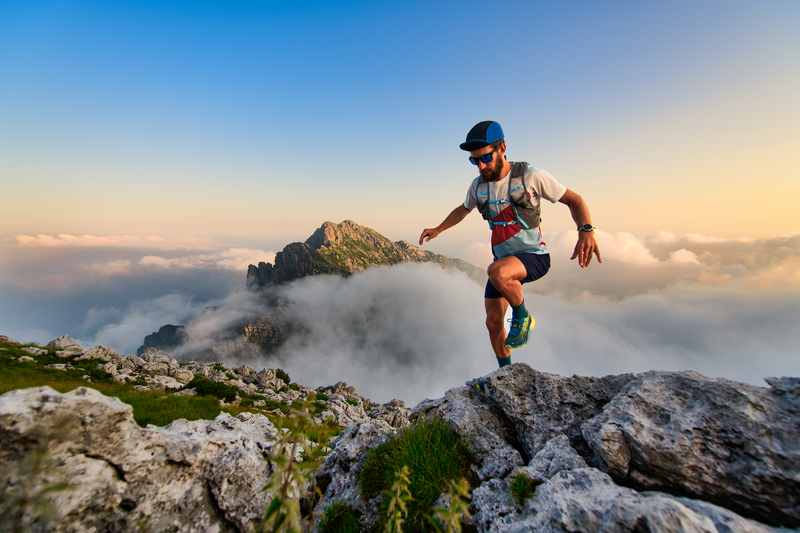
95% of researchers rate our articles as excellent or good
Learn more about the work of our research integrity team to safeguard the quality of each article we publish.
Find out more
MINI REVIEW article
Front. Cell Dev. Biol. , 21 March 2023
Sec. Cell Adhesion and Migration
Volume 11 - 2023 | https://doi.org/10.3389/fcell.2023.1171930
This article is part of the Research Topic Evolution, Emerging Functions and Structure of Actin-Binding Proteins - Volume II View all 11 articles
The actin cytoskeleton represents a highly dynamic filament system providing cell structure and mechanical forces to drive a variety of cellular processes. The dynamics of the actin cytoskeleton are controlled by a number of conserved proteins that maintain the pool of actin monomers, promote actin nucleation, restrict the length of actin filaments and cross-link filaments into networks or bundles. Previous work has been established that cytoplasmic calcium is an important signal to rapidly relay information to the actin cytoskeleton, but the underlying mechanisms remain poorly understood. Here, we summarize new recent perspectives on how calcium fluxes are transduced to the actin cytoskeleton in a physiological context. In this mini-review we will focus on three calcium-binding EF-hand-containing actin cross-linking proteins, α-actinin, plastin and EFHD2/Swiprosin-1, and how these conserved proteins affect the cell’s actin reorganization in the context of cell migration and wound closure in response to calcium.
Filamentous actin (F-actin) is a dynamic polymer providing mechanical forces to change cell morphology. The dynamic rearrangements and turnover of F-actin are pivotal corner stones for cellular functions such as intracellular transport, cytokinesis and cell migration. F-Actin polymerization and depolymerization are controlled by different families of actin binding proteins (ABPs). Profilin and sequestering thymosin-β4 compete for binding of actin monomers thereby modulating the concentration of free actin monomers available for polymerization (Pollard, 2016). Nucleation promoting factors (NPF) such as the WASp/SCAR family proteins at the cell membrane are activated through small Rho GTPases and can, in turn, activate the actin-related protein (Arp)-2/3-complex (Blanchoin et al., 2000). Growth of a filament is terminated by capping proteins and eventually filaments are disassembled through severing and debranching by ADF/cofilin. Severing exposes filament barbed ends that can continue growing and the dissociated actin monomers can enter a new actin polymerization cycle (Pollard and Borisy, 2003). Furthermore, actin filaments are incorporated into different μm-scale higher order structures: from loosely cross-linked isotropic networks to tight and highly organized bundles (Svitkina, 2020). While F-actin bundles are found in the cell cortex, in thin finger-like cell protrusions such as filopodia and are also prominent in contractile actomyosin-rings during cytokinesis and wound closure, cross-linked actin networks are important for the broad lamellipodium of a migrating cell.
More than 23 different classes of ABPs have been shown to crosslink or bundle F-actin (Schliwa, 1994; Tseng et al., 2005). Several studies suggest that actin crosslinking proteins have redundant roles (Rivero et al., 1999; Lieleg et al., 2010), which allows for finely adjusting the mechanical and viscoelastic F-actin network properties through cooperation and competition. Some proteins frequently also exhibit a dual activity by either cross-linking filaments into loose networks or laterally into tight bundles of filaments in vitro, often depending on protein concentration and solution crowding (Park et al., 2021). Interestingly, some ABPs are regulated by calcium ions (Ca2+) which allows to coordinate rapid actin remodeling driving morphological changes in response to diverse environmental stimuli (Chun and Santella, 2009; Izadi et al., 2018; Bootman and Bultynck, 2020). Prominent calcium-regulated ABPs are EF-hand containing proteins such as α-actinin (Burridge and Feramisco, 1981; Noegel et al., 1987) and fimbrins/plastins (Lin et al., 1988; de Arruda et al., 1990; Lin et al., 1993b) and EFHD/Swiprosin (Dutting et al., 2011; Mielenz and Gunn-Moore, 2016). Thus, the study of Ca2+ binding proteins in the context of actin cytoskeleton reorganization is important for our understanding of fundamental processes such as cell shape changes in cell migration and epithelial wound closure. Advances in biochemical structural analysis and in vivo live-cell microscopy have revealed new perspectives, how Ca2+ can regulate dynamic actin cytoskeletal changes by acting on ABPs. This review aims to outline recent findings on Ca2+ regulation of the most prominent actin cross-linking proteins α-actinin, plastin and EFHD2/Swiprosin-1.
