- 1Department of Pharmacology and Department of Pharmacy of the Second Affiliated Hospital, Key Laboratory of Medical Neurobiology of the Ministry of Health of China, Zhejiang University School of Medicine, Hangzhou, China
- 2Key Laboratory of Neuropharmacology and Translational Medicine of Zhejiang Province, Zhejiang Chinese Medical University, Hangzhou, China
Febrile seizures (FSs) are convulsions caused by a sudden increase in body temperature during a fever. FSs are one of the commonest presentations in young children, occurring in up to 4% of children between the ages of about 6 months and 5 years old. FSs not only endanger children’s health, cause panic and anxiety to families, but also have many adverse consequences. Both clinical and animal studies show that FSs have detrimental effects on neurodevelopment, that cause attention deficit hyperactivity disorder (ADHD), increased susceptibility to epilepsy, hippocampal sclerosis and cognitive decline during adulthood. However, the mechanisms of FSs in developmental abnormalities and disease occurrence during adulthood have not been determined. This article provides an overview of the association of FSs with neurodevelopmental outcomes, outlining both the underlying mechanisms and the possible appropriate clinical biomarkers, from histological changes to cellular molecular mechanisms. The hippocampus is the brain region most significantly altered after FSs, but the motor cortex and subcortical white matter may also be involved in the development disorders induced by FSs. The occurrence of multiple diseases after FSs may share common mechanisms, and the long-term role of inflammation and γ-aminobutyric acid (GABA) system are currently well studied.
1 Introduction of febrile seizures
1.1 Definition and symptoms of febrile seizures
Febrile seizures (FSs) refer to convulsions induced by a sudden increase in body temperature (>38°C) in the absence of other underlying causes or disorders that induce convulsions, such as central nervous system (CNS) infections, electrolyte abnormalities, withdrawal, trauma, genetic predisposition or known epilepsy (Xixis et al., 2022). Children between the ages of about 6 months and 5 years old are the most likely to experience FSs. FSs are extremely common, occurring in up to 4% of children in this age group. Some children have a single FS event, and others have multiple events over early childhood. Approximately 30–40 percent of children who experience one FS will have a recurrence (Patel et al., 2015; Smith et al., 2019).
According to National Institutes of Health, symptoms of FSs are described as following: lose consciousness, both arms and legs will shake uncontrollably, eye rolling and rigid limbs. Sometimes during a FS, a child may lose consciousness but will not noticeably shake or move. FSs are categorized as either simple FSs or complex FSs: 1) simple FSs last no more than 15 min and this type does not recur within a 24-h period; 2) complex FSs last longer than 15 min, occur more than once within 24 h.
In recent years, the pandemic caused by 2019 coronavirus disease (COVID-19) has aroused widespread concern. One of the commonest clinical manifestations of COVID-19 is fever, and COVID-19 has become a common cause of FSs. As of April 2022, 13 million cases of COVID-19 have been reported among children and adolescents in the United States, accounting for nearly 20% of all cases in the United States. Among 15,137 children hospitalized for COVID-19, the most common neurological complication was FSs (3.9%) (Antoon et al., 2022). Although the incidence of seizures in children with fever during the COVID-19 pandemic has significantly increased (Iijima et al., 2022; Joung et al., 2023), in the latest retrospective case-control study, the risk of FSs secondary to COVID-19 did not increase compared to other causes (Hanlon et al., 2023). Meanwhile, a retrospective observational cohort study showed an increased frequency of complex FSs diagnoses during the COVID-19 pandemic (Cadet et al., 2023). Further analysis of the clinical features of FSs in patients with and without COVID-19 revealed that patients with FSs in COVID-19 tended to be predominantly male and had a later age of onset compared with non-Covid-19 patients (Seo et al., 2023). Because of the atypical age of onset and the greater likelihood of multiple convulsive episodes, patients (especially males) with COVID-19 require vigilance for FSs.
1.2 Therapeutics of FSs
Since the 1990 s, hundreds of articles have been published on the drug management of FSs. However, this has been a controversial area, and there are various views on drug management. This controversy reflects, in part, the fact that it is uncertain whether prophylactic medications with antiepileptics and antipyretics are effective without important adverse effects. There is no specific treatment for simple or complex FSs other than appropriate management of underlying etiologies driving the persistent febrile illness. Antipyretic drugs do not reduce the recurrence of FSs in children with a history of FSs (Lux, 2010). Diazepam in combination with acetaminophen reduced more seizure recurrences without serious adverse events compared with acetaminophen alone (Tanaka et al., 2022). In most patients in whom a febrile illness leads to frequent recurrence of FSs, studies have evaluated the use of benzodiazepines as a bridging measure during a subsequent febrile episode for several days (Printz et al., 2016; Guedj et al., 2017; Renda et al., 2020). Febrile status epilepticus can occur in less than 10% of children during the first FS. Rectal diazepam is used to abort this disorder if it lasts more than 5 min. There are also recommendations for intranasal midazolam. However, such treatment may have adverse effects on the child’s behavior and cognitive development (Xixis et al., 2022). The decision to treat thus requires assessment of the potential risks and benefits to children. Phenobarbital at times of fever has been proven to be ineffective, probably because of the delay in achieving appropriate serum and tissue levels (Farwell 1990; Herranz 1988). To avoid the side effects of continuous antiepileptic drugs (AEDs), rapid-acting antiepileptics given only during fever periods have been used in an attempt to reduce the risk of recurrent FSs. To date, only prophylactic diazepam (administered orally or rectally) has been studied in placebo-controlled trials. Intermittent diazepam reduces the recurrence rate in children with FSs, but the incidence of adverse effects is as high as 30% (Offringa et al., 2017; Offringa et al., 2021). Current reports have confirmed the exact efficacy of levetiracetam with an extremely low incidence of adverse effects. If this effect is repeated in other studies, levetiracetam may be considered as a prophylactic treatment option in selected families where anxiety over potential FSs recurrence is high. Melatonin treatment had a similarly low incidence of adverse events, and further studies are needed to demonstrate its effectiveness versus placebo (Xixis et al., 2022).
2 Etiology of FSs
2.1 Generation of FSs
Any fever of adequate height may cause FSs. Some clinical studies found that upper respiratory tract infection was determined the commonest disease (81.6%) in FSs followed by acute gastroenteritis (15.4%) and urinary tract infection (3%), respectively (Gunes et al., 2021), but no specific febrile cause was more likely to cause FSs. A large number of clinical and preclinical studies have uncovered the pathological features and possible etiological factors of FSs, such as mutation of fever response genes and γ-aminobutyric acid (GABA) gene, ion channel activity and inflammation (Hessel et al., 2014; Chen et al., 2019). However, there is no generally acknowledged mechanism of FSs.
Briefly, mutation in SCN1A gene, encoding the voltage-gated sodium channel (VGSC) NaV1.1, is widely recognized as a leading cause of genetic FSs, due to the decrease in the Na+ current density, mainly affecting the inhibitory neuronal transmission (Kasperaviciute et al., 2013; Scalise et al., 2020; Scalise et al., 2022). However, failure to detect association between polymorphisms of SCN1A and FSs in Chinese patients suggests that the correlation between SCN1A mutation and FSs may just consist in specific races (Zhang et al., 2010). Mutation in Na+ -channel beta1 subunit gene SCN1B, which usually leads to loss of function, is also associated with FSs (Wallace et al., 1998; Audenaert et al., 2003; Steinlein, 2004). SCN2A is a well-established epilepsy gene, encoding the neuronal sodium channel NaV1.2, may also contribute to the generation of FSs (Saitoh et al., 2015; Berseem et al., 2022; Skotte et al., 2022). GABRG2 mutation associated with FSs has been elucidated (Audenaert et al., 2006; Todd et al., 2014; Haerian et al., 2016). Some other gene mutations are also identified, such as SRP9, ADGRV1 and the fever response genes PTGER3, IL-6 and IL-10 (Shahrokhi et al., 2017; Chen et al., 2019). Last year, the largest genetic study of FSs to date further identified several novel loci strongly associated with FSs. Variants at two loci were functionally associated with altered expression of the fever responsive genes PTGER10 and IL-2, and the other four loci contained genes (BSN, ERC2, GABRG1, HERC1) influencing neuronal excitability by regulating neurotransmitter release and binding, vesicular transport or membrane trafficking at synapses (Hessel et al., 2014; Han et al., 2020; Skotte et al., 2022). Mutations in several genes strongly associated with fever related epilepsy syndromes are also notable, such as STX1B, encoding a presynaptic protein, and CELF4 haploinsufficiency mutations, encoding a splicing regulator (Halgren et al., 2012; Schubert et al., 2014). FSs are serious adverse events following measles, mumps and rubella (MMR) vaccination. A genome-wide association scan identified two loci clearly associated with MMR related febrile seizures, harboring the interferon stimulated gene IFI44L and the measles virus receptor CD46. Two loci associated with FSs in general, ANO3 (also known as TMEM16C), and locus associated with serum magnesium levels have also been identified (Feenstra et al., 2014; Sisodiya, 2014).
Similar to epilepsy, the onset of FSs is also closely associated with neural excitation-inhibition imbalance. Ion channels are key gating channels that control intra and intercellular ion currents as the important regulators of neuronal network excitability. The direct effect of heat on ion channels localized to the site of action potential initiation potentially causes a profound increase in neuronal excitability, which is likely to contribute to FSs genesis (Thomas et al., 2009). At present, several ion channels are known to be involved in FSs, including KCC2 (K+ -Cl− co-transporter, the chloride exporter), NKCC1(Na+-K+-2Cl− cotransporter, the chloride importer) (Asisipo et al., 2020), TRPV1 (transient receptor potential vanilloid-1) (Barrett et al., 2018) and TRPC3 (a member of canonical transient receptor potential channel) (Sun et al., 2018).
Inflammatory elements have major roles in FSs pathogenesis. A meta-analysis showed that IL-6 (−572, −174, −597) polymorphisms were significantly associated with susceptibility to FSs (Chen et al., 2019). At the protein level, several meta-analyses and researches on animal models suggest that cerebrospinal fluid (CSF) cappase-1/IL-1β level, and serum high mobility group box protein 1 (HMGB1), tumor necrosis factor-α (TNF-α), IL-1β, IL-6 level are associated with an increased risk of FSs in children (Choi et al., 2011; Kwon et al., 2018; Tang et al., 2020; Carman et al., 2021). These inflammatory cytokines impact FSs through changing neuronal excitability, regulating GABAergic transmission, inhibiting BDNF-TrkB signaling or enhancing the NF-κB pathway (Wang et al., 2019; Sun et al., 2020; Tang et al., 2020).
There are other hypotheses about FSs, for instance, respiratory alkalosis induced by hyperthermia (Schuchmann et al., 2006; Barrett et al., 2018), iron deficiency (Vaswani et al., 2010; Papageorgiou et al., 2015), and the role of immunoreactive-arginine vasopressin and immunoreactive-somatostatin (Nagaki et al., 1996) (Supplementary Table S1).
2.2 Recurrence of FSs
According to some follow-up studies, the risk of FSs recurrence decreased linearly with increasing age (−2% per month) and the risk was higher among patients with abnormal electroencephalogram (EEG) (Cappellari et al., 2018). Younger age at first seizure, short duration of fever before the onset of first FS, lower temperature at onset, and family history of FS are risk factors of recurrence of FSs in children (Patel et al., 2015; Kumar et al., 2019).
Currently, however, the recurrence of FSs cannot be effectively predicted, and reliable biomarkers and diagnostic evidence are lacking. Current researches on the mechanisms of recurrence are still progressing. A case-control study in a Romanian pediatric population reported that recurrent crises and repeated episodes of seizures are more frequent in the GABRG2 Asn196Asn TT genotype polymorphism, with 8 times higher risk of developing recurrent FSs (Butila et al., 2018). TRPV1 is a nonselective cation channel, as a key component implicated several inflammatory diseases. A massive amount of evidence has demonstrated that TRPV1 is extensively expressed in the CNS and there might be a close relationship between TRPV1 and neuroinflammation (Kong et al., 2014; Yang et al., 2019; Zhang et al., 2021). It is found that TRPV1 promotes recurrent FSs by increasing pro-inflammatory cytokines (Huang et al., 2015), and TRPV1 was mainly derived from microglia to participate in neuroinflammatory response and recurrent FSs (Kong et al., 2019). There are also several blood analyses. C-reactive protein level, blood glucose level, serum sodium level, serum zinc level and vitamin D were indicated significant association with recurrent FSs (Hugen et al., 1995; Kiviranta and Airaksinen, 1995; Lee and Kim, 2012; Waqar Rabbani et al., 2013; Arul et al., 2020; Bhat et al., 2020; Kubota et al., 2021; Miyagi et al., 2022). To be noted, there is evidence from meta-analysis and cross-sectional studies that hyponatremia and hypozinc may be strongly associated with the recurrence of FSs. However, there is a lack of direct evidence to show whether sodium or zinc supplementation can effectively prevent or treat recurrence of FSs, and it is also unclear whether monitoring blood sodium or zinc in children after first FS is effective in predicting recurrence of FSs. At present, only randomized clinical trials with few sample sizes have found that zinc supplementation can effectively reduce the recurrence rate of FSs (Fallah et al., 2015). The association of hyponatremia with FSs recurrence may be related to mutations in sodium channels in the etiology of FSs, but the evidence is weak (Supplementary Table S2).
