- 1Division of Nephrology, Department of Medicine, The University of Hong Kong, Hong Kong, Hong Kong SAR, China
- 2Department of Anatomical and Cellular Pathology, State Key Laboratory of Translational Oncology, The Chinese University of Hong Kong, Hong Kong, China
Fibrotic signaling plays a pivotal role in the development and progression of solid cancers including renal cell carcinoma (RCC). Intratumoral fibrosis (ITF) and pseudo-capsule (PC) fibrosis are significantly correlated to the disease progression of renal cell carcinoma. Targeting classic fibrotic signaling processes such as TGF-β signaling and epithelial-to-mesenchymal transition (EMT) shows promising antitumor effects both preclinically and clinically. Therefore, a better understanding of the pathogenic mechanisms of fibrotic signaling in renal cell carcinoma at molecular resolution can facilitate the development of precision therapies against solid cancers. In this review, we systematically summarized the latest updates on fibrotic signaling, from clinical correlation and molecular mechanisms to its therapeutic strategies for renal cell carcinoma. Importantly, we examined the reported fibrotic signaling on the human renal cell carcinoma dataset at the transcriptome level with single-cell resolution to assess its translational potential in the clinic.
1 Introduction
Renal cell carcinoma accounts for approximately 3% of all adult malignant diseases and over 90% of kidney cancer (Ferlay et al., 2015). In 2020, the new cases of kidney cancer have reached 431,288 worldwide, with 179,368 related deaths reported accordingly (Siegel et al., 2021). Although the 5-year survival is 60% overall for kidney cancer, it drops to 10% in patients with metastasis (Sengupta et al., 2005; Bianchi et al., 2012; Howlader et al., 2019). Currently, over 10 histological and molecular subtypes of RCC have been identified (Lopez-Beltran et al., 2006; Moch et al., 2016), of which clear cell renal cell carcinoma (ccRCC) is the most common RCC subtype (75% in all RCCs) (Creighton et al., 2013), followed by papillary RCC (pRCC, about 15% in all RCCs) (Linehan et al., 2016) and chromophobe RCC (chRCC, about 5% in total RCCs) (Davis et al., 2014). The discovery of von Hippel–Lindau (VHL) and other epigenetic regulatory gene mutations further advanced the knowledge of RCC development (Creighton et al., 2013; Alaghehbandan et al., 2019; Lin et al., 2021). However, how immune escape and distant metastasis initiate in RCC remains obscure.
Cancer-associated fibrosis was found to play a critical role in tumorigenesis, immune evasion, metastasis, and drug resistance in various solid tumors (Coffman et al., 2016; Kalluri, 2016; Piersma et al., 2020). Epidemiological findings strongly indicate a prognostic relevance between tissue fibrosis and epithelial cancers, such as hepatic, gastroesophageal, lung, and renal cancers (Neglia et al., 1995; Leek et al., 1996; Maisonneuve et al., 2013; Joung et al., 2018; Ballester et al., 2019). Moreover, fibrosis in the tumor immune microenvironment (TME), characterized by ITF (Chandler et al., 2019; Liu et al., 2019), activation of cancer-associated fibroblasts (CAFs) (Kalluri, 2016), and extracellular matrix (ECM) deposition (Chandler et al., 2019), supports tumor growth by producing growth factors, stimulating angiogenesis (Ziani et al., 2018; Inoue et al., 2019). Fibrotic signaling can also mediate immunosuppression by facilitating Treg cell and myeloid-derived suppressor cell (MDSC) differentiation and recruitment (Costa et al., 2018; Givel et al., 2018; Lin et al., 2022), metabolic reprogramming (Hamanaka and Mutlu, 2021), and long non-coding RNA (lncRNA) regulation (Shi et al., 2021). Therefore, fibrotic signaling has become a promising therapeutic target for cancer.
In this review, we summarized the pathogenic roles and underlying mechanisms of various RCC-associated fibrosis in the development and progression of RCC. In addition, we validated the reported findings in RCC patients datasets by using gene set enrichment analysis (GSEA) and single-cell RNA sequencing (scRNA-seq) data mining. Furthermore, the translational potentials of new therapeutic strategies targeting fibrotic signaling in RCC were also discussed.
2 Prognostic relevance between fibrosis and RCC
Fibrosis is a common feature frequently observed in RCC (Cheville et al., 2003), characterized by ITF, CAFs, ECM deposition, peritumoral PC fibrosis, and EMT. CAFs play an essential and versatile role in all stages of RCC. They are a group of robustly proliferative and metabolically activated fibroblasts (Guido et al., 2012; Kalluri, 2016), characterized by high expression of α-smooth muscle actin (α-SMA), fibroblast activation protein (FAP), S100A4, and platelet-derived growth factor receptors α and β (PDGFα and PDGFβ) (Kalluri, 2016). CAFs can be derived from multiple sources including resident fibroblasts (Kojima et al., 2010), mesenchymal stem cells (MSCs) (Barcellos-de-Souza et al., 2016), and epithelial and endothelial cells (Petersen et al., 2003; Yeon et al., 2018). Mechanistically, CAFs continuously interplay with tumor cells by producing pro-tumor cytokines, activating immunosuppressive leukocytes, and promoting ECM deposition, thus offering a tumor-favorable microenvironment (Kalluri, 2016). A recent cohort study revealed that the CAFs are significantly correlated with shorter disease-free survival (DFS), poorer overall survival (OS), and lymph node metastasis among ccRCC patients (Ambrosetti et al., 2022). Xu et al. further specified CD248 + CAFs as the pivotal CAF phenotype that was remarkably related to poor prognosis and immunosuppressive TME during RCC progression (Xu et al., 2021).
CAFs can directly form fibrotic tumor stroma via cross-linked collagen matrix deposition. Such ITF is associated with decreased lymphocyte infiltration, poorer patient survival, and various carcinomas, including breast cancer (Solinas et al., 2017; Li et al., 2019), lung cancer (Ballester et al., 2019), colorectal cancer (Nazemalhosseini-Mojarad et al., 2019), pancreatic cancer (Sinn et al., 2014), and advanced rectal cancer (Ueno et al., 2004). A retrospective cohort study involving 204 RCC patients found that over 80% of ccRCC cases had intratumoral fibrosis (Joung et al., 2018). Although ITF itself does not have a significant association with ccRCC prognosis, it is correlated with other prognostic factors such as Fuhrman nuclear grade, intratumoral necrosis, and lymphovascular invasion (Joung et al., 2018).
CAFs are also the major source of tumor-associated ECM (Bond et al., 2021). A recent proteomics study revealed that the composition of ECM in ccRCC varies significantly from their respective counterparts in the neighboring healthy cortex. RCC-associated ECM is more abundant, denser, and stiffer, with increased deposition of fibronectin (FN1), collagen 1 (COL1A1 and COL1A2), and collagen 6 (COL6A1, COL6A2, and COL6A3) (Bond et al., 2021). Also, some of these overexpressed fibrotic ECM proteins, including fibronectin 1 and collagen 1, are correlated with a poorer prognosis among ccRCC and pRCC patients (Steffens et al., 2012; Majo et al., 2020).
Interestingly, in contrast to the previous findings, several studies show that tumor-related fibrosis might limit tumor growth and metastasis at the early stages of cancer (Bruno et al., 2013; Alkasalias et al., 2014). In terms of RCC, such protective fibrosis is referred to as the fibrotic PC (Xi et al., 2018). PC is a common pathologic feature that exists in almost all the early-stage RCCs and is composed of fibrous tissue, compressed normal renal tissue, and scaffolding of vascular tissues in the RCC surrounding area (Huang et al., 1992; Tsili et al., 2012; Minervini et al., 2014; Cheng et al., 2015; Cho et al., 2017). The frequency of PC appearance varies from 33% to 72% in RCC. As the only barrier interposing between RCC and the surrounding normal renal parenchyma, the intact PC indicates a limited tumor-to-immune cell and tumor-to-matrix interaction in the TME and lower aggressiveness of RCCs. Several clinical observations revealed that the tumor invasion of the PC suggested a poor prognosis with a higher risk of local recurrence and metastasis (Yamashita et al., 1996; Cho et al., 2009; Xi et al., 2018). Qin et al. (2020) mentioned that fibrosis in PCs might strengthen the vital barriers to prevent tumor penetration. By quantifying collagen distribution in PCs among RCC patients, they further noticed that fibrosis in PCs is an independent marker of PC integrity. Lower PC fibrosis is significantly associated with shorter progression-free survival.
3 Transcriptome profile uncovered enriched fibrotic signaling in RCC
With the emerging RNA sequencing technologies, from bulk RNA sequencing (bulk RNA-seq) to scRNA-seq, transcriptome profiling of RCC has been greatly utilized in biomarker discovery, cancer heterogeneity characterization, and studies regarding distant metastasis and therapy resistance. More importantly, understanding the role of fibrosis in RCC development enlightens further therapeutic target identification by data mining the transcriptomic database of RCC patients. Here, we performed gene set enrichment analysis, as previously described (Chen et al., 2022), of a public human RCC scRNA-seq dataset (Young et al., 2018) and summarized the activated fibrotic signaling pathways identified in different subtypes and stages of human RCC.
Early in 2010, López-Lago et al. (2010) used RNA sequencing to provide evidence for the association of increased metastatic activity with the acquisition of a myofibroblast-like feature in both RCC cell lines and human metastatic RCC biopsies. Later, activation of the pro-fibrotic TGF-β signaling pathway was further identified in the transcriptome profile of MiTF/TFE translocation RCC (Malouf et al., 2014). Another two bulk RNA-seq datasets, comparing mRNA expression between human ccRCC and normal kidney tissue, also revealed pro-fibrosis signatures ACTA2 (α-SMA), COL1A1, COL23A1, VEGFA, and TGFB1 as the top differentially expressed genes (DEGs) upregulated in ccRCC (Xiong et al., 2014; Eikrem et al., 2016). Elevated TGF-β signaling was also identified in the transcriptomic profile of 176 ccRCC patients, correlated with poor disease survival and tumor metastasis (Zhao et al., 2006; Sjölund et al., 2011). Sven Wach et al. (2019) reported over 5,000 DEGs with the criteria |log2 fold change| ≥1 in collecting duct carcinoma (CDC), in comparison with the normal kidney tissue. Enrichment analysis targeting those DEGs further identified the pro-fibrosis collagen signaling pathway as the top-ranked enriched pathway activated in the CDC group. KRT17 (keratin 17), a wounded stratified epithelium-induced filament protein, was identified as the top DEG with the highest fold change of expression in CDC [Wach et al. (2019)]. More recently, KRT17 high-expressing basal-like cells were defined in fibrotic hypersensitivity pneumonitis patients with higher expression levels of COL1A1, FN1, and COL6A2 and upregulated activities in ECM organization by scRNA-seq analysis (Wang et al., 2022b). Findings of the scRNA-seq profile, in combination with the CDC bulk RNA profile, suggest a potential pathogenic role of KRT17-mediated fibrosis during RCC development.
Nevertheless, the aforementioned studies mainly focused on biomarker identification and RCC heterogenetic phenotype characterization without an in-depth study of fibrotic signaling participation in RCC at single-cell resolution. To address this question, we performed GSEA of a published human RCC scRNA-seq dataset (Young et al., 2018) downloaded from the European Genome-phenome Archive (EGA) under study IDs EGAS00001002171, EGAS00001002486, EGAS00001002325, and EGAS00001002553. Subsequently, raw UMI counts of the scRNA-seq dataset were imported into R (version 4.1.2) using the Seurat package (version 4.0.5) for quality control. Next, sequencing data from normal control and RCC patients were normalized, scaled, and mapped via non-linear dimensionality reduction on the Uniform Manifold Approximation and Projection (UMAP) plot by using the Normalized, ScaleData, and FindVariableFeatures functions, respectively, in the Seurat package to map the comprehensive cell landscape as shown in Figure 1A. Next, fibrosis-related gene set enrichment was tested using GSEABase (version 1.56.0) and GSVA R packages (version 1.42.0) within a panel of annotated gene databases (Gene Ontology, Reactome, and Kyoto Encyclopedia of Genes and Genomes). The enrichment of gene sets at the single-cell level was visualized using the AUCell (version 1.16.0) R Bioconductor package. The transcriptomes of RCC cells were significantly enriched in pro-fibrotic EMT and VEGF signaling (Figure 1B). Fibrotic signaling pathways including WNT and Hippo signaling pathways were also significantly upregulated in RCC (Figure 1C). Although TGF-β signaling is less enriched in RCC cells, TGFB1 expression, together with three other key fibrosis regulators, FN1, VIM, and CXCR4, was also elevated under the RCC condition (Figure 1D). Taken together, the above findings provided solid evidence of the activation and participation of fibrotic signaling during RCC progression.
