- 1Key Laboratory of Molecular Biophysics of MOE, College of Life Science and Technology, Huazhong University of Science and Technology, Wuhan, Hubei, China
- 2State Key Laboratory for Diagnosis and Treatment of Infectious Diseases, National Clinical Research Center for Infectious Diseases, The First Affiliated Hospital, College of Medicine, Zhejiang University, Hangzhou, China
Micronutrients and cell death have a strong relationship and both are essential for human to maintain good body health. Dysregulation of any micronutrients causes metabolic or chronic diseases, including obesity, cardiometabolic condition, neurodegeneration, and cancer. The nematode Caenorhabditis elegans is an ideal genetic organism for researching the mechanisms of micronutrients in metabolism, healthspan, and lifespan. For example, C. elegans is a haem auxotroph, and the research of this special haem trafficking pathway contributes important reference to mammal study. Also, C. elegans characteristics including anatomy simply, clear cell lineage, well-defined genetics, and easily differentiated cell forms make it a powerful tool for studying the mechanisms of cell death including apoptosis, necrosis, autophagy, and ferroptosis. Here, we describe the understanding of micronutrient metabolism currently and also sort out the fundamental mechanisms of different kinds of cell death. A thorough understanding of these physiological processes not only builds a foundation for developing better treatments for various micronutrient disorders but also provides key insights into human health and aging.
Introduction
Nutrients are the foundations of organisms’ activities and survival. In addition to macromolecules carbohydrates, lipids, and proteins, many micronutrients including haem, vitamin, and amino acid are focused as essential elements of life. These micronutrients are principles to support biosynthetic and bioenergetic processes for growth, development, healthspan, and lifespan. Deficiency of micronutrients causes metabolic disorders and health damage (Figure 1). Organisms as seemingly different as nematode and mammals have much in common regarding nutrition metabolism. In C. elegans, different kinds of cell death are beneficial to overcome nutrient-limiting or stress conditions. For instance, vitamin B12 deficiency during early-life worms causes ferroptosis in germ-cell (Qin et al., 2022). Threonine supplementation protects against age-associated ferroptosis through the DAF-16 and HSF-1 pathways (Kim et al., 2022). Indeed, C. elegans is used as a genetic and visual model for cell death research due to easily observable morphology of cell death in living animals. Cell death is regulated by complex mechanisms during different cellular stages and research in vitro neglects many details of cell death. Studying cell death in vivo is more advantageous in C. elegans. Notably, regulation mechanism of cell death in C. elegans is similar to mammals. In this review, we discuss the essential molecule and regulatory pathway. It is hoped the researches on nutrition disorders and cell fate provide different understanding.
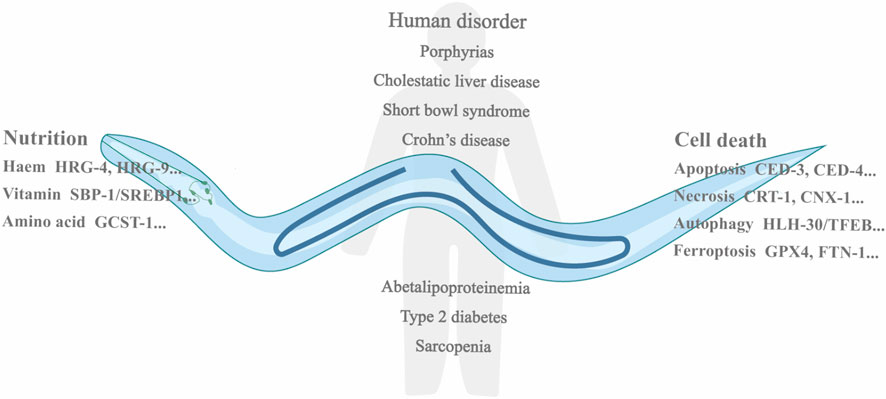
FIGURE 1. Showing micronutrient limitation related disorders in human and listing the genes in different types of nutrition metabolism and cell death in C. elegans. Nutrient deficiency causes multiple metabolism disorders in human, including porphyrias, cholestatic liver disease, short bowl syndrome, Crohm’s disease, and sarcopenia. In C. elegans, many genes are involved in the metabolisms of micronutrient, like HRG-4, HRG-9, SBP-1, and glycine cleavage system T-protein (GCST-1). Meanwhile, different kinds of cell death occur in C. elegans, and essential genes are also listed, for example, CED-3, CED-4, HLH-30/TFEB, and FTN-1.
The metabolism of haem
Haem is considered the essential biomolecule because of transferring electrons, binding oxygen, and catalyzing redox reactions with it in cellular (Koreny et al., 2022). Besides being used as an element of the electron transport chains, haem plays a vital function in the anabolic metabolism of steroids, fats, and further secondary metabolites, as well as the catabolism of exogenous substrates and endogenous metabolic complexes (Schenkman and Jansson, 2003). As a component of haemoglobin or myoglobin, haem is responsible for transporting diatomic gases to the blood in mammals (Chen et al., 2008). Haem in signal transduction, a sensor of nitric oxide (NO), is binding NO to the haem-containing domain, and subsequently soluble guanylyl cyclase is activated by the cyclase domain (Montfort et al., 2017). Haem plays a crucial regulatory molecule modulating the function of binding proteins like transcription factors and ion channels (Hou et al., 2006). The via of biosynthesizing haem is conserved, multi-step enzymatic pathway using amino acid, cofactors, and substance ferrous iron (Phillips, 2019). In the biosynthesis of haem, the initial and final three reactions happen in the mitochondria, while remaining steps take place in the cytosol (Koreny et al., 2022). The intermediates traverse organelle membranes for haem biosynthesis to proceed (Schultz et al., 2010). When haem biosynthetic pathway is defective, pathway intermediates are accumulated, which causes porphyrias. Porphyrias, a generic title for a type of metabolic disorder, leads to either neurologic or mitochondrial membrane photocutaneous symptoms phenotypically based on the haem metabolic intermediate that accumulates (Phillips, 2019).
Haem cannot be biosynthesized by C. elegans, so it is a favorable organism to research haem transferring pathways (Rao et al., 2005). In C. elegans, intestinal cells are responsible for the assimilation of haem exclusively from peripheral environment. Besides intaking haem from the diet and trafficking it to various tissue locations, C. elegans intestinal cell is involved in storing haem (Rajagopal et al., 2008; Chen et al., 2011; Korolnek et al., 2014). Haem-responsive gene 9 (HRG-9) in C. elegans, a haem chaperone, is conserved compared with yeasts and zebrafish and plays a crucial role in trafficking haem from its storage conditions to other tissue (Sun et al., 2022). HRG-4 traffics haem into worm intestinal cells, while HRG-1 is involved in mediating haem homeostasis in intracellular conditions (Rajagopal et al., 2008). HRG-3, a haem-trafficking protein, is responsible for intracellular carrier, but also for tissue-to-tissue transfer, including intestine-to-hypodermis, intestine-to-oocytes, intestine-to-muscle, intestine-to-neurons (Chen et al., 2011; Korolnek et al., 2014). Under HRG-3 deficiency, haem transport is dysregulated, which leads to either embryonic lethality or development arrest after hatching (Chen et al., 2011) (Figure 2).
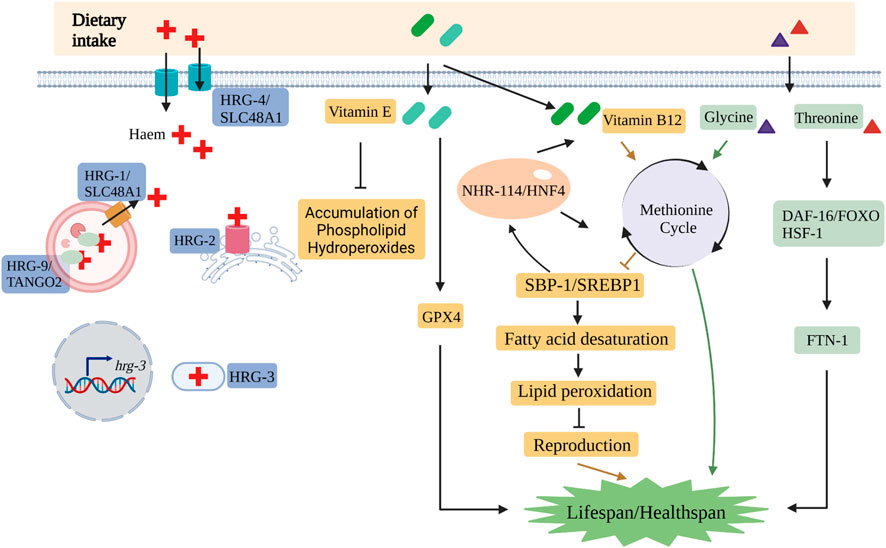
FIGURE 2. The nutrient metabolisms include many critical pathways in C. elegans. The key molecules involved in the trafficking pathways of haem are HRG-1/SLC48A1, HRG-2, HRG-3, HRG-4/SLC48A1 and HRG-9/TANGO2.1 in C. elegans. Vitamin B12 affects reproduction by methionine cycle-SBP-1/SREBP1-lipogenesis axis. Vitamin E suppresses the accumulation of phospholipid hydroperoxides and extends the lifespan of C. elegans via GPX4. Glycine and threonine play important roles in the lifespan and lipid peroxidation, respectively. (Created with BioRender.com).
