- 1Disease Modeling Laboratory, Department of Microbiology, Institute of Biomedical Sciences, University of São Paulo, São Paulo, Brazil
- 2Neurobiology Laboratory, Scientific Platform Pasteur-USP, São Paulo, Brazil
Peripheral nervous system (PNS) sensory alterations are present in several pathologies and syndromes. The use of induced pluripotent stem cell (iPSC) technology is an important strategy to produce sensory neurons in patients who are accomplished in terms of sensory symptoms. The iPSC technology relies on manipulating signaling pathways to resemble what occurs in vivo, and the iPSCs are known to carry a transcriptional memory after reprogramming, which can affect the produced cell. To this date, protocols described for sensory neuron production start using iPSCs derived from skin fibroblasts, which have the same ontogenetic origin as the central nervous system (CNS). Since it is already known that the cells somehow resemble their origin even after cell reprogramming, PNS cells should be produced from cells derived from the neural crest. This work aimed to establish a protocol to differentiate sensory neurons derived from stem cells from human exfoliated deciduous teeth (SHED) with the same embryonic origin as the PNS. SHED-derived iPSCs were produced and submitted to peripheral sensory neuron (PSN) differentiation. Our protocol used the dual-SMAD inhibition method, followed by neuronal differentiation, using artificial neurotrophic factors and molecules produced by human keratinocytes. We successfully established the first protocol for differentiating neural crest and PNS cells from SHED-derived iPSCs, enabling future studies of PNS pathologies.
1 Introduction
The use of induced pluripotent stem cells (iPSCs) in disease modeling and drug screening has been widely spread in biomedicine (Omole & Fakoya, 2018) but mainly as a tool for the study of neurodegenerative and neurodevelopmental disorders probably because it somehow allows access to the central nervous system (CNS), permitting the exploration of molecular pathways of certain subtypes of active human neurons and other neural cells (Marchetto et al., 2011; Russo et al., 2018). Additionally, iPSCs keep the genetic background of the donor, which is especially important to study polygenic and multifactorial disorders like autism spectrum disorder (ASD) (Marchetto et al., 2009). Human iPSCs have been used to generate several neuronal and glial subtypes (Tcw et al., 2017; Bianchi et al., 2018; Jerber et al., 2021; Johns & Maragakis, 2022; Xu et al., 2022), as well as whole-brain organoids (Lancaster et al., 2013) or organoids from specific brain regions (Qian et al., 2018; Jacob et al., 2020), to develop a basis for understanding neurological diseases.
Despite the numerous advances in iPSC-derived nerve cells, there are few protocols to produce sensory neurons, which are the cells that capture environmental or visceral stimuli and transmit them to the CNS (Chambers et al., 2012; Eberhardt et al., 2015; Bear et al., 2016; Guimarães et al., 2018; Schwartzentruber et al., 2018; Mis et al., 2019; Umehara et al., 2020). In addition, sensory neurons have recently been described as very much affected during viral infections, like in the coronavirus disease of 2019 (COVID-19) pandemic, in which 80% of infected people manifested alterations in senses related to the peripheral nervous system (PNS), like ageusia (loss of sense of taste) and anosmia (loss of sense of smell) (Cooper et al., 2020; Patel et al., 2020; Cunhados et al., 2021). Once again, sensorial stimuli seem to be affected in neurodevelopmental disorders, such as ASD and attention-deficit hyperactivity disorder (ADHD), in neuropathic pain, and specific sensory disorders, like sensory processing disorder (SPD) (Koziol et al., 2011; Weiland et al., 2011; Hazen et al., 2014; Labau et al., 2022).
Neurons from the sensory system still face difficulties in differentiation protocols, which impact their use for in vitro modeling (Labau et al., 2022). Sensory neuron differentiation protocols (Chambers et al., 2012; Eberhardt et al., 2015; Guimarães et al., 2018; Schwartzentruber et al., 2018; Mis et al., 2019; Nickolls et al., 2020; Umehara et al., 2020) have been tested on more than 100 different human iPSC (hiPSC) strains, all derived from pulmonary, dermal fibroblasts, or blood, which are cells of mesenchymal origin (LeBleu & Neilson, 2020). It is important to consider that sensory neurons belonging to the PNS originate in the neural crest, derived from ectoderm differentiation during the neurulation process (Huang & Saint- Jeannet, 2004). The neural crest cells act in the formation of several parts of the vertebrate body: in the formation of pigmented cells, originating the ganglia of the peripheral nervous system and composing part of the cartilages and bones of the face; in the cardiac formation (cells that form septa and valves, and muscle cells); and in tooth structure, originating cells forming dentin and, in mammals, pulp, cementum, periodontal ligament, and alveolar bone (Hall, 2009; Szobó and Mayor, 2018). In the pulp of human exfoliated deciduous teeth, there is a population of postnatal multipotent stem cells called stem cells from human exfoliated deciduous teeth (SHED), which have a high proliferation rate and the ability to form clusters, and are osteoinductive in vivo (Miura et al., 2003). Because they share the same embryonic origin as some neural cells, SHED express neuronal and glial markers (Miura et al., 2003), and the dental pulp cells produce neurotrophic factors and interact with peripheral nervous system neurons in vitro (Nosrat et al., 2001). Considering that even after reprogramming hiPSCs maintain a particular transcriptional signature from the donor cell (Marchetto et al., 2009), it is essential to choose for reprograming the cell that is derived from the same germ layer as the final differentiated cell, which is the target of the study. Here, we have established a protocol to obtain functional peripheral sensory neurons (fPSNs) derived from SHED to use as a platform for studying sensory alterations or diseases that affect this system.
