- 1Department of Plastic and Burn Surgery, West China Hospital, Sichuan University, Chengdu, China
- 2Department of Plastic Reconstructive and Aesthetic Surgery, West China Tianfu Hospital, Sichuan University, Chengdu, China
- 3Plastic Surgery Hospital, Chinese Academy of Medical Sciences and Peking Union Medical College, Beijing, China
Refractory skin defects such as pressure ulcers, diabetic ulcers, and vascular ulcers represent a challenge for clinicians and researchers in many aspects. The treatment strategies for wound healing have high cost and limited efficacy. To ease the financial and psychological burden on patients, a more effective therapeutic approach is needed to address the chronic wound. MSC-derived exosomes (MSC-exosomes), the main bioactive extracellular vesicles of the paracrine effect of MSCs, have been proposed as a new potential cell-free approach for wound healing and skin regeneration. The benefits of MSC-exosomes include their ability to promote angiogenesis and cell proliferation, increase collagen production, regulate inflammation, and finally improve tissue regenerative capacity. However, poor targeting and easy removability of MSC-exosomes from the wound are major obstacles to their use in clinical therapy. Thus, the concept of bioengineering technology has been introduced to modify exosomes, enabling higher concentrations and construction of particles of greater stability with specific therapeutic capability. The use of biomaterials to load MSC-exosomes may be a promising strategy to concentrate dose, create the desired therapeutic efficacy, and maintain a sustained release effect. The beneficial role of MSC-exosomes in wound healing is been widely accepted; however, the potential of bioengineering-modified MSC-exosomes remains unclear. In this review, we attempt to summarize the therapeutic applications of modified MSC-exosomes in wound healing and skin regeneration. The challenges and prospects of bioengineered MSC-exosomes are also discussed.
1 Introduction
Wounds are an underestimated health problem that lay a great financial burden on patients and healthcare systems (Frykberg and Banks, 2015). As the primary immune barrier against external damage, the skin is the largest organ in the human body that can regulate thermostability and sense extrinsic stimuli (Takeo et al., 2015). The wound healing process is complex and involves inflammation, cell migration, angiogenesis, and granulation tissue formation, and remodelling of the extracellular matrix (ECM) (Cavanagh et al., 2005; Gushiken et al., 2021). A chronic wound, however, is commonly characterized by prolonged inflammation, persistent infection, the formation of microbial biofilms and failure of epithelial cells respond to stimuli (Schultz et al., 2003; Frykberg and Banks, 2015). According to their etiology, the chronic wounds can be classified as diabetic ulcers, pressure ulcers, and vascular ulcers (Nunan et al., 2014). Due to the healing process’s intricacies, treating chronic wounds is still a great challenge for clinicians.
Exosomes derived from mesenchymal stem cells (MSC-exosomes) with regenerative and immunomodulatory functions have been shown to be beneficial in wound healing and to accelerate the process (Casado-Díaz et al., 2020). Compared with conventional treatment methods, MSC-exosome-based therapies have shown higher therapeutic efficacy that play a role throughout all stages of wound healing (Vu et al., 2021). In addition, MSC-exosome-based therapies avoid the risks of immune rejection and tumorigenesis associated with stem cell transplantation (Yin et al., 2019). Therefore, MSC-exosome-based therapy is considered to be a promising therapeutic approach in wound repair and skin regeneration.
In clinical settings, the application of exosome-based therapy may still face some challenges. The challenging task of isolation, purification, and large-scale production of exosomes represents a direct obstacle to the development of exosome-based treatment from bench to bed (Riau et al., 2019). Exosomes may also undergo rapid clearance in the blood circulation or show poor retention at the wound surface (Agrahari et al., 2016; Riau et al., 2019). In addition, safety concerns have limited the clinical applications of MSC-exosomes. The introduction of bioengineering technologies into MSC-exosome-based therapies is expected to address the practical problems mentioned above.
Ideally, modified exosomes could be loaded with luminal cargos or display specific surface molecules, thereby increasing the efficiency of the exosomes and giving them additional therapeutic effects (Riazifar et al., 2017; Wiklander et al., 2019; Salunkhe et al., 2020). Genetic engineering of progenitor cells and direct modification of exosomes are the main engineering strategies for altering exosomes and increasing their regenerative effectiveness (Luan et al., 2017; Shi et al., 2020a). Other strategies such as engineering of hybrid exosomes may also be used in exosome modification (Chen et al., 2020). Furthermore, encapsulating exosomes into hydrogel scaffolding can be used to form a sustained release system or wound dressing (Liang et al., 2019; Bai et al., 2022; Hu et al., 2022). Preclinical studies and clinical trials of chronic wound models have shown promising results, demonstrating that modified MSC-exosomes could promote angiogenesis, fibrogenesis, re-epithelization, and granulation tissue formation, as well as attenuating inflammation (Shi et al., 2020b; Li et al., 2020; Wu et al., 2021a; Xiong et al., 2022). The application of hydrogels may have a synergistic effect in wound closure (Das et al., 2022). Despite a lack of systematic evidence, bioengineered modified MSC-exosomes are increasingly recognized as beneficial to compensate for the limitations of natural exosome-based clinical therapies in wound healing applications.
In this review, we focus on promising bioengineering modifications of MSC-exosomes that enhance their potential therapeutic effectiveness in wound healing and skin regeneration. The applications of biomaterials loaded with modified MSC-exosomes in wound dressing strategies are also addressed. Finally, the challenges and prospects of tissue-engineered MSC-exosomes are discussed. We hope to provide a valuable overview of the role and the mechanisms of modified MSC-exosomes in cutaneous wound healing and elaborate on the potential of applying modified MSC-exosomes in clinical practice.
2 Wound healing and regenerative management
The primary molecular regulators of wound healing are proteins and polypeptides including growth factors, cytokines, and chemokines (Das et al., 2015; Guo et al., 2018; Kim et al., 2019; Su et al., 2019). During the past decades, with advances in regenerative medicine, significant efforts have been made to explore solutions to improve the tissue regeneration process, thereby repairing and correcting physiological deficiencies. Such approaches include using growth factors, stem cells, and biomaterials to induce a more effective healing process. However, despite promising therapeutic results in preclinical studies, the clinical applications of these strategies have been limited by concerns including tissue origin, standards for isolation and culture procedures, tumorigenicity, and ethical regulatory restrictions on the use of stem cells.
As stem cells have self-renewal capacity and multi-lineage differentiation potential, they have been extensively explored with respect to their potential applications in treatment of chronic wounds (Donati and Watt, 2015; Chen et al., 2016). However, the immunogenicity and tumorigenesis of stem cells are critical factors affecting their therapeutic function. Owing to their comparatively low immunogenicity, MSCs represent a promising source for cell-based therapies for wound healing (Lee et al., 2014). Accumulating evidence demonstrates that the multi-lineage differentiation of MSCs and their secretion of bioactive factors have potential therapeutic value in the treatment of various diseases (Pittenger et al., 1999; Zhou et al., 2021). However, despite promising results in preclinical studies and clinical trials, MSCs fall short of expectations in clinical settings.
The main limiting factors in the therapeutic use of MSCs are their heterogeneity and limited homing capacity in damaged tissue (Ullah et al., 2019; Levy et al., 2020). On the one hand, MSCs may undergo senescence during extended culture (Fang et al., 2018; Tabibzadeh, 2022). On the other hand, the transplantation of MSCs could induce tumorigenic scenarios. The most critical issue is that the preparation process of MSCs remains experimentally challenging. It is also difficult to reproduce the therapeutic potency of an MSC.
Previous research has attributed the therapeutic effects of MSC-based therapies to the multi-lineage differentiation and engrafting capacities of the MSCs. The current view is that the release of extracellular vesicles (EVs) through paracrine mechanisms is the major mediator of their therapeutic activities (Lener et al., 2015; Li et al., 2018a; Zhang et al., 2019a). Various in vitro studies and preclinical disease models have reported that EVs isolated from MSCs cultures display therapeutic activities that equivalent to those of MSCs (Giebel et al., 2017). Therefore, the secretome from MSCs has attracted much attention for its potential use in tissue repair and regeneration.
3 EVs from MSCs
3.1 EV biogenesis
The term “extracellular vesicles” refers to a group of cell-derived lipid bilayer membranous structures containing transmembrane proteins and RNAs (Lötvall et al., 2014; Théry et al., 2018; Mathieu et al., 2019). Work in the late 1960s first discovered and recorded the presence of vesicles around cells in human plasma (Wolf, 1967). However, the generic term “exosome” was not proposed until 1987 (Johnstone et al., 1987). According to their biogenesis processes, EVs can be classified into three major subtypes: (a) microvesicles, budding from the plasma membrane, with size between 50 and 1000 nm; (b) exosomes: a group of relatively smaller membrane vesicles (30–120 nm) secreted from the endosomal system and formed through the inward budding of multivesicular bodies (MVBs) that transfer molecules into target cells; and (c) apoptotic bodies: derived from fragments of apoptotic cells, sized between 800 and 5,000 nm. As there is no standard production protocol for MSC-EVs, the term “MSC-exosomes” was used to describe exosome-containing products derived from MSCs (Gimona et al., 2021).
The biogenesis of exosomes comprises an endosomal sorting complex required for transport (ESCRT)-dependent mechanism and an ESCRT-independent mechanism (Trajkovic et al., 2008; Kajimoto et al., 2013; Mathieu et al., 2019). The ESCRT-dependent process starts with the inward budding of the bilayer membrane of late endosomes, resulting in the formation of intraluminal vesicles. The bilayer membrane bubble-filled endosome is called an MVB. The maturation of MVBs ends with the excretion of the exosomes into the extracellular space. The ESCRT-independent mechanism can also produce intraluminal vesicles. The biogenesis of microvesicles mainly involves the outward budding of the cell plasma membrane and degradation of the cytoskeleton (Shao et al., 2018). However, other mechanisms may be also involved in the biogenesis of microvesicles, for instance, conversion between ceramide and sphingomyelins (van Niel et al., 2018).
3.2 EV isolation and characterization
Differential ultracentrifugation is the most frequently used method for isolation of EVs and remains the “gold standard” (Théry et al., 2018). However, differential ultracentrifugation, which can only be performed for up to about 500 ml of culture medium per run, is unsuitable for large-scale purification (Witwer et al., 2019). Density gradient centrifugation is an improved ultracentrifugation method that produces EVs with higher purity. Although other methods such as serial washes can improve purity, they are associated with low production, risk of structural damage, and contamination (Webber and Clayton, 2013). Therefore, ultracentrifugation may not be an optimal method for therapeutic applications.
Size-based isolation methods include ultrafiltration, size exclusion chromatography, and tangential flow filtration (Li et al., 2017; Willis et al., 2017; Wu et al., 2021b). These methods can produce EVs with comparable or superior purity and/or functional activity to those produced by conventional methods (Busatto et al., 2018; Ludwig et al., 2018); however, pore clogging may occur during the filtration process, resulting in low yield.
Polymer precipitation with polyethylene glycol (PEG) or other polymers could be an effective way to achieve “salting out” of EVs to aggregate (Doyle and Wang, 2019). In clinical applications, PEG or other polymers are considered as contaminants that need to be removed (Doyle and Wang, 2019). Immunoaffinity capture technology, in which antigens are bound to antibodies on the surface of EVs, allows isolation of specific EVs with certain surface proteins.
With the development of the EV research field, various novel strategies have been developed to isolate EVs. However, as there are no standard evaluation metrics for MSC-EV preparation, and no proprietary matrices have been specified, it is difficult to assess the clinical usability of the resulting EVs, as well as the safety of the ingredients used in these EV-harvesting strategies (Reiner et al., 2017).
According to the Minimal Information for Studies of EVs (MISEV) guidelines, the characterization of isolated EVs needs to address morphology, particle size, and surface biomarkers (Théry et al., 2018). The morphology of EVs is observed by scanning electron microscopy, transmission electron microscopy (Théry et al., 2018), electron cryo-microscopy, and atomic force microscopy (Wu et al., 2021b). The size distribution and concentration of EVs are usually determined by nanoparticle tracking analysis, dynamic light scattering, and tunable resistive pulse sensing (An et al., 2021). Common biochemical analysis methods for EVs include western blotting, flow cytometry, and liquid chromatography with mass spectrometry. As the size ranges of exosomes and microvesicles may overlap, these two subtypes cannot be differentiated by size alone.
According to minimal criteria for MSCs suggested by the International Society for Cell and Gene Therapy (ISCT), surface antigens may be the most detectable characteristics that can be transferred to EVs (Dominici et al., 2006). MSC-EVs could be identified based on recently reported MSC-specific surface antigens including CD73, CD90, and CD105, and by the absence of CD14, CD34, and CD11b by Western blot or ELISA (Witwer et al., 2019). The proteomic and lipidomic signatures of MSC-exosomes samples can also be utilized for the identification, characterization, and verification of MSC-exosomes. Previous research revealed that the origin of EV subtypes can be defined by identifying the specific binding affinity, such as cholera toxin B chain (CTB), annexin V (AV), and Shiga toxin B chain (ST) on lipid membrane (Lai and Lim, 2019). And the signature analysis of a specific set of proteins might help in quality control of the preparation and standardization of MSC-exosomes (van Balkom et al., 2019).
4 MSC-exosomes in skin regenerative medicine
MSCs that secrete effectors can be loaded into EVs, thus enabling them to exert bioactive effects (Fu et al., 2019). Exosomes are thought to be the critical bioactive EVs responsible for the paracrine effects of MSCs (Joo et al., 2020), which can be secreted from all types of cells in the human body. According to high-throughput exosome studies, exosomes consist of and carry a diverse load of proteins (Toh et al., 2018), lipids (Davidson et al., 2022), metabolites, RNAs (Fan et al., 2018; Zhang et al., 2019b). Almost 350,000 proteins and more than 27,000 mRNAs and 10,000 miRNAs have so far been detected in exosomes, based on data from Vesiclepedia (http://microvesicles.org/, accessed on 1 August 2022).
