- 1Department for Biochemistry and Molecular Medicine, Medical School EWL, Bielefeld University, Bielefeld, Germany
- 2Department of Child and Adolescent Health, University Medical Center, Göttingen, Germany
- 3German Center for Cardiovascular Research (DZHK), Partner Site Göttingen, Göttingen, Germany
Calcium is a central signal transduction element in biology. Peroxisomes are essential cellular organelles, yet calcium handling in peroxisomes has been contentious. Recent advances show that peroxisomes are part of calcium homeostasis in cardiac myocytes and therefore may contribute to or even shape their calcium-dependent functionality. However, the mechanisms of calcium movement between peroxisomes and other cellular sites and their mediators remain elusive. Here, we review calcium handling in peroxisomes in concert with other organelles and summarize the most recent knowledge on peroxisomal involvement in calcium dynamics with a focus on mammalian cells.
Introduction
Calcium ions (Ca2+) are among the most important intracellular second messengers with essential roles in various cellular processes such as embryonic development, muscle contraction, neuron excitability, and cell death (Berridge et al., 2000; Giorgi et al., 2018). Ca2+ is the only form of calcium with biological relevance and no mechanisms of its degradation or synthesis are known. Ca2+ is biologically active by two main mechanisms: The movement of charge along electrical currents across membranes, and binding and unbinding of target proteins translocate Ca2+ within cells (Görlach et al., 2015). In this context, calmodulin (CaM) is of particular importance as a Ca2+-binding messenger protein that acts on a wide range of cellular pathways (Cheung, 1980; Kahl and Means, 2003).
Calcium signaling can be initiated by calcium influx through the plasma membrane (PM), and by efflux from the endoplasmic reticulum (ER) (or sarcoplasmic reticulum (SR) in muscle cells), the major intracellular calcium store. ER calcium is released either into the cytosol, or through specialized compartments and membrane contact sites to juxtaposed organelles (Paupe and Prudent, 2018). Calcium release from intracellular stores activates store operated calcium entry (SOCE) from the extracellular space. Cytosolic calcium is either constantly pumped back to the ER through the sarco/endoplasmic reticulum Ca2+-ATPase (SERCA) or exits the cell by the plasma membrane calcium ATPase (PMCA) (Raffaello et al., 2016).
Peroxisomes are membrane-bound organelles originally identified as sites for production and degradation of hydrogen peroxide, and fatty acid metabolism (Wanders and Waterham, 2006; Islinger et al., 2018). Mutations in any of the 15 genes encoding essential peroxisomal biogenesis factors (peroxins) can cause rhizomelic chondrodysplasia punctata (RCDP) or disorders of the Zellweger syndrome spectrum (ZSS), a group of rare multisystem disorders marked by peroxisomal dysfunction and concomitant metabolic abnormalities (Klouwer et al., 2015). Peroxisomes are spherical or tubular with diameters ranging from 100 nm to 1 µM (Soliman et al., 2018; Sograte-Idrissi et al., 2020). The large range is due to species differences and depends on the methods used. The smallest diameters are detected by super-resolution microscopy (Soliman et al., 2018). Peroxisomes contain over 130 proteins participating in a large variety of metabolic pathways (Wanders, 2014). Peroxisomes play a crucial role, e.g., in ether lipid and bile acid biosynthesis, the metabolism of D-amino acids, reactive oxygen species (ROS), and the degradation of purines, polyamines and L-pipecolic acid in mammals (Wanders and Waterham, 2006; Sargsyan and Thoms, 2020). Furthermore, peroxisomes cooperate with mitochondria for the efficient β-oxidation of several fatty acid species and virtually all peroxisomal metabolic pathways require intimate communication of peroxisomes with other organelles (Sargsyan and Thoms, 2020).
In electron micrographs of rodent hearts, peroxisomes are found in immediate vicinity of T-tubules and with junctional SR (Hicks and Fahimi, 1977). T-tubule and SR interaction sites are the main determinants of excitation-contraction coupling and effective Ca2+ handling in cardiomyocytes (CMs) (Flucher et al., 1994). The defined localization of peroxisomes at these sites suggests that Ca2+ may be important for peroxisomes, and that peroxisomes may take up Ca2+ and are part of Ca2+ homeostasis in CMs (Sargsyan et al., 2021).
Calcium presence in peroxisomes
Peroxisomes are highly dynamic organelles capable of fast adaptation to nutritional and environmental changes (Islinger et al., 2012). The multiple interconnections of peroxisomal and extraperoxisomal metabolic pathways imply that peroxisomes may be involved in the regulation of cellular processes and be a part of signaling pathways (Islinger et al., 2012; Sargsyan and Thoms, 2020). Recently, peroxisomal ether lipid metabolism was found to be essential under hypoxic conditions (Jain et al., 2020). At the same time, peroxisomal ROS regulate the activity of mTOR signaling and autophagy (Zhang et al., 2013), suggesting peroxisomes are fine-tuning cellular homeostasis at different levels.