2 Materials and equipment
3 Methods
3.1 Ethics statement, human samples, and primary cell culture
This project was approved by the ethics committee (CEP 4.144.470). Subjects were recruited through The Tooth Fairy Project initiative (University of São Paulo—USP), with approval by the Ethics Committee of the Institute of Biosciences CEP- ICB/USP (Protocol CEP/ICB-USP 1001, Biorepository: CAAE 58219416.0.0000.5467). After a complete description of this research, parents were provided with informed consent for their participation in donating the deciduous tooth after the exfoliation. Dental pulp was extracted from the tooth, and the tissue was washed twice using sterile phosphate-buffered saline (PBS; 0.01 M, pH = 7.4) supplemented with 5% antibiotics (500 U/mL penicillin and 500 μg/mL streptomycin, Sigma). After washing, collagenase enzyme type 1 (Gibco, Life Technologies) was used for tissue matrix digestion isolating cells. SHED were cultivated with Dulbecco’s modified Eagle’s medium (DMEM)/Ham’s F12 (1:1, Invitrogen) supplemented with 15% fetal bovine serum (FBS), 1% penicillin–streptomycin, 1% glutamine, and 1% non-essential amino acids. Cells were passed every 4–5 days with the medium being refreshed daily. All cultures were incubated at 37°C with 5% CO2 in a high-humidity environment.
3.2 iPSC generation and maintenance
Cellular reprogramming experiments (Beltrão-Braga et al., 2011; Russo et al., 2018) were conducted using the Sendai virus (CytoTune® - Gibco, Life Technologies) carrying genetic material from Yamanaka’s factors (Oct4, Sox2, Kfl4, and c-Myc) (Takahashi et al., 2007). SHED were transduced, and after 2 days, the cells were transferred to a feeder-layer condition consisting of murine embryonic fibroblasts (MEFs, Millipore) and maintained with DMEM/F12 (Gibco, Life Technologies), 20% KnockOut Serum Replacement (Invitrogen), 1% non-essential amino acids, 100 μM beta-mercaptoethanol, and fibroblast growth factor 2 (FGF2) (30 ηg/mL). The iPSC colonies, identified after approximately 3 weeks, were transferred to Matrigel (BD Biosciences)-coated plates. Once iPSCs were on feeder-free plates, they were maintained in mTeSR media (Stem Cell Technologies) and changed daily. These cells underwent G-banding karyotype performed at Children’s Hospital of Los Angeles, CA, to confirm if all isolated clones maintained the normal karyotype after reprogramming, and all abnormal karyotype cell lines were discarded (Russo et al., 2018).
3.3 Conditioned medium for human epidermal keratinocyte culture
The human epidermal keratinocytes (HEKs), foreskin-originated, were cultured in KGM medium (Lonza), and when the plate reached 70% confluence, the keratinocyte medium was changed to 3 N media, which was described in the following section, and maintained for 48 h for conditioning. The conditioned medium was centrifuged before use to remove debris, and it was used in the final stage of the protocol for the generation of fPSN.
3.4 Functional peripheral sensory neuron differentiation
The differentiation protocol to produce fPSNs was based on Guimarães et al. (2018). The medium used for fPSN differentiation was 3 N medium: to prepare 100 mL of the medium, combine 48.5 mL of DMEM-F12 (Gibco), 48.5 mL of the Neurobasal medium (Gibco), 1 mL of Glutamax (100x; Gibco), 1 mL of non-essential amino acids (NEAAs) (100x; Gibco), 1 mL of N2 supplement (100x; Gibco), 2 mL of B27 supplement (50x; Gibco), 90 µL of beta-mercaptoethanol (1000x; Gibco), and 1 mL of penicillin–streptomycin (10,000 U/mL; Gibco).
When the iPSC reached approximately 60% confluence with small colonies (∼500µm in diameter), the mTeSR medium was changed to a 3 N medium supplemented with 500 nM of LDN-193189 and 10 µM of SB431541 for 24 h. After this period, 3 µM of CHIR-99021 and the other supplements were added. The next day, LDN-193189 was removed, and after another 24 h, SB431541 was also removed, leaving only CHIR-99021 for another 6 days, with media being changed every 2 days. After this period, the cells were transferred using Accutase diluted in 1 × PBS (1:1) to 6-cm plates treated with poly-L-ornithine (10 μg/mL) and laminin (2.5 μg/mL) (PO-lam). From this stage, they were named neural crest progenitor cells (NCPCs) and were cultured with a 3 N medium supplemented with 10 ng/mL of FGF2 and 10 ng/mL of epidermal growth factor (EGF) for expansion and stock. NCPC expansion was performed by adding Accutase to the plate and leaving it for 5 min in an incubator at 37°C to detach. Twice as much medium was added to the Accutase–cell mixture to stop the reaction, and the cells were centrifuged for 5 min at 1000 rpm. Cells were re-suspended in a 3 N medium supplemented with FGF and EGF, and plated on a PO-lam-treated plate. When the NCPC reached approximately 80% of confluence, cells were seeded in a 24-well plate treated with PO-lam using the same medium and supplements described previously. The differentiation of peripheral sensory neurons (PSNs) started 24 h after seeding. For this procedure, the FGF and EGF were removed, and other factors such as 10 ng/mL brain-derived neurotrophic factor (BDNF), 200 µM ascorbic acid, 10 ng/mL glial cell line-derived neurotrophic factor (GDNF), 10 ng/mL nerve growth factor (NGF), 10 ng/mL neurotrophin-3 (NT-3), and 0.5 mM cyclic adenosine monophosphate (cAMP) were added. This condition was maintained for 20 days with a media change every 5 days, and, in the end, the PSNs were obtained.
After this period, the PSN cultures were maintained with 75% of the conditioned medium on HEK and 25% of the new 3 N medium for another 10 days to mature and obtain fPSNs. All six supplements were also added in this stage.