There is a growing recognition that MSC-derived exosomes are the initial mediators of the therapeutic efficacy of MSCs, maintaining the biological activity and therapeutic effects of progenitor MSCs (Yeo et al., 2013; Brennan et al., 2017; Ramasubramanian et al., 2019; Yin et al., 2019). The mechanisms underlying the therapeutic potential of MSC-exosomes involve transmission of intercellular information through direct binding to surface ligands via ligand–receptor interactions (Chen et al., 2018; Theodoraki et al., 2018; Yang et al., 2018) or transfer of genetic information and proteins into acceptor cells through cellular internalization or membrane fusion (Skog et al., 2008; Möller and Lobb, 2020) to alter the biological properties of target cells. Research indicates that luminal vehicles within exosomes are absorbed by recipient cells by three methods: direct membrane fusion (Duan et al., 2021), endocytosis (Gurung et al., 2021), and receptor–ligand interactions (Salunkhe et al., 2020).
MSC exosome-based therapies are thought to circumvent the hurdles faced by MSC-based therapies. The main advantages of MSC-exosomes can be summarized as follows. First, the isolation, concentration, storage, and therapeutic dosage of exosomes are more controllable (Witwer et al., 2013). Second, the phospholipid bilayer structure can merge directly with target cells, releasing functional cargos in the receiving cells. Third, exosome-based therapies can avoid the risks of immune rejection and tumorigenesis (Tan et al., 2021). Therefore, MSC-exosomes, as a promising cell-free therapy, exhibit high potential in wound healing and skin regeneration.
4.1 Therapeutic potential of MSC-exosomes in skin tissue regeneration
The pathology of wound healing is regulated by a well-orchestrated processed including inflammation, re-epithelialization, reformation, angiogenesis, and collagen remodeling (Singer and Clark, 1999). MSC-exosomes are believed to have equivalent biological effects to MSCs in tissue regeneration, homeostasis, and wound healing (Yeo et al., 2013; Ramasubramanian et al., 2019; He et al., 2022; Luo et al., 2022; Yan et al., 2022). They can exert their effects on receptor cells by modifying gene expression and protein production. Research efforts have been undertaken to develop MSC-exosomes as therapies in wound healing.
Several studies have examined the effects of MSC-exosomes on the prolonged inflammatory phase in chronic wounds. Anti-inflammatory M2 macrophages have essential roles in promoting cell proliferation and tissue regeneration (Singer and Clark, 1999; Das et al., 2015; Guo et al., 2018). He et al. revealed that bone marrow MSC (BMMSC)-derived exosomes could modulate M2 polarization to promote cutaneous wound healing (He et al., 2019). Adipose tissue-derived stem cell (ADSC)-exosomes have been proven to attenuate macrophage infiltration and reactive oxygen species production (Xu et al., 2020).
Several studies have reported that MSC-exosomes contribute to wound healing with an emphasis on proliferation. Angiogenesis and re-epithelization are important tasks during the proliferation phase of wound healing (Tonnesen et al., 2000; Sorrell et al., 2009). ADSC-exosomes have been reported to promote the proliferation and migration of vascular endothelial cells and sprouting of vascular endothelial tip cells, and to rejuvenate senescent endothelial cells in vivo and in vitro. Ren et al. reported that the angiogenesis induced by ADSC-exosomes in human umbilical vein endothelial cells was associated with the upregulation of platelet-derived growth factors, VEGFA, EGF, and FGF via AKT and ERK signaling pathways (Ren et al., 2019). In a mouse model of a full-thickness skin defect, exosomes from fetal dermal MSCs could accelerate wound healing by activating Notch signaling (Wang et al., 2019a). In an ischemic disease model, miRNA-31 in ADSC-exosomes targeted factor inhibiting HIF-1 (FIH-1) in recipient cells to promote angiogenesis (Zhao et al., 2017; Bian et al., 2019). In addition, preclinical studies in mouse injury models have shown that BMMSC-exosomes accelerate wound healing by promoting collagen synthesis and angiogenesis (El-Tookhy et al., 2017; He et al., 2019).
Human-induced pluripotent stem cells can be generated from a variety of adult cell types after genetic modification. Exosomes from induced pluripotent stem cells (hiPSC)-MSCs can accelerate cutaneous wound healing by promoting vascularization and fibroblast proliferation in rats. After an injury, wounds treated with hiPSC-MSC-exosomes showed improved re-epithelialization and formation of skin appendices, including sebaceous glands and hair follicles. Increasing expression of CD-31 and α-SMA indicates proliferation of endothelial cells and smooth muscle cells that are closely related to vascularization (Zhang et al., 2015). Notably, hiPSC-MSC-exosomes were also found to enhance collagen synthesis and fibroblast proliferation in a dose-dependent manner of human umbilical vein endothelial cells in vitro. The proliferation of human keratinocytes (HaCaT cells) and human dermal fibroblasts was also found to increase significantly after treatment with hiPSC-MSC-exosomes (Kim et al., 2018).
MSC-derived exosomes also have prominent regenerative effects on collagen deposition and can reduce scar deposition. Data suggest that exosomes from human ADSCs can promote fibroblast proliferation and migration through the PI3K/Akt signaling pathways following subcutaneous injection of umbilical cord blood (UCB)-exosomes at full-thickness cutaneous wounds on the backs of mice (Zhang et al., 2018). Another study confirmed the beneficial effects of exosomes from human umbilical cord blood plasma (hUC-MSCs) on wound healing. Levels of collagen I, collagen III, and α-SMA were downregulated after treatment with hUC-MSC-derived exosomes, and expression of myofibroblast-related protein was decreased. In addition, hUC-MSCs have been found to inhibit the activation of the TGF-β1/Smad2/3 signaling pathway. These data indicate that hUC-MSC-derived exosomes could inhibit the transition of fibroblasts to myofibroblasts (Hu et al., 2020).
However, exosome therapeutics for wound healing still face challenges regarding their clinical applications, including poor targeting, a rapid clearance rate, and a relatively short half-life in the complex microenvironment of the wound (Liu et al., 2017). Quantitative analysis of exosome-internalized skin cells show a time-dependent decrease 24 h after injection (Hu et al., 2018). Furthermore, the functions of these cells may have been compromised by the time-consuming harvesting and passage processes and the low survival rate. For these reasons, tissue engineering technology has been introduced to modify the therapeutic potential of natural exosomes, enabling the design of exosomes higher stability and greater therapeutic capability in accelerating wound healing and skin regeneration.
4.2 Challenges in the development of MSC-exosomes-based therapies
Although several preclinical studies have shown promising result, some challenges still hinder the development of exosome-based treatments (Liu et al., 2017; Hu et al., 2018). In clinical settings, the drawbacks of exosome-based therapy may outweigh the advantages. Challenges in achieving a therapeutic effect in clinical settings are found throughout the whole process of MSC-exosome preparation.
To date, various studies are evaluating the biodistribution of exosomes obtained from different sources and used for treatment in several disease models. The underlying mechanism of action (MoA) of MSC-exosomes in each stage of wound healing needs further elucidating. To achieve the therapeutic effect, MSC-exosomes need to present on the target site in a spatiotemporal manner. However, no specific markers are yet available to specifically label and track the biodistribution of MSC-exosomes (He et al., 2019). Moreover, to elucidate the MoA of the therapeutic MSC-exosomes is often implemented by gene editing of the donor MSCs to down-regulated the candidate RNAs or proteins of MSC-exosomes, which may eventually lead to major alterations of MSC-exosomes products (Théry et al., 2018).
The variability of parental MSC sources, the processes for isolation, purification, and identification of exosomes, and the need for a standardized definition of MSC-exosomes need to be addressed (Witwer et al., 2019). In addition, the therapeutic efficacy of MSC-exosomes may be affected by local conditions, the delivery route, and the time window for intervention. For clinical applications of MSC-exosomes, xenogenic components should be avoided during the preparation process. Non-MSC-exosomes derived from the culture media may exist (Witwer et al., 2019).
After administration, the biological effects of MSC-exosomes should be elicited only after they have been engulfed by the target cells. Otherwise, MSC-exosomes would be depleted by immune cells in the circulation. Exosomes are often administered intravenously, subcutaneously, or intraperitoneally (Figure 1). There are also drawbacks also associated with the administration of the exosomes. Systemic administration of exosomes may cause an instant inflammatory reaction and rapid clearance from the blood circulation, resulting in insufficient residence time and homing. The half-life of local administration of exosomes may be even shorter with low bioavailability owing to flushing by body fluid, resulting in a short retention rate of exosomes (Agrahari et al., 2016; Riau et al., 2019). Moreover, therapeutic efficacy may differ between exosomes harvested from in vitro MSC culture and exosomes secreted by MSCs after administration in vivo.
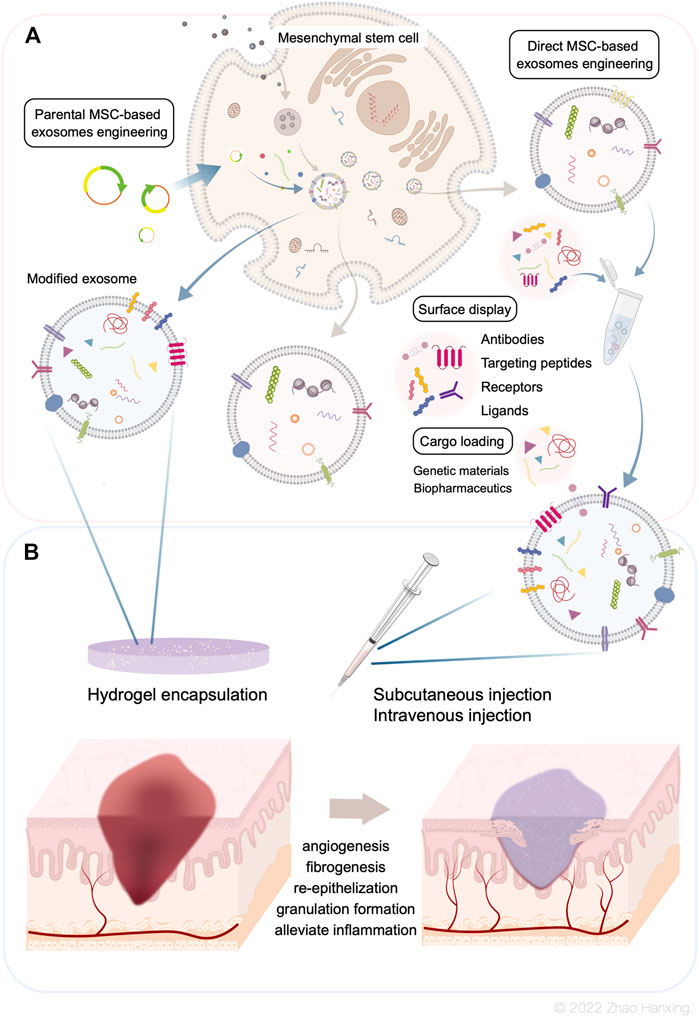
FIGURE 1. (A) Biogenesis of Mesenchymal stem cell-derived exosomes. The bioengineering modifications include genetic modification of MSCs and direct modification of exosomes. (B) Current administration procedures of modified exosomes are co-administration with hydrogels and administered by intravenous, subcutaneous, or intraperitoneal injections. Modified MSC-exosomes could accelerate skin wound repair by promoting angiogenesis, fibrogenesis, re-epithelization, and granulation tissue formation, as well as alleviate inflammation.
5 Bioengineering modified MSC-exosomes
To solve the problems of low effective concentration and limited therapeutic effect, researchers have introduced bioengineering methods into exosome-based therapy. This has resulted in the design of highly specialized bioengineering-modified MSC-exosomes. The features of modified MSC-exosomes are as follows: targeted binding to a specific cell type or tissue; the capacity for loading molecules, drugs, proteins, or genetic information into exosomes or on their surface; and enrichment of an endogenous molecule in the lumen of exosomes or on their surface (Kojima et al., 2018). Current bioengineering methods fall into two main categories: parental MSC-based exosome engineering and direct MSC-exosome engineering (Figure 1A). Several studies have shown the therapeutical roles of bioengineered MSC-exosomes in tissue regeneration and the treatment of CNS disease and cancer (Figure 2) (Haney et al., 2015; Samadikuchaksaraei et al., 2016; Togliatto et al., 2016; Gilligan and Dwyer, 2017; Tao et al., 2017; Yuan et al., 2017; Li et al., 2018b; Liao et al., 2018; Luo et al., 2019; Mathew et al., 2019; Sisa et al., 2019; Kaminski et al., 2020; Xuan et al., 2020; Nuzzi et al., 2021; Sandonà et al., 2021; Yao et al., 2021; Dong et al., 2022; Kurniawati et al., 2022; Miyasaki et al., 2022; Thomas et al., 2022; Rong et al., 2023).
