- 1Division of Developmental and Stem Cell Biology, Institute for Quantitative Health Science and Engineering (IQ), Michigan State University, East Lansing, MI, United States
- 2Department of Biomedical Engineering, College of Engineering, Michigan State University, East Lansing, MI, United States
Cardiovascular disease (CVD) is one of the leading causes of mortality worldwide, and frequently leads to massive heart injury and the loss of billions of cardiac muscle cells and associated vasculature. Critical work in the last 2 decades demonstrated that these lost cells can be partially regenerated by the epicardium, the outermost mesothelial layer of the heart, in a process that highly recapitulates its role in heart development. Upon cardiac injury, mature epicardial cells activate and undergo an epithelial-mesenchymal transition (EMT) to form epicardium-derived progenitor cells (EpiPCs), multipotent progenitors that can differentiate into several important cardiac lineages, including cardiomyocytes and vascular cells. In mammals, this process alone is insufficient for significant regeneration, but it might be possible to prime it by administering specific reprogramming factors, leading to enhanced EpiPC function. Here, we show that oxytocin (OXT), a hypothalamic neuroendocrine peptide, induces epicardial cell proliferation, EMT, and transcriptional activity in a model of human induced pluripotent stem cell (hiPSC)-derived epicardial cells. In addition, we demonstrate that OXT is produced after cardiac cryoinjury in zebrafish, and that it elicits significant epicardial activation promoting heart regeneration. Oxytocin signaling is also critical for proper epicardium development in zebrafish embryos. The above processes are significantly impaired when OXT signaling is inhibited chemically or genetically through RNA interference. RNA sequencing data suggests that the transforming growth factor beta (TGF-β) pathway is the primary mediator of OXT-induced epicardial activation. Our research reveals for the first time an evolutionary conserved brain-controlled mechanism inducing cellular reprogramming and regeneration of the injured mammalian and zebrafish heart, a finding that could contribute to translational advances for the treatment of cardiac injuries.
Introduction
Cardiovascular disease (CVD) is the leading cause of mortality in the United States and the rest of the developed world (Virani et al., 2020). These diseases often lead to severe cardiac events, such as a myocardial infarction (MI), which can result in the death of more than 25% of cardiac myocytes, the chief contractile cells of the adult heart. If left untreated, this condition can lead to heart failure (Laflamme & Murry, 2011). Cardiomyocytes (CMs) are terminally differentiated cells, and as such, their innate regenerative capacity is extremely limited and insufficient to restore function to lost myocardium. Instead, the injured heart typically resorts to wound repair, replacing dead cells with fibrotic scar tissue (Bergmann et al., 2009; Fan et al., 2012). Therefore, the ability to induce heart regeneration, which results in growth and proliferation of endogenous diseased cells (and subsequent restoration of cardiac function), would be paramount in the discovery of new therapeutic strategies for the successful treatment of MI and other types of CVD (Laflamme & Murry, 2011; Uygur & Lee, 2016).
The epicardium is the outermost mesothelial layer of the heart that serves a structural and protective role in healthy individuals (Riley, 2012). While its activity level decreases as development proceeds, in adults, it becomes activated upon injury and recapitulates its embryonic phenotype (Peralta et al., 2014; Simões & Riley, 2018). Upon activation, a subset of epicardial cells migrate into the subepicardial space and undergo epithelial-mesenchymal transition (EMT) to form epicardium-derived progenitor cells (EpiPCs) (Rao & Spees, 2017; Smits et al., 2018; Quijada et al., 2020). These EpiPCs are multipotent cardiac progenitors (the only widely accepted source) that contribute to multiple different cell lineages in the heart and can aid in the healing and repair process (Martínez-Estrada et al., 2010; Kikuchi et al., 2011a; Smart et al., 2011; Van Wijk et al., 2012; Streef & Smits, 2021). While there is substantial evidence to suggest that EpiPCs can differentiate into cardiomyocytes during development (Cai et al., 2008; Zhou et al., 2008) and in injured adult hearts (Smart et al., 2011; Van Wijk et al., 2012; Eroglu et al., 2022), this subject is still a matter of ongoing debate (Christoffels et al., 2009; Zhou et al., 2012). Notably, adult epicardial cells also secrete paracrine factors to modulate heart repair and regeneration post-MI (Zhou et al., 2011; Wei et al., 2015). Therefore, the activated epicardium might be the key to unlocking cardiac regeneration pathways in humans.