3.5 Cellular characterization by immunofluorescence assay
Cells were fixed in 4% paraformaldehyde (PFA) for 15 min at room temperature (RT) and permeabilized with 0.1% Triton X-100 for 15 min at RT. Fixed cells were blocked with 2% bovine serum albumin (BSA) (Sigma-Aldrich) for 4 h at RT and then incubated overnight with the primary antibodies (Table 1) at 4°C. The following day, cells were washed thrice with dPBS and blocked again for 1 hour with 2% BSA at RT. Secondary antibodies, such as goat anti-mouse Alexa Fluor 488 (Thermo Fisher Scientific), goat anti-rabbit Alexa Fluor 555 (Abcam), mouse anti-goat Alexa Fluor 555 (Abcam), goat anti-chicken Alexa Fluor 488 (Abcam), and goat anti-mouse Alexa Fluor 555 (Thermo Fisher Scientific), at 1:500 were added for 1 hour at RT. Cells were triple-washed with dPBS, and nuclei were stained using DAPI (Invitrogen) at 1:10,000 diluted in a 1x dPBS solution for 5 min. Cells were washed once after the DAPI addition with dPBS and mounted using the ProLong Gold Antifade Reagent (Invitrogen). Images were acquired using the AMG EVOS FL digital inverted microscope (Thermo Fisher Scientific.) and the LEICA DMi8 wide-field microscope (Leica Microsystems).
3.6 Immunofluorescence quantification and statistics
Image processing was performed using ImageJ and LASX software applications (Leica Microsystems). The quantification of synapsin and peripherin was obtained by LASX software, and the calculation of the relative fluorescence intensity (RFI) was performed by dividing the fluorescence intensity (F(t)) of each image by the fluorescence of the nuclear marker (F(0)), subtracting their respective background fluorescence (Fb). (RFI = [F(t)-Fb(t)]/[F(0)-Fb(0)]). Graphical and statistical analyses were based on the RFI of 20 images and were performed using Prism software (GraphPad), applying an unpaired t-test with Welch’s correction.
4 Results
Our group was the first to establish the protocol to produce fPSNs derived from SHED-iPSC. fPSNs were produced based on a protocol previously established for iPSCs derived from fibroblasts (Guimarães et al., 2018). The protocol is represented in Figure 1.
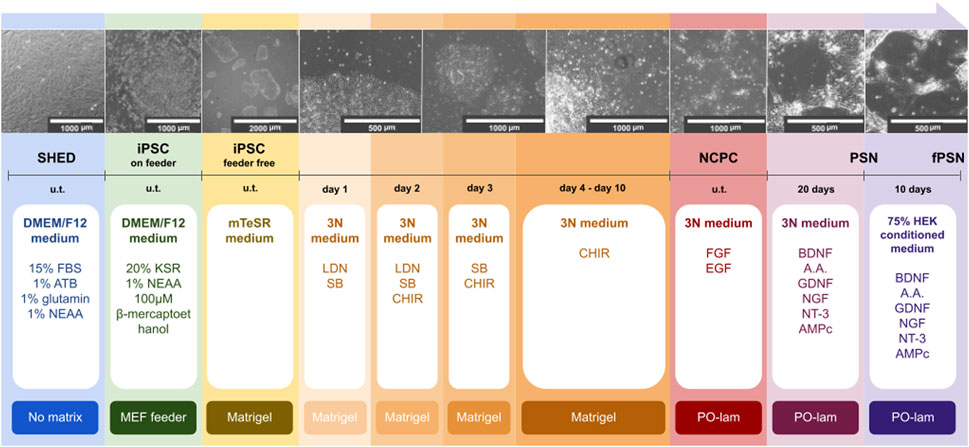
FIGURE 1. Schematic representation of the sensory neuron differentiation protocol. SHED were isolated from neurotypical individuals. Cells were reprogrammed into iPSCs using Sendai virus (u.t., undetermined time). The production of fPSNs was based on a previously described protocol (Guimarães et al., 2018). Scale bars: 2000, 1000, and 500 µm.
SHED were obtained from neurotypical individuals. Cellular reprogramming was highly effective, with small iPSC colonies being observed 25 days after transduction (Figures 2A–G), which were collected and established in feeder-free culture conditions (without a supporting cell layer) (Figure 2H). The iPSC colonies showed a delimited border with a phase-bright center, and the cells had a prominent nucleus and less cytoplasm (Figure 2I). The expression of pluripotent cell markers (Sox2, NANOG, and Oct4) was analyzed by immunofluorescence assays, confirming the success of cell reprogramming (Figures 2J–L).
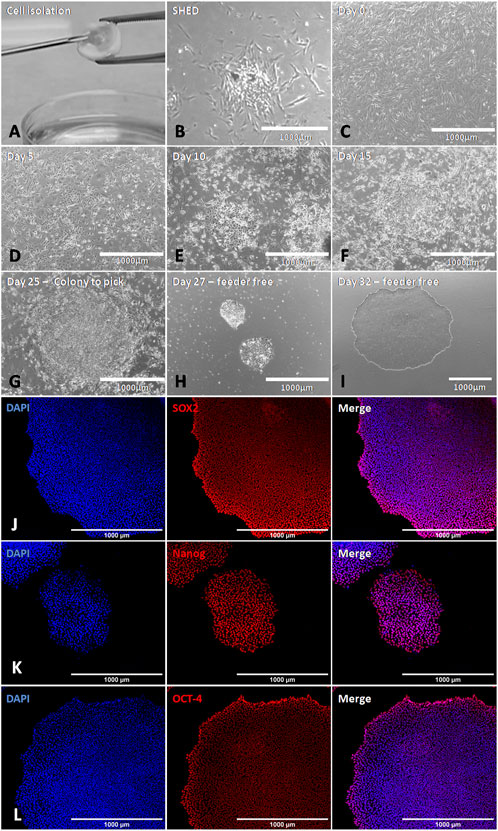
FIGURE 2. Generation of iPSCs derived from SHED using Sendai virus. (A,B) Representative images of SHED isolation and primary culture. (C) SHED before the virus infection with 1 × 105 cells (day 0). (D–F) SHED-iPSCs cultivated on MEF (days 5, 10, and 15 after transduction). We can observe the aspect of iPSC colonies growing on the feeder. (G) SHED-iPSCs on MEF 25 days after transduction. At this moment, the colony is ready to pick. (H,I) iPSC colonies on the feeder-free condition. (J–L) iPSCs showed expression for Sox2, NANOG, and Oct4. Scale bar: 1000 µm.
