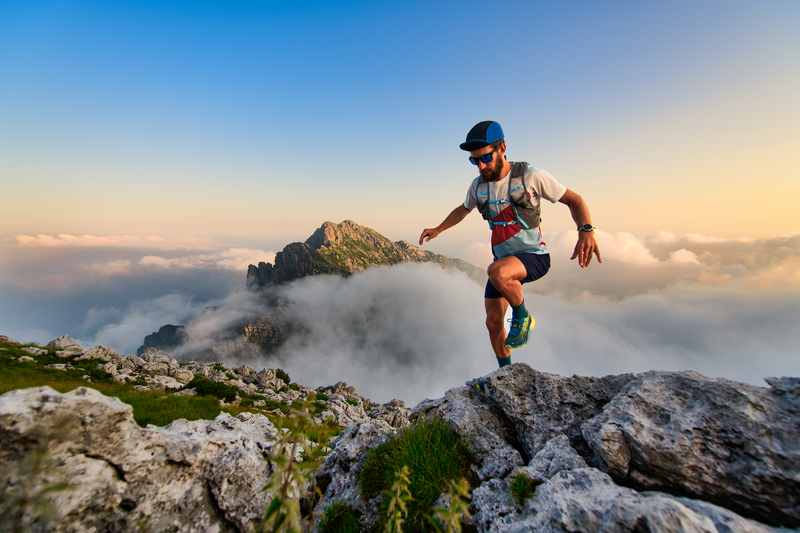
95% of researchers rate our articles as excellent or good
Learn more about the work of our research integrity team to safeguard the quality of each article we publish.
Find out more
REVIEW article
Front. Cell Dev. Biol. , 05 April 2023
Sec. Cancer Cell Biology
Volume 11 - 2023 | https://doi.org/10.3389/fcell.2023.1129343
This article is part of the Research Topic Modulation of T-cell Function and Survival by the Tumor Microenvironment View all 10 articles
Myeloid-derived suppressor cells (MDSCs) are pathologically activated neutrophils and monocytes that negatively regulate the immune response to cancer and chronic infections. Abnormal myelopoiesis and pathological activation of myeloid cells generate this heterogeneous population of myeloid-derived suppressor cells. They are characterized by their distinct transcription, phenotypic, biochemical, and functional features. In the tumor microenvironment (TME), myeloid-derived suppressor cells represent an important class of immunosuppressive cells that correlate with tumor burden, stage, and a poor prognosis. Myeloid-derived suppressor cells exert a strong immunosuppressive effect on T-cells (and a broad range of other immune cells), by blocking lymphocyte homing, increasing production of reactive oxygen and nitrogen species, promoting secretion of various cytokines, chemokines, and immune regulatory molecules, stimulation of other immunosuppressive cells, depletion of various metabolites, and upregulation of immune checkpoint molecules. Additionally, the heterogeneity of myeloid-derived suppressor cells in cancer makes their identification challenging. Overall, they serve as a major obstacle for many cancer immunotherapies and targeting them could be a favorable strategy to improve the effectiveness of immunotherapeutic interventions. However, in hematological malignancies, particularly B-cell malignancies, the clinical outcomes of targeting these myeloid-derived suppressor cells is a field that is still to be explored. This review summarizes the complex biology of myeloid-derived suppressor cells with an emphasis on the immunosuppressive pathways used by myeloid-derived suppressor cells to modulate T-cell function in hematological malignancies. In addition, we describe the challenges, therapeutic strategies, and clinical relevance of targeting myeloid-derived suppressor cells in these diseases.
Myeloid cells are highly diverse cells that are essential for the efficient functioning of innate and adaptive immunity. These cells are divided into two categories (a) monocytic myeloid cells which include monocytes, macrophages, and dendritic cells (DC), and (b) granulocytic/polymorphonuclear (PMN) myeloid cells including neutrophils, eosinophils, basophils, and mast cells. Under steady-state conditions, hematopoiesis is a well-regulated process that includes a series of cell lineage commitments, defined steps of cell differentiation (dedicated transition of hematopoietic stem cells to lymphoid cells/myeloid cells), and lastly, maturation/circulation of immune cells. During stress/pathological conditions such as cancer or infection, certain signals derived from the hematopoietic stem cell (HSC) niche modify the fate of HSCs, known as “emergency hematopoiesis”. This emergency hematopoiesis results in increased demand for both lymphoid and myeloid cells. The persistence of emergency myelopoiesis results in the accumulation of immature myeloid cells/neutrophils/monocytes which are characterized by their immune suppressor activity, now known as myeloid-derived suppressor cells (MDSCs). The immunosuppressive activity of neutrophils and monocytes was first reported around 30 years ago and these immunosuppressive cells were later named MDSCs (Gabrilovich et al., 2007). In healthy individuals, MDSCs are present in very low numbers and are involved in regulating immune responses and tissue repair (Gabrilovich and Nagaraj, 2009). However, MDSCs rapidly expand attesting to their critical role during pathological conditions such as chronic infections, autoimmune disorders, and cancer.
Given the adverse effects of MDSCs, the question arises as to why this population is evolutionary conserved. The first report by Köstlin et al. (2017) reported the expansion of polymorphonuclear MDSCs (PMN-MDSCs) during human pregnancy and the modulation of T-cell response. This provides maternal tolerance for the allogenic fetus. During pregnancy PMN-MDSCs accumulate in the peripheral blood of the mother and the human placenta/cord blood of the newborns. However, after giving birth, the mother’s MDSCs revert to their normal levels with the polarization of T-cell association with MDSCs (Köstlin et al., 2016). Additionally, Ostrand-Rosenberg and Fenselau (2018) found that MDSCs serve as a frontline barrier (in placenta) and facilitate maternal-fetal tolerance. These studies attest to the fact that MDSC’s play an important role in pregnancy and neonates (Veglia et al., 2018; Dorhoi et al., 2020).
On the other hand, activated MDSCs participate in several aspects of angiogenesis, tumor growth and metastasis, premetastatic niche formation, and epithelial-mesenchymal transition (EMT). The tumor microenvironment (TME) plays a critical role in supporting the growth and differentiation of MDSCs. The TME is a complex network of cellular and non-cellular components around the tumor cells which consists of lymphocytes, other immune cells, stromal cells, and extracellular matrix (ECM). The TME provides a permissive environment for tumor growth, invasion, and metastasis. The composition of TME in B-cell malignancies in divided into two parts: The immune microenvironment and the non-immune microenvironment (discussed below in section 4.1). The bidirectional interaction of MDSCs and tumor cells creates a haven in TME for the tumor growth. Accumulating evidence supports a pivotal role of MDSCs in cancer progression and immune suppression. Recent studies have reported the importance of the TME and the expansion of MDSCs in the pathogenesis of B-cell lymphoma (Höpken and Rehm, 2019; Liu et al., 2021). Briefly, B-cell lymphomas are a group of hematological malignancies that are characterized by complex clinical and biological heterogeneity. Lymphomas comprises Hodgkin lymphoma (HL) and non-Hodgkin lymphoma (NHL). Further, B-cell lymphoma constitutes for almost 95% of the total NHL cases. (Ennishi et al., 2020; Sehn and Salles, 2021).
In this review, we will discuss the phenotypic characteristics of MDSC, along with their genomic and metabolic profiles. We also describe how the characteristic features of MDSCs can be potentially used for therapeutic purposes in B-cell lymphomas. However, due to the short lifespan (Condamine et al., 2014) and immune escape properties of MDSCs, the therapeutic strategies in B-cell malignancies are currently somewhat limited. Therefore, we highlight the gaps in the literature which can be explored in emerging studies that will help to limit the severity of the disease. In summary, we define the challenges and clinical relevance of targeting MDSCs in hematological malignancies.
Hematopoietic stem cells (HSPCs) reside in bone marrow and differentiate into all cell types including lymphoid cells, myeloid cells, and red blood cells (RBCs.) Common myeloid progenitor cells in bone marrow generate myeloid-derived suppressor cells (MDSCs). The generation of MDSCs is considered as a two-phased, somewhat overlapping process,—(a) expansion of myeloid cells and (b) procurement of MDSCs features by myeloid cells. Generally, MDSCs develop in bone marrow, however, their generation may also take place in other organs including spleen or liver as demonstrated in tumor bearing mice (TB mice) and in patients diagnosed with cancer (Tavukcuoglu et al., 2020).
Classically, bone marrow derived immature myeloid cells (IMCs) differentiate into granulocytes, dendritic cells and macrophages. However, during demand adapted myelopoiesis (or emergency myelopoiesis), there is robust expansion of IMCs. If the inflammation is resolved, the mechanism of natural myelopoiesis is restored. However, a persistent low strength stimulus during chronic inflammation or cancer causes substantial changes in the biology of these IMCs resulting in their accumulation. During diseased conditions like cancer, the mechanism of emergency myelopoiesis is hijacked by cancer cells, releasing cytokines, chemokines, reactive radicals, immune checkpoint population, and metabolites. These secreted factors strongly contribute to tumor niche formation and to activation of immunosuppressive mechanisms.
The development of MDSC is a complex signalling network which can be understood by dividing the process into two categories: Firstly, signals which promote the accumulation of IMCs and secondly, signals which facilitate pathological activation of IMCs (Condamine et al., 2015a). The accumulation of MDSC in tumor bearing hosts is reported in many clinical studies (Sinha et al., 2008; Porembka et al., 2012; Capietto et al., 2013; Cassetta et al., 2020). Pathological activation of MDSC arises with the persistent stimulation of myeloid cells during prolonged exposure to inflammatory molecules and myeloid growth factors. These activation signals include granulocyte colony stimulating factor (G-CSF), granulocyte-macrophage colony stimulating factor (GM-CSF), macrophage colony stimulating factor (M-CSF), IL-6, IL-1β, VEGF, adenosine, hypoxia-inducible factor (HIF1α) and many more (Gabrilovich and Nagaraj, 2009). Once activated, these MDSC actively participate in various aspects of tumor progression including tumor invasion, angiogenesis, tumor microenvironment formation and epithelial-mesenchymal transition (Karakhanova et al., 2015; Li et al., 2017; Fleming et al., 2018). Furthermore, immune cell suppression is also a defining feature of MDSCs. Despite their profound effects, the characterization standards of MDSC are not very well defined and the defining phenotypic, morphological, and functional features of MDSC are still an area of intensive research.
The major subgroups of MDSCs in humans and mice are granulocytic/polymorphonuclear MDSC (PMN-MDSCs) and monocytic MDSC (M-MDSCs). The classification of PMN-MDSC and M-MDSC is based on their origin in granulocytic and monocytic cell lineages, respectively. Bronte et al. in 2016 reported another small group of MDSC in humans termed as early-MDSC (eMDSC) (Bronte et al., 2016). However, the precise characteristic features of the three subtypes of MDSC is still unclear. The three main populations of MDSCs are found at different frequencies in various cancer subtypes and every pathological condition such as cancer, autoimmunity, and infection may affect their existence (Youn and Gabrilovich, 2010). For instance, the fate and differentiation of hematopoietic cells in the bone marrow is affected by tumor cells and is clearly distinct from the effect of infection and sepsis related myelopoiesis. Specifically, the cues that lead to the expansion and mobilization of myeloid cells specially MDSCs, namely, endotoxins, cytokines, chemokines, and hormones, play an important role to define the plasticity and longevity and cytokine profile of MDSCs.
In mice, the MDSCs were first characterized as cells expressing Gr-1 and CD11b. These markers were subsequently improved by identifying the existence of subpopulations of MDSCs, namely, PMN-MDSCs (CD11b+ Ly6G+ Ly6Clow) and M-MDSC (CD11b+ Ly6G− Ly6Chi). Ginderachter and others validated the two distinct MDSC subfractions with a detailed description of their morphological, molecular and functional complexity (Movahedi et al., 2008). These cell surface characteristics have defined an initial framework for the MDSC characterization.
With the increased relevance of MDSCs in tumor immunology, uniformity in the classification of MDSC has gained interest. This challenge was explored by the Cancer Immuno guiding Program under the umbrella of the Association of Cancer Immunotherapy to establish the foundation of MDSC phenotypic characteristics. An interlaboratory collaboration of 23 laboratories (15 Europe and 8 US) coordinated a proficiency panel program which aimed at harmonizing the accepted MDSC phenotype. The results documented high variability in MDSC phenotyping which was associated with the variance of gating strategy. Therefore, a strong recommendation to harmonize the marker combinations and gating strategies for the identification of MDSC subsets was proposed (Mandruzzato et al., 2016). This collaborative research successfully described a specific gating strategy for the identification of human MDSC through flow cytometry.
Human peripheral mononuclear blood (PBMC) contains PMN-MDSC which are defined as CD11b+ CD14− CD15+ or CD11b+ CD14− CD66b+ cells and M-MDSC defined as CD11b+ CD14+ CD15− cells. CD33, another myeloid marker can also be used instead of CD11b+, since a very few CD15+ cells are CD11b−. Three markers, namely, Lin− (CD3, CD14, CD15, CD19, CD56), HLA-DRlow and CD33+ define an immature progenitor of MDSCs. Another immature subset of MDSC is now termed early MDSCs (eMDSC) which are CD11b+ and CD33+ but CD14−/CD15−. This group of cells comprises of myeloid progenitors and precursors, representing less than 5% of the MDSC population (Bronte et al., 2016). A recent study also reported a monocytic lineage termed monocyte-like precursors of granulocytes (MLPGs) which differentiates into PMN-MDSCs (Mastio et al., 2019). However, the possibility of differentiating PMN-MDSC from precursor cells requires additional validation.
Despite specific gating criteria for the identification of MDSC (both in human and mouse), discrimination of monocytes from M-MDSC and neutrophils from PMN-MDSCs is difficult because unique phenotypic markers for MDSCs. (Bronte et al., 2016). Functional studies using MDSCs are performed on the entire cell population, therefore the precise nature of M-MDSCs and PMN-MDSCs remains unclear.
Additionally, a major challenge is also observed in recognizing MDSC in tissue samples by immunohistochemistry (IHC). Gr-1 and Ly6G markers are used for identification of MDSC in mice by IHC. However, it is impossible to differentiate between neutrophils and monocytes in frozen and paraffin-embedded tissue samples. Similarly, in human samples, distinction of MDSC from monocytes and neutrophils is difficult. Generally, CD33 is considered for the identification of MDSC in human samples but this does not allow for the discrimination of MDSC from macrophages, dendritic cells (DC) and other myeloid cells. Therefore, a combination of CD33 and S100A9 is suggested for MDSC identification as this excludes DC and macrophages. Some recent studies have also suggested lectin-type oxidized LDL receptor 1 (LOX1; encoded by ORL1 gene) as a specific marker to identify human PMN-MDSCs in peripheral blood and patients with cancer (Condamine et al., 2016; Nan et al., 2018; Kim et al., 2019a; Chai et al., 2019). Similarly, M-MDSC can be distinguished from monocytes by the low expression of MHC class II. The challenge for future studies is the identification of unique cell surface markers of MDSCs so that, their function in various diseases can be explored.