For the rapid relay of a Ca2+ stimulus, Ca2+-regulated proteins can either bind to Ca2+/calmodulin (CaM) or bind Ca2+ directly through calmodulin-like domains (CaMD) to induce specific Ca2+-dependent processes (Nakayama and Kretsinger, 1994; Chin and Means, 2000). Calmodulin is a highly conserved Ca2+ sensor protein that contains the common Ca2+ binding motif composed of paired helix-loop-helix EF-hands. Binding of Ca2+ to the EF-hand domains leads to a conformational change of the target protein (Tufty and Kretsinger, 1975). EF-hand containing proteins with CaMDs comprise one of the largest protein family with a wide range of biological functions that bind Ca2+ with different affinities ranging from 10–6 M to 10–3 M (Bagur and Hajnoczky, 2017; Kawasaki and Kretsinger, 2017). Over twenty subfamilies have been defined by both evolutionary history and functions (Kawasaki and Kretsinger, 2017). Among them, three prominent EF-hand subfamily groups contain Ca2+-regulated ABPs including the Fimbrin/plastin group (FIMB), the α-actinin group (ACTN) and the EFHD2/Swiprosin group (EFHD2_DM) (Kawasaki and Kretsinger, 2017).
Moreover, Ca2+ can act indirectly on ABPs through activation of Ca2+-dependent kinases and small Rho GTPases. Therefore, maintaining cellular Ca2+ homeostasis is crucial for cytoskeletal dynamics. For the effectiveness of Ca2+ flux into the cytoplasm, an approximately 20.000-fold gradient between intracellular and extracellular Ca2+ concentration must be maintained (reviewed in Clapham, 2007). Special pumps like the plasma membrane Ca2+ ATPases (PMCA) or the sarcoendoplasmic reticular Ca2+ ATPases (SERCA) constantly remove Ca2+ under energy expenditure from the cytoplasm to the extracellular space or the endoplasmic reticulum (ER) which acts as an intracellular Ca2+ storage (Clapham, 2007). Ca2+ from the ER can also be transferred to mitochondria, dynamic organelles that have been shown to be important in locally releasing Ca2+ pulses to induce cytoskeletal rearrangements during migration and wound healing (reviewed in Paupe and Prudent, 2018). Moreover, influx of extracellular Ca2+ through Ca2+-permeable ion channels at the plasma membrane can be regulated by the actin cytoskeleton itself (Qian and Xiang, 2019).
In migrating cells there is a tightly controlled gradient of Ca2+ concentration from low at the leading edge to high at the cell rear. The low background level at the front of the cell permits even small changes in Ca2+ concentration to relay signals efficiently allowing, for example, small Ca2+ pulses to regulate cycles of local lamellipodia retraction and adhesion (Brundage et al., 1991; Wei et al., 2009; Tsai and Meyer, 2012; Wei et al., 2012; Tsai et al., 2015). Furthermore, tissue damage in a continuous epithelial sheet will cause an efflux of Ca2+ ions from the damaged cells into the extracellular environment which are then rapidly transported by mechanosensitive ion channels into the cells surrounding the wound site (Nakamura et al., 2018). This signal is then propagated within the epithelial tissue by gap junctions between neighboring cells (Razzell et al., 2013). The immediate increase of intracellular Ca2+ concentration leads to the massive cytoskeletal remodeling important for effective wound healing such as contractile ring and membrane protrusion formation at the wound margin (Antunes et al., 2013). But the underlying molecular mechanisms how Ca2+ signals result in local changes in cell shape and cell behavior are still poorly understood.
Calcium is a prominent regulatory cell signal which has also multiple effects on the structure and dynamics of the actin cytoskeleton (Chun and Santella, 2009; Izadi et al., 2018; Bootman and Bultynck, 2020). For the mechanical integrity of the actin cytoskeleton, overlapping actin filaments need to be attached to one another to rigidify and generate a stable F-actin network (Ydenberg et al., 2011; Svitkina, 2018; Svitkina, 2020). In the following we will discuss three highly evolutionarily conserved ABPs with Ca2+-regulated actin cross-linking activity (Figure 1).
FIGURE 1. α-Actinin, Plastin and EFHD2/Swiprosin-1—three conserved EF- hand containing actin cross-linking proteins regulated by Ca2+. Schematic diagram of the protein domains of α-Actinin, Plastin and EFHD2/Swiprosin-1 and structural and functional changes in response to calcium. Domains as indicated: ABD (actin bindind domain) consists of two CH (calponin homology) domains; EF-hands (helix-loop-helix motif) that bind Ca2+ (green spheres); R (spectrin-like repeats) facilitate dimerization of α-Actinin; LM (ligand mimic domain); CC (coiled-coil domain) facilitates dimerization of EFHD2/Swiprosin-1; H5 (fith helix).
α-Actinin belongs to the spectrin protein superfamily that dimerizes through four spectrin-like repeats (SLR) in their rod-shaped center (Figure 1). The central rod is connected on one side via a flexible neck region to the N-terminal actin binding domain (ABD), which is composed of two adjacent calponin homology domains (CH), and at the other side to a C-terminal CaMD, which is composed of two pairs of EF-hands (EF1/2 and EF3/4; see Murphy and Young, 2015). Antiparallel dimerization positions the terminal ABD and CaMD of opposing monomers at either end and in close proximity to each other allowing Ca2+-mediated regulation of the ABD (Djinović-Carugo et al., 1999; Sjöblom et al., 2008). The Ca2+ regulation has recently been shown to merely involve one single Ca2+ ion bound to the first EF-loop (Figure 1; Drmota Prebil et al., 2016; Backman, 2015). This domain is also affected by alternative splicing of the α-actinin gene which results in four different variants: Ca2+-insensitive muscle α-actinins and Ca2+-sensitive non-muscle α-actinins (Burridge and Feramisco, 1981; Sjöblom et al., 2008). In muscles, α-actinin is the predominant ABP cross-linking thin filaments from adjacent sarcomeres (Foley and Young, 2014). Here, Ca2+-independent cross-links are important for maintaining proper sarcomere structure during Ca2+-triggered muscle contraction. Instead, muscle α-actinins are regulated by phosphoinositides (Fukami et al., 1992). In non-muscle tissues, α-actinins are mainly involved in anchoring F-actin to cell-cell and cell-matrix adhesions (Foley and Young, 2014). In humans there are four α-actinin isoforms (1–4) with isoforms 1 and 4 widely expressed in non-muscle tissues (Murphy and Young, 2015). Mutations in the EF-hands of α-actinin-1 have been linked to congenital macrothrombocytopenia (CMTP), a rare blood disorder characterized by a reduced platelet count and increased platelet size due to aberrant actin cytoskeletal organization (Murphy and Young, 2015).
