- Institut d'Investigacions Biomèdiques August Pi i Sunyer (IDIBAPS), Gastroenterology Department, Centro de Investigación Biomédica en Red de Enfermedades Hepáticas y Digestivas (CIBEREHD), Hospital Clínic, Barcelona, Spain
The use of next-generation sequencing (NGS) technologies has been instrumental in the characterization of the mutational landscape of complex human diseases like cancer. But despite the enormous rise in the identification of disease candidate genetic variants, their functionality is yet to be fully elucidated in order to have a clear implication in patient care. Haploid human cell models have become the tool of choice for functional gene studies, since they only contain one copy of the genome and can therefore show the unmasked phenotype of genetic variants. Over the past few years, the human near-haploid cell line HAP1 has widely been consolidated as one of the favorite cell line models for functional genetic studies. Its rapid turnover coupled with the fact that only one allele needs to be modified in order to express the subsequent desired phenotype has made this human cell line a valuable tool for gene editing by CRISPR-Cas9 technologies. This review examines the recent uses of the HAP1 cell line model in functional genetic studies and high-throughput genetic screens using the CRISPR-Cas9 system. It covers its use in an attempt to develop new and relevant disease models to further elucidate gene function, and create new ways to understand the genetic basis of human diseases. We will cover the advantages and potential of the use of CRISPR-Cas9 technology on HAP1 to easily and efficiently study the functional interpretation of gene function and human single-nucleotide genetic variants of unknown significance identified through NGS technologies, and its implications for changes in clinical practice and patient care.
Introduction
A series of major and rapid scientific technical innovations over the past 20 years have enabled the advancement and refinement of DNA sequencing. This has opened a new window for better understanding the human genome and the genetic basis of human diseases. The rising need for high-throughput sequencing of whole genomes in any large quantity at lower costs, coupled with the development of potent and capable bioinformatic tools for data analysis, have propelled the implementation of NGS as a driving tool for translational medicine (Metzker, 2010; Beigh, 2016).
Joint efforts between scientists across the globe have made the complete sequencing of the Human Genome possible (Nurk et al., 2022). This has allowed the mapping of any sequence of interest and the identification of any existing polymorphic variants in a given genomic area. The routine implementation of high-throughput sequencing of exomes and whole genomes has provided a wealth of information on how our genes work to create a wondrous spectrum of phenotypes, including their variance across populations. NGS has enabled a deeper, but still minimal, understanding of gene function and their relationship with disease. It has allowed the discovery of many disease-causing genes and genes involved in disease predisposition (Lyon et al., 2012). This straightforward process of DNA sequencing is extremely successful in characterizing the causes of many clinical Mendelian disorders, where the mutation effect is dominant and the altered phenotype can be easily linked to the genomic change. On the other hand, complex diseases, caused by mutations in multiple genes and that have a less dominant effect, add a layer of difficulty to actually determine the implication a particular change has on a disease.
Next-generation sequencing and genome-engineering
The revolution in massive parallel sequencing has facilitated the discovery of hundreds of thousands of new single nucleotide polymorphisms (SNPs) associated with complex human diseases, like cancer. On these grounds, despite the undeniable power of NGS, there is an unquestionable bottleneck for analysis and interpretation of data. Identified genetic variants are mostly selected according to their rarity in the general population and in silico prediction tools. With this deluge of sequence data provided by NGS platforms there is a growing need to assess the functional impact of each variant to unequivocally associate mutations with disease predisposition, development, or progression. The unavailability to precisely edit genes had been a major roadblock to generating novel biological insights to develop new disease prevention therapeutic strategies (Wei et al., 2014; Bonjoch et al., 2019). In this sense, the advent of new easy-to handle human cellular models that enable a robust genomic editing in a high-throughput manner, is key to unravelling the functional consequences of SNP and their role in human diseases in order to have a clinical impact.
Nuclease-assisted gene-editing techniques have overcome this barrier and allow editing of any desired genomic site. The core techniques currently utilized for gene editing are clustered regularly interspaced short palindromic repeats (CRISPR)—CRISPR-associated protein 9 (Cas9)(CRISPR-Cas9), transcription activator-like effector nucleases (TALENs), zinc-finger nucleases (ZFN), and meganucleases (MegNs) (Gaj et al., 2016; Porteus, 2016; Khalil, 2020).
Genome engineering using the CRISPR-Cas system
The ground-breaking method in the genomic engineering field has been the CRISPR-Cas system. This adaptive ancient bacterial immune system recognizes DNA through RNA-DNA hybridization. The complex consists of a guide RNA (gRNA), complementary to the target DNA, and a Cas protein nuclease. There are different classes of Cas proteins (Makarova et al., 2018), but the current most commonly used is the type II nuclease Cas9, derived from Streptococcus pyogenes. Upon DNA recognition and binding, Cas9 nuclease domains are activated, generating DNA double-strand breaks (DBSs) and triggering the endogenous cellular DNA repair mechanisms (Jinek et al., 2012; Cong et al., 2013; Esvelt et al., 2013; Ran et al., 2013). Genome editing is achieved through the repair of the resulting DSBs. The cleaved DNA can be repaired through the error-prone, non-homologous end-joining (NHEJ) mechanism, or the homology-directed repair (HDR) pathway, which enables the introduction of specific point mutations or insertion of a desired sequence. Therefore, depending on the DNA repair pathway triggered, the CRISPR-Cas9 system can be used to generate gene knock-outs (KO) or gene knock-ins (KI).
Optimization and development of more precise strategies
Since its discovery and development, the CRISPR-Cas9 system has been improved to increase gene editing precision and efficiency. It has become the genome-editing technique of choice amongst other nuclease-based functional genomic approaches due to its unprecedented ease to modify the genome of a wide variety of organisms, from animals to plants.
Its incessant application in the field has resulted in its constant evolvement and optimization. A major hurdle still very much prevalent for all engineered nucleases is the risk of off-target effects, the acquisition of unintended changes at sites other than your target. This is a direct consequence of the ability of gRNA to bind DNA sequences with less than perfect complementarity (Hsu et al., 2013). One approach currently used to increase target specificity is the refinement in guide RNA engineering, RNA complex and guide selection. Through improving the gRNA spatial conformation and its ability to hybridize with target DNA, and the use of ameliorated engineered Cas9s it has been possible to substantially increase on-target activity and specificity (Mir et al., 2018). Tool websites for guide-design are available to automate the design of the best gRNA for your target DNA sequence, evaluating the guides according to their ON and OFF-target scores. Some examples of free online tools for guide and HDR template design can be found summarized in the Review from Cui et al. (2018).
CRISPR-Cas9 for gene knock-ins
In addition to generating knock-outs or indels, a more precise form of editing is a gene knock-in (insertion). As previously mentioned, KI rely on HDR where the cells repair DSB using a DNA template with the desired genetic change of interest. The DNA template is required to be homologous to the region immediately located around the DSB, and to be delivered simultaneously as either DNA or with the gRNA complexed with the Cas9, called ribonucleoprotein complexes (RNP). Therefore, coupled with the high specificity of CRISPR-Cas, DSB can be directed to a specific genomic site and the DNA donor sequence delivered used as template to precisely make various types of genome modifications, from single nucleotide substitutions to insertions of longer DNA sequences via HDR (Tyumentseva et al., 2021).
The ability to precisely and effectively introduce genetic changes into a host genome is invaluable to understand gene function and to model disease-causing mutations. Despite HDR-mediated repair being more accurate than NHEJ, its efficiency is usually very low compared to NHEJ-mediated DNA repair used to generate KO, hampering its development for clinical application (Petazzi et al., 2022).
To exploit the potential of CRISPR-Cas9 KI for clinical research, several approaches have been explored to improve HDR efficiency. From the use of chemical reagents that inhibit NHEJ or enhance HDR, such as KU-0060648 and RS-1 respectively (Chu et al., 2015; Maruyama et al., 2015; Robert et al., 2015; Yu et al., 2015; Song et al., 2016), to the use of drugs that transiently arrest cell cycle (Lin et al., 2014), since HDR only occurs in the late S and G2 phases of the cell cycle. The small molecule compound RS-1 enhanced the homologous recombination activity of hRAD51, and therefore enhanced HDR. On the other hand, KU-0060648 enriches for HDR-mediated repair events by inhibiting the DNA-PKcs pathway.