The iPSC colonies must be small (∼500µm) to start the NCPC differentiation (Figure 3A) since this makes the factors reach the colony’s interior and prevents them from growing uncontrollably until day 11. Around day 3, the colony’s border is no longer delimited, and it becomes possible to observe cytoplasmic expansions on it (Figure 3B). When the culture is only supplemented with CHIR-99021, on day 6, some cells begin to migrate out of the colonies (Figure 3C), and on day 8, colonies may show overgrowth and an increased risk of detachment (Figure 3D). After transferring the cells to PO-lam-treated plates, it may take a few days to become established, and cells can form cell aggregates at this stage (Figure 3E). As soon as the NCPC (Figure 3F) reached 70% confluence, expansion and storage of these cells were performed. Cellular characterization was performed after the fourth passage. The NCPC showed expression for nestin and peripherin, an intermediate filament protein from proliferation cells and peripheral nervous system neurons, respectively (Figure 3G).
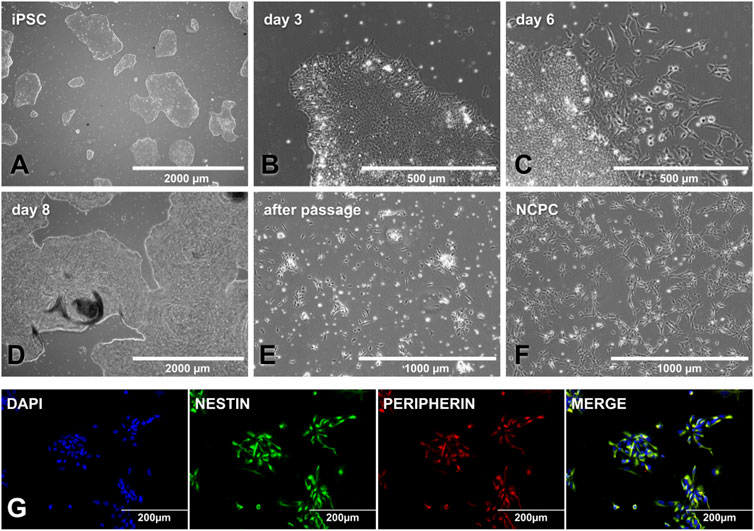
FIGURE 3. NCPC differentiation and characterization. (A) SHED-iPSC colonies ready to start differentiation, with a colony size of approximately 500 μm. (B) Day 3 NCPC differentiation, with the stretched cells in the colony edge. (C) Day 6 NCPC differentiation, when the cells start migrating from the colony. (D) Day 8 NCPC differentiation, where colonies may have overgrowth. (E) Cells as soon as they are transferred to the PO-lam plate, with some cellular clusters. (F). NCPC-established culture. (G). NCPC-expressed nestin (the neuroprogenitor marker) and peripherin (peripheral nervous system cell intermediate filament protein). Scale bar: 2000 µm (A and D), 1000 µm (E, F), 500 µm (B, C), and 200 µm (G).
From the NCPCs (Figure 4A), the PSN already assumed a neuronal morphology after 20 days of differentiation (Figure 4B), and, as expected, the neurite branches increased after the addition of HEK-conditioned medium during the last 10 days of the protocol (Figure 4C).
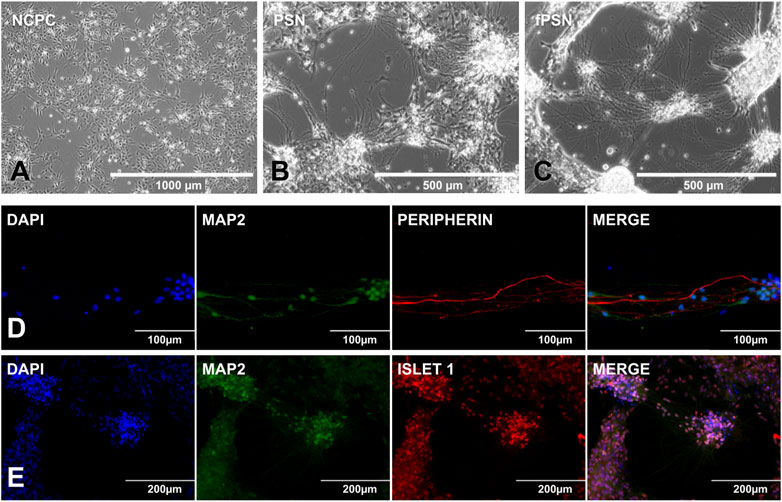
FIGURE 4. PSN differentiation and characterization. (A) NCPCs with ideal confluence to start PSN differentiation. (B) PSN after 20 days of differentiation. Cells showed a neuronal morphology with cells connected by cytoplasm extensions called neurites. (C) Functional PSNs (fPSNs) after 10 days of culture with the HEK-conditioned medium. fPSNs showed expression for MAP2, peripherin (D), and ISLET1 (E), confirming the protocol’s success. Scale bars: 1000 µm (A), 500 µm (B, C), 200 µm (E), and 100 µm (D).
The protocol allowed the differentiation of fPSNs with long neurites of more than 300 μm. fPSN characterization was performed with MAP2, a neuron marker; peripherin (Figure 4D), a marker for peripheral nervous system neurons; and Islet 1 (Figure 4E), highly expressed in fPSNs but not in NCPCs (Guimarães et al., 2018), concluding that the cells obtained using this protocol are specific neurons from the peripheral system. In order to confirm the need to use the conditioned medium to allow the neurons to mature, three culture conditions were tested as follows: 1) using the HEK-conditioned medium during the last 5 days of the protocol; 2) using the HEK-conditioned medium during the last 10 days of the protocol, and 3) without the HEK-conditioned medium during the last 10 days of the protocol.
