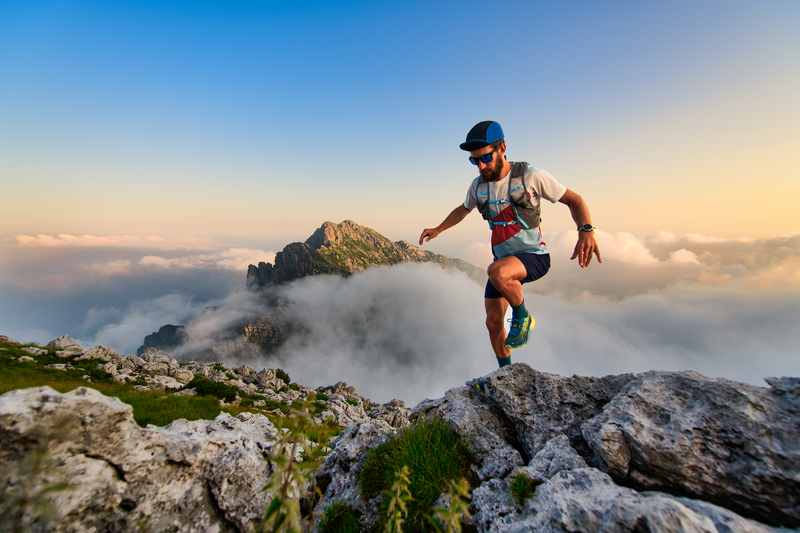
94% of researchers rate our articles as excellent or good
Learn more about the work of our research integrity team to safeguard the quality of each article we publish.
Find out more
REVIEW article
Front. Cell Dev. Biol. , 17 April 2023
Sec. Stem Cell Research
Volume 11 - 2023 | https://doi.org/10.3389/fcell.2023.1128134
CD34 is a cell surface antigen expressed in numerous stem/progenitor cells including hematopoietic stem cells (HSCs) and endothelial progenitor cells (EPCs), which are known to be rich sources of EPCs. Therefore, regenerative therapy using CD34+ cells has attracted interest for application in patients with various vascular, ischemic, and inflammatory diseases. CD34+ cells have recently been reported to improve therapeutic angiogenesis in a variety of diseases. Mechanistically, CD34+ cells are involved in both direct incorporation into the expanding vasculature and paracrine activity through angiogenesis, anti-inflammatory, immunomodulatory, and anti-apoptosis/fibrosis roles, which support the developing microvasculature. Preclinical, pilot, and clinical trials have well documented a track record of safety, practicality, and validity of CD34+ cell therapy in various diseases. However, the clinical application of CD34+ cell therapy has triggered scientific debates and controversies in last decade. This review covers all preexisting scientific literature and prepares an overview of the comprehensive biology of CD34+ cells as well as the preclinical/clinical details of CD34+ cell therapy for regenerative medicine.
The CD34 surface marker is a sialomucin transmembrane protein composed of the haematopoietic progenitor cells (HPCs) antigen, podocalyxin, and endoglycan, which is a marker of vascular endothelial cells (ECs), HPCs, and endothelial progenitor cells (EPC) (Nielsen and McNagny, 2008). Definitely, using this cell marker allowed phenotyping and separation of circulating EPCs, which incorporate into ischemic areas and facilitate vasculogenesis (Asahara et al., 1997). Following the recognition of EPCs in peripheral blood (PB)-derived CD34+ cells (Asahara et al., 1997; Shi et al., 1998), EPC biology has been developed for years. Based on the initial hypothesis of hemangioblast, a natural origin of hematopoietic and endothelial lineages in embryogenesis, the identification of EPCs used cell surface markers of hematopoietic stem cells (HSCs) or HPCs, such as CD34, CD133, VEGFR-2, CXCR4, CD105, etc., in human (Risau et al., 1988; Pardanaud et al., 1989; Flamme and Risau, 1992). Most of early research phase exploited CD34+ or CD133+ cell in human bone marrow (BM), PB or cord blood (CB) mononuclear cells (MNCs) and showed its commitment into endothelial lineage in vitro and its incorporation into ECs in neoangiogenesis in vivo (Murohara et al., 2000a; Gehling et al., 2000; Peichev et al., 2000; Quirici et al., 2001a; Kocher et al., 2001; Friedrich et al., 2006). These outcomes were backed up by the indisputable human clinical reports, indicating the incorporation of cells derived from CD34+ cells into the human vascular networks, in aortas of radiation syndrome patients (Suzuki et al., 2003), skin and gut from hematologic malignancy recipients (Jiang et al., 2004) and various cancer patients (Peters et al., 2005). Nevertheless, more than 2 decades of advances from the initial identification of EPCs, CD34+ or CD133+ cells are still pivoted as representative EPC-enriched cells for scientific EPC biology studies and therapeutical applications in clinical researches. As discussed later, the populations in circulation unintentionally comprehend both hematopoietic and non-hematopoietic lineage cell-derived EPCs (Figure 1).
CD34+ populations have been the subject of far-reaching investigations and have been believed to have potential applications in regenerative therapy because of their unique regenerative properties (Velagapudi et al., 2019). In addition to the safety, feasibility, and potential efficacy of CD34+ EPC therapy demonstrated in the previous outcomes, as EPCs, CD34+ cells naturally have the capacity for self-renewal, making them a highly valuable source of stem cells in clinical settings and suggesting an important role for EPCs in therapeutic neovascularization. This may provide a reasonable justification for transplanting purified CD34+ EPCs in garden variety of disease. Based on the scientific and medical progress in recent EPC biology, CD34+ cells are reviewed in terms of progenitor biology for vasculogenesis, paracrine functions for angiogenesis and anti-inflammation, potency to differentiate into ECs or transdifferentiate, and therapeutic development in ischemic and inflammatory diseases (Figure 2).
The fact that the phenotype and function of EPCs must be discussed in terms of their cultured phenotype, “EPCs ex vivo,” has been viewed as an issue in the previous studies (Figure 2). Biologically, primary immature cells differentiating into ECs in the living organism should be referred as EPCs. However, many discussions have come to the conclusion that EPC biology is based on studies on differentiated cells into ECs in culture ex vivo, as is unavoidable in the progress of biological research. We must consider whether these debates about EPCs ex vivo are eventually aimed at “EPCs in vivo” cell biology. In this respect, we explain EPC biology in terms of its in vivo origin. One can distinguish two types of circulating EPCs in vivo, hematopoietic lineage cell-derived EPCs (hEPCs) and non-hematopoietic lineage cells-derived EPCs (non-hEPCs) (Asahara et al., 2011). hEPCs are cells derived from HSCs or HPCs mainly derived from the BM and upon stimulation capable of circulating as blood cells, represented for example by colony-forming (CF)-EPCs, non-CF-EPCs as differentiating EPCs in short-term culture, myeloid EPCs, lymphatic EPCs, angiogenic cells, etc. Non-hEPCs are neither likely derived from HSCs nor HPCs and can be isolated as EC phenotype ex vivo in adhesive long-term cell cultures from blood or tissue samples. Non-hEPCs supposed to mobilize from resident EPCs in organ blood vessels. Resident EPCs are considered as endothelial colony forming cell (ECFC)-producing cells (Patel et al., 2013a; Lin et al., 2013; Alphonse et al., 2014; Green et al., 2017a), and have been identified in mice such as endothelial stem cells or endovascular progenitors (Patel et al., 2017; Wakabayashi et al., 2018), despite the fact that this has not yet been defined in human terms.
Non-hEPC is almost unified to one kind of endothelial outgrowth cells (EOCs). EOCs-produced from PB ex vivo reported initially by Lin et al. (2000). The concept of adhesive endothelial lineage cell stages using the original culture conditions disclosed clonal colony-forming units of outgrowth ECs cultured from MNCs or blood vessel ECs by Ingram and Yoder et al. (Ingram et al., 2004; Ingram et al., 2005a). These proliferative endothelial lineage cells were termed ECFC, also known in the literature as EOCs or late EPCs. ECFCs do not likely represent the primary “EPC in vivo” phenotype found in our body but rather reflect an EC phenotype emerging from cultured cells (Lin et al., 2000) or vessel wall ECs (Figure 1) (Ingram et al., 2005b). Questions regarding the definition of the primary EPC underlying the ECFC phenotype and in vivo origin are still missing. Initially, ECFCs were identified from the long-term culture of PB (Lin et al., 2000; Hur et al., 2004) or CB-MNCs (Ingram et al., 2004). These were followed by publication of Smadja et al. and Delorme et al., showing ECFCs can be derived from CD34+ cells or CD146+/CD34+/CD45+/CD133+ or CD177+ cells (Delorme et al., 2005; Smadja et al., 2005; Lee et al., 2013; Boisson-Vidal et al., 2018). Additional studies by Case et al. and Timmermans et al. revealed that CD34+/CD45− cells could give rise to ECFCs but not a hematopoietic colony (Case et al., 2007; Timmermans et al., 2007). A later publication by Tura et al. confirmed CD34+/CD146+/CD133− cells are the source of ECFC precursor cells (Tura et al., 2013). They also claimed ECFC precursors might not be derived from BM, because BM-MNCs did not contain CD34+/CD146+/CD133− cells and did not give rise to any ECFC colony. This discussion was partly supported by a recent publication using fluorescence in situ hybridization analysis of ECFCs derived from allogenic BM transplant patients (Fujisawa et al., 2019). The findings about the fraction of ECFC-producing cells in vivo are somewhat contradicting between the publications regarding CD45 positivity and CD133 positivity, while CD34 positivity is agreed by common consent.
ECFCs have been successfully isolated from not only CB and PB but also from fat tissue (Lin et al., 2013), placenta (Patel et al., 2013b), lungs (Alphonse et al., 2014) and saphenous vein (Green et al., 2017b); these results imply that ECFCs may derived from tissue resident vasculature progenitors. Recent reports indicate a candidate of ECFC origin locates in ECs of blood vessels (Salybekov et al., 2022). The mechanism of non-hEPC mobilization into circulation and physiological functions in kinetics are not defined yet.
Although the proof of BM-derived ECFCs is not approved by the former reports, there are several publication demonstrating that a pluripotent stem cell known as “very small embryonic-like stem cell” (VSEL) is a lineage-negative, CD34+, CD133+ and CD45− cell with very small size (<6 µm length) located in BM, and differentiate into ECFC-like ECs in human (Havens et al., 2014; Guerin et al., 2015; Lahlil et al., 2018; Domingues et al., 2022). Also other reports disclosed VSELs derived from cord blood CD34+ cell differentiated into ECFCs ex vivo (Lahlil et al., 2018) (Domingues et al., 2022). We need further insights and discussions regarding the origin of ECFCs.
Postnatal EPCs was thought closely related to that of HSC populations, both being isolated by cell surface antigens, including CD34, CD133, VEGFR-2, CXCR4, CD105, etc., in human, or c-Kit, Sca-1, Flk-1, etc., in mouse (Peichev et al., 2000; Quirici et al., 2001b; Eggermann et al., 2003; Handgretinger et al., 2003; Alessandri et al., 2004). However, the identification of an exact primary hEPC phenotype was missing, mainly due to the lack of a definitive assay system capable of determining and distinguishing an EPC unambiguously from an HSC.
The initially developed culture assays and colonies (Murohara et al., 2000b; Kalka et al., 2000; Hill et al., 2003) grouped multifarious EPCs into single qualitative class: “adhesive cultured EPCs”, without any hierarchy or proper identification of unwanted cells, while the colonies detected by Hill’s colony assay (Hill et al., 2003) turned out to consist of not colony-forming EPCs, but mainly other hematopoietic cells (Hur et al., 2007; Rohde et al., 2007). Later, an improved EPC-colony forming assay (EPC-CFA) was developed, challenging the field’s traditional views and opening the door to illustrating the developmental hierarchy of hEPCs (Masuda et al., 2011).
