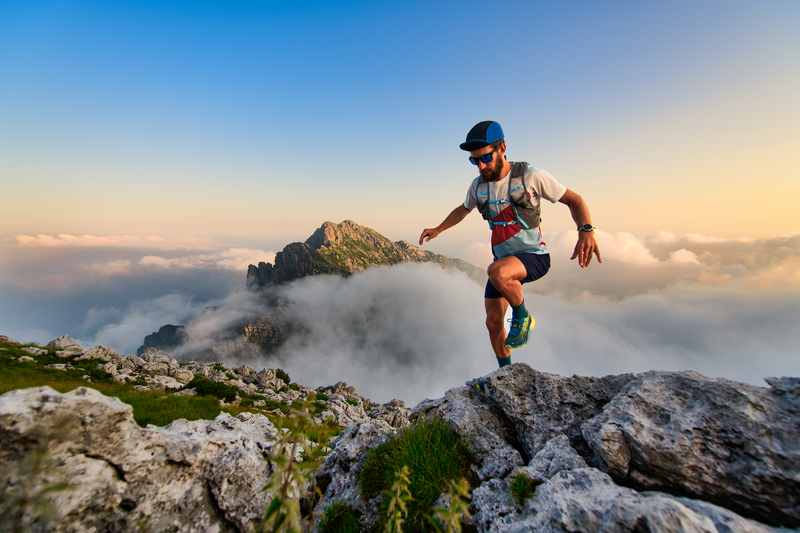
95% of researchers rate our articles as excellent or good
Learn more about the work of our research integrity team to safeguard the quality of each article we publish.
Find out more
ORIGINAL RESEARCH article
Front. Cell Dev. Biol. , 17 July 2023
Sec. Developmental Epigenetics
Volume 11 - 2023 | https://doi.org/10.3389/fcell.2023.1168643
This article is part of the Research Topic Epigenetic and Molecular Control of Development and Germ Cell Fate Determination View all 7 articles
Polycomb group (PcG) proteins are key regulators of gene expression and developmental programs via covalent modification of histones, but the factors that interpret histone modification marks to regulate embryogenesis are less studied. We previously identified Remodeling and Spacing Factor 1 (RSF1) as a reader of histone H2A lysine 119 ubiquitination (H2AK119ub), the histone mark deposited by Polycomb Repressive Complex 1 (PRC1). In the current study, we used Xenopus laevis as a model to investigate how RSF1 affects early embryonic development and whether recognition of H2AK119ub is important for the function of RSF1. We showed that knockdown of Xenopus RSF1, rsf1, not only induced gastrulation defects as reported previously, but specific targeted knockdown in prospective neural precursors induced neural and neural crest defects, with reductions of marker genes. In addition, similar to knockdown of PRC1 components in Xenopus, the anterior-posterior neural patterning was affected in rsf1 knockdown embryos. Binding of H2AK119ub appeared to be crucial for rsf1 function, as a construct with deletion of the UAB domain, which is required for RSF1 to recognize the H2AK119ub nucleosomes, failed to rescue rsf1 morphant embryos and was less effective in interfering with early Xenopus development when ectopically expressed. Furthermore, ectopic deposition of H2AK119ub on the Smad2 target gene gsc using a ring1a-smad2 fusion protein led to ectopic recruitment of RSF1. The fusion protein was inefficient in inducing mesodermal markers in the animal region or a secondary axis when expressed in the ventral tissues. Taken together, our results reveal that rsf1 modulates similar developmental processes in early Xenopus embryos as components of PRC1 do, and that RSF1 acts at least partially through binding to the H2AK119ub mark via the UAB domain during development.
Polycomb group (PcG) proteins were originally identified in Drosophila as repressors of homeobox (Hox) genes, mutations of which often cause homeotic transformation of posterior to anterior legs, characterized by a comb-like bristle phenotype (Lewis, 1978). Subsequently, homologues of Drosophila PcG proteins were identified in vertebrates and plants and emerged as key regulators of gene expression programs (Schuettengruber et al., 2017; Piunti and Shilatifard, 2021; Baile et al., 2022). In addition to regulating Hox genes and anterior-posterior axial identity, PcG proteins have been shown to influence expression of many central developmental regulators, including genes involved in signaling, such as components of the TGFβ, Wnt, and MAPK pathways, transcriptional controls, as well as metabolism (Brookes et al., 2012). PcG proteins and their target genes play pivotal roles in many aspects of cellular physiology, such as cell fate determination, epigenetic memory, cell lineage commitment, and X inactivation (Schuettengruber et al., 2017; Blackledge and Klose, 2021; Piunti and Shilatifard, 2021). Mutations in PcG proteins often lead to diverse developmental phenotypes in vertebrates, including humans, and have been causally linked to disease predisposition and progression, particularly cancer (Piunti and Shilatifard, 2021; Dong et al., 2022; German and Ellis, 2022).
The function of PcG proteins is achieved through forming multiple subunit complexes. Two major repressive complexes, Polycomb Repressive Complex 1 and 2, a.k.a. PRC1 and PRC2, have been studied extensively. PRC2 contains EZH2, SUZ12, and EED as core components and can methylate histone H3 at lysine 27 (H3K27me) (Margueron and Reinberg, 2011). PRC1 contains Ring1 (Ring1A in mouse), Ring2 (also called RNF2 or Ring1B in mouse), and Bmi1 as core components and mono-ubiquitinate histone H2A at lysine 119 (H2AK119ub) (de Napoles et al., 2004; Wang et al., 2004). These modifications on chromatin form a positive feed-back loop to enhance the repressive effects. H3K27me can facilitate PRC1 to target chromatin for H2AK119ub modification whereas H2AK119ub can also recruit PRC2 through the auxiliary subunit Jarid2 for H3K27me (Blackledge et al., 2014; Cooper et al., 2014; Kalb et al., 2014; Cooper et al., 2016). This positive feed-back, self-enhancement mechanism may help the establishment of large PcG repressive domains. These domains repress alternative cellular gene expression programs, which contribute to the stabilization and maintenance of cell type-specific programs (Boyer et al., 2006; Bracken et al., 2006; Lee et al., 2006). Deletion of PRC1 or PRC2 subunits in mouse embryonic stem cells (ESC) often results in the expression of differentiation-associated genes and causes these ESCs to differentiate spontaneously (Chamberlain et al., 2008; Loh et al., 2021; Thornton et al., 2014; Pasini et al., 2007; O'Carroll et al., 2001). Therefore, PcG proteins are critical for the maintenance of cell identity (German and Ellis, 2022; Loh and Veenstra, 2022).