In plants, Ca2+-sensitive targets involved in peroxisomal metabolism have been described. In Arabidopsis and tobacco, ROS-scavenging efficiency increases with Ca2+-mediated activation of peroxisomal catalase 3 (Yang and Poovaiah, 2002; Costa et al., 2010). Furthermore, the Ca2+-dependent protein kinase AtCPK1 is targeted to peroxisomes (Dammann et al., 2003) and peroxisomal Ca2+ and CaM are essential for protein import and functionality of peroxisomal enzymes (Corpas and Barroso, 2018), including nitric oxide (NO) synthase, which, in plants, has an inducible peroxisomal isoform and is associated with pathogen defense (Barroso et al., 1999; Corpas et al., 2004).
Peroxisomal calcium in mammalian cells
The study of purified hamster liver peroxisomes suggested that peroxisomes store Ca2+ and carry a vanadate-sensitive Ca2+-ATPase on the peroxisomal membrane (Raychaudhury et al., 2006). Drago et al. (2008) and Lasorsa et al. (2008) were the first to measure peroxisomal Ca2+ in intact mammalian cells. These studies gave conflicting results about the levels of Ca2+ in peroxisomes and the kinetics of peroxisomal Ca2+ dynamics. Lasorsa et al. (2008) did not find Ca2+-ATPase activity in resting peroxisomes. Using an aequorin sensor, they concluded that peroxisomal Ca2+ concentration in steady state is around 20-fold higher than in the cytosol, rise up to 50–100 µM depending on the cell type and reach 70 µM in HeLa cells. On the other side, Drago et al. (2008) showed that peroxisomal Ca2+ levels are similar to cytosolic Ca2+ and rise slowly when the concentration of the latter rises. Differences in measurement techniques and biophysical properties of the sensors can partially explain these contradicting results (Costa et al., 2013).
In our recent study, three genetically encoded ratiometric Ca2+ indicators covering a broad Ca2+ sensitivity range—Kd 0.6, 1.7, and 60 µM—were used to reassess the results of the aforementioned papers (Sargsyan et al., 2021). D1cpV-px with the highest Kd had the lowest dynamic range and only minimal calcium-dependent increase in fluorescence resonance energy transfer (FRET) could be detected upon maximal stimulation. The response of D3cpV-px (FRET sensor) and pericam-px (ratiometric sensor) were comparable and were not saturated, suggesting that peroxisomal Ca2+ levels are in the optimal detection range of these sensors (Sargsyan et al., 2021). Pericam as a classical EYFP-based sensor may be pH-sensitive in an acidic environment (Nagai et al., 2001). We did not detect signal changes of YFP variants that could be attributed to Ca2+-independent changes of the sensor, suggesting measurements with the more pH-sensitive pericam-px are also reliable.
Parts of the results of our work are based on measurements with the same sensor (D3cpV-KVK-SKL) as Drago et al. (2008), the only difference is a stronger PTS1 (peroxisomal targeting signal 1) signal in D3cpV-px. In this manner we could overcome the problem of unspecific targeting of the sensor described by Drago et al. (2008), which these authors solved by adding a linker before the PTS1 tripeptide.
In HeLa cells, we found basal peroxisomal Ca2+ levels with a mean value of 600 nM and increase upon stimulation up to 2.4 µM (Sargsyan et al., 2021). Of note, 7% of the analyzed cells had basal peroxisomal Ca2+ higher than 1 μM, which would be over 10-fold higher than the expected cytosolic level and would partially correspond to the findings of Lasorsa et al. (2008). Upon stimulation, again some rare cells showed an extremely high increase of peroxisomal Ca2+ up to 6.5 µM and higher. The absence of correlation between the high maximal response and basal Ca2+ values speaks against the hypothesis that peroxisomes have a strictly limited Ca2+ uptake capacity and may rather imply that Ca2+ increase in peroxisomes highly depends on the cell state and current cellular needs. Our findings are integrated in an updated overview of organellar calcium concentrations (Figure 1).
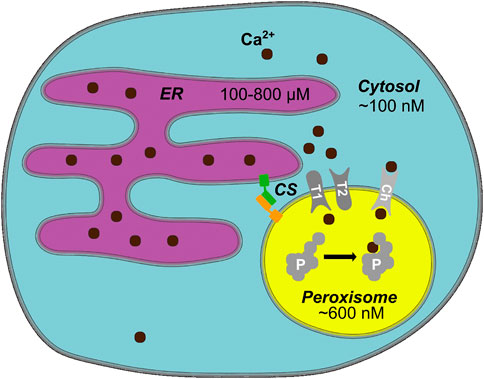
FIGURE 1. Peroxisomal Calcium—an Overview. In mammalian cells, cytosolic Ca2+ levels are around 100 nM, whereas the endoplasmic reticulum (ER) as a main cellular Ca2+ store has several hundred micromolar Ca2+ (Samtleben et al., 2013). An average peroxisome contains around 600 nM Ca2+ (Sargsyan et al., 2021). Ca2+ likely enters peroxisomes either through a channel/pore or a transporter (Ch). The entry and exit mechanism of Ca2+, however, may differ (T1/T2 hypothetical importer/exporter). Known protein tethers form contact sites (CS) between peroxisomes and the ER, potentially create microdomains that facilitate the exchange of Ca2+. Ca2+ may bind to a yet unknown intraperoxisomal protein (P) or membrane protein and affect its function, e.g., by inducing a conformational change when bound to Ca2+. Hypothetical elements of the model (channel, transporter, intraperoxisomal Ca2+-sensitive protein) are shown in gray.
