- 1Department of Gynecology, Xiangya Hospital, Central South University, Changsha, China
- 2Department of Pharmacy, Xiangya Hospital, Central South University, Changsha, China
- 3National Clinical Research Center for Geriatric Disorders, Xiangya Hospital, Central South University, Changsha, China
- 4Department of Pathology, Xiangya Hospital, Central South University, Changsha, China
- 5Department of Orthopedic Surgery, The Second Hospital University of South China, Hengyang, China
Ferroptosis is a novel type of cell death associated with iron accumulation and excessive lipid peroxidation. Elucidating the underlying molecular mechanisms of ferroptosis is intensively related to the development and treatment of multiple diseases, including musculoskeletal disorders. Moreover, in vitro and in vivo studies have shown the importance of oxidative stress in musculoskeletal conditions such as osteoporosis, osteoarthritis, rheumatoid arthritis, and osteosarcoma. Ferroptosis-derived clinical management of musculoskeletal diseases offers tremendous and attractive opportunities. Notably, ferroptosis agonists have been proven to enhance the sensitivity of osteosarcoma cells to conventional therapeutic strategies. In this review, we have mainly focused on the implications of ferroptosis regulation in the pathophysiology and therapeutic response of musculoskeletal disorders. Understanding roles of ferroptosis for controlling musculoskeletal diseases might provide directions for ferroptosis-driven therapies, which could be promising for the development of novel therapeutic strategies.
1 Introduction
Ferroptosis is a regulated cell death induced by iron-correlated lipid peroxidation. The crucial impetus of ferroptosis is the phospholipids peroxidation and formation of cellular membranes (Hadian and Stockwell, 2020). Ferroptosis induction through oxidative perturbations of the intracellular microenvironment controlled by glutathione (GSH) peroxidase 4 (GPX4) has been reported, essentially triggered by Fe2+ accumulation and lipid peroxidation (Su et al., 2020).
Numerous studies have demonstrated the importance of ferroptosis in human diseases, such as nervous system disorders, cardiovascular diseases, and cancers (Wei et al., 2020). Additionally, emerging evidence has supported the notion that ferroptosis and musculoskeletal diseases are closely linked (Zhang et al., 2022a); however, the underlying mechanisms require further investigation. Accordingly, ferroptosis and other types of programmed cell death have been studied extensively for their involvement in musculoskeletal diseases (Hwang and Kim, 2015; Spel and Martinon, 2020; Zhao et al., 2021a).
In this review, we have summarized and discussed the profiles and clinical implications of ferroptosis related to musculoskeletal disorders, which are currently under intensive investigation (Figure 1). These discoveries could provide a more detailed understanding of musculoskeletal diseases to determine potential therapeutic strategies.
2 Regulatory mechanisms of ferroptosis
Ferroptosis can be distinguished from other programmed cell death modes having characteristics of mitochondrial damage, such as mitochondrial swelling, decreased cristae, and an increase in autophagosomes (Friedmann Angeli et al., 2014). Because of the uncontrollable production of lipid peroxides, polyunsaturated fatty acid (PUFA) hydroperoxides accumulate within the phospholipid membranes, providing the fundamental lipid component necessary for ferroptotic cell death that leads to enhanced membrane permeability and cell swelling (Yang et al., 2016; Agmon et al., 2018). Additionally, in ferroptotic cells, the antioxidant defense systems, Xc-, ferroptosis suppressor protein 1 (FSP1), GTP cyclohydrolase 1 (GCH1), and other pathways, are critically responsible for the regulation of lipid peroxidation and ferroptosis (Figure 2). Intriguingly, ferroptosis-associated cell death may spread to neighboring cells in waves, further triggering their death (Riegman et al., 2020). Therefore, safeguarding these cells and rebuilding the cell membrane lipids is crucial before undergoing cellular ferroptosis (Doll et al., 2017).
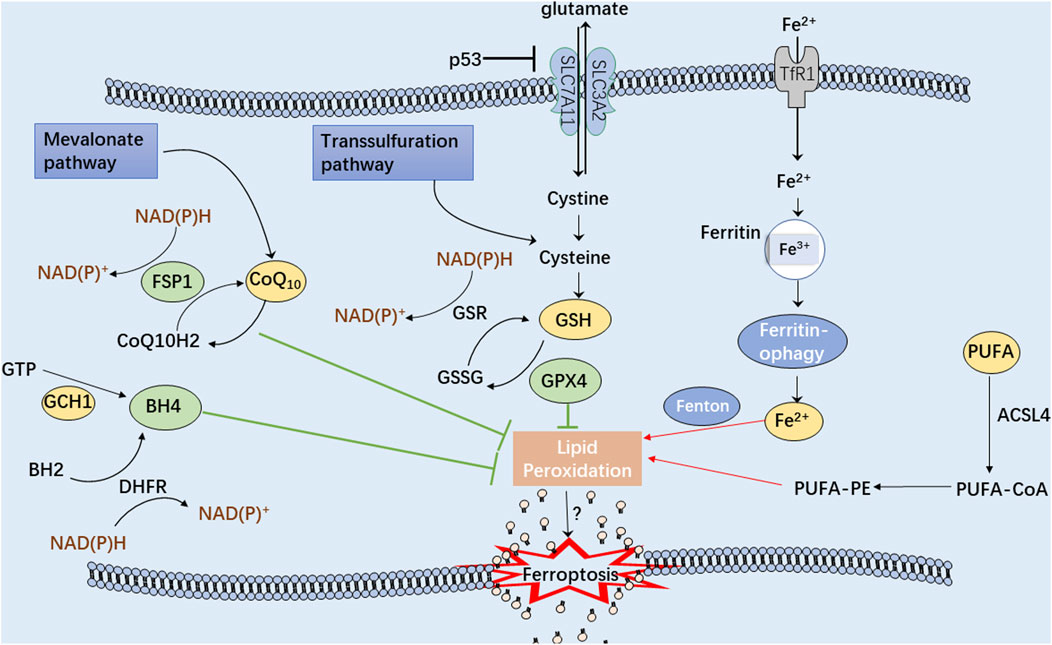
FIGURE 2. A schematic illustration showing the main mechanisms of the ferroptosis process. The green arrows represent three antioxidant systems: Xc-GSH-GPX4 pathway, FSP1-CoQ10-NAD(P)H pathway, and GCH1-BH4 pathway. Iron metabolism disturbance and polyunsaturated fatty acids (PUFA) peroxidation, marked with red arrows, significantly contribute to cellular ferroptosis.
2.1 Iron metabolism
Iron metabolism homeostasis may influence cell sensitivity to ferroptosis (Yu et al., 2020; Zheng and Conrad, 2020) because iron could produce large amounts of reactive oxygen species (ROS) through the Fenton reaction, thus, exacerbating oxidative damage to cells (Yoshida et al., 2019). Therefore, controlling iron transport and storage is critical to regulating ferroptosis (Chen et al., 2020). Hypoxia-inducible factor (HIF), generated under hypoxic conditions, has been reported to regulate the functioning of iron-transferring proteins, including transferrin receptor (TfR), divalent metal transporter 1 (DMT1) and iron transport protein (FPN) (Tacchini et al., 1999; Taylor et al., 2011; Xu et al., 2017; Yang et al., 2018). Moreover, ferritinophagy mediated by nuclear receptor coactivator 4 (NCOA4) has been implicated in maintaining iron homeostasis through converting ferritin into intracellular iron (Santana-Codina and Mancias, 2018; Fuhrmann et al., 2020; Santana-Codina et al., 2021). Iron chelators, including deferoxamine (DFO) and ciclopirox, have been demonstrated to prevent ferroptosis by forbidding the production of oxidized lipid species (Stockwell et al., 2017). Recent studies have suggested the functional roles of aberrant iron overload or iron deficiency on the osteoblast ferroptosis, which might contribute to the occurrence of osteoporosis (Toxqui and Vaquero, 2015; Jiang et al., 2022; Xu et al., 2022). It has been observed that intracellular iron overload caused by ferrous ammonium citrate (FAC) could improve ferroptosis sensitivity by downregulating the expression of Wnt target genes, Lef1, Bmp4, Smad6, and Cyclin D1, consequently blocking the development of mesenchymal stem cells into osteoblasts (Luo et al., 2022). Moreover, iron overload reduces the activity of end-plate chondrocytes and knee chondrocytes, hence participating in intervertebral disc degeneration and osteoarthritis (OA) (Cai et al., 2021; Chen et al., 2022a). Wang’s group constructed a mouse model of iron overload and found that iron overload could induce cartilage calcification in a dose-dependent manner (Wang et al., 2022a). Previous research has demonstrated that DMT1 plays a pivotal role in the prevention of iron overload and ferroptosis in chondrocytes in OA (Jing et al., 2020). In the OA mouse model induced by ferroptosis activator erastin, the iron overload in chondrocytes could facilitate the development of arthritis by downregulating type II collagen and upregulating matrix metalloproteinase 1333. Besides, distinct from normal cells, osteosarcoma cells display an exuberant iron demand due to their malignant proliferative characteristic and high cellular iron concentration (Xue et al., 2021). The salazosulfapyridine-induced ferroptosis in K7M2 osteosarcoma cells could be significantly reversed after depletion of iron (Liu et al., 2022a). Therefore, targeting iron metabolism could be served as an appealing strategy for regulating the cell death of osteosarcoma.
2.2 Lipid peroxidation
Uncontrolled lipid peroxides production due to oxidative stress generally causes mitochondrial lipid peroxidation and damage, resulting in ferroptosis. Based on the differences in hydrocarbon chain saturation, fatty acids can be divided into three categories: saturated fatty acids (SFAs), monounsaturated fatty acids (MUFAs), and PUFAs (Tang et al., 2021a). The density of PUFAs significantly impacts the degree of lipid peroxidation, thereby determining the sensitivity of ferroptosis (Stockwell et al., 2017). The free PUFAs, including arachidonic acid (AA) and adrenic acid (ADA), are catalyzed by acyl-CoA synthetase long-chain family member 4 (ACSL4) to produce AA/AdA-CoA derivatives. Next, AA/AdA-CoA and membrane PE are catalyzed by lysophosphatidylcholine acyltransferase 3 (LPCAT3) to obtain AA/AdA-PE (Doll et al., 2017; Kagan et al., 2017; Zou et al., 2019; Kagan et al., 2020). Upon the overactivation of lipid peroxide synthesis, PUFA depletion can alter the establishment and operation of lipid membranes (Hassannia et al., 2019). Thus, ACSL4 and LPCAT3 have been identified as crucial targets for ferroptosis regulation due to their involvement in PUFA catalysis. A recent study revealed that the natural flavonoid glycoside, baicalin, could suppress the expression of ACSL4 to prevent cell ferroptosis (Fan et al., 2021). Similarly, rosiglitazone, an ACSL4 inhibitor, has been reported to prevent acute kidney injury induced by ferroptosis (Wang et al., 2022b).
