- 1Department of Biology, Christian Brothers University, Memphis, TN, United States
- 2College of Public Health, University of South Florida, Tampa, FL, United States
- 3Placental Research Group LLC., Maplewood, NJ, United States
- 4Instituto Boliviano de Biología de Altura, Universidad de San Andreas Mayor, La Paz, Bolivia
Introduction: The placenta mediates fetal growth by regulating gas and nutrient exchange between the mother and the fetus. The cell type in the placenta where this nutrient exchange occurs is called the syncytiotrophoblast, which is the barrier between the fetal and maternal blood. Residence at high-altitude is strongly associated with reduced 3rd trimester fetal growth and increased rates of complications such as preeclampsia. We asked whether altitude and/or ancestry-related placental gene expression contributes to differential fetal growth under high-altitude conditions, as native populations have greater fetal growth than migrants to high-altitude.
Methods: We have previously shown that methylation differences largely accounted for altitude-associated differences in placental gene expression that favor improved fetal growth among high-altitude natives. We tested for differences in DNA methylation between Andean and European placental samples from Bolivia [La Paz (∼3,600 m) and Santa Cruz, Bolivia (∼400 m)]. One group of genes showing significant altitude-related differences are those involved in cell fusion and membrane repair in the syncytiotrophoblast. Dysferlin (DYSF) shows greater expression levels in high- vs. low-altitude placentas, regardless of ancestry. DYSF has a single nucleotide variant (rs10166384;G/A) located at a methylation site that can potentially stimulate or repress DYSF expression. Following up with individual DNA genotyping in an expanded sample size, we observed three classes of DNA methylation that corresponded to individual genotypes of rs10166384 (A/A < A/G < G/G). We tested whether these genotypes are under Darwinian selection pressure by sequencing a ∼2.5 kb fragment including the DYSF variants from 96 Bolivian samples and compared them to data from the 1000 genomes project.
Results: We found that balancing selection (Tajima’s D = 2.37) was acting on this fragment among Andeans regardless of altitude, and in Europeans at high-altitude (Tajima’s D = 1.85).
Discussion: This supports that balancing selection acting on dysferlin is capable of altering DNA methylation patterns based on environmental exposure to high-altitude hypoxia. This finding is analogous to balancing selection seen frequency-dependent selection, implying both alleles are advantageous in different ways depending on environmental circumstances. Preservation of the adenine (A) and guanine (G) alleles may therefore aid both Andeans and Europeans in an altitude dependent fashion.
Introduction
Natural selection is an evolutionary mechanism contributing to differences between populations at both the genotypic and phenotypic levels (Barreiro et al., 2008). Two major types of natural selection cause changes in gene allele frequencies; directional selection and balancing selection. A locus under directional selection in a population has one allele frequency that is significantly greater than the other suggesting the more frequent allele is beneficial compared to the less frequent allele (Smith and Haigh, 1974). An example of positive directional selection in humans is lactase persistence among individuals residing in arid regions (Tishkoff et al., 2007). Three major sequence variants within the lactase gene show positive selection. Functionally, these variants promote better absorption of nutrients and water from milk, providing an adaptive advantage in arid regions. Conversely, a locus experiencing balancing selection has two alleles of roughly equal frequency suggesting both alleles may be beneficial (Dobzhansky and Dobzhansky, 1970). A well-known example of balancing selection is seen in those individuals who are heterozygotes for the sickle cell allele associated with hemoglobin beta (Allison, 1954; Hoff et al., 2001). Heterozygous individuals have mostly healthy erythrocytes (i.e., RBC) but also have resistance to malaria infection of these same erythrocytes (Allison, 1954). In contrast, homozygous individuals either are more vulnerable to malaria infection or have sickled erythrocytes that impair oxygen delivery. Therefore, the heterozygote condition is favored by natural selection as it enables efficient transport of oxygen while simultaneously preventing malaria infection. In contrast, homozygotes are disadvantaged depending on environmental context. In evolutionary theory, this phenomenon is called heterozygote advantage. Balancing selection also occurs through frequency-dependent selection, where two alleles have intermediate frequencies in which both alleles have roughly equal allele frequencies, known as balanced polymorphisms suggesting that both alleles may be beneficial (Hedrick, 2007). Examples of frequency dependent selection seen in humans include allelic variation in the human leukocyte antigens where an intermediate number of alleles may allow for greater recognition of pathogens (Brandt et al., 2018). In contrast to selection pressures, there are also random events that change allele frequencies. Genetic drift, for example, is often found in small populations isolated by migration, geography and/or culture. Drift is caused by both founder effects, where a small founder population is not genetically representative of the parent population (Tournebize et al., 2022) and population bottlenecks (rapid loss of population resulting in a different genetic make-up of the remaining individuals) (Amos and Hoffman, 2010; Masel, 2011). We can distinguish between positive selection, balancing selection, and genetic drift by calculating the Tajima’s D value for a DNA sequence (Tajima, 1989).
The high-altitude environment exerts selection pressure, resulting in allele frequency changes in different regions of the human genome. Early studies among Tibetans (Beall et al., 2010) and Andeans (Bigham et al., 2013) found signatures of positive selection in genes associated with the hypoxia-inducible factors (HIFs), transcription factors critical to oxygen homeostasis. These include genes encoding endothelial PAS domain protein 1 (HIF-2 alpha) and Egl-9 family hypoxia inducible factor 1 (PHD2, a prolyl hydroxylase involved in HIF regulation).