Briefly, in other cancers, evidence suggests that M-MDSCs from tumor bearing (TB) mice can differentiate into PMN-MDSCs, attesting to the transition of monocytic cells to differentiated granulocytes (Youn et al., 2012; Youn et al., 2013). Transcriptional silencing of retinoblastoma (Rb1) gene by histone deacetylase 2 (HDAC-2) has been implicated in this process. Involvement of the Rb1 gene in PMN-MDSC differentiation is also supported by recent preclinical studies in breast cancer (Casbon et al., 2015). However, only a certain percentage of M-MDSCs population can be differentiated into PMN-MDSCs. This evidence raises a discussion as to whether M-MDSCs may constitute granulocytic progenitors. The phenotype and nature of these cells was explored by Mastio and others in 2019. They clearly demonstrated the existence of monocytic precursors of granulocytes which only expanded in mouse models but also in cancer patients. The mechanistic studies showed the myeloid differentiation pathway is controlled by the downregulation of Rb1 which is an important factor for the accumulation of PMN-MDSCs. However, the exact mechanism for abnormal myelopoiesis and reason for expansion of PMN-MDSCs in cancer patients requires additional study.
Phenotypic characteristics of hematological malignancies based on similar patterns of MDSCs appear to be shared by other cancers. For instance, in patients with B cell non-Hodgkin lymphoma (B-NHL) or T-NHL, M-MDSCs were increased as compared to healthy donors, andis associated with advanced stage of lymphoma (Wang et al., 2022). M-MDSC dependent T cell suppression correlated with the overexpression of Arg-1, IL-10, PD-L1 and S100A12 (Lin et al., 2011; Tadmor et al., 2013; Khalifa et al., 2014; Wu et al., 2015; Xiu et al., 2015; Azzaoui et al., 2016). In other lymphoid malignancies an enrichment has been also described in myeloma (Görgün et al., 2013), chronic lymphoid leukemia (CLL) (Jitschin et al., 2014), DLBCL (Tadmor et al., 2013; Azzaoui et al., 2016) and T-cell lymphoma (Movahedi et al., 2008). An expanded immunosuppressive population of CD66b+CD33 low HLA-DR− cells was observed within PBMCs from patients with Hodgkin and non-Hodgkin lymphoma. This was the first report on the presence of G-MDSCs in lymphomas. The study defined the G-MDSCs as CD66b+CD33dimHLA-DR−CD11b+CD16+ (Marini et al., 2016) and co-related with unfavorable prognosis and disease aggressiveness. M- and G-MDSCs were also reported in peripheral blood of DLBCL patients. Myeloid dependent T-cell suppression was co-related with the release of IL-10 and S100A12 and increase in PD-L1 expression. In vitro experiments noted the restoration of T-cell proliferation with the depletion of the M-MDSC population (Azzaoui et al., 2016). In multiple myeloma, MDSCs showed a strong tumor promoting and immune-suppressive activity. Both, M- and G-MDSCs were significantly increased with high expression of ROS and ARG1 in peripheral blood and bone marrow of MM patients as compared to healthy donors (Görgün et al., 2013).
The important characteristic that defines MDSC is their suppression of immune cells including B-cells (Li et al., 2014), NK cells (Elkabets et al., 2010; Hongo et al., 2014) and T-cells. Both M-MDSC and PMN-MDSC share similar biochemical features which enables the suppression of the immune response that includes upregulation of signal transducer and activator of transcription 3 (STAT3), expression of Arginase one and S100A8/A9, and induction of ER stress. Specifically, PMN-MDSC upregulate reactive oxygen species (ROS), peroxynitrite, prostaglandin E2 (PGE2) and arginase 1. Additionally, M-MDSC upregulate nitric oxide (NO), cytokines such as IL10 and TGFβ (Figure 1), and increase expression of PD-L1 (Gabrilovich, 2017). Therefore, MDSCs in the TME strongly contribute to the formation of a pre-metastatic niche and subsequent development of metastatic lesions. MDSCs also facilitate the escape of tumor cells from the primary site by suppressing the immune system via multiple signaling pathways which directly corelate to poor patient prognosis. Therefore, MDSC-directed cancer therapies usher in a new era of research for establishing novel anticancer therapies.
FIGURE 1. MDSCs suppressive mechanism targets both innate and adaptive immunity 1. Targeting T cell and NK cell function by TGFβ/IL10 induced inhibition. Also, MDSCs release ROS, H2O2 and peroxynitrite which dysregulate the TCR Z chain inhibiting T-cell/MDSC interaction 2. MDSCs maintain a pre-metastatic niche inhibiting T-cell migration, 3. MDSCs interact with Tregs via CCL4/5 and CD40 that recruitments them to the TME, 4. MDSCs induce a M2 macrophage phenotype by secretion of IL-10, thereby promoting immune escape for tumor cells. Furthermore, secretion of factors like IL-10 and TGF-b, and reduction of L-arginine by MDSCs induce Treg polarization. (iNOS-Inducible nitric oxide synthase, Arg- Arginase, PD-L1- Programmed death-ligand 1, TGFβ- Transforming growth factor beta, IL-10- Interleukin 10, 1L-6- Interleukin 6,CCL-2- C-C Motif Chemokine Ligand 2, CCL4- C-C Motif Chemokine Ligand 2, ADAM17-a disintegrin and metalloprotease domain).
MDSCs affect T-cell function by depleting the fundamental amino acids such as L-arginine and cysteine. Also, MDSC drive ROS production by increasing NADPH oxidase activity. Studies have found that administration of ROS inhibitors effectively regains T-cell activation (Corzo et al., 2009; Wei et al., 2015). The VEGF receptors on MDSC also support expansion and recruitment into theTME (Kusmartsev et al., 2008; Corzo et al., 2009). Other MDSC receptors such as adisintegrinandmetalloproteinase17 (ADAM17), downregulate L-selectin levels on CD4/CD8 T-cells preventing T-cell homing (Hanson et al., 2009). MDSCs have also been shown to increase PD-L1 expression which downregulates T cell mediated antitumor reactivity (Weber et al., 2018). Fuse et al. (2016) effectively blocked PD-L1 and reported a significant decrease in MDSC suppressing T-cell activity (Figure 1). MDSCs produce CCR5 ligands (CCL4 and CCL5) which recruit Tregs and promote immune escape for tumors (Figure 1). Schlecker et al. (2012) have shown that intratumoral injection of CCL4/CCL5 increased tumor infiltration of Treg cells (Figure 1). Similarly, lack of CCR5 reduced the recruitment of Tregs into the TME.
In 2015, Crook and others reported for the first time B-cell inhibition by MDSCs in a murine model of rheumatoid arthritis (Crook et al., 2015). Despite extensive research, limited studies attest to MDSC and B cell crosstalk. In human PBMC, PMN-MDSCs were shown to modulate B-cell function by suppressing cell proliferation. It was further demonstrated that arginase-1, NO, ROS were involved in the suppression of B-cells (Lelis et al., 2017). A novel finding revealed an interaction between MDSCs and B-cells in tumor bearing mice. It was observed that MDSCs accumulated in the germinal center of the spleens of tumor bearing mice and co-localized with B-cells. In vitro co-culture of MDSCs with B-cells promoted proliferation and differentiation of B-cells into plasma cells producing IgA. Further experimental validations suggested that IL-10 and TGF-β1 play an important role in promoting MDSCs mediated IgA production (Xu et al., 2017a).
Wang et al. (2018) and others found that MDSCs suppress B cell function in a murine lung cancer model. It was reported that B-cells were significantly reduced in the bone marrowand spleen of tumor bearing mice. Mechanistic studies revealed that IL-7 and STAT5, key regulators for B cell commitment, were also downregulated. The B-cell impairment was positively co-related with MDSC infiltration and cancer prognosis. Furthermore, IgG levels were decreased with B-cell dysfunction. MDSCs suppressed B-cell proliferation in an arginase-dependent manner, indicating cell-cell interactions between MDSCs and B-cells.
MDSCs are further known to inhibit NK cell cytotoxicity. Expression of indoleamine 2,3-dioxygenase (IDO) expression by MDSCs reduces tryptophan concentration in the TME. This not only stimulates Treg cells (Arandi et al., 2018) but also induces apoptosis of NK cells (Fang et al., 2022). Murine studies have reported MDSC related inhibition of NK cells mediated by TGFβ (Li et al., 2012). Membrane bound TGFβ was shown to impair NK cell cytotoxic activity, reduce NKG2D expression and decrease INF-γ production (Li et al., 2009). Ligands such as IL-33 (Fournié and Poupot, 2018; Shen et al., 2018)- produced by epithelial andendothelial cells and IL-1β (Elkabets et al., 2010) expressed by MDSCs have greater inhibitory properties for NK cells than other immune cells. In humans, MDSC inhibit the effects on INF-γ via NКp30-dependent mechanism (Hoechst et al., 2009) and interfere with NK FcR-mediated cytotoxicity which impairs NK cytotoxic and cytokine production (Stiff et al., 2018).
INF-γ and other molecules present in the TME promote MDSC expansion which results in the release of IL-10. The increased amount of IL-10, an anti-inflammatory molecule, inhibits the release of inflammatory cytokines thereby promoting a pro-tumorigenic microenvironment. The IL-10 mechanism strongly suppresses NK-cell and CD8+ T-lymphocyte activation (Figure 1) (Ibrahim et al., 2018; Yaseen et al., 2020). Additionally, NO production by MDSCs also arrests NK function and the secretion of INF-γ, granzyme B and TNF-α (Zhang et al., 2018). MDSCs produce IDO, which impairs development and activation of NK cells via STAT3 and TGF-β signaling (Sun et al., 2013; Sui et al., 2014). On the other hand, in-vitro studies on murine NK cells have shown Jak-3 inhibition and activation of STAT5 when co-cultured with MDSC (Hoechst et al., 2009). Furthermore, other NK-MDSCs cells interactions include TIGIT-CD155 (Sarhan et al., 2016) interactions and IL-1R8 (Molgora et al., 2017) signaling induced by interactions with MDSCs.
MDSCs also contribute to the progression of primary tumors by promoting angiogenesis and metastasis. Yang et al. (2004) demonstrated a tumor-promoting role for Gr+CD11b+ cells in tumor bearing animals. Co-injection of tumor cells with Gr+CD11b+ cells increased angiogenesis, reduced tumor necrosis and enhanced tumor growth. It was also reported that MMP9 produced by Gr+CD11b+ cells contributes to tumor angiogenesis and growth. In another study, MDSCs isolated from mouse tumors displayed STAT3 activation and induced angiogenesis by expressing VEGF (Kujawski et al., 2008). MDSCs also promote a mesenchymal phenotype in tumor cells by secreting several inflammatory factors such as TGF-β, IL-6, IL1-β and Hepatocyte Growth Factor (HGF) (Figure 1). These factors reduce E-cadherin expression in tumor cells which drives epithelial-mesenchymal transition (Toh et al., 2011; Ouzounova et al., 2017; Pastaki Khoshbin et al., 2019).
Studies in the literature have reported a critical role for MDSCs in establishing a pre-metastatic niche. Generally, primary tumors release signaling molecules before the onset of metastasis to regulate secondary tumor formation and recruitment of cells including neutrophils, macrophages, Treg and MDSCs. These cells communicate via signals that prepare healthy tissues at secondary sites to act as “soil” that encourage the inhabitation of circulating tumor cells (CTCs) (Peinado et al., 2017; Nasrollahzadeh et al., 2020; Wu et al., 2020). It has been reported that VEGFR1+ hematopoietic bone marrow progenitors promote the formation of a pre-metastatic niche that supports tumor metastasis. VEGFR1+ cells express VLA-4 (also known as integrin alpha4beta1) which can act in concert to promote a supportive niche for tumor growth (Kaplan et al., 2005). A study by Yan et al. (2010) reported an increased population of Gr-1+CD11b+ cells (immature myeloid cells) in the lungs of mice bearing mammary adenocarcinoma. The immature myeloid cells in the premetastatic lungs showed significant decrease in INF-gamma production with increased production of proinflammatory cytokines. Also, these cells expressed high levels of matrix metalloproteinase 9 (MMP9) and promoted vascular remodeling. The deletion of MMP9 diminished lung metastasis. Further studies have shown MDSC derived exosomes, containing TGF- β, VEGF and S100A8/A9, significantly induced metastasis, immunosuppression and promotes PMN-MDSC expansion (Wang et al., 2019a; Hsu et al., 2019).
The MDSCs genotype has benn widely studied in several cancers, namely, breast cancer, colorectal cancer, lung adenocarcinoma, etc. However, research on hematological malignancies is much more limited as indicated by gaps in the literature. For instance, an RNA sequencing analysis on non-small cell lung carcinoma (NSCLC) patients by Mastio and others defined of M-MDSCs and monocytes with distinct gene signature profiles. The gene expression profile of HLA-DR−/LOW CD14+ CD15− showed 619 differentially expressed genes as compared to monocytes. A notable upregulation of neutrophil-associated genes was observed including IL-8, MNDA (Myeloid cell nuclear differentiation antigen), CSF3R (G-CSF receptor), LYZ (lysozyme), NCF4 and NCF2 (neutrophil cytosolic factors 4 and 2). Additionally, downregulation of CSF1R (M-CSF receptor), NR4A1 (Nur77), CXCR1, ITGA4 (CD49d) and CD52 was observed (Table 1) (Mastio et al., 2019). The higher expression of neutrophil function genes in HLA-DR−/LOW CD14+ CD15− cells suggests that these cells may have neutrophil precursors. Further extensive analysis showed an upregulation of the CXCR1 gene, which is a chemokine receptor on neutrophils and is not associated with monocytes. Experimental validation on prostate cancer in the same study showed that 20% of M-MDSCs were CXCR1 positive and had potent immunosuppressive activity. Furthermore, these CXCR1+ M-MDSCs not only had the ability to differentiate into neutrophils but also shared features of macrophages (Mastio et al., 2019). This study therefore strongly attests to the enrichment of MLPGs (MDSC originating from M-MDSC and differentiating into PMN-MDSC) in humans.
Another study on pancreatic ductal carcinoma (PDAC) showed distinct cytological features (small size) and T cell suppressive function in M- MDSCs. Genome wide mRNA expression profile on M-MDSCs from three suppressive and four non-suppressive PDAC patients demonstrated an overall cancer-related gene signature including increased TNFα signaling via NF-κB, inflammatory response, IL6 JAK/STAT3 signaling and apoptosis categories. The suppressive CD14+ cells showed higher expression of FBN2, TSPAN16, LEPR, CLTA and CD163 which are genes associated with classic monocytes and differential expression patterns of MAP3K3, PRKRA, JAK2, as well as differential expression of components of the STAT family (STAT1, STAT2, STAT3, STAT5A, STAT5B and STAT6) (Trovato et al., 2019). This study also reported upregulation of metabolic genes linked to immunosuppression including fatty acid and lipoprotein metabolism-related genes, namely, CD36, LYPLA1 and CERS5, ATP and glucose metabolism genes such as ATP51C, ATP5G2, SDHB and PDK4, GXYLT1. Additionally, the BM-MDSCs and cancer patient immunosuppressive M-MDSCs shared a common gene signature profile, i.e., non-differentially expressed genes such as PTGS2, TNF, CD38, ARG1, AKT3, JAK1, JAK3, STAT1, STAT4, STAT5, STAT6 and STAT3, suggesting a common regulatory mechanism among the myeloid cells. Briefly, this study states an important immunosuppressive phenotype of M-MDSCs (Table 1).