The EPC-CFA enables the description and discrimination of two different forms of EPC-CFUs derived from a single cell; small cell-sized EPCs as “primitive CF-EPCs”, which are probably derived from more immature/proliferative EPCs, and large cell-sized EPCs as “definitive CF-EPCs”, which have more tendency to differentiation and functional participation in neovasculogenesis-related process (Table 1). The definitive CF-EPCs are more talented to differentiate into a non-colonizing, large cell-sized EPC phenotype, parallel to classical EPC culture-derived EPCs (Dimmeler et al., 2001; Vasa et al., 2001) and are considered as newly emerging EPCs, leaving the colony-forming EPCs niche (Figure 1). Masuda et al. demonstrated that approximately 22.0% of a single CB-CD133+ gave rise to primitive or definitive EPC colonies (16.8% or 5.2%, respectively) in EPC-CFA. This assay can further be combined with an HPC colony assay to elucidate the rationale of a possible “bloodline” between EPCs and HPCs. EPC-CFA along with HPC-CFA, opens the door for additional visions into the origin and characteristics of hematopoiesis and neovasculogenesis in the adult (Masuda et al., 2011). An only CB-CD133+ cell could produce both EPC and HPC colonies at a rate of 36.3% after particular culture conditioning for 7 days.
Several former publications claimed that myeloid early EPCs like myeloid angiogenic cells (MACs) and circulating angiogenic cells (CACs) should not be involved in the EPC category, because they were not committing into totally differentiated ECs but functioning as myeloid cells to deliver angiogenic mediators (Medina et al., 2017). As this partly agreeable, myeloid EPCs are not only MACs or CACs but also progenitor cells that differentiate into ECs of blood vessel in tumors and regenerative tissues. Bailey et al. described common myeloid progenitors (CMPs) and granulocyte/macrophage progenitors (GMPs) could differentiate into functional ECs by 1.3% and 0.8%, respectively (Bailey et al., 2004; Bailey et al., 2006), followed by Romangnani et al. showed that CD14+/CD34low MNCs with stem cell phenotypic and functional features represent the major source of circulating EPCs with high vasculogenic property (Romagnani et al., 2005). Furthermore, the research flow of myeloid EPCs is extended to lymphatic EPCs, which are derived from myeloid cells. The concept of lymphatic vessel development has recently been revised by evidence rising from both mouse and human studies for the critical role of lymphatic EPCs (LEPCs) (Ran and Wilber, 2017; Ran and Volk-Draper, 2020). Adult LEPCs have been found to be derived from wide variety of ancestors, including HSC (Jiang et al., 2008), MSC (Conrad et al., 2009), ADSC (Yang et al., 2015), and myeloid stem or progenitor cells (Lee et al., 2010), with the myeloid lineage gaining popularity as the primary source (Maruyama et al., 2005; Zumsteg et al., 2009; Li et al., 2014). BM-derived immature myeloid cells recruit to inflamed and ischemic sites (Maruyama et al., 2005) and tumors (Allavena et al., 2008; Pollard, 2008), where they help to develop vasculature (Schoppmann et al., 2006; Ding et al., 2012). One of the compelling lines of evidence for myeloid EPCs is the ability to differentiate from primary blood monocytes under in vitro conditions, demonstrating de novo property of lymphatic phenotype expressing such as VEGFR3 and LYVE-1, endothelial-specific phenotype, and functional behaviors limited to vasculature cells (Van’t Hull et al., 2014; Tan et al., 2014), concurrent with the loss or reduction of stem, myeloid, and hematopoietic markers (Salven et al., 2003; Nguyen et al., 2009).
EPCs are thought to release autocrine elements for their nutritional support, as well as paracrine factors to develop vasculature and repair of impaired vessels (Figure 2). In vivo EPCs, isolated as CD34+ from PB or BM, have been reported to secrete numerous angiogenic elements, such as vascular endothelial growth factor (VEGF), hepatic growth factor (HGF), insulin growth factor-1 (IGF-1), interleukin-8 (IL-8), fibroblast growth factor (FGF) (Majka et al., 2001; Jarajapu et al., 2014) angiopoietin-1, platelet-derived growth factor (PDGF) (Fang et al., 2020), etc. Ex vivo EPCs such as early EPCs as well as ECFCs also represented a similar finding to express a large number of angiogenic factors although the level of VEGF and IL-8 were higher in early EPCs than ECFCs (Table 2) (Cheng et al., 2013).
Collaborative factors with the capacity to promote both immunomodulation and anti-inflammation, such as TGF-β, IL-10, IL-4, IL-6, PGE2, M-CSF, G-CSF, adenosine, etc., have been investigated for years and reported to be expressed by properly stimulated hematopoietic lineage cells (Motz and Coukos, 2011; Rivera and Bergers, 2015). This co-creative property inherently applies to EPCs in regenerative tissues. EPCs secrete multiple factors for both anti-inflammation and immunosuppression, such as VEGF (Jarajapu et al., 2014), PGE2 (Carneiro et al., 2019), TGF-β (Acosta et al., 2019), IL-4, IL-6 (Kado et al., 2018), IL-10 (Zullo et al., 2015) and G-CSF (Wen et al., 2019; Alwjwaj et al., 2021; Yan et al., 2022). Especially, IL-10 and TGF-β are expressed and play a key role in immunomodulation and anti-inflammation in CD34+ cells from PB or BM (Majka et al., 2001; Ratajczak et al., 2013; Jarajapu et al., 2014). IL-10 is a strong anti-inflammatory and immunoregulatory cytokine with a variety of direct and indirect effects on innate and adaptive immunity through the induction of M2 macrophages and Treg polarization (Mosser and Zhang, 2008). In acute myocardial infarction (MI) models, IL-10 suppresses in vivo inflammatory responses, which contributes to improved myocardial recovery. TGF-β1 is a multifunctional cytokine involved in a variety of essential activities, such as embryonic evolution, cellular development, wound recovery, and immunomodulation, which contributes to the anti-inflammatory feedback by encouraging the proliferation of Tregs and inhibiting the differentiation of Th cells (Mantel and Schmidt-Weber, 2011).
MicroRNAs (miRNAs) are a class of non-coding RNAs and have critical functions in the modification of gene expression. Most of them are transcribed from DNA into primary miRNAs, modified into initial miRNAs, and ultimately made into mature miRNAs (Mohr and Mott, 2015). Seminal study showed that CD34+ EPC secreted extracellular vesicles (EVs) and activated an angiogenic program in ECs through horizontal mRNA transfer (Table 3) (Deregibus et al., 2007; Sahoo et al., 2011; Xu et al., 2020; Xiong et al., 2022).
Cardiovascular diseases are based on pathological process of ischemia caused by circulatory insufficiency and inflammation induced by hematopoietic cell invasion, requiring angiogenic and anti-inflammatory microenvironment for tissue recovery. Preclinical studies have shown that EVs derived from CD34+ have superior therapeutic effects on various ischemic diseases (Sahoo et al., 2011; Bitzer et al., 2012; Mathiyalagan et al., 2017). Sahoo et al. have shown pro-angiogenic activity of EVs from conditioned media of mobilized human CD34+ cells (CD34+-EVs) possibly through miR-126 and 130a (Sahoo et al., 2011). According to Mathiyalagan and colleagues, silencing miR-126-3p in CD34+-EVs derived from G-CSF mobilized PB-MNCs eliminated their angiogenic behavior and other constructive activities in vitro as well as in vivo. In addition, infusion of CD34+-EVs improved miR-126-3p levels in mouse ischemic limbs while having no impact on endogenous miR-126-3p synthesis, implying a horizontal transfer of efficient miR-126-3p to the ischemia site (Mathiyalagan et al., 2017). Dellet et al. documented highly expressed miRs identified in EVs produced by CD31+/CD34+/CD146+ ECFC and ECFC obtained from umbilical cord blood (UCB)-MNCs, including miR-10a/b, miR-21-5p, miR-30a-5p, miR-126-5p, miR151a-3p, let-7 families, etc. (Dellett et al., 2017). However, EPC-EVs works for anti-inflammatory effect on microenvironment of ischemia tissues as well. Later, Venkat et al. reported that EVs derived from CD133+EPC administration increased miR-126 levels while decreasing in MCP-1 and VCAM1, leading to decreased cardiac inflammation and oxidative stress after stroke in T2DM mice (Venkat et al., 2021). Also, BM-ECFC-derived EVs mitigate atherosclerosis, inflammation response, and atherosclerosis-related endothelial dysfunction in the experimental mice DM models (Bai et al., 2020). Ke et al. also reported that PB-EPC-EVs including miR-363-3p and miR-218-5p have cardioprotective effects on MI damage by targeting JMY-related apoptosis and mesenchymal-endothelial transition (Ke et al., 2021). Zhuo et al. realized that Rab27a deletion (as one of the major genes involved in EV biogenesis) weakened the therapeutic functionality of EPCs in MI conditions. Mechanistically, Rab27a deletion inhibits the PI3K/cyclinD1/Akt/FoxO3a pathway and reduces EV secretion in CD34+/VEGFR-2+ EPCs (Zhou et al., 2021). In turn, it is worth noting that suppression of PI3K/Akt induces inflammatory cascades (Schabbauer et al., 2004; Zhao et al., 2019). Therefore, it could be concluded that EV knockouts in EPCs initiate the inflammation process, which is another reason for the anti-inflammatory properties of intact EPCs for CVDs.
Acute lung injury/acute respiratory distress syndrome (ALI/ARDS) mortality rate is approximately 40% and mostly caused by sepsis, pneumonia, severe traumas, etc. (Dushianthan et al., 2011). Initial reports showed that BM derived CD31+/Flk1+/CD34+-EV infusion significantly decreased LPS-induced lung inflammatory process, representing convincing anti-inflammatory effects of these cells. The histologic analysis of CD34+-EVs injected group revealed restricted edema, neutrophil recruitment, and cytokines/chemokines level reduction in the bronchoalveolar lavage (BAL) (Wu et al., 2018). From a mechanistic viewpoint, miR-126 is abundant in CB-EPC-EVs, and upregulation of miR-126-3p may target PIK3R2, whereas upregulation of miR-126-5p prevents HMGB1 from acting as an inflammatory alarmin and VEGF- from acting as a permeability factor (Zhou et al., 2019). EPC-EVs has constructive functions to improve ALI/ARDS consequences, and additional investigations are mandatory to outline the best EV delivery method to damaged tissue.
Sepsis is systemic inflammatory condition provoked by pathogens causing in tissue/organ malfunction. Recently, reports have underlined that EPC therapy has advantageous effects on sepsis models (Fan et al., 2014; Sun et al., 2020). Fan et al. demonstrated that CB-CD34+/KDR+ cells and SDF-1α administration synergistically improve septic animal survival through overexpression of miR-126 and -125b, key miRs in preservation of EC functionalities (Fan et al., 2014). Following, they revealed that the main EPC defensive effects on the microvascular after sepsis emergence occur through exosomes-mediated transfer of miRs like miR-126-3p and miR-126-5p (Zhou et al., 2018). Mechanistically, Zhang et al. suggested that EPCs mitigated sepsis-induced kidney injury by secreting miR-21-5p-containing exosomes through the silencing of RUNX1 (Zhang et al., 2021). EPC-EVs miR-126-3p and 5p suppress damage-associated molecular patterns (DAMP)-stimulated HMGB1 and vascular cell adhesion molecule 1 (VCAM1), however blockage of miR-126-3p and 5p by miR-126-3p/5p inhibitors troubled the valuable functions of EPC-exosomes. Therefore, EPC-EVs prevent unfavorable septic problems through miR-1263p/5p delivery (Zhou et al., 2018).
Hereby, we highlight a small portion of the numerous animal investigations that have been conducted to examine the regenerative application of CD34+ EPC therapy for hypoxic organ regeneration (Table 4).
According to studies, CB-CD34+ EPCs concentrated in the regenerating muscles and improved vasculogenesis and in a murine hindlimb ischemia model, leading to enhanced limb salvage and hemodynamic recovery (Murohara et al., 2000a; Schatteman et al., 2000; Madeddu et al., 2004). Collectively, CD34+ EPCs efficiently improve blood flow and vasculogenesis in the hindlimb ischemic models.