We found that placental genes associated with cell fusion and proliferation, critical processes in placental development, were differentially expressed between individuals residing at high and low-altitude. Of 36 altitude-associated differentially expressed genes, 8 had significant correlation between gene expression and DNA methylation (Gundling et al., 2018). One of these is dysferlin (DYSF). Dysferlin is linked to the development of syncytial cells and membrane repair. DYSF is a gene spanning roughly 230 Kb with more than 50 coding exons. Within the third intron of DYSF, there is a single nucleotide polymorphism (SNP) that causes that site to either be a Guanine (G) or and Adenine (A) that changes a CpG site to a CpA. CpG sites are commonly methylated, whereas the change from a G to A makes methylation less likely as methylation rarely occurs at CpA sites (Patil et al., 2014). Methylation is a common epigenetic mark that can be induced not only in developmental processes as a regulated event, but also by environmental change (Uddin et al., 2010). DYSF encodes a membrane bound protein associated with calcium regulated membrane repair (Codding et al., 2016). DYSF plays an important role in the formation of syncytial tissues, which contain multinucleated cells formed by the fusion of mononucleated cells (De Luna et al., 2004; Han and Campbell, 2007; Omata et al., 2013) (Figure 1). In the placenta, dysferlin is expressed in the apical membrane (facing the maternal blood) of the multi-nucleated syncytiotrophoblast. Expression is induced when the mononucleated cytotrophoblast fuse during syncytiotrophoblast formation and repair (Vandre et al., 2007). Methylation likely plays a role in regulating gene expression of DYSF. It is known to be a methylation driven gene in other cells such as monocytes in cardiovascular diseases (Zhang et al., 2022).
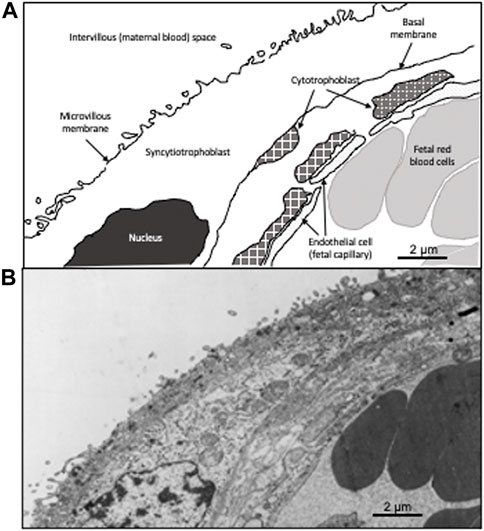
FIGURE 1. Drawing of a villous cross-section of a human placenta. A cross-section of the villous tissue of the human placenta. (A) is a schematic showing the composition the maternal fetal interface. The outer layer is comprised of multi-nucleated syncytiotrophoblast in contact with the maternal blood. The second layer is comprised of mononucleated cytotrophoblast, which fuse to form the syncytiotrophoblast. Below the cytotrophoblast is the endothelial wall of the fetal capillary. Fetal red blood cells (erythrocytes) are present in the fetal capillary. (B) shows the transmission electron micrograph of the placenta that was used to generate (A). The dark cells on the right are fetal erythrocytes while the space on the upper left is the maternal blood (intervillous) space. The two circulatory systems are separated by the syncytiotrophoblast and the cytotrophoblast.
We sought to test whether natural selection, induced by the high-altitude environment, acted on dysferlin ultimately changing allele frequency in relation to DNA methylation and gene expression. We examined whether the population was in Hardy-Weinberg equilibrium and distinguished between selection and genetic drift using statistical tests (i.e., Tajima’s D). We found that individuals residing at high-altitude had a signature of frequency-dependent balancing selection, suggesting that the possession of both alleles (A and G) may be beneficial for Andean and European individuals at high-altitude. We have previously posited that Andean individuals have genetically adapted to their high-altitude environment by methylation-favored, and therefore environmentally modifiable placental physiology (Gundling et al., 2018). In the case of dysferlin, we hypothesized that the heterozygote dysferlin alleles would be more prevalent among Andeans, whereas their European counterparts (i.e., those of Spanish descent) would not yet have evolved allele frequencies reflecting balancing selection. We tested this proposition by comparing allele frequencies in high- and low-altitude Bolivian populations of both indigenous and European descent.
Materials and methods
Samples
Term placentas from 96 Bolivian individuals were collected from La Paz, Bolivia (high-altitude; 3,600 m n = 48) and Santa Cruz, Bolivia (low-altitude; 400 m n = 48). Of our 96 individuals 48 were of European ancestry and 48 were of Andean ancestry. The placentas were from healthy, term (≥ 37 weeks gestation), singleton pregnancies in individuals without pregnancy complications delivered via elective caesarian section. All women gave written informed consent to protocols approved by the Bolivian National Bioethics Committee, the Universidad Mayor de San Andrés, Instituto Boliviano de Biología de Altura Consejo Tecnico, and the United States Institutional IRB from the University of Medicine and Dentistry, New Jersey Medical School in Newark, NJ (now Rutgers). Tissue for transmission electron microscopy was rinsed three times over a 24-h period in 0.1 M sodium cacodylate, pH 7.4, and then post-fixed in 1% osmium tetroxide in cacodylate buffer at 4°C for 1 h. After a brief rinse in buffer the tissue was dehydrated in ascending concentrations of alcohol, embedded in Taab resin, and polymerized at 60°C for 36–48 h. Ultrathin sections were cut on an LKB ultramicrotome, and grids were stained with uranyl acetate and Reynold’s lead citrate prior to examination in a Phillips EM 301 electron microscope (Figure 1).
Identification of differentially methylated sites associated with differential gene expression
DNA was extracted from the villous tissue of term placenta and used for DNA methylation analysis and SNP genotyping. DNA methylation data was generated using the HM450k methylation microarray chips using previously described methods (Gundling et al., 2018). Validation was performed using 350 ng of placental DNA from 8 individuals from our sample groups as well as high and low methylation controls (Zymo Research, Irvine, CA). Samples were bisulfite converted using the Zymo EZ DNA Methylation-Gold Kit (Zymo Research, Irvine, CA). The samples chosen for pyrosequencing were those that had methylation microarray data used in Gundling et al. (2018), and provided an even representation of each dysferlin genotype (G/G, G/A, or A/A). Primers developed for this study were designed using the PyroMark Q24 Assay Design Software 2.0 (Qiagen, Hilden, Germany) (Supplementary Table S1). The amplicon fragment was ∼60 bp containing three different CpG sites and was amplified using the Qiagen PyroMark PCR master mix and the manufacturer's suggested protocol (Supplementary Table S1). Pyrosequencing was performed on the PyroMarkQ24 Advanced using a sequencing primer (Supplementary Table S1) as well as the standard PyroMarkQ24 Advanced reagents and protocol.