Other studies have also reported CD33 as a specific marker expressed on MDSCs (Ortiz et al., 2015), which is one of the potential targets for cancer therapies (Jitschin et al., 2018). Other genes associated with gene ontology (GO) analysis included chemokine receptor 2 (CXCR2) (Veglia et al., 2019; Ugolini et al., 2020), colony Stimulating Factor 1 Receptor (CSF1R) (Zhou et al., 2018), and C-C Motif chemokine receptor 1(CCR1) (Cai et al., 2017), suggesting that MDSCs might communicate with recruitment signals from other sites of inflammation including the primary tumor or metastatic sites. This study also validated MDSC gene signatures from spleens of healthy and tumor bearing mice the upregulation of CD11B + Gr1+ cells (Alshetaiwi et al., 2020).
Leader et al. applied scRNA to corelate transcriptome profiles of tumor early-stage NSCLC and normal tissues from early-stage NSCLC. The interrogation into immune ecosystem revealed a remarkable heterogeneity and plasticity in the myeloid cells. According to analyses, M-MDSCs trajectory analysis M-MDSCs and PMN-MDSCs transitioned along the monocyte-to-M2 macrophage path. However, MDSCs subsets did not show any root or branch-level enrichment. Gene expression analysis of M-MDSCs and PMN-MDSCs showed a distinct gene signatures of IL-10, CD14, VEGFA and IL-6, OLR1, TGFβ1, respectively (Leader et al., 2021).
Studies in the literature attest to distinct transcriptome profiles of MDSCs with the upregulation of genes characterized by immunosuppression, high transcriptional activity, and increased pro-inflammatory pathways. These hallmark genes can be further explored in hematological malignancies and their functions can be studied. However, we also face challenges to clearly distinguish MDSCs from monocytes and neutrophils. Therefore, a more comprehensive analysis the gene expression signatures of the MDSCs subtypes will create new opportunities for selective targeting MDSCs populations and thereby designing new therapies.
Mostexperimental and clinical studies on solid tumors have reported dysregulation of MDSCs However, recent studies have defined their role in immune dysregulation in hematologic malignancies, immune-mediated cytopenias and allogeneic hemopoietic stem cell transplantation. Also, MDSCs potential role as biomarkers and therapeutic targets have started gaining attention in B-cell malignancies. The signaling pathways associated with the differentiation, expansion, circulation, and function of MDSCs, as well as the crosstalk between MDSCs and tumor cells make therapeutic strategies challenging. This section will summarize the research studies on the MDSCs in hematological malignancies and their tumor microenvironment.
The TME of B-cell lymphomas is highly variable, which consists of immune cells, stromal cells, blood vessels and extracellular matrix. Genetic aberrations harbored by tumor cells, their proliferation, increased angiogenesis, and an immune response by the host, contributes to the complex spatial cellular distribution of TME. Therefore, crosstalk between the TME elements is distinct in different types of lymphoma. Lymphomas can have variable amounts of tumor cell content, which ranges from a minimum of ∼1% in Hodgkin’s lymphoma to a maximum of 90+ % in Burkitt’s lymphoma (BL). Generally, the TME in hematological malignancies can be divided into three models based on the interaction between tumor cells and the TME: 1. ‘recruitment’, 2. ‘re-education,’ and 3. ‘effacement.’
Briefly, the ‘re-education’ pattern is commonly seen in FL, CLL, MM, and MALT lymphomas, which primarily affect lymph nodes. In this pattern, the malignant cells have a substantial degree of dependency on the TME for survival. Proliferation of lymphoma cells is partially supported by external stimuli in the TME, such as cytokines, chemokines, growth factors and signaling via stromal cells. The spatial arrangement of the tumor tissue is very similar to the normal lymphoid tissue, comprising of residual reactive germinal centers with distinct follicular dendritic cell (FDC) meshworks and follicular T-helper cells (TFH cells) (Scott and Gascoyne, 2014; Nathan et al., 2016).The second pattern is one of ‘recruitment’ and is typically observed in classical Hodgkin’s lymphoma (CHL). Here, the tumor comprises of a supportive non-malignant milieu which surrounds the infrequent malignant Hodgkin Reed-Sternberg cells (HRS cells). In this pattern, the tumor cells rely completely on the TME for survival and growth (Scott and Gascoyne, 2014; Nathan et al., 2016). Lastly, the third pattern is ‘effacement’ and includes BL where malignant cells express genetic aberrations such as MYC translocations and cooperative mutations (Schmitz et al., 2012). These changes remove the dependency of the malignant cell on the microenvironment for growth and proliferation, causing cell-autonomous survival (Scott and Gascoyne, 2014; Nathan et al., 2016).
As discussed earlier, the composition of TME in B-cell malignancies in divided into two parts: Immune microenvironment and non-immune microenvironment. The immune microenvironment consists of immune cells including T and B lymphocytes, MDSCs, tumor-associated macrophages (TAMs), tumor associated neutrophils (TANs), NK cells, dendritic cells, and many other cells. Furthermore, the non-immune microenvironment mostly includes fibroblasts, stromal cells, secretory molecules including growth factors, exosomes, cytokines, and chemokines.
TAMs are the most intensive immunosuppressive cells in TME. These cells inhibits T-cell activation and recruits at the tumor site by secreting cytokines, chemokines and other factors (Pathria et al., 2019). Based on immunomodulatory functions, TAMs are classified into M1 and M2 phenotypes. M1 macrophages are cytotoxic and play a critical role in tumor development. However, during the process of tumor growth M1-like macrophages are transformed into M2-like macrophages (Mantovani et al., 2017). M2-like macrophages produce cytokines and growth factors which contribute to tumor development, angiogenesis, inflammation and immunosuppression (Wang et al., 2019b) (Figure 1). As discussed in previous sections, the TME cells express specific cell surface markers which is associated with poor prognosis in B cell lymphoma patients. The cytokines and chemokines secreted by TME cells creates an immunosuppressive environment which modulates the activity of immune cells such as suppression of T cell function. These cytokines are divided into two groups. The first group includes VEGF, granulocyte-macrophage colony-stimulating factor (GM-CSF), macrophage colony-stimulating factor (M-CSF) and granulocyte colony-stimulating factor (G-CSF).Similarly, the second group of cytokines includes interferon-γ (INF- γ), IL-6, IL-4, IL13, high-mobility group box 1 (HMGB1), and tumor necrosis factor (TNF) (Sevko et al., 2013; Condamine et al., 2015b).
A direct relationship between MDSC and TME is reported in many cancers including prostate, breast, colon, melanoma and in B-cell malignancies. As an important immunosuppressive cell, MDSCs effectively suppress T-cell, B-cell, and NK cell activation, and recruits Tregs at the site of tumor. Therefore, tumor cells escape by the immune surveillance and increases tumor growth and metastasis. A recent report demonstrated the secretion of exosomes in TME. These tumor derived exosomes accelerate the activation and expansion of MDSCs (Tian et al., 2019) delivering molecules and proteins such as miRNAs (Ren et al., 2019), IL-10, 1L-16, PEG2 and TGF-β.
A preclinical study on murine A20 B-cell lymphoma model, Serafini and others identified a population of cells with a unique phenotype. These cells showed expression of Gr1, F4/80 and IL-4Rα, with low expression of MHC class I and II molecules. In subsequent studies, the population was confirmed to be MDSCs, and these cells suppressed CD8+ T cell activity and induced recruitment and expansion of Treg cells (Serafini et al., 2008) (Figure 1). The immunosuppressive activity of MDSCs was inhibited by lenalidomide, in that the drug reduced the frequency of MDSCs in A20 lymphoma mice. (Sakamaki et al., 2014). Furthermore, inflammatory changes promoted infiltration of MDSCs in the spleens of EL4-luc2 lymphoma mice model. These mice demonstrated elevated numbers of neutrophils resulting in abnormal myelopoiesis, which stimulated MDSCs thereby supporting tumor development (Abedi-Valugerdi et al., 2016). In murine B- cell lymphoma, the expression of mir-30 further promoted the development of MDSCs. Activation of JAK/STAT3 signaling pathway by miR-30 decreased the expression of suppressor of cytokine signaling-3 (SOCS3), thereby promoting differentiation of MDSCs and releasing immunosuppressive factors such as ARG-1, IL-10 and ROS (Xu et al., 2017b). To confirm increased levels of PDL-1 expression and expansion of MDSCs (at the tumor site) in DLBCL patients, PDL-1 expression was evaluated in an A20 B-cell lymphoma mouse model. It was observed that anti-PDL-1 treatment decreased MDSCs at the tumor site (Lu et al., 2021). Further, another study identified calmodulin kinase 2 (Camkk2) as an important target responsible for the accumulation and expansion of MDSCs in, E.G.,7-OVA TB mice (a mouse lymphoma model derived by electroporating T lymphoblast, EL4 cells). Knockdown of Camkk2 in mice decreased the accumulation of MDSCs, enhanced the anti-tumor response of T-cells and slowed tumor growth. Tumor growth was restored in Camkk2−/− mice when transplanted with MDSCs. This study confirmed the immune suppressive function of MDSCs and potential role of Camkk2 in targeting MDSCs expansion (Huang et al., 2021). Also, M-MDSC accumulation via an IL-35 mechanism was significantly decreased with the anti-IL-35 treatment in the Ly8DLBCL murine model (Wang et al., 2021a).
Clinically, Romano et al. (2015) reported an increase in M-MDSCs, PMN-MDSCs and CD34+ MDSCs in peripheral blood of Hodgkin’s lymphoma (HL) patients as compared to healthy individuals. It was observed that the levels of MDSCs were much lower in complete remission patients when compared to the patients who did not have a complete remission after chemotherapy. Amini et al. (2019) also observed increased numbers of PMN-MDSCs in the peripheral blood of 19 HL patients when compared to healthy individuals. A similar study found that PMN-MDSCs (CD66b+ CD33dim HLADR−) were higher in 124 B-cell lymphoma patients which included HL and B-NHL. The study found that depletion of CD66b+ MDSCs restored the T-cell response and proliferation (Marini et al., 2016). Furthermore, in DLBCL patients, there was a significant increase in MDSCs in the peripheral blood and this was a poor prognostic factor. However, only the increased number of M-MDSCs correlated with the international prognostic index (IPI), high numbers of circulating Treg cells and elevated expression of IL10, PDL-1 and S100A12. These factors were associated with the immunosuppressive mechanism of MDSCs, and inhibition of IL-10, PDL-1 and S100A12 increased T-cell activation and proliferation (Azzaoui et al., 2016). Some recent studies have reported that high levels of M-MDSCs in newly diagnosed and relapsed patients correlated with tumor progression and patient survival. Additionally, a clinical trial with lenalidomide and R-GDP (Rituximab plus gemcitabine, cisplatin and dexamethasone) chemotherapy in relapsed DLBCL patients showed decreased levels of MDSCs and Treg cells in individuals with a favorable 24-month survival rate. This study also demonstrated that Vit-D deficient DLBCL patients had increased numbers of MDSCs and Treg cells, suggesting that an enhanced treatment outcomes could be achieved by Vit-D supplementation in DLBCL-patients (Jiménez-Cortegana et al., 2021a; Jiménez-Cortegana et al., 2021b). Additionally, patients with NK/T-cell lymphoma (ENKL) had high MDSCs levels and specially M-MDSCs were responsible for a poorer disease-free survival and overall survival. Studies have found that IL-17 produced by CD4+Th17 cells in ENKL patients increases MDSC expansion and inhibits T cell proliferation (Zhang et al., 2015). The effect of MDSCs on other types of NHL including cell lymphoma and follicular lymphoma is still unexplored.
MDSCs expansion in bone marrow was observed during early stages of multiple myeloma (MM) in the 5TMM mouse model and in later stages, MDSCs could be detected in peripheral blood. Also, myeloid leukemia cell differentiation protein (Mcl-1) was reported as a potential target promoting MDSCs survival. In vitro studies have shown that mesenchymal stem cell (BMSC)- derived exosomes induced the survival of MM MDSCs and increased NO production in MDSCs by activating STAT3 and STAT1 signaling. These bone marrow-derived mesenchymal stem/stromal cells (BMSC) also increased the expression of anti-apoptotic proteins, namely, Bcl-xL and Mcl-1 suppressing T-cell activation and contributing to bortezomib and melphalan resistance (Wang et al., 2015a; Veirman et al., 2015; De Veirman et al., 2019). A mechanistic study reported the presence of S100A9 and its receptor TLR4 in MM MDSCs model mice which triggered MDSCs expansion and secreted inflammatory molecules such as TNF- α, IL-6 and IL-10 (Figure 1). However, blocking S100A9 did not affect the MDSCs expansion but rather decreased the cytokine production (De Veirman et al., 2017). Further, in a clinical study, a pro-tumorigenic affect of MM derived Galectin-1 was observed on the expansion of M-MDSCs with CD304 interaction, thereby facilitating MM progression following autologous stem cell transplant (ASCT) (Lim et al., 2021). These studies attest that MDSC in the MM microenvironment play an important role in interaction between cells, cytokines and other factors present in the tumor niche which together influence MM progression.
Clinically, for the first time in 2010, Brimnes et al. (2010) reported an increase in M-MDSCs in peripheral blood of MM patients as compared to controls. In later studies Wang et al. (2015b) showed a positive correlation of M-MDSCs with MM recurrence and negative correlation with treatment outcomes. A similar study found out that neutrophils from bone marrow were immune suppressive and had MDSCs like activity. Furthermore, it was found that CD11b+ CD113+ CD16+ neutrophils have G-MDSCs (Perez et al., 2020) phenotype.
Several studies have reported an accumulation of G-MDSCs in the bone marrow and peripheral blood of MM patients as compared to healthy individuals and the expansion is corelated with the disease pathogenesis (Ramachandran et al., 2013; Favaloro et al., 2014; Giallongo et al., 2016). In context to treatment with bortezomib, lenalidomide (immunomodulatory agent) and DC vaccination there are inconsistent results on its effect on MDSCs and MM patients (Gunes et al., 2020). A study by Kuwahara-Ota et al. (2020) identified lenalidomide and pomalidomide as potential inhibitors for MDSCs induction, which downregulated CCL5 and MIF in MM patients. This study also found out that downregulation of CCL5, and induction of interferon regulatory factor 8 (IRF8) affected MDSCs expansion. Additionally, it was also observed that high amount of M-MDSCs in MM patients inhibited the cytotoxicity of pre-autologous stem cell transplantation (ASCT) and was associated with poor clinical outcome (Lee et al., 2019). A co-culture study of MPC11 cells (B lymphocyte cell line) with MDSCs of MM patients showed high proliferation of MPC11 cells and reduction in T cell immune response when compared to no MDSCs, and decitabine (DAC) treated bone marrow cells (Zhou et al., 2019).
