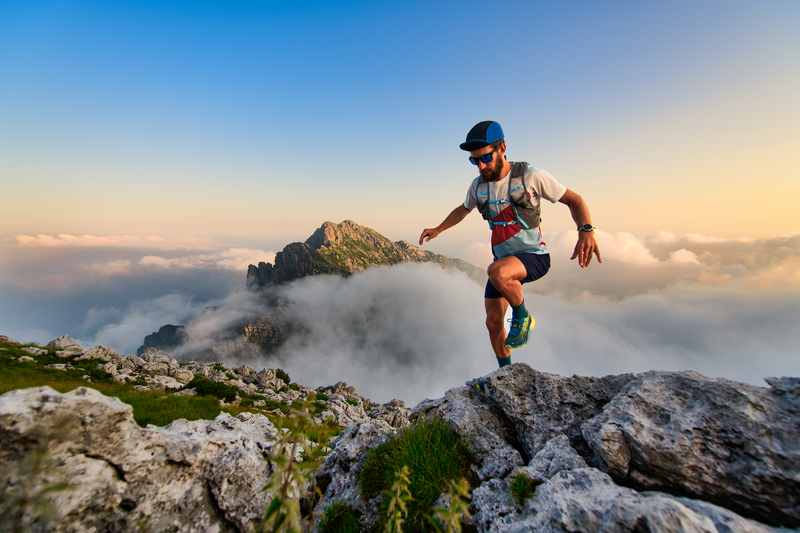
95% of researchers rate our articles as excellent or good
Learn more about the work of our research integrity team to safeguard the quality of each article we publish.
Find out more
REVIEW article
Front. Cell Dev. Biol. , 13 June 2023
Sec. Cancer Cell Biology
Volume 11 - 2023 | https://doi.org/10.3389/fcell.2023.1164301
This article is part of the Research Topic Role of non-coding RNAs in Development and Metastasis of Solid Tumors View all 5 articles
Cancer is a devastating disease and the primary cause of morbidity and mortality worldwide, with cancer metastasis responsible for 90% of cancer-related deaths. Cancer metastasis is a multistep process characterized by spreading of cancer cells from the primary tumor and acquiring molecular and phenotypic changes that enable them to expand and colonize in distant organs. Despite recent advancements, the underlying molecular mechanism(s) of cancer metastasis is limited and requires further exploration. In addition to genetic alterations, epigenetic changes have been demonstrated to play an important role in the development of cancer metastasis. Long non-coding RNAs (lncRNAs) are considered one of the most critical epigenetic regulators. By regulating signaling pathways and acting as decoys, guides, and scaffolds, they modulate key molecules in every step of cancer metastasis such as dissemination of carcinoma cells, intravascular transit, and metastatic colonization. Gaining a good knowledge of the detailed molecular basis underlying lncRNAs regulating cancer metastasis may provide previously unknown therapeutic and diagnostic lncRNAs for patients with metastatic disease. In this review, we concentrate on the molecular mechanisms underlying lncRNAs in the regulation of cancer metastasis, the cross-talk with metabolic reprogramming, modulating cancer cell anoikis resistance, influencing metastatic microenvironment, and the interaction with pre-metastatic niche formation. In addition, we also discuss the clinical utility and therapeutic potential of lncRNAs for cancer treatment. Finally, we also represent areas for future research in this rapidly developing field.
Cancer poses a significant threat to improving life expectancy as it remains one of the leading causes of global deaths (Bray et al., 2021). The preliminary data show that it is the leading cause of mortality in people before the age of 70 years in most countries worldwide. Since the last decade, cancer incidence and mortality rates have risen sharply around the globe with 19.3 million new cases and 10 million cancer deaths worldwide in 2020 (Sung et al., 2021). It is estimated that more than 1,670 people will die of cancer every day in the United States by 2023 (Siegel et al., 2023).
Metastasis, the spread of cancer cells from the primary site to distant organs, is a complex and multi-stage process that is the leading cause of cancer-related deaths. This process involves several key stages, which include local invasion, in which cancer cells invade surrounding tissues, and intravasation, in which cancer cells enter the bloodstream (Hanahan and Weinberg, 2011). The cancer cells then travel to distant sites, where they undergo extravasation into the surrounding tissues and form micro-metastatic colonies. Finally, these colonies can proliferate and become clinically identifiable macro-metastases, referred to as “cancer colonization” (Talmadge and Fidler, 2010; Lambert et al., 2017; Klein, 2020). The molecular mechanisms underlying metastasis are intricate and not yet fully understood, but research into this issue continues to evolve. This complexity highlights the need for new perspectives and approaches in the study of metastasis in order to better understand and ultimately combat this devastating aspect of cancer.
Ribonucleic acids (RNAs) play a critical role in the origin of life due to their unique properties as both catalysts and genetic materials (Higgs and Lehman, 2015). The “RNA world hypothesis” posits that early life on Earth was characterized by self-replicating RNA molecules before the evolution of proteins and DNA (Mercer et al., 2009). Despite the fact that only 2% of human RNA transcripts are translated into proteins, the remaining transcripts have been shown to play important regulatory functions through recent advancements in genomic technologies (Djebali et al., 2012). These transcripts, which do not code for proteins, are known as non-coding RNAs (ncRNAs) (Jarroux et al., 2017) and are divided into two main categories: regulatory and structural ncRNAs. Structural ncRNAs include ribosomal RNA (rRNA) and transfer RNA (tRNA), while regulatory ncRNAs are further divided into small non-coding RNAs (sncRNAs) and long non-coding RNAs (lncRNAs) based on their size (Esteller, 2011; Quinn and Chang, 2016). The most well-studied classes of sncRNAs are piwi-interacting RNAs (piRNAs), micro-RNAs (miRNAs), and endogenous small interfering RNAs (siRNAs) (Naqvi et al., 2009). These small non-coding RNAs play critical roles in the regulation of gene expression, which includes the repression of unwanted transcripts and the modulation of mRNA stability. Further research into the role of ncRNAs, particularly sncRNAs, in the regulation of gene expression holds the potential to reveal new insights into the biology of cells and pathogenesis of diseases.
Long non-coding RNAs (lncRNAs) are RNA transcripts longer than 200 nucleotides without the potential to code for proteins (Ming et al., 2021). These lncRNAs are usually located in the nucleus and are found in different nuclear compartments such as the chromatin and nucleoplasm. They play important roles in modulating nuclear organization and function (Yao et al., 2019). Some lncRNAs can be found in the cytoplasm (Wang and Chang, 2011; Chen, 2016). Based on their relative position to their proximate protein-coding genes (PCGs), lncRNAs are classified (Harrow et al., 2012; Hrdlickova et al., 2014) as (a) sense lncRNAs, which are within the PCG spanning multiple introns or exons; (b) antisense lncRNAs, which are transcribed from the opposite strand of a PCG; (c) intronic lncRNAs are found in the sense strand of an intron of a coding gene; (d) intergenic lncRNAs, which are transcripts located between two PCGs; and (e) bidirectional lncRNAs that are located on the opposite strand but within 1 kb of the promoter on the sense strand and are transcribed on the sense strand in the opposite direction to that of the promoter (Bär et al., 2016). The biological functions of lncRNAs can be classified into several categories, such as guides, scaffolds, decoys, and platforms (Wong et al., 2018). In addition, the expression of other genes is usually modulated by signals from lncRNAs (Grossi et al., 2020). As guides, lncRNAs can direct regulatory proteins such as transcription factors and epigenetic regulators to specific regions of the genome. As decoys, lncRNAs can modulate gene expression by binding with miRNAs, thereby blocking their ability to regulate target genes (Zhang et al., 2018). As scaffolds, lncRNAs can provide a platform for the assembly of protein complexes involved in various cellular processes (Zhang et al., 2019a). Finally, as platforms, lncRNAs can recruit various molecules through binding with different structural domains (Düzgün Ş et al., 2017). These various roles of lncRNAs highlight their importance in regulating gene expression and cellular processes, particularly in cancer.
For many years, researchers have suspected that lncRNAs could play a role in cancer but lacked concrete evidence to support this hypothesis (Rainier et al., 1993). However, with advancements in cancer transcriptome profiling and accumulating evidence that support lncRNA function, a number of differentially expressed lncRNAs have now been associated with cancer (Gibb et al., 2011). Importantly, several lncRNAs have been identified to be involved in different stages of the metastatic cascade, which includes invasion, intravasation, and extravasation. For example, the metastasis-associated lung adenocarcinoma transcript 1 (MALAT1) lncRNA was first identified as having a potential role in cancer during a comparative screening of non–small-cell lung cancer patients with and without metastatic tumors (Ji et al., 2003). This lncRNA is widely expressed in normal tissues in human (Ji et al., 2003; Hutchinson et al., 2007) and is found to be upregulated in a variety of cancers of the breast, prostate, colon, liver, and uterus in humans (Luo et al., 2006; Yamada et al., 2006; Lin et al., 2007; Guffanti et al., 2009). MALAT1 has been shown to interact with the protein SFPQ to promote the invasion and migration of colorectal cancer cells (Ji et al., 2014). One way in which lncRNAs contribute to metastasis is by regulating the expression of genes that are involved in metastatic processes. For example, the lncRNA HOX antisense intergenic RNA (HOTAIR), a 2.2-kb gene located in the mammalian HOXC locus on chromosome 12q13.13 (Rinn et al., 2007), has been shown to promote metastasis in breast cancer (BC) by repressing the expression of genes involved in cell adhesion and promoting the expression of genes involved in cell migration and invasion (Abba et al., 2021). This lncRNA was found to be highly upregulated in metastatic breast tumors, demonstrating up to 2000 times increased transcription over normal BC (Gupta et al., 2010). High levels of HOTAIR expression were found to be correlated with both metastasis and poor survival rate, linking this lncRNA with cancer invasiveness and patient prognosis (Gupta et al., 2010). lncRNAs also play an important role in regulating the epithelial–mesenchymal transition (EMT) process, which is crucial for the migration of cancer cells from the primary site to other parts of the body (Nieto et al., 2016; Brabletz et al., 2018; Nisar et al., 2021a). lncRNAs have been found to regulate important signaling pathways involved in EMT and are therefore considered key regulators of tumor metastasis (Guo et al., 2014; Jia et al., 2016; Heery et al., 2017). In particular, lncRNA H19, upregulated in a number of human cancers, such as in hepatocellular, bladder, and breast carcinomas (Berteaux et al., 2005; Barsyte-Lovejoy et al., 2006; Matouk et al., 2007), has been implicated in inducing EMT and cancer metastasis. This lncRNA has been shown to promote EMT in hepatocellular carcinoma by upregulating the expression of the transcription factor Snail (Wen et al., 2020; Yuan et al., 2021). In addition to these, multiple cancer–associated lncRNAs has been shown to regulate cancer invasion and metastasis (Raveh et al., 2015; Luo et al., 2018; Tang et al., 2018). Overall, many studies have suggested that lncRNAs are important regulators of cancer metastasis (Hunter et al., 2018; Slack and Chinnaiyan, 2019; Williams et al., 2019; Li et al., 2020a; Qin et al., 2020) and therefore serve as important therapeutic targets and disease biomarkers (Liu et al., 2021).
Metabolic reprogramming is a hallmark of cancer (Hanahan and Weinberg, 2011), which involves alterations in cellular metabolism to support the energy and biosynthetic demands of rapidly dividing cancer cells. Recent studies have shown that lncRNAs can modulate metabolic pathways and contribute to the metabolic reprogramming that occurs in cancer cells during metastasis. lncRNAs are involved in regulating the interplay between molecular signaling and metabolic reprogramming (Sellitto et al., 2021). This regulation is achieved through changes in cell metabolic processes during different stages of metastasis and by providing energy and essential metabolites for the continuous growth and proliferation of cancer cells (Phan et al., 2014). One example is the lncRNA MALAT1, which has been shown to promote metabolic reprogramming in BC cells by regulating the expression of genes involved in glycolysis and oxidative phosphorylation (Agostini et al., 2022). MALAT1 also enhances the Warburg effect by increasing lactate production and decreasing mitochondrial respiration, which in turn promotes the migration and invasion of multiple myeloma cells (Liu et al., 2020a). In addition, H19 has been shown to promote glycolysis in gastric cancer (GC) (Sun et al., 2021) and oral cancer (Yang et al., 2021) cells, thereby enhancing the Warburg effect and promoting the metastatic potential.
The tumor microenvironment (TME) regulates important tumor promotion and survival functions. Through a dynamic and multistep metastatic cascade, communication between the structural and cellular components of the TME permits cancer cells to disseminate from the primary site to distant areas and become invasive (Neophytou et al., 2021). On arrival at distant organ sites, metastatic cells form and interact with the TME, involving numerous processes like angiogenesis (De Palma et al., 2017), suppression and/or co-option of the innate and adaptive immune system (Kitamura et al., 2015; Karki and Kanneganti, 2019; Huntington et al., 2020), and the reprogramming of stromal populations to enable metastatic outgrowth (Peinado et al., 2017; Zanconato et al., 2019). The development of metastatic colonies depends on establishing and maintaining a supportive microenvironment, which includes innate and adaptive immune cells and resident stromal cells (Quail and Joyce, 2013; Lambert et al., 2017). In addition, the cellular and extracellular components of the metastatic microenvironment are crucial for metastatic colonization (Lin et al., 2018a; Altorki et al., 2019). Several facts have suggested that lncRNAs have a major influence on the TME (Fatima and Nawaz, 2017; Lin et al., 2018a; Sun et al., 2018a). Tumor cells after detachment from their primary site and traveling via the lymphatic and circulatory systems possess the potential to resist cell death, i.e., “anoikis”. The anoikis resistance (AR) is a cornerstone step for metastasis, promoting secondary tumor formation in distal organs (Simpson et al., 2008). Extensive studies have shown that lncRNAs can regulate the AR of cancer cells through modulating pathways, apoptosis-associated proteins, and other molecules (Lee et al., 2021). Primary tumors can influence the microenvironment of distant organs to create a pre-metastatic niche, which can facilitate the spread and colonization of cancer cells. This is achieved through changes in various factors such as inflammation, lymph angiogenesis, immunosuppression, angiogenesis, vascular permeability, and organotropism. These changes create a supportive environment for cancer cells to thrive and establish secondary tumors (Guo et al., 2019), which are important for metastasis development (Nogués et al., 2018). It is well established that lncRNAs play a crucial role in the regulation of tumor progression and can contribute to the development of metastasis. Imbalances of lncRNAs have been shown to be involved in the release of exosomes, alteration of remote tumor cells, creation of a pre-metastatic niche, and survival of disseminated tumor cells (Kim et al., 2016; Liu et al., 2019a). Many studies have also demonstrated that lncRNAs can act as mediators of both intra- and extracellular signaling pathways that drive metastasis, making the targeting of lncRNAs a potential strategy for treating metastatic cancer.
In this review article, the functions of lncRNAs in the development of metastasis will be discussed, such as their involvement in underlying molecular mechanisms, metabolic reprogramming, EMT programming, TME influence, AR, and interaction with pre-metastatic niche formation (Figure 1). The diagnostic, prognostic, and therapeutic potential of lncRNAs in cancers will also be examined. Further research in this field is necessary to gain a comprehensive understanding of the molecular basis of lncRNA-modulated cancer metastasis and develop new diagnostic and therapeutic approaches for patients with metastatic disease. Furthermore, we represented areas for future research in this rapidly developing field.
FIGURE 1. Mechanism of long non-coding RNAs (lncRNAs) in cancer metastasis. lncRNAs play a role in cancer development and growth by regulating various processes such as metabolic reprogramming, anoikis resistance, EMT, metastatic niche, immune escape, and oncogenes or tumor suppressors.
Otto Warburg in the 1920s identified cancer cells favoring glycolysis over the considerably more effective oxidative phosphorylation (OXPHOS) to generate energy (Warburg, 1956; Vander Heiden et al., 2009), resulting in considerably increased secretion of lactate and glucose uptake in tumor cells (Dang, 2010). This process is described as the Warburg effect, also known as aerobic glycolysis, and represents a new phase in the research of tumor metabolism. Deletions, gene mutations, and translocations impact cancer cells in various signaling pathways and mainly converges at the metabolism level (Cairns et al., 2011; Beloribi-Djefaflia et al., 2016). Cancer cell metabolites not only provide materials for metastasis and for their proliferation but also supply sustaining signals in tumor-specific microenvironments to meet their survival needs (Hanahan and Weinberg, 2011; Alderton, 2014; Weber, 2016). Moreover, cancer cells also perturb the metabolism of distant organs in order to disseminate and ease their growth and implantation (Fong et al., 2015). In turn, metabolic alterations of invaded tissues and organs also affect the survival and growth of carcinoma cells (Mashimo et al., 2014; Loo et al., 2015). Many researchers have recognized that the Warburg effect facilitates inhibition of anoikis (AR) and spread of tumor cells (Lu et al., 2015; Lu, 2019). This ability of tumor cells to resist anoikis (AR) and metastatic spread is provided by aerobic glycolysis, which accelerates glucose consumption, decreases the production of reactive oxygen species (ROS), and enhances the anti-oxidant capacity of tumor cells (Lu et al., 2015). However, despite the Warburg effect, oxidative metabolism is still a significant source of ATP in some cancers (Guppy et al., 2002; Marin-Valencia et al., 2012). Oxidative metabolism in mitochondria can generate reactive ROS that include hydroxyl radicals (HO−), hydrogen peroxide (H2O2), and superoxide (O−2) (Katritsis et al., 1991). These ROS have been shown to play a role in promoting tumor spread by preventing the death of detached cancer cells (anoikis) (Moloney and Cotter, 2018). A growing body of evidence has shown a connection between ncRNAs, particularly miRNA and lncRNA, and metabolic alterations in cancer (Singh et al., 2012; Sun et al., 2018b). lncRNAs regulate the signaling pathways in key metabolic reprogramming and promote cancer progression, tumorigenesis, and metastasis. Despite reduced glucose levels in solid tumors, enhanced glycolysis produces metabolic intermediate that serves as crucial synthetic components for developing tumors (DeBerardinis et al., 2008) and promotes metastasis (Warburg, 1956; Vander Heiden et al., 2009). More specifically, glycolysis promotes acidic and hypoxic TME associated with the protonation of significant pH-sensitive protein residues (Magalhaes et al., 2011; Choi et al., 2013) and regulates the function and sub-cellular localization of cytoskeleton proteins important for invasion and immune escape (Huber et al., 2017; Rohani et al., 2019). For example, in tumor tissue hexokinases (HKs), the essential glycolytic enzyme has been shown to keep a rapid rate of glycolysis and help cancer cell metastasis (Zhang et al., 2017a). More specifically, HK2 by regulating the matrix metalloproteinase 9 (MMP-9) expression, non-processed pseudogene (NANOG), and SRY box transcription factor (SOX)-9 facilitates the metastasis of ovarian cancer (OVC) cells (Siu et al., 2019). Interestingly, knocking down (KD) of ncRNA-TUG1 and suppressing the miR-455-3p expression leads to decreased activity of adenosine monophosphate–activated protein kinase subunit b2 (AMPKb2), which in turn affects HK2 and reduces the migration and invasion of hepatocellular carcinoma (HCC) cells (Lin et al., 2018b). In gallbladder cancer (GBC) tissues, increased expression of lncRNA PVT1 was negatively correlated with the overall survival (OS) of patients (Chen et al., 2019). Furthermore, KD of lncRNA PVT1 in GBC cells decreased HK2 expression, inhibited glycolysis, and decreased metastases via competitive interaction with endogenous miR-143 (Chen et al., 2019).