3 The long-term adverse outcomes associated with FSs
Electroencephalographic and biochemical long-lasting abnormalities have been reported both in clinical cases and animal models of FSs (Leaffer et al., 2013; Mohammed et al., 2017). At the outset, scholars focused on the association between infant FSs and epileptogenesis in adulthood. More and more data suggest that FSs may also contribute to damage of cognition, motor skills and mental diseases (Leaffer et al., 2013; Rajab et al., 2014; Crespo et al., 2018; Lin et al., 2021; Harris et al., 2022).
3.1 Diseases or pathological manifestations after FSs
3.1.1 Epileptogenesis
In 1997, a study of 44 adult patients suggests that a history of FSs is associated with the subsequent temporal lobe pathology (Barr et al., 1997). The association between temporal lobe epilepsy (TLE) and the history of FSs has since gradually come into public view. There have been numerous cohort studies designed one after another to investigate the association of FSs with epilepsy in adult, with numbers of patients involved ranging from hundreds to millions and time span more than 10–20 years. Although the proportions varied from 5 to 18 folds, these data suggest a significant increase in the incidence of epilepsy after FSs (Vestergaard et al., 2007; Neligan et al., 2012; Chiang et al., 2018; Tsai et al., 2018). Further, of those individuals who experienced FSs, the frequency of subsequent development of epilepsy was 2.15-fold greater in females, 4.846-fold greater in patients with recurrent FSs (Chiang et al., 2018). Researchers assessed predictive value of epileptiform discharges for subsequent epilepsy after FSs and found that patients with normal EEGs were unlikely to develop epilepsy (Kavcic and Rener-Primec, 2018). The important impact of FSs on subsequent epileptogenesis has been recognized, but over a large time span, it is clinically difficult to validate directly. Therefore, animal models of FSs are well-established and the research achievements are considerable.
Although there are some data showing that prolonged experimental FSs lead to adult-onset TLE (Dube et al., 2006), most data suggest that a single episode of prolonged FSs may not induce per se, but accelerates epileptogenesis, and increases seizure susceptibility and severity (Dai et al., 2014; Hamelin et al., 2014; Dutton et al., 2017; Alese et al., 2020). Notably, in animal models, FSs were found to cause interictal epileptifom EEG abnormalities, which is consistent with clinical studies, suggesting detection of EEG may serve as an important means of predicting epileptogenesis after FSs. These data were obtained in adulthood, and whether FS also has alterations in EEG during development requires more evidence. Current studies only found that the seizure susceptibility decreased in 35-day-old (P35) FS rats but increased in P60 FS rats (Feng et al., 2015), but the EEG changes during neurodevelopment until adult were not examined. Dai’s findings provide direct evidence of sex-dependent acquired seizure susceptibility after complex FSs (Dai et al., 2014). Female FSs rats were more susceptible to pentylenetetrazol and maximum electric shock than male FSs rats. The protein expression of interleukin-1β (IL-1β), an inflammatory factor associated with seizure susceptibility, was higher in adult FSs females than in males, which may reflect a gender-specific phenomenon of seizure susceptibility.
3.1.2 Cognitive and memory impairments
Recognition memory is impaired in children after FSs and this memory impairment can persist for at least 10 days to 1 year (Martinos et al., 2012). Cognition impairment, including perceptual reasoning and working memory defects, were identified in patients aged 6–12 years with FSs onset at the age of 2–2.5 years old (Tsai et al., 2015). A generation R study suggested that children with recurrent FSs might be at risk for delayed language development around 6 months after FSs (Visser et al., 2012). Similarly, 4-year-old children with FSs had been identified at child healthcare centers in Gothenburg. One-third of the children had at least one neurodevelopmental disorder diagnosis or marked developmental problems within areas of attention, speech and language or general cognition. No differences were found between children with single vs. recurrent FS or simple vs. complex FSs (Nilsson et al., 2019). These clinical studies did not analyze the key brain regions or molecular targets affected. In addition, reports on whether these cognitive and language impairments extend into adulthood are lacking.
In experimental FSs model, most agree that FSs cause moderate memory impairment, which focus on spatial, working and reference memory (Chang et al., 2003; Dube et al., 2009; Kloc et al., 2022). FSs had transient effects on spatial learning in immature rats in a few days (Yagoubi et al., 2015). But spatial memory problems were identified in male adult rats following experimental prolonged FSs. Remarkably, possible mechanisms underlying these deficits may involve hippocampal impairments of dendritic filtering of cortical inputs discoordination of entorhinal-hippocampal circuit, as well as neuron restrictive silencing factor mediated aberrant generation of excitatory synapses in the dentate gyrus, that results in dendritic loss in the hippocampus (Rajab et al., 2014; Patterson et al., 2017; Kloc et al., 2023). It was interesting that sex seemingly had a remarkable effect on spatial cognitive outcome where adult males with FSs fared worse than adult females with FSs (Kloc et al., 2022). At the same time, there are some reports that FSs also resulted in adult memory deficits in novel object recognition task, inhibitory avoidance task, and contextual fear conditioning task and inhibition of TRPV1 receptors during FSs in part prevented learning deficits in adult (Dai et al., 2019; Harris et al., 2022). Interestingly, prolonged FSs in infant rats caused adult memory deficits that could be transmitted to the next-generation, mainly through the mother, and may be associated with DNA methyltransferase (DNMT) 1 upregulation (Dai et al., 2019). These animal findings pinpoint that FSs may cause cognitive impairment that persists into adulthood. Mechanisms of sex differences and inheritance to the next-generation are insufficiently studied and will be research directions with great clinical significance.
3.1.3 Psychiatric disorders
It was showed the most common psychiatric diagnoses after FSs were anxiety, attention-deficit/hyperactivity, and personality disorders (Dreier et al., 2020). The cohort studies that found the association between FSs and epilepsy also identified a 11.26-fold higher frequency of comorbid autism after FSs compared with controls (Chiang et al., 2018). To exclude the disturbances of epilepsy and focused on analyzing the contribution of FSs per se to psychiatric disorders, one evaluation of long-term risk of psychiatric disorders among 2103232 children with recurrent FSs from Denmark at Aarhus University was conducted. A history of recurrent FSs appears to be associated with a risk of psychiatric disorders, including organic mental disorders, affective mental disorders, schizophrenia, mood disorders, somatoform disorders, intellectual disability, disorders of psychological development, etc. (Dreier et al., 2019). In animal studies, the results of depression-like behavior in rats after FSs showed that FSs significantly reduced the sucrose consumption in the sucrose preference test and increased the immobility time in the forced swim test in P37 and P60. This study also demonstrated that FSs caused depression-like behavioral changes that may be inherited to the next-generation of rats and leaded to increased mGluR3 mRNA expression and mGluR1 gene hypermethylation in two generations of rats (Alese and Mabandla, 2019). But there is no direct evidence that mGluR3 and mGluR1 regulate the inheritance of this depressive phenotype.
3.1.4 Attention deficit hyperactivity disorder (ADHD)
After comparison of relation between ADHD in children with and without FSs, it was concluded that hyperactivity has a significant relation with FSs in male gender (Salehi et al., 2016). After 11 years of follow-up and identified 1,081 children with FSs as the case cohort, Ku et al. found that FSs may increase the risk of subsequent ADHD occurrence in children (Ku et al., 2014). It is reported that the overall risk of ADHD in the FSs+/preterm + group was higher than that in the FSs+/preterm-group. Preterm birth may be a risk factor for subsequent ADHD in children with FSs (Lin et al., 2021). A population-based cohort of 906,379 children born in Denmark were followed up for 22 years. The findings indicated an association between FSs and subsequent development of ADHD, after adjusting for socioeconomic and perinatal risk factors, and family history of epilepsy, or psychiatric disorders (Bertelsen et al., 2016).
3.1.5 Mortality
In current research, the presence or absence of a history of FS episodes had little effect on long-term mortality. 132 of 100 000 children died within 2 years of FSs compared with 67 deaths per 100 000 children without a history of this disorder (Vestergaard et al., 2008). In the nested case-control study, children with simple FSs had a mortality rate similar to that of the background population, whereas mortality was increased for those with complex FSs. This finding can be partially explained by pre-existing neurological abnormalities and subsequent epilepsy. In aforementioned study from Denmark at Aarhus University, increased mortality was found in individuals with a history of FSs who later developed epilepsy (Dreier et al., 2019). In summary, long-term mortality is not increased in children with FS, but there seems to be a small excess mortality after complex FS (Vestergaard et al., 2008). So, we should be reassured that death after FSs is not increased.
3.2 Long-term alterations on tissues or cells after FSs
3.2.1 Temporal abnormalities: hippocampus and amygdala
At the time of the observation of increased epilepsy susceptibility, researchers found substantial hippocampal and amygdala changes after FSs. The abnormalities of hippocampus are the most clinically reported. There are some case reports, which found development of hippocampal sclerosis after FSs (Sokol et al., 2003; Merkenschlager et al., 2009). When magnetic resonance imaging (MRI) was done immediately (a few days after FS), it was showed that T2 weighted signal and the volume of hippocampus increased (swelling) (Sokol et al., 2003; Yokoi et al., 2019). In children with FSs from 3 to 23 months later, MRI imaging found a markedly hyperintense hippocampus (Provenzale et al., 2008). In their teens 2-3 years after FS onset, hippocampal subfield volumes were reduced (Grunewald et al., 2001; Finegersh et al., 2011; Peng et al., 2021), and these abnormalities were probably more pronounced in men (Auer et al., 2008). These suggest that FSs cause parenchymal lesions in the hippocampus, which may have undergone complex lesions during development. A more nuanced analysis noted an increase of hippocampal calretinin-immunoreactive neurons after FSs (Blumcke et al., 1999). Additional clues found that sulfur dioxide (SO2) content is overproduced during the development of FSs and related brain injury (Yang et al., 2018). Still, it remains to be elucidated, how these changes contribute to the pathogenesis of TLE and when is the key time nodes for intervention and treatment.
Preclinical studies have also found that FSs led to a temporal morphological disturbance. MRI histology showed increased fiber density and anisotropy in the hippocampus, and reduced neuronal surface area in the amygdala of rats. Diffusion tensor imaging (DTI) abnormalities were detected in the amygdala and persisted up to 8 weeks (Jansen et al., 2008). The cell numbers decreased by 10% in the CA1 and hilus but did not reduce in the CA3 or dentate gyrus areas, suggesting that the temporal abnormalities after FSs not only changed over time, but possibly also differed by brain regions. Meanwhile, functional impairments were more robust. Shortly after FSs, long-term potentiation (LTP) in CA3-CA1 synapses was strongly reduced (Postnikova et al., 2021). FSs reduced thresholds to chemical convulsants in the immature rat model and electrical stimulation in vitro, suggesting FSs enhanced hippocampal excitability long term during development (Dube et al., 2000). The modification of neuronal excitability of limbic circuits in the developing brain induced by FSs may last into adulthood (Chen et al., 1999). Thus, alterations in temporal lobe tissue morphology after FSs may stem from neuronal excitotoxicity that provoke more long-lasting signals or effects persisting into adulthood.
To identify molecular candidates, which entrain this structural and functional re-organization, Bart C Jongbloets et al. investigated temporal changes in mRNA expression profiles 1 h to 56 days after FSs. They screened 931 regulated genes and profiled several candidates using in situ hybridization and histology at 3 and 14 days after FSs. Temporal regulation of multiple processes was identified, such as stress-, immune- and inflammatory responses, glia activation, glutamate-glutamine cycle and myelination (Jongbloets et al., 2015), indicating the complex short- and long-term changes of hippocampus after FSs. More studies on specific molecules at specific developmental stages need to be further promoted to deconstruct the long-term effects of FS on the temporal lobe and subsequent epileptogenesis.