Base editors and prime editing
The discovery of new Cas proteins (Pickar-Oliver and Gersbach, 2019) and specially Cas9 protein engineering over the last few years, has diversified and improved CRISPR systems at high speed. Base editors were developed as DSB-free methods to introduce specific nucleotide changes, reducing unintended mutations and indels. By using a catalytically dead Cas9 (dCas9) or a Cas9 nickase (nCas9) coupled to deaminase enzymes, it is possible to make the direct conversion of one base to another relying on the base-excision repair (BER) system. Nowadays, cytidine base editors (CBE) (Komor et al., 2016), adenine base editors (ABE) (Gaudelli et al., 2017) and glycosylase base editors (GBE) (Zhao et al., 2021) allow C-to-T, A-to-G and C-to-G base changes, respectively. Although base editors have demonstrated a high on-target activity, they still have some limitations regarding base transversions and the editing window. In addition, such methods are unsuitable for introducing indel mutations.
One of the last cutting-edge CRISPR advances has been the Prime Editing (PE) methodology (Anzalone et al., 2019). PE utilizes an nCas9 fused with a reverse-transcriptase (RT) and an extended gRNA that encodes for both the primer binding site and the RT template sequence with the desired edits (pegRNA). The PE approach is more versatile than base editing since it allows all potential 12 nucleotide substitutions as well as modeling small indels, increasing the editing possibilities. PE has been a significant breakthrough in the gene editing field, but as with other CRISPR methods, there is still room for optimization and improvement at both pegRNA and enzymatic levels (Scholefield and Harrison, 2021).
Delivery systems of Cas9
Tremendous efforts have been devoted to improve the efficiency of the delivery of the CRISPR-Cas9 system. There are currently three main strategies to deliver the CRISPR-Cas9 system to edit genomes. The simplest and straight-forward is the delivery of plasmids containing vectors that encode for the Cas9 protein and the gRNA. Another approach is to deliver a mixture consisting of the gRNA and the mRNA of the Cas9. The third strategy is to directly deliver the Cas9-gRNA complex (Ran et al., 2013; Liu et al., 2017). Despite the plasmid-based CRISPR-Cas9 system being the most stable of the delivery systems, it still produces off-target effects, and its introduction to the nucleus of the cells remains challenging. On the other hand, direct delivery of the Cas9 mRNA and gRNA into the cytoplasm of the target cells reduces cytotoxicity, and most importantly, allows the transient expression of the Cas9 protein, which limits the duration of the genome-editing window, subsidizing off-target effects. The main obstacle of this strategy is the relatively poor stability of mRNA. Consequently, the most widely used strategy in recent years has been the direct delivery of RNP. Amongst the many advantages this strategy confers are its rapid action, high gene efficiency, and reduced toxicity and off-target effects (Liu et al., 2017). Upon delivery to the cell’s nucleus, the RNP complex is instantly functional without the need of transcription or translation. With RNP delivery, risks of spontaneous genome integration disappear and the short active window of the complex before degradation greatly reduces off-target effects (Schumann et al., 2015).
Various physical and non-viral delivery approaches have been extensively exploited to deliver the CRISPR-Cas9 system to target cells. Amongst the most widely used are hydrodynamic injections, electroporation, nucleofection, and nanoparticles (Gori et al., 2015; Qin et al., 2015; Zuris et al., 2015; Tyumentseva et al., 2021). The efficiency of viral vectors to deliver the plasmid-based CRISPR system is compromised by its safety, a concern that is greatly reduced when using non-viral vectors. Overall, over the past decade, tremendous efforts have been made to increase the efficiency of the system and reduce off-target effects to successfully use the technique for biological research.
Genome editing in functional studies
The development of precise and efficient genome-editing technologies has paved the way for the deciphering of the biological relevance of many genetic variants identified so far. The ability to modify genes to the precise nucleotide has made it possible for researchers to manipulate human genomes in an attempt to recapitulate particular diseases to understand their biological basis in health and disease. It is not only possible to do that on the human genome, but several model organisms have been used to functionally assess genes.
Yeast research has been pivotal in the establishment of the conceptual framework for systematic genetics, which has provided a view on the genetic landscape and molecular organization of a cell (Costanzo et al., 2010). The use of yeast, a haploid organism, enabled many advances in the field of genetics, allowing the discovery and classification of essential and non-essential genes through the performance of systematic genetic knock-out studies. Although gene essentiality is tissue and cell dependent, the characterization of such gene functionality was of extreme importance for future advancement in genetic studies (Giaever et al., 2002; Hillenmeyer et al., 2008; Ramani et al., 2012). This distinction in gene essentiality according to cell type is extremely relevant in humans. The ability to discern genes that are essential for human diseases, being genes that either drive the initiation, or progression of the disease is of utmost importance to develop clinically-relevant management strategies based on these specific genomic changes (Hart et al., 2015).
Despite technological advancements the main challenges that remain are the main research models utilized to recapitulate the genetic background of human diseases. In recent years, several attempts have been made to create relevant haploid human cell models to propel the genetic engineering field for biological research to the next level by creating relevant human models where gene function could be studied and exploited in a human-disease context.
Haploid human cell line HAP1
HAP1 is a near-haploid human cell line derived from KBM7, a human myeloid leukemia cell line developed from a 39-year-old male patient in the blastic phase of chronic myeloid leukemia (Andersson et al., 1987). KBM7 was a mixed ploidy line, constituted by two predominant but distinct near-haploid cell populations with disomies for chromosomes 8 and 8 and 15, and a hyper-diploid one. Its cytogenetic analysis revealed that all subclones had lost chromosome Y and carried the Philadelphia translocation, t (9q;22q), characteristic of myeloid leukemias (Andersson et al., 1995; Kotecki et al., 1999). Higher ploidy clones presented a lower doubling time than their near-haploid counterparts. Upon further cultivation, cells with greater DNA content quickly overgrew the near-haploid cells and became dominant in culture (Andersson et al., 1995). From the mixed ploidy line, a haploid stable subclone, except for a disomy of chromosome 8, was isolated and further stably cultured over several months (Kotecki et al., 1999), becoming the first human (near) haploid cell line model, known nowadays as KBM7. Since then, the near haploidy of KBM7 has been widely exploited to perform gene function studies, since baring a single copy of the genome unravels the effect of any given mutation (Li and Shuai, 2017).
In an attempt by Carette et al. to induce KBM7 cell’s pluripotency by expression of OCT4, SOX-2, c-MYC and KLF411, subclones with only one subset of chromosomes were obtained (Takahashi and Yamanaka, 2006; Carette et al., 2011). This set of subclones, termed HAP1, retained the Philadelphia chromosome, no longer expressed hematopoietic markers and, unlike KBM7, grew adherently. Spectral karyotyping analysis of HAP1 by Essletzbichler et al. revealed a fusion of a 30-megabse fragment of Chromosome 15 to Chromosome 19. They efficiently employed CRISPR-Cas9 genome engineering technology to excise the disomic region from Chromosome 15, acquiring the first fully haploid human cell line, eHAP1 (Essletzbichler et al., 2014). A few advantages HAP1 confers apart from a haploid karyotype are a rapid doubling time, sustained growth at single dilutions, and ease of transfection.
In biomedical research studies, the potential of an in vitro cell line used as a model system depends on its ability to maintain genome stability. Genomic disparities observed between “control” and ‘treated’ cells need to be a direct consequence of the effect of your target treatment and not off-target cytogenetic mutations. Something to take into consideration when working with HAP1 is their ploidy instability, a long-recognized trait of all haploid mammalian cells (Horii and Hatada, 2015). As happened with KBM7, HAP1 was karyotypically stable in culture for up to approximately 20 passages, further culturing reduced the percentage of haploid cells in culture, which was enriched in diploid cells. This phenomenon has long been considered as spontaneous diploidization (Egli et al., 2011; Leeb et al., 2014; Yilmaz et al., 2016; Sagi & Benvenisty, 2017; Banerjee et al., 2022), but it has recently been shown that the growth properties and reduced fitness of haploid cells is what is a disadvantage amongst diploid cells present in culture. Data on HAP1 from Olbrich et al. showed that the conversion from haploid to diploid is an infrequent event, but upon occurrence, the better growth properties of diploid cells allow them to overtake the culture. The reduced fitness is in part explained by the triggering of the p53-dependent cytotoxic response which limits the fitness of mammalian cells (Olbrich et al., 2017).