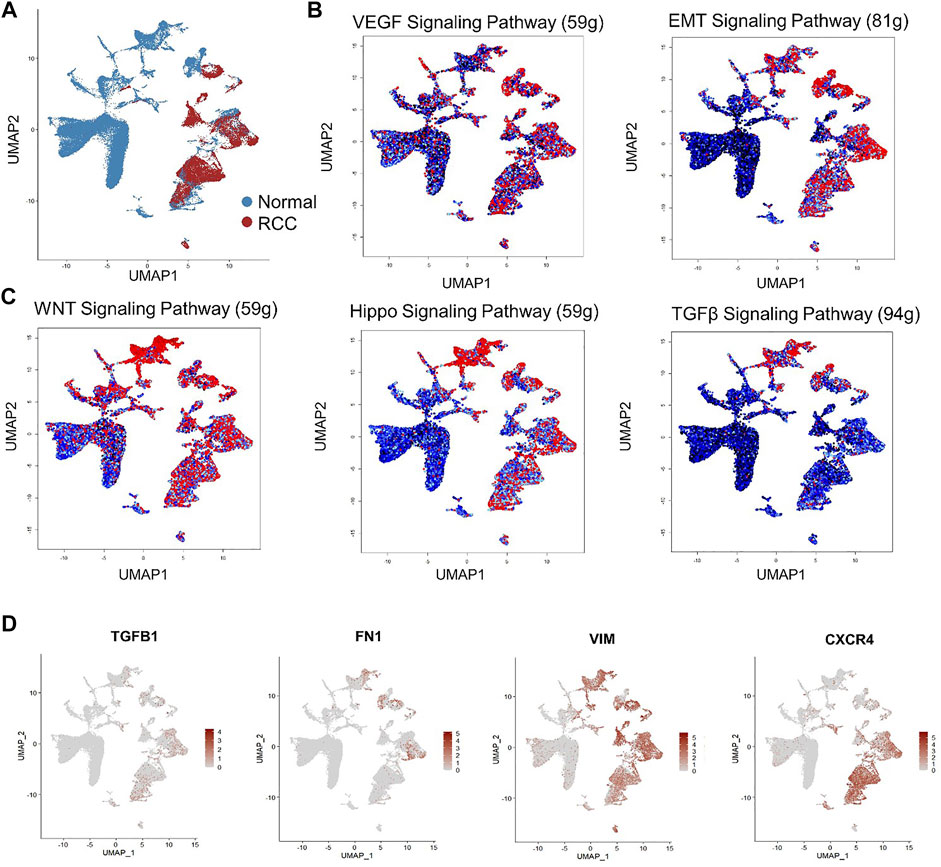
FIGURE 1. Single-cell RNA sequencing (scRNA-seq) reveals activated fibrotic signaling pathways in human renal cell carcinoma. (A) Non-linear dimensionality reduction on Uniform Manifold Approximation and Projection (UMAP) visualization of renal cells from RCC patients and the healthy control group. Each point depicts a single cell, colored according to group designation. Colored UMAP plots of highlighted cells with activated gene set expression in (B) VEGF and EMT signaling and (C) WNT, Hippo, and TGF-β signaling pathways in renal cells based on AUC scores. Each point represents a single cell. Cells with indicated signaling activation are colored in shades of red and those without signaling activation are colored in black–blue. (D) UMAP plots of gene expression gradients identified. Each point depicts a single cell, colored according to normalized expression levels. The average expression scale is shown on the right side of each UMAP plot.
4 Mechanism of the interplay between fibrosis and RCC
4.1 CAF-centered crosstalk between fibrotic stroma and RCC
CAFs have long been recognized as a substantial element in the TME that support tumor growth and invasion through diverse mechanisms, including ECM remodeling, cytokine production, immune regulation, and metabolic alteration. In this study, we reviewed and summarized the role of CAF-centered interaction and other critical fibrotic signaling pathways in RCC oncogenesis and metastasis (Figure 2). In RCC, activation of CAFs results from von Hippel–Lindau gene malfunction-induced HIF-1α accumulation (Razorenova et al., 2011; Shen and Kaelin, 2013; Yang et al., 2020). During tumor progression, CAFs continuously produce matrix-crosslinking enzymes, such as LOX family oxidases and matrix metalloproteinases (MMPs), to promote ECM remodeling. This leads to the reorganization of collagen and fibronectin fibers and consequently increases tumor stiffness and contributes to RCC invasion and metastasis (Gilkes et al., 2014). Higher expression levels of LOX family genes, for instance, LOX and LOXL2, indicate poor survival in ccRCC patients (Hase et al., 2014; Lin et al., 2020). Mechanistically, LOX and LOXL2 promote collagen stiffness increment, integrin α5β1 stabilization, and fiber formation while suppressing the protease and proteasome system in ccRCC (Hase et al., 2014). Furthermore, MMPs, including MMP-9 and MMP-2 produced by CAFs, also contribute to tumor invasion and metastasis and have been recognized as potential prognostic biomarkers in ccRCC (Awakura et al., 2006; Chen et al., 2017). In addition, microRNA mir-124-mediated suppression of MMP-9 can attenuate RCC invasiveness in vitro (Wang et al., 2018). Regulated by TGF-β1, MMP-13 is more related to bone metastasis of RCC than to primary RCC and healthy kidneys (Kominsky et al., 2008). Moreover, CAFs are also the major producers of the stromal cell-derived factor (SDF-1) (Orimo et al., 2005; Wang et al., 2021). In RCC, CAF-secreted SDF-1 interacts with the chemokine receptor 4 (CXCR4) on renal cancer cells and consequently promotes tumor angiogenesis and organ metabolism (Pan et al., 2006).
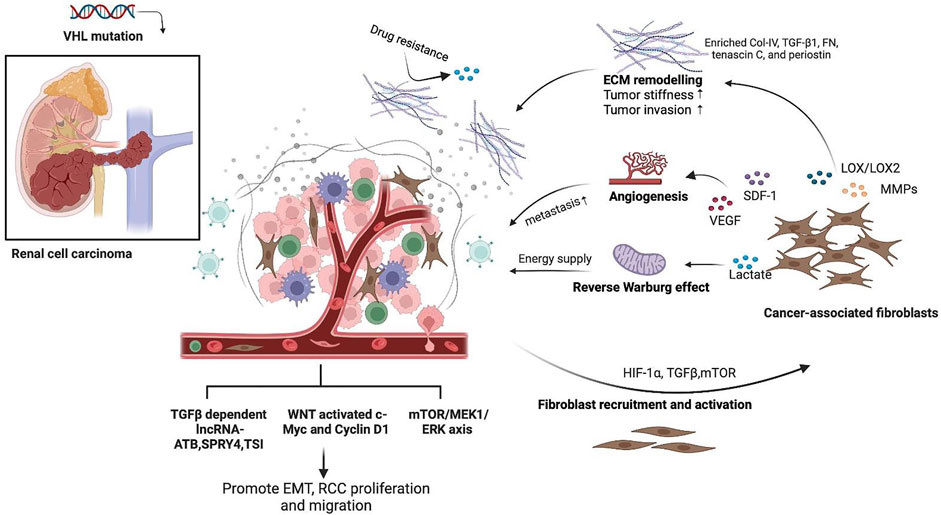
FIGURE 2. Schematic representation of the role of fibrotic signaling in renal cell carcinoma (RCC). CAFs are the major source of fibrotic stroma in the RCC TME, which could be activated due to HIF-1α accumulation and TGF-β and mTOR signaling regulation from cancer cells. Activated CAFs secreted MMPs and LOX to promote ECM remodeling with enriched collagen IV, TGF-β1, fibronectin, tenascin C, and periostin, thus promoting tumor stiffness, drug resistance, and tumor invasion. CAFs in RCC also produce SDF-1 and VEGF to promote tumor angiogenesis and favor RCC metastasis. CAFs fed cancer cells with increased lactate as a direct energy supply via aerobic glycolysis, which is known as the “reverse Warburg effect.” RCC also expressed pro-fibrotic signaling pathways, including TGF-β and its downstream lncRNA, WNT, and mTOR signaling pathways, to mediate the EMT process, tumor cell proliferation, and migration. This figure was created in BioRender.com.
CAFs are also capable of transducing the non-muscular myosin II- and PDGFRα-mediated contractility and traction forces to fibronectin via integrin α5β1, thus aligning the fibronectin-rich ECM to favor tumor migration (Erdogan et al., 2017; Gopal et al., 2017). Previous scRNA-seq transcriptomic analysis revealed CAF-specific feature expression and identified CAFs as the major sources of ECM in human ccRCC (Young et al., 2018; Bond et al., 2021; Liu et al., 2021). In contrast to the healthy cortex, CAF-contributed ECM production in ccRCC was characterized by high enrichment of collagen VI, fibronectin, tenascin C, TGF-β1, and periostin (Bond et al., 2021). Fibronectin and collagen were the most abundant components in CAF-produced ECM. CAF-induced fibronectin promotes the proliferation and inhibits the apoptosis of precancerous bronchial epithelial and carcinoma cells through the activation of PI3K/AKT signaling in the lung (Han et al., 2006; Han and Roman, 2006). As for RCC patients, higher fibronectin 1 expression showed an increased disease-related mortality rate (Steffens et al., 2012) and a more advanced clinical stage (Dong et al., 2021). Competing endogenous RNA networks further proved the direct correlations between FN1 and C3, FN1 and pro-fibrotic signaling pathways, including WNT, HIF, PI3K/AKT, MAPK, and TGF-β pathways, in ccRCC (Dong et al., 2021). The TGF-β1/Src axis is a well-studied pro-fibrotic signaling pathway that promotes renal fibrosis and tumor progression by promoting macrophage-to-myofibroblast transition (MMT) (Meng et al., 2016; Tang et al., 2018). An in vitro study on human RCC cells showed that silencing fibronectin in vitro attenuated cell proliferation and migration by suppressing TGF-β1/Src signaling (Ou et al., 2019). Collagen I, another essential CAF-produced ECM component, also facilitates EMT by upregulating MMP-2 and transcription factors ZEB2 and SNAIL in multiple RCC cell lines (Majo et al., 2020), which consequently enhances the proliferation, adhesion, and migration of RCC.
CAFs also influence tumor behavior by reprograming cancer cell metabolism (Martinez-Outschoorn et al., 2014). Aerobic glycolysis, also known as the Warburg effect (Warburg, 1925), has long been recognized as the hallmark metabolic pathway in various solid tumors, including RCC (Singer et al., 2011). During cancer development, anabolic cancer cells rapidly interact with the neighboring CAFs through metabolite exchange and oxidative stress induction and thus consequently promote aerobic glycolysis in CAFs. Such a “dual chamber” process is proposed as a CAF-dependent “reverse Warburg effect” (Pavlides et al., 2009; Benny et al., 2020). To be specific, aerobic glycolysis occurring within CAFs under Warburg metabolism results in lactate generation and deposition in RCC TME. Increased lactate not only acts as a major metabolic fuel for cancer cells directly (Guo et al., 2019), but also contributes to RCC progression and metastasis through indirect mechanisms. Accelerated lactate leads to acidification in TME, which consequently suppresses T-cell cytotoxicity against RCC (Sun et al., 2022). In addition, lactate also accelerates angiogenesis (Trabold et al., 2003), promotes EMT (Miranda-Gonçalves et al., 2020), and weakens cancer sensitivity to programmed cell death (Daneshmandi et al., 2019), thereby enhancing tumor aggressiveness.