The lncRNA SAMMSON and MALAT1 have been shown to play roles in the development of melanoma (Leucci et al., 2016) and HCC (Malakar et al., 2019), respectively. SAMMSON interacts with protein p32 to increase its pro-oncogenic function and enhance mitochondrial targeting (Leucci et al., 2016). MALAT1 contributes to HCC by modulating glucose metabolism and enhancing glycolysis while inhibiting gluconeogenesis. It does so by activating the mTORC1-4EBP1 axis, leading to increased translation of the transcription factor TCF7L2 (Malakar et al., 2019). Similarly, lncRNA MACC1-AS1 overexpression is associated with metastasis of gastric cancer (GC) cells to the lungs. The underlying mechanisms include the activation of AMPK/Lin28 pathway–mediated increased glycolysis and anti-oxidative abilities with strong metabolic plasticity (Zhao et al., 2018). lncRNA MACC1-AS1 in pancreatic cancer (PC) is also upregulated and associated with bad prognosis (Qi et al., 2019), its KD prevents the metastasis of PC cells by promoting the expression of paired-box gene 8 (PAX8), which is essential in activating NOTCH 1 signaling and enhancing cell aerobic glycolysis (Qi et al., 2019). An alternate spliced form of PK is pyruvate kinase M2 (PKM2), which is overexpressed in different types of cancerous cells. PKM2 regulates the final rate-limiting step of glycolysis and determines the efficiency of lactic acid production and glucose utilization (Chaneton and Gottlieb, 2012). Histone deacetylase 3 is recruited by direct interaction of transforming growth factor β (TGF-β)–induced factor homeobox 2 (TGIF2) in the nucleus with PKM2 and subsequent deacetylation to the E-cadherin promoter, thus suppressing E-cadherin transcription in colon cancer cells and promoting EMT (Hamabe et al., 2014). lncRNA FEZ finger zinc 1 antisense 1 (lncRNA FEZF1-AS1) is typically overexpressed in colorectal cancers (CRC) that leads to cell metastasis. Mechanistically, PKM2 is stabilized by the binding of FEZF1-AS1 to it, increases aerobic glycolysis, and stimulates the signal transducer and activator of transcription 3 (STAT3) signaling pathway (Bian et al., 2018). Transmembrane glycoprotein known as glucose transporter (GLUT) is an essential factor in the uptake of glucose by cancer cells. A high expression of GLUT1 increases glucose absorption, promoting glycolysis and cancer cell metastasis (Nagarajan et al., 2017). The gene expression of GLUT1 is correlated with the activity of MMP-2 and invasiveness in PC (Ito et al., 2004). lnc-p23154 regulates glycolysis by inhibiting the transcription of miR-378a-3p, resulting in increased expression of GLUT1, which contributes to the development of oral squamous cell carcinoma (OSCC) metastasis (Wang et al., 2018a). Lactate dehydrogenase A (LDHA) is the rate-limiting enzyme in the metabolic pathway that converts glucose into lactate and pyruvate. LDHA is an important enzyme that regulates the balance between energy production and energy utilization in the cell, and its activity has been shown to be altered in various diseases such as cancer. The progression of breast cancer (BC) metastasis is positively correlated with the phosphorylation of enzyme LDHA at Y10 (Jin et al., 2017). LDHA induces EMT and facilitates the metastasis of lung adenocarcinoma cells (LADCs) (Hou et al., 2019). In addition, many other kinases and glycolytic enzymes involved in glycolysis are also regulated by lncRNAs. For example, LINC00092 suppresses the enzyme 6-phosphofructo-2-kinase/fructose-2,6-biphosphatase 2 (PFKFB2), which is involved in the synthesis and breakdown of fructose-2,6-bisphosphate (Zhao et al., 2017). It is important to mention that chemokine-CXCL14 (C-X-C motif ligand 14) is overexpressed in cancer-associated fibroblasts (CAFs) in OVC and induces LINC00092 expression. For cancerous cells, a non-essential amino acid glutamine has vital biological importance with enhanced glutamine uptake and catabolism. Glutamate dehydrogenase (GDH) catalyzes the conversion of glutamine into glutamate, which is triggered by an enzyme glutaminase (GLS), and results in the production of α-ketoglutarate, an intermediary for the TCA cycle and an important source of energy. Glutamine has been demonstrated to increase hypoxia-inducible factor-1 (HIF1) expression and also increase the pro-autophagic function of BNIP3 (Bcl2/adenovirus E1B interacting protein 3), thus encouraging melanoma cell dissemination (Vara-Perez et al., 2019). Fumarate, an intermediate product of glutamine metabolism, induce metastasis by activating glutathione peroxidase, and reducing the ROS levels (Jin et al., 2015). The c-Myc protein is a transcription factor that plays a role in the regulation of gene expression and cell growth. One mechanism by which c-Myc contributes to cancer is by upregulating the expression of miR-23b, which in turn suppresses the expression of proline oxidase and leads to an increase in glutamine catabolism to promote cell proliferation and contribute to the development of cancer (Liu et al., 2012). Interestingly, lncRNA GLS-AS has been shown to regulate glutaminase and c-Myc feedback loop, thus contributing to the metastasis of PC cells (Deng et al., 2019). In bladder cancer (BDC), lncRNA UCA1 and GLS2 are positively correlated. UCA1 has a positive effect on human BDC cells by reducing ROS production and promoting mitochondrial glutaminolysis, as well as having sponge effects on miR-16. The binding of miR-16 “seed region” to the 3′-UTR of GLS2 mRNA regulates GLS2 expression (Li et al., 2015a). Similarly, lncRNA OIP5-AS1 is upregulated in melanoma by sponge miR-217, which leads to increased GLS expression and promotion of glutamine catabolism and melanoma growth (Luan et al., 2019). For maintaining cellular energy homeostasis, 5′ adenosine monophosphate–activated protein kinase (AMPK) is an essential detector. AMPK phosphorylation of sterol regulatory element binding protein-1 (SREBP1) and acetyl-CoA carboxylase (ACC1) restricts the production of cholesterol, fatty acids, and triglycerides while promoting the uptake of fatty acids. Moreover, AMPK also induces glycolysis by activating the phosphorylation of glycogen phosphorylase and PFKFB3 (Hardie et al., 2012). However, reduced AMPK activity is associated with increased fatty acid synthesis and cancer cell growth (Kim et al., 2011). Tumor suppressor liver kinase B1 (LKB1), an upstream kinase of AMPK, phosphorylates and activates AMPK during ATP-depleted conditions (Hezel and Bardeesy, 2008). Under the TME, activation of the LKB1-AMPK signaling pathway inhibits autophagy via miR-7 upregulation and decreases intracellular glucose supply, coupled with decreased proliferation and metastasis of PC cells (Gu et al., 2017). In HCC cells, taurine-upregulated gene 1 (TUG1) through the miR-455-3p/AMPKb2 axis upregulates the HK2 expression, promotes glycolysis, and induces metastasis (Lin et al., 2018b). Similar to AMPK, the hyperactivation of the PI3K/AKT/mTOR signaling pathway promotes cancer proliferation, growth, invasion, and metastasis (Jeong et al., 2014; Hua et al., 2018). LINC00963 promotes metastasis in non–small-cell lung cancer (NSCLC) by stimulating the AKT/mTOR pathway and by inhibiting the ubiquitination of phosphoglycerate kinase 1 (PGK1) (Yu et al., 2017). Likewise, the high expression of the long non-coding RNA MACC1-AS1 has been linked to the spread of GC cells to the lungs. This is due to the activation of the AMPK/Lin28 pathway, which enhances glycolysis and anti-oxidative capacity, resulting in improved metabolic adaptability (Zhao et al., 2018). In PC, lncRNA MACC1-AS1 is overexpressed and associated with poor prognosis (Qi et al., 2019). KD MACC1-AS1 has been shown to inhibit metastasis of PC cells by increasing the expression of paired-box gene 8 (PAX8), which is crucial for activating NOTCH 1 signaling and improving aerobic glycolysis of the cells (Qi et al., 2019). Furthermore, in HCC, lncRNA HULC is highly overexpressed and acts as an oncogene. It modulates lipid metabolism by involving miR-9, PPARA, and ACSL1 in a signaling pathway, strengthened by a feed-forward mechanism involving cholesterol and RXRA to drive HULC signaling (Cui et al., 2015) (Figure 2, Table 1).
FIGURE 2. Long non-coding RNAs (lncRNAs) by modulating metabolic reprogramming participate in tumor metastasis. lncRNAs such as PVT1, FEZF1-AS1, LINC00092, LINC00963, MACC1-AS1, HULC, GLS-AS, lnc-p23154, lnc-IGFBP4-1, TUG1, UCA1, OP15-AS1, and XLOC-006390 have been shown to modulate metabolic pathways such as glycolysis, lipid metabolism, and glutamine metabolism, leading to tumor metastasis in different organs.
EMT is an essential part in the cell dissemination process that enables cancer cells to leave the original site and migrate to distant regions (Nieto et al., 2016; Brabletz et al., 2018). EMT can be activated by numerous intracellular signaling pathways that are activated once the ligands that originate from the stroma bind to the appropriate receptors that are expressed in neoplastic cells (Jiang et al., 2017a; Dongre and Weinberg, 2019). Recent research have demonstrated that lncRNAs are significant EMT modulators in tumor metastasis (Guo et al., 2014; Jia et al., 2016; Heery et al., 2017). Accumulated evidence has demonstrated that NOTCH, TGF-β/SMAD, PI3K/AKT, Wnt/β-catenin, JAK/STAT, and MEK/ERK are responsible for inducing the EMT-activating transcription factors (EMT-TFs) expression, particularly SNAIL, TWIST, and ZEB, which simultaneously prevent the epithelial state–related gene expression and enhance the expression of genes linked to the mesenchymal state (Xu et al., 2009; Bray, 2016; Nieto et al., 2016; Yang et al., 2017; Browning et al., 2018; Cevenini et al., 2018; Tauriello et al., 2018; Dongre and Weinberg, 2019; Su et al., 2020). lncRNAs have been found to play a significant role in regulating the TGF-β signaling pathway in cancers (Wang et al., 2016). LINC00978 (also known as AK001796 and MIR4435-2HG) promotes EMT in GC (Fu et al., 2018). KD of LINC00978 inactivates SMAD2 and reduces TGF-β expression, leading to decreased expression of MMP-9, SNAIL2, and TWIST1 genes (Fu et al., 2018). Likewise, lncRNA TUG1 induces EMT in PC by controlling the TGF-β signaling pathway. It decreases SMAD4 expression and increases TGF-β and TGF-β receptor expressions, leading to the induction of EMT (Qin and Zhao, 2017). Similarly, lncRNA actin filament–associated protein-1 antisense RNA1 (lncRNA AFAP1-AS1) modulates the expression of several EMT-related genes (SLUG, SNAIL1, VIM, CADN, ZEB, and TWIST) in tongue squamous cell carcinoma (TSCC) by controlling the Wnt/β-catenin signaling pathway (Wang et al., 2018b). Additionally, the polycomb repressive complex 2 (PRC2) and lncRNA HOTAIR regulate WIF-1 expression by increasing H3K27 methylation in the promoter region, activating the Wnt/β-catenin signaling pathway in esophageal squamous cell carcinoma (ESCC). This activation is confirmed by the overexpression of downstream genes such as MMP-13, ZEB1, and SNAIL (Ge et al., 2013). NOTCH is a well-known oncogene involved in tumor cell proliferation, AR, and EMT in many cancers (Shao et al., 2015; Wieland et al., 2017; Jackstadt et al., 2019). lncRNAs have been shown to directly bind to the NOTCH pathway’s core components and regulate many EMT-TFs. For example, lncRNA hepatocyte nuclear factor-1 alpha antisense RNA 1 (lncRNA HNF1A-AS1) promotes EMT in oral squamous cell carcinoma (OSCC) by upregulating the NOTCH 1 and Hes-1 expression (Liu et al., 2019b). In addition, lncRNAs can also act as competitive endogenous RNAs (ceRNAs), indirectly influencing NOTCH signaling by working with the TGF-β pathway to advance EMT. By sponging miR-124, the lncRNA urothelial cancer–associated 1 (lncRNA UCA1) regulates the TGF-β1 expression to upregulate JAG1 and activate NOTCH signaling in tongue cancer (TC) cells (Zhang et al., 2019b).
All these studies conclusively establish that lncRNAs enhance EMT through the upregulation of the canonical Wnt/β-catenin and the activation of the NOTCH pathways. Multiple intercellular signaling pathways, such as PI3K/AKT/mTOR, are activated to induce EMT (Grotegut et al., 2006; Di Domenico and Giordano, 2017), and lncRNAs have been shown to regulate these intercellular signaling pathways in cancer metastasis. Specifically, lncRNA UCA1 positively modulates the PI3K/AKT/mTOR signaling pathway by sponging miR-582 and activating the target protein CAMP responsive element binding protein 1 (CREB1), inducing EMT in osteosarcoma cells (Ma et al., 2019). lncRNA TTN-AS1 activates the PI3K/AKT/mTOR signaling pathway by sponging miR-497 and promoting metastasis of colorectal cancer cells. lncRNA HOXA-AS3 regulates the MEK/ERK signaling pathway by targeting miR-29c and controlling BMP1 expression, which facilitates EMT in HCC (Cui et al., 2019a) (Figure 3). Further research is required to fully understand the molecular mechanisms of lncRNA regulation of signaling pathways in cancer metastasis.
FIGURE 3. Schematics showing pathways modulated by lncRNAs to induce EMT. (A) Interaction of TGF-β ligands with T-βR triggers the canonical TGF-pathway, leading to the formation of trimeric SMAD complexes (SMAD2–SMAD3–SMAD4) which act as transcription factors in the nucleus to regulate the expression of EMT-associated genes. lncRNAs, such as LINC00978 and TUG1, can modulate the TGF-β/SMAD signaling transduction by affecting the expression and phosphorylation of SMAD2/3 and SMAD4, respectively. (B) Interaction of Wnt ligands with frizzled receptors triggers the canonical Wnt pathway, leading to the release of β-catenin which binds with LEF and TCF to promote the expression of EMT-related genes. lncRNAs AFAP1-AS1 and HOTAIR modulate the Wnt/β-catenin pathway by affecting the phosphorylation of GSK3β and methylation of H3K27, respectively. (C) Interaction of Jagged/Delta-like ligands with Notch receptors triggers the canonical Notch pathway, leading to the generation of NICD which acts as a transcriptional co-activator in the nucleus. lncRNAs HNF1A-AS1 and UCA1 modulate the Notch pathway by regulating the expression of essential components and acting as ceRNA to regulate Notch signaling indirectly. (D) Growth factors activating the MEK-ERK and PI3K-AKT pathways also induce EMT through simultaneous activation of EMT-TFs. lncRNAs, such as UCA1 and HOXA-AS3, can act as ceRNA to regulate the expression of CREB1 and miR-29c, by promoting PIK3/AKT/mTOR pathway, and enhance the phosphorylation of MEK and ERK, respectively.
The TME comprises of the extracellular matrix (ECM), basement membrane (BM), tumor-infiltrating immune cells, neuroendocrine cells, endothelial cells, adipose cells, cancer-associated fibroblasts (CAFs), pericytes, cancer stem cells (CSCs), cytokines, and a plethora of signaling molecules that modulate tumor progression (Macha et al., 2020; Bhat et al., 2021a; Bhat et al., 2021b; Nisar et al., 2021b; Mehraj et al., 2021; Neophytou et al., 2021; Bhat et al., 2022). The interactions between tumor cells and their microenvironment are very critical for cell survival, and therefore impact the development and growth of tumors (Gajewski et al., 2013; Bhat et al., 2021a; Bhat et al., 2021b; Nisar et al., 2021b; Bhat et al., 2022). It also plays a crucial role in cancer metastasis by providing the necessary support and signals for tumor survival, growth, and migration to new sites in the body. lncRNAs are essential players in the crosstalk between cancer cells and the TME. Numerous studies have demonstrated that lncRNAs promote the formation of an immunosuppressive tumor immune microenvironment (TIME), which contributes to tumor escape from immune surveillance, promoting metastatic development and therapeutic resistance (Pei et al., 2018; Wei et al., 2019a). Neutrophils are immune cells that can exhibit different polarization states, such as the tumor-promoting N2 type and the anti-tumor N1 type, which are influenced by the TME. N2-type neutrophils promote tumors by increasing angiogenesis, facilitating tumor cell infiltration and ECM reconstruction, inhibiting T-cell activation, and inducing anti-inflammatory M2 macrophages (Guo et al., 2022). KD of LINC01116 in CAFs leads to the generation of tumor-associated neutrophils (TANs), which promote tumor growth through the secretion of cytokines (Wang et al., 2020). The lncRNA Morrbid regulates neutrophil lifespan and apoptosis, making it a potential therapeutic target in the TIME (Coffelt et al., 2016; Kotzin et al., 2016). In OVC cells, the lncRNA HOTTIP enhances immunosuppression by increasing PD-L1 expression in the neutrophils and upregulating IL-6 expression (Shang et al., 2019).