3.2.2 Alterations of cortex
The effects on the hippocampus after FSs are probably the most profound and thoroughly studied (Nazem et al., 2012; Raijmakers et al., 2016), but the role of FSs on other brain regions should not be ignored, especially we note that FSs not only cause alterations of hippocampus related disease in adulthood, but also similarly affect the occurrence of disorders related to cortex, such as ADHD and other psychiatric disorders. Moreover, in the previously mentioned study in Gothenburg, FSs children had motor functioning impairment (Nilsson et al., 2019). Experiment FSs caused fine motor coordination impairment and gait disturbances (Crespo et al., 2018). These suggest that motor related motor cortex and other brain regions may have been affected by FSs.
The vast majority of hippocampal recorded seizures were preceded by a drop in cortical EEG amplitude that began half a minute before hippocampal spasms and was sustained after seizure termination (Dube et al., 2006). This suggests that the limbic spontaneous seizures exert a substantial disturbance to normal cortical neuronal activity. A significant increase in A1 receptor density and mRNA coding A1 was observed in cerebral cortical area 48 h after FSs. In contrast, a significant decrease in A2A receptor density and 5′-nucleotidase activity was detected 48 h after FSs (Leon-Navarro et al., 2015). These results illustrated that long-term alterations after FSs may also exist in the cortex. Whether these cellular molecular abnormalities detected in the cortex are maintained to a longer time and thus provide an explanation for functional abnormalities in adulthood also awaits more in-depth studies.
3.2.3 White matter tract reorganization
MRI studies have demonstrated acute and long-term subcortical white matter changes following FSs (Takanashi et al., 2006; Pujar et al., 2017). Between 3 and 9 days after FSs, white matter lesions were observed and the diffusion abnormality disappeared between days 9 and 25 (Takanashi et al., 2006). In a homogeneous, population-based sample, fractional anisotropy in early maturing central white matter tracts and mean and axial diffusivity in several late-maturing peripheral white matter tracts were found to increase 8 years post-FSs (Pujar et al., 2017). Drug resistant TLE is increasingly recognized as a system level disorder affecting the structure and function of large-scale gray matter networks, while the superficial white matter, close to neocortical regions, plays a key role in maintaining cortical connectivity, which is associated with seizure outcome (Liu et al., 2016). Long lasting abnormalities of white matter after FSs therefore need to be paid more attention and may be one of the etiologies of drug-resistant TLE.
3.2.4 Activation of microglia
In the identification of mRNA expression profiles following FSs described above, glia was activated (Jongbloets et al., 2015). Among these, modulation of microglia in the structure and function of the hippocampus after early-life FSs received much attention (Andoh et al., 2020; Weninger et al., 2021). Massive microgliosis after FSs was found (Weninger et al., 2021). The microglial expression levels of Iba1 and ED1 (lysosomal markers) and the proportion of microglia with amoeboid morphology increased, indicating that microglia were activated after FSs (Kong et al., 2019). The expression levels of microglial cytokines like inflammatory cytokines such as TNF-α and IL-1β were elevated 4 h after FSs (Kim et al., 2015). In addition, microglial synaptic displacement in motor cortex is a protective event during the pathological process of complex FSs. There is a significant increase of perisomatic GABAergic synapses around neuronal soma after complex FSs. So, complex FSs caused enhanced neuronal GABA transmission and increased neuronal excitability, as GABAergic synaptic transmission plays an excitatory role in juvenile stage. Microglia extensively associated with glutamatergic neuronal soma, displacing but not engulfing GABAergic synapses around neuronal soma to reduce the GABA transmission and neuronal excitability through P2Y12 receptors. These studies provide a rationale to elucidate the protective role of microglia and may be a potential future treatment for complex FSs (Wan et al., 2020). Microglial displacement of GABAergic synapses also provides neuroprotection in the adult brain (Chen et al., 2014). In addition, microglia can also secrete numerous neurotrophic factors to elaborate repair of injured neurons after FSs (Parkhurst et al., 2013; Araki et al., 2021). The induction of brain-derived neurotrophic factor (BDNF) mRNA was firstly observed in the dentate gyrus at 30 min after FSs, peaked at 3 h and returned to basal level at 24 h. It was also observed in the CA3 of hippocampus from 2 to 3 h (Kim et al., 2001). BDNF play critical role in neuronal survival, synaptic plasticity and cognitive functions and is known to mediate its action through various intracellular signaling pathways triggered by activation of tyrosine kinase receptor B (TrkB) (Pandya et al., 2013). These observation times did not last into adulthood, and the long-term role of microglia after FSs remains to be explored.
4 Possible mechanisms for long-term neurodevelopmental outcomes of FSs
Clinically, it is somewhat difficult to find targets that dynamically change over prolonged periods after FSs. Most of the mechanistic studies are based on animal models of experimental FSs and often lack clinical proof of correspondence. But these studies remain valuable. Overall, the current mechanistic hypotheses for neuropsychiatric damage from FS are summarized (Figure 1).
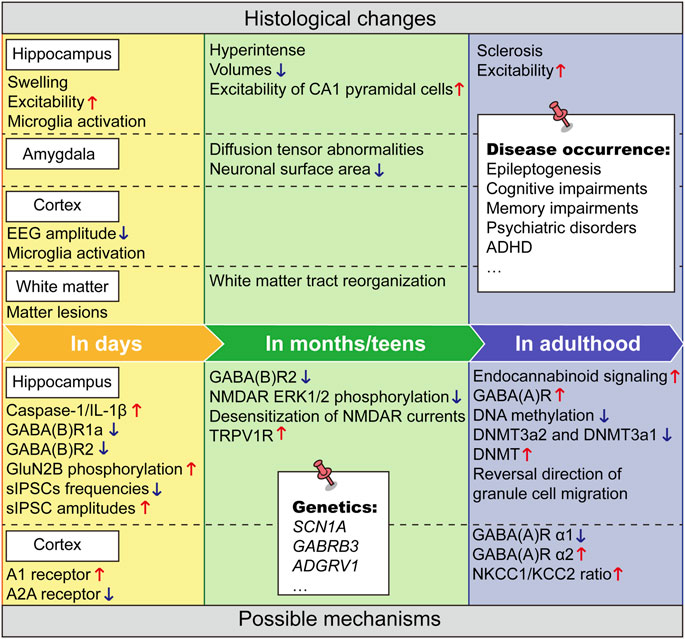
FIGURE 1. The neurodevelopmental changes after FSs and underlying mechanisms over time. The upper part summarizes the histological changes over time following FSs and the lower part summarizes the corresponding underlying mechanisms. Upward red arrows indicate increase or enhancement, and downward blue arrows indicate decrease, attenuation, or loss. The contents on the white notes indicate lesions or gene mutations that may span a long-time course throughout the developmental stage.
4.1 Endocannabinoid system
Feng’s work identified prolonged increase of endocannabinoid signaling in adult seizure susceptibility following FSs, as a potential therapeutic target for preventing the development of epilepsy after infantile FSs (Feng et al., 2016). In a study of long-term plasticity following FSs induced endocannabinoid signaling, the activity dependent retrograde inhibition of endogenous cannabinoids on GABA release continued to increase in the hippocampus of rats (Chen et al., 2003). Endogenous cannabinoid system has been reported to be associated with depression, ADHD and other diseases. Targeting the endocannabinoid system has the potential to alleviate depression (Bright and Akirav, 2022). Clinical randomized experiments found reduced symptoms and cognitive impairment in some ADHD patients after cannabinoid use (Cooper et al., 2017). Recent evidence suggests that modulation of endocannabinoid tone has anxiolytic or antipsychotic effects (Navarrete et al., 2020). This undoubtedly suggests that endocannabinoid signaling may not only be involved in hippocampal related epilepsy and functional alterations after FSs, but may also play a key role in other disease courses.
4.2 Inflammation
In examination of the molecular changes in the rat brain after FSs throughout the latent period, the largest changes were for genes involved in inflammation signaling. Reduction of early inflammatory responses after FSs reduced the risk of subsequent spontaneous seizures (Jung et al., 2011). An important role for caspase-1/IL-1β inflammatory signaling pathway was identified in the increased susceptibility to seizures induced by FSs. Caspase-1/IL-1β expression was upregulated transiently at the onset of FSs. In neonatal mice, caspase-1 inhibitor CZL80 markedly reduced neuronal excitability and incidence of FSs, and, in adult mice, relieved later enhanced epileptogenic susceptibility (Tang et al., 2020). When IL-1β was given after FSs, the incidence of spontaneous seizures in rats will be significantly increased (Fukuda et al., 2015). Increased GluN2B phosphorylation at Tyr1472 site mediated by the transient increase of IL-1β was involved in the enhanced adult seizure susceptibility after FSs and showed a 3-day therapeutic time-window for reversing the enhanced seizure susceptibility after FSs (Chen et al., 2016). It is interesting that FSs or early-life IL-1β treatment increased the expression of cannabinoid type 1 receptor (CB1R) for over 50 days, which was blocked by IL-1Ra or was absent in IL-1R1 knockout mice (Tang et al., 2020). Knockdown and synthesis inhibitor of endocannabinoid abolished FSs or IL-1β-enhanced seizure susceptibility. So, the long-term effects of FSs on IL-1β and endocannabinoid may have crosstalk. Furthermore, IL-6 and IL-8 in the IL-1 cytokine system are closely associated with FSs. Compared to children without hippocampal signal abnormalities, children with T2 hippocampal hyperintensity on MRI after FSs had significantly higher IL-8 and IL-6 levels and lower IL-1Ra/IL-6 and IL-1Ra/IL-8 ratios. The lower IL-1Ra/IL-6 ratio was highly predictive of T21 hyperintensity in hippocampus after FSs (Gallentine et al., 2017; Vezzani et al., 2019), suggesting that IL-1Ra and IL-6 may also be powerful biomarkers and potential therapeutic targets for hippocampal injury after FSs.
4.3 GABAergic and glutamatergic system
Within the molecular examined in the rat brain after FSs throughout the latent period, the largest changes were the genes involved in inflammation signaling, then were (GABA signaling and glutamatergic system (Jung et al., 2011). We know that the neural network is overexcited during the onset of FSs, and this excitement may last for some time after the onset (Reid et al., 2013). Swijsen et al. evaluated BrdU-labeled Devil Gundam (DG) cells for co-expression with GABA A receptors [GABA (A)Rs] and N-methyl-D-aspartate receptors (NMDARs). The number of BrdU-GABA (A)R co-labeled cells not BrdU-NMDAR co-expressing cells was increased in adult after FSs. The results demonstrate that developmental seizures cause a long-term increase in GABA (A)R expression in newborn DG cells (Swijsen et al., 2012b). In other research, increased adult hippocampal protein expression of NR2B was found after early life inflammation and FSs. The inflammation + FSs group had also decreased protein expression of GluR2 and GABAA α1 receptor subunits and mRNA and protein expression of KCC2 (Reid et al., 2013). In the adult sensorimotor cortex, significantly lower levels of the GABA (A) receptor α1 subunit, higher levels of the α2 subunit, and a higher NKCC1/KCC2 ratio were reported in FSs rat (Reid et al., 2012), but the functional outcome and disease occurrence during adulthood due to these alterations remain to be investigated. Therefore, the GABAergic system may be more markedly altered after FSs, while the glutamatergic system may be more likely to change concomitantly in expression due to other factors such as inflammation and different brain regions. It is also found that experimental FSs induce changes in GABA (A) R-mediated neurotransmission in the dentate gyrus. Frequencies of spontaneous inhibitory postsynaptic currents (sIPSCs) were reduced in FS rats, whereas sIPSC amplitudes were enhanced (Swijsen et al., 2012a). GABA (B)R1a and GABA (B)R2 subunits and the binding of the 2 subunits decreased in hippocampus after FSs in immature rats. To be noted, the decrease of GABA (B)R1a lasted for 15 days but that of GABA (B)R2 persisted for more than 30 days. These changes may result in long-lasting imbalance of excitation/inhibition function in hippocampus (Han et al., 2006). Aberrant migration of neonatal-generated granule cells resulted in granule cell ectopia that persists into adulthood. FSs induced an upregulation of GABA (A) receptors [GABA (A)-Rs] in neonatally generated granule cells, and hyperactivation of excitatory GABA (A)-Rs caused a reversal in the direction of granule cell migration. This abnormal migration was prevented by RNAi-mediated knockdown of the NKCC1, which regulates the excitatory action of GABA (Koyama et al., 2012). These all may be the neuropathological basis for the subsequent FSs induced excitation/inhibition imbalance in adulthood and holds promise as potential intervention targets for disorders such as epilepsy.