CAFs in RCC expressed common fibrotic signaling pathways, including TGF-β, mTOR, MAPK, and WNT/β-catenin signaling pathways, which are similar to those found in most solid tumors (Wu et al., 2021a). Meanwhile, it is increasingly recognized that CAFs and their fibrotic signaling exhibit phenotypic and functional heterogeneity in different tissues/organs and origins. For example, CAFs in lung cancer express distinctively high levels of elastin and collagen (Hao et al., 2019a). Diverse CAF subpopulations with a distinguished transcriptome profile have been recognized in breast cancer (Bartoschek et al., 2018; Costa et al., 2018). Knowledge of CAF heterogeneity will help develop a precision cancer treatment. However, our understanding of RCC-specific CAFs is still very limited. A recent study combining scRNA-seq and cell line sequencing profile has identified the transcriptome signatures of RCC-specific CAFs, including COL1A1, COL1A2, COL5A1, COL16A1, elastin microfibril interfacer 1 (EMILIN1), lysyl oxidase-like 1 (LOXL1), and lumican (LUM) (Liu et al., 2020). Functional enrichment analysis indicates that RCC-specific CAF signatures are significantly associated with extracellular matrix function, collagen synthesis, cell surface interaction, and cell adhesion. Further studies are required to validate the phenotype and functional heterogeneity of CAFs among RCC patients.
4.2 TGF-β-centered regulation of fibrosis in RCC progression
TGF-β is the master regulator of renal fibrosis and immune escape in renal cancer (Meng et al., 2015; Chung et al., 2021). Early in 1998, Wunderlich et al. (1998) observed that the latent TGF-β1 was commonly and significantly elevated among RCC patients and even higher among those with pyelonephritis, indicating the oncogenetic role of TGF-β in RCC. With the emerging in-depth studies targeting the pathological role of TGF-β in cancer development, researchers have revealed the dual roles of TGF-β and its downstream cascades during cancer development (Massagué, 2008; Kubiczkova et al., 2012; Chan et al., 2022; Tang et al., 2022). Under normal conditions or at precancerous stages, TGF-β mainly exhibits antitumor activities by maintaining tissue homeostasis and inducing cell cycle arrest to regulate epithelial cell differentiation and apoptosis (Song, 2007; Xu et al., 2018). Nevertheless, cancer cells can always find ways to bypass TGF-β-mediated inhibition, in turn taking advantage of TGF-β signaling or directly producing TGF-β to benefit themselves (Biswas et al., 2014; Yang et al., 2016a; Tang et al., 2017; Rasti et al., 2021). In TME, TGF-β is the key mediator of EMT during tumor progression (Hao et al., 2019b; Xue et al., 2020; Chung et al., 2021). Stimulation with recombinant TGF-β1 significantly promotes EMT in renal carcinoma cells in vitro (Boström et al., 2012; Tretbar et al., 2019). Inhibition of TGF-β-induced EMT resulted in suppressed metastasis capacity of RCC (Boström et al., 2013; Wang et al., 2020). TGF-β also promotes EMT by regulating long non-coding RNA (lncRNA) expression in RCC TME. TGF-β1-dependent activation of lncRNA-ATB enhances EMT and tumor cell invasion in hepatocellular carcinoma (Yuan et al., 2014). In RCC, lncRNA-ATB was found to be positively correlated with metastasis and poorer prognosis (Xiong et al., 2016; Qi et al., 2017). Mechanistically, lncRNA-ATB downregulates the tumor suppressor p53 through the regulation of the DNA methyltransferase enzyme DNMT1 and thus strengthens the proliferation and migration of RCC cells (Song et al., 2019). Meanwhile, silencing lncRNA-ATB represses EMT in RCC by suppressing the expression of mesenchymal signatures such as N-cadherin and vimentin (Xiong et al., 2016). Another TGF-β1-induced lncRNA SPRY4-IT1 also demonstrates similar pro-EMT functions both in human RCC and RCC cells in vitro (Zhang et al., 2014).
4.3 ITF shapes the immune landscape of RCC
Immune cells in TME are considered critical and versatile players in tumor development. Fibrotic tumoral stroma is a significant source of immunosuppressive activity in the TME (Yamauchi et al., 2018; Baker et al., 2021). By producing CXCL12, activated CAFs directly inhibit cytotoxic T-cell recruitment and upregulate immunosuppressive Treg cell infiltration via binding to the CXCR4 receptor on cytotoxic T cells and Treg cells in pancreatic cancer (Feig et al., 2013). Yang et al. (2016b) found that FAP + CAFs are the major producers of CCL2 through the uPAR-induced FAP/STAT3/CCL2 axis in the murine liver cancer model. CAF-derived CCL2 in turn induces the recruitment of MDSCs by interacting with CCR2 on circulating MDSCs, thus favoring tumor evasion from immune surveillance. Other molecules produced by CAFs such as MMPs, latent TGF-β, IL-10, and VEGFA were also found to reshape the immune system toward a pro-tumoral pattern (Baker et al., 2021). In addition, CAFs contribute to ECM remodeling by producing MMPs, fibronectin, and collagen, thus increasing ECM stiffness around tumors (Acerbi et al., 2015; Altorki et al., 2019). Consequently, the thickened ECM prevents T-cell infiltration and antitumor drug delivery to cancer cells. Till now, there are still very limited evidence regarding the fibrotic stroma and its interaction with immune activity in TME of kidney cancer. A recent cohort study of 45 ccRCC patients uncovered a significant association between intratumoral fibrosis and cytotoxic T-lymphocyte-associated protein 4 (CTLA4) expression (Han et al., 2022), indicating the potential regulatory role of fibrosis in the tumor immune microenvironment of RCC. However, the underlying mechanism of fibrosis interaction with TME of RCC needs further investigation.
4.4 The role of other fibrosis signaling in RCC tumorigenesis
Except for TGF-β/Smad signaling, other pro-fibrotic pathways such as WNT, mTOR, and NOTCH signaling pathways also contribute to tumorigenesis in renal cancer (Table 1). The WNT/β-catenin pathway is one of the most well-studied signaling pathways in renal fibrosis and is activated in human fibrotic chronic kidney disease and the unilateral ureteral obstruction (UUO) mouse model (Tan et al., 2014; Huffstater et al., 2020). In response to injury, renal cells from the tubular epithelium or interstitium ubiquitously produce WNT ligands, such as WNT1, WNT7A, and WNT10A (He et al., 2011; Kuma et al., 2014; Huffstater et al., 2020). The activation of WNT signaling upregulates β-catenin-mediated downstream gene expression, including fibronectin, Snail 1, MMP-7, PAI-1, FSP1, and HGF, which consequently leads to fibroblast activation, EMT, and renal fibrosis (Edeling et al., 2016; Zhou et al., 2017). In RCC, the activation of WNT signaling through WNT ligand secretion, WNT receptor overexpression, and function loss of WNT antagonists has been reported to promote tumorigenesis and metastasis (Xu et al., 2016). Ligands of the WNT canonical pathway, such as WNT1 and WNT10A, are associated with tumor progression, poor prognosis, and tumor invasiveness in ccRCC patients (Kruck et al., 2013; Piotrowska et al., 2020) by oncogene activation, such as c-Myc and cyclin D1 (Furge et al., 2007; Karim et al., 2016). On the contrary, some ligands of the WNT non-canonical pathway, including WNT5A and WNT7A, demonstrate a potential tumor-suppressive role in renal cancer (Tamimi et al., 2008; Kondratov et al., 2012). In addition, the functional loss of WNT antagonists is another vital trigger of WNT signaling activation in RCC. Early in 2006, Urakami et al. (2006) reported that RCC patients significantly demonstrated high methylation levels of WNT antagonists, including sFRP-1, sFRP-2, sFRP-4, sFRP-5, WIF-1, and Dkk-3, compared to healthy controls. Specifically, the methylation level of sFRP-1 serves as an independent biomarker for RCC prognosis. Later, the same team also identified that WIF-1, a WNT antagonist belonging to the secreted frizzled-related protein (sFRP) class, functions as a tumor suppressor in RCC cells. Overexpression of WIF-1 enhances RCC cell apoptosis and inhibits tumor growth in vivo (Kawakami et al., 2009). A similar antitumor potential has also been identified in IGFBP-4, DKK-1, and DKK-3 (Ueno et al., 2011; Xu et al., 2017; Chen et al., 2018).
Activation of the mTOR signaling cascade also plays a pro-fibrotic role during chronic kidney injury. In diabetic nephropathy, induced glomerular mesangial hypertrophy and matrix expansion were mediated by TGF-β1 and its downstream AKT/PRAS40/mTOR axis in glomerular mesangial cells (Dey et al., 2012; Maity et al., 2020). Further studies confirmed that the activation of either mTOR1 or mTOR2 promotes fibroblast activation and myofibroblast proliferation (Jiang et al., 2013; Li et al., 2015). Moreover, the activation of mTOR2 facilitates macrophage polarization toward the pro-fibrotic M2 phenotype through the Rictor/mTORC2/AKT axis in the UUO mouse model (Ren et al., 2017). Both activated myofibroblasts and M2 macrophages are the main contributors to collagen production and ECM deposition during renal fibrosis. Intriguingly, downstream cascades of mTOR signaling also contribute to RCC oncogenesis and metastasis. The activation of mTOR is correlated with poor prognosis and aggressive tumorigenesis in RCC (Pantuck et al., 2007; Damayanti et al., 2018). Wu et al. (2021b) further uncovered that the activation of the mTOR pathway initiated RCC development from renal proximal tubular cells. Mechanistically, activated mTOR signaling upregulates MEK1 expression and promotes ERK activation, which induces pro-proliferation cyclins and Myc expression. Meanwhile, activated mTOR also suppresses the anti-proliferation p53/p16 axis via MKK6/p38 MAPK signaling. As a result, the TSC1 or VHL mutation-induced pathologic mTOR activation and its downstream cascades lead to renal cyst formation and RCC carcinogenesis [Wu et al. (2021b)]. Other mTOR-regulated molecules, such as autophagy-related light chain (LC3), fatty acids, EMT-associated eIF4E-binding protein 1 (4E-BP1), and ribosomal S6 kinase (S6K), were also found to contribute to RCC development and invasion (Dey et al., 2019; Qu et al., 2020; Tao et al., 2020).
The pro-fibrotic Notch signaling pathway also contributes to tumorigenesis. The interaction of Notch2 with its receptor Jagged1 not only promotes renal fibrosis and metabolism by regulating TFAM and PGC1-α expression (Han et al., 2017; Huang et al., 2018) but is also associated with RCC proliferation and metastasis through histone modification and gene amplification of cell fate determination features, KLF4 and SOX9, renal development-related features, PAX2 and SALL1, the stem cell maintenance-associated features, PROM1 and ALDH1A, and chromatin modification-related feature, MYST3 (Fendler et al., 2020).
Efforts have also been made to identify how RCC-specific fibrotic signaling differs from that of other types of cancer. Yang et al. (2021) identified 11 cytokines that are significantly associated with fibrosis in ccRCC, including brevican, prolactin, presenilin 1, and GRO. The team further found that ccRCC expressed a distinctly higher level of prolactin and prolactin receptors, compared to other malignant tumors like lung, liver, and breast cancers. Such ligand–receptor interactions are also correlated with the prognosis of ccRCC patients.
5 Therapeutic implications targeting fibrosis signaling
Due to the highly fibrotic feature of the RCC intratumoral environment, therapies targeting fibrosis signaling are exploited to suppress the progression of RCC (Table 2). In 2009, the mTORC1 inhibitors everolimus and temsirolimus had already been approved by the U.S. Food and Drug Administration (FDA) as single agents in the second-line setting and in the first-line in RCC patients’ treatment at advanced stages (Hudes et al., 2007; Motzer et al., 2008). Interestingly, another mTORC1/2 dual inhibitor AZD-2014 was also able to downregulate HIF-1α/2α and cyclin D expression and further inhibit RCC cell proliferation preclinically (Zheng et al., 2015). However, the phase II randomized control study among 49 patients with VEGF-refractory metastatic ccRCC showed that AZD-2014 was less toxic but also less effective than everolimus in improving patients’ overall survival and preventing tumor progression (Powles et al., 2016). Roulin et al. (2011) revealed that combined treatment with sorafenib and NVP-BEZ235, a novel dual PI3K/mTOR inhibitor, demonstrated enhanced antitumor efficacy in RCC cell lines, 786-0 and Caki-1, compared to either of the single treatments. Other mTOR inhibitors, such as mTORC2 inhibitors, PP242 and PP30 (Feldman et al., 2009; Li et al., 2021), and the mTORC1/2 dual inhibitor, WYE-125132 (Yu et al., 2010), all demonstrated ideal antitumor capacity in RCC preclinically. The aforementioned findings provide new insights into the development of mTOR-targeted novel antitumor therapeutic strategies for RCC.