One essential question regarding gene regulation by histone modifications is how these histone marks are recognized and interpreted by other cellular proteins to affect chromatin structures and gene expression programs. We addressed this question in our previous studies by identifying a H2AK119ub-binding protein, Remodeling and Spacing Factor 1 (RSF1), the large subunit of RSF complex (LeRoy et al., 1998; Zhang et al., 2017). RSF1 can recognize H2AK119ub nucleosomes through a previously uncharacterized region we designated as the ubiquitinated H2A binding (UAB) domain. The UAB domain, spanning amino acid 770-807, interacts with H2AK119ub nucleosomes in a bi-partition model through a ubiquitin-interacting motif at its middle region and an arginine-anchoring mechanism at its N-terminal region. We showed in previous work that RSF1 is required for H2AK119ub target gene silencing and helps maintain regularly spaced H2AK119ub nucleosome patterns at promoter regions (Zhang et al., 2017). We also discovered that Rsf1 is required for vertebrate development. Specifically, loss of Rsf1 interrupted gastrulation and mesodermal induction in Xenopus laevis (Zhang et al., 2017). However, the importance of recognizing H2AK119ub via the UAB domain in RSF1 function was not addressed in these studies. In addition, the role of RSF1 during vertebrate embryogenesis has not been analyzed in depth. In this study, we extended our previous work and further investigated the activities of Rsf1 during development using the X. laevis model. While our previous studies established a role for Rsf1 in early stages of development, our investigation here revealed that Rsf1 also regulated other tissue types later in development. Another goal of this study was to determine whether the RSF1 UAB domain is important in embryos as it is in cells. We were interested in determining whether RSF1 also mediates the repressive effects of H2AK119ub on gene expression during embryonic development. Our studies revealed that the PRC1-H2AK119ub-RSF1 system, which was established with previous biochemical and cell-based assays, is also operating during normal Xenopus development, and that the UAB domain is important for the function of RSF1 in this system.
The utilization of X. laevis was under the institutional IACUC protocol 21854 at the University of Alabama at Birmingham and IACUC protocol AD20261 at Virginia Commonwealth University. X. laevis embryos were obtained by in vitro fertilization and micro-injected with RNAs or MOs, as described in previous publications (Tien et al., 2021; Mohammad Parast and Chang, 2022). The human RSF1 or RSF1ΔUAB (fused with GFP) sequences were moved from the pCDNA3 to the pCS105 vector, making them amenable for in vitro RNA synthesis for expression in Xenopus. The Xenopus ring1a (ring1a.L, XB-Gene-6493980, NCBI accession # XM_041572851) sequence was PCR-cloned into the EcoRI/XbaI cut CS105 vector using the primers: forward: 5’-GGAATTCACCAGTTTAAAGACAATGGC-3’ and reverse: 5’-GCTCTAGA CTATTTCTGCTCTTTGGT-3’. Ring1A R to Q mutation was generated by PCR using the primers: forward: 5’-AGAAGCTGGTGTCCAAGCAATCCCTACG-3’, reverse: 5’- TGGCCGTAGGGATTGCTTGGACACCA-3’. The Xenopus rnf2 (rnf2.L, XB-Gene-6538658, NCBI accession # XM_041589074) sequence was PCR-cloned into EcoRI/XhoI cut pCS105 vector using the primers: forward: 5’-GGAATTCACCATGAATTGCATCAGCATGC-3’ and reverse 5’-CCGCTCGAGTTATTTGTGCTCCTTGGTG-3’. For constructing ring1a-smad2, ring1a sequence was PCR-amplified from pCS105-ring1a with primers: forward, 5’-GGAATTCACCAGTTTAAAGACAATGGC-3’, reverse: 5’-GCTCTAGATTTCTGCTCTTTGGT-3’, digested with EcoRI/XbaI, ligated with Xba1/AscI digested PCR product amplified from pCS105-Smad2 with the primers: forward, 5’-GCTCTAGATCGTCCATCTTGCCATTCACG-3’, reverse, 5’-AGGCGCGCCGCGAATTAAAAAACCT-3’ into EcoRI/AscI cut pCS105 vector. For constructing rnf2-smad2, rnf2 sequence was PCR-amplified with primers: forward, 5’-GGAATTC ACCATGACGCAGGCAGTGCAGA-3’, reverse: 5’- CCGCTCGAGTTTGTGCTCCTTGGTG-3’, digested with EcoRI/XhoI, ligated with XhoI/AscI cut PCR product amplified from pCS105-Smad2 with the primers: forward, 5’-CCGCTCGAGTCGTCCATCTTGCCATTCACG-3’, reverse, 5’- AGGCGCGCCGCGAATTAAAAAACCT-3’ into the EcoRI/AscI cut pCS105 vector. RNAs were synthesized from linearized plasmids using the mMessage mMachine transcription kit (Ambion). The sequences for rsf1 splice-blocking MO are described previously (Zhang et al., 2017). The RNAs or MOs were injected into different regions of early Xenopus embryos, as indicated in the text and figure legends. The doses of RNAs or MOs used are indicated in the figures and/or figure legends.
Only one rsf1 homeolog (rsf1.S) has been identified in X. laevis (XB-GENE-988573, NCBI accession # XM_018250374.2) and will be from here on in referred to as rsf1. The antisense digoxygenin-labeled probe for rsf1 was made from the pBSKSII vector containing partial Xenopus rsf1 coding sequence made by PCR cloning using the primers: forward 5’-TACCAGAGCTGCAGGAAGCTGAAGC-3’ and reverse 5’-GATGTTCTCGAGGCTGATTCCAACG-3’. The plasmid was linearized with XhoI (antisense) or Pst1 (sense) and transcribed with T3 (antisense) or T7 (sense) polymerase. Sense probe labeled embryos did not display any staining (Supplementary Figure S1A). For marker gene expression analysis, MOs were co-injected with the 200 pg of RNA encoding the lineage tracer nuclear beta-galactosidase into animal regions of 2-cell stage or one dorsal animal blastomere of 4- to 8-cell stage embryos. The embryos were collected at the indicated stages, stained with the red-Gal substrate to mark the injected side, and subjected to WMISH for expression of neural, neural crest, and anterior-posterior neural patterning genes. Protocols for in situ hybridization and beta-galactosidase have been described (Zhang et al., 2017).
After injection with RSF1 MO or mRNA, embryos were collected at gastrula stages and lysed in lysis buffer [50 mM Tris-HCL (pH 7.5), 150 mM NaCl, 1 mM EDTA, 10% glycerol, 0.5% Triton X-100] with 10 μl buffer per embryo at 4°C on ice. The lysate was then centrifuged at 16,000 g for 15 min and the supernatant was used for SDS-PAGE, transferred to PVDF membrane. Immunoblots were performed with anti-RSF1 (Abcam ab109002, 1:1000), anti-GFP [Santa Cruz (B-2) sc-9996, 1:600], and anti-β-actin [Cell Signaling Technology (8H110D10) # 3700, 1:2000] antibodies. LI-COR Odyssey M imager was used to detect the signals.
The ChIP assay was performed using the method as described in recent publications (Tien et al., 2021; Mohammad Parast and Chang, 2022). Briefly, the embryos were injected with 1 ng GFP-RSF1 RNA and 0.4 ng Ring1A-Smad2 or Ring1A-RQ-Smad2, cultured to the stage 11 (mid-gastrula), and harvested by cross-linking with 1% formaldehyde in 1 X PBS for about 60 min at room temperature. Sheared chromatin was collected by sonication of the embryos on ice in cold RIPA buffer, split equally into separate tubes with about 40 embryo-worth of extract in each tube, and immunoprecipitated with 1–2 μg of anti-HA (Cell signaling Technology, 3724, negative control of the experiment), anti-GFP [Santa Cruz (B-2) sc-9996], anti-H2AK119ub (Cell signaling Technology, 8240) antibodies. After reverse crosslinking and DNA extraction, qPCR was performed on an Applied Biosystems StepOnePlus cycler using PowerUp SYBR Green Master Mix (ThermoFisher A25742). The potential smad2 binding sites upstream of the gsc gene (gsc.L upstream region, accession number # AB698641.1) were analyzed by motif-based sequence analysis tool FIMO (https://meme-suite.org/meme/tools/fimo) (Grant et al., 2011). The consensus SMAD2 binding motif was extracted from JASPAR database (http://jaspar.genereg.net/) (Fornes et al., 2019). Three putative smad2 binding sites were identified around the promoter of the gsc gene (Supplementary Figure S3A). Two sets of primer pairs were designed to investigate the DNA immunoprecipitated by the antibodies described above (Supplementary Figure S3A). The primer sequences for ChIP-qPCR are: gsc primers I: forward: 5’-CTTACATTCCCAAAAGATGAACAGT-3’ and reverse: 5’-GGTTTAATATTTGCCATGAAGCTAA-3’; gsc primers II: forward: 5’-AGAGAAACAAAACAGTCATTCCATT-3’ and reverse: 5’-ATCTGTGCCTCTTCCCTTATATAGC-3’. The experiment was repeated biologically three times, with each subjected to technical duplicates for qPCR. The percentage input method was used to calculate ChIP signals.