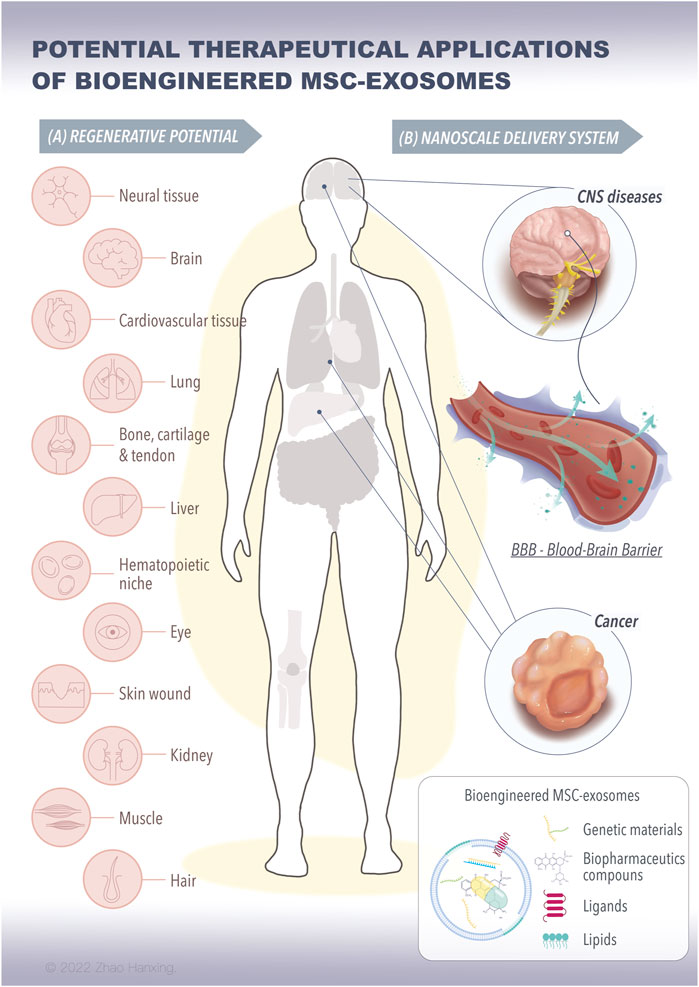
FIGURE 2. The potential therapeutical applications of bioengineered MSC-exosomes for tissue regeneration and nanoscale delivery system. (A) The bioengineered MSC-exosomes loading with specific signaling molecules, such as proteins, RNAs, and nanoparticles can achieve repair and regenerative effects on various tissues. (B) The bioengineered MSC-exosomes can cross the BBB in the brain or to the tumor for the treatment of CNS diseases and cancer. (MSC, mesenchymal stem cells, BBB, blood-brain barrier, CNS, central nervous system).
5.1 Parental MSC-based exosome engineering
The genetic engineering of parental MSCs produces specific exosomes loaded with functional surface display molecules to specificically label MSC-exosomes or increase exosome accumulation. The most common procedure for encapsulating a protein at the surface of exosomes is the use of an exosome signal peptide such as lysosome-associated membrane protein 2b (LAMP2b) (Alvarez-Erviti et al., 2011). The LAMP2b membrane protein combined with a neuron-targeted short peptide of rabies virus glycoprotein (RVG) is expressed. RVG displayed on the surface may lead to the accumulation of exosomes and enhance exosome delivery (Alvarez-Erviti et al., 2011; Barile and Vassalli, 2017). Vesicular stomatitis virus glycoprotein is an exosome-anchored transmembrane protein containing ectoplasmic, transmembrane helix, and cytoplasmic domains (Meyer et al., 2017). Specific proteins can replace the ectoplasmic and cytoplasmic domains without altering the structures of the exosome membrane. Other documented exosome surface proteins include tetraspanins (CD63, CD9, CD81) (Mathieu et al., 2021; Shi et al., 2021), glycosylphosphatidylinositol (GPI)-anchored cadherin (Nakamura et al., 2020), platelet-derived growth factor receptors (Ohno et al., 2013), and lactadherin (C1C2 domain) (Tian et al., 2021), which could be used for similar purposes.
The EXOtic device can be used to carry mRNAs into parental MSCs to improve exosome production, deliver mRNAs into recipient cells, and promote cell-to-cell communication (Kojima et al., 2018). CRISPR/Cas9 technologies are employed for in vivo gene editing. For example, CRISPR/Cas9 has been used to knock down specific gene sequences in human umbilical MSCs to enhance therapeutic effects (Shao et al., 2020). Such strategies have great potential for gene manipulation in exosome-based therapy and are awaiting further investigation. Transfection has been used to incorporate small RNAs and endogenous molecules into exosomes via loading into parental MSCs. Parental MSCs co-transfected with plasmids encoding production enhancement genes may increase exosome production by 15- to 40-fold as determined by measuring a luminescent reporter (Kojima et al., 2018).
Genetic modification of parental MSCs seems feasible for improving protein surface display, increasing yield of exosomes, loading specific mRNAs into the exosomes, and increasing exosome uptake by recipient cells without any alteration in exosome structures. However, alteration of genetic information may not be suitable for use in exosomes for clinical applications.
5.2 Direct MSC-exosome engineering
Direct exogenous loading approaches after exosome isolation include co-incubation, electroporation, and sonication; other methods such as freeze-thaw and extrusion are rarely used (Figure 1).
Co-incubation is a frequently used strategy to maintain the integrity of the exosome membrane and is especially suitable for loading of hydrophobic molecules such as drugs and RNAs (Xiao et al., 2022; Zhuang et al., 2022). Electroporation is a promising strategy for loading small-molecule drugs or nucleotides into exosomes by creating small-transit pores on the phospholipid bilayer (Lv et al., 2020; Xiong et al., 2022). The membrane gap is automatically restored without altering the morphology of the exosomes. Given that large compounds cannot easily be encapsulated in exosomes, the electroporation approach is particularly suitable for loading nucleotides (Luan et al., 2017). Sonication is also a popular method for exosome engineering (Haney et al., 2015). Exogenous cargo diffuses into the exosomes by passing through the membrane breaks caused by a probe’s mechanical force. Although the loading efficiency of the sonication method is higher than those of co-incubation and electroporation, this approach has some important shortcomings. For instance, the high power of sonication may cause irreversible deformation of exosomes and may disrupt the integrity of exosomes (Haney et al., 2015; Luan et al., 2017).
Extrusion can be used to load exogenous cargos such as gold nanoparticles into exosomes (Khongkow et al., 2019; Van Deun et al., 2020). Freeze–thaw methods have been used to load water-soluble molecules into exosomes by forming ice crystals within lipid membranes that temporarily break the integrity of the exosome membrane (Haney et al., 2015; Hajipour et al., 2021). According to a previous study, extrusion and freeze-thaw methods have notable drawbacks, including low efficiency, low throughput, and the risk of altering the membrane stability of exosomes, resulting in unpredictable side effects (Fuhrmann et al., 2015; Haney et al., 2015; Van Deun et al., 2020). Therefore, such methods are not conventionally used for loading cargo into exosomes.
Exosomes can be modified by using chemical engineering strategies such as click chemistry and metabolic glycoengineering to conjugate ligands to the exosome surface, enabling regulation of the directional migration and targeting of exosomes (You et al., 2021). The advantage of this method is that the highly effective reaction can maintain the size of exosomes without any undesirable side reaction related to the association between exosomes and recipient cells (Smyth et al., 2014; Yoon et al., 2017).
Engineered hybrid exosomes are emerging as an alternative strategy for encapsulating and transferring large nucleic acids such as DNA to target cells and organs and exert therapeutic effects (Lin et al., 2018). Fusing exosomes with synthetic liposomes modifies and tunes the exosome interface to decrease immunogenicity, increase colloidal stability, and improve the half-life in circulation (Lin et al., 2018). Composite carriers enable the efficiency of drug loading to be improved while retaining the function of exosomes. However, there have been few comprehensive evaluations of hybrid exosomes. Hybrid exosomes have been reported to show cytotoxicity slightly greater than that of pure exosomes, as demonstrated by their effects on cell proliferation, which was attributed to the conjugation of the nanoparticles (Chen et al., 2020). As the incubation time or the concentration of hybrid exosomes increased, the viability of progenitor cells decreased.
In summary, various therapeutic molecules and nanoparticles can be efficiently transferred to exosomes using the abovementioned modification procedures. However, these methods require repeated purification and centrifugation using detergents, enzymes or other methods, which might disrupt the integrity of exosomes or reduce bioactivity (Levy et al., 2022). Therefore, novel bioengineering procedures are needed to compensate for these limitations.
5.3 Co-administration with hydrogels
Deepening understanding of exosome-based therapies has spurred research combining biomaterials to formulate sustained-release therapeutic systems. Improving the ability of MSC-exosomes to accelerate wound healing is the driving force behind the research integrating biomaterials and bioengineering. Ideal biomaterials can be used to maximize the therapeutic functions of exosomes as wound dressings or scaffolds by increasing their durability and stability.
A variety of biomaterials, including membranes, electrospun nanofibers, colloidal nanoparticles, and hydrogels, have been used to facilitate the controlled release of bioactive molecules for skin regeneration (Liang et al., 2019; Bai et al., 2022; Hu et al., 2022). Among them, hydrogels have recently attracted attention for applications as delivery systems and scaffold dressings with multifunctional properties such as antibacterial activity, hemostatic ability, tissue adhesion, anti-ultraviolet activity, injectability, and self-healing (Lokhande et al., 2018; Safari et al., 2022). Hydrogels can mimic natural extracellular matrix (ECM) and provide a three-dimensional framework to support growth and proliferation of loaded cells and regulate biomolecule activation (Riha et al., 2021). Moreover, three-dimensional hydrophilic polymer networks can maintain moisture at the wound site.
The combination of hydrogels and exosomes plays a crucial part in adjusting the wound inflammation microenvironment, promoting vascularization, enhancing re-epithelialization, and accelerating wound healing (Qian et al., 2020; Zhang et al., 2021a; Kudinov et al., 2021; Geng et al., 2022). Furthermore, a comparative study has demonstrated a synergistic effect of hydrogel–exosome complexes compared with exosome therapy alone (Zhou et al., 2022).
In summary, although several studies have shown great potential for MSC-exosomes in clinical applications, many unresolved issues still limit their practical use. Before exosomes are introduced into clinical settings, safety concerns, cytotoxicity, and reproducibility of production need to be solved (Luan et al., 2017; Murphy et al., 2019). The development of bioengineering methodologies for exosome-based therapy could provide a means of bridging the gap.
6 Applications of bioengineering-modified MSC-derived exosomes in skin wound healing and regeneration
In this section, we review the therapeutic applications of modified MSC-exosomes in wound healing and skin regeneration and the improvements in regenerative efficiency that have been achieved compared with MSC-derived exosomes. As mentioned in the previous discussion on modified exosomes and bioengineering technology, there are two current trends for implementing modified MSC-exosomes in wound healing and skin regeneration.
6.1 Bioengineering the properties of MSC-exosomes
One solution is to bioengineer the properties of exosomes, such as their cargos or surface molecular functions, before administration (Table 1).
The RNA cargo of MSC exosomes is considered a pivotal factor in eliciting biological functions to target cells and has raised great attention. Previous mechanistic studies have shown that exosome-derived RNAs play a crucial role in immunomodulatory capacity (He et al., 2019; Zhao et al., 2021; Wang et al., 2022), stimulate the differentiation of fibroblasts, keratinocytes, and endothelial cells, promote angiogenesis (Liang et al., 2016), and prohibit tissue hyperplasia and scar formation (Fang et al., 2016). Such abilities can be enhanced after bioengineering modification. For example, modified exosomes derived from bone marrow MSCs (BMMSCs) enriched with miRNA-542-3p could enhance proliferation, migration, and angiogenesis of human skin fibroblasts/human dermal microvascular endothelial cells in vitro and in vivo (Xiong et al., 2022). The potential therapeutic value of exosomes loaded with circular RNAs has been studied in full-thickness skin wound repair in diabetic rats. The MSC-exosomes enriched with mmu_circ_0000250 could efficiently regulate miR-128-3p and the expression of SIRT1 to control inflammation (Shi et al., 2020b). Another study modified MSCs to generate exosomes enriched for long ncRNA H19, leading to the regulation of the PI3K/AKT signaling pathway and suppression of inflammatory responses and apoptosis in a diabetic foot ulcer mouse model (Li et al., 2020). Nanoparticles can be loaded into the exosomes to promote angiogenesis. BMSCs-exosomes loaded with magnetic Fe3O4 nanoparticles, with upregulation of exosome miR-1260a, were confirmed to enhance angiogenesis (Wu et al., 2021a).
Deep sequencing of MSC exosomal RNA revealed that MSC excretes exosomal miRNAs in a selective manner, many of which are precursor miRNAs incapable of protein-coding (Chen et al., 2010; Pritchard et al., 2012). In addition, a prerequisite for RNA-based MoA is that the exosome-derived miRNAs reach a therapeutic dose. However, the amount of miRNAs in standard preparation is approximately 60 ng per 100 μg exosome. Leaving aside the fact that the distribution of a specific miRNA in an exosome is about 1:100 (Chevillet et al., 2014; Albanese et al., 2021). According to the analysis of Toh WS et al., MSC-exosome-derived miRNAs are unable to achieve the therapeutic effect as their low concentration in single exosome, immature status, and the absence of RNA-induced silencing complex (RISC) (Toh et al., 2018).
The protein-mediated mechanism of MSC-exosomes in wound healing has raised attention (Sung et al., 2019; Bari et al., 2020; Kudinov et al., 2021; Camões et al., 2022; Zhang et al., 2022). Comprehensive proteomic analysis showed that the proteins TGF-β, ITGA1-3/5, IL-6, CDC151, S100A10, and Wnt5α, as being enriched in MSC-exosomes in 3D culture, were confirmed to be associated with wound healing (Camões et al., 2022). In infected burn injury, PGE2, IL-6, IL-8, or IFN-γ, IL-10, growth factors, and chemokines secreted by placental MSC secretome were found to accelerate dermal fibroblast and keratinocyte migration and proliferation, stimulate angiogenesis, and reduce scarring (Kudinov et al., 2021). Angiogenic protein cargos, including angiogenin, angiopoietin-1, HGF, and vascular endothelial growth factor (VEGF) in thrombin preconditioning MSC-exosomes were enhanced compared with that of naïve MSC-exosomes, thus boosting angiogenesis (Sung et al., 2019). As with RNA-based MoA, the proteins should be in therapeutic doses to elicit their therapeutic effect.