Single nucleotide polymorphism genotyping
We genotyped SNP rs10166384 within dysferlin (DYSF) using DNA from term placentas of 96 individuals (48 Andean, 48 European) collected from La Paz, Bolivia (3,600 m) and Santa Cruz, Bolivia (400 m). These included the 45 individuals from our prior study in which three methylation patterns for DYSF were observed (Gundling et al., 2018). We genotyped SNP rs10166384 in all 96 individuals using a TaqMan SNP genotyping assay (TaqMan assay C__387100_10). This probe was 50 nucleotides long with rs10166384 (A/G) being located at the 26th base. The Adenine was labeled with VIC and the Guanine was labeled with FAM. The assay was run on an ABI 7500 qPCR machine (Applied Biosystems, CA). Standard run conditions used were used by the TaqMan SNP genotyping assay provided in the ABI 7500 manual. We then calculated the allele frequencies of the A and G allele for each of the four sample populations. We then tested to see if the populations are in Hardy-Weinberg equilibrium. When a population is within Hardy-Weinberg equilibrium it is assumed to have no selection, no mutation, no migration, a large population size, and random mating. Chi-square analysis was performed to determine if the proportion of genotypes in each of the four sample groups were significantly outside of Hardy-Weinberg equilibrium (p < 0.05).
Detecting natural selection
To determine if natural selection was acting on the SNP of interest (rs10166384) we amplified a ∼2,500 base pair region using polymerase chain reaction from the placental DNA of the 96 pregnancies (Supplementary Table S1). PCR products were purified using the QIAquick PCR purification kit (Qiagen, Hilden, Germany). Between 30 and 50 μg of each PCR product was sequenced at the University of Illinois, Urbana Champaign (UIUC) core sequencing center (Urbana, IL). The sequences generated from each primer were aligned using Sequencher (Gene Codes, Ann Arbor, MI). The complete sequences from each sample were then aligned to each other using the ClustalW (Thompson et al., 1994) algorithm implemented in MEGA version 6.06 (Tamura et al., 2013). Once sequences were aligned, a variant call format file (vcf file) was generated using SNP-sites (Page et al., 2016). We calculated allele frequencies for each polymorphism, determined if each subpopulation was in Hardy-Weinberg equilibrium, and calculated Tajima’s D for all SNPs with a minor allele > 0.05 using vcftools version 0.1.13 (Tajima, 1989; Danecek et al., 2011).
Results
By analyzing the DNA methylation patterns of genes we previously found to be differentially expressed (Gundling et al., 2018), we found a probe (cg09829645) within the third intron of dysferlin that had three distinct levels of DNA methylation (Figure 2A).
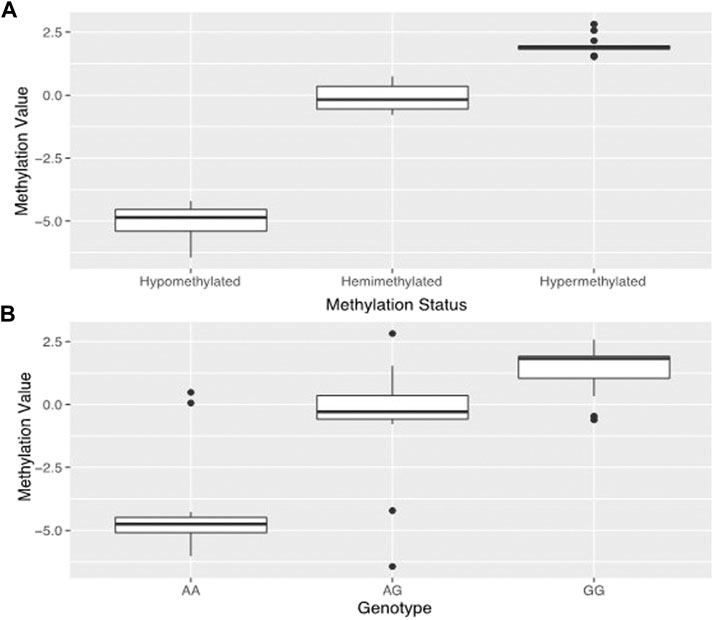
FIGURE 2. Estimated genotypes based on methylation. Prediction and confirmation of genotype using DNA methylation status at methylation probe cg09829645 which is in the same location as dysferlin variant rs10166384. Data used to generate (A) was taken from the 45 samples used in the original study. (A) shows the tripartite distribution of methylation levels detected for dysferlin at HM450k methylation probecg09829645 in the 45 placentas from our original study (Gundling et al., 2018). One group is hypomethylated, one group is hemimethylated, and one group is hypermethylated. (B) shows the mean methylation estimates for genotypes sampled at the rs10166384 variant in 96 placentas including the 45 from the 2018 study. Those with the AA genotype have low levels of methylation, those with GG genotype have high levels of DNA methylation and those with the AG genotype tend to have methylation levels that fall in between the two homozygous groups. Methylation values (M-values) were calculated according to Du et al., 2010.
One group showed hypomethylation with low levels of DNA methylation (M = −5.01 ± 0.67), one with hypermethylated, high levels of DNA methylation (M = 1.97 ± 0.36), and one hemi-methylated with median DNA methylation levels (M = −0.11 ± 0.49). This probe is in the same position as the polymorphism rs10166384 and can be either a Guanine (G) or an Adenine (A) nucleotide. By genotyping this site in the 96 individuals of Andean and European descent from high and low-altitude, we found that the levels of DNA methylation corresponded to the three possible different genotypes at rs10166384 (Figure 2B). Those that were homozygous for the A allele (A/A) had on average the lowest levels of DNA methylation (M = −4.21 ± 1.96), those that there homozygous for the G allele (G/G) had on average the highest levels of DNA methylation (M = 1.41 ± 0.97), and those that were heterozygous (A/G) had methylation values that fell in between the two homozygous groups (M = −0.51 ± 2.06) (Figure 2B). The genotype-specific DNA methylation was confirmed through pyrosequencing a region containing rs10166384 in a subset of individuals for which we have methylation microarray data for probe cg09829645 (Supplementary Figure S1) (Gundling et al., 2018).
