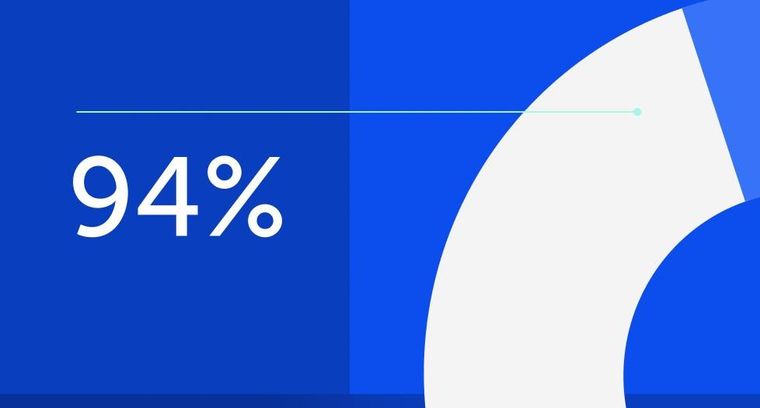
94% of researchers rate our articles as excellent or good
Learn more about the work of our research integrity team to safeguard the quality of each article we publish.
Find out more
ORIGINAL RESEARCH article
Front. Cell Dev. Biol., 25 April 2023
Sec. Morphogenesis and Patterning
Volume 11 - 2023 | https://doi.org/10.3389/fcell.2023.1126968
This article is part of the Research TopicEditors' Showcase 2022: Insights in Morphogenesis and PatterningView all 8 articles
Members of the Eya family, which are a class of transcription factors with phosphatase activity, are widely expressed in cranial sensory organs during development. However, it is unclear whether these genes are expressed in the taste system during development and whether they play any role in specifying taste cell fate. In this study, we report that Eya1 is not expressed during embryonic tongue development but that Eya1-expressing progenitors in somites or pharyngeal endoderm give rise to tongue musculature or taste organs, respectively. In the Eya1-deficient tongues, these progenitors do not proliferate properly, resulting in a smaller tongue at birth, impaired growth of taste papillae, and disrupted expression of Six1 in the papillary epithelium. On the other hand, Eya2 is specifically expressed in endoderm-derived circumvallate and foliate papillae located on the posterior tongue during development. In adult tongues, Eya1 is predominantly expressed in IP3R3-positive taste cells in the taste buds of the circumvallate and foliate papillae, while Eya2 is persistently expressed in these papillae at higher levels in some epithelial progenitors and at lower levels in some taste cells. We found that conditional knockout of Eya1 in the third week or Eya2 knockout reduced Pou2f3+, Six1+ and IP3R3+ taste cells. Our data define for the first time the expression patterns of Eya1 and Eya2 during the development and maintenance of the mouse taste system and suggest that Eya1 and Eya2 may act together to promote lineage commitment of taste cell subtypes.
The tongue, a muscular organ that manipulates food for chewing and swallowing, is one of the primary organs of taste. The tongue’s upper surface is covered with sensory taste buds, which are housed in the papillae. In mammals, there are three different types of taste papillae: fungiform papillae (FUP), which are located over the anterior two-thirds of the tongue, and circumvallate (CVP) and foliate (FOP) papillae, which are situated at the back of the tongue (Witt, 2019). While a single CVP is located in the midline of the tongue, the FOP consists of inwardly folded epithelial trenches on both sides. Each taste bud forms an onion-like shape and is composed of distinct types of cells, such as taste receptors (TRCs) that span the depth of the papilla epithelium and supporting cells (Finger and Barlow, 2021). The TRCs are maintained by a continuously renewing population of progenitor cells that reside along the basement membrane of the epithelium (Barlow and Klein, 2015). The taste buds that receive gustatory stimuli are innervated by cranial nerves that respond to chemicals from food transmitted through taste receptor cells, resulting in the sensations of salty, sour, bitter, sweet, and umami (Northcutt, 2004).
While taste bud differentiation is induced around or after birth, taste papilla development in mice begins around embryonic day (E) 12.5, when taste primordia emerge as epithelial thickenings or taste placodes, which undergo dynamic morphogenetic events of evaginations and invaginations to form the epithelial papillae. There are 70–90 FUPs on the anterior tongue, and each contains a single taste bud, which differentiates as clusters of TRCs in the center of the papillary epithelium around birth (Kim et al., 2003; Mistretta and Liu, 2006). In contrast, taste buds in the CVP and FOP develop postnatally. They originate initially in the papillary region and later in the trench epithelium, formed by the invagination of the epithelium into the underlying mesenchyme beginning around E14.5. These trench epithelial cells undergo an extensive growth period to generate sufficient progenitor cells before the induction of taste cell differentiation. From the base of trenches, minor salivary glands (von Ebner’s glands) develop to aid gustatory sensation (Lee et al., 2006). However, how tongue epithelial progenitors are induced to diversify and generate different cell types in the taste system remains poorly understood.
Cell fate diversification is known to be controlled by gene expression programs that are regulated spatiotemporally by tissue- and cell type-specific transcription factors. Several transcription factors have been identified to play important roles in controlling the differentiation of embryonic tongue epithelium into taste papillae and the generation of different types of TRCs. Among them, Sox2 is an essential regulator of taste bud development and TRC generation and maintenance in adults (Okubo et al., 2006; Nakayama et al., 2015; Castillo-Azofeifa et al., 2018; Ohmoto et al., 2020). In contrast, Pou2f3 and Mash1 are critical for the generation of distinct TRC subtypes (Matsumoto et al., 2011; Seta et al., 2011). Previous studies have also suggested a role for the homeodomain Six/So (Sine oculis) protein family members Six1 and Six4 in the taste papillae during mouse embryonic development (Suzuki et al., 2010a; Suzuki et al., 2010b; Suzuki et al., 2011). However, it is not well understood how these transcription factors interact to spatiotemporally regulate the gene expression programs that establish and maintain distinct taste structures.
The Eya (eyes absent) protein family represents a class of transcription factors without DNA binding ability but with phosphatase activity. It encodes a divergent N-terminus possessing a transcriptional activation function (Xu et al., 1997a) and a highly conserved C-terminal Eya domain (ED) (Xu et al., 1997b) that participates in protein-protein interactions (Buller et al., 2001; Li et al., 2003; Rayapureddi et al., 2003; Tootle et al., 2003). We previously demonstrated that Eya1 interacts with chromatin remodelers and DNA-binding proteins Six1 and Sox2 to regulate cranial sensory organ development (Ahmed et al., 2012a; Ahmed et al., 2012b; Wong et al., 2013; Xu et al., 2021) or with Six1/Six2/Six4 to regulate kidney formation (Xu et al., 2003; Xu, 2013; Xu et al., 2014; Xu and Xu, 2015; Li et al., 2021; Li et al., 2022; Xu et al., 2022). Deletion of either Eya1 or Six1 in mice results in similar organ defects (Xu et al., 1999; Zheng et al., 2003; Ozaki et al., 2004; Zou et al., 2004; Zou et al., 2006a; Zou et al., 2008; Ahmed et al., 2012a; Ahmed et al., 2012b; Xu et al., 2021), while mutations in either gene in humans cause branchio-oto-renal syndrome (Abdelhak et al., 1997a; Abdelhak et al., 1997b; Ruf et al., 2004; Shah et al., 2020). Eya2, another member of the Eya family, is also widely expressed in sensory structures during development, including the sensory nervous system, olfactory epithelium, inner ear sensory organs, and salivary glands, in a complementary or overlapping pattern with that of Eya1 (Xu et al., 1997b; Ishihara et al., 2008; Chen et al., 2009; Zhang et al., 2021). Eya2 knockout mice exhibit a normal appearance but have hearing loss (Zhang et al., 2021). In the taste system, RNA-seq analysis of chick oral tissues detected Eya2 transcripts (Cui et al., 2017). However, a recent in situ hybridization study reported the expression of Eya1 in subsets of taste bud cells in the CVP and FUP of mice aged 8–12 weeks, but not in FOP (Ohmoto et al., 2021). However, the expression patterns of these genes during the embryonic development of taste organs have not been studied. Furthermore, whether these genes play a role in taste organogenesis or TRC differentiation is not known.
