- Laboratory of Stem Cell and Neuro-Vascular Biology, National Heart, Lung, and Blood Institute, National Institutes of Health, Bethesda, MD, United States
The central nervous system (CNS) is considered as an immune privilege organ, based on experiments in the mid 20th century showing that the brain fails to mount an efficient immune response against an allogeneic graft. This suggests that in addition to the presence of the blood-brain barrier (BBB), the apparent absence of classical lymphatic vasculature in the CNS parenchyma limits the capacity for an immune response. Although this view is partially overturned by the recent discovery of the lymphatic-like hybrid vessels in the Schlemm’s canal in the eye and the lymphatic vasculature in the outmost layer of the meninges, the existence of lymphatic vessels in the CNS parenchyma has not been reported. Two potential mechanisms by which lymphatic vasculature may arise in the organs are: 1) sprouting and invasion of lymphatic vessels from the surrounding tissues into the parenchyma and 2) differentiation of blood endothelial cells into lymphatic endothelial cells in the parenchyma. Considering these mechanisms, we here discuss what causes the dearth of lymphatic vessels specifically in the CNS parenchyma.
1 Introduction
In mammals the circulatory system is composed of two independent and complementary vascular networks that cooperate to maintain the proper functions in peripheral tissues and the organism fluid balance: while the blood vascular system is responsible for the transport of essential nutrients, hormones, and oxygen, as well as removal of waste across the body via the blood circulation, the lymphatic vascular system is a unidirectional route required to drain the interstitial fluid (ISF) extravasated from the blood capillaries and return it to the blood circulation, contributing thereby to the maintenance of tissue homeostasis. Additional well-known functions of the lymphatic system are the dietary lipid uptake in the gut and the mediation of immune cell trafficking and antigen presentation in the lymph nodes in charge of immune surveillance (Oliver and Alitalo, 2005; Tammela and Alitalo, 2010; Oliver et al., 2020; Petrova and Koh, 2020). The study of the lymphatic system is a rapidly evolving field, and the knowledge about its tissue-specific features and functions has greatly advanced in recent years.
Due to its relevant role to maintain tissue homeostasis and immune surveillance, lymphatic vasculature is typically found in most of the vascularized tissues, with the notable exception of the bone marrow, cartilage, and the central nervous system (CNS): we should note that, although it was believed that the bone and bone marrow lack lymphatic vessels, very recent work has revealed the presence of lymphatic vessels in bone, supporting bone and hematopoietic cell regeneration (Biswas et al., 2023). The lack of classical lymphatic network in the brain and spinal cord parenchyma limiting the ability to induce an immune response to CNS-derived antigens was the main reason why the CNS has been considered an immune-privileged organ (Shirai, 1921; Murphy and Sturm, 1923; Medawar, 1948). This concept was revised in the last decade with the discovery of the lymphatic-like hybrid vessels in the Schlemm’s canal in the eye (Aspelund et al., 2014; Kizhatil et al., 2014; Park et al., 2014; Truong et al., 2014; Kim et al., 2017) and the meningeal lymphatics in the dura mater, the outermost layer of the three meninges located under the skull (Aspelund et al., 2015; Louveau et al., 2015).
An additional feature that makes CNS tissue a unique immune privileged site is the presence of a physical blood-brain barrier (BBB), a heavily restringing barrier that regulates the CNS homeostasis, protecting the brain parenchyma from the entry of pathogens, toxins, circulating immune cells, and other factors to maintain proper neuronal activity (reviewed in Zhao et al., 2015; Obermeier, Daneman, and Ransohoff, 2013; Daneman and Prat, 2015). It is well-characterized that the BBB is a multicellular structure where the brain microvasculature is embedded in a unique environment known as “neurovascular unit,” where endothelial cells (ECs) are in close association with numerous pericytes, astrocytes, microglia, neurons, vascular smooth muscle cells and extracellular matrix (ECM). Altogether they form an impermeable barrier that maintains brain homeostasis by regulating the trafficking of fluid and solutes in both directions and therefore minimizing the potentially toxic compounds. CNS ECs are highly specialized compared with ECs in other tissues’ vasculature. Their special attributes include the control of paracellular and transcellular passage pathways through the expression of continuous tight junction (TJ) proteins, and restricted transcytosis and fenestration to avoid non-specific transcellular transport (Hawkins and Davis, 2005; Abbott et al., 2010; Obermeier, Daneman, and Ransohoff, 2013; Daneman and Prat, 2015; Iadecola, 2017). These properties allow the strict regulation of the movement of molecules, ions and cells between the blood and the CNS. In addition, brain ECs express low levels of leukocyte adhesion molecules (LAMs), consequently limiting the number of immune cells that enter in the CNS. Consistently, it has been widely described that decreased expression of TJ proteins or enhanced transcytosis rate is linked to the BBB breakdown, which is associated with pathological inflammation in the brain tissue and many neurodegenerative diseases, including stroke, multiple sclerosis, Alzheimer’s disease, and Parkinson’s disease. While these disorders have their own triggers, all of them converge in similar modifications of the CNS vasculature, suggesting that the BBB disruption is a common hallmark in these disorders, culminating in neuronal dysfunction, neuroinflammation and neurodegeneration (Hawkins and Davis, 2005; Obermeier, Daneman, and Ransohoff, 2013; Daneman and Prat, 2015; Zhao et al., 2015).
In this review, we discuss the unique anatomical aspects of the CNS vasculature, which include the presence of highly specialized ECs that comprise the BBB and the absence of a proper lymphatic system inside the brain and spinal cord parenchyma. We will debate whether the lack of lymphatic vessels in the CNS is developed by preventing 1) the invasion of lymphatic vessels from surrounding tissues (i.e., meningeal layers) and/or 2) the differentiation of CNS blood ECs into LECs. Considering that CNS blood ECs are specialized cells to form the BBB, we discuss the hypothesis that the lack of lymphatic differentiation potential of CNS blood ECs is tightly linked to their BBB formation. With a special interest in the absence of Prospero Homeobox protein 1 (Prox1) in CNS ECs (Antila et al., 2017; Izen et al., 2018), the key transcriptional regulator of lymphatic differentiation (Sabin, 1902; Wigle and Oliver, 1999; Srinivasan et al., 2007), here we provide new insights into the possible relationship between these two immune-privileged features.
2 An overview of the lymphatic vascular development
The lymphatic vasculature is a thin-walled and blind-ended system that transports the fluids and other components extravasated from the blood circulation (called lymph) through the lymph nodes back to the bloodstream (Wigle and Oliver, 1999; Tammela and Alitalo, 2010). Hierarchically, highly permeable initial lymphatic vessels characterized by the presence of interconnected and discontinuous button-like junctions between the lymphatic endothelial cells (LECs), and the absence of continuous basement membrane and perivascular mural cells facilitate the uptake of the ISF through passive drainage (Baluk et al., 2007). This ISF released from the blood vasculature is then transported through the pre-collecting and then collecting lymphatics, in which LECs are tightly connected with continuous zipper-like junctions (Baluk et al., 2007) where the presence of specialized perivascular smooth muscle cells induce contractile activity that promotes the unidirectional lymph flow (Muthuchamy and Zawieja, 2008; Norrmen et al., 2009; Alitalo, 2011; Bazigou and Makinen, 2013; Sabine et al., 2016).
The formation of the lymphatic network in mice begins during embryonic development, after a primitive but functional circulatory system is established. The first LEC progenitors are detected during embryonic days (E) 9.5–10.0, when a subset of venous ECs located in the jugular region of the cardinal vein starts to express Prox1 (Sabin, 1902; Wigle and Oliver, 1999; Srinivasan et al., 2007). Prox1 expression is initiated by Sox18 and Nr2f2 (also known as COUP-TF II) in embryonic venous ECs (Francois et al., 2008; Srinivasan et al., 2010). Prox1 transcription factor is widely considered as the master regulator of lymphatic vascular development, because its expression in blood ECs (BECs) induces the commitment toward a LECs fate by inducing LEC-specific transcriptional program as well as the repression of BEC-specific genes (reviewed in Oliver and Alitalo, 2005; Oliver and Srinivasan, 2010). Prox1+ LEC progenitors bud off from the cardinal vein, sprout and migrate to form the initial lymphatic plexus in response to vascular endothelial growth factor C (VEGF-C) (Karkkainen et al., 2004), which binds to its receptor VEGF receptor 3 (VEGFR3) expressed by the LECs (Dumont et al., 1998; Makinen et al., 2001; Karkkainen et al., 2004). The identity of LECs is maintained by a feedback loop between Prox1 and VEGFR3: Prox1 activates the expression of VEGFR3, and VEGF-C/VEGFR3 signaling regulates Prox1 (Srinivasan et al., 2014). The process of expansion of the initial lymphatic vessels by sprouting from pre-existing VEGFR3+ lymphatic capillaries in response to the morphogen VEGF-C is known as lymphangiogenesis (Vaahtomeri et al., 2017). When the primary lymphatic plexus has been established during embryonic development, it continues maturing postnatally to give rise to a hierarchical network of blind-ended capillaries, pre-collecting and collecting lymphatic vessels, characterized by the transformation from zipper-like to button-like junctions and the recruitment of smooth muscle cells that cover the collecting vessels. The development of lymphatic valves secures the unidirectional lymph flow whereas the lymphovenous valves restrict the entrance of the blood into the lymphatic vasculature. LECs can be distinguished from BECs based on their unique molecular signature. In addition to Prox1 and VEGFR3, LECs are distinguished by the expression of lymphatic vessels endothelial hyaluronan receptor 1 (LYVE-1) and glycoprotein podoplanin (PDPN) (Oliver and Srinivasan, 2010).
For decades, the origin of the lymphatic vasculature has been extensively debated. Consistent with the centrifugal theory described by Sabin in 1902 (Sabin, 1902), the major contribution from the venous vasculature that gives rise to the lymphatic network through lymphangiogenesis (sprouting of pre-existing vessels) (Vaahtomeri et al., 2017) was corroborated both in mice and zebrafish with a series of lineage tracing experiments and high-resolution imaging, indicating that the process is highly conserved across vertebrates (Sabin, 1902; Huntington and McClure, 1910; Yaniv et al., 2006; Srinivasan et al., 2007). Moreover, the most recent lineage tracing studies using the paraxial mesoderm specific Pax3-Cre and Myf5-Cre drivers demonstrated that the majority of LECs originate from the paraxial mesoderm-derived endothelium of the cardinal vein (Stone and Stainier, 2019). However, additional findings in recent years demonstrated that, while there is no doubt that the main source of lymphatics derives from the cardinal and intersomitic veins, further contribution from non-venous-derived progenitors, as described by McClure in the centripetal theory (Huntington and McClure, 1910) was found in specific tissues, in a process called lymphvasculogenesis (formation de novo of LECs). Examples of these non-venous origins are described as the contribution of local dermal blood capillary plexus to dermal lymphatics vasculature (Martinez-Corral et al., 2015; Pichol-Thievend et al., 2018), the contribution of second heart field cells and yolk sac hematopoietic derivatives to cardiac lymphatic vasculature (Klotz et al., 2015; Maruyama et al., 2019; Lioux et al., 2020) and the contribution of c-kit+ hemogenic endothelium to mesenteric lymphatics (Stanczuk et al., 2015). In a similar fashion, studies in zebrafish using lineage-tracing and live imaging techniques reinforced the idea that LECs emerge from mixed venous and non-venous origins (Yaniv et al., 2006; Nicenboim et al., 2015; Eng et al., 2019). However, the roles of lymphatics from different sources in organ development and diseases are currently unclear.
3 The CNS is an immune privileged organ… devoid of lymphatic vasculature?
Whilst the lymphatic system is present in most of the vascularized tissues in the body, the CNS comprises one of the few exceptions lacking lymphatic vessels within the brain and spinal cord parenchyma. Indeed, whole-mount imaging of Prox1-BAC-GFP mouse tissues (Choi et al., 2011) allows the direct identification of Prox1-expressing lymphatic vasculature in vivo. We here show a representative sagittal section image of Prox1-BAC-GFP mouse brain (Choi et al., 2011) at postnatal day 3 (P3) to illustrate the presence of lymphatic vessels (PECAM1+ Prox1-GFP+ LYVE-1+) in the skin but not inside the brain parenchyma (Figure 1). This feature in combination with the presence of a restrictive BBB provides an extremely unique environment for the immune cells, where immune surveillance is not achieved under physiological conditions.