A study by Nakamura et al. (2018) have reported the high expression of IL-18 in the bone marrow microenvironment of MM patients. It was observed that, IL-18 driven MDSCs immune suppression via C/EBβ was associated with poor prognosis. The migratory inhibitor factor in the MM bone marrow microenvironment is an important factor which leads to the expansion and differentiation of MDSCs, increases levels of PDL-1 expression on MDSCs and suppresses T-cell function (Lewinsky et al., 2021). But now we know that some MM targeting drugs such as Daratumumab (targeting CD38) successfully diminished the immune suppressive cells, namely, Tregs, Bregs and MDSCs (van de Donk and Usmani, 2018). Additionally, estrogen has also been associated with MM disease progression (Ozerova and Nefedova, 2019). Together, all the studies identified new molecules which promotes MDSC formation/expansion and targeting them could improve drug therapeutics for MM.
In earlier studies, Sun et al. (2015) found expansion of MDSCs in acute myeloid leukemia (AML) and a complete remission group of patients as compared to partial and no remission group of patients. This study also reported the overexpression of WT1 (Wilms tumor gene) gene in the bone marrow samples of AML patients which had positive correlation with MDSCs expansion. Another study identified higher amounts of M-MDSCs and e-MDSCs in the peripheral blood of AML patients (Lv et al., 2021). Further, an elevated expression of MDSCs corelated with the poor remission, higher relapse rates and reduced long term survival (Wang et al., 2020).
In AML, it was also observed the cytarabine (Ara-C)- triggered increased expression of TNFα from the AML cells resulting in the expansion and survival of the MDSC by activating IL-6/STAT3 and NFКB signaling (Bai et al., 2022). It has also been observed that the combination of Ara-C, Plerixafor (CXCR4 inhibitor) and PDL-1 in AML murine model decreased the number of Tregs and MDSCs in peripheral blood and bone marrow (Hwang et al., 2019). Additionally, MDSC expansion was observed in B-cell acute lymphoblastic leukemia (B-ALL) (Zahran et al., 2021). Hohtari et al. (2019) noted that in all the AML bone marrow (n = 52) there was a decrease in M1-type macrophages and effector T cells with an increase in M2-type macrophages and MDSCs as compared to healthy individuals. They also observed an elevation of PD-1 and CTLA-4 (negative immune check point) which was responsible for immune suppressive role in AML patients. Furthermore, in acute promyelocytic leukemia (APL) patients innate lymphoid cells (ILC2s) were increased and hyper-activated which in turn activated M-MDSCs via IL-13 secretion. After treatment with all trans retinoic acid (ATRA), the levels of PGD2, NKp30, ILC2s, IL-13 and M-MDSCs was restored (Trabanelli et al., 2017).
In chronic granulocytic leukemia (CML), an increased number of MDSCs and its immune suppressive markers such as IL10 and ARG1 was observed. Further, it was noted that imatinib and dasatinib (tyrosine kinase inhibitor) treatment reduced MDSCs numbers to the normal range (Giallongo et al., 2014; Christiansson et al., 2015). Additional studies also reported the tyrosine kinase inhibitor (TKI) treatments reduced G-MDSCs in patients, however, only dasatinib showed a significant reduction in M-MDSCs. Therefore, dasatinib can serve as an important targeting agent for M-MDSCs in chronic myeloid lymphoma (CML) patients (Giallongo et al., 2018). Also, an elevated number of M-MDSCs was observed in the peripheral blood of fifty CLL patients and was associated with poor survival (Zahran et al., 2020). It was also seen that Tregs and MDSCs can be reduced to normal range with ibrutinib treatment in 1–2 years (Solman et al., 2021). Furthermore, PMN-MDSCs showed greater immunosuppressive impact on M-MDSCs in CLL patients. However, ibrutinib successfully lowered the PMN-MDSCs, targeted MDSCs differentiation, induced naïve T cells and improve the tumor microenvironment (Ferrer et al., 2021).
The first study on MDSC expansion in myeloproliferative syndromes (MDS) was shared by Chen et al. (2013). Using multiple cell transfection models, it was noted that the expansion of MDSCs was driven by S100A9 and CD33. These two important proteins form functional ligand/receptor pairs which suppress immunoreceptor tyrosine-based inhibition motif and induce secretion of suppressive cytokines such as IL-10 and TGFβ. In MDS, MDSCs suppress T cells by recruitment and proliferation of Tregs in the bone marrow (Kittang et al., 2016). Also, Gal-9, an important ligand for immune checkpoint molecule TIM3 is highly expressed on the MDSCs of MDS patients. This Gal9 was observed to bind TIM3 on CD8+T cells, suppressing their immune function and cause T-cell exhaustion (Tao et al., 2020). Studies have also shown that ARG1, an immune suppressive molecule suppresses the ani-tumor response of CD8+ T cells via STAT3 signaling pathway (Qi et al., 2021) (Figure 1). Therefore, targeting these negatively corelated immune checkpoint molecules of MDSCs in MDS patients could suppress the activation and proliferation of MDSCs.
In previous sections we have discussed the immune suppressive and tumor promoting role of MDSCs in hematological malignancies. However, in hematological stem cell transplantation (HSCT), the relationship between graft versus leukemia (GVL), graft versus host disease (GVHD) and MDSCs is complicated (Figure 2).
FIGURE 2. Role of MDSCs in hematological malignancies and Hematopoietic stem cell transplantation. MDSCs have a favorable role in that they suppress T cell activity thereby preventing GVHD after HSCT (Left side of figure). In contrast, the unfavorable expansion of MDSCs promotes tumor growth by increasing angiogenesis, creating a pre-metastatic niche, suppressing T-cell activity and recruiting Tregs in the TME (Right side of figure).
In mice and humans, the expression of MDSCs have been detected in the peripheral blood during allogenic hematopoietic stem cell transplantation (allo-HSCT) (Luyckx et al., 2012). A clinical study by Fan et al. (2017) showed that G-CSF mobilization improved relapse free survival and GVHD in bone marrow hematopoietic transplantation (G-BM) as compared to G-PBMC transplantation. A similar study also showed that mobilization with pegylated-G-CSF in allo-HSCT elevates M-MDSCs in the donors and improved the rates of severe GVHD (Li et al., 2021).
A transcriptomic study by Andrea et al. reported that G-CSF mobilized PMN-MDSCs had upregulation of genes promoting DNA replication, cell cycle, and cell division, namely, Ki-67, topoisomerase II alpha, and cyclin B, respectively. This study showed that G-CSF-mobilized PMN-MDSCs enrichment in the graft displayed remarkable molecular characteristics with significant inhibition of donor NK cells (Pelosi et al., 2021). A retrospective study reported encouraging improvement in ani-leukemic affect of donor lymphocyte infusion (DLI) after G-CSF treatment as compared to standard DLI after relapsed allo-HCT (Schneidawind et al., 2019). Wang et al. (2021b) described that human umbical cord mesenchymal stem cells causes MDSCs expansion and prevents GVHD after HSCT by secreting CXCL1 and HLA-G (Yang et al., 2020). The mechanistic study on GVHD mice models revealed that MDSCs suppresses GVHD and preserves GVL activities by inducing NKG2D expression on T cells and suppresses GVHD by increasing Tregs (Zhang et al., 2019). It was also observed that the delay in M-MDSCs recovery and invariant natural killer cells (iNKT) after transplantation was associated with a high incidence of grade III-IV acute GVHD. However, low levels of M-MDSCs and high levels of iNKT cells significantly reduced leukemic relapse (Kim et al., 2019b). This study suggests that the balance between MDSCs and other immune cells is critical for achieving good outcomes in HSCT.
The major challenges in the field of GVHD during transfusion of immune suppressive cells is the inefficient immune system, dysregulation in growth of immune cells and the risk of infection. Pre-transplantation measures together with stem cell infusion and inflammation in the host causes M-MDSCs expansion, and this expansion of MDSCs causes non-relapse mortality (Lee et al., 2018). In recent studies treatment with cyclophosphamide (an immunosuppressant) after allo-HSCT caused early differentiation of MDSCs with reduced GVHD incidence (D'Aveni et al., 2020). It was observed that post-transplant cyclophosphamide (PTCy) patients showed healthy MDSC recovery, particularly PMN-MDSCs than standard of care (SOC) recipients, with active T-cell suppressive function (Oshrine et al., 2022). For the first time, it was also found that mitochondrial permeability transition pore (MPTP) opened in the PMN-MDSCs is due to the intense inflammatory environment of GVHD causing mitochondrial damage, oxidative stress, and apoptosis of PMN-MDSCs. By obstructing MPTP opening by cyclosporine A (CsA) (an immunosuppressant), restored the immunosuppressive function and viability of PMN-MDSCs in vitro and in vivo. Therefore, MPTP blockade by CsA can preserve/active PMN-MDSCs which can improve efficiency bone marrow transplantation. Also, restoration of immunosuppressive function of PMN-MDSCs by enhancing mitochondrial health could serve as a novel therapeutic strategy for aGVHD (Figure 2).
Cyclosporin A blocks mitochondrial permeability transition pore (MPTP) of PMN-MDSCs therefore, inhibiting MDSCs damage in GVHD inflammatory environment (Li et al., 2022). These studies strongly attest that MDSCs could enhance the function of immune suppressive drug in GVHD. Therefore, more studies are required to confirm whether MDSCs regulating GVHD incidence regulates tumor suppression and whether MDSCs can cause tumor recurrence?
Although MDSCs have a short lifespan, their continuous recruitment to the sites of inflammation enables a significantly long-lasting affect at that TME site. Also, as the life span of MDSCs in the tissue is short, it is very difficult to reverse its pathological state. Therefore, targeting MDSCs for effective therapies could be achieved by 1. Targeting MDSC recruitment, 2. MDSC depletion and 3. reprograming MDSCs to enhance antitumor immunity.
Strategies to target MDSCs in hematological malignancies have provided some initially promising results. STAT3 and cyclooxygenase 2 (COX2)/PGE2 plays an important role in MDSCs formation, differentiation, and accumulation. Veltman et al. (2010) reported ‘celecoxib’, an inhibitor of COX2, as a potential tool to improve dendritic cell based immunotherapy and can also effectively suppress MDSC function. A retrospective population-based study on DLBCL patients showed a survival benefit to COX-2 inhibitors prior to chemo-immunotherapy treatment (Smyth et al., 2020). A pre-clinical study by De Henau et al. (2016) showed that resistance to immune checkpoint blockade (ICB) is corelated with the infiltration of myeloid cells in various tumors. These findings strongly suggest the inhibition of PI3Kγ (gamma isoforms of phosphoinositide 3-kinase, which are highly expressed in myeloid cells) could be a potential target to overcome immune checkpoint blockade resistance in tumor immune landscape. Furthermore, in HL, it has been reported that RP6530, a PI3Kδ/γ inhibitor decreases the MDSC percentage, and downregulates iNOS which results in tumor regression (Locatelli et al., 2019).
Additionally, miRNAs are also known to affect MDSC function. In a B-cell lymphoma mouse model, mir-30a was upregulated M- and G-MDSCs which further increased the immunosuppressive function of MDSCs by inhibiting the expression of the suppressor of cytokine signaling (SOCS3) gene (Xu et al., 2017c). Li et al. (2020) reported R96A, ‘c-Rel’, which is a member of NF-B family, as a myeloid checkpoint for cancer immunotherapy. A deficiency of c-Rel in MDSCs was reported to inhibit cancer growth in mice which was further confirmed by pharmaceutical inhibition of c-Rel. Furthermore, a combinational therapy of c-Rel and PD1 blockade was more effective in cancer treatment than either strategy alone.
Chimeric antigen receptor (CAR) T-cell therapy, also known as a “living drug”, works on the principle of ‘engineering the patient’s T-cells for the treatment of cancer’. This treatment is routinely used in blood cancers, including lymphomas, some forms of leukemia, and, most recently, multiple myeloma (Neelapu et al., 2017; Schuster et al., 2017; Maude et al., 2018). However, Jain et al. (2021) reported that CAR T-cell failure in large B-cell lymphoma (LBCL) was associated with increased numbers of circulating M-MDSC and tumor associated IFN signaling. Some more clinical studies ongoing are listed in Table 2.
TABLE 2. Clinical trials performed worldwide for MDSCs (listed in clinicaltrials.gov).
Emerging evidence suggests a significant role for MDSCs in maintaining homeostasis in the immune system. Research studies have explored the immunosuppressive function of MDSCs and identified their pro-tumor characteristics as well as their negative prognostic impact for patients with malignant hematological diseases. In contrast, MDSCs may also have a favorable role in hematological diseases, for example, by preventing GVHD after HSCT (Figure 2). With the clear understanding of the origin, development, and differentiation of MDSCs, the genomic and metabolic mechanisms could be manipulated to optimize the relationship between MDSCs and other cells in the tumor microenvironment and utilize their function to improve patient outcomes. Aside from the role of MDSCs in suppressing the antitumor immune response in the TME, MDSCs also substantially impact the efficacy of immunotherapy, particularly CAR-T cell treatment (Lindo et al., 2021; Tumino et al., 2021). Several studies have developed ways to inhibit or remove MDSCs by modulating their differentiation (Luo et al., 2022), or by developing NK cells expressing chimeric activated receptors that eliminate MDSCs and improve the anti-tumor affects of CAR-T cells (Parihar et al., 2019). Therefore, treating hematological malignancies by targeting MDSCs will be an important future therapeutic direction. However, a critical challenge will be how best to identify and then target specific subsets of MDSC to improve the clinical outcome of patients. Clearly, substantial additional research is needed to achieve this goal.
VB and SA write the manuscript.
We thank Prithviraj Mukherjee from Mayo clinic, Division of Hematology Research for reviewing the manuscript.
The authors declare that the research was conducted in the absence of any commercial or financial relationships that could be construed as a potential conflict of interest.
All claims expressed in this article are solely those of the authors and do not necessarily represent those of their affiliated organizations, or those of the publisher, the editors and the reviewers. Any product that may be evaluated in this article, or claim that may be made by its manufacturer, is not guaranteed or endorsed by the publisher.