In this study, we investigated the potential roles of Eya1 and Eya2 in the development and maintenance of the mouse taste system from the embryonic stage to adulthood. Specifically, we examined the expression of Eya1LacZ and Eya2LacZ knockin reporter alleles in the tongues by staining for β-Gal activity and investigated whether deletion of either gene disrupted taste organogenesis and taste cell generation. We found that although Eya1 is not expressed in the tongue during embryonic development, Eya1-expressing progenitors in the pharyngeal endoderm or somites at E9.5 give rise to the lingual epithelium and epithelium-derived taste organs or tongue musculature, respectively. In Eya1-deficient tongues, these progenitor cells fail to proliferate normally, resulting in a smaller tongue at birth, impaired growth of taste papillae, and disrupted expression of Six1 in the papilla epithelium. After birth, Eya1 expression is detectable from 1 week to adulthood. It is expressed in some basal progenitors and a subset of IP3R3+ TRCs of the endoderm-derived CVP and FOP in the posterior tongue but not in the ectoderm-derived FUP in the anterior tongue. Eya1 conditional knockout (cKO) during the third week using Sox2CreER resulted in a reduced number of Type II TRCs. In contrast, we found that Eya2 is expressed in the CVP and FOP from E14.5 shortly after the onset of their morphogenesis. Soon after birth, Eya2 is expressed throughout the CVP and FOP trench epithelium. However, in the adult tongue, Eya2LacZ is expressed in some cells near the taste pore surrounding the taste bud and some basal precursors and taste cells, and the number of Type II TRCs is also reduced in Eya2-deficient mice. Together, our results define the expression patterns of Eya1 and Eya2 during the development and maintenance of the mouse taste system for the first time. Our results suggest that Eya1 and Eya2 may function together to promote the lineage specification of taste cell subsets in adult taste buds at the posterior tongue.
Mice carrying Eya1+/− (Xu et al., 1999), Eya1LacZ (Zou et al., 2008), Eya1CreER (Xu et al., 2014), Eya1Flox10/Flox10, R26LacZ, Eya2+/− and Eya2LacZ (Zhang et al., 2021), and Sox2CreER (Arnold et al., 2011) were housed at the Icahn School of Medicine at Mount Sinai Animal Facility, on a mixed background of 129/Sv and C57BL/6J strains. Eya1Flox10 was generated by Biocytogen through the use of LoxP sites to flank two exons—exons 10 and 11.
PCR genotype was performed as previously reported. All animal protocols were approved by Animal Care and Use Committee of the Icahn School of Medicine at Mount Sinai (protocol #06-0807).
Mice were anesthetized with isoflurane and transcardially perfused with phosphate-buffered saline (PBS), followed by 4% paraformaldehyde (PFA) in PBS. Tissue organs were then fixed with 4% PFA for 30 min, cryoprotected in 30% sucrose overnight, embedded in OCT compound (Tissue-Tek; Sakura Finetek USA, Torrance, CA), and sectioned on a cryotome at 6 μm thickness. Sections were air-dried, rinsed in PBS, and stained in a solution containing 0.8 mg/mL X-Gal in 35 mM K3Fe(CN)6, 35 mM MK4Fe(CN)6, 2 mM MgCl2 in PBS at room temperature until the blue staining appeared. Sections were then dehydrated through a series of graded ethanol, cleared in xylenes, and cover-slipped with Permount. For whole-mount staining, the embryos were fixed with 4% PFA for 30 min, rinsed in PBS, and stained with the same chemicals listed above.
Histology and immunostaining were performed following standard procedures. Briefly, tongues were collected in ice-cold PBS and fixed in 4% PFA for 30 min at room temperature. Samples were cryoprotected in 30% sucrose at 4°C overnight and then submerged in OCT compound and flash frozen on dry ice. Frontal sections were generated at 6 μm. Some sections were pretreated with citrate buffer (10 mM, pH6.0) at 65°C for 3 h for antigen retrieval before IHC.
Primary antibodies used were before IHC rabbit anti-Sox2 (PA1-094, ThermoFisher), rabbit anti-Six1 (50-204-6211, Cell Signaling), mouse anti-TUBB3 (66375-1-Ig, Proteintech), rabbit anti-β-Gal (08559761, MP Biomedicals), rabbit anti-Pou2f3 (PA5-40556, ThermoFisher), mouse anti-IP3R3 (610312, BD Biosciences), rabbit anti-Ncam (ab220360, Abcam), and mouse monoclonal Keratin 8 (Krt8) (NBP2-44940, Novusbio). Cy3-or Cy2-conjugated secondary antibodies were used. Nuclear staining was performed using Hoechst 33342.
The height of taste crypts in serial sections across the entire papilla was measured for three CVPs of wild-type control and Eya1−/− littermates, respectively, using Photoshop software, and the measurements were plotted using Prism software.
The EdU labeling assay was performed using a kit (cat# C10640, Life Technologies) following the manufacturer’s instructions. EdU was injected 90 min before harvest. Serial sections from three wild-type (35 sections, 6 μm/section) and three Eya1-deficient (33 sections, 6 μm/section) E15.5 CVPs and FOPs were prepared, and EdU+ cells were counted in the epithelial trenches. Statistical significance was assessed using a two-tailed t-test.
The TUNEL assay was performed using the ApopTag kit for in situ apoptosis fluorescein detection (cat# NC9815837, Millipore) following the manufacturer’s instructions. TUNEL+ cells were counted in the CVP epithelium. Statistical significance was assessed using a two-tailed t-test.
During the course of our phenotypic analysis of craniofacial defects associated with Eya1 deficiency, we noticed that the tongues of Eya1−/− mice that die at birth (Xu et al., 1999) were severely reduced in size compared to wild-type control littermates (Figure 1A). Although not directly related to the development of taste papillae, the tongue primordium forms from two lateral lingual swellings called the tongue buds, which originate from the floor of the first and second pharyngeal arches at E11.5 (Paulson et al., 1985; Shuler and Dalrymple, 2001; Torii et al., 2016). In Eya1−/− mice, the overall size of the tongue was noticeably smaller as early as E12.5 and drastically reduced in size by E18.5 compared to wild-type littermates (Figure 1A). We examined a total of 6-8 embryos of each genotype, and the phenotype was reproducible in all embryos. Thus, Eya1 is required for normal tongue formation during embryonic development.
FIGURE 1. Eya1 deficiency leads to growth arrest of tongue and taste papillae during development. (A) Tongues of wild-type and Eya1-null mice at E12.5, E14.5 and E18.5. (B, D) Hematoxylin-stained sections of E18.5 tongues showing FUP (B) and trenches of CVP and FOP with von Ebner’s glands (vEG) (D) in the wild-type and Eya1−/−. (C, E) Immunostaining for anti-TUBB3 showing association of cranial gustatory nerves with papillae and trenches at E18.5, but reduced or lack of nerve contact in the mutant. Sections for FOP in D,E were sagittal, and all others were frontal. Arrows indicate well-developed taste buds with tightly clustered cells in wild-type FUP, and arrowheads indicate abnormal taste bud development in Eya1−/− FUP. Asterisks indicate stunted CVP and FOP epithelium. (F) Statistical analysis showing the height of crypts across the entire CVP from three entire CVPs. Scale bars: 1 mm (A), 200 μm (lower magnification in B), 50 μm (higher magnification in B), 30 μm (FUP in C), 90 μm (CVP in C) and 50 μm (FOP in C).
The three types of taste papillae—FUP, CVP, and FOP—also displayed abnormal morphology or growth arrest (Figures 1B, D). The wild-type tongue has well-developed FUP with surrounding flattened keratinocytes near the taste pore (arrow, Figure 1B). In Eya1−/− embryos, the FUP formed, but the cells did not appear tightly clustered (arrowhead, Figure 1C), suggesting defective taste bud differentiation. The epithelial trenches of the CVP (indicated by bars) failed to invaginate deeply into the mesenchyme and appeared shortened but were still able to give rise to von Ebner’s glands. However, fewer von Ebner’s gland-like structures were identifiable in serial sections of the entire papilla regions compared to those typically observed in wild-type controls (Figure 1D). The CVP epithelium also appeared stunted (asterisk, Figure 1D) compared to the control. Likewise, the FOP trench invagination and papillary epithelium development were also disrupted (Figure 1D). We measured the height of the taste crypt in serial sections across the entire papilla for three CVPs, and the data confirmed shortened taste crypt in Eya1−/− (Figure 1F). These data indicate that Eya1 activity is required for the development of epithelium-derived taste papillae.
We then analyzed taste papillae at earlier stages. The development of taste papillae initiates around E12.5 with the emergence of taste primordia as epithelial thickenings or taste placodes (Supplementary Figure S1A–C). In Eya1−/− mice, taste placodes were formed at E12.5 (Supplementary Figure S1A–C), indicating that Eya1 is not necessary for the initial formation of taste placodes.