6.2 Hydrogel encapsulation of MSC-exosomes
Another bioengineering strategy combines exosomes and hydrogels to exert synergistic functions after administration (Table 2) (Wang et al., 2019b). Hydrogels act as three-dimensional porous scaffolds to provide a favorable environment for cell proliferation and ECM remodeling (Yang et al., 2020). A complex of exosomes and hydrogels can also act as a sustainable release system for an extended period and exert lasting curative functions. The flexible morphology of biomaterials enables the construction of more variable forms of exosomes (Wang et al., 2019c).
MSC-exosomes encapsulated by biomaterials could avoid being rapidly released into the bloodstream and exert their therapeutic effect in a dose-dependent manner at designated sites. Hydrogels can be used as loading vehicles to enhance exosome retention rates and achieve synergistic therapeutic efficacy (Wang et al., 2019b; Das et al., 2022). A thermoactivated polymer has been shown to enable sustained administration of exosomes, maintaining 65% of the initial number of exosomes after 25 days (Das et al., 2022). This study also found no cytotoxic effects on cells in contact with the hydrogels or when cells were embedded in the hydrogel constructs. Surface modification with nanoparticles increases the production of exosomes. Bioavailable nanoparticles based on iron oxide with poly(lactic-co-glycolic acid) were designed to improve the yield of exosomes by stimulating MVB formation (Park et al., 2020).
Biomaterials may also combine engineered exosomes to improve the therapeutic efficiency of exosomes in wound healing (Table 3). As three-dimensional porous scaffolds, hydrogels provide exosomes with a favorable environment that promotes cell proliferation and ECM remodeling and have thus gained more research attention. Furthermore, the transformation of biomaterials provides a greater scope for applications of exosomes (Wang et al., 2019c; Yang et al., 2020). An in vivo study showed that incorporating gingival-MSC-derived exosomes into a chitosan/silk-based hydrogel sponge could facilitate wound healing by promoting re-epithelialization, angiogenesis, and neuronal ingrowth, as well as collagen remodeling (Shi et al., 2017). In a study by Wang et al., an injectable methylcellulose-chitosan hydrogel loaded with exosomes derived from human placental MSCs was constructed to synergistically promote angiogenesis and inhibit apoptosis (Wang et al., 2019b). The modified exosome hydrogel complex forms a suitable injectable wound dressing with appropriate gelation time, mechanical properties, and high self-healing efficiency. In a diabetic wound-infected model, BMSC-exosomes were loaded in an antibacterial hydrogel and enabled the wound inflammation microenvironment to be adjusted, as well as promoting neovascularization and accelerating wound healing (Geng et al., 2022).
6.3 Clinical trials of MSC-EVs for wound healing and skin regeneration
Overall, bioengineering technologies have been implemented in exosome-based therapy to make MSC-derived exosomes more effective in treating chronic wounds. Previous valuable research findings have expanded the scope of applications of exosomes in treatment of cutaneous wounds and established a theoretical basis for clinical trials of bioengineered MSC- exosomes.
7 Prospects and conclusion
Skin wound healing is a complex multi-stage biological process. Chronic wounds characterized by poor vascularization and prolonged inflammation still threaten patients’ health and quality of life. Traditional treatments for skin damage are time-consuming and costly, and existing therapies have little therapeutic effect in promoting chronic wound healing. Therefore, new therapeutic options are needed.
Over the past decades, therapies based on MSCs with multi-lineage potential have shown impressive therapeutic efficacy in regenerative medicine (Pittenger et al., 1999). However, significant challenges regarding immunocompatibility, stability, migration capacity, and pluripotent development still need to be addressed (Jiang et al., 2002). Current opinion is that MSCs exert their therapeutic effects mainly via their paracrine activity, mostly through the release of EVs (Herrera et al., 2010; Lai et al., 2010; Lener et al., 2015). As the main bioactive factor of the MSC secretome, MSC-exosomes are reported to have similar therapeutic effects to MSCs that transfer genetic information or biologically active molecules into target cells (Zhang et al., 2014).
In light of these properties, considerable research efforts have been put into MSC-exosomes therapies. In preclinical models, MSC-exosomes have been shown to have several advantages compared with MSCs, including minimal immune rejection and tumorigenesis risk (Zhang et al., 2014) and no side effects in vivo (Kordelas et al., 2014). However, before translation of MSC-exosome-based therapeutics into clinical settings, several issues need to be addressed.
The challenges include the manufacturing process of MSC-exosomes, such as characterization, isolation, purification, clinical safety of donor cells and recipient cells, and the manufacturing process. In addition, quality control hinders the translation of MSC-exosome-based therapies into clinical applications.
Microvesicles and exosomes have some common characteristics; for instance, they both have a bilateral phospholipid membranous structure and contain specific proteins, lipid, as well as RNAs, and their size ranges overlap; thus, current isolation procedures cannot discriminate different EV subtypes (Gould and Raposo, 2013; Giebel et al., 2017). Moreover, different manufacturing processes and apparently homogenous EV-releasing MSCs can result in different EV subtypes (Börger et al., 2017).
Currently, the definition of MSC-exosomes is based on the ISCT minimal criteria and the MISEV recommendations (Dominici et al., 2006; Théry et al., 2018). However, both of these sources of guidance are insufficient to distinguish MSC-exosomes from non-MSC-exosomes, and there is no process for functional assessment of MSC-exosomes. Therefore, in this review, the term “MSC-exosome” was used to describe exosome-containing products derived from MSCs. Another challenge during the application of MSC-exosomes in clinical studies is the lack of a standardized manufacturing process to produce the final product. Quality tests for batch size, purity, potency, reproducibility, safety, and storage stability of the MSC-exosome products need to be established. Currently, there is no optimal isolation method to achieve high purity without compromising the integrity, yield, or functionality of the product (Reiner et al., 2017). Finally, storage and transport conditions of MSC-exosome products must also be addressed. Recently, several studies on the storage conditions and biological activity of the native and cargo-loading MSC-exosomes have carried out the ideal storage conditions (Levy et al., 2022). These results may suggest that lyophilization and storage at room temperature can preserve the enhanced bioactivity of cargo-loading exosomes. In this case, additional steps are required to verify the producibility of the MSC-exosomes as a clinical product.
With advances in engineering and biotechnology (Alvarez-Erviti et al., 2011; Haney et al., 2015; You et al., 2021; Xiao et al., 2022; Zhuang et al., 2022), MSC-exosomes produced under given conditions may mitigate some of the shortcomings of natural MSC-exosomes mentioned above. MSC-exosomes, as natural carriers of genetic information and proteins, can potentially be modified as targeted therapeutic delivery systems by genetic editing of parental MSCs (Alvarez-Erviti et al., 2011) or by direct modification of MSC-exosomes (Haney et al., 2015; You et al., 2021; Xiao et al., 2022; Zhuang et al., 2022). Comparing with synthetic nanoparticles delivery system, EVs delivery RNA and protein cargos to cells shown higher efficacy (Murphy et al., 2021). However, the underlying MoA, feasibility, efficiency and producibility of engineering MSC-exosomes products need to be confirmed.
Taken together, the studies reviewed here suggest that MSC-exosomes could provide an alternative option for wound healing. However, more research is required to make modified MSC-exosomes more widely available and suitable for clinical applications in wound healing and skin regeneration.
Author contributions
HZ and ZL contributed equally to this work. Conceptualization, HZ, ZL, and ZZ; validation, HZ and ZZ; investigation, HZ, YZ, KZ, HL, and YW; resources, WW, YH, BP, PB, and ZC; original draft preparation, HZ; review and editing, ZL and ZZ; visualization, HZ, JT, and BW; supervision, ZZ; project administration, ZZ; funding acquisition, ZZ and YL. All authors have read and agreed to the published version of the manuscript. All authors have accepted responsibility for the entire content of this manuscript and approved its submission.
Funding
This work was supported by grants from the National Natural Science Foundation of China (No. 81871574), Sichuan Natural Science Foundation (No. 2022NSFSC0717 and No. 2022NSFSC1579), 135 project for disciplines of excellence, West China Hospital Sichuan University (No. ZYPY20003 and No. ZYPY20004).
Conflict of interest
The authors declare that the research was conducted in the absence of any commercial or financial relationships that could be construed as a potential conflict of interest.
Publisher’s note
All claims expressed in this article are solely those of the authors and do not necessarily represent those of their affiliated organizations, or those of the publisher, the editors and the reviewers. Any product that may be evaluated in this article, or claim that may be made by its manufacturer, is not guaranteed or endorsed by the publisher.
Abbreviations
MSCs, mesenchymal stem cells; MSC-exosomes, MSC-derived exosomes; EVs, extracellular vehicles; MiRNAs, microRNAs; NcRNAs, non-coding RNAs; LAMP2b, lysosome-associated membrane protein 2b; RVG, rabies virus glycoprotein; VSVG, Vesicular stomatitis virus glycoprotein; GPI, glycosylphosphatidylinositol; PDGFRs, platelet-derived growth factor receptors; hUMSCs, human umbilical mesenchymal stem cells; MGE, metabolic glycoengineering; BMSCs, bone marrow mesenchymal stem cells; CircRNAs, circular RNAs; DFU, diabetic foot ulcer; NPs, nanoparticles; PLGA, poly (lactic-co-glycolic acid); ECM, extracellular matrix; GMSC, gingival mesenchymal stem cells; ADMSC, adipose-derived mesenchymal stem cell; SMSC, Synovial mesenchymal stem cells.
References
Agrahari, V., Mandal, A., Agrahari, V., Trinh, H. M., Joseph, M., Ray, A., et al. (2016). A comprehensive insight on ocular pharmacokinetics. Drug Deliv. Transl. Res. 6 (6), 735–754. doi:10.1007/s13346-016-0339-2
Albanese, M., Chen, Y. A., Hüls, C., Gärtner, K., Tagawa, T., Mejias-Perez, E., et al. (2021). MicroRNAs are minor constituents of extracellular vesicles that are rarely delivered to target cells. PLoS Genet. 17 (12), e1009951. doi:10.1371/journal.pgen.1009951
Alvarez-Erviti, L., Seow, Y., Yin, H., Betts, C., Lakhal, S., and Wood, M. J. (2011). Delivery of siRNA to the mouse brain by systemic injection of targeted exosomes. Nat. Biotechnol. 29 (4), 341–345. doi:10.1038/nbt.1807
An, Y., Lin, S., Tan, X., Zhu, S., Nie, F., Zhen, Y., et al. (2021). Exosomes from adipose-derived stem cells and application to skin wound healing. Cell Prolif. 54 (3), e12993. doi:10.1111/cpr.12993
Bai, L., Liu, L., Esquivel, M., Tardy, B. L., Huan, S., Niu, X., et al. (2022). Nanochitin: Chemistry, structure, assembly, and applications. Chem. Rev. 122 (13), 11604–11674. doi:10.1021/acs.chemrev.2c00125