Individuals residing at high-altitude were more likely to have the A allele (Frequency of A = 0.59) and those residing at low-altitude were more likely to have the G allele (Frequency of G = 0.58, Table 1). The allele frequencies varied depending on ancestry as well. Europeans residing at high-altitude had higher A allele frequency than Europeans residing at low-altitude and a greater number of individuals that were homozygous for the A allele (Table 1). Those of primarily European descent had a negative correlation between the A allele frequency and Native American admixture, previously estimated in Zamudio et al. (2007). Europeans residing at high-altitude had 26% indigenous Bolivian admixture and an A allele frequency of 0.64 while low-altitude Europeans had greater indigenous admixture (33%) and the frequency of the A allele was 0.38 (Table 1). Among Andeans there was a less drastic change between high and low-altitude Andeans with A allele frequences of 0.54 and 0.46 respectively. There was also less admixture among the Andean populations compared to the European populations. The Andeans residing at high-altitude had ∼3% European admixture and those Andeans residing at low-altitude had ∼5% European admixture.
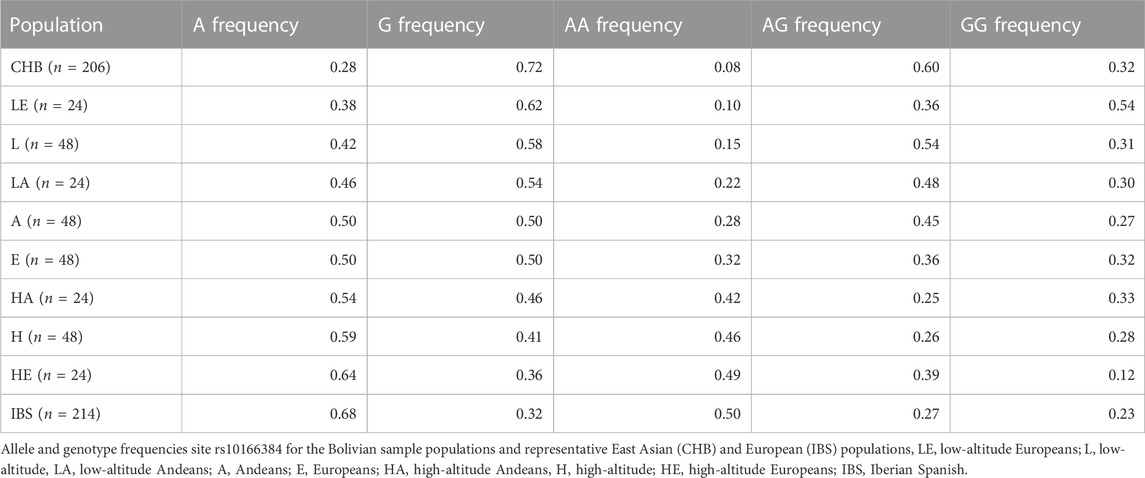
TABLE 1. Allele frequency and genotype proportions for site rs10166384 among the Bolivian samples as well as East Asian and European populations from the 1,000 genomes project.
We tested to see if natural selection induced by the high-altitude environment was contributing to the change in allele frequency at the location of SNP rs10166384, which is associated with the shift in DNA methylation. Hardy-Weinberg equilibrium was present in both Andeans and Europeans residing at low-altitude. Andeans residing at high-altitude were not in Hardy-Weinberg equilibrium (χ2 = 5.916, p = 0.015), indicating the population is being influenced by selection pressure with respect to these alleles. High-altitude Andeans had fewer heterozygotes than a population in accordance with Hardy-Weinberg equilibrium (Table 1). For high-altitude Europeans being out of Hardy Weinberg equilibrium was of borderline significance (χ2 = 3.711, p = 0.054). European high-altitude residents, like Andeans had fewer heterozygotes than would be expected for a population in Hardy-Weinberg equilibrium.
We then tested whether the change in genotype proportions was influenced by directional selection, balancing selection, or random genetic drift by calculating Tajima’s on a 2.5 kb region within the third intron of DYSF containing six polymorphisms with minor allele frequencies > 0.05 (Tajima, 1989). We found that the Andeans, regardless of altitude, had the highest estimated Tajima’s D (Tajima’s D = 2.35) suggesting balancing selection on roughly equal frequencies of each allele. While lower than in Andeans, Europeans, regardless of altitude, also had a Tajima’s D suggesting balancing selection (Tajima’s D = 1.86).
Discussion
Nucleotide adaptation in dysferlin
The dysferlin single nucleotide variant rs10166384 (G/A) is located directly downstream of a cytosine causing a CpG site to potentially become a CpA site. This variation reduces the likelihood of DNA methylation (McClay et al., 2015). rs10166384 is located within a TEAD4 transcription factor binding site as seen using the UCSC genomes browser, involved in the formation of syncytial tissues This included the syncytiotrophoblast, the outer layer of the placental villi (Figure 1) (Benhaddou et al., 2012) facing the maternal intervillous blood that provides the oxygen and nutrients required by the placenta and fetus. Increased DNA methylation along the TEAD4 transcription factor binding sites limits binding of the TEAD4 transcription factor, resulting in decreased DYSF gene expression (Gornikiewicz et al., 2016) Recent studies indicate DYSF expression is driven by DNA methylation in other cell types as well (Zhang et al., 2022). We would therefore expect that DYSF AA homozygotes would have more, GG homozygotes less, and AG heterozygotes intermediate levels of dysferlin protein in the syncytiotrophoblast.