Previous studies have demonstrated that gustatory nerves are essential for the development of taste papillae, with taste buds in FUP innervated by the VIIth nerve and those in CVP and FOP innervated by the IXth nerve (Gibbons and Sadiq, 2022). Gustatory nerve fibers are critical for taste bud cell renewal [reviewed in (54)], and ablation or poor growth of these fibers can result in abnormal and distorted papillae during late embryonic and early postnatal stages (Barlow et al., 1996; Oakley and Witt, 2004). A recent study has also highlighted the importance of innervation in the embryonic development of taste buds, as genetic ablation of Neurog2, a key transcription factor involved in the development of neurogenic placode-derived sensory neurons, disrupts taste bud formation (Fan et al., 2019). Given that our previous work demonstrated severe defects in neurogenic placode-derived cranial ganglia, including geniculate (VIIth) and petrosal (IXth) ganglia, in Eya1−/− mice (Zou et al., 2004), we examined whether gustatory nerve innervation was disrupted in Eya1-deficient mice. Immunostaining for the neuronal marker TUBB3 at E15.5 and E18.5 revealed that FUP taste buds on the top were fully innervated by nerve fibers in wild-type mice by E18.5 (arrow, Figure 1C; Supplementary Figure S1D), whereas only a few TUBB3+ nerve fibers were observed in the mutant FUP region at E15.5 and E18.5 (Supplementary Figure S1D; Figure 1C, arrowhead). The CVP and FOP regions of wild-type embryos at E15.5 and E18.5 showed nerve fiber association, as evidenced by TUBB3 staining (Supplementary Figure S1E, F; Figure 1E). In contrast, the mutant tongue had significantly fewer nerve fibers in the CVP and FOP regions (Supplementary Figure S1E, F; Figure 1E, asterisks indicate stunted papillary epithelium). Therefore, the lack of nerve contacts in the epithelial cells of taste papillae could disrupt taste bud formation and lead to a defective differentiation program of taste bud cells in the mutant.
Next, to define the molecular basis underlying abnormal papilla morphogenesis, we performed marker gene analysis. We previously found that Eya1 acts upstream of Sox2 and Six1 in a genetic network controlling the formation of sensory organs in the inner ear (Ahmed et al., 2012a; Li et al., 2020). Therefore, we examined the regulatory relationship between these genes in the taste system. Sox2 is expressed in the lingual epithelium and is required for the differentiation of epithelial progenitors into taste cells (Okubo et al., 2006). Immunostaining showed that Sox2 was strongly detected in well-developed taste bud cells but was relatively weak in surrounding epithelial cells in the FUP region of the wild-type tongue at E18.5 (Figure 2A). In Eya1−/− tongues, Sox2 expression was also strongly detected in the FUP epithelial cells, but the taste bud did not appear as onion-shaped, with tightly clustered and elongated cells (Figure 2A), similar to that seen histologically (Figure 1C). Although morphologically abnormal, the levels of Sox2 expression in the trenches and epithelium of the CVP and FOP as well as in von Ebner’s gland appeared unaffected in Eya1−/− (Figure 2A). Thus, Sox2 expression in the taste epithelium is Eya1-independent.
FIGURE 2. Loss of Six1 expression in CVP and FOP in Eya1-deficient tongues and absence of Eya1 expression in embryonic tongues. (A) Immunostaining on frontal sections showing Sox2 expression in E18.5 wild-type and Eya1−/− FUP, CVP and FOP regions. Arrows indicate well-developed taste buds with clustered cells in wild-type FUP but not in Eya1−/−. (B) Immunostaining on frontal sections showing Six1 expression in CVP epithelium (arrow) and trenches, FOP trenches, von Ebner’s gland and muscle cells in E18.5 wild-type, but its expression in the papilla and trench epithelium is lost in Eya1−/−. (C) Whole-mount X-Gal staining of Eya1LacZ/+ and Eya1LacZ/LacZ tongues at E18.5. (D) Frontal sections showing X-Gal staining of Eya1LacZ/+ and Eya1LacZ/LacZ tongues at E14.5 and E18.5. Red arrows point to the end of the trench epithelium, which was followed by a cluster of epithelial cells. Abb.: t, tooth; vEG, von Ebner’s gland. Scale bars: 30 μm (for top panels of FUP in A), 50 μm (for CVP and FOP in A,B), 1 mm (C) and 300 μm (D).
Six1 is expressed in the trenches of the CVP and FOP at E18.5, previously detected by ISH (Suzuki et al., 2010b). Consistent with this, immunostaining detected Six1 expression at the bottom of the trench epithelium and von Ebner’s glands at E18.5 (Figure 2B). We also observed Six1+ cells in the top CVP epithelium (arrow, Figure 2B). In contrast, Eya1−/− tongues lacked Six1 expression in these structures, but Six1 expression was detectable in the muscle cells (Figure 2B). This suggests that Eya1 is required for Six1 expression in the epithelium of trenches and papillae during development.
Since the expression of Eya1 during embryonic tongue and taste organ development has not been studied, we performed X-Gal staining of tongues isolated from Eya1LacZ knockin reporter mice (Zou et al., 2008; Xu et al., 2014). To our surprise, whole-mount or section staining at E12.5-18.5 failed to detect β-Gal activity in the developing tongue epithelium or taste papillae of either Eya1LacZ/+ heterozygotes or Eya1LacZ/LacZ homozygotes (Figures 2C, D; Supplementary Figure S2A). In contrast, some β-Gal activity was observed near the midline in the tip region (inset panel in Figure 2C) and other regions of the oral cavity (Figure 2D). Since Eya1 is not actively expressed in the tongue epithelium and epithelium-derived taste papillae, it is unlikely that Eya1 plays a direct role in the morphogenesis of the taste papillae during embryonic development. Therefore, the abnormal growth of taste papillae observed in Eya1−/− may be primarily due to the absence of associated afferent nerves.
Previous lineage tracing studies have indicated that taste papillae located in the anterior and posterior tongue have different embryonic origins, with FUP being ectoderm-derived and both CVP and FOP being endoderm-derived (Engert et al., 2009; Rothova et al., 2012). We showed that Eya1 is coexpressed with Six1 in pharyngeal endoderm, ectoderm and mesenchyme at E9.5-10.5 (Xu et al., 1997b; Xu et al., 2002), but the expression of Six1 in the pharyngeal endoderm and ectoderm is absent in Eya1−/− embryo (Xu et al., 2002; Zou et al., 2006b). This led us to hypothesize that early Eya1-expressing pharyngeal progenitors might contribute to tongue formation and that in the absence of Eya1, these progenitors might not be able to undergo normal cellular proliferation. To test this, we performed lineage tracing using Eya1CreER (Xu et al., 2014) and Cre-dependent R26RLacZ reporter by administering tamoxifen at E9.5-E14.5 and staining for β-Gal activity in the tongue at E17.5-18.5. Indeed, one dose of tamoxifen administration at E9.5 induced LacZ-marked cells in the tongue epithelium and epithelium-derived taste papillae (Figure 3A). Additionally, since both genes are also coexpressed in somites, where Six1 has a primary role in myogenesis (Laclef et al., 2003), the tongue muscle cells, which predominantly derive from the myoblasts that originate in the occipital somites (Iwata et al., 2013), were marked by β-Gal (Figure 3A). However, no labeled cells were observed in some cells surrounding the muscles or the subepithelial mesenchyme adjacent to the epithelium (red asterisks, Figure 3A). These mesenchymal cells also do not appear to express Six1 (Figure 2B). Notably, however, tamoxifen administration from E10.5 to E14.5 failed to induce Eya1-lineage cells in the tongue and taste papillae, indicating that Eya1-expressing progenitors from E10.5–14.5 did not contribute to the formation of tongue and taste papillae (Figures 3B, C). These data also confirmed the absence of Eya1 expression in the developing tongue and taste papillae (Figures 2C, D; Supplementary Figure S2A). Since the peak of CreER activation occurs over a subsequent period of 12–24 h (Danielian et al., 1998; Zervas et al., 2004), and the efficiency of CreER activation is affected by the timing and amount of tamoxifen received by different cells, the stage of cell development/differentiation and the age of the animals, multiple doses of tamoxifen treatment are commonly used in genetic ablation studies. Thus, one dose of tamoxifen treatment may not be sufficient to induce simultaneous CreER activation in all Eya1-expressing progenitors, explaining why not all epithelial cells in taste papillae and trenches were β-Gal+. Nonetheless, these results suggest that early Eya1-expressing progenitors at E9.5–10.5 can give rise to tongue epithelium, taste papillae and tongue muscle tissues.
FIGURE 3. Lineage tracing reveals the contribution of Eya1-expressing progenitors before E10.5 to tongue epithelium, taste papillae, and tongue musculature. (A–C) X-Gal stained frontal sections of Eya1CreER;R26RLacZ at E17.5–18.5 showing labeled cells in the tongue muscles, lingual epithelium, and taste papilla regions when tamoxifen (tam) was administered at E9.5 (A), but not at E10.5 and 11.5 (B) or E13.5 and 14.5 (C). Bottom panels in A show a higher magnification of areas indicated by arrows. The FOP was outlined by dashed lines. Red asterisks indicate unmarked mesenchymal cells. Abb.: vEG, von Ebner’s gland. Scale bars: 200 μm for the top panels in A and 50 μm for all other panels.