Bari, E., Di Silvestre, D., Mastracci, L., Grillo, F., Grisoli, P., Marrubini, G., et al. (2020). GMP-compliant sponge-like dressing containing MSC lyo-secretome: Proteomic network of healing in a murine wound model. Eur. J. Pharm. Biopharm. 155, 37–48. doi:10.1016/j.ejpb.2020.08.003
Barile, L., and Vassalli, G. (2017). Exosomes: Therapy delivery tools and biomarkers of diseases. Pharmacol. Ther. 174, 63–78. doi:10.1016/j.pharmthera.2017.02.020
Bian, X., Ma, K., Zhang, C., and Fu, X. (2019). Therapeutic angiogenesis using stem cell-derived extracellular vesicles: An emerging approach for treatment of ischemic diseases. Stem Cell Res. Ther. 10 (1), 158. doi:10.1186/s13287-019-1276-z
Börger, V., Bremer, M., Ferrer-Tur, R., Gockeln, L., Stambouli, O., Becic, A., et al. (2017). Mesenchymal stem/stromal cell-derived extracellular vesicles and their potential as novel immunomodulatory therapeutic agents. Int. J. Mol. Sci. 18 (7), 1450. doi:10.3390/ijms18071450
Born, L. J., Chang, K. H., Shoureshi, P., Lay, F., Bengali, S., Hsu, A. T. W., et al. (2022). HOTAIR-loaded mesenchymal stem/stromal cell extracellular vesicles enhance angiogenesis and wound healing. Adv. Healthc. Mater 11 (5), e2002070. doi:10.1002/adhm.202002070
Brennan, M. A., Renaud, A., Guilloton, F., Mebarki, M., Trichet, V., Sensebé, L., et al. (2017). Inferior in vivo osteogenesis and superior angiogenesis of human adipose-derived stem cells compared with bone marrow-derived stem cells cultured in xeno-free conditions. Stem Cells Transl. Med. 6 (12), 2160–2172. doi:10.1002/sctm.17-0133
Busatto, S., Vilanilam, G., Ticer, T., Lin, W. L., Dickson, D. W., Shapiro, S., et al. (2018). Tangential flow filtration for highly efficient concentration of extracellular vesicles from large volumes of fluid. Cells 7 (12), 273. doi:10.3390/cells7120273
Camões, S. P., Bulut, O., Yazar, V., Gaspar, M. M., Simões, S., Ferreira, R., et al. (2022). 3D-MSCs A151 ODN-loaded exosomes are immunomodulatory and reveal a proteomic cargo that sustains wound resolution. J. Adv. Res. 41, 113–128. doi:10.1016/j.jare.2022.01.013
Casado-Díaz, A., Quesada-Gómez, J. M., and Dorado, G. (2020). Extracellular vesicles derived from mesenchymal stem cells (MSC) in regenerative medicine: Applications in skin wound healing. Front. Bioeng. Biotechnol. 8, 146. doi:10.3389/fbioe.2020.00146
Cavanagh, P. R., Lipsky, B. A., Bradbury, A. W., and Botek, G. (2005). Treatment for diabetic foot ulcers. Lancet 366 (9498), 1725–1735. doi:10.1016/s0140-6736(05)67699-4
Chachques, J. C., Gardin, C., Lila, N., Ferroni, L., Migonney, V., Falentin-Daudre, C., et al. (2021). Elastomeric cardiowrap scaffolds functionalized with mesenchymal stem cells-derived exosomes induce a positive modulation in the inflammatory and wound healing response of mesenchymal stem cell and macrophage. Biomedicines 9 (7), 824. doi:10.3390/biomedicines9070824
Chen, D., Hao, H., Fu, X., and Han, W. (2016). Insight into reepithelialization: How do mesenchymal stem cells perform? Stem Cells Int. 2016, 6120173. doi:10.1155/2016/6120173
Chen, G., Huang, A. C., Zhang, W., Zhang, G., Wu, M., Xu, W., et al. (2018). Exosomal PD-L1 contributes to immunosuppression and is associated with anti-PD-1 response. Nature 560 (7718), 382–386. doi:10.1038/s41586-018-0392-8
Chen, H., Luo, C., Yang, M., Li, J., Ma, P., and Zhang, X. (2020). Intracellular uptake of and sensing with SERS-active hybrid exosomes: Insight into a role of metal nanoparticles. Nanomedicine (Lond) 15 (9), 913–926. doi:10.2217/nnm-2019-0419
Chen, T. S., Lai, R. C., Lee, M. M., Choo, A. B., Lee, C. N., and Lim, S. K. (2010). Mesenchymal stem cell secretes microparticles enriched in pre-microRNAs. Nucleic Acids Res. 38 (1), 215–224. doi:10.1093/nar/gkp857
Chevillet, J. R., Kang, Q., Ruf, I. K., Briggs, H. A., Vojtech, L. N., Hughes, S. M., et al. (2014). Quantitative and stoichiometric analysis of the microRNA content of exosomes. Proc. Natl. Acad. Sci. U. S. A. 111 (41), 14888–14893. doi:10.1073/pnas.1408301111
Das, A., Nikhil, A., and Kumar, A. (2022). Preparation of thermo-responsive polymer encapsulated exosomes and its role as a therapeutic agent for blood clot lysis. Colloids Surf. B Biointerfaces 216, 112580. doi:10.1016/j.colsurfb.2022.112580
Das, A., Sinha, M., Datta, S., Abas, M., Chaffee, S., Sen, C. K., et al. (2015). Monocyte and macrophage plasticity in tissue repair and regeneration. Am. J. Pathol. 185 (10), 2596–2606. doi:10.1016/j.ajpath.2015.06.001
Davidson, S. M., Boulanger, C. M., Aikawa, E., Badimon, L., Barile, L., Binder, C. J., et al. (2022). Methods for the identification and characterization of extracellular vesicles in cardiovascular studies - from exosomes to microvesicles. Cardiovasc Res., cvac031. doi:10.1093/cvr/cvac031
Dominici, M., Le Blanc, K., Mueller, I., Slaper-Cortenbach, I., Marini, F., Krause, D., et al. (2006). Minimal criteria for defining multipotent mesenchymal stromal cells. The International Society for Cellular Therapy position statement. Cytotherapy 8 (4), 315–317. doi:10.1080/14653240600855905
Donati, G., and Watt, F. M. (2015). Stem cell heterogeneity and plasticity in epithelia. Cell Stem Cell 16 (5), 465–476. doi:10.1016/j.stem.2015.04.014
Dong, Z., Gu, H., Guo, Q., Liu, X., Li, F., Liu, H., et al. (2022). Circulating small extracellular vesicle-derived miR-342-5p ameliorates beta-amyloid formation via targeting beta-site APP cleaving enzyme 1 in alzheimer's disease. Cells 11 (23), 3830. doi:10.3390/cells11233830
Doyle, L. M., and Wang, M. Z. (2019). Overview of extracellular vesicles, their origin, composition, purpose, and methods for exosome isolation and analysis. Cells 8 (7), 727. doi:10.3390/cells8070727
Duan, L., Xu, L., Xu, X., Qin, Z., Zhou, X., Xiao, Y., et al. (2021). Exosome-mediated delivery of gene vectors for gene therapy. Nanoscale 13 (3), 1387–1397. doi:10.1039/d0nr07622h
El-Tookhy, O. S., Shamaa, A. A., Shehab, G. G., Abdallah, A. N., and Azzam, O. M. (2017). Histological evaluation of experimentally induced critical size defect skin wounds using exosomal solution of mesenchymal stem cells derived microvesicles. Int. J. Stem Cells 10 (2), 144–153. doi:10.15283/ijsc17043
Fan, Q., Yang, L., Zhang, X., Peng, X., Wei, S., Su, D., et al. (2018). The emerging role of exosome-derived non-coding RNAs in cancer biology. Cancer Lett. 414, 107–115. doi:10.1016/j.canlet.2017.10.040
Fang, J., Wei, Y., Teng, X., Zhao, S., and Hua, J. (2018). Immortalization of canine adipose-derived mesenchymal stem cells and their seminiferous tubule transplantation. J. Cell Biochem. 119 (4), 3663–3670. doi:10.1002/jcb.26574
Fang, S., Xu, C., Zhang, Y., Xue, C., Yang, C., Bi, H., et al. (2016). Umbilical cord-derived mesenchymal stem cell-derived exosomal MicroRNAs suppress myofibroblast differentiation by inhibiting the transforming growth factor-β/SMAD2 pathway during wound healing. Stem Cells Transl. Med. 5 (10), 1425–1439. doi:10.5966/sctm.2015-0367
Frykberg, R. G., and Banks, J. (2015). Challenges in the treatment of chronic wounds. Adv. Wound Care (New Rochelle) 4 (9), 560–582. doi:10.1089/wound.2015.0635
Fu, X., Liu, G., Halim, A., Ju, Y., Luo, Q., and Song, A. G. (2019). Mesenchymal stem cell migration and tissue repair. Cells 8 (8), 784. doi:10.3390/cells8080784
Fuhrmann, G., Serio, A., Mazo, M., Nair, R., and Stevens, M. M. (2015). Active loading into extracellular vesicles significantly improves the cellular uptake and photodynamic effect of porphyrins. J. Control Release 205, 35–44. doi:10.1016/j.jconrel.2014.11.029
Geng, X., Qi, Y., Liu, X., Shi, Y., Li, H., and Zhao, L. (2022). A multifunctional antibacterial and self-healing hydrogel laden with bone marrow mesenchymal stem cell-derived exosomes for accelerating diabetic wound healing. Biomater. Adv. 133, 112613. doi:10.1016/j.msec.2021.112613
Giebel, B., Kordelas, L., and Börger, V. (2017). Clinical potential of mesenchymal stem/stromal cell-derived extracellular vesicles. Stem Cell Investig. 4, 84. doi:10.21037/sci.2017.09.06
Gilligan, K. E., and Dwyer, R. M. (2017). Engineering exosomes for cancer therapy. Int. J. Mol. Sci. 18 (6), 1122. doi:10.3390/ijms18061122
Gimona, M., Brizzi, M. F., Choo, A. B. H., Dominici, M., Davidson, S. M., Grillari, J., et al. (2021). Critical considerations for the development of potency tests for therapeutic applications of mesenchymal stromal cell-derived small extracellular vesicles. Cytotherapy 23 (5), 373–380. doi:10.1016/j.jcyt.2021.01.001
Gould, S. J., and Raposo, G. (2013). As we wait: Coping with an imperfect nomenclature for extracellular vesicles. J. Extracell. Vesicles 2, 20389. doi:10.3402/jev.v2i0.20389
Guo, J., Qiu, X., Zhang, L., and Wei, R. (2018). Smurf1 regulates macrophage proliferation, apoptosis and migration via JNK and p38 MAPK signaling pathways. Mol. Immunol. 97, 20–26. doi:10.1016/j.molimm.2018.03.005
Gurung, S., Perocheau, D., Touramanidou, L., and Baruteau, J. (2021). The exosome journey: From biogenesis to uptake and intracellular signalling. Cell Commun. Signal 19 (1), 47. doi:10.1186/s12964-021-00730-1
Gushiken, L. F. S., Beserra, F. P., Bastos, J. K., Jackson, C. J., and Pellizzon, C. H. (2021). Cutaneous wound healing: An update from physiopathology to current therapies. Life (Basel) 11 (7), 665. doi:10.3390/life11070665
Hajipour, H., Farzadi, L., Roshangar, L., Latifi, Z., Kahroba, H., Shahnazi, V., et al. (2021). A human chorionic gonadotropin (hCG) delivery platform using engineered uterine exosomes to improve endometrial receptivity. Life Sci. 275, 119351. doi:10.1016/j.lfs.2021.119351
Haney, M. J., Klyachko, N. L., Zhao, Y., Gupta, R., Plotnikova, E. G., He, Z., et al. (2015). Exosomes as drug delivery vehicles for Parkinson's disease therapy. J. Control Release 207, 18–30. doi:10.1016/j.jconrel.2015.03.033
He, X., Dong, Z., Cao, Y., Wang, H., Liu, S., Liao, L., et al. (2019). MSC-derived exosome promotes M2 polarization and enhances cutaneous wound healing. Stem Cells Int. 2019, 7132708. doi:10.1155/2019/7132708
He, X., Zhang, C., Amirsaadat, S., Jalil, A. T., Kadhim, M. M., Abasi, M., et al. (2022). Curcumin-loaded mesenchymal stem cell-derived exosomes efficiently attenuate proliferation and inflammatory response in rheumatoid arthritis fibroblast-like synoviocytes. Appl. Biochem. Biotechnol. 195, 51–67. doi:10.1007/s12010-022-04090-5
Herrera, M. B., Fonsato, V., Gatti, S., Deregibus, M. C., Sordi, A., Cantarella, D., et al. (2010). Human liver stem cell-derived microvesicles accelerate hepatic regeneration in hepatectomized rats. J. Cell Mol. Med. 14 (6), 1605–1618. doi:10.1111/j.1582-4934.2009.00860.x
Hu, H., Zhai, X., Li, W., Ji, S., Dong, W., Chen, W., et al. (2022). A photo-triggering double cross-linked adhesive, antibacterial, and biocompatible hydrogel for wound healing. iScience 25 (7), 104619. doi:10.1016/j.isci.2022.104619
Hu, J., Chen, Y., Huang, Y., and Su, Y. (2020). Human umbilical cord mesenchymal stem cell-derived exosomes suppress dermal fibroblasts-myofibroblats transition via inhibiting the TGF-β1/Smad 2/3 signaling pathway. Exp. Mol. Pathol. 115, 104468. doi:10.1016/j.yexmp.2020.104468
Hu, Y., Rao, S. S., Wang, Z. X., Cao, J., Tan, Y. J., Luo, J., et al. (2018). Exosomes from human umbilical cord blood accelerate cutaneous wound healing through miR-21-3p-mediated promotion of angiogenesis and fibroblast function. Theranostics 8 (1), 169–184. doi:10.7150/thno.21234
Huang, J., Yu, M., Yin, W., Liang, B., Li, A., Li, J., et al. (2021). Development of a novel RNAi therapy: Engineered miR-31 exosomes promoted the healing of diabetic wounds. Bioact. Mater 6 (9), 2841–2853. doi:10.1016/j.bioactmat.2021.02.007
Jiang, L., Zhang, Y., Liu, T., Wang, X., Wang, H., Song, H., et al. (2020). Exosomes derived from TSG-6 modified mesenchymal stromal cells attenuate scar formation during wound healing. Biochimie 177, 40–49. doi:10.1016/j.biochi.2020.08.003