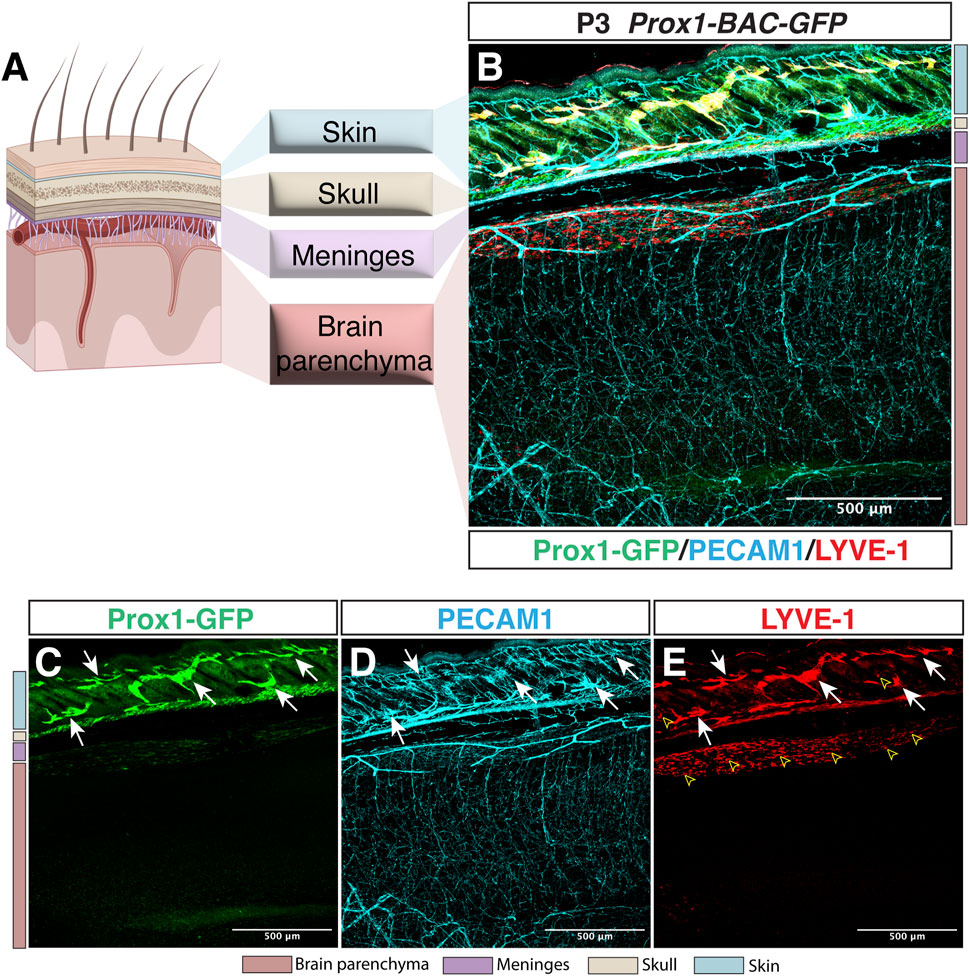
FIGURE 1. Lymphatic avascularity in the brain parenchyma. (A) Schematic representation of the brain, meningeal layers, skull bone, and skin on the left panel (Partially created with BioRender.com). (B–E) Whole-mount immunostaining of Prox1-BAC-GFP brain at postnatal day 3 (P3) is shown. Prox1-GFP labels lymphatic vessels in the skin [(B,C) green, arrows] as well as a subset of neural progenitors (not shown) in the brain parenchyma. All the vasculature is labeled with PECAM-1 [(B,D) cyan]. LYVE-1 labels lymphatic vessels [(B,E) red, arrows] and macrophages (open arrowheads) in the skin as well as the pia surface of the brain. Note that no lymphatic vessels are found in the brain parenchyma. Scale bar 500 μm.
During the early 20th century many experiments were performed transplanting grafts from a variety of tissues into anatomically unnatural sites in the body from the same or different species. Whereas in most of the cases the rejection from the host to the alien graft was observed, in some cases they could grow and survive, giving rise to the identification of “immunologically privileged” organs, including the anterior chamber of the eye, the cornea or the brain. The first evidence provided by Ebeling and Roffo is that allografts of a particular carcinoma were more successful when transplanted in the brain rather in the subcutaneous space using mice and rats, respectively (reviewed in Barker and Billingham, 1977). Xenografted rat sarcoma in the mouse brain could grow, whereas it was destroyed when placed subcutaneously or intramuscularly (Shirai, 1921). According to the observation that animals deprived of lymphoid tissue failed to destroy foreign tissue grafts, the brain could be an uncongenial environment for these immune cells, which in peripheral tissues were able to invade and destroy the tumors (Murphy and Sturm, 1923). Lastly, critical experiments carried out by Medawar described that, once primed peripherally, immune cells could induce an accelerated rejection against the foreign tissue, despite the brain’s status an immune-privileged site unable to induce an efficient adaptive immune response due to the lack of lymphatic drainage in this tissue (Medawar, 1948). Whereas in non-immunized animals the brain was unable to induce an immune response against the skin graft, in those animals where the skin was first grafted peripherally and then transplanted into the brain, a severe rejection was observed. These results showed that once peripherally activated, immune cells could migrate and enter the brain parenchyma, but the brain itself could not elicit such an immune response (Medawar, 1948).
Based on these transplant experiments, the eye and brain have been long considered immunologically unique and devoid of lymphatic vasculature; however this notion has been revised in the last decade due to the discovery of blood-lymphatic hybrid vessels in the Schlemm’s canal in the eye (Kizhatil et al., 2014; Park et al., 2014; Truong et al., 2014; Aspelund et al., 2015; Kim et al., 2017), the presence of classical lymphatic vessels in the meningeal dura mater of the brain (Aspelund et al., 2015; Louveau et al., 2015), and non-lumenized LECs in the surface of the brain in zebrafish and leptomeninges in mammals (Bower et al., 2017; van Lessen et al., 2017; Venero Galanternik et al., 2017; Shibata-Germanos et al., 2020). Although it is still maintained by the lack of classical lymphatic network inside the brain and spinal cord parenchyma, the concept of “immune privileged site” in the CNS has been recently redefined with these new insights (Figure 2).
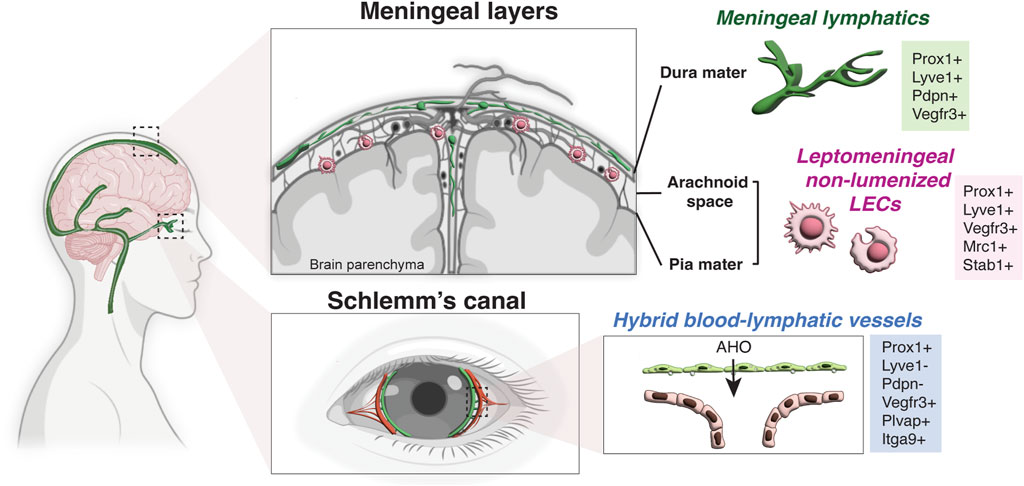
FIGURE 2. Lymphatic endothelial cells in the CNS. Schematic representation of a human brain showing the lymphatic endothelial cells in the meningeal layers and Schlemm’s canal. While there is no lymphatic vasculature in the brain parenchyma, meningeal lymphatic vessels are found in the dura mater under the skull and non-lumenized mural LECs are found in the leptomeninges (the arachnoid space and the pia mater). Meningeal lymphatics express the classical hallmark of lymphatic vessels (Prox1+ LYVE-1+ PDPN+ VEGFR3+), while non-lumenized mural LECs have macrophage-like shape and express the mannose receptor Mrc1 and Stab1. Note that Schlemm’s canal in the eye has hybrid blood-lymphatic vessels, expressing the lymphatic marker Prox1 but not LYVE-1 and PDPN. The hybrid vessels also express high level of VEGFR3, PLVAP and Itgα9. AHO, aqueous humor outflow (Partially created with BioRender.com).
3.1 Lymphatic-like vessels in the Schlemm’s canal in the eye
The Schlemm’s canal (SC) is a specialized ring-shaped vasculature at the periphery of the cornea which drains the aqueous humor outflow (AHO) from the intraocular chamber and delivers it back to the venous circulation to maintain fluid homeostasis in the eye. Malfunction in this drainage where the AHO is obstructed is frequently correlated with the development of glaucoma in humans. ECs lining the inner wall of the SC share morphologic and functional similarities with both BECs and LECs (Table 1). In addition to its vascular-derived origin, SC is a secondary structure that develops postnatally. Its ECs form a continuous monolayer lying on a discontinuous basement membrane, lack pericyte coverage, and establish a blind-ended tube that transports AHO and antigen-presenting cells in basal-to-apical flow to the systemic circulation. Altogether this special vasculature was considered more analogous to LECs rather than BECs, although initial studies did not examine the expression of LEC markers (reviewed in Ramos et al., 2007). The discovery of Prox1 expression in the SC ECs by different groups almost simultaneously was the milestone to consider this structure as hybrid vessels sharing blood and lymphatic properties but with lymphatic-like functions (Aspelund et al., 2014; Kizhatil et al., 2014; Park et al., 2014; Truong et al., 2014).
Despite the SC ECs expressing the lymphatic master regulator Prox1, they can be distinguished from classical lymphatic vasculature by the lack of other LEC markers such as LYVE-1 or PDPN (Aspelund et al., 2014; Truong et al., 2014) (Table 1), suggesting a partial lymphatic reprogramming. In a similar line of evidence, Sox18, the abovementioned upstream regulator of Prox1, was not detected in the SC ECs (Park et al., 2014). Moreover, SC ECs bud from the choroidal veins at postnatal day P1, and after the primordial SC is formed they upregulate the lymphatic master regulator Prox1 to acquire the hybrid lymphatic-blood phenotype (Park et al., 2014): SC expresses a mixture of BEC and LEC markers including the pan-endothelial platelet and endothelial cell adhesion molecule 1 (PECAM-1), the venous marker endomucin (EMCN), the chemokine (C-C motif) ligand 21 (CCL21), and VEGFR3, in addition to Prox1. They also express the lymphatic valve markers integrin alpha 9 (Itgα9) and Forkhead Box C2 protein (Foxc2), even though they do not have luminal valves (Aspelund et al., 2014; Park et al., 2014). Interestingly, abundant plasmalemma vesicle–associated protein (PLVAP), a marker for endothelial transcytosis and permeability, was also detected in the ECs of the inner SC wall (Figure 2; Table 1).
What controls the expression of Prox1 that is important for the SC integrity and functionality? Park et al. revealed that the shear stress-responsive transcription factor Klf4 might be an upstream regulator of Prox1 in SC ECs by directly binding to the first intron of the Prox1 gene. Likewise, a mouse ocular puncture model was used to confirm in vivo the requirement of flow to maintain the proper expression of Prox1. Indeed, reduction in AHO simultaneously downregulated Prox1 and Klf4 expression compared with control eyes (Park et al., 2014), proposing Prox1 as an accurate biosensor for SC functionality, which might be used for the early detection and prevention of glaucoma in humans.
Angiopoietin/Tie2 (Angpt/Tie2) signaling is required for the correct postnatal development and adult maintenance of the SC hybrid vessels to sustain the normal AHO (Thomson et al., 2014; Kim et al., 2017). Postnatal deletion of Angpt1/Angpt2 or Tie2 results in primary congenital glaucoma in mice due to increased intraocular pressure (Thomson et al., 2014). Meanwhile, Tie2-activating antibody (ABTAA) rescues the phenotype of glaucoma in double Angpt1/Angpt2-deficient mice (Kim et al., 2017), raising the possibility that this signaling pathway could be an interesting therapeutic target for the treatment of glaucoma.
3.2 The discovery of meningeal lymphatic vessels surrounding the brain
The surface of the brain is covered by three layers of meninges that comprise a superficial barrier between the cerebrospinal fluid (CSF) and the CNS: the dura mater is the outermost layer in contact with the skull bone, containing arteries, veins and fenestrated capillaries that do not form BBB; the arachnoid mater establishes a barrier between the dura and the subarachnoid space (SAS), which contains the CSF and resident immune cells; the pia mater is a monolayer of cells that covers the CNS separating it from the perivascular compartment (Engelhardt, Vajkoczy, and Weller, 2017). Given that no lymphatic vasculature exists in the CNS parenchyma (Figures 1, 2), the perivascular spaces of cerebral blood vessels have been proposed to serve as a pathway for the exchange of CSF and interstitial fluid to clear waste products (Iliff et al., 2012; Nedergaard, 2013). The recent identification of meningeal lymphatic vessels established another pathway for CSF outflow into deep cervical lymph nodes.
An extensive network of Prox1+ lymphatic vasculature was found in the dura mater (Figure 2), expressing hallmarks of classical LECs (PECAM-1, LYVE-1, PDPN, VEGFR3, CCL21) (Aspelund et al., 2015; Louveau et al., 2015; Antila et al., 2017; Izen et al., 2018; Ahn et al., 2019) (Figure 2; Table 1). Although the first evidence of the existence of lymphatics in the human dura mater was already described by Paolo Mascagni in 1787 (reviewed in Sandrone et al., 2019), due to their difficult location it is not until recently that the use of state-of-the-art confocal imaging and genetic models allowed a precise characterization and functional description of this lymphatic network surrounding the brain parenchyma.