2.3 Xc-GSH-GPX4 pathway
In mammals, the cyst(e)ine-GSH-GPX4 signaling axis has been identified as the primary regulatory system for ferroptosis. ROS are the byproducts of aerobic metabolism, and unrestrained ROS production significantly contributes to cellular ferroptosis (Sun et al., 2018). GSH is an essential antioxidant tripeptide consisting of glutamic acid, cysteine, and glycine (Kuang et al., 2020). The system Xc− and transsulfuration pathways are the two main pathways that produce cysteine for GSH synthesis (Liu et al., 2020; Martis et al., 2020). System Xc− has the function of transporting cystine into cells and constitutes the subunit solute carrier family 7 member 11 (SLC7A11) and solute carrier family 3 member 2 (SLC3A2) (Lewerenz et al., 2013). After cellular entry, cystine is oxidized to cysteine, which is utilized in GSH synthesis (Ursini and Maiorino, 2020). Besides, the transsulfuration pathway is related to the reciprocal conversion of cysteine to homocysteine (McBean, 2012). GPX4 (a selenium-containing protein) plays a crucial role in the inhibitory function of GSH on cellular ferroptosis by reducing the phospholipid hydroperoxide production (AA/AdA-PE-OOH) to the corresponding phosphatidyl alcohol (PLOH) (Ingold et al., 2018; Zhang et al., 2021). Another molecule, erastin (a classical inducer of ferroptosis), can inhibit extracellular cystine from entering cells by blocking the system Xc−, thus, lowering intracellular GSH expression and inducing ferroptosis (Zhao et al., 2020). Further research has revealed more compounds that participate in the regulation of the system Xc−pathway, such as RSL3, FIN56 and FINO2 (Yang et al., 2014; Gaschler et al., 2018; Wen et al., 2019; Yu et al., 2021).
2.4 FSP1-CoQ10-nad(P)H pathway
Ferroptosis and phospholipid peroxidation are also controlled by FSP1/ubiquinone (CoQ10)/NAD(P)H pathway, which is a GPX4-independent non-mitochondrial coenzyme Q10 (CoQ10)-based antioxidant system. FSP1 can catalyze the production of ubiquinol, the reduced form of CoQ10, at the cell membrane via NAD(P)H and subsequently traps lipid peroxides to inhibit ferroptosis (Doll et al., 2019). Interestingly, another study reported that CoQ10 could also suppress ferroptosis via the endosomal sorting complex required for transport (ESCRT)-III membrane repair, which is a distinct mechanism independent of ubiquinol (Dai et al., 2020). In addition, UBIA prenyltransferase domain-containing protein 1 (UBIAD1) is an essential antioxidant enzyme involved in the biosynthesis of non-mitochondrial CoQ10. UBIAD1/CoQ10 inhibition has been proposed as a potential strategy for treating melanoma by promoting lipid peroxidation and ferroptotic cell death (Arslanbaeva et al., 2022).
2.5 GCH1-BH4 pathway
GCH1/tetrahydrobiopterin (BH4)/phospholipid signaling axis has been recently reported to function as a highly potent ferroptosis suppressor. Moreover, the GCH1-BH4 pathway has been demonstrated as an endogenous antioxidant pathway independent of GSH-GPX4 (Kraft et al., 2020). BH4 serves as a cofactor in redox metabolism and regulates the metabolism of monoamines and aromatic compounds (Vancassel et al., 2022; Vasquez-Vivar et al., 2022). Aberrantly expressed BH4 is capable of trapping oxidative free radicals and preventing the production of lipid peroxides. Furthermore, dihydrofolate reductase (DHFR) can efficiently regenerate BH4 to defend against lipid peroxidation and ferroptosis (Soula et al., 2020). In addition, Hu et al. have delineated that genetic or pharmacological inhibition of GCH1 could improve the sensitivity of colorectal cancer cells to ferroptosis-inducing agents by downregulating BH4 metabolism (Hu et al., 2022a).
2.6 Other pathways regulating ferroptosis
Mounting evidence indicates the existence of other anti-lipid oxidation routes participating in the regulation of ferroptosis. For instance, an in vivo study suggests that iPLA2 inhibition may increase the susceptibility of cancer cells to p53-driven ferroptosis, which is independent of GPX4 (Chen et al., 2021a). In another report, Zou et al. identified P450 oxidoreductase (POR) as a significant enzyme contributing to lipid peroxidation and ferroptosis in cancer cells in response to distinct ferroptotic stress, using CRISPR-Cas9-mediated suppressor screens (Zou et al., 2020). Furthermore, energy stress led to the inactivation of AMP-activated protein kinase (AMPK) signaling that impaired the protective effects of energy stress on ferroptosis, contributing to ferroptosis-associated diseases (Lee et al., 2020). These findings collectively imply that clarifying the underlying molecular signals for ferroptotic cell death could provide a viable possibility to develop ferroptosis-based strategies, thus, impacting the therapeutic efficacy in human diseases.
3 Modulators that induce or inhibit ferroptosis in musculoskeletal diseases
Recent emerging reports have demonstrated the critical role of cellular ferroptosis in musculoskeletal diseases (Figure 3). Additionally, several ferroptosis regulators have shown promising therapeutic effects in the experimental models of human musculoskeletal disorders (Table 1). During oxidative stress conditions, the ferroportin (FPN)-dependent iron homeostasis weakened ferroptosis in bone cells in vitro and in vivo, suggesting the protective role of FPN in the pathogenesis of skeletal diseases (Lu et al., 2021).
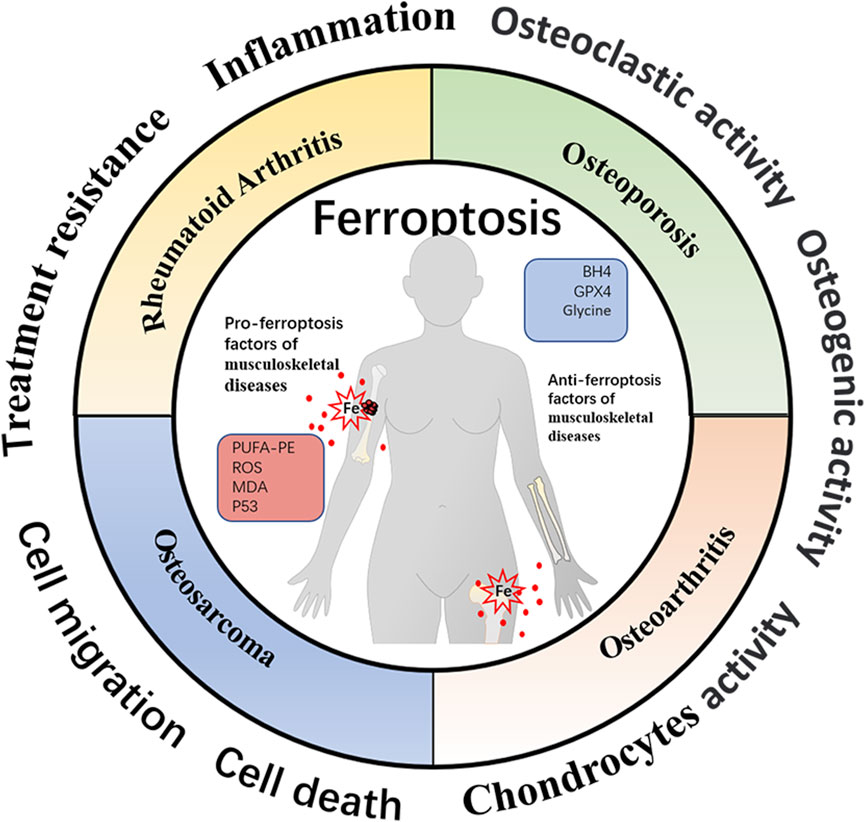
FIGURE 3. Schematic representation of ferroptosis-associated biomarkers in four different musculoskeletal diseases. The profiles of ferroptosis-related molecules have been proven to be well-established mediators affecting musculoskeletal disease progression and therapeutic response. The organic hallmarks present the biological functions of musculoskeletal diseases, including inflammation, cell death, cell migration, treatment resistance, etc.
3.1 Osteoarthritis
Being an important process in inflammatory diseases (Keuters et al., 2021; Chang et al., 2022), ferroptosis-based strategies have presented a long-time challenge for the clinical development of OA. In a rat OA model, treatment with interleukin (IL)-1β resulted in the decrease of collagen II and GPX4 and an increase in matrix metalloproteinases (MMPs), ROS and ion concentration, contributing to extracellular matrix (ECM) degradation and ferroptosis sensitivity (Lv et al., 2022). Additionally, two ferroptosis inhibitors, ferrostatin-1 (a lipophilic antioxidant) and DFO (an iron chelator), have demonstrated protective effects on OA cells, further supporting the predominant role of ferroptosis in OA pathology (Miao et al., 2022). Under inflammatory and iron overload conditions, intra-articular injection of ferrostatin-1 enhanced ferroptosis resistance in chondrocytes, leading to OA progression (Yao et al., 2021). In another report, treatment with IL-1β and erastin induced ferroptosis in mouse chondrocytes marked with excessive accumulation of lipid ROS and malondialdehyde (MDA), which could be reversed by DFO (Guo et al., 2022).
Recently, aberrant activation of HIFs displayed a functional role in controlling cellular metabolism and was involved in the progression of inflammatory diseases (Gonzalez et al., 2018). Under inflammatory conditions, D-mannose impaired glucose metabolism by inhibiting the succinate-induced activation of HIF-1α and lipopolysaccharide (LPS)-mediated activation of macrophages (Torretta et al., 2020). Other studies reported that HIF-2α (encoded by Epas1) influenced the metabolic phenotypes of cartilage and OA pathogenesis (Saito et al., 2010; Yang et al., 2010). The nicotinamide phosphoribosyl transferase (NAMPT) has been reported to be a direct target for HIF-2α; its overexpression could significantly increase the expression levels of matrix-degrading enzymes, causing cartilage destruction and accelerated OA development (Yang et al., 2015). Zhou et al. documented that mannitol reduced the sensitivity of chondrocytes to ferroptosis by inhibiting HIF-2α, thereby protecting chondrocytes and alleviating OA progession (Zhou et al., 2021). However, the detailed role of ferroptosis in OA pathogenesis and the therapeutic response has not been deeply explored. Therefore, ferroptosis-mediated occurrence and development of OA require more clinical and experimental research.