Abedi-Valugerdi, M., Wolfsberger, J., Pillai, P. R., Zheng, W., Sadeghi, B., Zhao, Y., et al. (2016). Suppressive effects of low-dose 5-fluorouracil, busulfan or treosulfan on the expansion of circulatory neutrophils and myeloid derived immunosuppressor cells in tumor-bearing mice. Int. Immunopharmacol. 40, 41–49. doi:10.1016/j.intimp.2016.08.023
Alshetaiwi, H., Pervolarakis, N., McIntyre, L. L., Ma, D., Nguyen, Q., Rath, J. A., et al. (2020). Defining the emergence of myeloid-derived suppressor cells in breast cancer using single-cell transcriptomics. Sci. Immunol. 5 (44), eaay6017. doi:10.1126/sciimmunol.aay6017
Amini, R. M., Enblad, G., Hollander, P., Laszlo, S., Eriksson, E., Ayoola Gustafsson, K., et al. (2019). Altered profile of immune regulatory cells in the peripheral blood of lymphoma patients. BMC Cancer 19 (1), 316. doi:10.1186/s12885-019-5529-0
Arandi, N., Ramzi, M., Safaei, F., and Monabati, A. (2018). Overexpression of indoleamine 2,3-dioxygenase correlates with regulatory T cell phenotype in acute myeloid leukemia patients with normal karyotype. Blood Res. 53 (4), 294–298. doi:10.5045/br.2018.53.4.294
Azzaoui, I., Uhel, F., Rossille, D., Pangault, C., Dulong, J., Le Priol, J., et al. (2016). T-cell defect in diffuse large B-cell lymphomas involves expansion of myeloid-derived suppressor cells. Blood 128 (8), 1081–1092. doi:10.1182/blood-2015-08-662783
Bai, H., Peng, Y., Li, Y., Duan, J., Fu, W., Liang, X., et al. (2022). Cytarabine-induced TNFα promotes the expansion and suppressive functions of myeloid-derived suppressor cells in acute myeloid leukaemia. Scand. J. Immunol. 95 (6), e13158. doi:10.1111/sji.13158
Brimnes, M. K., Vangsted, A. J., Knudsen, L. M., Gimsing, P., Gang, A. O., Johnsen, H. E., et al. (2010). Increased level of both CD4+FOXP3+ regulatory T cells and CD14+HLA-DR−/low myeloid-derived suppressor cells and decreased level of dendritic cells in patients with multiple myeloma. Scand. J. Immunol. 72 (6), 540–547. doi:10.1111/j.1365-3083.2010.02463.x
Bronte, V., Brandau, S., Chen, S. H., Colombo, M. P., Frey, A. B., Greten, T. F., et al. (2016). Recommendations for myeloid-derived suppressor cell nomenclature and characterization standards. Nat. Commun. 7, 12150. doi:10.1038/ncomms12150
Cai, T. T., Ye, S. B., Liu, Y. N., He, J., Chen, Q. Y., Mai, H. Q., et al. (2017). LMP1-mediated glycolysis induces myeloid-derived suppressor cell expansion in nasopharyngeal carcinoma. PLoS Pathog. 13 (7), e1006503. doi:10.1371/journal.ppat.1006503
Capietto, A. H., Kim, S., Sanford, D. E., Linehan, D. C., Hikida, M., Kumosaki, T., et al. (2013). Down-regulation of PLCγ2-β-catenin pathway promotes activation and expansion of myeloid-derived suppressor cells in cancer. J. Exp. Med. 210 (11), 2257–2271. doi:10.1084/jem.20130281
Casbon, A. J., Reynaud, D., Park, C., Khuc, E., Gan, D. D., Schepers, K., et al. (2015). Invasive breast cancer reprograms early myeloid differentiation in the bone marrow to generate immunosuppressive neutrophils. Proc. Natl. Acad. Sci. U. S. A. 112 (6), E566–E575. doi:10.1073/pnas.1424927112
Cassetta, L., Bruderek, K., Skrzeczynska-Moncznik, J., Osiecka, O., Hu, X., Rundgren, I. M., et al. (2020). Differential expansion of circulating human MDSC subsets in patients with cancer, infection and inflammation. J. Immunother. Cancer 8 (2), e001223. doi:10.1136/jitc-2020-001223
Chai, E., Zhang, L., and Li, C. (2019). LOX-1+ PMN-MDSC enhances immune suppression which promotes glioblastoma multiforme progression. Cancer Manag. Res. 11, 7307–7315. doi:10.2147/CMAR.S210545
Chen, X., Eksioglu, E. A., Zhou, J., Zhang, L., Djeu, J., Fortenbery, N., et al. (2013). Induction of myelodysplasia by myeloid-derived suppressor cells. J. Clin. Investigation 123 (11), 4595–4611. doi:10.1172/JCI67580
Christiansson, L., Söderlund, S., Mangsbo, S., Hjorth-Hansen, H., Höglund, M., Markevärn, B., et al. (2015). The tyrosine kinase inhibitors imatinib and dasatinib reduce myeloid suppressor cells and release effector lymphocyte responses. Mol. Cancer Ther. 14 (5), 1181–1191. doi:10.1158/1535-7163.MCT-14-0849
Condamine, F. L., Nagalingum, N. S., Marshall, C. R., and Morlon, H. (2015). Origin and diversification of living cycads: A cautionary tale on the impact of the branching process prior in bayesian molecular dating. BMC Evol. Biol. 15 (1), 65. doi:10.1186/s12862-015-0347-8
Condamine, T., Dominguez, G. A., Youn, J. I., Kossenkov, A. V., Mony, S., Alicea-Torres, K., et al. (2016). Lectin-type oxidized LDL receptor-1 distinguishes population of human polymorphonuclear myeloid-derived suppressor cells in cancer patients. Sci. Immunol. 1 (2), aaf8943. doi:10.1126/sciimmunol.aaf8943
Condamine, T., and Gabrilovich, D. I. (2011). Molecular mechanisms regulating myeloid-derived suppressor cell differentiation and function. Trends Immunol. 32 (1), 19–25. doi:10.1016/j.it.2010.10.002
Condamine, T., Kumar, V., Ramachandran, I. R., Youn, J. I., Celis, E., Finnberg, N., et al. (2014). ER stress regulates myeloid-derived suppressor cell fate through TRAIL-R-mediated apoptosis. J. Clin. Invest. 124 (6), 2626–2639. doi:10.1172/JCI74056
Condamine, T., Mastio, J., and Gabrilovich, D. I. (2015). Transcriptional regulation of myeloid-derived suppressor cells. J. Leukoc. Biol. 98 (6), 913–922. doi:10.1189/jlb.4RI0515-204R
Corzo, C. A., Cotter, M. J., Cheng, P., Cheng, F., Kusmartsev, S., Sotomayor, E., et al. (2009). Mechanism regulating reactive oxygen species in tumor-induced myeloid-derived suppressor cells. J. Immunol. 182 (9), 5693–5701. doi:10.4049/jimmunol.0900092
Crook, K. R., Jin, M., Weeks, M. F., Rampersad, R. R., Baldi, R. M., Glekas, A. S., et al. (2015). Myeloid-derived suppressor cells regulate T cell and B cell responses during autoimmune disease. J. Leukoc. Biol. 97 (3), 573–582. doi:10.1189/jlb.4A0314-139R
D'Aveni, M., Notarantonio, A. B., Bertrand, A., Boulangé, L., Pochon, C., and Rubio, M. T. (2020). Myeloid-derived suppressor cells in the context of allogeneic hematopoietic stem cell transplantation. Front. Immunol. 11, 989. doi:10.3389/fimmu.2020.00989
De Henau, O., Rausch, M., Winkler, D., Campesato, L. F., Liu, C., Cymerman, D. H., et al. (2016). Overcoming resistance to checkpoint blockade therapy by targeting PI3Kγ in myeloid cells. Nature 539 (7629), 443–447. doi:10.1038/nature20554
De Veirman, K., De Beule, N., Maes, K., Menu, E., De Bruyne, E., De Raeve, H., et al. (2017). Extracellular S100A9 protein in bone marrow supports multiple myeloma survival by stimulating angiogenesis and cytokine secretion. Cancer Immunol. Res. 5 (10), 839–846. doi:10.1158/2326-6066.CIR-17-0192
De Veirman, K., Menu, E., Maes, K., De Beule, N., De Smedt, E., Maes, A., et al. (2019). Myeloid-derived suppressor cells induce multiple myeloma cell survival by activating the AMPK pathway. Cancer Lett. 442, 233–241. doi:10.1016/j.canlet.2018.11.002
Dorhoi, A., Kotzé, L. A., Berzofsky, J. A., Sui, Y., Gabrilovich, D. I., Garg, A., et al. (2020). Therapies for tuberculosis and AIDS: Myeloid-derived suppressor cells in focus. J. Clin. Invest. 130 (6), 2789–2799. doi:10.1172/JCI136288
Elkabets, M., Ribeiro, V. S., Dinarello, C. A., Ostrand-Rosenberg, S., Di Santo, J. P., Apte, R. N., et al. (2010). IL-1β regulates a novel myeloid-derived suppressor cell subset that impairs NK cell development and function. Eur. J. Immunol. 40 (12), 3347–3357. doi:10.1002/eji.201041037
Ennishi, D., Hsi, E. D., Steidl, C., and Scott, D. W. (2020). Toward a new molecular taxonomy of diffuse large B-cell lymphoma. Cancer Discov. 10 (9), 1267–1281. doi:10.1158/2159-8290.CD-20-0174
Fan, Q., Liu, H., Liang, X., Yang, T., Fan, Z., Huang, F., et al. (2017). Superior GVHD-free, relapse-free survival for G-BM to G-PBSC grafts is associated with higher MDSCs content in allografting for patients with acute leukemia. J. Hematol. Oncol. 10 (1), 135. doi:10.1186/s13045-017-0503-2
Fang, X., Guo, L., Xing, Z., Shi, L., Liang, H., Li, A., et al. (2022). Ido1 can impair NK cells function against non-small cell lung cancer by downregulation of NKG2D Ligand via ADAM10. Pharmacol. Res. 177, 106132. doi:10.1016/j.phrs.2022.106132
Favaloro, J., Liyadipitiya, T., Brown, R., Yang, S., Suen, H., Woodland, N., et al. (2014). Myeloid derived suppressor cells are numerically, functionally and phenotypically different in patients with multiple myeloma. Leukemia Lymphoma 55 (12), 2893–2900. doi:10.3109/10428194.2014.904511
Feng, P. H., Lee, K. Y., Chang, Y. L., Chan, Y. F., Kuo, L. W., Lin, T. Y., et al. (2012). CD14(+)S100A9(+) monocytic myeloid-derived suppressor cells and their clinical relevance in non-small cell lung cancer. Am. J. Respir. Crit. Care Med. 186 (10), 1025–1036. doi:10.1164/rccm.201204-0636OC
Ferrer, G., Jung, B., Chiu, P. Y., Aslam, R., Palacios, F., Mazzarello, A. N., et al. (2021). Myeloid-derived suppressor cell subtypes differentially influence T-cell function, T-helper subset differentiation, and clinical course in CLL. Leukemia 35 (11), 3163–3175. doi:10.1038/s41375-021-01249-7
Fleming, V., Hu, X., Weber, R., Nagibin, V., Groth, C., Altevogt, P., et al. (2018). Targeting myeloid-derived suppressor cells to bypass tumor-induced immunosuppression. Front. Immunol. 9, 398. doi:10.3389/fimmu.2018.00398
Fournié, J. J., and Poupot, M. (2018). The pro-tumorigenic IL-33 involved in antitumor immunity: A yin and yang cytokine. Front. Immunol. 9, 2506. doi:10.3389/fimmu.2018.02506
Fuse, H., Tomihara, K., Heshiki, W., Yamazaki, M., Akyu-Takei, R., Tachinami, H., et al. (2016). Enhanced expression of PD-L1 in oral squamous cell carcinoma-derived CD11b(+)Gr-1(+) cells and its contribution to immunosuppressive activity. Oral Oncol. 59, 20–29. doi:10.1016/j.oraloncology.2016.05.012
Gabrilovich, D. I., Bronte, V., Chen, S. H., Colombo, M. P., Ochoa, A., Ostrand-Rosenberg, S., et al. (2007). The terminology issue for myeloid-derived suppressor cells. Cancer Res. 67 (1), 425; author reply 426. author reply 6. doi:10.1158/0008-5472.CAN-06-3037
Gabrilovich, D. I. (2017). Myeloid-derived suppressor cells. Cancer Immunol. Res. 5 (1), 3–8. doi:10.1158/2326-6066.CIR-16-0297
Gabrilovich, D. I., and Nagaraj, S. (2009). Myeloid-derived suppressor cells as regulators of the immune system. Nat. Rev. Immunol. 9 (3), 162–174. doi:10.1038/nri2506
Giallongo, C., Parrinello, N., Tibullo, D., La Cava, P., Romano, A., Chiarenza, A., et al. (2014). Myeloid derived suppressor cells (MDSCs) are increased and exert immunosuppressive activity together with polymorphonuclear leukocytes (PMNs) in chronic myeloid leukemia patients. PLOS ONE 9 (7), e101848. doi:10.1371/journal.pone.0101848
Giallongo, C., Parrinello, N. L., La Cava, P., Camiolo, G., Romano, A., Scalia, M., et al. (2018). Monocytic myeloid-derived suppressor cells as prognostic factor in chronic myeloid leukaemia patients treated with dasatinib. J. Cell. Mol. Med. 22 (2), 1070–1080. doi:10.1111/jcmm.13326
Giallongo, C., Tibullo, D., Parrinello, N. L., Cava, P. L., Rosa, M. D., Bramanti, V., et al. (2016). Granulocyte-like myeloid derived suppressor cells (G-MDSC) are increased in multiple myeloma and are driven by dysfunctional mesenchymal stem cells (MSC). Oncotarget 7 (52), 85764–85775. doi:10.18632/oncotarget.7969
Görgün, G. T., Whitehill, G., Anderson, J. L., Hideshima, T., Maguire, C., Laubach, J., et al. (2013). Tumor-promoting immune-suppressive myeloid-derived suppressor cells in the multiple myeloma microenvironment in humans. Blood 121 (15), 2975–2987. doi:10.1182/blood-2012-08-448548
Gunes, E. G., Rosen, S. T., and Querfeld, C. (2020). The role of myeloid-derived suppressor cells in hematologic malignancies. Curr. Opin. Oncol. 32 (5), 518–526. doi:10.1097/CCO.0000000000000662
Hanson, E. M., Clements, V. K., Sinha, P., Ilkovitch, D., and Ostrand-Rosenberg, S. (2009). Myeloid-derived suppressor cells down-regulate L-selectin expression on CD4+ and CD8+ T cells. J. Immunol. 183 (2), 937–944. doi:10.4049/jimmunol.0804253
Hoechst, B., Voigtlaender, T., Ormandy, L., Gamrekelashvili, J., Zhao, F., Wedemeyer, H., et al. (2009). Myeloid derived suppressor cells inhibit natural killer cells in patients with hepatocellular carcinoma via the NKp30 receptor. Hepatology 50 (3), 799–807. doi:10.1002/hep.23054
Hohtari, H., Brück, O., Blom, S., Turkki, R., Sinisalo, M., Kovanen, P. E., et al. (2019). Immune cell constitution in bone marrow microenvironment predicts outcome in adult ALL. Leukemia 33 (7), 1570–1582. doi:10.1038/s41375-018-0360-1