The metabolism of vitamin
An organism always intakes diet which are converted available substances for reproduction, cell regeneration, and wound repair, as well as energy to support daily cellular and organismal function. Micronutrients including vitamins, cofactors, and essential minerals indirectly contribute to the generation of biomass and energy (Yilmaz and Walhout, 2014). Vitamin is an essential micronutrient for health that is much less abundant. Many vitamins are not synthesized by human or animal themselves and hence need to be obtained from daily diet. Recent studies revealed that body development and health were negatively affected because of vitamin deficiency. For instance, the current studies focus on the impact of maternal vitamin B12 deficiency during developmental offspring, specifically, it also causes the increase of obesity and insulin resistance in subsequent generations (Yajnik et al., 2008; Stewart et al., 2011; Ghosh et al., 2017; Tanwar et al., 2020). Vitamin C deficiency severely leads to scurvy, a potentially fatal condition (Carr and McCall, 2017). Vitamin D insufficiency is associated with autoimmune diseases, hypertension, diabetes, and cancer, particularly chronic kidney disease (Holick, 2005; Holick, 2007; Chau and Kumar, 2012). Shortage of vitamin E is linked to intestinal fat absorption diseases in human, such as hepatic cholestasis, short bowl syndrome, and Crohn’s disease (Muller et al., 1985). Vitamin supplementations with pharmacologic doses are beneficial for a healthy lifecycle in all kinds of organisms.
Because C. elegans lifespan is about 2–3 weeks at 20°C, it is an ideal model organism to systematically examine how vitamin affects longevity. C. elegans absorbs micronutrients by diet for growth and development. Depletion of specific vitamins induces developmental slowing or developmental arrest. For example, the study has revealed that vitamin B12 insufficiency during early-life worms occurred fat accumulation and reactive oxygen species (ROS) production in mature worms that subsequently triggered germline disorders due to ferroptosis (Qin et al., 2022). When vitamin B12 deficiency happened in the early life of worms, adult worms’ characteristics were affected by the methionine circuit, SBP-1/SREBP1 activity, and synthesis of lipid (Qin et al., 2022). Additionally, SBP-1/SREBP1 is involved in crucial feedback metabolism to regulate organismal vitamin B12 homeostasis (Qin et al., 2022). In C. elegans, administration of vitamin E not only suppressed the accumulation of phospholipid hydroperoxides but also prolonged the lifespan of mutant that lacks all homologous genes of GPX4 (Sakamoto et al., 2020). Moreover, intake of vitamin E during reproductive stage extended the C. elegans lifespan significantly (Sakamoto et al., 2020) (Figure 2).
The metabolism of nutrient amino acid
In the studies of model animals, dietary restriction (DR) refers to the targeted decrease of dietary components not including vitamins and minerals, and has been shown to promote longevity and improve adult worms’ healthspan (Green et al., 2022). Amino acid metabolism sustains organism life by serving as protein subunit, energy substrate, glutathione, and neurotransmitters. Disorder of nutrient amino acid has been studied and found to have serious effects on many physical functions, like maintaining healthspan and the modulation of aging. Leucine is a pharmaconutrient. In type 2 diabetes and sarcopenia disease, leucine supplementation has primarily been evaluated as a potential factor (van Loon, 2012). Leucine stimulates release of insulin and suppresses muscle protein damage and biosynthesis (van Loon, 2012). Administration of glycine from dietary supplementation is effective in treatment of metabolic disorders cardiovascular diseases, inflammatory diseases, obesity, diabetes, and cancer (Razak et al., 2017). Dysregulation of methionine metabolism is also associated with chronic liver diseases (Li et al., 2020a).
In C. elegans, many researchers have concentrated on the connection between nutrient amino acid supply and the regulation of longevity. Glycine extended the lifespan by the effect of methionine cycle in C. elegans (Liu et al., 2018). In aging C. elegans, glycine is accumulated steadily and significantly because the expression level of glycine cleavage and purine synthesis pathway genes decrease (Liu et al., 2018). However, by supplementing with glycine in diet, the lifespan in C. elegans is rescued with mutations in two methionine synthase enzymes, metr-1(ok521) and sams-1(ok3033) (Liu et al., 2018). The worms daf-2(e1370) and eat-2(ad456) are long-lived phenotypes, where glycine mediated longevity via the methionine cycle-dependent manner, and all of these findings remain consistent with serine supplementation (Liu et al., 2018). A biguanide drug metformin is extensively treated for type 2 diabetes and metabolic syndrome. The impact of metformin is mediated by E. coli that is co-cultured with it, with metformin inhibiting folate production and methionine circuit of E. coli. Consequently, the changed methionine metabolism induced by the co-cultured E. coli extended C. elegans lifespan (Cabreiro et al., 2013). A responsive complex via the methylglyoxal-regulated proteasome is activated by disturbed threonine catabolism, which further enhanced healthspan of C. elegans (Kim et al., 2022). The longevity effects of threonine rely on cellular oxidative stress and transcription factors daf-16 and hsf-1 (Kim et al., 2022) (Figure 2).
The mechanism of apoptosis
Apoptosis is one of programmed cell death and is critically important for genome stability, stress response, immunity, development, and differentiation (Fuchs and Steller, 2011; Kuranaga, 2011). Apoptosis is conserved across the evolutionary tree from lower creatures to mammals. In mammals, apoptosis is induced by the intrinsic and extrinsic pathways (Ow et al., 2008). Among metazoans, the intrinsic pathway is highly conserved. However, the critical stage from mitochondria, the release of cytochrome C, is not detected in C. elegans and Drosophila (Oberst et al., 2008). In apoptosis, the transcriptional level of BH3 (one of the BCL-2 family), and release of cytochrome C, is increased by intrinsic controlled and genotoxic agents within mitochondria. Caspase 9 is activated by combination of cytochrome C and Apaf-1, which further causes the activation of caspases 3 and 7 (Oberst et al., 2008). The fas ligand (FasL), tumor necrosis factor (TNF), and TNF-related apoptosis-inducing ligand (TRAIL) are all involved in activating the extrinsic death pathway (Nagata, 1997; Krammer, 2000; Strasser et al., 2009). When FasL and its receptor Fas are combined, it triggers the generation of death-inducing signaling complex (DISC) (Oberst et al., 2008). The complex is composed of Fas, called FADD (an adaptor protein), and procaspase 8 (Oberst et al., 2008). The DISC generation results in the activation of caspase 8, which subsequently triggers two different cell types. One type (like thymocytes), caspase 8 directly triggers caspase 3 to lead to cell death. Another type (like hepatocytes), caspase 8 cleaves the protein Bid, which is the BCL-2 family. Bid promotes to increase of cytochrome C, which further activates caspase 9 and caspase 3.