To account for such differences it is crucial to ensure HAP1 cell ploidy status. Some fundamental differences between haploid and diploid HAP1 have been described (Olbrich et al., 2017, 2019; Beigl et al., 2020), hence the underlying importance of controlling the karyotype of the cells before experimentation and phenotypic characterization. If used for genetic engineering, haploid HAP1 single-cell subclones have been found to be more ploidy stable (Olbrich et al., 2017). In order to minimize interfering variables in gene function studies, it is recommended to perform haploid HAP1 cell sorting by flow cytometry (Beigl et al., 2020). An alternative haploidy selection method would be the administration of the chemical compound 10-Deacetylbaccatin-III (DAB) and Diclazuril, which have been proven capable of selecting haploidy in HAP1 cells (Olbrich et al., 2019). Olbrich et al. performed a phenotypic chemical screen in HAP1 cell line to identify compounds capable of selecting cells with lower ploidy in mixed cultures. The authors identified DAB and Diclazuril, and further studied their mechanistic effect. The compounds were found to affect the microtubule dynamics of interphase cells, extending the duration of mitosis. While haploid cells easily overcame the mitotic arrest, higher ploidy cells presented very prolonged arrests followed by cell death. Therefore, the compounds were shown to facilitate the maintenance of haploid cells by delaying the formation of the metaphase palate. (Olbrich et al., 2019).
Recently, Banerjee et al. (2022) performed a detailed analysis of cytogenetic characterization of HAP1 cell lines using the single cell-based assay M-FISH karyotyping. The group karyotypically characterized 19 CRISPR-Cas9 engineered HAP1 cells to assess their rates of chromosomal instability and explore their possible underlying causes. Sixteen out of 19 cell lines with passage numbers ranging from 10 to 35 revealed a diploidy percentage range of 2%–35%, results in accordance with a previous study from Beigl et al. (2020) which show that HAP1 cell cultures are haploidy-stable for at least 20 passages. These recent studies highlight the importance of using cell lines at low passage for biomedical research and the implementation of routine karyotype controls to avoid accumulation of mutations that can induce further genome instability.
The inherent ability of HAP1 to diploidize is one of the tremendous advantages of using HAP1. It has so far been proven to not affect engineered cells and therefore entailing the conversion of haploid into diploid human cells representing homozygotes for the genetic change of interest (Drazic et al., 2018; Oishi et al., 2018).
Functional studies using HAP1
HAP1 is an easy and amenable model to use for research. Since its establishment, the haploid HAP1 human cell line, along with its mother cell line, has been used for a wide array of studies across fields, from cell biology to virology, metabolism, apoptosis, drug testing, genome integrity, and functional genomics to name a few (Agrotis et al., 2019; Depetter et al., 2019; Guiducci et al., 2019; Moskovskich et al., 2019; Nixon et al., 2019). A summary of studies using HAP1 is found in Table 1. The primary advantage of haploid human cell lines is the fact that genes are hemizygous and therefore, upon mutations, phenotypes are immediately exposed which enables to study the effect of specific mutations in a human-related context (Wutz, 2014). Its second most precious attribute is its doubling time, at around 48 h, and ability to efficiently grow as single-cells, which enables the rapid cultivation of the cells for experimentation.
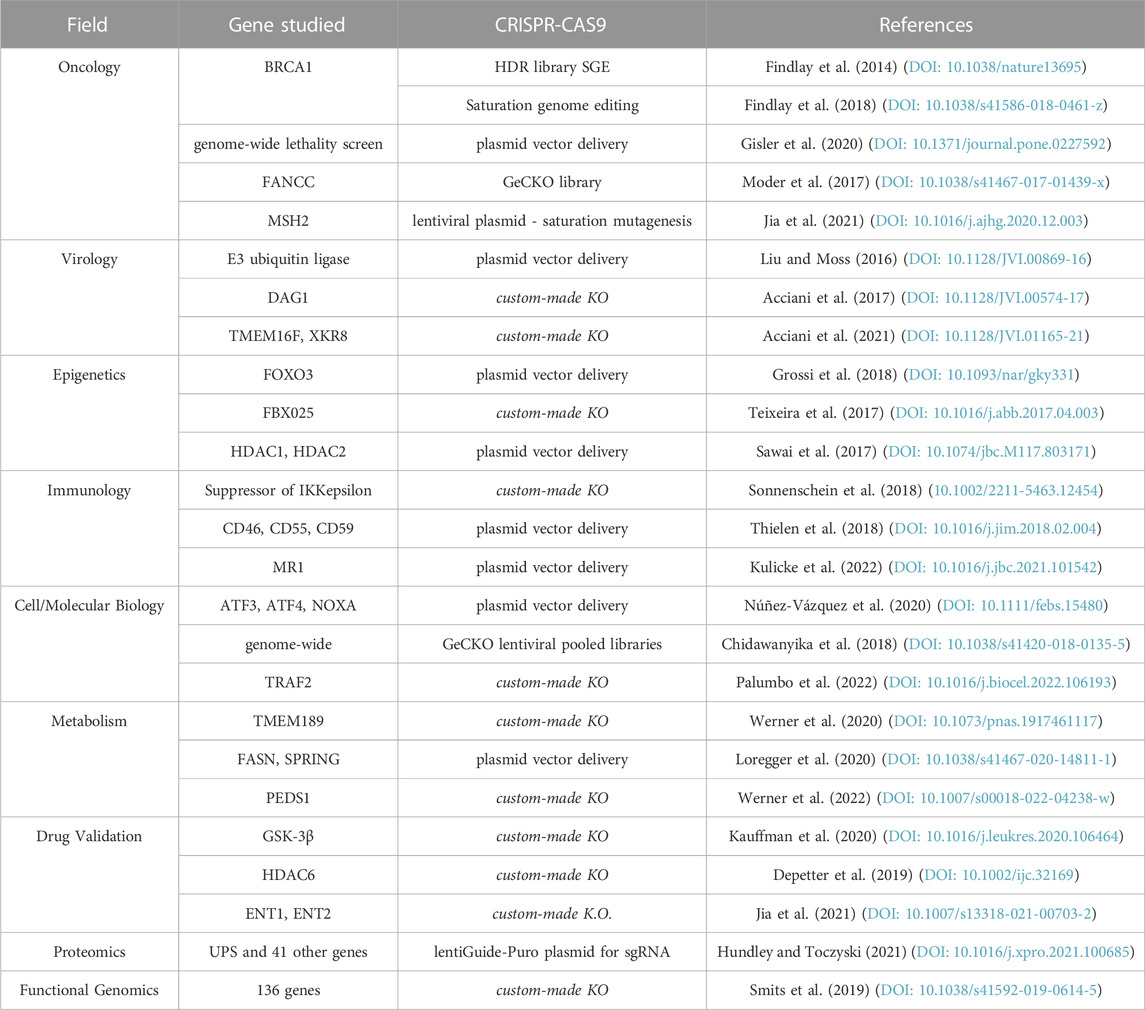
TABLE 1. Summary table of functional studies using HAP1. Summary of some of the genes studied using the haploid human cell line model HAP1, classified by field, gene studied and method of CRISPR-Cas9. The full table can be found in Supplementary Table S1.
The first use of human haploid cell lines consisted of forward genetic screens using KBM7. For the first time a human haploid cell line was easing the performance of biological research to study the functionality of human genes. The first haploid genetic screen in human cells used KBM7 to develop a screening method to generate null alleles. Insertional mutagenesis was chosen as the approach of interest to inactivate genes due to its high level of mutagenicity across species, and because the DNA sequences integrated in the genome can be used as tags to identify the disrupted gene (Carette et al., 2009). As previously mentioned, subsequent studies using haploid genetic screens by Carette et al. (2011) led to the generation of HAP1 as a failed attempt to induce pluripotency in KBM7. The first genome wide study using HAP1 was conducted using a retroviral gene-trap vector to mutagenize early-passage HAP1 cells, which enabled the identification of essential host factors for the entry pathway of Ebola virus. With the ease haploidy confers for forward genetic experiments, as only one allele needs to be mutated, another approach performed using HAP1 enabled the identification of essential factors for Lassa viral infection (Jae et al., 2014).
Due to their said relative rapid growth and ease of transfection, HAP1 cells have been used extensively in CRISPR-Cas9 screens. Due to their great amenability, several researchers have exploited HAP1s’ culture properties to conduct high-throughput functional screens to enhance their study’s potential. To facilitate the wide application of HAP1, some researchers have developed protocols to use HAP1 as a cell model to perform various gene-editing studies. Lackner et al. (2015) developed a protocol for gene tagging based on CRISPR-Cas9 using HAP1, allowing the authors to establish a strategy to endogenously tag genes at both the N and C terminus, without the integration of adjacent plasmid sequence. More recently, Hundley and others developed a detailed protocol to perform high-throughput system-specific chemical-genetic CRISPR-Cas9 screens in the human HAP1 cell line using a pathway-specific library or sub-genomic library (Hundley and Toczyski, 2021).