According to data from a mouse model of experimental acute myocardial infarction (AMI), human CD34+ cells imbedded in the hypoxic/ischemic region and shifted toward cardiomyocytes and ECs, which related to improved heart utility (Kawamoto et al., 2006). Shalaby et al. compared the efficacy of human CB-CD34+ and CB-MSCs for the regenerate of heart tissue by stimulation of neoangiogenesis. They reported that the CD34+-treated group notably reduced the infarct zone and the amplitude of the T-wave and increased VEGF, Ang-1, HIF-1α, and Tie-2 levels compared to the MSC-treated subjects, suggesting the supremacy of CD34+ therapy vs. MSCs in the induction of regenerative pothential (Shalaby et al., 2016). Supportively, human CD34+ cells increased angiogenesis and reduced pro-inflammatory cytokines in the infarct zones, leading to improved cardiac function in a rat AMI model (Kim et al., 2016). Moreover, Kawamoto et al. found that PB-derived CD34+ EPCs improved regional wall motion, fractional shortening, left ventricle ejection fraction (LVEF), and myocardial neovascularization (Kawamoto et al., 2001). Also, in another study, they showed that CD34+ EPCs increased capillary density and decreased the fibrosis rate of the infarct area in the experimental AMI model (Kawamoto et al., 2003). Supportively, Yeh et al. reported that human G-CSF mobilized PB-CD34+ cells increased angiogenesis through migration and retention into the myocardium and trans-differentiation into ECs in ischemic tissues (Yeh et al., 2003). Furthermore, transplanting of PB-CD34+ cells reduce structural changes in the infarct zone and eliminates any signs of inflammatory cell recruitment seen in the transplantation of total MNCs in a rat MI model (Kawamoto et al., 2006). Also, myocardial injection of BM-CD34+ cells improved the vascular network and fractional shortening in the macaque MI model, supporting the efficacy of CD34+ cells in a large animal model of MI (Yoshioka et al., 2005). Taken together, records from preclinical studies propose that CD34+ cells differentiate into ECs, integrate into vascular system, and secrete angiogenic factors, promoting vascular regeneration in the microcirculation and improving myocardial perfusion in ischemia-induced tissue damage.
The effectiveness of CD34+ EPC therapy on the protection of CKD (chronic kidney disease) renal function has only been documented in a small number of experimental investigations. Two previous experimental studies using small and large preclinical experiments of CKD revealed that renal CD34+ EPC therapy considerably refurbished kidney function. In detail, Sangidorj et al. demonstrated that the administration of BM-CD34+ EPCs immunologically trafficked in the injured kidney site and postponed the collapse of kidney function aside from reduced proteinuria. As well, angiogenic molecules were increased and proinflammatory cytokines and adhesion molecules were decreased, resulting in functional and structural renal preservation in a mouse model of chronic renal failure (Sangidorj et al., 2010). In a large animal model, Chade et al. reported that intrarenal autologous PB-derived KDR+/CD34+ EPC injection preserved microvascular architecture and function and diminished microvascular remodeling in an experimental stenotic kidney, showing renoprotective effects of CD34+ EPC in a pig model of chronic renal artery stenosis (Chade et al., 2009). Also, Ohtake et al. reported the effectiveness of G-CSF mobilized human CD34+ therapy in a mice experimental model of ischemic/reperfusion acute kidney injury (AKI), which drastically upgraded renal function and repaired loss of peritubular capillaries because of ischemic condition (Ohtake et al., 2018a). Mechanistically, Vinas et al. reported that delivery of ECFC exosomes reduces ischemic kidney injury via transfer of miR-486-5p through targeting PTEN signaling pathway (Viñas et al., 2016). Recently, Huang et al. demonstrated that PB-CD34+ EPC therapy excellently inhibits CKD progression and kidney homeostasis deterioration by means of improvement of neoangiogenesis, blood stream, and potent anti-oxidative, anti-inflammatory, and anti-apoptosis/fibrosis capacities in a rat model (Huang et al., 2015). Taken together, the present knowledge regarding CD34+ therapy for kidney-related diseases supports CD34+ as a promising therapeutic intervention for preserving kidney functions in renovascular disease.
As above mentioned, CD34+ cells supply anti-inflammatory factors via paracrine activities. Comparing the cardioprotective effects of MSCs and CD34+ cells, Shalaby et al. demonstrated that CB-CD34+ cell therapy significantly decreased recruitment of inflammatory cells and increased angiogenic factors compared to the MSCs-treated group, which discloses the superiority of CD34+ therapy over MSCs in therapeutic potential (Shalaby et al., 2016). Of note, CB-derived CD133+/CD34+ stem/progenitors exhibit elevated amount of migratory (CXCR4)- and adhesive (LFA-1)-related antigens, allowing them to migrate and home to inflammatory areas that express higher levels of SDF-1α, a CXCR4 ligand (Das et al., 2009). Furthermore, CD34+ cell therapy reduces the secretion of inflammatory factors, namely TNF-α, IL-1, IL-6, and NOS2A in the lesion context while overexpression of IL-10. Moreover, human CB-CD34+ therapy mitigates NF-κB signaling pathway in dermal fibroblasts by increasing IL-10, providing novel mechanistic evidence of CD34+ cell-mediated anti-inflammatory response in a mouse model of wound healing. It worth noting that IL-10 binds to NF-κB and inhibits transcriptional function of this pathway (Kanji et al., 2014). Also, it has been shown that CD34+ cells enhanced epicardial and coronary microcirculation via paracrine activity–mediated angiogenic response and secretion of anti-inflammatory factors (Mackie and Losordo, 2011).
Regarding the therapeutic effects of CD34+ cells in cerebral ischemia, Taguchi et al. have clarified that CB-derived CD34+ cells ameliorated angiogenesis and neurogenesis rates (Taguchi et al., 2004). Of note, injections of PB-derived CD34+ cells developed vasculature, neurogenesis, and locomotor activity in a chronic stroke rat experiment (Shyu et al., 2006). Supportively, Tsuji and colleagues reported that CB-CD34+ cells increased blood stream to the ischemic zone and decreased missing of ipsilateral hemisphere volume in a neonatal stroke model (Tsuji et al., 2014). Also, CB-CD34+ cells alleviates pathological brain injury and improves the neurobehavioral status in a cerebral palsy model (Chang et al., 2021). More recently, Ogawa et al. illustrated that transplantation of PB-CD34+ cells improved neurological functions, including grip strength test (to evaluate neuromuscular function), the water maze assay (to evaluate the spatial education skill), and the rotarod test (to evaluate sensorimotor skills) in a murine SCID chronic stroke model, providing another preclinical proof of the CD34+ therapy concept in ischemic strokes (Ogawa et al., 2022).
As a result of strong preclinical background supporting CD34+ cells’ safety and efficacy as a treatment for injured tissues, clinical trials are now underway to use their innate abilities in the context of ischemic disorders (Leuschner et al., 2012). Here, we highlight the significant turning points of CD34+ cell therapy in clinical trials (Table 5).
For the first time, Tateishi et al. introduced the clinical utility of PB-CD34+ cells in an ischemic limb setting. Based on their results, autologous BM-CD34+ therapy ameliorate pain rate and increase walking distance 7 days after transplantation (Tateishi-Yuyama et al., 2002).
In a phase I/IIa trial of CLTI, Kawamoto and coworkers illustrated that G-CSF mobilized CD34+ cell transplantation enhanced pain grade, pain-free walking distance, toe brachial pressure index (TBPI), transcutaneous partial pressure of oxygen (TcPO2), and decreased ulcer size after 3 months of injection (Kawamoto et al., 2009); Supportively, Kinoshita et al. found that TBPI and TcPO2 parameters improved significantly 4 years after treatment, and no major amputations occurred in this period, indicating the immunity, feasibility, and efficiency of G-CSF mobilized PB-CD34+ cell transplantation in CLTI individuals (Kinoshita et al., 2012). Fujita et al. conducted a phase II clinical trial to determine endpoint selection and timing, which revealed significant improvements in perfusion pressure, pain grade, TcPO2, pain-free walking distance, and TBPI (Fujita et al., 2014). Ohtake et al. reported that G-CSF-mobilized CD34+ therapy increased the amputation- and CLTI-free survival index in CLTI individuals without any cell therapy-related side effects (Ohtake et al., 2018b). Tanaka and colleagues studied five dialysis individuals having non-healing diabetic feet in a phase I/IIa trial of G-CSF-mobilized CD34+ therapy. 18 weeks after treatment, all of the patients’ wounds had healed completely without serious adverse events (Tanaka et al., 2014). According to the initial randomized and double-blind pilot study regarding CD34+ therapy of CLTI, autologous G-CSF mobilized CD34+ therapy boosts myogenic and angiogenic activity while decreasing the amputation-free survival rate in a dose-dependent trend at 12 months (Losordo et al., 2012). This finding was later confirmed by a double-blind randomized pilot trial, which suggested that patients with severe intermittent claudication receiving autologous G-CSF mobilized CD34+ therapy experienced improved quality of life and longer walking distances (Losordo et al., 2011a). Fang et al. evaluated the long-term efficacy of G-CSF mobilized CD34+ cells in CLTI patients. Based on their results, CD34+ cells not only increased the amputation-free survival rate, peak pain-free walking time (PPFWT), ulcer healing rate, and SF-36v2 score, but also decreased the Wong-Baker faces pain rating scale and the recurrence frequency during 5years follow-up period (Fang et al., 2018).
It is worth noting that since 2017, Japan’s ministry of health (MHLW) has permitted the G-CSF-mobilized CD34+ cell transplantation for CLTI patients as an advanced type B medical treatment. In addition, a multicenter comparative trial of CLTI subjects aimed at guiding authorization of CD34+ therapy as a novel regenerative medicine-made merchandise (Identifier: NCT02501018) was launched in Japan in 2017. The MHLW designated this regenerative medicine product as an applicable pharmaceutical composition for Sakigake Strategy in 2018. The trial was finished in May 2022 with the concluding data still pending.
Coronary microvascular dysfunction leads to angina and poor outcomes in ischemic coronary artery disease, and no specific treatment exists. As mentioned above, CD34+ cell transplantation expands microcirculation and improves symptoms, exercise tolerance, and mortality in preclinical studies of refractory angina patients. Nowadays, CD34+ transplantation has been assessed in several double-blind and randomized trials in these patients. In phase I/IIa clinical trial using the NOGATM mapping system, autologous G-CSF mobilized PB-CD34+ therapy improved the frequency of angina, exercise tolerance, and CCS scale, providing preliminary evidence of the immunity and bioactivity of CD34+ cell injection in patients with refractory angina (Losordo et al., 2007). Furthermore, in a prospective, double-blind, randomized, phase II study of refractory angina, autologous G-CSF mobilized CD34+ therapy considerably decreased angina frequency and upgraded exercise endurance, angina onset time, and CCS classification without any adverse cardiovascular side effects (Losordo et al., 2011b). A second year of follow-up study shows that autologous G-CSF mobilized CD34+ cell transplantation diminishes angina frequency, unwanted heart outcomes, and mortality rates (Henry et al., 2016). These findings are further validated in phase III of the randomized, double-blind study, which shows improved immunity and organ homeostasis in refractory angina patients undergo autologous G-CSF mobilized CD34+ therapy (Povsic et al., 2016). Most recently, Henry et al. demonstrated that autologous G-CSF mobilized CD34+ cells improves coronary flow reserve, CCS class, and life quality (based on the Seattle Angina Questionnaire (SAQ)) and mitigates angina frequency at 6 months after treatment (Henry et al., 2022). Supportively, Corban et al. documented that autologous G-CSF mobilized CD34+ therapy improved microvascular blood flow and SAQ scores and decreased CCS class, nitroglycerin use, and Wilcoxon signed-rank test at 6 months of follow-up (Corban et al., 2022). Johnson et al. reported that autologous G-CSF mobilized CD34+ therapy for refractory angina patients is associated with an improved mortality rate and reduced cardiac-related hospitalization in the year following injection compared to the year prior to transplantation (Johnson et al., 2020). In a one more general note, it could be said that CD34+ therapy is immune and could be a potent operative regenerative approach for microvascular dysfunction and angina patients.