Our previous work demonstrated that Xenopus rsf1, as well as the PRC1 complex members ring1 (ring1a) and rnf2 (ring1b) are expressed during early embryogenesis (Zhang et al., 2017). While we have shown previously that rsf1 has a role in mesodermal development, we also wondered if it could additionally have roles in the development of other structures. Therefore, the spatiotemporal expression of rsf1 was examined using a whole mount in situ hybridization (WMISH) assay. The transcripts of rsf1 were distributed ubiquitously during blastula and gastrula stages (Supplementary Figure S1A). At neurula stages, rsf1 was also widely expressed through the entire embryo but additionally appeared to be enriched in a region consistent with the presumptive neural crest (Figure 1Ai). Later, the signal was further enriched in migrating neural crest, eyes, brain, and ventral mesoderm, at the tailbud stage (approximately 26 hpf at 23°C) (Figure 1Aii). After another day of development, at tadpole stages, rsf1 also appeared in the developing branchial arches, which give rise to the craniofacial structures, and the anterior somites, which give rise to muscles and skeleton (Figure 1Aiii). The expression pattern suggests that rsf1 may participate in the development of multiple tissues at different stages, including mesoderm, neural and neural crest.
FIGURE 1. Rsf1 is expressed in multiple tissues of Xenopus laevis during early development and decreasing the levels of Rsf1 causes developmental malformations. (A) Whole mount in situ hybridization of rsf1 mRNA (purple) in Xenopus embryos. Representative lateral views of embryos (anterior to the right) at stages 15, 27 and 37 were shown. Rsf1 sense probe and rsf1 anti-sense probe with no antibody controls were included in Supplementary Figure S1A. (B) Immunoblots showing Rsf1 levels in control and rsf1 MO injected embryos. The amounts of rsf1 MO were indicated on the top of the panel, beta-actin was used as a loading control. Additional and raw images of blots are shown in Supplementary Figure S1C. (C) Quantification of western blots based on three replicates. One-way ANOVA revealed statistical differences among the groups (p < 0.001) and Holm-Sidak posthoc pair-wise comparisons showed statistical differences with each dose and control [p = 0.001 (uninj vs. 5 ng), p = 0.003 (uninj vs. 10 ng), p = 0.006 (ininj vs. 20 ng) SigmaPlot]. (D) Representative images of embryos injected with rsf1 MO revealing malformations that were more severe with increasing concentrations. The amounts of rsf1 MO were indicated on the top of the panel. Anterior is to the right. (E) Quantification of rsf1 morphant embryos displaying various phenotypes. A mild phenotype includes small eyes, reduced head size (e.g., arrowhead). A severe phenotype includes small or no eyes, reduced head size or no head, shortened and bent body axis, failure of blastopore closure (white arrow) (n = 20, 2 biological replicates). nc, neural crest, np, neural plate, cg, cement gland, ov, otic vesicle, bas, branchial arches.
An antisense morpholino oligo (MO) that targets intron-1/exon 2 junction was designed to disrupt splicing (Supplementary Figure S1B). Consistently, when the rsf1 MO was injected into embryos, there was a statistically significant dose-dependent reduction of Rsf1 protein levels (Figures 1B, C). Correspondingly, these doses of rsf1 MOs also resulted in dose-dependent developmental defects (Figures 1D, E). Low doses of the MO, for the most part, induced only minor changes in embryonic morphology, including shorter length and smaller head size (Figure 1Dii, arrowhead). 85% of the embryos injected with 5 ng of the MO appeared to have more severe defects, including shortened and bent body axis as well as smaller heads (Figure 1Dii). The effects were even more severe at 10 ng where 100% of the embryos had severe malformations. In some cases, these embryos had blastopore closure defects, indicating impaired gastrulation (Figure 1Div, arrow). These effects were never observed in the sibling un-injected controls. However, to ensure the effects were not simply due to an injection effect, we also demonstrated that un-injected embryos closely resembled embryos injected with a standard control MO (Supplementary Figure S1D). Moreover, to demonstrate the specificity of the rsf1 MO, we also determined that the defects in the Rsf1 morphants could be ameliorated by co-injecting RSF1 mRNA (Figure 3D).
Previously, we noted the Rsf1 has a role in mesodermal development (Zhang et al., 2017). Consistently, we observed expression of rsf1 throughout the gastrula stages, including the presumptive mesoderm, as well as gastrulation-type defects in the morphants. Additionally, rsf1 expression was also observed in neural and neural crest tissues and the rsf1 morphants had head defects (Figure 1A). These data prompted us to further investigate the role for Rsf1 in the specification of these tissues. To do so, we performed targeted injections of the MO and determined how this would affect the expression of genes that not only mark neural and neural crest but also regulate of neural or neural crest development. In these experiments, rsf1-MO was co-injected with an RNA lineage tracer that encodes nuclear β-galactosidase. Both the MOs and lineage tracers were injected into the animal region of one dorsal blastomere of four-to eight-cell stage embryos. As a result, these reagents would be localized to one half of the embryo in tissues fated to become the neural and neural crest. The embryos were collected at early stages when the neural and neural crest are specified. Then they were stained with a substrate of β-galactosidase (red-Gal) to mark the injected side, and subjected to WMISH for expression of neural and neural crest markers. We found that the pan-neural genes nrp1 and ncam were slightly reduced, especially in the posterior regions of 80% and 76% of the embryos respectively (Figure 2A). Decreased Rsf1 resulted in an even more dramatic reduction in neural crest marker genes slug, sox10, sox9, and twist on the MO-injected side when compared with that in the un-injected side (70%–91%, Figure 2A). Another important modulator of neural development is OCT4. This protein is thought to be integral to maintaining cell pluripotency and delay cell differentiation (Tien et al., 2021). Therefore, we examined the expression of the Xenopus OCT4 ortholog pou5f3.2, also called oct25. Results showed that the expression of this gene was expanded by Rsf1 knockdown in 67% of the morphant embryos (Figure 2B). These results point to a hypothesis where Rsf1-mediated PRC1 function is required for repressing pluripotency in the neural and neural crest domains to allow proper cell differentiation of these tissues.