Peroxisomal calcium in cardiomyocytes
The role of Ca2+ is even broader for CMs than in other tissues. Here, Ca2+ interconnects the electrical stimulation of cardiac myocytes and their contraction—a process termed excitation-contraction coupling (Bers, 2008). The specific crucial molecular players of Ca2+ handling in CMs are ryanodine receptors (RyR) on the SR, voltage-operated Ca2+ channels in the T-tubules and Na+/Ca2+-exchanger on the plasma membrane. Strict control of cellular Ca2+ levels is of particular importance in cardiomyocytes. Ca2+ overload in CMs results in malfunction of the heart by affecting both the electrical and contractile properties of cardiomyocytes (Vassalle and Lin, 2004). Electrical abnormalities of CMs present as arrhythmias with varying severity from relatively harmless to life-threatening (Vassalle and Lin, 2004; Landstrom et al., 2017).
The localization of peroxisomes in proximity to the T-tubular system and SR in cardiac myocytes (Hicks and Fahimi, 1977) hinted at a role for peroxisomes in Ca2+ handling. Intracellular store-depletion by the activation of RyRs on the SR through Ca2+ from the T-tubule localized L-type Ca2+ channel (LTCC, Ca2+-induced Ca2+-release) is the main source of Ca2+ increase in CMs in the excitation-contraction coupling (Bers and Perez-Reyes, 1999). Using chemical stimulation, we have shown that Ca2+ enters peroxisomes upon intracellular Ca2+-store depletion in neonatal rat CMs (NRCMs) and human induced pluripotent stem cell-derived CMs (hiPSC-CMs) (Sargsyan et al., 2021). We hypothesized that cardiac peroxisomes take up Ca2+ on a beat-to-beat basis. Indeed, we showed that upon electrical field stimulation with 1 Hz frequency, peroxisomes in NRCM take up Ca2+ in beat-to-beat manner (Sargsyan et al., 2021).
Peroxisomes in hiPSC-CMs occasionally localize in vicinity of ER protein RyR2 and rarely to T-tubular system and LTCC (Sargsyan et al., 2021). The striation pattern is not well-developed in hiPSC-CMs and therefore the relative localization of RyR2, LTCC, and peroxisomes in hiPSC-CMs may differ from that in CMs in the beating heart. It is known from monkey kidney fibroblast-like COS-7 cell line that over 90% of peroxisomes are in contact with the ER (Valm et al., 2017). ER-peroxisome contact is dependent on ACBD4/5-VAPB, and on Miro1v4-VPS13D-VAP (Costello et al., 2017a, 2017b; Hua et al., 2017; Guillén-Samander et al., 2021). However, it is not known whether membrane contact sites are relevant for Ca2+ entry to peroxisomes, if there are tissue or cell type-specific molecular composition and/or abundance of contact sites, and which of these are relevant for CMs.
As peroxisomes take up Ca2+ from the SR and localize in the proximity of RyR receptors in CMs, it is plausible that peroxisomes may contribute to or even be essential for normal excitation-contraction coupling. This hypothesis is supported by the fact that patients with mild forms of ZSS occasionally present with cardiac arrhythmias that may become the cause of lethal outcome (Wanders and Komen, 2007). The metabolic role of peroxisomes could be the reason for these arrhythmias (Colasante et al., 2015). However, a direct contribution to efficient Ca2+ handling by the peroxisomes is another plausible reason.
Candidates of peroxisomal calcium channels
Although peroxisomal Ca2+ changes largely follow cytosolic Ca2+, our experiments with maximal Ca2+ mobilization through ionophore addition showed a slower Ca2+ increase and even slower decline in peroxisomes compared to the cytosol (Sargsyan et al., 2021). The slow but constant increase in peroxisomal Ca2+ when the cytosolic Ca2+ rises suggests that the transfer mechanism may have limited capacity and can be saturated. Potential peroxisomal Ca2+ channels and pores are PEX11 (Mindthoff et al., 2016), PXMP2 (Rokka et al., 2009), PMP34 (Wylin et al., 1998), or any other peroxisomal membrane protein with a transport function (Chornyi et al., 2020). PEX11 and PXMP2 are reported to be unspecific peroxisomal channel-forming proteins with permeability to small solutes in in vitro experiments on artificial membranes (Rokka et al., 2009; Mindthoff et al., 2016). PMP34 has been suggested as a coenzyme A, FAD, and NAD+ transporter across peroxisomal membranes using liposomes with reconstituted recombinant protein (Agrimi et al., 2012). An in vivo study investigating the channel function of PXMP2 and PEX11 examined their role in hydrogen peroxide transport across peroxisomal membrane (Lismont et al., 2019). Judging from the molecular weight of the hydrogen peroxide it could freely pass through both PXMP2 and PEX11. Nonetheless, a fluorescent biosensor for H2O2 in PXMP2- and/or PEX11-deficient cells, showed that neither PXMP2 nor PEX11 are essential for H2O2 trafficking across the peroxisomal membrane (Lismont et al., 2019). Altogether, this suggests that the search for peroxisomal Ca2+ transport machinery may present a challenging task.