Identifying potential therapeutic agents for OA treatment and elucidating their underlying molecular mechanisms would be beneficial for developing novel strategies against OA. A recent study has shown that icariin (a major pharmacological component of a natural compound isolated from Epimedium) suppressed the expression of OA-related risk factors, such as IL-1β, MMPs, and GRP78, and improved the treatment efficacy against OA (Pan et al., 2017). Moreover, in vitro experiments have demonstrated that icariin could activate the Xc-/GPX4 signaling pathway to inhibit ferroptosis in response to the LPS-stimulated inflammation, thereby protecting the synovial cells from death (Luo and Zhang, 2021).
3.2 Osteoporosis
Osteoporosis is a major public health concern known to cause serious health risks and economic costs. Several factors have been demonstrated to contribute to the development of osteoporosis, such as inflammatory response, metabolic disorders, and genetic conditions (Ebeling et al., 2022). A few recent studies have established the involvement of ferroptosis in the progression of osteoporosis and the disruption of the balance between osteoclastic and osteogenic activities (Liu et al., 2022b; Gao et al., 2022; Yan et al., 2022). Another report showed that dexamethasone could weaken the activity of intracellular antioxidant systems through downregulation of the SLC7A11/GPX4 signaling, thereby enhancing the ferroptosis sensitivity of MC3T3-E1 and MOLY4 preosteoblasts and contributing to glucocorticoid-induced osteonecrosis (Sun et al., 2022). In a mouse model of steroid-induced osteoporosis, Lu et al. observed that the extracellular vesicles from bone marrow-derived endothelial progenitor cells (EPC-EVs) could alleviate the pathological changes related to osteoporosis by suppressing a series of ferroptosis associated features of osteoblasts (Lu et al., 2019). In a similar report, endothelial cell-secreted exosomes (EC-Exos) suppressed dexamethasone-induced osteoblast ferroptosis and reversed the inhibitory effect of osteoblast activity (Yang et al., 2021). Altogether these findings confirm the crucial role of ferroptosis in regulating the osteogenic activity during osteoporosis. Moreover, ferroptosis suppression could be a promising therapeutic strategy for osteoporosis management.
Ferroptosis induction by a high-fat and high-glucose diet may be the initiating factor for diabetic osteoporosis. High-fat and high-glucose conditions have resulted in osteoblastic ferroptosis by promoting METTL3-dependent m6A methylation of apoptosis signal-regulating kinase 1 (ASK1) and activating p38 signaling. On the contrary, METTL3 knockdown significantly abrogated the activation of ASK1-p38 signaling axis, resulting in ferroptosis attenuation and diabetic bone loss (Lin et al., 2022). In addition, mitochondrial ferritin (FtMt) is a key protein responsible for maintaining iron homeostasis and protecting cells from iron imbalance-induced death (Wang et al., 2021a). Increased FtMt expression can reduce cellular ROS concentration and inhibit ferroptosis in type 2 diabetic osteoporosis. Conversely, decreased FtMt expression may cause mitochondrial autophagy, further disturbing iron homeostasis and activating cell ferroptosis (Wang et al., 2022c). Furthermore, Yang et al. identified high levels of several pro-ferroptotic genes, such as heme oxygenase-1 (HO-1), in murine models of diabetic osteoporosis. Targeting HO-1 prohibited the occurrence of lipid peroxidation in osteocytes and effectively ameliorated the deterioration of bone trabeculae (Yang et al., 2022a). Moreover, the activation of Nrf2/HO-1 signaling pathway aided in the antagonistic effect of ferroptosis inhibitor melatonin against the high glucose-induced osteoporosis (Ma et al., 2020). These results suggest that pro-ferroptotic genes could serve as potential biomarkers for clinical therapy of diabetic osteoporosis.
3.3 Rheumatoid arthritis
Rheumatoid arthritis (RA) is a systemic inflammatory disease whose pathogenesis is often characterized by the infiltration of pro-inflammatory synovial fibroblasts, elevated frequency of synovial osteoclasts, and progressive joint destruction (Smolen et al., 2016; Zhao et al., 2022). Multiple strategies to inhibit the proliferation of synovial fibroblasts and reinstate synovial homeostasis in RA could provide promising therapeutic directions (Chen et al., 2022b; Ji et al., 2022; Sandhu and Thelma, 2022). Recent studies have reported that the increased risk of RA disease might be associated with the dysfunction of the antioxidant system in fibroblast-like synoviocytes (FLS). Additionally, the molecular characteristics of ferroptosis play an important role in maintaining the death and survival balance of synovium in RA patients, suggesting the involvement of ferroptosis in the pathogenesis and development of RA (Luczaj et al., 2016; England et al., 2018). Using a collagen-induced arthritis mouse model, Wu et al. reported that the combination of imidazole ketone erastin (a ferroptosis inducer) and etanercept (a tumor necrosis factor (TNF) inhibitor) could induce ferroptotic cell death and inhibit cell proliferation in synovial fibroblasts, thereby attenuating the RA development (Wu et al., 2022). In another report, glycine administration significantly induced the S-adenosyl methionine-dependent promoter methylation of GPX4 and decreased the expression of ferritin heavy chain 1 (FTH1) in FLS cells, followed by activation of FLS ferroptosis hindering RA development (Ling et al., 2022). These findings indicate the potential of ferroptosis-inducing compounds for exploitation as therapeutic candidates for RA patients.
Ferroptosis incentive influences RA progression; thus, explicating its underlying molecular mechanism could capture significant attention for RA treatment. Aberrant expression of FSP1, a molecular biomarker for ferroptosis inhibition (Bersuker et al., 2019), may be associated with RA development. Mechanistically, FSP1 overexpression interferes with the underlying signaling for lipid ROS generation, including TNF-α and p38/JNK signaling pathway. Based on the inhibition of ROS-induced peroxidation, FSP1 could protect chondrocytes from ferroptosis and delay the process of RA (Xie et al., 2021). In addition, serotransferrin (TF, a ferroptosis-inducer) (Hong et al., 2021) has been implicated in stimulating the anti-RA response. A corresponding study suggested the reduction of serum TF levels in antirheumatic drug-resistance patients (Chen et al., 2021b).
4 Approaches specifically targeting ferroptosis in musculoskeletal tumors
4.1 Ferroptosis inhibits osteosarcoma progression
Exploring the ferroptosis-related molecular mechanisms could provide new directions to study carcinogenesis and treatment response (Lin et al., 2021a; Qu et al., 2021). Previous studies have demonstrated the significance of targeting ferroptosis in anti-osteosarcoma treatment (Zhao et al., 2021b) (Table 2). Another intriguing research pointed out that iron zinc finger protein 36 (ZFP36), a ferroptosis-related gene, was significantly overexpressed in osteosarcoma patients, displaying a negative correlation with progression-free survival and overall survival; targeting ZFP36 could serve as a promising therapeutic biomarker for osteosarcoma patients (Song et al., 2021). Besides, traditional medicines, such as bavachin, might act as prospective drugs for the treatment of osteosarcoma patients (Luo et al., 2021). For example, administration of curcumin analogue EF24 in osteosarcoma U2OS and Saos-2 cells induced ferroptotic cell death characterized by increased concentration of intracellular MDA, lipid ROS, and ferric ions. However, the knockdown of ferroptosis-associated HMOX1 inversely attenuated the EF24-induced cytotoxicity effects (Lin et al., 2021b). Treatment with theaflavin-3,3′-digallate (TF3) could raise ROS levels and activate MAPK signaling pathways, causing ferroptotic cell death in OS cells MG63 and HOS (He et al., 2022). In addition, under hypoxia conditions, the prodrug tirapazamine was reported to decrease proliferation and induce ferroptosis in osteosarcoma 143B and U2OS cells; the biological functions were linked to the inhibition of SLC7A11 and GPX4 (Shi et al., 2021). Wang et al. (2022d) constructed the MH-PLGA-IR780 nanoparticles with homologous targeting ability, and found that MH-PLGA-IR780 could improve the photodynamic therapy (PDT)-induced ferroptosis sensitivity in human OS cell HOS, thus effectively killing tumors. Knockdown of Yes1 associated transcriptional regulator (YAP) in combination with ferroptosis induction obviously enhanced the sensitivity to pyropheophorbide-methyl ester-mediated PDT (MPPa-PDT) in HOS cells (Zhan et al., 2022).
4.2 Ferroptosis reduces chemotherapy resistance in osteosarcoma
The efficacy of osteosarcoma therapy is often limited due to the development of resistance. Ferroptosis modulation using pharmacological agents or genetic methods is required to sensitize osteosarcoma cells to enhance treatment benefits. MiR-1287-5p inhibited the activity of GPX4 via binding to its 3′-untranslated region, hence inducing ferroptosis in osteosarcoma cells. Notably, the intervention of miR-1287-5p led to osteosarcoma cells being more sensitive to cisplatin chemotherapy (Xu et al., 2021). In addition, KDM4A, a histone demethylase with the function of demethylating H3K9me3 in the promoter region of SLC7A11, inhibited ferroptosis-related cell death in osteosarcoma. Moreover, decreased KDM4A expression resulted in increased cell ferroptosis, attenuated migration ability, and enhanced cisplatin sensitivity in osteosarcoma 143B and HOS cells (Chen et al., 2021c). In another study, Tang et al. showed that ursolic acid, a natural component derived from radix Actinidiae chinensis, promoted ferroptosis sensitivity of osteosarcoma 143B and HOS cells by decomposing ferritin and caused intracellular iron accumulation through the activation of ferritinophagy. Additionally, ursolic acid effectively enhanced the cytotoxic effects of cisplatin on osteosarcoma cells (Tang et al., 2021b). Likewise, the combination of ferroptosis agonists (erastin and RSL3) and STAT3 (signal transducer and activator of transcription 3) inhibitors synergistically impaired the cisplatin resistance in osteosarcoma MG63 and Saos-2 cells (Liu and Wang, 2019). Recently, nanomedicine-based therapeutic methods have been applied to overcome treatment resistance and efficiently inhibit tumor development by inducing ferroptosis of tumor cells (Wang et al., 2021b). Fu et al. constructed a nano-carrier loaded with ferrate and doxorubicin that could initiate the Fenton reaction to promote ROS overproduction and iron-dependent ferroptotic cell death, thus overcoming chemotherapy resistance in osteosarcoma cells both in vitro and in vivo (Fu et al., 2021).
