- 1Shanghai Key Laboratory of Maternal Fetal Medicine, Clinical and Translational Research Center of Shanghai First Maternity and Infant Hospital, Tongji University School of Medicine, Shanghai, China
- 2Ninth People’s Hospital, Shanghai Jiao Tong University School of Medicine, Shanghai, China
- 3Shanghai Institute of Precision Medicine, Shanghai, China
- 4Ming Wai Lau Centre for Reparative Medicine, Karolinska Institutet, Sha Tin, Hong kong SAR, China
- 5School of Life Sciences, Fudan University, Shanghai, China
- 6AoBio Medical, Shanghai, China
Endoplasmic reticulum (ER) is the largest membrane-bound compartment in all cells and functions as a key regulator in protein biosynthesis, lipid metabolism, and calcium balance. Mammalian endoplasmic reticulum has evolved with an orchestrated protein quality control system to handle defective proteins and ensure endoplasmic reticulum homeostasis. Nevertheless, the accumulation and aggregation of misfolded proteins in the endoplasmic reticulum may occur during pathological conditions. The inability of endoplasmic reticulum quality control system to clear faulty proteins and aggregates from the endoplasmic reticulum results in the development of many human disorders. The efforts to comprehensively understand endoplasmic reticulum quality control network and protein aggregation will benefit the diagnostics and therapeutics of endoplasmic reticulum storage diseases. Herein, we overview recent advances in mammalian endoplasmic reticulum protein quality control system, describe protein phase transition model, and summarize the approaches to monitor protein aggregation. Moreover, we discuss the therapeutic applications of enhancing endoplasmic reticulum protein quality control pathways in endoplasmic reticulum storage diseases.
Introduction
The ER is a single membrane-enclosed organelle within eukaryotic cells and plays crucial roles in diverse cell functions such as protein synthesis, lipid trafficking, calcium homeostasis, redox regulation, and cellular metabolism (Schwarz and Blower, 2016; Harada et al., 2021). Although the ER subunits have obvious differences in shape and functions, they are interconnected with each other to enable the free movement of luminal molecules throughout the ER. For instance, the calcium traveling freely inside the ER lumen may reach up to a high concentration from 60 to 400 μM (Bygrave and Benedetti, 1996; Rossi and Taylor, 2020), which facilitates proper folding and quality control of nascent proteins in the ER via the activation of calcium binding chaperones calreticulin (CRT) and calnexin (CNX). Due to its extremely large size, the ER accounts for a great proportion of the intracellular membrane system and has associations with nearly all other organelles (e.g., mitochondria and nucleus) by the membrane contact sites (Rowland and Voeltz, 2012; Matteis and Rega, 2015; Wu et al., 2018; Lee et al., 2020; Qin et al., 2020). The close connection between ER and mitochondria is critical in the maintenance of an oxidative environment inside the ER lumen (Csordás et al., 2006; Gilady et al., 2010; Booth et al., 2016; Yoboue et al., 2018), which benefits disulfide bond formation and oxidative protein folding. As such, the association between ER and other organelles has an important role in the modulation of cytosolic positioning and functions of cellular organelles. When facing extracellular and intracellular signals, the ER is able to undergo rapid changes in morphology and composition for the maintenance of ER homeostasis and cellular functions (Baumann and Walz, 2001; Schwarz and Blower, 2016). It has been estimated that ER-membrane-resident proteins and lipids are updated once every 10 days (Omura et al., 1967). Foremost, the ER is responsible for the biogenesis of appropriate one-quarter to one-third of all proteins in eukaryotic cells (Chen et al., 2013; Kumari and Brodsky, 2021). After nascent peptides move into the ER from cytosolic ribosomes, they are recognized by ER chaperones and folding enzymes to guide their proper assembly and posttranslational modifications. Once achieving native conformation, functional proteins leave the ER and travel to the Golgi via the secretion pathway. Since nascent proteins are vulnerable to errors, a proportion of them needs to be cleared owing to their intrinsically defective conformation. This process is implemented by ER protein quality control system comprising unfolded protein response (UPR), ER-associated protein degradation (ERAD), and ER-related autophagy (ER-phagy) (Shoulders et al., 2013; Schultz et al., 2018; Christianson and Carvalho, 2022; Wiseman et al., 2022; Chino and Mizushima, 2023; Thepsuwan et al., 2023). Although these three distinct pathways primarily work in parallel, all of them cooperatively function to remove ER-resident faulty proteins, decrease ER stress, and sustain ER proteostasis (Figure 1).
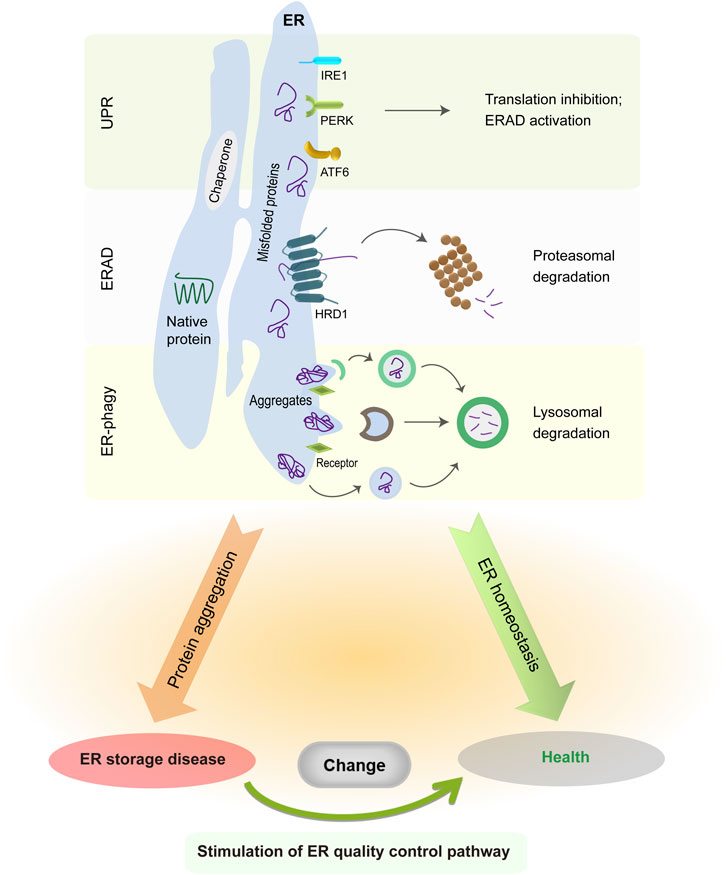
FIGURE 1. ER protein quality control system in normal and pathological conditions. The UPR is initiated by three distinct sensors, whose activation suppresses global protein translation but enhances ER folding and degradation capabilities. ER-resident faulty proteins can be transported to the cytosol via the ERAD complex and are degraded in the cytoplasmic proteasome. Meanwhile, insoluble protein aggregates are recruited to the ER-phagy receptors and then delivered into the lysosomes for degradation. The accumulation of misfolded protein aggregates in the ER causes illness, whereas enhancing ERAD or ER-phagy activity is sufficient to alleviate disease manifestations.
When ER protein quality control system is insufficient to clear misfolded proteins during pathological conditions, faulty proteins accumulate progressively and may form large protein aggregates, thereby disrupting ER homeostasis and cellular functions. Both ER stress and UPR dysfunction have been shown to play important roles in the etiology of many cancers and neurodegenerative disorders such as Alzheimer disease and Huntington disease (Imai et al., 2000; Saxena et al., 2009; Lee et al., 2010; Urra et al., 2016; Ajoolabady et al., 2022). Notably, ER stress responses are found to exert protective effects in the early disease progression with the aim to remove faulty proteins, but later exacerbate the neurodegeneration at the late stage (Hoozemans et al., 2009; Leitman et al., 2014; Mercado et al., 2016; Marcora et al., 2017). In fact, the accumulation and aggregation of faulty proteins happen within the cytoplasm of neurons in most cases of neurodegenerative diseases (Colla, 2019; Shacham et al., 2019; Bachmann et al., 2021), but leads to abnormal ER stress via the sequestration of ERAD factors, the impairment of ER-to-Golgi vesicle trafficking, the perturbation of UPR signaling cascades, and/or the disruption of ER calcium homeostasis (Yang et al., 2010; Abisambra et al., 2013; Hyrskyluoto et al., 2014; Hetz and Saxena, 2017; Remondelli and Renna, 2017; Betzer et al., 2018). Aberrant protein accumulation and aggregation in the ER have been known as the root cause of ER storage diseases (ERSDs) (Kim and Arvan, 1998; Rutishauser and Spiess, 2002; Callea and Desmet, 2021; Padilla-Godínez et al., 2021). Aberrant protein aggregates may further impede the function of normal proteins via blocking their exit from the ER. Since the majority of ERSDs arise from gene mutations at the genetic level (Ito et al., 1999; Sørensen et al., 2006; Schaeffer et al., 2017; Hilado and Randhawa, 2018; Kuscuoglu et al., 2018; Sun et al., 2020; Wang et al., 2020), there is no curative therapy to permanently correct genetic mutation and abolish mutant protein generation. The comprehensive understanding of ER quality control network and protein aggregation process has a chance to offer valuable cues for early diagnosis and medicinal development of ERSDs.
In this work, we outline ER protein quality control system, summarize protein aggregation model and detecting tools, and exemplify the therapeutic targets of ER protein quality control machinery in two different ERSDs.
ER protein quality control system
Oxidative protein folding
There exists an oxidative environment involving thiol-disulfide pairs in the ER lumen, which is crucial for disulfide formation and proper protein folding (Robinson et al., 2020; Wang and Wang, 2023). The redox state of the ER also plays an important role in the modulation of calcium balance through affecting ER calcium channels and calcium-binding proteins (Ushioda et al., 2016). The majority of membrane and secretory proteins are known to contain disulfide bonds, and their folding process involves the formation of disulfide bridges between two cysteine residues. The ER has many folding enzymes and chaperones such as protein disulfide isomerase (PDI) and ER sulfhydryl oxidase 1 (Ero1) for the fidelity and efficiency of this oxidative protein folding (Wang and Wang, 2023).
The Ero1-PDI redox cycle is pivotal in ER oxidative protein folding and highly conserved across different species. The glycoprotein Ero1 introduces the formation of de novo disulfide bridge via oxidating the PDI and transferring electrons to molecular oxygen, in which hydrogen peroxide is synthesized as a byproduct. Mammalian Ero1 exists in two different types: Ero1α has a broad expression pattern, while Ero1β is present in secretory cells (Cabibbo et al., 2000; Pagani et al., 2000; Zito et al., 2010). The Ero1 oxidase activity is dynamic and under the regulation of diversely post-translational modifications and intracellular cues. When ER environment is reductive, the regulatory disulfide bonds of Ero1 are reduced by the PDI to induce its activation. Meanwhile, the phosphorylation of Ero1 Ser145 by Fam20C is recently found to increase its oxidase activity (Zhang et al., 2018). On the contrary, the regulatory bonds of the Ero1 are oxidized again to inactivate Ero1 during oxidative stress conditions. The S-nitrosylation of Cys166 in the Ero1α is capable of lowering Ero1α activity and decreasing oxidative stress (Moilanen et al., 2018; Qiao et al., 2022). The PDI is responsible for the oxidation, reduction, and isomerization of disulfide bonds in both nascent substrates and misfolded proteins. There are 21 distinct members of the PDI oxidoreductase family in mammals (Braakman and Bulleid, 2011), each of which is thought to possess a division of labor during ER oxidative protein folding. The PDIA1 has the highest expression abundance among the PDI family and is thus the dominant player in the oxidative protein folding (Li and Sun, 2021). It should be noted that the PDIs also exhibit the chaperone activity (Wang et al., 2015; Okumura et al., 2019; Yu et al., 2020). The chaperone activity of PDIs is especially useful for the inhibition of aberrant protein accumulation and the proper maturation of secretory proteins. Additionally, the largest oxidoreductase ERDj5 functions as a central player in the reduction of non-native disulfide bridges in misfolded proteins (Ushioda et al., 2008; Oka et al., 2013). Upon the interruption of aberrant disulfide bonds, faulty protein substrates can be efficiently delivered into the ERAD for degradation.
UPR
Mammalian UPR is to sense and transmit ER stress signals to the cytosol and other organelles such as the nucleus. The UPR consists of three different signaling cascades triggered separately by protein kinase RNA-like ER kinase (PERK), activating transcription factor 6 (ATF6), and inositol-requiring protein 1α (IRE1α) (Hetz et al., 2020; Wiseman et al., 2022). All these sensors are ER membrane proteins and possess both ER-luminal and cytoplasmic domains in the meantime. Both PERK and IRE1α have kinase activity in their cytosolic region, while the cytosolic domain of ATF6 comprises a bZIP transcription factor. They are inactive in the absence of ER stresses, but rapidly exit the quiescence in the disruption of ER homeostasis. Upon stimulation of protein misfolding, the UPR sensors can be activated either by dissociating from ER chaperone BiP or by interacting directly with faulty proteins (Bertolotti et al., 2000; Zhou et al., 2006; Pincus et al., 2010; Gardner and Walter, 2011; Wiseman et al., 2022). Beyond the BiP, many molecular chaperones and proteins such as PDIs and ERp18 also participate in the activation of UPR sensors (He et al., 2012; Sepulveda et al., 2018; Oka et al., 2019; Yu et al., 2020; Wiseman et al., 2022). The three UPR pathways work together with the aim to reestablish ER proteostasis and functions.
The activation of PERK and IRE1α involves their oligomerization and autophosphorylation in situ, whereas the ATF6 activation is accompanied by its disulfide reduction and cleavage in the Golgi (Leitman et al., 2014; Preissler and Ron, 2019; Wiseman et al., 2022). The newly formed transcription factor ATF6(N) then travels into the nucleus where it induces the expression of ER quality control-related genes (Yamamoto et al., 2007; Adachi et al., 2008; Shoulders et al., 2013). Unlike the ATF6 pathway, the active PERK stimulates cytoplasmic eIF2α/eIF2B signaling cascade to decrease protein translation, but selectively increases the amounts of transcription factor ATF4 and C/EBP Homologous Protein (CHOP) (Lu et al., 2004; Han et al., 2013). Consequently, nascent peptides entering the ER have a significant reduction, whereas the genes controlling redox modulation and amino acid metabolism are unexpectedly increased. More complexly, three downstream signaling pathways are coupled to the activation of mammalian IRE1α (Wiseman et al., 2022). The active IRE1α primarily acts on the cytoplasmic XBP1 transcript and abscises 26 bases by means of its endonuclease activity (Yanagitani et al., 2011; Kanda et al., 2016). The spliced XBP1 mRNA encodes a bZIP transcription factor XBP1s to enhance the expressions of genes involved in ER protein quality control, lipid metabolism, and gluconeogenesis (Lee et al., 2003; Yamamoto et al., 2007; Shoulders et al., 2013). Meanwhile, the IRE1α could trigger the selective degradation of mRNAs and precursor miRNAs containing a consensus sequence via the regulated IRE1α-dependent decay (Hollien and Weissman, 2006; Upton et al., 2012), which reduces the protein load into the ER and boosts lysosomal flux. Thirdly, the active IRE1α may provoke the c-Jun N-terminal kinase (JNK) pathway through binding the immune sensor TRAF2 in response to severe ER stresses (Urano et al., 2000; Han et al., 2009). It is notable that both PERK and IRE1α could evoke cellular apoptotic program in the cases of acute and long-term ER stress (Wang and Kaufman, 2016; Hetz and Papa, 2018).