FIGURE 2. Targeted knockdown of rsf1 in presumptive neural territories interferes with specification of neural and neural crest tissues and affects anterior-posterior neural patterning. Embryos were all injected with 2.5 ng of MO into one dorsal blastomere. (A) Targeted knockdown of Rsf1 in presumptive neural territories downregulated neural and neural crest marker genes. Dorsal views of representative embryos at stage 17, anterior to the top and the injected side is on the left. The neural (nrp1 and ncam) and neural crest (slug, sox10, sox9, and twist) markers were reduced in the embryos on the side of targeted injection. 100% of controls had expression pattern similar to the representative embryos shown (black arrows, n = 33–35, 2 biological replicates for each marker). (B) The pluripotent marker gene oct25 was expanded on the rsf1 MO injected side. 100% of controls had expression pattern similar to the representative embryos shown (n = 24, 2 biological replicates). (C) Targeted knockdown of rsf1 in presumptive neural territories affected A-P axis gene expression. Dorsal views of representative embryos at stage 24, anterior to the top and the injected side is on the left. Bracketed regions indicate expression domains, and black arrows point to areas where there is a reduced expression. The numbers of the morphant embryos with expression pattern changes similar to those shown are indicated in the bottom right of each panel. 100% of controls had expression pattern similar to the representative embryos shown (n = 10–12, 2 biological replicates).
PRC1 has also been shown to regulate anterior-posterior (A-P) body patterning in both invertebrates and vertebrates, at least partially via its ability to regulate its canonical targets, the hox genes (Soshnikova, 2014; Gentile and Kmita, 2020). To investigate whether Rsf1 shares this function with PRC1, specifically in the developing nervous system, we again injected rsf1 MO unilaterally into the animal region of one dorsal blastomere of four- to eight-cell stage embryos with the lineage tracer as described above. Marker gene expression was analyzed at approximately 24 hpf at 23°C when the A-P axis of the brain is forming. Analysis of the anterior neural patterning genes otx2 and engrailed (en) revealed that rsf1 MO reduced the expression of these genes in the head (Figure 2C, arrows). Moreover, decreasing the levels of Rsf1 also caused a posterior shift in the expression domains of krox20, hoxd10, hoxd4, and hoxd9 on the MO-injected side of the embryos (Figure 2C). Collectively, these data suggest that Rsf1 indeed has a role in the patterning of the A-P axis of the nervous system.
The UAB domain in RSF1 was identified in our previous studies as a crucial region for binding to H2AK119ub nucleosomes and mediates its repressive activity on gene expression (Zhang et al., 2017). To determine whether the UAB domain is important for RSF1 to regulate Xenopus development, we examined the effects of wild-type human RSF1 and the UAB domain-deleted mutant (RSF1ΔUAB) on Xenopus embryogenesis. These sequences were fused with GFP to serve as a tracer (Figure 3A). Capped mRNA generated from these constructs were injected into the dorsal marginal zone of 4-cell stage embryos, and the effects on embryogenesis were tested at a variety of concentrations (not shown). We determined that 4 ng of RSF1 mRNA produced a significant defect. The levels of ectopically expressed RSF1 or RSF1ΔUAB proteins were similar in embryos injected with this concentration of mRNA (Figure 3B). Embryos injected with RSF1 mRNA had moderate-to-severe defects, such as curved body axis, smaller heads, and gastrulation defects (Figure 3C). In contrast, injection of RSF1ΔUAB mRNA in sibling embryos resulted in much less severe defects (Figure 3C). The notable difference in phenotype severity upon expression of similar levels of RSF1 and RSF1ΔUAB suggests that the UAB domain is important for the function of RSF1 during Xenopus development (Figure 3C).
FIGURE 3. The UAB domain is required for RSF1 to regulate Xenopus embryonic development. (A) Schematic representation of the two constructs used in the study consisting of a human RSF fused to GFP and a mutant form of RSF1 with the UAB region deleted and fused to GFP. (B) Immunoblots of RSF protein in extracts prepared from Xenopus embryos injected with RSF1 or RSF1ΔUAB mRNA. The levels of GFP fusion proteins appear the same. β-actin was used as loading controls. Additional images and raw data are included in Supplementary Figure S2A. (C) Embryos injected with 4 ng of RSF1 mRNA resulted in major malformations. However, much less severe developmental defects were observed in embryos injected with 4 ng RSF1ΔUAB mRNA. Representative embryos are shown with anterior to the right (n = 18, two biological replicates). (D) RSF1 but not UAB deleted RSF1ΔUAB rescued the developmental defects caused by rsf1 knockdown. The malformations induced by 5 ng of rsf1 MO were partially rescued by co-injection of low concentrations (0.25 ng) of RSF1 mRNA but not 0.25 ng of RSFΔUAB mRNA (compare embryos with white arrows). Control embryos are un-injected siblings (n = 20, 2 biological replicates). Four representative embryos are shown with anterior to the right.
To further confirm the importance of the UAB domain for the function of RSF1 in development, we compared whether RSF1ΔUAB mRNA could ameliorate the effects of rsf1 MO knockdown as effectively as wild-type RSF1 mRNA. For this purpose, we titrated down the concentrations of RSF1 mRNA or RSF1ΔUAB mRNA, so that no obvious malformations could be observed (0.25 ng/embryo, Figure 3D). We injected a moderate dose of rsf1 MO (5 ng/embryo) alone and observed a shortened body axis induced in approximately 50% of the embryos. Using the sub-phenotypic dose of RSF1 mRNA in combination with a moderate dose of rsf1 MO, we observed that this shortened body axis could be rescued (Figure 3D). In contrast, a sub-phenotypic dose of RSF1ΔUAB mRNA did not rescue the rsf1 MO effects as effectively (Figure 3D). Taken together, these data suggest that the UAB domain is important for the function of RSF1 and that recognition of H2AK119ub may underlie the activity of Rsf1 to regulate Xenopus embryogenesis. Furthermore, these experiments also demonstrate the specificity of the rsf1 MO as well as the conservation of Rsf1 function, since we used human RSF1 sequences.
Our next goal was to test the hypothesis that Rsf1 mediates the gene repressive effects of H2AK119ub during PRC1-regulated embryonic development. To do this, we created an artificial system that would generate ectopic H2AK119ub marks on target genes. We then used this system to examine the role of Rsf1 in regulating the expression at the ectopic gene locus (see the next section). Our artificial system consisted of the PRC1 subunit Ring1a, which we have shown in our previous work to play major roles in Xenopus development (Zhang et al., 2017). The Ring1a protein was fused to Smad2, which is a well-studied signaling molecule acting downstream of the mesodermal inducing Nodal (Schier, 2003). The prediction was that Ring1a, when fused to Smad2, would deposit ubiquitin marks at histone H2AK119 on target genes and these genes would then be repressed rather than induced (Figure 4A).
FIGURE 4. Fusion of ring1a to smad2 limits the function of smad2 in mesoderm induction. (A) Schematic showing the experimental system. (B) Embryos dorsally injected with (0.25 ng) of mRNA were examined for changes in pigmentation during gastrulation and inappropriate expression of mesodermal genes, all of which indicate mesoderm induction. Additional pigment and mesodermal gene expression are indicated by white arrows (n = 19–20, two biological replicates). (C) Embryos ventrally injected with (0.25 ng) of mRNA were examined for protrusions, indicatives of secondary axis formation. Overt protrusions are indicated by black arrows. N = 18–20, two biological replicates. ba’s, branchial arches.