4.3 The ferroptosis-based strategies in other musculoskeletal tumors
Growing knowledge of ferroptosis in musculoskeletal tumors has led to the discovery of potential molecular targets for the development and identification of anti-cancer drugs. Moreover, the ferroptosis displays a crucial regulatory effect on the pathogenesis and therapeutic response of other musculoskeletal tumors, such as rhabdomyosarcoma and fibrosarcoma (Liu et al., 2022c).
Of note, Efimova et al. (2020) revealed that, in fibrosarcoma MCA205 cells, ferroptosis induced by RSL3 could cause HMGB1 and ATP-dependent immunogenic cell death (ICD). According to other studies, indocyanine green (ICG)-based PDT combined with NIR could cause ferroptosis in HT1080 fibrosarcoma cells (Tseng et al., 2022). Interestingly, the photosens (PS)-based PDT on MCA205 fibrosarcoma cells could also induce cellular ferroptosis and ICD (Turubanova et al., 2019). In addition, the advancements of nanomedical technology bring new hopefulness for the clinical manegment of musculoskeletal tumors. Zhou and his colleagues prepared siRNA@ABMBP-COF containing HK2 inhibitor 3-bromopyruvate and SLC7A11 siRNA. They found that siRNA@ABMBP-COF could induce ferroptosis and apoptosis in HT1080 fibrosarcoma cells by downregulating SLC7A11 and HK2, suggesting the significant anti-tumor capacity in a tumor-bearing nude mouse model (Zhou et al., 2022). Iron oxide nanoparticles (IONP-GA/PAA) enrobed with gallic acid and polyacrylic acid may be effective in killing HT1080 fibrosarcoma cells via HMOX1-dependent ferroptosis (Fernandez-Acosta et al., 2022). The agents, DO264 and salazosulfapyridine, cause ferroptosis in HT-1080 fibrosarcoma cells by inhibiting ABHD12 and system Xc-, resectively (Kathman et al., 2020; Yin et al., 2022). Additionally, the inhibitors targeting mitochondrial NADP-dependent isocitrate dehydrogenase (IDH2) and lysosome could efficiently prevent ferroptosis in HT-1080 fibrosarcoma cells by increasing GSH levels and decreasing intracellular ROS generation (Torii et al., 2016; Kim et al., 2020). Erastin or RSL3 have been shown to cause ferroptosis in rhabdomyosarcoma cells, which could be blocked by ferroptosis inhibitor ferrostatin-1 (Schott et al., 2015). Meanwhile, PKC inhibitors could also prevent erastin-induced ferroptosis in rhabdomyosarcoma cells (Dachert et al., 2020). These studies might provide novel insights into the ferroptosis-based therapeutic strategies in musculoskeletal tumors.
5 Discussion and future remarks
Ferroptosis has been considered a complex and anomalous metabolic process associated with pathological alterations in a wide array of disorders. Although ferroptosis-related research in musculoskeletal conditions is still in the infancy stage, the strategies interfering with disease progression through modulation of the ferroptosis pathway display enormous clinical potential (Table 3), including enhanced viability of osteoblasts and diminished therapeutic resistance.
Considering the chronic nature of a large proportion of musculoskeletal diseases, the drugs activated dose should be maintained for an extended period to treat the abnormal state. Therefore, local drug delivery with precise dose control should be monitored carefully to reduce adverse effects. For instance, the non-specific toxicity and rapid metabolism of DFO restrict its clinical application. In vivo experiments have shown that ROS-responsive polymeric nanogels containing DFO moieties (rNG-DFO) could reduce iron-mediated oxidative stress and enhance the safety profile of DFO (Liu et al., 2018). Abbina et al. demonstrated that iron-chelating nanoconjugate could be used to slow down drug metabolism rates and ameliorate the drug toxicity of DFO (Abbina et al., 2019). Thus, further investigation of ferroptosis-modulating drugs and the corresponding drug delivery methods is crucial in exploiting new treatment schedules for musculoskeletal disorders.
Ferroptosis has been defined as a distinguished type of programmed cell death compared to others, such as apoptosis, necroptosis, and pyroptosis; however, ferroptosis may not occur in isolation but is intricately linked with other forms of cell death in musculoskeletal conditions. For instance, NCOA4 induced ferritinophagy, a selective type of autophagy leading to iron accumulation, which could further induce osteoclast ferroptosis and reduce bone resorption in ovariectomy mouse (Ni et al., 2021). To adequately understand the effect of ferroptosis-associated diseases, the relationships among various cell deaths remain to be addressed. Verifying their roles in musculoskeletal disorders as mutually antagonistic or facilitative would provide new insights and prospects for disease treatment.
Emerging signaling pathways, such as Xc-GSH-GPX4, FSP1-CoQ10-NAD(P)H, and GCH1-BH4, have been confirmed to be crucial for ferroptosis regulation in musculoskeletal diseases. In the cellular model of TNF-α-induced RA, the bioactive peptide G1dP3 was demonstrated to be a potential therapeutic agent against RA; this effect was correlated with the increased p53-mediated ferroptosis in synovial fibroblasts (Hu et al., 2022b). However, the involvement of ferroptosis regulatory molecules, such as p53, in musculoskeletal diseases still requires further elaboration. Previous studies have indicated that CoQ10 has tremendous potential for application in treating musculoskeletal diseases, including RA (Abdollahzad et al., 2015; Jhun et al., 2020) and OA (Chang et al., 2020). In addition, Zhang et al. (2022b) reported that CoQ10 could improve oxidative stress, as indicated by the increased MDA concentration. Another report by Yang et al. supported the functional regulatory effects of CoQ10 in ferroptosis, controlling sodium iodate-induced pathologies in vitro and in vivo (Yang et al., 2022b). However, the efficacy of CoQ10 as a ferroptosis-modulating agent against musculoskeletal disorders is not yet fully elucidated. Determining whether targeting CoQ10 could regulate ferroptosis to treat musculoskeletal diseases or any modulators of CoQ10-related signaling pathways could be exploited as potential ferroptosis-modulating therapeutic agents indicate a promising role of ferroptosis in controlling cell death and may provide new avenues for identifying treatment targets for musculoskeletal diseases.
Author contributions
FZ, YY, and ZX: conception and design. YC, QL, YL, and BP: literature collection and data curation. FZ, ZX, and WL: writing the manuscript and revision of the manuscript. All authors contributed to the article and approved the submitted version.
Funding
This study is supported by grants from the Science and Technology Innovation Program of Hunan Province (2022RC1210, 2021RC3029) and the National Natural Science Foundation of China (82272659).
Conflict of interest
The authors declare that the research was conducted in the absence of any commercial or financial relationships that could be construed as a potential conflict of interest.
Publisher’s note
All claims expressed in this article are solely those of the authors and do not necessarily represent those of their affiliated organizations, or those of the publisher, the editors and the reviewers. Any product that may be evaluated in this article, or claim that may be made by its manufacturer, is not guaranteed or endorsed by the publisher.
References
Abbina, S., Abbasi, U., Gill, A., Wong, K., Kalathottukaren, M. T., and Kizhakkedathu, J. N. (2019). Design of safe nanotherapeutics for the excretion of excess systemic toxic iron. ACS Cent. Sci. 5 (5), 917–926. doi:10.1021/acscentsci.9b00284
Abdollahzad, H., Aghdashi, M. A., Asghari Jafarabadi, M., and Alipour, B. (2015). Effects of coenzyme Q10 supplementation on inflammatory cytokines (TNF-alpha, IL-6) and oxidative stress in rheumatoid arthritis patients: A randomized controlled trial. Arch. Med. Res. 46 (7), 527–533. doi:10.1016/j.arcmed.2015.08.006
Agmon, E., Solon, J., Bassereau, P., and Stockwell, B. R. (2018). Modeling the effects of lipid peroxidation during ferroptosis on membrane properties. Sci. Rep. 8 (1), 5155. doi:10.1038/s41598-018-23408-0
Arslanbaeva, L., Tosi, G., Ravazzolo, M., Simonato, M., Tucci, F. A., Pece, S., et al. (2022). UBIAD1 and CoQ10 protect melanoma cells from lipid peroxidation-mediated cell death. Redox Biol. 51, 102272. doi:10.1016/j.redox.2022.102272