In C. elegans, apoptosis takes place in embryonic development and adult germline (Conradt et al., 2016). In 2002, Sydney Brenner, John E. Sulston, and H. Robert Horvitz received the Nobel Prize for Medicine because of significant contributions to discovering the cell lineage and identifying the molecular mechanism of cell death in C. elegans. The main proteins playing a vital role in apoptosis in C. elegans are EGL-1 and CED-family members (Adams and Cory, 2001) (Figure 3). EGL-1 is a crucial activator of apoptotic cell that is destined to die. CED-9 plays an important role like BCL-2 in preventing apoptosis (Adams and Cory, 2001). CED-4 (homolog of APAF-1 in mammalian) functions as a trigger for CED-3, leading to the initiation of cell death (Gartner et al., 2008). Elevated activity of EGL-1 blocks the activity of CED-9, activating CED-4 and CED-3, which ultimately triggers the process of apoptosis (Horvitz, 2003; Gartner et al., 2008; Conradt et al., 2016). CED-3, CED-4, EGL-1, and CED-9 are all participated in a pathway in that EGL-1 initiates cell death by acting upstream of CED-9, CED-9 prevents cell death by acting upstream of CED-4, and CED-4 activities cell death via upstream of CED-3 (Aballay and Ausubel, 2001). CED-8 acts as a substrate for CED-3 caspase cleavage, which induces apoptosis (Chen et al., 2013; Suzuki et al., 2013).
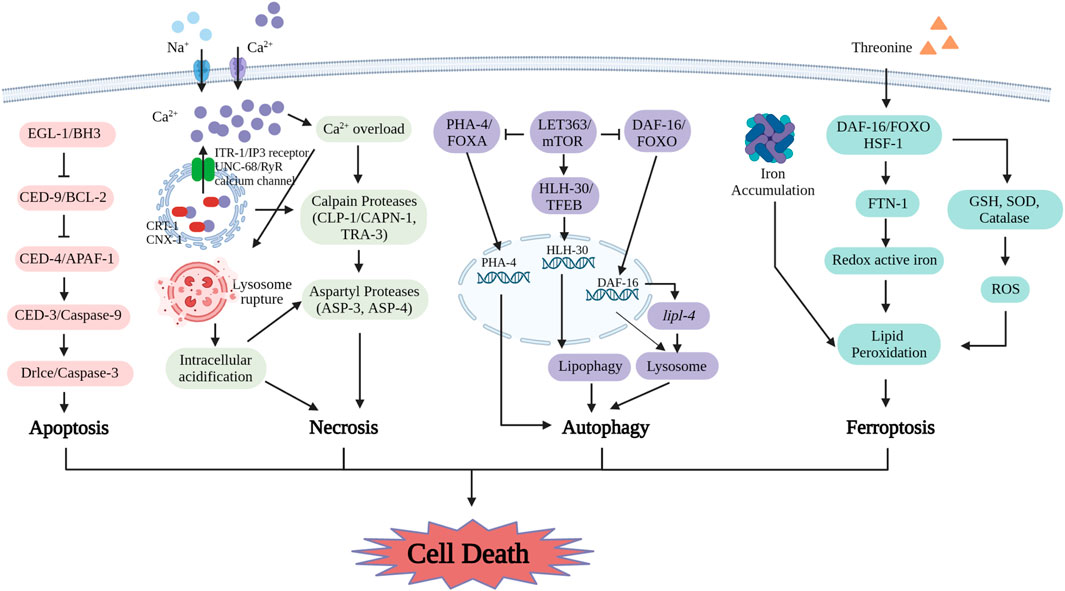
FIGURE 3. The mechanisms of cell death including apoptosis, necrosis, autophagy and ferroptosis in C. elegans are uncovered with key detail. The proteins involved in apoptosis are EGL-1/BH3, CED-9/BCL-2, CED-4/APAF-1, CED-3/Caspase-9 and Drlce/Caspase-3. In necrosis, these molecules include ITR-1/IP3 receptor, UNC-68/RyR calcium channel, CRT-1, CNX-1, CLP-1/CAPN-1, TRA-3, ASP-3 and ASP-4. The key regulators in autophagy include LET363/mTOR, PHA-4/FOXA, DAF-16/FOXO and HLH-30/TFEB. Ferroptosis process is induced by ferritin-dependent manner. Supplement of threonine affects ferroptosis by lipid peroxidation including the levels of DAF-16/FOXO, FTN-1, redox active iron, GSH, SOD, catalase and ROS. (Created with BioRender.com).
The mechanism of necrosis
Necrosis, a form of programmed cell death, frequently occurs in severe environments (Walker et al., 1988). Characteristics of necrosis are cellular and organelle swelling, breakdown of the premature membrane, and the release of intracellular substances to surrounding tissues, which leads to inflammation (Majno and Joris, 1995; Festjens et al., 2006).
The initial molecular mediator of necrosis pathway was cyclophilin D, a positive regulator that modulates the opening probability of the mitochondrial permeability transition pore (mPTP) (Baines et al., 2005; Halestrap, 2009). The initiation of necrosis via the mitochondrial pathway is triggered by the activation of mPTP in the inner mitochondrial membrane (IMM), which is activated by Ca2+ influx. In necrosis, the rapid activation of the mPTP leads to the dissipation of the proton that is responsible for driving ATP biosynthesis in mitochondrial across the IMM (Del Re et al., 2019). The mPTP opening by Ca2+ induced in the mitochondrial pathway plays a crucial role during necrosis. Another form of necrosis is affected by apoptosis receptors as well as the receptor-interacting protein kinases 1 and 3 (RIPK1 and RIPK3) (Galluzzi et al., 2009). In the death receptor pathway, the activation of RIPK3, a serine/threonine kinase, is the key event that triggers necroptosis, which is carried out through phosphorylation by its homologous RIPK1. Once activated, RIPK3 phosphorylates and a pseudokinase called mixed lineage kinase-like domain (MLKL), which oligomerizes and permeabilizes the plasma membrane, resulting in necrosis. RIPK3-MLKL pathway is recognized as the specific mechanism during necrosis (Del Re et al., 2019). Another type of induced necrosis occurs when poly ADP-ribose polymerase (PARP) is excessively triggered due to DNA damage (Zong et al., 2004). PARP1 is a nuclear enzyme, which is quickly triggered by the break of DNA and induces DNA restoration by adding ADP-ribose to chromatin-correlated proteins (Degterev and Yuan, 2008). Alkylating DNA damage induces to decrease of NAD+ by PARP1 in cytosolic that results in necrosis via ‘energy collapse’ during glycolysis that relies on cytosolic NAD+ for energy metabolism (Zong et al., 2004).
In C. elegans, the mechanisms and morphological features of necrosis resulting from aberration channel function and ionic imbalance exhibit similarities to excitotoxicity observed in vertebrates (Nikoletopoulou and Tavernarakis, 2014). Previous research discussed a novel example for excitotoxicity by examining the systemic effects of various triggers on glutamate-induced necrosis (Mano and Driscoll, 2009). Excitotoxicity critically depends on Ca2+ influx through glutamate-gated ion channels. Intracellular calcium overload is considered one of the initial steps in the necrosis pathway (Kourtis and Tavernarakis, 2007). In the necrosis pathway, endoplasmic reticulum Ca2+ binding proteins (CRT-1 and CNX-1) and channels (UNC-68 and ITR-1), Ca2+ dependent proteases (CLP-1 and TRA-3), and mitochondria permeability transition (MPT) are involved in regulating Ca2+ signaling (Geng et al., 2012). Gain-of-function mutations mec-4, the DEG/ENaC subunit, activate six mechanosensory neurons to result in excitotoxic necrosis of the cells in C. elegans (Syntichaki and Tavernarakis, 2003; Furuta et al., 2021). The gain-of-function mec-4 mutation alters the generation of the mechanosensory Na+ channel and makes it permeable to extracellular Ca2+ (Bianchi et al., 2004). The mutant mec-4 also causes necrosis and is partially suppressed by calreticulin. The Ca2+ chaperone residing in the endoplasmic reticulum (ER) assists in accumulating Ca2+ within the ER while deactivating channels that release Ca2+ from the ERs (Xu et al., 2001). Intracellular pH is a crucial inducing factor of necrosis in C. elegans. During necrosis, the vacuolar H+-ATPase is a pump that is responsible for acidifying lysosomes and other organelles within cell is necessary to promote necrosis after cytoplasmic calcium overload (Syntichaki et al., 2005). In necrosis, overload calcium actives calpain, which disrupted lysosomal membranes leading to the increase of acidic contents in cytoplasm (Syntichaki and Tavernarakis, 2003; Syntichaki et al., 2005) (Figure 3).
The mechanism of autophagy
Autophagy is an evolutionarily conserved cell death in that intracellular macromolecules are decomposed into multiple substances in the lysosomes (Mizushima and Komatsu, 2011). The main classifications of autophagy are macroautophagy, microautophagy, and chaperone-mediated autophagy. Macroautophagy is the most extensive type of autophagy compared to microautophagy and chaperone-mediated autophagy. The crucial functions of autophagy are to eliminate impaired or aged organelles and sustain energy homeostasis and engage intracellular nutrients to satisfy energy demands during nutrient deficiency (Klionsky and Emr, 2000).