Jiang, Y., Jahagirdar, B. N., Reinhardt, R. L., Schwartz, R. E., Keene, C. D., Ortiz-Gonzalez, X. R., et al. (2002). Pluripotency of mesenchymal stem cells derived from adult marrow. Nature 418 (6893), 41–49. doi:10.1038/nature00870
Johnstone, R. M., Adam, M., Hammond, J. R., Orr, L., and Turbide, C. (1987). Vesicle formation during reticulocyte maturation. Association of plasma membrane activities with released vesicles (exosomes). J. Biol. Chem. 262 (19), 9412–9420. doi:10.1016/s0021-9258(18)48095-7
Joo, H. S., Suh, J. H., Lee, H. J., Bang, E. S., and Lee, J. M. (2020). Current knowledge and future perspectives on mesenchymal stem cell-derived exosomes as a new therapeutic agent. Int. J. Mol. Sci. 21 (3), 727. doi:10.3390/ijms21030727
Kajimoto, T., Okada, T., Miya, S., Zhang, L., and Nakamura, S. (2013). Ongoing activation of sphingosine 1-phosphate receptors mediates maturation of exosomal multivesicular endosomes. Nat. Commun. 4, 2712. doi:10.1038/ncomms3712
Kaminski, N., Köster, C., Mouloud, Y., Börger, V., Felderhoff-Müser, U., Bendix, I., et al. (2020). Mesenchymal stromal cell-derived extracellular vesicles reduce neuroinflammation, promote neural cell proliferation and improve oligodendrocyte maturation in neonatal hypoxic-ischemic brain injury. Front. Cell Neurosci. 14, 601176. doi:10.3389/fncel.2020.601176
Khongkow, M., Yata, T., Boonrungsiman, S., Ruktanonchai, U. R., Graham, D., and Namdee, K. (2019). Surface modification of gold nanoparticles with neuron-targeted exosome for enhanced blood-brain barrier penetration. Sci. Rep. 9 (1), 8278. doi:10.1038/s41598-019-44569-6
Kim, H. S., Sun, X., Lee, J. H., Kim, H. W., Fu, X., and Leong, K. W. (2019). Advanced drug delivery systems and artificial skin grafts for skin wound healing. Adv. Drug Deliv. Rev. 146, 209–239. doi:10.1016/j.addr.2018.12.014
Kim, S., Lee, S. K., Kim, H., and Kim, T. M. (2018). Exosomes secreted from induced pluripotent stem cell-derived mesenchymal stem cells accelerate skin cell proliferation. Int. J. Mol. Sci. 19 (10), 3119. doi:10.3390/ijms19103119
KimParaiso, H. T., Rebecca, V. W., Ethan, V. A., Ann Chen, Y., Cecilia Munko, A., InnaFedorenko, V., et al. (2011). PTEN loss confers BRAF inhibitor resistance to melanoma cells through the suppression of BIM expression. Cancer Res. 71 (7), 2750–2760. doi:10.1158/0008-5472.CAN-10-2954
Kojima, R., Bojar, D., Rizzi, G., Hamri, G. C., El-Baba, M. D., Saxena, P., et al. (2018). Designer exosomes produced by implanted cells intracerebrally deliver therapeutic cargo for Parkinson's disease treatment. Nat. Commun. 9 (1), 1305. doi:10.1038/s41467-018-03733-8
Kordelas, L., Rebmann, V., Ludwig, A. K., Radtke, S., Ruesing, J., Doeppner, T. R., et al. (2014). MSC-Derived exosomes: A novel tool to treat therapy-refractory graft-versus-host disease. Leukemia 28 (4), 970–973. doi:10.1038/leu.2014.41
Kudinov, V. A., Artyushev, R. I., Zurina, I. M., Lapshin, R. D., Snopova, L. B., Mukhina, I. V., et al. (2021). Antimicrobial and regenerative effects of placental multipotent mesenchymal stromal cell secretome-based chitosan gel on infected burns in rats. Pharm. (Basel) 14 (12), 1263. doi:10.3390/ph14121263
Kurniawati, I., Liu, M. C., Hsieh, C. L., Do, A. D., and Sung, S. Y. (2022). Targeting castration-resistant prostate cancer using mesenchymal stem cell exosomes for therapeutic MicroRNA-let-7c delivery. Front. Biosci. (Landmark Ed. 27 (9), 256. doi:10.31083/j.fbl2709256
Lai, R. C., Arslan, F., Lee, M. M., Sze, N. S., Choo, A., Chen, T. S., et al. (2010). Exosome secreted by MSC reduces myocardial ischemia/reperfusion injury. Stem Cell Res. 4 (3), 214–222. doi:10.1016/j.scr.2009.12.003
Lai, R. C., and Lim, S. K. (2019). Membrane lipids define small extracellular vesicle subtypes secreted by mesenchymal stromal cells. J. Lipid Res. 60 (2), 318–322. doi:10.1194/jlr.R087411
Lee, M., Jeong, S. Y., Ha, J., Kim, M., Jin, H. J., Kwon, S. J., et al. (2014). Low immunogenicity of allogeneic human umbilical cord blood-derived mesenchymal stem cells in vitro and in vivo. Biochem. Biophys. Res. Commun. 446 (4), 983–989. doi:10.1016/j.bbrc.2014.03.051
Lener, T., Gimona, M., Aigner, L., Börger, V., Buzas, E., Camussi, G., et al. (2015). Applying extracellular vesicles based therapeutics in clinical trials - an ISEV position paper. J. Extracell. Vesicles 4, 30087. doi:10.3402/jev.v4.30087
Levy, D., Jeyaram, A., Born, L. J., Chang, K. H., Abadchi, S. N., Hsu, A. T. W., et al. (2022). Impact of storage conditions and duration on function of native and cargo-loaded mesenchymal stromal cell extracellular vesicles. Cytotherapy. doi:10.1016/j.jcyt.2022.11.006
Levy, O., Kuai, R., Siren, E. M. J., Bhere, D., Milton, Y., Nissar, N., et al. (2020). Shattering barriers toward clinically meaningful MSC therapies. Sci. Adv. 6 (30), eaba6884. doi:10.1126/sciadv.aba6884
Li, B., Luan, S., Chen, J., Zhou, Y., Wang, T., Li, Z., et al. (2020). The MSC-derived exosomal lncRNA H19 promotes wound healing in diabetic foot ulcers by upregulating PTEN via MicroRNA-152-3p. Mol. Ther. Nucleic Acids 19, 814–826. doi:10.1016/j.omtn.2019.11.034
Li, D., Zhang, P., Yao, X., Li, H., Shen, H., Li, X., et al. (2018). Exosomes derived from miR-133b-modified mesenchymal stem cells promote recovery after spinal cord injury. Front. Neurosci. 12, 845. doi:10.3389/fnins.2018.00845
Li, M., Ke, Q. F., Tao, S. C., Guo, S. C., Rui, B. Y., and Guo, Y. P. (2016). Fabrication of hydroxyapatite/chitosan composite hydrogels loaded with exosomes derived from miR-126-3p overexpressed synovial mesenchymal stem cells for diabetic chronic wound healing. J. Mater Chem. B 4 (42), 6830–6841. doi:10.1039/c6tb01560c
Li, P., Kaslan, M., Lee, S. H., Yao, J., and Gao, Z. (2017). Progress in exosome isolation techniques. Theranostics 7 (3), 789–804. doi:10.7150/thno.18133
Li, W., Liu, Y., Zhang, P., Tang, Y., Zhou, M., Jiang, W., et al. (2018). Tissue-engineered bone immobilized with human adipose stem cells-derived exosomes promotes bone regeneration. ACS Appl. Mater Interfaces 10 (6), 5240–5254. doi:10.1021/acsami.7b17620
Liang, X., Zhang, L., Wang, S., Han, Q., and Zhao, R. C. (2016). Exosomes secreted by mesenchymal stem cells promote endothelial cell angiogenesis by transferring miR-125a. J. Cell Sci. 129 (11), 2182–2189. doi:10.1242/jcs.170373
Liang, Y., Zhao, X., Hu, T., Chen, B., Yin, Z., Ma, P. X., et al. (2019). Adhesive hemostatic conducting injectable composite hydrogels with sustained drug release and photothermal antibacterial activity to promote full-thickness skin regeneration during wound healing. Small 15 (12), e1900046. doi:10.1002/smll.201900046
Liao, F. L., Tan, L., Liu, H., Wang, J. J., Ma, X. T., Zhao, B., et al. (2018). Hematopoietic stem cell-derived exosomes promote hematopoietic differentiation of mouse embryonic stem cells in vitro via inhibiting the miR126/Notch1 pathway. Acta Pharmacol. Sin. 39 (4), 552–560. doi:10.1038/aps.2017.130
Lin, Y., Wu, J., Gu, W., Huang, Y., Tong, Z., Huang, L., et al. (2018). Exosome-liposome hybrid nanoparticles deliver CRISPR/Cas9 system in MSCs. Adv. Sci. (Weinh) 5 (4), 1700611. doi:10.1002/advs.201700611
Liu, X., Yang, Y., Li, Y., Niu, X., Zhao, B., Wang, Y., et al. (2017). Integration of stem cell-derived exosomes with in situ hydrogel glue as a promising tissue patch for articular cartilage regeneration. Nanoscale 9 (13), 4430–4438. doi:10.1039/c7nr00352h
Liu, Y., Liu, Y., Zhao, Y., Wu, M., Mao, S., Cong, P., et al. (2022). Application of adipose mesenchymal stem cell-derived exosomes-loaded β-chitin nanofiber hydrogel for wound healing. Folia Histochem Cytobiol. 60 (2), 167–178. doi:10.5603/FHC.a2022.0015
Lokhande, G., Carrow, J. K., Thakur, T., Xavier, J. R., Parani, M., Bayless, K. J., et al. (2018). Nanoengineered injectable hydrogels for wound healing application. Acta Biomater. 70, 35–47. doi:10.1016/j.actbio.2018.01.045
Lötvall, J., Hill, A. F., Hochberg, F., Buzás, E. I., Di Vizio, D., Gardiner, C., et al. (2014). Minimal experimental requirements for definition of extracellular vesicles and their functions: A position statement from the international society for extracellular vesicles. J. Extracell. Vesicles 3, 26913. doi:10.3402/jev.v3.26913
Luan, X., Sansanaphongpricha, K., Myers, I., Chen, H., Yuan, H., and Sun, D. (2017). Engineering exosomes as refined biological nanoplatforms for drug delivery. Acta Pharmacol. Sin. 38 (6), 754–763. doi:10.1038/aps.2017.12
Ludwig, A. K., De Miroschedji, K., Doeppner, T. R., Börger, V., Ruesing, J., Rebmann, V., et al. (2018). Precipitation with polyethylene glycol followed by washing and pelleting by ultracentrifugation enriches extracellular vesicles from tissue culture supernatants in small and large scales. J. Extracell. Vesicles 7 (1), 1528109. doi:10.1080/20013078.2018.1528109
Luo, Z., Sun, Y., Qi, B., Lin, J., Chen, Y., Xu, Y., et al. (2022). Human bone marrow mesenchymal stem cell-derived extracellular vesicles inhibit shoulder stiffness via let-7a/Tgfbr1 axis. Bioact. Mater 17, 344–359. doi:10.1016/j.bioactmat.2022.01.016
Luo, Z. W., Li, F. X., Liu, Y. W., Rao, S. S., Yin, H., Huang, J., et al. (2019). Aptamer-functionalized exosomes from bone marrow stromal cells target bone to promote bone regeneration. Nanoscale 11 (43), 20884–20892. doi:10.1039/c9nr02791b
Lv, Q., Deng, J., Chen, Y., Wang, Y., Liu, B., and Liu, J. (2020). Engineered human adipose stem-cell-derived exosomes loaded with miR-21-5p to promote diabetic cutaneous wound healing. Mol. Pharm. 17 (5), 1723–1733. doi:10.1021/acs.molpharmaceut.0c00177
Mathew, B., Ravindran, S., Liu, X., Torres, L., Chennakesavalu, M., Huang, C. C., et al. (2019). Mesenchymal stem cell-derived extracellular vesicles and retinal ischemia-reperfusion. Biomaterials 197, 146–160. doi:10.1016/j.biomaterials.2019.01.016
Mathieu, M., Martin-Jaular, L., Lavieu, G., and Théry, C. (2019). Specificities of secretion and uptake of exosomes and other extracellular vesicles for cell-to-cell communication. Nat. Cell Biol. 21 (1), 9–17. doi:10.1038/s41556-018-0250-9
Mathieu, M., Névo, N., Jouve, M., Valenzuela, J. I., Maurin, M., Verweij, F. J., et al. (2021). Specificities of exosome versus small ectosome secretion revealed by live intracellular tracking of CD63 and CD9. Nat. Commun. 12 (1), 4389. doi:10.1038/s41467-021-24384-2
Meyer, C., Losacco, J., Stickney, Z., Li, L., Marriott, G., and Lu, B. (2017). Pseudotyping exosomes for enhanced protein delivery in mammalian cells. Int. J. Nanomedicine 12, 3153–3170. doi:10.2147/ijn.S133430
Miyasaki, D. M., Senegaglia, A. C., de Moura, S. A. B., Leitolis, A., Capriglione, L. G. A., Fracaro, L., et al. (2022). Treatment of chronic kidney disease with extracellular vesicles from mesenchymal stem cells and CD133(+) expanded cells: A comparative preclinical analysis. Int. J. Mol. Sci. 23 (5), 2521. doi:10.3390/ijms23052521
Möller, A., and Lobb, R. J. (2020). The evolving translational potential of small extracellular vesicles in cancer. Nat. Rev. Cancer 20 (12), 697–709. doi:10.1038/s41568-020-00299-w
Murphy, D. E., de Jong, O. G., Brouwer, M., Wood, M. J., Lavieu, G., Schiffelers, R. M., et al. (2019). Extracellular vesicle-based therapeutics: Natural versus engineered targeting and trafficking. Exp. Mol. Med. 51 (3), 1–12. doi:10.1038/s12276-019-0223-5
Murphy, D. E., de Jong, O. G., Evers, M. J. W., Nurazizah, M., Schiffelers, R. M., and Vader, P. (2021). Natural or synthetic RNA delivery: A stoichiometric comparison of extracellular vesicles and synthetic nanoparticles. Nano Lett. 21 (4), 1888–1895. doi:10.1021/acs.nanolett.1c00094
Nakamura, Y., Kita, S., Tanaka, Y., Fukuda, S., Obata, Y., Okita, T., et al. (2020). Adiponectin stimulates exosome release to enhance mesenchymal stem-cell-driven therapy of heart failure in mice. Mol. Ther. 28 (10), 2203–2219. doi:10.1016/j.ymthe.2020.06.026