The brain plays a central role in several energetically demanding processes that serve to maintain body homeostasis (Guyenet & Bayliss, 2015; Dampney, 2016; Morrison, 2016; Roh et al., 2016). In addition, the brain-heart signaling axis regulates heart rate, blood pressure, and systolic and diastolic function (Palma & Benarroch, 2014; Dampney, 2016). The neural endocrine structures, such as the hypothalamus and pituitary, play a particularly important role in maintaining normal cardiovascular function through the release of various hormones (Rhee & Pearce, 2011; Gordan et al., 2015). Therefore, it stands to reason that a process as biologically and energetically demanding as heart regeneration does not occur cell-autonomously and might be under central hormonal control. In fact, there is evidence that several hormones such as estrogen (Xu et al., 2020), thyroid hormones (Hirose et al., 2019), and cortisol (Sallin & Jaźwińska, 2016) affect cardiac regeneration. In addition, a recent study indicates that damage to the hypothalamus inhibits endogenous regenerative processes in vertebrates (Zhang et al., 2018). These findings, combined with the fact that injury-induced epicardial activation can be primed by specific signaling factors (Smart et al., 2011), led us to hypothesize that after cardiac injury, one or more neuroendocrine hormones are released from the hypothalamus into the bloodstream to facilitate heart regeneration. Proper identification and characterization of these hormones will be critical in advancing the translational potential of cardiac regeneration research.
Here, we present evidence that oxytocin (OXT), a nine amino acid neuropeptide produced by the hypothalamus and released by the posterior pituitary, may be the missing link in achieving sufficient EpiPC proliferation, activation, and migration to fully regenerate the lost myocardium in the injured heart. We utilize both in vitro and in vivo injury models to identify and describe the direct involvement of OXT in these processes. First, we differentiate and characterize a mature-like model of human iPSC-derived epicardial cells (hEpiCs) and show that the peptide induces proliferation and activation in these cells by acting through its receptor (OXTR). We then show that oxytocin is released from the brain after cardiac cryoinjury in zebrafish, a process that is critical for heart regeneration and epicardial activation. In addition, inhibition of OXT signaling delays regeneration of adult hearts and epicardium formation in zebrafish embryos. RNA sequencing (RNA-seq) analyses suggest that the TGF-β pathway is the primary mediator of OXT-induced epicardial activation. The findings of this study reveal a novel role for the brain-heart signaling axis in regulating cardiac regeneration and elucidate the critical importance of the epicardium in this process.
Materials and Methods
Stem cell culture
Human iPSCs (hiPSC-L1) were cultured on six well plates coated with growth factor-reduced Matrigel (Corning) in an incubator at 37°C, 5% CO2. Stem cells were maintained in Essential 8 Flex medium (E8 Flex; Thermo Fisher Scientific) containing 1% penicillin/streptomycin until ∼70% confluency was reached, at which point cells were split into new wells using ReLeSR passaging reagent (Stem Cell Technologies) with 2 µM ROCK inhibitor (Thiazovivin/TZV; Selleck Chemicals) added to prevent cells from undergoing apoptosis while in suspension. All hiPSC lines were periodically validated for pluripotency and genomic stability.
hiPSC differentiation to hEpiCs
hiPSCs were differentiated to epicardial monolayers using a modified version of a previously described protocol (Bao et al., 2016, 2017). Cells were dissociated using Accutase (Innovative Cell Technologies), re-plated on Matrigel coated plates, and incubated overnight in E8 Flex +2 µM TZV. Once hiPSC monolayers reached ∼90% confluency, epicardial differentiation was started via the addition of 12 µM CHIR99021 (Selleck Chemicals) in RPMI/B-27 minus insulin (Gibco; Day 0) for 24 h. On Day 3, cells were exposed to 2 µM Wnt-C59 (Selleck Chemicals) in RPMI/B-27 minus insulin for 48 h. On Day 6, cardiac progenitors were then re-plated in RPMI/B-27 (Gibco) with 100 μg/ml Vitamin C (Vit. C) using Accutase and TZV. Cells were directed down the epicardial lineage by the consecutive addition of 9 µM CHIR99021 on Days 7 and 8 and maintained in RPMI/B-27/Vit. C until passage. On day 12, hEpiCs were again re-plated using Accutase and TZV and 2 µM SB431542 (TGF-β receptor inhibitor; Selleck Chemicals) was added to the media to prevent cells from spontaneously undergoing EMT. Epicardial cells were cultured long-term in RPMI/B-27/Vit. C + 2 µM SB431542 and split when full confluency was reached. To ensure that hEpiCs were as mature as possible for experiments, Vitamin C and SB431542 were removed from the culture system 5 days prior to experiments. OXT (Sigma-Aldrich), thymosin β4 (Tocris Bioscience), and other compounds were added directly to the media 2 days later, for a total exposure time of 3 days, unless otherwise indicated. This process was repeated for experimental replicates, with a fresh batch of epicardial cells prepared from hiPSCs each time. Automated cell counting of hEpiCs in 12, 24, 48, and 96-well plate format was conducted using the Cytation 3 and Cytation 5 Cell Imaging Multi-Mode Readers (Biotek), with initial plating densities determined based on the surface area of the wells.