Next, we investigated whether the early progenitors had a defect in cell proliferation or survival associated with Eya1 deficiency. To measure cell proliferation, we injected the mitotic tracer 5-ethynyl-2′-deoxyurindine (EdU) at E15.5 and harvested the tongue 1.5 h after injection. EdU-labeling assays confirmed that while EdU incorporation appeared to be reduced in the muscle cells, EdU-incorporated epithelial cells in the taste papilla regions, especially at the bottom of the growing CVP and FOP trenches, were significantly reduced in the mutant compared to the control littermates (Figures 4A–F). We counted EdU+ cells in the CVP and FOP trenches, and quantitative analysis indicated that EdU-incorporated cells in the mutant CVP and FOP trenches were reduced to ∼52.2% (CVP trench) or ∼40.7% (FOP trench) of those in the littermate controls (Figure 4I). Thus, in the absence of Eya1, the trench epithelial progenitor cells are likely unable to undergo normal proliferation and expansion, which may partially lead to the growth defect of the trench epithelium.
FIGURE 4. Reduced cell proliferation in the proliferating zone of the CVP and FOP trenches and increased apoptosis in the CVP epithelium of Eya1−/− tongues. (A–F) EdU (red) on sections of FUP, CVP and FOP regions from E15.5 tongues injected with EdU 1.5 h before harvesting. Right panels in A,B are bright-field images showing higher magnification of the boxed areas and the arrow in A,B points to FUP. Invaginating trenches of FOP (C, D) or CVP are outlined by dashed lines. Right panels in C,D are bright-field images. (G, H) TUNEL assay on sections of the CVP and FOP regions from E15.5 tongues showing increased apoptotic cells (green) in the CVP epithelium on the top (arrow). (I) Number of EdU+ cells in the outlined trenches (see Methods for quantification). p-values were measured for +/+ and Eya1−/− using two-tailed Student’s t-test. (J) Quantitative analysis of the number of TUNEL+ cells in the CVP epithelium region. Scale bars: 50 μm (A, B, E, F) and 30 μm (C, D, G, H).
We also performed a TUNEL assay to investigate if the tongue progenitor cells undergo abnormal cell death and found that the number of apoptotic cells was only increased in the top epithelium of the mutant CVP at E15.5 (∼27.5-fold increase) compared to the littermate controls (Figures 4G, H, J). No abnormal apoptosis was observed in the growing trenches, other papilla regions, or the musculature. This suggests that CVP epithelial growth arrest may in turn lead to epithelial apoptosis.
As noted earlier, Eya1 has been reported to be expressed in a subset of Pou2f3+ Type II TRCs and some immature precursors in the endoderm-derived CVP and ectoderm-derived FUP but not in the endoderm-derived FOP at 8–12 weeks of age (Ohmoto et al., 2021). To test whether Eya1 regulates taste cell differentiation, we first clarified the expression of Eya1 at various postnatal stages. X-Gal staining of Eya1LacZ/+ tongues at 3 weeks to adulthood showed weak β-Gal activity in taste buds of CVP and FOP (Figure 5A), but no β-Gal activity was observed in the ectoderm-derived FUP at any stage after birth (Figure 5A). X-Gal staining on sections confirmed β-Gal activity in the endoderm-derived CVP and FOP taste buds (Figure 5B). Analysis of P14 and P7 tongues also revealed β-Gal activity in the CVP and FOP taste buds (Figures 5C, D). Thus, our analysis redefines the expression of Eya1 as restricted to the endoderm-derived CVP and FOP taste buds located at the back of the tongue, but not the ectoderm-derived FUP situated in the anterior part of the tongue.
FIGURE 5. Eya1 is expressed in a subset of taste cells and some basal progenitors after birth. (A) Whole-mount X-Gal staining of the tongue in Eya1lacZ/+ mice at P21. (B) Sections of X-Gal stained tongue in (A) showing the CVP and FOP regions. (C, D) X-gal stained sections of CVP and FOP regions at P14 (C) and P7 (D). (E) Schematic drawing showing different types of taste bud cells. (F–H) X-Gal stained sections showing β-Gal+ cells in the CVP taste buds at P7 (F), P21 (G), and 8 weeks (H). Arrows point to basal progenitors. (I) Co-immunostaining for β-Gal and IP3R3 in taste buds at 8 weeks. Asterisks indicate IP3R3+ cells are also β-Gal+. Scale bars: 50 μm (A–D) and 20 μm (F–I).
In the adult tongue, taste buds are maintained by continuous renewal of cells from surrounding epithelial stem progenitors throughout life. The taste bud cells are classified into four types: elongated and tightly clustered Type I, II, and III TRCs, and IV basal postmitotic precursors (Figure 5E) (Feng et al., 2014; Barlow and Klein, 2015; Yang et al., 2020; Finger and Barlow, 2021). Upon higher magnification of X-Gal-stained sections, strong β-Gal activity was observed in some taste cells and cells at the basal regions at P7, P21 and 8 weeks (arrows, Figures 5F–H). To clarify whether Eya1+ taste cells are a subset of Type II cells, we performed co-immunostaining with anti-β-Gal and -IP3R3 (a marker for Type II cells) and found that approximately 96% of β-Gal + cells were also IP3R3+ (Figure 5I; Table 1). This confirms that Eya1 expression is predominantly restricted to Type II TRCs.
After confirming the expression pattern of Eya1 in postnatal taste buds, we investigated whether it plays a role in the differentiation or maintenance of Type II TRCs by generating an Eya1 conditional knockout (Eya1cKO). Previous studies have shown that Sox2 is expressed in some proliferative progenitors surrounding adult taste buds, postmitotic precursors, and taste cells (fewer Type II and Type III and more Type I) (Suzuki, 2008). We analyzed Sox2 expression in postnatal and adult taste buds in Sox2GFP/+ knockin reporter mice (Arnold et al., 2011) and found that it is expressed in some basal progenitors and some taste cells (Supplementary Figure S3). As Eya1 was found to be expressed in many basal progenitor cells at P7-P21, we therefore used Sox2CreER and administered tamoxifen treatment at P15-P19. We harvested tongues at ∼6 weeks and immunostained for markers labeling different types of TRCs. Pou2f3 is specifically expressed in Type II TRCs, and inactivation of Pou2f3 in mice results in loss of Type II TRCs and expansion of Type III cells (Matsumoto et al., 2011). In Eya1cKO, immunostaining showed a clear reduction in the number of Pou2f3+ cells in the taste buds (Figure 6A; Table 2), and the trench also appeared abnormal, with wider cavity space compared to the control (Figure 6A). Six1 has also been reported to be expressed in Type II cells (Suzuki et al., 2010a). Immunostaining for Six1 or co-staining with the pan-taste cell marker Krt8 (Mbiene and Roberts, 2003; Boggs et al., 2016) also showed a reduction in the number of Six1+ cells in the mutant taste buds (Figures 6B, C; Table 2). Immunostaining for IP3R3 (Golden et al., 2021) or co-immunostaining for IP3R3 and Ncam (a marker for Type III cells) further confirmed the reduction of Type II cells in Eya1cKO (Figures 6D, E; Table 2). However, no significant difference was observed in the number of Ncam+ Type III cells between wild-type and Eya1cKO mice (Figure 6E; Table 2). Thus, these results suggest that deletion of Eya1 activity in Sox2-expressing cells may affect gene expression levels or Type II cell turnover rates.
FIGURE 6. Conditional deletion of Eya1 leads to a reduction in the numbers of Pou2f3+, Six1+ and IP3R3+ taste cells. (A–E) Immunostaining for Pou2f3 (A), Six1 (B), or co-immunostaining for Six1/Krt8 (C), IP3R3 (D) or co-immunostaining for IP3R3/Ncam (E) in wild-type control and Eya1cKO taste buds at ∼6–8 weeks of age. Sections were also counterstained with diluted hematoxylin, and bright-field images were taken to outline the taste buds. Scale bars: 50 μm (A–C) and 20 μm (D, E).
Eya2 is broadly expressed in cranial sensory organs in an overlapping or complementary pattern with Eya1 during mouse development (Xu et al., 1997b; Zhang et al., 2021). Eya2-deficent mice are viable but develop hearing loss (Zhang et al., 2021), However, it has not been reported whether Eya2 is expressed in the tongue and whether it plays a role in taste organ development. To address these questions, we first examined the expression pattern of Eya2 during tongue development by performing X-Gal staining of Eya2LacZ/+ or Eya2LacZ/LacZ from E14.5 to E18.5. Although we did not observe a clear difference in tongue appearance between Eya2LacZ/+ and Eya2LacZ/LacZ, we detected β-Gal activity in the CVP and FOP, but not in the FUP (Figure 7A; Supplementary Figure S2B). Eya2 expression became detectable in the CVP epithelium at E14.5 as revealed by weak β-Gal activity in Eya2LacZ/+ heterozygotes and stronger in Eya2LacZ/LacZ homozygotes (Figure 7B). As development proceeded, intense β-Gal activity was detected in the invaginating trenches and in the epithelium of CVP at E16.5-E18.5 (Figure 7B). At these stages, von Ebner’s glands appeared and showed strong Eya2 expression (Figure 7B). Similarly, Eya2 was expressed in the FOP trench epithelium (Figure 7B). Immunostaining for Six1 at E18.5 did not detect any significant differences in its expression levels between control and Eya2-deficient tongues (Figure 7C), suggesting that Six1 expression is not dependent on Eya2.