In mice, multiple groups including ours, carried out an exhaustive examination of the meningeal lymphatic formation during postnatal and adult stages using Prox1-BAC-GFP mice (Antila et al., 2017; Izen et al., 2018). Whereas most lymphatic vessels in the peripheral tissues develop during embryonic stages, meningeal lymphatics are formed during the first postnatal month, appearing first at the base of the skull (Antila et al., 2017; Izen et al., 2018), as it was also reported in the Schlemm’s canal (Aspelund et al., 2014) and lacteal vessels in the gut (Kim, Sung, and Koh, 2007). Like classical lymphatic vessel development, meningeal lymphatics require Prox1 and VEGF-C/VEGFR3 signaling for their proper formation and maintenance during adult stages (Absinta et al., 2017). Indeed, blockade of this signaling impairs meningeal lymphatic development, while boosting VEGF-C induces meningeal lymphangiogenesis, suggesting promising regenerative potential (Aspelund et al., 2015; Antila et al., 2017; Hsu et al., 2019; Patel et al., 2019; Bolte et al., 2020; Song et al., 2020; Yanev et al., 2020). Nevertheless, a recent work showed that mice lacking Plcγ2 have impaired structural remodeling and maturation of meningeal lymphatics, accompanied with reduced lymph flow, suggesting that meningeal lymphatic formation might not be only dependent on the VEGF-C/VEGFR3 signaling axis (Balint et al., 2019a). Intriguingly, after the re-discovery of the lymphatic vasculature in the dura mater in mice (Aspelund et al., 2015; Louveau et al., 2015), meningeal vessels were carefully examined in humans (Absinta et al., 2017; Eide et al., 2018; Jani and Sekula, 2018; Shibata-Germanos et al., 2020; Jacob et al., 2022), non-human primates (Absinta et al., 2017), and zebrafish (Castranova et al., 2021). Meningeal lymphatic vasculature that wraps the spinal cord also develops postnatally, through VEGF-C/VEGFR3 signaling (Antila et al., 2017; Louveau et al., 2018; Jacob et al., 2019).
The presence of immune cells in the meningeal vessels under the steady state suggests their involvement in immune cell trafficking and immunosurveillance of the CNS (Louveau et al., 2015). Recent studies elegantly demonstrated the skull and vertebrae bones as new bone marrow niches for hematopoiesis supplying blood-borne myeloid cells (including monocytes, neutrophils, and macrophages) and B cells to the dura mater (Brioschi et al., 2021; Cugurra et al., 2021; Schafflick et al., 2021). These cells transit from the bone to the meninges through specialized vascular connections, challenging the previous accepted idea that meningeal adaptative immunity originates from the systemic circulation.
High-resolution imaging with the injection of fluorescent tracers into the brain parenchyma or the CSF in the cisterna magna clearly demonstrated that lymphatic vessels in the dura mater play an essential role in the clearance of macromolecules and immune cells from the CSF within the SAS into the deep cervical lymph nodes (dcLNs) (Aspelund et al., 2015; Louveau et al., 2015; Absinta et al., 2017; Da Mesquita et al., 2018; Louveau et al., 2018; Ahn et al., 2019; Balint et al., 2019b; Bolte et al., 2020; Chen et al., 2020). Interestingly, there are anatomical differences in meningeal vasculature between the dorsal and basal part of the skull. While dorsal meningeal lymphatics mainly present zipper-like junctional pattern of LECs, in basal vessels the LECs are loosely joined by intermittent button-like junctions, which allow them a better uptake of the CSF macromolecules and subsequent drainage outside the brain (Ahn et al., 2019). Altogether, these recent findings about key roles of meningeal lymphatics in the CNS clearance and immunosurveillance under physiological conditions generated substantial excitement in medical research due to their potential contributions in a variety of pathological models, including autoimmune diseases, cerebrovascular injury, brain tumors or age-related neurological diseases, which implied promising avenues for the therapeutic treatment of cerebrovascular injuries and age-related neurodegenerative diseases.
Extraordinary work by Jacob et al. showed a similar anatomy in the meningeal lymphatic vasculature between mice and humans (Jacob et al., 2022). 3D characterization with unprecedented resolution was performed combining the imaging of intact whole head in mice and improved non-invasive magnetic resonance imaging (MRI) in humans, filling gaps in the knowledge about the vascular connections between the meninges and the collecting lymph nodes. More importantly, the innovative use of MRI combining elliptic venography, T1 SPACE and DANTE sequences provided enough resolution to compare the meningeal lymphatic network in human patients and identify morphological anomalies. As an example, the authors examined 11 patients with neurovascular diseases and identified that only those with Gorham-Stout disease had significantly altered meningeal lymphatics. Thus, this work provides new insights in the detection of meningeal alterations between individuals with neuropathological conditions (Jacob et al., 2022).
3.3 Non-lumenized LECs in the surface of zebrafish brain and mammalian leptomeninges: Potential roles in neurological diseases and regeneration
Recently, multiple groups described the presence of a distinctive LEC population in the surface of the brain (Figure 2). These LECs appear to be unusual isolated perivascular cells with a macrophage-like morphology, and they do not form lumenized tubes under physiological conditions, although they express Prox1 and exhibit features of LECs due to their molecular markers (LYVE-1, VEGFR3) and their venous derived origin, which is dependent on VEGF-C/VEGFR3 signaling (Bower et al., 2017; van Lessen et al., 2017; Venero Galanternik et al., 2017; Castranova et al., 2021).
Initially identified in the zebrafish model, brain perivascular LECs are known as mural LECs (muLECs) (Bower et al., 2017), brain LECs (bLECs) (van Lessen et al., 2017) or fluorescent granular perithelial cells (FGPs) (Venero Galanternik et al., 2017). Interestingly, an analogous population was also found in the mouse and human leptomeninges, the brain-associated meningeal layers that includes the arachnoid mater and pia mater, which is why they were termed leptomeningeal LECs (LLECs) (Shibata-Germanos et al., 2020). In mammals, FGPs or “Mato Cells” were previously characterized as perivascular macrophages with a bone marrow-derived origin (Mato and Ookawara, 1981; Audoy-Remus et al., 2008; Faraco et al., 2016) due to the expression of the Mannose Receptor 1 (Mrc1) and their scavenger potential, which was thought to protect the brain from toxic waste products by phagocytosis and pinocytosis (reviewed in Suarez and Schulte-Merker, 2021). Nevertheless, studies with extensive live imaging and sophisticated lineage tracing discard the pericytes-, neural crest- or hematopoietic-derived origins, demonstrating instead that this unique population derives from pre-existing venous vessels in the CNS (Bower et al., 2017; van Lessen et al., 2017; Shibata-Germanos et al., 2020), which sprout to cover the surface of the brain before acquiring their mesenchymal/perivascular morphology (Bower et al., 2017). In a similar fashion, genetic deletion of the myelopoietic lineage in PU.1 mutants did not affect the formation of these muLECs/bLECs/FGPs/LLECs (van Lessen et al., 2017; Shibata-Germanos et al., 2020), but the loss of Ccbe1, a VEGF-C-activating protease, gave rise to abnormal sprouting and asymmetrical distribution of these cells on the surface of the brain (van Lessen et al., 2017), clearly demonstrating their lymphatic origin and dependence on VEGF-C/VEGFR3 signaling.
A key hallmark of perivascular LECs is the presence of large cytoplasmic vesicles and the expression of scavenger receptors Mrc1 and Stab1 (Table 1), suggesting their endocytic role to uptake and clear waste products that enter into the interstitial space for degradation. Previous studies with tracer injections in the parenchyma or CSF in mouse and zebrafish showed the ability of perivascular LECs to actively take up polysaccharides and glycoproteins with at least 150 KDa size and internalize them through endocytosis with higher efficiency than macrophages or microglia, whereas lower efficiency was proved in the uptake of molecules higher than 500 KDa (Venero Galanternik et al., 2017; Shibata-Germanos et al., 2020; Huisman et al., 2022). Such endocytic capacity quickly raised the interest in examining whether they could play a relevant role in the surveillance and clearance of Aβ peptides in the brain, the toxic driver of Alzheimer Disease’s progression. Real-time in vivo analysis in zebrafish allows the investigation of the clearance capacity of Amyloid-β isoform 42 (Aβ1–42). Remarkably, Jeong et al. (2021) demonstrated that the dynamic uptake of Aβ42 depends on its aggregation status, where Aβ1-42 monomers are selectively internalized and cleared by perivascular LECs but not Aβ1–42 oligomers. The discovery of these isolated LECs in the surface of the brain involved in the homeostasis and uptake of Aβ peptides provides new therapeutic avenues in AD, where the enhancement of their function in combination with the reduction of Aβ aggregates might contribute to new approaches to prevent their accumulation. Similarly, Amyloid β1–40 clearance by LLECs was shown in mice, illustrating their conserved functions in vertebrates (Shibata-Germanos et al., 2020).
Under physiological conditions, muLECs/bLECs/FGPs/LLECs stay as loose single cells without forming lumenized lymphatic vessels; however, elegant studies by Chen et al. (2019) described sticking roles for perivascular LECs in resolving tissue damage caused by vascular dysfunction in the zebrafish brain. Using a model of brain cerebrovascular injury in zebrafish, the authors demonstrated that some perivascular LECs could form lymphatic tubes that penetrated the brain parenchyma to drain the ISF and resolve the edema. Although such transient invasion of lymphatic vessels has not been reported in mammals, it demonstrates a potential role of perivascular LECs in brain regeneration in the zebrafish model.
4 Potential mechanisms of CNS lymphatic avascularity
The continuous emerging of clearing tissue protocols in combination with the use of 3D imaging from intact tissues with cell-specific markers is revolutionizing the identification of lymphatic vasculature in tissues that were long considered devoid of them, including the brain and spinal cord in the CNS, the eye, and the bone. The most recent example was the sighting of lymphatic vessels inside the bone in mice and humans (Biswas et al., 2023). However, in the CNS, despite the finding of lymphatic vessels and non-lumenized LECs in the meningeal layers surrounding the surface of the brain (dura mater and leptomeninges, respectively), there is no evidence of the presence of classical lymphatic vasculature inside the brain parenchyma in mammals under physiological conditions.
Potential mechanisms that could promote the appearance of lymphatic vessels in the different organs are 1) LEC sprouting and invasion of pre-existing lymphatic vessels (lymphangiogenesis) in response to lymphangiogenic signaling such as VEGF-C/VEGFR3, or 2) differentiation of BECs or non-ECs into LECs (lymphvasculogenesis), which requires the expression of the lymphatic transcriptional regulator Prox1. We discuss how the CNS develops lymphatic avascularity by preventing LEC invasion and differentiation.
4.1 LEC invasion into the CNS parenchyma in the zebrafish vasculature model
Multiple reports have suggested the boosting of VEGF-C signaling as a promising therapeutic approach to enhance meningeal lymphangiogenesis to halt pathologies like edemas, or toxic peptides accumulation (Aspelund et al., 2015; Antila et al., 2017; Hsu et al., 2019; Patel et al., 2019; Bolte et al., 2020; Song et al., 2020; Yanev et al., 2020). Nevertheless, lymphatic vessels or non-lumenized LECs have not been observed inside the brain and spinal cord parenchyma in mammals under steady-state or disordered conditions. Still the molecular cues that might prevent the CNS environment from the invasion of meningeal lymphatics remain unelucidated. Similarly, an extensive population of LYVE-1+ macrophages are found in the borders of the brain, but never inside the parenchyma (Figure 1E). LYVE-1 is a well-established receptor for hyaluronan (HA), a key component of the extracellular matrix in many tissues (Banerji et al., 1999). Considering the recent studies that LYVE-1+ cells are associated with HA-enriched regions in the mammary gland (Wang et al., 2020a), HA accumulation in the boundaries of the brain and spinal cord may function as a physical barrier to prevent the invasion of LYVE-1-expressing cells. Further studies would be required to elucidate such a mechanism underlying lack of LYVE-1-expressing cells in the CNS parenchyma.
In addition to the apparent incapability of meningeal lymphatics to penetrate the brain parenchyma in mammals, a transient lymphatic invasion of the abovementioned non-lumenized LECs in response to cerebrovascular injury was described in zebrafish model. By using NTR-Mtz system and photochemical thrombosis to induce brain vascular injury, Chen et al. (2019) proved the capability of LECs located in the borders of zebrafish brain to activate the ingrowth of LECs upon cerebrovascular injury, dependent on VEGF-C/VEGFR3 signaling, and penetrate the injured brain parenchyma to drain the ISF and resolve brain edema. In this work, the authors classified the ingrowth lymphatic vessels (iLVs) in two different subpopulations depending on their functions: “stand-alone iLVs” and “track iLVs.” The stand-alone iLVs become lumenized lymphatics to drain the ISF and resolve the edema in the injured brain parenchyma whereas the other iLVs transdifferentiate into blood vessels (iLVs-to-BVs transdifferentiation), giving rise to early-formed blood vessels in the ischemic area. The track iLVs serve as a migratory scaffold (or “growing tracks”) to guide and support the growth of late-generated blood vessels necessary to promote the functional recovery in the damaged tissue without inducing uncontrolled vessel growth (Chen et al., 2019; Chen et al. 2021; Chen et al., 2022). Mechanistically, iLV-to-BV transdifferentiation exclusively occurs in stand-alone iLVs through activation of Notch signaling (Chen et al., 2019). These new early formed blood vessels are covered by pericytes and become functional with blood flow within 2 days after injury. By contrast, track iLVs are unable to transdifferentiate since Notch signaling is suppressed. Surrounding blood vessels express ephrinB2a, activating the expression of EphB4 in the track iLVs, which in turn promotes Notch inhibition (Chen et al., 2021). Moreover, the authors described that the formation of new vessels guided by the “growing tracks” is regulated by CXCL12/CXCR4 signaling axis (Chen et al., 2022). Whilst the chemokine receptor Cxcr4a is transcriptionally activated in track iLVs after cerebrovascular damage, its ligand Cxcl12b is expressed by the surrounding blood vessels, guiding the directionality of iLV ingrowth. By using Cxcr4a and Cxcl12b mutants, the authors highlighted the critical role of CXCL12/CXCR4 signaling in controlling the appropriate directionality and vessel patterning during cerebrovascular regeneration, avoiding increased branching and uncontrolled formation of blood vessels (Chen et al., 2022). This is a transient invasion of lymphatic vessels inside the brain parenchyma, in that stand-alone iLVs gradually disappear after the completion of cerebrovascular regeneration by apoptosis, maintaining the lymphatic avascular status inside the brain parenchyma once the brain tissue is fully recovered (Chen et al., 2021).