According to Hofmann et al. injected CD34+ cells may effectively accumulate in the area of ischemia and improve cardiac regeneration, While a significant portion of the injected-MNCs cramped in the spleen, liver, and the infarcted myocardium’s center, providing another proof of the superiority of CD34+ cells over MNCs (Hofmann et al., 2005). In 2009, Pasquet et al. launched a pilot study using autologous G-CSF-mobilized PB-CD34+ cells to treat AMI subjects, which resulted in increased LVEF and sustained structural/functional scar repair (Pasquet et al., 2009). In a phase I dose-dependent investigation of subjects with LVEF≤ 50% after angioplasty for STEMI, Quyyumi et al. found that BM-CD34+ cells improved myocardial perfusion and reduced infarct size in a dose-dependent manner (Quyyumi et al., 2011). The PreSERVE-AMI was the largest randomized phase II trial that used autologous BM-CD34+ cell transplantation of LV dysfunction post-STEMI. After 12 months, transplanted subjects showed decreased infarct size, hospitalized days, and mortality compared to the non-treated group and increased LVEF in a dose-dependent manner, providing additional proof of the immunity and efficiency of CD34+ therapy in STEMI subjects (Quyyumi et al., 2017). Besides, Poglajen et al. demonstrated potential antiarrhythmic benefits of autologous G-CSF mobilized CD34+ cells on ventricular arrhythmias subjects (Poglajen et al., 2019). These findings further supported the immunity and efficiency of CD34+ cell transplantation in STEMI-suffered individuals.
According to the first report of using autologous G-CSF mobilized CD34+ therapy in acute ischemic stroke patients, CD34+ cells improved modified rankin score (MRS) and national institutes of health stroke score (NIHSS) in the 6-month follow-up (Banerjee et al., 2014). Similarly, Chen et al. showed in a randomized, single-blind, controlled trial that autologous G-CSF mobilized CD34+ therapy improved the NIHSS, the European stroke score (ESS), and the ESS motor score without any serious adverse events in patients with a MCAI (Chen et al., 2014). Furthermore, Sung et al. reported that autologous G-CSF mobilized PB-CD34+ cell transplantation increased angiogenesis, the Barthel index, and cognitive ability screening instrument score (CASI) at 6-month follow-up after transplantation without any recurrent stroke or tumorigenesis (Sung et al., 2018). Based on these findings, a randomized double-blind study of CD34+ therapy in chronic ischemic stroke (Identifier: jRCT2052200112) began in 2020 and is still recruiting subjects.
Regarding CD34+ clinical trials of kidney disease, in a randomized controlled study, Yang et al. recently found that intra-renal transplantation of autologous G-CSF mobilized CD34+ cells for CKD patients significantly reduced adverse clinical outcomes (dialysis and death) at 1 year compared to the control group, but did not improve kidney function (Yang et al., 2020). Furthermore, according to Lee et al., G-CSF-mobilized CD34+ transplantation into the right renal artery is 100% safe and maintains the renal function in a stationary state, suggesting that CD34+ transplantation could mitigate the collapse of renal homeostasis in CKD subjects (Lee et al., 2017). Besides, in phase I/II study, Suzuki and colleagues reported that direct injection of G-CSF-mobilized CD34+ cells to both renal arteries of a 36 years old AKI patient significantly improved the serum creatinine level, GFR, angiogenic-related cytokines, and consequently kidney function 23 weeks after cell therapy without major adverse events (Suzuki et al., 2021). Therefore, CD34+ cell transplantation could apply renoprotective effects by improved angiogenesis and anti-inflammation capability and could be considered a new therapeutic tool for kidney disorders.
Since the discovery of CD34+ population in PB as the EPC-fortified fraction in 1997, a growing body of preclinical and clinical studies using CD34+ cells, a naturally occurring microvascular repair cell, provide undeniable evidence for the immunity and efficiency of CD34+ EPC treatment. In fact, until now, there has been no unsuccessful CD34+ cell therapy studies for tissue regeneration that has failed to provide a piece of evidence for safety and clinical effect. Remarkably, these reports were carried out on patients who were at the end of their treatment options. Despite being given to these seriously ill patients, a single CD34+ cell therapy administration has produced convincing proof that ischemia can be reversed. Principally, a multicenter trial in CLTI-suffered individuals was initiated in 2017in Japan with the aim of guiding authorization of CD34+ therapy as a novel regenerative medicine-made merchandise, which has been nominated as an applicable approach for the Sakigake Strategy. In case of therapeutically approval of this product from now on, it is anticipated to be a breakthrough for developing signals for diseases aside from CLTI. Furthermore, in the United States, the FDA has designated CD34+ cell products as “regenerative medicine advanced therapy” for refractory angina. If these studies are completed, approved, and expanded successfully, CD34+ therapy may result in the world’s first approval of a regenerative therapeutic approach for CVD and other vascular-related diseases.
TA designed the study, prepared figures, and put forward the conception; MH literature review and wrote the manuscript; SK, AS, and TA contributed to manuscript writing and put forward the conception. All authors reviewed and approved the manuscript.
The authors wish to thank the personnel of the Shonan Research Institute of Innovative Medicine and the Center for Cell therapy and Regenerative Medicine of Shonan Kamakura General Hospital for kindest guidance and help.
The authors declare that the research was conducted in the absence of any commercial or financial relationships that could be construed as a potential conflict of interest.
All claims expressed in this article are solely those of the authors and do not necessarily represent those of their affiliated organizations, or those of the publisher, the editors and the reviewers. Any product that may be evaluated in this article, or claim that may be made by its manufacturer, is not guaranteed or endorsed by the publisher.
Acosta, S. A., Lee, J. Y., Nguyen, H., Kaneko, Y., and Borlongan, C. V. (2019). Endothelial progenitor cells modulate inflammation-associated stroke vasculome. Stem Cell Rev. Rep. 15 (2), 256–275. doi:10.1007/s12015-019-9873-x
Alessandri, G., Emanueli, C., and Madeddu, P. (2004). Genetically engineered stem cell therapy for tissue regeneration. Ann. N. Y. Acad. Sci. 1015, 271–284. doi:10.1196/annals.1302.023
Allavena, P., Sica, A., Solinas, G., Porta, C., and Mantovani, A. (2008). The inflammatory micro-environment in tumor progression: The role of tumor-associated macrophages. Crit. Rev. Oncol. Hematol. 66 (1), 1–9. doi:10.1016/j.critrevonc.2007.07.004
Alphonse, R. S., Vadivel, A., Fung, M., Shelley, W. C., Critser, P. J., Ionescu, L., et al. (2014). Existence, functional impairment, and lung repair potential of endothelial colony-forming cells in oxygen-induced arrested alveolar growth. Circulation 129 (21), 2144–2157. doi:10.1161/CIRCULATIONAHA.114.009124
Alwjwaj, M., Kadir, R. R. A., and Bayraktutan, U. (2021). The secretome of endothelial progenitor cells: A potential therapeutic strategy for ischemic stroke. Neural Regen. Res. 16 (8), 1483–1489. doi:10.4103/1673-5374.303012
Asahara, T., Kawamoto, A., and Masuda, H. (2011). Concise review: Circulating endothelial progenitor cells for vascular medicine. Stem Cells 29 (11), 1650–1655. doi:10.1002/stem.745
Asahara, T., Murohara, T., Sullivan, A., Silver, M., and van der Zee, R. (1997). Isolation of putative progenitor endothelial cells for angiogenesis. Science 275 (5302), 964–967. doi:10.1126/science.275.5302.964
Bai, S., Yin, Q., Dong, T., Dai, F., Qin, Y., Ye, L., et al. (2020). Endothelial progenitor cell–derived exosomes ameliorate endothelial dysfunction in a mouse model of diabetes. Biomed. Pharmacother. 131, 110756. doi:10.1016/j.biopha.2020.110756
Bailey, A. S., Jiang, S., Afentoulis, M., Baumann, C. I., Schroeder, D. A., Olson, S. B., et al. (2004). Transplanted adult hematopoietic stems cells differentiate into functional endothelial cells. Blood 103 (1), 13–19. doi:10.1182/blood-2003-05-1684
Bailey, A. S., Willenbring, H., Jiang, S., Anderson, D. A., Schroeder, D. A., Wong, M. H., et al. (2006). Myeloid lineage progenitors give rise to vascular endothelium. Proc. Natl. Acad. Sci. U. S. A. 103 (35), 13156–13161. doi:10.1073/pnas.0604203103
Banerjee, S., Bentley, P., Hamady, M., Marley, S., Davis, J., Shlebak, A., et al. (2014). Intra-arterial immunoselected CD34+ stem cells for acute ischemic stroke. Stem cells Transl. Med. 3 (11), 1322–1330. doi:10.5966/sctm.2013-0178
Bitzer, M., Ben-Dov, I. Z., and Thum, T. (2012). Microparticles and microRNAs of endothelial progenitor cells ameliorate acute kidney injury. Kidney Int. 82 (4), 375–377. doi:10.1038/ki.2012.152
Boisson-Vidal, C., Benslimane-Ahmim, Z., Lokajczyk, A., Heymann, D., and Smadja, D. M. (2018). Osteoprotegerin induces CD34(+) differentiation in endothelial progenitor cells. Front. Med. (Lausanne) 5, 331. doi:10.3389/fmed.2018.00331