hiPSC lentiviral transduction
Bacteria carrying the plasmid for shRNA-mediated knockdown of OXTR and a scrambled plasmid (both designed with VectorBuilder) were grown on LB agar plates and isolated colonies were expanded in LB broth, both containing ampicillin. The plasmids carried an ampicillin resistance cassette, allowing for their growth. Plasmid DNA was isolated from the bacteria by midiprep (Zymo Research), and purified DNA was transfected into 40% confluent HEK293T cells using Lipofectamine (Invitrogen). Lentiviral packaging plasmids (pVSVg, psPAX2) were also transfected at this time, thereby allowing the generation of a functional lentivirus containing the shRNA molecules of interest. Viral supernatant was collected and concentrated after 48 h and transduced directly into hiPSCs at low to mid-confluency along with 8 μg/ml polybrene (Fisher Scientific). Because all plasmids contained puromycin-resistance cassettes, the stem cell media was changed the next day to E8 Flex containing 0.5 μg/ml puromycin (Thermo Fisher Scientific) to facilitate colony selection. hiPSCs were maintained in puromycin for 5 days and surviving monoclonal colonies were re-plated and expanded to generate new hiPSC lines.
Zebrafish cardiac cryoinjury
Adult zebrafish hearts were subjected to cardiac cryoinjury as described previously (González-Rosa et al., 2011; González-Rosa & Mercader, 2012). Briefly, fish were anesthetized with 0.65 mM tricaine (MS-222) and placed on a wet sponge ventral side up under a stereomicroscope (Leica). The chest cavity was opened by cutting through the pericardium until the point where the beating heart was clearly visible. For cryoinjury, a liquid nitrogen cooled metal probe was gently placed on the apex of the ventricle for ∼45 s. Sham operations were carried out by simply opening the chest cavity without injury. Fish were closely monitored for the first 2 h after surgery and twice daily thereafter until ready for organ collection. Pharmacological inhibition of oxytocin signaling was achieved by injecting 2,3 µl of water or 1 µM L-368,899 (a non-peptide OXTR antagonist) directly into the intrathoracic cavity, as described previously (Bise & Jaźwińska, 2019). Initial injections were administered 24 h after cryoinjury with repeat injections every other day throughout the experiment. All zebrafish were maintained in a dedicated facility between 27,28°C on a 14-h light/10-h dark cycle. Equal numbers of male and female fish were used for cryoinjuries.
Zebrafish embryo experiments
One female and one male adult fish were placed in specialized breeding tanks with a partition between them the night before experiments. The partition was removed early the next morning and fish were given ∼15 min to breed. Fertilized embryos were collected and placed in embryo medium containing 4.96 mM NaCl, 0.179 mM KCl, 0.329 mM CaCl2 • 2H2O, and 0.401 mM MgCl2 • 6H2O dissolved in water. The appropriate concentration of L-368,899 or atosiban (competitive OXTR antagonist) was added directly to the medium and embryos were stored in an incubator at 28 °C for the duration of the experiment. Dead embryos and discarded chorions were removed daily. Developing fish were imaged at 1, 2, 3, 5, and 7 days post-fertilization (dpf) with the Leica M165 FC stereomicroscope with a DFC7000 T fluorescence camera so that epicardium and myocardium formation could be observed in real time. Epicardial cell number was counted manually at 3, 5, and 7 dpf.