We first tested this fusion construct on mesoderm development in vivo using our Xenopus model. When smad2 is expressed in the animal region, it induced ectopic mesoderm, as visualized by dark pigmented cells indicative of ectopic bottle cells at gastrula stages (Schier, 2003). Indeed, smad2 mRNA injected embryos appeared to have abundant pigmented cells in the ectodermal region (Figure 4B, white arrow). On the other hand, ring1a-smad2 mRNA was less effective in inducing such ectopic pigmented cells, indicating a reduction in mesoderm induction (Figure 4B). To further assess mesoderm development, we next examined the expression of mesodermal marker genes, which are expressed in the early mesoderm. This analysis revealed that while smad2 mRNA induced the expression of the mesodermal markers brachyury (xbra), chordin, and xnr1, the ring1a-smad2 mRNA failed to induce these markers (Figure 4B). To ensure that the effect of Ring1a-Smad2 fusion protein on mesoderm induction was specific, we performed additional controls. First, ring1a mRNA alone was injected into embryos and it was determined that Ring1a alone did not induce excess pigmentation at gastrula stages, nor did it induce secondary axis formation (Supplementary Figure S2B). One might argue that the artificial fusion construct might affect mesodermal gene expression due to the steric hindrance of the added Ring1a. Therefore, we included another control, in which an R to Q point mutation in the catalytic domain of the Ring1a protein that abolishes its ubiquitin ligase activity was introduced into the ring1a-smad2 construct. Embryos injected with ring1a(RQ)-smad2 RNA appeared similar to embryos injected with smad2 mRNA alone in inducing pigmented cells and expression of mesodermal markers (Figure 4B). These results indicate that the fusion protein likely acted as predicted and its repressive effects on mesoderm induction required the H2AK119ub activity of Ring1a. Finally, we also constructed a fusion of Smad2 with the other PRC1 complex member Ring1b (also called Rnf2 in Xenopus). Results revealed that embryos injected with smad2-rnf2 mRNA did not abolish the Smad2-induced expression of xbra, however, it did reduce the expression of mesodermal markers chordin and xnr1 (Supplementary Figure S2C). These results suggest that Ring1a is the primary regulator of Xenopus mesodermal development, consistent with previous reports (Zhang et al., 2017).
Overexpression of smad2 can also induce ectopic secondary axis formation when expressed in the ventral region (Schier, 2003). Indeed, when we injected smad2 RNA in the ventral marginal zone of 4-cell stage embryos, we observed the formation of partial secondary axis as a large protrusion (Figure 4C). However, expression of the ring1a-smad2 mRNA in the ventral region did not induce these protrusions as effectively, some were absent or much smaller (Figure 4C). Embryos injected with ring1a(R/Q)-smad2 RNA, however, developed secondary axis protrusions, similar to the smad2 mRNA alone (Figure 4C). These results thus validate our artificial system, showing that the Smad2-Ring1a fusion has repressive properties that rely on the Ring1a ubiquitin ligase activity. These results are consistent with our hypothesis that Ring1a is recruited to the Smad2 target genes by the fusion protein, deposits ectopic H2AK119ub marks at the regulatory regions of these genes, and leads to inefficient activation or even repression of Smad2 transcriptional activity (Figure 4A).
Now that we have established an artificial system to test for Ring1a-dependent H2AK119ub activity and Rsf1 recruitment in vivo, we next asked whether Ring1a-Smad2 indeed deposits ectopic H2AK119ub marks directly to a Smad2 target and then recruits RSF1 for gene repression (Figure 5A). To do this, we chose a potential direct target of Smad2 in Xenopus development, the gooscoid (gsc) gene (Kim et al., 2011). This gene has also been well established to be an integral regulator of mesoderm induction and organizer formation (Sudou et al., 2012). To identify the Smad2 binding sites in Xenopus gsc, we first used JASPAR, an online open-access database storing manually curated transcription factor binding profiles (Fornes et al., 2019). This database identified a SMAD2 consensus binding sequence CCAGAC (Figure 5B). We then searched the promoter regions of the Xenopus gsc gene using the online motif-searching tool FIMO (Grant et al., 2011). The result revealed three Smad2 consensus motifs within the promoter of this gene and primers were designed to flank these motifs (Supplementary Figure S3A). Chromatin immunoprecipitation (ChIP) assay with anti-H2AK119ub antibody revealed that embryos injected with ring1a-smad2 mRNA had enhanced H2AK119ub signals at the gsc gene promoter (Figure 5C), as compared with embryos injected with mutant ring1a(R/Q)-smad2 mRNA (Figure 5C).
FIGURE 5. Ring1a-smad2 deposits H2AK119ub marks on the smad2 target gene gsc and recruits RSF1. (A) Schematic of the experimental hypothesis. (B) SMAD2 binding motif. (C) Real-time PCR of input and chromatin immunoprecipitated DNA by the indicated antibody. Embryos were co-injected with GFP-RSF1 (1 ng) and ring1a-smad2 or ring1a-R/Q-smad2 (0.4 ng) and subjected to immunoprecipitation using anti-H2AK119ub antibody. IgG was used as negative controls. ChIP signals were normalized to input signals and percentage inputs were plotted and shown for two primer pairs. (D). Real-time PCR of input and chromatin immunoprecipitated DNA by the indicated antibodies. Embryos were injected as in (C) and subjected to immunoprecipitation using anti-GFP antibody. Anti-HA antibody was used as negative controls. Percentage input results from each technical repeat are represented by symbols and median is shown as a short bar. Wilcoxon matched-pairs signed rank test revealed statistical differences between ring1a-smad2 and ring1a(R/Q)-smad2 groups (p < 0.05). Raw data were included in Supplementary Figure S3B.
To explore whether ectopic H2AK119ub marks could cause the recruitment of the RSF1 protein, we also performed ChIP against GFP in embryos co-injected with GFP-RSF1 and ring1a-smad2 or ring1a(R/Q)-smad2 mRNA. We observed that embryos injected with ring1a-smad2 indeed showed higher signal for GFP (indicative of RSF1 binding) at the gcs promoter regions when compared with embryos injected with ring1a(RQ)-smad2 (Figure 5D). In these experiments, we included an uninjected group as negative control and normalized our ChIP data against uninjected group signals (Figures 5C, D). The data provide direct evidence that the PRC1-H2Aub-RSF1 system, which was originally identified in biochemical and cell-based assay, is also operating in vivo during vertebrate development.
RSF1 was first identified as the non-catalytic subunit of RSF complex, together with the ATP-dependent subunit SNF2H, to facilitate activator-dependent transcription initiation on chromatin templates (LeRoy et al., 1998). Subsequently, RSF was shown to mediate nucleosome deposition and generate regularly spaced nucleosome arrays in an ATP-dependent manner (Loyola et al., 2001; Loyola et al., 2003). In Drosophila, mutant Rsf-1 behaved as a dominant suppressor of position effect variegation (Hanai et al., 2008). Kdm2, a subunit of the variant PRC1.1 complex, specifically pulled down CG8677, the Dosohplia homolog of RSF1 (Kang et al., 2022). Together, these data suggested that RSF1 may be a missing factor in the PcG system. Indeed, our previous work showed that RSF1 can recognize H2AK119ub nucleosomes and is involved in PRC1-mediated gene repression (Zhang et al., 2017).