FIGURE 7. Eya2 is expressed in the epithelium of CVP and FOP from the onset of papilla morphogenesis. (A) Whole-mount X-Gal staining of tongues from Eya2LacZ/+ and Eya2LacZ/LaZ at E14.5, E16.5, and E18.5. (B) Frontal sections of whole-mount X-Gal stained tongues showing β-Gal activity the CVP epithelium and trenches, FOP trenches, and von Ebner’s gland in Eya2LacZ/+ and Eya2LacZ/LacZ respectively. (C) Immunostaining on frontal sections showing Six1 expression in the trenches of CVP and FOP of wild-type and Eya2-null tongues at E18.5. Scale bars: 50 μm.
After birth, Eya2 continues to be widely expressed in the CVP and FOP but not in the FUP (Supplementary Figure S2C) and its expression spans the entire trench epithelium, including the taste buds and surrounding epithelium (Figure 8A). In the adult tongue, X-gal staining of sections from the Eya2LacZ/+ CVP and FOP regions revealed β-Gal activity in some cells near the taste pores surrounding the buds (Figures 8B, C, red asterisks) and some cells in the basal regions (Figures 8B, C, arrows), but relatively weaker β-Gal activity was detected in a subset of taste cells (Figures 8B, C).
FIGURE 8. Eya2 is expressed in the CVP and FOP taste buds, and Eya2-deficient mice show a reduced number of Type II taste cells. (A, B) X-Gal staining on sections showing broad expression of Eya2LacZ in the CVP and FOP trench epithelium at P14 (A) and the adult CVP trench epithelium (B). (C) Higher magnification showing β-Gal+ cells in some basal progenitors (arrows) and cells surrounding the taste pore (red asterisks) and inside the taste buds. Some sections were counterstained with diluted hematoxylin (A and two lower panels in (C). (D–F) Immunostaining for Pou2f3 (D), Six1(E), and co-immunostaining for IP3R3/Ncam (F) in wild-type control and Eya2-deficient adult taste buds. Scale bars: 50 μm (A, B) and 20 μm (C–E).
To investigate whether Eya2 has a role in the differentiation of the TRCs in the adult CVP and FOP taste buds, we performed marker gene analysis. Similar to that observed in the Eya1cKO, the number of Pou2f3+, Six1+ or IP3R3+ Type II cells was also reduced in the mutant taste buds of the CVP and FOP (Figures 8D–F; Table 2). However, the number of Ncam+ Type III cells appeared unaffected in Eya2-deficient tongues (Table 2). Collectively, these results suggest that Eya1 and Eya2 may function together to regulate Type II TRC generation.
Transcription factors are known to spatiotemporally regulate gene expression programs, generating cellular diversity during development and maintaining cellular specificity over time. However, our understanding of how the taste system develops, and how taste cells are diversified and maintained, remains limited. The Eya transcription factor was initially identified as a critical regulator of compound eye formation in Drosophila, interacting with the homeodomain protein So/Six and Dachshund (Dach) to promote ectopic eye formation synergistically (Castillo-Azofeifa et al., 2018; Ohmoto et al., 2020). Genetically, eya is upstream of so because the expression of so is dependent on eya but not vice versa (Bonini et al., 1997; Pignoni et al., 1997; Halder et al., 1998). During mammalian development, members of the Eya gene family are coexpressed with the Six family genes in multiple organ systems, and the Eya-Six regulatory relationship is evolutionarily conserved (Wong et al., 2013; Xu, 2013). Although previous studies reported the expression patterns of Six1 and Six4 during embryonic taste papilla morphogenesis and Six1 expression in a subset of Type II TRCs in adult mice, it remains unexplored whether Six genes play any role in the differentiation of TRCs after birth or whether the Eya-Six regulatory network also operates in the taste system. In this study, we described previously unreported expression patterns of Eya1 and Eya2 in the taste system from embryonic stages to adulthood. By using Eya1LacZ or Eya2LacZ knockin reporter mice, we show for the first time that these two genes are predominantly expressed in the endoderm-derived CVP and FOP taste buds located at the back of the tongue. Furthermore, our analyses reveal an early role for Eya1 in regulating embryonic tongue patterning and a later role for Eya1 and Eya2 in Type II cell generation or maintenance. Our results also indicate the importance of the Eya-Six network in regulating gustatory system morphogenesis.
Our expression studies and lineage tracing provide evidence that Eya1 is not actively expressed in the tongue from E10.5-E18.5, whereas Eya1-expressing progenitors at E9.5 give rise to tongue muscles, lingual epithelium, and epithelium-derived taste papillae. Without Eya1, these progenitors may not expand properly, resulting in growth arrest of the tongue and taste papillae. Thus, a primary function for Eya1 during embryonic tongue development may be to maintain the competence of these different progenitor cells to expand and generate tongue musculature and epithelial structures. This was supported by the observation that Eya1−/− tongues showed reduced cell proliferation and increased apoptosis in the Eya1-derived lineage cells. Since a single dose of tamoxifen treatment at E9.5 resulted in a mosaic pattern of β-Gal activity in the tongue epithelium and taste papillae/trench epithelium (Figure 4), it will be interesting to explore whether earlier administration of tamoxifen from E8.5, E9.0 or E9.25 will lead to more β-Gal-labeled cells in the epithelium of taste papillae and trenches.
In Eya1−/− tongues, we observed that Six1 expression was absent in the taste papillae and trench epithelium but remained present in the muscle cells (Figure 2). This finding is consistent with previous reports that Six1 expression is lost in the pharyngeal endoderm and ectoderm but not in the somites of Eya1−/− embryos (Xu et al., 2002; Giordani et al., 2007; Grifone et al., 2007; Niro et al., 2010). Additionally, Six1-null mice exhibit stunned trench formation in the CVP and FOP and abnormal taste papilla morphogenesis (Suzuki et al., 2010b). Our results further suggest that Eya1 is crucial for cranial neurogenesis (Zou et al., 2004) and that Eya1−/− tongues lack contact with nerve endings in developing papillae and trenches (Figure 1; Supplemental Figure S1), indicating a role for Eya1 in taste papilla development and differentiation. Interestingly, Six1 expression is also Eya1-dependent in cranial sensory neurogenesis (Zou et al., 2004), and Six1−/− taste papillae show a decreased nerve supply (Suzuki et al., 2010b). However, unlike Eya1, Six1 is actively expressed in taste papillae and trenches during their morphogenesis. Thus, it is unclear whether impaired trench formation and abnormal taste papilla morphogenesis in Six1−/− mice is due to the loss of early Six1 expression in pharyngeal progenitors before E10.5, Six1 expression during tongue morphogenesis, or both. Future inducible deletion of Six1 in the tongue may clarify this issue.
In contrast to Eya1, we found that Eya2, which is not expressed in pharyngeal endoderm or ectoderm at E9.5–10.5, is specifically expressed in the endoderm-derived CVP and FOP, shortly after the onset of their morphogenesis. It is also expressed in von Ebner’s glands. However, if Eya2 has a direct role in the development of these papillae and acts upstream of Six1, one might expect abnormal CVP and FOP development and loss of Six1 expression in Eya2−/− mice. Instead, Eya2−/− mice did not show noticeable anomalies in the initial papillary elevation and trench invagination as well as the pattern of Six1 expression. Thus, it is possible that the other two Eya family members, Eya3 or Eya4, may be coexpressed with Eya2 and function redundantly in tongue epithelial cells to regulate Six1 expression during CVP and FOP morphogenesis at embryonic stages. Future studies are required to address this issue.
Our analysis has shown that Eya1 is not expressed in the ectoderm-derived FUP distributed in the anterior part of the tongue. Instead, its expression is restricted to the endoderm-derived CVP and FOP taste buds in the posterior tongue, which differentiate postnatally. As Eya2 expression is also restricted to these endoderm-derived CVP and FOP taste buds, these two genes may function synergistically to regulate the CVP and FOP taste bud formation.
In the adult tongue, each taste bud is composed of three types of distinct TRCs. To maintain the taste bud tissues, the TRCs are all replaced over a period of weeks (∼every 2 weeks) by proliferative multipotent keratinocytes in the surrounding epithelium (Finger and Barlow, 2021). These epithelial stem/progenitor cells express the transcription factor Sox2 (Castillo-Azofeifa et al., 2018). The Sox2+ progenitors are induced to become postmitotic taste lineage-committed Type IV precursors located in the basal compartment of taste buds, and these committed precursors are capable of giving rise to Type I, II or III in adult buds (Miura et al., 2014). Type I cells make up approximately 50% of the differentiated cells in each bud and are glial-like cells thought to function as support cells within taste buds (Bartel et al., 2006; Yang et al., 2020). Type II cells make up approximately 20%–40% of TRCs detecting sweet, bitter, or umami stimuli, while Type III cells are the least common type sensitive to sour stimuli (Finger and Barlow, 2021). However, little is known about how distinct types of TRCs acquire their fate. To date, functional roles for transcription factors in TRC generation have only been demonstrated for Mash1 (Ascl1), which is required for Type III TRC development (Seta et al., 2011), and Pou2f3, which is required for Type II TRC generation (Matsumoto et al., 2011). Pou2f3-null tongues show loss of Type II TRCs and an increase in the number of Type III cells, with no detectable changes in the number of Type I cells. Six1 was previously reported to be expressed in a subset of Type II TRCs (Suzuki et al., 2010a); however, it remains unknown whether Six1 plays any role in the generation of Type II TRCs.