Human non-muscle α-actinin-1 mainly localizes to stress fibers and focal adhesion sites. Its actin cross-linking activity is inhibited by cytoplasmic Ca2+ concentrations above 0.1 mM (Burridge and Feramisco, 1981). Recently it has been shown that upon Ca2+ binding to the first of four EF-loops (EF1) in the CaMD, the local domain flexibility is altered leading to a more stabilized structure (Figure 1). It has been suggested that this modifies the CaMD interaction with the neck domain and therefore changing the ABD orientation in the dimer to effectively cross-link F-actin while actin binding is unchanged (Drmota Prebil et al., 2016). Similarly, Ca2+ has been described as allosteric regulator of F-actin bundling activity by modulating ABD orientational flexibility in Entamoeba histolytica α-actinin-2 (EhActn2) (Pinotsis et al., 2020). EhActn2 is similar to ancestral α-actinin containing only two SLR in the rod domain. Binding of Ca2+ was shown to support the interaction of the neck domain with EF-loops 3 and 4 thereby reducing the flexibility of a hinge region at the neck domain that allows ABD rotation around the rod axis. Interestingly, increasing the flexibility of the ABD also resulted in loss of actin bundling activity. Hence, Ca2+ binding to EhActn2 has been proposed to lead to an interdomain cross-talk increasing overall protein rigidity and therefore decreasing the conformational flexibility of the ABD which inhibits F-actin bundling (Pinotsis et al., 2020).
Plastins, also known as fimbrins, harbor two ABDs (Figure 1; ABD1 and 2) of tandem CH domains to effectively stabilize parallel F-actin bundles with a distance between adjacent filaments of approximately 120Å (Volkmann et al., 2001). There are three conserved plastin isoforms, namely I-, L-, and T-plastin (also called plastin 1-3, respectively) (Lin et al., 1993a), each encoded by a distinct gene and expressed in a tissue-specific manner. I-plastin is found in microvilli of intestinal and kidney epithelia (Lin et al., 1994) and in stereocilia of cochlear hair cells, where also T-plastin is expressed, though temporally restricted to stereocilia maturation (Daudet and Lebart, 2002). T-plastin is the most ubiquitously expressed isoform found in cells from solid tissues such as fibroblasts, endo- and epithelial cells but also neuronal cell such as microglia (Arpin et al., 1994; Shinomiya, 2012). L-plastin is expressed in hematopoetic cells serving fundamental functions in immunity including migration and adhesion (Morley, 2012). Further highlighting their importance for cell migration, L- and T-plastin are often associated with the invasiveness of metastatic cancer cells (reviewed in Lin et al., 1993b; Delanote et al., 2005).
The bundling activity of plastins is negatively regulated by Ca2+ binding. Plastins have two N-terminal EF-hands arranged in a so-called headpiece domain that is connected to ABD1 by a short 40 aa linker. Within this linker region lies a conserved fifth helix (H5) reminiscent of a calmodulin binding motif (Figure 1). This helix specifically interacts with the helices of the Ca2+-bound EF-hands. It has been suggested that in the tertiary protein structure of L-plastin, H5 acts as a wedge possibly between CH1 and CH2 of ABD1 changing their relative position and stabilizing an orientation that is favorable to actin binding (Ishida et al., 2017). The authors proposed that binding of Ca2+ to the EF-hands leads to sequestration of H5 from ABD1, destabilizing the orientation and thus decreasing actin bundling (Ishida et al., 2017). Another study looked at Ca2+ binding and its effect on actin binding in all three human plastin isoforms. They, however, identified ABD1 of L-plastin to bind F-actin Ca2+-independently while ABD2 is regulated by Ca2+ binding to the EF-hands (Schwebach et al., 2017). Therefore, the former hypothesis could still be applicable to CH3 and CH4 of ABD2. Another recent finding concerns the localization of T-plastin in osteoblast, osteocyte and fibroblast cells depending on Ca2+ binding. Wildtypic T-plastin can cycle between the lamellipodium and focal adhesions while Ca2+-hyposensitive T-plastin mutants localized exclusively to focal adhesions and Ca2+-hypersensitive mutants only in the lamellipodium. Chelation of Ca2+ led the latter to localize to focal adhesions as well, identifying Ca2+ binding as the regulator for T-plastin cycling between the leading edge and adhesion complexes. The authors proposed that in the presence of Ca2+, CH3 and CH4 of ABD2 are locked in an inhibited state by the headpiece domain therefore only ABD1 binds to F-actin. Binding of Ca2+ leads to detachment of the H5 from the EF-hands therefore releasing ABD2 from its structural constraints (Schwebach et al., 2020). However, the crystal structures of Ca2+-bound and -unbound human T-plastin has not been solved yet to reveal the exact inhibitory mechanism proposed for H5.