Although NMDARs expression does not change obviously after FSs, changes in its phosphorylation have been detected by several groups. It is demonstrated that GluN2B phosphorylation at Tyr1472 site was involved in the enhanced adult seizure susceptibility after FSs (Chen et al., 2016). Furthermore, a selective long-term deficit in NMDA receptor-mediated ERK1/2 phosphorylation was observed in the hippocampus after FSs. There was a specific alteration in NR2A, but not NR2B in subunit tyrosine phosphorylation in adult (Chang et al., 2005). LTP in CA3-CA1 synapses was strongly reduced, which contributed to the insufficient activity of NMDARs. Whole cell recordings found a greater desensitization of NMDAR currents in teens after FSs, probably due to insufficient glycine site activation of NMDARs, as application of D-serine (a glycine site agonist) allowed LTP to return to control values of rats (Postnikova et al., 2021). Taken together, intervening on specific phosphorylation sites of NMDARs and downstream signals rather than directly regulating their expression may be more helpful to prevent long-term pathological changes after FSs. The dentate gyrus of rats showed impaired paired pulse suppression and excitation ratio, and increased VGLUT-1 (Vesicular Glutamate Transporter 1) immunoreactivity 10–12 weeks after FSs (Kwak et al., 2008)). Due to the importance of VGLUTs in maintaining low extracellular glutamate concentrations and their association with epilepsy syndromes (van der Hel et al., 2009; Santolini et al., 2017), it is highly recommended to conduct more research to discover more drug targets.
4.4 Genetics
Patients with SCN1A mutations often experience prolonged early-life FSs, raising the possibility that these events may influence epileptogenesis and lead to more severe adult phenotypes. To test this hypothesis, Dutton et al. subjected 21–23-day-old mice expressing the human SCN1A GEFS + mutation R1648H to prolonged hyperthermia, and then examined seizure and behavioral phenotypes during adulthood. They found that early-life FSs resulted in lower latencies to induced seizures, increased severity of spontaneous seizures, hyperactivity, and impairments in social behavior and recognition memory during adulthood (Dutton et al., 2017). The underlying sodium current of this mutant exhibited a significantly shifted hyperpolarizing inactivation threshold at room temperature and elevated temperature (Roemmich et al., 2021). Since this change is constitutive, it is likely to interact with thermally induced changes in other cellular properties, leading to sustained depolarization and thermally induced increases in seizure activity. Meta-analysis also revealed a genome-wide significant association for TLE and early-life FSs at the sodium channel gene cluster on chromosome 2q24.3 within an intron of the SCN1A gene (Kasperaviciute et al., 2013). Functional studies of the mutations showed that it caused biophysical defects of Na (V)1.2 and impaired its cell surface expression (Shi et al., 2012; Orlando et al., 2022). But more direct evidence for the therapeutic effect of intervention of SCN1A on adult diseases after FSs need to be provided. Massive parallel sequencing of GABRB3 was performed in 416 patients with a range of epileptic encephalopathies and childhood-onset epilepsies. The results indicated that GABRB3 mutations, which will reduce GABAergic receptors functions, are associated with a broad phenotypic spectrum of epilepsies and that reduced receptor function causing GABAergic disinhibition represents the relevant disease mechanism (Moller et al., 2017). Considering the important role of the GABAergic system in the neurological abnormalities induced by FSs, this mutation should receive more attention. In addition, adhesion G protein-coupled receptor V1 (ADGRV1) is potentially associated with FS-related epilepsy as a susceptibility gene, encoding a very large G protein-coupled receptor-1 (VLGR1), which is localized at synaptic junctions and cooperatively regulates synaptic function (Togashi et al., 2002). The variation in ADGRV1 results in a significant loss of major functional domains of the VLGR1 protein. ADGRV1 variants associated with FSs/epilepsy respond well to antiepileptic drugs, implying a clinical significance (Zhou et al., 2022).
4.5 DNA methylation levels
A cross-sectional pilot study investigated whether global DNA methylation levels (5-mC and 5-hmC markers) and DNMT isoforms (DNMT1, DNMT3a1, and DNMT3a2) expression would be different in hippocampal and neocortical tissues between controls and TLE patients with or without a history of FSs. Compared with the control group, the overall level of DNA methylation and the expression of DNMT3a2 subtype in the hippocampus of all TLE groups were lower, while the decline in the TLE group with a history of FSs was greater. Interestingly, compared with the control group and other TLE groups, the expression of DNMT3a1 in the hippocampus of TLE patients with FSs history was significantly reduced (de Nijs et al., 2019). They did not investigate the epigenetic and functional changes following the methylation changes. In addition, tri-methylation of histone 3 at Lys9 and Lys27 was decreased in the FSs group, suggesting a potential mechanism for the long-term effects of FSs on energy metabolism via histone methylation (Heng et al., 2016).
As previously mentioned, FSs caused adult memory deficits and are transmitted to the next-generation, mostly through the mother. For both generations, DNMT 1 is upregulated, leading to transcriptional repression of the synaptic plasticity protein, coilin, but not memory inhibitory protein phosphatase 1. DNMT inhibitors prevented the high expression of DNMT1 and hypermethylation of curly genes, reversing transgenerational memory deficits (Dai et al., 2019). The above results suggest that the effects of DNA methylation levels not only persist long term but may also persist across generations.
4.6 Ion channel
In the hippocampus of rats that had FSs, the long-lasting enhancement of the widely expressed intrinsic membrane conductance Ih converts the potentiated synaptic inhibition to hyperexcitability in a frequency-dependent manner (Chen et al., 2001). Kamal et al. determined long-term changes in neuronal excitability of rat hippocampal CA1 pyramidal cells after FSs. They showed that FSs induced an increase in the hyperpolarization-activated current Ih and a reduction in the amplitude of the slow afterhyperpolarization following FSs, which is likely to contribute to the hyperexcitability of the hippocampus 3–5 weeks after FS (Kamal et al., 2006). These studies have not been able to identify an intervention molecular target, but Harris et al. found that inhibiting TRPV1 receptors during FSs prevented learning deficits in young adult female rats (Harris et al., 2022). Whether modulation of other ion channels can also alter the long-term neurological effects caused by FSs and whether the downstream series of signaling pathways resulting from these current alterations are the true culprits remain unclear.
5 Conclusion
With FSs as one of the highest incidences in early childhood, it is important to assess and prevent the adverse long-term neurodevelopmental effects that FSs causes. In this review, the long-term adverse effects of FSs on neurodevelopment and the underlying mechanisms are systematically reviewed and summarized, and some specific novel targets with therapeutic value are specifically proposed. Overall, because the onset of FSs is precisely at the stage of rapid infantile neural-glial development, a series of neural-glial changes are triggered after FSs. Some protein or molecular alterations are long-term, even until disease onset in adulthood. Some alterations, although transient in short term, cause long-term evolution of some downstream signaling pathways, which may ultimately lead to disease.
Long term follow-up of more patients after FSs may be necessary, in conjunction with experimental animal models, to elucidate the complex effects of FSs on neurodevelopment. Indeed, several important questions remain open. First, is the frequency and duration of FSs linked to the type and severity of illness occurring later? Second, are there common lesions or targets in different diseases caused by FSs? Third, is there optimal time window for neuropsychiatric disorders resulting from FSs? Is prophylactic treatment necessary? If these issues can be addressed, they will undoubtedly better guide clinical treatment and greatly benefit patients. Gene mutations will have long-term stable effects in patients, which could be important underlying mechanism to explain the occurrence of adult disease after FSs. For example, SCN1A GEFS + mutations cause FSs and genetic epilepsies. However, it remains unclear how most individual mutations in SCN1A lead to seizures. Recent studies have identified a significant depolarizing shift in action potential threshold in parvalbumin expressing inhibitory hippocampal CA1 interneurons but not in firing properties of excitatory pyramidal neurons, suggesting that mutations in the same gene on different cells may cause different consequences (Das et al., 2021). Thus, if single-cell sequencing developed in recent years is used in the future, it will help to identify more specific molecules and also to explain shared mechanisms of different diseases. A variety of intravital imaging techniques that have revolutionized rapidly also offer more possibilities for long-term observations in preclinical studies. A general genetic strategy for precise control of copy number of fluorescently labeled molecules in cells could help visualizing long-term single-molecule dynamics in vivo (Liu et al., 2018). At the same time, probes for long-term in vivo imaging without autofluorescence have also been developed due to the disadvantages that cannot be easily overcome by fluorescent proteins, such as long maturation time, low brightness, photobleaching, broad emission spectrum, and sample autofluorescence. Although it can still only be applied to transparent zebrafish (Cardoso Dos Santos et al., 2020), it is also a new idea for long-term observation. Label free atraumatic large-scale photoacoustic microscopy methods have also emerged that can enable long-term imaging of angiogenesis in an undisturbed environment (Rebling et al., 2021). The application of these new methods can facilitate long-term observation after FSs and the elucidation of mechanisms. More powerful tools may also improve the situation in which a hundred flowers bloom but the main line is unclear in the current mechanism research. Based on that, future research should be engaged in discovering biomarkers of disease after FSs and critical drug targets, accelerating the development of drugs with less side effects, and providing more effective therapeutic strategies.
Author contributions
YY, CZ, and HW-W contributed to conception and design of the review. YY wrote the first draft of the manuscript. CZ and HW-W wrote sections of the manuscript. All authors contributed to the article and approved the submitted version.
Funding
This work was supported by National Key R&D Program of China (No. 2020YFA0803900).
Conflict of interest
The authors declare that the research was conducted in the absence of any commercial or financial relationships that could be construed as a potential conflict of interest.
Publisher’s note
All claims expressed in this article are solely those of the authors and do not necessarily represent those of their affiliated organizations, or those of the publisher, the editors and the reviewers. Any product that may be evaluated in this article, or claim that may be made by its manufacturer, is not guaranteed or endorsed by the publisher.