Biological relevance of peroxisomal calcium
Highly localized calcium dynamics play a central role in controlling cellular processes. At the same time, excessive increase of intracellular Ca2+ to levels that cannot be handled by the cell is known as Ca2+ overload and can have detrimental consequences (Vassalle and Lin, 2004). For example, mitochondrial calcium stimulates energy gain from Krebs cycle and respiratory chain but can also induce cell death in case of mitochondrial Ca2+ overload (Görlach et al., 2015). Thus, the subcellular sequestration of Ca2+ in different compartments is vital for the regulation of physiological processes.
The interplay of redox and Ca2+ signaling is well described for mitochondria, where Ca2+-dependent opening of calcium channels is regulated by interaction with the oxidoreductase Mia40 (Petrungaro et al., 2015). Additionally, metabolic processes also regulate Ca2+ uptake by mitochondria (Nemani et al., 2018). Whether redox or metabolic processes influence peroxisomal Ca2+ is not known. There is also no consensus about the drivers of Ca2+ transport to peroxisomes and the role of known ion transporters like Ca2+/H+ antiporter, Ca2+/Na+ exchanger, and V-ATPase for peroxisomal Ca2+ levels (Drago et al., 2008; Lasorsa et al., 2008).
Presently, in contrast to plant catalases and kinases (Yang and Poovaiah, 2002; Dammann et al., 2003), no mammalian peroxisomal enzymes are known to bind Ca2+. Weber et al. (1997) suggested the presence of a Ca2+-dependent mitochondrial solute carrier Efinal (gene SLC25A24) also on peroxisomes based on immunoelectron microscopy in rabbit small intestinal tissue. Along with peroxisomal malate dehydrogenase and lactate dehydrogenase (Schueren et al., 2014; Hofhuis et al., 2016; Schueren and Thoms, 2016), they would serve as crucial components of malate and lactate shuttles across peroxisomal membranes for reduction equivalent reoxidation (McClelland et al., 2003). However, in human cell lines, Efinal homologues—identified as members of the short calcium-binding mitochondrial carriers (SCaMC) protein subfamily—were found exclusively in mitochondria (del Arco and Satrústegui, 2004).
Direct evidence for a functional role of intra-peroxisomal Ca2+ is still missing. For the mammalian peroxisome, however, Ca2+ appears to be important. Particularly, Ca2+ channel blockers nifedipine, diltiazem and nicardipine suppress peroxisomal fatty acid oxidation enzymes and peroxisome proliferation (Watanabe and Suga, 1988; Itoga et al., 1990; Zhang et al., 1996). These findings might be due to direct regulation of a peroxisomal enzyme through Ca2+ or by indirect regulation of peroxisomal functions by extraperoxisomal Ca2+.
In case of the latter, peroxisomal membrane protein Miro1v4 could be a potential target. Miro1v4 is a peroxisomal variant of mitochondrial the Miro1 protein that form ER contact sites through VPS13D to exchange lipids and has been shown to mediate the linkage of mitochondria to motor proteins in a calcium-dependent manner (MacAskill et al., 2009). Miro1v4 has two potentially Ca2+ binding EF-hands which are essential for Miro-VPS13D interaction on peroxisome-ER contact sites (Guillén-Samander et al., 2021). Whether Ca2+ binding really regulates peroxisome-ER contact was not studied experimentally. Still, no Ca2+-dependent interaction of the mitochondrial-ER contact site mediated by Miro-VPS13D could be found in experiments (Guillén-Samander et al., 2021). Other lipid transfer mechanisms, like the vesicle-based lipid exchange mediated by synaptotagmin, have been shown to be Ca2+-dependent (Yu et al., 2016). Similarly to the findings for mitochondria, peroxisomal Miro isoforms have been implicated in peroxisome motility (Castro and Schrader, 2018) although there is no evidence on involvement of Ca2+ in peroxisome motility in this context.
The role of peroxisomal Ca2+ might not necessarily be the regulation of peroxisomal processes. Drago et al. (2008) have suggested that peroxisomes may serve as an additional cytosolic Ca2+ buffer compartment. This idea is supported by the fact that peroxisomal Ca2+ rises after ER-store depletion in case of knockdown of mitochondrial calcium uniporter (MCU) beyond its initial maximum (Sargsyan et al., 2021). This suggests that at least in some situations of cellular Ca2+ overload peroxisomes may take up more Ca2+ than under near-physiological standard conditions, therefore buffering potentially deleterious effects of excess Ca2+ on the cell. Consequently, the protective effect of peroxisomal Ca2+ uptake may be necessary only in some special situations such as Ca2+ overload.
As Ca2+ concentration in the peroxisome is higher than in the cytosol, peroxisomes may also in extreme situations play a role of additional Ca2+ store for the cytosol. The buffering function of peroxisomes may be protective in some cases of arrhythmia, such as catecholaminergic polymorphic ventricular tachycardia, when increased predisposition to inadequate SR Ca2+ release events (so called Ca2+ sparks) occur. On the other hand, the absence of peroxisomes may contribute to cardiac pathogenesis and promote the development or extent of arrhythmias.