The classic pro-fibrosis TGF-β signaling pathway is another promising therapeutic target of RCC. Early in 2014, a phase I clinical study used the human TGF-β1/β2/β3 neutralizer GC1008 (fresolimumab) to treat patients with advanced malignant melanoma and RCC (Morris et al., 2014). However, only seven out of the total 29 patients achieved a partial response. Later, in 2017, another phase I study among advanced cancer patients using the TGF-β receptor 2 monoclonal antibody LY3022859 failed to determine the maximum tolerated dose due to adverse side effects (Tolcher et al., 2017). The difficulties in the clinical application of anti-TGF-β antibodies among cancer patients might be related to the dual functions of the diverse TGF-β-dependent downstream cascades (Meng et al., 2012a). Smad3, one of the key downstream transcription factors of TGF-β signaling, plays a major pathogenic role during renal fibrosis and inflammation. However, the activation of TGF-β-dependent Smad2 and Smad7 pathways demonstrates anti-fibrosis and renal protective effects (Meng et al., 2012b). Nevertheless, efforts have been continuously made to improve the therapeutic effects of TGF-β-targeted treatments. Strauss et al. (2018) led another phase I study using a novel bifunctional fusion protein M7824 against PD-L1 and TGF-β among patients with an advanced solid tumor, and this novel targeted agent demonstrated encouraging antitumor effects with a good safety profile (Strauss et al., 2018). Previously, Lian et al. (2018) used a Smad7 inducer asiatic acid (AA) in combination with a Smad3 inhibitor naringenin (NG) to restore the balance of Smad3 and Smad7 signaling pathways in invasive melanoma and lung cancer mouse models and achieved significant anticancer effects by enhancing natural killer (NK) cell-mediated cytotoxicity through attenuating Smad3-induced suppression on two transcription factors essential for NK development and functions, namely, ID2 and IRF2. In addition, the same group discovered SIS3, a small-molecule inhibitor of Smad3, which effectively delays tumor development by suppressing TGF-β-mediated angiogenesis and immune escape in lung cancer (Tang et al., 2017; Lian et al., 2022). Although there is still very limited clinical evidence, preclinical studies have already revealed the promising anti-RCC potential of TGF-β signal-targeted therapies. A study using valproic acid, a Smad4 suppressor, significantly decreased cancer cell viability by inducing cell apoptosis and inhibiting EMT marker (E-cadherin and vimentin) expression in RCC cell lines (Mao et al., 2017). More recently, the anti-fibrotic drug pirfenidone (PFD), which has been approved by the FDA for the treatment of renal fibrosis, has been shown to be capable of suppressing RCC progression in vivo (Wang et al., 2022a). Mechanistically, PFD significantly downregulates TGF-β production in the RCC mouse model, thus mitigating TGF-β-mediated EMT and immunosuppressive MDSC infiltration into the TME.
6 Conclusion
Fibrosis has long been recognized as a major contributor to cancer progression and invasion. In this review, we systematically summarized the clinical association between renal fibrosis and poorer RCC outcomes and how a fibrotic microenvironment interacts with RCC in the form of ITF, CAFs, and PC fibrosis. In addition, we further conducted transcriptomic analysis on an up-to-date scRNA-seq profile of human RCC to confirm the participation of diverse fibrotic signaling in RCC development at single-cell resolution. The crosstalk between common pro-fibrotic pathways, including TGF-β, WNT, mTOR, and NOTCH signaling pathways, and RCC has also been discussed. In conclusion, the discovery of the mechanisms through which fibrotic signaling promotes tumorigenesis and aggressiveness in RCC provides inspiration for the development of anti-fibrotic therapies, such as mTOR inhibitors or anti-TGF-β antibodies, as novel therapeutic strategies for renal cancer.
Author contributions
J-YC conceived and designed the study, analyzed the scRNA-seq dataset, and coordinated this work. JY-C and W-HY collected and reviewed the literature and composed the draft. PT contributed to the draft and revision. J-YC and ST organized the manuscript. All authors contributed to data interpretation and manuscript editing.
Funding
This project was supported by the Research Grants Council of Hong Kong (General Research Fund 14111720, 17117923), Dr. Rita T. Liu SBS of L&T Charitable Foundation Ltd., and Bingei Family of Indo Café, Mr. Winston Leung, Mr. K.K. Chan, Ms. Siu Suet Lau, and Dr. Y.Y. Cheung and an endowment fund established at the University of Hong Kong for the Yu Professorship in Nephrology awarded to ST.
Conflict of interest
The authors declare that the research was conducted in the absence of any commercial or financial relationships that could be construed as a potential conflict of interest.
Publisher’s note
All claims expressed in this article are solely those of the authors and do not necessarily represent those of their affiliated organizations, or those of the publisher, the editors, and the reviewers. Any product that may be evaluated in this article, or claim that may be made by its manufacturer, is not guaranteed or endorsed by the publisher.
References
Acerbi, I., Cassereau, L., Dean, I., Shi, Q., Au, A., Park, C., et al. (2015). Human breast cancer invasion and aggression correlates with ECM stiffening and immune cell infiltration. Integr. Biol. (Camb) 7, 1120–1134. doi:10.1039/c5ib00040h
Alaghehbandan, R., Perez Montiel, D., Luis, A. S., and Hes, O. (2019). Molecular genetics of renal cell tumors: A practical diagnostic approach. Cancers (Basel) 12, 85. doi:10.3390/cancers12010085
Alkasalias, T., Flaberg, E., Kashuba, V., Alexeyenko, A., Pavlova, T., Savchenko, A., et al. (2014). Inhibition of tumor cell proliferation and motility by fibroblasts is both contact and soluble factor dependent. Proc. Natl. Acad. Sci. 111, 17188–17193. doi:10.1073/pnas.1419554111
Altorki, N. K., Markowitz, G. J., Gao, D., Port, J. L., Saxena, A., Stiles, B., et al. (2019). The lung microenvironment: An important regulator of tumour growth and metastasis. Nat. Rev. Cancer 19, 9–31. doi:10.1038/s41568-018-0081-9
Ambrosetti, D., Coutts, M., Paoli, C., Durand, M., Borchiellini, D., Montemagno, C., et al. (2022). Cancer-associated fibroblasts in renal cell carcinoma: Implication in prognosis and resistance to anti-angiogenic therapy. BJU Int. 129, 80–92. doi:10.1111/bju.15506
Awakura, Y., Ito, N., Nakamura, E., Takahashi, T., Kotani, H., Mikami, Y., et al. (2006). Matrix metalloproteinase-9 polymorphisms and renal cell carcinoma in a Japanese population. Cancer Lett. 241, 59–63. doi:10.1016/j.canlet.2005.10.005
Baker, A. T., Abuwarwar, M. H., Poly, L., Wilkins, S., and Fletcher, A. L. (2021). Cancer-associated fibroblasts and T cells: From mechanisms to outcomes. J. Immunol. 206, 310–320. doi:10.4049/jimmunol.2001203
Ballester, B., Milara, J., and Cortijo, J. (2019). Idiopathic pulmonary fibrosis and lung cancer: Mechanisms and molecular targets. Int. J. Mol. Sci. 20, 593. doi:10.3390/ijms20030593
Barcellos-De-Souza, P., Comito, G., Pons-Segura, C., Taddei, M. L., Gori, V., Becherucci, V., et al. (2016). Mesenchymal stem cells are recruited and activated into carcinoma-associated fibroblasts by prostate cancer microenvironment-derived TGF-β1. Stem Cells 34, 2536–2547. doi:10.1002/stem.2412
Bartoschek, M., Oskolkov, N., Bocci, M., Lövrot, J., Larsson, C., Sommarin, M., et al. (2018). Spatially and functionally distinct subclasses of breast cancer-associated fibroblasts revealed by single cell RNA sequencing. Nat. Commun. 9, 5150. doi:10.1038/s41467-018-07582-3
Benny, S., Mishra, R., Manojkumar, M. K., and Aneesh, T. (2020). From Warburg effect to Reverse Warburg effect; the new horizons of anti-cancer therapy. Med. Hypotheses 144, 110216. doi:10.1016/j.mehy.2020.110216
Bianchi, M., Sun, M., Jeldres, C., Shariat, S. F., Trinh, Q. D., Briganti, A., et al. (2012). Distribution of metastatic sites in renal cell carcinoma: A population-based analysis. Ann. Oncol. 23, 973–980. doi:10.1093/annonc/mdr362
Biswas, T., Gu, X., Yang, J., Ellies, L. G., and Sun, L. Z. (2014). Attenuation of TGF-β signaling supports tumor progression of a mesenchymal-like mammary tumor cell line in a syngeneic murine model. Cancer Lett. 346, 129–138. doi:10.1016/j.canlet.2013.12.018
Bond, K. H., Chiba, T., Wynne, K. P. H., Vary, C. P. H., Sims-Lucas, S., Coburn, J. M., et al. (2021). The extracellular matrix environment of clear cell renal cell carcinoma determines cancer associated fibroblast growth. Cancers (Basel) 13, 5873. doi:10.3390/cancers13235873
Boström, A.-K., Möller, C., Nilsson, E., Elfving, P., Axelson, H., and Johansson, M. E. (2012). Sarcomatoid conversion of clear cell renal cell carcinoma in relation to epithelial-to-mesenchymal transition. Hum. Pathol. 43, 708–719. doi:10.1016/j.humpath.2011.06.019
Boström, A. K., Lindgren, D., Johansson, M. E., and Axelson, H. (2013). Effects of TGF-β signaling in clear cell renal cell carcinoma cells. Biochem. Biophys. Res. Commun. 435, 126–133. doi:10.1016/j.bbrc.2013.04.054
Bruno, S., Collino, F., Deregibus, M. C., Grange, C., Tetta, C., and Camussi, G. (2013). Microvesicles derived from human bone marrow mesenchymal stem cells inhibit tumor growth. Stem cells Dev. 22, 758–771. doi:10.1089/scd.2012.0304
Chan, M. K., Chung, J. Y., Tang, P. C., Chan, A. S., Ho, J. Y., Lin, T. P., et al. (2022). TGF-β signaling networks in the tumor microenvironment. Cancer Lett. 550, 215925. doi:10.1016/j.canlet.2022.215925
Chandler, C., Liu, T., Buckanovich, R., and Coffman, L. G. (2019). The double edge sword of fibrosis in cancer. Transl. Res. 209, 55–67. doi:10.1016/j.trsl.2019.02.006
Chen, J., Huang, X. R., Yang, F., Yiu, W. H., Yu, X., Tang, S. C. W., et al. (2022). Single-cell RNA sequencing identified novel Nr4a1(+) Ear2(+) anti-inflammatory macrophage phenotype under myeloid-TLR4 dependent regulation in anti-glomerular basement membrane (GBM) crescentic glomerulonephritis (cGN). Adv. Sci. (Weinh) 9, e2200668. doi:10.1002/advs.202200668
Chen, S., Liu, W., Wang, K., Fan, Y., Chen, J., Ma, J., et al. (2017). Tetrandrine inhibits migration and invasion of human renal cell carcinoma by regulating Akt/NF-κB/MMP-9 signaling. PLoS One 12, e0173725. doi:10.1371/journal.pone.0173725
Chen, Z. Y., Du, Y., Wang, L., Liu, X. H., Guo, J., and Weng, X. D. (2018). MiR-543 promotes cell proliferation and metastasis of renal cell carcinoma by targeting Dickkopf 1 through the Wnt/β-catenin signaling pathway. J. Cancer 9, 3660–3668. doi:10.7150/jca.27124
Cheng, X., He, J., Gan, W., Fan, X., Yang, J., Zhu, B., et al. (2015). Pseudocapsule of renal cell carcinoma associated with Xp11.2 translocation/TFE3 gene fusion: A clue for tumor enucleation? Int. J. Clin. Exp. Pathol. 8, 5403–5410.