There are only 3 true syncytial tissues in humans: muscle fibers, osteoclasts or giant cells and the placental syncytiotrophoblast (the latter is shown in Figure 1) (Benhaddou et al., 2012). It is unsurprising, given dysferlin’s role in the muscle sarcolemma, that numerous pathogenic mutations in the dysferlin gene are linked to muscle wasting diseases known collectively as dysferlinopathies and attributable to failure of muscle repair mechanisms (Charnay et al., 2021). In the human placenta, dysferlin localizes strictly to the syncytiotrophoblast (Robinson et al., 2009) DYSF plays a key role in syncytiotrophoblast fusion with the underlying mononuclear cytotrophoblast (Omata et al., 2013) (Robinson et al., 2009). Cytotrophoblasts are progressively incorporated into the syncytium via fusion over the course of pregnancy. This critically important process allows the syncytium to expand as the placenta grows. From this perspective an increase in dysferlin at high-altitude might be beneficial. This is supported by placental morphological studies indicating reduced perivillous fibrin deposition is a prominent difference between high- and low-altitude placentae (Zamudio, 2003) Perivillous fibrin deposition is related to syncytial damage and breaks, occasionally pathological, and associated with the placental hypoxia seen in preeclampsia IUGR (Lang et al., 2009). Additional support for the idea that elevated DYSF would be beneficial at high-altitude derives from the well-supported evidence that hypoxia, including that due to high-altitude, causes ballooning of the tertiary villi, expanding and thinning the syncytium due to capillary growth, reducing syncytiotrophoblast thickness and permitting greater oxygen diffusion (Burton et al., 1996; Mayhew, 2003). Nonetheless, other studies suggest altitude decreases trophoblast growth (Mayhew et al., 2002), while molecular studies indicate the mechanisms regulating trophoblast turnover into the syncytium are decreased (Soleymanlou et al., 2007).
The population sub-group with the highest A allele frequencies are the high-altitude Europeans (A allele frequency = 0.64). The higher A allele frequency corresponds with a decrease in methylation compared to low-altitude Europeans (ΔM = −2.62). Lower DNA methylation may contribute to the higher DYSF gene expression within the high-altitude European population compared to the low-altitude European population. The A allele is also more abundant among those individuals residing in Spain who were genotyped as part of the 1,000 genomes project, suggesting that the A allele is the ancestral European allele. The high frequency of A alleles among Europeans residing at high-altitude, which differed significantly from that of low-altitude Europeans (0.64 at 3,600 m vs. 0.38 at 400 m) could be related to the fact that we only examined individuals with healthy pregnancies. While no single factor can be said to influence placental health, the large difference in gene frequency suggests the A allele may be under greater selection pressure among migrants than natives to high-altitude because it may be linked to more effective DYSF transcription and therefore better cytotrophoblast fusion and syncytiotrophoblast repair. We predict that if we were to genotype Europeans residing at high-altitude who experienced problematic pregnancies, we would see increased G allele frequencies.
We compared allele frequencies of our sample populations to those of East Asian, European, and South American descent from the 1000 genomes project (1000 Genomes Project Consortium et al., 2015). The allele frequencies of our Bolivian samples lie in between those of the East Asian and European 1000 genomes populations (Figure 3). We chose to look at the east Asian populations because, while the founding population for North and South American paleo-Indian migrations is lost, they likely contain the descendants of the founding population for Native Americans, which crossed over the Bering Land Bridge roughly 15,000–17,000 years ago (Perego et al., 2010). The East Asian participants that were part of the 1,000 genomes project had G as the major allele for SNP rs10166384 with an average G allele frequency of 0.74, whilst Andeans had frequencies of 0.46 and 0.54 at low and high-altitude respectively. Apart from the East Asians, the European samples from the 1,000 genomes had the A as the major allele at this site with an A allele frequency of 0.68, close to that of high-altitude Europeans. The fact that both the Andeans and Europeans residing in Bolivia (regardless of altitude) had roughly equal frequencies of both the A and G allele suggests that there has been East Asian and European admixture resulting in the Andeans gaining more of the European A allele and the Europeans gaining more of the East Asian G allele (Figure 3). This implies that the more generations Europeans have resided at high-altitude the greater the chance that they will inherit native admixture. This would result in an increase in the frequency of G allele causing them to become significantly out of Hardy-Weinberg equilibrium.
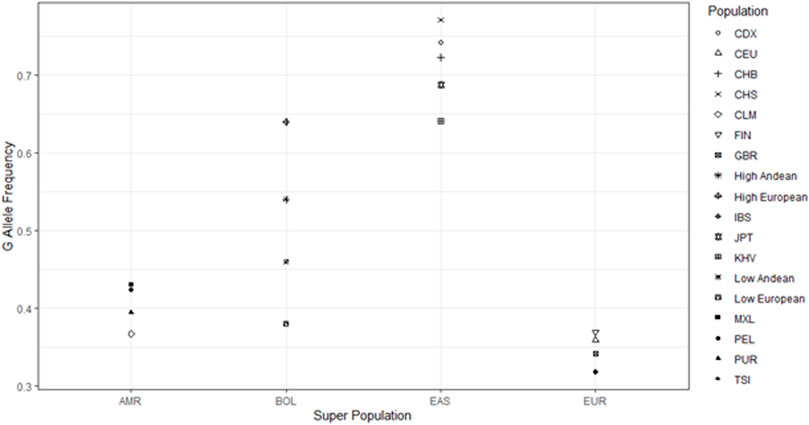
FIGURE 3. Comparisons of Andean allele frequency to 1,000 genomes data. Figure shows the comparison of the G allele frequency among our Andean sample populations in relation to the 1,000 genomes populations. AMR = Americans from the 1,000 genomes project, BOL = Samples from Bolivia residing at both high and low-altitude, EAS = East Asians from the 1,000 genomes project, EUR = Europeans from the 1,000 genomes project. The Populations include groups from the 1,000 genomes project (CDX = Chinese Dai, CEU = European from Utah, United States, CHB = Han Chinese from Beijing, CHS = Han Chinese from South China, CLM = Colombian, FIN = Finnish, GBR = British, IBS = Ibererian from Spain, JPT = Japanese, KHV = Vietnamese, MXL = Mexican from Los Angeles, PEL = Peruvian, PUR = Puerto Rican, TSI = Italian). We also included our 4 sample populations from Bolivia (High Andean = High altitude Andean, High European = High altitude European, Low Andean = Low altitude Andean, Low European = Low altitude European).