Carneiro, G. D., Sielski, M. S., Vieira, C. P., Costa, F. T. M., Werneck, C. C., and Vicente, C. P. (2019). Administration of endothelial progenitor cells accelerates the resolution of arterial thrombus in mice. Cytotherapy 21 (4), 444–459. doi:10.1016/j.jcyt.2019.01.005
Case, J., Mead, L. E., Bessler, W. K., Prater, D., White, H. A., Saadatzadeh, M. R., et al. (2007). Human CD34+AC133+VEGFR-2+ cells are not endothelial progenitor cells but distinct, primitive hematopoietic progenitors. Exp. Hematol. 35 (7), 1109–1118. doi:10.1016/j.exphem.2007.04.002
Chade, A. R., Zhu, X., Lavi, R., Krier, J. D., Pislaru, S., Simari, R. D., et al. (2009). Endothelial progenitor cells restore renal function in chronic experimental renovascular disease. Circulation 119 (4), 547–557. doi:10.1161/CIRCULATIONAHA.108.788653
Chang, Y., Lin, S., Li, Y., Liu, S., Ma, T., and Wei, W. (2021). Umbilical cord blood CD34+ cells administration improved neurobehavioral status and alleviated brain injury in a mouse model of cerebral palsy. Child's Nerv. Syst. 37 (7), 2197–2205. doi:10.1007/s00381-021-05068-0
Chen, D.-C., Lin, S. Z., Fan, J. R., Lin, C. H., Lee, W., Lin, C. C., et al. (2014). Intracerebral implantation of autologous peripheral blood stem cells in stroke patients: A randomized phase II study. Cell Transplant. 23 (12), 1599–1612. doi:10.3727/096368914X678562
Cheng, C.-C., Chang, S. J., Chueh, Y. N., Huang, T. S., Huang, P. H., Cheng, S. M., et al. (2013). Distinct angiogenesis roles and surface markers of early and late endothelial progenitor cells revealed by functional group analyses. BMC genomics 14 (1), 1–10. doi:10.1186/1471-2164-14-182
Conrad, C., Niess, H., Huss, R., Huber, S., von Luettichau, I., Nelson, P. J., et al. (2009). Multipotent mesenchymal stem cells acquire a lymphendothelial phenotype and enhance lymphatic regeneration in vivo. Circulation 119 (2), 281–289. doi:10.1161/CIRCULATIONAHA.108.793208
Corban, M. T., Toya, T., Albers, D., Sebaali, F., Lewis, B. R., Bois, J., et al. (2022). IMPROvE-CED trial: Intracoronary autologous CD34+ cell therapy for treatment of coronary endothelial dysfunction in patients with angina and nonobstructive coronary arteries. Circulation Res. 130 (3), 326–338. doi:10.1161/CIRCRESAHA.121.319644
Das, H., Abdulhameed, N., Joseph, M., Sakthivel, R., Mao, H. Q., and Pompili, V. J. (2009). Ex vivo nanofiber expansion and genetic modification of human cord blood-derived progenitor/stem cells enhances vasculogenesis. Cell Transplant. 18 (3), 305–318. doi:10.3727/096368909788534870
Dellett, M., Brown, E. D., Guduric-Fuchs, J., O'Connor, A., Stitt, A. W., Medina, R. J., et al. (2017). MicroRNA-containing extracellular vesicles released from endothelial colony-forming cells modulate angiogenesis during ischaemic retinopathy. J. Cell Mol. Med. 21 (12), 3405–3419. doi:10.1111/jcmm.13251
Delorme, B., Basire, A., Gentile, C., Sabatier, F., Monsonis, F., Desouches, C., et al. (2005). Presence of endothelial progenitor cells, distinct from mature endothelial cells, within human CD146+ blood cells. Thromb. Haemost. 94 (6), 1270–1279. doi:10.1160/TH05-07-0499
Deregibus, M. C., Cantaluppi, V., Calogero, R., Lo Iacono, M., Tetta, C., Biancone, L., et al. (2007). Endothelial progenitor cell derived microvesicles activate an angiogenic program in endothelial cells by a horizontal transfer of mRNA. Blood 110 (7), 2440–2448. doi:10.1182/blood-2007-03-078709
Dimmeler, S., Aicher, A., Vasa, M., Mildner-Rihm, C., Adler, K., Tiemann, M., et al. (2001). HMG-CoA reductase inhibitors (statins) increase endothelial progenitor cells via the PI 3-kinase/Akt pathway. J. Clin. Invest. 108 (3), 391–397. doi:10.1172/JCI13152
Ding, M., Fu, X., Tan, H., Wang, R., Chen, Z., and Ding, S. (2012). The effect of vascular endothelial growth factor C expression in tumor-associated macrophages on lymphangiogenesis and lymphatic metastasis in breast cancer. Mol. Med. Rep. 6 (5), 1023–1029. doi:10.3892/mmr.2012.1043
Domingues, A., Rossi, E., Bujko, K., Detriche, G., Richez, U., Blandinieres, A., et al. (2022). Human CD34(+) very small embryonic-like stem cells can give rise to endothelial colony-forming cells with a multistep differentiation strategy using UM171 and nicotinamide acid. Leukemia 36 (5), 1440–1443. doi:10.1038/s41375-022-01517-0
Dushianthan, A., Grocott, M. P. W., Postle, A. D., and Cusack, R. (2011). Acute respiratory distress syndrome and acute lung injury. Postgrad. Med. J. 87 (1031), 612–622. doi:10.1136/pgmj.2011.118398
Eggermann, J., Kliche, S., Jarmy, G., Hoffmann, K., Mayr-Beyrle, U., Debatin, K. M., et al. (2003). Endothelial progenitor cell culture and differentiation in vitro: A methodological comparison using human umbilical cord blood. Cardiovasc Res. 58 (2), 478–486. doi:10.1016/s0008-6363(03)00252-9
Fan, H., Goodwin, A. J., Chang, E., Zingarelli, B., Borg, K., Guan, S., et al. (2014). Endothelial progenitor cells and a stromal cell-derived factor-1α analogue synergistically improve survival in sepsis. Am. J. Respir. Crit. Care Med. 189 (12), 1509–1519. doi:10.1164/rccm.201312-2163OC
Fang, J., Huang, X., Han, X., Zheng, Z., Hu, C., Chen, T., et al. (2020). Endothelial progenitor cells promote viability and nerve regenerative ability of mesenchymal stem cells through PDGF-BB/PDGFR-β signaling. Aging (Albany NY) 12 (1), 106–121. doi:10.18632/aging.102604
Fang, Y., Wei, Z., Chen, B., Pan, T., Gu, S., Liu, P., et al. (2018). A five-year study of the efficacy of purified CD34+ cell therapy for angiitis-induced no-option critical limb ischemia. Stem cells Transl. Med. 7 (8), 583–590. doi:10.1002/sctm.17-0252
Flamme, I., and Risau, W. (1992). Induction of vasculogenesis and hematopoiesis in vitro. Development 116 (2), 435–439. doi:10.1242/dev.116.2.435
Friedrich, E. B., Walenta, K., Scharlau, J., Nickenig, G., and Werner, N. (2006). CD34−/CD133+/VEGFR-2+ endothelial progenitor cell subpopulation with potent vasoregenerative capacities. Circulation Res. 98 (3), e20–e25. doi:10.1161/01.RES.0000205765.28940.93
Fujisawa, T., Tura-Ceide, O., Hunter, A., Mitchell, A., Vesey, A., Medine, C., et al. (2019). Endothelial progenitor cells do not originate from the bone marrow. Circulation 140 (18), 1524–1526. doi:10.1161/CIRCULATIONAHA.119.042351
Fujita, Y., Kinoshita, M., Furukawa, Y., Nagano, T., Hashimoto, H., Hirami, Y., et al. (2014). Phase II clinical trial of CD34+ cell therapy to explore endpoint selection and timing in patients with critical limb ischemia. Circulation J. 78 (2), 490–501. doi:10.1253/circj.cj-13-0864
Gehling, U. M., Ergün, S., Schumacher, U., Wagener, C., Pantel, K., Otte, M., et al. (2000). In vitro differentiation of endothelial cells from AC133-positive progenitor cells. Blood 95 (10), 3106–3112. doi:10.1182/blood.v95.10.3106.010k08_3106_3112
Green, L., Ofstein, R. H., Rapp, B., Saadatzadeh, M. R., Bhavsar, J. R., Fajardo, A., et al. (2017). Adult venous endothelium is a niche for highly proliferative and vasculogenic endothelial colony-forming cells. J. Vasc. Surg. 66 (6), 1854–1863. doi:10.1016/j.jvs.2016.11.059
Green, L., Ofstein, R. H., Rapp, B., Saadatzadeh, M. R., Bhavsar, J. R., Fajardo, A., et al. (2017). Adult venous endothelium is a niche for highly proliferative and vasculogenic endothelial colony-forming cells. J. Vasc. Surg. 66 (6), 1854–1863. doi:10.1016/j.jvs.2016.11.059
Guerin, C. L., Loyer, X., Vilar, J., Cras, A., Mirault, T., Gaussem, P., et al. (2015). Bone-marrow-derived very small embryonic-like stem cells in patients with critical leg ischaemia: Evidence of vasculogenic potential. Thromb. Haemost. 113 (5), 1084–1094. doi:10.1160/TH14-09-0748
Handgretinger, R., Gordon, P. R., Leimig, T., Chen, X., Buhring, H. J., Niethammer, D., et al. (2003). Biology and plasticity of CD133+ hematopoietic stem cells. Ann. N. Y. Acad. Sci. 996, 141–151. doi:10.1111/j.1749-6632.2003.tb03242.x
Havens, A. M., Sun, H., Shiozawa, Y., Jung, Y., Wang, J., Mishra, A., et al. (2014). Human and murine very small embryonic-like cells represent multipotent tissue progenitors, in vitro and in vivo. Stem Cells Dev. 23 (7), 689–701. doi:10.1089/scd.2013.0362
Henry, T. D., Bairey Merz, C. N., Wei, J., Corban, M. T., Quesada, O., Joung, S., et al. (2022). Autologous CD34+ stem cell therapy increases coronary flow reserve and reduces angina in patients with coronary microvascular dysfunction. Circ. Cardiovasc. Interv. 15 (2), e010802. doi:10.1161/CIRCINTERVENTIONS.121.010802
Henry, T. D., Schaer, G. L., Traverse, J. H., Povsic, T. J., Davidson, C., Lee, J. S., et al. (2016). Autologous CD34+ cell therapy for refractory angina: 2-year outcomes from the ACT34-CMI study. Cell Transplant. 25 (9), 1701–1711. doi:10.3727/096368916X691484
Hill, J. M., Zalos, G., Halcox, J. P. J., Schenke, W. H., Waclawiw, M. A., Quyyumi, A. A., et al. (2003). Circulating endothelial progenitor cells, vascular function, and cardiovascular risk. N. Engl. J. Med. 348 (7), 593–600. doi:10.1056/NEJMoa022287
Hofmann, M., Wollert, K. C., Meyer, G. P., Menke, A., Arseniev, L., Hertenstein, B., et al. (2005). Monitoring of bone marrow cell homing into the infarcted human myocardium. Circulation 111 (17), 2198–2202. doi:10.1161/01.CIR.0000163546.27639.AA
Huang, T. H., Chen, Y. T., Sung, P. H., Chiang, H. J., Chen, Y. L., Chai, H. T., et al. (2015). Peripheral blood-derived endothelial progenitor cell therapy prevented deterioration of chronic kidney disease in rats. Am. J. Transl. Res. 7 (5), 804–824.