PcG proteins are essential for maintaining cell type-specific gene expression programs and, therefore, cell identity (Boyer et al., 2006; Bracken et al., 2006; Lee et al., 2006; Thornton et al., 2014). For example, mouse embryonic stem cells lacking PcG proteins such as EZH2, SUZ12, EED and Ring1B, are often unstable and tend to differentiate spontaneously (Pasini et al., 2007; Chamberlain et al., 2008; Voncken et al., 2003; O'Carroll et al., 2001). When induced to differentiate, these knockout ESC lines also fail to undergo successful lineage commitment, demonstrating a clear role for PcG proteins in cell fate. Since PcG complexes such as PCR1 are critical for defining cell identity and RSF1 is required for PRC1-mediated gene repression, it was an obvious next step to explore a role for RSF1 in embryonic development. Understanding how RSF1 functions in the embryo has the potential to begin to fill a gap in our understanding of gene repression during specification of cell fates. However, PcG protein knockout mice die at the embryonic stage after implantation but before finishing gastrulation. The early lethality prevents further dissection of the function of PcG protein in mammalian embryogenesis. Therefore, we have turned to another vertebrate model, X. laevis. This model has been widely used for studying epigenetic modulators during development (Bajpai et al., 2010; Tahir et al., 2014; Greenberg et al., 2019; Wyatt et al., 2021; Mohammad Parast and Chang, 2022) and has several advantages (Bowes et al., 2008; Tandon et al., 2017). For example, Xenopus embryos are free-living, develop rapidly, and can be obtained simultaneously (by in vitro fertilization) in great numbers with synchronous development. This allows us to monitor changes in development in real time and is ideal for biochemical analyses. In addition, cell fates have been mapped to early cleavage stage embryos (Moody, 1987; Moody, 2019), thus allowing targeted injection of reagents into defined regions to specifically affect gene levels in tissue-specific manners. Importantly, tools to modify levels of gene expression are titratable and thus we can avoid the lethality of gene knockout, which occur in mouse models (Eisen and Smith, 2008).
Here, using X. laevis as a model, we examined the PRC1-H2AK119ub-RSF1 pathway in embryogenesis. One major goal was to demonstrate that this system worked in a similar way during development. We have previously defined a specific domain, called UAB, in RSF1 that can recognize H2AK119ub nucleosomes. Here, we demonstrated that this domain is indeed critical for RSF1 function in embryos as well. This provides additional evidence that this is a conserved pathway. Importantly, we also determined that, like in mammalian cells, RSF1 is recruited to PRC1-mediated H2AK119ub. To show this, we created an artificial system using the known role of Smad2 in mesoderm development. Smad2 is activated by Nodal signaling during induction of mesoderm during gastrulation (Schier, 2003). For this reason, ectopic expression of Smad2 in the animal region (where ectoderm forms) results in the inappropriate induction of mesoderm. As we expected, when Smad2 was fused to Ring1a, mesoderm was no longer induced, suggesting that Ring1a deposited the repressive H2AK119ub marks on genes required for mesodermal development. This was not due to ring1a expression nor due to the steric hindrance of the fused proteins, since a point mutation to ring1a did not have the same repressive effect. Similarly, when smad2 is overexpressed in a ventral blastomere, embryos form a secondary axis due to additional and inappropriate organizer induction (Schier, 2003). Again, as expected, the Smad2-Ring1a fusion could repress this developmental anomaly. Next, we used this system to directly examine changes in H2AK119ub marks on consensus Smad2 binding motifs in a Smad2 target gene, gsc. The ChIP analysis demonstrated that the Smad2-Ring1 fusion protein deposited H2AK119ub marks. Importantly, the epitope H2AK119ub marks recruit RSF1. Thus, these results support our model established with cell-based assays (Figure 5A).
In previous work, we revealed a role for Rsf1 in gastrulation and mesoderm development (Zhang et al., 2017). In the present study, we extended the data to reveal additional roles for Rsf1 in neural and neural crest development. Specification of each of these tissues requires their own sophisticated orchestration of signaling and transcriptional regulators [reviewed in (Sargent, 2006; Prasad et al., 2012; Mayor and Theveneau, 2013; Bronner and Simões-Costa, 2016)]. Targeted injection of rsf1 antisense oligonucleotides (morpholinos) into blastomeres that are fated to the neural and neural crest progenitors resulted in a reduction in the expression of markers of these tissues, which are also critical for their development. These results are consistent with rsf1 morphant embryos having a smaller head, possibly due to the failure of brain and cranial neural crest cell proliferation and differentiation. Interestingly, we also observed an expansion in oct25, the Xenopus ortholog of OCT4. It has been proposed that OCT4 has been co-opted by neurons and neural crest cells to expand their developmental potential and maintain a multipotent state (Patel and Parchem, 2022). Future work in dissecting the role of PRC1-H2AK119ub-RSF1 regulation of gene expression could provide a better understanding of the balance between pluripotency and differentiation during both neural and crest development. PcG proteins are also known to regulate homeobox transcription factors (Gentile and Kmita, 2020). These proteins are critical regulators of cell identity and positioning in embryonic development, specifically in the nervous system (Soshnikova, 2014; Parker et al., 2018; Saito and Suzuki, 2020). In this study, Rsf1 knockdown resulted in posterior shifts in the expression of two Hox genes and the homeobox-containing gene krox20, all expressed in the developing nervous system. Our data suggests that Rsf1 could also be important for delineating embryonic hox gene expression domains.
In conclusion, based on the work presented here and in previous studies (Zhang et al., 2017), we propose that RSF1 is an integral component of the PRC1-H2AK119ub epigenetic system. Importantly, we solidify that the PRC1-H2AK119ub-RSF1 system is also operating in the specification of several fundamental tissue types in vertebrate embryonic development.
The raw data supporting the conclusion of this article will be made available by the authors, without undue reservation.
The animal study was reviewed and approved by the UAB IACUC 21854 and VCU IACUC AD20261.
SP and DY: Performing experiment, data analyses, and manuscript draft. CXC: Manuscript preparation. AD, CBC, and HW: Experiment design and manuscript preparation. All authors contributed to the article and approved the submitted version.
This work was supported by the NIH grant RO1 GM 130696 and DOD W81XWH2110714 (to HW).
The authors declare that the research was conducted in the absence of any commercial or financial relationships that could be construed as a potential conflict of interest.
All claims expressed in this article are solely those of the authors and do not necessarily represent those of their affiliated organizations, or those of the publisher, the editors and the reviewers. Any product that may be evaluated in this article, or claim that may be made by its manufacturer, is not guaranteed or endorsed by the publisher.