There was an increase in the number of pre-synaptic markers when 75% of the HEK-conditioned medium was used, in contrast to the protocol using only the 3 N medium (Figures 5B,C,G). Although a variation in the expression of peripherin appears, it is not significant when compared to the use of the HEK-conditioned medium for 5 or 10 days (Figures 5A,B,G). The expression of pre-synaptic markers does not vary significantly between the three culture conditions, although image analysis seems to show a subtle increase in the protocol’s original condition (using a conditioned medium) (Figures 5D–F,H). It lacks other evaluation methods to verify how sensitive this protocol is to changes.
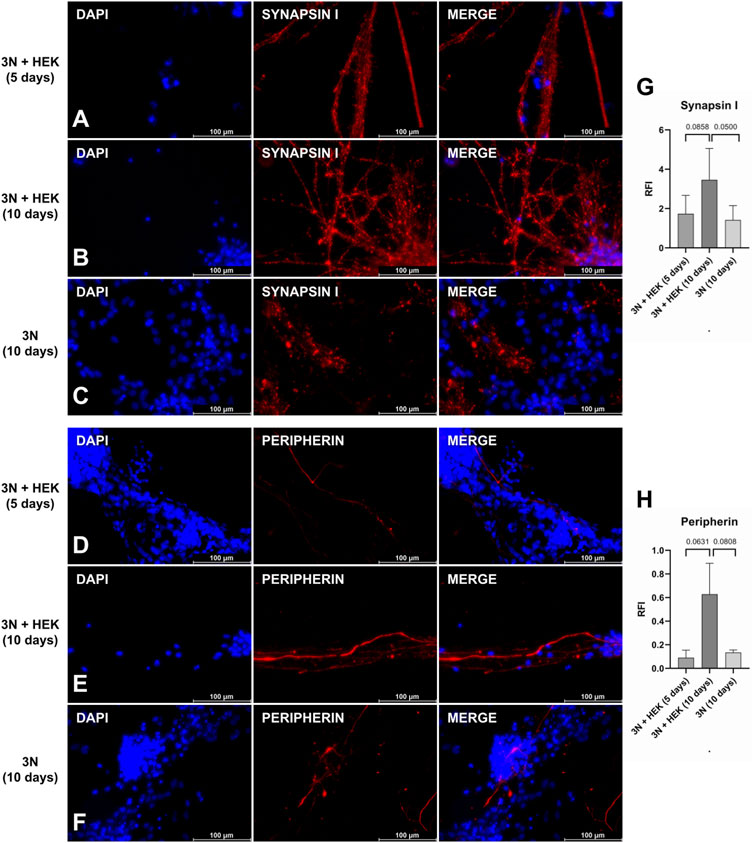
FIGURE 5. Peripheral sensory neurons under protocol modifications. Synapsin I (A–C) and peripherin (D–F) staining: after the application of the original protocol (B and E), in which the last 10 days of culture contain 75% of the HEK-conditioned medium; with reduction of the final phase of the protocol to 5 days, maintaining the conditioned medium (A and D), and keeping the 10-days protocol, but without the conditioned medium, only with 3 N medium (C and F). Quantification of relative fluorescence intensity (RFI) of images stained with synapsin I (G) and peripherin (H) under protocol modification conditions (p-value represented at the top of the graph). A p-value above 0.05 is considered non-significant. Scale bar: 100 µm.
5 Discussion
In this study, we established a protocol of differentiation to obtain NCPCs and fPSNs from iPSCs derived from SHED, aiming at their use in studies related to sensory disorders.
SHED were isolated from the dental pulp after exfoliation. Therefore, it is non-invasive, easy to obtain and isolate, and has an optimal application for studies, in which a patient’s skin biopsy would be impracticable and potentially traumatic, for example, in cases of pediatric diseases or syndromes.
This protocol is similar to the dual-SMAD inhibition method described by Chambers et al. (2012), in which what triggers neuronal differentiation are small inhibitory molecules such as LDN-193189, SB431542, and CHIR-99021, but this method and those with little modifications (Eberhardt et al., 2015; Schwartzentruber et al., 2018; Mis et al., 2019; Umehara et al., 2020) use fibroblast- or blood-derived iPSC lines. The other differentiation protocols for human sensory neurons, those that do not use this dual-SMAD method, start from hiPSCs derived from fibroblasts (Nickolls et al., 2020), from human embryonic stem cells (hESCs) (Bajpai et al., 2010; Schrenk-Siemens et al., 2014; Alshawaf et al., 2018; Hulme et al., 2020) or from murine fibroblasts (Wainger et al., 2014; Blanchard et al., 2015). We were the first group to establish this protocol using human SHED-iPSCs, whose cell source comes from accessible tissues.
This whole protocol takes around 2 months without going through a suspension culture stage, which includes three principal phases: NCPC induction, NCPC maintenance and expansion, and PSN induction and maturation; the latter is divided into two stages, one with only 3 N medium and supplements for 20 days and the other with the addition of the HEK-conditioned medium for 10 days, which seems to be essential for neuronal maturation (Guimarães et al., 2018). To start the protocol, iPSCs should have approximately 60% confluence composed of small colonies as failure to comply with this determination increases the risk of plate detachment, inadequate NCPC differentiation, and low densities in the initial plating in the dual-SMAD inhibition method, and promotes differentiation to neural crest cells (Chambers et al., 2009). In the first passages, NCPC differentiation is not fully established and may show slight changes in morphology and characterization, probably depending on the genetic background of the cell lineage.
Keratinocytes stimulate axonal growth in sensory neurons via the release of NGF and BDNF (Ulmann et al., 2007). In our protocol, these two factors were added exogenously, independent of the presence of the conditioned medium produced from the keratinocytes, and probably were sufficient to promote axonal growth despite the time and the presence of the conditioned medium. It would be interesting to test a co-culture model with sensory neurons and keratinocytes to improve PSN differentiation because recent studies have shown synaptic communication between them (Talagas et al., 2020).