Hur, J., Yang, H. M., Yoon, C. H., Lee, C. S., Park, K. W., Kim, J. H., et al. (2007). Identification of a novel role of T cells in postnatal vasculogenesis: Characterization of endothelial progenitor cell colonies. Circulation 116 (15), 1671–1682. doi:10.1161/CIRCULATIONAHA.107.694778
Hur, J., Yoon, C. H., Kim, H. S., Choi, J. H., Kang, H. J., Hwang, K. K., et al. (2004). Characterization of two types of endothelial progenitor cells and their different contributions to neovasculogenesis. Arterioscler. Thromb. Vasc. Biol. 24 (2), 288–293. doi:10.1161/01.ATV.0000114236.77009.06
Ingram, D. A., Caplice, N. M., and Yoder, M. C. (2005). Unresolved questions, changing definitions, and novel paradigms for defining endothelial progenitor cells. Blood 106 (5), 1525–1531. doi:10.1182/blood-2005-04-1509
Ingram, D. A., Mead, L. E., Moore, D. B., Woodard, W., Fenoglio, A., and Yoder, M. C. (2005). Vessel wall-derived endothelial cells rapidly proliferate because they contain a complete hierarchy of endothelial progenitor cells. Blood 105 (7), 2783–2786. doi:10.1182/blood-2004-08-3057
Ingram, D. A., Mead, L. E., Tanaka, H., Meade, V., Fenoglio, A., Mortell, K., et al. (2004). Identification of a novel hierarchy of endothelial progenitor cells using human peripheral and umbilical cord blood. Blood 104 (9), 2752–2760. doi:10.1182/blood-2004-04-1396
Jarajapu, Y. P., Hazra, S., Segal, M., Li Calzi, S., Jadhao, C., Qian, K., et al. (2014). Vasoreparative dysfunction of CD34+ cells in diabetic individuals involves hypoxic desensitization and impaired autocrine/paracrine mechanisms. PLoS One 9 (4), e93965. doi:10.1371/journal.pone.0093965
Jiang, S., Bailey, A. S., Goldman, D. C., Swain, J. R., Wong, M. H., Streeter, P. R., et al. (2008). Hematopoietic stem cells contribute to lymphatic endothelium. PLoS One 3 (11), e3812. doi:10.1371/journal.pone.0003812
Jiang, S., Walker, L., Afentoulis, M., Anderson, D. A., Jauron-Mills, L., Corless, C. L., et al. (2004). Transplanted human bone marrow contributes to vascular endothelium. Proc. Natl. Acad. Sci. U. S. A. 101 (48), 16891–16896. doi:10.1073/pnas.0404398101
Johnson, G. L., Henry, T. D., Povsic, T. J., Losordo, D. W., Garberich, R. F., Stanberry, L. I., et al. (2020). CD34+ cell therapy significantly reduces adverse cardiac events, health care expenditures, and mortality in patients with refractory angina. Stem Cells Transl. Med. 9 (10), 1147–1152. doi:10.1002/sctm.20-0046
Kado, M., Tanaka, R., Arita, K., Okada, K., Ito-Hirano, R., Fujimura, S., et al. (2018). Human peripheral blood mononuclear cells enriched in endothelial progenitor cells via quality and quantity controlled culture accelerate vascularization and wound healing in a porcine wound model. Cell Transplant. 27 (7), 1068–1079. doi:10.1177/0963689718780307
Kalka, C., Masuda, H., Takahashi, T., Kalka-Moll, W. M., Silver, M., Kearney, M., et al. (2000). Transplantation of ex vivo expanded endothelial progenitor cells for therapeutic neovascularization. Proc. Natl. Acad. Sci. U. S. A. 97 (7), 3422–3427. doi:10.1073/pnas.97.7.3422
Kanji, S., Das, M., Aggarwal, R., Lu, J., Joseph, M., Basu, S., et al. (2014). Nanofiber-expanded human umbilical cord blood-derived CD34+ cell therapy accelerates murine cutaneous wound closure by attenuating pro-inflammatory factors and secreting IL-10. Stem Cell Res. 12 (1), 275–288. doi:10.1016/j.scr.2013.11.005
Kawamoto, A., Gwon, H. C., Iwaguro, H., Yamaguchi, J. I., Uchida, S., Masuda, H., et al. (2001). Therapeutic potential of ex vivo expanded endothelial progenitor cells for myocardial ischemia. Circulation 103 (5), 634–637. doi:10.1161/01.cir.103.5.634
Kawamoto, A., Iwasaki, H., Kusano, K., Murayama, T., Oyamada, A., Silver, M., et al. (2006). CD34-positive cells exhibit increased potency and safety for therapeutic neovascularization after myocardial infarction compared with total mononuclear cells. Circulation 114 (20), 2163–2169. doi:10.1161/CIRCULATIONAHA.106.644518
Kawamoto, A., Katayama, M., Handa, N., Kinoshita, M., Takano, H., Horii, M., et al. (2009). Intramuscular transplantation of G-CSF-mobilized CD34+ cells in patients with critical limb ischemia: A phase I/IIa, multicenter, single-blinded, dose-escalation clinical trial. Stem cells 27 (11), 2857–2864. doi:10.1002/stem.207
Kawamoto, A., Tkebuchava, T., Yamaguchi, J. I., Nishimura, H., Yoon, Y. S., Milliken, C., et al. (2003). Intramyocardial transplantation of autologous endothelial progenitor cells for therapeutic neovascularization of myocardial ischemia. Circulation 107 (3), 461–468. doi:10.1161/01.cir.0000046450.89986.50
Ke, X., Yang, R., Wu, F., Wang, X., Liang, J., Hu, X., et al. (2021). Exosomal miR-218-5p/miR-363-3p from endothelial progenitor cells ameliorate myocardial infarction by targeting the P53/JMY signaling pathway. Oxid. Med. Cell Longev. 2021, 5529430. doi:10.1155/2021/5529430
Kim, J.-H., Choi, S. C., Park, C. Y., Park, J. H., Choi, J. H., Joo, H. J., et al. (2016). Transplantation of immortalized CD34+ and CD34-adipose-derived stem cells improve cardiac function and mitigate systemic pro-inflammatory responses. PLoS One 11 (2), e0147853. doi:10.1371/journal.pone.0147853
Kinoshita, M., Fujita, Y., Katayama, M., Baba, R., Shibakawa, M., Yoshikawa, K., et al. (2012). Long-term clinical outcome after intramuscular transplantation of granulocyte colony stimulating factor-mobilized CD34 positive cells in patients with critical limb ischemia. Atherosclerosis 224 (2), 440–445. doi:10.1016/j.atherosclerosis.2012.07.031
Kocher, A., Schuster, M. D., Szabolcs, M. J., Takuma, S., Burkhoff, D., Wang, J., et al. (2001). Neovascularization of ischemic myocardium by human bone-marrow–derived angioblasts prevents cardiomyocyte apoptosis, reduces remodeling and improves cardiac function. Nat. Med. 7 (4), 430–436. doi:10.1038/86498
Lahlil, R., Scrofani, M., Barbet, R., Tancredi, C., Aries, A., and Hénon, P. (2018). VSELs maintain their pluripotency and competence to differentiate after enhanced ex vivo expansion. Stem Cell Rev. Rep. 14 (4), 510–524. doi:10.1007/s12015-018-9821-1
Lee, J. H., Lee, S. H., Yoo, S. Y., Asahara, T., and Kwon, S. M. (2013). CD34 hybrid cells promote endothelial colony-forming cell bioactivity and therapeutic potential for ischemic diseases. Arterioscler. Thromb. Vasc. Biol. 33 (7), 1622–1634. doi:10.1161/ATVBAHA.112.301052
Lee, J. Y., Park, C., Cho, Y. P., Lee, E., Kim, H., Kim, P., et al. (2010). Podoplanin-expressing cells derived from bone marrow play a crucial role in postnatal lymphatic neovascularization. Circulation 122 (14), 1413–1425. doi:10.1161/CIRCULATIONAHA.110.941468
Lee, M. S., Lee, F. Y., Chen, Y. L., Sung, P. H., Chiang, H. J., Chen, K. H., et al. (2017). Investigated the safety of intra-renal arterial transfusion of autologous CD34+ cells and time courses of creatinine levels, endothelial dysfunction biomarkers and micro-RNAs in chronic kidney disease patients-phase I clinical trial. Oncotarget 8 (11), 17750–17762. doi:10.18632/oncotarget.14831
Leuschner, F., Rauch, P. J., Ueno, T., Gorbatov, R., Marinelli, B., Lee, W. W., et al. (2012). Rapid monocyte kinetics in acute myocardial infarction are sustained by extramedullary monocytopoiesis. J. Exp. Med. 209 (1), 123–137. doi:10.1084/jem.20111009
Li, T., Wang, G. d., Tan, Y. z., and Wang, H. j. (2014). Inhibition of lymphangiogenesis of endothelial progenitor cells with VEGFR-3 siRNA delivered with PEI-alginate nanoparticles. Int. J. Biol. Sci. 10 (2), 160–170. doi:10.7150/ijbs.6719
Lin, R. Z., Moreno-Luna, R., Muñoz-Hernandez, R., Li, D., Jaminet, S. C. S., Greene, A. K., et al. (2013). Human white adipose tissue vasculature contains endothelial colony-forming cells with robust in vivo vasculogenic potential. Angiogenesis 16 (4), 735–744. doi:10.1007/s10456-013-9350-0
Lin, Y., Weisdorf, D. J., Solovey, A., and Hebbel, R. P. (2000). Origins of circulating endothelial cells and endothelial outgrowth from blood. J. Clin. Investigation 105 (1), 71–77. doi:10.1172/JCI8071
Losordo, D. W., Henry, T. D., Davidson, C., Sup Lee, J., Costa, M. A., Bass, T., et al. (2011). Intramyocardial, autologous CD34+ cell therapy for refractory angina. Circulation Res. 109 (4), 428–436. doi:10.1161/CIRCRESAHA.111.245993
Losordo, D. W., Kibbe, M. R., Mendelsohn, F., Martson, W., Driver, D. R., Carman, T., et al. (2011). Randomized, double-blind, placebo-controlled pilot trial of autologous CD34+ cell therapy for severe intermittent claudication: Interim results. Circ. Cardiovasc Interv. 5, 821. doi:10.1161/CIRCINTERVENTIONS.112.968321
Losordo, D. W., Kibbe, M. R., Mendelsohn, F., Marston, W., Driver, V. R., Sharafuddin, M., et al. (2012). A randomized, controlled pilot study of autologous CD34+ cell therapy for critical limb ischemia. Circ. Cardiovasc. Interv. 5 (6), 821–830. doi:10.1161/CIRCINTERVENTIONS.112.968321
Losordo, D. W., Schatz, R. A., White, C. J., Udelson, J. E., Veereshwarayya, V., Durgin, M., et al. (2007). Intramyocardial transplantation of autologous CD34+ stem cells for intractable angina: A phase I/IIa double-blind, randomized controlled trial. Circulation 115 (25), 3165–3172. doi:10.1161/CIRCULATIONAHA.106.687376
Mackie, A. R., and Losordo, D. W. (2011). CD34-positive stem cells in the treatment of heart and vascular disease in human beings. Tex. Heart Inst. J. 38 (5), 474–485.
Madeddu, P., Emanueli, C., Pelosi, E., Salis, M. B., Cerio, A. M., Bonanno, G., et al. (2004). Transplantation of low dose CD34+ KDR+ cells promotes vascular and muscular regeneration in ischemic limbs. FASEB J. 18 (14), 1737–1739. doi:10.1096/fj.04-2192fje
Majka, M., Janowska-Wieczorek, A., Ratajczak, J., Ehrenman, K., Pietrzkowski, Z., Kowalska, M. A., et al. (2001). Numerous growth factors, cytokines, and chemokines are secreted by human CD34(+) cells, myeloblasts, erythroblasts, and megakaryoblasts and regulate normal hematopoiesis in an autocrine/paracrine manner. Blood 97 (10), 3075–3085. doi:10.1182/blood.v97.10.3075
Mantel, P. Y., and Schmidt-Weber, C. B. (2011). Transforming growth factor-beta: Recent advances on its role in immune tolerance. Methods Mol. Biol. 677, 303–338. doi:10.1007/978-1-60761-869-0_21
Maruyama, K., Ii, M., Cursiefen, C., Jackson, D. G., Keino, H., Tomita, M., et al. (2005). Inflammation-induced lymphangiogenesis in the cornea arises from CD11b-positive macrophages. J. Clin. Invest. 115 (9), 2363–2372. doi:10.1172/JCI23874
Masuda, H., Alev, C., Akimaru, H., Ito, R., Shizuno, T., Kobori, M., et al. (2011). Methodological development of a clonogenic assay to determine endothelial progenitor cell potential. Circ. Res. 109, 20. doi:10.1161/CIRCRESAHA.110.231837
Mathiyalagan, P., Liang, Y., Kim, D., Misener, S., Thorne, T., Kamide, C. E., et al. (2017). Angiogenic mechanisms of human CD34(+) stem cell exosomes in the repair of ischemic hindlimb. Circ. Res. 120 (9), 1466–1476. doi:10.1161/CIRCRESAHA.116.310557
Medina, R. J., Barber, C. L., Sabatier, F., Dignat-George, F., Melero-Martin, J. M., Khosrotehrani, K., et al. (2017). Endothelial progenitors: A consensus statement on nomenclature. Stem Cells Transl. Med. 6 (5), 1316–1320. doi:10.1002/sctm.16-0360