Conclusion
Ca2+ enters peroxisomes of non-excitable and excitable mammalian cells upon near-physiological electrical and chemical stimulation. Peroxisomal Ca2+ handling presents an exciting research area with many open questions: For example, the existence of Ca2+-dependent transporters in peroxisomes as suggested (Weber et al., 1997) is still unclear. Similarly, Ca2+-sensitive targets in the mammalian peroxisome, as known for the plant peroxisome, may yet be identified. Furthermore, it is conceivable that peroxisomal Ca2+ homeostasis may be important in the absence of luminal Ca2+ binding proteins, by buffering local calcium. In excitable cells, peroxisomal Ca2+ dynamics might be of special importance, also with regard to their pathophysiology. The cellular function of peroxisomal Ca2+ and the role of peroxisomal Ca2+ in pathology remain to be studied further.
Author contributions
YS and ST conceived the article, YS wrote the first draft, JK and ST contributed to the writing, YS drafted the figure, JK and ST edited the figure. All authors edited the article.
Funding
This work was supported by the following grants: Deutsche Forschungsgemeinschaft TH 1538/3-1 to ST, Collaborate Research Council “Modulatory units in heart failure” SFB 1002/3 TP A10 to ST, “Shared expertise” funding by the DZHK (German Centre for Cardiovascular Research), and a PhD stipend by the DAAD program 57381412 ID 91572398 to YS. We acknowledge the financial support of the German Research Foundation (DFG) and the Open Access Publication Fund of Bielefeld University for the article processing charge.
Acknowledgments
We thank Leonie Burghardt, Anna-Lena Toschke, and Dennis Lebeda for comments. We also thank the reviewers for their constructive comments on the manuscript.
Conflict of interest
The authors declare that the research was conducted in the absence of any commercial or financial relationships that could be construed as a potential conflict of interest.
Publisher’s note
All claims expressed in this article are solely those of the authors and do not necessarily represent those of their affiliated organizations, or those of the publisher, the editors and the reviewers. Any product that may be evaluated in this article, or claim that may be made by its manufacturer, is not guaranteed or endorsed by the publisher.
References
Agrimi, G., Russo, A., Scarcia, P., and Palmieri, F. (2012). The human gene SLC25A17 encodes a peroxisomal transporter of coenzyme A, FAD and NAD+. Biochem. J. 443, 241–247. doi:10.1042/BJ20111420
Barroso, J. B., Corpas, F. J., Carreras, A., Sandalio, L. M., Valderrama, R., Palma, JoséM., et al. (1999). Localization of nitric-oxide synthase in plant peroxisomes. J. Biol. Chem. 274, 36729–36733. doi:10.1074/jbc.274.51.36729
Berridge, M. J., Lipp, P., and Bootman, M. D. (2000). The versatility and universality of calcium signalling. Nat. Rev. Mol. Cell Biol. 1, 11–21. doi:10.1038/35036035
Bers, D. M. (2008). Calcium cycling and signaling in cardiac myocytes. Annu. Rev. Physiol. 70, 23–49. doi:10.1146/annurev.physiol.70.113006.100455
Bers, D. M., and Perez-Reyes, E. (1999). Ca channels in cardiac myocytes: Structure and function in Ca influx and intracellular Ca release. Cardiovasc. Res. 42, 339–360. doi:10.1016/s0008-6363(99)00038-3
Castro, I. G., and Schrader, M. (2018). Miro1 – The missing link to peroxisome motility. Commun. Integr. Biol. 11, e1526573. doi:10.1080/19420889.2018.1526573
Cheung, W. Y. (1980). Calmodulin plays a pivotal role in cellular regulation. Science 207, 19–27. doi:10.1126/science.6243188
Chornyi, S., IJlst, L., van Roermund, C. W. T., Wanders, R. J. A., and Waterham, H. R. (2020). Peroxisomal metabolite and cofactor transport in humans. Front. Cell Dev. Biol. 8, 613892. doi:10.3389/fcell.2020.613892
Colasante, C., Chen, J., Ahlemeyer, B., and Baumgart-Vogt, E. (2015). Peroxisomes in cardiomyocytes and the peroxisome/peroxisome proliferator-activated receptor-loop. Thromb. Haemost. 113, 452–463. doi:10.1160/TH14-06-0497
Corpas, F. J., and Barroso, J. B. (2018). Calmodulin antagonist affects peroxisomal functionality by disrupting both peroxisomal Ca2+ and protein import. J. Cell Sci. 131, jcs201467. doi:10.1242/jcs.201467