4.2 No lymphatic differentiation in the CNS vasculature
The transcription factor Prox1 is involved in many developmental processes including neurogenesis and tissue development such as heart, eye lens, liver, and pancreas. In the vascular system, it serves as the “master regulator” that controls the switch from BEC into LEC fate. During the last two decades, a series of pioneering studies showed that Prox1 is required for the acquisition and maintenance of the lymphatic phenotype (Wigle and Oliver, 1999; Hong et al., 2002; Petrova et al., 2002; Johnson et al., 2008; Kim et al., 2010; Kim et al., 2013). The expression of Prox1 in the LEC progenitors that bud off from the cardinal vein is necessary not only to activate the expression of LEC-specific genes, but also to repress BEC-specific transcriptional programs (Johnson et al., 2008). Numerous Prox1 loss-of-function and gain-of-function studies clearly demonstrated the malleability of ECs to acquire BEC or LEC fate. In vivo and in vitro assays clearly showed that Prox1 is necessary and sufficient to induce the lymphatic phenotype and repress the BEC program (Wigle and Oliver, 1999; Hong et al., 2002; Petrova et al., 2002; Francois et al., 2008; Johnson et al., 2008). Likewise, in the absence of this transcription factor the suppression of the BEC program is not achieved and ECs lose their lymphatic identity (Johnson et al., 2008; Kim et al., 2010; Kim et al., 2013).
Considering the lack of Prox1 expression in the CNS vasculature, in this section we discuss the hypothesis that Prox1 expression might be strictly controlled in the CNS ECs to prevent LEC differentiation and preserve the BBB properties. Here we provide a general overview about regulatory mechanisms of Prox1 expression in the lymphatic system, and we debate potential mechanisms that might regulate Prox1 expression in the CNS vasculature, with a particular focus on Wnt/β-catenin signaling required for the BBB development and maintenance.
4.2.1 Prox1 regulation in LEC development
Diverse transcriptional and epigenetic regulators have been proposed to control Prox1 expression in the vascular system (summarized in Figure 3). The initiation of Prox1 expression in the LEC progenitors requires the expression of Sox18 and Nr2f2 transcription factors (Francois et al., 2008; Srinivasan et al., 2010). While Sox18 is only needed for the initial specification of LECs (Francois et al., 2008), Nr2f2 is critical for the venous specification, which is required for the subsequent lymphatic specification and migration of these progenitors away from the cardinal vein (Srinivasan et al., 2007; Srinivasan et al., 2010), but not for the maintenance of the lymphatic fate once the lymphatic system has been established. A binding motif for Nr2f2 was found in the −9.5 kb upstream open reading frame of the Prox1 gene, whereas two different binding sites have been described for Sox18 (−1.1 and −0.8 kb, respectively, Figure 4). Epigenetically, the histone H3K79 methyltransferase DOT1L controls Sox18 transcriptional regulation, which subsequently regulates Prox1 expression, as well as other lymphatic essential genes such as Foxc2 and VEGFR3, by inducing chromatin opening that promotes their transcriptional activation (Yoo et al., 2020). Mafb is another upstream regulator of the lymphatic differentiation factors Prox1, Sox18 and Nr2f2 during embryonic and postnatal development (Dieterich et al., 2015). By directly binding to the first intron in the Prox1 gene, the combination Mafb and Prox1 generates a positive feedback loop in which the increased expression of Prox1 also maintains the expression of Mafb. In a similar line of work, another well-known positive feedback loop in the lymphatic vasculature is the Prox1/VEGF-C/VEGFR3 axis. The Prox1-VEGFR3 autoregulatory loop is critical for controlling the number of specified LEC progenitors budding from the cardinal vein in response to VEGF-C and the subsequent maintenance of the lymphatic identity (Srinivasan et al., 2014). Furthermore, the hematopoietically expressed homeobox (HHEX) transcription factor was recently found as an upstream regulator of Prox1 as well as VEGF-C and FLT4 during lymphatic development. Indeed, HHEX binds to the −0.8 kb upstream of the Prox1 transcriptional start site (Gauvrit et al., 2018) (Figures 3, 4).
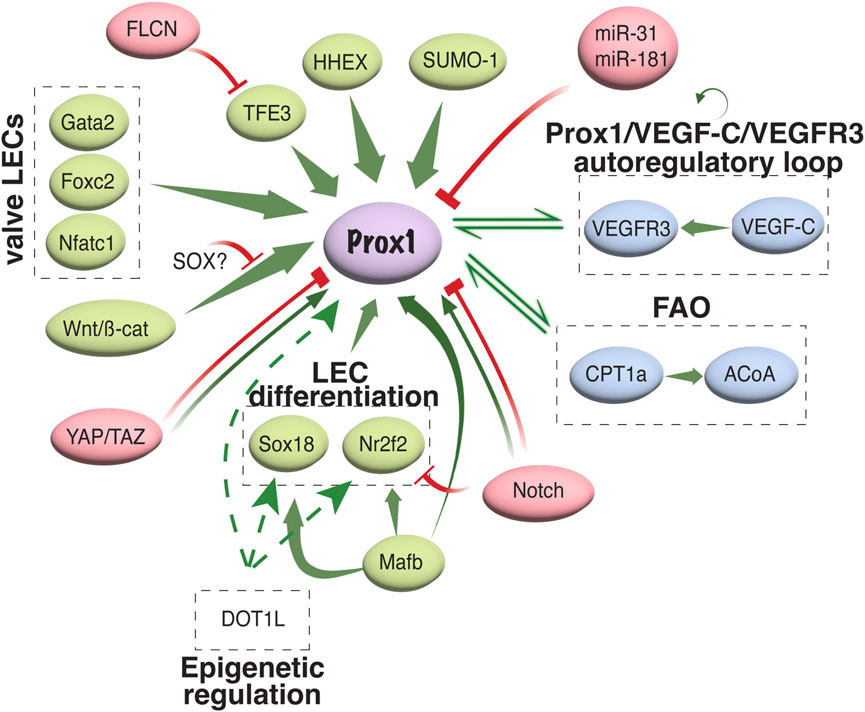
FIGURE 3. Transcriptional and epigenetic regulation of Prox1 in the vascular system. Schematic representation of different activators/repressors of Prox1 expression in the vascular system is shown. FAO, fatty acid oxidation; ACoA, acetyl coenzyme A.
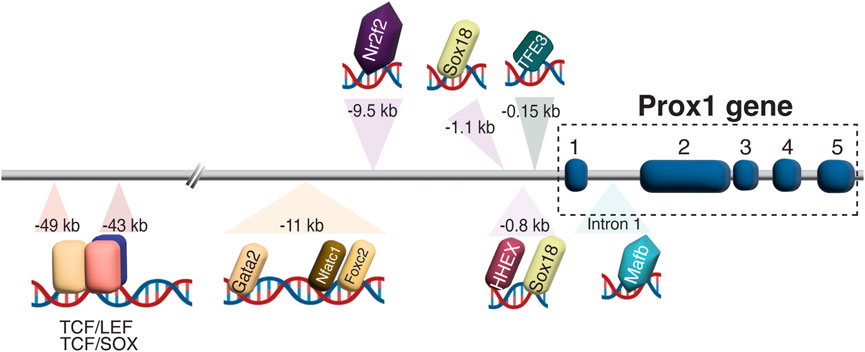
FIGURE 4. Prox1 regulation in LEC development. Schematic representation of biding motifs for multiple transcriptions factors and regulators in the upstream of the Prox1 gene is shown. In the −43/−49 kb region, TCF:LEF and TCF:SOX overlapping binding sites are found, suggesting a possible competition between LEF and SOX factors to bind to the same motif.
An interesting regulatory region including the binding sites of Gata2, Foxc2, Nfatc1, and Prox1 itself was identified in the −11 kb upstream of the Prox1 transcriptional start site. Oscillatory shear stress from the lymph flow upregulates the levels of these factors, which therefore binds to the −11 kb enhancer region to increase its expression in the valvular LECs (Kazenwadel et al., 2015). Genome editing of the GATA-binding site in this enhancer element abolished its activity and impaired lymphatic vascular development, demonstrating the crucial role of GATA2 in activating the Prox1 −11 kb regulatory element (Kazenwadel et al., 2023). Moreover, the authors revealed that mutants with a reduced expression of Prox1 showed an enhanced hemogenic capacity, submitting the first evidence of lymphatic endothelium as a source of hematopoietic cells, and suggesting the role of Prox1 in repressing this property (Kazenwadel et al., 2023). Wnt/β-catenin signaling also interacts with Prox1 in a feedback loop to induce the expression of Gata2 and Foxc2 in the valvular ECs (Cha et al., 2016). Folliculin (FLCN) suppresses the lymphatic specification in venous ECs through the degradation of TFE3 transcription factor, which binds to the −0.15 kb upstream of the Prox1 transcription start site, and therefore prevents the expression of the lymphatic master regulator: lack of folliculin allows TFE3 to translocate into the nucleus and upregulate Prox1 expression by binding to its promoter in venous ECs (Tai-Nagara et al., 2020) (Figures 3, 4).
Suppression of Prox1 expression has been proposed to control the balance between LEC and BEC specification. In vitro studies suggest that miR-31 inhibits lymphatic fate by downregulating Prox1 expression (Pedrioli et al., 2010). Similarly, miR-181 downregulates Prox1 expression by binding to its 3-UTR region, which leads to its translational inhibition and transcript degradation (Kazenwadel, Michael, and Harvey, 2010). Notch signaling functions as a negative regulator of the Nr2f2/Prox1 transcriptional axis to block lymphatic specification (Kang et al., 2010; Zheng et al., 2011; Murtomaki et al., 2013; Choi et al., 2017). By contrast, opposite results were found in zebrafish and postnatal mice, where Dll4/Notch signaling is required for the correct lymphatic development (Geudens et al., 2010; Niessen et al., 2011). These contrary roles of Notch signaling in regulating LEC fate could be context-dependent to maintain the precise balance between BEC and LEC specification. In a similar line of research, YAP/TAZ signaling functions as a positive regulator or inhibitor of Prox1 expression in the different studies (Cho et al., 2019; Cha et al., 2020). Whereas Cho et al. (2019) found that YAP and TAZ act as important negative regulators of Prox1 during lymphatic remodeling and postnatal lymphatic valve morphogenesis, Cha et al. (2020) described thar YAP/TAZ signaling was required to maintain the valvular ECs and experiments in vitro demonstrated that YAP/TAZ positively regulates Prox1 in primary human LECs (HLECs). The discrepancies found between both studies might be due to the differences in the genetic models used, nevertheless both groups obtained the same results that the hyperactivation of YAP/TAZ signaling results in the downregulation of Prox1 in vitro. These results suggested that a proper level of YAP and TAZ is required to maintain the expression of Prox1 in LECs (Figure 3).
Post-translational regulations of Prox1 influence the transcriptional response (reviewed in Bui and Hong, 2020). Several lysine sites on Prox1 can be modified by small ubiquitin-related modifier (SUMO) (Shan et al., 2008). Sumoylation modulates Prox1 DNA binding to their target genes, enhancing the transcriptional activation of downstream lymphatic-related genes (Pan et al., 2009). In cancer cells, the Ser79 phosphorylation of Prox1 by AMP-activated protein kinase promotes Prox1 degradation through the recruitment of the CUL4-DDB1 E3 ubiquitin ligase complex (Wang et al., 2022). Despite the importance in post-translational regulations to modulate Prox1 transcriptional activity, how different modifications of Prox1 in response to distinct upstream signals regulate LEC development and maintenance remain elusive.
Lastly, novel findings spotlighted the involvement of metabolism in the regulation of Prox1 and lymphangiogenesis. A beautiful work by Wong et al. described a role of fatty acid β-oxidation (FAO) in LEC differentiation. Prox1-mediated transcriptional upregulation of carnitine palmitoyltransferase 1a (CPT1a) promotes mitochondrial-dependent FAO which increases the level of acetyl coenzyme A (ACoA). The ACoA is utilized by Prox1/p300 complex to promote the histone acetylation of key lymphangiogenic genes, including Prox1 itself. Therefore, the upregulation of CPT1a by Prox1 is also a positive feedback loop to maintain Prox1 expression active in LECs (Wong et al., 2017). Moreover, recent exciting study by Ma et al. demonstrated that mitochondrial complex III is required to maintain the epigenetic regulation that promoted the Prox1-VEGFR3 autoregulatory loop (Ma et al., 2021).
Altogether, the expression levels of Prox1 in the vascular system encompass multiple transcriptional and epigenetic players that maintain the proper balance between blood endothelial and lymphatic endothelial specification.
4.2.2 Specific regulation of Prox1 in the CNS vasculature
Although some Prox1+ LECs are derived from venous capillaries in the developing Schlemm’s canal and meninges, no Prox1 expression is observed in the vasculature of the brain and spinal cord parenchyma (Antila et al., 2017; Izen et al., 2018) (Figure 5). Considering the observation that in vivo overexpression of Prox1 in the vascular bed using Tie1 promoter decreased the expression of junctional proteins such as zonula occludens 1 (ZO-1), Occludin (Ocln) and JAM-1, as well as triggered increase permeability (Kim et al., 2010; Kim et al., 2013), the lack of lymphatic vessels and suppression of Prox1 in ECs might be compatible with the BBB function which regulates the brain homeostasis and protects the CNS. CNS ECs might avoid the expression of Prox1 to maintain the BBB properties and functions; however, little is known about whether ectopic Prox1 expression affects the BBB properties and functions and what regulates Prox1 expression in the CNS ECs.