The Supplementary Material for this article can be found online at: https://www.frontiersin.org/articles/10.3389/fcell.2023.1168643/full#supplementary-material
Baile, F., Gómez-Zambrano, Á., and Calonje, M. (2022). Roles of Polycomb complexes in regulating gene expression and chromatin structure in plants. Plant Commun. 3, 100267. doi:10.1016/j.xplc.2021.100267
Bajpai, R., Chen, D. A., Rada-Iglesias, A., Zhang, J., Xiong, Y., Helms, J., et al. (2010). CHD7 cooperates with PBAF to control multipotent neural crest formation. Nature 463, 958–962. doi:10.1038/nature08733
Blackledge, N. P., Farcas, A. M., Kondo, T., King, H. W., Mcgouran, J. F., Hanssen, L. L. P., et al. (2014). Variant PRC1 complex-dependent H2A ubiquitylation drives PRC2 recruitment and polycomb domain formation. Cell 157, 1445–1459. doi:10.1016/j.cell.2014.05.004
Blackledge, N. P., and Klose, R. J. (2021). The molecular principles of gene regulation by Polycomb repressive complexes. Nat. Rev. Mol. Cell Biol. 22, 815–833. doi:10.1038/s41580-021-00398-y
Bowes, J. B., Snyder, K. A., Segerdell, E., Gibb, R., Jarabek, C., Noumen, E., et al. (2008). Xenbase: A Xenopus biology and genomics resource. Nucleic Acids Res. 36, D761–D767. doi:10.1093/nar/gkm826
Boyer, L. A., Plath, K., Zeitlinger, J., Brambrink, T., Medeiros, L. A., Lee, T. I., et al. (2006). Polycomb complexes repress developmental regulators in murine embryonic stem cells. Nature 441, 349–353. doi:10.1038/nature04733
Bracken, A. P., Dietrich, N., Pasini, D., Hansen, K. H., and Helin, K. (2006). Genome-wide mapping of Polycomb target genes unravels their roles in cell fate transitions. Genes Dev. 20, 1123–1136. doi:10.1101/gad.381706
Bronner, M. E., and Simões-Costa, M. (2016). The neural crest migrating into the twenty-first century. Curr. Top. Dev. Biol. 116, 115–134. doi:10.1016/bs.ctdb.2015.12.003
Brookes, E., de Santiago, I., Hebenstreit, D., Morris, K. J., Carroll, T., Xie, S. Q., et al. (2012). Polycomb associates genome-wide with a specific RNA polymerase II variant, and regulates metabolic genes in ESCs. Cell Stem Cell 10, 157–170. doi:10.1016/j.stem.2011.12.017
Chamberlain, S. J., Yee, D., and Magnuson, T. (2008). Polycomb repressive complex 2 is dispensable for maintenance of embryonic stem cell pluripotency. Stem Cells 26, 1496–1505. doi:10.1634/stemcells.2008-0102
Cooper, S., Dienstbier, M., Hassan, R., Schermelleh, L., Sharif, J., Blackledge, N. P., et al. (2014). Targeting polycomb to pericentric heterochromatin in embryonic stem cells reveals a role for H2AK119u1 in PRC2 recruitment. Cell Rep. 7, 1456–1470. doi:10.1016/j.celrep.2014.04.012
Cooper, S., Grijzenhout, A., Underwood, E., Ancelin, K., Zhang, T., Nesterova, T. B., et al. (2016). Jarid2 binds mono-ubiquitylated H2A lysine 119 to mediate crosstalk between Polycomb complexes PRC1 and PRC2. Nat. Commun. 7, 13661. doi:10.1038/ncomms13661
de Napoles, M., Mermoud, J. E., Wakao, R., Tang, Y. A., Endoh, M., Appanah, R., et al. (2004). Polycomb group proteins Ring1A/B link ubiquitylation of histone H2A to heritable gene silencing and X inactivation. Dev. Cell 7, 663–676. doi:10.1016/j.devcel.2004.10.005
Dong, G. J., Xu, J. L., Qi, Y. R., Yuan, Z. Q., and Zhao, W. (2022). Critical roles of polycomb repressive complexes in transcription and cancer. Int. J. Mol. Sci. 23, 9574. doi:10.3390/ijms23179574
Eisen, J. S., and Smith, J. C. (2008). Controlling morpholino experiments: don't stop making antisense. Development 135, 1735–1743. doi:10.1242/dev.001115
Fornes, O., Castro-Mondragon, J. A., Khan, A., van der Lee, R., Zhang, X., Richmond, P. A., et al. (2019). Jaspar 2020: Update of the open-access database of transcription factor binding profiles. Nucleic Acids Res. 48, D87–D92. doi:10.1093/nar/gkz1001
Gentile, C., and Kmita, M. (2020). Polycomb repressive complexes in hox gene regulation: Silencing and beyond: The functional dynamics of polycomb repressive complexes in hox gene regulation. Bioessays 42, e1900249. doi:10.1002/bies.201900249
German, B., and Ellis, L. (2022). Polycomb directed cell fate decisions in development and cancer. Epigenomes 6, 28. doi:10.3390/epigenomes6030028
Grant, C. E., Bailey, T. L., and Noble, W. S. (2011). Fimo: Scanning for occurrences of a given motif. Bioinformatics 27, 1017–1018. doi:10.1093/bioinformatics/btr064
Greenberg, R. S., Long, H. K., Swigut, T., and Wysocka, J. (2019). Single amino acid change underlies distinct roles of H2A.Z subtypes in human syndrome. Cell 178, 1421–1436.e24. doi:10.1016/j.cell.2019.08.002
Hanai, K., Furuhashi, H., Yamamoto, T., Akasaka, K., and Hirose, S. (2008). RSF governs silent chromatin formation via histone H2Av replacement. PLoS Genet. 4, e1000011. doi:10.1371/journal.pgen.1000011
Kalb, R., Latwiel, S., Baymaz, H. I., Jansen, P. W., Müller, C. W., Vermeulen, M., et al. (2014). Histone H2A monoubiquitination promotes histone H3 methylation in Polycomb repression. Nat. Struct. Mol. Biol. 21, 569–571. doi:10.1038/nsmb.2833
Kang, H., Cabrera, J. R., Zee, B. M., Kang, H. A., Jobe, J. M., Hegarty, M. B., et al. (2022). Variant Polycomb complexes in Drosophila consistent with ancient functional diversity. Sci. Adv. 8, eadd0103. doi:10.1126/sciadv.add0103
Kim, S. W., Yoon, S. J., Chuong, E., Oyolu, C., Wills, A. E., Gupta, R., et al. (2011). Chromatin and transcriptional signatures for Nodal signaling during endoderm formation in hESCs. Dev. Biol. 357, 492–504. doi:10.1016/j.ydbio.2011.06.009
Lee, T. I., Jenner, R. G., Boyer, L. A., Guenther, M. G., Levine, S. S., Kumar, R. M., et al. (2006). Control of developmental regulators by Polycomb in human embryonic stem cells. Cell 125, 301–313. doi:10.1016/j.cell.2006.02.043
Leroy, G., Orphanides, G., Lane, W. S., and Reinberg, D. (1998). Requirement of RSF and FACT for transcription of chromatin templates in vitro. Science 282, 1900–1904. doi:10.1126/science.282.5395.1900
Lewis, E. B. (1978). A gene complex controlling segmentation in Drosophila. Nature 276, 565–570. doi:10.1038/276565a0
Loh, C. H., van Genesen, S., Perino, M., Bark, M. R., and Veenstra, G. J. C. (2021). Loss of PRC2 subunits primes lineage choice during exit of pluripotency. Nat. Commun. 12, 6985. doi:10.1038/s41467-021-27314-4
Loh, C. H., and Veenstra, G. J. C. (2022). The role of polycomb proteins in cell lineage commitment and embryonic development. Epigenomes 6, 23. doi:10.3390/epigenomes6030023
Loyola, A., Huang, J. Y., Leroy, G., Hu, S., Wang, Y. H., Donnelly, R. J., et al. (2003). Functional analysis of the subunits of the chromatin assembly factor RSF. Mol. Cell Biol. 23, 6759–6768. doi:10.1128/MCB.23.19.6759-6768.2003