Cheville, J. C., Lohse, C. M., Zincke, H., Weaver, A. L., and Blute, M. L. (2003). Comparisons of outcome and prognostic features among histologic subtypes of renal cell carcinoma. Am. J. Surg. Pathology 27, 612–624. doi:10.1097/00000478-200305000-00005
Cho, H. J., Kim, S. J., Ha, U. S., Hong, S. H., Kim, J. C., Choi, Y. J., et al. (2009). Prognostic value of capsular invasion for localized clear-cell renal cell carcinoma. Eur. Urol. 56, 1006–1012. doi:10.1016/j.eururo.2008.11.031
Cho, S., Lee, J. H., Jeon, S. H., Park, J., Lee, S. H., Kim, C. H., et al. (2017). A prospective, multicenter analysis of pseudocapsule characteristics: Do all stages of renal cell carcinoma have complete pseudocapsules? Urol. Oncol. 35, 370–378. doi:10.1016/j.urolonc.2017.01.003
Chung, J. Y., Chan, M. K., Li, J. S., Chan, A. S., Tang, P. C., Leung, K. T., et al. (2021). TGF-Β signaling: From tissue fibrosis to tumor microenvironment. Int. J. Mol. Sci. 22, 7575. doi:10.3390/ijms22147575
Coffman, L. G., Choi, Y. J., Mclean, K., Allen, B. L., Di Magliano, M. P., and Buckanovich, R. J. (2016). Human carcinoma-associated mesenchymal stem cells promote ovarian cancer chemotherapy resistance via a BMP4/HH signaling loop. Oncotarget 7, 6916–6932. doi:10.18632/oncotarget.6870
Costa, A., Kieffer, Y., Scholer-Dahirel, A., Pelon, F., Bourachot, B., Cardon, M., et al. (2018). Fibroblast heterogeneity and immunosuppressive environment in human breast cancer. Cancer Cell 33, 463–479. doi:10.1016/j.ccell.2018.01.011
Creighton, C. J., Morgan, M., Gunaratne, P. H., Wheeler, D. A., Gibbs, R. A., Gordon Robertson, A., et al. (2013). Comprehensive molecular characterization of clear cell renal cell carcinoma. Nature 499, 43–49. doi:10.1038/nature12222
Damayanti, N. P., Budka, J. A., Khella, H. W. Z., Ferris, M. W., Ku, S. Y., Kauffman, E., et al. (2018). Therapeutic targeting of TFE3/IRS-1/PI3K/mTOR Axis in translocation renal cell carcinoma. Clin. Cancer Res. 24, 5977–5989. doi:10.1158/1078-0432.CCR-18-0269
Daneshmandi, S., Wegiel, B., and Seth, P. (2019). Blockade of lactate dehydrogenase-A (LDH-A) improves efficacy of anti-programmed cell death-1 (PD-1) therapy in melanoma. Cancers (Basel) 11, 450. doi:10.3390/cancers11040450
Davis, C. F., Ricketts, C. J., Wang, M., Yang, L., Cherniack, A. D., Shen, H., et al. (2014). The somatic genomic landscape of chromophobe renal cell carcinoma. Cancer Cell 26, 319–330. doi:10.1016/j.ccr.2014.07.014
Dey, N., Ghosh-Choudhury, N., Kasinath, B. S., and Choudhury, G. G. (2012). TGFβ-stimulated microRNA-21 utilizes PTEN to orchestrate AKT/mTORC1 signaling for mesangial cell hypertrophy and matrix expansion. PLoS One 7, e42316. doi:10.1371/journal.pone.0042316
Dey, P., Son, J. Y., Kundu, A., Kim, K. S., Lee, Y., Yoon, K., et al. (2019). Knockdown of pyruvate kinase M2 inhibits cell proliferation, metabolism, and migration in renal cell carcinoma. Int. J. Mol. Sci. 20, 5622. doi:10.3390/ijms20225622
Dong, Y., Ma, W. M., Yang, W., Hao, L., Zhang, S. Q., Fang, K., et al. (2021). Identification of C3 and FN1 as potential biomarkers associated with progression and prognosis for clear cell renal cell carcinoma. BMC Cancer 21, 1135. doi:10.1186/s12885-021-08818-0
Edeling, M., Ragi, G., Huang, S., Pavenstädt, H., and Susztak, K. (2016). Developmental signalling pathways in renal fibrosis: The roles of notch, wnt and hedgehog. Nat. Rev. Nephrol. 12, 426–439. doi:10.1038/nrneph.2016.54
Eikrem, O. S., Strauss, P., Beisland, C., Scherer, A., Landolt, L., Flatberg, A., et al. (2016). Development and confirmation of potential gene classifiers of human clear cell renal cell carcinoma using next-generation RNA sequencing. Scand. J. Urol. 50, 452–462. doi:10.1080/21681805.2016.1238007
Erdogan, B., Ao, M., White, L. M., Means, A. L., Brewer, B. M., Yang, L., et al. (2017). Cancer-associated fibroblasts promote directional cancer cell migration by aligning fibronectin. J. Cell Biol. 216, 3799–3816. doi:10.1083/jcb.201704053
Feig, C., Jones, J. O., Kraman, M., Wells, R. J., Deonarine, A., Chan, D. S., et al. (2013). Targeting CXCL12 from FAP-expressing carcinoma-associated fibroblasts synergizes with anti-PD-L1 immunotherapy in pancreatic cancer. Proc. Natl. Acad. Sci. U. S. A. 110, 20212–20217. doi:10.1073/pnas.1320318110
Feldman, M. E., Apsel, B., Uotila, A., Loewith, R., Knight, Z. A., Ruggero, D., et al. (2009). Active-site inhibitors of mTOR target rapamycin-resistant outputs of mTORC1 and mTORC2. PLoS Biol. 7, e38. doi:10.1371/journal.pbio.1000038
Fendler, A., Bauer, D., Busch, J., Jung, K., Wulf-Goldenberg, A., Kunz, S., et al. (2020). Inhibiting WNT and NOTCH in renal cancer stem cells and the implications for human patients. Nat. Commun. 11, 929. doi:10.1038/s41467-020-14700-7
Ferlay, J., Soerjomataram, I., Dikshit, R., Eser, S., Mathers, C., Rebelo, M., et al. (2015). Cancer incidence and mortality worldwide: Sources, methods and major patterns in GLOBOCAN 2012. Int. J. Cancer 136, E359–E386. doi:10.1002/ijc.29210
Furge, K. A., Chen, J., Koeman, J., Swiatek, P., Dykema, K., Lucin, K., et al. (2007). Detection of DNA copy number changes and oncogenic signaling abnormalities from gene expression data reveals MYC activation in high-grade papillary renal cell carcinoma. Cancer Res. 67, 3171–3176. doi:10.1158/0008-5472.CAN-06-4571
Gilkes, D. M., Semenza, G. L., and Wirtz, D. (2014). Hypoxia and the extracellular matrix: Drivers of tumour metastasis. Nat. Rev. Cancer 14, 430–439. doi:10.1038/nrc3726
Givel, A.-M., Kieffer, Y., Scholer-Dahirel, A., Sirven, P., Cardon, M., Pelon, F., et al. (2018). miR200-regulated CXCL12β promotes fibroblast heterogeneity and immunosuppression in ovarian cancers. Nat. Commun. 9, 1056. doi:10.1038/s41467-018-03348-z
Gopal, S., Veracini, L., Grall, D., Butori, C., Schaub, S., Audebert, S., et al. (2017). Fibronectin-guided migration of carcinoma collectives. Nat. Commun. 8, 14105. doi:10.1038/ncomms14105
Guido, C., Whitaker-Menezes, D., Capparelli, C., Balliet, R., Lin, Z., Pestell, R. G., et al. (2012). Metabolic reprogramming of cancer-associated fibroblasts by TGF-β drives tumor growth: Connecting TGF-β signaling with "Warburg-like" cancer metabolism and L-lactate production. Cell Cycle 11, 3019–3035. doi:10.4161/cc.21384
Guo, C., Huang, T., Wang, Q.-H., Li, H., Khanal, A., Kang, E.-H., et al. (2019). Monocarboxylate transporter 1 and monocarboxylate transporter 4 in cancer-endothelial co-culturing microenvironments promote proliferation, migration, and invasion of renal cancer cells. Cancer Cell Int. 19, 170. doi:10.1186/s12935-019-0889-8
Hamanaka, R. B., and Mutlu, G. M. (2021). The role of metabolic reprogramming and de novo amino acid synthesis in collagen protein production by myofibroblasts: Implications for organ fibrosis and cancer. Amino Acids 53, 1851–1862. doi:10.1007/s00726-021-02996-8
Han, S. H., Wu, M. Y., Nam, B. Y., Park, J. T., Yoo, T. H., Kang, S. W., et al. (2017). PGC-1α protects from notch-induced kidney fibrosis development. J. Am. Soc. Nephrol. 28, 3312–3322. doi:10.1681/ASN.2017020130
Han, S., Khuri, F. R., and Roman, J. (2006). Fibronectin stimulates non-small cell lung carcinoma cell growth through activation of Akt/mammalian target of rapamycin/S6 kinase and inactivation of LKB1/AMP-activated protein kinase signal pathways. Cancer Res. 66, 315–323. doi:10.1158/0008-5472.CAN-05-2367
Han, S. W., and Roman, J. (2006). Fibronectin induces cell proliferation and inhibits apoptosis in human bronchial epithelial cells: Pro-oncogenic effects mediated by PI3-kinase and NF-kappa B. Oncogene 25, 4341–4349. doi:10.1038/sj.onc.1209460
Han, S., Yang, W., Qin, C., Du, Y., Ding, M., Yin, H., et al. (2022). Intratumoral fibrosis and patterns of immune infiltration in clear cell renal cell carcinoma. BMC Cancer 22, 661. doi:10.1186/s12885-022-09765-0
Hao, J., Zeltz, C., Pintilie, M., Li, Q., Sakashita, S., Wang, T., et al. (2019a). Characterization of distinct populations of carcinoma-associated fibroblasts from non-small cell lung carcinoma reveals a role for ST8SIA2 in cancer cell invasion. Neoplasia 21, 482–493. doi:10.1016/j.neo.2019.03.009
Hao, Y., Baker, D., and Ten Dijke, P. (2019b). TGF-β-Mediated epithelial-mesenchymal transition and cancer metastasis. Int. J. Mol. Sci. 20, 2767. doi:10.3390/ijms20112767
Hase, H., Jingushi, K., Ueda, Y., Kitae, K., Egawa, H., Ohshio, I., et al. (2014). LOXL2 status correlates with tumor stage and regulates integrin levels to promote tumor progression in ccRCC. Mol. Cancer Res. 12, 1807–1817. doi:10.1158/1541-7786.MCR-14-0233
He, W., Kang, Y. S., Dai, C., and Liu, Y. (2011). Blockade of Wnt/β-catenin signaling by paricalcitol ameliorates proteinuria and kidney injury. J. Am. Soc. Nephrol. 22, 90–103. doi:10.1681/ASN.2009121236
Howlader, N., Noone, A., Krapcho, M. E., Miller, D., Brest, A., Yu, M., et al. (2019).SEER cancer statistics review, 1. National Cancer Institute, 1975–2016.
Huang, S., Park, J., Qiu, C., Chung, K. W., Li, S. Y., Sirin, Y., et al. (2018). Jagged1/Notch2 controls kidney fibrosis via Tfam-mediated metabolic reprogramming. PLoS Biol. 16, e2005233. doi:10.1371/journal.pbio.2005233
Huang, S. Q., Zou, S. S., and Huang, Q. L. (1992). MR appearance of the pseudocapsule of renal cell carcinoma and its pathologic basis. Urol. Radiol. 13, 158–161.