ERAD
When misfolded proteins fail to be resolved by the UPR, the majority of them are cleared from the ER through the ERAD pathway (Li and Sun, 2021; Christianson and Carvalho, 2022; Thepsuwan et al., 2023). The ERAD pathway is made up of molecular chaperones, ER-membrane-resident E3 ubiquitin ligases and adaptor proteins, and cytoplasmic adaptors and proteasome. The ERAD is conserved across distinct mammalian species (Christianson and Carvalho, 2022). The recognition of ERAD substrates is implemented by ER chaperones and lectins such as BiP, glucose-regulated protein 170 (GRP170), Os9, and xanthosine 50-triphosphate-B (Plemper et al., 1997; Brodsky et al., 1999; Olivari et al., 2005; Christianson et al., 2008; Hosokawa et al., 2008; Ushioda et al., 2008; Shenkman et al., 2013). Molecular chaperones discriminate faulty protein from native protein pools by recognizing the exposed hydrophobic domain of non-glycosylated clients or by catching the trimmed glycans of glycoproteins. The PDIs are involved in the recruitment and transportation of faulty proteins with disulfide bonds (Ushioda et al., 2008; Grubb et al., 2012; Oka et al., 2013; He et al., 2015).
The retrotranslocation channels in the ER membrane transport client substrates into the cytosol for proteasome degradation. A multi-protein complex comprising the suppressor/enhancer of lin-12-like (Sel1L) and hydroxymethylglutaryl reductase degradation protein 1 (Hrd1) is the best-studied translocation channel in mammalian cells (Needham et al., 2019; Lemberg and Strisovsky, 2021; Christianson and Carvalho, 2022). The Sel1L, a type-I transmembrane protein, not only transfers client substrates to the Hrd1 but also supports the stability and activity of the Hrd1 (Olzmann et al., 2013; Sun et al., 2014; Jeong et al., 2016). Hrd1 is an ER-membrane-resident E3 ubiquitin ligase having eight transmembrane segments and can self-associate into dimeric or higher oligomeric molecules (Hwang et al., 2017; Schoebel et al., 2017; Vasic et al., 2020). A multitude of adaptor proteins such as the degradation in endoplasmic reticulum (Derlin) and HERP is involved in the organization of the Hrd1 complex and the retrotranslocation of target clients (Horn et al., 2009; Neal et al., 2018; Schmidt et al., 2020; Lemberg and Strisovsky, 2021). It has been shown that yeast Hrd1 may assemble into two-halves of a channel with the Der1, the yeast ortholog of mammalian Derlin-1 (Wu et al., 2020). Derlins are ER-membrane-resident multifunctional proteins with six transmembrane spans, while their N- and C-terminal regions face the cytosol. Notably, human Derlin-1 can form a tetrameric translocation tunnel whose cavity allows the pass of specific proteins (Rao et al., 2021), supporting the complexity of retrotranslocation channels. When client substrates access the cytosolic cavity of the Hrd1, they are ubiquitinated by the cytosolic RING finger domain of the Hrd1, thus facilitating their engagement of valosin-containing protein (VCP) for the extraction. Beyond the Hrd1, more than twenty E3 ubiquitin ligases, such as March6, TRC8, and RNF170, are implicated in the ubiquitination of ERAD substrates (Mehrtash and Hochstrasser, 2019; Lemberg and Strisovsky, 2021), which cooperate with the Hrd1 or function in parallel. The ubiquitination events driven by E3 ubiquitin ligase may get assistance from cytoplasmic E2 ubiquitin-conjugating proteins Ube2j and Ube2g2 (Christianson and Ye, 2014). Once the ubiquitinated substrates have an association with the cytosolic VCP-Ufd1-Npl4 complex, they are pulled out from the ER membrane, in which the driving force arises from ATP hydrolysis of the AAA + ATPase VCP (Pye et al., 2007; Meyer et al., 2012; Christianson and Carvalho, 2022). The VCP, known as p97, is a mechanoenzyme with two ATPase domains and participates in the retrotranslocation of nearly all ERAD substrates. The recruitment of the VCP-Ufd1-Npl4 complex to the retrotranslocation sites on the ER membrane is triggered by many ERAD factors, such as Derlin1, UbxD8, and RHBDL4 (Mueller et al., 2008; Klemm et al., 2011; Sato et al., 2019; Lemberg and Strisovsky, 2021). It should be noted that the two ATPase domains of the VCP form a hexameric ATPase ring with a central pore (Cooney et al., 2019; Twomey et al., 2019). Thus, the client substrate needs to be unfolded, at least partially, all the way when passing the VCP central pore. The disulfide bonds in disulfide-containing substrates are also disrupted to make them in the linear state (Ushioda et al., 2008; Grubb et al., 2012). The last step of ERAD pathway is transferring the retrotranslocated substrates to the cytosolic 26S proteasome, which involves many cytoplasmic chaperones such as Ubl4A and BAG6 (Ahner et al., 2007; Wang et al., 2011; Xu et al., 2012). The Ubl4A, BAG6, and Trc35 form a holdase complex to maintain the solubilization of the retrotranslocated proteins in the cytosol. The interaction between this holdase complex and the proteasome facilitates the efficient and rapid delivery of client substrates to the proteasome.
Apart from the misfolded proteins, ERAD has the capacity to manage properly folded proteins and thus governs their expression abundance (Adle et al., 2009; Bhattacharya and Qi, 2019). This kind of constitutive ERAD is independent of the UPR stimulation and functions as a protein quantity control system to maintain ER and cellular homeostasis. The endogenous substrates of constitutive ERAD include but not limited to the IRE1α, CREBH, pre-BCR, CPT2, Pgc1β, Rmnd1, Nrf2, and CDKN1B (Wu et al., 2014; Fujita et al., 2015; Sun et al., 2015; Ji et al., 2016; Xu et al., 2016; Wei et al., 2018; Wei et al., 2018). Notably, ERAD deficiency has been associated with physiological dysregulation and disease occurrence (Sun et al., 2016; Bhattacharya et al., 2018; Yang et al., 2018). The specific deletion of the Sel1L in mouse liver leads to the accumulation of transcriptional factor CREBH in hepatocellular ER, the impairment of energy metabolism, and the growth retardation (Bhattacharya et al., 2018). Likewise, the accumulation and hyperactivation of IRE1α in the Sel1L-knockout mice results in the development of colitis (Sun et al., 2015; Sun et al., 2016).
ER-phagy
ER-phagy is an orchestrated system to remove ERAD-resistant misfolded proteins and insoluble protein aggregates from the ER via the lysosomal degradation pathway (Iavarone et al., 2022; Chino and Mizushima, 2023). Beyond the elimination of misfolded proteins, ER-phagy also plays important roles in the control of ER level and the degradation of the nuclear membrane (Chino and Mizushima, 2023). ER-phagy receptors mediate the selective recognition of ER subdomains by the cytoplasmic degradation machinery. Thus far, eleven ER-phagy receptors have been discovered in mammals: eight receptors (FAM134B, FAM134A, FAM134C, RTN3L, CCPG1, SEC62, TEX264, and ATL3) are resident in the ER membrane, and the other three receptors (CALCOCO1, C53, and p62) exist in soluble forms (Smith et al., 2018; Chen et al., 2019; Chino et al., 2019; Ji et al., 2019; Liang et al., 2020; Nthiga et al., 2020; Stefely et al., 2020; Stephani et al., 2020; Reggio et al., 2021; Stephani et al., 2021). Despite lacking transmembrane regions, soluble ER-phagy receptors may be recruited to ER membrane via the interaction with ER-membrane-resident proteins (Nthiga et al., 2020; Stefely et al., 2020; Stephani et al., 2020; Stephani et al., 2021). All of ER-phagy receptors possess at least an LC3-interacting region or GABARAP-interaction motif to bind the Atg8 family protein LC3 or GABARAP in autophagosomal membranes, respectively. Most ER-phagy receptors contain a long intrinsically disordered region to facilitate the curvature and scission of ER membranes (Busch et al., 2015; Snead et al., 2017). Growing evidence indicate that the ER-phagy receptors show preference in mediating the degradation of different ER regions and protein substrates (Grumati et al., 2017; Chen et al., 2019; Nthiga et al., 2020). For example, RTN3, ATL3, and CALCOCO1 mainly participate in the fragmentation of ER tubules, while FAM134B, TEX264, CCPG1, and SEC62 are involved in the degradation of ER sheets (Grumati et al., 2017; Smith et al., 2018; Chen et al., 2019; Nthiga et al., 2020). It is noteworthy that ER-phagy receptors exhibit diverse tissue expression patterns in mammals (Smith et al., 2018; Chino et al., 2019; Kohno et al., 2019; Keles et al., 2020).
There are three distinct forms of ER-phagy in mammalian cells: macro-ER-phagy, micro-ER-phagy, and the vesicular transport pathway (Molinari, 2021; Chino and Mizushima, 2023). Macro-ER-phagy harnesses the autophagosome to engulf and deliver ER fragments to the lysosomes, whereas the other two types of ER-phagy involve the direct fusion of ER fragments with the endosomes and/or lysosomes. During macro-ER-phagy, ER-phagy receptors mediate the recruitment of double-membrane autophagosomes to the ER subdomains containing misfolded protein aggregates (Rogov et al., 2014; Grumati et al., 2018). A set of ATG factors (e.g., ATG5, ATG7, and ULK1/2) participating in autophagosome biogenesis and ATG8 lipidation are necessary for this process. Once the autophagosomal cargos integrate into the lysosomes, protein aggregates, ER membrane, and the inner autophagosomal membrane are scheduled for degradation. The majority of ERAD-resistant protein aggregates such as vasopressin and Akita proinsulin mutants are disposed from the ER by this macro-ER-phagy (Cunningham et al., 2019; Forrester et al., 2019). Unlike the macro-ER-phagy, micro-ER-phagy needs the endosomal sorting complex required for transport (ESCRT) machinery rather than the autophagosome. Albeit the core autophagy factors are not involved in the micro-ER-phagy, some components of the LC3 lipidation system such as ATG4B and ATG16L1 have been observed in mammalian micro-ER-phagy during recovery from ER stresses (Schuck et al., 2014; Loi et al., 2019; Molinari, 2021). For instance, p62-dependent micro-ER-phagy is shown to handle the procollagen mutant bearing a Gly610-to-Cys (G610C) substitution in mouse cells (Omari et al., 2018). Similar to the micro-ER-phagy, the vesicular transport pathway has nothing to do with the autophagy initiation factors, but depends on the LC3-conjugation machinery (Fregno et al., 2018; Chino and Mizushima, 2020). The single-membrane vesicles detaching from the ER fuses directly with the lysosomes, which is implemented by the interaction between ER-membrane-resident syntaxin 17 and lysosomal membrane protein VAMP8 (Itakura et al., 2012; Fregno and Molinari, 2019). The Z variant of alpha1-antitrypsin (Z-AAT) with a Glu342-to-Lys (E342K) mutation is removed from the ER by this LC3-dependent transport pathway (Fregno et al., 2018; Fregno and Molinari, 2019).
Crosstalk
The UPR, ERAD, and ER-phagy are believed to work together for the high fidelity of protein synthesis and folding in the ER. When misfolded proteins appear in the ER, UPR sensors are activated with the attempt to enhance ER protein folding, translocation, secretion and degradation. To this end, the three branches of mammalian UPR have to activate ERAD and ER-phagy at certain circumstances (Hwang and Qi, 2018; Song et al., 2018; Sun and Brodsky, 2019; Molinari, 2021). The IRE1α/XBP1s pathway has been shown to boost the transcriptional expressions of core ERAD components and autophagy gene Beclin-1 (Travers et al., 2000; Margariti et al., 2013; Song et al., 2018). Moreover, the IRE1α/XBP1s signaling can stimulate the expression of ER-phagy receptor CCPG1 via regulating transcription factor MIST1 (Smith et al., 2018; Molinari, 2021). Likewise, the PERK/eIF2α/ATF4 pathway has been found to increase the expressions of ER-phagy receptors and autophagosome-related ATG5 and ATG12 genes at the transcriptional level (B'Chir et al., 2013; Adamson et al., 2016; Song et al., 2018; Zielke et al., 2020). As such, both ERAD and ER-phagy activation following UPR stimulation assist in the maintenance of proper ER functions and contents by disposing of aberrant proteins and ER subdomains. The UPR signaling is also under the control of ERAD and ER-phagy. Both IRE1α and ATF6 have been identified as the substrates of the ERAD complex (Horimoto et al., 2013; Sun et al., 2015). For example, the Sel1L-Hrd1 ERAD can regulate normal IRE1α abundance at the protein level with the help of ER luminal chaperone BiP and Os9 (Sun et al., 2015), thus limiting the hyperactivation of IRE1α/XBP1s pathway during physiological circumstance. On the contrary, the aberrant accumulation and hyperactivation of IRE1α molecule following ERAD deficiency contribute to the development of experimental colitis and inflammation dysbiosis (Sun et al., 2015). Beyond the ERAD, ER-phagy has been shown to participate in the degradation of IRE1α oligomers when ER stress occurs (Tschurtschenthaler et al., 2017).
The ERAD has ability to cope with most misfolded proteins rather than large oligomers and aggregates. The incapacity of faulty proteins to enter the ERAD pathway may be due to their large size, the absence of ERAD-responsive signal, or their aggregation state (Fregno and Molinari, 2019). On the other side, the ER-phagy is known to handle protein aggregates as well as particular ER subregions. It should be noted that some aberrant proteins such as Akita proinsulin and NPC1 I1061T mutant are the targets of both ERAD and ER-phagy (Schultz et al., 2018; Cunningham et al., 2019; Molinari, 2021). In this case, their soluble monomers are delivered into the ERAD for clearance, while their large aggregates are managed by the ER-phagy pathway. Hence, the degradation selection for misfolded proteins closely depends on protein size, mutation severity, aggregation propensity, the kinetics and thermodynamics of folding, and the site of folding lesion.