Autophagy is strongly activated by nutrient deficiency and multiple stresses and quickly produces autophagosomes (Mizushima and Komatsu, 2011). Autophagy is activated by dephosphorylation of the serine or threonine protein kinase (ULK1). The ULK1 complex, consisting ULK1, ATG13, ATG101, and FIP200, breaks up from mechanistic/mammalian target of rapamycin complex 1(mTORC1) complex. The initiation of autophagy is activated by ULK1 and the phosphorylates ATG13 and FIP200 (Chang and Neufeld, 2009; Hosokawa et al., 2009; Jung et al., 2009). Phosphorylation of both Ambra1 and Beclin-1 by ULK1 complex results in the activation of the Beclin-1-Vps34 complex (Beclin-1, Vps34, Vps15, and ATG14L) (Di Bartolomeo et al., 2010). Autophagy is triggered by the interplay of Ambra1 and TRAF6, which modulates the balance and activity of ULK1 (Nazio et al., 2013). Autophagy is also induced through phosphorylation of Beclin-1 by AKT and EGFR1 (Wang et al., 2012; Wei et al., 2013). Autophagosome generation is enhanced by the recruitment of effector proteins through phosphatidylinositol-3-phosphate (PI3P) produced by Vps34 (Axe et al., 2008; Polson et al., 2010; Lu et al., 2011). The extension of the autophagosome is promoted by two systems, ATG12–ATG5–ATG16L and LC3–phosphatidylethanolamine (Ichimura et al., 2000; Kuma et al., 2002; Fujita et al., 2008). After the establishment of fully developed autophagic vesicles, mature autophagosomes, and lysosomes together merge, resulting in autolysosomes responsible for the decomposition of molecules and organelles (Mizushima and Komatsu, 2011).
In C. elegans, autophagy occurs mainly due to dietary restrictions and stress conditions. In C. elegans, initiation of autophagy is modulated by critical elements, such as mTOR signaling, a serine/threonine kinase, and DAF-16(a homolog of mammalian FOXO) that acting as an activator (Dall and Færgeman, 2019) (Figure 3). Both dietary restriction and suppression of the mTOR pathway activate autophagy in C. elegans. Conversely, inactivation of autophagy-related genes prevents the lifespan extension caused by these conditions (Hansen et al., 2008). Extended lifespan due to LET-363, DAF-15, or RSKS-1 mutant was eliminated by the autophagy modulatory transcription factor HLH30/TFEB and autophagy proteins (Hansen et al., 2008; Lapierre et al., 2013). let-363 or rsks-1 RNAi results in extending lifespan, which requires the involvement of PHA-4, which manages autophagy and is counteracted by mTORC1 pathway (Sheaffer et al., 2008). Autophagy has beneficial effects on metabolism. Autophagy is activated by upregulated transcriptionally of lysosomal acid lipase lipl-4, which extends lifespan via lipid regulatory (Lapierre et al., 2011; Folick et al., 2017). LGG-1 and LGG-2, are two ATG8 orthologs in C. elegans that function to degrade sequestosome-related (SQST-1) at different stages of the aggrephagy pathway (Wu et al., 2015). LGG-1 is necessary for the disassembly of SQST-1 throughout development, whereas the necessity for LGG-2 is temporal (Wu et al., 2015). In the embryonic period, a degradation defect of SQST-1 aggregates occurs when LGG-2 disables its role, whereas in the larval, SQST-1 is eliminated as normal (Wu et al., 2015). Autophagy mutants show distinct models of SQST-1 aggregation in various tissues and different period (Zheng et al., 2020). Different autophagy mutants exhibit distinct modes of SQST-1 aggregates in various tissues and periods (Zheng et al., 2020). Calpain family member CLP-2 degrades SQST-1 in a tissue- and stage-specific way in C. elegans (Zheng et al., 2020). Regulation of lipid phase separation of PGL particles by mTORC1 signaling under heat stress during embryonic period contributes to coordinating heat stress adaptation and autophagic degradation (Zhang et al., 2018a).
The mechanism of ferroptosis
Ferroptosis is a form of cell death, which depends on iron and is distinguished by oxidative injury to cellular membranes (Dixon et al., 2012; Galluzzi et al., 2018). The occurrence of ferroptosis has been established in diverse mammals, such as murine and cancer cell lines (Ingold et al., 2018). The production of ferric iron (Fe3+) triggers the production of ROS and activates lipoxygenases, ultimately resulting in damage of cell membrane (Yang et al., 2016). Ferroptosis displayed unique morphological features and dense, compact mitochondria that distinguish from apoptosis and necrosis (Dixon et al., 2012). Biochemically, the solute carrier family 7 member 11-glutathione-glutathione peroxidase 4 (SLC7A11-GSH-GPX4) signaling axis is thought to comprise the major defense system in ferroptosis (Koppula et al., 2018). SLC7A11, which is also called xCT, serves as a critical constituent of the cystine/glutamate antiporter system Xc− (Koppula et al., 2018). By facilitating the import of cystine and the export of glutamate in cellular, this antiporter activity relies on SLC7A11 (Koppula et al., 2018). Cysteine acts as the precursor that limits synthesis of GSH, a vital cofactor required by GPX4 to facilitate the detoxification of lipid peroxides (Koppula et al., 2021). SLC7A11, as a main BAP1 target gene in ferroptosis of cancers cell, is regulated by BRCA1-associated protein 1 (BAP1) on epigenetic mechanism (Zhang et al., 2018b). Specially, previous studies demonstrate that BAP1 downregulates SLC7A11 expression by reducing histone 2A ubiquitination occupancy on its promoter (Zhang et al., 2018b). As a result, cystine uptake is inhibited, resulting in increased lipid peroxidation and ultimately ferroptosis (Zhang et al., 2018b). Also, SLC7A11 and H2Aub location on its promoter are under the control of polycomb repressive complex 1 (PRC1), a key H2Aub ubiquitin ligase (Zhang et al., 2019). SLC7A11-regulted uptake of cystine and cysteine leads to activation of mTORC1 and subsequently promotes GPX4 protein biosynthesis via the Rag-mTORC1-4EBP pathway (Zhang et al., 2021). Furthermore, inactivation of mTORC1 makes cancer cells sensitive to ferroptosis by inhibiting GPX4 biosynthesis (Zhang et al., 2021). The process of ferroptosis is triggered by intracellular depletion of GSH and subsequent reduction in GPX4 activity. As a result, lipid peroxides cannot be efficiently regulated through the GPX4-catalyzed reaction, resulting in the accumulation of ROS. In the presence of Fe2+, lipids are oxidized in a Fenton-like way, further increasing ROS levels and ultimately inducing ferroptosis (Yang and Stockwell, 2008; Friedmann Angeli et al., 2014). Recently, a study termed a new cell death, named disulfidptosis (Liu et al., 2020; Liu et al., 2023). Under glucose starvation, disulfidptosis is observed in cancer cells, where SLC7A11 expresses abnormally, cystine uptake increases, cystine reduces to cysteine, and the NADPH pool depletes, which results in excess accumulation of intracellular disulfide molecules and rapid cell death (Liu et al., 2020; Liu et al., 2023).
The crucial endogenous mechanism of ferroptosis to counter lipid peroxidation is GPX4 (Chen et al., 2014; Matsuda et al., 2016). Glutathione serves as the primary co-ordinating substrate for cytosolic ferrous iron and is utilized by GPX4 as a precursor to elimination of the lipid peroxides subsequently inducing ferroptosis (Hider and Kong, 2011; Dixon and Stockwell, 2014; Friedmann Angeli et al., 2014; Yang et al., 2014). The effect of GPX4 depends on GSH produced by the plasma membrane Xc− (Dixon et al., 2012). SLC7A11 imports extracellular cystine into cells to promote GSH synthesis, resulting in protection from oxidative stress and ferroptosis (Zhang et al., 2021). Suppression of system Xc−-activity has a major impact on GSH synthesis by decreasing the intake of cystine, resulting in decrease of GPX activity and cell antioxidant capacity, ROS accumulation, and subsequently oxidative damage and ferroptosis (Li et al., 2020b).