Bersuker, K., Hendricks, J. M., Li, Z., Magtanong, L., Ford, B., Tang, P. H., et al. (2019). The CoQ oxidoreductase FSP1 acts parallel to GPX4 to inhibit ferroptosis. Nature 575 (7784), 688–692. doi:10.1038/s41586-019-1705-2
Cai, C., Hu, W., and Chu, T. (2021). Interplay between iron overload and osteoarthritis: Clinical significance and cellular mechanisms. Front. Cell Dev. Biol. 9, 817104. doi:10.3389/fcell.2021.817104
Chang, P. S., Yen, C. H., Huang, Y. Y., Chiu, C. J., and Lin, P. T. (2020). Associations between coenzyme Q10 status, oxidative stress, and muscle strength and endurance in patients with osteoarthritis. Antioxidants (Basel) 9 (12), 1275. doi:10.3390/antiox9121275
Chang, S., Tang, M., Zhang, B., Xiang, D., and Li, F. (2022). Ferroptosis in inflammatory arthritis: A promising future. Front. Immunol. 13, 955069. doi:10.3389/fimmu.2022.955069
Chen, D., Chu, B., Yang, X., Liu, Z., Jin, Y., Kon, N., et al. (2021a). iPLA2β-mediated lipid detoxification controls p53-driven ferroptosis independent of GPX4. Nat. Commun. 12 (1), 3644. doi:10.1038/s41467-021-23902-6
Chen, D., Li, S., Ge, Y., Kang, J., Liao, J. F., Du, J. F., et al. (2021b). iTRAQ and PRM-based proteomic analysis provides new insights into mechanisms of response to triple therapy in patients with rheumatoid arthritis. J. Inflamm. Res. 14, 6993–7006. doi:10.2147/JIR.S340351
Chen, J., Yang, X., Feng, Y., Li, Q., Ma, J., Wang, L., et al. (2022a). Targeting ferroptosis holds potential for intervertebral disc degeneration therapy. Cells 11 (21), 3508. doi:10.3390/cells11213508
Chen, J., Zhu, G., Sun, Y., Wu, Y., Wu, B., Zheng, W., et al. (2022b). 7-deacetyl-gedunin suppresses proliferation of Human rheumatoid arthritis synovial fibroblast through activation of Nrf2/ARE signaling. Int. Immunopharmacol. 107, 108557. doi:10.1016/j.intimp.2022.108557
Chen, J., Jiang, Y., and Sun, Y. (2021c). KDM4A-mediated histone demethylation of SLC7A11 inhibits cell ferroptosis in osteosarcoma. Biochem. Biophys. Res. Commun. 550, 77–83. doi:10.1016/j.bbrc.2021.02.137
Chen, X., Yu, C., Kang, R., and Tang, D. (2020). Iron metabolism in ferroptosis. Front. Cell Dev. Biol. 8, 590226. doi:10.3389/fcell.2020.590226
Dachert, J., Ehrenfeld, V., Habermann, K., Dolgikh, N., and Fulda, S. (2020). Targeting ferroptosis in rhabdomyosarcoma cells. Int. J. Cancer 146 (2), 510–520. doi:10.1002/ijc.32496
Dai, E., Zhang, W., Cong, D., Kang, R., Wang, J., and Tang, D. (2020). AIFM2 blocks ferroptosis independent of ubiquinol metabolism. Biochem. Biophys. Res. Commun. 523 (4), 966–971. doi:10.1016/j.bbrc.2020.01.066
Doll, S., Freitas, F. P., Shah, R., Aldrovandi, M., da Silva, M. C., Ingold, I., et al. (2019). FSP1 is a glutathione-independent ferroptosis suppressor. Nature 575 (7784), 693–698. doi:10.1038/s41586-019-1707-0
Doll, S., Proneth, B., Tyurina, Y. Y., Panzilius, E., Kobayashi, S., Ingold, I., et al. (2017). ACSL4 dictates ferroptosis sensitivity by shaping cellular lipid composition. Nat. Chem. Biol. 13 (1), 91–98. doi:10.1038/nchembio.2239
Ebeling, P. R., Nguyen, H. H., Aleksova, J., Vincent, A. J., Wong, P., and Milat, F. (2022). Secondary osteoporosis. Endocr. Rev. 43 (2), 240–313. doi:10.1210/endrev/bnab028
Efimova, I., Catanzaro, E., Van der Meeren, L., Turubanova, V. D., Hammad, H., Mishchenko, T. A., et al. (2020). Vaccination with early ferroptotic cancer cells induces efficient antitumor immunity. J. Immunother. Cancer 8 (2), e001369. doi:10.1136/jitc-2020-001369
England, B. R., Thiele, G. M., Anderson, D. R., and Mikuls, T. R. (2018). Increased cardiovascular risk in rheumatoid arthritis: Mechanisms and implications. BMJ 361, k1036. doi:10.1136/bmj.k1036
Fan, Z., Cai, L., Wang, S., Wang, J., and Chen, B. (2021). Baicalin prevents myocardial ischemia/reperfusion injury through inhibiting ACSL4 mediated ferroptosis. Front. Pharmacol. 12, 628988. doi:10.3389/fphar.2021.628988
Fernandez-Acosta, R., Iriarte-Mesa, C., Alvarez-Alminaque, D., Hassannia, B., Wiernicki, B., Diaz-Garcia, A. M., et al. (2022). Novel iron oxide nanoparticles induce ferroptosis in a panel of cancer cell lines. Molecules 27 (13), 3970. doi:10.3390/molecules27133970
Friedmann Angeli, J. P., Schneider, M., Proneth, B., Tyurina, Y. Y., Tyurin, V. A., Hammond, V. J., et al. (2014). Inactivation of the ferroptosis regulator Gpx4 triggers acute renal failure in mice. Nat. Cell Biol. 16 (12), 1180–1191. doi:10.1038/ncb3064
Fu, J., Li, T., Yang, Y., Jiang, L., Wang, W., Fu, L., et al. (2021). Activatable nanomedicine for overcoming hypoxia-induced resistance to chemotherapy and inhibiting tumor growth by inducing collaborative apoptosis and ferroptosis in solid tumors. Biomaterials 268, 120537. doi:10.1016/j.biomaterials.2020.120537
Fuhrmann, D. C., Mondorf, A., Beifuss, J., Jung, M., and Brune, B. (2020). Hypoxia inhibits ferritinophagy, increases mitochondrial ferritin, and protects from ferroptosis. Redox Biol. 36, 101670. doi:10.1016/j.redox.2020.101670
Gao, Z., Chen, Z., Xiong, Z., and Liu, X. (2022). Ferroptosis - a new target of osteoporosis. Exp. Gerontol. 165, 111836. doi:10.1016/j.exger.2022.111836
Gaschler, M. M., Andia, A. A., Liu, H., Csuka, J. M., Hurlocker, B., Vaiana, C. A., et al. (2018). FINO2 initiates ferroptosis through GPX4 inactivation and iron oxidation. Nat. Chem. Biol. 14 (5), 507–515. doi:10.1038/s41589-018-0031-6
Gonzalez, F. J., Xie, C., and Jiang, C. (2018). The role of hypoxia-inducible factors in metabolic diseases. Nat. Rev. Endocrinol. 15 (1), 21–32. doi:10.1038/s41574-018-0096-z
Guo, Z., Lin, J., Sun, K., Guo, J., Yao, X., Wang, G., et al. (2022). Deferoxamine alleviates osteoarthritis by inhibiting chondrocyte ferroptosis and activating the Nrf2 pathway. Front. Pharmacol. 13, 791376. doi:10.3389/fphar.2022.791376
Hadian, K., and Stockwell, B. R. (2020). SnapShot: Ferroptosis. Cell 181 (5), 1188–1188 e1181. doi:10.1016/j.cell.2020.04.039
Hassannia, B., Vandenabeele, P., and Vanden Berghe, T. (2019). Targeting ferroptosis to iron out cancer. Cancer Cell 35 (6), 830–849. doi:10.1016/j.ccell.2019.04.002
He, T., Lin, X., Yang, C., Chen, Z., Wang, L., Li, Q., et al. (2022). Theaflavin-3,3'-Digallate plays a ROS-mediated dual role in ferroptosis and apoptosis via the MAPK pathway in human osteosarcoma cell lines and xenografts. Oxid. Med. Cell Longev. 2022, 8966368. doi:10.1155/2022/8966368
Hong, X., Roh, W., Sullivan, R. J., Wong, K. H. K., Wittner, B. S., Guo, H., et al. (2021). The lipogenic regulator SREBP2 induces transferrin in circulating melanoma cells and suppresses ferroptosis. Cancer Discov. 11 (3), 678–695. doi:10.1158/2159-8290.CD-19-1500
Hu, J., Zhang, R., Chang, Q., Ji, M., Geng, R., et al. (2022b). p53: A regulator of ferroptosis induced by galectin-1 derived peptide 3 in MH7A cells. Front. Genet. 13, 920273. doi:10.3389/fgene.2022.920273
Hu, J., Wei, W., Wu, D., Huang, F., Li, M., Li, W., et al. (2022a). Blockade of GCH1/BH4 Axis activates ferritinophagy to mitigate the resistance of colorectal cancer to erastin-induced ferroptosis. Front. Cell Dev. Biol. 10, 810327. doi:10.3389/fcell.2022.810327
Hwang, H. S., and Kim, H. A. (2015). Chondrocyte apoptosis in the pathogenesis of osteoarthritis. Int. J. Mol. Sci. 16 (11), 26035–26054. doi:10.3390/ijms161125943
Ingold, I., Berndt, C., Schmitt, S., Doll, S., Poschmann, G., Buday, K., et al. (2018). Selenium utilization by GPX4 is required to prevent hydroperoxide-induced ferroptosis. Cell 172 (3), 409–422. doi:10.1016/j.cell.2017.11.048
Jhun, J., Moon, J., Ryu, J., Shin, Y., Lee, S., Cho, K. H., et al. (2020). Liposome/gold hybrid nanoparticle encoded with CoQ10 (LGNP-CoQ10) suppressed rheumatoid arthritis via STAT3/Th17 targeting. PLoS One 15 (11), e0241080. doi:10.1371/journal.pone.0241080
Ji, M., Ryu, H. J., Baek, H. M., Shin, D. M., and Hong, J. H. (2022). Dynamic synovial fibroblasts are modulated by NBCn1 as a potential target in rheumatoid arthritis. Exp. Mol. Med. 54 (4), 503–517. doi:10.1038/s12276-022-00756-6
Jiang, Z., Wang, H., Qi, G., Jiang, C., Chen, K., and Yan, Z. (2022). Iron overload-induced ferroptosis of osteoblasts inhibits osteogenesis and promotes osteoporosis: An in vitro and in vivo study. IUBMB Life 74 (11), 1052–1069. doi:10.1002/iub.2656