Nunan, R., Harding, K. G., and Martin, P. (2014). Clinical challenges of chronic wounds: Searching for an optimal animal model to recapitulate their complexity. Dis. Model Mech. 7 (11), 1205–1213. doi:10.1242/dmm.016782
Nuzzi, R., Buono, L., Scalabrin, S., De Iuliis, M., and Bussolati, B. (2021). Effect of stem cell-derived extracellular vesicles on damaged human corneal endothelial cells. Stem Cells Int. 2021, 6644463. doi:10.1155/2021/6644463
Ohno, S., Takanashi, M., Sudo, K., Ueda, S., Ishikawa, A., Matsuyama, N., et al. (2013). Systemically injected exosomes targeted to EGFR deliver antitumor microRNA to breast cancer cells. Mol. Ther. 21 (1), 185–191. doi:10.1038/mt.2012.180
Park, D. J., Yun, W. S., Kim, W. C., Park, J. E., Lee, S. H., Ha, S., et al. (2020). Improvement of stem cell-derived exosome release efficiency by surface-modified nanoparticles. J. Nanobiotechnology 18 (1), 178. doi:10.1186/s12951-020-00739-7
Pittenger, M. F., Mackay, A. M., Beck, S. C., Jaiswal, R. K., Douglas, R., Mosca, J. D., et al. (1999). Multilineage potential of adult human mesenchymal stem cells. Science 284 (5411), 143–147. doi:10.1126/science.284.5411.143
Pritchard, C. C., Cheng, H. H., and Tewari, M. (2012). MicroRNA profiling: Approaches and considerations. Nat. Rev. Genet. 13 (5), 358–369. doi:10.1038/nrg3198
Qian, Z., Bai, Y., Zhou, J., Li, L., Na, J., Fan, Y., et al. (2020). A moisturizing chitosan-silk fibroin dressing with silver nanoparticles-adsorbed exosomes for repairing infected wounds. J. Mater Chem. B 8 (32), 7197–7212. doi:10.1039/d0tb01100b
Ramasubramanian, L., Kumar, P., and Wang, A. (2019). Engineering extracellular vesicles as nanotherapeutics for regenerative medicine. Biomolecules 10 (1), 48. doi:10.3390/biom10010048
Reiner, A. T., Witwer, K. W., van Balkom, B. W. M., de Beer, J., Brodie, C., Corteling, R. L., et al. (2017). Concise review: Developing best-practice models for the therapeutic use of extracellular vesicles. Stem Cells Transl. Med. 6 (8), 1730–1739. doi:10.1002/sctm.17-0055
Ren, S., Chen, J., Duscher, D., Liu, Y., Guo, G., Kang, Y., et al. (2019). Microvesicles from human adipose stem cells promote wound healing by optimizing cellular functions via AKT and ERK signaling pathways. Stem Cell Res. Ther. 10 (1), 47. doi:10.1186/s13287-019-1152-x
Riau, A. K., Ong, H. S., Yam, G. H. F., and Mehta, J. S. (2019). Sustained delivery system for stem cell-derived exosomes. Front. Pharmacol. 10, 1368. doi:10.3389/fphar.2019.01368
Riazifar, M., Pone, E. J., Lötvall, J., and Zhao, W. (2017). Stem cell extracellular vesicles: Extended messages of regeneration. Annu. Rev. Pharmacol. Toxicol. 57, 125–154. doi:10.1146/annurev-pharmtox-061616-030146
Riha, S. M., Maarof, M., and Fauzi, M. B. (2021). Synergistic effect of biomaterial and stem cell for skin tissue engineering in cutaneous wound healing: A concise review. Polym. (Basel) 13 (10), 1546. doi:10.3390/polym13101546
Rong, Y., Wang, Z., Tang, P., Wang, J., Ji, C., Chang, J., et al. (2023). Engineered extracellular vesicles for delivery of siRNA promoting targeted repair of traumatic spinal cord injury. Bioact. Mater 23, 328–342. doi:10.1016/j.bioactmat.2022.11.011
Safari, B., Aghazadeh, M., Davaran, S., and Roshangar, L. (2022). Exosome-loaded hydrogels: A new cell-free therapeutic approach for skin regeneration. Eur. J. Pharm. Biopharm. 171, 50–59. doi:10.1016/j.ejpb.2021.11.002
Salunkhe, S., Dheeraj, M. B., Chitkara, D., and Mittal, A. (2020). Surface functionalization of exosomes for target-specific delivery and in vivo imaging & tracking: Strategies and significance. J. Control Release 326, 599–614. doi:10.1016/j.jconrel.2020.07.042
Samadikuchaksaraei, A., Mehdipour, A., Habibi Roudkenar, M., Verdi, J., Joghataei, M. T., As'adi, K., et al. (2016). A dermal equivalent engineered with TGF-β3 expressing bone marrow stromal cells and amniotic membrane: Cosmetic healing of full-thickness skin wounds in rats. Artif. Organs 40 (12), E266–e279. doi:10.1111/aor.12807
Sandonà, M., Di Pietro, L., Esposito, F., Ventura, A., Silini, A. R., Parolini, O., et al. (2021). Mesenchymal stromal cells and their secretome: New therapeutic perspectives for skeletal muscle regeneration. Front. Bioeng. Biotechnol. 9, 652970. doi:10.3389/fbioe.2021.652970
Schultz, G. S., Sibbald, R. G., Falanga, V., Ayello, E. A., Dowsett, C., Harding, K., et al. (2003). Wound bed preparation: A systematic approach to wound management. Wound Repair Regen. 11 (1), S1–S28. doi:10.1046/j.1524-475x.11.s2.1.x
Shao, H., Im, H., Castro, C. M., Breakefield, X., Weissleder, R., and Lee, H. (2018). New technologies for analysis of extracellular vesicles. Chem. Rev. 118 (4), 1917–1950. doi:10.1021/acs.chemrev.7b00534
Shao, L., Zhang, Y., Pan, X., Liu, B., Liang, C., Zhang, Y., et al. (2020). Knockout of beta-2 microglobulin enhances cardiac repair by modulating exosome imprinting and inhibiting stem cell-induced immune rejection. Cell Mol. Life Sci. 77 (5), 937–952. doi:10.1007/s00018-019-03220-3
Shi, A., Li, J., Qiu, X., Sabbah, M., Boroumand, S., Huang, T. C., et al. (2021). TGF-β loaded exosome enhances ischemic wound healing in vitro and in vivo. Theranostics 11 (13), 6616–6631. doi:10.7150/thno.57701
Shi, Q., Qian, Z., Liu, D., Sun, J., Wang, X., Liu, H., et al. (2017). GMSC-derived exosomes combined with a chitosan/silk hydrogel sponge accelerates wound healing in a diabetic rat skin defect model. Front. Physiol. 8, 904. doi:10.3389/fphys.2017.00904
Shi, R., Jin, Y., Hu, W., Lian, W., Cao, C., Han, S., et al. (2020). Exosomes derived from mmu_circ_0000250-modified adipose-derived mesenchymal stem cells promote wound healing in diabetic mice by inducing miR-128-3p/SIRT1-mediated autophagy. Am. J. Physiol. Cell Physiol. 318 (5), C848–c856. doi:10.1152/ajpcell.00041.2020
Shi, X., Cheng, Q., Hou, T., Han, M., Smbatyan, G., Lang, J. E., et al. (2020). Genetically engineered cell-derived nanoparticles for targeted breast cancer immunotherapy. Mol. Ther. 28 (2), 536–547. doi:10.1016/j.ymthe.2019.11.020
Singer, A. J., and Clark, R. A. (1999). Cutaneous wound healing. N. Engl. J. Med. 341 (10), 738–746. doi:10.1056/nejm199909023411006
Sisa, C., Kholia, S., Naylor, J., Herrera Sanchez, M. B., Bruno, S., Deregibus, M. C., et al. (2019). Mesenchymal stromal cell derived extracellular vesicles reduce hypoxia-ischaemia induced perinatal brain injury. Front. Physiol. 10, 282. doi:10.3389/fphys.2019.00282
Skog, J., Würdinger, T., van Rijn, S., Meijer, D. H., Gainche, L., Sena-Esteves, M., et al. (2008). Glioblastoma microvesicles transport RNA and proteins that promote tumour growth and provide diagnostic biomarkers. Nat. Cell Biol. 10 (12), 1470–1476. doi:10.1038/ncb1800
Smyth, T., Petrova, K., Payton, N. M., Persaud, I., Redzic, J. S., Graner, M. W., et al. (2014). Surface functionalization of exosomes using click chemistry. Bioconjug Chem. 25 (10), 1777–1784. doi:10.1021/bc500291r
Sorrell, J. M., Baber, M. A., and Caplan, A. I. (2009). Influence of adult mesenchymal stem cells on in vitro vascular formation. Tissue Eng. Part A 15 (7), 1751–1761. doi:10.1089/ten.tea.2008.0254
Su, D., Tsai, H. I., Xu, Z., Yan, F., Wu, Y., Xiao, Y., et al. (2019). Exosomal PD-L1 functions as an immunosuppressant to promote wound healing. J. Extracell. Vesicles 9 (1), 1709262. doi:10.1080/20013078.2019.1709262
Su, Y., Sharma, N. S., John, J. V., Ganguli-Indra, G., Indra, A. K., Gombart, A. F., et al. (2022). Engineered exosomes containing cathelicidin/LL-37 exhibit multiple biological functions. Adv. Healthc. Mater 11, e2200849. doi:10.1002/adhm.202200849
Sun, B., Wu, F., Wang, X., Song, Q., Ye, Z., Mohammadniaei, M., et al. (2022). An optimally designed engineering exosome-reductive COF integrated nanoagent for synergistically enhanced diabetic fester wound healing. Small 18 (26), e2200895. doi:10.1002/smll.202200895
Sung, D. K., Chang, Y. S., Sung, S. I., Ahn, S. Y., and Park, W. S. (2019). Thrombin preconditioning of extracellular vesicles derived from mesenchymal stem cells accelerates cutaneous wound healing by boosting their biogenesis and enriching cargo content. J. Clin. Med. 8 (4), 533. doi:10.3390/jcm8040533
Tabibzadeh, S. (2022). Repair, regeneration and rejuvenation require un-entangling pluripotency from senescence. Ageing Res. Rev. 80, 101663. doi:10.1016/j.arr.2022.101663
Takeo, M., Lee, W., and Ito, M. (2015). Wound healing and skin regeneration. Cold Spring Harb. Perspect. Med. 5 (1), a023267. doi:10.1101/cshperspect.a023267
Tan, T. T., Lai, R. C., Padmanabhan, J., Sim, W. K., Choo, A. B. H., and Lim, S. K. (2021)., 14. Pharmaceuticals (Basel), 345. doi:10.3390/ph14040345Assessment of tumorigenic potential in mesenchymal-stem/stromal-cell-derived small extracellular vesicles (MSC-sEV)Pharmaceuticals4
Tao, S. C., Yuan, T., Zhang, Y. L., Yin, W. J., Guo, S. C., and Zhang, C. Q. (2017). Exosomes derived from miR-140-5p-overexpressing human synovial mesenchymal stem cells enhance cartilage tissue regeneration and prevent osteoarthritis of the knee in a rat model. Theranostics 7 (1), 180–195. doi:10.7150/thno.17133
Theodoraki, M. N., Yerneni, S. S., Hoffmann, T. K., Gooding, W. E., and Whiteside, T. L. (2018). Clinical significance of PD-L1(+) exosomes in plasma of head and neck cancer patients. Clin. Cancer Res. 24 (4), 896–905. doi:10.1158/1078-0432.Ccr-17-2664
Théry, C., Witwer, K. W., Aikawa, E., Alcaraz, M. J., Anderson, J. D., Andriantsitohaina, R., et al. (2018). Minimal information for studies of extracellular vesicles 2018 (MISEV2018): A position statement of the international society for extracellular vesicles and update of the MISEV2014 guidelines. J. Extracell. Vesicles 7 (1), 1535750. doi:10.1080/20013078.2018.1535750
Thomas, M. A., Fahey, M. J., Pugliese, B. R., Irwin, R. M., Antonyak, M. A., and Delco, M. L. (2022). Human mesenchymal stromal cells release functional mitochondria in extracellular vesicles. Front. Bioeng. Biotechnol. 10, 870193. doi:10.3389/fbioe.2022.870193
Tian, T., Cao, L., He, C., Ye, Q., Liang, R., You, W., et al. (2021). Targeted delivery of neural progenitor cell-derived extracellular vesicles for anti-inflammation after cerebral ischemia. Theranostics 11 (13), 6507–6521. doi:10.7150/thno.56367
Togliatto, G., Dentelli, P., Gili, M., Gallo, S., Deregibus, C., Biglieri, E., et al. (2016). Obesity reduces the pro-angiogenic potential of adipose tissue stem cell-derived extracellular vesicles (EVs) by impairing miR-126 content: Impact on clinical applications. Int. J. Obes. (Lond) 40 (1), 102–111. doi:10.1038/ijo.2015.123
Toh, W. S., Lai, R. C., Zhang, B., and Lim, S. K. (2018). MSC exosome works through a protein-based mechanism of action. Biochem. Soc. Trans. 46 (4), 843–853. doi:10.1042/bst20180079
Tonnesen, M. G., Feng, X., and Clark, R. A. (2000). Angiogenesis in wound healing. J. Investig. Dermatol Symp. Proc. 5 (1), 40–46. doi:10.1046/j.1087-0024.2000.00014.x
Trajkovic, K., Hsu, C., Chiantia, S., Rajendran, L., Wenzel, D., Wieland, F., et al. (2008). Ceramide triggers budding of exosome vesicles into multivesicular endosomes. Science 319 (5867), 1244–1247. doi:10.1126/science.1153124
Ullah, M., Liu, D. D., and Thakor, A. S. (2019). Mesenchymal stromal cell homing: Mechanisms and strategies for improvement. iScience 15, 421–438. doi:10.1016/j.isci.2019.05.004
van Balkom, B. W. M., Gremmels, H., Giebel, B., and Lim, S. K. (2019). Proteomic signature of mesenchymal stromal cell-derived small extracellular vesicles. Proteomics 19 (1-2), e1800163. doi:10.1002/pmic.201800163
Van Deun, J., Roux, Q., Deville, S., Van Acker, T., Rappu, P., Miinalainen, I., et al. (2020). Feasibility of mechanical extrusion to coat nanoparticles with extracellular vesicle membranes. Cells 9 (8), 1797. doi:10.3390/cells9081797