EFHD2/Swiprosin-1 (Swip-1) is also an EF-hand containing ABP that has recently been identified to create orthogonal F-actin cross-links aiding the rapid cytoskeletal rearrangements necessary for lamellipodia formation (Lehne et al., 2022). Physiologically, Swip-1 has been associated with a plethora of pathologies such as neurodegenerative diseases, acute and chronic inflammation and cancer especially invasive stages of malignant melanoma (Dütting et al., 2011; Mielenz and Gunn-Moore, 2016; Thylur et al., 2018). It has also been reported that Swip-1 is involved in BCR-induced Ca2+ flux controlling BCR signaling to activate B cells (Kroczek et al., 2010; Hagen et al., 2012) and in Drosophila embryos it was hypothesized that it regulates Ca2+-dependent exocytosis of electron dense vesicles during myoblast fusion (Hornbruch-Freitag et al., 2011).
Swip-1 contains a disordered region at the N-terminus that varies across species, two functional EF-hand domains, a connecting short α-helix called ligand mimic (LM) helix and a coiled-coil (CC) domain at its C-terminus allowing self-dimerization (Avramidou et al., 2007; Vega et al., 2008; Hagen et al., 2012; Ferrer-Acosta et al., 2013). After gene duplication in Euteleostomi, the efhd2 gene was duplicated giving rise to the efhd1 gene encoding EFHD1/Swiprosin-2 (Swip-2) (Dutting et al., 2011). While Swip-2 also binds Ca2+, it localizes in the inner mitochondria membrane and will therefore not be further discussed here. Although it is known that the cytoplasmically expressed Swip-1 binds F-actin (Huh et al., 2013; Kwon et al., 2013; Huh et al., 2015; Tu et al., 2018), the localization and number of ABDs has not been fully addressed, yet. Most probable, a binding site is located within the first EF-hand domain because its deletion has been shown to sufficiently dimmish F-actin binding (Moreno-Layseca et al., 2021). Swip-1’s role in cell motility and lamellipodia formation has been established (Huh et al., 2013; Kwon et al., 2013), but the mechanism how it regulates the underlying necessary F-actin rearrangements in response to Ca2+ has only recently been elucidated (Lehne et al., 2022). In vivo studies in Drosophila showed that Swip-1 is enriched in the forming lamellipodium of both migrating immune cells and at epidermal wound edges where it drives the initial phase of wound healing to re-establish tissue integrity (Lehne et al., 2022). In the absence of Ca2+, Swip-1-mediated cross-links stabilizes the F-actin network while Ca2+ binding to Swip-1 results in transient cross-links. Therefore, upon Ca2+ binding to Swip-1, the actin meshwork becomes relaxed making the filaments accessible for severing and branching (Figure 2). A model has been proposed in which increased Ca2+ concentrations reduce Swip-1 cross-linking activity to promote rapid reorganization of existing actin networks, to drive fast and efficient epithelial wound closure by extension of newly formed lamellipodia (Figure 2). Defective constriction of the wound margin in mutant epidermal cells further suggests that Swip-1 function might also be involved in the subsequent formation of the contractile supracellular acto-myosin ring forming along the wound margin (Figure 2; Lehne et al., 2022). Thus, Swip-1 could act as a Ca2+ sensor protein responding to the transient local changes of Ca2+ concentration at the leading edge of migrating cells and in wounded epidermis (Lehne et al., 2022).
FIGURE 2. Swip-1 cross-linked lamellipodial actin networks drive single cell migration and epithelial wound closure. Upper panel: Schematic of the in vivo model to study calcium-dependent wound healing in the abdominal epidermis in Drosophila. Laser-induced single-cell ablation starts at t = 0 min. In the first two minutes (t = 2 min) F-actin assembled into broad lamellipodial protrusions within cells at the wound edge; lamellipodial protrusions reached a maximum size between 5–10 min after wounding. Later on (t = 15 min) lamellipodia formation decreased and a supracellular acto-myosin ring is formed at the leading edge of the wound, contracted laterally to pull cells forward and increasingly contributes to wound closure. Lower panel: Schematic showing the proposed role of Swip-1 in regulating lamellipodial actin networks and supracellular acto-myosin rings upon laser-induced single-cell wounding within the epidermis (see also text; adapted from Lehne et al., 2022). Left: The actin crosslinking activity of Swip-1 (blue spheres) synergizes with WRC-Arp2/3-branched actin nucleation (red stars) promoting generation of a stable and densely branched actin filament network. Plasma membrane damage or mechanical activation of calcium channels (green) allows a rapid reorganization of the actin cytoskeleton through Swip-1. Right: After initial calcium wave propagation, the levels of intracellular calcium decreased mediated by special pumps (green) like the sarcoendoplasmic reticular calcium ATPases (SERCA) which constantly remove calcium from the cytoplasm to the ER lumen. A supracellular actin-myosin ring is formed stabilized by stable actin cross-links induced by Swip-1.
Human Swip-1 also bundles efficiently actin filaments, however this activity is calcium-insensitive (Lehne et al., 2022). Of note here, Ca2+ concentrations of up to 1 mM did not completely abolish Swip-1-mediated cross-links but only shifted them from stable to transient. Structurally, binding of Ca2+ at the two EF-hands does not alter the overall secondary structure nor the actin-binding properties (Ferrer-Acosta et al., 2013). However, it is believed that upon depletion of Ca2+ the local conformation at the EF-hand domains and LM helix becomes more flexible (Park et al., 2016). Because recent findings have been contradictory in regards to positive or negative regulation of Swip-1 by Ca2+, the underlying structural changes and how it influences Swip-1 cross-linking activity are of great interest. Remarkably, a recent study also identified a calcium-independent role of Swip-1 in regulated exocytosis where it contributes to the recruitment of Rho-GTPase regulating actomyosin activity to drive proper vesicle membrane crumpling and expulsion of cargo (Lehne and Bogdan, 2023).