Supplementary material
The Supplementary Material for this article can be found online at: https://www.frontiersin.org/articles/10.3389/fcell.2023.1186050/full#supplementary-material
References
Alese, O. O., and Mabandla, M. V. (2019). Transgenerational deep sequencing revealed hypermethylation of hippocampal mGluR1 gene with altered mRNA expression of mGluR5 and mGluR3 associated with behavioral changes in Sprague Dawley rats with history of prolonged febrile seizure. PLoS One 14 (11), e0225034. doi:10.1371/journal.pone.0225034
Alese, O. O., Rakgantsho, C., Mkhize, N. V., Zulu, S., and Mabandla, M. V. (2020). Prolonged febrile seizure history exacerbates seizure severity in a pentylenetetrazole rat model of epilepsy. Brain Res. Bull. 155, 137–144. doi:10.1016/j.brainresbull.2019.11.021
Andoh, M., Ikegaya, Y., and Koyama, R. (2020). Microglia modulate the structure and function of the hippocampus after early-life seizures. J. Pharmacol. Sci. 144 (4), 212–217. doi:10.1016/j.jphs.2020.09.003
Antoon, J. W., Hall, M., Howard, L. M., Herndon, A., Freundlich, K. L., Grijalva, C. G., et al. (2022). COVID-19 and acute neurologic complications in children. Pediatrics 150 (5), e2022058167. doi:10.1542/peds.2022-058167
Araki, T., Ikegaya, Y., and Koyama, R. (2021). The effects of microglia- and astrocyte-derived factors on neurogenesis in health and disease. Eur. J. Neurosci. 54 (5), 5880–5901. doi:10.1111/ejn.14969
Arul, J., Kommu, P. P. K., Kasinathan, A., Ray, L., and Krishnan, L. (2020). Zinc status and febrile seizures: Results from a cross-sectional study. J. Neurosci. Rural. Pract. 11 (4), 597–600. doi:10.1055/s-0040-1715992
Asisipo, M., Gwladys, N. T., and Musa, M. V. (2020). Effect of a novel prolonged febrile seizure model on GABA associated ion channels. Metab. Brain Dis. 35 (3), 441–449. doi:10.1007/s11011-019-00492-3
Audenaert, D., Claes, L., Ceulemans, B., Lofgren, A., Van Broeckhoven, C., and De Jonghe, P. (2003). A deletion in SCN1B is associated with febrile seizures and early-onset absence epilepsy. Neurology 61 (6), 854–856. doi:10.1212/01.wnl.0000080362.55784.1c
Audenaert, D., Schwartz, E., Claeys, K. G., Claes, L., Deprez, L., Suls, A., et al. (2006). A novel GABRG2 mutation associated with febrile seizures. Neurology 67 (4), 687–690. doi:10.1212/01.wnl.0000230145.73496.a2
Auer, T., Barsi, P., Bone, B., Angyalosi, A., Aradi, M., Szalay, C., et al. (2008). History of simple febrile seizures is associated with hippocampal abnormalities in adults. Epilepsia 49 (9), 1562–1569. doi:10.1111/j.1528-1167.2008.01679.x
Barr, W. B., Ashtari, M., and Schaul, N. (1997). Bilateral reductions in hippocampal volume in adults with epilepsy and a history of febrile seizures. J. Neurol. Neurosurg. Psychiatry 63 (4), 461–467. doi:10.1136/jnnp.63.4.461
Barrett, K. T., Roy, A., Rivard, K. B., Wilson, R. J. A., and Scantlebury, M. H. (2018). Vagal TRPV1 activation exacerbates thermal hyperpnea and increases susceptibility to experimental febrile seizures in immature rats. Neurobiol. Dis. 119, 172–189. doi:10.1016/j.nbd.2018.08.004
Berseem, N. F., Khattab, E., Saad, D. S., and Abd Elnaby, S. A. (2022). Role of SCN2A c.56G/A gene polymorphism in Egyptian children with genetic epilepsy with febrile seizure plus. CNS Neurol. Disord. Drug Targets 21 (5), 450–457. doi:10.2174/1871527320666211004123731
Bertelsen, E. N., Larsen, J. T., Petersen, L., Christensen, J., and Dalsgaard, S. (2016). Childhood epilepsy, febrile seizures, and subsequent risk of ADHD. Pediatrics 138 (2), e20154654. doi:10.1542/peds.2015-4654
Bhat, J. A., Bhat, T. A., Sheikh, S. A., Wani, Z. A., and Ara, R. (2020). Status of 25-hydroxy vitamin D level in simple febrile seizures and its correlation with recurrence of seizures. Avicenna J. Med. 10 (1), 6–9. doi:10.4103/ajm.ajm_57_19
Blumcke, I., Beck, H., Suter, B., Hoffmann, D., Fodisch, H. J., Wolf, H. K., et al. (1999). An increase of hippocampal calretinin-immunoreactive neurons correlates with early febrile seizures in temporal lobe epilepsy. Acta Neuropathol. 97 (1), 31–39. doi:10.1007/s004010050952
Bright, U., and Akirav, I. (2022). Modulation of endocannabinoid system components in depression: Pre-clinical and clinical evidence. Int. J. Mol. Sci. 23 (10), 5526. doi:10.3390/ijms23105526
Butila, A. T., Zazgyva, A., Sin, A. I., Szabo, E. R., and Tilinca, M. C. (2018). GABRG2 C588T gene polymorphisms might be a predictive genetic marker of febrile seizures and generalized recurrent seizures: A case-control study in a Romanian pediatric population. Arch. Med. Sci. 14 (1), 157–166. doi:10.5114/aoms.2016.63739
Cadet, K., Ceneviva, G. D., Walter, V., Thomas, N. J., and Krawiec, C. (2023). Impact of the COVID-19 pandemic on diagnostic frequency of febrile seizures: An electronic health record database observational study. Neurohospitalist 13 (1), 46–52. doi:10.1177/19418744221123208
Cappellari, A. M., Brizio, C., Mazzoni, M. B., Bertolozzi, G., Vianello, F., Rocchi, A., et al. (2018). Predictive value of EEG for febrile seizure recurrence. Brain Dev. 40 (4), 311–315. doi:10.1016/j.braindev.2017.12.004
Cardoso Dos Santos, M., Colin, I., Ribeiro Dos Santos, G., Susumu, K., Demarque, M., Medintz, I. L., et al. (2020). Time-gated FRET nanoprobes for autofluorescence-free long-term in vivo imaging of developing zebrafish. Adv. Mater 32 (39), e2003912. doi:10.1002/adma.202003912
Carman, K. B., Karal, Y., Gul Mert, G., Ekici, A., Perk, P., Arslantas, D., et al. (2021). Expression of MicroRNA 146a, 155, 181 and 223 in febrile seizure. Turk J. Pediatr. 63 (4), 594–601. doi:10.24953/turkjped.2021.04.006
Chang, Y. C., Huang, A. M., Kuo, Y. M., Wang, S. T., Chang, Y. Y., and Huang, C. C. (2003). Febrile seizures impair memory and cAMP response-element binding protein activation. Ann. Neurol. 54 (6), 706–718. doi:10.1002/ana.10789
Chang, Y. C., Kuo, Y. M., Huang, A. M., and Huang, C. C. (2005). Repetitive febrile seizures in rat pups cause long-lasting deficits in synaptic plasticity and NR2A tyrosine phosphorylation. Neurobiol. Dis. 18 (3), 466–475. doi:10.1016/j.nbd.2004.12.012
Chen, B., Feng, B., Tang, Y., You, Y., Wang, Y., Hou, W., et al. (2016). Blocking GluN2B subunits reverses the enhanced seizure susceptibility after prolonged febrile seizures with a wide therapeutic time-window. Exp. Neurol. 283, 29–38. doi:10.1016/j.expneurol.2016.05.034
Chen, K., Aradi, I., Thon, N., Eghbal-Ahmadi, M., Baram, T. Z., and Soltesz, I. (2001). Persistently modified h-channels after complex febrile seizures convert the seizure-induced enhancement of inhibition to hyperexcitability. Nat. Med. 7 (3), 331–337. doi:10.1038/85480
Chen, K., Baram, T. Z., and Soltesz, I. (1999). Febrile seizures in the developing brain result in persistent modification of neuronal excitability in limbic circuits. Nat. Med. 5 (8), 888–894. doi:10.1038/11330
Chen, K., Ratzliff, A., Hilgenberg, L., Gulyas, A., Freund, T. F., Smith, M., et al. (2003). Long-term plasticity of endocannabinoid signaling induced by developmental febrile seizures. Neuron 39 (4), 599–611. doi:10.1016/s0896-6273(03)00499-9
Chen, Q., Li, M., Zhang, X., Zhang, X., Zhong, R., and Lin, W. (2019). Association between interleukin-6 gene polymorphisms and febrile seizure risk: A meta-analysis. Med. Baltim. 98 (39), e17167. doi:10.1097/MD.0000000000017167
Chen, Z., Jalabi, W., Hu, W., Park, H. J., Gale, J. T., Kidd, G. J., et al. (2014). Microglial displacement of inhibitory synapses provides neuroprotection in the adult brain. Nat. Commun. 5, 4486. doi:10.1038/ncomms5486
Chiang, L. M., Huang, G. S., Sun, C. C., Hsiao, Y. L., Hui, C. K., and Hu, M. H. (2018). Association of developing childhood epilepsy subsequent to febrile seizure: A population-based cohort study. Brain Dev. 40 (9), 775–780. doi:10.1016/j.braindev.2018.05.006
Choi, J., Min, H. J., and Shin, J. S. (2011). Increased levels of HMGB1 and pro-inflammatory cytokines in children with febrile seizures. J. Neuroinflammation 8, 135. doi:10.1186/1742-2094-8-135
Cooper, R. E., Williams, E., Seegobin, S., Tye, C., Kuntsi, J., and Asherson, P. (2017). Cannabinoids in attention-deficit/hyperactivity disorder: A randomised-controlled trial. Eur. Neuropsychopharmacol. 27 (8), 795–808. doi:10.1016/j.euroneuro.2017.05.005
Crespo, M., Leon-Navarro, D. A., and Martin, M. (2018). Cerebellar oxidative stress and fine motor impairment in adolescent rats exposed to hyperthermia-induced seizures is prevented by maternal caffeine intake during gestation and lactation. Eur. J. Pharmacol. 822, 186–198. doi:10.1016/j.ejphar.2018.01.023
Dai, Y. J., Wu, D. C., Feng, B., Chen, B., Tang, Y. S., Jin, M. M., et al. (2019). Prolonged febrile seizures induce inheritable memory deficits in rats through DNA methylation. CNS Neurosci. Ther. 25 (5), 601–611. doi:10.1111/cns.13088
Dai, Y. J., Xu, Z. H., Feng, B., Xu, C. L., Zhao, H. W., Wu, D. C., et al. (2014). Gender difference in acquired seizure susceptibility in adult rats after early complex febrile seizures. Neurosci. Bull. 30 (6), 913–922. doi:10.1007/s12264-014-1482-8
Das, A., Zhu, B., Xie, Y., Zeng, L., Pham, A. T., Neumann, J. C., et al. (2021). Interneuron dysfunction in a new mouse model of SCN1A GEFS+. eNeuro 8 (2), ENEURO.0394–20.2021. doi:10.1523/ENEURO.0394-20.2021
de Nijs, L., Choe, K., Steinbusch, H., Schijns, O., Dings, J., van den Hove, D. L. A., et al. (2019). DNA methyltransferase isoforms expression in the temporal lobe of epilepsy patients with a history of febrile seizures. Clin. Epigenetics 11 (1), 118. doi:10.1186/s13148-019-0721-2
Dreier, J. W., Li, J., Sun, Y., and Christensen, J. (2019). Evaluation of long-term risk of epilepsy, psychiatric disorders, and mortality among children with recurrent febrile seizures: A national cohort study in Denmark. JAMA Pediatr. 173 (12), 1164–1170. doi:10.1001/jamapediatrics.2019.3343
Dreier, J. W., Sun, Y., and Christensen, J. (2020). Limited clinical application and concerns of bias in long-term risk of epilepsy, psychiatric disorders, and mortality following febrile seizures-reply. JAMA Pediatr. 174 (7), 730–732. doi:10.1001/jamapediatrics.2020.0046
Dube, C., Chen, K., Eghbal-Ahmadi, M., Brunson, K., Soltesz, I., and Baram, T. Z. (2000). Prolonged febrile seizures in the immature rat model enhance hippocampal excitability long term. Ann. Neurol. 47 (3), 336–344. doi:10.1002/1531-8249(200003)47:3<336::aid-ana9>3.0.co;2-w
Dube, C. M., Zhou, J. L., Hamamura, M., Zhao, Q., Ring, A., Abrahams, J., et al. (2009). Cognitive dysfunction after experimental febrile seizures. Exp. Neurol. 215 (1), 167–177. doi:10.1016/j.expneurol.2008.10.003
Dube, C., Richichi, C., Bender, R. A., Chung, G., Litt, B., and Baram, T. Z. (2006). Temporal lobe epilepsy after experimental prolonged febrile seizures: Prospective analysis. Brain 129, 911–922. doi:10.1093/brain/awl018