We found that the Native Americans residing at high-altitude had genotype proportions that were significantly out of Hardy-Weinberg equilibrium (p = 0.015). This suggests that over the millennia the Andeans have resided at high-altitude the selection pressure of the environment has caused a shift in genotype proportions. This shift, exemplified by rs10166384 is an example of how evolution may favor DNA methylation to alter not just DYSF, but other gene expression as populations shifts between high and low-altitude environments (Gundling et al., 2018). Likewise, Europeans residing at high-altitude are of borderline significance for being out of Hardy-Weinberg equilibrium (p = 0.054). This indicates that the selection pressure of high-altitude may also be resulting in sequence level change at site rs10166384. However, the Europeans have not resided at high-altitude for enough generations to cause the population to be significantly out of Hardy-Weinberg equilibrium. We speculate that after more generations at high-altitude, or even with a larger sample size of European ancestry high-altitude residents rs10166384 will be out of Hardy-Weinberg equilibrium.
That our Bolivian Andean and European populations regardless of altitude had A and G allele frequencies of ∼0.5 supports that balancing selection is acting on and around variant rs10166384. This is supported by the positive Tajima’s D value of 2.37 and 1.85 across Andean and European populations respectively. A significantly positive Tajima’s D (D > 1.80) indicates balancing selection suggesting that both the A and G allele maybe beneficial in the high-altitude environment (Tajima, 1989). Those possessing a G allele are more likely to be methylated limiting the binding of the TEAD4 transcription factor resulting in decreased DYSF gene expression. This is supported by the findings in our prior study where were found increased gene expression of DYSF at high altitude suggesting that the increase frequency of the A allele at high-altitude is increasing gene expression by limiting DNA methylation (Gundling et al., 2018). Methylation at this site may allow for more transient regulation of dysferlin allowing for a smoother transition between high and low-altitude. Those that have the A allele may be regulating gene expression by limiting the ability of this CpG site to be methylated potentially resulting in higher gene expression. The intermediate allele frequencies suggest that balancing selection is acting in a frequency dependent manner rather than through heterozygous advantage (Brandt et al., 2018). While genotypes cannot be induced, methylation status of particular genotypes may potentially be inducible based on environmental conditions. The preservation of both the A and G alleles among native Andeans regardless of altitude suggests that both the ability to be methylated and consequent impacts on transcription factor binding may aid the Andeans in attaining greater fetal growth and better placental health regardless of the altitude they reside in at the time.
One of the major limitations of our study is the small sample size for our Bolivian population, although we did increase our sample size from 45 to 96 samples to help combat this issue. Increasing our sample size further may help increase our statistical power to detect meaningful differences among groups. Another limitation is that we focused only on one site within one gene to test for balancing selection. Future studies should involve increased number of sites associated with altitude dependent differentially expressed genes to detect whether selection occurred at sites impacted by DNA methylation.
Conclusion
We identified an environmentally challenged population (high-altitude) in which ancestry dependent natural selection may induce altered DNA methylation status, thereby benefitting placental function. In the gene dysferlin, we found a variant (rs10166384) located within a transcription factor-binding site that can alter dysferlin gene expression. Those individuals with the A allele who reside at high-altitude are likely to have decreased DNA methylation and higher dysferlin gene expression. The higher dysferlin gene expression is likely to contribute to more effective syncytiotrophoblast turnover and repair in response to the high-altitude environment, which may lead to more successful pregnancies at high-altitude. Those with the G allele are more likely to have DNA methylation along the transcription factor-binding site and decreased gene expression. This may be advantageous among those of Andean ancestry because it may allow them a way to easily modify gene expression of those genes regulated by the TEAD4 transcription factor as they move between high and low-altitude. As native Andeans move to high-altitude the DNA methylation may be removed increasing dysterlin gene expression and allowing for increased membrane repair compared to low-altitude.
Data availability statement
The datasets presented in this study can be found in online repositories. The names of the repository/repositories and accession number(s) can be found below: https://www.ncbi.nlm.nih.gov/geo/, GSE100988 data released by NIH on publication.
Ethics statement
All women gave written informed consent to protocols approved by the Bolivian National Bioethics Committee, the Universidad Mayor de San Andrés, Instituto Boliviano de Biología de Altura Consejo Tecnico, and the USA Institutional IRB from the University of Medicine and Dentistry, New Jersey Medical School in Newark, NJ (now Rutgers).
Author contributions
WG, NI, DW, and SZ designed the research. WG, NI, DW, and SZ performed the research. LE, NI, and SZ provided clinical information. WG, NI, SP, DW, and SZ wrote the manuscript.
Funding
The parent project from which these samples derived was supported by the National Science Foundation BCS 0309142, Fogarty International Research Collaboration Award R03 TW007444 and the National Institutes of Health R01 HD42737 (SZ and NI). The genomic research was supported by National Institutes of Health R21 HD068954 (SZ and DW).
Acknowledgments
The Authors acknowledge L Raetzman, M. Bagchi, and K Clancy from the University of Illinois for their advice and guidance while preparing the manuscript. The staff at the Roy J. Carver Biotechnology Center is acknowledged for performing the DNA sequencing. We thank Claudio Barbeito and Maria Miglino for organizing the research topic of Cellular and Processes in Placental Morphogenesis and inviting us to submit this manuscript. We thank C Valero for her technical assistance and informative comments on early drafts. We are indebted to the scientists and medical colleagues in La Paz and Santa Cruz Bolivia, at the Instituto Boliviano de Biología de Altura, Universidad Mayor de Andrés, and Centro Nacionál de Enfermedades Tropicales (CENETROP) who assisted in every way possible. Finally, we are grateful to our participating Bolivian mothers and their babies, who so generously gave of their time to support the research.
Conflict of interest
Authors NI and SZ are consultants for Placental Research Group LLC.
The remaining authors declare that the research was conducted in the absence of any commercial or financial relationships that could be construed as a potential conflict of interest.
Publisher’s note
All claims expressed in this article are solely those of the authors and do not necessarily represent those of their affiliated organizations, or those of the publisher, the editors and the reviewers. Any product that may be evaluated in this article, or claim that may be made by its manufacturer, is not guaranteed or endorsed by the publisher.
Supplementary material
The Supplementary Material for this article can be found online at: https://www.frontiersin.org/articles/10.3389/fcell.2023.1125972/full#supplementary-material
SUPPLEMENTARY FIGURE S1 | Correlation of 450 k methylation with pyrosequencing. Correlation of beta values generated using the Illumina 450 k methylation microarray (x-axis) and percent methylation calculated from the pyrosequencing analysis. The gray band surrounding the blue regression line represents the 95% confidence interval. We see that those samples with the AA genotype demonstrated minimal methylation (i.e., Beta values close to 0%) when analyzed through methylation array as well as pyrosequencing. Those with the heterozygous genotype (GA) had roughly (beta values representing 50% percent methylation). Lastly those that were homozygous for the G allele had the highest methylation values with Beta values indicating approximately 70% methylation.