In C. elegans, the increase of unstable ferric iron is correlated with genetic factors that expedite aging and pose a threat to lifespan (Xu et al., 2010; James et al., 2015; James et al., 2016) (Figure 3). In C. elegans, inhibiting ferroptosis by preventing lipid peroxidation and iron accumulation, reduce age-related cell death and significantly improves lifespan and healthspan (Jenkins et al., 2020). The increased threonine concentration diminished ferroptosis via ferritin-dependent way, activated ferritin 1 and antioxidant reaction, and extended longevity and health via a DAF-16 and HSF-1-dependent mechanism in C. elegans (Kim et al., 2022). Dietary lipids also can impact cell fates. In germ cells of C. elegans, ferroptosis is activated by dietary dihomo gamma linolenic acid (DGLA), which was modulated by redox reaction and iron metabolism (Perez et al., 2020). Endogenous ether-lipid synthesis and elevating monounsaturated fatty acid (MUFA) levels with genetic or dietary intervention are adequate to protect DGLA-induced germ-cell ferroptosis (Perez et al., 2020).
Conclusion and future directions
For both human and worm, nutrition metabolism and cell death are the basis of life. In this review, we listed many crucial molecules and regulatory pathways in nematode and human. In C. elegans, haem cannot be biosynthesized by itself, so the multi-step enzymatic pathway of biosynthesizing haem is not conserved between human and worms. However, haem uptake and transport systems exist in cellular compartments in C. elegans, and haem binding proteins are evolutionarily conserved haem chaperone in yeast and mammalian cells. Vitamin and nutrient amino acid deficiency in both human and worms has negative effects on development and health. The crucial components of cell death are also conserved, such as EGL-1 and CED-family member (homolog of BCL-2 family, APAF-1 and Caspase in mammalian) in apoptosis, calpain proteases in necrosis, initiation of autophagy LET363 (homolog of mTOR) and autophagy modulatory transcription factor HLH-30 (homolog of TFEB). And ferroptosis also depends on iron overload, which further triggers the production of ROS and activities lipoxygenases in C. elegans. Nevertheless, many micronutrients and their metabolic pathway remain elusive. New types of cell death have been reported recently, like disulfidptosis. However, many novel cell death pathways remain undiscovered. C. elegans, as a power model, plays a crucial role for the basic research of nutrition metabolism and cell death.
Absorbing nutrients and processing cell death in nematode and human are related to survival. How does C. elegans intake micronutrients from environment, transport them to cell, and what are the details about enzymes and membrane carriers in these processes? What are the storage form, location, and utilization of micronutrients? The answers to these questions are still not clear. Finally, how can we apply better treatments for nutrition disorders? Similarly, the rigorous mechanism of cell death remains to be further explored. The probes for the identification research in cell death type rapidly need to be further exploited. The micronutrition metabolism and cell death, the genetic and functional characterization study about essential molecules and regulatory pathways in nematode is complex, which can still reveal the fundamental biology principles in human.
Author contributions
All authors listed have made a substantial, direct, and intellectual contribution to the work and approved it for publication. All authors contributed to the article and approved the submitted version.
Funding
This work is funded by the National Natural Science Foundation of China (82071851 and 32100604), the program for HUST Academic Frontier Youth Team (5001170068), Fundamental Research Funds for the Central Universities (2022ZFJH003), and National Key Research and Development Program (2020YFE0204300).
Conflict of interest
The authors declare that the research was conducted in the absence of any commercial or financial relationships that could be construed as a potential conflict of interest.
Publisher’s note
All claims expressed in this article are solely those of the authors and do not necessarily represent those of their affiliated organizations, or those of the publisher, the editors and the reviewers. Any product that may be evaluated in this article, or claim that may be made by its manufacturer, is not guaranteed or endorsed by the publisher.
References
Aballay, A., and Ausubel, F. M. (2001). Programmed cell death mediated by ced-3 and ced-4 protects Caenorhabditis elegans from Salmonella typhimurium-mediated killing. Proc. Natl. Acad. Sci. 98, 2735–2739. doi:10.1073/pnas.041613098
Adams, J. M., and Cory, S. (2001). Life-or-death decisions by the Bcl-2 protein family. Trends Biochem. Sci. 26, 61–66. doi:10.1016/s0968-0004(00)01740-0
Axe, E. L., Walker, S. A., Manifava, M., Chandra, P., Roderick, H. L., Habermann, A., et al. (2008). Autophagosome formation from membrane compartments enriched in phosphatidylinositol 3-phosphate and dynamically connected to the endoplasmic reticulum. J. Cell. Biol. 182, 685–701. doi:10.1083/jcb.200803137
Baines, C. P., Kaiser, R. A., Purcell, N. H., Blair, N. S., Osinska, H., Hamblaton, M. A., et al. (2005). Loss of cyclophilin D reveals a critical role for mitochondrial permeability transition in cell death. Nature 434, 658–662. doi:10.1038/nature03434
Bianchi, L., Gerstbrein, B., Frokjaer-Jensen, C., Royal, D. C., Mukherjee, G., Royal, M. A., et al. (2004). The neurotoxic MEC-4(d) DEG/ENaC sodium channel conducts calcium: Implications for necrosis initiation. Nat. Neurosci. 7, 1337–1344. doi:10.1038/nn1347
Cabreiro, F., Au, C., Leung, K. Y., Vergara-Irigaray, N., Cocheme, H. M., Noori, T., et al. (2013). Metformin retards aging in C. elegans by altering microbial folate and methionine metabolism. Cell. 153, 228–239. doi:10.1016/j.cell.2013.02.035
Carr, A. C., and McCall, C. (2017). The role of vitamin C in the treatment of pain: New insights. J. Transl. Med. 15, 77. doi:10.1186/s12967-017-1179-7
Chang, Y. Y., and Neufeld, T. P. (2009). An Atg1/Atg13 complex with multiple roles in TOR-mediated autophagy regulation. Mol. Biol. Cell. 20, 2004–2014. doi:10.1091/mbc.e08-12-1250
Chau, Y. Y., and Kumar, J. (2012). Vitamin D in chronic kidney disease. Indian J. Pediatr. 79, 1062–1068. doi:10.1007/s12098-012-0765-1
Chen, C., Samuel, T. K., Sinclair, J., Dailey, H. A., and Hamza, I. (2011). An intercellular heme-trafficking protein delivers maternal heme to the embryo during development in C. elegans. Cell. 145, 720–731. doi:10.1016/j.cell.2011.04.025
Chen, H., Ikeda-Saito, M., and Shaik, S. (2008). Nature of the Fe-O2 bonding in oxy-myoglobin: Effect of the protein. J. Am. Chem. Soc. 130, 14778–14790. doi:10.1021/ja805434m
Chen, X., Li, W., Ren, J., Huang, D., He, W. T., Song, Y., et al. (2014). Translocation of mixed lineage kinase domain-like protein to plasma membrane leads to necrotic cell death. Cell. Res. 24, 105–121. doi:10.1038/cr.2013.171
Chen, Y. Z., Mapes, J., Lee, E. S., Skeen-Gaar, R. R., and Xue, D. (2013). Caspase-mediated activation of Caenorhabditis elegans CED-8 promotes apoptosis and phosphatidylserine externalization. Nat. Commun. 4, 2726. doi:10.1038/ncomms3726
Conradt, B., Wu, Y. C., and Xue, D. (2016). Programmed cell death during Caenorhabditis elegans development. Genetics 203, 1533–1562. doi:10.1534/genetics.115.186247
Dall, K. B., and Færgeman, N. J. (2019). Metabolic regulation of lifespan from a C. elegans perspective. Genes. & Nutr. 14, 25. doi:10.1186/s12263-019-0650-x
Degterev, A., and Yuan, J. (2008). Expansion and evolution of cell death programmes. Nat. Rev. Mol. Cell. Biol. 9, 378–390. doi:10.1038/nrm2393
Del Re, D. P., Amgalan, D., Linkermann, A., Liu, Q., and Kitsis, R. N. (2019). Fundamental mechanisms of regulated cell death and implications for heart disease. Physiol. Rev. 99, 1765–1817. doi:10.1152/physrev.00022.2018
Di Bartolomeo, S., Corazzari, M., Nazio, F., Oliverio, S., Lisi, G., Antonioli, M., et al. (2010). The dynamic interaction of AMBRA1 with the dynein motor complex regulates mammalian autophagy. J. Cell. Biol. 191, 155–168. doi:10.1083/jcb.201002100
Dixon, S. J., Lemberg, K. M., Lamprecht, M. R., Skouta, R., Zaitsev, E. M., Gleason, C. E., et al. (2012). Ferroptosis: An iron-dependent form of nonapoptotic cell death. Cell. 149, 1060–1072. doi:10.1016/j.cell.2012.03.042
Dixon, S. J., and Stockwell, B. R. (2014). The role of iron and reactive oxygen species in cell death. Nat. Chem. Biol. 10, 9–17. doi:10.1038/nchembio.1416
Festjens, N., Vanden Berghe, T., and Vandenabeele, P. (2006). Necrosis, a well-orchestrated form of cell demise: Signalling cascades, important mediators and concomitant immune response. Biochim. Biophys. Acta 1757, 1371–1387. doi:10.1016/j.bbabio.2006.06.014
Folick, A., OakleyHolly, D. Y., Armstrong, H. E., Kumari, M., and Sanor, L. (2017). Lysosomal signaling molecules regulate longevity in Caenorhabditis elegans.