Ca2+ is an important second messenger, whose change in concentration induces and controls spatiotemporally pathways of many physiological processes such as proliferation or cell death and many processes dependent on cell migration such as development, immune response or cancer metastases (Clapham, 2007; Evans and Falke, 2007; Tsai et al., 2015). The main pillars of cellular migration are protrusions, forming new adhesion sites allowing traction force generation and lastly release of the cell rear by dissolving mature adhesions (Lauffenburger and Horwitz, 1996). These processes rely on dynamic cellular changes where Ca2+-dependent actin cross-linkers can respond to the fast-acting second messenger. Low cytoplasmic Ca2+ concentration at the protruding edge would stabilize F-actin cross-links that can be rearranged by local Ca2+ pulses while high Ca2+ at the trailing edge destabilizes adhesion complexes (Drmota Prebil et al., 2016). It is therefore not surprising that aberrant Ca2+ signaling as well as the described cross-linkers, α-actinin, plastins and Swip-1, all have been implicated in migration-linked pathologies such as invasive cancers (Shinomiya, 2012; Huh et al., 2015; Tsai et al., 2015). A common theme seems to be that on a structural level, binding of Ca2+ influences protein domain flexibility through interdomain cross-talk. Further investigations like comparing Ca2+-bound and -unbound states can help elucidate the regulatory mechanisms of structural changes and possibly uncover new strategies for cancer treatment.
Not to be dismissed, actin cross-linkers inherently bind actin so other regulating mechanisms independently of their bundling activity are conceivable such as regulating actin turnover. For instance, Swip-1 has been implicated to regulate small Rho GTPases (Huh et al., 2015; Lehne and Bogdan, 2023) and has been shown to regulate actin depolymerization by recruiting cofilin (Huh et al., 2013). On the other hand, T-plastin has been suggested to control actin turnover by displacing cofilin therefore decreasing the actin depolymerization rate and possibly recruiting other ABPs such as α-actinin (Giganti et al., 2005). This also shows that functional cross-linker synergy poses interesting new ways of deciphering dynamic actin cytoskeleton regulation. In line with this, for future investigations and interpretation of obtained results one should also consider that the concentration of a cross-linking protein is crucial for its function (Lieleg et al., 2010). For example, α-actinin has been shown to cross-link actin at low but bundle it at high concentrations (Tseng et al., 2002; Lieleg et al., 2010). Thus, in vitro experiments are crucial to dissect how nanoscale structural changes of a cross-linking protein can alter the mesoscale geometric and mechanical properties of the actin cytoskeleton network. On the other hand, the limitations of in vitro studies should be emphasized. ABPs often interact with multiple proteins that integrate signaling pathways between the plasma membrane and the actin cytoskeleton in a living cell. Thus, structure-function in vivo studies using genetic model systems such as Drosophila will further improve our understanding of how the cross-linkers function in a physiological context.
FL and SB made the figures and wrote the manuscript.
The authors declare that the research was conducted in the absence of any commercial or financial relationships that could be construed as a potential conflict of interest.
All claims expressed in this article are solely those of the authors and do not necessarily represent those of their affiliated organizations, or those of the publisher, the editors and the reviewers. Any product that may be evaluated in this article, or claim that may be made by its manufacturer, is not guaranteed or endorsed by the publisher.
ABD, actin binding domain; ABP, actin binding protein; Ca2+, calcium; CaMD, calmodulin-like domain; CH, calponin homology domains; F-actin, filamentous actin; SLR, spectrin-like repeats; Swip-1; EFHD2/Swiprosin-1.
Antunes, M., Pereira, T., Cordeiro, J. V., Almeida, L., and Jacinto, A. (2013). Coordinated waves of actomyosin flow and apical cell constriction immediately after wounding. J. Cell Biol. 202, 365–379. doi:10.1083/jcb.201211039
Arpin, M., Friederich, E., Algrain, M., Vernel, F., and Louvard, D. (1994). Functional differences between L- and T-plastin isoforms. J. Cell Biol. 127, 1995–2008. doi:10.1083/jcb.127.6.1995
Avramidou, A., Kroczek, C., Lang, C., Schuh, W., Jäck, H. M., and Mielenz, D. (2007). The novel adaptor protein Swiprosin-1 enhances BCR signals and contributes to BCR-induced apoptosis. Cell Death Differ. 14, 1936–1947. doi:10.1038/sj.cdd.4402206
Bagur, R., and Hajnoczky, G. (2017). Intracellular Ca(2+) sensing: Its role in calcium homeostasis and signaling. Mol. Cell 66, 780–788. doi:10.1016/j.molcel.2017.05.028
Blanchoin, L., Amann, K. J., Higgs, H. N., Marchand, J.-b., Kaiser, D. A., and Pollard, T. D. (2000). Direct observation of dendritic actin filament networks nucleated by Arp2/3 complex and WASP/Scar proteins. Nature 404, 1007–1011. doi:10.1038/35010008