Gene expression analysis
Total RNA was extracted from samples using the RNeasy Mini Kit (Qiagen). Cells were lysed directly in their culture wells and tissues were lysed and homogenized using the Bead Mill 4 Homogenizer (Fisher Scientific). Once extracted, RNA was quantified using a NanoDrop (Mettler Toledo), with a concentration of at least 10 ng/μl being required to proceed with reverse transcription. cDNA was synthesized using the Quantitect Reverse Transcription Kit (Qiagen) and stored at -20 °C for further use. Primers for qRT-PCR were designed using the Primer Quest tool (Integrated DNA Technologies) and SYBR Green (Qiagen) was used as the DNA intercalating dye. qRT-PCR plates were run using the QuantStudio 5 Real-Time PCR system (Applied Biosystems) with a total reaction volume of 20 µL. Expression levels of genes of interest were normalized to HPRT1 levels and fold change values were obtained using the 2−ΔΔCT method. At least three to five independent samples were run for each gene expression assay.
Immunofluorescence
hEpiC samples were first transferred onto Millicell EZ slides (MilliporeSigma), fixed in 4% paraformaldehyde (PFA) solution, and washed with PBS +1.5 g/L glycine. Zebrafish tissue samples were extracted, washed in PBS +0.5 mM EDTA solution, and fixed in 4% PFA for 1 hour. After fixation, organs were washed with PBS-glycine and transferred into a PBS +30% sucrose solution for at least 48 h. Samples were then embedded in Optimal Cutting Temperature (OCT) compound (Electron Microscopy Sciences), frozen in a mold, and sectioned at 10 µm thickness using the Leica CM3050 S cryostat. Sectioned tissue was stored at 4 °C for further use. Once ready for staining, antigen retrieval was performed by immersing slides in a sodium citrate buffer (10 mM sodium citrate, 0.05% Tween 20, pH 6.0) for ∼45 min at 95–100°C. All samples (cells and tissues) were blocked and permeabilized with 10% normal donkey serum, 0.5% bovine serum albumin (BSA), and 0.5% Triton X-100 in PBS for 1 h at room temperature. After washing, primary antibodies were diluted in antibody solution (1% normal donkey serum, 0.5% BSA, 0.5% Triton X-100 for tissues, 0.05% Triton X-100 for cells, all dissolved in PBS), added to the samples, and incubated overnight at 4°C. The next day, cells and tissues were washed, and secondary antibodies were diluted in antibody solution (with 0.05% Triton X-100) and added for 2 h at room temperature. 4’,6-diamidino-2-phenylindole (DAPI, Thermo Fisher Scientific) was then added immediately at a concentration of 1:1000 to label DNA. Stained slides were washed 3 times in PBS and No.1 coverslips (VWR) were added using ProLong Gold Antifade Mountant (Thermo Fisher Scientific).
Histology
Masson’s trichrome staining was carried out using a kit from IMEB Inc. and following manufacturer instructions. Briefly, sectioned tissue was immersed in Bouin’s solution for ∼50 min at 56–64°C to improve staining quality and washed in tap water until the yellow color was fully removed from the hearts. Slides were then stained with Biebrich’s scarlet/acid fuchsin solution for 7 min, washed in deionized (DI) H2O, and immersed in phosphomolybdic/phosphotungstic acid solution for 18 min to decolorize fibrous tissue. Slides were then transferred directly to aniline blue solution for 12 min to stain collagen fibers, washed in DI H2O, and immersed in 1% acetic acid for 3 min to stabilize the staining. After washing, tissue was dehydrated by successive exposure to 95% ethanol, 100% ethanol, and xylene and coverslips were added using Eukitt quick-hardening mounting medium (Sigma-Aldrich). Scar size was quantified manually by tracing the injured myocardial area in ImageJ and dividing it by the total myocardial area. At least two sections from at least three independent hearts were analyzed for each condition at each time point.
Confocal microscopy and image analysis
All samples were imaged using the Zeiss LSM880 NLO Confocal Microscope system or the Nikon A1 Confocal Laser Microscope. Images were analyzed and prepared for publication using Fiji software. Cell counts were performed manually using the “Wand” tool and automatically using the “Threshold” and “Analyze Particles” tools. Individuals who conducted manual cell counting of hEpiCs were blinded to experimental conditions and at least five images were analyzed for each group. For analysis of zebrafish confocal images, at least three independent fish from each condition were analyzed. Epicardial cell number was quantified by counting the number of GFP + cells on the surface of the heart and in the subepicardial space per field of view. To ensure consistency in analysis, each image was taken immediately adjacent to the injured area. The number of h3p+/wt1b + cells in the subepicardial region was normalized to the number of DAPI-stained nuclei. Cardiomyocyte proliferation was quantified by manually counting the number of PCNA + cells per field of view in the myocardium of the infarct border zone. At least two regions per heart were analyzed. Vascularization was quantified by measuring the GFP + area within the injury region and dividing that value by the total injured area. In all cases, TNNT2 was utilized as a counterstain to allow for visualization of the cryoinjury. Quantification analyses were performed in a blinded fashion.