ER protein aggregation and phase transition
Although the models and mechanisms of misfolded protein aggregation within the ER remain elusive, the kinetics and research tools of protein aggregation in other cellular compartments may be applicable to the formation of ER protein aggregates. Protein aggregation in the cytoplasm is a phase transition process from soluble monomers to solid insoluble aggregates (Boeynaems et al., 2018; Dhouafli et al., 2018; Soto and Pritzkow, 2018; Wang and Roberts, 2018; Verdile et al., 2019; Zbinden et al., 2020). In the classic seeding-nucleation model, there are two sequential phases, one nucleation phase, and the other elongation stage (Dhouafli et al., 2018; Soto and Pritzkow, 2018; Wang and Roberts, 2018). During the nucleation phase, soluble protein monomers firstly assemble into non-native oligomers, which is driven by the non-covalent intermolecular interactions between hydrophobic domains or by the disulfide-mediated covalent linkage (Wang and Roberts, 2018; Franzmann and Alberti, 2019). The protein nucleation rate is closely associated with the contents of hydrophobic patches or aromatic amino acids in the monomer. The size of protein oligomers is heterogeneous from dimers to decamers, depending partially on specific protein species. Due to its reversibility and thermodynamical instability, the nucleation phase serves as the rate-limiting step in protein aggregation. Thus, the addition of oligomeric seeds is sufficient to shorten nucleation phase and accelerate protein aggregation process (Soto and Pritzkow, 2018; Wang and Roberts, 2018; Zbinden et al., 2020). In the elongation stage, oligomeric seeds can grow into large polymers quickly by recruiting misfolded and even normal proteins. Growing oligomers make use of the sticky pockets to engage other protein molecules, induce their conformational fluctuation, and then render them into the preexisting oligomeric scaffold. The growth of small protein oligomers is in an exponential manner via the monomer addition and/or oligomer-oligomer fusion (Philo and Arakawa, 2009; Wang and Roberts, 2018). As the size of protein polymers continuously increases, they could form into insoluble aggregates (Feng et al., 2019), which may involve the liquid-to-solid phase transition. The morphology of protein precipitates is manifold and determined by the degree of intermolecular arrangement (Barz and Urbanc, 2014; Mitrea and Kriwacki, 2016; Mudogo et al., 2020).
The formation of protein aggregates is concentration dependent. In theory, a high protein concentration can decrease intermolecular interaction distance and thus accelerate protein aggregation. Once the concentration of accumulated proteins reaches over the nucleation threshold, protein aggregation should take place instantaneously. Indeed, the high concentration of κ chain of human immunoglobulin G2 (IgG2) in a neutral pH solution is found to self-assemble into protein crystals in vitro (Hasegawa et al., 2011; Hasegawa et al., 2014). Overexpressing human IgG2/κ protein in CHO cells leads to the accumulation of IgG2/κ molecules and the formation of rod-shaped protein crystals in the ER (Hasegawa et al., 2011). The overexpression of human neuraminidase-1 cleaving the N-terminal sialic acids of glycoconjugates allows spontaneous formation of cuboid protein crystals in the ER of human embryonic kidney 293 cells (Hasegawa, 2019). Unlike faulty protein aggregates, these intra-ER protein crystals are made of properly folded proteins whose accumulation results from the inability of the ER to export the excess molecules. Recently, the overexpressed Z-AAT molecules are shown to form a solid protein matrix in the ER of CHO cells and retard the motility and functions of ER proteins (Chambers et al., 2022). These observations suggest that protein aggregation in the ER may be a liquid-solid phase separation event.
Detecting methods for protein aggregation and phase separation
There exist limitable approaches to characterize protein aggregates (Hamrang et al., 2013; Mitrea and Kriwacki, 2016; Wang and Roberts, 2018; Sinnige et al., 2020), most of which are used to measure protein aggregation in vitro. Since it is difficult to forecast when and where the aggregation of faulty proteins happens in multi-cellular organisms, the in vivo assessment of protein aggregation is extremely challenging. Thus, the spontaneous aggregation of purified recombinant proteins in vitro provides a chance to assess the kinetic process of protein aggregation.
Size exclusion chromatography (SEC), dynamic light scattering (DLS), analytical ultracentrifugation (AUC), and atomic force microscopy (AFM) have been employed to capture the early aggregation events of soluble protein oligomers (Mahler et al., 2009; Hamrang et al., 2013; Wang and Roberts, 2018). Each detection tool has its advantages and limitations. The SEC is sensitive and reproducible, but only detects small oligomers less than 50 nm. Besides, the necessary dilution of detection samples in the SEC application may trigger the dissociation of reversible protein aggregates. The DLS is a non-destructive technique with high sensitivity to large protein aggregates, but is unsuitable for polydisperse samples. The AUC could be taken to identify the content of protein aggregates upon its high resolution, but its wide application is limited by the low throughput and intricate data analysis. Importantly, the combinational application of SEC and DLS offers an effective strategy to monitor the size alteration of protein oligomers, while the SEC together with AUC would be very useful in characterizing the components of small protein aggregates. Regarding insoluble protein aggregates, the visual inspection can be used to identify visible protein particles via the evaluation of opalescence and turbidity, whereas light obscuration, flow imaging analysis, and microscopic technology have been harnessed to detect subvisible protein aggregates (Mahler et al., 2009; den Engelsman et al., 2011; Mitrea and Kriwacki, 2016; Wang and Roberts, 2018). Light obscuration measurement is a rapid quantitative method, but likely generates false-positive outcomes and not works for translucent particles. The flow imaging analysis is highly sensitive in analyzing the morphology of subvisible protein aggregates less than 400 microns, but its mass data volume restrains its broad application. Unlike the low resolution of optical microscopy measurement, fluorescence microscopic analysis has ability to characterize the morphology and size of protein aggregates, and its high sensitivity enables it to monitor early-stage protein aggregation. As extrinsic fluorescence dyes in fluorescence microscopic analysis may disturb protein aggregation at some extent, other detecting tools should be synergistically applied with the fluorescence microscopic analysis for the identification of protein aggregates.
Since the in vitro characterization of protein aggregation may mistakenly recapitulate protein aggregation process in vivo, the overexpression of fluorescently labeled proteins in cell line has been utilized to characterize the dynamic features of protein aggregation in living cells (Frottin et al., 2019; Hasegawa, 2019; Sinnige et al., 2020; Zbinden et al., 2020). Single-molecule fluorescence correlation spectroscopy (smFSC), single-molecule förster resonance energy transfer (smFRET), and electron paramagnetic resonance (EPR) spectroscopy are found to be effective in profiling the formation of protein aggregates in the cellular models. Notably, the inducible expression of fluorescently tagged proteins in the cellular model has been described to visualize the early events of protein aggregation (Miyazaki et al., 2016). Moreover, fluorescence recovery after photobleaching (FRAP) technique enables the observation of the kinetic addition of fluorescent protein molecules into protein aggregates in the living cells (Wang et al., 2013; Mitrea and Kriwacki, 2016). Both electron microscopy and immunohistochemistry technology have been taken to determine the relative amount of protein aggregates in vivo (Sung et al., 2015; Noda et al., 2020; Zbinden et al., 2020). Electron microscopy such as transmission electron microscopy and 4-dimensional ultrafast electron microscopy utilizes the greyscale contrast of particles to determine protein aggregates, whereas the immunohistochemistry analysis needs specific protein antibodies to measure the aggregated proteins. Given the limitation of a single detection tool, one detection technology is unable to cover all features of protein aggregation. Thus, the combination of multiple detecting tools should be adopted to gain insight into the protein aggregation.
Therapeutic applications in the ER storage diseases
Albeit the ER devotes numerous efforts to safeguard protein fidelity, protein misfolding and accumulation may appear in the ER owing to gene mutations, oxidative stress, or nutrient deprivation. The aggregation of misfolded proteins and the dysfunction of the ER play crucial roles in the development of ERSDs. Thus far, more than 11 types of ERSDs have been identified in humans (Kim et al., 1996; Ito et al., 1999; Brennan et al., 2002; Marini et al., 2007; Schaeffer et al., 2017; Hilado and Randhawa, 2018; Wang et al., 2020; Li and Sun, 2021). The retention of defective protein aggregates in the ER is a typical characteristic of these ERSDs. Notably, the enhancement of ERAD or ER-phagy activity has been reported to boost the clearance of mutant protein aggregates from the ER in preclinical animal models of human ERSDs (Hidvegi et al., 2010; Kaushal et al., 2010; Pastore et al., 2013; Xu et al., 2018; Miyata et al., 2020). This selective degradation of pathological protein aggregates suggests the therapeutic potential of increasing ER protein clearance activity in the ERSDs. Since some ERSDs have been discussed elsewhere (Kuscuoglu et al., 2018; Callea et al., 2021; Li and Sun, 2021), here we pay more attention on two representative forms of ERADs.
Proopiomelanocortin (POMC) mutation-driven early-onset obesity
POMC mutation-driven obesity is a recessive genetic disease characterized by hyperphagia and excessive body weight (Creemers et al., 2008; Hilado and Randhawa, 2018). The POMC is a multivalent polypeptide synthesized in the ER sheets. Once entering the secretory granules, native POMC is split into around 10 bioactive peptides such as α-melanocyte-stimulating hormone (α-MSH) and β-endorphin under the action of prohormone convertases (Millington, 2006; Harno et al., 2018). POMC derivatives are essential for numerous physiological processes including but not limited to the appetite, melanocyte stimulation, immune modulation, and skin pigmentation. For example, α-MSH is the major POMC derivative in hypothalamic neurons and acts as a key player in regulating food intake and energy balance (Kim et al., 2018; Fujima et al., 2020). Upon the importance of POMC derivatives, the deficiency of functional POMC and derivatives leads to hyperphagia, obesity, hypopigmentation, and adrenal insufficiency (Creemers et al., 2008; Hilado and Randhawa, 2018).
The folding of POMC molecules is error prone due to the presence of multiple disulfide bonds, but faulty POMC in the ER can be timely removed by the ERAD pathway during normal conditions. On the contrary, pathogenic POMC-C28F mutant harboring a cysteine-to-phenylalanine mutation at position 28 escapes ERAD-mediated destruction and eventually forms large aggregates inside the ER (Creemers et al., 2008; Kim et al., 2018). The POMC-C28F mutant is unstable because of its phenylalanine side chain, so the intermolecular disulfide linkage mediates its spontaneous aggregation (Kim et al., 2018). Supporting this notion, the creation of an intragenic cysteine-to-serine or cysteine-to-alanine mutation at position 50 is found to abolish the aggregation of POMC-C28F mutant (Kim et al., 2018; Bhattacharya and Qi, 2019). As the C28F mutation resides in the N-terminal domain guiding POMC trafficking, POMC-C28F mutant can't be transported into the secretory granules and is stuck in the ER (Creemers et al., 2008). The incapacity of POMC-C28F mutant to generate functional derivates eventually results in the occurrence of hyperphagia and severe obesity (Creemers et al., 2008; Kim et al., 2018).
The disease progression of POMC mutant-driven obesity can be recapitulated in the mice with either ERAD or autophagy deficiency (He et al., 2015; Kim et al., 2018). When the Sel1L is specifically deleted in the POMC neurons, nearly all POMC molecules are detained in the ER of neural cells, leading to the shortage of POMC derivatives in Sel1L-knockout mice (Kim et al., 2018). The mice with Sel1L deletion become hyperphagic and ultimately develop age-associated obesity, hyperleptinemia, and insulin resistance (Kim et al., 2018). Likewise, the deletion of autophagy-related ATG5 or ATG7 in hypothalamic POMC neurons is found to trigger abnormal glycolipid metabolism and severe obesity in the ATG5-or ATG7-deficient mice (Coupe et al., 2012; Kaushik et al., 2012; Malhotra et al., 2015), suggesting the involvement of ER-phagy in the degradation of misfolded POMC. Recently, the macro-ER-phagy driven by RTN3 has been reported to battle with POMC-C28F mutant aggregates (He et al., 2015). At present, there is no curative option for POMC-mutant-driven obesity. The application of melanocortin-4 receptor agonist setmelanotide has been manifested to reduce food uptake and induce substantial weight loss in patients with POMC deficiency (Table 1) (Kühnen et al., 2016; Clément et al., 2020). However, the setmelanotide treatment is offered only for the mitigation of secondary symptoms of genetic obesity without removing the POMC gene mutation. Given the pivotal roles of ER quality control system in aberrant protein clearance, it is worth exploring whether the enhancement of ERAD or ER-phagy activity may efficiently treat genetic obesity caused by POMC mutations.
Alpha1-antitrypsin deficiency (AATD)
The congenital AATD is caused by genetic mutations in the SERPINA1 gene encoding the alpha1-antitrypsin (AAT) (Sveger, 1976; Karatas and Bouchecareilh, 2020; Strnad et al., 2020). The AAT is a secretory glycoprotein whose folding and modification occur in the ER of hepatic cells. Once maturation, the AAT leaves the CNX/CRT cycle and is secreted into extracellular space via the secretory pathway. AAT is the most abundant serine protease inhibitor in the bloodstream and functions to protect distant tissues from protease-mediated destruction (Esra et al., 2019; Karatas and Bouchecareilh, 2020). Nevertheless, pathological AAT mutants are unable to be recognized by the ER-Golgi intermediate compartment that delivers native AAT from ER to Golgi (Callea et al., 1992; Leon and Bouchecareilh, 2021), thereby causing their retention in the hepatocyte ER. Due to the insufficiency of functional AAT, AATD patients suffer from pulmonary emphysema and liver cirrhosis.
Among over 100 AAT mutations, the Z-AAT is the most severe mutant affecting ∼1 in 1,500 newborns worldwide (Karatas and Bouchecareilh, 2020; Leon and Bouchecareilh, 2021). The folding of Z-AAT mutant is slow even with the assistance of many ER chaperones (e.g., BiP, GRP170, and PDIs), so Z-AAT molecules yield fault conformation and are stuck in the ER. Although both ERAD and ER-phagy can deal with Z-AAT mutants (Qu et al., 1996; Teckman and Perlmutter, 2000; Teckman et al., 2001; Kruse et al., 2006; Fregno et al., 2018), the continuous generation of Z-AAT eventually overwhelms the clearance ability of ER quality control machinery. As a consequence, the Z-AAT mutant accumulates in the ER lumen and self-assembles into large aggregates by the classic loop-sheet model or by the C-terminal domain swap mechanism (Shin and Yu, 2002; Yamasaki et al., 2011; Karatas and Bouchecareilh, 2020). It should be noted that inclusion body is found in the hepatocytes of AATD individuals (Granell and Baldini, 2008; Granell et al., 2008). Inclusion bodies are a small portion of ER sheets and are filled up with insoluble Z-AAT aggregates. The inability of the inclusion body to sequestrate Z-AAT aggregates has been shown to impair normal protein trafficking in the ER and evoke cell shrinkage (Granell et al., 2008), suggesting the protective roles of inclusion body formation in ER functions. Moreover, the durative accumulation of Z-AAT aggregates triggers oxidative stress, impairs calcium balance, and provokes inflammatory reactions in the liver (Lomas et al., 1992; Miranda et al., 2010; Bouchecareilh, 2020), thereby contributing to hepatic dysfunctions and other organ damages.
Although functional AAT administration can be used to treat AATD patients with emphysema (Wewers et al., 1987; Sandhaus, 2004), this augmentation treatment is shown to not improve lung function and reduce exacerbation events in AATD patients with obstructive pulmonary disease (Chapman et al., 2015; Stolk et al., 2019). Hence, it is necessary to develop novel therapeutic approaches for the treatment of AATD patients. The augment of ERAD or ER-phagy activity may represent a promising therapeutic strategy for AATD (Table 1). Consistent with the clearance of Z-AAT aggregates by FAM134B-driven ER-phagy (Fregno et al., 2018), the specific overexpression of autophagy regulator TFEB in mouse liver is found to suppress Z-AAT aggregation and alleviate hepatic damages in the Z-AAT transgenic mice (Pastore et al., 2013). The systemic application of autophagy inducer rapamycin, carbamazepine, or norursodeoxycholic acid in the Z-AAT transgenic mice has been described to ameliorate liver fibrosis and inflammation via enhancing autophagic flux and reducing Z-AAT aggregates (Hidvegi et al., 2010; Kaushal et al., 2010; Tang et al., 2016; Wang et al., 2019). Additionally, the enhancement of ERAD activity by overexpressing the Hrd1 could increase Z-AAT degradation and decrease intracellular A-AAT accumulation in the cellular model (Wang et al., 2011).