Jing, X., Lin, J., Du, T., Jiang, Z., Wang, G., et al. (2020). Iron overload is associated with accelerated progression of osteoarthritis: The role of DMT1 mediated iron homeostasis. Front. Cell Dev. Biol. 8, 594509. doi:10.3389/fcell.2020.594509
Kagan, V. E., Mao, G., Qu, F., Angeli, J. P. F., Doll, S., Croix, C. S., et al. (2017). Oxidized arachidonic and adrenic PEs navigate cells to ferroptosis. Nat. Chem. Biol. 13 (1), 81–90. doi:10.1038/nchembio.2238
Kagan, V. E., Tyurina, Y. Y., Vlasova, , Kapralov, A. A., Amoscato, A. A., Anthonymuthu, T. S., et al. (2020). Redox epiphospholipidome in programmed cell death signaling: Catalytic mechanisms and regulation. Front. Endocrinol. (Lausanne) 11, 628079. doi:10.3389/fendo.2020.628079
Kathman, S. G., Boshart, J., Jing, H., and Cravatt, B. F. (2020). Blockade of the lysophosphatidylserine lipase ABHD12 potentiates ferroptosis in cancer cells. ACS Chem. Biol. 15 (4), 871–877. doi:10.1021/acschembio.0c00086
Keuters, M. H., Keksa-Goldsteine, V., Dhungana, H., Huuskonen, M. T., Pomeshchik, Y., Savchenko, E., et al. (2021). An arylthiazyne derivative is a potent inhibitor of lipid peroxidation and ferroptosis providing neuroprotection in vitro and in vivo. Sci. Rep. 11 (1), 3518. doi:10.1038/s41598-021-81741-3
Kim, H., Lee, J. H., and Park, J. W. (2020). Down-regulation of IDH2 sensitizes cancer cells to erastin-induced ferroptosis. Biochem. Biophys. Res. Commun. 525 (2), 366–371. doi:10.1016/j.bbrc.2020.02.093
Kraft, V. A. N., Bezjian, C. T., Pfeiffer, S., Ringelstetter, L., Muller, C., Zandkarimi, F., et al. (2020). GTP cyclohydrolase 1/tetrahydrobiopterin counteract ferroptosis through lipid remodeling. ACS Cent. Sci. 6 (1), 41–53. doi:10.1021/acscentsci.9b01063
Kuang, F., Liu, J., Tang, D., and Kang, R. (2020). Oxidative damage and antioxidant defense in ferroptosis. Front. Cell Dev. Biol. 8, 586578. doi:10.3389/fcell.2020.586578
Lee, H., Zandkarimi, F., Zhang, Y., Meena, J. K., Kim, J., Zhuang, L., et al. (2020). Energy-stress-mediated AMPK activation inhibits ferroptosis. Nat. Cell Biol. 22 (2), 225–234. doi:10.1038/s41556-020-0461-8
Lewerenz, J., Hewett, S. J., Huang, Y., Lambros, M., Gout, P. W., Kalivas, P. W., et al. (2013). The cystine/glutamate antiporter system x(c)(-) in health and disease: From molecular mechanisms to novel therapeutic opportunities. Antioxid. Redox Signal 18 (5), 522–555. doi:10.1089/ars.2011.4391
Lin, H. Y., Chen, X., Zhang, C., Yang, T., Deng, Z., Song, Y., et al. (2021b). EF24 induces ferroptosis in osteosarcoma cells through HMOX1. Biomed. Pharmacother. 136, 111202. doi:10.1016/j.biopha.2020.111202
Lin, H. Y., Ho, H. W., Chang, Y. H., Wei, C. J., and Chu, P. Y. (2021a). The evolving role of ferroptosis in breast cancer: Translational implications present and future. Cancers (Basel) 13 (18), 4576. doi:10.3390/cancers13184576
Lin, Y., Shen, X., Ke, Y., Lan, C., Chen, X., Liang, B., et al. (2022). Activation of osteoblast ferroptosis via the METTL3/ASK1-p38 signaling pathway in high glucose and high fat (HGHF)-induced diabetic bone loss. FASEB J. 36 (3), e22147. doi:10.1096/fj.202101610R
Ling, H., Li, M., Yang, C., Sun, S., Zhang, W., Zhao, L., et al. (2022). Glycine increased ferroptosis via SAM-mediated GPX4 promoter methylation in rheumatoid arthritis. Rheumatol. Oxf. 61, 4521–4534. doi:10.1093/rheumatology/keac069
Liu, J., Lou, C., Zhen, C., Wang, Y., Shang, P., and Lv, H. (2022a). Iron plays a role in sulfasalazine-induced ferroptosis with autophagic flux blockage in K7M2 osteosarcoma cells. Metallomics 14 (5), mfac027. doi:10.1093/mtomcs/mfac027
Liu, N., Lin, X., and Huang, C. (2020). Activation of the reverse transsulfuration pathway through NRF2/CBS confers erastin-induced ferroptosis resistance. Br. J. Cancer 122 (2), 279–292. doi:10.1038/s41416-019-0660-x
Liu, J., Wang, W., Li, Z., Li, Y., Yu, X., Tu, J., et al. (2022b). Ferroptosis: A new regulatory mechanism in osteoporosis. Oxid. Med. Cell Longev. 2022, 2634431. doi:10.1155/2022/2634431
Liu, Q., and Wang, K. (2019). The induction of ferroptosis by impairing STAT3/Nrf2/GPx4 signaling enhances the sensitivity of osteosarcoma cells to cisplatin. Cell Biol. Int. 43 (11), 1245–1256. doi:10.1002/cbin.11121
Liu, J., Du, S., Wang, S., and Ye, K. (2022c). Ferroptosis in osteosarcoma: A promising future. Front. Oncol. 12, 1031779. doi:10.3389/fonc.2022.1031779
Liu, Z., Qiao, J., Nagy, T., and Xiong, M. P. (2018). ROS-triggered degradable iron-chelating nanogels: Safely improving iron elimination in vivo. J. Control Release 283, 84–93. doi:10.1016/j.jconrel.2018.05.025
Lu, J., Yang, J., Zheng, Y., Chen, X., and Fang, S. (2019). Extracellular vesicles from endothelial progenitor cells prevent steroid-induced osteoporosis by suppressing the ferroptotic pathway in mouse osteoblasts based on bioinformatics evidence. Sci. Rep. 9 (1), 16130. doi:10.1038/s41598-019-52513-x
Lu, S., Song, Y., Luo, R., Li, S., Li, G., Wang, K., et al. (2021). Ferroportin-dependent iron homeostasis protects against oxidative stress-induced nucleus pulposus cell ferroptosis and ameliorates intervertebral disc degeneration in vivo. Oxid. Med. Cell Longev. 2021, 6670497. doi:10.1155/2021/6670497
Luczaj, W., Gindzienska-Sieskiewicz, E., Jarocka-Karpowicz, I., Andrisic, L., Sierakowski, S., Zarkovic, N., et al. (2016). The onset of lipid peroxidation in rheumatoid arthritis: Consequences and monitoring. Free Radic. Res. 50 (3), 304–313. doi:10.3109/10715762.2015.1112901
Luo, C., Xu, W., Tang, X., Liu, X., Cheng, Y., Wu, Y., et al. (2022). Canonical Wnt signaling works downstream of iron overload to prevent ferroptosis from damaging osteoblast differentiation. Free Radic. Biol. Med. 188, 337–350. doi:10.1016/j.freeradbiomed.2022.06.236
Luo, H., and Zhang, R. (2021). Icariin enhances cell survival in lipopolysaccharide-induced synoviocytes by suppressing ferroptosis via the Xc-/GPX4 axis. Exp. Ther. Med. 21 (1), 72. doi:10.3892/etm.2020.9504
Luo, Y., Gao, X., Zou, L., Lei, M., Feng, J., and Hu, Z. (2021). Bavachin induces ferroptosis through the STAT3/P53/slc7a11 Axis in osteosarcoma cells. Oxid. Med. Cell Longev. 2021, 1783485. doi:10.1155/2021/1783485
Lv, M., Cai, Y., Hou, W., Peng, K., Xu, K., Lu, C., et al. (2022). The RNA-binding protein SND1 promotes the degradation of GPX4 by destabilizing the HSPA5 mRNA and suppressing HSPA5 expression, promoting ferroptosis in osteoarthritis chondrocytes. Inflamm. Res. 71 (4), 461–472. doi:10.1007/s00011-022-01547-5
Ma, H., Wang, X., Zhang, W., Li, H., Zhao, W., Sun, J., et al. (2020). Melatonin suppresses ferroptosis induced by high glucose via activation of the Nrf2/HO-1 signaling pathway in type 2 diabetic osteoporosis. Oxid. Med. Cell Longev. 2020, 9067610. doi:10.1155/2020/9067610
Martis, R. M., Knight, L. J., Donaldson, P. J., and Lim, J. C. (2020). Identification, expression, and roles of the cystine/glutamate antiporter in ocular tissues. Oxid. Med. Cell Longev. 2020, 4594606. doi:10.1155/2020/4594606
McBean, G. J. (2012). The transsulfuration pathway: A source of cysteine for glutathione in astrocytes. Amino Acids 42 (1), 199–205. doi:10.1007/s00726-011-0864-8
Miao, Y., Chen, Y., Xue, F., Liu, K., Zhu, B., Gao, J., et al. (2022). Contribution of ferroptosis and GPX4's dual functions to osteoarthritis progression. EBioMedicine 76, 103847. doi:10.1016/j.ebiom.2022.103847
Ni, S., Yuan, Y., Qian, Z., Zhong, Z., Lv, T., Kuang, Y., et al. (2021). Hypoxia inhibits RANKL-induced ferritinophagy and protects osteoclasts from ferroptosis. Free Radic. Biol. Med. 169, 271–282. doi:10.1016/j.freeradbiomed.2021.04.027
Pan, L., Zhang, Y., Chen, N., and Yang, L. (2017). Icariin regulates cellular functions and gene expression of osteoarthritis patient-derived human fibroblast-like synoviocytes. Int. J. Mol. Sci. 18 (12), 2656. doi:10.3390/ijms18122656
Qu, M., Zhang, H., Chen, Z., Sun, X., Zhu, S., Nan, K., et al. (2021). The role of ferroptosis in acute respiratory distress syndrome. Front. Med. (Lausanne) 8, 651552. doi:10.3389/fmed.2021.651552
Riegman, M., Sagie, L., Galed, C., Levin, T., Steinberg, N., Dixon, S. J., et al. (2020). Ferroptosis occurs through an osmotic mechanism and propagates independently of cell rupture. Nat. Cell Biol. 22 (9), 1042–1048. doi:10.1038/s41556-020-0565-1
Saito, T., Fukai, A., Mabuchi, A., Ikeda, T., Yano, F., Ohba, S., et al. (2010). Transcriptional regulation of endochondral ossification by HIF-2alpha during skeletal growth and osteoarthritis development. Nat. Med. 16 (6), 678–686. doi:10.1038/nm.2146