Hudes, G., Carducci, M., Tomczak, P., Dutcher, J., Figlin, R., Kapoor, A., et al. (2007). Temsirolimus, interferon alfa, or both for advanced renal-cell carcinoma. N. Engl. J. Med. 356, 2271–2281. doi:10.1056/NEJMoa066838
Huffstater, T., Merryman, W. D., and Gewin, L. S. (2020). Wnt/β-Catenin in acute kidney injury and progression to chronic kidney disease. Semin. Nephrol. 40, 126–137. doi:10.1016/j.semnephrol.2020.01.004
Inoue, K. I., Kishimoto, S., Akimoto, K., Sakuma, M., Toyoda, S., Inoue, T., et al. (2019). Cancer-associated fibroblasts show heterogeneous gene expression and induce vascular endothelial growth factor A (VEGFA) in response to environmental stimuli. Ann. Gastroenterol. Surg. 3, 416–425. doi:10.1002/ags3.12249
Jiang, L., Xu, L., Mao, J., Li, J., Fang, L., Zhou, Y., et al. (2013). Rheb/mTORC1 signaling promotes kidney fibroblast activation and fibrosis. J. Am. Soc. Nephrol. 24, 1114–1126. doi:10.1681/ASN.2012050476
Joung, J. W., Oh, H. K., Lee, S. J., Kim, Y. A., and Jung, H. J. (2018). Significance of intratumoral fibrosis in clear cell renal cell carcinoma. J. Pathol. Transl. Med. 52, 323–330. doi:10.4132/jptm.2018.07.21
Kalluri, R. (2016). The biology and function of fibroblasts in cancer. Nat. Rev. Cancer 16, 582–598. doi:10.1038/nrc.2016.73
Karim, S., Al-Maghrabi, J. A., Farsi, H. M., Al-Sayyad, A. J., Schulten, H. J., Buhmeida, A., et al. (2016). Cyclin D1 as a therapeutic target of renal cell carcinoma-a combined transcriptomics, tissue microarray and molecular docking study from the Kingdom of Saudi Arabia. BMC Cancer 16, 741. doi:10.1186/s12885-016-2775-2
Kawakami, K., Hirata, H., Yamamura, S., Kikuno, N., Saini, S., Majid, S., et al. (2009). Functional significance of Wnt inhibitory factor-1 gene in kidney cancer. Cancer Res. 69, 8603–8610. doi:10.1158/0008-5472.CAN-09-2534
Kojima, Y., Acar, A., Eaton, E. N., Mellody, K. T., Scheel, C., Ben-Porath, I., et al. (2010). Autocrine TGF-beta and stromal cell-derived factor-1 (SDF-1) signaling drives the evolution of tumor-promoting mammary stromal myofibroblasts. Proc. Natl. Acad. Sci. U. S. A. 107, 20009–20014. doi:10.1073/pnas.1013805107
Kominsky, S. L., Doucet, M., Thorpe, M., and Weber, K. L. (2008). MMP-13 is over-expressed in renal cell carcinoma bone metastasis and is induced by TGF-beta1. Clin. Exp. Metastasis 25, 865–870. doi:10.1007/s10585-008-9202-2
Kondratov, A. G., Kvasha, S. M., Stoliar, L. A., Romanenko, A. M., Zgonnyk, Y. M., Gordiyuk, V. V., et al. (2012). Alterations of the WNT7A gene in clear cell renal cell carcinomas. PLoS One 7, e47012. doi:10.1371/journal.pone.0047012
Kruck, S., Eyrich, C., Scharpf, M., Sievert, K. D., Fend, F., Stenzl, A., et al. (2013). Impact of an altered Wnt1/β-catenin expression on clinicopathology and prognosis in clear cell renal cell carcinoma. Int. J. Mol. Sci. 14, 10944–10957. doi:10.3390/ijms140610944
Kubiczkova, L., Sedlarikova, L., Hajek, R., and Sevcikova, S. (2012). TGF-β - an excellent servant but a bad master. J. Transl. Med. 10, 183. doi:10.1186/1479-5876-10-183
Kuma, A., Yamada, S., Wang, K. Y., Kitamura, N., Yamaguchi, T., Iwai, Y., et al. (2014). Role of WNT10A-expressing kidney fibroblasts in acute interstitial nephritis. PLoS One 9, e103240. doi:10.1371/journal.pone.0103240
Leek, R. D., Lewis, C. E., Whitehouse, R., Greenall, M., Clarke, J., and Harris, A. L. (1996). Association of macrophage infiltration with angiogenesis and prognosis in invasive breast carcinoma. Cancer Res. 56, 4625–4629.
Li, B., Zhang, X., Ren, Q., Gao, L., and Tian, J. (2021). NVP-BEZ235 inhibits renal cell carcinoma by targeting TAK1 and PI3K/Akt/mTOR pathways. Front. Pharmacol. 12, 781623. doi:10.3389/fphar.2021.781623
Li, J., Ren, J., Liu, X., Jiang, L., He, W., Yuan, W., et al. (2015). Rictor/mTORC2 signaling mediates TGFβ1-induced fibroblast activation and kidney fibrosis. Kidney Int. 88, 515–527. doi:10.1038/ki.2015.119
Li, Y., Wei, Y., Tang, W., Luo, J., Wang, M., Lin, H., et al. (2019). Association between the degree of fibrosis in fibrotic focus and the unfavorable clinicopathological prognostic features of breast cancer. PeerJ 7, e8067. doi:10.7717/peerj.8067
Lian, G. Y., Wan, Y., Mak, T. S., Wang, Q. M., Zhang, J., Chen, J., et al. (2022). Self-carried nanodrug (SCND-SIS3): A targeted therapy for lung cancer with superior biocompatibility and immune boosting effects. Biomaterials 288, 121730. doi:10.1016/j.biomaterials.2022.121730
Lian, G. Y., Wang, Q. M., Tang, P. M., Zhou, S., Huang, X. R., and Lan, H. Y. (2018). Combination of asiatic acid and Naringenin modulates NK cell anti-cancer immunity by rebalancing smad3/smad7 signaling. Mol. Ther. 26, 2255–2266. doi:10.1016/j.ymthe.2018.06.016
Lin, P. H., Huang, C. Y., Yu, K. J., Kan, H. C., Liu, C. Y., Chuang, C. K., et al. (2021). Genomic characterization of clear cell renal cell carcinoma using targeted gene sequencing. Oncol. Lett. 21, 169. doi:10.3892/ol.2021.12430
Lin, S., Zheng, L., Lu, Y., Xia, Q., Zhou, P., and Liu, Z. (2020). Comprehensive analysis on the expression levels and prognostic values of LOX family genes in kidney renal clear cell carcinoma. Cancer Med. 9, 8624–8638. doi:10.1002/cam4.3472
Lin, Y., Cai, Q., Chen, Y., Shi, T., Liu, W., Mao, L., et al. (2022). CAFs shape myeloid-derived suppressor cells to promote stemness of intrahepatic cholangiocarcinoma through 5-lipoxygenase. Hepatology 75, 28–42. doi:10.1002/hep.32099
Linehan, W. M., Spellman, P. T., Ricketts, C. J., Creighton, C. J., Fei, S. S., Davis, C., et al. (2016). Comprehensive molecular characterization of papillary renal-cell carcinoma. N. Engl. J. Med. 374, 135–145. doi:10.1056/NEJMoa1505917
Liu, B., Chen, X., Zhan, Y., Wu, B., and Pan, S. (2020). Identification of a gene signature for renal cell carcinoma-associated fibroblasts mediating cancer progression and affecting prognosis. Front. Cell Dev. Biol. 8, 604627. doi:10.3389/fcell.2020.604627
Liu, B., Chen, X., Zhan, Y., Wu, B., and Pan, S. (2021). Identification of a gene signature for renal cell carcinoma–associated fibroblasts mediating cancer progression and affecting prognosis. Front. Cell Dev. Biol. 8, 604627. doi:10.3389/fcell.2020.604627
Liu, T., Zhou, L., Li, D., Andl, T., and Zhang, Y. (2019). Cancer-associated fibroblasts build and secure the tumor microenvironment. Front. Cell Dev. Biol. 7, 60. doi:10.3389/fcell.2019.00060
Lopez-Beltran, A., Scarpelli, M., Montironi, R., and Kirkali, Z. (2006). 2004 WHO classification of the renal tumors of the adults. Eur. Urol. 49, 798–805. doi:10.1016/j.eururo.2005.11.035
López-Lago, M. A., Thodima, V. J., Guttapalli, A., Chan, T., Heguy, A., Molina, A. M., et al. (2010). Genomic deregulation during metastasis of renal cell carcinoma implements a myofibroblast-like program of gene expression. Cancer Res. 70, 9682–9692. doi:10.1158/0008-5472.CAN-10-2279
Maisonneuve, P., Marshall, B. C., Knapp, E. A., and Lowenfels, A. B. (2013). Cancer risk in cystic fibrosis: A 20-year nationwide study from the United States. J. Natl. Cancer Inst. 105, 122–129. doi:10.1093/jnci/djs481
Maity, S., Das, F., Kasinath, B. S., Ghosh-Choudhury, N., and Ghosh Choudhury, G. (2020). TGFβ acts through PDGFRβ to activate mTORC1 via the Akt/PRAS40 axis and causes glomerular mesangial cell hypertrophy and matrix protein expression. J. Biol. Chem. 295, 14262–14278. doi:10.1074/jbc.RA120.014994
Majo, S., Courtois, S., Souleyreau, W., Bikfalvi, A., and Auguste, P. (2020). Impact of extracellular matrix components to renal cell carcinoma behavior. Front. Oncol. 10, 625. doi:10.3389/fonc.2020.00625
Malouf, G. G., Su, X., Yao, H., Gao, J., Xiong, L., He, Q., et al. (2014). Next-generation sequencing of translocation renal cell carcinoma reveals novel RNA splicing partners and frequent mutations of chromatin-remodeling genes. Clin. Cancer Res. 20, 4129–4140. doi:10.1158/1078-0432.CCR-13-3036
Mao, S., Lu, G., Lan, X., Yuan, C., Jiang, W., Chen, Y., et al. (2017). Valproic acid inhibits epithelial-mesenchymal transition in renal cell carcinoma by decreasing SMAD4 expression. Mol. Med. Rep. 16, 6190–6199. doi:10.3892/mmr.2017.7394
Martinez-Outschoorn, U. E., Lisanti, M. P., and Sotgia, F. (2014). Catabolic cancer-associated fibroblasts transfer energy and biomass to anabolic cancer cells, fueling tumor growth. Semin. Cancer Biol. 25, 47–60. doi:10.1016/j.semcancer.2014.01.005
Meng, X.-M., Nikolic-Paterson, D. J., and Lan, H. Y. (2016). TGF-Β: The master regulator of fibrosis. Nat. Rev. Nephrol. 12, 325–338. doi:10.1038/nrneph.2016.48
Meng, X. M., Huang, X. R., Xiao, J., Chen, H. Y., Zhong, X., Chung, A. C., et al. (2012a). Diverse roles of TGF-β receptor II in renal fibrosis and inflammation in vivo and in vitro. J. Pathol. 227, 175–188. doi:10.1002/path.3976
Meng, X. M., Huang, X. R., Xiao, J., Chung, A. C., Qin, W., Chen, H. Y., et al. (2012b). Disruption of Smad4 impairs TGF-β/Smad3 and Smad7 transcriptional regulation during renal inflammation and fibrosis in vivo and in vitro. Kidney Int. 81, 266–279. doi:10.1038/ki.2011.327
Meng, X. M., Tang, P. M., Li, J., and Lan, H. Y. (2015). TGF-β/Smad signaling in renal fibrosis. Front. Physiol. 6, 82. doi:10.3389/fphys.2015.00082
Minervini, A., Rosaria Raspollini, M., Tuccio, A., Di Cristofano, C., Siena, G., Salvi, M., et al. (2014). Pathological characteristics and prognostic effect of peritumoral capsule penetration in renal cell carcinoma after tumor enucleation. Urol. Oncol. 32, 50.e15–e22. doi:10.1016/j.urolonc.2013.07.018
Miranda-Gonçalves, V., Lameirinhas, A., Macedo-Silva, C., Lobo, J., Dias, P, C., Ferreira, V., et al. (2020). Lactate increases renal cell carcinoma aggressiveness through sirtuin 1-dependent epithelial mesenchymal transition Axis regulation. Cells 9, 1053. doi:10.3390/cells9041053
Moch, H., Cubilla, A. L., Humphrey, P. A., Reuter, V. E., and Ulbright, T. M. (2016). The 2016 WHO classification of tumours of the urinary system and male genital organs-Part A: Renal, penile, and testicular tumours. Eur. Urol. 70, 93–105. doi:10.1016/j.eururo.2016.02.029