Studies using CRISPR-Cas9 system to edit HAP1
The exuberant development of genome-editing techniques has revolutionized research on the human genome, allowing the generation and introduction of genetic variants in a wide variety of cell models for their functional characterization at a great speed. General approaches to generating informative functional data are still too slow and expensive to meet the growing need. The possibility to apply the CRISPR-Cas9 technique to precisely edit the genome at the nucleotide level offers a new avenue in research to start functionally characterizing mutations relevant to complex human diseases like cancer. Multiplex assays of variant effect allow the engineering and testing of variants in a pooled format, making them highly scalable, drastically reducing cost and minimizing sample processing (Gasperini et al., 2016; Weile and Roth, 2018; Findlay, 2021).
In the past decade, HAP1 has been widely established as a cell line model for a broad range of gene-editing studies, from forward genetic screens to high-throughput CRISPR-Cas9 screens. Despite being a leukemia cell line, it has become the cell model of choice across different scientific fields due to its ease of transfection and acquisition of single-cell clones, which allows results in a time-efficient manner. In addition, the utilization of the CRISPR-Cas9 system on a haploid human cell model like HAP1 can facilitate the gene editing process and increase editing efficiencies, since only one allele needs to be modified. A few examples of studies using HAP1 are summarized below.
Oncology
Back in 2014, Findlay and others demonstrated the feasibility of generating and functionally analyzing thousands of variants of programmed genome edits in a single experiment using saturation mutagenesis in HAP1. The authors coupled CRISPR/Cas9 RNA-guided cleavage with multiplex HDR using a complex library of donor templates to assess all possible SNVs so far identified on exon 18 of BRCA1 in breast cancer (Findlay et al., 2014). A of couple years later the group presented saturation genome editing (SGE) as an innovative approach to assess the pathogenicity of BRCA1 variants. The researchers used HAP1 to assess the clinical significance of nearly 4000 SNV in 13 exons encoding functionally crucial domains in BRCA1 (Findlay et al., 2018). Similarly, Radford et al. used HAP1 to perform SGE of DDX3X gene to study the functional impact of 12776 SNV (Radford et al., 2022). In an attempt to prospectively and systematically measure the functional impact of missense variants in MSH2, one of the major Lynch syndrome genes, Jia et al. (2021) performed a massively parallel screen in human cells (including HAP1) to identify loss-of-function (LOF) missense variants in the DNA mismatch repair factor MSH2.
The CRISPR-based alternative technologies base and prime editing have emerged as tools to continue to improve the creation of programmed variants. Recently, the first large-scale base editor screens were published using HAP1, where Hanna et al. (2021) assessed more than 52,000 variants enabling the discovery of LOF variants in BRCA1 and BRCA2 genes and mapping protein residues where variants confer sensitivity or resistance to targeted therapies. In a similar high-throughput manner, BE was used in HAP1 to create missense variants across 86 DNA damage response genes, discovering functionally critical loss- and gain-of-function protein domains and providing evidence for reclassification of variants of uncertain significance for breast cancer (Cuella-Martin et al., 2021).
For a more therapeutic target based approach, Billaud et al. developed a rapid functional assay using HAP1 to characterize the functional implication of variants in tumor suppressor genes BRCA1, BRCA2 and POLE to patients’ response to therapy (Billaud et al., 2021). Alternatively, Neggers et al. created a CRISPR-mediated NHEJ repair genetic screening approach to rapidly derive and identify drug-resistance mutations in essential genes (gain of function mutations) (Neggers et al., 2018). To study the role of kinase deregulation in tumor initiation and/or progression, Robinson-Garcia et al. developed a CRISPR-Cas9 systematic approach to identify synthetic lethal interactions for kinase deficiencies to different DNA-damaging chemotherapeutic agents using HAP1 (Robinson-Garcia et al., 2019). Another technique to exploit synthetic lethality in HAP1 was conducted by Gisler et al., by performing a genome-wide screen for gene disruptors that could result in resistance to pharmacological inhibition of BMI1 on HAP1 by exposing mutagenized HAP1 to low concentrations of the BMI1-inhibitor PTC-318 (Gisler et al., 2020).
Other diseases in the field of oncology have also benefited from the scalability of experiments when performed with HAP1. Moder et al. introduced an approach to systematically study synthetic viability using HAP1, where a genome-wide screen was performed on cells with LOF for the Fanconi anemia complementation group C (FANCC), and used insertional mutagenesis coupled with genome-wide CRISPR libraries to identify genetic suppressor interactions for Fanconi anemia (Moder et al., 2017). In a similar way, a genome wide genetic screen on HAP1 was conducted to uncover tumor-intrinsic genes that regulate tumor killing by major histocompatibility complex-unrestricted cytotoxic lymphocytes (Menasche et al., 2020).
Virology
In the virology field Guedán et al. also exploited HAP1 haploidy to generate knock-outs for PKD1 and PKD2 genes using CRISPR-Cas9 to study Human Rhinovirus replication. Although the authors did not identify the molecular mechanisms by which PKD regulates viral replication, the use of HAP1 PKD knock-out cell lines allowed the study of small-molecule inhibitors targeting PKD, suggesting it as a novel antiviral target for drug discovery (Guedán et al., 2017).
Immunology
In the immunology field, Sonnenschein et al. used HAP1 to create CRISPR-Cas9 knockout cells to study the type I interferon response of the innate immune system. The authors wanted to study the interaction between the suppressor of IKKepsilon (SIKE) and TANK-binding kinase 1, associated with the activation of interferon regulatory factors to initiate a type I interferon response. Using the CRISPR-cas9 system, expression of SIKE was knocked out in HAP1 to investigate the protein interactions of SIKE during the innate immune response, which enabled the identification of the direct interaction of SIKE with the cytoskeleton of cells to promote cytoskeletal rearrangements to initiate cell movement either for phagocytosis or migration (Sonnenschein et al., 2018).
The complement system is a part of the immune system that plays a pivotal role in the innate defence against pathogens and host homeostasis. The complement is initiated upon sensing danger signals that are caused by confirmational changes of molecular complexes, initiating a tightly regulated cascade of enzymatic reactions to modulate the adaptative immune response and regulate host homeostasis. Thielen et al. generated knockout HAP1 cells for the complement regulatory proteins CD46, CD55 and CD59 to investigate the complement activation and regulation of human cells (Thielen et al., 2018).
Epigenetics
HAP1 has also been used in the epigenetic field to study SUMOylation. Important cellular processes like transcription, nuclear transport and maintenance of genome integrity are regulated by the reversible post-translational modification of SUMOylation. Rodríguez-Castañeda et al. used HAP1 to generate CRISPR-Cas9 knock-outs for SENP1 and CHD3 genes by generating frameshift mutations in the coding region of each gene. They reported a novel level of coordinated gene expression control between SENP1 and CHD3 (Rodríguez-Castañeda et al., 2018).
Cell biology
In a more high-throughput manner Chidawanyika et al. conducted a genome-wide CRISPR-Cas9 loss of function screen against HAP1 cells to study the stress-induced endoplasmatic reticulum (ER) cell death. With the aim to identify genes essential for cell death induced by the classical pharmacological ER stressors thapsigargin, tunicamycin, and brefeldin, the authors used pooled CRISPR-Cas9 human libraries to perform thorough and unbiased loss-of function screen against thapsigargin, tunicamycin, and brefeldin. With this approach the authors were able to identify a novel essential gene, SEC24A, for thapsigargin-induced cytotoxicity (Chidawanyika et al., 2018).
Researchers studying the translational apparatus developed a novel method for targeting one of the two dedicated RNA components of the re-coding machinery, the selenocysteine-tRNA. In their study, Vindry et al. developed a novel CRISPR strategy based on Cas9-sgRNA ribonucleoproteins loaded with murine leukaemia virus-like particles (VLPs) to inactivate the Sec-tRNA gene in human cell lines, HAP1 amongst them. The authors successfully reduced selenoprotein expression by the development of CRISPR-Cas9-VLP, important for regulating the activation of selenoproteins (Vindry et al., 2019).
Metabolism
In the metabolism field, HAP1 has also been used to study calcium activity in eukaryotic cellular processes. Karakus et al. used HAP1 to functionally assess for the first time the molecular function of a newly identified negative regulator of intracellular calcium signalling, SLC10A7. The authors used SLC10A7 KO HAP1 cells to functionally study the gene and its genomic variants to further clarify the pathogenesis of SLC10A7-associated diseases (Karakus et al., 2020).