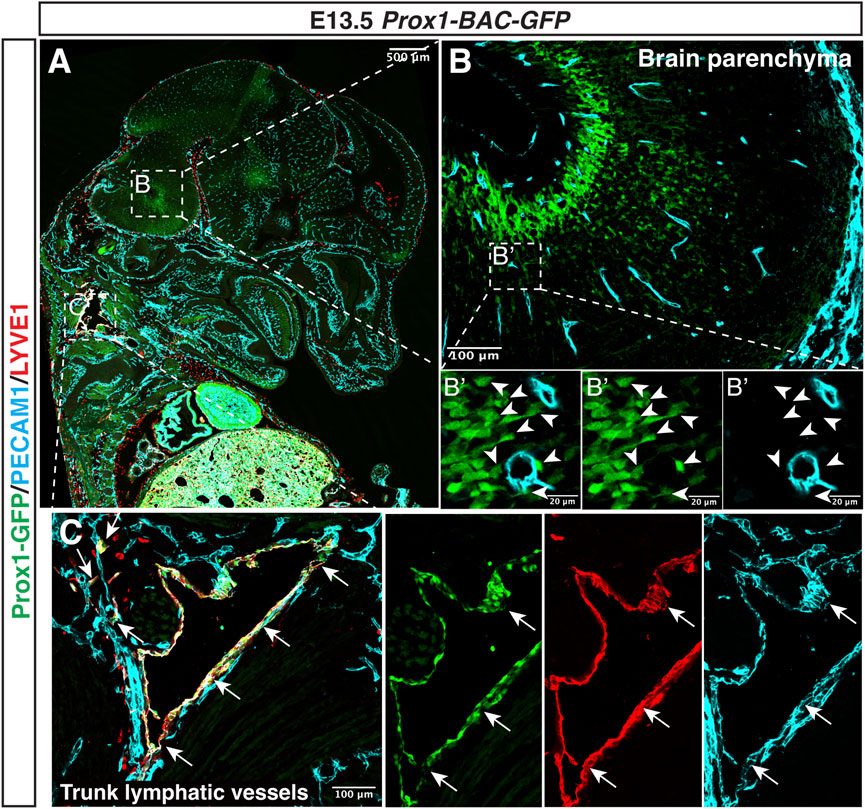
FIGURE 5. Prox1 is not expressed in the developing CNS vasculature. Section immunostaining of Prox1-BAC-GFP brain at E13.5 with anti-PECAM1 (cyan) and anti-LYVE-1 (red) is shown. The boxed regions in (A) are magnified in (B,C), and the boxed region in (B) is magnified in (B’). In the brain parenchyma (B,B’), Prox1-GFP expression is found in a subset of neural progenitors [(B) green, white arrowheads], but this GFP expression does not colocalize with the PECAM1+ endothelial cells [(B’) green vs. cyan]. In the trunk (C), Prox1-GFP + LYVE-1+ PECAM1+ lymphatic vessels are found [(C) arrows]. Scale bars 500, 100, and 20 μm.
The canonical Wnt/β-catenin signaling is essential for the acquisition and maintenance of the BBB properties (Liebner et al., 2008; Daneman et al., 2009). Activation of T-cell factor/lymphoid enhancer factor (TCF/LEF1) in brain ECs is required to control the expression of BBB-specific programs that induce the expression of TJ proteins and the repression of PLVAP to inhibit fenestration, permeability, and transcytosis. Given that Wnt/β-catenin signaling declines at late embryonic and postnatal stages (Corada et al., 2019), Wnt/β-catenin signaling appears to be maintained at the minimum active levels to maintain the integrity of the adult BBB: EC-specific deletion of β-catenin in adult mice compromises the BBB integrity by downregulating the expression of TJ proteins Claudin 5 (Cldn5), ZO-1 and Ocln (Zhou et al., 2014; Tran et al., 2016; Hussain et al., 2022). Moreover, these mutants increase transcytosis due to a reduction in the expression of major facilitator superfamily domain containing 2A (Mfsd2a), which represses the caveolae-dependent transcytosis (Ben-Zvi et al., 2014; Andreone et al., 2017; Wang et al., 2020b; Cui et al., 2021; Hussain et al., 2022).
Studies by Boye et al. (2022) described the transmembrane receptor Unc5b and its ligand Netrin-1 as upstream regulators of β-catenin in brain ECs, since their absence provokes cerebrovascular leak in postnatal and adult mice. Endothelial-specific deletion of Unc5b in mice generated BBB leakage for tracers up to 40 KDa and the brain microvasculature and displayed decreased Cldn5 and increased PLVAP expression, common features under the BBB breakdown.
Interestingly, there is wide evidence that Prox1 is a downstream target of Wnt/β-catenin/TCF signaling in many different cell types, including colon cancer cells, neural stem cells (NSCs), LECs, lymphatic valves, and hematopoietic stem cells (HSCs) (Petrova et al., 2008; Karalay et al., 2011; Nicenboim et al., 2015; Cha et al., 2016; Cha et al., 2018; Lin et al., 2022). TCF/LEF binding sites are identified in the −43 and −49 kb regions of the Prox1 transcriptional start site, which are conserved in human, mouse, rat, and chicken (Petrova et al., 2008; Karalay et al., 2011) (Figure 4). ChIP assay demonstrated that TCF4 binds specifically to the −49 kb region to activate Prox1 expression in intestinal cancer cells (Petrova et al., 2008), whereas in murine hippocampal NSCs, Prox1 activation requires the binding of TCF/LEF to both the −43 and −49 kb regions (Karalay et al., 2011). The canonical Wnt/β-catenin signaling regulates LEC specification in zebrafish and human embryonic stem cells (hESCs) (Nicenboim et al., 2015) by promoting the angioblast-to-lymphatic transition, as well as inducing Prox1 expression in HSCs (Lin et al., 2022).
The Sry-related HMG box 17 (Sox17) transcription factor exerts a positive role on Wnt/β-catenin signaling in CNS ECs to maintain the BBB (Corada et al., 2019) and blood-retina barrier (BRB) properties (Yang et al., 2020). Sox17 is one of the major transcriptional targets of Wnt/β-catenin signaling during CNS vascular development, while Sox17 modulates Wnt/β-catenin signaling. Indeed, EC-specific deletion of Sox17, which causes BBB disruptions, can be rescued by the expression of a constitutive active form of β-catenin (Corada et al., 2019). Considering that Sox17 expression increases during development and after birth, Sox17 and β-catenin act in synergy to maintain the integrity of the brain microcirculation. Interestingly, TCF/LEF and SOX binding sites overlap in the −43 and −49 kb regions upstream Prox1 locus (Figure 4), indicating that the presence of SOX family proteins may repress the effects of Wnt/β-catenin on Prox1 transcriptional activation (Kuwabara et al., 2009; Karalay et al., 2011).
Altogether, although the regulation of the lymphatic master regulator Prox1 in the CNS vasculature is poorly understood, there is indirect evidence that supports that its expression should be downregulated in the brain and spinal cord ECs to keep the BBB properties, which include the high expression of TJ proteins, lack of fenestration and low rate of transcytosis and permeability across the barrier. Future studies to determine whether SOX family transcription factors expressed in the brain microvasculature could be inactivating the effect of Wnt/β-catenin signaling on Prox1 locus to control its expression as it has been already shown in adult neurogenesis (Kuwabara et al., 2009) would be interesting.
5 Concluding remarks and outstanding questions
Traditionally, the CNS has been considered an immune-privileged site due to two main features that differentiate this tissue from the others in the body: 1) the lack of efficient immune response, which is linked with the absence of lymphatic vasculature in the brain and spinal cord parenchyma, and 2) the presence of a restricted blood-brain barrier composed by unique microvasculature, characterized by the expression of high level of TJ proteins and restricted transcytosis and fenestration, properties required to maintain the barrier function. Considering the functional and structural differences between the lymphatic and the CNS vascular beds, the specialization of the CNS ECs to form the BBB is incompatible with the acquisition of the lymphatic phenotype in the brain and spinal cord parenchyma. But how does the CNS microenvironment prevent lymphatic vessel development?
We here discuss two potential mechanisms by which the CNS prevents the emergence of lymphatic vasculature inside the brain and spinal cord parenchyma. One potential mechanism is that, albeit the extensive network of meningeal lymphatics in the skull-associated meninges and non-lumenized LECs in the surface of the brain, the CNS may prevent the LEC sprouting and invasion from the pre-existing lymphatic vessels in adjacent tissues. The other is that the CNS may prevent the differentiation of BECs into LECs, through the suppression of Prox1 expression in CNS ECs.
It seems that the CNS microenvironment provides a permissive environment for the development of blood vasculature but not lymphatic vasculature. VEGF-A is expressed and released by neural progenitors to promote the ingrowth of capillaries in the developing brain and spinal cord parenchyma. Likewise, VEGF-C is also expressed in embryonic and early postnatal brain and induces proliferation of neural progenitors (Le Bras et al., 2006; Ward and Cunningham, 2015). However, CNS ECs express lower level of VEGFR3, possibly due to the lack of the positive feedback loop between Prox1 and VEGFR3. These observations suggest that the lack of lymphatic vessels in the brain and spinal cord parenchyma is unlikely to result from an insufficient microenvironment for lymphatic vessel development.
VEGF-C/VEGFR3 signaling activates lymphangiogenesis in development as well as pathological settings. In the bone, the tissue long considered devoid of lymphatic vasculature until the recent findings (Biswas et al., 2023), the exogenous expression of VEGF-C was enough to induce lymphangiogenesis and invasion of pre-existing lymphatic vessels in the neighbor tissues (Hominick et al., 2018; Monroy et al., 2020). Similarly, taking advantage of the plasticity shown by the lymphatic system, several groups promoted the expansion of meningeal lymphatics by the exogenous expression of VEGF-C, resulting in a better immune response against brain tumors or improvement of CSF drainage to reduce neuroinflammation (Antila et al., 2017; Song et al., 2020).
Brain mural LECs in the zebrafish vasculature model invade into the brain parenchyma and become lumenized lymphatics that can drain the ISF and resolve the edema (Chen et al., 2019). However, in the murine CNS vasculature model, LEC invasion from the meningeal lymphatic vessels or leptomeningeal non-lumenized LECs into the brain parenchyma has not been reported under the steady or pathological conditions. Further studies are necessary to elucidate the impact of this change during evolution.
The transcriptional and epigenetic regulators controlling Prox1 expression in the vascular system maintain the balance between BEC and LEC fate; nonetheless, little is known about specific Prox1 regulation in the CNS BECs. There are examples of non-CNS organs where Prox1 expression is prevented to ensure the segregation between blood and lymphatic vasculatures. Findings from Tai-Nagara et al. (2020) highlighted the role of FLCN gene as a gatekeeper to avoid Prox1 expression in those BECs that must keep the blood specification. The expression of FLCN in BECs prevents the translocation of the TFE3 to the nuclei, impairing the activation of Prox1 and therefore the induction of lymphatic specification.
What is the link between the lack of lymphatic vessels and BBB formation? The forced expression of Prox1 in BECs leads to a reduced expression of junctional proteins and an increase in the vascular permeability (Kim et al., 2010; Kim et al., 2013). In the CNS vasculature, these changes are incompatible with the maintenance of the BBB integrity, raising the possibility that Prox1 expression should be tightly suppressed in BBB ECs. Which signaling controls Prox1 suppression and BBB formation? Wnt/β-catenin signaling is crucial for the acquisition and maintenance of the BBB properties, including the induction of TJ and adherens junction proteins as well as the inhibition of fenestration, permeability, and transcytosis. Of note, identification of TCF/LEF binding sites upstream of the Prox1 locus suggested this transcription factor as a downstream target of Wnt/β-catenin/TCF pathway in different cell types. In a similar line of research, Sox17 is a major downstream target and regulator of Wnt/β-catenin signaling, and TCF/LEF and SOX binding sites overlap in the −43 and −49 kb regions upstream Prox1 locus. Whether inactivation of Wnt/β-catenin signaling and Sox17 affects Prox1 expression in CNS ECs remains unclear. Elegant studies in zebrafish by Das et al. (2022) demonstrated that anal fin vascularization requires transdifferentiation of previously formed lymphatic vessels into blood vessels. Notably, the loss of LEC fate in this model is related to the upregulation of several BEC markers, where Sox17 plays a relevant role in the suppression of Prox1 expression to promote the LEC-to-BEC transition. Considering that Sox17 expression increases in CNS ECs during BBB development and maturation, Sox17 might repress LEC fate and maintain CNS EC properties. Further studies will provide the fundamental mechanism of lymphatic avascularity in the brain and spinal cord parenchyma. Moreover, a possible dysregulation of Prox1 expression could lead to BBB alterations in pathological scenarios.
Author contributions
SG-H contributed to the concepts, writing, editing of the manuscript, and making the figures. Y-SM contributed to the concepts and editing of the manuscript.
Funding
This work was supported by the Intramural Research Program of the National Heart, Lung, and Blood Institute, National Institutes of Health (HL006115-12).
Acknowledgments
We thank K. Gill for laboratory management and editorial advice and V. Sam for administrative assistance. Thanks also to members of the Laboratory of Stem Cell and Neuro-Vascular Biology for valuable discussion.
Conflict of interest
The authors declare that the research was conducted in the absence of any commercial or financial relationships that could be construed as a potential conflict of interest.
Publisher’s note
All claims expressed in this article are solely those of the authors and do not necessarily represent those of their affiliated organizations, or those of the publisher, the editors and the reviewers. Any product that may be evaluated in this article, or claim that may be made by its manufacturer, is not guaranteed or endorsed by the publisher.