Perspectives
Despite recent advances, there are many open questions to be addressed in the future. The identification of mutant protein aggregates and inclusions in the ER is qualitative without the description of dynamic protein aggregation. Thus, more studies are needed to explore whether the aggregation of misfolded proteins in the ER follows the seeding-nucleation model. The cellular models have been taken to investigate ER protein aggregation by stably overexpressing protein in living cells (Hasegawa et al., 2011; Hasegawa et al., 2014; Hasegawa, 2019), but they aren’t the ideal systems to mirror the in vivo process of protein aggregation. The stable overexpression of specific protein in cell lines may facilitate protein aggregation behavior via inducing protein overcrowding and cellular stress, which contributes to the artificial peculiarity of cellular models. In this scenario, it will be reasonable to employ primary cell culture or zebrafish model to investigate the dynamic features of ER protein aggregation in vivo. Moreover, as liquid-liquid phase separation conduces to the formation of membraneless compartments in eukaryotic cells (Feng et al., 2019; Noda et al., 2020; Zbinden et al., 2020), it is interesting to assess whether the liquid-liquid phase separation participates in the aggregation of ER-resident proteins. Another valuable direction is to measure the contribution level of each ER quality control pathway to the degradation of misfolded and aggregated proteins in vivo, especially in animal disease models. Since the UPR, ERAD, and ER-phagy exist in all mammalian cells, the feasible path to address the above issue is utilizing the animal model with deletion of at least one ER quality control pathway. The aging has been associated with ER stress and UPR impairment (Chadwick and Lajoie, 2019; Bhattarai et al., 2020; Taylor and Hetz, 2020). Chronic ER stress and the impaired UPR activation might be important inducers in the death of aged cells during aging. The upregulation of UPR activity by overexpressing XBP1s is reported to extend the life span of C. elegans (Taylor and Dillin, 2013), while the XBP1s overexpression in aged mouse brain significantly improves age-related behaviors and brain dysfunctions in murine (Cabral-Miranda et al., 2020). As the linkage between aging and ERAD or ER-phage remains elusive, it is necessary to investigate how the aging changes ER quality control pathways and how the manipulation of ERAD or ER-phagy regulates the aging process. It should be noted here that the improper activation of UPR pathways conduces to the progression of some human diseases including the diabetes, viral infection, and hepatic steatosis (Chen et al., 2008; Kammoun et al., 2009; Allagnat et al., 2012; Han et al., 2015; Li et al., 2019; Bhattarai et al., 2021). For example, the hyperactivation of IRE1α and ATF6 signaling pathways has been found to induce pancreatic cell death and exacerbate insulin resistance in the animal models of diabetes (Chen et al., 2008; Allagnat et al., 2012; Han et al., 2015). In these circumstances, it is highly reasonable to suppress the activity of UPR signaling for the efficient alleviation of disease symptoms. However, more researches are needed to explore whether inhibiting the UPR is beneficial to health in animal disease models or clinical settings. With the advent of novel detection techniques, this exploration during pathological conditions holds great promise for the development of medicinal targets in ER protein quality control system.
Author contributions
GC performed literature search, wrote the manuscript, and revised the manuscript; TW performed literature search and wrote the manuscript; FJ reviewed and revised the manuscript; HL initiated the study, devised conceptual ideas, revised the manuscript, and supervised the project.
Funding
This work was funded by the Natural Science Foundation of Shanghai (22ZR1449100) and the National Natural Science Foundation of China (82273475).
Conflict of interest
HL was employed by AoBio Medical Co., Ltd.
The remaining authors declare that the research was conducted in the absence of any commercial or financial relationships that could be construed as a potential conflict of interest.
Publisher’s note
All claims expressed in this article are solely those of the authors and do not necessarily represent those of their affiliated organizations, or those of the publisher, the editors and the reviewers. Any product that may be evaluated in this article, or claim that may be made by its manufacturer, is not guaranteed or endorsed by the publisher.
References
Abisambra, J. F., Jinwal, U. K., Blair, L. J., O'Leary, J. C., Li, Q., Brady, S., et al. (2013). Tau accumulation activates the unfolded protein response by impairing endoplasmic reticulum-associated degradation. J. Neurosci. 33, 9498–9507. doi:10.1523/JNEUROSCI.5397-12.2013
Adachi, Y., Yamamoto, K., Okada, T., Yoshida, H., Harada, A., and Mori, K. (2008). ATF6 is a transcription factor specializing in the regulation of quality control proteins in the endoplasmic reticulum. Cell Struct. Funct. 33, 75–89. doi:10.1247/csf.07044
Adamson, B., Norman, T. M., Jost, M., Cho, M. Y., Nuñez, J. K., Chen, Y., et al. (2016). A multiplexed single-cell CRISPR screening platform enables systematic dissection of the unfolded protein response. Cell 167, 1867–1882. doi:10.1016/j.cell.2016.11.048
Adle, D. J., Wei, W., Smith, N., Bies, J. J., and Lee, J. (2009). Cadmium-mediated rescue from ER-associated degradation induces expression of its exporter. Proc. Natl. Acad. Sci. U. S. A. 106, 10189–10194. doi:10.1073/pnas.0812114106
Ahner, A., Nakatsukasa, K., Zhang, H., Frizzell, R. A., and Brodsky, J. L. (2007). Small heat-shock proteins select deltaF508-CFTR for endoplasmic reticulum-associated degradation. Mol. Biol. Cell 18, 806–814. doi:10.1091/mbc.e06-05-0458
Ajoolabady, A., Lindholm, D., Ren, J., and Pratico, D. (2022). ER stress and UPR in alzheimer's disease: Mechanisms, pathogenesis, treatments. Cell Death Dis. 13, 706. doi:10.1038/s41419-022-05153-5
Allagnat, F., Fukaya, M., Nogueira, T. C., Delaroche, D., Welsh, N., Marselli, L., et al. (2012). C/EBP homologous protein contributes to cytokine-induced pro-inflammatory responses and apoptosis in β-cells. Cell Death Differ. 19, 1836–1846. doi:10.1038/cdd.2012.67
B'Chir, W., Maurin, A. C., Carraro, V., Averous, J., Jousse, C., Muranishi, Y., et al. (2013). The eIF2α/ATF4 pathway is essential for stress-induced autophagy gene expression. Nucleic Acids Res. 41, 7683–7699. doi:10.1093/nar/gkt563
Bachmann, S., Bell, M., Klimek, J., and Zempel, H. (2021). Differential effects of the six human TAU isoforms: Somatic retention of 2N-tau and increased microtubule number induced by 4R-TAU. Front. Neurosci. 15, 643115. doi:10.3389/fnins.2021.643115
Barz, B., and Urbanc, B. (2014). Minimal model of self-assembly: Emergence of diversity and complexity. J. Phys. Chem. B 118, 3761–3770. doi:10.1021/jp412819j
Baumann, O., and Walz, B. (2001). Endoplasmic reticulum of animal cells and its organization into structural and functional domains. Int. Rev. Cytol. 205, 149–214. doi:10.1016/s0074-7696(01)05004-5
Bertolotti, A., Zhang, Y., Hendershot, L. M., Harding, H. P., and Ron, D. (2000). Dynamic interaction of BiP and ER stress transducers in the unfolded-protein response. Nat. Cell Biol. 2, 326–332. doi:10.1038/35014014
Betzer, C., Lassen, L. B., Olsen, A., Kofoed, R. H., Reimer, L., Gregersen, E., et al. (2018). Alpha-synuclein aggregates activate calcium pump SERCA leading to calcium dysregulation. EMBO Rep. 19, e44617. doi:10.15252/embr.201744617
Bhattacharya, A., and Qi, L. (2019). ER-associated degradation in health and disease - from substrate to organism. J. Cell Sci. 132, jcs232850. doi:10.1242/jcs.232850
Bhattacharya, A., Sun, S., Wang, H., Liu, M., Long, Q., Yin, L., et al. (2018). Hepatic Sel1L-Hrd1 ER-associated degradation (ERAD) manages FGF21 levels and systemic metabolism via CREBH. EMBO J. 37, e99277. doi:10.15252/embj.201899277
Bhattarai, K. R., Chaudhary, M., Kim, H. R., and Chae, H. J. (2020). Endoplasmic reticulum (ER) stress response failure in diseases. Trends Cell Biol. 30, 672–675. doi:10.1016/j.tcb.2020.05.004
Bhattarai, K. R., Riaz, T. A., Kim, H. R., and Chae, H. J. (2021). The aftermath of the interplay between the endoplasmic reticulum stress response and redox signaling. Exp. Mol. Med. 53, 151–167. doi:10.1038/s12276-021-00560-8
Boeynaems, S., Alberti, S., Fawzi, N. L., Mittag, T., Polymenidou, M., Rousseau, F., et al. (2018). Protein phase separation: A new phase in cell biology. Trends Cell Biol. 28, 420–435. doi:10.1016/j.tcb.2018.02.004
Booth, D. M., Enyedi, B., Geiszt, M., Várnai, P., and Hajnóczky, G. (2016). Redox nanodomains are induced by and control calcium signaling at the ER-mitochondrial interface. Cell 63, 240–248. doi:10.1016/j.molcel.2016.05.040
Bouchecareilh, M. (2020). Alpha-1 antitrypsin deficiency-mediated liver toxicity: Why do some patients do poorly? What do we know so far? Chronic. Obstr. Pulm. Dis. 7, 172–181. doi:10.15326/jcopdf.7.3.2019.0148
Braakman, I., and Bulleid, N. J. (2011). Protein folding and modification in the mammalian endoplasmic reticulum. Annu. Rev. Biochem. 80, 71–99. doi:10.1146/annurev-biochem-062209-093836
Brennan, S. O., Maghzal, G., Shneider, B. L., Gordon, R., Magid, M. S., and George, P. M. (2002). Novel fibrinogen gamma375 Arg-->Trp mutation (fibrinogen aguadilla) causes hepatic endoplasmic reticulum storage and hypofibrinogenemia. Hepatology 36, 652–658. doi:10.1053/jhep.2002.35063
Brodsky, J. L., Werner, E. D., Dubas, M. E., Goeckeler, J. L., Kruse, K. B., and McCracken, A. A. (1999). The requirement for molecular chaperones during endoplasmic reticulum-associated protein degradation demonstrates that protein export and import are mechanistically distinct. J. Biol. Chem. 274, 3453–3460. doi:10.1074/jbc.274.6.3453
Busch, D. J., Houser, J. R., Hayden, C. C., Sherman, M. B., Lafer, E. M., and Stachowiak, J. C. (2015). Intrinsically disordered proteins drive membrane curvature. Nat. Commun. 6, 7875. doi:10.1038/ncomms8875
Bygrave, F. L., and Benedetti, A. (1996). What is the concentration of calcium ions in the endoplasmic reticulum? Cell Calcium 19, 547–551. doi:10.1016/s0143-4160(96)90064-0
Cabibbo, A., Pagani, M., Fabbri, M., Rocchi, M., Farmery, M. R., Bulleid, N. J., et al. (2000). ERO1-L, a human protein that favors disulfide bond formation in the endoplasmic reticulum. J. Biol. Chem. 275, 4827–4833. doi:10.1074/jbc.275.7.4827
Cabral-Miranda, F., Tamburini, G., Martinez, G., Medinas, D., Gerakis, Y., Miedema, T., et al. (2020). Control of mammalian brain aging by the unfolded protein response (UPR). bioRxiv.