Similar to neutrophils, macrophages are immune cells that play a crucial role in both innate and adaptive immune responses. The two main phenotypes, M1 and M2, have distinct functions in the body. The M1-type macrophage prevents pathogen invasion and destroys tumor cells while M2 macrophages predominantly enhance metastasis, invasion, and tumor development. These specific M2 macrophages are known as tumor-associated macrophages (TAMs) by exerting immunosuppressive and tumor-promoting effects (Ruffell et al., 2012; DeNardo and Ruffell, 2019). M2 macrophages have anti-inflammatory characteristics and facilitate the formation of tumors, by promoting angiogenesis, tumor cell infiltration, tumor cell proliferation, and metastasis, and suppressing immune function and chemotherapy resistance (Murdoch et al., 2008; De Palma and Lewis, 2013; Shu and Cheng, 2020). The expression of lncRNAs in the macrophages also impacts macrophage recruitment, tumor development, and progression, affecting factors such as invasion, metastasis, and vascularization. Calcium (Ca2+)-dependent signaling and Ca2+ flux play significant roles in the development and progression of tumors. In response to hypoxia-induced Ca2+ influx, lncRNA calcium-dependent kinase activation (lncRNA CamK-A) activates NF-κB by degrading IκB to upregulate the expressions of IL6, IL8, and VEGF in BCs, promoting angiogenesis and macrophage recruitment in patient-derived BC xenografts (Sang et al., 2018) (Figure 4). The expression of FOXO1 can be targeted and inhibited by lncRNA ANCR, which promotes M2-macrophage polarization, and thus enhances tumor cell migration and invasion (Xie et al., 2020). TAMs, which have similar effects to M2-macrophages, can facilitate tumor development and angiogenesis through lncRNA RP11-361F15.2, which acts as a ceRNA and sponges miR-30c-5p, activating and binding CPEB4 and enhancing the progression and metastasis of osteosarcoma (Yang et al., 2020). The endothelial cells play a crucial role in supporting blood vessel formation and tumor neovasculature. Tumor-associated endothelial cells display a high expression of TGF-β1 and CD105, and TGF-β1 acts as a chemoattractant for CD105-expressing endothelial cells, promoting angiogenesis (Benetti et al., 2008). In vitro studies have shown that KD of taurine upregulated gene 1 (TUG1) leads to remarkable suppression of tumor-induced endothelial cell proliferation, migration, and angiogenesis (Dong et al., 2016). Cytokines such as TNF-α, IFN, and IL-17 are major target molecules in various inflammatory conditions, with targeted therapies already in clinical use (Budhu and Wang, 2006; Rider et al., 2016; Yu et al., 2018). TGF-β plays a complex role in tumor progression (Fabregat and Caballero-Díaz, 2018; Marquardt, 2018), and the lncRNA ATB is induced by TGF-β1 (Yuan et al., 2014). In HCC specimens, lncRNA ATB was found to be overexpressed, and it enhanced EMT and metastasis by increasing the colonization of migrating cells via the IL-11/STAT3 signaling pathway. The ECM is produced by stromal cells in the microenvironment and its components, which include laminin, collagens, fibronectin, and proteoglycans, are associated with altering the phenotype and function of HCC cells. ECM production and reorganization can promote tumor cell proliferation and invasion, alter gene expression in different stromal and cancer cell types, and lead to tumor progression (Frantz et al., 2010). Extracellular proteinases, such as matrix metalloproteinases (MMPs), mediate many of the changes in the TME during tumor progression. In HCC, the amplification of the lncRNA ZFAS1 gene is positively correlated with hepatic invasion and metastasis through modulation of the miR-150/ZEB1/MMP-14/MMP-16 cascade (Li et al., 2015b). Cancer is a heterogeneous population of cells, and CSCs are responsible for metastasis and resistance to traditional therapies (Chiba et al., 2016; Xiao et al., 2017). CSCs or TICs have been identified in multiple cancer types, such as HCC, and are proposed as critical promotors of tumor initiation, development, metastasis, and recurrence. The upregulation of lncRNA UCA1 in liver CSCs plays a critical role in governing their growth and differentiation through regulation of multiple pathways (Gui et al., 2015; Pu et al., 2015).
FIGURE 4. Long non-coding RNAs modulate tumor microenvironment. (A) lncRNA NKILA inactivates the nuclear factor-kappa beta (NF-kB) pathway in breast cancer cells. (B) Downregulation of lncRNA NKILA in tumor-infiltrating T lymphocytes leads to decreased metastatic potential. (C) In BC cells, in response to micro environmental hypoxia, activation of CamK-A triggers the activation of the NF-kB pathway, leading to increased expression of IL6, IL8, and VEGF, and enhances angiogenesis. (D) In bladder cancer metastasis, the lncRNA LNMAT1 recruits hnRNPL to the CCL2 promoter, resulting in increased expression of CCL2 through CCL2-dependent macrophage recruitment.
T cells play a crucial role in both cancer development and immune responses. lncRNAs play a role in T-cell activation, differentiation, development, function, and cancer immunology. T-cell activation by foreign antigens triggers immune responses that are controlled by activation-induced cell death (AICD) of T lymphocytes. Cancer cells use AICD to evade the immune system. lncRNA has been shown to be involved in AICD of T lymphocytes, and NF-KappaB Interacting LncRNA (lncRNA NKILA) has been found to modulate T-cell sensitivity to AICD by inhibiting NF-kB activity (Huang et al., 2018). KD of NKILA has been shown to suppress BC progression and enhance the infiltration of cytotoxic T lymphocytes (CTLs) (Huang et al., 2018). A previous study found that in BC cells, cytoplasmic lncRNA that directly inhibits the NF-kB complex leads to increased metastatic potential when downregulated (Liu et al., 2021) (Figure 4). In the TME, T cells have the ability to activate and differentiate into regulatory T cells (Tregs). Tregs play a crucial role in the regulation of tumor immunity by inhibiting the anti-tumor immunity of immune cells such as NK cells, CD8+ T cells, and DCs (Wang et al., 2011). lncRNAs have been found to influence the function of Tregs in the TME and inhibit immune surveillance (Jiang et al., 2017b). Some lncRNAs, such as SNHG1, lnc-epidermal growth factor receptor (lnc-EGFR), Flicr, and Flatr, have been documented to modulate Tregs (Jiang et al., 2017b; Zemmour et al., 2017; Brajic et al., 2018). lncRNA LNMAT1 has also been shown to induce metastasis of the lymphatic system in BDC patients by recruiting macrophages and enhancing H3K4 tri-methylation and CCL2 expression by recruiting hnRNPL (heterogeneous nuclear ribonucleoprotein L) to its promoter (Chen et al., 2018) (Figure 4).
CAFs are one of the core components of the TME, which have a significant role in reshaping the ECM structure. This can lead to collagen tracking and promote the migration of cancer cells (Guo et al., 2022). lncRNAs have also been found to be key drivers of CAF-mediated metastatic progression. For example, LINC00092 interacts with a glycolytic enzyme, 6-phosphofructo-2-kinase/fructose-2,6-biphosphatase 2 (PFKFB2), to regulate the level of glycolysis and support CAF activity, thereby promoting OVC metastasis (Zhao et al., 2017). Additionally, exosomal LINC00659 transferred from CAFs can increase the expression of ANXA2 by binding with miR-342-3p, thereby enhancing EMT(, and migration and proliferation of CRC cells (Zhou et al., 2021).
The TME also plays a crucial role in immune escape, as tumor-related immunosuppressive factors can contribute to the ability of cancerous cells to resist the body’s immune surveillance (Ott et al., 2013; Postow et al., 2015). Immune checkpoint inhibitors (ICIs), such as monoclonal antibodies, are designed to block the communication between immune cells and tumor cells and are used to enhance the function of the immune system in the fight against cancer. Tumor-associated immune checkpoint molecules include LAG3, TIM-3, PD-1, and CTLA4, among others (Tumeh et al., 2014). PD-L1 on tumor cells can interact with PD-1 on the surface of relevant lymphocytes, leading to the production of cytokines and lymphocyte apoptosis, thereby allowing tumor cells to evade immune surveillance (Tumeh et al., 2014). lncRNAs have been found to play a crucial role in the regulation of tumor immunity and the development of drug resistance. Some lncRNAs have been shown to promote an immunosuppressive microenvironment that allows tumors to escape the immune system and become resistant to drugs. For example, lncRNA MALAT1 indirectly increases the expression of the immune checkpoint protein PD-L1 through miR-200a-3 and miR-195 binding (Wei et al., 2019b; Wang et al., 2019), while the lncRNA NKX2-1-AS1 has been shown to decrease the PD-L1 expression (Kathuria et al., 2018). Immunotherapy, particularly using PD-1/PD-L1 inhibitors, has made significant advancements in the treatment of solid tumors (Kato et al., 2019; Motzer et al., 2019). However, the modulation of lncRNAs can play a key role in determining the resistance to these ICI therapies. Therefore, it is essential to understand the regulatory functions of lncRNAs in the TME in order to improve the effectiveness of immunotherapy and overcome resistance to these treatments. In conclusion, lncRNAs play a crucial role in regulating various aspects of tumorigenesis, angiogenesis, immunosuppression, and tumor cell progression. A deeper understanding of the regulation of lncRNAs in the TME is necessary for advancing the treatment of metastatic tumors.
AR is a cellular function that plays a critical role in the spread of cancer by allowing cancer cells to resist death when they detach from the ECM (Sakamoto and Kyprianou, 2010; Tajbakhsh et al., 2019; Lee et al., 2021), mainly during metastasis (Paoli et al., 2013). This capability enables cancer cells to dissociate from the primary tumor site and invade distant areas, establishing a metastatic lesion (Simpson et al., 2008; Sakamoto and Kyprianou, 2010). Therefore, AR is considered a crucial process for tumor cell metastasis and has been the target of research for developing new cancer therapies (Coates et al., 2010; Sakamoto and Kyprianou, 2010). Multiple factors and mechanisms have been linked to AR in cancer cells, such as changes in integrin expression, growth factors, oxidative stress, autophagy, EMT, metabolic alterations, and signaling pathways (Paoli et al., 2013; Adeshakin et al., 2021). Additionally, various ncRNAs, particularly lncRNAs, have been associated with AR in several types of cancer (Lee et al., 2021). One specific lncRNA, HOX transcript antisense intergenic RNA (HOTAIR), has been shown to be upregulated in many cancers and linked to metastasis, aggressiveness, and poor patient prognosis (Liu et al., 2013; Tan et al., 2018). While lncRNA HOTAIR increased the AR of OVC cells by recruiting EZH2 and prompting H3K27 methylation (Dai et al., 2021), its KD decreased the ability of AR, migration, invasion, and spheroid formation (Dai et al., 2021). HOTAIR also facilitates AR in GC (Okugawa et al., 2014) and HCC cells through c-Met signaling (Topel et al., 2020). ANRIL, for example, is positively correlated with glioma and modulates caspase-3/8/9 and AKT signaling pathways by sponging miR-203a (Dai et al., 2018). APOC1P1-3 suppresses early apoptosis and promotes AR by reducing activated caspase 3, 8, 9, and PARP through the specific sponge of target miRNA-188-3p (Lu et al., 2021). lncRNA H19 imprints maternally expressed transcripts and promotes EMT and metastasis in various cancers (Yoshimura et al., 2018), and the downregulation of lncRNA H19 decreases liver and lung metastases in PC cells (Yoshimura et al., 2018). lncRNA H19 also activates the Wnt signaling pathway by sponging miR-29-3b and stimulates the onset of EMT in CRC (Ding et al., 2018). Telomerase reverse transcriptase (TERT), in addition to potentiating cancer stemness, metastasis, and telomere length maintenance (Hannen and Bartsch, 2018), also promotes anchorage-independent growth of cancer cells. lncRNA FOXD2-AS1, by sponging miR-7, promotes anchorage-independent growth of thyroid cancer (THC) cells by targeting TERT (Fleisig and Wong, 2012). lncRNA FOXD2 adjacent opposite strand RNA 1 (lncRNA FOXD2-AS1) has been shown to promote the anchorage-independent growth of THC cells by sponging miR-7 and targeting TERT (Cui et al., 2019b). The inactivation of the Hippo signaling pathway is associated with tumor progression and metastasis in many cancers (Calses et al., 2019; Dey et al., 2020). lncRNA MAPK8IP1P2, by sponging miR-146b-3p, activates the Hippo pathway and inhibits anchorage-independent growth and lymphatic metastasis of thyroid cancer in vitro and in vivo (Calses et al., 2019; Liu et al., 2020b; Dey et al., 2020). Analysis of the Cancer Genome Atlas (TCGA) database has revealed that lncRNA NEAT1 is dysregulated in several cancers (Li et al., 2018a) and correlated with lymph node metastasis in cervical cancer (CC) (Shen et al., 2020). Increased lncRNA MALAT1 expression has also been associated with advanced tumor stage, recurrence, and reduced survival in OVC (Gordon et al., 2019). In addition, KD of lncRNA MALAT1 expression in anoikis-resistant OVC cells induced apoptosis by modulating RBFOX2-mediated alternative splicing of KIF1B (pro-apoptotic isoform) (Gordon et al., 2019). Furthermore, lncRNA NEAT1 promotes the metastatic potential of endometrial cancer (EndC) by sponging anti-metastatic miR-361 (Dong et al., 2019). lncRNA VAL induces AR by directly abrogating Trim16-dependent vimentin poly-ubiquitination and degradation (Tian et al., 2020). LINC00958 is upregulated in bladder tumor samples (BDC) compared with normal samples, and KD attenuated an AR of BDC cells (Seitz et al., 2017). miR-7 regulates p65 subunit of NF-kB (known as RELA proto-oncogene, NF-kB subunit (RELA), and KLF4 expression to control invasion, angiogenesis, progression, and metastasis (Okuda et al., 2013; Cui et al., 2017; Li et al., 2020b). These functions of miR-7 in breast cancer (BC) can be diminished by the expression of lncRNA TINCR, which is enhanced by the Sp1 transcription factor. Silencing of lncRNA TINCR leads to a reduction in the anchorage-independent growth, migration, invasion, cell survival, and in vivo growth of BC cells (Liu et al., 2018) (Table 2). Further research into the mechanisms of AR and its association with lncRNAs may lead to the development of new treatments for cancer.
The concept of stem cell niche was first postulated by Schofield (1978) as a distinctive microenvironment that modulates stem cell activity during hematopoiesis. Different stem cell models have well-characterized specialized cellular niches, which play an important role in balancing stem cell activity and quiescence (Scadden, 2014). In the stromal microenvironment of stem cells, it is a distinct local region that combines signals reflecting tissue and organismal state (Schofield, 1978) and modulates epithelial cell plasticity and stem cell fate commitment during tissue regeneration and homeostasis (Blanpain and Fuchs, 2014). In the context of tumor development, tumor cells subvert and shape the niche to create a compatible metastatic niche (Barcellos-Hoff et al., 2013) that supports the growth and survival of disseminated tumor cells (DTCs) and result in the development and progression of disease (Psaila and Lyden, 2009; Sleeman, 2012). Metastatic niches can be formed either on arrival of DTCs in the recipient tissue (Sleeman, 2012) or under the regulation of secreted factors and/or exosomes released by the primary tumor cells before the seeding of DTCs (also termed the pre-metastatic niche) (Kaplan et al., 2006; Hoshino et al., 2015; Peinado et al., 2017). Tumor-derived molecular components (TDMCs), often referred to as non-vesicle factors, and extracellular vesicles are adaptable intercellular communication vehicles that can modulate signaling in the TME while developing the pre-metastatic niche. TDMCs are subdivided into non-vesicle tumor-derived secreted factors (TDSFs) and tumor-derived secreted extracellular vehicles (EVs) which carry a variety of molecular components, such as RNA, DNA proteins, and lipid molecules (Liu and Cao, 2016). In recent years, ncRNAs, such as lncRNAs and sncRNAs, have been identified as an essential part of pre-metastatic niche formation and a new intercellular communication mechanism (Xie et al., 2019). These ncRNAs, as part of TDMCs, are mostly found in EVs with only a small percentage being free (Das et al., 2019). EVs have a unique repertoire of lncRNAs and sncRNAs, highlighting the importance of ncRNAs in the formation of the pre-metastatic niche (Ma et al., 2017; Pardini and Calin, 2019).
Previously, primary tumor–derived vesicles have been linked to the development of the pre-metastatic niche (Kaiser, 2016). In addition, exosomes secreted by primary tumor cells have been shown to enter the bloodstream and alter the metastatic microenvironment for invasion (Szatanek et al., 2017). Recently, lncRNAs, for example, lincROR, DREH, MALAT, CCAT2, HOTAIR, BCAR4, and H19, have been shown to contribute to metastasis in vitro and in vivo (Weidle et al., 2017). In addition to modulating the release of exosomes, lncRNAs modify the cell physiology of distant non-tumor cells and, at the pre-metastatic niche, allow the early survival of disseminate tumor cells (Liu et al., 2019a). Endothelial cells transfer and internalize lncRNA H19 in CD90+ HCC cells and promote angiogenesis and intracellular adhesion by enhancing the release and production of VEGF (Conigliaro et al., 2015; Zhang et al., 2019c). In Hepatocellular cancer (HCC)/liver cancer (LiC) cells, the integrin β1/α5/JNK/c-JUN signaling pathway participates in higher matrix stiffness, which is induced by LOXL2 (lysyl oxidase homolog 2). LOXL2 stimulates the expression of CXCL12 and MMP-9, the production of fibrin, and the recruitment of bone marrow–derived dendritic cells (BMDCs), supporting the development of the pre-metastatic niche (Wu et al., 2018). In pancreatic ductal adenocarcinoma (PDAC), exosome-derived protein macrophage migration inhibitory factor (MIF) enhances metastasis of the liver by promoting the development of the hepatic pre-metastatic niche. Additionally, the lncRNA SOX2OT has been found to regulate the expression of Sox2 via competitively binding with the miR-200 family, leading to the EMT process and stem cell–like characteristics, which are hallmarks of cancer metastasis (Li et al., 2018b), thereby leading to metastasis and invasion of PDAC. Researchers from the School of Public Health and Medicine University of Wisconsin, USA have proposed that cancer stem-like cells (CSCs) and anaplastic TC (ATC) release lncRNAs (HOTAIR, lincROR, MALAT1, and PVT1) transferred by the exosome and therefore help to induct EMT, modulate host immunity to escape immune response, and inculcate the TME to form a metastatic niche (Hardin et al., 2018).