Corpas, F. J., Barroso, J. B., Carreras, A., Quirós, M., León, A. M., Romero-Puertas, M. C., et al. (2004). Cellular and subcellular localization of endogenous nitric oxide in young and senescent pea plants. Plant Physiol. 136, 2722–2733. doi:10.1104/pp.104.042812
Costa, A., Drago, I., Behera, S., Zottini, M., Pizzo, P., Schroeder, J. I., et al. (2010). H2O2 in plant peroxisomes: An in vivo analysis uncovers a Ca2+-dependent scavenging system. Plant J. 62, 760–772. doi:10.1111/j.1365-313X.2010.04190.x
Costa, A., Drago, I., Zottini, M., Pizzo, P., and Pozzan, T. (2013). Peroxisome Ca(2+) homeostasis in animal and plant cells. Subcell. Biochem. 69, 111–133. doi:10.1007/978-94-007-6889-5_7
Costello, J. L., Castro, I. G., Hacker, C., Schrader, T. A., Metz, J., Zeuschner, D., et al. (2017a). ACBD5 and VAPB mediate membrane associations between peroxisomes and the ER. J. Cell Biol. 216, 331–342. doi:10.1083/jcb.201607055
Costello, J. L., Castro, I. G., Schrader, T. A., Islinger, M., and Schrader, M. (2017b). Peroxisomal ACBD4 interacts with VAPB and promotes ER-peroxisome associations. Cell cycle 16, 1039–1045. doi:10.1080/15384101.2017.1314422
Dammann, C., Ichida, A., Hong, B., Romanowsky, S. M., Hrabak, E. M., Harmon, A. C., et al. (2003). Subcellular targeting of nine calcium-dependent protein kinase isoforms from Arabidopsis. Plant Physiol. 132, 1840–1848. doi:10.1104/pp.103.020008
del Arco, A., and Satrústegui, J. (2004). Identification of a novel human subfamily of mitochondrial carriers with calcium-binding domains. J. Biol. Chem. 279, 24701–24713. doi:10.1074/jbc.M401417200
Drago, I., Giacomello, M., Pizzo, P., and Pozzan, T. (2008). Calcium dynamics in the peroxisomal lumen of living cells. J. Biol. Chem. 283, 14384–14390. doi:10.1074/jbc.M800600200
Flucher, B. E., Andrews, S. B., and Daniels, M. P. (1994). Molecular organization of transverse tubule/sarcoplasmic reticulum junctions during development of excitation-contraction coupling in skeletal muscle. Mol. Biol. Cell 5, 1105–1118. doi:10.1091/mbc.5.10.1105
Giorgi, C., Danese, A., Missiroli, S., Patergnani, S., and Pinton, P. (2018). Calcium dynamics as a machine for decoding signals. Trends Cell Biol. 28, 258–273. doi:10.1016/j.tcb.2018.01.002
Görlach, A., Bertram, K., Hudecova, S., and Krizanova, O. (2015). Calcium and ROS: A mutual interplay. Redox Biol. 6, 260–271. doi:10.1016/j.redox.2015.08.010
Guillén-Samander, A., Leonzino, M., Hanna, M. G., Tang, N., Shen, H., and De Camilli, P. (2021). VPS13D bridges the ER to mitochondria and peroxisomes via Miro. J. Cell Biol. 220, e202010004. doi:10.1083/jcb.202010004
Hicks, L., and Fahimi, H. D. (1977). Peroxisomes (microbodies) in the myocardium of rodents and primates. A comparative Ultrastructural cytochemical study. Cell Tissue Res. 175, 467–481. doi:10.1007/BF00222413
Hofhuis, J., Schueren, F., Nötzel, C., Lingner, T., Gärtner, J., Jahn, O., et al. (2016). The functional readthrough extension of malate dehydrogenase reveals a modification of the genetic code. Open Biol. 6, 160246. doi:10.1098/rsob.160246
Hua, R., Cheng, D., Coyaud, É., Freeman, S., Di Pietro, E., Wang, Y., et al. (2017). VAPs and ACBD5 tether peroxisomes to the ER for peroxisome maintenance and lipid homeostasis. J. Cell Biol. 216, 367–377. doi:10.1083/jcb.201608128
Islinger, M., Grille, S., Fahimi, H. D., and Schrader, M. (2012). The peroxisome: An update on mysteries. Histochem. Cell Biol. 137, 547–574. doi:10.1007/s00418-012-0941-4
Islinger, M., Voelkl, A., Fahimi, H. D., and Schrader, M. (2018). The peroxisome: An update on mysteries 2.0. Histochem. Cell Biol. 150, 443–471. doi:10.1007/s00418-018-1722-5
Itoga, H., Tamura, H., Watanabe, T., and Suga, T. (1990). Characteristics of the suppressive effect of nicardipine on peroxisome induction in rat liver. Biochim. Biophys. Acta 1051, 21–28. doi:10.1016/0167-4889(90)90169-e
Jain, I. H., Calvo, S. E., Markhard, A. L., Skinner, O. S., To, T.-L., Ast, T., et al. (2020). Genetic screen for cell fitness in high or low oxygen highlights mitochondrial and lipid metabolism. Cell 181, 716–727. e11. doi:10.1016/j.cell.2020.03.029