References
1000 Genomes Project Consortium Auton, A., Brooks, L. D., Durbin, R. M., Garrison, E. P., Kang, H. M., et al. (2015). A global reference for human genetic variation. Nature 526, 68–74. doi:10.1038/nature15393
Allison, A. C. (1954). Protection afforded by sickle-cell trait against subtertian malareal infection. Br. Med. J. 1, 290–294. doi:10.1136/bmj.1.4857.290
Amos, W., and Hoffman, J. I. (2010). Evidence that two main bottleneck events shaped modern human genetic diversity. Proc. Biol. Sci. 277, 131–137. doi:10.1098/rspb.2009.1473
Barreiro, L. B., Laval, G., Quach, H., Patin, E., and Quintana-Murci, L. (2008). Natural selection has driven population differentiation in modern humans. Nat. Genet. 40, 340–345. doi:10.1038/ng.78
Beall, C. M., Cavalleri, G. L., Deng, L., Elston, R. C., Gao, Y., Knight, J., et al. (2010). Natural selection on EPAS1 (HIF2alpha) associated with low hemoglobin concentration in Tibetan highlanders. Proc. Natl. Acad. Sci. U. S. A. 107, 11459–11464. doi:10.1073/pnas.1002443107
Benhaddou, A., Keime, C., Ye, T., Morlon, A., Michel, I., Jost, B., et al. (2012). Transcription factor TEAD4 regulates expression of myogenin and the unfolded protein response genes during C2C12 cell differentiation. Cell Death Differ. 19, 220–231. doi:10.1038/cdd.2011.87
Bigham, A. W., Wilson, M. J., Julian, C. G., Kiyamu, M., Vargas, E., Leon-Velarde, F., et al. (2013). Andean and Tibetan patterns of adaptation to high altitude. Am. J. Hum. Biol. 25, 190–197. doi:10.1002/ajhb.22358
Brandt, D. Y. C., Cesar, J., Goudet, J., and Meyer, D. (2018). The effect of balancing selection on population differentiation: A study with hla genes. G3 (Bethesda) 8, 2805–2815. doi:10.1534/g3.118.200367
Burton, G. J., Reshetnikova, O. S., Milovanov, A. P., and Teleshova, O. V. (1996). Stereological evaluation of vascular adaptations in human placental villi to differing forms of hypoxic stress. Placenta 17, 49–55. doi:10.1016/s0143-4004(05)80643-5
Charnay, T., Blanck, V., Cerino, M., Bartoli, M., Riccardi, F., Bonello-Palot, N., et al. (2021). Retrospective analysis and reclassification of DYSF variants in a large French series of dysferlinopathy patients. Genet. Med. 23, 1574–1577. doi:10.1038/s41436-021-01164-3
Codding, S. J., Marty, N., Abdullah, N., and Johnson, C. P. (2016). Dysferlin binds SNAREs (soluble N-Ethylmaleimide-sensitive factor (NSF) attachment protein receptors) and stimulates membrane fusion in a calcium-sensitive manner. J. Biol. Chem. 291, 14575–14584. doi:10.1074/jbc.M116.727016
Danecek, P., Auton, A., Abecasis, G., Albers, C. A., Banks, E., Depristo, M. A., et al. (2011). The variant call format and VCFtools. Bioinformatics 27, 2156–2158. doi:10.1093/bioinformatics/btr330
De Luna, N., Gallardo, E., and Illa, I. (2004). In vivo and in vitro dysferlin expression in human muscle satellite cells. J. Neuropathol. Exp. Neurol. 63, 1104–1113. doi:10.1093/jnen/63.10.1104
Dobzhansky, T., and Dobzhansky, T. G. (1970). Genetics of the evolutionary process. Columbia University Press.
Du, P., Zhang, X., Huang, C. C., Jafari, N., Kibbe, W. A., Hou, L., et al. (2010). Comparison of Beta-value and M-value methods for quantifying methylation levels by microarray analysis. BMC Bioinforma. 11, 587. doi:10.1186/1471-2105-11-587
Gornikiewicz, B., Ronowicz, A., Krzeminski, M., and Sachadyn, P. (2016). Changes in gene methylation patterns in neonatal murine hearts: Implications for the regenerative potential. BMC Genomics 17, 231. doi:10.1186/s12864-016-2545-1
Gundling, W. E., Pantham, P., Illsley, N. P., Echalar, L., Zamudio, S., and Wildman, D. E. (2018). DNA methylation explains a subset of placental gene expression differences based on ancestry and altitude. bioRxiv.