Current treatments for metastasis are similar to those for primary tumors and include immunotherapy, chemotherapy, targeted therapy, and a combination of these (Massagué and Obenauf, 2016). However, patients with metastatic cancer frequently exhibit increased therapeutic resistance in multiple carcinomas (Jolly et al., 2019), indicating that exploring new strategies for diagnosis and therapeutics is an urgent priority. The expression of certain lncRNAs is modified during transformation from primary to metastatic cancer cells, and these changes can serve as potential diagnostic biomarkers for cancer. In addition to identifying the molecular mechanisms of lncRNAs in cancer metastasis, considerable efforts should be acquired to investigate the promising strategies for clinical implications. The expression of certain lncRNAs can be detected not only in the cells themselves but also in exosomes found in the serum. One example is the lncRNA HOTAIR which has shown promise as a diagnostic marker for thyroid cancer and can differentiate benign thyroid nodules from migratory. Clinical trials are ongoing to further explore its potential use as a diagnostic tool for THC (NCT03469544) (Zhang et al., 2017b; Lai and Cheng, 2018). Early detection of lncRNA expression changes in patients can lead to personalized and precision medicine by allowing for early treatment at a time when the disease is more responsive to medication. Although direct targeting of lncRNAs as a therapeutic intervention is still in its early stages, various methods are being developed to modulate lncRNA expression, such as transcription blocking, degradation, and gene-editing technology. DNA binding elements can modulate lncRNA transcription, while techniques such as ribozymes, antisense oligonucleotides (ASOs), and siRNAs via activating an RNA-induced silencing complex (RISC) can downregulate lncRNAs by inducing their degradation. For instance, lncRNA MALAT1 can be efficiently silenced by zinc finger nucleases (ZFNs)–based genomic manipulation (Gutschner et al., 2011). Depletion of oncogenic lncRNAs in cancer cells has shown anti-cancer properties, such as inhibiting the proliferation and cloning of lung cancer cells. For instance, siRNA-induced KD of the lncRNA LL22NC03-N64E9.1 inhibited the proliferation of lung cancer (LC) cells (Jing et al., 2018). Likewise, intra-tumor administration of siRNA targeting lncRNA MALAT1 in a prostate cancer (PCa) xenograft model decreased metastasis and improved mice survival (Ren et al., 2013). Similarly, siRNAs targeting lncRNA OIP5-AS1 reduced migration, invasion, and proliferation of glioma U87 cells (Sun et al., 2019). siRNA mediated KD of lncRNA PCGEM1 (PCa gene expression marker 1) has been shown to increase G2- and S-phase cells, inhibit colony formation, and enhance sensitivity to baicalein in PCa LNCaP cells (Han et al., 2020). ASOs are chemically synthesized RNA-targeting molecules that range from 12 to 30 nucleotides in length. They bind to specific RNA targets through Watson–Crick base pairing (Bennett, 2019) and modulate gene expression by initiating target degradation, blocking translation, steric hindrance, altering splicing (Arun et al., 2018), and premature transcriptional termination (Lai et al., 2020; Lee and Mendell, 2020). ASOs have shown promising results as a therapeutic approach for lncRNA targeting and have been shown to slowdown tumor growth and reduce metastasis in mouse mammary cancer models (Zhou et al., 2016). In the mouse mammary tumor virus (MMTV)-PyMT cancer model, the KD of lncRNA MALAT1 by ASOs results in slower tumor growth and a reduction in metastasis (Arun et al., 2016). However, optimizing the delivery of ASOs is a crucial issue to improve their efficacy for targeting lncRNAs or protein-coding genes (Yamamoto et al., 2015; Lai et al., 2020). Recently developed miR-CLIP-seq technology is used to identify the miRNA-mRNA (Ahadi et al., 2017) and miRNA-lncRNA interactions (Cao et al., 2019). For instance, the interactions between miR-106a and lncRNA H19 and their effect on the upregulation of downstream-associated mRNAs have been studied (Imig et al., 2015).
Another method of targeting lncRNAs is through competitive binding using specific small molecules or aptamers. Aptamers are short RNA or DNA oligonucleotides that can bind to specific regions of lncRNA and block its interactions with binding partners. Small molecule inhibitors target the RNA binding pockets of lncRNAs, preventing the interactions between lncRNAs and proteins (Parasramka et al., 2016). However, the potential for off-target effects and high costs in targeting the interaction between lncRNAs and their targets require further research before these methods can be used in therapeutic applications. CRISPR/Cas9 or CRISPR/Cas13–based targeting technology is becoming increasingly important due to recent advancements in genome editing techniques, making it a promising alternative for lncRNA regulation (Cox et al., 2017; Zhen and Li, 2019). In animal models, lncRNA GMAN was successfully targeted using CRISPR/Cas9, resulting in a significant decrease in GC metastasis (Zhuo et al., 2019). Despite the fact that lncRNAs are promising therapeutic targets for metastatic diseases, their in vivo inhibition still remains a challenge because of their quick degradation in biological fluids by nucleases, and the difficulty of delivering them to specific target cells and the activation of innate immunity. A variety of materials are being researched to overcome these challenges, which include lipid nanoparticles (Pirollo et al., 2007), polymers (Shu et al., 2014), cell-penetrating peptides (CPPs) (Yang et al., 2015), monoclonal antibodies (Yao et al., 2012), and small-molecule inhibitors (Thomas et al., 2009). However, further research is required before these findings can be translated into clinical applications.
Metastasis is the leading cause of cancer-related deaths and a major barrier to successful cancer treatment. The complex process of metastasis is influenced by interrelated signaling pathways caused by genetic heterogeneity or epigenetic modification changes and the metastatic microenvironment. lncRNAs have gained attention in recent years due to their important role in promoting and maintaining tumor initiation and progression (Schmitt and Chang, 2016). This article focuses on the role of lncRNAs in the regulation of key processes in metastasis, which includes metabolic reprogramming (glucose metabolism and OXPHOS), EMT, metastatic microenvironment, development of pre-metastatic niches, drug resistance, and AR.
Due to their unique and diverse functions, lncRNAs have been identified as potential targets for cancer therapy. For example, lncRNAs have been found to be involved in regulating gene expression and chromatin structure, both of which are critical processes in cancer progression and metastasis. However, the majority of research on lncRNAs and metastasis to date has been concentrated on metastasis specific to organs. However, many patients suffer from metastasis in multiple organs, making it difficult to understand the molecular mechanisms mediated by lncRNAs. Because of this intricacy, it is difficult to understand scientific molecular mechanisms mediated by lncRNAs, and therefore it has been challenging to generalize the functions of lncRNAs in metastasis across different types of cancers due to the cell type–specific nature of most lncRNAs. Generalizing the functions of lncRNAs in metastasis across different types of cancers has also been elusive, and perhaps this should be expected based on the cell type–specific function of most lncRNAs. The contribution of lncRNAs to the steps in the invasion–metastasis cascade that includes intravasation, extravasation, distant colonization, and formation of micrometastases is not well established. Therefore, more patient-matched molecular profile data from granular stages of metastasis are necessary to overcome these limitations (for example, CTCs, micrometastases, primary tumor, and well-defined metastases).
lncRNAs appear to be an underappreciated cache of novel therapeutic targets. They play a variety of roles in the progression of cancer and ensure new opportunities for undermining metastases in the clinical setting. Thus, future research may focus on identifying lncRNA mediators of metastasis as potential targeted therapies common in various types of tumors. The development of lncRNA-based therapeutics requires the identification and validation of specific lncRNA targets involved in metastasis. To achieve this, researchers have to develop reliable technologies that are capable of targeting lncRNAs in vivo (Bassett et al., 2014; Liu and Lim, 2018), such as antisense oligonucleotides (Wurster and Ludolph, 2018) that can specifically bind to lncRNAs and modulate their expression. The availability of these technologies will significantly facilitate the development of lncRNA-based therapeutics for the treatment of metastatic cancer. However, there are still many challenges in lncRNA research, such as the lack of conservation of lncRNAs across species, which can hinder the study of their function in animal models, complexity and heterogeneity in function, and tissue specificity. Furthermore, there is a requirement for more sensitive and specific technologies to effectively target lncRNAs in vivo. Despite these challenges, the potential of lncRNAs as therapeutic targets in cancer is evident, and future research in this area has the potential to impact the successful treatment of metastatic cancer.
SnB, SdB, AAB, TM, and MM wrote the manuscript and generated figures. MM contributed to the concept and design and critically edited the manuscript. RM, IE, MU, JB, FA, MA, SM, TM, ASAA, AAB, and MM performed critical revision and editing of the scientific content. All authors contributed to the article and have approved the submitted version.
This study was supported by the Ramalingaswami Re-entry Fellowship (Grant number: D.O. NO.BT/HRD/35/02/2006) from the Department of Biotechnology, Govt. of India, New Delhi, and Core Research Grant (CRG/2021/003805) from the Science and Engineering Research Board (SERB), Govt. of India, New Delhi, to MM. Promotion of University Research and Scientific Excellence (SR/PURSE/2022/121) grant from the Department of Biotechnology, Govt. of India, New Delhi to the Islamic University of Science and Technology (IUST), Awantipora, Kashmir.
The authors declare that the research was conducted in the absence of any commercial or financial relationships that could be construed as a potential conflict of interest.
All claims expressed in this article are solely those of the authors and do not necessarily represent those of their affiliated organizations, or those of the publisher, editors, and reviewers. Any product that may be evaluated in this article, or claim that may be made by its manufacturer, is not guaranteed or endorsed by the publisher.
Abba, M. C., Fabre, M. L., Lee, J., Tatineni, P., Kil, H., and Aldaz, C. M. (2021). HOTAIR modulated pathways in early-stage breast cancer progression. Front. Oncol. 11, 783211. doi:10.3389/fonc.2021.783211
Adeshakin, F. O., Adeshakin, A. O., Afolabi, L. O., Yan, D., Zhang, G., and Wan, X. (2021). Mechanisms for modulating anoikis resistance in cancer and the relevance of metabolic reprogramming. Front. Oncol. 11, 626577. doi:10.3389/fonc.2021.626577
Agostini, M., Mancini, M., and Candi, E. (2022). Long non-coding RNAs affecting cell metabolism in cancer. Biol. direct 17 (1), 26. doi:10.1186/s13062-022-00341-x
Ahadi, A., Sablok, G., and Hutvagner, G. (2017). miRTar2GO: a novel rule-based model learning method for cell line specific microRNA target prediction that integrates Ago2 CLIP-Seq and validated microRNA-target interaction data. Nucleic acids Res. 45 (6), e42. doi:10.1093/nar/gkw1185
Alderton, G. K. (2014). Metastasis: Metabolic reprogramming in disseminated cells. Nat. Rev. Cancer 14 (11), 703. doi:10.1038/nrc3842
Altorki, N. K., Markowitz, G. J., Gao, D., Port, J. L., Saxena, A., Stiles, B., et al. (2019). The lung microenvironment: An important regulator of tumour growth and metastasis. Nat. Rev. Cancer 19 (1), 9–31. doi:10.1038/s41568-018-0081-9
Arun, G., Diermeier, S., Akerman, M., Chang, K. C., Wilkinson, J. E., Hearn, S., et al. (2016). Differentiation of mammary tumors and reduction in metastasis upon Malat1 lncRNA loss. Genes & Dev. 30 (1), 34–51. doi:10.1101/gad.270959.115
Arun, G., Diermeier, S. D., and Spector, D. L. (2018). Therapeutic targeting of long non-coding RNAs in cancer. Trends Mol. Med. 24 (3), 257–277. doi:10.1016/j.molmed.2018.01.001
Bär, C., Chatterjee, S., and Thum, T. (2016). Long noncoding RNAs in cardiovascular pathology, diagnosis, and therapy. Circulation 134 (19), 1484–1499. doi:10.1161/CIRCULATIONAHA.116.023686
Barcellos-Hoff, M. H., Lyden, D., and Wang, T. C. (2013). The evolution of the cancer niche during multistage carcinogenesis. Nat. Rev. Cancer 13 (7), 511–518. doi:10.1038/nrc3536
Barsyte-Lovejoy, D., Lau, S. K., Boutros, P. C., Khosravi, F., Jurisica, I., Andrulis, I. L., et al. (2006). The c-Myc oncogene directly induces the H19 noncoding RNA by allele-specific binding to potentiate tumorigenesis. Cancer Res. 66 (10), 5330–5337. doi:10.1158/0008-5472.CAN-06-0037
Bassett, A. R., Akhtar, A., Barlow, D. P., Bird, A. P., Brockdorff, N., Duboule, D., et al. (2014). Considerations when investigating lncRNA function in vivo. eLife 3, e03058. doi:10.7554/eLife.03058
Beloribi-Djefaflia, S., Vasseur, S., and Guillaumond, F. (2016). Lipid metabolic reprogramming in cancer cells. Oncogenesis 5 (1), e189. doi:10.1038/oncsis.2015.49
Benetti, A., Berenzi, A., Gambarotti, M., Garrafa, E., Gelati, M., Dessy, E., et al. (2008). Transforming growth factor-beta1 and CD105 promote the migration of hepatocellular carcinoma-derived endothelium. Cancer Res. 68 (20), 8626–8634. doi:10.1158/0008-5472.CAN-08-1218
Bennett, C. F. (2019). Therapeutic antisense oligonucleotides are coming of age. Annu. Rev. Med. 70, 307–321. doi:10.1146/annurev-med-041217-010829
Berteaux, N., Lottin, S., Monté, D., Pinte, S., Quatannens, B., Coll, J., et al. (2005). H19 mRNA-like noncoding RNA promotes breast cancer cell proliferation through positive control by E2F1. J. Biol. Chem. 280 (33), 29625–29636. doi:10.1074/jbc.M504033200
Bhat, A. A., Nisar, S., Maacha, S., Carneiro-Lobo, T. C., Akhtar, S., Siveen, K. S., et al. (2021). Cytokine-chemokine network driven metastasis in esophageal cancer; promising avenue for targeted therapy. Mol. cancer 20 (1), 2. doi:10.1186/s12943-020-01294-3
Bhat, A. A., Nisar, S., Singh, M., Ashraf, B., Masoodi, T., Prasad, C. P., et al. (2022). Cytokine- and chemokine-induced inflammatory colorectal tumor microenvironment: Emerging avenue for targeted therapy. Cancer Commun. Lond. Engl. 42 (8), 689–715. doi:10.1002/cac2.12295
Bhat, A. A., Yousuf, P., Wani, N. A., Rizwan, A., Chauhan, S. S., Siddiqi, M. A., et al. (2021). Correction: Tumor microenvironment: An evil nexus promoting aggressive head and neck squamous cell carcinoma and avenue for targeted therapy. Signal Transduct. Target. Ther. 6 (1), 93. doi:10.1038/s41392-021-00503-9
Bian, Z., Zhang, J., Li, M., Feng, Y., Wang, X., Zhang, J., et al. (2018). LncRNA-FEZF1-AS1 promotes tumor proliferation and metastasis in colorectal cancer by regulating PKM2 signaling. Clin. cancer Res. official J. Am. Assoc. Cancer Res. 24 (19), 4808–4819. doi:10.1158/1078-0432.CCR-17-2967
Blanpain, C., and Fuchs, E. (2014). Stem cell plasticity. Plasticity of epithelial stem cells in tissue regeneration. Sci. (New York, NY) 344 (6189), 1242281. doi:10.1126/science.1242281