Sensory neurons capture and transmit environmental or visceral stimuli to the CNS (Bear et al., 2016). In addition to the entire PNS, sensory neurons differentiate from neural crest cells during embryogenesis (Hall, 2009; Szobó& Mayor, 2018) from a portion of cells at the edge of the neural plate that starts to separate and migrate during the formation of the neural tube, leading to the origin of CNS cells (de Lahunta et al., 2016). Our protocol differentiates sensory neurons from somatic cells that also originated from the neural crest (Labau et al., 2022), and, as cells after reprogramming still carry their transcriptional signature, it is important that when working with iPSC technology, whenever possible, using the same embryonic origin of the cell source and the cell target of the study after the final differentiation is considered. An advantage presented is that the PSNs generated by this method do not present significant changes in their functionality according to variations in the final phase of the protocol, indicating that they are not as sensitive as those originating from fibroblasts (Schwartzentruber et al., 2018; Umehara et al., 2020), although additional analyses are necessary to confirm this result. Moreover, another benefit of using cells from the dental pulp of the exfoliated deciduous tooth is the low age of the donor, probably with fewer mitochondrial DNA mutations, which can alter the metabolism of the iPSCs (Kang et al., 2016).
This work showed the production of NCPCs and PSNs from SHED, cells with the same ontogenetic origin. Obtaining sensory neurons in vitro can open a wide variety of applications consistently, such as studies of pain or pruritus, the effects of peripheral inflammation and infections, drug testing, and altered sensitivity alterations in diseases or syndromes. Last, starting from the NCPCs established here, it is possible to develop new differentiation protocols to achieve other of its various cellular destinations.
Data availability statement
The original contributions presented in the study are included in the article, further inquiries can be directed to the corresponding author.
Ethics statement
This project was approved by the ethics committee (CEP4.144.470). Subjects were recruited through The Tooth Fairy Project initiative (University of S\xE3o Paulo \x96 USP), with approval from the Ethics Committee of the Institute of Biosciences CEP- ICB/USP (Protocol CEP/ICB-USP 1001, Biorepository: CAAE 58219416.0.0000.5467). Parents were provided with informed consent for their participation to donate the deciduous tooth after the exfoliation.
Author contributions
NO and PB-B designed the experiments. NO carried out the experiments and data analysis. NO, FR, and PB-B wrote the manuscript. All authors contributed to the article and approved the submitted version.
Funding
This work was supported by grants from FAPESP (2018/16,748-8), the NGO The Tooth Fairy Project, the Institut Pasteur (COVID Task Force), and the Coordenação de Aperfeiçoamento de Pessoal de Nível Superior—Brasil (CAPES)—Finance Code 001.
Acknowledgments
The authors would like to thank the individuals that donated the teeth to the NGO “the tooth fairy project, Brazil” and their families. They would also like to thank the agency FAPESP, CAPES, and the University of São Paulo for their support. They are thankful to Doctor Beatriz McGilvray for the text edition.
Conflict of interest
The authors declare that the research was conducted in the absence of any commercial or financial relationships that could be construed as a potential conflict of interest.
Publisher’s note
All claims expressed in this article are solely those of the authors and do not necessarily represent those of their affiliated organizations, or those of the publisher, the editors, and the reviewers. Any product that may be evaluated in this article, or claim that may be made by its manufacturer, is not guaranteed or endorsed by the publisher.
References
Alshawaf, A. J., Viventi, S., Qiu, W., D’Abaco, G., Nayagam, B., Erlichster, M., et al. (2018). Phenotypic and functional characterization of peripheral sensory neurons derived from human embryonic stem cells. Sci. Rep. 8 (1), 603. doi:10.1038/s41598-017-19093-0
Bajpai, R., Chen, D. A., Rada-Iglesias, A., Zhang, J., Xiong, Y., Helms, J., et al. (2010). CHD7 cooperates with PBAF to control multipotent neural crest formation. Nature 463 (7283), 958–962. doi:10.1038/nature08733
Bear, M. F., Connors, B. W., and Paradiso, M. A. (2016). Neuroscience: Exploring the brain. 4th ed. Philadelphia ; Baltimore ; New York: Wolters Kluwer, Cop.
Beltrão-Braga, P. C. B., Pignatari, G. C., Maiorka, P. C., Oliveira, N. A. J., Lizier, N. F., Wenceslau, C. V., et al. (2011). Feeder-free derivation of induced pluripotent stem cells from human immature dental pulp stem cells. Cell Transplant. 20 (11-12), 1707–1719. doi:10.3727/096368911x566235
Bianchi, F., Malboubi, M., Li, Y., George, J. H., Jerusalem, A., Szele, F., et al. (2018). Rapid and efficient differentiation of functional motor neurons from human iPSC for neural injury modelling. Stem Cell Res. 32, 126–134. doi:10.1016/j.scr.2018.09.006