Friedmann Angeli, J. P., Schneider, M., Proneth, B., Tyurina, Y. Y., Tyurin, V. A., Hammond, V. J., et al. (2014). Inactivation of the ferroptosis regulator Gpx4 triggers acute renal failure in mice. Nat. Cell. Biol. 16, 1180–1191. doi:10.1038/ncb3064
Fuchs, Y., and Steller, H. (2011). Programmed cell death in animal development and disease. Cell. 147, 742–758. doi:10.1016/j.cell.2011.10.033
Fujita, N., Itoh, T., Omori, H., Fukuda, M., Noda, T., and Yoshimori, T. (2008). The Atg16L complex specifies the site of LC3 lipidation for membrane biogenesis in autophagy. Mol. Biol. Cell. 19, 2092–2100. doi:10.1091/mbc.e07-12-1257
Furuta, Y., Pena-Ramos, O., Li, Z., Chiao, L., and Zhou, Z. (2021). Calcium ions trigger the exposure of phosphatidylserine on the surface of necrotic cells. PLoS Genet. 17, e1009066. doi:10.1371/journal.pgen.1009066
Galluzzi, L., Kepp, O., and Kroemer, G. (2009). RIP kinases initiate programmed necrosis. J. Mol. Cell. Biol. 1, 8–10. doi:10.1093/jmcb/mjp007
Galluzzi, L., Vitale, I., Aaronson, S. A., Abrams, J. M., Adam, D., Agostinis, P., et al. (2018). Molecular mechanisms of cell death: Recommendations of the nomenclature committee on cell death 2018. Cell. Death Differ. 25, 486–541. doi:10.1038/s41418-017-0012-4
Gartner, A., Boag, P. R., and Blackwell, T. K. (2008). “Germline survival and apoptosis,” in Woembook: The C. elegans Research Community. Available at: http://wormbook.org/chapters/www_germlinesurvival/germlinesurvival.html, http://www.wormbook.org/ (Accessed September 4, 2008).
Geng, X., Harry, B. L., Zhou, Q., Skeen-Gaar, R. R., Ge, X., Lee, E. S., et al. (2012). Hepatitis B virus X protein targets the Bcl-2 protein CED-9 to induce intracellular Ca2+ increase and cell death in Caenorhabditis elegans. Proc. Natl. Acad. Sci. U. S. A. 109, 18465–18470. doi:10.1073/pnas.1204652109
Ghosh, S., Sinha, J. K., Muralikrishna, B., Putcha, U. K., and Raghunath, M. (2017). Chronic transgenerational vitamin B12 deficiency of severe and moderate magnitudes modulates adiposity-probable underlying mechanisms. Biofactors 43, 400–414. doi:10.1002/biof.1350
Green, C. L., Lamming, D. W., and Fontana, L. (2022). Molecular mechanisms of dietary restriction promoting health and longevity. Nat. Rev. Mol. Cell. Biol. 23, 56–73. doi:10.1038/s41580-021-00411-4
Halestrap, A. P. (2009). What is the mitochondrial permeability transition pore? J. Mol. Cell. Cardiol. 46, 821–831. doi:10.1016/j.yjmcc.2009.02.021
Hansen, M., Chandra, A., Mitic, L. L., Onken, B., Driscoll, M., and Kenyon, C. (2008). A role for autophagy in the extension of lifespan by dietary restriction in C. elegans. PLoS Genet. 4, e24. doi:10.1371/journal.pgen.0040024
Hider, R. C., and Kong, X. L. (2011). Glutathione: A key component of the cytoplasmic labile iron pool. Biometals 24, 1179–1187. doi:10.1007/s10534-011-9476-8
Holick, M. F. (2005). Vitamin D in health and disease: Vitamin D for health and in chronic kidney disease: Vitamin D for health and ckd. Semin. Dial. 18, 266–275. doi:10.1111/j.1525-139x.2005.18402.x
Horvitz, H. R. (2003). Worms, life, and death (Nobel lecture). Chembiochem 4, 697–711. doi:10.1002/cbic.200300614
Hosokawa, N., Hara, T., Kaizuka, T., Kishi, C., Takamura, A., Miura, Y., et al. (2009). Nutrient-dependent mTORC1 association with the ULK1-Atg13-FIP200 complex required for autophagy. Mol. Biol. Cell. 20, 1981–1991. doi:10.1091/mbc.e08-12-1248
Hou, S., Reynolds, M. F., Horrigan, F. T., Heinemann, S. H., and Hoshi, T. (2006). Reversible binding of heme to proteins in cellular signal transduction. Acc. Chem. Res. 39, 918–924. doi:10.1021/ar040020w
Ichimura, Y., Kirisako, T., Takao, T., Satomi, Y., Shimonishi, Y., Ishihara, N., et al. (2000). A ubiquitin-like system mediates protein lipidation. Nature 408, 488–492. doi:10.1038/35044114
Ingold, I., Berndt, C., Schmitt, S., Doll, S., Poschmann, G., Buday, K., et al. (2018). Selenium utilization by GPX4 is required to prevent hydroperoxide-induced ferroptosis. Cell. 172, 409–422. doi:10.1016/j.cell.2017.11.048
James, S. A., Hare, D. J., Jenkins, N. L., de Jonge, M. D., Bush, A. I., and McColl, G. (2016). φXANES: In vivo imaging of metal-protein coordination environments. Sci. Rep. 6, 20350. doi:10.1038/srep20350
James, S. A., Roberts, B. R., Hare, D. J., de Jonge, M. D., Birchall, I. E., Jenkins, N. L., et al. (2015). Direct in vivo imaging of ferrous iron dyshomeostasis in ageing Caenorhabditis elegans. Chem. Sci. 6, 2952–2962. doi:10.1039/c5sc00233h
Jenkins, N. L., James, S. A., Salim, A., Sumardy, F., Speed, T. P., Conrad, M., et al. (2020). Changes in ferrous iron and glutathione promote ferroptosis and frailty in aging Caenorhabditis elegans. Elife 9, e56580. doi:10.7554/eLife.56580
Jung, C. H., Jun, C. B., Ro, S. H., Kim, Y. M., Otto, N. M., Cao, J., et al. (2009). ULK-Atg13-FIP200 complexes mediate mTOR signaling to the autophagy machinery. Mol. Biol. Cell. 20, 1992–2003. doi:10.1091/mbc.e08-12-1249
Kim, J., Jo, Y., Cho, D., and Ryu, D. (2022). L-threonine promotes healthspan by expediting ferritin-dependent ferroptosis inhibition in C. elegans. Nat. Commun. 13, 6554. doi:10.1038/s41467-022-34265-x
Klionsky, D. J., and Emr, S. D. (2000). Autophagy as a regulated pathway of cellular degradation. Science 290, 1717–1721. doi:10.1126/science.290.5497.1717
Koppula, P., Zhang, Y., Zhuang, L., and Gan, B. (2018). Amino acid transporter SLC7A11/xCT at the crossroads of regulating redox homeostasis and nutrient dependency of cancer. Cancer Commun. (Lond) 38, 12. doi:10.1186/s40880-018-0288-x
Koppula, P., Zhuang, L., and Gan, B. (2021). Cystine transporter slc7a11/xCT in cancer: Ferroptosis, nutrient dependency, and cancer therapy. Protein Cell. 12, 599–620. doi:10.1007/s13238-020-00789-5
Koreny, L., Obornik, M., Horakova, E., Waller, R. F., and Lukes, J. (2022). The convoluted history of haem biosynthesis. Biol. Rev. Camb Philos. Soc. 97, 141–162. doi:10.1111/brv.12794
Korolnek, T., Zhang, J., Beardsley, S., Scheffer, G. L., and Hamza, I. (2014). Control of metazoan heme homeostasis by a conserved multidrug resistance protein. Cell. Metab. 19, 1008–1019. doi:10.1016/j.cmet.2014.03.030
Kourtis, N., and Tavernarakis, N. (2007). Non-developmentally programmed cell death in Caenorhabditis elegans. Semin. Cancer Biol. 17, 122–133. doi:10.1016/j.semcancer.2006.11.004