In this study, we found that Eya1 is expressed in a subset of Type II cells, and conditional deletion of Eya1 using Sox2CreER resulted in ∼50% reduction in the number of Pou2f3+ and IP3R3+ Type II cells in each taste bud (Table 2), whereas Six1+ Type II cells were reduced to ∼24% of those observed in the control. In contrast, although Eya2 showed continuous expression in the CVP and FOP after birth, its expression level was higher in cells located in the basal regions and some cells near the taste pore but relatively weak in the taste cells. While less severe than that observed in Eya1cKO mice, since Eya2-deficient mice also showed reduced numbers of Type II TRCs (Table 2), Eya2 may also have a role in the Type II TRC subtype lineage commitment. It should be noted that we used Eya2LacZ/+ heterozygous samples for the expression analyses. Thus, future work using an anti-Eya2 specific antibody for immunohistochemistry, when it becomes available, will clarify whether Eya2 is also specifically expressed in Type II TRC subsets. Nonetheless, since deletion of either gene reduces the number of Type II cells, it is possible that these two genes may cooperate to regulate Type II TRC lineage commitment or maintenance. Therefore, they may function together to regulate the expression of Six1, which could explain why some Six1+ TRCs are still present in adult Eya1cKO or Eya2-deficient buds. Our data show that some Eya1-or Eya2-β-Gal+ cells are located in the basal region of taste buds, which leads us to speculate that these cells may be progenitors or precursors. Hence, it is plausible that these genes may be required to regulate gene expression programs that direct Type II TRC-specific lineage commitment. However, future studies using progenitor- or precursor-specific markers are necessary to confirm whether the basal β-Gal+ cells are proliferative progenitors or postmitotic taste precursors.
It will be interesting to investigate whether Eya1 and Eya2 have redundant roles in the CVP and FOP taste buds in Eya1/Eya2 double mutant mice. Analysis of different types or subtypes of TRC marker genes in Eya1 and Eya2 double mutants will determine whether these two genes are involved in TRC differentiation. Future analyses on the possible role of other Eya genes and the Eya-Six pathway in regulating Type II TRC differentiation, as well as the regulatory relationship among Pou2f3, Eya, and Six genes, will help to elucidate the transcriptional network that controls the acquisition of Type II cell fate.
The original contributions presented in the study are included in the article/Supplementary Material, further inquiries can be directed to the corresponding author.
All animal protocols were approved by the Animal Care and Use Committee of the Icahn School of Medicine at Mount Sinai (protocol #06-0807).
TZ and P-XX designed the study, TZ conducted the experiments, TZ and P-XX analyzed the data and wrote the manuscript.
This work was supported by the National Institute on Deafness and Other Communication Disorders grant RO1DC 014718 (PXX).
We thank all Xu lab members for technical assistance and critical discussion.
The authors declare that the research was conducted in the absence of any commercial or financial relationships that could be construed as a potential conflict of interest.
All claims expressed in this article are solely those of the authors and do not necessarily represent those of their affiliated organizations, or those of the publisher, the editors and the reviewers. Any product that may be evaluated in this article, or claim that may be made by its manufacturer, is not guaranteed or endorsed by the publisher.
The Supplementary Material for this article can be found online at: https://www.frontiersin.org/articles/10.3389/fcell.2023.1126968/full#supplementary-material
Abdelhak, S., Kalatzis, V., Heilig, R., Compain, S., Samson, D., Vincent, C., et al. (1997). Clustering of mutations responsible for branchio-oto-renal (BOR) syndrome in the eyes absent homologous region (eyaHR) of EYA1. Hum. Mol. Genet. 6, 2247–2255. doi:10.1093/hmg/6.13.2247
Abdelhak, S., Kalatzis, V., Heilig, R., Compain, S., Samson, D., Vincent, C., et al. (1997). A human homologue of the Drosophila eyes absent gene underlies branchio-oto-renal (BOR) syndrome and identifies a novel gene family. Nat. Genet. 15 (2), 157–164. doi:10.1038/ng0297-157
Ahmed, M., Wong, E. Y., Sun, J., Xu, J., Wang, F., and Xu, P. X. (2012). Eya1-Six1 interaction is sufficient to induce hair cell fate in the cochlea by activating Atoh1 expression in cooperation with Sox2. Dev. Cell. 22 (2), 377–390. doi:10.1016/j.devcel.2011.12.006
Ahmed, M., Xu, J., and Xu, P. X. (2012). EYA1 and SIX1 drive the neuronal developmental program in cooperation with the SWI/SNF chromatin-remodeling complex and SOX2 in the mammalian inner ear. Development 139 (11), 1965–1977. doi:10.1242/dev.071670
Arnold, K., Sarkar, A., Yram, M. A., Polo, J. M., Bronson, R., Sengupta, S., et al. (2011). Sox2(+) adult stem and progenitor cells are important for tissue regeneration and survival of mice. Cell. Stem Cell. 9 (4), 317–329. doi:10.1016/j.stem.2011.09.001
Barlow, L. A., Chien, C. B., and Northcutt, R. G. (1996). Embryonic taste buds develop in the absence of innervation. Development 122 (4), 1103–1111. doi:10.1242/dev.122.4.1103
Barlow, L. A., and Klein, O. D. (2015). Developing and regenerating a sense of taste. Neural Crest Placodes 111, 401–419. doi:10.1016/bs.ctdb.2014.11.012
Bartel, D. L., Sullivan, S. L., Lavoie, E. G., Sevigny, J., and Finger, T. E. (2006). Nucleoside triphosphate diphosphohydrolase-2 is the ecto-ATPase of type I cells in taste buds. J. Comp. Neurol. 497 (1), 1–12. doi:10.1002/cne.20954
Boggs, K., Venkatesan, N., Mederacke, I., Komatsu, Y., Stice, S., Schwabe, R. F., et al. (2016). Contribution of underlying connective tissue cells to taste buds in mouse tongue and soft palate. PLoS One 11 (1), e0146475. doi:10.1371/journal.pone.0146475
Bonini, N. M., Bui, Q. T., Gray-Board, G. L., and Warrick, J. M. (1997). The Drosophila eyes absent gene directs ectopic eye formation in a pathway conserved between flies and vertebrates. Development 124 (23), 4819–4826. doi:10.1242/dev.124.23.4819
Buller, C., Xu, X., Marquis, V., Schwanke, R., and Xu, P. X. (2001). Molecular effects of Eya1 domain mutations causing organ defects in BOR syndrome. Hum. Mol. Genet. 10 (24), 2775–2781. doi:10.1093/hmg/10.24.2775
Castillo-Azofeifa, D., Seidel, K., Gross, L., Golden, E. J., Jacquez, B., Klein, O. D., et al. (2018). SOX2 regulation by hedgehog signaling controls adult lingual epithelium homeostasis. Development 145 (14), dev164889. doi:10.1242/dev.164889
Chen, B., Kim, E. H., and Xu, P. X. (2009). Initiation of olfactory placode development and neurogenesis is blocked in mice lacking both Six1 and Six4. Dev. Biol. 326 (1), 75–85. doi:10.1016/j.ydbio.2008.10.039
Cui, X. G., Marshall, B., Shi, N., Chen, S. Y., Rekaya, R., and Liu, H. X. (2017). RNA-Seq analysis on chicken taste sensory organs: An ideal system to study organogenesis. Sci. Rep. 7, 9131. doi:10.1038/s41598-017-09299-7
Danielian, P. S., Muccino, D., Rowitch, D. H., Michael, S. K., and McMahon, A. P. (1998). Modification of gene activity in mouse embryos in utero by a tamoxifen-inducible form of Cre recombinase. Curr. Biol. 8 (24), 1323–1326. doi:10.1016/s0960-9822(07)00562-3
Engert, S., Liao, W. P., Burtscher, I., and Lickert, H. (2009). Sox17-2A-iCre: A knock-in mouse line expressing Cre recombinase in endoderm and vascular endothelial cells. Genesis 47 (9), 603–610. doi:10.1002/dvg.20540
Fan, D., Chettouh, Z., Consalez, G. G., and Brunet, J. F. (2019). Taste bud formation depends on taste nerves. Elife 8, e49226. doi:10.7554/eLife.49226
Feng, P., Huang, L., and Wang, H. (2014). Taste bud homeostasis in health, disease, and aging. Chem. Senses 39 (1), 3–16. doi:10.1093/chemse/bjt059
Finger, T. E., and Barlow, L. A. (2021). Cellular diversity and regeneration in taste buds. Curr. Opin. Physiol. 20, 146–153. doi:10.1016/j.cophys.2021.01.003
Gibbons, J. R., and Sadiq, N. M. (2022). Neuroanatomy, neural taste pathway (Treasure Island (FL):: StatPearls Publishing).