Bootman, M. D., and Bultynck, G. (2020). Fundamentals of cellular calcium signaling: A primer. Cold Spring Harb. Perspect. Biol. 12, a038802. doi:10.1101/cshperspect.a038802
Brundage, R. A., Fogarty, K. E., Tuft, R. A., and Fay, F. S. (1991). Calcium gradients underlying polarization and chemotaxis of eosinophils. Science 254, 703–706. doi:10.1126/science.1948048
Burridge, K., and Feramisco, J. R. (1981). Non-muscle alpha actinins are calcium-sensitive actin-binding proteins. Nature 294, 565–567. doi:10.1038/294565a0
Chin, D., and Means, A. R. (2000). Calmodulin: A prototypical calcium sensor. Trends Cell Biol. 10, 322–328. doi:10.1016/s0962-8924(00)01800-6
Chun, J. T., and Santella, L. (2009). Roles of the actin-binding proteins in intracellular Ca2+ signalling. Acta Physiol. (Oxf) 195, 61–70. doi:10.1111/j.1748-1716.2008.01921.x
Daudet, N., and Lebart, M. C. (2002). Transient expression of the T-isoform of plastins/fimbrin in the stereocilia of developing auditory hair cells. Cell Motil. Cytoskelet. 53, 326–336. doi:10.1002/cm.10092
de Arruda, M. V., Watson, S., Lin, C. S., Leavitt, J., and Matsudaira, P. (1990). Fimbrin is a homologue of the cytoplasmic phosphoprotein plastin and has domains homologous with calmodulin and actin gelation proteins. J. Cell Biol. 111, 1069–1079. doi:10.1083/jcb.111.3.1069
Delanote, V., Vandekerckhove, J., and Gettemans, J. (2005). Plastins: Versatile modulators of actin organization in (patho)physiological cellular processes. Acta Pharmacol. Sin. 26, 769–779. doi:10.1111/j.1745-7254.2005.00145.x
Djinović-Carugo, K., Young, P., Gautel, M., and Saraste, M. (1999). Structure of the α-actinin rod: Molecular basis for cross-linking of actin filaments. Cell 98, 537–546. doi:10.1016/s0092-8674(00)81981-9
Drmota Prebil, S., Slapšak, U., Pavšič, M., Ilc, G., Puž, V., de Almeida Ribeiro, E., et al. (2016). Structure and calcium-binding studies of calmodulin-like domain of human non-muscle α-actinin-1. Sci. Rep. 6, 27383. doi:10.1038/srep27383
Dutting, S., Brachs, S., and Mielenz, D. (2011). Fraternal twins: Swiprosin-1/EFhd2 and Swiprosin-2/EFhd1, two homologous EF-hand containing calcium binding adaptor proteins with distinct functions. Cell Commun. Signal 9, 2. doi:10.1186/1478-811X-9-2
Evans, J. H., and Falke, J. J. (2007). Ca2+ influx is an essential component of the positive-feedback loop that maintains leading-edge structure and activity in macrophages. Proc. Natl. Acad. Sci. U. S. A. 104, 16176–16181. doi:10.1073/pnas.0707719104
Ferrer-Acosta, Y., Rodriguez Cruz, N. E., Vaquer, d. C., and Vega, E. I. (2013). Functional and structural analysis of the conserved EFhd2 protein. Protein & Peptide Lett. 20, 573–583. doi:10.2174/0929866511320050011
Foley, K. S., and Young, P. W. (2014). The non-muscle functions of actinins: An update. Biochem. J. 459, 1–13. doi:10.1042/BJ20131511
Fukami, K., Furuhashi, K., Inagaki, M., Endo, T., Hatano, S., and Takenawa, T. (1992). Requirement of phosphatidylinositol 4,5-bisphosphate for α-actinin function. Nature 359, 150–152. doi:10.1038/359150a0
Giganti, A., Plastino, J., Janji, B., Van Troys, M., Lentz, D., Ampe, C., et al. (2005). Actin-filament cross-linking protein T-plastin increases Arp2/3-mediated actin-based movement. J. Cell Sci. 118, 1255–1265. doi:10.1242/jcs.01698
Hagen, S., Brachs, S., Kroczek, C., Fürnrohr, B. G., Lang, C., and Mielenz, D. (2012). The B cell receptor-induced calcium flux involves a calcium mediated positive feedback loop. Cell Calcium 51, 411–417. doi:10.1016/j.ceca.2012.01.004
Hornbruch-Freitag, C., Griemert, B., Buttgereit, D., and Renkawitz-Pohl, R. (2011). Drosophila Swiprosin-1/EFHD2 accumulates at the prefusion complex stage during Drosophila myoblast fusion. J. Cell Sci. 124, 3266–3278. doi:10.1242/jcs.083907
Huh, Y. H., Kim, S. H., Chung, K.-H., Oh, S., Kwon, M.-S., Choi, H.-W., et al. (2013). Swiprosin-1 modulates actin dynamics by regulating the F-actin accessibility to cofilin. Cell. Mol. Life Sci. 70, 4841–4854. doi:10.1007/s00018-013-1447-5
Huh, Y. H., Oh, S., Yeo, Y. R., Chae, I. H., Kim, S. H., Lee, J. S., et al. (2015). Swiprosin-1 stimulates cancer invasion and metastasis by increasing the Rho family of GTPase signaling. Oncotarget 6, 13060–13071. doi:10.18632/oncotarget.3637
Ishida, H., Jensen, K. V., Woodman, A. G., Hyndman, M. E., and Vogel, H. J. (2017). The calcium-dependent switch helix of L-plastin regulates actin bundling. Sci. Rep. 7, 40662–40712. doi:10.1038/srep40662
Izadi, M., Hou, W., Qualmann, B., and Kessels, M. M. (2018). Direct effects of Ca(2+)/calmodulin on actin filament formation. Biochem. Biophys. Res. Commun. 506, 355–360. doi:10.1016/j.bbrc.2018.07.159
Kawasaki, H., and Kretsinger, R. H. (2017). Structural and functional diversity of EF-hand proteins: Evolutionary perspectives. Protein Sci. 26, 1898–1920. doi:10.1002/pro.3233
Kroczek, C., Lang, C., Brachs, S., Grohmann, M., Dütting, S., Schweizer, A., et al. (2010). Swiprosin-1/EFhd2 controls B cell receptor signaling through the assembly of the B cell receptor, Syk, and phospholipase C gamma2 in membrane rafts. J. Immunol. 184, 3665–3676. doi:10.4049/jimmunol.0903642