References
Abbott, N. J., Patabendige, A. A., Dolman, D. E., Yusof, S. R., and Begley, D. J. (2010). 'Structure and function of the blood-brain barrier. Neurobiol. Dis. 37, 13–25. doi:10.1016/j.nbd.2009.07.030
Absinta, M., Ha, S. K., Nair, G., Sati, P., Luciano, N. J., Palisoc, M., et al. (2017). 'Human and nonhuman primate meninges harbor lymphatic vessels that can be visualized noninvasively by MRI. Elife 6, e29738. doi:10.7554/eLife.29738
Ahn, J. H., Cho, H., Kim, J. H., Kim, S. H., Ham, J. S., Park, I., et al. (2019). 'Meningeal lymphatic vessels at the skull base drain cerebrospinal fluid. Nature 572, 62–66. doi:10.1038/s41586-019-1419-5
Alitalo, K. (2011). 'The lymphatic vasculature in disease. Nat. Med. 17, 1371–1380. doi:10.1038/nm.2545
Andreone, B. J., Chow, B. W., Tata, A., Lacoste, B., Ben-Zvi, A., Bullock, K., et al. (2017). 'Blood-Brain barrier permeability is regulated by lipid transport-dependent suppression of caveolae-mediated transcytosis. Neuron 94, 581–594. doi:10.1016/j.neuron.2017.03.043
Antila, S., Karaman, S., Nurmi, H., Airavaara, M., Voutilainen, M. H., Mathivet, T., et al. (2017). 'Development and plasticity of meningeal lymphatic vessels. J. Exp. Med. 214, 3645–3667. doi:10.1084/jem.20170391
Aspelund, A., Antila, S., Proulx, S. T., Karlsen, T. V., Karaman, S., Detmar, M., et al. (2015). 'A dural lymphatic vascular system that drains brain interstitial fluid and macromolecules. J. Exp. Med. 212, 991–999. doi:10.1084/jem.20142290
Aspelund, A., Tammela, T., Antila, S., Nurmi, H., Leppanen, V. M., Zarkada, G., et al. (2014). 'The Schlemm's canal is a VEGF-C/VEGFR-3-responsive lymphatic-like vessel. J. Clin. Investigation 124, 3975–3986. doi:10.1172/JCI75395
Audoy-Remus, J., Richard, J. F., Soulet, D., Zhou, H., Kubes, P., and Vallieres, L. (2008). 'Rod-Shaped monocytes patrol the brain vasculature and give rise to perivascular macrophages under the influence of proinflammatory cytokines and angiopoietin-2. J. Neurosci. 28, 10187–10199. doi:10.1523/JNEUROSCI.3510-08.2008
Balint, L., Ocskay, Z., Deak, B. A., Aradi, P., and Jakus, Z. (2019a). 'Lymph flow induces the postnatal formation of mature and functional meningeal lymphatic vessels. Front. Immunol. 10, 3043. doi:10.3389/fimmu.2019.03043
Balint, L., Ocskay, Z., Deak, B. A., and Jakus, Z. (2019b). 'Lymph flow mediated postnatal remodeling of meningeal lymphatics is required for the uptake and transport of macromolecules from the central nervous system. J. Vasc. Res. 56, 9.
Baluk, P., Fuxe, J., Hashizume, H., Romano, T., Lashnits, E., Butz, S., et al. (2007). 'Functionally specialized junctions between endothelial cells of lymphatic vessels. J. Exp. Med. 204, 2349–2362. doi:10.1084/jem.20062596
Banerji, S., Ni, J., Wang, S. X., Clasper, S., Su, J., Tammi, R., et al. (1999). 'LYVE-1, a new homologue of the CD44 glycoprotein, is a lymph-specific receptor for hyaluronan. J. Cell Biol. 144, 789–801. doi:10.1083/jcb.144.4.789
Barker, C. F., and Billingham, R. E. (1977). 'Immunologically privileged sites. Adv. Immunol. 25, 1–54.
Bazigou, E., and Makinen, T. (2013). Flow control in our vessels: Vascular valves make sure there is no way back. Cell Mol. Life Sci. 70, 1055–1066. doi:10.1007/s00018-012-1110-6
Ben-Zvi, A., Lacoste, B., Kur, E., Andreone, B. J., Mayshar, Y., Yan, H., et al. (2014). 'Mfsd2a is critical for the formation and function of the blood-brain barrier. Nature 509, 507–511. doi:10.1038/nature13324
Biswas, L., Chen, J., De Angelis, J., Singh, A., Owen-Woods, C., Ding, Z., et al. (2023). 'Lymphatic vessels in bone support regeneration after injury. Cell 186, 382–397. doi:10.1016/j.cell.2022.12.031
Bolte, A. C., Dutta, A. B., Hurt, M. E., Smirnov, I., Kovacs, M. A., McKee, C. A., et al. (2020). 'Meningeal lymphatic dysfunction exacerbates traumatic brain injury pathogenesis. Nat. Commun. 11, 4524. doi:10.1038/s41467-020-18113-4
Bower, N. I., Koltowska, K., Pichol-Thievend, C., Virshup, I., Paterson, S., Lagendijk, A. K., et al. (2017). 'Mural lymphatic endothelial cells regulate meningeal angiogenesis in the zebrafish. Nat. Neurosci. 20, 774–783. doi:10.1038/nn.4558
Boye, K., Geraldo, L. H., Furtado, J., Pibouin-Fragner, L., Poulet, M., Kim, D., et al. (2022). 'Endothelial Unc5B controls blood-brain barrier integrity. Nat. Commun. 13, 1169. doi:10.1038/s41467-022-28785-9
Brioschi, S., Wang, W. L., Peng, V., Wang, M., Shchukina, I., Greenberg, Z. J., et al. (2021). 'Heterogeneity of meningeal B cells reveals a lymphopoietic niche at the CNS borders. Science 373, eabf9277. doi:10.1126/science.abf9277
Bui, K., and Hong, Y. K. (2020). 'Ras pathways on Prox1 and lymphangiogenesis: Insights for therapeutics. Front. Cardiovasc Med. 7, 597374. doi:10.3389/fcvm.2020.597374
Castranova, D., Samasa, B., Venero Galanternik, M., Jung, H. M., Pham, V. N., and Weinstein, B. M. (2021). 'Live imaging of intracranial lymphatics in the zebrafish. Circ. Res. 128, 42–58. doi:10.1161/CIRCRESAHA.120.317372
Cha, B., Geng, X., Mahamud, M. R., Fu, J., Mukherjee, A., Kim, Y., et al. (2016). Mechanotransduction activates canonical Wnt/β-catenin signaling to promote lymphatic vascular patterning and the development of lymphatic and lymphovenous valves. Genes Dev. 30, 1454–1469. doi:10.1101/gad.282400.116
Cha, B., Geng, X., Mahamud, M. R., Zhang, J. Y., Chen, L., Kim, W., et al. (2018). Complementary Wnt sources regulate lymphatic vascular development via PROX1-dependent wnt/β-catenin signaling. Cell Rep. 25, 571–584. doi:10.1016/j.celrep.2018.09.049
Cha, B., Ho, Y. C., Geng, X., Mahamud, M. R., Chen, L., Kim, Y., et al. (2020). 'YAP and TAZ maintain PROX1 expression in the developing lymphatic and lymphovenous valves in response to VEGF-C signaling. Development 147, dev195453. doi:10.1242/dev.195453
Chen, J., He, J., and Luo, L. (2022). 'Brain vascular damage-induced lymphatic ingrowth is directed by Cxcl12b/Cxcr4a. Development 149, dev200729. doi:10.1242/dev.200729
Chen, J., He, J., Ni, R., Yang, Q., Zhang, Y., and Luo, L. (2019). Cerebrovascular injuries induce lymphatic invasion into brain parenchyma to guide vascular regeneration in zebrafish. Dev. Cell 49, 697–710. doi:10.1016/j.devcel.2019.03.022
Chen, J., Li, X., Ni, R., Chen, Q., Yang, Q., He, J., et al. (2021). 'Acute brain vascular regeneration occurs via lymphatic transdifferentiation. Dev. Cell 56, 3115–3127. doi:10.1016/j.devcel.2021.09.005
Chen, J., Wang, L., Xu, H., Xing, L., Zhuang, Z., Zheng, Y., et al. (2020). 'Meningeal lymphatics clear erythrocytes that arise from subarachnoid hemorrhage. Nat. Commun. 11, 3159. doi:10.1038/s41467-020-16851-z
Cho, H., Kim, J., Ahn, J. H., Hong, Y. K., Makinen, T., Lim, D. S., et al. (2019). 'YAP and TAZ negatively regulate Prox1 during developmental and pathologic lymphangiogenesis. Circ. Res. 124, 225–242. doi:10.1161/CIRCRESAHA.118.313707
Choi, D., Park, E., Jung, E., Seong, Y. J., Yoo, J., Lee, E., et al. (2017). 'Laminar flow downregulates Notch activity to promote lymphatic sprouting. J. Clin. Investigation 127, 1225–1240. doi:10.1172/JCI87442
Choi, I., Chung, H. K., Ramu, S., Lee, H. N., Kim, K. E., Lee, S., et al. (2011). 'Visualization of lymphatic vessels by Prox1-promoter directed GFP reporter in a bacterial artificial chromosome-based transgenic mouse. Blood 117, 362–365. doi:10.1182/blood-2010-07-298562
Corada, M., Orsenigo, F., Bhat, G. P., Conze, L. L., Breviario, F., Cunha, S. I., et al. (2019). Fine-tuning of Sox17 and canonical Wnt coordinates the permeability properties of the blood-brain barrier. Circ. Res. 124, 511–525. doi:10.1161/CIRCRESAHA.118.313316
Cugurra, A., Mamuladze, T., Rustenhoven, J., Dykstra, T., Beroshvili, G., Greenberg, Z. J., et al. (2021). 'Skull and vertebral bone marrow are myeloid cell reservoirs for the meninges and CNS parenchyma. Science 373, eabf7844. doi:10.1126/science.abf7844
Cui, Y., Wang, Y., Song, X., Ning, H., Zhang, Y., Teng, Y., et al. (2021). 'Brain endothelial PTEN/AKT/NEDD4-2/MFSD2A axis regulates blood-brain barrier permeability. Cell Rep. 36, 109327. doi:10.1016/j.celrep.2021.109327
Da Mesquita, S., Louveau, A., Vaccari, A., Smirnov, I., Cornelison, R. C., Kingsmore, K. M., et al. (2018). Functional aspects of meningeal lymphatics in ageing and Alzheimer's disease. Nature 560, 185–191. doi:10.1038/s41586-018-0368-8
Daneman, R., Agalliu, D., Zhou, L., Kuhnert, F., Kuo, C. J., and Barres, B. A. (2009). Wnt/β-catenin signaling is required for CNS, but not non-CNS, angiogenesis. Proc. Natl. Acad. Sci. U. S. A. 106, 641–646. doi:10.1073/pnas.0805165106
Daneman, R., and Prat, A. (2015). 'The blood-brain barrier. Cold Spring Harb. Perspect. Biol. 7, a020412. doi:10.1101/cshperspect.a020412
Das, R. N., Tevet, Y., Safriel, S., Han, Y., Moshe, N., Lambiase, G., et al. (2022). 'Generation of specialized blood vessels via lymphatic transdifferentiation. Nature 606, 570–575. doi:10.1038/s41586-022-04766-2
Dieterich, L. C., Klein, S., Mathelier, A., Sliwa-Primorac, A., Ma, Q., Hong, Y. K., et al. (2015). DeepCAGE transcriptomics reveal an important role of the transcription factor MAFB in the lymphatic endothelium. Cell Rep. 13, 1493–1504. doi:10.1016/j.celrep.2015.10.002
Dumont, D. J., Jussila, L., Taipale, J., Lymboussaki, A., Mustonen, T., Pajusola, K., et al. (1998). 'Cardiovascular failure in mouse embryos deficient in VEGF receptor-3. Science 282, 946–949. doi:10.1126/science.282.5390.946
Eide, P. K., Vatnehol, S. A. S., Emblem, K. E., and Ringstad, G. (2018). 'Magnetic resonance imaging provides evidence of glymphatic drainage from human brain to cervical lymph nodes. Sci. Rep. 8, 7194. doi:10.1038/s41598-018-25666-4
Eng, T. C., Chen, W., Okuda, K. S., Misa, J. P., Padberg, Y., Crosier, K. E., et al. (2019). 'Zebrafish facial lymphatics develop through sequential addition of venous and non-venous progenitors. EMBO Rep. 20, e47079. doi:10.15252/embr.201847079
Engelhardt, B., Vajkoczy, P., and Weller, R. O. (2017). The movers and shapers in immune privilege of the CNS. Nat. Immunol. 18, 123–131. doi:10.1038/ni.3666
Faraco, G., Sugiyama, Y., Lane, D., Garcia-Bonilla, L., Chang, H., Santisteban, M. M., et al. (2016). 'Perivascular macrophages mediate the neurovascular and cognitive dysfunction associated with hypertension. J. Clin. Investigation 126, 4674–4689. doi:10.1172/JCI86950