Hongo, D., Tang, X., Baker, J., Engleman, E. G., and Strober, S. (2014). Requirement for interactions of natural killer T cells and myeloid-derived suppressor cells for transplantation tolerance. Am. J. Transpl. 14 (11), 2467–2477. doi:10.1111/ajt.12914
Höpken, U. E., and Rehm, A. (2019). Targeting the tumor microenvironment of leukemia and lymphoma. Trends Cancer 5 (6), 351–364. doi:10.1016/j.trecan.2019.05.001
Hsu, Y. L., Yen, M. C., Chang, W. A., Tsai, P. H., Pan, Y. C., Liao, S. H., et al. (2019). CXCL17-derived CD11b(+)Gr-1(+) myeloid-derived suppressor cells contribute to lung metastasis of breast cancer through platelet-derived growth factor-BB. Breast Cancer Res. 21 (1), 23. doi:10.1186/s13058-019-1114-3
Huang, W., Liu, Y., Luz, A., Berrong, M., Meyer, J. N., Zou, Y., et al. (2021). Calcium/calmodulin dependent protein kinase kinase 2 regulates the expansion of tumor-induced myeloid-derived suppressor cells. Front. Immunol. 12, 754083. doi:10.3389/fimmu.2021.754083
Hwang, H-S., Han, A. R., Lee, J. Y., Park, G. S., Min, W-S., and Kim, H-J. (2019). Enhanced anti-leukemic effects through induction of immunomodulating microenvironment by blocking CXCR4 and PD-L1 in an AML mouse model. Immunol. Investig. 48 (1), 96–105. doi:10.1080/08820139.2018.1497057
Ibrahim, M. L., Klement, J. D., Lu, C., Redd, P. S., Xiao, W., Yang, D., et al. (2018). Myeloid-derived suppressor cells produce IL-10 to elicit DNMT3b-dependent IRF8 silencing to promote colitis-associated colon tumorigenesis. Cell Rep. 25 (11), 3036–3046.e6. doi:10.1016/j.celrep.2018.11.050
Jain, M. D., Zhao, H., Wang, X., Atkins, R., Menges, M., Reid, K., et al. (2021). Tumor interferon signaling and suppressive myeloid cells are associated with CAR T-cell failure in large B-cell lymphoma. Blood 137 (19), 2621–2633. doi:10.1182/blood.2020007445
Jiménez-Cortegana, C., Palazón-Carrión, N., Martin Garcia-Sancho, A., Nogales-Fernandez, E., Carnicero-González, F., Ríos-Herranz, E., et al. (2021). Circulating myeloid-derived suppressor cells and regulatory T cells as immunological biomarkers in refractory/relapsed diffuse large B-cell lymphoma: Translational results from the R2-GDP-GOTEL trial. J. Immunother. Cancer 9 (6), e002323. doi:10.1136/jitc-2020-002323
Jiménez-Cortegana, C., Sánchez-Martínez, P. M., Palazón-Carrión, N., Nogales-Fernández, E., Henao-Carrasco, F., Martín García-Sancho, A., et al. (2021). Lower survival and increased circulating suppressor cells in patients with relapsed/refractory diffuse large B-cell lymphoma with deficit of vitamin D levels using R-GDP plus lenalidomide (R2-GDP): Results from the R2-GDP-GOTEL trial. Cancers 13 (18), 4622. doi:10.3390/cancers13184622
Jitschin, R., Braun, M., Büttner, M., Dettmer-Wilde, K., Bricks, J., Berger, J., et al. (2014). CLL-cells induce IDOhi CD14+HLA-DRlo myeloid-derived suppressor cells that inhibit T-cell responses and promote TRegs. Blood 124 (5), 750–760. doi:10.1182/blood-2013-12-546416
Jitschin, R., Saul, D., Braun, M., Tohumeken, S., Völkl, S., Kischel, R., et al. (2018). CD33/CD3-bispecific T-cell engaging (BiTE®) antibody construct targets monocytic AML myeloid-derived suppressor cells. J. Immunother. Cancer 6 (1), 116. doi:10.1186/s40425-018-0432-9
Kaplan, R. N., Riba, R. D., Zacharoulis, S., Bramley, A. H., Vincent, L., Costa, C., et al. (2005). VEGFR1-positive haematopoietic bone marrow progenitors initiate the pre-metastatic niche. Nature 438 (7069), 820–827. doi:10.1038/nature04186
Karakhanova, S., Link, J., Heinrich, M., Shevchenko, I., Yang, Y., Hassenpflug, M., et al. (2015). Characterization of myeloid leukocytes and soluble mediators in pancreatic cancer: Importance of myeloid-derived suppressor cells. Oncoimmunology 4 (4), e998519. doi:10.1080/2162402X.2014.998519
Khalifa, K. A., Badawy, H. M., Radwan, W. M., Shehata, M. A., and Bassuoni, M. A. (2014). CD14(+) HLA-DR low/(-) monocytes as indicator of disease aggressiveness in B-cell non-Hodgkin lymphoma. Int. J. Lab. Hematol. 36 (6), 650–655. doi:10.1111/ijlh.12203
Kim, H. R., Park, S. M., Seo, S. U., Jung, I., Yoon, H. I., Gabrilovich, D. I., et al. (2019). The ratio of peripheral regulatory T cells to lox-1(+) polymorphonuclear myeloid-derived suppressor cells predicts the early response to anti-PD-1 therapy in patients with non-small cell lung cancer. Am. J. Respir. Crit. Care Med. 199 (2), 243–246. doi:10.1164/rccm.201808-1502LE
Kim, T. W., Park, S-S., Lim, J-Y., Min, G. J., Park, S., Jeon, Y-W., et al. (2019). Predictive role of circulating immune cell subtypes early after allogeneic hematopoietic stem cell transplantation in patients with acute leukemia. IJSC 12 (1), 73–83. doi:10.15283/ijsc18094
Kittang, A. O., Kordasti, S., Sand, K. E., Costantini, B., Kramer, A. M., Perezabellan, P., et al. (2016). Expansion of myeloid derived suppressor cells correlates with number of T regulatory cells and disease progression in myelodysplastic syndrome. OncoImmunology 5 (2), e1062208. doi:10.1080/2162402X.2015.1062208
Köstlin, N., Hofstädter, K., Ostermeir, A. L., Spring, B., Leiber, A., Haen, S., et al. (2016). Granulocytic myeloid-derived suppressor cells accumulate in human placenta and polarize toward a Th2 phenotype. J. Immunol. 196 (3), 1132–1145. doi:10.4049/jimmunol.1500340
Köstlin, N., Ostermeir, A. L., Spring, B., Schwarz, J., Marmé, A., Walter, C. B., et al. (2017). HLA-G promotes myeloid-derived suppressor cell accumulation and suppressive activity during human pregnancy through engagement of the receptor ILT4. Eur. J. Immunol. 47 (2), 374–384. doi:10.1002/eji.201646564
Kujawski, M., Kortylewski, M., Lee, H., Herrmann, A., Kay, H., and Yu, H. (2008). Stat3 mediates myeloid cell-dependent tumor angiogenesis in mice. J. Clin. Invest. 118 (10), 3367–3377. doi:10.1172/JCI35213
Kusmartsev, S., Eruslanov, E., Kübler, H., Tseng, T., Sakai, Y., Su, Z., et al. (2008). Oxidative stress regulates expression of VEGFR1 in myeloid cells: Link to tumor-induced immune suppression in renal cell carcinoma. J. Immunol. 181 (1), 346–353. doi:10.4049/jimmunol.181.1.346
Kuwahara-Ota, S., Shimura, Y., Steinebach, C., Isa, R., Yamaguchi, J., Nishiyama, D., et al. (2020). Lenalidomide and pomalidomide potently interfere with induction of myeloid-derived suppressor cells in multiple myeloma. Br. J. Haematol. 191 (5), 784–795. doi:10.1111/bjh.16881
Leader, A. M., Grout, J. A., Maier, B. B., Nabet, B. Y., Park, M. D., Tabachnikova, A., et al. (2021). Single-cell analysis of human non-small cell lung cancer lesions refines tumor classification and patient stratification. Cancer cell. 12, 1594–1609.e12. doi:10.1016/j.ccell.2021.10.009
Lee, S-E., Lim, J-Y., Kim, T. W., Jeon, Y-W., Yoon, J-H., Cho, B-S., et al. (2018). Matrix metalloproteinase-9 in monocytic myeloid-derived suppressor cells correlate with early infections and clinical outcomes in allogeneic hematopoietic stem cell transplantation. Biol. Blood Marrow Transplant. 24 (1), 32–42. doi:10.1016/j.bbmt.2017.08.017
Lee, S-E., Lim, J-Y., Kim, T. W., Ryu, D-B., Park, S. S., Jeon, Y-W., et al. (2019). Different role of circulating myeloid-derived suppressor cells in patients with multiple myeloma undergoing autologous stem cell transplantation. J. Immunother. Cancer 7 (1), 35. doi:10.1186/s40425-018-0491-y
Lelis, F. J. N., Jaufmann, J., Singh, A., Fromm, K., Teschner, A. C., Pöschel, S., et al. (2017). Myeloid-derived suppressor cells modulate B-cell responses. Immunol. Lett. 188, 108–115. doi:10.1016/j.imlet.2017.07.003
Lewinsky, H., Gunes, E. G., David, K., Radomir, L., Kramer, M. P., Pellegrino, B., et al. (2021). CD84 is a regulator of the immunosuppressive microenvironment in multiple myeloma. JCI Insight 6 (4), e141683. doi:10.1172/jci.insight.141683
Li, C., Liu, T., Bazhin, A. V., and Yang, Y. (2017). The sabotaging role of myeloid cells in anti-angiogenic therapy: Coordination of angiogenesis and immune suppression by hypoxia. J. Cell Physiol. 232 (9), 2312–2322. doi:10.1002/jcp.25726
Li, H., Han, Y., Guo, Q., Zhang, M., and Cao, X. (2009). Cancer-expanded myeloid-derived suppressor cells induce anergy of NK cells through membrane-bound TGF-beta 1. J. Immunol. 182 (1), 240–249. doi:10.4049/jimmunol.182.1.240
Li, L., Yin, J., Li, Y., Wang, C., Mao, X., Wei, J., et al. (2021). Allogeneic hematopoietic stem cell transplantation mobilized with pegylated granulocyte colony-stimulating factor ameliorates severe acute graft-versus-host disease through enrichment of monocytic myeloid-derived suppressor cells in the graft: A real world experience. Front. Immunol. 12, 801313. doi:10.3389/fimmu.2021.801313
Li, T., Li, X., Zamani, A., Wang, W., Lee, C. N., Li, M., et al. (2020). c-Rel is a myeloid checkpoint for cancer immunotherapy. Nat. Cancer 1 (5), 507–517. doi:10.1038/s43018-020-0061-3
Li, X., Kong, D., Yu, Q., Si, X., Yang, L., Zeng, X., et al. (2022). Cyclosporine A regulates PMN-MDSCs viability and function through MPTP in acute GVHD: Old medication, new target. Transplant. Cell. Ther. 28 (7), 411.e1–411411.e9. doi:10.1016/j.jtct.2022.04.010
Li, Y., Tu, Z., Qian, S., Fung, J. J., Markowitz, S. D., Kusner, L. L., et al. (2014). Myeloid-derived suppressor cells as a potential therapy for experimental autoimmune myasthenia gravis. J. Immunol. 193 (5), 2127–2134. doi:10.4049/jimmunol.1400857
Li, Z., Pang, Y., Gara, S. K., Achyut, B. R., Heger, C., Goldsmith, P. K., et al. (2012). Gr-1+CD11b+ cells are responsible for tumor promoting effect of TGF-β in breast cancer progression. Int. J. Cancer 131 (11), 2584–2595. doi:10.1002/ijc.27572
Lim, J-Y., Kim, T-W., Ryu, D-B., Park, S-S., Lee, S-E., Kim, B-S., et al. (2021). Myeloma-secreted galectin-1 potently interacts with CD304 on monocytic myeloid-derived suppressor cells. Cancer Immunol. Res. 9 (5), 503–513. doi:10.1158/2326-6066.CIR-20-0663
Lin, Y., Gustafson, M. P., Bulur, P. A., Gastineau, D. A., Witzig, T. E., and Dietz, A. B. (2011). Immunosuppressive CD14+HLA-DR(low)/- monocytes in B-cell non-Hodgkin lymphoma. Blood 117 (3), 872–881. doi:10.1182/blood-2010-05-283820
Lindo, L., Wilkinson, L. H., and Hay, K. A. (2021). Befriending the hostile tumor microenvironment in CAR T-cell therapy. Front. Immunol. 11, 618387. doi:10.3389/fimmu.2020.618387
Liu, Y., Zhou, X., and Wang, X. (2021). Targeting the tumor microenvironment in B-cell lymphoma: Challenges and opportunities. J. Hematol. Oncol. 14 (1), 125. doi:10.1186/s13045-021-01134-x
Locatelli, S. L., Careddu, G., Serio, S., Consonni, F. M., Maeda, A., Viswanadha, S., et al. (2019). Targeting cancer cells and tumor microenvironment in preclinical and clinical models of Hodgkin lymphoma using the dual PI3Kδ/γ inhibitor RP6530. Clin. Cancer Res. 25 (3), 1098–1112. doi:10.1158/1078-0432.CCR-18-1133
Lu, F., Zhao, Y., Pang, Y., Ji, M., Sun, Y., Wang, H., et al. (2021). NLRP3 inflammasome upregulates PD-L1 expression and contributes to immune suppression in lymphoma. Cancer Lett. 497, 178–189. doi:10.1016/j.canlet.2020.10.024
Luo, W., Napoleon, J. V., Zhang, F., Lee, Y. G., Wang, B., Putt, K. S., et al. (2022). Repolarization of tumor-infiltrating myeloid cells for augmentation of CAR T cell therapies. Front. Immunol. 13, 816761. doi:10.3389/fimmu.2022.816761
Luyckx, A., Schouppe, E., Rutgeerts, O., Lenaerts, C., Fevery, S., Devos, T., et al. (2012). G-CSF stem cell mobilization in human donors induces polymorphonuclear and mononuclear myeloid-derived suppressor cells. Clin. Immunol. 143 (1), 83–87. doi:10.1016/j.clim.2012.01.011
Lv, J., Zhao, Y., Zong, H., Ma, G., Wei, X., and Zhao, Y. (2021). Increased levels of circulating monocytic- and early-stage myeloid-derived suppressor cells (MDSC) in acute myeloid leukemia. Clin. Lab. 67 (3). doi:10.7754/Clin.Lab.2020.200719
Mandruzzato, S., Brandau, S., Britten, C. M., Bronte, V., Damuzzo, V., Gouttefangeas, C., et al. (2016). Toward harmonized phenotyping of human myeloid-derived suppressor cells by flow cytometry: Results from an interim study. Cancer Immunol. Immunother. 65 (2), 161–169. doi:10.1007/s00262-015-1782-5
Mantovani, A., Marchesi, F., Malesci, A., Laghi, L., and Allavena, P. (2017). Tumour-associated macrophages as treatment targets in oncology. Nat. Rev. Clin. Oncol. 14 (7), 399–416. doi:10.1038/nrclinonc.2016.217
Marini, O., Spina, C., Mimiola, E., Cassaro, A., Malerba, G., Todeschini, G., et al. (2016). Identification of granulocytic myeloid-derived suppressor cells (G-MDSCs) in the peripheral blood of Hodgkin and non-Hodgkin lymphoma patients. Oncotarget 7 (19), 27676–27688. doi:10.18632/oncotarget.8507