The wide range of studies from various areas in biological research referenced here using HAP1 are proof for the broad applicability of HAP1 for genome engineering studies. Researchers face the challenge to recreate human disease in animal models, and such a thing is in itself extremely laborious. As technical innovations help improve the methods which researchers use to better understand human diseases, new more amendable human cell models are being generated to better recapitulate human diseases for their deeper understanding. In this case, as previously mentioned, the major benefit of HAP1 is its stable haploid karyotype, which only requires the modification of only one allele to study the effect of your mutation/change of interest. With its spontaneous diploidization over subsequent culturing, the edited allele will duplicate and give rise to the diploid edited state. The power of haploid human cells can aid in the functional validation of many variants that have been identified through NGS, and help prove their functional roles as well as implication in disease. Nevertheless, some drawbacks should also be considered. As with most cell lines of tumor origin, the HAP1 genetic background must be taken into account when studying certain cell functions or molecular pathways, such as the already described TP53 alteration (Moder et al., 2017). Also, its ploidy instability could be a limitation for prolonged in-vitro cell culturing, such as assessing the mutational burden over time when focusing on those genes involved in DNA damage and repair pathways.
Conclusion
In this review, the use of HAP1 in the recent years to perform genome-engineering using the CRISPR-Cas9 system has been explored. All the studies cited here demonstrate the power of CRISPR-Cas9 on HAP1 to study the functional consequences of genomic alterations. Again, researchers need powerful and relevant human models to study diseases. The haploidy of HAP1 offers a tremendous advantage amongst diploid human cells when generating genetic variants to elucidate the implication of specific genomic changes in the development and/or progression of diseases. With more precise genome engineering techniques and the use of relevant human cell models, researchers will be able to precisely recapitulate human diseases. Models will enable to study the function of such genomic changes with the aim to identify clinically-relevant molecular events related to disease to develop new management strategies based on these specific genomic changes.
Author contributions
The idea of the review was conceived and planned by all authors. GL-S took the lead in writing the manuscript. LB provided critical feedback and helped writing and shaping the final version of the review. SC-B supervised and checked the manuscript editing process.
Funding
This research was supported by grants from Fundación Científica de la Asociación Española contra el Cáncer (PRYGN211085CAST), Fundació La Marató de TV3 (2019-202008-10), Fondo de Investigación Sanitaria/FEDER (17/00878, 20/00113), CERCA Program (Generalitat de Catalunya) and Agència de Gestió d'Ajuts Universitaris i de Recerca (Generalitat de Catalunya, GRPRE 2017SGR21). CIBEREHD is funded by the Instituto de Salud Carlos III.
Acknowledgments
The work was carried out (in part) at the Esther Koplowitz Centre.
Conflict of interest
The authors declare that the research was conducted in the absence of any commercial or financial relationships that could be construed as a potential conflict of interest.
Publisher’s note
All claims expressed in this article are solely those of the authors and do not necessarily represent those of their affiliated organizations, or those of the publisher, the editors and the reviewers. Any product that may be evaluated in this article, or claim that may be made by its manufacturer, is not guaranteed or endorsed by the publisher.
Supplementary material
The Supplementary Material for this article can be found online at: https://www.frontiersin.org/articles/10.3389/fcell.2023.1111488/full#supplementary-material
Supplementary Table S1 | Summary table of the genes studied using HAP1 since its establishment.
References
Acciani, M., Alston, J. T., Zhao, G., Reynolds, H., Ali, A. M., Xu, B., et al. (2017). Mutational analysis of lassa virus glycoprotein highlights regions required for alpha-dystroglycan utilization. J. Virol., 91 (18), e00574–17. doi:10.1128/JVI.00574-17
Acciani, M. D., Mendoza, M. F. L., Havranek, K. E., Duncan, A. M., Iyer, H., Linn, O. L., et al. (2021). Ebola virus requires phosphatidylserine scrambling activity for efficient budding and optimal infectivity. J. Virol., 95 (20), e01165–21. doi:10.1128/JVI.01165-21
Agrotis, A., Pengo, N., Burden, J. J., and Ketteler, R. (2019). Redundancy of human ATG4 protease isoforms in autophagy and LC3/GABARAP processing revealed in cells. Autophagy 15 (6), 976–997. doi:10.1080/15548627.2019.1569925
Andersson, B. S., Beran, M., Pathak, S., Goodacre, A., Barlogie, B., and Mccredie, K. B. (1987). Ph-positive chronic myeloid leukemia with near-haploid conversion in vivo and establishment of a continuously growing cell line with similar cytogenetic pattern. Cancer Genet. Cytogenet. 24, 335–343. doi:10.1016/0165-4608(87)90116-6
Andersson, B. S., Collins, V. P., Kurzrock, R., Larkin, D. W., Childs, C., Ost, A., et al. (1995). KBM-7, a human myeloid leukemia cell line with double Philadelphia chromosomes lacking normal c-ABL and BCR transcripts. Leukemia 9 (12), 2100–2108.
Anzalone, A., Randolph, P. B., Davis, J. R., Sousa, A. A., Koblan, L. W., Levy, J. M., et al. (2019). Search-and-replace genome editing without double-strand breaks or donor DNA. Nature 576 (7785), 149–157. doi:10.1038/s41586-019-1711-4
Banerjee, R., Sotero-Caio, C. G., Fu, B., and Yang, F. (2022). Chromosomal instability (CIN) in HAP1 cell lines revealed by multiplex fluorescence in situ hybridisation (M-FISH). Mol. Cytogenet. 15 (1), 46. doi:10.1186/s13039-022-00625-x
Beigh, M. (2016). Next-generation sequencing: The translational medicine approach from “bench to bedside to population. Medicines 3 (2), 14. doi:10.3390/medicines3020014
Beigl, T. B., Kjosås, I., Seljeseth, E., Glomnes, N., and Aksnes, H. (2020). Efficient and crucial quality control of HAP1 cell ploidy status. Biol. Open 9, bio057174. doi:10.1242/bio.057174
Billaud, A., Chevalier, L. M., Augereau, P., Frenel, J. S., Passot, C., Campone, M., et al. (2021). Functional pre-therapeutic evaluation by genome editing of variants of uncertain significance of essential tumor suppressor genes. Genome Med. 13, 174. doi:10.1186/s13073-021-00976-x
Bonjoch, L., Mur, P., Arnau-Collell, C., Vargas-Parra, G., Shamloo, B., Franch-Expósito, S., et al. (2019). Approaches to functionally validate candidate genetic variants involved in colorectal cancer predisposition. Mol. Aspects Med. 69, 27–40. doi:10.1016/j.mam.2019.03.004
Carette, J. E., Guimaraes, C. P., Varadarajan, M., Park, A. S., Wuethrich, I., Godarova, A., et al. (2009). Haploid genetic screens in human cells identify host factors used by pathogens. Science 326, 1231–1235. doi:10.1126/science.1178955