Krammer, P. H. (2000). CD95's deadly mission in the immune system. Nature 407, 789–795. doi:10.1038/35037728
Kuma, A., Mizushima, N., Ishihara, N., and Ohsumi, Y. (2002). Formation of the approximately 350-kDa Apg12-Apg5.Apg16 multimeric complex, mediated by Apg16 oligomerization, is essential for autophagy in yeast. J. Biol. Chem. 277, 18619–18625. doi:10.1074/jbc.M111889200
Kuranaga, E. (2011). Caspase signaling in animal development. Dev. Growth Differ. 53, 137–148. doi:10.1111/j.1440-169X.2010.01237.x
Lapierre, L. R., De Magalhaes Filho, C. D., McQuary, P. R., Chu, C.-C., Visvikis, O., Chang, J. T., et al. (2013). The TFEB orthologue HLH-30 regulates autophagy and modulates longevity in Caenorhabditis elegans. Nat. Commun. 4, 2267. doi:10.1038/ncomms3267
Lapierre, L. R., Gelino, S., Melendez, A., and Hansen, M. (2011). Autophagy and lipid metabolism coordinately modulate life span in germline-less C. elegans. Curr. Biol. 21, 1507–1514. doi:10.1016/j.cub.2011.07.042
Li, J., Cao, F., Yin, H. L., Huang, Z. J., Lin, Z. T., Mao, N., et al. (2020). Ferroptosis: Past, present and future. Cell. Death Dis. 11, 88. doi:10.1038/s41419-020-2298-2
Li, Z., Wang, F., Liang, B., Su, Y., Sun, S., Xia, S., et al. (2020). Methionine metabolism in chronic liver diseases: An update on molecular mechanism and therapeutic implication. Signal Transduct. Target Ther. 5, 280. doi:10.1038/s41392-020-00349-7
Liu, X., Nie, L., Zhang, Y., Yan, Y., Wang, C., Colic, M., et al. (2023). Actin cytoskeleton vulnerability to disulfide stress mediates disulfidptosis. Nat. Cell. Biol. 25, 404–414. doi:10.1038/s41556-023-01091-2
Liu, X., Olszewski, K., Zhang, Y., Lim, E. W., Shi, J., Zhang, X., et al. (2020). Cystine transporter regulation of pentose phosphate pathway dependency and disulfide stress exposes a targetable metabolic vulnerability in cancer. Nat. Cell. Biol. 22, 476–486. doi:10.1038/s41556-020-0496-x
Lu, Q., Yang, P., Huang, X., Hu, W., Guo, B., Wu, F., et al. (2011). The WD40 repeat PtdIns(3)P-binding protein EPG-6 regulates progression of omegasomes to autophagosomes. Dev. Cell. 21, 343–357. doi:10.1016/j.devcel.2011.06.024
Majno, G., and Joris, I. (1995). Apoptosis, oncosis, and necrosis. An overview of cell death. Am. J. Pathology 146, 3–15.
Mano, I., and Driscoll, M. (2009). Caenorhabditis elegans glutamate transporter deletion induces AMPA-receptor/adenylyl cyclase 9-dependent excitotoxicity. J. Neurochem. 108, 1373–1384. doi:10.1111/j.1471-4159.2008.05804.x
Razak, M. A., Begum, P. S., Viswanath, B., and Rajagopal, S. (2017), Multifarious beneficial effect of nonessential amino acid, Glycine: A review. Oxid. Med. Cell. Longev. 2017, 1716701, doi:10.1155/2017/1716701
Matsuda, T., Zhai, P., Sciarretta, S., Zhang, Y., Jeong, J. I., Ikeda, S., et al. (2016). NF2 activates hippo signaling and promotes ischemia/reperfusion injury in the heart. Circ. Res. 119, 596–606. doi:10.1161/CIRCRESAHA.116.308586
Mizushima, N., and Komatsu, M. (2011). Autophagy: Renovation of cells and tissues. Cell. 147, 728–741. doi:10.1016/j.cell.2011.10.026
Montfort, W. R., Wales, J. A., and Weichsel, A. (2017). Structure and activation of soluble guanylyl cyclase, the nitric oxide sensor. Antioxidants Redox Signal. 26, 107–121. doi:10.1089/ars.2016.6693
Muller, D. P., Lloyd, J. K., and Wolff, O. H. (1985). The role of vitamin E in the treatment of the neurological features of abetalipoproteinaemia and other disorders of fat absorption. J. Inherit. Metab. Dis. 8 (1), 88–92. doi:10.1007/BF01800666
Nazio, F., Strappazzon, F., Antonioli, M., Bielli, P., Cianfanelli, V., Bordi, M., et al. (2013). mTOR inhibits autophagy by controlling ULK1 ubiquitylation, self-association and function through AMBRA1 and TRAF6. Nat. Cell. Biol. 15, 406–416. doi:10.1038/ncb2708
Nikoletopoulou, V., and Tavernarakis, N. (2014). Necrotic cell death in Caenorhabditis elegans. Methods Enzymol. 545, 127–155. doi:10.1016/B978-0-12-801430-1.00006-8
Oberst, A., Bender, C., and Green, D. R. (2008). Living with death: The evolution of the mitochondrial pathway of apoptosis in animals. Cell. Death Differ. 15, 1139–1146. doi:10.1038/cdd.2008.65
Ow, Y. P., Green, D. R., Hao, Z., and Mak, T. W. (2008). Cytochrome c: Functions beyond respiration. Nat. Rev. Mol. Cell. Biol. 9, 532–542. doi:10.1038/nrm2434
Perez, M. A., Magtanong, L., Dixon, S. J., and Watts, J. L. (2020). Dietary lipids induce ferroptosis in Caenorhabditis elegans and human cancer cells. Dev. Cell. 54, 447–454. doi:10.1016/j.devcel.2020.06.019
Phillips, J. D. (2019). Heme biosynthesis and the porphyrias. Mol. Genet. Metab. 128, 164–177. doi:10.1016/j.ymgme.2019.04.008
Polson, H. E., de Lartigue, J., Rigden, D. J., Reedijk, M., Urbe, S., Clague, M. J., et al. (2010). Mammalian Atg18 (WIPI2) localizes to omegasome-anchored phagophores and positively regulates LC3 lipidation. Autophagy 6, 506–522. doi:10.4161/auto.6.4.11863
Qin, S., Wang, Y., Li, L., Liu, J., Xiao, C., Duan, D., et al. (2022). Early-life vitamin B12 orchestrates lipid peroxidation to ensure reproductive success via SBP-1/SREBP1 in Caenorhabditis elegans. Cell. Rep. 40, 111381. doi:10.1016/j.celrep.2022.111381
Rajagopal, A., Rao, A. U., Amigo, J., Tian, M., Upadhyay, S. K., Hall, C., et al. (2008). Haem homeostasis is regulated by the conserved and concerted functions of HRG-1 proteins. Nature 453, 1127–1131. doi:10.1038/nature06934
Rao, A. U., Carta, L. K., Lesuisse, E., and Hamza, I. (2005). Lack of heme synthesis in a free-living eukaryote. Proc. Natl. Acad. Sci. U. S. A. 102, 4270–4275. doi:10.1073/pnas.0500877102
Sakamoto, T., Maebayashi, K., Tsunoda, Y., and Imai, H. (2020). Inhibition of lipid peroxidation during the reproductive period extend s the lifespan of <i>Caenorhabditis elegans</i>. J. Clin. Biochem. Nutr. 66, 116–123. doi:10.3164/jcbn.19-51
Schenkman, J. B., and Jansson, I. (2003). The many roles of cytochrome b5. Pharmacol. Ther. 97, 139–152. doi:10.1016/s0163-7258(02)00327-3
Schultz, I. J., Chen, C., Paw, B. H., and Hamza, I. (2010). Iron and porphyrin trafficking in heme biogenesis. J. Biol. Chem. 285, 26753–26759. doi:10.1074/jbc.R110.119503
Sheaffer, K. L., Updike, D. L., and Mango, S. E. (2008). The Target of Rapamycin pathway antagonizes pha-4/FoxA to control development and aging. Curr. Biol. 18, 1355–1364. doi:10.1016/j.cub.2008.07.097