Dutton, S. B. B., Dutt, K., Papale, L. A., Helmers, S., Goldin, A. L., and Escayg, A. (2017). Early-life febrile seizures worsen adult phenotypes in Scn1a mutants. Exp. Neurol. 293, 159–171. doi:10.1016/j.expneurol.2017.03.026
Fallah, R., Sabbaghzadegan, S., Karbasi, S. A., and Binesh, F. (2015). Efficacy of zinc sulfate supplement on febrile seizure recurrence prevention in children with normal serum zinc level: A randomised clinical trial. Nutrition 31 (11-12), 1358–1361. doi:10.1016/j.nut.2015.05.024
Feenstra, B., Pasternak, B., Geller, F., Carstensen, L., Wang, T., Huang, F., et al. (2014). Common variants associated with general and MMR vaccine-related febrile seizures. Nat. Genet. 46 (12), 1274–1282. doi:10.1038/ng.3129
Feng, B., Tang, Y., Chen, B., Xu, C., Wang, Y., Dai, Y., et al. (2016). Transient increase of interleukin-1β after prolonged febrile seizures promotes adult epileptogenesis through long-lasting upregulating endocannabinoid signaling. Sci. Rep. 6, 21931. doi:10.1038/srep21931
Feng, B., Tang, Y. S., Chen, B., Xu, Z. H., Wang, Y., Wu, D. C., et al. (2015). Early hypoactivity of hippocampal rhythms during epileptogenesis after prolonged febrile seizures in freely-moving rats. Neurosci. Bull. 31 (3), 297–306. doi:10.1007/s12264-014-1524-2
Finegersh, A., Avedissian, C., Shamim, S., Dustin, I., Thompson, P. M., and Theodore, W. H. (2011). Bilateral hippocampal atrophy in temporal lobe epilepsy: Effect of depressive symptoms and febrile seizures. Epilepsia 52 (4), 689–697. doi:10.1111/j.1528-1167.2010.02928.x
Fukuda, M., Ito, M., Yano, Y., Takahashi, H., Motoie, R., Yano, A., et al. (2015). Postnatal interleukin-1β administration after experimental prolonged febrile seizures enhances epileptogenesis in adulthood. Metab. Brain Dis. 30 (3), 813–819. doi:10.1007/s11011-014-9648-7
Gallentine, W. B., Shinnar, S., Hesdorffer, D. C., Epstein, L., Nordli, D. R., Lewis, D. V., et al. (2017). Plasma cytokines associated with febrile status epilepticus in children: A potential biomarker for acute hippocampal injury. Epilepsia 58 (6), 1102–1111. doi:10.1111/epi.13750
Grunewald, R. A., Farrow, T., Vaughan, P., Rittey, C. D., and Mundy, J. (2001). A magnetic resonance study of complicated early childhood convulsion. J. Neurol. Neurosurg. Psychiatry 71 (5), 638–642. doi:10.1136/jnnp.71.5.638
Guedj, R., Chappuy, H., Titomanlio, L., De Pontual, L., Biscardi, S., Nissack-Obiketeki, G., et al. (2017). Do all children who present with a complex febrile seizure need a lumbar puncture? Ann. Emerg. Med. 70 (1), 52–62. doi:10.1016/j.annemergmed.2016.11.024
Gunes, A., Fidan, S., Dulkadir, R., and Unlu, E. (2021). Evaluation of risk factors associated with first episode febrile seizure. Eur. Rev. Med. Pharmacol. Sci. 25 (22), 7089–7092. doi:10.26355/eurrev_202111_27261
Haerian, B. S., Baum, L., Kwan, P., Cherny, S. S., Shin, J. G., Kim, S. E., et al. (2016). Contribution of GABRG2 polymorphisms to risk of epilepsy and febrile seizure: A multicenter cohort study and meta-analysis. Mol. Neurobiol. 53 (8), 5457–5467. doi:10.1007/s12035-015-9457-y
Halgren, C., Bache, I., Bak, M., Myatt, M. W., Anderson, C. M., Brondum-Nielsen, K., et al. (2012). Haploinsufficiency of CELF4 at 18q12.2 is associated with developmental and behavioral disorders, seizures, eye manifestations, and obesity. Eur. J. Hum. Genet. 20 (12), 1315–1319. doi:10.1038/ejhg.2012.92
Hamelin, S., Pouyatos, B., Khalaf-Nazzal, R., Chabrol, T., Francis, F., David, O., et al. (2014). Long-term modifications of epileptogenesis and hippocampal rhythms after prolonged hyperthermic seizures in the mouse. Neurobiol. Dis. 69, 156–168. doi:10.1016/j.nbd.2014.05.025
Han, J. Y., Lee, H. J., Lee, Y. M., and Park, J. (2020). Identification of missense ADGRV1 mutation as a candidate genetic cause of familial febrile seizure 4. Child. (Basel) 7 (9), 144. doi:10.3390/children7090144
Han, Y., Qin, J., Bu, D. F., Chang, X. Z., and Yang, Z. X. (2006). Successive alterations of hippocampal gamma-aminobutyric acid B receptor subunits in a rat model of febrile seizure. Life Sci. 78 (25), 2944–2952. doi:10.1016/j.lfs.2005.11.023
Hanlon, S. M., Sim, D., Schneider, J. G., Yang, Z., and Thompson, S. M. (2023). The association between COVID-19 and febrile seizure: A retrospective case-control study. Pediatr. Emerg. Care 39 (5), 360–363. doi:10.1097/PEC.0000000000002935
Harris, S. A., George, A. G., Barrett, K. T., Scantlebury, M. H., and Teskey, G. C. (2022). Febrile seizures lead to prolonged epileptiform activity and hyperoxia that when blocked prevents learning deficits. Epilepsia 63 (10), 2650–2663. doi:10.1111/epi.17371
Heng, D., Wang, Z., Fan, Y., Li, L., Fang, J., Han, S., et al. (2016). Long-term metabolic alterations in a febrile seizure model. Int. J. Neurosci. 126 (4), 374–380. doi:10.3109/00207454.2015.1018385
Hessel, E. V., de Wit, M., Wolterink-Donselaar, I. G., Karst, H., de Graaff, E., van Lith, H. A., et al. (2014). Identification of Srp9 as a febrile seizure susceptibility gene. Ann. Clin. Transl. Neurol. 1 (4), 239–250. doi:10.1002/acn3.48
Huang, W. X., Yu, F., Sanchez, R. M., Liu, Y. Q., Min, J. W., Hu, J. J., et al. (2015). TRPV1 promotes repetitive febrile seizures by pro-inflammatory cytokines in immature brain. Brain Behav. Immun. 48, 68–77. doi:10.1016/j.bbi.2015.01.017
Hugen, C. A., Oudesluys-Murphy, A. M., and Hop, W. C. (1995). Serum sodium levels and probability of recurrent febrile convulsions. Eur. J. Pediatr. 154 (5), 403–405. doi:10.1007/BF02072115
Iijima, H., Kubota, M., and Ogimi, C. (2022). Change in seizure incidence in febrile children with COVID-19 in the era of omicron variant of concern. J. Pediatr. Infect. Dis. Soc. 11 (11), 514–517. doi:10.1093/jpids/piac085
Jansen, J. F., Lemmens, E. M., Strijkers, G. J., Prompers, J. J., Schijns, O. E., Kooi, M. E., et al. (2008). Short- and long-term limbic abnormalities after experimental febrile seizures. Neurobiol. Dis. 32 (2), 293–301. doi:10.1016/j.nbd.2008.07.010
Jongbloets, B. C., van Gassen, K. L., Kan, A. A., Olde Engberink, A. H., de Wit, M., Wolterink-Donselaar, I. G., et al. (2015). Expression profiling after prolonged experimental febrile seizures in mice suggests structural remodeling in the Hippocampus. PLoS One 10 (12), e0145247. doi:10.1371/journal.pone.0145247
Joung, J., Yang, H., Choi, Y. J., Lee, J., and Ko, Y. (2023). The impact of omicron wave on pediatric febrile seizure. J. Korean Med. Sci. 38 (3), e18. doi:10.3346/jkms.2023.38.e18
Jung, K. H., Chu, K., Lee, S. T., Park, K. I., Kim, J. H., Kang, K. M., et al. (2011). Molecular alterations underlying epileptogenesis after prolonged febrile seizure and modulation by erythropoietin. Epilepsia 52 (3), 541–550. doi:10.1111/j.1528-1167.2010.02916.x
Kamal, A., Notenboom, R. G., de Graan, P. N., and Ramakers, G. M. (2006). Persistent changes in action potential broadening and the slow afterhyperpolarization in rat CA1 pyramidal cells after febrile seizures. Eur. J. Neurosci. 23 (8), 2230–2234. doi:10.1111/j.1460-9568.2006.04732.x
Kasperaviciute, D., Catarino, C. B., Matarin, M., Leu, C., Novy, J., Tostevin, A., et al. (2013). Epilepsy, hippocampal sclerosis and febrile seizures linked by common genetic variation around SCN1A. Brain 136, 3140–3150. doi:10.1093/brain/awt233
Kavcic, A., and Rener-Primec, Z. (2018). Predictive value of epileptiform discharges for subsequent epilepsy after febrile seizures. J. Child. Neurol. 33 (12), 772–775. doi:10.1177/0883073818787064
Kim, I., Mlsna, L. M., Yoon, S., Le, B., Yu, S., Xu, D., et al. (2015). A postnatal peak in microglial development in the mouse hippocampus is correlated with heightened sensitivity to seizure triggers. Brain Behav. 5 (12), e00403. doi:10.1002/brb3.403
Kim, Y. H., Rhyu, I. J., Park, K. W., Eun, B. L., Kim, Y. I., Rha, H. K., et al. (2001). The induction of BDNF and c-fos mRNA in the hippocampal formation after febrile seizures. Neuroreport 12 (15), 3243–3246. doi:10.1097/00001756-200110290-00020
Kiviranta, T., and Airaksinen, E. M. (1995). Low sodium levels in serum are associated with subsequent febrile seizures. Acta Paediatr. 84 (12), 1372–1374. doi:10.1111/j.1651-2227.1995.tb13571.x
Kloc, M. L., Chen, Y., Daglian, J. M., Holmes, G. L., Baram, T. Z., and Barry, J. M. (2023). Spatial learning impairments and discoordination of entorhinal-hippocampal circuit coding following prolonged febrile seizures. Hippocampus. doi:10.1002/hipo.23541
Kloc, M. L., Marchand, D. H., Holmes, G. L., Pressman, R. D., and Barry, J. M. (2022). Cognitive impairment following experimental febrile seizures is determined by sex and seizure duration. Epilepsy Behav. 126, 108430. doi:10.1016/j.yebeh.2021.108430
Kong, W. L., Min, J. W., Liu, Y. L., Li, J. X., He, X. H., and Peng, B. W. (2014). Role of TRPV1 in susceptibility to PTZ-induced seizure following repeated hyperthermia challenges in neonatal mice. Epilepsy Behav. 31, 276–280. doi:10.1016/j.yebeh.2013.10.022
Kong, W., Wang, X., Yang, X., Huang, W., Han, S., Yin, J., et al. (2019). Activation of TRPV1 contributes to recurrent febrile seizures via inhibiting the microglial M2 phenotype in the immature brain. Front. Cell Neurosci. 13, 442. doi:10.3389/fncel.2019.00442
Koyama, R., Tao, K., Sasaki, T., Ichikawa, J., Miyamoto, D., Muramatsu, R., et al. (2012). GABAergic excitation after febrile seizures induces ectopic granule cells and adult epilepsy. Nat. Med. 18 (8), 1271–1278. doi:10.1038/nm.2850
Ku, Y. C., Muo, C. H., Ku, C. S., Chen, C. H., Lee, W. Y., Shen, E. Y., et al. (2014). Risk of subsequent attention deficit-hyperactivity disorder in children with febrile seizures. Arch. Dis. Child. 99 (4), 322–326. doi:10.1136/archdischild-2013-304647
Kubota, J., Higurashi, N., Hirano, D., Okabe, S., Yamauchi, K., Kimura, R., et al. (2021). Body temperature predicts recurrent febrile seizures in the same febrile illness. Brain Dev. 43 (7), 768–774. doi:10.1016/j.braindev.2021.03.002
Kumar, N., Midha, T., and Rao, Y. K. (2019). Risk factors of recurrence of febrile seizures in children in a tertiary care hospital in kanpur: A one year follow up study. Ann. Indian Acad. Neurol. 22 (1), 31–36. doi:10.4103/aian.AIAN_472_17
Kwak, S. E., Kim, J. E., Kim, S. C., Kwon, O. S., Choi, S. Y., and Kang, T. C. (2008). Hyperthermic seizure induces persistent alteration in excitability of the dentate gyrus in immature rats. Brain Res. 1216, 1–15. doi:10.1016/j.brainres.2008.04.026
Kwon, A., Kwak, B. O., Kim, K., Ha, J., Kim, S. J., Bae, S. H., et al. (2018). Cytokine levels in febrile seizure patients: A systematic review and meta-analysis. Seizure 59, 5–10. doi:10.1016/j.seizure.2018.04.023
Leaffer, E. B., Hinton, V. J., and Hesdorffer, D. C. (2013). Longitudinal assessment of skill development in children with first febrile seizure. Epilepsy Behav. 28 (1), 83–87. doi:10.1016/j.yebeh.2013.03.034
Lee, J. H., and Kim, J. H. (2012). Comparison of serum zinc levels measured by inductively coupled plasma mass spectrometry in preschool children with febrile and afebrile seizures. Ann. Lab. Med. 32 (3), 190–193. doi:10.3343/alm.2012.32.3.190