Golden, E. J., Larson, E. D., Shechtman, L. A., Trahan, G. D., Gaillard, D., Fellin, T. J., et al. (2021). Onset of taste bud cell renewal starts at birth and coincides with a shift in SHH function. Elife 10, e64013. doi:10.7554/eLife.64013
Grifone, R., Demignon, J., Giordani, J., Niro, C., Souil, E., Bertin, F., et al. (2007). Eya1 and Eya2 proteins are required for hypaxial somitic myogenesis in the mouse embryo. Dev. Biol. 302 (2), 602–616. doi:10.1016/j.ydbio.2006.08.059
Halder, G., Callaerts, P., Flister, S., Walldorf, U., Kloter, U., and Gehring, W. J. (1998). Eyeless initiates the expression of both sine oculis and eyes absent during Drosophila compound eye development. Development 125 (12), 2181–2191. doi:10.1242/dev.125.12.2181
Ishihara, T., Ikeda, K., Sato, S., Yajima, H., and Kawakami, K. (2008). Differential expression of Eya1 and Eya2 during chick early embryonic development. Gene Expr. Patterns 8 (5), 357–367. doi:10.1016/j.gep.2008.01.003
Iwata, J., Suzuki, A., Pelikan, R. C., Ho, T. V., and Chai, Y. (2013). Noncanonical transforming growth factor β (TGFβ) signaling in cranial neural crest cells causes tongue muscle developmental defects. J. Biol. Chem. 288 (41), 29760–29770. doi:10.1074/jbc.M113.493551
Giordani, J., Bajard, L., Demignon, J., Daubas, P., Buckingham, M., and Maire, P. (2007). Six proteins regulate the activation of Myf5 expression in embryonic mouse limbs. Proc. Natl. Acad. Sci. U. S. A. 104 (27), 11310–11315. doi:10.1073/pnas.0611299104
Kim, J. Y., Mochizuki, T., Akita, K., and Jung, H. S. (2003). Morphological evidence of the importance of epithelial tissue during mouse tongue development. Exp. Cell. Res. 290 (2), 217–226. doi:10.1016/s0014-4827(03)00319-7
Laclef, C., Hamard, G., Demignon, J., Souil, E., Houbron, C., and Maire, P. (2003). Altered myogenesis in Six1-deficient mice. Development 130 (10), 2239–2252. doi:10.1242/dev.00440
Lee, M. J., Kim, J. Y., Lee, S. I., Sasaki, H., Lunny, D. P., Lane, E. B., et al. (2006). Association of Shh and Ptc with keratin localization in the initiation of the formation of circumvallate papilla and von Ebner's gland. Cell. Tissue Res. 325 (2), 253–261. doi:10.1007/s00441-006-0160-1
Li, J., Cheng, C., Xu, J., Zhang, T., Tokat, B., Dolios, G., et al. (2022). The transcriptional coactivator Eya1 exerts transcriptional repressive activity by interacting with REST corepressors and REST-binding sequences to maintain nephron progenitor identity. Nucleic Acids Res. 50 (18), 10343–10359. doi:10.1093/nar/gkac760
Li, J., Xu, J., Jiang, H., Zhang, T., Ramakrishnan, A., Shen, L., et al. (2021). Chromatin remodelers interact with Eya1 and Six2 to target enhancers to control nephron progenitor cell maintenance. J. Am. Soc. Nephrol. 32 (11), 2815–2833. doi:10.1681/ASN.2021040525
Li, J., Zhang, T., Ramakrishnan, A., Fritzsch, B., Xu, J., Wong, E. Y. M., et al. (2020). Dynamic changes in cis-regulatory occupancy by Six1 and its cooperative interactions with distinct cofactors drive lineage-specific gene expression programs during progressive differentiation of the auditory sensory epithelium. Nucleic Acids Res. 48 (6), 2880–2896. doi:10.1093/nar/gkaa012
Li, X., Oghi, K. A., Zhang, J., Krones, A., Bush, K. T., Glass, C. K., et al. (2003). Eya protein phosphatase activity regulates Six1-Dach-Eya transcriptional effects in mammalian organogenesis. Nature 426 (6964), 247–254. doi:10.1038/nature02083
Matsumoto, I., Ohmoto, M., Narukawa, M., Yoshihara, Y., and Abe, K. (2011). Skn-1a (Pou2f3) specifies taste receptor cell lineage. Nat. Neurosci. 14 (6), 685–687. doi:10.1038/nn.2820
Mbiene, J. P., and Roberts, J. D. (2003). Distribution of keratin 8-containing cell clusters in mouse embryonic tongue: Evidence for a prepattern for taste bud development. J. Comp. Neurol. 457 (2), 111–122. doi:10.1002/cne.10551
Mistretta, C. M., and Liu, H. X. (2006). Development of fungiform papillae: Patterned lingual gustatory organs. Arch. Histol. Cytol. 69 (4), 199–208. doi:10.1679/aohc.69.199
Miura, H., Scott, J. K., Harada, S., and Barlow, L. A. (2014). Sonic hedgehog-expressing basal cells are general post-mitotic precursors of functional taste receptor cells. Dev. Dyn. 243 (10), 1286–1297. doi:10.1002/dvdy.24121
Nakayama, A., Miura, H., Ooki, M., and Harada, S. (2015). During development intense Sox2 expression marks not only Prox1-expressing taste bud cell but also perigemmal cell lineages. Cell. Tissue Res. 359 (3), 743–753. doi:10.1007/s00441-014-2076-5
Niro, C., Demignon, J., Vincent, S., Liu, Y., Giordani, J., Sgarioto, N., et al. (2010). Six1 and Six4 gene expression is necessary to activate the fast-type muscle gene program in the mouse primary myotome. Dev. Biol. 338 (2), 168–182. doi:10.1016/j.ydbio.2009.11.031
Northcutt, R. G. (2004). Taste buds: Development and evolution. Brain Behav. Evol. 64 (3), 198–206. doi:10.1159/000079747
Oakley, B., and Witt, M. (2004). Building sensory receptors on the tongue. J. Neurocytol. 33 (6), 631–646. doi:10.1007/s11068-005-3332-0
Ohmoto, M., Kitamoto, S., and Hirota, J. (2021). Expression of Eya1 in mouse taste buds. Cell. Tissue Res. 383 (3), 979–986. doi:10.1007/s00441-020-03311-9
Ohmoto, M., Lei, W., Yamashita, J., Hirota, J., Jiang, P., and Matsumoto, I. (2020). SOX2 regulates homeostasis of taste bud cells and lingual epithelial cells in posterior tongue. PLoS One 15 (10), e0240848. doi:10.1371/journal.pone.0240848
Okubo, T., Pevny, L. H., and Hogan, B. L. (2006). Sox2 is required for development of taste bud sensory cells. Genes. Dev. 20 (19), 2654–2659. doi:10.1101/gad.1457106
Ozaki, H., Nakamura, K., Funahashi, J., Ikeda, K., Yamada, G., Tokano, H., et al. (2004). Six1 controls patterning of the mouse otic vesicle. Development 131 (3), 551–562. doi:10.1242/dev.00943
Paulson, R. B., Hayes, T. G., and Sucheston, M. E. (1985). Scanning electron microscope study of tongue development in the CD-1 mouse fetus. J. Craniofac Genet. Dev. Biol. 5 (1), 59–73.
