Y., McAlindon, T. E., Gibofsky, A., Lane, N. E., Clauw, D., Jones, M., et al. (2020). Lorecivivint, a novel intraarticular CDC-like kinase 2 and dual-specificity tyrosine phosphorylation-regulated kinase 1A inhibitor and wnt pathway modulator for the treatment of knee osteoarthritis: A phase II randomized trial. Arthritis Rheumatol. 72, 1694–1706. doi:10.1002/art.41315
Zachos, T. A., Shields, K. M., and Bertone, A. L. (2006). Gene-Mediated osteogenic differentiation of stem cells by bone morphogenetic proteins-2 or -6. J. Orthop. Res. 24, 1279–1291. doi:10.1002/jor.20068
Zachos, T., Diggs, A., Weisbrode, S., Bartlett, J., and Bertone, A. (2007). Mesenchymal stem cell-mediated gene delivery of bone morphogenetic protein-2 in an articular fracture model. Mol. Ther. 15, 1543–1550. doi:10.1038/sj.mt.6300192
Zhang, F.-X., Liu, P., Ding, W., Meng, Q.-B., Su, D.-H., Zhang, Q.-C., et al. (2021). Injectable mussel-inspired highly adhesive hydrogel with exosomes for endogenous cell recruitment and cartilage defect regeneration. Biomaterials 278, 121169. doi:10.1016/j.biomaterials.2021.121169
Zhang, J., Wehrle, E., Adamek, P., Paul, G. R., Qin, X.-H., Rubert, M., et al. (2020). Optimization of mechanical stiffness and cell density of 3D bioprinted cell-laden scaffolds improves extracellular matrix mineralization and cellular organization for bone tissue engineering. Acta Biomater. 114, 307–322. doi:10.1016/j.actbio.2020.07.016
Zhang, W., De La Vega, R. E., Coenen, M. J., Müller, S. A., Peniche Silva, C. J., Aneja, M. K., et al. (2019). An improved, chemically modified RNA encoding BMP-2 enhances osteogenesis in vitro and in vivo. Tissue Eng. Part A 25, 131–144. doi:10.1089/ten.TEA.2018.0112
Keywords: osteoarthritis, regenerative medicine, orthobiologics, stem cells, extracellular vesicles, gene therapy, RNA therapeutics
Citation: Velot É, Balmayor ER, Bertoni L, Chubinskaya S, Cicuttini F, de Girolamo L, Demoor M, Grigolo B, Jones E, Kon E, Lisignoli G, Murphy M, Noël D, Vinatier C, van Osch GJVM and Cucchiarini M (2023) Women’s contribution to stem cell research for osteoarthritis: an opinion paper. Front. Cell Dev. Biol. 11:1209047. doi: 10.3389/fcell.2023.1209047
Received: 20 April 2023; Accepted: 18 September 2023;
Published: 19 December 2023.
Edited by:
Giovanna Orsini, Marche Polytechnic University, ItalyReviewed by:
Matilde Cescon, University of Padua, ItalyCopyright © 2023 Velot, Balmayor, Bertoni, Chubinskaya, Cicuttini, de Girolamo, Demoor, Grigolo, Jones, Kon, Lisignoli, Murphy, Noël, Vinatier, van Osch and Cucchiarini. This is an open-access article distributed under the terms of the Creative Commons Attribution License (CC BY). The use, distribution or reproduction in other forums is permitted, provided the original author(s) and the copyright owner(s) are credited and that the original publication in this journal is cited, in accordance with accepted academic practice. No use, distribution or reproduction is permitted which does not comply with these terms.
*Correspondence: Émilie Velot, ZW1pbGllLnZlbG90QHVuaXYtbG9ycmFpbmUuZnI=; Magali Cucchiarini, bW1jdWNjaGlhcmluaUBob3RtYWlsLmNvbQ==
Disclaimer: All claims expressed in this article are solely those of the authors and do not necessarily represent those of their affiliated organizations, or those of the publisher, the editors and the reviewers. Any product that may be evaluated in this article or claim that may be made by its manufacturer is not guaranteed or endorsed by the publisher.
Research integrity at Frontiers
Learn more about the work of our research integrity team to safeguard the quality of each article we publish.