Brabletz, T., Kalluri, R., Nieto, M. A., and Weinberg, R. A. (2018). EMT in cancer. Nat. Rev. Cancer 18 (2), 128–134. doi:10.1038/nrc.2017.118
Brajic, A., Franckaert, D., Burton, O., Bornschein, S., Calvanese, A. L., Demeyer, S., et al. (2018). The long non-coding RNA Flatr anticipates Foxp3 expression in regulatory T cells. Front. Immunol. 9, 1989. doi:10.3389/fimmu.2018.01989
Bray, F., Laversanne, M., Weiderpass, E., and Soerjomataram, I. (2021). The ever-increasing importance of cancer as a leading cause of premature death worldwide. Cancer 127 (16), 3029–3030. doi:10.1002/cncr.33587
Bray, S. J. (2016). Notch signalling in context. Nat. Rev. Mol. Cell Biol. 17 (11), 722–735. doi:10.1038/nrm.2016.94
Browning, L., Patel, M. R., Horvath, E. B., Tawara, K., and Jorcyk, C. L. (2018). IL-6 and ovarian cancer: Inflammatory cytokines in promotion of metastasis. Cancer Manag. Res. 10, 6685–6693. doi:10.2147/CMAR.S179189
Budhu, A., and Wang, X. W. (2006). The role of cytokines in hepatocellular carcinoma. J. Leukoc. Biol. 80 (6), 1197–1213. doi:10.1189/jlb.0506297
Cairns, R. A., Harris, I. S., and Mak, T. W. (2011). Regulation of cancer cell metabolism. Nat. Rev. Cancer 11 (2), 85–95. doi:10.1038/nrc2981
Calses, P. C., Crawford, J. J., Lill, J. R., and Dey, A. (2019). Hippo pathway in cancer: Aberrant regulation and therapeutic opportunities. Trends cancer 5 (5), 297–307. doi:10.1016/j.trecan.2019.04.001
Cao, M., Zhao, J., and Hu, G. (2019). Genome-wide methods for investigating long noncoding RNAs. Biomed. Pharmacother. = Biomedecine Pharmacother. 111, 395–401. doi:10.1016/j.biopha.2018.12.078
Cevenini, A., Orrù, S., Mancini, A., Alfieri, A., Buono, P., and Imperlini, E. (2018). Molecular signatures of the insulin-like growth factor 1-mediated epithelial-mesenchymal transition in breast, lung and gastric cancers. Int. J. Mol. Sci. 19 (8), 2411. doi:10.3390/ijms19082411
Chaneton, B., and Gottlieb, E. (2012). Rocking cell metabolism: Revised functions of the key glycolytic regulator PKM2 in cancer. Trends Biochem. Sci. 37 (8), 309–316. doi:10.1016/j.tibs.2012.04.003
Chen, C., He, W., Huang, J., Wang, B., Li, H., Cai, Q., et al. (2018). LNMAT1 promotes lymphatic metastasis of bladder cancer via CCL2 dependent macrophage recruitment. Nat. Commun. 9 (1), 3826. doi:10.1038/s41467-018-06152-x
Chen, J., Yu, Y., Li, H., Hu, Q., Chen, X., He, Y., et al. (2019). Long non-coding RNA PVT1 promotes tumor progression by regulating the miR-143/HK2 axis in gallbladder cancer. Mol. cancer 18 (1), 33. doi:10.1186/s12943-019-0947-9
Chen, L. L. (2016). Linking long noncoding RNA localization and function. Trends Biochem. Sci. 41 (9), 761–772. doi:10.1016/j.tibs.2016.07.003
Chiba, T., Iwama, A., and Yokosuka, O. (2016). Cancer stem cells in hepatocellular carcinoma: Therapeutic implications based on stem cell biology. Hepatology Res. official J. Jpn. Soc. Hepatology 46 (1), 50–57. doi:10.1111/hepr.12548
Choi, C. H., Webb, B. A., Chimenti, M. S., Jacobson, M. P., and Barber, D. L. (2013). pH sensing by FAK-His58 regulates focal adhesion remodeling. J. Cell Biol. 202 (6), 849–859. doi:10.1083/jcb.201302131
Coates, J. M., Galante, J. M., and Bold, R. J. (2010). Cancer therapy beyond apoptosis: Autophagy and anoikis as mechanisms of cell death. J. Surg. Res. 164 (2), 301–308. doi:10.1016/j.jss.2009.07.011
Coffelt, S. B., Wellenstein, M. D., and de Visser, K. E. (2016). Neutrophils in cancer: Neutral no more. Nat. Rev. Cancer 16 (7), 431–446. doi:10.1038/nrc.2016.52
Conigliaro, A., Costa, V., Lo Dico, A., Saieva, L., Buccheri, S., Dieli, F., et al. (2015). CD90+ liver cancer cells modulate endothelial cell phenotype through the release of exosomes containing H19 lncRNA. Mol. cancer 14, 155. doi:10.1186/s12943-015-0426-x
Cox, D. B. T., Gootenberg, J. S., Abudayyeh, O. O., Franklin, B., Kellner, M. J., Joung, J., et al. (2017). RNA editing with CRISPR-Cas13. Sci. (New York, NY) 358 (6366), 1019–1027. doi:10.1126/science.aaq0180
Cui, M., Xiao, Z., Wang, Y., Zheng, M., Song, T., Cai, X., et al. (2015). Long noncoding RNA HULC modulates abnormal lipid metabolism in hepatoma cells through an miR-9-mediated RXRA signaling pathway. Cancer Res. 75 (5), 846–857. doi:10.1158/0008-5472.CAN-14-1192
Cui, Y., Xu, H. F., Liu, M. Y., Xu, Y. J., He, J. C., Zhou, Y., et al. (2019). Mechanism of exosomal microRNA-224 in development of hepatocellular carcinoma and its diagnostic and prognostic value. World J. gastroenterology 25 (15), 1890–1898. doi:10.3748/wjg.v25.i15.1890
Cui, Y. X., Bradbury, R., Flamini, V., Wu, B., Jordan, N., and Jiang, W. G. (2017). MicroRNA-7 suppresses the homing and migration potential of human endothelial cells to highly metastatic human breast cancer cells. Br. J. cancer 117 (1), 89–101. doi:10.1038/bjc.2017.156
Cui, Z., Han, B., Wang, X., Li, Z., Wang, J., and Lv, Y. (2019). Long non-coding RNA TTN-AS1 promotes the proliferation and invasion of colorectal cancer cells by activating miR-497-mediated PI3K/Akt/mTOR signaling. OncoTargets Ther. 12, 11531–11539. doi:10.2147/OTT.S229104
Dai, W., Tian, C., and Jin, S. (2018). Effect of lncRNA ANRIL silencing on anoikis and cell cycle in human glioma via microRNA-203a. OncoTargets Ther. 11, 5103–5109. doi:10.2147/OTT.S169809
Dai, Z. Y., Jin, S. M., Luo, H. Q., Leng, H. L., and Fang, J. D. (2021). LncRNA HOTAIR regulates anoikis-resistance capacity and spheroid formation of ovarian cancer cells by recruiting EZH2 and influencing H3K27 methylation. Neoplasma 68 (3), 509–518. doi:10.4149/neo_2021_201112N1212
Dang, C. V. (2010). Rethinking the Warburg effect with Myc micromanaging glutamine metabolism. Cancer Res. 70 (3), 859–862. doi:10.1158/0008-5472.CAN-09-3556
Das, S., Ansel, K. M., Bitzer, M., Breakefield, X. O., Charest, A., Galas, D. J., et al. (2019). The extracellular RNA communication consortium: Establishing foundational knowledge and technologies for extracellular RNA research. Cell 177 (2), 231–242. doi:10.1016/j.cell.2019.03.023
De Palma, M., Biziato, D., and Petrova, T. V. (2017). Microenvironmental regulation of tumour angiogenesis. Nat. Rev. Cancer 17 (8), 457–474. doi:10.1038/nrc.2017.51
De Palma, M., and Lewis, C. E. (2013). Macrophage regulation of tumor responses to anticancer therapies. Cancer Cell 23 (3), 277–286. doi:10.1016/j.ccr.2013.02.013
DeBerardinis, R. J., Lum, J. J., Hatzivassiliou, G., and Thompson, C. B. (2008). The biology of cancer: Metabolic reprogramming fuels cell growth and proliferation. Cell metab. 7 (1), 11–20. doi:10.1016/j.cmet.2007.10.002
DeNardo, D. G., and Ruffell, B. (2019). Macrophages as regulators of tumour immunity and immunotherapy. Nat. Rev. Immunol. 19 (6), 369–382. doi:10.1038/s41577-019-0127-6
Deng, S. J., Chen, H. Y., Zeng, Z., Deng, S., Zhu, S., Ye, Z., et al. (2019). Nutrient stress-dysregulated antisense lncRNA GLS-AS impairs GLS-mediated metabolism and represses pancreatic cancer progression. Cancer Res. 79 (7), 1398–1412. doi:10.1158/0008-5472.CAN-18-0419
Dey, A., Varelas, X., and Guan, K. L. (2020). Targeting the Hippo pathway in cancer, fibrosis, wound healing and regenerative medicine. Nat. Rev. Drug Discov. 19 (7), 480–494. doi:10.1038/s41573-020-0070-z
Di Domenico, M., and Giordano, A. (2017). Signal transduction growth factors: The effective governance of transcription and cellular adhesion in cancer invasion. Oncotarget 8 (22), 36869–36884. doi:10.18632/oncotarget.16300
Ding, D., Li, C., Zhao, T., Li, D., Yang, L., and Zhang, B. (2018). LncRNA H19/miR-29b-3p/PGRN Axis promoted epithelial-mesenchymal transition of colorectal cancer cells by acting on Wnt signaling. Mol. cells 41 (5), 423–435. doi:10.14348/molcells.2018.2258
Djebali, S., Davis, C. A., Merkel, A., Dobin, A., Lassmann, T., Mortazavi, A., et al. (2012). Landscape of transcription in human cells. Nature 489 (7414), 101–108. doi:10.1038/nature11233
Dong, P., Xiong, Y., Yue, J., Xu, D., Ihira, K., Konno, Y., et al. (2019). Long noncoding RNA NEAT1 drives aggressive endometrial cancer progression via miR-361-regulated networks involving STAT3 and tumor microenvironment-related genes. J. Exp. Clin. cancer Res. CR 38 (1), 295. doi:10.1186/s13046-019-1306-9
Dong, R., Liu, G. B., Liu, B. H., Chen, G., Li, K., Zheng, S., et al. (2016). Targeting long non-coding RNA-TUG1 inhibits tumor growth and angiogenesis in hepatoblastoma. Cell death Dis. 7 (6), e2278. doi:10.1038/cddis.2016.143
Dongre, A., and Weinberg, R. A. (2019). New insights into the mechanisms of epithelial-mesenchymal transition and implications for cancer. Nat. Rev. Mol. Cell Biol. 20 (2), 69–84. doi:10.1038/s41580-018-0080-4
Düzgün Ş, A., Yerlikaya, A., Zeren, S., Bayhan, Z., Okur, E., and Boyacı, İ. (2017). Differential effects of p38 MAP kinase inhibitors SB203580 and SB202190 on growth and migration of human MDA-MB-231 cancer cell line. Cytotechnology 69 (4), 711–724. doi:10.1007/s10616-017-0079-2
Esteller, M. (2011). Non-coding RNAs in human disease. Nat. Rev. Genet. 12 (12), 861–874. doi:10.1038/nrg3074
Fabregat, I., and Caballero-Díaz, D. (2018). Transforming growth factor-β-induced cell plasticity in liver fibrosis and hepatocarcinogenesis. Front. Oncol. 8, 357. doi:10.3389/fonc.2018.00357
Fatima, F., and Nawaz, M. (2017). Vesiculated long non-coding RNAs: Offshore packages deciphering trans-regulation between cells, cancer progression and resistance to therapies. Non-coding RNA 3 (1), 10. doi:10.3390/ncrna3010010
Fleisig, H. B., and Wong, J. M. (2012). Telomerase promotes efficient cell cycle kinetics and confers growth advantage to telomerase-negative transformed human cells. Oncogene 31 (8), 954–965. doi:10.1038/onc.2011.292
Fong, M. Y., Zhou, W., Liu, L., Alontaga, A. Y., Chandra, M., Ashby, J., et al. (2015). Breast-cancer-secreted miR-122 reprograms glucose metabolism in premetastatic niche to promote metastasis. Nat. Cell Biol. 17 (2), 183–194. doi:10.1038/ncb3094
Frantz, C., Stewart, K. M., and Weaver, V. M. (2010). The extracellular matrix at a glance. J. Cell Sci. 123 (24), 4195–4200. doi:10.1242/jcs.023820
Fu, M., Huang, Z., Zang, X., Pan, L., Liang, W., Chen, J., et al. (2018). Long noncoding RNA LINC00978 promotes cancer growth and acts as a diagnostic biomarker in gastric cancer. Cell Prolif. 51 (1), e12425. doi:10.1111/cpr.12425
Gajewski, T. F., Schreiber, H., and Fu, Y. X. (2013). Innate and adaptive immune cells in the tumor microenvironment. Nat. Immunol. 14 (10), 1014–1022. doi:10.1038/ni.2703
Ge, X. S., Ma, H. J., Zheng, X. H., Ruan, H. L., Liao, X. Y., Xue, W. Q., et al. (2013). HOTAIR, a prognostic factor in esophageal squamous cell carcinoma, inhibits WIF-1 expression and activates Wnt pathway. Cancer Sci. 104 (12), 1675–1682. doi:10.1111/cas.12296
Gibb, E. A., Brown, C. J., and Lam, W. L. (2011). The functional role of long non-coding RNA in human carcinomas. Mol. cancer 10, 38. doi:10.1186/1476-4598-10-38
Gordon, M. A., Babbs, B., Cochrane, D. R., Bitler, B. G., and Richer, J. K. (2019). The long non-coding RNA MALAT1 promotes ovarian cancer progression by regulating RBFOX2-mediated alternative splicing. Mol. Carcinog. 58 (2), 196–205. doi:10.1002/mc.22919
Grossi, E., Raimondi, I., Goñi, E., González, J., Marchese, F. P., Chapaprieta, V., et al. (2020). A lncRNA-SWI/SNF complex crosstalk controls transcriptional activation at specific promoter regions. Nat. Commun. 11 (1), 936. doi:10.1038/s41467-020-14623-3
Grotegut, S., von Schweinitz, D., Christofori, G., and Lehembre, F. (2006). Hepatocyte growth factor induces cell scattering through MAPK/Egr-1-mediated upregulation of Snail. EMBO J. 25 (15), 3534–3545. doi:10.1038/sj.emboj.7601213
Gu, D. N., Jiang, M. J., Mei, Z., Dai, J. J., Dai, C. Y., Fang, C., et al. (2017). microRNA-7 impairs autophagy-derived pools of glucose to suppress pancreatic cancer progression. Cancer Lett. 400, 69–78. doi:10.1016/j.canlet.2017.04.020
Guffanti, A., Iacono, M., Pelucchi, P., Kim, N., Soldà, G., Croft, L. J., et al. (2009). A transcriptional sketch of a primary human breast cancer by 454 deep sequencing. BMC genomics 10, 163. doi:10.1186/1471-2164-10-163
Gui, X., Li, H., Li, T., Pu, H., and Lu, D. (2015). Long noncoding RNA CUDR regulates HULC and β-catenin to govern human liver stem cell malignant differentiation. Mol. Ther. J. Am. Soc. Gene Ther. 23 (12), 1843–1853. doi:10.1038/mt.2015.166
Guo, F., Parker Kerrigan, B. C., Yang, D., Hu, L., Shmulevich, I., Sood, A. K., et al. (2014). Post-transcriptional regulatory network of epithelial-to-mesenchymal and mesenchymal-to-epithelial transitions. J. Hematol. Oncol. 7, 19. doi:10.1186/1756-8722-7-19
Guo, Y., Ji, X., Liu, J., Fan, D., Zhou, Q., Chen, C., et al. (2019). Effects of exosomes on pre-metastatic niche formation in tumors. Mol. cancer 18 (1), 39. doi:10.1186/s12943-019-0995-1
Guo, Y., Xie, Y., and Luo, Y. (2022). The role of long non-coding RNAs in the tumor immune microenvironment. Front. Immunol. 13, 851004. doi:10.3389/fimmu.2022.851004
Guppy, M., Leedman, P., Zu, X., and Russell, V. (2002). Contribution by different fuels and metabolic pathways to the total ATP turnover of proliferating MCF-7 breast cancer cells. Biochem. J. 364 (1), 309–315. doi:10.1042/bj3640309
Gupta, R. A., Shah, N., Wang, K. C., Kim, J., Horlings, H. M., Wong, D. J., et al. (2010). Long non-coding RNA HOTAIR reprograms chromatin state to promote cancer metastasis. Nature 464 (7291), 1071–1076. doi:10.1038/nature08975
Gutschner, T., Baas, M., and Diederichs, S. (2011). Noncoding RNA gene silencing through genomic integration of RNA destabilizing elements using zinc finger nucleases. Genome Res. 21 (11), 1944–1954. doi:10.1101/gr.122358.111
Hamabe, A., Konno, M., Tanuma, N., Shima, H., Tsunekuni, K., Kawamoto, K., et al. (2014). Role of pyruvate kinase M2 in transcriptional regulation leading to epithelial-mesenchymal transition. Proc. Natl. Acad. Sci. U. S. A. 111 (43), 15526–15531. doi:10.1073/pnas.1407717111
Han, Z., He, J., Zou, M., Chen, W., Lv, Y., and Li, Y. (2020). Small interfering RNA target for long noncoding RNA PCGEM1 increases the sensitivity of LNCaP cells to baicalein. Anat. Rec. 303(8), 2077–2085. doi:10.1002/ar.24454
Hanahan, D., and Weinberg, R. A. (2011). Hallmarks of cancer: The next generation. Cell 144 (5), 646–674. doi:10.1016/j.cell.2011.02.013
Hannen, R., and Bartsch, J. W. (2018). Essential roles of telomerase reverse transcriptase hTERT in cancer stemness and metastasis. FEBS Lett. 592 (12), 2023–2031. doi:10.1002/1873-3468.13084
Hardie, D. G., Ross, F. A., and Hawley, S. A. (2012). Ampk: A nutrient and energy sensor that maintains energy homeostasis. Nat. Rev. Mol. Cell Biol. 13 (4), 251–262. doi:10.1038/nrm3311
Hardin, H., Helein, H., Meyer, K., Robertson, S., Zhang, R., Zhong, W., et al. (2018). Thyroid cancer stem-like cell exosomes: Regulation of EMT via transfer of lncRNAs. Laboratory investigation; a J. Tech. methods pathology 98 (9), 1133–1142. doi:10.1038/s41374-018-0065-0
Harrow, J., Frankish, A., Gonzalez, J. M., Tapanari, E., Diekhans, M., Kokocinski, F., et al. (2012). Gencode: The reference human genome annotation for the ENCODE project. Genome Res. 22 (9), 1760–1774. doi:10.1101/gr.135350.111
Heery, R., Finn, S. P., Cuffe, S., and Gray, S. G. (2017). Long non-coding RNAs: Key regulators of epithelial-mesenchymal transition, tumour drug resistance and cancer stem cells. Cancers 9 (4), 38. doi:10.3390/cancers9040038
Hezel, A. F., and Bardeesy, N. (2008). LKB1; linking cell structure and tumor suppression. Oncogene 27 (55), 6908–6919. doi:10.1038/onc.2008.342
Higgs, P. G., and Lehman, N. (2015). The RNA world: Molecular cooperation at the origins of life. Nat. Rev. Genet. 16 (1), 7–17. doi:10.1038/nrg3841
Hoshino, A., Costa-Silva, B., Shen, T. L., Rodrigues, G., Hashimoto, A., Tesic Mark, M., et al. (2015). Tumour exosome integrins determine organotropic metastasis. Nature 527 (7578), 329–335. doi:10.1038/nature15756