Loyola, A., Leroy, G., Wang, Y. H., and Reinberg, D. (2001). Reconstitution of recombinant chromatin establishes a requirement for histone-tail modifications during chromatin assembly and transcription. Genes Dev. 15, 2837–2851. doi:10.1101/gad.937401
Margueron, R., and Reinberg, D. (2011). The Polycomb complex PRC2 and its mark in life. Nature 469, 343–349. doi:10.1038/nature09784
Mayor, R., and Theveneau, E. (2013). The neural crest. Development 140, 2247–2251. doi:10.1242/dev.091751
Mohammad Parast, S., and Chang, C. (2022). Ash2l, an obligatory component of H3K4 methylation complexes, regulates neural crest development. Dev. Biol. 492, 14–24. doi:10.1016/j.ydbio.2022.09.008
Moody, S. A. (2019). Analysis of cell fate commitment in Xenopus embryos. Cold Spring Harb. Protoc. 2019, 097246. doi:10.1101/pdb.top097246
Moody, S. A. (1987). Fates of the blastomeres of the 32-cell-stage Xenopus embryo. Dev. Biol. 122, 300–319. doi:10.1016/0012-1606(87)90296-x
O'Carroll, D., Erhardt, S., Pagani, M., Barton, S. C., Surani, M. A., and Jenuwein, T. (2001). The polycomb-group gene Ezh2 is required for early mouse development. Mol. Cell Biol. 21, 4330–4336. doi:10.1128/MCB.21.13.4330-4336.2001
Parker, H. J., Pushel, I., and Krumlauf, R. (2018). Coupling the roles of Hox genes to regulatory networks patterning cranial neural crest. Dev. Biol. 444 (1), S67–s78. doi:10.1016/j.ydbio.2018.03.016
Pasini, D., Bracken, A. P., Hansen, J. B., Capillo, M., and Helin, K. (2007). The polycomb group protein Suz12 is required for embryonic stem cell differentiation. Mol. Cell Biol. 27, 3769–3779. doi:10.1128/MCB.01432-06
Patel, I., and Parchem, R. J. (2022). Regulation of Oct4 in stem cells and neural crest cells. Birth Defects Res. 114, 983–1002. doi:10.1002/bdr2.2007
Piunti, A., and Shilatifard, A. (2021). The roles of Polycomb repressive complexes in mammalian development and cancer. Nat. Rev. Mol. Cell Biol. 22, 326–345. doi:10.1038/s41580-021-00341-1
Prasad, M. S., Sauka-Spengler, T., and Labonne, C. (2012). Induction of the neural crest state: Control of stem cell attributes by gene regulatory, post-transcriptional and epigenetic interactions. Dev. Biol. 366, 10–21. doi:10.1016/j.ydbio.2012.03.014
Saito, S., and Suzuki, T. (2020). How do signaling and transcription factors regulate both axis elongation and Hox gene expression along the anteroposterior axis? Dev. Growth Differ. 62, 363–375. doi:10.1111/dgd.12682
Sargent, T. D. (2006). Transcriptional regulation at the neural plate border. Adv. Exp. Med. Biol. 589, 32–44. doi:10.1007/978-0-387-46954-6_3
Schier, A. F. (2003). Nodal signaling in vertebrate development. Annu. Rev. Cell Dev. Biol. 19, 589–621. doi:10.1146/annurev.cellbio.19.041603.094522
Schuettengruber, B., Bourbon, H. M., di Croce, L., and Cavalli, G. (2017). Genome regulation by polycomb and trithorax: 70 Years and counting. Cell 171, 34–57. doi:10.1016/j.cell.2017.08.002
Soshnikova, N. (2014). Hox genes regulation in vertebrates. Dev. Dyn. 243, 49–58. doi:10.1002/dvdy.24014
Sudou, N., Yamamoto, S., Ogino, H., and Taira, M. (2012). Dynamic in vivo binding of transcription factors to cis-regulatory modules of cer and gsc in the stepwise formation of the Spemann-Mangold organizer. Development 139, 1651–1661. doi:10.1242/dev.068395
Tahir, R., Kennedy, A., Elsea, S. H., and Dickinson, A. J. (2014). Retinoic acid induced-1 (Rai1) regulates craniofacial and brain development in Xenopus. Mech. Dev. 133, 91–104. doi:10.1016/j.mod.2014.05.004
Tandon, P., Conlon, F., Furlow, J. D., and Horb, M. E. (2017). Expanding the genetic toolkit in Xenopus: Approaches and opportunities for human disease modeling. Dev. Biol. 426, 325–335. doi:10.1016/j.ydbio.2016.04.009
Thornton, S. R., Butty, V. L., Levine, S. S., and Boyer, L. A. (2014). Polycomb Repressive Complex 2 regulates lineage fidelity during embryonic stem cell differentiation. PLoS One 9, e110498. doi:10.1371/journal.pone.0110498
Tien, C. L., Mohammadparast, S., and Chang, C. (2021). Heterochromatin protein 1 beta regulates neural and neural crest development by repressing pluripotency-associated gene pou5f3.2/oct25 in Xenopus. Dev. Dyn. 250, 1113–1124. doi:10.1002/dvdy.319
Voncken, J. W., Roelen, B. A., Roefs, M., de Vries, S., Verhoeven, E., Marino, S., et al. (2003). Rnf2 (Ring1b) deficiency causes gastrulation arrest and cell cycle inhibition. Proc. Natl. Acad. Sci. U. S. A. 100, 2468–2473. doi:10.1073/pnas.0434312100
Wang, H., Wang, L., Erdjument-Bromage, H., Vidal, M., Tempst, P., Jones, R. S., et al. (2004). Role of histone H2A ubiquitination in Polycomb silencing. Nature 431, 873–878. doi:10.1038/nature02985
Wyatt, B. H., Raymond, T. O., Lansdon, L. A., Darbro, B. W., Murray, J. C., Manak, J. R., et al. (2021). Using an aquatic model, Xenopus laevis, to uncover the role of chromodomain 1 in craniofacial disorders. Genesis 59, e23394. doi:10.1002/dvg.23394
Keywords: RSF1, PRC1, H2AK119ub, UAB domain, mesoderm, neural and neural crest, development, Xenopus laevis
Citation: Parast SM, Yu D, Chen C, Dickinson AJ, Chang C and Wang H (2023) Recognition of H2AK119ub plays an important role in RSF1-regulated early Xenopus development. Front. Cell Dev. Biol. 11:1168643. doi: 10.3389/fcell.2023.1168643
Received: 17 February 2023; Accepted: 07 July 2023;
Published: 17 July 2023.
Edited by:
Alejandro Vaquero, Josep Carreras Leukaemia Research Institute (IJC), SpainReviewed by:
Puli Chandramouli Reddy, Shiv Nadar University, IndiaCopyright © 2023 Parast, Yu, Chen, Dickinson, Chang and Wang. This is an open-access article distributed under the terms of the Creative Commons Attribution License (CC BY). The use, distribution or reproduction in other forums is permitted, provided the original author(s) and the copyright owner(s) are credited and that the original publication in this journal is cited, in accordance with accepted academic practice. No use, distribution or reproduction is permitted which does not comply with these terms.
*Correspondence: Hengbin Wang, aGVuZ2Jpbi53YW5nQHZjdWhlYWx0aC5vcmc=
†These authors have contributed equally to this work
Disclaimer: All claims expressed in this article are solely those of the authors and do not necessarily represent those of their affiliated organizations, or those of the publisher, the editors and the reviewers. Any product that may be evaluated in this article or claim that may be made by its manufacturer is not guaranteed or endorsed by the publisher.
Research integrity at Frontiers
Learn more about the work of our research integrity team to safeguard the quality of each article we publish.