Kwon, M.-S., Park, K. R., Kim, Y.-D., Na, B.-R., Kim, H.-R., Choi, H.-J., et al. (2013). Swiprosin-1 is a novel actin bundling protein that regulates cell spreading and migration. PLoS ONE 8, e71626. doi:10.1371/journal.pone.0071626
Lauffenburger, D. A., and Horwitz, A. F. (1996). Cell migration: A physically integrated molecular process. Cell 84, 359–369. doi:10.1016/s0092-8674(00)81280-5
Lehne, F., and Bogdan, S. (2023). Swip-1 promotes exocytosis of glue granules in the exocrine Drosophila salivary gland. J. Cell Sci. 136, jcs260366. doi:10.1242/jcs.260366
Lehne, F., Pokrant, T., Parbin, S., Salinas, G., Großhans, J., Rust, K., et al. (2022). Calcium bursts allow rapid reorganization of EFhD2/Swip-1 cross-linked actin networks in epithelial wound closure. Nat. Commun. 13, 2492. doi:10.1038/s41467-022-30167-0
Lieleg, O., Claessens, M. M. A. E., and Bausch, A. R. (2010). Structure and dynamics of cross-linked actin networks. Soft Matter 6, 218–225. doi:10.1039/b912163n
Lin, C. S., Aebersold, R. H., Kent, S. B., Varma, M., and Leavitt, J. (1988). Molecular cloning and characterization of plastin, a human leukocyte protein expressed in transformed human fibroblasts. Mol. Cell Biol. 8, 4659–4668. doi:10.1128/mcb.8.11.4659
Lin, C. S., Park, T., Chen, Z. P., and Leavitt, J. (1993a). Human plastin genes. Comparative gene structure, chromosome location, and differential expression in normal and neoplastic cells. J. Biol. Chem. 268, 2781–2792. doi:10.1016/s0021-9258(18)53842-4
Lin, C. S., Park, T., Zong Ping, C., and Leavitt, J. (1993b). Human plastin genes. Comparative gene structure, chromosome location, and differential expression in normal and neoplastic cells. J. Biol. Chem. 268, 2781–2792. doi:10.1016/s0021-9258(18)53842-4
Lin, C. S., Shen, W., Chen, Z. P., Tu, Y. H., and Matsudaira, P. (1994). Identification of I-plastin, a human fimbrin isoform expressed in intestine and kidney. Mol. Cell. Biol. 14, 2457–2467. doi:10.1128/mcb.14.4.2457
Mielenz, D., and Gunn-Moore, F. (2016). Physiological and pathophysiological functions of Swiprosin-1/EFhd2 in the nervous system. Biochem. J. 473, 2429–2437. doi:10.1042/BCJ20160168
Moreno-Layseca, P., Jäntti, N. Z., Godbole, R., Sommer, C., Jacquemet, G., Al-Akhrass, H., et al. (2021). Cargo-specific recruitment in clathrin- and dynamin-independent endocytosis. Nat. Cell Biol. 23, 1073–1084. doi:10.1038/s41556-021-00767-x
Morley, S. C. (2012). The actin-bundling protein L-plastin: A critical regulator of immune cell function. Int. J. Cell Biol. 2012, 935173, doi:10.1155/2012/935173
Murphy, A. C., and Young, P. W. (2015). The actinin family of actin cross-linking proteins - a genetic perspective. Cell Biosci. 5, 49. doi:10.1186/s13578-015-0029-7
Nakamura, M., Dominguez, A. N. M., Decker, J. R., Hull, A. J., Verboon, J. M., and Parkhurst, S. M. (2018). Into the breach: How cells cope with wounds. Open Biol. 8, 180135. doi:10.1098/rsob.180135
Nakayama, S., and Kretsinger, R. H. (1994). Evolution of the EF-hand family of proteins. Annu. Rev. Biophysics Biomol. Struct. 23, 473–507. doi:10.1146/annurev.bb.23.060194.002353
Noegel, A., Witke, W., and Schleicher, M. (1987). Calcium-sensitive non-muscle alpha-actinin contains EF-hand structures and highly conserved regions. FEBS Lett. 221, 391–396. doi:10.1016/0014-5793(87)80962-6
Park, J., Lee, M., Lee, B., Castaneda, N., Tetard, L., and Kang, E. H. (2021). Crowding tunes the organization and mechanics of actin bundles formed by crosslinking proteins. FEBS Lett. 595, 26–40. doi:10.1002/1873-3468.13949
Park, K. R., Kwon, M.-S., An, J. Y., Lee, J.-G., Youn, H.-S., Lee, Y., et al. (2016). Structural implications of Ca2+-dependent actin-bundling function of human EFhd2/Swiprosin-1. Sci. Rep. 6, 39095. doi:10.1038/srep39095
Paupe, V., and Prudent, J. (2018). New insights into the role of mitochondrial calcium homeostasis in cell migration. Biochem. Biophysical Res. Commun. 500, 75–86. doi:10.1016/j.bbrc.2017.05.039
Pinotsis, N., Zielinska, K., Babuta, M., Arolas, J. L., Kostan, J., Khan, M. B., et al. (2020). Calcium modulates the domain flexibility and function of an α-actinin similar to the ancestral α-actinin. Proc. Natl. Acad. Sci. U. S. A. 117, 22101–22112. doi:10.1073/pnas.1917269117
Pollard, T. D. (2016). Actin and actin-binding proteins. Cold Spring Harb. Perspect. Biol. 8, 0182266–a18317. doi:10.1101/cshperspect.a018226
Pollard, T. D., and Borisy, G. G. (2003). Cellular motility driven by assembly and disassembly of actin filaments. Cell 112, 453–465. doi:10.1016/s0092-8674(03)00120-x
Qian, D., and Xiang, Y. (2019). Actin cytoskeleton as actor in upstream and downstream of calcium signaling in plant cells. Int. J. Mol. Sci. 20, 1403–1416. doi:10.3390/ijms20061403
Razzell, W., Evans, I. R., Martin, P., and Wood, W. (2013). Calcium flashes orchestrate the wound inflammatory response through DUOX activation and hydrogen peroxide release. Curr. Biol. 23, 424–429. doi:10.1016/j.cub.2013.01.058
Rivero, F., Furukawa, R., Fechheimer, M., and Noegel, A. A. (1999). Three actin cross-linking proteins, the 34 kDa actin-bundling protein, alpha-actinin and gelation factor (ABP-120), have both unique and redundant roles in the growth and development of Dictyostelium. J. Cell Sci. 112, 2737–2751. doi:10.1242/jcs.112.16.2737