Carette, J. E., Guimaraes, C. P., Wuethrich, I., Blomen, V. A., Varadarajan, M., Sun, C., et al. (2011). Global gene disruption in human cells to assign genes to phenotypes by deep sequencing. Nat. Biotechnol. 29 (6), 542–546. doi:10.1038/nbt.1857
Chidawanyika, T., Sergison, E., Cole, M., Mark, K., and Supattapone, S. (2018). SEC24A identified as an essential mediator of thapsigargin-induced cell death in a genome-wide CRISPR/Cas9 screen. Cell Death Discov. 4 (1), 115. doi:10.1038/s41420-018-0135-5
Chu, V. T., Weber, T., Wefers, B., Wurst, W., Sander, S., Rajewsky, K., et al. (2015). Increasing the efficiency of homology-directed repair for CRISPR-Cas9-induced precise gene editing in mammalian cells. Nat. Biotechnol. 33 (5), 543–548. doi:10.1038/nbt.3198
Cong, L., Ran, F. A., Cox, D., Lin, S., Barretto, R., Habib, N., et al. (2013). Multiplex genome engineering using CRISPR/Cas systems. Science 339 (6121), 819–823. doi:10.1126/science.1231143
Costanzo, M., Baryshnikova, A., Bellay, J., Kim, Y., Spear, E. D., Sevier, C. S., et al. (2010). The genetic landscape of a cell. Science 327 (5964), 425–431. doi:10.1126/science.1180823
Cuella-Martin, R., Hayward, S. B., Fan, X., Chen, X., Huang, J. W., Taglialatela, A., et al. (2021). Functional interrogation of DNA damage response variants with base editing screens. Cell 184 (4), 1081–1097.e19. doi:10.1016/j.cell.2021.01.041
Cui, Y., Xu, J., Cheng, M., Liao, X., and Peng, S. (2018). Review of CRISPR/Cas9 sgRNA design tools. Interdiscip. Sci. Comput. Life Sci. 10 (2), 455–465. doi:10.1007/s12539-018-0298-z
Depetter, Y., Geurs, S., de Vreese, R., Goethals, S., Vandoorn, E., Laevens, A., et al. (2019). Selective pharmacological inhibitors of HDAC6 reveal biochemical activity but functional tolerance in cancer models. Int. J. Cancer 145 (3), 735–747. doi:10.1002/ijc.32169
Drazic, A., Aksnes, H., Marie, M., Boczkowska, M., Varland, S., Timmerman, E., et al. (2018). NAA80 is actin’s N-terminal acetyltransferase and regulates cytoskeleton assembly and cell motility. Proc. Natl. Acad. Sci. 115 (17), 4399–4404. doi:10.1073/pnas.1718336115
Egli, D., Chen, A., Saphier, G., Powers, D., Alper, M., Katz, K., et al. (2011). Impracticality of egg donor recruitment in the absence of compensation. Cell Stem Cell 9, 293–294. doi:10.1016/j.stem.2011.08.002
Essletzbichler, P., Konopka, T., Santoro, F., Chen, D., Gapp, B., Kralovics, R., et al. (2014). Megabase-scale deletion using CRISPR/Cas9 to generate a fully haploid human cell line. Genome Res. 24 (12), 2059–2065. doi:10.1101/gr.177220.114
Esvelt, K. M., Mali, P., Braff, J. L., Moosburner, M., Yaung, S. J., and Church, G. M. (2013). Orthogonal Cas9 proteins for RNA-guided gene regulation and editing. Nat. Methods 10 (11), 1116–1121. doi:10.1038/nmeth.2681
Findlay, G. M. (2021). Linking genome variants to disease: Scalable approaches to test the functional impact of human mutations. Hum. Mol. Genet. 30, R187–R197. doi:10.1093/hmg/ddab219
Findlay, G. M., Boyle, E. A., Hause, R. J., Klein, J. C., and Shendure, J. (2014). Saturation editing of genomic regions by multiplex homology-directed repair. Nature 513 (7516), 120–123. doi:10.1038/nature13695
Findlay, G. M., Daza, R. M., Martin, B., Zhang, M. D., Leith, A. P., Gasperini, M., et al. (2018). Accurate classification of BRCA1 variants with saturation genome editing. Nature 562 (7726), 217–222. doi:10.1038/s41586-018-0461-z
Gaj, T., Sirk, S. J., Shui, S. L., and Liu, J. (2016). Genome-editing technologies: Principles and applications. Cold Spring Harb. Perspect. Biol. 8, a023754. doi:10.1101/cshperspect.a023754
Gasperini, M., Starita, L., and Shendure, J. (2016). The power of multiplexed functional analysis of genetic variants. Nat. Protoc. 11 (10), 1782–1787. doi:10.1038/nprot.2016.135
Gaudelli, N. M., Komor, A. C., Rees, H. A., Packer, M. S., Badran, A. H., Bryson, D. I., et al. (2017). Programmable base editing of A•T to G•C in genomic DNA without DNA cleavage. Nature 551 (7681), 464–471. doi:10.1038/nature24644
Giaever, G., Chu, A. M., Ni, L., Connelly, C., Riles, L., and Johnston, M. (2002). Functional profiling of the Saccharomyces cerevisiae genome. Nature 418, 387–391. doi:10.1038/nature00935
Gisler, S., Maia, A. R. R., Chandrasekaran, G., Kopparam, J., and van Lohuizen, M. (2020). A genome-wide enrichment screen identifies NUMA1-loss as a resistance mechanism against mitotic cell-death induced by BMI1 inhibition. PLoS ONE 15, e0227592. doi:10.1371/journal.pone.0227592
Gori, J. L., Hsu, P. D., Maeder, M. L., Shen, S., Welstead, G. G., and Bumcrot, D. (2015). Delivery and specificity of CRISPR/Cas9 genome editing technologies for human gene therapy. Hum. Gene Ther. 26 (7), 443–451. doi:10.1089/hum.2015.074
Grossi, V., Forte, G., Sanese, P., Peserico, A., Tezil, T., Lepore Signorile, M., et al. (2018). The longevity SNP rs2802292 uncovered: HSF1 activates stress-dependent expression of FOXO3 through an intronic enhancer. Nucleic Acids Res. 46 (11), 5587–5600. doi:10.1093/nar/gky331
Guedán, A., Swieboda, D., Charles, M., Toussaint, M., Johnston, S. L., Asfor, A., et al. (2017). Investigation of the role of protein kinase D in human Rhinovirus replication. J. Virology 91, e00217. doi:10.1128/JVI.00217-17
Guiducci, G., Paone, A., Tramonti, A., Giardina, G., Rinaldo, S., Bouzidi, A., et al. (2019). The moonlighting RNA-binding activity of cytosolic serine hydroxymethyltransferase contributes to control compartmentalization of serine metabolism. Nucleic Acids Res. 47 (8), 4240–4254. doi:10.1093/nar/gkz129
Hanna, R. E., Hegde, M., Fagre, C. R., DeWeirdt, P. C., Sangree, A. K., Szegletes, Z., et al. (2021). Massively parallel assessment of human variants with base editor screens. Cell 184 (4), 1064–1080.e20. doi:10.1016/j.cell.2021.01.012
Hart, T., Chandrashekhar, M., Aregger, M., Steinhart, Z., Brown, K. R., MacLeod, G., et al. (2015). High-resolution CRISPR screens reveal fitness genes and genotype-specific cancer liabilities. Cell 163 (6), 1515–1526. doi:10.1016/j.cell.2015.11.015
Hillenmeyer, M. E., Fung, E., Wildenhain, J., Pierce, S. E., Hoon, S., Lee, W., et al. (2008). The chemical genomic portrait of yeast: Uncovering a phenotype for all genes. Science 320 (5874), 362–365. doi:10.1126/science.1150021
Horii, T., and Hatada, I. (2015). Genome editing using mammalian haploid cells. Int. J. Mol. Sci. 16, 23604–23614. doi:10.3390/ijms161023604
Hsu, P. D., Scott, D. A., Weinstein, J. A., Ran, F. A., Konermann, S., Agarwala, V., et al. (2013). DNA targeting specificity of RNA-guided Cas9 nucleases. Nat. Biotechnol. 31 (9), 827–832. doi:10.1038/nbt.2647
Hundley, F., and Toczyski, D. P. (2021). Chemical-genetic CRISPR-Cas9 screens in human cells using a pathway-specific library. Star. Protoc. 2, 100685. doi:10.1016/j.xpro.2021.100685
Jae, L. T., Raaben, M., Herbert, A. S., Kuehne, A. I., Wirchnianski, A. S., Soh, T. K., et al. (2014). Virus entry. Lassa virus entry requires a trigger-induced receptor switch. Science 344 (6191), 1506–1510. doi:10.1126/science.1252480
Jia, X., Burugula, B. B., Chen, V., Lemons, R. M., Jayakody, S., Maksutova, M., et al. (2021). Massively parallel functional testing of MSH2 missense variants conferring Lynch syndrome risk. Am. J. Hum. Genet. 108 (1), 163–175. doi:10.1016/j.ajhg.2020.12.003