Sandhu, G., and Thelma, B. K. (2022). New druggable targets for rheumatoid arthritis based on insights from synovial Biology. Front. Immunol. 13, 834247. doi:10.3389/fimmu.2022.834247
Santana-Codina, N., Gikandi, A., and Mancias, J. D. (2021). The role of NCOA4-mediated ferritinophagy in ferroptosis. Adv. Exp. Med. Biol. 1301, 41–57. doi:10.1007/978-3-030-62026-4_4
Santana-Codina, N., and Mancias, J. D. (2018). The role of NCOA4-mediated ferritinophagy in health and disease. Pharm. (Basel) 11 (4), 114. doi:10.3390/ph11040114
Schott, C., Graab, U., Cuvelier, N., Hahn, H., and Fulda, S. (2015). Oncogenic RAS mutants confer resistance of RMS13 rhabdomyosarcoma cells to oxidative stress-induced ferroptotic cell death. Front. Oncol. 5, 131. doi:10.3389/fonc.2015.00131
Shi, Y., Gong, M., Deng, Z., Liu, H., Chang, Y., Yang, Z., et al. (2021). Tirapazamine suppress osteosarcoma cells in part through SLC7A11 mediated ferroptosis. Biochem. Biophys. Res. Commun. 567, 118–124. doi:10.1016/j.bbrc.2021.06.036
Smolen, J. S., Aletaha, D., and McInnes, I. B. (2016). Rheumatoid arthritis. Lancet 388 (10055), 2023–2038. doi:10.1016/S0140-6736(16)30173-8
Song, P., Xie, Z., Chen, C., Chen, L., Wang, X., Wang, F., et al. (2021). Identification of a novel iron zinc finger protein 36 (ZFP36) for predicting the overall survival of osteosarcoma based on the Gene Expression Omnibus (GEO) database. Ann. Transl. Med. 9 (20), 1552. doi:10.21037/atm-21-5086
Soula, M., Weber, R. A., Zilka, O., Alwaseem, H., La, K., Yen, F., et al. (2020). Metabolic determinants of cancer cell sensitivity to canonical ferroptosis inducers. Nat. Chem. Biol. 16 (12), 1351–1360. doi:10.1038/s41589-020-0613-y
Spel, L., and Martinon, F. (2020). Inflammasomes contributing to inflammation in arthritis. Immunol. Rev. 294 (1), 48–62. doi:10.1111/imr.12839
Stockwell, B. R., Friedmann Angeli, J. P., Bayir, H., Bush, A. I., Conrad, M., Dixon, S. J., et al. (2017). Ferroptosis: A regulated cell death nexus linking metabolism, redox Biology, and disease. Cell 171 (2), 273–285. doi:10.1016/j.cell.2017.09.021
Su, Y., Zhao, B., Zhou, L., Zhang, Z., Shen, Y., Lv, H., et al. (2020). Ferroptosis, a novel pharmacological mechanism of anti-cancer drugs. Cancer Lett. 483, 127–136. doi:10.1016/j.canlet.2020.02.015
Sun, F., Zhou, J. L., Liu, Z. L., Jiang, Z. W., and Peng, H. (2022). Dexamethasone induces ferroptosis via P53/SLC7A11/GPX4 pathway in glucocorticoid-induced osteonecrosis of the femoral head. Biochem. Biophys. Res. Commun. 602, 149–155. doi:10.1016/j.bbrc.2022.02.112
Sun, Y., Zheng, Y., Wang, C., and Liu, Y. (2018). Glutathione depletion induces ferroptosis, autophagy, and premature cell senescence in retinal pigment epithelial cells. Cell Death Dis. 9 (7), 753. doi:10.1038/s41419-018-0794-4
Tacchini, L., Bianchi, L., Bernelli-Zazzera, A., and Cairo, G. (1999). Transferrin receptor induction by hypoxia. HIF-1-mediated transcriptional activation and cell-specific post-transcriptional regulation. J. Biol. Chem. 274 (34), 24142–24146. doi:10.1074/jbc.274.34.24142
Tang, D., Chen, X., Kang, R., and Kroemer, G. (2021a). Ferroptosis: Molecular mechanisms and health implications. Cell Res. 31 (2), 107–125. doi:10.1038/s41422-020-00441-1
Tang, D., Dong, H., Li, T., Wang, N., Wei, X., Wu, H., et al. (2021b). The synergistic reducing drug resistance effect of cisplatin and ursolic acid on osteosarcoma through a multistep mechanism involving ferritinophagy. Oxid. Med. Cell Longev. 2021, 5192271. doi:10.1155/2021/5192271
Taylor, M., Qu, A., Anderson, E. R., Matsubara, T., Martin, A., Gonzalez, F. J., et al. (2011). Hypoxia-inducible factor-2α mediates the adaptive increase of intestinal ferroportin during iron deficiency in mice. Gastroenterology 140 (7), 2044–2055. doi:10.1053/j.gastro.2011.03.007
Torii, S., Shintoku, R., Kubota, C., Yaegashi, M., Torii, R., Sasaki, M., et al. (2016). An essential role for functional lysosomes in ferroptosis of cancer cells. Biochem. J. 473 (6), 769–777. doi:10.1042/BJ20150658
Torretta, S., Scagliola, A., Ricci, L., Mainini, F., Di Marco, S., Cuccovillo, I., et al. (2020). D-mannose suppresses macrophage IL-1β production. Nat. Commun. 11 (1), 6343. doi:10.1038/s41467-020-20164-6
Toxqui, L., and Vaquero, M. P. (2015). Chronic iron deficiency as an emerging risk factor for osteoporosis: A hypothesis. Nutrients 7 (4), 2324–2344. doi:10.3390/nu7042324
Tseng, H. C., Kuo, C. Y., Liao, W. T., Chou, T. S., and Hsiao, J. K. (2022). Indocyanine green as a near-infrared theranostic agent for ferroptosis and apoptosis-based, photothermal, and photodynamic cancer therapy. Front. Mol. Biosci. 9, 1045885. doi:10.3389/fmolb.2022.1045885
Turubanova, V. D., Balalaeva, I. V., Mishchenko, T. A., Catanzaro, E., Alzeibak, R., Peskova, N. N., et al. (2019). Immunogenic cell death induced by a new photodynamic therapy based on photosens and photodithazine. J. Immunother. Cancer 7 (1), 350. doi:10.1186/s40425-019-0826-3
Ursini, F., and Maiorino, M. (2020). Lipid peroxidation and ferroptosis: The role of GSH and GPx4. Free Radic. Biol. Med. 152, 175–185. doi:10.1016/j.freeradbiomed.2020.02.027
Vancassel, S., Fanet, H., Castanon, N., Monchaux De Oliveira, C., Cussotto, S., and Capuron, L. (2022). Tetrahydrobiopterin modulates the behavioral neuroinflammatory response to an LPS challenge in mice. Brain Behav. Immun. 105, 139–148. doi:10.1016/j.bbi.2022.06.016
Vasquez-Vivar, J., Shi, Z., and Tan, S. (2022). Tetrahydrobiopterin in cell function and death mechanisms. Antioxid. Redox Signal 37 (1-3), 171–183. doi:10.1089/ars.2021.0136
Wang, P., Cui, Y., Ren, Q., Yan, B., Zhao, Y., Yu, P., et al. (2021a). Mitochondrial ferritin attenuates cerebral ischaemia/reperfusion injury by inhibiting ferroptosis. Cell Death Dis. 12 (5), 447. doi:10.1038/s41419-021-03725-5
Wang, W., Jing, X., Du, T., Ren, J., Liu, X., Chen, F., et al. (2022a). Iron overload promotes intervertebral disc degeneration via inducing oxidative stress and ferroptosis in endplate chondrocytes. Free Radic. Biol. Med. 190, 234–246. doi:10.1016/j.freeradbiomed.2022.08.018
Wang, W., Ma, H., Sun, J., Zheng, T., Zhao, P., Li, H., et al. (2022c). Mitochondrial ferritin deficiency promotes osteoblastic ferroptosis via mitophagy in type 2 diabetic osteoporosis. Biol. Trace Elem. Res. 200 (1), 298–307. doi:10.1007/s12011-021-02627-z
Wang, P., Liu, T., Li, X., Sheng, H., Ma, X., and Hao, L. (2021b). Ferroptosis-inducing nanomedicine for cancer therapy. Front. Pharmacol. 12, 735965. doi:10.3389/fphar.2021.735965
Wang, W., Zhang, L., Zhao, G., Zhan, F., Chen, Z., et al. (2022d). Homologous targeting nanoparticles for enhanced PDT against osteosarcoma HOS cells and the related molecular mechanisms. J. Nanobiotechnology 20 (1), 83. doi:10.1186/s12951-021-01201-y
Wang, W., Zhang, M., Bi, R., Su, Y., Quan, F., Lin, Y., et al. (2022b). ACSL4 deficiency confers protection against ferroptosis-mediated acute kidney injury. Redox Biol. 51, 102262. doi:10.1016/j.redox.2022.102262
Wei, X., Yi, X., Zhu, X. H., and Jiang, D. S. (2020). Posttranslational modifications in ferroptosis. Oxid. Med. Cell Longev. 2020, 8832043. doi:10.1155/2020/8832043
Wen, Q., Liu, J., Kang, R., Zhou, B., and Tang, D. (2019). The release and activity of HMGB1 in ferroptosis. Biochem. Biophys. Res. Commun. 510 (2), 278–283. doi:10.1016/j.bbrc.2019.01.090
Wu, J., Feng, Z., Chen, L., Li, Y., Bian, H., Geng, J., et al. (2022). TNF antagonist sensitizes synovial fibroblasts to ferroptotic cell death in collagen-induced arthritis mouse models. Nat. Commun. 13 (1), 676. doi:10.1038/s41467-021-27948-4
Xie, Z., Hou, H., Luo, D., An, R., Zhao, Y., and Qiu, C. (2021). ROS-dependent lipid peroxidation and reliant antioxidant ferroptosis-suppressor-protein 1 in rheumatoid arthritis: A covert clue for potential therapy. Inflammation 44 (1), 35–47. doi:10.1007/s10753-020-01338-2
Xu, J., Ma, J., Chen, J., Zhang, S., Zheng, C., Si, H., et al. (2022). No genetic causal association between iron status and osteoporosis: A two-sample mendelian randomization. Front. Endocrinol. (Lausanne) 13, 996244. doi:10.3389/fendo.2022.996244
Xu, M. M., Wang, J., and Xie, J. X. (2017). Regulation of iron metabolism by hypoxia-inducible factors. Sheng Li Xue Bao 69 (5), 598–610.