Blanchard, J. W., Eade, K. T., Szűcs, A., Lo Sardo, V., Tsunemoto, R. K., Williams, D., et al. (2015). Selective conversion of fibroblasts into peripheral sensory neurons. Nat. Neurosci. 18 (1), 25–35. doi:10.1038/nn.3887
Chambers, S. M., Fasano, C. A., Papapetrou, E. P., Tomishima, M., Sadelain, M., and Studer, L. (2009). Highly efficient neural conversion of human ES and iPS cells by dual inhibition of SMAD signaling. Nat. Biotechnol. 27 (3), 275–280. doi:10.1038/nbt.1529
Chambers, S. M., Qi, Y., Mica, Y., Lee, G., Zhang, X.-J., Niu, L., et al. (2012). Combined small-molecule inhibition accelerates developmental timing and converts human pluripotent stem cells into nociceptors. NatureBiotechnology 30 (7), 715–720. doi:10.1038/nbt.2249
Cooper, K. W., Brann, D. H., Farruggia, M. C., Bhutani, S., Pellegrino, R., Tsukahara, T., et al. (2020). COVID-19 and the chemical senses: Supporting players take center stage. Neuron 107 (2), 219–233. doi:10.1016/j.neuron.2020.06.032
Cunha, M., dos, P., Vilela, A. P. P., Molina, C. V., Acuña, S. M., Muxel, S. M., et al. (2021). Atypical prolonged viral shedding with intra-host SARS-CoV-2 evolution in a mildly affected symptomatic patient. Front. Med. 8, 760170. doi:10.3389/fmed.2021.760170
de Lahunta, A., Glass, E. N., and Kent, M. (2016). Embryonic development of the central nervous system. Veterinary Clin. N. Am Small Animal Pract. 46 (2), 193–216. doi:10.1016/j.cvsm.2015.10.011
Eberhardt, E., Havlicek, S., Schmidt, D., Link, Andrea S., Neacsu, C., Kohl, Z., et al. (2015). Pattern of functional TTX-resistant sodium channels reveals a developmental stage of human iPSC- and ESC-derived nociceptors. Stem Cell Rep. 5 (3), 305–313. doi:10.1016/j.stemcr.2015.07.010
Guimarães, M. Z. P., De Vecchi, R., Vitória, G., Sochacki, J. K., Paulsen, B. S., Lima, I., et al. (2018). Generation of iPSC-derived human peripheral sensory neurons releasing substance P elicited by TRPV1 agonists. Front. Mol. Neurosci. 11, 277. doi:10.3389/fnmol.2018.00277
B. K. Hall (Editor) (2009). The neural crest and neural crest cells in vertebrate development and evolution. (Boston, MA: Springer US). doi:10.1007/978-0-387-09846-3
Hazen, E. P., Stornelli, J. L., O’Rourke, J. A., Koesterer, K., and McDougle, C. J. (2014). Sensory symptoms in autism Spectrum disorders. Harv. Rev. Psychiatry 22 (2), 112–124. doi:10.1097/01.hrp.0000445143.08773.58
Huang, X., and Saint-Jeannet, J.-P. (2004). Induction of the neural crest and the opportunities of life on the edge. Dev. Biol. 275 (1), 1–11. doi:10.1016/j.ydbio.2004.07.033
Hulme, A. J., McArthur, J. R., Maksour, S., Miellet, S., Ooi, L., Adams, D. J., et al. (2020). Molecular and functional characterization of neurogenin-2 induced human sensory neurons. Front. Cell. Neurosci. 14, 600895. doi:10.3389/fncel.2020.600895
Jacob, F., Pather, S. R., Huang, W.-K., Zhang, F., Wong, S. Z. H., Zhou, H., et al. (2020). Human pluripotent stem cell-derived neural cells and brain organoids reveal SARS-CoV-2 neurotropism predominates in choroid plexus epithelium. Cell Stem Cell 27 (6), 937–950.e9. doi:10.1016/j.stem.2020.09.016
Jerber, J., Seaton, D. D., Cuomo, A. S. E., Kumasaka, N., Haldane, J., Steer, J., et al. (2021). Population-scale single-cell RNA-seq profiling across dopaminergic neuron differentiation. Nat. Genet. 53 (3), 304–312. doi:10.1038/s41588-021-00801-6
Johns, A. E., and Maragakis, N. J. (2022). Exploring motor neuron diseases using iPSC platforms. Stem Cells 40, 2–13. doi:10.1093/stmcls/sxab006
Kang, E., Wang, X., Tippner-Hedges, R., Ma, H., Folmes, Clifford D. L., Gutierrez, N., et al. (2016). Age-related accumulation of somatic mitochondrial DNA mutations in adult-derived human iPSCs. Cell Stem Cell 18 (5), 625–636. doi:10.1016/j.stem.2016.02.005
Koziol, L. F., Budding, D. E., and Chidekel, D. (2011). Sensory integration, sensory processing, and sensory modulation disorders: Putative functional neuroanatomic underpinnings. Cerebellum 10 (4), 770–792. doi:10.1007/s12311-011-0288-8
Labau, J. I. R., Andelic, M., Faber, C. G., Waxman, S. G., Lauria, G., and Dib-Hajj, S. D. (2022). Recent advances for using human induced-pluripotent stem cells as pain-in-a-dish models of neuropathic pain. Exp. Neurol. 358, 114223. doi:10.1016/j.expneurol.2022.114223
Lancaster, M. A., Renner, M., Martin, C.-A., Wenzel, D., Bicknell, L. S., Hurles, M. E., et al. (2013). Cerebral organoids model human brain development and microcephaly. Nature 501 (7467), 373–379. doi:10.1038/nature12517
LeBleu, V. S., and Neilson, E. G. (2020). Origin and functional heterogeneity of fibroblasts. FASEB J. 34 (3), 3519–3536. doi:10.1096/fj.201903188r
Marchetto, M. C., Brennand, K. J., Boyer, L. F., and Gage, F. H. (2011). Induced pluripotent stem cells (iPSCs) and neurological disease modeling: Progress and promises. Hum. Mol. Genet. 20 (R2), R109–R115. doi:10.1093/hmg/ddr336
Marchetto, M. C. N., Yeo, G. W., Kainohana, O., Marsala, M., Gage, F. H., and Muotri, A. R. (2009). Transcriptional signature and memory retention of human-induced pluripotent stem cells. PLoS ONE 4 (9), e7076. doi:10.1371/journal.pone.0007076
Mis, M. A., Yang, Y., Tanaka, B. S., Gomis-Perez, C., Liu, S., Dib-Hajj, F., et al. (2019). Resilience to pain: A peripheral component identified using induced pluripotent stem cells and dynamic clamp. J. Neurosci. 39 (3), 382–392. doi:10.1523/JNEUROSCI.2433-18.2018