Callea, F., Brisigotti, M., Fabbretti, G., Bonino, F., and Desmet, V. J. (1992). Hepatic endoplasmic reticulum storage diseases. Liver 12, 357–362. doi:10.1111/j.1600-0676.1992.tb00589.x
Callea, F., and Desmet, V. (2021). The discovery of endoplasmic reticulum storage disease. The connection between an H&E slide and the brain. Int. J. Mol. Sci. 22, 2899. doi:10.3390/ijms22062899
Callea, F., Francalanci, P., and Giovannoni, I. (2021). Hepatic and extrahepatic sources and manifestations in endoplasmic reticulum storage diseases. Int. J. Mol. Sci. 22, 5778. doi:10.3390/ijms22115778
Chadwick, S. R., and Lajoie, P. (2019). Endoplasmic reticulum stress coping mechanisms and lifespan regulation in health and diseases. Front. Cell Dev. Biol. 7, 84. doi:10.3389/fcell.2019.00084
Chambers, J. E., Zubkov, N., Kubánková, M., Nixon-Abell, J., Mela, I., Abreu, S., et al. (2022). Z-α(1)-antitrypsin polymers impose molecular filtration in the endoplasmic reticulum after undergoing phase transition to a solid state. Sci. Adv. 8, eabm2094. doi:10.1126/sciadv.abm2094
Chapman, K. R., Burdon, J. G., Piitulainen, E., Sandhaus, R. A., Seersholm, N., Stocks, J. M., et al. (2015). Intravenous augmentation treatment and lung density in severe α1 antitrypsin deficiency (RAPID): A randomised, double-blind, placebo-controlled trial. Lancet 386, 360–368. doi:10.1016/S0140-6736(15)60860-1
Chen, J., Hui, S. T., Couto, F. M., Mungrue, I. N., Davis, D. B., Attie, A. D., et al. (2008). Thioredoxin-interacting protein deficiency induces Akt/Bcl-xL signaling and pancreatic beta-cell mass and protects against diabetes. FASEB J. 22, 3581–3594. doi:10.1096/fj.08-111690
Chen, Q., Xiao, Y., Chai, P., Zheng, P., Teng, J., and Chen, J. (2019). ATL3 is a tubular ER-phagy receptor for GABARAP-mediated selective autophagy. Curr. Biol. 29, 846–855. doi:10.1016/j.cub.2019.01.041
Chen, S., Novick, P., and Ferro-Novick, S. (2013). ER structure and function. Curr. Opin. Cell Bio.l 25, 428–433. doi:10.1016/j.ceb.2013.02.006
Chino, H., Hatta, T., Natsume, T., and Mizushima, N. (2019). Intrinsically disordered protein TEX264 mediates ER-phagy. Cell 74, 909–921. doi:10.1016/j.molcel.2019.03.033
Chino, H., and Mizushima, N. (2023). ER-Phagy: Quality and quantity control of the endoplasmic reticulum by autophagy. Cold spring harb perspect. Biol 15, a041256. doi:10.1101/cshperspect.a041256
Chino, H., and Mizushima, N. (2020). ER-Phagy: Quality control and turnover of endoplasmic reticulum. Trends Cell Biol. 30, 384–398. doi:10.1016/j.tcb.2020.02.001
Christianson, J. C., and Carvalho, P. (2022). Order through destruction: How ER-associated protein degradation contributes to organelle homeostasis. EMBO J. 41, e109845. doi:10.15252/embj.2021109845
Christianson, J. C., Shaler, T. A., Tyler, R. E., and Kopito, R. R. (2008). OS-9 and GRP94 deliver mutant alpha1-antitrypsin to the Hrd1-SEL1L ubiquitin ligase complex for ERAD. Nat. Cell Biol. 10, 272–282. doi:10.1038/ncb1689
Christianson, J. C., and Ye, Y. (2014). Cleaning up in the endoplasmic reticulum: Ubiquitin in charge. Nat. Struct. Mol. Biol. 21, 325–335. doi:10.1038/nsmb.2793
Clément, K., van den Akker, E., Argente, J., Bahm, A., Chung, W. K., Connors, H., et al. (2020). Efficacy and safety of setmelanotide, an MC4R agonist, in individuals with severe obesity due to LEPR or POMC deficiency: Single-arm, open-label, multicentre, phase 3 trials. Lancet Diabetes Endocrinol. 8, 960–970. doi:10.1016/S2213-8587(20)30364-8
Colla, E. (2019). Linking the endoplasmic reticulum to Parkinson's disease and alpha-synucleinopathy. Front. Neurosci. 13, 560. doi:10.3389/fnins.2019.00560
Cooney, I., Han, H., Stewart, M. G., Carson, R. H., Hansen, D. T., Iwasa, J. H., et al. (2019). Structure of the Cdc48 segregase in the act of unfolding an authentic substrate. Science 365, 502–505. doi:10.1126/science.aax0486
Coupe, B., Ishii, Y., Dietrich, M. O., Komatsu, M., Horvath, T. L., and Bouret, S. G. (2012). Loss of autophagy in pro-opiomelanocortin neurons perturbs axon growth and causes metabolic dysregulation. Cell Metab. 15, 247–255. doi:10.1016/j.cmet.2011.12.016
Creemers, J. W., Lee, Y. S., Oliver, R. L., Bahceci, M., Tuzcu, A., Gokalp, D., et al. (2008). Mutations in the amino-terminal region of proopiomelanocortin (POMC) in patients with early-onset obesity impair POMC sorting to the regulated secretory pathway. J. Clin. Endocrinol. Metab. 93, 4494–4499. doi:10.1210/jc.2008-0954
Csordás, G., Renken, C., Várnai, P., Walter, L., Weaver, D., Buttle, K. F., et al. (2006). Structural and functional features and significance of the physical linkage between ER and mitochondria. J. Cell Biol. 174, 915–921. doi:10.1083/jcb.200604016
Cunningham, C. N., Williams, J. M., Knupp, J., Arunagiri, A., Arvan, P., and Tsai, B. (2019). Cells deploy a two-pronged strategy to rectify misfolded proinsulin aggregates. Cell 75, 442–456. doi:10.1016/j.molcel.2019.05.011
den Engelsman, J., Garidel, P., Smulders, R., Koll, H., Smith, B., Bassarab, S., et al. (2011). Strategies for the assessment of protein aggregates in pharmaceutical biotech product development. Pharm. Res. 28, 920–933. doi:10.1007/s11095-010-0297-1
Dhouafli, Z., Cuanalo-Contreras, K., Hayouni, E. A., Mays, C. E., Soto, C., and Moreno-Gonzalez, I. (2018). Inhibition of protein misfolding and aggregation by natural phenolic compounds. Cell. Mol. Life Sci. 75, 3521–3538. doi:10.1007/s00018-018-2872-2
Esra, K. S., Nathalie, D.-S., Alain, L., and Marion, B. (2019). Overview of alpha-1 antitrypsin deficiency-mediated liver disease. EMJ Hepatol. 7, 65–79.
Feng, Z., Chen, X., Wu, X., and Zhang, M. (2019). Formation of biological condensates via phase separation: Characteristics, analytical methods, and physiological implications. J. Biol. Chem. 294, 14823–14835. doi:10.1074/jbc.REV119.007895
Forrester, A., De Leonibus, C., Grumati, P., Fasana, E., Piemontese, M., Staiano, L., et al. (2019). A selective ER-phagy exerts procollagen quality control via a Calnexin-FAM134B complex. EMBO J. 38, e99847. doi:10.15252/embj.201899847
Franzmann, T. M., and Alberti, S. (2019). Protein phase separation as a stress survival strategy. Cold Spring Harb. Perspect. Biol. 11, a034058. doi:10.1101/cshperspect.a034058a034058
Fregno, I., Fasana, E., Bergmann, T. J., Raimondi, A., Loi, M., Soldà, T., et al. (2018). ER-to-lysosome-associated degradation of proteasome-resistant ATZ polymers occurs via receptor-mediated vesicular transport. EMBO J. 37, e99259. doi:10.15252/embj.201899259
Fregno, I., and Molinari, M. (2019). Proteasomal and lysosomal clearance of faulty secretory proteins: ER-associated degradation (ERAD) and ER-to-lysosome-associated degradation (ERLAD) pathways. Crit. Rev. Biochem. Mol. Biol. 54, 153–163. doi:10.1080/10409238.2019.1610351
Frottin, F., Schueder, F., Tiwary, S., Gupta, R., Körner, R., Schlichthaerle, T., et al. (2019). The nucleolus functions as a phase-separated protein quality control compartment. Science 365, 342–347. doi:10.1126/science.aaw9157
Fujima, S., Amemiya, N., Arima, T., Sano, Y., and Furuichi, T. (2020). CAPS2 deficiency induces proopiomelanocortin accumulation in pituitary and affects food intake behavior in mice. Neurosci. Lett. 738, 135335. doi:10.1016/j.neulet.2020.135335
Fujita, H., Yagishita, N., Aratani, S., Saito-Fujita, T., Morota, S., Yamano, Y., et al. (2015). The E3 ligase synoviolin controls body weight and mitochondrial biogenesis through negative regulation of PGC-1β. EMBO J. 34, 1042–1055. doi:10.15252/embj.201489897
Gardner, B. M., and Walter, P. (2011). Unfolded proteins are Ire1-activating ligands that directly induce the unfolded protein response. Science 333, 1891–1894. doi:10.1126/science.1209126
Gilady, S. Y., Bui, M., Lynes, E. M., Benson, M. D., Watts, R., Vance, J. E., et al. (2010). Ero1alpha requires oxidizing and normoxic conditions to localize to the mitochondria-associated membrane (MAM). Cell Stress Chaperones 15, 619–629. doi:10.1007/s12192-010-0174-1
Granell, S., and Baldini, G. (2008). Inclusion bodies and autophagosomes: Are ER-derived protective organelles different than classical autophagosomes? Autophagy 4, 375–377. doi:10.4161/auto.5605
Granell, S., Baldini, G., Mohammad, S., Nicolin, V., Narducci, P., Storrie, B., et al. (2008). Sequestration of mutated alpha1-antitrypsin into inclusion bodies is a cell-protective mechanism to maintain endoplasmic reticulum function. Mol. Biol. Cell 19, 572–586. doi:10.1091/mbc.e07-06-0587
Grubb, S., Guo, L., Fisher, E. A., and Brodsky, J. L. (2012). Protein disulfide isomerases contribute differentially to the endoplasmic reticulum-associated degradation of apolipoprotein B and other substrates. Mol. Biol. Cell 23, 520–532. doi:10.1091/mbc.E11-08-0704
Grumati, P., Dikic, I., and Stolz, A. (2018). ER-phagy at a glance. J. Cell Sci. 131, jcs217364. doi:10.1242/jcs.217364
Grumati, P., Morozzi, G., Hölper, S., Mari, M., Harwardt, M. I., Yan, R., et al. (2017). Full length RTN3 regulates turnover of tubular endoplasmic reticulum via selective autophagy. Elife 6, e25555. doi:10.7554/eLife.25555
Hamrang, Z., Rattray, N. J., and Pluen, A. (2013). Proteins behaving badly: Emerging technologies in profiling biopharmaceutical aggregation. Trends Biotechnol. 31, 448–458. doi:10.1016/j.tibtech.2013.05.004
Han, D., Lerner, A. G., Vande Walle, L., Upton, J. P., Xu, W., Hagen, A., et al. (2009). IRE1alpha kinase activation modes control alternate endoribonuclease outputs to determine divergent cell fates. Cell 138, 562–575. doi:10.1016/j.cell.2009.07.017
Han, J., Back, S. H., Hur, J., Lin, Y. H., Gildersleeve, R., Shan, J., et al. (2013). ER-stress-induced transcriptional regulation increases protein synthesis leading to cell death. Nat. Cell Biol. 15, 481–490. doi:10.1038/ncb2738
Han, J., Li, E., Chen, L., Zhang, Y., Wei, F., Liu, J., et al. (2015). The CREB coactivator CRTC2 controls hepatic lipid metabolism by regulating SREBP1. Nature 524, 243–246. doi:10.1038/nature14557
Harada, Y., Ohkawa, Y., Maeda, K., and Taniguchi, N. (2021). Glycan quality control in and out of the endoplasmic reticulum of mammalian cells. FEBS J. 289, 7147–7162. doi:10.1111/febs.16185
Harno, E., Gali Ramamoorthy, T., Coll, A. P., and White, A. (2018). Pomc: The physiological power of hormone processing. Physiol. Rev. 98, 2381–2430. doi:10.1152/physrev.00024.2017
Hasegawa, H. (2019). Simultaneous induction of distinct protein phase separation events in multiple subcellular compartments of a single cell. Exp. Cell Res. 379, 92–109. doi:10.1016/j.yexcr.2019.03.010
Hasegawa, H., Wendling, J., He, F., Trilisky, E., Stevenson, R., Franey, H., et al. (2011). In vivo crystallization of human IgG in the endoplasmic reticulum of engineered Chinese hamster ovary (CHO) cells. J. Biol. Chem. 286, 19917–19931. doi:10.1074/jbc.M110.204362
Hasegawa, H., Woods, C. E., Kinderman, F., He, F., and Lim, A. C. (2014). Russell body phenotype is preferentially induced by IgG mAb clones with high intrinsic condensation propensity: Relations between the biosynthetic events in the ER and solution behaviors in vitro. MAbs 6, 1518–1532. doi:10.4161/mabs.36242
He, K., Cunningham, C. N., Manickam, N., Liu, M., Arvan, P., and Tsai, B. (2015). PDI reductase acts on Akita mutant proinsulin to initiate retrotranslocation along the Hrd1/Sel1L-p97 axis. Mol. Biol. Cell 26, 3413–3423. doi:10.1091/mbc.E15-01-0034
He, Y., Beatty, A., Han, X., Ji, Y., Ma, X., Adelstein, R. S., et al. (2012). Nonmuscle myosin IIB links cytoskeleton to IRE1α signaling during ER stress. Dev. Cell 23, 1141–1152. doi:10.1016/j.devcel.2012.11.006
Hetz, C., and Papa, F. R. (2018). The unfolded protein response and cell fate control. Cell 69, 169–181. doi:10.1016/j.molcel.2017.06.017
Hetz, C., and Saxena, S. (2017). ER stress and the unfolded protein response in neurodegeneration. Nat. Rev. Neurol. 13, 477–491. doi:10.1038/nrneurol.2017.99
Hetz, C., Zhang, K., and Kaufman, R. J. (2020). Mechanisms, regulation and functions of the unfolded protein response. Nat. Rev. Mol. Cell Biol. 21, 421–438. doi:10.1038/s41580-020-0250-z
Hidvegi, T., Ewing, M., Hale, P., Dippold, C., Beckett, C., Kemp, C., et al. (2010). An autophagy-enhancing drug promotes degradation of mutant alpha1-antitrypsin Z and reduces hepatic fibrosis. Science 329, 229–232. doi:10.1126/science.1190354
Hilado, M. A., and Randhawa, R. S. (2018). A novel mutation in the proopiomelanocortin (POMC) gene of a hispanic child: Metformin treatment shows a beneficial impact on the body mass index. J. Pediatr. Endocrinol. Metab. 31, 815–819. doi:10.1515/jpem-2017-0467
Hollien, J., and Weissman, J. S. (2006). Decay of endoplasmic reticulum-localized mRNAs during the unfolded protein response. Science 313, 104–107. doi:10.1126/science.1129631
Hoozemans, J. J., van Haastert, E. S., Nijholt, D. A., Rozemuller, A. J., Eikelenboom, P., and Scheper, W. (2009). The unfolded protein response is activated in pretangle neurons in Alzheimer's disease hippocampus. Am. J. Pathol. 174, 1241–1251. doi:10.2353/ajpath.2009.080814