Francois, M., Caprini, A., Hosking, B., Orsenigo, F., Wilhelm, D., Browne, C., et al. (2008). 'Sox18 induces development of the lymphatic vasculature in mice. Nature 456, 643–647. doi:10.1038/nature07391
Gauvrit, S., Villasenor, A., Strilic, B., Kitchen, P., Collins, M. M., Marin-Juez, R., et al. (2018). 'HHEX is a transcriptional regulator of the VEGFC/FLT4/PROX1 signaling axis during vascular development. Nat. Commun. 9, 2704. doi:10.1038/s41467-018-05039-1
Geudens, I., Herpers, R., Hermans, K., Segura, I., Ruiz de Almodovar, C., Bussmann, J., et al. (2010). Role of delta-like-4/Notch in the formation and wiring of the lymphatic network in zebrafish. Arterioscler. Thromb. Vasc. Biol. 30, 1695–1702. doi:10.1161/ATVBAHA.110.203034
Hawkins, B. T., and Davis, T. P. (2005). The blood-brain barrier/neurovascular unit in health and disease. Pharmacol. Rev. 57, 173–185. doi:10.1124/pr.57.2.4
Hominick, D., Silva, A., Khurana, N., Liu, Y., Dechow, P. C., Feng, J. Q., et al. (2018). 'VEGF-C promotes the development of lymphatics in bone and bone loss. Elife 7, e34323. doi:10.7554/eLife.34323
Hong, Y. K., Harvey, N., Noh, Y. H., Schacht, V., Hirakawa, S., Detmar, M., et al. (2002). 'Prox1 is a master control gene in the program specifying lymphatic endothelial cell fate. Dev. Dyn. 225, 351–357. doi:10.1002/dvdy.10163
Hsu, M., Rayasam, A., Kijak, J. A., Choi, Y. H., Harding, J. S., Marcus, S. A., et al. (2019). Neuroinflammation-induced lymphangiogenesis near the cribriform plate contributes to drainage of CNS-derived antigens and immune cells. Nat. Commun. 10, 229. doi:10.1038/s41467-018-08163-0
Huisman, Y., Uphoff, K., Berger, M., Dobrindt, U., Schelhaas, M., Zobel, T., et al. (2022). 'Meningeal lymphatic endothelial cells fulfill scavenger endothelial cell function and cooperate with microglia in waste removal from the brain. Glia 70, 35–49. doi:10.1002/glia.24081
Huntington, G. S., and McClure, C. F. W. (1910). 'The anatomy and development of the jugular lymph sacs in the domestic cat (Felis domestica). Am. J. Anat. 10, 177–312. doi:10.1002/aja.1000100108
Hussain, B., Fang, C., Huang, X., Feng, Z., Yao, Y., Wang, Y., et al. (2022). 'Endothelial beta-catenin deficiency causes blood-brain barrier breakdown via enhancing the paracellular and transcellular permeability. Front. Mol. Neurosci. 15, 895429. doi:10.3389/fnmol.2022.895429
Iadecola, C. (2017). The neurovascular unit coming of age: A journey through neurovascular coupling in health and disease. Neuron 96, 17–42. doi:10.1016/j.neuron.2017.07.030
Iliff, J. J., Wang, M., Liao, Y., Plogg, B. A., Peng, W., Gundersen, G. A., et al. (2012). A paravascular pathway facilitates CSF flow through the brain parenchyma and the clearance of interstitial solutes, including amyloid β. Sci. Transl. Med. 4, 147ra111. doi:10.1126/scitranslmed.3003748
Izen, R. M., Yamazaki, T., Nishinaka-Arai, Y., Hong, Y. K., and Mukouyama, Y. S. (2018). Postnatal development of lymphatic vasculature in the brain meninges. Dev. Dyn. 247, 741–753. doi:10.1002/dvdy.24624
Jacob, L., Boisserand, L. S. B., Geraldo, L. H. M., de Brito Neto, J., Mathivet, T., Antila, S., et al. (2019). 'Anatomy and function of the vertebral column lymphatic network in mice. Nat. Commun. 10, 4594. doi:10.1038/s41467-019-12568-w
Jacob, L., de Brito Neto, J., Lenck, S., Corcy, C., Benbelkacem, F., Geraldo, L. H., et al. (2022). 'Conserved meningeal lymphatic drainage circuits in mice and humans. J. Exp. Med. 219, e20220035. doi:10.1084/jem.20220035
Jani, R. H., and Sekula, R. F. (2018). Magnetic resonance imaging of human dural meningeal lymphatics. Neurosurgery 83, E10–E12. doi:10.1093/neuros/nyy171
Jeong, Y. M., Lee, J. G., Cho, H. J., Lee, W. S., Jeong, J., and Lee, J. S. (2021). Differential clearance of Aβ species from the brain by brain lymphatic endothelial cells in zebrafish. Int. J. Mol. Sci. 22, 11883. doi:10.3390/ijms222111883
Johnson, N. C., Dillard, M. E., Baluk, P., McDonald, D. M., Harvey, N. L., Frase, S. L., et al. (2008). 'Lymphatic endothelial cell identity is reversible and its maintenance requires Prox1 activity. Genes & Dev. 22, 3282–3291. doi:10.1101/gad.1727208
Kang, J., Yoo, J., Lee, S., Tang, W., Aguilar, B., Ramu, S., et al. (2010). An exquisite cross-control mechanism among endothelial cell fate regulators directs the plasticity and heterogeneity of lymphatic endothelial cells. Blood 116, 140–150. doi:10.1182/blood-2009-11-252270
Karalay, O., Doberauer, K., Vadodaria, K. C., Knobloch, M., Berti, L., Miquelajauregui, A., et al. (2011). 'Prospero-related homeobox 1 gene (Prox1) is regulated by canonical Wnt signaling and has a stage-specific role in adult hippocampal neurogenesis. Proc. Natl. Acad. Sci. U. S. A. 108, 5807–5812. doi:10.1073/pnas.1013456108
Karkkainen, M. J., Haiko, P., Sainio, K., Partanen, J., Taipale, J., Petrova, T. V., et al. (2004). Vascular endothelial growth factor C is required for sprouting of the first lymphatic vessels from embryonic veins. Nat. Immunol. 5, 74–80. doi:10.1038/ni1013
Kazenwadel, J., Betterman, K. L., Chong, C. E., Stokes, P. H., Lee, Y. K., Secker, G. A., et al. (2015). 'GATA2 is required for lymphatic vessel valve development and maintenance. J. Clin. Investigation 125, 2979–2994. doi:10.1172/JCI78888
Kazenwadel, J., Michael, M. Z., and Harvey, N. L. (2010). 'Prox1 expression is negatively regulated by miR-181 in endothelial cells. Blood 116, 2395–2401. doi:10.1182/blood-2009-12-256297
Kazenwadel, J., Venugopal, P., Oszmiana, A., Toubia, J., Arriola-Martinez, L., Panara, V., et al. (2023). A Prox1 enhancer represses haematopoiesis in the lymphatic vasculature. Nature 614, 343–348. doi:10.1038/s41586-022-05650-9
Kim, H., Cruz, M., Bourdeau, A., and Dumont, D. J. (2013). 'Cell-Cell interactions influence vascular reprogramming by Prox1 during embryonic development. Plos One 8, e52197. doi:10.1371/journal.pone.0052197
Kim, H., Nguyen, V. P. K. H., Petrova, T. V., Cruz, M., Alitalo, K., and Dumont, D. J. (2010). 'Embryonic vascular endothelial cells are malleable to reprogramming via Prox1 to a lymphatic gene signature. Bmc Dev. Biol. 10, 72. doi:10.1186/1471-213X-10-72
Kim, J., Park, D. Y., Bae, H., Park, D. Y., Kim, D., Lee, C. K., et al. (2017). 'Impaired angiopoietin/Tie2 signaling compromises Schlemm's canal integrity and induces glaucoma. J. Clin. Investigation 127, 3877–3896. doi:10.1172/JCI94668
Kim, K. E., Sung, H. K., and Koh, G. Y. (2007). 'Lymphatic development in mouse small intestine. Dev. Dyn. 236, 2020–2025. doi:10.1002/dvdy.21200
Kizhatil, K., Ryan, M., Marchant, J. K., Henrich, S., and John, S. W. (2014). 'Schlemm's canal is a unique vessel with a combination of blood vascular and lymphatic phenotypes that forms by a novel developmental process. PLoS Biol. 12, e1001912. doi:10.1371/journal.pbio.1001912
Klotz, L., Norman, S., Vieira, J. M., Masters, M., Rohling, M., Dube, K. N., et al. (2015). Cardiac lymphatics are heterogeneous in origin and respond to injury. Nature 522, 62–67. doi:10.1038/nature14483
Kuwabara, T., Hsieh, J., Muotri, A., Yeo, G., Warashina, M., Lie, D. C., et al. (2009). 'Wnt-mediated activation of NeuroD1 and retro-elements during adult neurogenesis. Nat. Neurosci. 12, 1097–1105. doi:10.1038/nn.2360
Le Bras, B., Barallobre, M. J., Homman-Ludiye, J., Ny, A., Wyns, S., Tammela, T., et al. (2006). 'VEGF-C is a trophic factor for neural progenitors in the vertebrate embryonic brain. Nat. Neurosci. 9, 340–348. doi:10.1038/nn1646
Liebner, S., Corada, M., Bangsow, T., Babbage, J., Taddei, A., Czupalla, C. J., et al. (2008). 'Wnt/beta-catenin signaling controls development of the blood-brain barrier. J. Cell Biol. 183, 409–417. doi:10.1083/jcb.200806024
Lin, Q., Wu, L., Chatla, S., Chowdhury, F. A., Atale, N., Joseph, J., et al. (2022). 'Hematopoietic stem cell regeneration through paracrine regulation of the Wnt5a/Prox1 signaling axis. J. Clin. Investigation 132, e155914. doi:10.1172/JCI155914
Lioux, G., Liu, X., Temino, S., Oxendine, M., Ayala, E., Ortega, S., et al. (2020). 'A second heart field-derived vasculogenic niche contributes to cardiac lymphatics. Dev. Cell 52, 350–363.e6. doi:10.1016/j.devcel.2019.12.006
Louveau, A., Herz, J., Alme, M. N., Salvador, A. F., Dong, M. Q., Viar, K. E., et al. (2018). 'CNS lymphatic drainage and neuroinflammation are regulated by meningeal lymphatic vasculature. Nat. Neurosci. 21, 1380–1391. doi:10.1038/s41593-018-0227-9
Louveau, A., Smirnov, I., Keyes, T. J., Eccles, J. D., Rouhani, S. J., Peske, J. D., et al. (2015). 'Structural and functional features of central nervous system lymphatic vessels. Nature 523, 337–341. doi:10.1038/nature14432
Ma, W., Gil, H. J., Liu, X., Diebold, L. P., Morgan, M. A., Oxendine-Burns, M. J., et al. (2021). 'Mitochondrial respiration controls the Prox1-Vegfr3 feedback loop during lymphatic endothelial cell fate specification and maintenance. Sci. Adv. 7, eabe7359. doi:10.1126/sciadv.abe7359
Makinen, T., Veikkola, T., Mustjoki, S., Karpanen, T., Catimel, B., Nice, E. C., et al. (2001). Isolated lymphatic endothelial cells transduce growth, survival and migratory signals via the VEGF-C/D receptor VEGFR-3. EMBO J. 20, 4762–4773. doi:10.1093/emboj/20.17.4762
Martinez-Corral, I., Ulvmar, M. H., Stanczuk, L., Tatin, F., Kizhatil, K., John, S. W., et al. (2015). 'Nonvenous origin of dermal lymphatic vasculature. Circ. Res. 116, 1649–1654. doi:10.1161/CIRCRESAHA.116.306170
Maruyama, K., Miyagawa-Tomita, S., Mizukami, K., Matsuzaki, F., and Kurihara, H. (2019). 'Isl1-expressing non-venous cell lineage contributes to cardiac lymphatic vessel development. Dev. Biol. 452, 134–143. doi:10.1016/j.ydbio.2019.05.002
Mato, M., and Ookawara, S. (1981). 'Influences of age and vasopressin on the uptake capacity of fluorescent granular perithelial cells (FGP) of small cerebral vessels of the rat. Am. J. Anat. 162, 45–53. doi:10.1002/aja.1001620105
Medawar, P. B. (1948). 'Immunity to homologous grafted skin; the fate of skin homografts transplanted to the brain, to subcutaneous tissue, and to the anterior chamber of the eye. Br. J. Exp. Pathol. 29, 58–69.