Mohr, A. M., and Mott, J. L. (2015). Overview of microRNA biology. Seminars liver Dis. 35, 3. doi:10.1055/s-0034-1397344
Mosser, D. M., and Zhang, X. (2008). Interleukin-10: New perspectives on an old cytokine. Immunol. Rev. 226, 205–218. doi:10.1111/j.1600-065X.2008.00706.x
Motz, G. T., and Coukos, G. (2011). The parallel lives of angiogenesis and immunosuppression: Cancer and other tales. Nat. Rev. Immunol. 11 (10), 702–711. doi:10.1038/nri3064
Murohara, T., Ikeda, H., Duan, J., Shintani, S., Sasaki, K. i., Eguchi, H., et al. (2000). Transplanted cord blood-derived endothelial precursor cells augment postnatal neovascularization. J. Clin. Invest. 105 (11), 1527–1536. doi:10.1172/JCI8296
Murohara, T., Ikeda, H., Duan, J., Shintani, S., Sasaki, K. i., Eguchi, H., et al. (2000). Transplanted cord blood–derived endothelial precursor cells augment postnatal neovascularization. J. Clin. investigation 105 (11), 1527–1536. doi:10.1172/JCI8296
Nguyen, V. A., Fürhapter, C., Obexer, P., Stössel, H., Romani, N., and Sepp, N. (2009). Endothelial cells from cord blood CD133+CD34+ progenitors share phenotypic, functional and gene expression profile similarities with lymphatics. J. Cell Mol. Med. 13 (3), 522–534. doi:10.1111/j.1582-4934.2008.00340.x
Nielsen, J. S., and McNagny, K. M. (2008). Novel functions of the CD34 family. J. Cell Sci. 121 (22), 3683–3692. doi:10.1242/jcs.037507
Ogawa, Y., Okinaka, Y., Kikuchi-Taura, A., Saino, O., Tani-Yokoyama, A., Masuda, S., et al. (2022). Pre-clinical proof of concept: Intra-carotid injection of autologous CD34-positive cells for chronic ischemic stroke. Front. Med. 9, 681316. doi:10.3389/fmed.2022.681316
Ohtake, T., Kobayashi, S., Slavin, S., Mochida, Y., Ishioka, K., Moriya, H., et al. (2018). Human peripheral blood mononuclear cells incubated in vasculogenic conditioning medium dramatically improve ischemia/reperfusion acute kidney injury in mice. Cell Transplant. 27 (3), 520–530. doi:10.1177/0963689717753186
Ohtake, T., Mochida, Y., Ishioka, K., Oka, M., Maesato, K., Moriya, H., et al. (2018). Autologous granulocyte colony-stimulating factor-mobilized peripheral blood CD34 positive cell transplantation for hemodialysis patients with critical limb ischemia: A prospective phase II clinical trial. Stem cells Transl. Med. 7 (11), 774–782. doi:10.1002/sctm.18-0104
Pardanaud, L., Yassine, F., and Dieterlen-Lievre, F. (1989). Relationship between vasculogenesis, angiogenesis and haemopoiesis during avian ontogeny. Development 105 (3), 473–485. doi:10.1242/dev.105.3.473
Pasquet, S., Sovalat, H., Hénon, P., Bischoff, N., Arkam, Y., Ojeda-Uribe, M., et al. (2009). Long-term benefit of intracardiac delivery of autologous granulocyte–colony-stimulating factor-mobilized blood CD34+ cells containing cardiac progenitors on regional heart structure and function after myocardial infarct. Cytotherapy 11 (8), 1002–1015. doi:10.3109/14653240903164963
Patel, J., Seppanen, E., Chong, M. S. K., Yeo, J. S. L., Teo, E. Y. L., Chan, J. K. Y., et al. (2013). Prospective surface marker-based isolation and expansion of fetal endothelial colony-forming cells from human term placenta. Stem cells Transl. Med. 2 (11), 839–847. doi:10.5966/sctm.2013-0092
Patel, J., Seppanen, E., Chong, M. S. K., Yeo, J. S. L., Teo, E. Y. L., Chan, J. K. Y., et al. (2013). Prospective surface marker-based isolation and expansion of fetal endothelial colony-forming cells from human term placenta. Stem Cells Transl. Med. 2 (11), 839–847. doi:10.5966/sctm.2013-0092
Patel, J., Seppanen, E. J., Rodero, M. P., Wong, H. Y., Donovan, P., Neufeld, Z., et al. (2017). Functional definition of progenitors versus mature endothelial cells reveals key SoxF-dependent differentiation process. Circulation 135 (8), 786–805. doi:10.1161/CIRCULATIONAHA.116.024754
Peichev, M., Naiyer, A. J., Pereira, D., Zhu, Z., Lane, W. J., Williams, M., et al. (2000). Expression of VEGFR-2 and AC133 by circulating human CD34+ cells identifies a population of functional endothelial precursors. Blood, J. Am. Soc. Hematol. 95 (3), 952–958. doi:10.1182/blood.v95.3.952.003k27_952_958
Peters, B. A., Diaz, L. A., Polyak, K., Meszler, L., Romans, K., Guinan, E. C., et al. (2005). Contribution of bone marrow-derived endothelial cells to human tumor vasculature. Nat. Med. 11 (3), 261–262. doi:10.1038/nm1200
Poglajen, G., Zemljič, G., Cerar, A., Frljak, S., Jaklič, M., Androcec, V., et al. (2019). Transendocardial CD34+ cell therapy does not increase the risk of ventricular arrhythmias in patients with chronic heart failure. Cell Transplant. 28 (7), 856–863. doi:10.1177/0963689719840351
Pollard, J. W. (2008). Macrophages define the invasive microenvironment in breast cancer. J. Leukoc. Biol. 84 (3), 623–630. doi:10.1189/jlb.1107762
Povsic, T. J., Henry, T. D., Traverse, J. H., Fortuin, F. D., Schaer, G. L., Kereiakes, D. J., et al. (2016). The RENEW trial: Efficacy and safety of intramyocardial autologous CD34+ cell administration in patients with refractory angina. JACC Cardiovasc. Interv. 9 (15), 1576–1585. doi:10.1016/j.jcin.2016.05.003
Quirici, N., Soligo, D., Caneva, L., Servida, F., Bossolasco, P., and Deliliers, G. L. (2001). Differentiation and expansion of endothelial cells from human bone marrow CD133+ cells. Br. J. Haematol. 115 (1), 186–194. doi:10.1046/j.1365-2141.2001.03077.x
Quirici, N., Soligo, D., Caneva, L., Servida, F., Bossolasco, P., and Deliliers, G. L. (2001). Differentiation and expansion of endothelial cells from human bone marrow CD133(+) cells. Br. J. Haematol. 115 (1), 186–194. doi:10.1046/j.1365-2141.2001.03077.x
Quyyumi, A. A., Vasquez, A., Kereiakes, D. J., Klapholz, M., Schaer, G. L., Abdel-Latif, A., et al. (2017). PreSERVE-AMI: A randomized, double-blind, placebo-controlled clinical trial of intracoronary administration of autologous CD34+ cells in patients with left ventricular dysfunction post STEMI. Circulation Res. 120 (2), 324–331. doi:10.1161/CIRCRESAHA.115.308165
Quyyumi, A. A., Waller, E. K., Murrow, J., Esteves, F., Galt, J., Oshinski, J., et al. (2011). CD34+ cell infusion after ST elevation myocardial infarction is associated with improved perfusion and is dose dependent. Am. heart J. 161 (1), 98–105. doi:10.1016/j.ahj.2010.09.025
Ran, S., and Volk-Draper, L. (2020). Lymphatic endothelial cell progenitors in the tumor microenvironment. Adv. Exp. Med. Biol. 1234, 87–105. doi:10.1007/978-3-030-37184-5_7
Ran, S., and Wilber, A. (2017). Novel role of immature myeloid cells in formation of new lymphatic vessels associated with inflammation and tumors. J. Leukoc. Biol. 102 (2), 253–263. doi:10.1189/jlb.1MR1016-434RR
Ratajczak, J., Kucia, M., Mierzejewska, K., Marlicz, W., Pietrzkowski, Z., Wojakowski, W., et al. (2013). Paracrine proangiopoietic effects of human umbilical cord blood-derived purified CD133+ cells--implications for stem cell therapies in regenerative medicine. Stem Cells Dev. 22 (3), 422–430. doi:10.1089/scd.2012.0268
Risau, W., Sariola, H., Zerwes, H. G., Sasse, J., Ekblom, P., Kemler, R., et al. (1988). Vasculogenesis and angiogenesis in embryonic-stem-cell-derived embryoid bodies. Development 102 (3), 471–478. doi:10.1242/dev.102.3.471
Rivera, L. B., and Bergers, G. (2015). Intertwined regulation of angiogenesis and immunity by myeloid cells. Trends Immunol. 36 (4), 240–249. doi:10.1016/j.it.2015.02.005
Rohde, E., Bartmann, C., Schallmoser, K., Reinisch, A., Lanzer, G., Linkesch, W., et al. (2007). Immune cells mimic the morphology of endothelial progenitor colonies in vitro. Stem Cells 25 (7), 1746–1752. doi:10.1634/stemcells.2006-0833
Romagnani, P., Annunziato, F., Liotta, F., Lazzeri, E., Mazzinghi, B., Frosali, F., et al. (2005). CD14+CD34low cells with stem cell phenotypic and functional features are the major source of circulating endothelial progenitors. Circ. Res. 97 (4), 314–322. doi:10.1161/01.RES.0000177670.72216.9b
Sahoo, S., Klychko, E., Thorne, T., Misener, S., Schultz, K. M., Millay, M., et al. (2011). Exosomes from human CD34(+) stem cells mediate their proangiogenic paracrine activity. Circ. Res. 109 (7), 724–728. doi:10.1161/CIRCRESAHA.111.253286
Salven, P., Mustjoki, S., Alitalo, R., Alitalo, K., and Rafii, S. (2003). VEGFR-3 and CD133 identify a population of CD34+ lymphatic/vascular endothelial precursor cells. Blood 101 (1), 168–172. doi:10.1182/blood-2002-03-0755
Salybekov, A. A., Kobayashi, S., and Asahara, T. (2022). Characterization of endothelial progenitor cell: Past, present, and future. Int. J. Mol. Sci. 23 (14), 7697. doi:10.3390/ijms23147697
Sangidorj, O., Yang, S. H., Jang, H. R., Lee, J. P., Cha, R. h., Kim, S. M., et al. (2010). Bone marrow-derived endothelial progenitor cells confer renal protection in a murine chronic renal failure model. Am. J. Physiol. Ren. Physiol. 299 (2), F325–F335. doi:10.1152/ajprenal.00019.2010
Schabbauer, G., Tencati, M., Pedersen, B., Pawlinski, R., and Mackman, N. (2004). PI3K-Akt pathway suppresses coagulation and inflammation in endotoxemic mice. Arteriosclerosis, thrombosis, Vasc. Biol. 24 (10), 1963–1969. doi:10.1161/01.ATV.0000143096.15099.ce
Schatteman, G. C., Hanlon, H. D., Jiao, C., Dodds, S. G., and Christy, B. A. (2000). Blood-derived angioblasts accelerate blood-flow restoration in diabetic mice. J. Clin. investigation 106 (4), 571–578. doi:10.1172/JCI9087
Schoppmann, S. F., Fenzl, A., Nagy, K., Unger, S., Bayer, G., Geleff, S., et al. (2006). VEGF-C expressing tumor-associated macrophages in lymph node positive breast cancer: Impact on lymphangiogenesis and survival. Surgery 139 (6), 839–846. doi:10.1016/j.surg.2005.12.008
Shalaby, S. M., El-Shal, A. S., Zidan, H. E., Mazen, N. F., Abd El-Haleem, M. R., and Abd El Motteleb, D. M. (2016). Comparing the effects of MSCs and CD34+ cell therapy in a rat model of myocardial infarction. IUBMB life 68 (5), 343–354. doi:10.1002/iub.1487
Shi, Q., Rafii, S., Wu, M. H., Wijelath, E. S., Yu, C., Ishida, A., et al. (1998). Evidence for circulating bone marrow-derived endothelial cells. Blood 92 (2), 362–367. doi:10.1182/blood.v92.2.362.414k38_362_367
Shyu, W.-C., Lin, S. Z., Chiang, M. F., Su, C. Y., and Li, H. (2006). Intracerebral peripheral blood stem cell (CD34+) implantation induces neuroplasticity by enhancing beta1 integrin-mediated angiogenesis in chronic stroke rats. J. Neurosci. 26 (13), 3444–3453. doi:10.1523/JNEUROSCI.5165-05.2006
Smadja, D. M., Bièche, I., Uzan, G., Bompais, H., Muller, L., Boisson-Vidal, C., et al. (2005). PAR-1 activation on human late endothelial progenitor cells enhances angiogenesis in vitro with upregulation of the SDF-1/CXCR4 system. Arterioscler. Thromb. Vasc. Biol. 25 (11), 2321–2327. doi:10.1161/01.ATV.0000184762.63888.bd
Sun, R., Huang, J., and Sun, B. (2020). Mobilization of endothelial progenitor cells in sepsis. Inflamm. Res. 69 (1), 1–9. doi:10.1007/s00011-019-01299-9
Sung, P.-H., Lin, H. S., Lin, W. C., Chang, C. C., Pei, S. N., Ma, M. C., et al. (2018). Intra-carotid arterial transfusion of autologous circulatory derived CD34+ cells for old ischemic stroke patients-a phase I clinical trial to evaluate safety and tolerability. Am. J. Transl. Res. 10 (9), 2975–2989.