Horimoto, S., Ninagawa, S., Okada, T., Koba, H., Sugimoto, T., Kamiya, Y., et al. (2013). The unfolded protein response transducer ATF6 represents a novel transmembrane-type endoplasmic reticulum-associated degradation substrate requiring both mannose trimming and SEL1L protein. J. Biol. Chem. 288, 31517–31527. doi:10.1074/jbc.M113.476010
Horn, S. C., Hanna, J., Hirsch, C., Volkwein, C., Schütz, A., Heinemann, U., et al. (2009). USA1 functions as a scaffold of the HRD-ubiquitin ligase. Mol. Cell 36, 782–793. doi:10.1016/j.molcel.2009.10.015
Hosokawa, N., Wada, I., Nagasawa, K., Moriyama, T., Okawa, K., and Nagata, K. (2008). Human XTP3-B forms an endoplasmic reticulum quality control scaffold with the HRD1-SEL1L ubiquitin ligase complex and BiP. J. Biol. Chem. 283, 20914–20924. doi:10.1074/jbc.M709336200
Hwang, J., and Qi, L. (2018). Quality control in the endoplasmic reticulum: Crosstalk between ERAD and UPR pathways. Trends biochem. Sci. 43, 593–605. doi:10.1016/j.tibs.2018.06.005
Hwang, J., Walczak, C. P., Shaler, T. A., Olzmann, J. A., Zhang, L., Elias, J. E., et al. (2017). Characterization of protein complexes of the endoplasmic reticulum-associated degradation E3 ubiquitin ligase Hrd1. J. Biol. Chem. 292, 9104–9116. doi:10.1074/jbc.M117.785055
Hyrskyluoto, A., Bruelle, C., Lundh, S. H., Do, H. T., Kivinen, J., Rappou, E., et al. (2014). Ubiquitin-specific protease-14 reduces cellular aggregates and protects against mutant huntingtin-induced cell degeneration: Involvement of the proteasome and ER stress-activated kinase IRE1α. Hum. Mol. Genet. 23, 5928–5939. doi:10.1093/hmg/ddu317
Iavarone, F., Di Lorenzo, G., and Settembre, C. (2022). Regulatory events controlling ER-phagy. Curr. Opin. Cell Biol. 76, 102084. doi:10.1016/j.ceb.2022.102084
Imai, Y., Soda, M., and Takahashi, R. (2000). Parkin suppresses unfolded protein stress-induced cell death through its E3 ubiquitin-protein ligase activity. J. Biol. Chem. 275, 35661–35664. doi:10.1074/jbc.C000447200
Itakura, E., Kishi-Itakura, C., and Mizushima, N. (2012). The hairpin-type tail-anchored SNARE syntaxin 17 targets to autophagosomes for fusion with endosomes/lysosomes. Cell 151, 1256–1269. doi:10.1016/j.cell.2012.11.001
Ito, M., Yu, R. N., and Jameson, J. L. (1999). Mutant vasopressin precursors that cause autosomal dominant neurohypophyseal diabetes insipidus retain dimerization and impair the secretion of wild-type proteins. J. Biol. Chem. 274, 9029–9037. doi:10.1074/jbc.274.13.9029
Jeong, H., Sim, H. J., Song, E. K., Lee, H., Ha, S. C., Jun, Y., et al. (2016). Crystal structure of SEL1L: Insight into the roles of SLR motifs in ERAD pathway. Sci. Rep. 6, 20261. doi:10.1038/srep20261
Ji, C. H., Kim, H. Y., Heo, A. J., Lee, S. H., Lee, M. J., Kim, S. B., et al. (2019). The N-degron pathway mediates ER-phagy. Cell 75, 1058–1072. doi:10.1016/j.molcel.2019.06.028
Ji, Y., Kim, H., Yang, L., Sha, H., Roman, C. A., Long, Q., et al. (2016). The Sel1L-hrd1 endoplasmic reticulum-associated degradation complex manages a key checkpoint in B cell development. Cell Rep. 16, 2630–2640. doi:10.1016/j.celrep.2016.08.003
Kammoun, H. L., Chabanon, H., Hainault, I., Luquet, S., Magnan, C., Koike, T., et al. (2009). GRP78 expression inhibits insulin and ER stress-induced SREBP-1c activation and reduces hepatic steatosis in mice. J. Clin. Invest. 119, 1201–1215. doi:10.1172/JCI37007
Kanda, S., Yanagitani, K., Yokota, Y., Esaki, Y., and Kohno, K. (2016). Autonomous translational pausing is required for XBP1u mRNA recruitment to the ER via the SRP pathway. Proc. Natl. Acad. Sci. U. S. A. 113, E5886–E5895. doi:10.1073/pnas.1604435113
Karatas, E., and Bouchecareilh, M. (2020). Alpha 1-antitrypsin deficiency: A disorder of proteostasis-mediated protein folding and trafficking pathways. Int. J. Mol. Sci. 21, 1493. doi:10.3390/ijms21041493
Kaushal, S., Annamali, M., Blomenkamp, K., Rudnick, D., Halloran, D., Brunt, E. M., et al. (2010). Rapamycin reduces intrahepatic alpha-1-antitrypsin mutant Z protein polymers and liver injury in a mouse model. Exp. Biol. Med. 235, 700–709. doi:10.1258/ebm.2010.009297
Kaushik, S., Arias, E., Kwon, H., Lopez, N. M., Athonvarangkul, D., Sahu, S., et al. (2012). Loss of autophagy in hypothalamic POMC neurons impairs lipolysis. EMBO Rep. 13, 258–265. doi:10.1038/embor.2011.260
Keles, U., Iscan, E., Yilmaz, H. E., Karakülah, G., Suner, A., Bal, E., et al. (2020). Differential expression of full-length and NH(2) terminally truncated FAM134B isoforms in normal physiology and cancer. Am. J. Physiol. Gastrointest. Liver Physiol. 319, G733–G747. doi:10.1152/ajpgi.00094.2020
Kim, G. H., Shi, G., Somlo, D. R., Haataja, L., Song, S., Long, Q., et al. (2018). Hypothalamic ER-associated degradation regulates POMC maturation, feeding, and age-associated obesity. J. Clin. Invest. 128, 1125–1140. doi:10.1172/JCI96420
Kim, P. S., and Arvan, P. (1998). Endocrinopathies in the family of endoplasmic reticulum (ER) storage diseases: Disorders of protein trafficking and the role of ER molecular chaperones. Endocr. Rev. 19, 173–202. doi:10.1210/edrv.19.2.0327
Kim, P. S., Kwon, O. Y., and Arvan, P. (1996). An endoplasmic reticulum storage disease causing congenital goiter with hypothyroidism. J. Cell Biol. 133, 517–527. doi:10.1083/jcb.133.3.517
Klemm, E. J., Spooner, E., and Ploegh, H. L. (2011). Dual role of ancient ubiquitous protein 1 (AUP1) in lipid droplet accumulation and endoplasmic reticulum (ER) protein quality control. J. Biol. Chem. 286, 37602–37614. doi:10.1074/jbc.M111.284794
Kohno, S., Shiozaki, Y., Keenan, A. L., Miyazaki-Anzai, S., and Miyazaki, M. (2019). An N-terminal-truncated isoform of FAM134B (FAM134B-2) regulates starvation-induced hepatic selective ER-phagy. Alliance 2, e201900340. doi:10.26508/lsa.201900340
Kruse, K. B., Brodsky, J. L., and McCracken, A. A. (2006). Characterization of an ERAD gene as VPS30/ATG6 reveals two alternative and functionally distinct protein quality control pathways: One for soluble Z variant of human alpha-1 proteinase inhibitor (A1PiZ) and another for aggregates of A1PiZ. Mol. Biol. Cell 17, 203–212. doi:10.1091/mbc.e04-09-0779
Kühnen, P., Clément, K., Wiegand, S., Blankenstein, O., Gottesdiener, K., Martini, L. L., et al. (2016). Proopiomelanocortin deficiency treated with a melanocortin-4 receptor agonist. N. Engl. J. Med. 375, 240–246. doi:10.1056/NEJMoa1512693
Kumari, D., and Brodsky, J. L. (2021). The targeting of native proteins to the endoplasmic reticulum-associated degradation (ERAD) pathway: An expanding repertoire of regulated substrates. Biomolecules 11, 1185. doi:10.3390/biom11081185
Kuscuoglu, D., Janciauskiene, S., Hamesch, K., Haybaeck, J., Trautwein, C., and Strnad, P. (2018). Liver - master and servant of serum proteome. J. Hepatol. 69, 512–524. doi:10.1016/j.jhep.2018.04.018
Lee, A. H., Iwakoshi, N. N., and Glimcher, L. H. (2003). XBP-1 regulates a subset of endoplasmic reticulum resident chaperone genes in the unfolded protein response. Mol. Cell Biol. 23, 7448–7459. doi:10.1128/mcb.23.21.7448-7459.2003
Lee, J. E., Cathey, P. I., Wu, H., Parker, R., and Voeltz, G. K. (2020). Endoplasmic reticulum contact sites regulate the dynamics of membraneless organelles. Science 367, eaay7108. doi:10.1126/science.aay7108
Lee, J. H., Won, S. M., Suh, J., Son, S. J., Moon, G. J., Park, U. J., et al. (2010). Induction of the unfolded protein response and cell death pathway in Alzheimer's disease, but not in aged Tg2576 mice. Exp. Mol. Med. 42, 386–394. doi:10.3858/emm.2010.42.5.040
Leitman, J., Barak, B., Benyair, R., Shenkman, M., Ashery, U., Hartl, F. U., et al. (2014). ER stress-induced eIF2-alpha phosphorylation underlies sensitivity of striatal neurons to pathogenic huntingtin. PLoS One 9, e90803. doi:10.1371/journal.pone.0090803
Lemberg, M. K., and Strisovsky, K. (2021). Maintenance of organellar protein homeostasis by ER-associated degradation and related mechanisms. Mol. Cell 81, 2507–2519. doi:10.1016/j.molcel.2021.05.004
Leon, C., and Bouchecareilh, M. (2021). The autophagy pathway: A critical route in the disposal of alpha 1-antitrypsin aggregates that holds many mysteries. Int. J. Mol. Sci. 22, 1875. doi:10.3390/ijms22041875
Li, H., and Sun, S. (2021). Protein aggregation in the ER: Calm behind the storm. Cells 10, 3337. doi:10.3390/cells10123337
Li, Y., Xia, Y., Cheng, X., Kleiner, D. E., Hewitt, S. M., Sproch, J., et al. (2019). Hepatitis B surface antigen activates unfolded protein response in forming ground glass hepatocytes of chronic hepatitis B. Viruses 11, 386. doi:10.3390/v11040386
Liang, J. R., Lingeman, E., Luong, T., Ahmed, S., Muhar, M., Nguyen, T., et al. (2020). A genome-wide ER-phagy screen highlights key roles of mitochondrial metabolism and ER-resident UFMylation. Cell 180, 1160–1177. doi:10.1016/j.cell.2020.02.017
Loi, M., Raimondi, A., Morone, D., and Molinari, M. (2019). ESCRT-III-driven piecemeal micro-ER-phagy remodels the ER during recovery from ER stress. Nat. Commun. 10, 5058. doi:10.1038/s41467-019-12991-z
Lomas, D. A., Evans, D. L., Finch, J. T., and Carrell, R. W. (1992). The mechanism of Z alpha 1-antitrypsin accumulation in the liver. Nature 357, 605–607. doi:10.1038/357605a0
Lu, P. D., Harding, H. P., and Ron, D. (2004). Translation reinitiation at alternative open reading frames regulates gene expression in an integrated stress response. J. Cell Biol. 167, 27–33. doi:10.1083/jcb.200408003
Mahler, H. C., Friess, W., Grauschopf, U., and Kiese, S. (2009). Protein aggregation: Pathways, induction factors and analysis. J. Pharm. Sci. 98, 2909–2934. doi:10.1002/jps.21566
Malhotra, R., Warne, J. P., Salas, E., Xu, A. W., and Debnath, J. (2015). Loss of Atg12, but not Atg5, in pro-opiomelanocortin neurons exacerbates diet-induced obesity. Autophagy 11, 145–154. doi:10.1080/15548627.2014.998917
Marcora, M. S., Belfiori-Carrasco, L. F., Bocai, N. I., Morelli, L., and Castaño, E. M. (2017). Amyloid-β42 clearance and neuroprotection mediated by X-box binding protein 1 signaling decline with aging in the Drosophila brain. Neurobiol. Aging 60, 57–70. doi:10.1016/j.neurobiolaging.2017.08.012
Margariti, A., Li, H., Chen, T., Martin, D., Vizcay-Barrena, G., Alam, S., et al. (2013). XBP1 mRNA splicing triggers an autophagic response in endothelial cells through BECLIN-1 transcriptional activation. J. Biol. Chem. 288, 859–872. doi:10.1074/jbc.M112.412783
Marini, J. C., Forlino, A., Cabral, W. A., Barnes, A. M., Antonio, S. J. D., Milgrom, S., et al. (2007). Consortium for osteogenesis imperfecta mutations in the helical domain of type I collagen: Regions rich in lethal mutations align with collagen binding sites for integrins and proteoglycans. Hum. Mutat. 28, 209–221. doi:10.1002/humu.20429
Matteis, De M. A., and Rega, L. R. (2015). Endoplasmic reticulum-Golgi complex membrane contact sites. Curr. Opin. Cell Biol. 35, 43. doi:10.1016/j.ceb.2015.04.001
Mehrtash, A. B., and Hochstrasser, M. (2019). Ubiquitin-dependent protein degradation at the endoplasmic reticulum and nuclear envelope. Semin. Cell Dev. Biol. 93, 111–124. doi:10.1016/j.semcdb.2018.09.013
Mercado, G., Castillo, V., Soto, P., and Sidhu, A. (2016). ER stress and Parkinson's disease: Pathological inputs that converge into the secretory pathway. Brain Res. 1648, 626–632. doi:10.1016/j.brainres.2016.04.042
Meyer, H., Bug, M., and Bremer, S. (2012). Emerging functions of the VCP/p97 AAA-ATPase in the ubiquitin system. Nat. Cell Biol. 14, 117–123. doi:10.1038/ncb2407
Millington, G. W. (2006). Proopiomelanocortin (POMC): The cutaneous roles of its melanocortin products and receptors. Clin. Exp. Dermatol. 31, 407–412. doi:10.1111/j.1365-2230.2006.02128.x
Miranda, E., Pérez, J., Ekeowa, U. I., Hadzic, N., Kalsheker, N., Gooptu, B., et al. (2010). A novel monoclonal antibody to characterize pathogenic polymers in liver disease associated with alpha1-antitrypsin deficiency. Hepatology 52, 1078–1088. doi:10.1002/hep.23760
Mitrea, D. M., and Kriwacki, R. W. (2016). Phase separation in biology; functional organization of a higher order. Cell Commun. Signal. 14, 1. doi:10.1186/s12964-015-0125-7
Miyata, T., Hagiwara, D., Hodai, Y., Miwata, T., Kawaguchi, Y., Kurimoto, J., et al. (2020). Degradation of mutant protein aggregates within the endoplasmic reticulum of vasopressin neurons. iScience 23, 101648. doi:10.1016/j.isci.2020.101648
Miyazaki, Y., Mizumoto, K., Dey, G., Kudo, T., Perrino, J., Chen, L. C., et al. (2016). A method to rapidly create protein aggregates in living cells. Nat. Commun. 7, 11689. doi:10.1038/ncomms11689
Moilanen, A., Korhonen, K., Saaranen, M. J., and Ruddock, L. W. (2018). Molecular analysis of human Ero1 reveals novel regulatory mechanisms for oxidative protein folding. Life Sci. Alliance 1, e201800090. doi:10.26508/lsa.201800090
Molinari, M. (2021). ER-phagy responses in yeast, plants, and mammalian cells and their crosstalk with UPR and ERAD. Dev. Cell 56, 949–966. doi:10.1016/j.devcel.2021.03.005
Mudogo, C. N., Falke, S., Brognaro, H., Duszenko, M., and Betzel, C. (2020). Protein phase separation and determinants of in cell crystallization. Traffic 21, 220–230. doi:10.1111/tra.12711