Pignoni, F., Hu, B., Zavitz, K. H., Xiao, J., Garrity, P. A., and Zipursky, S. L. (1997). The eye-specification proteins So and Eya form a complex and regulate multiple steps in Drosophila eye development. Cell. 91 (7), 881–891. doi:10.1016/s0092-8674(00)80480-8
Rayapureddi, J. P., Kattamuri, C., Steinmetz, B. D., Frankfort, B. J., Ostrin, E. J., Mardon, G., et al. (2003). Eyes absent represents a class of protein tyrosine phosphatases. Nature 426 (6964), 295–298. doi:10.1038/nature02093
Rothova, M., Thompson, H., Lickert, H., and Tucker, A. S. (2012). Lineage tracing of the endoderm during oral development. Dev. Dyn. 241 (7), 1183–1191. doi:10.1002/dvdy.23804
Ruf, R. G., Xu, P. X., Silvius, D., Otto, E. A., Beekmann, F., Muerb, U. T., et al. (2004). SIX1 mutations cause branchio-oto-renal syndrome by disruption of EYA1-SIX1-DNA complexes. Proc. Natl. Acad. Sci. U. S. A. 101 (21), 8090–8095. doi:10.1073/pnas.0308475101
Seta, Y., Oda, M., Kataoka, S., Toyono, T., and Toyoshima, K. (2011). Mash1 is required for the differentiation of AADC-positive type III cells in mouse taste buds. Dev. Dyn. 240 (4), 775–784. doi:10.1002/dvdy.22576
Shah, A. M., Krohn, P., Baxi, A. B., Tavares, A. L. P., Sullivan, C. H., Chillakuru, Y. R., et al. (2020). Six1 proteins with human branchio-oto-renal mutations differentially affect cranial gene expression and otic development. Dis. Models Mech. 13 (3), dmm043489. doi:10.1242/dmm.043489
Shuler, C. F., and Dalrymple, K. R. (2001). Molecular regulation of tongue and craniofacial muscle differentiation. Crit. Rev. Oral Biol. Med. 12 (1), 3–17. doi:10.1177/10454411010120010201
Suzuki, Y. (2008). Expression of Sox2 in mouse taste buds and its relation to innervation. Cell. Tissue Res. 332 (3), 393–401. doi:10.1007/s00441-008-0600-1
Suzuki, Y., Ikeda, K., and Kawakami, K. (2011). Development of gustatory papillae in the absence of Six1 and Six4. J. Anat. 219 (6), 710–721. doi:10.1111/j.1469-7580.2011.01435.x
Suzuki, Y., Ikeda, K., and Kawakami, K. (2010). Expression of Six1 and Six4 in mouse taste buds. J. Mol. Histol. 41 (4-5), 205–214. doi:10.1007/s10735-010-9280-8
Suzuki, Y., Ikeda, K., and Kawakami, K. (2010). Regulatory role of Six1 in the development of taste papillae. Cell. Tissue Res. 339 (3), 513–525. doi:10.1007/s00441-009-0917-4
Tootle, T. L., Silver, S. J., Davies, E. L., Newman, V., Latek, R. R., Mills, I. A., et al. (2003). The transcription factor Eyes absent is a protein tyrosine phosphatase. Nature 426 (6964), 299–302. doi:10.1038/nature02097
Torii, D., Soeno, Y., Fujita, K., Sato, K., Aoba, T., and Taya, Y. (2016). Embryonic tongue morphogenesis in an organ culture model of mouse mandibular arches: Blocking sonic hedgehog signaling leads to microglossia. Vitro Cell. Dev. Biol. Anim. 52 (1), 89–99. doi:10.1007/s11626-015-9951-6
Witt, M. (2019). Anatomy and development of the human taste system. Handb. Clin. Neurol. 164, 147–171. doi:10.1016/B978-0-444-63855-7.00010-1
Wong, E. Y., Ahmed, M., and Xu, P. X. (2013). EYA1-SIX1 complex in neurosensory cell fate induction in the mammalian inner ear. Hear Res. 297, 13–19. doi:10.1016/j.heares.2012.09.009
Xu, J., Li, J., Ramakrishnan, A., Yan, H., Shen, L., and Xu, P. X. (2022). Six1 and Six2 of the sine oculis homeobox subfamily are not functionally interchangeable in mouse nephron formation. Front. Cell. Dev. Biol. 10, 815249. doi:10.3389/fcell.2022.815249
Xu, J., Li, J., Zhang, T., Jiang, H., Ramakrishnan, A., Fritzsch, B., et al. (2021). Chromatin remodelers and lineage-specific factors interact to target enhancers to establish proneurosensory fate within otic ectoderm. Proc. Natl. Acad. Sci. U. S. A. 118 (12), e2025196118. doi:10.1073/pnas.2025196118
Xu, J., Wong, E. Y., Cheng, C., Li, J., Sharkar, M. T., Xu, C. Y., et al. (2014). Eya1 interacts with Six2 and Myc to regulate expansion of the nephron progenitor pool during nephrogenesis. Dev. Cell. 31 (4), 434–447. doi:10.1016/j.devcel.2014.10.015
Xu, J., and Xu, P. X. (2015). Eya-six are necessary for survival of nephrogenic cord progenitors and inducing nephric duct development before ureteric bud formation. Dev. Dyn. 244 (7), 866–873. doi:10.1002/dvdy.24282
Xu, P. X., Adams, J., Peters, H., Brown, M. C., Heaney, S., and Maas, R. (1999). Eya1-deficient mice lack ears and kidneys and show abnormal apoptosis of organ primordia. Nat. Genet. 23 (1), 113–117. doi:10.1038/12722
Xu, P. X., Cheng, J., Epstein, J. A., and Maas, R. L. (1997). Mouse Eya genes are expressed during limb tendon development and encode a transcriptional activation function. Proc. Natl. Acad. Sci. U. S. A. 94 (22), 11974–11979. doi:10.1073/pnas.94.22.11974
Xu, P. X. (2013). The EYA-SO/SIX complex in development and disease. Pediatr. Nephrol. 28 (6), 843–854. doi:10.1007/s00467-012-2246-1
Xu, P. X., Woo, I., Her, H., Beier, D. R., and Maas, R. L. (1997). Mouse Eya homologues of the Drosophila eyes absent gene require Pax6 for expression in lens and nasal placode. Development 124 (1), 219–231. doi:10.1242/dev.124.1.219
Xu, P. X., Zheng, W., Huang, L., Maire, P., Laclef, C., and Silvius, D. (2003). Six1 is required for the early organogenesis of mammalian kidney. Development 130 (14), 3085–3094. doi:10.1242/dev.00536
Xu, P. X., Zheng, W., Laclef, C., Maire, P., Maas, R. L., Peters, H., et al. (2002). Eya1 is required for the morphogenesis of mammalian thymus, parathyroid and thyroid. Development 129 (13), 3033–3044. doi:10.1242/dev.129.13.3033
Yang, R., Dzowo, Y. K., Wilson, C. E., Russell, R. L., Kidd, G. J., Salcedo, E., et al. (2020). Three-dimensional reconstructions of mouse circumvallate taste buds using serial blockface scanning electron microscopy: I. Cell types and the apical region of the taste bud. J. Comp. Neurol. 528 (5), 756–771. doi:10.1002/cne.24779
Zervas, M., Millet, S., Ahn, S., and Joyner, A. L. (2004). Cell behaviors and genetic lineages of the mesencephalon and rhombomere 1. Neuron 43 (3), 345–357. doi:10.1016/j.neuron.2004.07.010
Zhang, T., Xu, J., and Xu, P. X. (2021). Eya2 expression during mouse embryonic development revealed by Eya2(lacZ) knockin reporter and homozygous mice show mild hearing loss. Dev. Dyn. 250 (10), 1450–1462. doi:10.1002/dvdy.326
Zheng, W., Huang, L., Wei, Z. B., Silvius, D., Tang, B., and Xu, P. X. (2003). The role of Six1 in mammalian auditory system development. Development 130 (17), 3989–4000. doi:10.1242/dev.00628
Zou, D., Erickson, C., Kim, E. H., Jin, D., Fritzsch, B., and Xu, P. X. (2008). Eya1 gene dosage critically affects the development of sensory epithelia in the mammalian inner ear. Hum. Mol. Genet. 17 (21), 3340–3356. doi:10.1093/hmg/ddn229
Zou, D., Silvius, D., Davenport, J., Grifone, R., Maire, P., and Xu, P. X. (2006). Patterning of the third pharyngeal pouch into thymus/parathyroid by Six and Eya1. Dev. Biol. 293 (2), 499–512. doi:10.1016/j.ydbio.2005.12.015
Zou, D., Silvius, D., Fritzsch, B., and Xu, P. X. (2004). Eya1 and Six1 are essential for early steps of sensory neurogenesis in mammalian cranial placodes. Development 131 (22), 5561–5572. doi:10.1242/dev.01437
Keywords: Eya1, Eya2, tongue, taste bud, type II taste cells, circumvallate and foliate papillae
Citation: Zhang T and Xu P-X (2023) The role of Eya1 and Eya2 in the taste system of mice from embryonic stage to adulthood. Front. Cell Dev. Biol. 11:1126968. doi: 10.3389/fcell.2023.1126968
Received: 18 December 2022; Accepted: 10 April 2023;
Published: 25 April 2023.
Edited by:
De-Li Shi, Sorbonne Université, FranceReviewed by:
Kathryn Medler, University at Buffalo, United StatesCopyright © 2023 Zhang and Xu. This is an open-access article distributed under the terms of the Creative Commons Attribution License (CC BY). The use, distribution or reproduction in other forums is permitted, provided the original author(s) and the copyright owner(s) are credited and that the original publication in this journal is cited, in accordance with accepted academic practice. No use, distribution or reproduction is permitted which does not comply with these terms.
*Correspondence: Pin-Xian Xu, cGlueGlhbi54dUBtc3NtLmVkdQ==
Disclaimer: All claims expressed in this article are solely those of the authors and do not necessarily represent those of their affiliated organizations, or those of the publisher, the editors and the reviewers. Any product that may be evaluated in this article or claim that may be made by its manufacturer is not guaranteed or endorsed by the publisher.
Research integrity at Frontiers
Learn more about the work of our research integrity team to safeguard the quality of each article we publish.