Xu, Z., Chen, L., Wang, C., Zhang, L., and Xu, W. (2021). MicroRNA-1287-5p promotes ferroptosis of osteosarcoma cells through inhibiting GPX4. Free Radic. Res. 55 (11-12), 1119–1129. doi:10.1080/10715762.2021.2024816
Xue, Y., Zhang, G., Zhou, S., Wang, S., Lv, H., Zhou, L., et al. (2021). Iron chelator induces apoptosis in osteosarcoma cells by disrupting intracellular iron homeostasis and activating the MAPK pathway. Int. J. Mol. Sci. 22 (13), 7168. doi:10.3390/ijms22137168
Yan, C., Zhang, J., An, F., Wang, J., Shi, Y., Yuan, L., et al. (2022). Research progress of ferroptosis regulatory network and bone remodeling in osteoporosis. Front. Public Health 10, 910675. doi:10.3389/fpubh.2022.910675
Yang, L., Wang, D., Wang, X. T., Lu, Y. P., and Zhu, L. (2018). The roles of hypoxia-inducible Factor-1 and iron regulatory protein 1 in iron uptake induced by acute hypoxia. Biochem. Biophys. Res. Commun. 507 (1-4), 128–135. doi:10.1016/j.bbrc.2018.10.185
Yang, M., Tsui, M. G., Tsang, J. K. W., Goit, R. K., Yao, K. M., So, K. F., et al. (2022b). Involvement of FSP1-CoQ10-NADH and GSH-GPx-4 pathways in retinal pigment epithelium ferroptosis. Cell Death Dis. 13 (5), 468. doi:10.1038/s41419-022-04924-4
Yang, R. Z., Xu, W. N., Zheng, H. L., Zheng, X. F., Li, B., Jiang, L. S., et al. (2021). Exosomes derived from vascular endothelial cells antagonize glucocorticoid-induced osteoporosis by inhibiting ferritinophagy with resultant limited ferroptosis of osteoblasts. J. Cell Physiol. 236 (9), 6691–6705. doi:10.1002/jcp.30331
Yang, S., Kim, J., Ryu, J. H., Oh, H., Chun, C. H., Kim, B. J., et al. (2010). Hypoxia-inducible factor-2alpha is a catabolic regulator of osteoarthritic cartilage destruction. Nat. Med. 16 (6), 687–693. doi:10.1038/nm.2153
Yang, S., Ryu, J. H., Oh, H., Jeon, J., Kwak, J. S., Kim, J. H., et al. (2015). NAMPT (visfatin), a direct target of hypoxia-inducible factor-2α, is an essential catabolic regulator of osteoarthritis. Ann. Rheum. Dis. 74 (3), 595–602. doi:10.1136/annrheumdis-2013-204355
Yang, W. S., Kim, K. J., Gaschler, M. M., Patel, M., Shchepinov, M. S., and Stockwell, B. R. (2016). Peroxidation of polyunsaturated fatty acids by lipoxygenases drives ferroptosis. Proc. Natl. Acad. Sci. U. S. A. 113 (34), E4966–E4975. doi:10.1073/pnas.1603244113
Yang, W. S., SriRamaratnam, R., Welsch, M. E., Shimada, K., Skouta, R., Viswanathan, V. S., et al. (2014). Regulation of ferroptotic cancer cell death by GPX4. Cell 156 (1-2), 317–331. doi:10.1016/j.cell.2013.12.010
Yang, M., Lin, Y., Wang, M., Yuan, K., Wang, Q., Mu, P., et al. (2022a). Targeting ferroptosis suppresses osteocyte glucolipotoxicity and alleviates diabetic osteoporosis. Bone Res. 10 (1), 26. doi:10.1038/s41413-022-00198-w
Yao, X., Sun, K., Yu, S., Luo, J., Guo, J., Lin, J., et al. (2021). Chondrocyte ferroptosis contribute to the progression of osteoarthritis. J. Orthop. Transl. 27, 33–43. doi:10.1016/j.jot.2020.09.006
Yin, L., Liu, P., Jin, Y., Ning, Z., Yang, Y., and Gao, H. (2022). Ferroptosis-related small-molecule compounds in cancer therapy: Strategies and applications. Eur. J. Med. Chem. 244, 114861. doi:10.1016/j.ejmech.2022.114861
Yoshida, M., Minagawa, S., Araya, J., Sakamoto, T., Hara, H., Tsubouchi, K., et al. (2019). Involvement of cigarette smoke-induced epithelial cell ferroptosis in COPD pathogenesis. Nat. Commun. 10 (1), 3145. doi:10.1038/s41467-019-10991-7
Yu, M., Lin, Z., Tian, X., Chen, S., Liang, X., Qin, M., et al. (2021). Downregulation of Cx43 reduces cisplatin-induced acute renal injury by inhibiting ferroptosis. Food Chem. Toxicol. 158, 112672. doi:10.1016/j.fct.2021.112672
Yu, Y., Jiang, L., Wang, H., Shen, Z., Cheng, Q., Zhang, P., et al. (2020). Hepatic transferrin plays a role in systemic iron homeostasis and liver ferroptosis. Blood 136 (6), 726–739. doi:10.1182/blood.2019002907
Zhan, F., Zhang, Y., Zuo, Q., Xie, C., Li, H., Tian, L., et al. (2022). YAP knockdown in combination with ferroptosis induction increases the sensitivity of HOS human osteosarcoma cells to pyropheophorbide-alpha methyl ester-mediated photodynamic therapy. Photodiagnosis Photodyn. Ther. 39, 102964. doi:10.1016/j.pdpdt.2022.102964
Zhang, Y., Huang, X., Liu, N., Liu, M., Sun, C., Qi, B., et al. (2022b). Discovering the potential value of coenzyme Q10 in oxidative stress: Enlightenment from a synthesis of clinical evidence based on various population. Front. Pharmacol. 13, 936233. doi:10.3389/fphar.2022.936233
Zhang, Y., Huang, X., Qi, B., Sun, C., Sun, K., Liu, N., et al. (2022a). Ferroptosis and musculoskeletal diseases: "Iron maiden" cell death may be a promising therapeutic target. Front. Immunol. 13, 972753. doi:10.3389/fimmu.2022.972753
Zhang, Y., Swanda, R. V., Nie, L., Liu, X., Wang, C., Lee, H., et al. (2021). mTORC1 couples cyst(e)ine availability with GPX4 protein synthesis and ferroptosis regulation. Nat. Commun. 12 (1), 1589. doi:10.1038/s41467-021-21841-w
Zhao, J., Jiang, P., Guo, S., Schrodi, S. J., and He, D. (2021a). Apoptosis, autophagy, NETosis, necroptosis, and pyroptosis mediated programmed cell death as targets for innovative therapy in rheumatoid arthritis. Front. Immunol. 12, 809806. doi:10.3389/fimmu.2021.809806
Zhao, J., Zhao, Y., Ma, X., Zhang, B., and Feng, H. (2021b). Targeting ferroptosis in osteosarcoma. J. Bone Oncol. 30, 100380. doi:10.1016/j.jbo.2021.100380
Zhao, T., Yang, Q., Xi, Y., Xie, Z., Shen, J., Li, Z., et al. (2022). Ferroptosis in rheumatoid arthritis: A potential therapeutic strategy. Front. Immunol. 13, 779585. doi:10.3389/fimmu.2022.779585
Zhao, Y., Li, Y., Zhang, R., Wang, F., Wang, T., and Jiao, Y. (2020). The role of erastin in ferroptosis and its prospects in cancer therapy. Onco Targets Ther. 13, 5429–5441. doi:10.2147/OTT.S254995
Zheng, J., and Conrad, M. (2020). The metabolic underpinnings of ferroptosis. Cell Metab. 32 (6), 920–937. doi:10.1016/j.cmet.2020.10.011
Zhou, L. L., Guan, Q., Zhou, W., Kan, J. L., and Dong, Y. B. (2022). Ambient synthesis of an iminium-linked covalent organic framework for synergetic RNA interference and metabolic therapy of fibrosarcoma. Chem. Sci. 13 (26), 7846–7854. doi:10.1039/d2sc02297d
Zhou, X., Zheng, Y., Sun, W., Zhang, Z., Liu, J., Yang, W., et al. (2021). D-mannose alleviates osteoarthritis progression by inhibiting chondrocyte ferroptosis in a HIF-2α-dependent manner. Cell Prolif. 54 (11), e13134. doi:10.1111/cpr.13134
Zou, Y., Li, H., Graham, E. T., Deik, A. A., Eaton, J. K., Wang, W., et al. (2020). Cytochrome P450 oxidoreductase contributes to phospholipid peroxidation in ferroptosis. Nat. Chem. Biol. 16 (3), 302–309. doi:10.1038/s41589-020-0472-6
Zou, Y., Palte, M. J., Deik, A. A., Li, H., Eaton, J. K., Wang, W., et al. (2019). A GPX4-dependent cancer cell state underlies the clear-cell morphology and confers sensitivity to ferroptosis. Nat. Commun. 10 (1), 1617. doi:10.1038/s41467-019-09277-9
Glossary
GSH glutathione
GPX4 glutathione peroxidase 4 (GPX4)
PUFA polyunsaturated fatty acid
FSP1 ferroptosis suppressor protein 1
GCH1 GTP cyclohydrolase 1
ROS reactive oxygen species
HIF hypoxia-inducible factor
TfR transferrin receptor
DMT divalent metal transporter 1
NCOA4 nuclear receptor coactivator 4
DFO deferoxamine
SFAs saturated fatty acids
MUFAs monounsaturated fatty acids
AA arachidonic acid
ADA adrenic acid
ACSL4 acyl-CoA synthetase long-chain family member 4
LPCAT3 lysophosphatidylcholine acyltransferase 3
SLC7A1 solute carrier family 7 member 11
SLC3A2 solute carrier family 3 member 2
ESCRT endosomal sorting complex required for transport
UBIAD1 UBIA prenyltransferase domain-containing protein 1
CoQ10 coenzyme Q10
DHFR dihydrofolate reductase
POR P450 oxidoreductase
AMPK AMP-activated protein kinase
FPN ferroportin
OA osteoarthritis
MMPs matrix metalloproteinases
ECM extracellular matrix
MDA malondialdehyde
LPS lipopolysaccharide
NAMPT nicotinamide phosphoribosyl transferase
ASK1 apoptosis signal-regulating kinase 1
FtMt mitochondrial ferritin
HO-1 heme oxygenase-1
RA rheumatoid arthritis
FLS fibroblast-like synoviocytes
FTH1 ferritin heavy chain 1
TF serotransferrin
ZFP36 zinc finger protein 36.
Keywords: clinical implications, ferroptosis, musculoskeletal diseases, oxidative stress, therapeutic strategies
Citation: Zhang F, Yan Y, Cai Y, Liang Q, Liu Y, Peng B, Xu Z and Liu W (2023) Current insights into the functional roles of ferroptosis in musculoskeletal diseases and therapeutic implications. Front. Cell Dev. Biol. 11:1112751. doi: 10.3389/fcell.2023.1112751
Received: 01 December 2022; Accepted: 20 January 2023;
Published: 03 February 2023.
Edited by:
Markus Ritter, Paracelsus Medical University, AustriaCopyright © 2023 Zhang, Yan, Cai, Liang, Liu, Peng, Xu and Liu. This is an open-access article distributed under the terms of the Creative Commons Attribution License (CC BY). The use, distribution or reproduction in other forums is permitted, provided the original author(s) and the copyright owner(s) are credited and that the original publication in this journal is cited, in accordance with accepted academic practice. No use, distribution or reproduction is permitted which does not comply with these terms.
*Correspondence: Zhijie Xu, eHpqMTMyMjAwN0Bjc3UuZWR1LmNu; Wei Liu, MjAyMTIxMDIyMTE2ODdAc3R1LnVzYy5lZHUuY24=