van Niel, G., D'Angelo, G., and Raposo, G. (2018). Shedding light on the cell biology of extracellular vesicles. Nat. Rev. Mol. Cell Biol. 19 (4), 213–228. doi:10.1038/nrm.2017.125
Vu, N. B., Nguyen, H. T., Palumbo, R., Pellicano, R., Fagoonee, S., and Pham, P. V. (2021). Stem cell-derived exosomes for wound healing: Current status and promising directions. Minerva Med. 112 (3), 384–400. doi:10.23736/s0026-4806.20.07205-5
Wang, C., Liang, C., Wang, R., Yao, X., Guo, P., Yuan, W., et al. (2019). The fabrication of a highly efficient self-healing hydrogel from natural biopolymers loaded with exosomes for the synergistic promotion of severe wound healing. Biomater. Sci. 8 (1), 313–324. doi:10.1039/c9bm01207a
Wang, C., Wang, M., Xu, T., Zhang, X., Lin, C., Gao, W., et al. (2019). Engineering bioactive self-healing antibacterial exosomes hydrogel for promoting chronic diabetic wound healing and complete skin regeneration. Theranostics 9 (1), 65–76. doi:10.7150/thno.29766
Wang, L., Cai, Y., Zhang, Q., and Zhang, Y. (2022). Pharmaceutical activation of Nrf2 accelerates diabetic wound healing by exosomes from bone marrow mesenchymal stem cells. Int. J. Stem Cells 15 (2), 164–172. doi:10.15283/ijsc21067
Wang, M., Wang, C., Chen, M., Xi, Y., Cheng, W., Mao, C., et al. (2019). Efficient angiogenesis-based diabetic wound healing/skin reconstruction through bioactive antibacterial adhesive ultraviolet shielding nanodressing with exosome release. ACS Nano 13 (9), 10279–10293. doi:10.1021/acsnano.9b03656
Wang, X., Jiao, Y., Pan, Y., Zhang, L., Gong, H., Qi, Y., et al. (2019). Fetal dermal mesenchymal stem cell-derived exosomes accelerate cutaneous wound healing by activating Notch signaling. Stem Cells Int. 2019, 2402916. doi:10.1155/2019/2402916
Webber, J., and Clayton, A. (2013). How pure are your vesicles? J. Extracell. Vesicles 2, 19861. doi:10.3402/jev.v2i0.19861
Wiklander, O. P. B., Brennan, M., Lötvall, J., Breakefield, X. O., and El Andaloussi, S. (2019). Advances in therapeutic applications of extracellular vesicles. Sci. Transl. Med. 11 (492), eaav8521. doi:10.1126/scitranslmed.aav8521
Willis, G. R., Kourembanas, S., and Mitsialis, S. A. (2017). Toward exosome-based therapeutics: Isolation, heterogeneity, and fit-for-purpose potency. Front. Cardiovasc Med. 4, 63. doi:10.3389/fcvm.2017.00063
Witwer, K. W., Buzás, E. I., Bemis, L. T., Bora, A., Lässer, C., Lötvall, J., et al. (2013). Standardization of sample collection, isolation and analysis methods in extracellular vesicle research. J. Extracell. Vesicles 2, 20360. doi:10.3402/jev.v2i0.20360
Witwer, K. W., Van Balkom, B. W. M., Bruno, S., Choo, A., Dominici, M., Gimona, M., et al. (2019). Defining mesenchymal stromal cell (MSC)-derived small extracellular vesicles for therapeutic applications. J. Extracell. Vesicles 8 (1), 1609206. doi:10.1080/20013078.2019.1609206
Wolf, P. (1967). The nature and significance of platelet products in human plasma. Br. J. Haematol. 13 (3), 269–288. doi:10.1111/j.1365-2141.1967.tb08741.x
Wu, D., Chang, X., Tian, J., Kang, L., Wu, Y., Liu, J., et al. (2021). Bone mesenchymal stem cells stimulation by magnetic nanoparticles and a static magnetic field: Release of exosomal miR-1260a improves osteogenesis and angiogenesis. J. Nanobiotechnology 19 (1), 209. doi:10.1186/s12951-021-00958-6
Wu, P., Zhang, B., Ocansey, D. K. W., Xu, W., and Qian, H. (2021). Extracellular vesicles: A bright star of nanomedicine. Biomaterials 269, 120467. doi:10.1016/j.biomaterials.2020.120467
Xiao, T., Qu, H., Zeng, Z., Li, C., and Wan, J. (2022). Exosomes from M2-polarized macrophages relieve oxygen/glucose deprivation/normalization-induced neuronal injury by activating the Nrf2/HO-1 signaling. Arch. Biochem. Biophys. 721, 109193. doi:10.1016/j.abb.2022.109193
Xiong, Q. H., Zhao, L., Wan, G. Q., Hu, Y. G., and Li, X. L. (2022). Engineered BMSCs-derived exosomal miR-542-3p promotes cutaneous wound healing. Endocr. Metab. Immune Disord. Drug Targets 23, 336–346. doi:10.2174/1871530322666220523151713
Xu, P., Xin, Y., Zhang, Z., Zou, X., Xue, K., Zhang, H., et al. (2020). Extracellular vesicles from adipose-derived stem cells ameliorate ultraviolet B-induced skin photoaging by attenuating reactive oxygen species production and inflammation. Stem Cell Res. Ther. 11 (1), 264. doi:10.1186/s13287-020-01777-6
Xuan, W., Khan, M., and Ashraf, M. (2020). Extracellular vesicles from Notch activated cardiac mesenchymal stem cells promote myocyte proliferation and neovasculogenesis. Front. Cell Dev. Biol. 8, 11. doi:10.3389/fcell.2020.00011
Yan, T., Huang, L., Yan, Y., Zhong, Y., Xie, H., and Wang, X. (2022). Bone marrow mesenchymal stem cell-derived exosome miR-29b-3p alleviates UV irradiation-induced photoaging in skin fibroblast. Photodermatol. Photoimmunol. Photomed. doi:10.1111/phpp.12827
Yang, J., Chen, Z., Pan, D., Li, H., and Shen, J. (2020). Umbilical cord-derived mesenchymal stem cell-derived exosomes combined pluronic F127 hydrogel promote chronic diabetic wound healing and complete skin regeneration. Int. J. Nanomedicine 15, 5911–5926. doi:10.2147/ijn.S249129
Yang, Y., Li, C. W., Chan, L. C., Wei, Y., Hsu, J. M., Xia, W., et al. (2018). Exosomal PD-L1 harbors active defense function to suppress T cell killing of breast cancer cells and promote tumor growth. Cell Res. 28 (8), 862–864. doi:10.1038/s41422-018-0060-4
Yao, X., Mao, Y., Wu, D., Zhu, Y., Lu, J., Huang, Y., et al. (2021). Exosomal circ_0030167 derived from BM-MSCs inhibits the invasion, migration, proliferation and stemness of pancreatic cancer cells by sponging miR-338-5p and targeting the Wif1/Wnt8/β-catenin axis. Cancer Lett. 512, 38–50. doi:10.1016/j.canlet.2021.04.030
Yeo, R. W., Lai, R. C., Zhang, B., Tan, S. S., Yin, Y., Teh, B. J., et al. (2013). Mesenchymal stem cell: An efficient mass producer of exosomes for drug delivery. Adv. Drug Deliv. Rev. 65 (3), 336–341. doi:10.1016/j.addr.2012.07.001
Yin, K., Wang, S., and Zhao, R. C. (2019). Exosomes from mesenchymal stem/stromal cells: A new therapeutic paradigm. Biomark. Res. 7, 8. doi:10.1186/s40364-019-0159-x
Yoon, H. Y., Koo, H., Kim, K., and Kwon, I. C. (2017). Molecular imaging based on metabolic glycoengineering and bioorthogonal click chemistry. Biomaterials 132, 28–36. doi:10.1016/j.biomaterials.2017.04.003
You, D. G., Lim, G. T., Kwon, S., Um, W., Oh, B. H., Song, S. H., et al. (2021). Metabolically engineered stem cell-derived exosomes to regulate macrophage heterogeneity in rheumatoid arthritis. Sci. Adv. 7 (23), eabe0083. doi:10.1126/sciadv.abe0083
Yuan, R., Dai, X., Li, Y., Li, C., and Liu, L. (2021). Exosomes from miR-29a-modified adipose-derived mesenchymal stem cells reduce excessive scar formation by inhibiting TGF-β2/Smad3 signaling. Mol. Med. Rep. 24 (5), 758. doi:10.3892/mmr.2021.12398
Yuan, X., Li, D., Chen, X., Han, C., Xu, L., Huang, T., et al. (2017). Extracellular vesicles from human-induced pluripotent stem cell-derived mesenchymal stromal cells (hiPSC-MSCs) protect against renal ischemia/reperfusion injury via delivering specificity protein (SP1) and transcriptional activating of sphingosine kinase 1 and inhibiting necroptosis. Cell Death Dis. 8 (12), 3200. doi:10.1038/s41419-017-0041-4
Zhang, B., Yin, Y., Lai, R. C., Tan, S. S., Choo, A. B., and Lim, S. K. (2014). Mesenchymal stem cells secrete immunologically active exosomes. Stem Cells Dev. 23 (11), 1233–1244. doi:10.1089/scd.2013.0479
Zhang, J., Guan, J., Niu, X., Hu, G., Guo, S., Li, Q., et al. (2015). Exosomes released from human induced pluripotent stem cells-derived MSCs facilitate cutaneous wound healing by promoting collagen synthesis and angiogenesis. J. Transl. Med. 13, 49. doi:10.1186/s12967-015-0417-0
Zhang, W., Bai, X., Zhao, B., Li, Y., Zhang, Y., Li, Z., et al. (2018). Cell-free therapy based on adipose tissue stem cell-derived exosomes promotes wound healing via the PI3K/Akt signaling pathway. Exp. Cell Res. 370 (2), 333–342. doi:10.1016/j.yexcr.2018.06.035
Zhang, X. F., Wang, T., Wang, Z. X., Huang, K. P., Zhang, Y. W., Wang, G. L., et al. (2021). Hypoxic ucMSC-secreted exosomal miR-125b promotes endothelial cell survival and migration during wound healing by targeting TP53INP1. Mol. Ther. Nucleic Acids 26, 347–359. doi:10.1016/j.omtn.2021.07.014
Zhang, Y., Hao, Z., Wang, P., Xia, Y., Wu, J., Xia, D., et al. (2019). Exosomes from human umbilical cord mesenchymal stem cells enhance fracture healing through HIF-1α-mediated promotion of angiogenesis in a rat model of stabilized fracture. Cell Prolif. 52 (2), e12570. doi:10.1111/cpr.12570
Zhang, Y., Liu, Y., Liu, H., and Tang, W. H. (2019). Exosomes: Biogenesis, biologic function and clinical potential. Cell Biosci. 9, 19. doi:10.1186/s13578-019-0282-2
Zhang, Y., Zhang, P., Gao, X., Chang, L., Chen, Z., and Mei, X. (2021). Preparation of exosomes encapsulated nanohydrogel for accelerating wound healing of diabetic rats by promoting angiogenesis. Mater Sci. Eng. C Mater Biol. Appl. 120, 111671. doi:10.1016/j.msec.2020.111671
Zhang, Z., Mi, T., Jin, L., Li, M., Zhanghuang, C., Wang, J., et al. (2022). Comprehensive proteomic analysis of exosome mimetic vesicles and exosomes derived from human umbilical cord mesenchymal stem cells. Stem Cell Res. Ther. 13 (1), 312. doi:10.1186/s13287-022-03008-6
Zhao, B., Zhang, X., Zhang, Y., Lu, Y., Zhang, W., Lu, S., et al. (2021). Human exosomes accelerate cutaneous wound healing by promoting collagen synthesis in a diabetic mouse model. Stem Cells Dev. 30 (18), 922–933. doi:10.1089/scd.2021.0100
Zhao, L., Johnson, T., and Liu, D. (2017). Therapeutic angiogenesis of adipose-derived stem cells for ischemic diseases. Stem Cell Res. Ther. 8 (1), 125. doi:10.1186/s13287-017-0578-2
Zhou, T., Yuan, Z., Weng, J., Pei, D., Du, X., He, C., et al. (2021). Challenges and advances in clinical applications of mesenchymal stromal cells. J. Hematol. Oncol. 14 (1), 24. doi:10.1186/s13045-021-01037-x
Zhou, Y., Zhang, X. L., Lu, S. T., Zhang, N. Y., Zhang, H. J., Zhang, J., et al. (2022). Human adipose-derived mesenchymal stem cells-derived exosomes encapsulated in pluronic F127 hydrogel promote wound healing and regeneration. Stem Cell Res. Ther. 13 (1), 407. doi:10.1186/s13287-022-02980-3
Zhuang, Z., Liu, M., Luo, J., Zhang, X., Dai, Z., Zhang, B., et al. (2022). Exosomes derived from bone marrow mesenchymal stem cells attenuate neurological damage in traumatic brain injury by alleviating glutamate-mediated excitotoxicity. Exp. Neurol. 357, 114182. doi:10.1016/j.expneurol.2022.114182
Keywords: bioengineering, wound healing, skin regeneration, mesenchymal stem cell, exosomes
Citation: Zhao H, Li Z, Wang Y, Zhou K, Li H, Bi S, Wang Y, Wu W, Huang Y, Peng B, Tang J, Pan B, Wang B, Chen Z and Zhang Z (2023) Bioengineered MSC-derived exosomes in skin wound repair and regeneration. Front. Cell Dev. Biol. 11:1029671. doi: 10.3389/fcell.2023.1029671
Received: 27 August 2022; Accepted: 16 February 2023;
Published: 27 February 2023.
Edited by:
Xiaodan Wang, Kunming Medical University, ChinaReviewed by:
Jessica Cao, Kolling Institute of Medical Research, AustraliaWenjing Yu, University of Pennsylvania, United States
Copyright © 2023 Zhao, Li, Wang, Zhou, Li, Bi, Wang, Wu, Huang, Peng, Tang, Pan, Wang, Chen and Zhang. This is an open-access article distributed under the terms of the Creative Commons Attribution License (CC BY). The use, distribution or reproduction in other forums is permitted, provided the original author(s) and the copyright owner(s) are credited and that the original publication in this journal is cited, in accordance with accepted academic practice. No use, distribution or reproduction is permitted which does not comply with these terms.
*Correspondence: Zhenyu Zhang, emhhbmd6eS53Y2hAZm94bWFpbC5jb20=