Mastio, J., Condamine, T., Dominguez, G., Kossenkov, A. V., Donthireddy, L., Veglia, F., et al. (2019). Identification of monocyte-like precursors of granulocytes in cancer as a mechanism for accumulation of PMN-MDSCs. J. Exp. Med. 216 (9), 2150–2169. doi:10.1084/jem.20181952
Maude, S. L., Laetsch, T. W., Buechner, J., Rives, S., Boyer, M., Bittencourt, H., et al. (2018). Tisagenlecleucel in children and young adults with B-cell lymphoblastic leukemia. N. Engl. J. Med. 378 (5), 439–448. doi:10.1056/NEJMoa1709866
Molgora, M., Bonavita, E., Ponzetta, A., Riva, F., Barbagallo, M., Jaillon, S., et al. (2017). IL-1R8 is a checkpoint in NK cells regulating anti-tumour and anti-viral activity. Nature 551 (7678), 110–114. doi:10.1038/nature24293
Movahedi, K., Guilliams, M., Van den Bossche, J., Van den Bergh, R., Gysemans, C., Beschin, A., et al. (2008). Identification of discrete tumor-induced myeloid-derived suppressor cell subpopulations with distinct T cell–suppressive activity. Blood 111 (8), 4233–4244. doi:10.1182/blood-2007-07-099226
Nakamura, K., Kassem, S., Cleynen, A., Chrétien, M-L., Guillerey, C., Putz, E. M., et al. (2018). Dysregulated IL-18 is a key driver of immunosuppression and a possible therapeutic target in the multiple myeloma microenvironment. Cancer Cell 33 (4), 634–648.e5. doi:10.1016/j.ccell.2018.02.007
Nan, J., Xing, Y. F., Hu, B., Tang, J. X., Dong, H. M., He, Y. M., et al. (2018). Endoplasmic reticulum stress induced LOX-1(+) CD15(+) polymorphonuclear myeloid-derived suppressor cells in hepatocellular carcinoma. Immunology 154 (1), 144–155. doi:10.1111/imm.12876
Nasrollahzadeh, E., Razi, S., Keshavarz-Fathi, M., Mazzone, M., and Rezaei, N. (2020). Pro-tumorigenic functions of macrophages at the primary, invasive and metastatic tumor site. Cancer Immunol. Immunother. 69 (9), 1673–1697. doi:10.1007/s00262-020-02616-6
Nathan, H. F., Chan Yoon, C., Randy, D. G., John, G., Sattva, S. N., Paolo, G., et al. (2016). Role of the tumor microenvironment in mature B-cell lymphoid malignancies. Haematologica 101 (5), 531–540. doi:10.3324/haematol.2015.139493
Neelapu, S. S., Locke, F. L., Bartlett, N. L., Lekakis, L. J., Miklos, D. B., Jacobson, C. A., et al. (2017). Axicabtagene ciloleucel CAR T-cell therapy in refractory large B-cell lymphoma. N. Engl. J. Med. 377 (26), 2531–2544. doi:10.1056/NEJMoa1707447
Okła, K., Rajtak, A., Czerwonka, A., Bobiński, M., Wawruszak, A., Tarkowski, R., et al. (2020). Accumulation of blood-circulating PD-L1-expressing M-MDSCs and monocytes/macrophages in pretreatment ovarian cancer patients is associated with soluble PD-L1. J. Transl. Med. 18 (1), 220. doi:10.1186/s12967-020-02389-7
Ortiz, M. L., Kumar, V., Martner, A., Mony, S., Donthireddy, L., Condamine, T., et al. (2015). Immature myeloid cells directly contribute to skin tumor development by recruiting IL-17-producing CD4+ T cells. J. Exp. Med. 212 (3), 351–367. doi:10.1084/jem.20140835
Oshrine, B., Innamarato, P., Branthoover, H., Nagle, L., Verdugo, P., Pilon-Thomas, S., et al. (2022). Early recovery of myeloid-derived suppressor cells after allogeneic hematopoietic transplant: Comparison of post-transplantation cyclophosphamide to standard graft-versus-host disease prophylaxis. Transplant. Cell. Ther. 28 (4), 203.e1–203203.e7. doi:10.1016/j.jtct.2021.12.019
Ostrand-Rosenberg, S., and Fenselau, C. (2018). Myeloid-derived suppressor cells: Immune-suppressive cells that impair antitumor immunity and are sculpted by their environment. J. Immunol. 200 (2), 422–431. doi:10.4049/jimmunol.1701019
Ouzounova, M., Lee, E., Piranlioglu, R., El Andaloussi, A., Kolhe, R., Demirci, M. F., et al. (2017). Monocytic and granulocytic myeloid derived suppressor cells differentially regulate spatiotemporal tumour plasticity during metastatic cascade. Nat. Commun. 8, 14979. doi:10.1038/ncomms14979
Ozerova, M., and Nefedova, Y. (2019). Estrogen promotes multiple myeloma through enhancing the immunosuppressive activity of MDSC. Leukemia Lymphoma 60 (6), 1557–1562. doi:10.1080/10428194.2018.1538511
Parihar, R., Rivas, C., Huynh, M., Omer, B., Lapteva, N., Metelitsa, L. S., et al. (2019). NK cells expressing a chimeric activating receptor eliminate MDSCs and rescue impaired CAR-T cell activity against solid tumors. Cancer Immunol. Res. 7 (3), 363–375. doi:10.1158/2326-6066.CIR-18-0572
Pastaki Khoshbin, A., Eskian, M., Keshavarz-Fathi, M., and Rezaei, N. (2019). Roles of myeloid-derived suppressor cells in cancer metastasis: Immunosuppression and beyond. Arch. Immunol. Ther. Exp. Warsz. 67 (2), 89–102. doi:10.1007/s00005-018-0531-9
Pathria, P., Louis, T. L., and Varner, J. A. (2019). Targeting tumor-associated macrophages in cancer. Trends Immunol. 40 (4), 310–327. doi:10.1016/j.it.2019.02.003
Peinado, H., Zhang, H., Matei, I. R., Costa-Silva, B., Hoshino, A., Rodrigues, G., et al. (2017). Pre-metastatic niches: Organ-specific homes for metastases. Nat. Rev. Cancer 17 (5), 302–317. doi:10.1038/nrc.2017.6
Pelosi, A., Besi, F., Tumino, N., Merli, P., Quatrini, L., Li Pira, G., et al. (2021). NK cells and PMN-MDSCs in the graft from G-CSF mobilized haploidentical donors display distinct gene expression profiles from those of the non-mobilized counterpart. Front. Immunol. 12, 657329. doi:10.3389/fimmu.2021.657329
Perez, C., Botta, C., Zabaleta, A., Puig, N., Cedena, M-T., Goicoechea, I., et al. (2020). Immunogenomic identification and characterization of granulocytic myeloid-derived suppressor cells in multiple myeloma. Blood 136 (2), 199–209. doi:10.1182/blood.2019004537
Porembka, M. R., Mitchem, J. B., Belt, B. A., Hsieh, C. S., Lee, H. M., Herndon, J., et al. (2012). Pancreatic adenocarcinoma induces bone marrow mobilization of myeloid-derived suppressor cells which promote primary tumor growth. Cancer Immunol. Immunother. 61 (9), 1373–1385. doi:10.1007/s00262-011-1178-0
Qi, X., Jiang, H., Liu, P., Xie, N., Fu, R., Wang, H., et al. (2021). Increased myeloid-derived suppressor cells in patients with myelodysplastic syndromes suppress CD8+ T lymphocyte function through the STAT3-ARG1 pathway. Leukemia Lymphoma 62 (1), 218–223. doi:10.1080/10428194.2020.1817431
Ramachandran, I. R., Martner, A., Pisklakova, A., Condamine, T., Chase, T., Vogl, T., et al. (2013). Myeloid-derived suppressor cells regulate growth of multiple myeloma by inhibiting T cells in bone marrow. J. Immunol. 190 (7), 3815–3823. doi:10.4049/jimmunol.1203373
Ren, W., Zhang, X., Li, W., Feng, Q., Feng, H., Tong, Y., et al. (2019). Exosomal miRNA-107 induces myeloid-derived suppressor cell expansion in gastric cancer. Cancer Manag. Res. 11, 4023–4040. doi:10.2147/CMAR.S198886
Romano, A., Parrinello, N. L., Vetro, C., Forte, S., Chiarenza, A., Figuera, A., et al. (2015). Circulating myeloid-derived suppressor cells correlate with clinical outcome in Hodgkin Lymphoma patients treated up-front with a risk-adapted strategy. Br. J. Haematol. 168 (5), 689–700. doi:10.1111/bjh.13198
Sakamaki, I., Kwak, L. W., Cha, S. C., Yi, Q., Lerman, B., Chen, J., et al. (2014). Lenalidomide enhances the protective effect of a therapeutic vaccine and reverses immune suppression in mice bearing established lymphomas. Leukemia 28 (2), 329–337. doi:10.1038/leu.2013.177
Sarhan, D., Cichocki, F., Zhang, B., Yingst, A., Spellman, S. R., Cooley, S., et al. (2016). Adaptive NK cells with low TIGIT expression are inherently resistant to myeloid-derived suppressor cells. Cancer Res. 76 (19), 5696–5706. doi:10.1158/0008-5472.CAN-16-0839
Schlecker, E., Stojanovic, A., Eisen, C., Quack, C., Falk, C. S., Umansky, V., et al. (2012). Tumor-infiltrating monocytic myeloid-derived suppressor cells mediate CCR5-dependent recruitment of regulatory T cells favoring tumor growth. J. Immunol. 189 (12), 5602–5611. doi:10.4049/jimmunol.1201018
Schmitz, R., Young, R. M., Ceribelli, M., Jhavar, S., Xiao, W., Zhang, M., et al. (2012). Burkitt lymphoma pathogenesis and therapeutic targets from structural and functional genomics. Nature 490 (7418), 116–120. doi:10.1038/nature11378
Schneidawind, C., Jahnke, S., Schober-Melms, I., Schumm, M., Handgretinger, R., Faul, C., et al. (2019). G-CSF administration prior to donor lymphocyte apheresis promotes anti-leukaemic effects in allogeneic HCT patients. Br. J. Haematol. 186 (1), 60–71. doi:10.1111/bjh.15881
Schuster, S. J., Svoboda, J., Chong, E. A., Nasta, S. D., Mato, A. R., Anak, Ö., et al. (2017). Chimeric antigen receptor T cells in refractory B-cell lymphomas. N. Engl. J. Med. 377 (26), 2545–2554. doi:10.1056/NEJMoa1708566
Scott, D. W., and Gascoyne, R. D. (2014). The tumour microenvironment in B cell lymphomas. Nat. Rev. Cancer 14 (8), 517–534. doi:10.1038/nrc3774
Sehn, L. H., and Salles, G. (2021). Diffuse large B-cell lymphoma. N. Engl. J. Med. 384 (9), 842–858. doi:10.1056/NEJMra2027612
Serafini, P., Mgebroff, S., Noonan, K., and Borrello, I. (2008). Myeloid-derived suppressor cells promote cross-tolerance in B-cell lymphoma by expanding regulatory T cells. Cancer Res. 68 (13), 5439–5449. doi:10.1158/0008-5472.CAN-07-6621
Sevko, A., Michels, T., Vrohlings, M., Umansky, L., Beckhove, P., Kato, M., et al. (2013). Antitumor effect of paclitaxel is mediated by inhibition of myeloid-derived suppressor cells and chronic inflammation in the spontaneous melanoma model. J. Immunol. 190, 2464–2471. doi:10.4049/jimmunol.1202781
Shen, J. X., Liu, J., and Zhang, G. J. (2018). Interleukin-33 in malignancies: Friends or foes? Front. Immunol. 9, 3051. doi:10.3389/fimmu.2018.03051
Sinha, P., Okoro, C., Foell, D., Freeze, H. H., Ostrand-Rosenberg, S., and Srikrishna, G. (2008). Proinflammatory S100 proteins regulate the accumulation of myeloid-derived suppressor cells. J. Immunol. 181 (7), 4666–4675. doi:10.4049/jimmunol.181.7.4666
Smyth, L., Blunt, D. N., Gatov, E., Nagamuthu, C., Croxford, R., Mozessohn, L., et al. (2020). Statin and cyclooxygenase-2 inhibitors improve survival in newly diagnosed diffuse large B-cell lymphoma: A large population-based study of 4913 subjects. Br. J. Haematol. 191 (3), 396–404. doi:10.1111/bjh.16635
Solman, I. G., Blum, L. K., Burger, J. A., Kipps, T. J., Dean, J. P., James, D. F., et al. (2021). Impact of long-term ibrutinib treatment on circulating immune cells in previously untreated chronic lymphocytic leukemia. Leukemia Res. 102, 106520. doi:10.1016/j.leukres.2021.106520
Stiff, A., Trikha, P., Mundy-Bosse, B., McMichael, E., Mace, T. A., Benner, B., et al. (2018). Nitric oxide production by myeloid-derived suppressor cells plays a role in impairing fc receptor-mediated natural killer cell function. Clin. Cancer Res. 24 (8), 1891–1904. doi:10.1158/1078-0432.CCR-17-0691
Sui, Q., Zhang, J., Sun, X., Zhang, C., Han, Q., and Tian, Z. (2014). NK cells are the crucial antitumor mediators when STAT3-mediated immunosuppression is blocked in hepatocellular carcinoma. J. Immunol. 193 (4), 2016–2023. doi:10.4049/jimmunol.1302389
Sun, H., Li, Y., Zhang, Z., Ju, Y., Li, L., Zhang, B., et al. (2015). Increase in myeloid-derived suppressor cells (MDSCs) associated with minimal residual disease (MRD) detection in adult acute myeloid leukemia. Int. J. Hematol. 102 (5), 579–586. doi:10.1007/s12185-015-1865-2
Sun, X., Sui, Q., Zhang, C., Tian, Z., and Zhang, J. (2013). Targeting blockage of STAT3 in hepatocellular carcinoma cells augments NK cell functions via reverse hepatocellular carcinoma-induced immune suppression. Mol. Cancer Ther. 12 (12), 2885–2896. doi:10.1158/1535-7163.MCT-12-1087
Tadmor, T., Fell, R., Polliack, A., and Attias, D. (2013). Absolute monocytosis at diagnosis correlates with survival in diffuse large B-cell lymphoma-possible link with monocytic myeloid-derived suppressor cells. Hematol. Oncol. 31 (2), 65–71. doi:10.1002/hon.2019
Tao, J., Han, D., Gao, S., Zhang, W., Yu, H., Liu, P., et al. (2020). CD8+ T cells exhaustion induced by myeloid-derived suppressor cells in myelodysplastic syndromes patients might be through TIM3/Gal-9 pathway. J. Cell. Mol. Med. 24 (1), 1046–1058. doi:10.1111/jcmm.14825
Tavukcuoglu, E., Horzum, U., Yanik, H., Uner, A., Yoyen-Ermis, D., Nural, S. K., et al. (2020). Human splenic polymorphonuclear myeloid-derived suppressor cells (PMN-MDSC) are strategically located immune regulatory cells in cancer. Eur. J. Immunol. 50 (12), 2067–2074. doi:10.1002/eji.202048666
Tian, X., Shen, H., Li, Z., Wang, T., and Wang, S. (2019). Tumor-derived exosomes, myeloid-derived suppressor cells, and tumor microenvironment. J. Hematol. Oncol. 12 (1), 84. doi:10.1186/s13045-019-0772-z