Kahl, C. R., and Means, A. R. (2003). Regulation of cell cycle progression by calcium/calmodulin-dependent pathways. Endocr. Rev. 24, 719–736. doi:10.1210/er.2003-0008
Klouwer, F. C. C., Berendse, K., Ferdinandusse, S., Wanders, R. J. A., Engelen, M., and Poll-The, B. T. (2015). Zellweger spectrum disorders: Clinical overview and management approach. Orphanet J. Rare Dis. 10, 151. doi:10.1186/s13023-015-0368-9
Landstrom, A. P., Dobrev, D., and Wehrens, X. H. T. (2017). Calcium signaling and cardiac arrhythmias. Circ. Res. 120, 1969–1993. doi:10.1161/CIRCRESAHA.117.310083
Lasorsa, F. M., Pinton, P., Palmieri, L., Scarcia, P., Rottensteiner, H., Rizzuto, R., et al. (2008). Peroxisomes as novel players in cell calcium homeostasis. J. Biol. Chem. 283, 15300–15308. doi:10.1074/jbc.M800648200
Lismont, C., Koster, J., Provost, S., Baes, M., Van Veldhoven, P. P., Waterham, H. R., et al. (2019). Deciphering the potential involvement of PXMP2 and PEX11B in hydrogen peroxide permeation across the peroxisomal membrane reveals a role for PEX11B in protein sorting. Biochim. Biophys. Acta. Biomembr. 1861, 182991. doi:10.1016/j.bbamem.2019.05.013
MacAskill, A. F., Rinholm, J. E., Twelvetrees, A. E., Arancibia-Carcamo, I. L., Muir, J., Fransson, A., et al. (2009). Miro1 is a calcium sensor for glutamate receptor-dependent localization of mitochondria at synapses. Neuron 61, 541–555. doi:10.1016/j.neuron.2009.01.030
McClelland, G. B., Khanna, S., González, G. F., Butz, C. E., and Brooks, G. A. (2003). Peroxisomal membrane monocarboxylate transporters: Evidence for a redox shuttle system? Biochem. Biophys. Res. Commun. 304, 130–135. doi:10.1016/s0006-291x(03)00550-3
Mindthoff, S., Grunau, S., Steinfort, L. L., Girzalsky, W., Hiltunen, J. K., Erdmann, R., et al. (2016). Peroxisomal Pex11 is a pore-forming protein homologous to TRPM channels. Biochim. Biophys. Acta 1863, 271–283. doi:10.1016/j.bbamcr.2015.11.013
Nagai, T., Sawano, A., Park, E. S., and Miyawaki, A. (2001). Circularly permuted green fluorescent proteins engineered to sense Ca2+. Proc. Natl. Acad. Sci. U. S. A. 98, 3197–3202. doi:10.1073/pnas.051636098
Nemani, N., Shanmughapriya, S., and Madesh, M. (2018). Molecular regulation of MCU: Implications in physiology and disease. Cell Calcium 74, 86–93. doi:10.1016/j.ceca.2018.06.006
Paupe, V., and Prudent, J. (2018). New insights into the role of mitochondrial calcium homeostasis in cell migration. Biochem. Biophys. Res. Commun. 500, 75–86. doi:10.1016/j.bbrc.2017.05.039
Petrungaro, C., Zimmermann, K. M., Küttner, V., Fischer, M., Dengjel, J., Bogeski, I., et al. (2015). The Ca(2+)-dependent release of the mia40-induced MICU1-MICU2 dimer from MCU regulates mitochondrial Ca(2+) uptake. Cell Metab. 22, 721–733. doi:10.1016/j.cmet.2015.08.019
Raffaello, A., Mammucari, C., Gherardi, G., and Rizzuto, R. (2016). Calcium at the center of cell signaling: Interplay between endoplasmic reticulum, mitochondria, and lysosomes. Trends biochem. Sci. 41, 1035–1049. doi:10.1016/j.tibs.2016.09.001
Raychaudhury, B., Gupta, S., Banerjee, S., and Datta, S. C. (2006). Peroxisome is a reservoir of intracellular calcium. Biochim. Biophys. Acta 1760, 989–992. doi:10.1016/j.bbagen.2006.02.022
Rokka, A., Antonenkov, V. D., Soininen, R., Immonen, H. L., Pirilä, P. L., Bergmann, U., et al. (2009). Pxmp2 is a channel-forming protein in Mammalian peroxisomal membrane. PloS one 4, e5090. doi:10.1371/journal.pone.0005090
Samtleben, S., Jaepel, J., Fecher, C., Andreska, T., Rehberg, M., and Blum, R. (2013). Direct imaging of ER calcium with targeted-esterase induced dye loading (TED). J. Vis. Exp. (75), e50317. doi:10.3791/50317
Sargsyan, Y., Bickmeyer, U., Gibhardt, C. S., Streckfuss-Bömeke, K., Bogeski, I., and Thoms, S. (2021). Peroxisomes contribute to intracellular calcium dynamics in cardiomyocytes and non-excitable cells. Life Sci. Alliance 4, e202000987. doi:10.26508/lsa.202000987
Sargsyan, Y., and Thoms, S. (2020). Staying in healthy contact: How peroxisomes interact with other cell organelles. Trends Mol. Med. 26, 201–214. doi:10.1016/j.molmed.2019.09.012
Schueren, F., Lingner, T., George, R., Hofhuis, J., Dickel, C., Gärtner, J., et al. (2014). Peroxisomal lactate dehydrogenase is generated by translational readthrough in mammals. eLife 3, e03640. doi:10.7554/eLife.03640