Mueller, B., Klemm, E. J., Spooner, E., Claessen, J. H., and Ploegh, H. L. (2008). SEL1L nucleates a protein complex required for dislocation of misfolded glycoproteins. Proc. Natl. Acad. Sci. U. S. A. 105, 12325–12330. doi:10.1073/pnas.0805371105
Neal, S., Jaeger, P. A., Duttke, S. H., Benner, C., Glass, C. K., Ideker, T., et al. (2018). The Dfm1 Derlin is required for ERAD retrotranslocation of integral membrane proteins. Cell 69, 306–320. doi:10.1016/j.molcel.2017.12.012
Needham, P. G., Guerriero, C. J., and Brodsky, J. L. (2019). Chaperoning endoplasmic reticulum-associated degradation (ERAD) and protein conformational diseases. Cold Spring Harb. Perspect. Biol. 11, a033928. doi:10.1101/cshperspect.a033928
Noda, N. N., Wang, Z., and Zhang, H. (2020). Liquid-liquid phase separation in autophagy. J. Cell Biol. 219, e202004062. doi:10.1083/jcb.202004062
Nthiga, T. M., Kumar Shrestha, B., Sjøttem, E., Bruun, J. A., Bowitz Larsen, K., Bhujabal, Z., et al. (2020). CALCOCO1 acts with VAMP-associated proteins to mediate ER-phagy. EMBO J. 39, e103649. doi:10.15252/embj.2019103649
Oka, O. B., Pringle, M. A., Schopp, I. M., Braakman, I., and Bulleid, N. J. (2013). ERdj5 is the ER reductase that catalyzes the removal of non-native disulfides and correct folding of the LDL receptor. Mol. Cell 50, 793–804. doi:10.1016/j.molcel.2013.05.014
Oka, O. B., van Lith, M., Rudolf, J., Tungkum, W., Pringle, M. A., and Bulleid, N. J. (2019). ERp18 regulates activation of ATF6α during unfolded protein response. EMBO J. 38, e100990. doi:10.15252/embj.2018100990
Okumura, M., Noi, K., Kanemura, S., Kinoshita, M., Saio, T., Inoue, Y., et al. (2019). Dynamic assembly of protein disulfide isomerase in catalysis of oxidative folding. Nat. Chem. Biol. 15, 499–509. doi:10.1038/s41589-019-0268-8
Olivari, S., Galli, C., Alanen, H., Ruddock, L., and Molinari, M. (2005). A novel stress-induced EDEM variant regulating endoplasmic reticulum-associated glycoprotein degradation. J. Biol. Chem. 280, 2424–2428. doi:10.1074/jbc.C400534200
Olzmann, J. A., Kopito, R. R., and Christianson, J. C. (2013). The mammalian endoplasmic reticulum-associated degradation system. Cold Spring Harb. Perspect. Biol. 5, a013185. doi:10.1101/cshperspect.a013185
Omari, S., Makareeva, E., Roberts-Pilgrim, A., Mirigian, L., Jarnik, M., Ott, C., et al. (2018). Noncanonical autophagy at ER exit sites regulates procollagen turnover. Proc. Natl. Acad. Sci. U. S. A. 115, E10099–E10108. doi:10.1073/pnas.1814552115
Omura, T., Siekevitz, P., and Palade, G. E. (1967). Turnover of constituents of the endoplasmic reticulum membranes of rat hepatocytes. J. Biol. Chem. 242, 2389–2396. doi:10.1016/s0021-9258(18)95974-0
Padilla-Godínez, F. J., Ramos-Acevedo, R., Martínez-Becerril, H. A., Bernal-Conde, L. D., Garrido-Figueroa, J. F., Hiriart, M., et al. (2021). Protein misfolding and aggregation: The relatedness between Parkinson's disease and hepatic endoplasmic reticulum storage disorders. Int. J. Mol. Sci. 22, 12467. doi:10.3390/ijms222212467
Pagani, M., Fabbri, M., Benedetti, C., Fassio, A., Pilati, S., Bulleid, N. J., et al. (2000). Endoplasmic reticulum oxidoreductin 1-lbeta (ERO1-Lbeta), a human gene induced in the course of the unfolded protein response. J. Biol. Chem. 275, 23685–23692. doi:10.1074/jbc.M003061200
Pastore, N., Ballabio, A., and Brunetti-Pierri, N. (2013). Autophagy master regulator TFEB induces clearance of toxic SERPINA1/α-1-antitrypsin polymers. Autophagy 9, 1094–1096. doi:10.4161/auto.24469
Pastore, N., Blomenkamp, K., Annunziata, F., Piccolo, P., Mithbaokar, P., Maria Sepe, R., et al. (2013). Gene transfer of master autophagy regulator TFEB results in clearance of toxic protein and correction of hepatic disease in alpha-1-anti-trypsin deficiency. EMBO Mol. Med. 5, 397–412. doi:10.1002/emmm.201202046
Philo, J. S., and Arakawa, T. (2009). Mechanisms of protein aggregation. Curr. Pharm. Biotechnol. 10, 348–351. doi:10.2174/138920109788488932
Pincus, D., Chevalier, M. W., Aragón, T., van Anken, E., Vidal, S. E., El-Samad, H., et al. (2010). BiP binding to the ER-stress sensor Ire1 tunes the homeostatic behavior of the unfolded protein response. PLoS Biol. 8, e1000415. doi:10.1371/journal.pbio.1000415
Plemper, R. K., Böhmler, S., Bordallo, J., Sommer, T., and Wolf, D. H. (1997). Mutant analysis links the translocon and BiP to retrograde protein transport for ER degradation. Nature 388, 891–895. doi:10.1038/42276
Preissler, S., and Ron, D. (2019). Erratum: Early events in the endoplasmic reticulum unfolded protein response. Cold Spring Harb. Perspect. Biol. 10, a037309. doi:10.1101/cshperspect.a037309
Pye, V. E., Beuron, F., Keetch, C. A., McKeown, C., Robinson, C. V., Meyer, H. H., et al. (2007). Structural insights into the p97-Ufd1-Npl4 complex. Proc. Natl. Acad. Sci. U. S. A. 104, 467–472. doi:10.1073/pnas.0603408104
Qiao, X., Zhang, Y., Ye, A., Zhang, Y., Xie, T., Lv, Z., et al. (2022). ER reductive stress caused by Ero1α S-nitrosation accelerates senescence. Free. Radic. Biol. Med. 180, 165–178. doi:10.1016/j.freeradbiomed.2022.01.006
Qin, J., Guo, Y., Xue, B., Shi, P., Chen, Y., Su, Q. P., et al. (2020). ER-mitochondria contacts promote mtDNA nucleoids active transportation via mitochondrial dynamic tubulation. Nat. Commun. 11, 4471. doi:10.1038/s41467-020-18202-4
Qu, D., Teckman, J. H., Omura, S., and Perlmutter, D. H. (1996). Degradation of a mutant secretory protein, alpha1-antitrypsin Z, in the endoplasmic reticulum requires proteasome activity. J. Biol. Chem. 271, 22791–22795. doi:10.1074/jbc.271.37.22791
Rao, B., Li, S., Yao, D., Wang, Q., Xia, Y., Jia, Y., et al. (2021). The cryo-EM structure of an ERAD protein channel formed by tetrameric human Derlin-1. Sci. Adv. 7, eabe8591. doi:10.1126/sciadv.abe8591
Reggio, A., Buonomo, V., Berkane, R., Bhaskara, R. M., Tellechea, M., Peluso, I., et al. (2021). Role of FAM134 paralogues in endoplasmic reticulum remodeling, ER-phagy, and Collagen quality control. EMBO Rep. 22, e52289. doi:10.15252/embr.202052289
Remondelli, P., and Renna, M. (2017). The endoplasmic reticulum unfolded protein response in neurodegenerative disorders and its potential therapeutic significance. Front. Mol. Neurosci. 10, 187. doi:10.3389/fnmol.2017.00187
Robinson, P. J., Kanemura, S., Cao, X., and Bulleid, N. J. (2020). Protein secondary structure determines the temporal relationship between folding and disulfide formation. J. Biol. Chem. 295, 2438–2448. doi:10.1074/jbc.RA119.011983
Rogov, V., Dötsch, V., Johansen, T., and Kirkin, V. (2014). Interactions between autophagy receptors and ubiquitin-like proteins form the molecular basis for selective autophagy. Mol. Cell 53, 167–178. doi:10.1016/j.molcel.2013.12.014
Rossi, A. M., and Taylor, C. W. (2020). Reliable measurement of free Ca(2+) concentrations in the ER lumen using Mag-Fluo-4. Cell Calcium 87, 102188. doi:10.1016/j.ceca.2020.102188
Rowland, A. A., and Voeltz, G. K. (2012). Endoplasmic reticulum-mitochondria contacts: Function of the junction. Nat. Rev. Mol. Cell Biol. 13, 607–625. doi:10.1038/nrm3440
Rutishauser, J., and Spiess, M. (2002). Endoplasmic reticulum storage diseases. Swiss Med. Wkly. 132, 211–222. doi:10.4414/smw.2002.09861
Sandhaus, R. A. (2004). alpha1-Antitrypsin deficiency. 6: new and emerging treatments for alpha1-antitrypsin deficiency. Thorax 59, 904–909. doi:10.1136/thx.2003.006551
Sato, Y., Tsuchiya, H., Yamagata, A., Okatsu, K., Tanaka, K., Saeki, Y., et al. (2019). Structural insights into ubiquitin recognition and Ufd1 interaction of Npl4. Nat. Commun. 10, 5708. doi:10.1038/s41467-019-13697-y
Saxena, S., Cabuy, E., and Caroni, P. (2009). A role for motoneuron subtype-selective ER stress in disease manifestations of FALS mice. Nat. Neurosci. 12, 627–636. doi:10.1038/nn.229712
Schaeffer, C., Merella, S., Pasqualetto, E., Lazarevic, D., and Rampoldi, L. (2017). Mutant uromodulin expression leads to altered homeostasis of the endoplasmic reticulum and activates the unfolded protein response. PLoS One 12, e0175970. doi:10.1371/journal.pone.0175970
Schmidt, C. C., Vasic, V., and Stein, A. (2020). Doa10 is a membrane protein retrotranslocase in ER-associated protein degradation. Elife 9, e56945. doi:10.7554/eLife.56945
Schoebel, S., Mi, W., Stein, A., Ovchinnikov, S., Pavlovicz, R., DiMaio, F., et al. (2017). Cryo-EM structure of the protein-conducting ERAD channel Hrd1 in complex with Hrd3. Nature 548, 352–355. doi:10.1038/nature23314
Schuck, S., Gallagher, C. M., and Walter, P. (2014). ER-phagy mediates selective degradation of endoplasmic reticulum independently of the core autophagy machinery. J. Cell Sci. 127, 4078–4088. doi:10.1242/jcs.154716
Schultz, M. L., Krus, K. L., Kaushik, S., Dang, D., Chopra, R., Qi, L., et al. (2018). Coordinate regulation of mutant NPC1 degradation by selective ER autophagy and MARCH6-dependent ERAD. Nat. Commun. 9, 3671. doi:10.1038/s41467-018-06115-2
Schwarz, D. S., and Blower, M. D. (2016). The endoplasmic reticulum: Structure, function and response to cellular signaling. Cell. Mol. Life Sci. 73, 79–94. doi:10.1007/s00018-015-2052-6
Sepulveda, D., Rojas-Rivera, D., Rodríguez, D. A., Groenendyk, J., Köhler, A., Lebeaupin, C., et al. (2018). Interactome screening identifies the ER luminal chaperone Hsp47 as a regulator of the unfolded protein response transducer IRE1α. Mol. Cell 69, 238–252. doi:10.1016/j.molcel.2017.12.028
Shacham, T., Sharma, N., and Lederkremer, G. Z. (2019). Protein misfolding and ER stress in huntington's disease. Front. Mol. Biosci. 6, 20. doi:10.3389/fmolb.2019.00020
Shenkman, M., Groisman, B., Ron, E., Avezov, E., Hendershot, L. M., and Lederkremer, G. Z. (2013). A shared endoplasmic reticulum-associated degradation pathway involving the EDEM1 protein for glycosylated and nonglycosylated proteins. J. Biol. Chem. 288, 2167–2178. doi:10.1074/jbc.M112.438275
Shin, J. S., and Yu, M. H. (2002). Kinetic dissection of alpha 1-antitrypsin inhibition mechanism. J. Biol. Chem. 277, 11629–11635. doi:10.1074/jbc.M111168200
Shoulders, M. D., Ryno, L. M., Genereux, J. C., Moresco, J. J., Tu, P. G., Wu, C., et al. (2013). Stress-independent activation of XBP1s and/or ATF6 reveals three functionally diverse ER proteostasis environments. Cell Rep. 3, 1279–1292. doi:10.1016/j.celrep.2013.03.024
Sinnige, T., Stroobants, K., Dobson, C. M., and Vendruscolo, M. (2020). Biophysical studies of protein misfolding and aggregation in in vivo models of Alzheimer's and Parkinson's diseases. Q. Rev. Biophys. 49, e22. doi:10.1017/S0033583520000025
Smith, M. D., Harley, M. E., Kemp, A. J., Wills, J., Lee, M., Arends, M., et al. (2018). CCPG1 is a non-canonical autophagy cargo receptor essential for ER-phagy and pancreatic ER proteostasis. Proteostasis. Dev. Cell 44, 217–232. doi:10.1016/j.devcel.2017.11.024
Snead, W. T., Hayden, C. C., Gadok, A. K., Zhao, C., Lafer, E. M., Rangamani, P., et al. (2017). Membrane fission by protein crowding. Proc. Natl. Acad. Sci. U. S. A. 114, E3258–E3267. doi:10.1073/pnas.1616199114
Song, S., Tan, J., Miao, Y., and Zhang, Q. (2018). Crosstalk of ER stress-mediated autophagy and ER-phagy: Involvement of UPR and the core autophagy machinery. J. Cell. Physiol. 233, 3867–3874. doi:10.1002/jcp.26137
Sørensen, S., Ranheim, T., Bakken, K. S., Leren, T. P., and Kulseth, M. A. (2006). Retention of mutant low density lipoprotein receptor in endoplasmic reticulum (ER) leads to ER stress. J. Biol. Chem. 281, 468–476. doi:10.1074/jbc.M507071200
Soto, C., and Pritzkow, S. (2018). Protein misfolding, aggregation, and conformational strains in neurodegenerative diseases. Nat. Neurosci. 21, 1332–1340. doi:10.1038/s41593-018-0235-9
Stefely, J. A., Zhang, Y., Freiberger, E. C., Kwiecien, N. W., Thomas, H. E., Davis, A. M., et al. (2020). Mass spectrometry proteomics reveals a function for mammalian CALCOCO1 in MTOR-regulated selective autophagy. Autophagy 16, 2219–2237. doi:10.1080/15548627.2020.1719746
Stephani, M., Picchianti, L., and Dagdas, Y. (2021). C53 is a cross-kingdom conserved reticulophagy receptor that bridges the gap betweenselective autophagy and ribosome stalling at the endoplasmic reticulum. Autophagy 17, 586–587. doi:10.1080/15548627.2020.1846304
Stephani, M., Picchianti, L., Gajic, A., Beveridge, R., Skarwan, E., Sanchez de Medina Hernandez, V., et al. (2020). A cross-kingdom conserved ER-phagy receptor maintains endoplasmic reticulum homeostasis during stress. Elife 9, e58396. doi:10.7554/eLife.58396
Stolk, J., Tov, N., Chapman, K. R., Fernandez, P., MacNee, W., Hopkinson, N. S., et al. (2019). Efficacy and safety of inhaled α1-antitrypsin in patients with severe α1-antitrypsin deficiency and frequent exacerbations of COPD. Eur. Respir. J. 54, 1900673. doi:10.1183/13993003.00673-2019
Strnad, P., McElvaney, N. G., and Lomas, D. A. (2020). Alpha(1)-Antitrypsin deficiency. N. Engl. J. Med. 382, 1443–1455. doi:10.1056/NEJMra1910234
Sun, J., Xiong, Y., Li, X., Haataja, L., Chen, W., Mir, S. A., et al. (2020). Role of proinsulin self-association in mutant INS gene-induced diabetes of youth. Diabetes 69, 954–964. doi:10.2337/db19-1106