Schwebach, C. L., Agrawal, R., Lindert, S., Kudryashova, E., and Kudryashov, D. S. (2017). The roles of actin-binding domains 1 and 2 in the calcium-dependent regulation of actin filament bundling by human plastins. J. Mol. Biol. 429, 2490–2508. doi:10.1016/j.jmb.2017.06.021
Schwebach, C. L., Kudryashova, E., Zheng, W., Orchard, M., Smith, H., Runyan, L. A., et al. (2020). Osteogenesis imperfecta mutations in plastin 3 lead to impaired calcium regulation of actin bundling. Bone Res. 8, 21. doi:10.1038/s41413-020-0095-2
Shinomiya, H. (2012). Plastin family of actin-bundling proteins: Its functions in leukocytes, neurons, intestines, and cancer. Int. J. Cell Biol. 2012, 213492, doi:10.1155/2012/213492
Sjöblom, B., Salmazo, A., and Djinović-Carugo, K. (2008). Alpha-actinin structure and regulation. Cell. Mol. Life Sci. 65, 2688–2701. doi:10.1007/s00018-008-8080-8
Svitkina, T. M. (2020). Actin cell cortex: Structure and molecular organization. Trends Cell Biol. 30, 556–565. doi:10.1016/j.tcb.2020.03.005
Svitkina, T. (2018). The actin cytoskeleton and actin-based motility. Cold Spring Harb. Perspect. Biol. 10, a018267. doi:10.1101/cshperspect.a018267
Thylur, R. P., Gowda, R., Mishra, S., and Jun, C.-D. (2018). Swiprosin-1: Its expression and diverse biological functions. J. Cell. Biochem. 119, 150–156. doi:10.1002/jcb.26199
Tsai, F.-C., Kuo, G.-H., Chang, S.-W., and Tsai, P.-J. (2015). Ca2+ signaling in cytoskeletal reorganization, cell migration, and cancer metastasis. BioMed Res. Int. 2015, 409245, doi:10.1155/2015/409245
Tsai, F. C., and Meyer, T. (2012). Ca2+ pulses control local cycles of lamellipodia retraction and adhesion along the front of migrating cells. Curr. Biol. 22, 837–842. doi:10.1016/j.cub.2012.03.037
Tseng, Y., Kole, T. P., Lee, J. S. H., Fedorov, E., Alino, S. C., Schafer, B. W., et al. (2005). How actin crosslinking and bundling proteins cooperate to generate an enhanced cell mechanical response. Biochem. Bioph Res. Co. 334, 183–192. doi:10.1016/j.bbrc.2005.05.205
Tseng, Y., Schafer, B. W., Almo, S. C., and Wirtz, D. (2002). Functional synergy of actin filament cross-linking proteins. J. Biol. Chem. 277, 25609–25616. doi:10.1074/jbc.M202609200
Tu, Y., Zhang, L., Tong, L., Wang, Y., Zhang, S., Wang, R., et al. (2018). EFhd2/swiprosin-1 regulates LPS-induced macrophage recruitment via enhancing actin polymerization and cell migration. Int. Immunopharmacol. 55, 263–271. doi:10.1016/j.intimp.2017.12.030
Tufty, R. M., and Kretsinger, R. H. (1975). Troponin and parvalbumin calcium binding regions predicted in myosin light chain and T4 lysozyme. Science 187, 167–169. doi:10.1126/science.1111094
Vega, I. E., Traverso, E. E., Ferrer-Acosta, Y., Matos, E., Colon, M., Gonzalez, J., et al. (2008). A novel calcium-binding protein is associated with tau proteins in tauopathy. J. Neurochem. 106, 96–106. doi:10.1111/j.1471-4159.2008.05339.x
Volkmann, N., Derosier, D., Matsudaira, P., and Hanein, D. (2001). An atomic model of actin filaments cross-linked by fimbrin and its implications for bundle assembly and function. J. Cell Biol. 153, 947–956. doi:10.1083/jcb.153.5.947
Wei, C., Wang, X., Chen, M., Ouyang, K., Song, L. S., and Cheng, H. (2009). Calcium flickers steer cell migration. Nature 457, 901–905. doi:10.1038/nature07577
Wei, C., Wang, X., Zheng, M., and Cheng, H. (2012). Calcium gradients underlying cell migration. Curr. Opin. Cell Biol. 24, 254–261. doi:10.1016/j.ceb.2011.12.002
Keywords: actin-binding protein (ABP), crosslinker, calcium, EF-hand, α-actinin, plastin/fimbrin, EFhd2/Swiprosin-1, wound healing
Citation: Lehne F and Bogdan S (2023) Getting cells into shape by calcium-dependent actin cross-linking proteins. Front. Cell Dev. Biol. 11:1171930. doi: 10.3389/fcell.2023.1171930
Received: 22 February 2023; Accepted: 09 March 2023;
Published: 21 March 2023.
Edited by:
Kai Murk, Charité University Medicine Berlin, GermanyReviewed by:
Klaus-Dieter Schlüter, University of Giessen, GermanyCopyright © 2023 Lehne and Bogdan. This is an open-access article distributed under the terms of the Creative Commons Attribution License (CC BY). The use, distribution or reproduction in other forums is permitted, provided the original author(s) and the copyright owner(s) are credited and that the original publication in this journal is cited, in accordance with accepted academic practice. No use, distribution or reproduction is permitted which does not comply with these terms.
*Correspondence: Sven Bogdan, c3Zlbi5ib2dkYW5Ac3RhZmYudW5pLW1hcmJ1cmcuZGU=
Disclaimer: All claims expressed in this article are solely those of the authors and do not necessarily represent those of their affiliated organizations, or those of the publisher, the editors and the reviewers. Any product that may be evaluated in this article or claim that may be made by its manufacturer is not guaranteed or endorsed by the publisher.
Research integrity at Frontiers
Learn more about the work of our research integrity team to safeguard the quality of each article we publish.