Miura, M., Gronthos, S., Zhao, M., Lu, B., Fisher, L. W., Robey, P. G., et al. (2003). Shed: Stem cells from human exfoliated deciduous teeth. Proc. Natl. Acad. Sci. 100 (10), 5807–5812. doi:10.1073/pnas.0937635100
Nickolls, A. R., Lee, M. M., Espinoza, D. F., Szczot, M., Lam, R. M., Wang, Q., et al. (2020). Transcriptional programming of human mechanosensory neuron subtypes from pluripotent stem cells. Cell Rep. 30 (3), 932–946.e7. doi:10.1016/j.celrep.2019.12.062
Nosrat, I. V., Widenfalk, J., Olson, L., and Nosrat, C. A. (2001). Dental pulp cells produce neurotrophic factors, interact with trigeminal neurons in vitro, and rescue motoneurons after spinal cord injury. Dev. Biol. 238 (1), 120–132. doi:10.1006/dbio.2001.0400
Omole, A. E., and Fakoya, A. O. J. (2018). Ten years of progress and promise of induced pluripotent stem cells: Historical origins, characteristics, mechanisms, limitations, and potential applications. PeerJ 6, e4370. doi:10.7717/peerj.4370
Patel, A., Charani, E., Ariyanayagam, D., Abdulaal, A., Denny, S. J., Mughal, N., et al. (2020). New-onset anosmia and ageusia in adult patients diagnosed with SARS-CoV-2 infection. Clin. Microbiol. Infect. 26 (9), 1236–1241. doi:10.1016/j.cmi.2020.05.026
Qian, X., Jacob, F., Song, M. M., Nguyen, H. N., Song, H., and Ming, G. (2018). Generation of human brain region–specific organoids using a miniaturized spinning bioreactor. Nat. Protoc. 13 (3), 565–580. doi:10.1038/nprot.2017.152
Russo, F. B., Freitas, B. C., Pignatari, G. C., Fernandes, I. R., Sebat, J., Muotri, A. R., et al. (2018). Modeling the interplay between neurons and astrocytes in autism using human induced pluripotent stem cells. Biol. Psychiatry 83 (7), 569–578. doi:10.1016/j.biopsych.2017.09.021
Schrenk-Siemens, K., Wende, H., Prato, V., Song, K., Rostock, C., Loewer, A., et al. (2014). PIEZO2 is required for mechanotransduction in human stem cell–derived touch receptors. Nat. Neurosci. 18 (1), 10–16. doi:10.1038/nn.3894
Schwartzentruber, J., Foskolou, S., Kilpinen, H., Rodrigues, J., Alasoo, K., Knights, A. J., et al. (2018). Molecular and functional variation in iPSC-derived sensory neurons. Nat. Genet. 50 (1), 54–61. doi:10.1038/s41588-017-0005-8
Szabó, A., and Mayor, R. (2018). Mechanisms of neural crest migration. Annu. Rev. Genet. 52 (1), 43–63. doi:10.1146/annurev-genet-120417-031559
Takahashi, K., Tanabe, K., Ohnuki, M., Narita, M., Ichisaka, T., Tomoda, K., et al. (2007). Induction of pluripotent stem cells from adult human fibroblasts by defined factors. Cell 131 (5), 861–872. doi:10.1016/j.cell.2007.11.019
Talagas, M., Lebonvallet, N., Leschiera, R., Sinquin, G., Elies, P., Haftek, M., et al. (2020). Keratinocytes communicate with sensory neurons via synaptic-like contacts. Ann. Neurology 88 (6), 1205–1219. doi:10.1002/ana.25912
Tcw, J., Wang, M., Pimenova, A. A., Bowles, K. R., Hartley, B. J., Lacin, E., et al. (2017). An efficient platform for astrocyte differentiation from human induced pluripotent stem cells. Stem Cell Rep. 9 (2), 600–614. doi:10.1016/j.stemcr.2017.06.018
Ulmann, L., Rodeau, J.-L., Danoux, L., Contet-Audonneau, J.-L., Pauly, G., and Schlichter, R. (2007). Trophic effects of keratinocytes on the axonal development of sensory neurons in a coculture model. Eur. J. Neurosci. 26 (1), 113–125. doi:10.1111/j.1460-9568.2007.05649.x
Umehara, Y., Toyama, S., Tominaga, M., Matsuda, H., Takahashi, N., Kamata, Y., et al. (2020). Robust induction of neural crest cells to derive peripheral sensory neurons from human induced pluripotent stem cells. Sci. Rep. 10 (1), 4360. doi:10.1038/s41598-020-60036-z
Wainger, B. J., Buttermore, E. D., Oliveira, J. T., Mellin, C., Lee, S., Saber, W. A., et al. (2014). Modeling pain in vitro using nociceptor neurons reprogrammed from fibroblasts. Nat. Neurosci. 18 (1), 17–24. doi:10.1038/nn.3886
Weiland, R., Macht, M., Ellgring, H., GroB-Lesch, S., Lesch, K.-P., and Pauli, P. (2011). Olfactory and gustatory sensitivity in adults with attention-deficit/hyperactivity disorder. ADHD Atten. Deficit Hyperactivity Disord. 3 (1), 53–60. doi:10.1007/s12402-010-0052-9
Keywords: iPSC differentiation, peripheral nervous system, peripheral sensory neuron, neural crest cell, SHED
Citation: Oliveira NC, Russo FB and Beltrão-Braga PCB (2023) Differentiation of peripheral sensory neurons from iPSCs derived from stem cells from human exfoliated deciduous teeth (SHED). Front. Cell Dev. Biol. 11:1203503. doi: 10.3389/fcell.2023.1203503
Received: 10 April 2023; Accepted: 26 June 2023;
Published: 13 July 2023.
Edited by:
Zhiping P. Pang, The State University of New Jersey, United StatesReviewed by:
Mohan Chari Vemuri, Jivakana Cell Technologies, Fredrick, MD, USANazmul Haque, TotiCell Limited, Bangladesh
Copyright © 2023 Oliveira, Russo and Beltrão-Braga. This is an open-access article distributed under the terms of the Creative Commons Attribution License (CC BY). The use, distribution or reproduction in other forums is permitted, provided the original author(s) and the copyright owner(s) are credited and that the original publication in this journal is cited, in accordance with accepted academic practice. No use, distribution or reproduction is permitted which does not comply with these terms.
*Correspondence: Patricia C. B. Beltrão-Braga, cGF0cmljaWFjYmJicmFnYUB1c3AuYnI=