Morris, J. C., Tan, A. R., Olencki, T. E., Shapiro, G. I., Dezube, B. J., Reiss, M., et al. (2014). Phase I study of GC1008 (fresolimumab): A human anti-transforming growth factor-beta (TGFβ) monoclonal antibody in patients with advanced malignant melanoma or renal cell carcinoma. PLoS One 9, e90353. doi:10.1371/journal.pone.0090353
Motzer, R. J., Escudier, B., Oudard, S., Hutson, T. E., Porta, C., Bracarda, S., et al. (2008). Efficacy of everolimus in advanced renal cell carcinoma: A double-blind, randomised, placebo-controlled phase III trial. Lancet 372, 449–456. doi:10.1016/S0140-6736(08)61039-9
Nazemalhosseini-Mojarad, E., Mohammadpour, S., Torshizi Esafahani, A., Gharib, E., Larki, P., Moradi, A., et al. (2019). Intratumoral infiltrating lymphocytes correlate with improved survival in colorectal cancer patients: Independent of oncogenetic features. J. Cell Physiol. 234, 4768–4777. doi:10.1002/jcp.27273
Neglia, J. P., Fitzsimmons, S. C., Maisonneuve, P., Schöni, M. H., Schöni-Affolter, F., Corey, M., et al. (1995). The risk of cancer among patients with cystic fibrosis. Cystic Fibrosis and Cancer Study Group. N. Engl. J. Med. 332, 494–499. doi:10.1056/NEJM199502233320803
Orimo, A., Gupta, P. B., Sgroi, D. C., Arenzana-Seisdedos, F., Delaunay, T., Naeem, R., et al. (2005). Stromal fibroblasts present in invasive human breast carcinomas promote tumor growth and angiogenesis through elevated SDF-1/CXCL12 secretion. Cell 121, 335–348. doi:10.1016/j.cell.2005.02.034
Ou, Y. C., Li, J. R., Wang, J. D., Chang, C. Y., Wu, C. C., Chen, W. Y., et al. (2019). Fibronectin promotes cell growth and migration in human renal cell carcinoma cells. Int. J. Mol. Sci. 20, 2792. doi:10.3390/ijms20112792
Pan, J., Mestas, J., Burdick, M. D., Phillips, R. J., Thomas, G. V., Reckamp, K., et al. (2006). Stromal derived factor-1 (SDF-1/CXCL12) and CXCR4 in renal cell carcinoma metastasis. Mol. Cancer 5, 56. doi:10.1186/1476-4598-5-56
Pantuck, A. J., Seligson, D. B., Klatte, T., Yu, H., Leppert, J. T., Moore, L., et al. (2007). Prognostic relevance of the mTOR pathway in renal cell carcinoma: Implications for molecular patient selection for targeted therapy. Cancer 109, 2257–2267. doi:10.1002/cncr.22677
Pavlides, S., Whitaker-Menezes, D., Castello-Cros, R., Flomenberg, N., Witkiewicz, A. K., Frank, P. G., et al. (2009). The reverse Warburg effect: Aerobic glycolysis in cancer associated fibroblasts and the tumor stroma. Cell Cycle 8, 3984–4001. doi:10.4161/cc.8.23.10238
Petersen, O. W., Nielsen, H. L., Gudjonsson, T., Villadsen, R., Rank, F., Niebuhr, E., et al. (2003). Epithelial to mesenchymal transition in human breast cancer can provide a nonmalignant stroma. Am. J. Pathol. 162, 391–402. doi:10.1016/S0002-9440(10)63834-5
Piersma, B., Hayward, M. K., and Weaver, V. M. (2020). Fibrosis and cancer: A strained relationship. Biochim. Biophys. Acta Rev. Cancer 1873, 188356. doi:10.1016/j.bbcan.2020.188356
Piotrowska, Ż., Niezgoda, M., Młynarczyk, G., Acewicz, M., and Kasacka, I. (2020). Comparative assessment of the WNT/β-Catenin pathway, CacyBP/SIP, and the immunoproteasome subunit LMP7 in various histological types of renal cell carcinoma. Front. Oncol. 10, 566637. doi:10.3389/fonc.2020.566637
Powles, T., Wheater, M., Din, O., Geldart, T., Boleti, E., Stockdale, A., et al. (2016). A randomised phase 2 study of AZD2014 versus everolimus in patients with VEGF-refractory metastatic clear cell renal cancer. Eur. Urol. 69, 450–456. doi:10.1016/j.eururo.2015.08.035
Qi, J. J., Liu, Y. X., and Lin, L. (2017). High expression of long non-coding RNA ATB is associated with poor prognosis in patients with renal cell carcinoma. Eur. Rev. Med. Pharmacol. Sci. 21, 2835–2839.
Qin, C., Yin, H., Liu, H., Liu, F., Du, Y., and Xu, T. (2020). The significance of fibrosis quantification as a marker in assessing pseudo-capsule status and clear cell renal cell carcinoma prognosis. Diagn. (Basel) 10, 895. doi:10.3390/diagnostics10110895
Qu, Y. Y., Zhao, R., Zhang, H. L., Zhou, Q., Xu, F. J., Zhang, X., et al. (2020). Inactivation of the AMPK-GATA3-ECHS1 pathway induces fatty acid synthesis that promotes clear cell renal cell carcinoma growth. Cancer Res. 80, 319–333. doi:10.1158/0008-5472.CAN-19-1023
Rasti, A., Madjd, Z., Saeednejad Zanjani, L., Babashah, S., Abolhasani, M., Asgari, M., et al. (2021). SMAD4 expression in renal cell carcinomas correlates with a stem-cell phenotype and poor clinical outcomes. Front. Oncol. 11, 581172. doi:10.3389/fonc.2021.581172
Razorenova, O. V., Finger, E. C., Colavitti, R., Chernikova, S. B., Boiko, A. D., Chan, C. K. F., et al. (2011). VHL loss in renal cell carcinoma leads to up-regulation of CUB domain-containing protein 1 to stimulate PKCδ-driven migration. Proc. Natl. Acad. Sci. 108, 1931–1936. doi:10.1073/pnas.1011777108
Ren, J., Li, J., Feng, Y., Shu, B., Gui, Y., Wei, W., et al. (2017). Rictor/mammalian target of rapamycin complex 2 promotes macrophage activation and kidney fibrosis. J. Pathol. 242, 488–499. doi:10.1002/path.4921
Roulin, D., Waselle, L., Dormond-Meuwly, A., Dufour, M., Demartines, N., and Dormond, O. (2011). Targeting renal cell carcinoma with NVP-BEZ235, a dual PI3K/mTOR inhibitor, in combination with sorafenib. Mol. Cancer 10, 90. doi:10.1186/1476-4598-10-90
Sengupta, S., Lohse, C. M., Leibovich, B. C., Frank, I., Thompson, R. H., Webster, W. S., et al. (2005). Histologic coagulative tumor necrosis as a prognostic indicator of renal cell carcinoma aggressiveness. Cancer 104, 511–520. doi:10.1002/cncr.21206
Shen, C., and Kaelin, W. G. (2013). The VHL/HIF axis in clear cell renal carcinoma. Semin. Cancer Biol. 23, 18–25. doi:10.1016/j.semcancer.2012.06.001
Shi, T., Morishita, A., Kobara, H., and Masaki, T. (2021). The role of long non-coding RNA and microRNA networks in hepatocellular carcinoma and its tumor microenvironment. Int. J. Mol. Sci. 22, 10630. doi:10.3390/ijms221910630
Siegel, R. L., Miller, K. D., Fuchs, H. E., and Jemal, A. (2021). Cancer Statistics, 2021. CA Cancer J. Clin. 71 (1), 7–33. doi:10.3322/caac.21654
Singer, K., Kastenberger, M., Gottfried, E., Hammerschmied, C. G., Büttner, M., Aigner, M., et al. (2011). Warburg phenotype in renal cell carcinoma: High expression of glucose-transporter 1 (GLUT-1) correlates with low CD8(+) T-cell infiltration in the tumor. Int. J. Cancer 128, 2085–2095. doi:10.1002/ijc.25543
Sinn, M., Denkert, C., Striefler, J. K., Pelzer, U., Stieler, J. M., Bahra, M., et al. (2014). α-Smooth muscle actin expression and desmoplastic stromal reaction in pancreatic cancer: Results from the CONKO-001 study. Br. J. Cancer 111, 1917–1923. doi:10.1038/bjc.2014.495
Sjölund, J., Boström, A. K., Lindgren, D., Manna, S., Moustakas, A., Ljungberg, B., et al. (2011). The notch and TGF-β signaling pathways contribute to the aggressiveness of clear cell renal cell carcinoma. PLoS One 6, e23057. doi:10.1371/journal.pone.0023057
Solinas, C., Carbognin, L., De Silva, P., Criscitiello, C., and Lambertini, M. (2017). Tumor-infiltrating lymphocytes in breast cancer according to tumor subtype: Current state of the art. Breast 35, 142–150. doi:10.1016/j.breast.2017.07.005
Song, C., Xiong, Y., Liao, W., Meng, L., and Yang, S. (2019). Long noncoding RNA ATB participates in the development of renal cell carcinoma by downregulating p53 via binding to DNMT1. J. Cell Physiol. 234, 12910–12917. doi:10.1002/jcp.27957
Song, J. (2007). EMT or apoptosis: A decision for TGF-beta. Cell Res. 17, 289–290. doi:10.1038/cr.2007.25
Steffens, S., Schrader, A. J., Vetter, G., Eggers, H., Blasig, H., Becker, J., et al. (2012). Fibronectin 1 protein expression in clear cell renal cell carcinoma. Oncol. Lett. 3, 787–790. doi:10.3892/ol.2012.566
Strauss, J., Heery, C. R., Schlom, J., Madan, R. A., Cao, L., Kang, Z., et al. (2018). Phase I trial of M7824 (MSB0011359C), a bifunctional fusion protein targeting PD-L1 and TGFβ, in advanced solid tumors. Clin. Cancer Res. 24, 1287–1295. doi:10.1158/1078-0432.CCR-17-2653
Sun, Z., Tao, W., Guo, X., Jing, C., Zhang, M., Wang, Z., et al. (2022). Construction of a lactate-related prognostic signature for predicting prognosis, tumor microenvironment, and immune response in kidney renal clear cell carcinoma. Front. Immunol. 13, 818984. doi:10.3389/fimmu.2022.818984
Tamimi, Y., Ekuere, U., Laughton, N., and Grundy, P. (2008). WNT5A is regulated by PAX2 and may be involved in blastemal predominant Wilms tumorigenesis. Neoplasia 10, 1470–1480. doi:10.1593/neo.08442
Tan, R. J., Zhou, D., Zhou, L., and Liu, Y. (2014). Wnt/β-catenin signaling and kidney fibrosis. Kidney Int. Suppl. 4 (4), 84–90. doi:10.1038/kisup.2014.16
Tang, P. C., Chung, J. Y., Liao, J., Chan, M. K., Chan, A. S., Cheng, G., et al. (2022). Single-cell RNA sequencing uncovers a neuron-like macrophage subset associated with cancer pain. Sci. Adv. 8, eabn5535. eabn5535. doi:10.1126/sciadv.abn5535
Tang, P. M.-K., Zhou, S., Meng, X.-M., Wang, Q.-M., Li, C.-J., Lian, G.-Y., et al. (2017). Smad3 promotes cancer progression by inhibiting E4BP4-mediated NK cell development. Nat. Commun. 8, 14677. doi:10.1038/ncomms14677
Tang, P. M., Zhou, S., Li, C. J., Liao, J., Xiao, J., Wang, Q. M., et al. (2018). The proto-oncogene tyrosine protein kinase Src is essential for macrophage-myofibroblast transition during renal scarring. Kidney Int. 93, 173–187. doi:10.1016/j.kint.2017.07.026
Tao, R., Niu, W. B., Dou, P. H., Ni, S. B., Yu, Y. P., Cai, L. C., et al. (2020). Nucleobindin-2 enhances the epithelial-mesenchymal transition in renal cell carcinoma. Oncol. Lett. 19, 3653–3664. doi:10.3892/ol.2020.11526
Tolcher, A. W., Berlin, J. D., Cosaert, J., Kauh, J., Chan, E., Piha-Paul, S. A., et al. (2017). A phase 1 study of anti-TGFβ receptor type-II monoclonal antibody LY3022859 in patients with advanced solid tumors. Cancer Chemother. Pharmacol. 79, 673–680. doi:10.1007/s00280-017-3245-5
Trabold, O., Wagner, S., Wicke, C., Scheuenstuhl, H., Hussain, M. Z., Rosen, N., et al. (2003). Lactate and oxygen constitute a fundamental regulatory mechanism in wound healing. Wound Repair Regen. 11, 504–509. doi:10.1046/j.1524-475x.2003.11621.x