Stewart, C. P., Christian, P., Schulze, K. J., Arguello, M., LeClerq, S. C., Khatry, S. K., et al. (2011). Low maternal vitamin B12 status is associated with offspring insulin resistance regardless of antenatal micronutrient supplementation in rural Nepal. J. Nutr. 141, 1912–1917. doi:10.3945/jn.111.144717
Strasser, A., Jost, P. J., and Nagata, S. (2009). The many roles of FAS receptor signaling in the immune system. Immunity 30, 180–192. doi:10.1016/j.immuni.2009.01.001
Sun, F., Zhao, Z., Willoughby, M. M., Shen, S., Zhou, Y., Shao, Y., et al. (2022). HRG-9 homologues regulate haem trafficking from haem-enriched compartments. Nature 610, 768–774. doi:10.1038/s41586-022-05347-z
Suzuki, J., Denning, D., Imanishi, E., Horvitz, H., and Nagata, S. (2013). Xk-related protein 8 and CED-8 promote phosphatidylserine exposure in apoptotic cells. Sci. (New York, N.Y.) 341, 403–406. doi:10.1126/science.1236758
Syntichaki, P., Samara, C., and Tavernarakis, N. (2005). The vacuolar H+ -ATPase mediates intracellular acidification required for neurodegeneration in C. elegans. Curr. Biol. 15, 1249–1254. doi:10.1016/j.cub.2005.05.057
Syntichaki, P., and Tavernarakis, N. (2003). The biochemistry of neuronal necrosis: Rogue biology? Nat. Rev. Neurosci. 4, 672–684. doi:10.1038/nrn1174
Tanwar, V. S., Ghosh, S., Sati, S., Ghose, S., Kaur, L., Kumar, K. A., et al. (2020). Maternal vitamin B(12) deficiency in rats alters DNA methylation in metabolically important genes in their offspring. Mol. Cell. Biochem. 468, 83–96. doi:10.1007/s11010-020-03713-x
van Loon, L. J. (2012). Leucine as a pharmaconutrient in health and disease. Curr. Opin. Clin. Nutr. Metab. Care 15, 71–77. doi:10.1097/MCO.0b013e32834d617a
Walker, N. I., Harmon, B. V., Gobé, G., and Kerr, J. F. (1988). Patterns of cell death. Methods & Achiev. Exp. Pathology 13, 18–54.
Wang, R. C., Wei, Y., An, Z., Zou, Z., Xiao, G., Bhagat, G., et al. (2012). Akt-mediated regulation of autophagy and tumorigenesis through Beclin 1 phosphorylation. Science 338, 956–959. doi:10.1126/science.1225967
Wei, Y., Zou, Z., Becker, N., Anderson, M., Sumpter, R., Xiao, G., et al. (2013). EGFR-mediated Beclin 1 phosphorylation in autophagy suppression, tumor progression, and tumor chemoresistance. Cell. 154, 1269–1284. doi:10.1016/j.cell.2013.08.015
Wu, F., Watanabe, Y., Guo, X. Y., Qi, X., Wang, P., Zhao, H. Y., et al. (2015). Structural basis of the differential function of the two C. elegans Atg8 homologs, LGG-1 and LGG-2, in autophagy. Mol. Cell. 60, 914–929. doi:10.1016/j.molcel.2015.11.019
Xu, J., Marzetti, E., Seo, A. Y., Kim, J. S., Prolla, T. A., and Leeuwenburgh, C. (2010). The emerging role of iron dyshomeostasis in the mitochondrial decay of aging. Mech. Ageing Dev. 131, 487–493. doi:10.1016/j.mad.2010.04.007
Xu, K., Tavernarakis, N., and Driscoll, M. (2001). Necrotic cell death in C. elegans requires the function of calreticuli n and regulators of Ca(2+) release from the endoplasmic reticulum. Neuron 31, 957–971. doi:10.1016/s0896-6273(01)00432-9
Yajnik, C. S., Deshpande, S. S., Jackson, A. A., Refsum, H., Rao, S., Fisher, D. J., et al. (2008). Vitamin B12 and folate concentrations during pregnancy and insulin resistance in the offspring: The pune maternal nutrition study. Diabetologia 51, 29–38. doi:10.1007/s00125-007-0793-y
Yang, S. W., Katherine, J. G., MichaelPatel, M., Milesh, S., Mikhail, S., and Stockwell, B. R. (2016). Peroxidation of polyunsaturated fatty acids by lipoxygenases drives ferroptosis.
Yang, W. S., SriRamaratnam, R., Welsch, M. E., Shimada, K., Skouta, R., Viswanathan, V. S., et al. (2014). Regulation of ferroptotic cancer cell death by GPX4. Cell. 156, 317–331. doi:10.1016/j.cell.2013.12.010
Yang, W. S., and Stockwell, B. R. (2008). Synthetic lethal screening identifies compounds activating iron-dependent, nonapoptotic cell death in oncogenic-RAS-harboring cancer cells. Chem. Biol. 15, 234–245. doi:10.1016/j.chembiol.2008.02.010
Yilmaz, L. S., and Walhout, A. J. (2014). Worms, bacteria, and micronutrients: An elegant model of our diet. Trends Genet. 30, 496–503. doi:10.1016/j.tig.2014.07.010
Liu, Y. J., Janssens, G. E., Kamble, R., Gao, A. W., Jongejan, A., Weeghel, M. V., et al. (2018). Mitochondrial translation and dynamics synergistically extend lifespan in C. elegans through HLH-30. J Cell Biol. 219, e201907067. doi:10.1083/jcb.201907067
Zhang, G., Wang, Z., Du, Z., and Zhang, H. (2018). mTOR regulates phase separation of PGL granules to modulate their autophagic degradation. Cell. 174, 1492–1506. doi:10.1016/j.cell.2018.08.006
Zhang, Y., Koppula, P., and Gan, B. (2019). Regulation of H2A ubiquitination and SLC7A11 expression by BAP1 and PRC1. Cell. Cycle 18, 773–783. doi:10.1080/15384101.2019.1597506
Zhang, Y., Shi, J., Liu, X., Feng, L., Gong, Z., Koppula, P., et al. (2018). BAP1 links metabolic regulation of ferroptosis to tumour suppression. Nat. Cell. Biol. 20, 1181–1192. doi:10.1038/s41556-018-0178-0
Zhang, Y., Swanda, R. V., Nie, L., Liu, X., Wang, C., Lee, H., et al. (2021). mTORC1 couples cyst(e)ine availability with GPX4 protein synthesis and ferroptosis regulation. Nat. Commun. 12, 1589. doi:10.1038/s41467-021-21841-w
Zheng, H., Yuan, C., Zhang, H., Chen, Y., and Zhang, H. (2020). The tissue- and developmental stage-specific involvement of autophagy genes in aggrephagy. Autophagy 16, 589–599. doi:10.1080/15548627.2019.1632121
Keywords: cell death, nutrition, metabolism disorder, signal pathway, Caenorhabditis elegans
Citation: Wang Y, Wu W and Gong J (2023) Live or death in cells: from micronutrition metabolism to cell fate. Front. Cell Dev. Biol. 11:1185989. doi: 10.3389/fcell.2023.1185989
Received: 14 March 2023; Accepted: 28 April 2023;
Published: 12 May 2023.
Edited by:
Yilei Zhang, Xi’an Jiaotong University, ChinaCopyright © 2023 Wang, Wu and Gong. This is an open-access article distributed under the terms of the Creative Commons Attribution License (CC BY). The use, distribution or reproduction in other forums is permitted, provided the original author(s) and the copyright owner(s) are credited and that the original publication in this journal is cited, in accordance with accepted academic practice. No use, distribution or reproduction is permitted which does not comply with these terms.
*Correspondence: Wei Wu, MTE5ODA0MkB6anUuZWR1LmNu; Jianke Gong, amlhbmtlZ0BodXN0LmVkdS5jbg==
†ORCID: Wei Wu, orcid.org/0000-0003-4657-4088