Leon-Navarro, D. A., Albasanz, J. L., and Martin, M. (2015). Hyperthermia-induced seizures alter adenosine A1 and A2A receptors and 5'-nucleotidase activity in rat cerebral cortex. J. Neurochem. 134 (3), 395–404. doi:10.1111/jnc.13130
Lin, C. H., Lin, W. D., Chou, I. C., Lee, I. C., and Hong, S. Y. (2021). Is preterm birth a risk factor for subsequent autism spectrum disorder and attention deficit hyperactivity disorder in children with febrile seizure?-A retrospective study. Life (Basel) 11 (8), 854. doi:10.3390/life11080854
Liu, H., Dong, P., Ioannou, M. S., Li, L., Shea, J., Pasolli, H. A., et al. (2018). Visualizing long-term single-molecule dynamics in vivo by stochastic protein labeling. Proc. Natl. Acad. Sci. U. S. A. 115 (2), 343–348. doi:10.1073/pnas.1713895115
Liu, M., Bernhardt, B. C., Hong, S. J., Caldairou, B., Bernasconi, A., and Bernasconi, N. (2016). The superficial white matter in temporal lobe epilepsy: A key link between structural and functional network disruptions. Brain 139, 2431–2440. doi:10.1093/brain/aww167
Lux, A. L. (2010). Antipyretic drugs do not reduce recurrences of febrile seizures in children with previous febrile seizure. Evid. Based Med. 15 (1), 15–16. doi:10.1136/ebm.15.1.15
Martinos, M. M., Yoong, M., Patil, S., Chin, R. F., Neville, B. G., Scott, R. C., et al. (2012). Recognition memory is impaired in children after prolonged febrile seizures. Brain 135, 3153–3164. doi:10.1093/brain/aws213
Merkenschlager, A., Todt, H., Pfluger, T., and Bernhard, M. K. (2009). Development of hippocampal sclerosis after a complex febrile seizure. Eur. J. Pediatr. 168 (4), 487–490. doi:10.1007/s00431-008-0776-0
Miyagi, Y., Sasano, T., Kato, H., and Kin, K. (2022). Hyponatremia and recurrent febrile seizures during febrile episodes: A meta-analysis. Cureus 14 (4), e24398. doi:10.7759/cureus.24398
Mohammed, H. S., Aboul Ezz, H. S., Sayed, H. M., and Ali, M. A. (2017). Electroencephalographic and biochemical long-lasting abnormalities in animal model of febrile seizure. Biochim. Biophys. Acta Mol. Basis Dis. 1863 (9), 2120–2125. doi:10.1016/j.bbadis.2017.05.024
Moller, R. S., Wuttke, T. V., Helbig, I., Marini, C., Johannesen, K. M., Brilstra, E. H., et al. (2017). Mutations in GABRB3: From febrile seizures to epileptic encephalopathies. Neurology 88 (5), 483–492. doi:10.1212/WNL.0000000000003565
Nagaki, S., Nagaki, S., Minatogawa, Y., Sadamatsu, M., Kato, N., Osawa, M., et al. (1996). The role of vasopressin, somatostatin and GABA in febrile convulsion in rat pups. Life Sci. 58 (24), 2233–2242. doi:10.1016/0024-3205(96)00218-4
Navarrete, F., Garcia-Gutierrez, M. S., Jurado-Barba, R., Rubio, G., Gasparyan, A., Austrich-Olivares, A., et al. (2020). Endocannabinoid system components as potential biomarkers in psychiatry. Front. Psychiatry 11, 315. doi:10.3389/fpsyt.2020.00315
Nazem, A., Jafarian, A. H., Sadraie, S. H., Gorji, A., Kheradmand, H., Radmard, M., et al. (2012). Neuronal injury and cytogenesis after simple febrile seizures in the hippocampal dentate gyrus of juvenile rat. Childs Nerv. Syst. 28 (11), 1931–1936. doi:10.1007/s00381-012-1817-6
Neligan, A., Bell, G. S., Giavasi, C., Johnson, A. L., Goodridge, D. M., Shorvon, S. D., et al. (2012). Long-term risk of developing epilepsy after febrile seizures: A prospective cohort study. Neurology 78 (15), 1166–1170. doi:10.1212/WNL.0b013e31824f807a
Nilsson, G., Westerlund, J., Fernell, E., Billstedt, E., Miniscalco, C., Arvidsson, T., et al. (2019). Neurodevelopmental problems should be considered in children with febrile seizures. Acta Paediatr. 108 (8), 1507–1514. doi:10.1111/apa.14716
Offringa, M., Newton, R., Cozijnsen, M. A., and Nevitt, S. J. (2017). Prophylactic drug management for febrile seizures in children. Cochrane Database Syst. Rev. 2 (2), CD003031. doi:10.1002/14651858.CD003031.pub3
Offringa, M., Newton, R., Nevitt, S. J., and Vraka, K. (2021). Prophylactic drug management for febrile seizures in children. Cochrane Database Syst. Rev. 6 (6), CD003031. doi:10.1002/14651858.CD003031.pub3
Orlando, V., Di Tommaso, S., Alesi, V., Loddo, S., Genovese, S., Catino, G., et al. (2022). A complex genomic rearrangement resulting in loss of function of SCN1A and SCN2A in a patient with severe developmental and epileptic encephalopathy. Int. J. Mol. Sci. 23 (21), 12900. doi:10.3390/ijms232112900
Pandya, C. D., Kutiyanawalla, A., and Pillai, A. (2013). BDNF-TrkB signaling and neuroprotection in schizophrenia. Asian J. Psychiatr. 6 (1), 22–28. doi:10.1016/j.ajp.2012.08.010
Papageorgiou, V., Vargiami, E., Kontopoulos, E., Kardaras, P., Economou, M., Athanassiou-Mataxa, M., et al. (2015). Association between iron deficiency and febrile seizures. Eur. J. Paediatr. Neurol. 19 (5), 591–596. doi:10.1016/j.ejpn.2015.05.009
Parkhurst, C. N., Yang, G., Ninan, I., Savas, J. N., Yates, J. R., Lafaille, J. J., et al. (2013). Microglia promote learning-dependent synapse formation through brain-derived neurotrophic factor. Cell 155 (7), 1596–1609. doi:10.1016/j.cell.2013.11.030
Patel, N., Ram, D., Swiderska, N., Mewasingh, L. D., Newton, R. W., and Offringa, M. (2015). Febrile seizures. BMJ 351, h4240. doi:10.1136/bmj.h4240
Patterson, K. P., Barry, J. M., Curran, M. M., Singh-Taylor, A., Brennan, G., Rismanchi, N., et al. (2017). Enduring memory impairments provoked by developmental febrile seizures are mediated by functional and structural effects of neuronal restrictive silencing factor. J. Neurosci. 37 (14), 3799–3812. doi:10.1523/JNEUROSCI.3748-16.2017
Peng, S. J., Hsieh, K. L., Lin, Y. K., Tsai, M. L., Wong, T. T., and Chang, H. (2021). Febrile seizures reduce hippocampal subfield volumes but not cortical thickness in children with focal onset seizures. Epilepsy Res. 179, 106848. doi:10.1016/j.eplepsyres.2021.106848
Postnikova, T. Y., Griflyuk, A. V., Amakhin, D. V., Kovalenko, A. A., Soboleva, E. B., Zubareva, O. E., et al. (2021). Early life febrile seizures impair hippocampal synaptic plasticity in young rats. Int. J. Mol. Sci. 22 (15), 8218. doi:10.3390/ijms22158218
Printz, V., Hobbs, A. M., Teuten, P., and Paul, S. P. (2016). Clinical update: Assessment and management of febrile children. Community Pract. 89 (6), 32–37.
Provenzale, J. M., Barboriak, D. P., VanLandingham, K., MacFall, J., Delong, D., and Lewis, D. V. (2008). Hippocampal MRI signal hyperintensity after febrile status epilepticus is predictive of subsequent mesial temporal sclerosis. AJR Am. J. Roentgenol. 190 (4), 976–983. doi:10.2214/AJR.07.2407
Pujar, S. S., Seunarine, K. K., Martinos, M. M., Neville, B. G. R., Scott, R. C., Chin, R. F. M., et al. (2017). Long-term white matter tract reorganization following prolonged febrile seizures. Epilepsia 58 (5), 772–780. doi:10.1111/epi.13724
Raijmakers, M., Clynen, E., Smisdom, N., Nelissen, S., Brone, B., Rigo, J. M., et al. (2016). Experimental febrile seizures increase dendritic complexity of newborn dentate granule cells. Epilepsia 57 (5), 717–726. doi:10.1111/epi.13357
Rajab, E., Abdeen, Z., Hassan, Z., Alsaffar, Y., Mandeel, M., Al Shawaaf, F., et al. (2014). Cognitive performance and convulsion risk after experimentally-induced febrile-seizures in rat. Int. J. Dev. Neurosci. 34, 19–23. doi:10.1016/j.ijdevneu.2014.01.001
Rebling, J., Ben-Yehuda Greenwald, M., Wietecha, M., Werner, S., and Razansky, D. (2021). Long-term imaging of wound angiogenesis with large scale optoacoustic microscopy. Adv. Sci. (Weinh) 8 (13), 2004226. doi:10.1002/advs.202004226
Reid, A. Y., Pittman, Q. J., and Teskey, G. C. (2012). A prolonged experimental febrile seizure results in motor map reorganization in adulthood. Neurobiol. Dis. 45 (2), 692–700. doi:10.1016/j.nbd.2011.10.013
Reid, A. Y., Riazi, K., Campbell Teskey, G., and Pittman, Q. J. (2013). Increased excitability and molecular changes in adult rats after a febrile seizure. Epilepsia 54 (4), e45–e48. doi:10.1111/epi.12061
Renda, R., Yuksel, D., and Gurer, Y. K. Y. (2020). Evaluation of patients with febrile seizure: Risk factors, reccurence, treatment and prognosis. Pediatr. Emerg. Care 36 (4), 173–177. doi:10.1097/PEC.0000000000001173
Roemmich, A. J., Vu, T., Lukacsovich, T., Hawkins, C., Schutte, S. S., and O'Dowd, D. K. (2021). Seizure phenotype and underlying cellular defects in Drosophila knock-in models of DS (R1648C) and GEFS+ (R1648H) SCN1A epilepsy. eNeuro 8 (5), ENEURO.0002–21.2021. doi:10.1523/ENEURO.0002-21.2021
Saitoh, M., Ishii, A., Ihara, Y., Hoshino, A., Terashima, H., Kubota, M., et al. (2015). Missense mutations in sodium channel SCN1A and SCN2A predispose children to encephalopathy with severe febrile seizures. Epilepsy Res. 117, 1–6. doi:10.1016/j.eplepsyres.2015.08.001
Salehi, B., Yousefichaijan, P., Safi Arian, S., Ebrahimi, S., and Naziri, M. (2016). Comparison of relation between attention deficit hyperactivity disorder in children with and without simple febrile seizure admitted in arak central Iran. Iran. J. Child. Neurol. 10 (4), 56–61.
Santolini, I., Celli, R., Cannella, M., Imbriglio, T., Guiducci, M., Parisi, P., et al. (2017). Alterations in the α2 δ ligand, thrombospondin-1, in a rat model of spontaneous absence epilepsy and in patients with idiopathic/genetic generalized epilepsies. Epilepsia 58 (11), 1993–2001. doi:10.1111/epi.13898
Scalise, S., Scaramuzzino, L., Lucchino, V., Esposito, C., Malatesta, P., Grillone, K., et al. (2020). Generation of iPSC lines from two patients affected by febrile seizure due to inherited missense mutation in SCN1A gene. Stem Cell Res. 49, 102083. doi:10.1016/j.scr.2020.102083
Scalise, S., Zannino, C., Lucchino, V., Lo Conte, M., Scaramuzzino, L., Cifelli, P., et al. (2022). Human iPSC modeling of genetic febrile seizure reveals aberrant molecular and physiological features underlying an impaired neuronal activity. Biomedicines 10 (5), 1075. doi:10.3390/biomedicines10051075
Schubert, J., Siekierska, A., Langlois, M., May, P., Huneau, C., Becker, F., et al. (2014). Mutations in STX1B, encoding a presynaptic protein, cause fever-associated epilepsy syndromes. Nat. Genet. 46 (12), 1327–1332. doi:10.1038/ng.3130
Schuchmann, S., Schmitz, D., Rivera, C., Vanhatalo, S., Salmen, B., Mackie, K., et al. (2006). Experimental febrile seizures are precipitated by a hyperthermia-induced respiratory alkalosis. Nat. Med. 12 (7), 817–823. doi:10.1038/nm1422
Seo, M. J., Yum, M. S., and Park, J. S. (2023). Comparison of febrile seizures in children with or without coronavirus disease-2019: A single-center observational study. Pediatr. Int. 65 (1), e15461. doi:10.1111/ped.15461
Shahrokhi, A., Zare-Shahabadi, A., Naeimi Poor, M., Sajedi, F., Soltani, S., Zoghi, S., et al. (2017). Association of the single nucleotide polymorphisms of the genes encoding IL-2 and IFN-gamma with febrile seizure. Acta Med. Iran. 55 (6), 354–359.