Hou, X. M., Yuan, S. Q., Zhao, D., Liu, X. J., and Wu, X. A. (2019). LDH-A promotes malignant behavior via activation of epithelial-to-mesenchymal transition in lung adenocarcinoma. Biosci. Rep. 39 (1). doi:10.1042/BSR20181476
Hrdlickova, B., de Almeida, R. C., Borek, Z., and Withoff, S. (2014). Genetic variation in the non-coding genome: Involvement of micro-RNAs and long non-coding RNAs in disease. Biochimica biophysica acta 1842 (10), 1910–1922. doi:10.1016/j.bbadis.2014.03.011
Hua, H., Zhu, Y., and Song, Y. H. (2018). Ruscogenin suppressed the hepatocellular carcinoma metastasis via PI3K/Akt/mTOR signaling pathway. Biomed. Pharmacother. = Biomedecine Pharmacother. 101, 115–122. doi:10.1016/j.biopha.2018.02.031
Huang, D., Chen, J., Yang, L., Ouyang, Q., Li, J., Lao, L., et al. (2018). NKILA lncRNA promotes tumor immune evasion by sensitizing T cells to activation-induced cell death. Nat. Immunol. 19 (10), 1112–1125. doi:10.1038/s41590-018-0207-y
Huber, V., Camisaschi, C., Berzi, A., Ferro, S., Lugini, L., Triulzi, T., et al. (2017). Cancer acidity: An ultimate frontier of tumor immune escape and a novel target of immunomodulation. Seminars cancer Biol. 43, 74–89. doi:10.1016/j.semcancer.2017.03.001
Hunter, K. W., Amin, R., Deasy, S., Ha, N. H., and Wakefield, L. (2018). Genetic insights into the morass of metastatic heterogeneity. Nat. Rev. Cancer 18 (4), 211–223. doi:10.1038/nrc.2017.126
Huntington, N. D., Cursons, J., and Rautela, J. (2020). The cancer-natural killer cell immunity cycle. Nat. Rev. Cancer 20 (8), 437–454. doi:10.1038/s41568-020-0272-z
Hutchinson, J. N., Ensminger, A. W., Clemson, C. M., Lynch, C. R., Lawrence, J. B., and Chess, A. (2007). A screen for nuclear transcripts identifies two linked noncoding RNAs associated with SC35 splicing domains. BMC genomics 8, 39. doi:10.1186/1471-2164-8-39
Imig, J., Brunschweiger, A., Brümmer, A., Guennewig, B., Mittal, N., Kishore, S., et al. (2015). miR-CLIP capture of a miRNA targetome uncovers a lincRNA H19-miR-106a interaction. Nat. Chem. Biol. 11 (2), 107–114. doi:10.1038/nchembio.1713
Ito, H., Duxbury, M., Zinner, M. J., Ashley, S. W., and Whang, E. E. (2004). Glucose transporter-1 gene expression is associated with pancreatic cancer invasiveness and MMP-2 activity. Surgery 136 (3), 548–556. doi:10.1016/j.surg.2004.05.032
Jackstadt, R., van Hooff, S. R., Leach, J. D., Cortes-Lavaud, X., Lohuis, J. O., Ridgway, R. A., et al. (2019). Epithelial NOTCH signaling rewires the tumor microenvironment of colorectal cancer to drive poor-prognosis subtypes and metastasis. Cancer Cell 36 (3), 319–336. doi:10.1016/j.ccell.2019.08.003
Jarroux, J., Morillon, A., and Pinskaya, M. (2017). History, discovery, and classification of lncRNAs. Adv. Exp. Med. Biol. 1008, 1–46. doi:10.1007/978-981-10-5203-3_1
Jeong, Y. J., Choi, Y., Shin, J. M., Cho, H. J., Kang, J. H., Park, K. K., et al. (2014). Melittin suppresses EGF-induced cell motility and invasion by inhibiting PI3K/Akt/mTOR signaling pathway in breast cancer cells. Food Chem. Toxicol. 68, 218–225. doi:10.1016/j.fct.2014.03.022
Ji, P., Diederichs, S., Wang, W., Böing, S., Metzger, R., Schneider, P. M., et al. (2003). MALAT-1, a novel noncoding RNA, and thymosin beta4 predict metastasis and survival in early-stage non-small cell lung cancer. Oncogene 22 (39), 8031–8041. doi:10.1038/sj.onc.1206928
Ji, Q., Zhang, L., Liu, X., Zhou, L., Wang, W., Han, Z., et al. (2014). Long non-coding RNA MALAT1 promotes tumour growth and metastasis in colorectal cancer through binding to SFPQ and releasing oncogene PTBP2 from SFPQ/PTBP2 complex. Br. J. cancer 111 (4), 736–748. doi:10.1038/bjc.2014.383
Jia, M., Jiang, L., Wang, Y. D., Huang, J. Z., Yu, M., and Xue, H. Z. (2016). lincRNA-p21 inhibits invasion and metastasis of hepatocellular carcinoma through Notch signaling-induced epithelial-mesenchymal transition. Hepatology Res. official J. Jpn. Soc. Hepatology 46 (11), 1137–1144. doi:10.1111/hepr.12659
Jiang, J., Wang, K., Chen, Y., Chen, H., Nice, E. C., and Huang, C. (2017). Redox regulation in tumor cell epithelial-mesenchymal transition: Molecular basis and therapeutic strategy. Signal Transduct. Target. Ther. 2, 17036. doi:10.1038/sigtrans.2017.36
Jiang, R., Tang, J., Chen, Y., Deng, L., Ji, J., Xie, Y., et al. (2017). The long noncoding RNA lnc-EGFR stimulates T-regulatory cells differentiation thus promoting hepatocellular carcinoma immune evasion. Nat. Commun. 8, 15129. doi:10.1038/ncomms15129
Jin, L., Chun, J., Pan, C., Alesi, G. N., Li, D., Magliocca, K. R., et al. (2017). Phosphorylation-mediated activation of LDHA promotes cancer cell invasion and tumour metastasis. Oncogene 36 (27), 3797–3806. doi:10.1038/onc.2017.6
Jin, L., Li, D., Alesi, G. N., Fan, J., Kang, H. B., Lu, Z., et al. (2015). Glutamate dehydrogenase 1 signals through antioxidant glutathione peroxidase 1 to regulate redox homeostasis and tumor growth. Cancer Cell 27 (2), 257–270. doi:10.1016/j.ccell.2014.12.006
Jing, H., Qu, X., Liu, L., and Xia, H. (2018). A novel long noncoding RNA (lncRNA), LL22NC03-N64E9.1, promotes the proliferation of lung cancer cells and is a potential prognostic molecular biomarker for lung cancer. Med. Sci. Monit. Int. Med. J. Exp. Clin. Res. 24, 4317–4323. doi:10.12659/MSM.908359
Jolly, M. K., Somarelli, J. A., Sheth, M., Biddle, A., Tripathi, S. C., Armstrong, A. J., et al. (2019). Hybrid epithelial/mesenchymal phenotypes promote metastasis and therapy resistance across carcinomas. Pharmacol. Ther. 194, 161–184. doi:10.1016/j.pharmthera.2018.09.007
Kaiser, J. (2016). Malignant messengers. Sci. (New York, NY) 352 (6282), 164–166. doi:10.1126/science.352.6282.164
Kaplan, R. N., Psaila, B., and Lyden, D. (2006). Bone marrow cells in the 'pre-metastatic niche': Within bone and beyond. Cancer metastasis Rev. 25 (4), 521–529. doi:10.1007/s10555-006-9036-9
Karki, R., and Kanneganti, T. D. (2019). Diverging inflammasome signals in tumorigenesis and potential targeting. Nat. Rev. Cancer 19 (4), 197–214. doi:10.1038/s41568-019-0123-y
Kathuria, H., Millien, G., McNally, L., Gower, A. C., Tagne, J. B., Cao, Y., et al. (2018). NKX2-1-AS1 negatively regulates CD274/PD-L1, cell-cell interaction genes, and limits human lung carcinoma cell migration. Sci. Rep. 8 (1), 14418. doi:10.1038/s41598-018-32793-5
Kato, K., Cho, B. C., Takahashi, M., Okada, M., Lin, C. Y., Chin, K., et al. (2019). Nivolumab versus chemotherapy in patients with advanced oesophageal squamous cell carcinoma refractory or intolerant to previous chemotherapy (ATTRACTION-3): A multicentre, randomised, open-label, phase 3 trial. Lancet Oncol. 20 (11), 1506–1517. doi:10.1016/S1470-2045(19)30626-6
Katritsis, D., Wilmshurst, P. T., Wendon, J. A., Davies, M. J., and Webb-Peploe, M. M. (1991). Primary restrictive cardiomyopathy: Clinical and pathologic characteristics. J. Am. Coll. Cardiol. 18 (5), 1230–1235. doi:10.1016/0735-1097(91)90540-p
Kim, H. J., Eoh, K. J., Kim, L. K., Nam, E. J., Yoon, S. O., Kim, K. H., et al. (2016). The long noncoding RNA HOXA11 antisense induces tumor progression and stemness maintenance in cervical cancer. Oncotarget 7 (50), 83001–83016. doi:10.18632/oncotarget.12863
Kim, J., Kundu, M., Viollet, B., and Guan, K. L. (2011). AMPK and mTOR regulate autophagy through direct phosphorylation of Ulk1. Nat. Cell Biol. 13 (2), 132–141. doi:10.1038/ncb2152
Kitamura, T., Qian, B. Z., and Pollard, J. W. (2015). Immune cell promotion of metastasis. Nat. Rev. Immunol. 15 (2), 73–86. doi:10.1038/nri3789
Klein, C. A. (2020). Cancer progression and the invisible phase of metastatic colonization. Nat. Rev. Cancer 20 (11), 681–694. doi:10.1038/s41568-020-00300-6
Kotzin, J. J., Spencer, S. P., McCright, S. J., Kumar, D. B. U., Collet, M. A., Mowel, W. K., et al. (2016). The long non-coding RNA Morrbid regulates Bim and short-lived myeloid cell lifespan. Nature 537 (7619), 239–243. doi:10.1038/nature19346
Lai, F., Damle, S. S., Ling, K. K., and Rigo, F. (2020). Directed RNase H cleavage of nascent transcripts causes transcription termination. Mol. Cell 77 (5), 1032–1043. doi:10.1016/j.molcel.2019.12.029
Lai, X. J., and Cheng, H. F. (2018). LncRNA colon cancer-associated transcript 1 (CCAT1) promotes proliferation and metastasis of ovarian cancer via miR-1290. Eur. Rev. Med. Pharmacol. Sci. 22 (2), 322–328. doi:10.26355/eurrev_201801_14175
Lambert, A. W., Pattabiraman, D. R., and Weinberg, R. A. (2017). Emerging biological principles of metastasis. Cell 168 (4), 670–691. doi:10.1016/j.cell.2016.11.037
Lee, H. Y., Son, S. W., Moeng, S., Choi, S. Y., and Park, J. K. (2021). The role of noncoding RNAs in the regulation of anoikis and anchorage-independent growth in cancer. Int. J. Mol. Sci. 22 (2), 627. doi:10.3390/ijms22020627
Lee, J. S., and Mendell, J. T. (2020). Antisense-mediated transcript knockdown triggers premature transcription termination. Mol. Cell 77 (5), 1044–1054. doi:10.1016/j.molcel.2019.12.011
Leucci, E., Vendramin, R., Spinazzi, M., Laurette, P., Fiers, M., Wouters, J., et al. (2016). Melanoma addiction to the long non-coding RNA SAMMSON. Nature 531 (7595), 518–522. doi:10.1038/nature17161
Li, B., Jiang, J., Assaraf, Y. G., Xiao, H., Chen, Z. S., and Huang, C. (2020). Surmounting cancer drug resistance: New insights from the perspective of N(6)-methyladenosine RNA modification. Drug Resist. Updat. 53, 100720. doi:10.1016/j.drup.2020.100720
Li, H. J., Li, X., Pang, H., Pan, J. J., Xie, X. J., and Chen, W. (2015). Long non-coding RNA UCA1 promotes glutamine metabolism by targeting miR-16 in human bladder cancer. Jpn. J. Clin. Oncol. 45 (11), 1055–1063. doi:10.1093/jjco/hyv132
Li, M., Pan, M., Wang, J., You, C., Zhao, F., Zheng, D., et al. (2020). miR-7 reduces breast cancer stem cell metastasis via inhibiting RELA to decrease ESAM expression. Mol. Ther. oncolytics 18, 70–82. doi:10.1016/j.omto.2020.06.002
Li, S., Li, J., Chen, C., Zhang, R., and Wang, K. (2018). Pan-cancer analysis of long non-coding RNA NEAT1 in various cancers. Genes & Dis. 5 (1), 27–35. doi:10.1016/j.gendis.2017.11.003
Li, T., Xie, J., Shen, C., Cheng, D., Shi, Y., Wu, Z., et al. (2015). Amplification of long noncoding RNA ZFAS1 promotes metastasis in hepatocellular carcinoma. Cancer Res. 75 (15), 3181–3191. doi:10.1158/0008-5472.CAN-14-3721
Li, Z., Jiang, P., Li, J., Peng, M., Zhao, X., Zhang, X., et al. (2018). Tumor-derived exosomal lnc-Sox2ot promotes EMT and stemness by acting as a ceRNA in pancreatic ductal adenocarcinoma. Oncogene 37 (28), 3822–3838. doi:10.1038/s41388-018-0237-9
Lin, R., Maeda, S., Liu, C., Karin, M., and Edgington, T. S. (2007). A large noncoding RNA is a marker for murine hepatocellular carcinomas and a spectrum of human carcinomas. Oncogene 26 (6), 851–858. doi:10.1038/sj.onc.1209846
Lin, Y. H., Wu, M. H., Huang, Y. H., Yeh, C. T., Cheng, M. L., Chi, H. C., et al. (2018). Taurine up-regulated gene 1 functions as a master regulator to coordinate glycolysis and metastasis in hepatocellular carcinoma. Hepatol. Baltim. Md) 67 (1), 188–203. doi:10.1002/hep.29462
Lin, Y. H., Wu, M. H., Yeh, C. T., and Lin, K. H. (2018). Long non-coding RNAs as mediators of tumor microenvironment and liver cancer cell communication. Int. J. Mol. Sci. 19 (12), 3742. doi:10.3390/ijms19123742
Liu, N., Feng, S., Li, H., Chen, X., Bai, S., and Liu, Y. (2020). Long non-coding RNA MALAT1 facilitates the tumorigenesis, invasion and glycolysis of multiple myeloma via miR-1271-5p/SOX13 axis. J. cancer Res. Clin. Oncol. 146 (2), 367–379. doi:10.1007/s00432-020-03127-8
Liu, R., Li, X., Zhu, W., Wang, Y., Zhao, D., Wang, X., et al. (2019). Cholangiocyte-derived exosomal long noncoding RNA H19 promotes hepatic stellate cell activation and cholestatic liver fibrosis. Hepatol. Baltim. Md) 70 (4), 1317–1335. doi:10.1002/hep.30662
Liu, S. J., Dang, H. X., Lim, D. A., Feng, F. Y., and Maher, C. A. (2021). Long noncoding RNAs in cancer metastasis. Nat. Rev. Cancer 21 (7), 446–460. doi:10.1038/s41568-021-00353-1
Liu, S. J., and Lim, D. A. (2018). Modulating the expression of long non-coding RNAs for functional studies. EMBO Rep. 19 (12), e46955. doi:10.15252/embr.201846955
Liu, W., Le, A., Hancock, C., Lane, A. N., Dang, C. V., Fan, T. W., et al. (2012). Reprogramming of proline and glutamine metabolism contributes to the proliferative and metabolic responses regulated by oncogenic transcription factor c-MYC. Proc. Natl. Acad. Sci. U. S. A. 109 (23), 8983–8988. doi:10.1073/pnas.1203244109
Liu, X., Fu, Q., Bian, X., Fu, Y., Xin, J., Liang, N., et al. (2020). Long non-coding RNA MAPK8IP1P2 inhibits lymphatic metastasis of thyroid cancer by activating Hippo signaling via sponging miR-146b-3p. Front. Oncol. 10, 600927. doi:10.3389/fonc.2020.600927
Liu, X., Fu, Q., Li, S., Liang, N., Li, F., Li, C., et al. (2019). LncRNA FOXD2-AS1 functions as a competing endogenous RNA to regulate TERT expression by sponging miR-7-5p in thyroid cancer. Front. Endocrinol. 10, 207. doi:10.3389/fendo.2019.00207
Liu, X. H., Liu, Z. L., Sun, M., Liu, J., Wang, Z. X., and De, W. (2013). The long non-coding RNA HOTAIR indicates a poor prognosis and promotes metastasis in non-small cell lung cancer. BMC cancer 13, 464. doi:10.1186/1471-2407-13-464
Liu, Y., and Cao, X. (2016). Characteristics and significance of the pre-metastatic niche. Cancer Cell 30 (5), 668–681. doi:10.1016/j.ccell.2016.09.011
Liu, Y., Du, Y., Hu, X., Zhao, L., and Xia, W. (2018). Up-regulation of ceRNA TINCR by SP1 contributes to tumorigenesis in breast cancer. BMC cancer 18 (1), 367. doi:10.1186/s12885-018-4255-3
Liu, Z., Li, H., Fan, S., Lin, H., and Lian, W. (2019). STAT3-induced upregulation of long noncoding RNA HNF1A-AS1 promotes the progression of oral squamous cell carcinoma via activating Notch signaling pathway. Cancer Biol. Ther. 20 (4), 444–453. doi:10.1080/15384047.2018.1529119
Loo, J. M., Scherl, A., Nguyen, A., Man, F. Y., Weinberg, E., Zeng, Z., et al. (2015). Extracellular metabolic energetics can promote cancer progression. Cell 160 (3), 393–406. doi:10.1016/j.cell.2014.12.018
Lu, J., Tan, M., and Cai, Q. (2015). The Warburg effect in tumor progression: Mitochondrial oxidative metabolism as an anti-metastasis mechanism. Cancer Lett. 356 (2), 156–164. doi:10.1016/j.canlet.2014.04.001
Lu, J. (2019). The Warburg metabolism fuels tumor metastasis. Cancer metastasis Rev. 38 (1-2), 157–164. doi:10.1007/s10555-019-09794-5
Lu, Q., Wang, L., Gao, Y., Zhu, P., Li, L., Wang, X., et al. (2021). lncRNA APOC1P1-3 promoting anoikis-resistance of breast cancer cells. Cancer Cell Int. 21 (1), 232. doi:10.1186/s12935-021-01916-w
Luan, W., Zhang, X., Ruan, H., Wang, J., and Bu, X. (2019). Long noncoding RNA OIP5-AS1 acts as a competing endogenous RNA to promote glutamine catabolism and malignant melanoma growth by sponging miR-217. J. Cell. physiology 234, 16609–16618. doi:10.1002/jcp.28335
Luo, J. H., Ren, B., Keryanov, S., Tseng, G. C., Rao, U. N., Monga, S. P., et al. (2006). Transcriptomic and genomic analysis of human hepatocellular carcinomas and hepatoblastomas. Hepatol. Baltim. Md) 44 (4), 1012–1024. doi:10.1002/hep.21328
Luo, X., Qiu, Y., Jiang, Y., Chen, F., Jiang, L., Zhou, Y., et al. (2018). Long non-coding RNA implicated in the invasion and metastasis of head and neck cancer: Possible function and mechanisms. Mol. cancer 17 (1), 14. doi:10.1186/s12943-018-0763-7
Ma, H., Su, R., Feng, H., Guo, Y., and Su, G. (2019). Long noncoding RNA UCA1 promotes osteosarcoma metastasis through CREB1-mediated epithelial-mesenchymal transition and activating PI3K/AKT/mTOR pathway. J. bone Oncol. 16, 100228. doi:10.1016/j.jbo.2019.100228