Suzuki, H., Ohtake, T., Tsukiyama, T., Morota, M., Ishioka, K., Moriya, H., et al. (2021). Acute kidney injury successfully treated with autologous granulocyte colony-stimulating factor-mobilized peripheral blood CD34-positive cell transplantation: A first-in-human report. Stem Cells Transl. Med. 10 (9), 1253–1257. doi:10.1002/sctm.20-0561
Suzuki, T., Nishida, M., Futami, S., Fukino, K., Amaki, T., Aizawa, K., et al. (2003). Neoendothelialization after peripheral blood stem cell transplantation in humans: A case report of a tokaimura nuclear accident victim. Cardiovasc Res. 58 (2), 487–492. doi:10.1016/s0008-6363(02)00780-0
Taguchi, A., Soma, T., Tanaka, H., Kanda, T., Nishimura, H., Yoshikawa, H., et al. (2004). Administration of CD34+ cells after stroke enhances neurogenesis via angiogenesisin a mouse model. J. Clin. investigation 114 (3), 330–338. doi:10.1172/JCI20622
Tan, Y. Z., Wang, H. j., Zhang, M. h., Quan, Z., and He, Q. z. (2014). CD34+ VEGFR-3+ progenitor cells have a potential to differentiate towards lymphatic endothelial cells. J. Cell Mol. Med. 18 (3), 422–433. doi:10.1111/jcmm.12233
Tanaka, R., Masuda, H., Kato, S., Imagawa, K., Kanabuchi, K., Nakashioya, C., et al. (2014). Autologous G-CSF-mobilized peripheral blood CD34+ cell therapy for diabetic patients with chronic nonhealing ulcer. Cell Transplant. 23 (2), 167–179. doi:10.3727/096368912X658007
Tateishi-Yuyama, E., Matsubara, H., Murohara, T., Ikeda, U., Shintani, S., Masaki, H., et al. (2002). Therapeutic angiogenesis for patients with limb ischaemia by autologous transplantation of bone-marrow cells: A pilot study and a randomised controlled trial. Lancet 360 (9331), 427–435. doi:10.1016/S0140-6736(02)09670-8
Timmermans, F., Van Hauwermeiren, F., De Smedt, M., Raedt, R., Plasschaert, F., De Buyzere, M. L., et al. (2007). Endothelial outgrowth cells are not derived from CD133+ cells or CD45+ hematopoietic precursors. Arterioscler. Thromb. Vasc. Biol. 27 (7), 1572–1579. doi:10.1161/ATVBAHA.107.144972
Tsuji, M., Taguchi, A., Ohshima, M., Kasahara, Y., Sato, Y., Tsuda, H., et al. (2014). Effects of intravenous administration of umbilical cord blood CD34+ cells in a mouse model of neonatal stroke. Neuroscience 263, 148–158. doi:10.1016/j.neuroscience.2014.01.018
Tura, O., Skinner, E. M., Barclay, G. R., Samuel, K., Gallagher, R. C. J., Brittan, M., et al. (2013). Late outgrowth endothelial cells resemble mature endothelial cells and are not derived from bone marrow. Stem Cells 31 (2), 338–348. doi:10.1002/stem.1280
Van't Hull, E. F., Bron, S., Henry, L., Ifticene-Treboux, A., Turrini, R., Coukos, G., et al. (2014). Bone marrow-derived cells are implicated as a source of lymphatic endothelial progenitors in human breast cancer. Oncoimmunology 3, e29080. doi:10.4161/onci.29080
Vasa, M., Fichtlscherer, S., Aicher, A., Adler, K., Urbich, C., Martin, H., et al. (2001). Number and migratory activity of circulating endothelial progenitor cells inversely correlate with risk factors for coronary artery disease. Circ. Res. 89 (1), E1–E7. doi:10.1161/hh1301.093953
Velagapudi, P., Turagam, M., Kolte, D., Khera, S., Hyder, O., Gordon, P., et al. (2019). Intramyocardial autologous CD34+ cell therapy for refractory angina: A meta-analysis of randomized controlled trials. Cardiovasc. Revascularization Med. 20 (3), 215–219. doi:10.1016/j.carrev.2018.05.018
Venkat, P., Cui, C., Chen, Z., Chopp, M., Zacharek, A., Landschoot-Ward, J., et al. (2021). CD133+ exosome treatment improves cardiac function after stroke in type 2 diabetic mice. Transl. stroke Res. 12 (1), 112–124. doi:10.1007/s12975-020-00807-y
Viñas, J. L., Burger, D., Zimpelmann, J., Haneef, R., Knoll, W., Campbell, P., et al. (2016). Transfer of microRNA-486-5p from human endothelial colony forming cell–derived exosomes reduces ischemic kidney injury. Kidney Int. 90 (6), 1238–1250. doi:10.1016/j.kint.2016.07.015
Wakabayashi, T., Naito, H., Suehiro, J. I., Lin, Y., Kawaji, H., Iba, T., et al. (2018). CD157 marks tissue-resident endothelial stem cells with homeostatic and regenerative properties. Cell Stem Cell 22 (3), 384–397 e6. doi:10.1016/j.stem.2018.01.010
Wen, Q., Kong, Y., Zhao, H. Y., Zhang, Y. Y., Han, T. T., Wang, Y., et al. (2019). G-CSF-induced macrophage polarization and mobilization may prevent acute graft-versus-host disease after allogeneic hematopoietic stem cell transplantation. Bone Marrow Transplant. 54 (9), 1419–1433. doi:10.1038/s41409-019-0449-9
Wu, X., Liu, Z., Hu, L., Gu, W., and Zhu, L. (2018). Exosomes derived from endothelial progenitor cells ameliorate acute lung injury by transferring miR-126. Exp. Cell Res. 370 (1), 13–23. doi:10.1016/j.yexcr.2018.06.003
Xiong, W., Tan, M. x., Chen, Z. l., Liu, Y., Liu, Y., Zou, X. l., et al. (2022). Endothelial progenitor cell (EPCs)-derived exosomal miR-30d-5p inhibits the inflammatory response of high glucose-impaired fibroblasts by affecting the M1/M2 polarization of macrophages. Rev. Romana Med. Lab. 30 (4), 435–451. doi:10.2478/rrlm-2022-0032
Xu, J., Bai, S., Cao, Y., Liu, L., Fang, Y., Du, J., et al. (2020). miRNA-221-3p in endothelial progenitor cell-derived exosomes accelerates skin wound healing in diabetic mice. Diabetes, metabolic syndrome Obes. targets Ther. 13, 1259–1270. doi:10.2147/DMSO.S243549
Yan, F., Liu, X., Ding, H., and Zhang, W. (2022). Paracrine mechanisms of endothelial progenitor cells in vascular repair. Acta histochem. 124 (1), 151833. doi:10.1016/j.acthis.2021.151833
Yang, C.-C., Sung, P. H., Cheng, B. C., Li, Y. C., Chen, Y. L., Lee, M. S., et al. (2020). Safety and efficacy of intrarenal arterial autologous CD34+ cell transfusion in patients with chronic kidney disease: A randomized, open-label, controlled phase II clinical trial. Stem Cells Transl. Med. 9 (8), 827–838. doi:10.1002/sctm.19-0409
Yang, Y., Chen, X. h., Li, F. g., Chen, Y. x., Gu, L. q., Zhu, J. k., et al. (2015). In vitro induction of human adipose-derived stem cells into lymphatic endothelial-like cells. Cell Reprogr. 17 (1), 69–76. doi:10.1089/cell.2014.0043
Yeh, E. T., Zhang, S., Wu, H. D., Körbling, M., Willerson, J. T., and Estrov, Z. (2003). Transdifferentiation of human peripheral blood CD34+-enriched cell population into cardiomyocytes, endothelial cells, and smooth muscle cells in vivo. Circulation 108 (17), 2070–2073. doi:10.1161/01.CIR.0000099501.52718.70
Yoshioka, T., Ageyama, N., Shibata, H., Yasu, T., Misawa, Y., Takeuchi, K., et al. (2005). Repair of infarcted myocardium mediated by transplanted bone marrow–derived CD34+ stem cells in a nonhuman primate model. Stem cells 23 (3), 355–364. doi:10.1634/stemcells.2004-0200
Zhang, Y., Huang, H., Liu, W., Liu, S., Wang, X. Y., Diao, Z. L., et al. (2021). Endothelial progenitor cells-derived exosomal microRNA-21-5p alleviates sepsis-induced acute kidney injury by inhibiting RUNX1 expression. Cell death Dis. 12 (4), 335–413. doi:10.1038/s41419-021-03578-y
Zhao, L., Yang, X. R., and Han, X. (2019). MicroRNA-146b induces the PI3K/Akt/NF-κB signaling pathway to reduce vascular inflammation and apoptosis in myocardial infarction by targeting PTEN. Exp. Ther. Med. 17 (2), 1171–1181. doi:10.3892/etm.2018.7087
Zhou, W., Zheng, X., Cheng, C., Guo, G., Zhong, Y., Liu, W., et al. (2021). Rab27a deletion impairs the therapeutic potential of endothelial progenitor cells for myocardial infarction. Mol. Cell. Biochem. 476 (2), 797–807. doi:10.1007/s11010-020-03945-x
Zhou, Y., Goodwin, A. J., Cook, J. A., Halushka, P. V., and Chang, E. (2019). Exosomes from endothelial progenitor cells improve outcomes of the lipopolysaccharide-induced acute lung injury. Crit. Care 23 (1), 44. doi:10.1186/s13054-019-2339-3
Zhou, Y., Li, P., Goodwin, A. J., Cook, J. A., Halushka, P. V., Chang, E., et al. (2018). Exosomes from endothelial progenitor cells improve the outcome of a murine model of sepsis. Mol. Ther. 26 (5), 1375–1384. doi:10.1016/j.ymthe.2018.02.020
Zullo, J. A., Nadel, E. P., Rabadi, M. M., Baskind, M. J., Rajdev, M. A., Demaree, C. M., et al. (2015). The secretome of hydrogel-coembedded endothelial progenitor cells and mesenchymal stem cells instructs macrophage polarization in endotoxemia. Stem cells Transl. Med. 4 (7), 852–861. doi:10.5966/sctm.2014-0111
Keywords: CD34 positive cells, endothelial progenitor cells, vasculogenesis, exosome, regenerative medicine
Citation: Hassanpour M, Salybekov AA, Kobayashi S and Asahara T (2023) CD34 positive cells as endothelial progenitor cells in biology and medicine. Front. Cell Dev. Biol. 11:1128134. doi: 10.3389/fcell.2023.1128134
Received: 20 December 2022; Accepted: 03 April 2023;
Published: 17 April 2023.
Edited by:
David M. Smadja, Université Paris Cité, FranceReviewed by:
Neslihan Meriç, Kutahya Health Sciences University, TürkiyeCopyright © 2023 Hassanpour, Salybekov, Kobayashi and Asahara. This is an open-access article distributed under the terms of the Creative Commons Attribution License (CC BY). The use, distribution or reproduction in other forums is permitted, provided the original author(s) and the copyright owner(s) are credited and that the original publication in this journal is cited, in accordance with accepted academic practice. No use, distribution or reproduction is permitted which does not comply with these terms.
*Correspondence: Takayuki Asahara, dF9hc2FoYXJhQHNob25hbmthbWFrdXJhLm9yLmpw
Disclaimer: All claims expressed in this article are solely those of the authors and do not necessarily represent those of their affiliated organizations, or those of the publisher, the editors and the reviewers. Any product that may be evaluated in this article or claim that may be made by its manufacturer is not guaranteed or endorsed by the publisher.
Research integrity at Frontiers
Learn more about the work of our research integrity team to safeguard the quality of each article we publish.