Jinek, M., Chylinski, K., Fonfara, I., Hauer, M., Doudna, J. A., and Charpentier, E. (2012). A programmable dual RNA-guided DNA endonuclease in adaptive bacterial immunity One-Sentence Summary. Science 337, 816–821. doi:10.1126/science.1225829
Karakus, E., Wannowius, M., Müller, S., Leiting, S., Leidolf, R., Noppes, S., et al. (2020). The orphan solute carrier SLC10A7 is a novel negative regulator of intracellular calcium signaling. Sci. Rep. 10, 7248. doi:10.1038/s41598-020-64006-3
Kauffman, M. R., Nazemidashtarjandi, S., Ghazanfari, D., Allen, A. E., Reynolds, N. M., Faik, A., et al. (2020). Evidence that knock down of GSK-3β in Chronic Myelogenous Leukemia cells augments IFN-γ-induced apoptosis. Leukemia Research 99, 106464. doi:10.1016/j.leukres.2020.106464
Khalil, A. M. (2020). The genome editing revolution: Review. J. Genet. Eng. Biotechnol. 18, 68. doi:10.1186/s43141-020-00078-y
Komor, A. C., Kim, Y. B., Packer, M. S., Zuris, J. A., and Liu, D. R. (2016). Programmable editing of a target base in genomic DNA without double-stranded DNA cleavage. Nature 533 (7603), 420–424. doi:10.1038/nature17946
Kotecki, M., Reddy, P. S., and Cochran, B. H. (1999). Isolation and characterization of a near-haploid human cell line. Exp. Cell. Res. 252, 273–280. doi:10.1006/excr.1999.4656
Kulicke, C. A., de Zan, E., Hein, Z., Gonzalez-Lopez, C., Ghanwat, S., Veerapen, N., et al. (2022). The P5-type ATPase ATP13A1 modulates major histocompatibility complex I-related protein 1 (MR1)-mediated antigen presentation. J. Biol. Chem. 298 (2), 101542. doi:10.1016/j.jbc.2021.101542
Lackner, D. H., Carré, A., Guzzardo, P. M., Banning, C., Mangena, R., Henley, T., et al. (2015). A generic strategy for CRISPR-Cas9-mediated gene tagging. Nat. Commun. 6 (1), 10237. doi:10.1038/ncomms10237
Leeb, M., Dietmann, S., Paramor, M., Niwa, H., and Smith, A. (2014). Genetic exploration of the exit from self-renewal using haploid embryonic stem cells. Cell Stem Cell 14 (3), 385–393. doi:10.1016/j.stem.2013.12.008
Li, Y., and Shuai, L. (2017). A versatile genetic tool: Haploid cells. Stem Cell Res. Ther. 8, 197. doi:10.1186/s13287-017-0657-4
Lin, S., Staahl, B. T., Alla, R. K., and Doudna, J. A. (2014). Enhanced homology-directed human genome engineering by controlled timing of CRISPR/Cas9 delivery. ELife 3, e04766. doi:10.7554/eLife.04766
Liu, R., and Moss, B. (2016). Opposing roles of double-stranded RNA effector pathways and viral defense proteins revealed with CRISPR-Cas9 knockout cell lines and vaccinia virus mutants. J. Virol. 90 (17), 7864–7879. doi:10.1128/JVI.00869-16
Liu, C., Zhang, L., Liu, H., and Cheng, K. (2017). Delivery strategies of the CRISPR-Cas9 gene-editing system for therapeutic applications. J. Control. Release 266, 17–26. doi:10.1016/j.jconrel.2017.09.012
Loregger, A., Raaben, M., Nieuwenhuis, J., Tan, J. M. E., Jae, L. T., van den Hengel, L. G., et al. (2020). Haploid genetic screens identify SPRING/C12ORF49 as a determinant of SREBP signaling and cholesterol metabolism. Nature Communications 11(1), 1128. doi:10.1038/s41467-020-14811-1
Lyon, G. J., Wang, K., and Lyon, W. (2012). A new era in genomic medicine identifying disease mutations in genomic medicine settings: Current challenges and how to accelerate progress. Genome Med. 4, 58. doi:10.1186/gm359
Makarova, K. S., Wolf, Y. I., and Kooninv, E. (2018). Classification and nomenclature of CRISPR-cas systems: Where from here? CRISPR J. 1 (5), 325–336. doi:10.1089/crispr.2018.0033
Maruyama, T., Dougan, S. K., Truttmann, M. C., Bilate, A. M., Ingram, J. R., and Ploegh, H. L. (2015). Increasing the efficiency of precise genome editing with CRISPR-Cas9 by inhibition of nonhomologous end joining. Nat. Biotechnol. 33 (5), 538–542. doi:10.1038/nbt.3190
Menasche, B. L., Davis, E. M., Wang, S., Ouyang, Y., Li, S., Yu, H., et al. (2020). PBRM1 and the glycosylphosphatidylinositol biosynthetic pathway promote tumor killing mediated by MHC-unrestricted cytotoxic lymphocytes. Sci. Adv. 6, eabc3243. doi:10.1126/sciadv.abc3243
Metzker, M. L. (2010). Sequencing technologies the next generation. Nat. Rev. Genet. 11, 31–46. doi:10.1038/nrg2626
Mir, A., Edraki, A., Lee, J., and Sontheimer, E. J. (2018). Type II-C CRISPR-cas9 biology, mechanism, and application. ACS Chem. Biol. 13, 357–365. doi:10.1021/acschembio.7b00855
Moder, M., Velimezi, G., Owusu, M., Mazouzi, A., Wiedner, M., Ferreira Da Silva, J., et al. (2017). Parallel genome-wide screens identify synthetic viable interactions between the BLM helicase complex and Fanconi anemia. Nat. Commun. 8, 1238. doi:10.1038/s41467-017-01439-x
Moskovskich, A., Goldmann, U., Kartnig, F., Lindinger, S., Konecka, J., Fiume, G., et al. (2019). The transporters SLC35A1 and SLC30A1 play opposite roles in cell survival upon VSV virus infection. Sci. Rep. 9 (1), 10471. doi:10.1038/s41598-019-46952-9
Neggers, J. E., Kwanten, B., Dierckx, T., Noguchi, H., Voet, A., Bral, L., et al. (2018). Target identification of small molecules using large-scale CRISPR-Cas mutagenesis scanning of essential genes. Nat. Commun. 9, 502. doi:10.1038/s41467-017-02349-8
Nixon, A. M. L., Duque, A., Yelle, N., McLaughlin, M., Davoudi, S., Pedley, N. M., et al. (2019). A rapid in vitro methodology for simultaneous target discovery and antibody generation against functional cell subpopulations. Sci. Rep. 9 (1), 842. doi:10.1038/s41598-018-37462-1
Núñez-Vázquez, S., Sánchez-Vera, I., Saura-Esteller, J., Cosialls, A. M., Noisier, A. F. M., Albericio, F., et al. (2021). NOXA upregulation by the prohibitin-binding compound fluorizoline is transcriptionally regulated by integrated stress response-induced ATF3 and ATF4. FEBS J. 288 (4), 1271–1285. doi:10.1111/febs.15480
Nurk, S., Koren, S., Rhie, A., Rautiainen, M., Bzikadze, A., and Phillippy, A. M. (2022). The complete sequence of a human genome. Science 376, 44–53. doi:10.1126/science.abj6987
Oishi, K., Yamayoshi, S., Kozuka-Hata, H., Oyama, M., and Kawaoka, Y. (2018). N-terminal acetylation by NatB is required for the shutoff activity of influenza A virus PA-X. Cell Rep. 24 (4), 851–860. doi:10.1016/j.celrep.2018.06.078
Olbrich, T., Mayor-Ruiz, C., Vega-Sendino, M., Gomez, C., Ortega, S., Ruiz, S., et al. (2017). A p53-dependent response limits the viability of mammalian haploid cells. Proc. Natl. Acad. Sci. U.S.A. 114 (35), 9367–9372. doi:10.1073/pnas.1705133114
Olbrich, T., Vega-Sendino, M., Murga, M., de Carcer, G., Malumbres, M., Ortega, S., et al. (2019). A chemical screen identifies compounds capable of selecting for haploidy in mammalian cells. Cell Rep. 28 (3), 597–604. doi:10.1016/j.celrep.2019.06.060
Palumbo, C., Mecchia, A., Bocedi, A., Aquilano, K., Lettieri-Barbato, D., et al. (2022). Revisited role of TRAF2 and TRAF2 C-terminal domain in endoplasmic reticulum stress-induced autophagy in HAP1 leukemia cells. Inter. J. Biochem. Cell Biol. 145, 106193. doi:10.1016/j.biocel.2022.106193
Petazzi, P., Menéndez, P., and Sevilla, A. (2022). CRISPR/Cas9-Mediated gene knockout and knockin human iPSCs. Methods Mol. Biol. 2454, 2559–2574. doi:10.1007/7651_2020_337
Pickar-Oliver, A., and Gersbach, C. A. (2019). The next generation of CRISPR–Cas technologies and applications. Nat. Rev. Mol. Cell Biol. 20 (8), 490–507. doi:10.1038/s41580-019-0131-5