Ma, P., Pan, Y., Li, W., Sun, C., Liu, J., Xu, T., et al. (2017). Extracellular vesicles-mediated noncoding RNAs transfer in cancer. J. Hematol. Oncol. 10 (1), 57. doi:10.1186/s13045-017-0426-y
Macha, M. A., Wani, N. A., Ganai, R. A., Bhat, A. A., Hamid, A., Hashem, S., et al. (2020). Recent advances in head and neck tumor microenvironment-based therapy. Adv. Exp. Med. Biol. 1296, 11–31. doi:10.1007/978-3-030-59038-3_2
Magalhaes, M. A., Larson, D. R., Mader, C. C., Bravo-Cordero, J. J., Gil-Henn, H., Oser, M., et al. (2011). Cortactin phosphorylation regulates cell invasion through a pH-dependent pathway. J. Cell Biol. 195 (5), 903–920. doi:10.1083/jcb.201103045
Malakar, P., Stein, I., Saragovi, A., Winkler, R., Stern-Ginossar, N., Berger, M., et al. (2019). Long noncoding RNA MALAT1 regulates cancer glucose metabolism by enhancing mTOR-mediated translation of TCF7L2. Cancer Res. 79 (10), 2480–2493. doi:10.1158/0008-5472.CAN-18-1432
Marin-Valencia, I., Yang, C., Mashimo, T., Cho, S., Baek, H., Yang, X. L., et al. (2012). Analysis of tumor metabolism reveals mitochondrial glucose oxidation in genetically diverse human glioblastomas in the mouse brain in vivo. Cell metab. 15 (6), 827–837. doi:10.1016/j.cmet.2012.05.001
Marquardt, J. U. (2018). The role of transforming growth factor-β in human hepatocarcinogenesis: Mechanistic and therapeutic implications from an integrative multiomics approach. Gastroenterology 154 (1), 17–20. doi:10.1053/j.gastro.2017.11.015
Mashimo, T., Pichumani, K., Vemireddy, V., Hatanpaa, K. J., Singh, D. K., Sirasanagandla, S., et al. (2014). Acetate is a bioenergetic substrate for human glioblastoma and brain metastases. Cell 159 (7), 1603–1614. doi:10.1016/j.cell.2014.11.025
Massagué, J., and Obenauf, A. C. (2016). Metastatic colonization by circulating tumour cells. Nature 529 (7586), 298–306. doi:10.1038/nature17038
Matouk, I. J., DeGroot, N., Mezan, S., Ayesh, S., Abu-lail, R., Hochberg, A., et al. (2007). The H19 non-coding RNA is essential for human tumor growth. PloS one 2 (9), e845. doi:10.1371/journal.pone.0000845
Mehraj, U., Ganai, R. A., Macha, M. A., Hamid, A., Zargar, M. A., Bhat, A. A., et al. (2021). The tumor microenvironment as driver of stemness and therapeutic resistance in breast cancer: New challenges and therapeutic opportunities. Cell. Oncol. Dordr. 44 (6), 1209–1229. doi:10.1007/s13402-021-00634-9
Mercer, T. R., Dinger, M. E., and Mattick, J. S. (2009). Long non-coding RNAs: Insights into functions. Nat. Rev. Genet. 10 (3), 155–159. doi:10.1038/nrg2521
Ming, H., Li, B., Zhou, L., Goel, A., and Huang, C. (2021). Long non-coding RNAs and cancer metastasis: Molecular basis and therapeutic implications. Biochimica biophysica acta Rev. cancer 1875 (2), 188519. doi:10.1016/j.bbcan.2021.188519
Moloney, J. N., and Cotter, T. G. (2018). ROS signalling in the biology of cancer. Seminars Cell & Dev. Biol. 80, 50–64. doi:10.1016/j.semcdb.2017.05.023
Motzer, R. J., Rini, B. I., McDermott, D. F., Arén Frontera, O., Hammers, H. J., Carducci, M. A., et al. (2019). Nivolumab plus ipilimumab versus sunitinib in first-line treatment for advanced renal cell carcinoma: Extended follow-up of efficacy and safety results from a randomised, controlled, phase 3 trial. Lancet Oncol. 20 (10), 1370–1385. doi:10.1016/S1470-2045(19)30413-9
Murdoch, C., Muthana, M., Coffelt, S. B., and Lewis, C. E. (2008). The role of myeloid cells in the promotion of tumour angiogenesis. Nat. Rev. Cancer 8 (8), 618–631. doi:10.1038/nrc2444
Nagarajan, A., Dogra, S. K., Sun, L., Gandotra, N., Ho, T., Cai, G., et al. (2017). Paraoxonase 2 facilitates pancreatic cancer growth and metastasis by stimulating GLUT1-mediated glucose transport. Mol. Cell 67 (4), 685–701. doi:10.1016/j.molcel.2017.07.014
Naqvi, A. R., Islam, M. N., Choudhury, N. R., and Haq, Q. M. (2009). The fascinating world of RNA interference. Int. J. Biol. Sci. 5 (2), 97–117. doi:10.7150/ijbs.5.97
Neophytou, C. M., Panagi, M., Stylianopoulos, T., and Papageorgis, P. (2021). The role of tumor microenvironment in cancer metastasis: Molecular mechanisms and therapeutic opportunities. Cancers 13 (9), 2053. doi:10.3390/cancers13092053
Nieto, M. A., Huang, R. Y., Jackson, R. A., and Thiery, J. P. (2016). Emt: 2016. Cell 166 (1), 21–45. doi:10.1016/j.cell.2016.06.028
Nisar, S., Bhat, A. A., Singh, M., Karedath, T., Rizwan, A., Hashem, S., et al. (2021). Insights into the role of CircRNAs: Biogenesis, characterization, functional, and clinical impact in human malignancies. Front. Cell Dev. Biol. 9, 617281. doi:10.3389/fcell.2021.617281
Nisar, S., Yousuf, P., Masoodi, T., Wani, N. A., Hashem, S., Singh, M., et al. (2021). Chemokine-cytokine networks in the head and neck tumor microenvironment. Int. J. Mol. Sci. 22 (9), 4584. doi:10.3390/ijms22094584
Nogués, L., Benito-Martin, A., Hergueta-Redondo, M., and Peinado, H. (2018). The influence of tumour-derived extracellular vesicles on local and distal metastatic dissemination. Mol. aspects Med. 60, 15–26. doi:10.1016/j.mam.2017.11.012
Okuda, H., Xing, F., Pandey, P. R., Sharma, S., Watabe, M., Pai, S. K., et al. (2013). miR-7 suppresses brain metastasis of breast cancer stem-like cells by modulating KLF4. Cancer Res. 73 (4), 1434–1444. doi:10.1158/0008-5472.CAN-12-2037
Okugawa, Y., Toiyama, Y., Hur, K., Toden, S., Saigusa, S., Tanaka, K., et al. (2014). Metastasis-associated long non-coding RNA drives gastric cancer development and promotes peritoneal metastasis. Carcinogenesis 35 (12), 2731–2739. doi:10.1093/carcin/bgu200
Ott, P. A., Hodi, F. S., and Robert, C. (2013). CTLA-4 and PD-1/PD-L1 blockade: New immunotherapeutic modalities with durable clinical benefit in melanoma patients. Clin. cancer Res. 19 (19), 5300–5309. doi:10.1158/1078-0432.CCR-13-0143
Paoli, P., Giannoni, E., and Chiarugi, P. (2013). Anoikis molecular pathways and its role in cancer progression. Biochimica biophysica acta 1833 (12), 3481–3498. doi:10.1016/j.bbamcr.2013.06.026
Parasramka, M. A., Maji, S., Matsuda, A., Yan, I. K., and Patel, T. (2016). Long non-coding RNAs as novel targets for therapy in hepatocellular carcinoma. Pharmacol. Ther. 161, 67–78. doi:10.1016/j.pharmthera.2016.03.004
Pardini, B., and Calin, G. A. (2019). MicroRNAs and long non-coding RNAs and their hormone-like activities in cancer. Cancers 11 (3), 378. doi:10.3390/cancers11030378
Pei, X., Wang, X., and Li, H. (2018). LncRNA SNHG1 regulates the differentiation of Treg cells and affects the immune escape of breast cancer via regulating miR-448/Ido. Int. J. Biol. Macromol. 118, 24–30. doi:10.1016/j.ijbiomac.2018.06.033
Peinado, H., Zhang, H., Matei, I. R., Costa-Silva, B., Hoshino, A., Rodrigues, G., et al. (2017). Pre-metastatic niches: Organ-specific homes for metastases. Nat. Rev. Cancer 17 (5), 302–317. doi:10.1038/nrc.2017.6
Phan, L. M., Yeung, S. C., and Lee, M. H. (2014). Cancer metabolic reprogramming: Importance, main features, and potentials for precise targeted anti-cancer therapies. Cancer Biol. Med. 11 (1), 1–19. doi:10.7497/j.issn.2095-3941.2014.01.001
Pirollo, K. F., Rait, A., Zhou, Q., Hwang, S. H., Dagata, J. A., Zon, G., et al. (2007). Materializing the potential of small interfering RNA via a tumor-targeting nanodelivery system. Cancer Res. 67 (7), 2938–2943. doi:10.1158/0008-5472.CAN-06-4535
Postow, M. A., Callahan, M. K., and Wolchok, J. D. (2015). Immune checkpoint blockade in cancer therapy. J. Clin. Oncol. official J. Am. Soc. Clin. Oncol. 33 (17), 1974–1982. doi:10.1200/JCO.2014.59.4358
Psaila, B., and Lyden, D. (2009). The metastatic niche: Adapting the foreign soil. Nat. Rev. Cancer 9 (4), 285–293. doi:10.1038/nrc2621
Pu, H., Zheng, Q., Li, H., Wu, M., An, J., Gui, X., et al. (2015). CUDR promotes liver cancer stem cell growth through upregulating TERT and C-Myc. Oncotarget 6 (38), 40775–40798. doi:10.18632/oncotarget.5805
Qi, C., Xiaofeng, C., Dongen, L., Liang, Y., Liping, X., Yue, H., et al. (2019). Long non-coding RNA MACC1-AS1 promoted pancreatic carcinoma progression through activation of PAX8/NOTCH1 signaling pathway. J. Exp. Clin. cancer Res. CR 38 (1), 344. doi:10.1186/s13046-019-1332-7
Qin, C. F., and Zhao, F. L. (2017). Long non-coding RNA TUG1 can promote proliferation and migration of pancreatic cancer via EMT pathway. Eur. Rev. Med. Pharmacol. Sci. 21 (10), 2377–2384.
Qin, S., Jiang, J., Lu, Y., Nice, E. C., Huang, C., Zhang, J., et al. (2020). Emerging role of tumor cell plasticity in modifying therapeutic response. Signal Transduct. Target. Ther. 5 (1), 228. doi:10.1038/s41392-020-00313-5
Quail, D. F., and Joyce, J. A. (2013). Microenvironmental regulation of tumor progression and metastasis. Nat. Med. 19 (11), 1423–1437. doi:10.1038/nm.3394
Quinn, J. J., and Chang, H. Y. (2016). Unique features of long non-coding RNA biogenesis and function. Nat. Rev. Genet. 17 (1), 47–62. doi:10.1038/nrg.2015.10
Rainier, S., Johnson, L. A., Dobry, C. J., Ping, A. J., Grundy, P. E., and Feinberg, A. P. (1993). Relaxation of imprinted genes in human cancer. Nature 362 (6422), 747–749. doi:10.1038/362747a0
Raveh, E., Matouk, I. J., Gilon, M., and Hochberg, A. (2015). The H19 Long non-coding RNA in cancer initiation, progression and metastasis - a proposed unifying theory. Mol. cancer 14, 184. doi:10.1186/s12943-015-0458-2
Ren, S., Liu, Y., Xu, W., Sun, Y., Lu, J., Wang, F., et al. (2013). Long noncoding RNA MALAT-1 is a new potential therapeutic target for castration resistant prostate cancer. J. urology 190 (6), 2278–2287. doi:10.1016/j.juro.2013.07.001
Rider, P., Carmi, Y., and Cohen, I. (2016). Biologics for targeting inflammatory cytokines, clinical uses, and limitations. Int. J. Cell Biol. 2016, 9259646. doi:10.1155/2016/9259646
Rinn, J. L., Kertesz, M., Wang, J. K., Squazzo, S. L., Xu, X., Brugmann, S. A., et al. (2007). Functional demarcation of active and silent chromatin domains in human HOX loci by noncoding RNAs. Cell 129 (7), 1311–1323. doi:10.1016/j.cell.2007.05.022
Rohani, N., Hao, L., Alexis, M. S., Joughin, B. A., Krismer, K., Moufarrej, M. N., et al. (2019). Acidification of tumor at stromal boundaries drives transcriptome alterations associated with aggressive phenotypes. Cancer Res. 79 (8), 1952–1966. doi:10.1158/0008-5472.CAN-18-1604
Ruffell, B., Affara, N. I., and Coussens, L. M. (2012). Differential macrophage programming in the tumor microenvironment. Trends Immunol. 33 (3), 119–126. doi:10.1016/j.it.2011.12.001
Sakamoto, S., and Kyprianou, N. (2010). Targeting anoikis resistance in prostate cancer metastasis. Mol. aspects Med. 31 (2), 205–214. doi:10.1016/j.mam.2010.02.001
Sang, L. J., Ju, H. Q., Liu, G. P., Tian, T., Ma, G. L., Lu, Y. X., et al. (2018). LncRNA CamK-A regulates Ca(2+)-signaling-mediated tumor microenvironment remodeling. Mol. Cell 72 (1), 601–683. doi:10.1016/j.molcel.2018.10.024
Scadden, D. T. (2014). Nice neighborhood: Emerging concepts of the stem cell niche. Cell 157 (1), 41–50. doi:10.1016/j.cell.2014.02.013
Schmitt, A. M., and Chang, H. Y. (2016). Long noncoding RNAs in cancer pathways. Cancer Cell 29 (4), 452–463. doi:10.1016/j.ccell.2016.03.010
Schofield, R. (1978). The relationship between the spleen colony-forming cell and the haemopoietic stem cell. Blood cells 4 (1-2), 7–25.
Seitz, A. K., Christensen, L. L., Christensen, E., Faarkrog, K., Ostenfeld, M. S., Hedegaard, J., et al. (2017). Profiling of long non-coding RNAs identifies LINC00958 and LINC01296 as candidate oncogenes in bladder cancer. Sci. Rep. 7 (1), 395. doi:10.1038/s41598-017-00327-0
Sellitto, A., Pecoraro, G., Giurato, G., Nassa, G., Rizzo, F., Saggese, P., et al. (2021). Regulation of metabolic reprogramming by long non-coding RNAs in cancer. Cancers 13 (14), 3485. doi:10.3390/cancers13143485
Shang, A., Wang, W., Gu, C., Chen, C., Zeng, B., Yang, Y., et al. (2019). Long non-coding RNA HOTTIP enhances IL-6 expression to potentiate immune escape of ovarian cancer cells by upregulating the expression of PD-L1 in neutrophils. J. Exp. Clin. cancer Res. CR 38 (1), 411. doi:10.1186/s13046-019-1394-6
Shao, S., Zhao, X., Zhang, X., Luo, M., Zuo, X., Huang, S., et al. (2015). Notch1 signaling regulates the epithelial-mesenchymal transition and invasion of breast cancer in a Slug-dependent manner. Mol. cancer 14 (1), 28. doi:10.1186/s12943-015-0295-3
Shen, X., Zhao, W., Zhang, Y., and Liang, B. (2020). Long non-coding RNA-NEAT1 promotes cell migration and invasion via regulating miR-124/NF-κB pathway in cervical cancer. OncoTargets Ther. 13, 3265–3276. doi:10.2147/OTT.S220306
Shu, Y., and Cheng, P. (2020). Targeting tumor-associated macrophages for cancer immunotherapy. Biochimica biophysica acta Rev. cancer 1874 (2), 188434. doi:10.1016/j.bbcan.2020.188434
Shu, Y., Pi, F., Sharma, A., Rajabi, M., Haque, F., Shu, D., et al. (2014). Stable RNA nanoparticles as potential new generation drugs for cancer therapy. Adv. drug Deliv. Rev. 66, 74–89. doi:10.1016/j.addr.2013.11.006
Siegel, R. L., Miller, K. D., Wagle, N. S., and Jemal, A. (2023). Cancer statistics, 2023. CA a cancer J. Clin. 73 (1), 17–48. doi:10.3322/caac.21763
Simpson, C. D., Anyiwe, K., and Schimmer, A. D. (2008). Anoikis resistance and tumor metastasis. Cancer Lett. 272 (2), 177–185. doi:10.1016/j.canlet.2008.05.029
Singh, P. K., Brand, R. E., and Mehla, K. (2012). MicroRNAs in pancreatic cancer metabolism. Nat. Rev. Gastroenterology hepatology 9 (6), 334–344. doi:10.1038/nrgastro.2012.63
Siu, M. K. Y., Jiang, Y. X., Wang, J. J., Leung, T. H. Y., Han, C. Y., Tsang, B. K., et al. (2019). Hexokinase 2 regulates ovarian cancer cell migration, invasion and stemness via FAK/ERK1/2/MMP9/NANOG/SOX9 signaling cascades. Cancers 11 (6), 813. doi:10.3390/cancers11060813
Slack, F. J., and Chinnaiyan, A. M. (2019). The role of non-coding RNAs in oncology. Cell 179 (5), 1033–1055. doi:10.1016/j.cell.2019.10.017
Sleeman, J. P. (2012). The metastatic niche and stromal progression. Cancer metastasis Rev. 31 (3-4), 429–440. doi:10.1007/s10555-012-9373-9
Su, J., Morgani, S. M., David, C. J., Wang, Q., Er, E. E., Huang, Y. H., et al. (2020). TGF-β orchestrates fibrogenic and developmental EMTs via the RAS effector RREB1. Nature 577 (7791), 566–571. doi:10.1038/s41586-019-1897-5
Sun, H., Huang, Z., Sheng, W., and Xu, M. D. (2018). Emerging roles of long non-coding RNAs in tumor metabolism. J. Hematol. Oncol. 11 (1), 106. doi:10.1186/s13045-018-0648-7
Sun, L., Li, J., Yan, W., Yao, Z., Wang, R., Zhou, X., et al. (2021). H19 promotes aerobic glycolysis, proliferation, and immune escape of gastric cancer cells through the microRNA-519d-3p/lactate dehydrogenase A axis. Cancer Sci. 112 (6), 2245–2259. doi:10.1111/cas.14896
Sun, W. L., Kang, T., Wang, Y. Y., Sun, J. P., Li, C., Liu, H. J., et al. (2019). Long noncoding RNA OIP5-AS1 targets Wnt-7b to affect glioma progression via modulation of miR-410. Biosci. Rep. 39 (1). doi:10.1042/BSR20180395
Sun, Z., Yang, S., Zhou, Q., Wang, G., Song, J., Li, Z., et al. (2018). Emerging role of exosome-derived long non-coding RNAs in tumor microenvironment. Mol. cancer 17 (1), 82. doi:10.1186/s12943-018-0831-z
Sung, H., Ferlay, J., Siegel, R. L., Laversanne, M., Soerjomataram, I., Jemal, A., et al. (2021). Global cancer statistics 2020: GLOBOCAN estimates of incidence and mortality worldwide for 36 cancers in 185 countries. CA a cancer J. Clin. 71 (3), 209–249. doi:10.3322/caac.21660
Szatanek, R., Baj-Krzyworzeka, M., Zimoch, J., Lekka, M., Siedlar, M., and Baran, J. (2017). The methods of choice for extracellular vesicles (EVs) characterization. Int. J. Mol. Sci. 18 (6), 1153. doi:10.3390/ijms18061153
Tajbakhsh, A., Rivandi, M., Abedini, S., Pasdar, A., and Sahebkar, A. (2019). Regulators and mechanisms of anoikis in triple-negative breast cancer (tnbc): A review. Crit. Rev. oncology/hematology 140, 17–27. doi:10.1016/j.critrevonc.2019.05.009