Shi, X., Yasumoto, S., Kurahashi, H., Nakagawa, E., Fukasawa, T., Uchiya, S., et al. (2012). Clinical spectrum of SCN2A mutations. Brain Dev. 34 (7), 541–545. doi:10.1016/j.braindev.2011.09.016
Sisodiya, S. (2014). Feverish prospects for seizure genetics. Nat. Genet. 46 (12), 1255–1256. doi:10.1038/ng.3150
Skotte, L., Fadista, J., Bybjerg-Grauholm, J., Appadurai, V., Hildebrand, M. S., Hansen, T. F., et al. (2022). Genome-wide association study of febrile seizures implicates fever response and neuronal excitability genes. Brain 145 (2), 555–568. doi:10.1093/brain/awab260
Smith, D. K., Sadler, K. P., and Benedum, M. (2019). Febrile seizures: Risks, evaluation, and prognosis. Am. Fam. Physician 99 (7), 445–450.
Sokol, D. K., Demyer, W. E., Edwards-Brown, M., Sanders, S., and Garg, B. (2003). From swelling to sclerosis: Acute change in mesial hippocampus after prolonged febrile seizure. Seizure 12 (4), 237–240. doi:10.1016/s1059-1311(02)00195-4
Steinlein, O. K. (2004). Genetic mechanisms that underlie epilepsy. Nat. Rev. Neurosci. 5 (5), 400–408. doi:10.1038/nrn1388
Sun, D., Ma, H., Ma, J., Wang, J., Deng, X., Hu, C., et al. (2018). Canonical transient receptor potential channel 3 contributes to febrile seizure inducing neuronal cell death and neuroinflammation. Cell Mol. Neurobiol. 38 (6), 1215–1226. doi:10.1007/s10571-018-0586-5
Sun, X., Xue, H., Zan, B., Zhao, Y., Li, Y., Wang, T., et al. (2020). Anti-convulsant effects of cultures bear bile powder in febrile seizure via regulation of neurotransmission and inhibition of neuroinflammation. J. Ethnopharmacol. 262, 112998. doi:10.1016/j.jep.2020.112998
Swijsen, A., Avila, A., Brone, B., Janssen, D., Hoogland, G., and Rigo, J. M. (2012a). Experimental early-life febrile seizures induce changes in GABA(A) R-mediated neurotransmission in the dentate gyrus. Epilepsia 53 (11), 1968–1977. doi:10.1111/j.1528-1167.2012.03694.x
Swijsen, A., Brone, B., Rigo, J. M., and Hoogland, G. (2012b). Long-lasting enhancement of GABA(A) receptor expression in newborn dentate granule cells after early-life febrile seizures. Dev. Neurobiol. 72 (12), 1516–1527. doi:10.1002/dneu.22016
Takanashi, J., Oba, H., Barkovich, A. J., Tada, H., Tanabe, Y., Yamanouchi, H., et al. (2006). Diffusion MRI abnormalities after prolonged febrile seizures with encephalopathy. Neurology 66 (9), 1304–1309. ; discussion 1291. doi:10.1212/01.wnl.0000210487.36667.a5
Tanaka, M., Wang, Q., Morikawa, Y., Tsukamoto, J., Sammori, H., Takehira, K., et al. (2022). Efficacy, safety, and economic impact of diazepam suppositories with as-needed acetaminophen for prevention of seizure recurrence during the same fever episode in children with suspected simple febrile seizures. Epilepsia 63 (7), 1704–1713. doi:10.1111/epi.17271
Tang, Y., Feng, B., Wang, Y., Sun, H., You, Y., Yu, J., et al. (2020). Structure-based discovery of CZL80, a caspase-1 inhibitor with therapeutic potential for febrile seizures and later enhanced epileptogenic susceptibility. Br. J. Pharmacol. 177 (15), 3519–3534. doi:10.1111/bph.15076
Thomas, E. A., Hawkins, R. J., Richards, K. L., Xu, R., Gazina, E. V., and Petrou, S. (2009). Heat opens axon initial segment sodium channels: A febrile seizure mechanism? Ann. Neurol. 66 (2), 219–226. doi:10.1002/ana.21712
Todd, E., Gurba, K. N., Botzolakis, E. J., Stanic, A. K., and Macdonald, R. L. (2014). GABAA receptor biogenesis is impaired by the γ2 subunit febrile seizure-associated mutation, GABRG2(R177G). Neurobiol. Dis. 69, 215–224. doi:10.1016/j.nbd.2014.05.013
Togashi, H., Abe, K., Mizoguchi, A., Takaoka, K., Chisaka, O., and Takeichi, M. (2002). Cadherin regulates dendritic spine morphogenesis. Neuron 35 (1), 77–89. doi:10.1016/s0896-6273(02)00748-1
Tsai, J. D., Mou, C. H., Chang, H. Y., Li, T. C., Tsai, H. J., and Wei, C. C. (2018). Trend of subsequent epilepsy in children with recurrent febrile seizures: A retrospective matched cohort study. Seizure 61, 164–169. doi:10.1016/j.seizure.2018.08.019
Tsai, M. L., Hung, K. L., Tsan, Y. Y., and Tung, W. T. (2015). Long-term neurocognitive outcome and auditory event-related potentials after complex febrile seizures in children. Epilepsy Behav. 47, 55–60. doi:10.1016/j.yebeh.2015.04.067
van der Hel, W. S., Verlinde, S. A., Meijer, D. H., de Wit, M., Rensen, M. G., van Gassen, K. L., et al. (2009). Hippocampal distribution of vesicular glutamate transporter 1 in patients with temporal lobe epilepsy. Epilepsia 50 (7), 1717–1728. doi:10.1111/j.1528-1167.2009.02054.x
Vaswani, R. K., Dharaskar, P. G., Kulkarni, S., and Ghosh, K. (2010). Iron deficiency as a risk factor for first febrile seizure. Indian Pediatr. 47 (5), 437–439. doi:10.1007/s13312-010-0080-8
Vestergaard, M., Pedersen, C. B., Sidenius, P., Olsen, J., and Christensen, J. (2007). The long-term risk of epilepsy after febrile seizures in susceptible subgroups. Am. J. Epidemiol. 165 (8), 911–918. doi:10.1093/aje/kwk086
Vestergaard, M., Pedersen, M. G., Ostergaard, J. R., Pedersen, C. B., Olsen, J., and Christensen, J. (2008). Death in children with febrile seizures: A population-based cohort study. Lancet 372 (9637), 457–463. doi:10.1016/S0140-6736(08)61198-8
Vezzani, A., Balosso, S., and Ravizza, T. (2019). Neuroinflammatory pathways as treatment targets and biomarkers in epilepsy. Nat. Rev. Neurol. 15 (8), 459–472. doi:10.1038/s41582-019-0217-x
Visser, A. M., Jaddoe, V. W., Ghassabian, A., Schenk, J. J., Verhulst, F. C., Hofman, A., et al. (2012). Febrile seizures and behavioural and cognitive outcomes in preschool children: The generation R study. Dev. Med. Child. Neurol. 54 (11), 1006–1011. doi:10.1111/j.1469-8749.2012.04405.x
Wallace, R. H., Wang, D. W., Singh, R., Scheffer, I. E., George, A. L., Phillips, H. A., et al. (1998). Febrile seizures and generalized epilepsy associated with a mutation in the Na+-channel beta1 subunit gene SCN1B. Nat. Genet. 19 (4), 366–370. doi:10.1038/1252
Wan, Y., Feng, B., You, Y., Yu, J., Xu, C., Dai, H., et al. (2020). Microglial displacement of GABAergic synapses is a protective event during complex febrile seizures. Cell Rep. 33 (5), 108346. doi:10.1016/j.celrep.2020.108346
Wang, J., Xu, F., Zheng, Y., Cheng, X., Zhang, P., and Zhao, H. (2019). Protein kinase C-ε contributes to a chronic inhibitory effect of IL-1β on voltage-gated sodium channels in mice with febrile seizure. J. Integr. Neurosci. 18 (2), 173–179. doi:10.31083/j.jin.2019.02.145
Waqar Rabbani, M., Ali, I., Zahid Latif, H., Basit, A., and Rabbani, M. A. (2013). Serum zinc level in children presenting with febrile seizures. Pak J. Med. Sci. 29 (4), 1008–1011.
Weninger, J., Meseke, M., Rana, S., and Forster, E. (2021). Heat-shock induces granule cell dispersion and microgliosis in hippocampal slice cultures. Front. Cell Dev. Biol. 9, 626704. doi:10.3389/fcell.2021.626704
Xixis, K. L., Samanta, D., and Keenaghan, M. (2022). “Febrile seizure,” in StatPearls (Treasure Island: StatPearls Publishing).
Yagoubi, N., Jomni, Y., and Sakly, M. (2015). Hyperthermia-induced febrile seizures have moderate and transient effects on spatial learning in immature rats. Behav. Neurol. 2015, 924303. doi:10.1155/2015/924303
Yang, S., Wen, X., Yang, X., Li, Y., Guo, C., Zhou, Y., et al. (2018). Visualizing endogenous sulfur dioxide derivatives in febrile-seizure-induced hippocampal damage by a two-photon energy transfer cassette. Anal. Chem. 90 (24), 14514–14520. doi:10.1021/acs.analchem.8b04355
Yang, X. L., Wang, X., Shao, L., Jiang, G. T., Min, J. W., Mei, X. Y., et al. (2019). TRPV1 mediates astrocyte activation and interleukin-1β release induced by hypoxic ischemia (HI). J. Neuroinflammation 16 (1), 114. doi:10.1186/s12974-019-1487-3
Yokoi, S., Kidokoro, H., Yamamoto, H., Ohno, A., Nakata, T., Kubota, T., et al. (2019). Hippocampal diffusion abnormality after febrile status epilepticus is related to subsequent epilepsy. Epilepsia 60 (7), 1306–1316. doi:10.1111/epi.16059
Zhang, C., Wong, V., Ng, P. W., Lui, C. H., Sin, N. C., Wong, K. S., et al. (2010). Failure to detect association between polymorphisms of the sodium channel gene SCN1A and febrile seizures in Chinese patients with epilepsy. Epilepsia 51 (9), 1878–1881. doi:10.1111/j.1528-1167.2010.02587.x
Zhang, Y., Hou, B., Liang, P., Lu, X., Wu, Y., Zhang, X., et al. (2021). TRPV1 channel mediates NLRP3 inflammasome-dependent neuroinflammation in microglia. Cell Death Dis. 12 (12), 1159. doi:10.1038/s41419-021-04450-9
Keywords: febrile seizures, disease occurrence, neurodevelopment, hippocampus, cortex
Citation: Yi Y, Zhong C and Wei-wei H (2023) The long-term neurodevelopmental outcomes of febrile seizures and underlying mechanisms. Front. Cell Dev. Biol. 11:1186050. doi: 10.3389/fcell.2023.1186050
Received: 14 March 2023; Accepted: 16 May 2023;
Published: 25 May 2023.
Edited by:
Seungwoo Kang, Augusta University, United StatesReviewed by:
Laurence De Nijs, Maastricht University, NetherlandsRao Fu, Sun Yat-sen University, China
Hongliu Sun, Binzhou Medical University, China
Copyright © 2023 Yi, Zhong and Wei-wei. This is an open-access article distributed under the terms of the Creative Commons Attribution License (CC BY). The use, distribution or reproduction in other forums is permitted, provided the original author(s) and the copyright owner(s) are credited and that the original publication in this journal is cited, in accordance with accepted academic practice. No use, distribution or reproduction is permitted which does not comply with these terms.
*Correspondence: Hu Wei-wei, aHV3d0B6anUuZWR1LmNu