Tretbar, S., Krausbeck, P., Müller, A., Friedrich, M., Vaxevanis, C., Bukur, J., et al. (2019). TGF-β inducible epithelial-to-mesenchymal transition in renal cell carcinoma. Oncotarget 10, 1507–1524. doi:10.18632/oncotarget.26682
Tsili, A. C., Argyropoulou, M. I., Gousia, A., Kalef-Ezra, J., Sofikitis, N., Malamou-Mitsi, V., et al. (2012). Renal cell carcinoma: Value of multiphase MDCT with multiplanar reformations in the detection of pseudocapsule. AJR Am. J. Roentgenol. 199, 379–386. doi:10.2214/AJR.11.7747
Ueno, H., Jones, A. M., Wilkinson, K. H., Jass, J. R., and Talbot, I. C. (2004). Histological categorisation of fibrotic cancer stroma in advanced rectal cancer. Gut 53, 581–586. doi:10.1136/gut.2003.028365
Ueno, K., Hirata, H., Majid, S., Tabatabai, Z. L., Hinoda, Y., and Dahiya, R. (2011). IGFBP-4 activates the Wnt/beta-catenin signaling pathway and induces M-CAM expression in human renal cell carcinoma. Int. J. Cancer 129, 2360–2369. doi:10.1002/ijc.25899
Urakami, S., Shiina, H., Enokida, H., Hirata, H., Kawamoto, K., Kawakami, T., et al. (2006). Wnt antagonist family genes as biomarkers for diagnosis, staging, and prognosis of renal cell carcinoma using tumor and serum DNA. Clin. Cancer Res. 12, 6989–6997. doi:10.1158/1078-0432.CCR-06-1194
Wach, S., Taubert, H., Weigelt, K., Hase, N., Köhn, M., Misiak, D., et al. (2019). RNA sequencing of collecting duct renal cell carcinoma suggests an interaction between miRNA and target genes and a predominance of deregulated solute carrier genes. Cancers (Basel) 12, 64. doi:10.3390/cancers12010064
Wang, G., Zhou, X., Guo, Z., Huang, N., Li, J., Lv, Y., et al. (2022a). The Anti-fibrosis drug Pirfenidone modifies the immunosuppressive tumor microenvironment and prevents the progression of renal cell carcinoma by inhibiting tumor autocrine TGF-β. Cancer Biol. Ther. 23, 150–162. doi:10.1080/15384047.2022.2035629
Wang, J., Zhang, L., Luo, L., He, P., Xiong, A., Jiang, M., et al. (2022b). Characterizing cellular heterogeneity in fibrotic hypersensitivity pneumonitis by single-cell transcriptional analysis. Cell Death Discov. 8, 38. doi:10.1038/s41420-022-00831-x
Wang, P., Chen, W., Ma, T., Lin, Z., Liu, C., Liu, Y., et al. (2020). lncRNA lnc-TSI inhibits metastasis of clear cell renal cell carcinoma by suppressing TGF-β-induced epithelial-mesenchymal transition. Mol. Ther. Nucleic Acids 22, 1–16. doi:10.1016/j.omtn.2020.08.003
Wang, P., Zhang, L. D., Sun, M. C., Gu, W. D., and Geng, H. Z. (2018). Over-expression of mir-124 inhibits MMP-9 expression and decreases invasion of renal cell carcinoma cells. Eur. Rev. Med. Pharmacol. Sci. 22, 6308–6314. doi:10.26355/eurrev_201810_16041
Wang, Y., Lan, W., Xu, M., Song, J., Mao, J., Li, C., et al. (2021). Cancer-associated fibroblast-derived SDF-1 induces epithelial-mesenchymal transition of lung adenocarcinoma via CXCR4/β-catenin/PPARδ signalling. Cell Death Dis. 12, 214. doi:10.1038/s41419-021-03509-x
Warburg, O. H. (1925). The metabolism of carcinoma cells. J. Cancer Res. 9, 148–163. doi:10.1158/jcr.1925.148
Wu, F., Yang, J., Liu, J., Wang, Y., Mu, J., Zeng, Q., et al. (2021a). Signaling pathways in cancer-associated fibroblasts and targeted therapy for cancer. Signal Transduct. Target Ther. 6, 218. doi:10.1038/s41392-021-00641-0
Wu, H., He, D., Biswas, S., Shafiquzzaman, M., Zhou, X., Charron, J., et al. (2021b). mTOR activation initiates renal cell carcinoma development by coordinating ERK and p38MAPK. Cancer Res. 81, 3174–3186. doi:10.1158/0008-5472.CAN-20-3979
Wunderlich, H., Steiner, T., Kosmehl, H., Junker, U., Reinhold, D., Reichelt, O., et al. (1998). Increased transforming growth factor beta1 plasma level in patients with renal cell carcinoma: A tumor-specific marker? Urol. Int. 60, 205–207. doi:10.1159/000030255
Xi, W., Wang, J., Liu, L., Xiong, Y., Qu, Y., Lin, Z., et al. (2018). Evaluation of tumor pseudocapsule status and its prognostic significance in renal cell carcinoma. J. Urol. 199, 915–920. doi:10.1016/j.juro.2017.10.043
Xiong, J., Liu, Y., Jiang, L., Zeng, Y., and Tang, W. (2016). High expression of long non-coding RNA lncRNA-ATB is correlated with metastases and promotes cell migration and invasion in renal cell carcinoma. Jpn. J. Clin. Oncol. 46, 378–384. doi:10.1093/jjco/hyv214
Xiong, Z., Yu, H., Ding, Y., Feng, C., Wei, H., Tao, S., et al. (2014). RNA sequencing reveals upregulation of RUNX1-runx1t1 gene signatures in clear cell renal cell carcinoma. BioMed Res. Int. 2014, 450621. doi:10.1155/2014/450621
Xu, C., Zhang, K., Yang, F., Zhou, X., Liu, S., Li, Y., et al. (2021). CD248+ cancer-associated fibroblasts: A novel prognostic and therapeutic target for renal cell carcinoma. Front. Oncol. 11, 773063. doi:10.3389/fonc.2021.773063
Xu, J., Sadahira, T., Kinoshita, R., Li, S. A., Huang, P., Wada, K., et al. (2017). Exogenous DKK-3/REIC inhibits Wnt/β-catenin signaling and cell proliferation in human kidney cancer KPK1. Oncol. Lett. 14, 5638–5642. doi:10.3892/ol.2017.6833
Xu, Q., Krause, M., Samoylenko, A., and Vainio, S. (2016). Wnt signaling in renal cell carcinoma. Cancers (Basel) 8, 57. doi:10.3390/cancers8060057
Xu, X., Zheng, L., Yuan, Q., Zhen, G., Crane, J. L., Zhou, X., et al. (2018). Transforming growth factor-β in stem cells and tissue homeostasis. Bone Res. 6, 2. doi:10.1038/s41413-017-0005-4
Xue, V. W., Chung, J. Y., Córdoba, C. a. G., Cheung, A. H., Kang, W., Lam, E. W., et al. (2020). Transforming growth factor-β: A multifunctional regulator of cancer immunity. Cancers (Basel) 12, 3099. doi:10.3390/cancers12113099
Yamashita, Y., Honda, S., Nishiharu, T., Urata, J., and Takahashi, M. (1996). Detection of pseudocapsule of renal cell carcinoma with MR imaging and CT. AJR Am. J. Roentgenol. 166, 1151–1155. doi:10.2214/ajr.166.5.8615260
Yamauchi, M., Barker, T. H., Gibbons, D. L., and Kurie, J. M. (2018). The fibrotic tumor stroma. J. Clin. Invest. 128, 16–25. doi:10.1172/JCI93554
Yang, D., He, Y., Wu, B., Deng, Y., Li, M., Yang, Q., et al. (2020). Drinking water and sanitation conditions are associated with the risk of malaria among children under five years old in sub-saharan africa: A logistic regression model analysis of national survey data. J. Adv. Res. 21, 1–13. doi:10.1016/j.jare.2019.09.001
Yang, J., Lu, Y., Lin, Y. Y., Zheng, Z. Y., Fang, J. H., He, S., et al. (2016a). Vascular mimicry formation is promoted by paracrine TGF-β and SDF1 of cancer-associated fibroblasts and inhibited by miR-101 in hepatocellular carcinoma. Cancer Lett. 383, 18–27. doi:10.1016/j.canlet.2016.09.012
Yang, W., Qin, C., Han, J., Han, S., Bai, W., Du, Y., et al. (2021). What mediates fibrosis in the tumor microenvironment of clear renal cell carcinoma. Front. Genet. 12, 725252. doi:10.3389/fgene.2021.725252
Yang, X., Lin, Y., Shi, Y., Li, B., Liu, W., Yin, W., et al. (2016b). FAP promotes immunosuppression by cancer-associated fibroblasts in the tumor microenvironment via STAT3-CCL2 signaling. Cancer Res. 76, 4124–4135. doi:10.1158/0008-5472.CAN-15-2973
Yeon, J. H., Jeong, H. E., Seo, H., Cho, S., Kim, K., Na, D., et al. (2018). Cancer-derived exosomes trigger endothelial to mesenchymal transition followed by the induction of cancer-associated fibroblasts. Acta Biomater. 76, 146–153. doi:10.1016/j.actbio.2018.07.001
Young, M. D., Mitchell, T. J., Vieira Braga, F. A., Tran, M. G. B., Stewart, B. J., Ferdinand, J. R., et al. (2018). Single-cell transcriptomes from human kidneys reveal the cellular identity of renal tumors. Science 361, 594–599. doi:10.1126/science.aat1699
Yu, K., Shi, C., Toral-Barza, L., Lucas, J., Shor, B., Kim, J. E., et al. (2010). Beyond rapalog therapy: Preclinical pharmacology and antitumor activity of WYE-125132, an ATP-competitive and specific inhibitor of mTORC1 and mTORC2. Cancer Res. 70, 621–631. doi:10.1158/0008-5472.CAN-09-2340
Yuan, J.-H., Yang, F., Wang, F., Ma, J.-Z., Guo, Y.-J., Tao, Q.-F., et al. (2014). A long noncoding RNA activated by TGF-β promotes the invasion-metastasis cascade in hepatocellular carcinoma. Cancer Cell 25, 666–681. doi:10.1016/j.ccr.2014.03.010
Zhang, H. M., Yang, F. Q., Yan, Y., Che, J. P., and Zheng, J. H. (2014). High expression of long non-coding RNA SPRY4-IT1 predicts poor prognosis of clear cell renal cell carcinoma. Int. J. Clin. Exp. Pathol. 7, 5801–5809.
Zhao, H., Ljungberg, B., Grankvist, K., Rasmuson, T., Tibshirani, R., and Brooks, J. D. (2006). Gene expression profiling predicts survival in conventional renal cell carcinoma. PLoS Med. 3, e13. doi:10.1371/journal.pmed.0030013
Zheng, B., Mao, J. H., Qian, L., Zhu, H., Gu, D. H., Pan, X. D., et al. (2015). Pre-clinical evaluation of AZD-2014, a novel mTORC1/2 dual inhibitor, against renal cell carcinoma. Cancer Lett. 357, 468–475. doi:10.1016/j.canlet.2014.11.012
Zhou, D., Fu, H., Zhang, L., Zhang, K., Min, Y., Xiao, L., et al. (2017). Tubule-derived wnts are required for fibroblast activation and kidney fibrosis. J. Am. Soc. Nephrol. 28, 2322–2336. doi:10.1681/ASN.2016080902
Keywords: renal fibrosis, renal cell carcinoma, cancer-associated fibroblast, mTOR, TGF-β
Citation: Chen J-Y, Yiu W-H, Tang PM-K and Tang SC-W (2023) New insights into fibrotic signaling in renal cell carcinoma. Front. Cell Dev. Biol. 11:1056964. doi: 10.3389/fcell.2023.1056964
Received: 29 September 2022; Accepted: 17 January 2023;
Published: 21 February 2023.
Edited by:
Jianping Chen, Shenzhen Traditional Chinese Medicine Hospital, ChinaReviewed by:
Ila Pant, Icahn School of Medicine at Mount Sinai, United StatesLibang Yang, University of Minnesota Twin Cities, United States
Copyright © 2023 Chen, Yiu, Tang and Tang. This is an open-access article distributed under the terms of the Creative Commons Attribution License (CC BY). The use, distribution or reproduction in other forums is permitted, provided the original author(s) and the copyright owner(s) are credited and that the original publication in this journal is cited, in accordance with accepted academic practice. No use, distribution or reproduction is permitted which does not comply with these terms.
*Correspondence: Sydney Chi-Wai Tang, c2N3dGFuZ0Boa3UuaGs=