Talmadge, J. E., and Fidler, I. J. (2010). AACR centennial series: The biology of cancer metastasis: Historical perspective. Cancer Res. 70 (14), 5649–5669. doi:10.1158/0008-5472.CAN-10-1040
Tan, S. K., Pastori, C., Penas, C., Komotar, R. J., Ivan, M. E., Wahlestedt, C., et al. (2018). Serum long noncoding RNA HOTAIR as a novel diagnostic and prognostic biomarker in glioblastoma multiforme. Mol. cancer 17 (1), 74. doi:10.1186/s12943-018-0822-0
Tang, Y., He, Y., Zhang, P., Wang, J., Fan, C., Yang, L., et al. (2018). LncRNAs regulate the cytoskeleton and related Rho/ROCK signaling in cancer metastasis. Mol. cancer 17 (1), 77. doi:10.1186/s12943-018-0825-x
Tauriello, D. V. F., Palomo-Ponce, S., Stork, D., Berenguer-Llergo, A., Badia-Ramentol, J., Iglesias, M., et al. (2018). TGFβ drives immune evasion in genetically reconstituted colon cancer metastasis. Nature 554 (7693), 538–543. doi:10.1038/nature25492
Thomas, M., Kularatne, S. A., Qi, L., Kleindl, P., Leamon, C. P., Hansen, M. J., et al. (2009). Ligand-targeted delivery of small interfering RNAs to malignant cells and tissues. Ann. N. Y. Acad. Sci. 1175, 32–39. doi:10.1111/j.1749-6632.2009.04977.x
Tian, H., Lian, R., Li, Y., Liu, C., Liang, S., Li, W., et al. (2020). AKT-induced lncRNA VAL promotes EMT-independent metastasis through diminishing Trim16-dependent Vimentin degradation. Nat. Commun. 11 (1), 5127. doi:10.1038/s41467-020-18929-0
Topel, H., Bagirsakci, E., Comez, D., Bagci, G., Cakan-Akdogan, G., and Atabey, N. (2020). lncRNA HOTAIR overexpression induced downregulation of c-Met signaling promotes hybrid epithelial/mesenchymal phenotype in hepatocellular carcinoma cells. Cell Commun. Signal. CCS 18 (1), 110. doi:10.1186/s12964-020-00602-0
Tumeh, P. C., Harview, C. L., Yearley, J. H., Shintaku, I. P., Taylor, E. J., Robert, L., et al. (2014). PD-1 blockade induces responses by inhibiting adaptive immune resistance. Nature 515 (7528), 568–571. doi:10.1038/nature13954
Vander Heiden, M. G., Cantley, L. C., and Thompson, C. B. (2009). Understanding the Warburg effect: The metabolic requirements of cell proliferation. Sci. (New York, NY) 324 (5930), 1029–1033. doi:10.1126/science.1160809
Vara-Perez, M., Maes, H., Van Dingenen, S., and Agostinis, P. (2019). BNIP3 contributes to the glutamine-driven aggressive behavior of melanoma cells. Biol. Chem. 400 (2), 187–193. doi:10.1515/hsz-2018-0208
Wang, J., Shao, N., Ding, X., Tan, B., Song, Q., Wang, N., et al. (2016). Crosstalk between transforming growth factor-β signaling pathway and long non-coding RNAs in cancer. Cancer Lett. 370 (2), 296–301. doi:10.1016/j.canlet.2015.11.007
Wang, K. C., and Chang, H. Y. (2011). Molecular mechanisms of long noncoding RNAs. Mol. Cell 43 (6), 904–914. doi:10.1016/j.molcel.2011.08.018
Wang, Q. M., Lian, G. Y., Song, Y., Huang, Y. F., and Gong, Y. (2019). LncRNA MALAT1 promotes tumorigenesis and immune escape of diffuse large B cell lymphoma by sponging miR-195. Life Sci. 231, 116335. doi:10.1016/j.lfs.2019.03.040
Wang, T., Cao, L., Dong, X., Wu, F., De, W., Huang, L., et al. (2020). LINC01116 promotes tumor proliferation and neutrophil recruitment via DDX5-mediated regulation of IL-1β in glioma cell. Cell death Dis. 11 (5), 302. doi:10.1038/s41419-020-2506-0
Wang, Y., Su, M. A., and Wan, Y. Y. (2011). An essential role of the transcription factor GATA-3 for the function of regulatory T cells. Immunity 35 (3), 337–348. doi:10.1016/j.immuni.2011.08.012
Wang, Y., Zhang, X., Wang, Z., Hu, Q., Wu, J., Li, Y., et al. (2018). LncRNA-p23154 promotes the invasion-metastasis potential of oral squamous cell carcinoma by regulating Glut1-mediated glycolysis. Cancer Lett. 434, 172–183. doi:10.1016/j.canlet.2018.07.016
Wang, Z. Y., Hu, M., Dai, M. H., Xiong, J., Zhang, S., Wu, H. J., et al. (2018). Upregulation of the long non-coding RNA AFAP1-AS1 affects the proliferation, invasion and survival of tongue squamous cell carcinoma via the Wnt/β-catenin signaling pathway. Mol. cancer 17 (1), 3. doi:10.1186/s12943-017-0752-2
Warburg, O. (1956). On the origin of cancer cells. Sci. (New York, NY) 123 (3191), 309–314. doi:10.1126/science.123.3191.309
Weber, G. F. (2016). Time and circumstances: Cancer cell metabolism at various stages of disease progression. Front. Oncol. 6, 257. doi:10.3389/fonc.2016.00257
Wei, B., Kong, W., Mou, X., and Wang, S. (2019). Comprehensive analysis of tumor immune infiltration associated with endogenous competitive RNA networks in lung adenocarcinoma. Pathology, Res. Pract. 215 (1), 159–170. doi:10.1016/j.prp.2018.10.032
Wei, S., Wang, K., Huang, X., Zhao, Z., and Zhao, Z. (2019). LncRNA MALAT1 contributes to non-small cell lung cancer progression via modulating miR-200a-3p/programmed death-ligand 1 axis. Int. J. Immunopathol. Pharmacol. 33, 2058738419859699. doi:10.1177/2058738419859699
Weidle, U. H., Birzele, F., Kollmorgen, G., and Rüger, R. (2017). Long non-coding RNAs and their role in metastasis. Cancer genomics & proteomics 14 (3), 143–160. doi:10.21873/cgp.20027
Wen, Z., Lian, L., Ding, H., Hu, Y., Xiao, Z., Xiong, K., et al. (2020). LncRNA ANCR promotes hepatocellular carcinoma metastasis through upregulating HNRNPA1 expression. RNA Biol. 17 (3), 381–394. doi:10.1080/15476286.2019.1708547
Wieland, E., Rodriguez-Vita, J., Liebler, S. S., Mogler, C., Moll, I., Herberich, S. E., et al. (2017). Endothelial Notch1 activity facilitates metastasis. Cancer Cell 31 (3), 355–367. doi:10.1016/j.ccell.2017.01.007
Williams, E. D., Gao, D., Redfern, A., and Thompson, E. W. (2019). Controversies around epithelial-mesenchymal plasticity in cancer metastasis. Nat. Rev. Cancer 19 (12), 716–732. doi:10.1038/s41568-019-0213-x
Wong, C. M., Tsang, F. H., and Ng, I. O. (2018). Non-coding RNAs in hepatocellular carcinoma: Molecular functions and pathological implications. Nat. Rev. Gastroenterology hepatology 15 (3), 137–151. doi:10.1038/nrgastro.2017.169
Wu, S., Zheng, Q., Xing, X., Dong, Y., Wang, Y., You, Y., et al. (2018). Matrix stiffness-upregulated LOXL2 promotes fibronectin production, MMP9 and CXCL12 expression and BMDCs recruitment to assist pre-metastatic niche formation. J. Exp. Clin. cancer Res. CR 37 (1), 99. doi:10.1186/s13046-018-0761-z
Wurster, C. D., and Ludolph, A. C. (2018). Antisense oligonucleotides in neurological disorders. Ther. Adv. neurological Disord. 11, 1756286418776932. doi:10.1177/1756286418776932
Xiao, Y., Lin, M., Jiang, X., Ye, J., Guo, T., Shi, Y., et al. (2017)., 2017. Amsterdam, 5108653. doi:10.1155/2017/5108653The recent advances on liver cancer stem cells: Biomarkers, separation, and therapyAnal. Cell. Pathol.
Xie, C., Guo, Y., and Lou, S. (2020). LncRNA ANCR promotes invasion and migration of gastric cancer by regulating FoxO1 expression to inhibit macrophage M1 polarization. Dig. Dis. Sci. 65 (10), 2863–2872. doi:10.1007/s10620-019-06019-1
Xie, Y., Dang, W., Zhang, S., Yue, W., Yang, L., Zhai, X., et al. (2019). The role of exosomal noncoding RNAs in cancer. Mol. cancer 18 (1), 37. doi:10.1186/s12943-019-0984-4
Xu, J., Lamouille, S., and Derynck, R. (2009). TGF-beta-induced epithelial to mesenchymal transition. Cell Res. 19 (2), 156–172. doi:10.1038/cr.2009.5
Yamada, K., Kano, J., Tsunoda, H., Yoshikawa, H., Okubo, C., Ishiyama, T., et al. (2006). Phenotypic characterization of endometrial stromal sarcoma of the uterus. Cancer Sci. 97 (2), 106–112. doi:10.1111/j.1349-7006.2006.00147.x
Yamamoto, Y., Loriot, Y., Beraldi, E., Zhang, F., Wyatt, A. W., Al Nakouzi, N., et al. (2015). Generation 2.5 antisense oligonucleotides targeting the androgen receptor and its splice variants suppress enzalutamide-resistant prostate cancer cell growth. Clin. cancer Res. 21 (7), 1675–1687. doi:10.1158/1078-0432.CCR-14-1108
Yang, D., Liu, K., Fan, L., Liang, W., Xu, T., Jiang, W., et al. (2020). LncRNA RP11-361F15.2 promotes osteosarcoma tumorigenesis by inhibiting M2-Like polarization of tumor-associated macrophages of CPEB4. Cancer Lett. 473, 33–49. doi:10.1016/j.canlet.2019.12.041
Yang, E. Y., Kronenfeld, J. P., Gattás-Asfura, K. M., Bayer, A. L., and Stabler, C. L. (2015). Engineering an "infectious" T(reg) biomimetic through chemoselective tethering of TGF-β1 to PEG brush surfaces. Biomaterials 67, 20–31. doi:10.1016/j.biomaterials.2015.07.009
Yang, J., Shi, X., Yang, M., Luo, J., Gao, Q., Wang, X., et al. (2021). Glycolysis reprogramming in cancer-associated fibroblasts promotes the growth of oral cancer through the lncRNA H19/miR-675-5p/PFKFB3 signaling pathway. Int. J. oral Sci. 13 (1), 12. doi:10.1038/s41368-021-00115-7
Yang, S., Liu, Y., Li, M. Y., Ng, C. S. H., Yang, S. L., Wang, S., et al. (2017). FOXP3 promotes tumor growth and metastasis by activating Wnt/β-catenin signaling pathway and EMT in non-small cell lung cancer. Mol. cancer 16 (1), 124. doi:10.1186/s12943-017-0700-1
Yao, R. W., Wang, Y., and Chen, L. L. (2019). Cellular functions of long noncoding RNAs. Nat. Cell Biol. 21 (5), 542–551. doi:10.1038/s41556-019-0311-8
Yao, Y. D., Sun, T. M., Huang, S. Y., Dou, S., Lin, L., Chen, J. N., et al. (2012). Targeted delivery of PLK1-siRNA by ScFv suppresses Her2+ breast cancer growth and metastasis. Sci. Transl. Med. 4 (130), 130ra48. doi:10.1126/scitranslmed.3003601
Yoshimura, H., Matsuda, Y., Yamamoto, M., Michishita, M., Takahashi, K., Sasaki, N., et al. (2018). Reduced expression of the H19 long non-coding RNA inhibits pancreatic cancer metastasis. Laboratory investigation; a J. Tech. methods pathology 98 (6), 814–824. doi:10.1038/s41374-018-0048-1
Yu, L. X., Ling, Y., and Wang, H. Y. (2018). Role of nonresolving inflammation in hepatocellular carcinoma development and progression. NPJ Precis. Oncol. 2 (1), 6. doi:10.1038/s41698-018-0048-z
Yu, T., Zhao, Y., Hu, Z., Li, J., Chu, D., Zhang, J., et al. (2017). MetaLnc9 facilitates lung cancer metastasis via a PGK1-activated AKT/mTOR pathway. Cancer Res. 77 (21), 5782–5794. doi:10.1158/0008-5472.CAN-17-0671
Yuan, J. H., Yang, F., Wang, F., Ma, J. Z., Guo, Y. J., Tao, Q. F., et al. (2014). A long noncoding RNA activated by TGF-β promotes the invasion-metastasis cascade in hepatocellular carcinoma. Cancer Cell 25 (5), 666–681. doi:10.1016/j.ccr.2014.03.010
Yuan, S., Si, W., Zhuang, K., Li, Y., Zhang, Y., Liu, J., et al. (2021). LncRNA UCID promotes hepatocellular carcinoma metastasis via stabilization of Snail. OncoTargets Ther. 14, 725–736. doi:10.2147/OTT.S277951
Zanconato, F., Cordenonsi, M., Piccolo, S., and Yap and, T. A. Z. (2019). YAP and TAZ: A signalling hub of the tumour microenvironment. Nat. Rev. Cancer 19 (8), 454–464. doi:10.1038/s41568-019-0168-y
Zemmour, D., Pratama, A., Loughhead, S. M., Mathis, D., and Benoist, C. (2017). Flicr, a long noncoding RNA, modulates Foxp3 expression and autoimmunity. Proc. Natl. Acad. Sci. U. S. A. 114 (17), E3472–e3480. doi:10.1073/pnas.1700946114
Zhang, G., Lan, Y., Xie, A., Shi, J., Zhao, H., Xu, L., et al. (2019). Comprehensive analysis of long noncoding RNA (lncRNA)-chromatin interactions reveals lncRNA functions dependent on binding diverse regulatory elements. J. Biol. Chem. 294 (43), 15613–15622. doi:10.1074/jbc.RA119.008732
Zhang, G., Li, S., Lu, J., Ge, Y., Wang, Q., Ma, G., et al. (2018). LncRNA MT1JP functions as a ceRNA in regulating FBXW7 through competitively binding to miR-92a-3p in gastric cancer. Mol. cancer 17 (1), 87. doi:10.1186/s12943-018-0829-6
Zhang, J., Han, C., Ungerleider, N., Chen, W., Song, K., Wang, Y., et al. (2019). A transforming growth factor-β and H19 signaling Axis in tumor-initiating hepatocytes that regulates hepatic carcinogenesis. Hepatol. Baltim. Md) 69 (4), 1549–1563. doi:10.1002/hep.30153
Zhang, J., Wang, S., Jiang, B., Huang, L., Ji, Z., Li, X., et al. (2017). c-Src phosphorylation and activation of hexokinase promotes tumorigenesis and metastasis. Nat. Commun. 8, 13732. doi:10.1038/ncomms13732
Zhang, S., Xiao, J., Chai, Y., Du, Y. Y., Liu, Z., Huang, K., et al. (2017). LncRNA-CCAT1 promotes migration, invasion, and EMT in intrahepatic cholangiocarcinoma through suppressing miR-152. Dig. Dis. Sci. 62 (11), 3050–3058. doi:10.1007/s10620-017-4759-8
Zhang, T. H., Liang, L. Z., Liu, X. L., Wu, J. N., Su, K., Chen, J. Y., et al. (2019). LncRNA UCA1/miR-124 axis modulates TGFβ1-induced epithelial-mesenchymal transition and invasion of tongue cancer cells through JAG1/Notch signaling. J. Cell. Biochem. 120 (6), 10495–10504. doi:10.1002/jcb.28334
Zhao, L., Ji, G., Le, X., Wang, C., Xu, L., Feng, M., et al. (2017). Long noncoding RNA LINC00092 acts in cancer-associated fibroblasts to drive glycolysis and progression of ovarian cancer. Cancer Res. 77 (6), 1369–1382. doi:10.1158/0008-5472.CAN-16-1615
Zhao, Y., Liu, Y., Lin, L., Huang, Q., He, W., Zhang, S., et al. (2018). The lncRNA MACC1-AS1 promotes gastric cancer cell metabolic plasticity via AMPK/Lin28 mediated mRNA stability of MACC1. Mol. cancer 17 (1), 69. doi:10.1186/s12943-018-0820-2
Zhen, S., and Li, X. (2019). Application of CRISPR-cas9 for long noncoding RNA genes in cancer research. Hum. gene Ther. 30 (1), 3–9. doi:10.1089/hum.2018.063
Zhou, L., Li, J., Tang, Y., and Yang, M. (2021). Exosomal LncRNA LINC00659 transferred from cancer-associated fibroblasts promotes colorectal cancer cell progression via miR-342-3p/ANXA2 axis. J. Transl. Med. 19 (1), 8. doi:10.1186/s12967-020-02648-7
Zhou, T., Kim, Y., and MacLeod, A. R. (2016). Targeting long noncoding RNA with antisense oligonucleotide technology as cancer therapeutics. Methods Mol. Biol. Clift. NJ) 1402, 199–213. doi:10.1007/978-1-4939-3378-5_16
Zhuo, W., Liu, Y., Li, S., Guo, D., Sun, Q., Jin, J., et al. (2019). Long noncoding RNA GMAN, up-regulated in gastric cancer tissues, is associated with metastasis in patients and promotes translation of ephrin A1 by competitively binding GMAN-AS. Gastroenterology 156 (3), 676–691. doi:10.1053/j.gastro.2018.10.054
Keywords: cancer, metastasis, long non-coding RNAs, tumor microenvironment, anoikis resistance, metabolic reprogramming, immune modulation
Citation: Baba SK, Baba SK, Mir R, Elfaki I, Algehainy N, Ullah MF, Barnawi J, Altemani FH, Alanazi M, Mustafa SK, Masoodi T, Akil ASA, Bhat AA and Macha MA (2023) Long non-coding RNAs modulate tumor microenvironment to promote metastasis: novel avenue for therapeutic intervention. Front. Cell Dev. Biol. 11:1164301. doi: 10.3389/fcell.2023.1164301
Received: 12 February 2023; Accepted: 22 May 2023;
Published: 13 June 2023.
Edited by:
Samikshan Dutta, University of Nebraska Medical Center, United StatesReviewed by:
Shailendra Kumar Maurya, University of Nebraska Medical Center, United StatesCopyright © 2023 Baba, Baba, Mir, Elfaki, Algehainy, Ullah, Barnawi, Altemani, Alanazi, Mustafa, Masoodi, Akil, Bhat and Macha. This is an open-access article distributed under the terms of the Creative Commons Attribution License (CC BY). The use, distribution or reproduction in other forums is permitted, provided the original author(s) and the copyright owner(s) are credited and that the original publication in this journal is cited, in accordance with accepted academic practice. No use, distribution or reproduction is permitted which does not comply with these terms.
*Correspondence: Muzafar A. Macha, bXV6YWZhci5tYWNoYUBpdXN0LmFjLmlu, bXV6YWZhci5haWlpbXNAZ21haWwuY29t
Disclaimer: All claims expressed in this article are solely those of the authors and do not necessarily represent those of their affiliated organizations, or those of the publisher, the editors and the reviewers. Any product that may be evaluated in this article or claim that may be made by its manufacturer is not guaranteed or endorsed by the publisher.
Research integrity at Frontiers
Learn more about the work of our research integrity team to safeguard the quality of each article we publish.