Sun, S., Lourie, R., Cohen, S. B., Ji, Y., Goodrich, J. K., Poole, A. C., et al. (2016). Epithelial Sel1L is required for the maintenance of intestinal homeostasis. Mol. Biol. Cell 27, 483–490. doi:10.1091/mbc.E15-10-0724
Sun, S., Shi, G., Han, X., Francisco, A. B., Ji, Y., Mendonça, N., et al. (2014). Sel1L is indispensable for mammalian endoplasmic reticulum-associated degradation, endoplasmic reticulum homeostasis, and survival. Proc. Natl. Acad. Sci. U. S. A. 111, E582–E591. doi:10.1073/pnas.1318114111
Sun, S., Shi, G., Sha, H., Ji, Y., Han, X., Shu, X., et al. (2015). IRE1α is an endogenous substrate of endoplasmic-reticulum-associated degradation. Nat. Cell Biol. 17, 1546–1555. doi:10.1038/ncb3266
Sun, Z., and Brodsky, J. L. (2019). Protein quality control in the secretory pathway. J. Cell Biol. 218, 3171–3187. doi:10.1083/jcb.201906047
Sung, J. J., Pardeshi, N. N., Mulder, A. M., Mulligan, S. K., Quispe, J., On, K., et al. (2015). Transmission electron microscopy as an orthogonal method to characterize protein aggregates. J. Pharm. Sci. 104, 750–759. doi:10.1002/jps.24157
Sveger, T. (1976). Liver disease in alpha1-antitrypsin deficiency detected by screening of 200,000 infants. N. Engl. J. Med. 294, 1316–1321. doi:10.1056/NEJM197606102942404
Tang, Y., Fickert, P., Trauner, M., Marcus, N., Blomenkamp, K., and Teckman, J. (2016). Autophagy induced by exogenous bile acids is therapeutic in a model of α-1-AT deficiency liver disease. Am. J. Physiol. Gastrointest. Liver Physiol. 311, G156–G165. doi:10.1152/ajpgi.00143.2015
Taylor, R. C., and Dillin, A. (2013). XBP-1 is a cell-nonautonomous regulator of stress resistance and longevity. Cell 153, 1435–1447. doi:10.1016/j.cell.2013.05.042
Taylor, R. C., and Hetz, C. (2020). Mastering organismal aging through the endoplasmic reticulum proteostasis network. Aging Cell 19, e13265. doi:10.1111/acel.13265
Teckman, J. H., Burrows, J., Hidvegi, T., Schmidt, B., Hale, P. D., and Perlmutter, D. H. (2001). The proteasome participates in degradation of mutant alpha 1-antitrypsin Z in the endoplasmic reticulum of hepatoma-derived hepatocytes. J. Biol. Chem. 276, 44865–44872. doi:10.1074/jbc.M103703200
Teckman, J. H., and Perlmutter, D. H. (2000). Retention of mutant alpha(1)-antitrypsin Z in endoplasmic reticulum is associated with an autophagic response. Am. J. Physiol. Gastrointest. Liver Physiol. 279, G961–G974. doi:10.1152/ajpgi.2000.279.5.G961
Thepsuwan, P., Bhattacharya, A., Song, Z., Hippleheuser, S., Feng, S., Wei, X., et al. (2023). Hepatic SEL1L-HRD1 ER-associated degradation regulates systemic iron homeostasis via ceruloplasmin. Proc. Natl. Acad. Sci. U. S. A. 120, e2212644120. doi:10.1073/pnas.2212644120
Travers, K. J., Patil, C. K., Wodicka, L., Lockhart, D. J., Weissman, J. S., and Walter, P. (2000). Functional and genomic analyses reveal an essential coordination between the unfolded protein response and ER-associated degradation. Cell 101, 249–258. doi:10.1016/s0092-8674(00)80835-1
Tschurtschenthaler, M., Adolph, T. E., Ashcroft, J. W., Niederreiter, L., Bharti, R., Saveljeva, S., et al. (2017). Defective ATG16L1-mediated removal of IRE1α drives Crohn's disease-like ileitis. J. Exp. Med. 214, 401–422. doi:10.1084/jem.20160791
Twomey, E., Ji, Z., Wales, T., Bodnar, N., Ficarro, S., Marto, J., et al. (2019). Substrate processing by the Cdc48 ATPase complex is initiated by ubiquitin unfolding. Science 365, eaax1033. doi:10.1126/science.aax1033
Upton, J. P., Wang, L., Han, D., Wang, E. S., Huskey, N. E., Lim, L., et al. (2012). IRE1α cleaves select microRNAs during ER stress to derepress translation of proapoptotic Caspase-2. Science 338, 818–822. doi:10.1126/science.1226191
Urano, F., Wang, X., Bertolotti, A., Zhang, Y., Chung, P., Harding, H. P., et al. (2000). Coupling of stress in the ER to activation of JNK protein kinases by transmembrane protein kinase IRE1. Science 287, 664–666. doi:10.1126/science.287.5453.664
Urra, H., Dufey, E., Avril, T., Chevet, E., and Hetz, C. (2016). Endoplasmic reticulum stress and the hallmarks of cancer. Trends Cancer 2, 252–262. doi:10.1016/j.trecan.2016.03.007
Ushioda, R., Hoseki, J., Araki, K., Jansen, G., Thomas, D. Y., and Nagata, K. (2008). ERdj5 is required as a disulfide reductase for degradation of misfolded proteins in the ER. Science 321, 569–572. doi:10.1126/science.1159293
Ushioda, R., Miyamoto, A., Inoue, M., Watanabe, S., Okumura, M., Maegawa, K. I., et al. (2016). Redox-assisted regulation of Ca2+ homeostasis in the endoplasmic reticulum by disulfide reductase ERdj5. Proc. Natl. Acad. Sci. U. S. A. 113, E6055–E6063. doi:10.1073/pnas.1605818113
Vasic, V., Denkert, N., Schmidt, C. C., Riedel, D., Stein, A., and Meinecke, M. (2020). Hrd1 forms the retrotranslocation pore regulated by auto-ubiquitination and binding of misfolded proteins. Nat. Cell Biol. 22, 274–281. doi:10.1038/s41556-020-0473-4
Verdile, V., De Paola, E., and Paronetto, M. P. (2019). Aberrant phase transitions: Side effects and novel therapeutic strategies in human disease. Front. Genet. 10, 173. doi:10.3389/fgene.2019.00173
Wang, H., Li, Q., Shen, Y., Sun, A., Zhu, X., Fang, S., et al. (2011). The ubiquitin ligase Hrd1 promotes degradation of the Z variant alpha 1-antitrypsin and increases its solubility. Mol. Cell. Biochem. 346, 137–145. doi:10.1007/s11010-010-0600-9
Wang, H., Saint-Martin, C., Xu, J., Ding, L., Wang, R., Feng, W., et al. (2020). Biological behaviors of mutant proinsulin contribute to the phenotypic spectrum of diabetes associated with insulin gene mutations. Mol. Cell. Endocrinol. 518, 111025. doi:10.1016/j.mce.2020.111025
Wang, L., Chen, M., Yang, J., and Zhang, Z. (2013). LC3 fluorescent puncta in autophagosomes or in protein aggregates can be distinguished by FRAP analysis in living cells. Autophagy 9, 756–769. doi:10.4161/auto.23814
Wang, L., and Wang, C. C. (2023). Oxidative protein folding fidelity and redoxtasis in the endoplasmic reticulum. Trends biochem. Sci. 48, 40–52. doi:10.1016/j.tibs.2022.06.011
Wang, L., Wang, X., and Wang, C. C. (2015). Protein disulfide-isomerase, a folding catalyst and a redox-regulated chaperone. Free Radic. Biol. Med. 83, 305–313. doi:10.1016/j.freeradbiomed.2015.02.007
Wang, M., and Kaufman, R. J. (2016). Protein misfolding in the endoplasmic reticulum as a conduit to human disease. Nature 529, 326–335. doi:10.1038/nature17041
Wang, Q., Liu, Y., Soetandyo, N., Baek, K., Hegde, R., and Ye, Y. (2011). A ubiquitin ligase-associated chaperone holdase maintains polypeptides in soluble states for proteasome degradation. Mol. Cell 42, 758–770. doi:10.1016/j.molcel.2011.05.010
Wang, W., and Roberts, C. J. (2018). Protein aggregation - mechanisms, detection, and control. Int. J. Pharm. 550, 251–268. doi:10.1016/j.ijpharm.2018.08.043
Wang, Y., Cobanoglu, M. C., Li, J., Hidvegi, T., Hale, P., Ewing, M., et al. (2019). An analog of glibenclamide selectively enhances autophagic degradation of misfolded α1-antitrypsin Z. PLoS One 14, e0209748. doi:10.1371/journal.pone.0209748
Wei, J., Chen, L., Li, F., Yuan, Y., Wang, Y., Xia, W., et al. (2018). HRD1-ERAD controls production of the hepatokine FGF21 through CREBH polyubiquitination. EMBO J. 37, e98942. doi:10.15252/embj.201898942
Wei, J., Yuan, Y., Chen, L., Xu, Y., Zhang, Y., Wang, Y., et al. (2018). ER-associated ubiquitin ligase HRD1 programs liver metabolism by targeting multiple metabolic enzymes. Nat. Commun. 9, 3659. doi:10.1038/s41467-018-06091-7
Wewers, M. D., Casolaro, M. A., Sellers, S. E., Swayze, S. C., McPhaul, K. M., Wittes, J. T., et al. (1987). Replacement therapy for alpha 1-antitrypsin deficiency associated with emphysema. N. Engl. J. Med. 316, 1055–1062. doi:10.1056/NEJM198704233161704
Wiseman, R. L., Mesgarzadeh, J. S., and Hendershot, L. M. (2022). Reshaping endoplasmic reticulum quality control through the unfolded protein response. Mol. Cell 82, 1477–1491. doi:10.1016/j.molcel.2022.03.025
Wu, H., Carvalho, P., and Voeltz, G. K. (2018). Here, there, and everywhere: The importance of ER membrane contact sites. Science 361, eaan5835. doi:10.1126/science.aan5835
Wu, T., Zhao, F., Gao, B., Tan, C., Yagishita, N., Nakajima, T., et al. (2014). Hrd1 suppresses Nrf2-mediated cellular protection during liver cirrhosis. Genes Dev. 28, 708–722. doi:10.1101/gad.238246.114
Wu, X., Siggel, M., Ovchinnikov, S., Mi, W., Svetlov, V., Nudler, E., et al. (2020). Structural basis of ER-associated protein degradation mediated by the Hrd1 ubiquitin ligase complex. Science 368, eaaz2449. doi:10.1126/science.aaz2449
Xu, B., Allard, C., Alvarez-Mercado, A. I., Fuselier, T., Kim, J. H., Coons, L. A., et al. (2018). Estrogens promote misfolded proinsulin degradation to protect insulin production and delay diabetes. Cell Rep. 24, 181–196. doi:10.1016/j.celrep.2018.06.019
Xu, Y., Cai, M., Yang, Y., Huang, L., and Ye, Y. (2012). SGTA recognizes a noncanonical ubiquitin-like domain in the Bag6-Ubl4A-Trc35 complex to promote endoplasmic reticulum-associated degradation. Cell Rep. 2, 1633–1644. doi:10.1016/j.celrep.2012.11.010
Xu, Y., Zhao, F., Qiu, Q., Chen, K., Wei, J., Kong, Q., et al. (2016). The ER membrane-anchored ubiquitin ligase Hrd1 is a positive regulator of T-cell immunity. Nat. Commun. 7, 12073. doi:10.1038/ncomms12073
Yamamoto, K., Sato, T., Matsui, T., Sato, M., Okada, T., Yoshida, H., et al. (2007). Transcriptional induction of mammalian ER quality control proteins is mediated by single or combined action of ATF6alpha and XBP1. Dev. Cell 13, 365–376. doi:10.1016/j.devcel.2007.07.018
Yamasaki, M., Sendall, T. J., Pearce, M. C., Whisstock, J. C., and Huntington, J. A. (2011). Molecular basis of α1-antitrypsin deficiency revealed by the structure of a domain-swapped trimer. EMBO Rep. 12, 1011–1017. doi:10.1038/embor.2011.171
Yanagitani, K., Kimata, Y., Kadokura, H., and Kohno, K. (2011). Translational pausing ensures membrane targeting and cytoplasmic splicing of XBP1u mRNA. Science 331, 586–589. doi:10.1126/science.1197142
Yang, H., Liu, C., Zhong, Y., Luo, S., Monteiro, M. J., and Fang, S. (2010). Huntingtin interacts with the cue domain of gp78 and inhibits gp78 binding to ubiquitin and p97/VCP. PLoS One 5, e8905. doi:10.1371/journal.pone.0008905
Yang, Y., Kong, S., Zhang, Y., Melo-Cardenas, J., Gao, B., Zhang, Y., et al. (2018). The endoplasmic reticulum-resident E3 ubiquitin ligase Hrd1 controls a critical checkpoint in B cell development in mice. J. Biol. Chem. 293, 12934–12944. doi:10.1074/jbc.RA117.001267
Yoboue, E. D., Sitia, R., and Simmen, T. (2018). Redox crosstalk at endoplasmic reticulum (ER) membrane contact sites (MCS) uses toxic waste to deliver messages. Cell Death Dis. 9, 331. doi:10.1038/s41419-017-0033-4
Yu, J., Li, T., Liu, Y., Wang, X., Zhang, J., Wang, X., et al. (2020). Phosphorylation switches protein disulfide isomerase activity to maintain proteostasis and attenuate ER stress. EMBO J. 39, e103841. doi:10.15252/embj.2019103841
Zbinden, A., Pérez-Berlanga, M., De Rossi, P., and Polymenidou, M. (2020). Phase separation and neurodegenerative diseases: A disturbance in the force. Cell 55, 45–68. doi:10.1016/j.devcel.2020.09.014
Zhang, J., Zhu, Q., Wang, X., Yu, J., Chen, X., Wang, J., et al. (2018). Secretory kinase Fam20C tunes endoplasmic reticulum redox state via phosphorylation of Ero1α. EMBO J. 37, e98699. doi:10.15252/embj.201798699
Zhou, J., Liu, C. Y., Back, S. H., Clark, R. L., Peisach, D., Xu, Z., et al. (2006). The crystal structure of human IRE1 luminal domain reveals a conserved dimerization interface required for activation of the unfolded protein response. Proc. Natl. Acad. Sci. U. S. A. 103, 14343–14348. doi:10.1073/pnas.0606480103
Zielke, S., Kardo, S., Zein, L., Mari, M., Covarrubias-Pinto, A., Kinzler, M. N., et al. (2020). ATF4 links ER stress with reticulophagy in glioblastoma cells. Autophagy 17, 2432–2448. doi:10.1080/15548627.2020.1827780
Keywords: ER, protein quality control, protein aggregate, phase transition, ER storage disease
Citation: Chen G, Wei T, Ju F and Li H (2023) Protein quality control and aggregation in the endoplasmic reticulum: From basic to bedside. Front. Cell Dev. Biol. 11:1156152. doi: 10.3389/fcell.2023.1156152
Received: 02 February 2023; Accepted: 10 April 2023;
Published: 19 April 2023.
Edited by:
Roger Schneiter, University of Fribourg, SwitzerlandReviewed by:
Deepika Vasudevan, University of Pittsburgh, United StatesJianchao Zhang, University of Michigan, United States
Copyright © 2023 Chen, Wei, Ju and Li. This is an open-access article distributed under the terms of the Creative Commons Attribution License (CC BY). The use, distribution or reproduction in other forums is permitted, provided the original author(s) and the copyright owner(s) are credited and that the original publication in this journal is cited, in accordance with accepted academic practice. No use, distribution or reproduction is permitted which does not comply with these terms.
*Correspondence: Haisen Li, bGloczEyNkAxNjMuY29t
†These authors have contributed equally to this work