Han, R., and Campbell, K. P. (2007). Dysferlin and muscle membrane repair. Curr. Opin. Cell Biol. 19, 409–416. doi:10.1016/j.ceb.2007.07.001
Hedrick, P. W. (2007). Balancing selection. Curr. Biol. 17, R230–R231. doi:10.1016/j.cub.2007.01.012
Hoff, C., Thorneycroft, I., Wilson, F., and Williams-Murphy, M. (2001). Protection afforded by sickle-cell trait (hb AS): What happens when malarial selection pressures are alleviated? Hum. Biol. 73, 583–586. doi:10.1353/hub.2001.0053
Lang, C. T., Markham, K. B., Behrendt, N. J., Suarez, A. A., Samuels, P., Vandre, D. D., et al. (2009). Placental dysferlin expression is reduced in severe preeclampsia. Placenta 30, 711–718. doi:10.1016/j.placenta.2009.05.008
Mayhew, T., Bowles, C., and Yücel, F. (2002). Hypobaric hypoxia and villous trophoblast: Evidence that human pregnancy at high altitude (3600 m) perturbs epithelial turnover and coagulation-fibrinolysis in the intervillous space. Placenta 23, 154–162. doi:10.1053/plac.2001.0775
Mayhew, T. M. (2003). Changes in fetal capillaries during preplacental hypoxia: Growth, shape remodelling and villous capillarization in placentae from high-altitude pregnancies. Placenta 24, 191–198. doi:10.1053/plac.2002.0895
Mcclay, J. L., Shabalin, A. A., Dozmorov, M. G., Adkins, D. E., Kumar, G., Nerella, S., et al. (2015). High density methylation QTL analysis in human blood via next-generation sequencing of the methylated genomic DNA fraction. Genome Biol. 16, 291. doi:10.1186/s13059-015-0842-7
Omata, W., Ackerman, W. E. T., Vandre, D. D., and Robinson, J. M. (2013). Trophoblast cell fusion and differentiation are mediated by both the protein kinase C and a pathways. PLoS One 8, e81003. doi:10.1371/journal.pone.0081003
Page, A. J., Taylor, B., Delaney, A. J., Soares, J., Seemann, T., Keane, J. A., et al. (2016). SNP-Sites: Rapid efficient extraction of SNPs from multi-FASTA alignments. Microb. Genom 2, e000056. doi:10.1099/mgen.0.000056
Patil, V., Ward, R. L., and Hesson, L. B. (2014). The evidence for functional non-CpG methylation in mammalian cells. Epigenetics 9, 823–828. doi:10.4161/epi.28741
Perego, U. A., Angerhofer, N., Pala, M., Olivieri, A., Lancioni, H., Hooshiar Kashani, B., et al. (2010). The initial peopling of the americas: A growing number of founding mitochondrial genomes from beringia. Genome Res. 20, 1174–1179. doi:10.1101/gr.109231.110
Robinson, J. M., Ackerman, W. E. T., Behrendt, N. J., and Vandre, D. D. (2009). While dysferlin and myoferlin are coexpressed in the human placenta, only dysferlin expression is responsive to trophoblast fusion in model systems. Biol. Reprod. 81, 33–39. doi:10.1095/biolreprod.108.074591
Smith, J. M., and Haigh, J. (1974). The hitch-hiking effect of a favourable gene. Genet. Res. 23, 23–35. doi:10.1017/s0016672300014634
Soleymanlou, N., Jurisicova, A., Wu, Y., Chijiiwa, M., Ray, J. E., Detmar, J., et al. (2007). Hypoxic switch in mitochondrial myeloid cell leukemia factor-1/Mtd apoptotic rheostat contributes to human trophoblast cell death in preeclampsia. Am. J. Pathol. 171, 496–506. doi:10.2353/ajpath.2007.070094
Tajima, F. (1989). Statistical method for testing the neutral mutation hypothesis by DNA polymorphism. Genetics 123, 585–595. doi:10.1093/genetics/123.3.585
Tamura, K., Stecher, G., Peterson, D., Filipski, A., and Kumar, S. (2013). MEGA6: Molecular evolutionary genetics analysis version 6.0. Mol. Biol. Evol. 30, 2725–2729. doi:10.1093/molbev/mst197
Thompson, J. D., Higgins, D. G., and Gibson, T. J. (1994). CLUSTAL W: Improving the sensitivity of progressive multiple sequence alignment through sequence weighting, position-specific gap penalties and weight matrix choice. Nucleic Acids Res. 22, 4673–4680. doi:10.1093/nar/22.22.4673
Tishkoff, S. A., Reed, F. A., Ranciaro, A., Voight, B. F., Babbitt, C. C., Silverman, J. S., et al. (2007). Convergent adaptation of human lactase persistence in Africa and Europe. Nat. Genet. 39, 31–40. doi:10.1038/ng1946
Tournebize, R., Chu, G., and Moorjani, P. (2022). Reconstructing the history of founder events using genome-wide patterns of allele sharing across individuals. PLoS Genet. 18, e1010243. doi:10.1371/journal.pgen.1010243
Uddin, M., Aiello, A. E., Wildman, D. E., Koenen, K. C., Pawelec, G., De Los Santos, R., et al. (2010). Epigenetic and immune function profiles associated with posttraumatic stress disorder. Proc. Natl. Acad. Sci. U. S. A. 107, 9470–9475. doi:10.1073/pnas.0910794107
Vandre, D. D., Ackerman, W. E. T., Kniss, D. A., Tewari, A. K., Mori, M., Takizawa, T., et al. (2007). Dysferlin is expressed in human placenta but does not associate with caveolin. Biol. Reprod. 77, 533–542. doi:10.1095/biolreprod.107.062190
Zamudio, S., Postigo, L., Illsley, N. P., Rodriguez, C., Heredia, G., Brimacombe, M., et al. (2007). Maternal oxygen delivery is not related to altitude- and ancestry-associated differences in human fetal growth. J. Physiol. 582, 883–895. doi:10.1113/jphysiol.2007.130708
Zamudio, S. (2003). The placenta at high altitude. High. Alt. Med. Biol. 4, 171–191. doi:10.1089/152702903322022785
Keywords: placenta, cell fusion, dysferlin, methylation, genomics
Citation: Gundling WE Jr, Post S, Illsley NP, Echalar L, Zamudio S and Wildman DE (2023) Ancestry dependent balancing selection of placental dysferlin at high-altitude. Front. Cell Dev. Biol. 11:1125972. doi: 10.3389/fcell.2023.1125972
Received: 16 December 2022; Accepted: 06 March 2023;
Published: 21 March 2023.
Edited by:
Claudio Gustavo Barbeito, National University of La Plata, ArgentinaReviewed by:
Nandor Gabor Than, Hungarian Academy of Sciences (MTA), HungaryPhelipe Favaron, State University of Londrina, Brazil
Copyright © 2023 Gundling, Post, Illsley, Echalar, Zamudio and Wildman. This is an open-access article distributed under the terms of the Creative Commons Attribution License (CC BY). The use, distribution or reproduction in other forums is permitted, provided the original author(s) and the copyright owner(s) are credited and that the original publication in this journal is cited, in accordance with accepted academic practice. No use, distribution or reproduction is permitted which does not comply with these terms.
*Correspondence: Derek E. Wildman, ZHdpbGRtYW5AdXNmLmVkdQ==; William E. Gundling, d2d1bmRsaW5AY2J1LmVkdQ==