Tobin, R. P., Jordan, K. R., Kapoor, P., Spongberg, E., Davis, D., Vorwald, V. M., et al. (2019). IL-6 and IL-8 are linked with myeloid-derived suppressor cell accumulation and correlate with poor clinical outcomes in melanoma patients. Front. Oncol. 9, 1223. doi:10.3389/fonc.2019.01223
Toh, B., Wang, X., Keeble, J., Sim, W. J., Khoo, K., Wong, W. C., et al. (2011). Mesenchymal transition and dissemination of cancer cells is driven by myeloid-derived suppressor cells infiltrating the primary tumor. PLoS Biol. 9 (9), e1001162. doi:10.1371/journal.pbio.1001162
Trabanelli, S., Chevalier, M. F., Martinez-Usatorre, A., Gomez-Cadena, A., Salomé, B., Lecciso, M., et al. (2017). Tumour-derived PGD2 and NKp30-B7H6 engagement drives an immunosuppressive ILC2-MDSC axis. Nat. Commun. 8 (1), 593. doi:10.1038/s41467-017-00678-2
Trovato, R., Fiore, A., Sartori, S., Canè, S., Giugno, R., Cascione, L., et al. (2019). Immunosuppression by monocytic myeloid-derived suppressor cells in patients with pancreatic ductal carcinoma is orchestrated by STAT3. J. Immunother. Cancer 7 (1), 255. doi:10.1186/s40425-019-0734-6
Tumino, N., Weber, G., Besi, F., Del Bufalo, F., Bertaina, V., Paci, P., et al. (2021). Polymorphonuclear myeloid-derived suppressor cells impair the anti-tumor efficacy of GD2.CAR T-cells in patients with neuroblastoma. J. Hematol. Oncol. 14 (1), 191. doi:10.1186/s13045-021-01193-0
Ugolini, A., Tyurin, V. A., Tyurina, Y. Y., Tcyganov, E. N., Donthireddy, L., Kagan, V. E., et al. (2020). Polymorphonuclear myeloid-derived suppressor cells limit antigen cross-presentation by dendritic cells in cancer. JCI Insight 5 (15), e138581. doi:10.1172/jci.insight.138581
van de Donk, N. W. C. J., and Usmani, S. Z. (2018). CD38 antibodies in multiple myeloma: Mechanisms of action and modes of resistance. Front. Immunol. 9, 2134. doi:10.3389/fimmu.2018.02134
Veglia, F., Hashimoto, A., Dweep, H., Sanseviero, E., De Leo, A., Tcyganov, E., et al. (2021). Analysis of classical neutrophils and polymorphonuclear myeloid-derived suppressor cells in cancer patients and tumor-bearing mice. J. Exp. Med. 218 (4), e20201803. doi:10.1084/jem.20201803
Veglia, F., Perego, M., and Gabrilovich, D. (2018). Myeloid-derived suppressor cells coming of age. Nat. Immunol. 19 (2), 108–119. doi:10.1038/s41590-017-0022-x
Veglia, F., Tyurin, V. A., Blasi, M., De Leo, A., Kossenkov, A. V., Donthireddy, L., et al. (2019). Fatty acid transport protein 2 reprograms neutrophils in cancer. Nature 569 (7754), 73–78. doi:10.1038/s41586-019-1118-2
Veirman, K. D., Van Ginderachter, J. A., Lub, S., Beule, N. D., Thielemans, K., Bautmans, I., et al. (2015). Multiple myeloma induces Mcl-1 expression and survival of myeloid-derived suppressor cells. Oncotarget 6 (12), 10532–10547. doi:10.18632/oncotarget.3300
Veltman, J. D., Lambers, M. E. H., van Nimwegen, M., Hendriks, R. W., Hoogsteden, H. C., Aerts, J. G. J. V., et al. (2010). COX-2 inhibition improves immunotherapy and is associated with decreased numbers of myeloid-derived suppressor cells in mesothelioma. Celecoxib influences MDSC function. BMC Cancer 10 (1), 464. doi:10.1186/1471-2407-10-464
Wang, H., Tao, Q., Wang, Z., Zhang, Q., Xiao, H., Zhou, M., et al. (2020). Circulating monocytic myeloid-derived suppressor cells are elevated and associated with poor prognosis in acute myeloid leukemia. J. Immunol. Res. 2020, 7363084. doi:10.1155/2020/7363084
Wang, J., Li, D., Cang, H., and Guo, B. (2019). Crosstalk between cancer and immune cells: Role of tumor-associated macrophages in the tumor microenvironment. Cancer Med. 8 (10), 4709–4721. doi:10.1002/cam4.2327
Wang, J., Veirman, K. D., Beule, N. D., Maes, K., Bruyne, E. D., Valckenborgh, E. V., et al. (2015). The bone marrow microenvironment enhances multiple myeloma progression by exosome-mediated activation of myeloid-derived suppressor cells. Oncotarget 6 (41), 43992–44004. doi:10.18632/oncotarget.6083
Wang, R., Wang, X., Yang, S., Xiao, Y., Jia, Y., Zhong, J., et al. (2021). Umbilical cord-derived mesenchymal stem cells promote myeloid-derived suppressor cell enrichment by secreting CXCL1 to prevent graft-versus-host disease after hematopoietic stem cell transplantation. Cytotherapy 23 (11), 996–1006. doi:10.1016/j.jcyt.2021.07.009
Wang, Y., Ding, Y., Guo, N., and Wang, S. (2019). MDSCs: Key criminals of tumor pre-metastatic niche formation. Front. Immunol. 10, 172. doi:10.3389/fimmu.2019.00172
Wang, Y., Schafer, C. C., Hough, K. P., Tousif, S., Duncan, S. R., Kearney, J. F., et al. (2018). Myeloid-derived suppressor cells impair B cell responses in lung cancer through IL-7 and STAT5. J. Immunol. 201 (1), 278–295. doi:10.4049/jimmunol.1701069
Wang, Y., Wang, J., Zhu, F., Wang, H., Yi, L., Huang, K., et al. (2022). Elevated circulating myeloid-derived suppressor cells associated with poor prognosis in B-cell non-Hodgkin's lymphoma patients. Immun. Inflamm. Dis. 10 (5), e616. doi:10.1002/iid3.616
Wang, Z., Jiang, R., Li, Q., Wang, H., Tao, Q., and Zhai, Z. (2021). Elevated M-MDSCs in circulation are indicative of poor prognosis in diffuse large B-cell lymphoma patients. J. Clin. Med. 10 (8), 1768. doi:10.3390/jcm10081768
Wang, Z., Zhang, L., Wang, H., Xiong, S., Li, Y., Tao, Q., et al. (2015). Tumor-induced CD14+HLA-DR−/low myeloid-derived suppressor cells correlate with tumor progression and outcome of therapy in multiple myeloma patients. Cancer Immunol. Immunother. 64 (3), 389–399. doi:10.1007/s00262-014-1646-4
Weber, R., Fleming, V., Hu, X., Nagibin, V., Groth, C., Altevogt, P., et al. (2018). Myeloid-derived suppressor cells hinder the anti-cancer activity of immune checkpoint inhibitors. Front. Immunol. 9, 1310. doi:10.3389/fimmu.2018.01310
Wei, J., Zhang, M., and Zhou, J. (2015). Myeloid-derived suppressor cells in major depression patients suppress T-cell responses through the production of reactive oxygen species. Psychiatry Res. 228 (3), 695–701. doi:10.1016/j.psychres.2015.06.002
Wu, C., Wu, X., Zhang, X., Chai, Y., Guo, Q., Li, L., et al. (2015). Prognostic significance of peripheral monocytic myeloid-derived suppressor cells and monocytes in patients newly diagnosed with diffuse large b-cell lymphoma. Int. J. Clin. Exp. Med. 8 (9), 15173–15181.
Wu, M., Ma, M., Tan, Z., Zheng, H., and Liu, X. (2020). Neutrophil: A new player in metastatic cancers. Front. Immunol. 11, 565165. doi:10.3389/fimmu.2020.565165
Xiu, B., Lin, Y., Grote, D. M., Ziesmer, S. C., Gustafson, M. P., Maas, M. L., et al. (2015). IL-10 induces the development of immunosuppressive CD14(+)HLA-DR(low/-) monocytes in B-cell non-Hodgkin lymphoma. Blood Cancer J. 5 (7), e328. doi:10.1038/bcj.2015.56
Xu, X., Meng, Q., Erben, U., Wang, P., Glauben, R., Kühl, A. A., et al. (2017). Myeloid-derived suppressor cells promote B-cell production of IgA in a TNFR2-dependent manner. Cell. Mol. Immunol. 14 (7), 597–606. doi:10.1038/cmi.2015.103
Xu, Z., Ji, J., Xu, J., Li, D., Shi, G., Liu, F., et al. (2017). MiR-30a increases MDSC differentiation and immunosuppressive function by targeting SOCS3 in mice with B-cell lymphoma. FEBS J. 284 (15), 2410–2424. doi:10.1111/febs.14133
Xu, Z., Ji, J., Xu, J., Li, D., Shi, G., Liu, F., et al. (2017). MiR-30a increases MDSC differentiation and immunosuppressive function by targeting SOCS3 in mice with B-cell lymphoma. Febs J. 284 (15), 2410–2424. doi:10.1111/febs.14133
Yan, H. H., Pickup, M., Pang, Y., Gorska, A. E., Li, Z., Chytil, A., et al. (2010). Gr-1+CD11b+ myeloid cells tip the balance of immune protection to tumor promotion in the premetastatic lung. Cancer Res. 70 (15), 6139–6149. doi:10.1158/0008-5472.CAN-10-0706
Yang, L., DeBusk, L. M., Fukuda, K., Fingleton, B., Green-Jarvis, B., Shyr, Y., et al. (2004). Expansion of myeloid immune suppressor Gr+CD11b+ cells in tumor-bearing host directly promotes tumor angiogenesis. Cancer Cell 6 (4), 409–421. doi:10.1016/j.ccr.2004.08.031
Yang, S., Wei, Y., Sun, R., Lu, W., Lv, H., Xiao, X., et al. (2020). Umbilical cord blood-derived mesenchymal stromal cells promote myeloid-derived suppressor cell proliferation by secreting HLA-G to reduce acute graft-versus-host disease after hematopoietic stem cell transplantation. Cytotherapy 22 (12), 718–733. doi:10.1016/j.jcyt.2020.07.008
Yaseen, M. M., Abuharfeil, N. M., Darmani, H., and Daoud, A. (2020). Mechanisms of immune suppression by myeloid-derived suppressor cells: The role of interleukin-10 as a key immunoregulatory cytokine. Open Biol. 10 (9), 200111. doi:10.1098/rsob.200111
Youn, J. I., Collazo, M., Shalova, I. N., Biswas, S. K., and Gabrilovich, D. I. (2012). Characterization of the nature of granulocytic myeloid-derived suppressor cells in tumor-bearing mice. J. Leukoc. Biol. 91 (1), 167–181. doi:10.1189/jlb.0311177
Youn, J. I., and Gabrilovich, D. I. (2010). The biology of myeloid-derived suppressor cells: The blessing and the curse of morphological and functional heterogeneity. Eur. J. Immunol. 40 (11), 2969–2975. doi:10.1002/eji.201040895
Youn, J. I., Kumar, V., Collazo, M., Nefedova, Y., Condamine, T., Cheng, P., et al. (2013). Epigenetic silencing of retinoblastoma gene regulates pathologic differentiation of myeloid cells in cancer. Nat. Immunol. 14 (3), 211–220. doi:10.1038/ni.2526
Zahran, A. M., Moeen, S. M., Thabet, A. F., Rayan, A., Abdel-Rahim, M. H., Mohamed, W. M. Y., et al. (2020). Monocytic myeloid-derived suppressor cells in chronic lymphocytic leukemia patients: A single center experience. Leukemia Lymphoma 61 (7), 1645–1652. doi:10.1080/10428194.2020.1728747
Zahran, A. M., Shibl, A., Rayan, A., Mohamed, M. A. E. H., Osman, A. M. M., Saad, K., et al. (2021). Increase in polymorphonuclear myeloid-derived suppressor cells and regulatory T-cells in children with B-cell acute lymphoblastic leukemia. Sci. Rep. 11 (1), 15039. doi:10.1038/s41598-021-94469-x
Zhang, H., Li, Z-L., Ye, S-B., Ouyang, L-Y., Chen, Y-S., He, J., et al. (2015). Myeloid-derived suppressor cells inhibit T cell proliferation in human extranodal NK/T cell lymphoma: A novel prognostic indicator. Cancer Immunol. Immunother. 64 (12), 1587–1599. doi:10.1007/s00262-015-1765-6
Zhang, J., Chen, H-M., Ma, G., Zhou, Z., Raulet, D., Rivera, A. L., et al. (2019). The mechanistic study behind suppression of GVHD while retaining GVL activities by myeloid-derived suppressor cells. Leukemia 33 (8), 2078–2089. doi:10.1038/s41375-019-0394-z
Zhang, J., Han, X., Hu, X., Jin, F., Gao, Z., Yin, L., et al. (2018). Ido1 impairs NK cell cytotoxicity by decreasing NKG2D/NKG2DLs via promoting miR-18a. Mol. Immunol. 103, 144–155. doi:10.1016/j.molimm.2018.09.011
Zhou, J., Nefedova, Y., Lei, A., and Gabrilovich, D. (2018). Neutrophils and PMN-MDSC: Their biological role and interaction with stromal cells. Semin. Immunol. 35, 19–28. doi:10.1016/j.smim.2017.12.004
Keywords: myeloid-derived suppressor cells, hematological malignancies, tumor microenvironment, t cells, immunosuppression
Citation: Bhardwaj V and Ansell SM (2023) Modulation of T-cell function by myeloid-derived suppressor cells in hematological malignancies. Front. Cell Dev. Biol. 11:1129343. doi: 10.3389/fcell.2023.1129343
Received: 21 December 2022; Accepted: 15 March 2023;
Published: 05 April 2023.
Edited by:
Brunie H. Felding, The Scripps Research Institute, United StatesReviewed by:
Laura Patrussi, University of Siena, ItalyCopyright © 2023 Bhardwaj and Ansell. This is an open-access article distributed under the terms of the Creative Commons Attribution License (CC BY). The use, distribution or reproduction in other forums is permitted, provided the original author(s) and the copyright owner(s) are credited and that the original publication in this journal is cited, in accordance with accepted academic practice. No use, distribution or reproduction is permitted which does not comply with these terms.
*Correspondence: Stephen M. Ansell, YW5zZWxsLnN0ZXBoZW5AbWF5by5lZHU=
Disclaimer: All claims expressed in this article are solely those of the authors and do not necessarily represent those of their affiliated organizations, or those of the publisher, the editors and the reviewers. Any product that may be evaluated in this article or claim that may be made by its manufacturer is not guaranteed or endorsed by the publisher.
Research integrity at Frontiers
Learn more about the work of our research integrity team to safeguard the quality of each article we publish.