Schueren, F., and Thoms, S. (2016). Functional translational readthrough: A systems Biology perspective. PLoS Genet. 12, e1006196. doi:10.1371/journal.pgen.1006196
Sograte-Idrissi, S., Schlichthaerle, T., Duque-Afonso, C. J., Alevra, M., Strauss, S., Moser, T., et al. (2020). Circumvention of common labelling artefacts using secondary nanobodies. Nanoscale 12, 10226–10239. doi:10.1039/d0nr00227e
Soliman, K., Göttfert, F., Rosewich, H., Thoms, S., and Gärtner, J. (2018). Super-resolution imaging reveals the sub-diffraction phenotype of Zellweger Syndrome ghosts and wild-type peroxisomes. Sci. Rep. 8, 7809. doi:10.1038/s41598-018-24119-2
Valm, A. M., Cohen, S., Legant, W. R., Melunis, J., Hershberg, U., Wait, E., et al. (2017). Applying systems-level spectral imaging and analysis to reveal the organelle interactome. Nature 546, 162–167. doi:10.1038/nature22369
Vassalle, M., and Lin, C.-I. (2004). Calcium overload and cardiac function. J. Biomed. Sci. 11, 542–565. doi:10.1007/BF02256119
Wanders, R. J. A., and Komen, J. C. (2007). Peroxisomes, Refsum’s disease and the alpha- and omega-oxidation of phytanic acid. Biochem. Soc. Trans. 35, 865–869. doi:10.1042/BST0350865
Wanders, R. J. A. (2014). Metabolic functions of peroxisomes in health and disease. Biochimie 98, 36–44. doi:10.1016/j.biochi.2013.08.022
Wanders, R. J. A., and Waterham, H. R. (2006). Biochemistry of mammalian peroxisomes revisited. Annu. Rev. Biochem. 75, 295–332. doi:10.1146/annurev.biochem.74.082803.133329
Watanabe, T., and Suga, T. (1988). Suppression of clofibrate-induced peroxisome proliferation in rat liver by nicardipine, a calcium antagonist. FEBS Lett. 232, 293–297. doi:10.1016/0014-5793(88)80756-7
Weber, F. E., Minestrini, G., Dyer, J. H., Werder, M., Boffelli, D., Compassi, S., et al. (1997). Molecular cloning of a peroxisomal Ca2+-dependent member of the mitochondrial carrier superfamily. Proc. Natl. Acad. Sci. U. S. A. 94, 8509–8514. doi:10.1073/pnas.94.16.8509
Wylin, T., Baes, M., Brees, C., Mannaerts, G. P., Fransen, M., and Van Veldhoven, P. P. (1998). Identification and characterization of human PMP34, a protein closely related to the peroxisomal integral membrane protein PMP47 of Candida boidinii. Eur. J. Biochem. 258, 332–338. doi:10.1046/j.1432-1327.1998.2580332.x
Yang, T., and Poovaiah, B. W. (2002). Hydrogen peroxide homeostasis: Activation of plant catalase by calcium/calmodulin. Proc. Natl. Acad. Sci. U. S. A. 99, 4097–4102. doi:10.1073/pnas.052564899
Yu, H., Liu, Y., Gulbranson, D. R., Paine, A., Rathore, S. S., and Shen, J. (2016). Extended synaptotagmins are Ca 2+ -dependent lipid transfer proteins at membrane contact sites. Proc. Natl. Acad. Sci. U. S. A. 113, 4362–4367. doi:10.1073/pnas.1517259113
Zhang, H., Tamura, H., Yamada, J., Watanabe, T., and Suga, T. (1996). Effect of nicardipine, a calcium antagonist, on induction of peroxisomal enzymes by dehydroepiandrosterone sulfate in cultured rat hepatocytes. J. Toxicol. Sci. 21, 235–241. doi:10.2131/jts.21.4_235
Keywords: peroxisomes, calcium, Ca2+, cell organelle, cardiomyocyte, FRET sensor
Citation: Sargsyan Y, Kalinowski J and Thoms S (2022) Calcium in peroxisomes: An essential messenger in an essential cell organelle. Front. Cell Dev. Biol. 10:992235. doi: 10.3389/fcell.2022.992235
Received: 12 July 2022; Accepted: 10 August 2022;
Published: 30 August 2022.
Edited by:
Joe Costello, University of Exeter, United KingdomReviewed by:
Werner Josef Kovacs, ETH Zürich, SwitzerlandCarlos Bas Orth, Heidelberg University, Germany
Copyright © 2022 Sargsyan, Kalinowski and Thoms. This is an open-access article distributed under the terms of the Creative Commons Attribution License (CC BY). The use, distribution or reproduction in other forums is permitted, provided the original author(s) and the copyright owner(s) are credited and that the original publication in this journal is cited, in accordance with accepted academic practice. No use, distribution or reproduction is permitted which does not comply with these terms.
*Correspondence: Sven Thoms, c3Zlbi50aG9tc0B1bmktYmllbGVmZWxkLmRl