Monroy, M., McCarter, A. L., Hominick, D., Cassidy, N., and Dellinger, M. T. (2020). 'Lymphatics in bone arise from pre-existing lymphatics. Development 147, dev184291. doi:10.1242/dev.184291
Murphy, J. B., and Sturm, E. (1923). 'Conditions determining the transplantability of tissues in the brain. J. Exp. Med. 38, 183–197. doi:10.1084/jem.38.2.183
Murtomaki, A., Uh, M. K., Choi, Y. K., Kitajewski, C., Borisenko, V., Kitajewski, J., et al. (2013). 'Notch1 functions as a negative regulator of lymphatic endothelial cell differentiation in the venous endothelium. Development 140, 2365–2376. doi:10.1242/dev.083865
Muthuchamy, M., and Zawieja, D. (2008). 'Molecular regulation of lymphatic contractility. Ann. N. Y. Acad. Sci. 1131, 89–99. doi:10.1196/annals.1413.008
Nedergaard, M. (2013). 'Neuroscience. Garbage truck of the brain. Science 340, 1529–1530. doi:10.1126/science.1240514
Nicenboim, J., Malkinson, G., Lupo, T., Asaf, L., Sela, Y., Mayseless, O., et al. (2015). 'Lymphatic vessels arise from specialized angioblasts within a venous niche. Nature 522, 56–61. doi:10.1038/nature14425
Niessen, K., Zhang, G., Ridgway, J. B., Chen, H., Kolumam, G., Siebel, C. W., et al. (2011). The Notch1-Dll4 signaling pathway regulates mouse postnatal lymphatic development. Blood 118, 1989–1997. doi:10.1182/blood-2010-11-319129
Norrmen, C., Ivanov, K. I., Cheng, J., Zangger, N., Delorenzi, M., Jaquet, M., et al. (2009). 'FOXC2 controls formation and maturation of lymphatic collecting vessels through cooperation with NFATc1. J. Cell Biol. 185, 439–457. doi:10.1083/jcb.200901104
Obermeier, B., Daneman, R., and Ransohoff, R. M. (2013). 'Development, maintenance and disruption of the blood-brain barrier. Nat. Med. 19, 1584–1596. doi:10.1038/nm.3407
Oliver, G., and Alitalo, K. (2005). 'The lymphatic vasculature: Recent progress and paradigms. Annu. Rev. Cell Dev. Biol. 21, 457–483. doi:10.1146/annurev.cellbio.21.012704.132338
Oliver, G., Kipnis, J., Randolph, G. J., and Harvey, N. L. (2020). The lymphatic vasculature in the 21(st) century: Novel functional roles in homeostasis and disease. Cell 182, 270–296. doi:10.1016/j.cell.2020.06.039
Oliver, G., and Srinivasan, R. S. (2010). Endothelial cell plasticity: How to become and remain a lymphatic endothelial cell. Development 137, 363–372. doi:10.1242/dev.035360
Pan, M. R., Chang, T. M., Chang, H. C., Su, J. L., Wang, H. W., and Hung, W. C. (2009). Sumoylation of Prox1 controls its ability to induce VEGFR3 expression and lymphatic phenotypes in endothelial cells. J. Cell Sci. 122, 3358–3364. doi:10.1242/jcs.050005
Park, D. Y., Lee, J., Park, I., Choi, D., Lee, S., Song, S., et al. (2014). 'Lymphatic regulator PROX1 determines Schlemm's canal integrity and identity. J. Clin. Investigation 124, 3960–3974. doi:10.1172/JCI75392
Patel, T. K., Habimana-Griffin, L., Gao, X., Xu, B., Achilefu, S., Alitalo, K., et al. (2019). 'Dural lymphatics regulate clearance of extracellular tau from the CNS. Mol. Neurodegener. 14, 11. doi:10.1186/s13024-019-0312-x
Pedrioli, D. M., Karpanen, T., Dabouras, V., Jurisic, G., van de Hoek, G., Shin, J. W., et al. (2010). 'miR-31 functions as a negative regulator of lymphatic vascular lineage-specific differentiation in vitro and vascular development in vivo. Mol. Cell Biol. 30, 3620–3634. doi:10.1128/MCB.00185-10
Petrova, T. V., and Koh, G. Y. (2020). 'Biological functions of lymphatic vessels. Science 369, eaax4063. doi:10.1126/science.aax4063
Petrova, T. V., Makinen, T., Makela, T. P., Saarela, J., Virtanen, I., Ferrell, R. E., et al. (2002). 'Lymphatic endothelial reprogramming of vascular endothelial cells by the Prox-1 homeobox transcription factor. EMBO J. 21, 4593–4599. doi:10.1093/emboj/cdf470
Petrova, T. V., Nykanen, A., Norrmen, C., Ivanov, K. I., Andersson, L. C., Haglund, C., et al. (2008). Transcription factor PROX1 induces colon cancer progression by promoting the transition from benign to highly dysplastic phenotype. Cancer Cell 13, 407–419. doi:10.1016/j.ccr.2008.02.020
Pichol-Thievend, C., Betterman, K. L., Liu, X., Ma, W., Skoczylas, R., Lesieur, E., et al. (2018). 'A blood capillary plexus-derived population of progenitor cells contributes to Genesis of the dermal lymphatic vasculature during embryonic development. Development 145.
Ramos, R. F., Hoying, J. B., Witte, M. H., and Daniel Stamer, W. (2007). Schlemm's canal endothelia, lymphatic, or blood vasculature? J. Glaucoma 16, 391–405. doi:10.1097/IJG.0b013e3180654ac6
Sabin, F. R. (1902). 'On the origin of the lymphatic system from the veins and the development of the lymph hearts and thoracic duct in the pig. Am. J. Anat. 1, 367–389. doi:10.1002/aja.1000010310
Sabine, A., Saygili Demir, C., and Petrova, T. V. (2016). Endothelial cell responses to biomechanical forces in lymphatic vessels. Antioxid. Redox Signal 25, 451–465. doi:10.1089/ars.2016.6685
Sandrone, S., Moreno-Zambrano, D., Kipnis, J., and van Gijn, J. (2019). 'A (delayed) history of the brain lymphatic system. Nat. Med. 25, 538–540. doi:10.1038/s41591-019-0417-3
Schafflick, D., Wolbert, J., Heming, M., Thomas, C., Hartlehnert, M., Borsch, A. L., et al. (2021). 'Single-cell profiling of CNS border compartment leukocytes reveals that B cells and their progenitors reside in non-diseased meninges. Nat. Neurosci. 24, 1225–1234. doi:10.1038/s41593-021-00880-y
Shan, S. F., Wang, L. F., Zhai, J. W., Qin, Y., Ouyang, H. F., Kong, Y. Y., et al. (2008). 'Modulation of transcriptional corepressor activity of prospero-related homeobox protein (Prox1) by SUMO modification. Febs Lett. 582, 3723–3728. doi:10.1016/j.febslet.2008.09.057
Shibata-Germanos, S., Goodman, J. R., Grieg, A., Trivedi, C. A., Benson, B. C., Foti, S. C., et al. (2020). 'Structural and functional conservation of non-lumenized lymphatic endothelial cells in the mammalian leptomeninges. Acta Neuropathol. 139, 383–401. doi:10.1007/s00401-019-02091-z
Shirai, Y. (1921). On the transplantation of the rat sarcoma in adult heterogenous animals. Tokyo, Japan: Jap Med World 1, 14-15.
Song, E., Mao, T., Dong, H., Boisserand, L. S. B., Antila, S., Bosenberg, M., et al. (2020). 'VEGF-C-driven lymphatic drainage enables immunosurveillance of brain tumours. Nature 577, 689–694. doi:10.1038/s41586-019-1912-x
Srinivasan, R. S., Dillard, M. E., Lagutin, O. V., Lin, F. J., Tsai, S., Tsai, M. J., et al. (2007). Lineage tracing demonstrates the venous origin of the mammalian lymphatic vasculature. Genes & Dev. 21, 2422–2432. doi:10.1101/gad.1588407
Srinivasan, R. S., Escobedo, N., Yang, Y., Interiano, A., Dillard, M. E., Finkelstein, D., et al. (2014). 'The Prox1-Vegfr3 feedback loop maintains the identity and the number of lymphatic endothelial cell progenitors. Genes Dev. 28, 2175–2187. doi:10.1101/gad.216226.113
Srinivasan, R. S., Geng, X., Yang, Y., Wang, Y. D., Mukatira, S., Studer, M., et al. (2010). The nuclear hormone receptor Coup-TFII is required for the initiation and early maintenance of Prox1 expression in lymphatic endothelial cells. Genes & Dev. 24, 696–707. doi:10.1101/gad.1859310
Stanczuk, L., Martinez-Corral, I., Ulvmar, M. H., Zhang, Y., Lavina, B., Fruttiger, M., et al. (2015). 'cKit lineage hemogenic endothelium-derived cells contribute to mesenteric lymphatic vessels. Cell Rep. 10, 1708–1721. doi:10.1016/j.celrep.2015.02.026
Stone, O. A., and Stainier, D. Y. R. (2019). 'Paraxial mesoderm is the major source of lymphatic endothelium. Dev. Cell 50, 247–255.e3. doi:10.1016/j.devcel.2019.04.034
Suarez, I., and Schulte-Merker, S. (2021). 'Cells with many talents: Lymphatic endothelial cells in the brain meninges. Cells 10, 799. doi:10.3390/cells10040799
Tai-Nagara, I., Hasumi, Y., Kusumoto, D., Hasumi, H., Okabe, K., Ando, T., et al. (2020). 'Blood and lymphatic systems are segregated by the FLCN tumor suppressor. Nat. Commun. 11, 6314. doi:10.1038/s41467-020-20156-6
Tammela, T., and Alitalo, K. (2010). 'Lymphangiogenesis: Molecular mechanisms and future promise. Cell 140, 460–476. doi:10.1016/j.cell.2010.01.045
Thomson, B. R., Heinen, S., Jeansson, M., Ghosh, A. K., Fatima, A., Sung, H. K., et al. (2014). 'A lymphatic defect causes ocular hypertension and glaucoma in mice. J. Clin. Investigation 124, 4320–4324. doi:10.1172/JCI77162
Tran, K. A., Zhang, X., Predescu, D., Huang, X., Machado, R. F., Gothert, J. R., et al. (2016). Endothelial beta-catenin signaling is required for maintaining adult blood-brain barrier integrity and central nervous system homeostasis. Circulation 133, 177–186. doi:10.1161/CIRCULATIONAHA.115.015982
Truong, T. N., Li, H., Hong, Y. K., and Chen, L. (2014). Novel characterization and live imaging of Schlemm's canal expressing Prox-1. Plos One 9, e98245. doi:10.1371/journal.pone.0098245
Vaahtomeri, K., Karaman, S., Makinen, T., and Alitalo, K. (2017). 'Lymphangiogenesis guidance by paracrine and pericellular factors. Genes Dev. 31, 1615–1634. doi:10.1101/gad.303776.117
van Lessen, M., Shibata-Germanos, S., van Impel, A., Hawkins, T. A., Rihel, J., and Schulte-Merker, S. (2017). 'Intracellular uptake of macromolecules by brain lymphatic endothelial cells during zebrafish embryonic development. Elife 6, e25932. doi:10.7554/eLife.25932
Venero Galanternik, M., Castranova, D., Gore, A. V., Blewett, N. H., Jung, H. M., Stratman, A. N., et al. (2017). A novel perivascular cell population in the zebrafish brain. Elife 6, e24369. doi:10.7554/eLife.24369
Wang, Y., Chaffee, T. S., LaRue, R. S., Huggins, D. N., Witschen, P. M., Ibrahim, A. M., et al. (2020a). 'Tissue-resident macrophages promote extracellular matrix homeostasis in the mammary gland stroma of nulliparous mice. Elife 9, e57438. doi:10.7554/eLife.57438
Wang, Y., Luo, M., Wang, F., Tong, Y., Li, L., Shu, Y., et al. (2022). 'AMPK induces degradation of the transcriptional repressor PROX1 impairing branched amino acid metabolism and tumourigenesis. Nat. Commun. 13, 7215. doi:10.1038/s41467-022-34747-y
Wang, Z., Liu, C. H., Huang, S., Fu, Z., Tomita, Y., Britton, W. R., et al. (2020b). 'Wnt signaling activates MFSD2A to suppress vascular endothelial transcytosis and maintain blood-retinal barrier. Sci. Adv. 6, eaba7457. doi:10.1126/sciadv.aba7457
Ward, M. C., and Cunningham, A. M. (2015). 'Developmental expression of vascular endothelial growth factor receptor 3 and vascular endothelial growth factor C in forebrain. Neuroscience 303, 544–557. doi:10.1016/j.neuroscience.2015.04.063
Wigle, J. T., and Oliver, G. (1999). 'Prox1 function is required for the development of the murine lymphatic system. Cell 98, 769–778. doi:10.1016/s0092-8674(00)81511-1
Wong, B. W., Wang, X., Zecchin, A., Thienpont, B., Cornelissen, I., Kalucka, J., et al. (2017). The role of fatty acid beta-oxidation in lymphangiogenesis. Nature 542, 49–54. doi:10.1038/nature21028
Yanev, P., Poinsatte, K., Hominick, D., Khurana, N., Zuurbier, K. R., Berndt, M., et al. (2020). Impaired meningeal lymphatic vessel development worsens stroke outcome. J. Cereb. Blood Flow. Metab. 40, 263–275. doi:10.1177/0271678X18822921
Yang, J. M., Park, C. S., Kim, S. H., Noh, T. W., Kim, J. H., Park, S., et al. (2020). 'Dll4 suppresses transcytosis for arterial blood-retinal barrier homeostasis. Circ. Res. 126, 767–783. doi:10.1161/CIRCRESAHA.119.316476
Yaniv, K., Isogai, S., Castranova, D., Dye, L., Hitomi, J., and Weinstein, B. M. (2006). Live imaging of lymphatic development in the zebrafish. Nat. Med. 12, 711–716. doi:10.1038/nm1427
Yoo, H., Lee, Y. J., Park, C., Son, D., Choi, D. Y., Park, J. H., et al. (2020). Epigenetic priming by Dot1l in lymphatic endothelial progenitors ensures normal lymphatic development and function. Cell Death Dis. 11, 14. doi:10.1038/s41419-019-2201-1
Zhao, Z., Nelson, A. R., Betsholtz, C., and Zlokovic, B. V. (2015). Establishment and dysfunction of the blood-brain barrier. Cell 163, 1064–1078. doi:10.1016/j.cell.2015.10.067
Zheng, W., Tammela, T., Yamamoto, M., Anisimov, A., Holopainen, T., Kaijalainen, S., et al. (2011). Notch restricts lymphatic vessel sprouting induced by vascular endothelial growth factor. Blood 118, 1154–1162. doi:10.1182/blood-2010-11-317800
Keywords: blood-brain barrier, central nervous system, Prox1, meningeal lymphatics, lymphatic vessels, hybrid vessels
Citation: González-Hernández S and Mukouyama Y-s (2023) Lymphatic vasculature in the central nervous system. Front. Cell Dev. Biol. 11:1150775. doi: 10.3389/fcell.2023.1150775
Received: 24 January 2023; Accepted: 28 March 2023;
Published: 07 April 2023.
Edited by:
Tsutomu Kume, Northwestern University, United StatesReviewed by:
Benjamin Thomson, Northwestern University, United StatesYoung-Kwon Hong, University of Southern California, United States
Copyright © 2023 González-Hernández and Mukouyama. This is an open-access article distributed under the terms of the Creative Commons Attribution License (CC BY). The use, distribution or reproduction in other forums is permitted, provided the original author(s) and the copyright owner(s) are credited and that the original publication in this journal is cited, in accordance with accepted academic practice. No use, distribution or reproduction is permitted which does not comply with these terms.
*Correspondence: Yoh-suke Mukouyama, bXVrb3lhbWF5QG1haWwubmloLmdvdg==