Porteus, M. (2016). Genome editing: A new approach to human therapeutics. Annu. Rev. Pharmacol. Toxicol. 56, 163–190. doi:10.1146/annurev-pharmtox-010814-124454
Qin, W., Dion, S. L., Kutny, P. M., Zhang, Y., Cheng, A. W., Jillette, N. L., et al. (2015). Efficient CRISPR/cas9-mediated genome editing in mice by zygote electroporation of nuclease. Genetics 200 (2), 423–430. doi:10.1534/genetics.115.176594
Radford, E. J., Tan, H. K., Andersson, M. H. L., Stephenson, J. D., Gardner, E. J., Ironfield, H., et al. (2022). Saturation genome editing of DDX3X clarifies pathogenicity of germline and somatic variation. MedRxiv. doi:10.1101/2022.06.10.22276179
Ramani, A. K., Chuluunbaatar, T., Verster, A. J., Na, H., Vu, V., Pelte, N., et al. (2012). The majority of animal genes are required for wild-type fitness. Cell 148 (4), 792–802. doi:10.1016/j.cell.2012.01.019
Ran, F. A., Hsu, P. D., Wright, J., Agarwala, V., Scott, D. A., and Zhang, F. (2013). Genome engineering using the CRISPR-Cas9 system. Nat. Protoc. 8 (11), 2281–2308. doi:10.1038/nprot.2013.143
Robert, F., Barbeau, M., Éthier, S., Dostie, J., and Pelletier, J. (2015). Pharmacological inhibition of DNA-PK stimulates Cas9-mediated genome editing. Genome Med. 7 (1), 93. doi:10.1186/s13073-015-0215-6
Robinson-Garcia, L., da Silva, J. F., and Loizou, J. (2019). Synthetic lethal interactions for kinase deficiencies to DNA damage chemotherapeutics. Cancer Res. 79, 5693–5698. doi:10.1158/0008-5472.CAN-19-1364
Rodríguez-Castañeda, F., Lemma, R. B., Cuervo, I., Bengtsen, M., Moen, L. M., Ledsaak, M., et al. (2018). The SUMO protease SENP1 and the chromatin remodeler CHD3 interact and jointly affect chromatin accessibility and gene expression. J. Biol. Chem. 293, 15439–15454. doi:10.1074/jbc.RA118.002844
Sagi, I., and Benvenisty, N. (2017). Haploidy in humans: An evolutionary and developmental perspective. Dev. Cell 41, 581–589. doi:10.1016/j.devcel.2017.04.019
Sawai, M., Uchida, Y., Ohno, Y., Miyamoto, M., Nishioka, C., Itohara, S., et al. (2017). The 3-hydroxyacyl-CoA dehydratases HACD1 and HACD2 exhibit functional redundancy and are active in a wide range of fatty acid elongation pathways. J. Biol. Chem. 292 (37), 15538–15551. doi:10.1074/jbc.M117.803171
Scholefield, J., and Harrison, P. T. (2021). Prime editing – An update on the field. Gene Ther. 28 (7), 396–401. doi:10.1038/s41434-021-00263-9
Schumann, K., Lin, S., Boyer, E., Simeonov, D. R., Subramaniam, M., Gate, R. E., et al. (2015). Generation of knock-in primary human T cells using Cas9 ribonucleoproteins. Proc. Natl. Acad. Sci. 112 (33), 10437–10442. doi:10.1073/pnas.1512503112
Smits, A. H., Ziebell, F., Joberty, G., Zinn, N., Mueller, W. F., Clauder-Münster, S., et al. (2019). Biological plasticity rescues target activity in CRISPR knock outs. Nature Methods 16 (11), 1087–1093. doi:10.1038/s41592-019-0614-5
Song, J., Yang, D., Xu, J., Zhu, T., Chen, Y. E., and Zhang, J. (2016). RS-1 enhances CRISPR/Cas9-and TALEN-mediated knock-in efficiency. Nat. Commun. 7 (1), 10548. doi:10.1038/ncomms10548
Sonnenschein, H. A., Lawrence, K. F., Wittenberg, K. A., Slykas, F. A., Dohleman, E. L., Knoublauch, J. B., et al. (2018). Suppressor of IKKepsilon forms direct interactions with cytoskeletal proteins, tubulin and α-actinin, linking innate immunity to the cytoskeleton. FEBS Open Bio 8 (7), 1064–1082. doi:10.1002/2211-5463.12454
Takahashi, K., and Yamanaka, S. (2006). Induction of pluripotent stem cells from mouse embryonic and adult fibroblast cultures by defined factors. Cell 126, 663–676. doi:10.1016/j.cell.2006.07.024
Teixeira, F. R., Manfiolli, A. O., Vieira, N. A., Medeiros, A. C., de Coelho, O. P., Santiago Guimarães, D., et al. (2017). FBXO25 regulates MAPK signaling pathway through inhibition of ERK1/2 phosphorylation. Archives Biochem. Biophy. 621, 38–45. doi:10.1016/j.abb.2017.04.003
Thielen, A., van Baarsen, I., Jongsma, M., Zeerleder, S., Spaapen, R., and Wouters, D. (2018). CRISPR/Cas9 generated human CD46, CD55 and CD59 knockout cell lines as a tool for complement research. J. Immunol. Methods 456, 15–22. doi:10.1016/j.jim.2018.02.004
Tyumentseva, A., Tyumentsev, A., and Akimkin, V. (2021). Protocol for assessment of the efficiency of CRISPR/Cas RNP delivery to different types of target cells. PLoS ONE 16 (11), e0259812. doi:10.1371/journal.pone.0259812
Vindry, C., Guillin, O., Mangeot, P. E., Ohlmann, T., and Chavatte, L. (2019). A versatile strategy to reduce uga-selenocysteine recoding efficiency of the ribosome using crispr-cas9-viral-like-particles targeting selenocysteine-trna[ser]sec gene. Cells 8 (6), 574. doi:10.3390/cells8060574
Wei, X., Das, J., Fragoza, R., Liang, J., Bastos de Oliveira, F. M., Lee, H. R., et al. (2014). A massively parallel pipeline to clone DNA variants and examine molecular phenotypes of human disease mutations. PLoS Genet. 10, e1004819. doi:10.1371/journal.pgen.1004819
Weile, J., and Roth, F. P. (2018). Multiplexed assays of variant effects contribute to a growing genotype–phenotype atlas. Hum. Genet. 137 (9), 665–678. doi:10.1007/s00439-018-1916-x
Werner, E. R., Fernández-Quintero, M. L., Hulo, N., Golderer, G., Sailer, S., Lackner, K., et al. (2022). Essential role of a conserved aspartate for the enzymatic activity of plasmanylethanolamine desaturase. Cell. Mol. Life Sci. 79 (4), 214. doi:10.1007/s00018-022-04238-w
Yilmaz, A., Peretz, M., Sagi, I., and Benvenisty, N. (2016). Haploid human embryonic stem cells: Half the genome, double the value. Cell Stem Cell 19. 569–572. doi:10.1016/j.stem.2016.10.009
Yu, C., Liu, Y., Ma, T., Liu, K., Xu, S., Zhang, Y., et al. (2015). Small molecules enhance CRISPR genome editing in pluripotent stem cells. Cell Stem Cell 16 (2), 142–147. doi:10.1016/j.stem.2015.01.003
Zhao, D., Li, J., Li, S., Xin, X., Hu, M., Price, M. A., et al. (2021). Glycosylase base editors enable C-to-A and C-to-G base changes. Nat. Biotechnol. 39 (1), 35–40. doi:10.1038/s41587-020-0592-2
Keywords: HAP1, CRISPR-cas9, genome-engineering, functional validation, haploidy
Citation: Llargués-Sistac G, Bonjoch L and Castellvi-Bel S (2023) HAP1, a new revolutionary cell model for gene editing using CRISPR-Cas9. Front. Cell Dev. Biol. 11:1111488. doi: 10.3389/fcell.2023.1111488
Received: 29 November 2022; Accepted: 22 February 2023;
Published: 03 March 2023.
Edited by:
Nidhi Jalan-Sakrikar, Mayo Clinic, United StatesReviewed by:
Haiwei Mou, Wistar Institute, United StatesKankan Wang, Purdue University, United States
Copyright © 2023 Llargués-Sistac, Bonjoch and Castellvi-Bel. This is an open-access article distributed under the terms of the Creative Commons Attribution License (CC BY). The use, distribution or reproduction in other forums is permitted, provided the original author(s) and the copyright owner(s) are credited and that the original publication in this journal is cited, in accordance with accepted academic practice. No use, distribution or reproduction is permitted which does not comply with these terms.
*Correspondence: Sergi Castellvi-Bel, c2JlbEByZWNlcmNhLmNsaW5pYy5jYXQ=; Gemma Llargués-Sistac, bGxhcmd1ZXNAcmVjZXJjYS5jbGluaWMuY2F0