RNA sequencing
RNA was extracted from 3 control and 3 hEpiC samples treated with 100 nM OXT as described above. RNA was quantified using a Qubit Fluorometer (Thermo Fisher Scientific) and samples were sent to the MSU Genomics core, where their quality was tested using the Agilent 2,100 Bioanalyzer. Samples were sequenced using an Illumina HiSeq 4,000. For RNA-seq sample processing, a pipeline was created in Galaxy. Briefly, sample run quality was assessed with FASTQC and alignment to hg38 was carried out using HISAT2. Counts were obtained using featureCounts and differential expression analysis was performed with EdgeR. Further downstream bioinformatic analysis was performed using Phantasus 1.11.0 (artyomovlab.wustl.edu/phantasus), ToppGene Suite (http://toppgene.cchmc.org), and Enrichr (https://maayanlab.cloud/Enrichr/).
Statistical analysis
All graphs were made using GraphPad software. Statistical significance was evaluated with a standard unpaired Student’s t-test (2-tailed) when comparing two groups or with a 1-way ANOVA with Tukey’s or Dunnett’s post-test for multiple comparison analysis. Regardless of the test used, a p-value of less than 0.05 was considered statistically significant. All data are presented as mean ± SEM and represent a minimum of three independent experiments with at least three technical replicates unless otherwise stated. For RNA-seq analysis, a false discovery rate (FDR) was used to determine statistical significance.
Results
Establishing human iPSC-derived epicardial progenitor and mature-like epicardial cell cultures
To produce mature-like epicardial cells from human iPSCs (hEpiCs) we started by deriving epicardial progenitor cells using a 3-step Wnt modulation strategy (Bao et al., 2016, 2017) (Figure 1A). After 12 days of culture, hEpiCs display classic epithelial morphology (Figure 1B), express epicardial progenitor markers WT1 and TCF21 (Figures 1C,D), and show increased WT1 and CDH1 (epithelial cadherin) levels as they age (Figures 1E,F), suggesting an increase in epicardial nature. These cells can be routinely maintained and cultured in medium containing Vitamin C and SB431542 (a TGF-β receptor inhibitor), allowing them to retain their epithelial morphology for at least 20 passages (Bao et al., 2016, 2017), however, we found that these compounds prevent hEpiC maturation. As the epicardium develops and matures, its resident cells transition from a highly proliferative, migratory, and EMT state to a more mature, quiescent phenotype. In the mouse heart, key genes involved in epicardial activation are barely detectable by 3 months of age (Smart et al., 2011; Zhou et al., 2011; Smits et al., 2018). We hypothesized that removing Vitamin C and SB431542 from the hEpiC media for a short time would allow the cells to mature further in the epicardial lineage providing a better model to study epicardial activation in vitro. Thus, we removed these two factors and cultured hEpiCs for 5 days at confluence without passaging. Cells were then assayed for gene expression of common epicardial, smooth muscle, and fibroblast markers to assess potential transdifferentiation into other cell types. Nearly 100% of hEpiCs maintained TJP1 expression (the highly specific epithelial tight junction protein ZO-1) (Figure 1G), and no significant difference in expression of smooth muscle and fibroblast markers CNN1, VIM, and ACTA2 was observed, suggesting maturated hEpiCs maintained their epithelial nature and did not undergo EMT (Figure 1H). We found that maturation conditions reduced hEpiC proliferation (cell counts were 2-fold higher when cultured in media containing Vitamin C and SB431542 compared to when they were absent) (Figure 1I) and decreased progenitor and EMT marker expression (WT1, TCF21, NT5E), while at the same time increasing mature epithelial marker expression (CDH1) (Figure 1J), confirming our hypothesis. Therefore, all experiments with epicardial cells were performed using this final media formulation to ensure that hEpiCs resembled epicardial cells in the adult heart as much as possible.
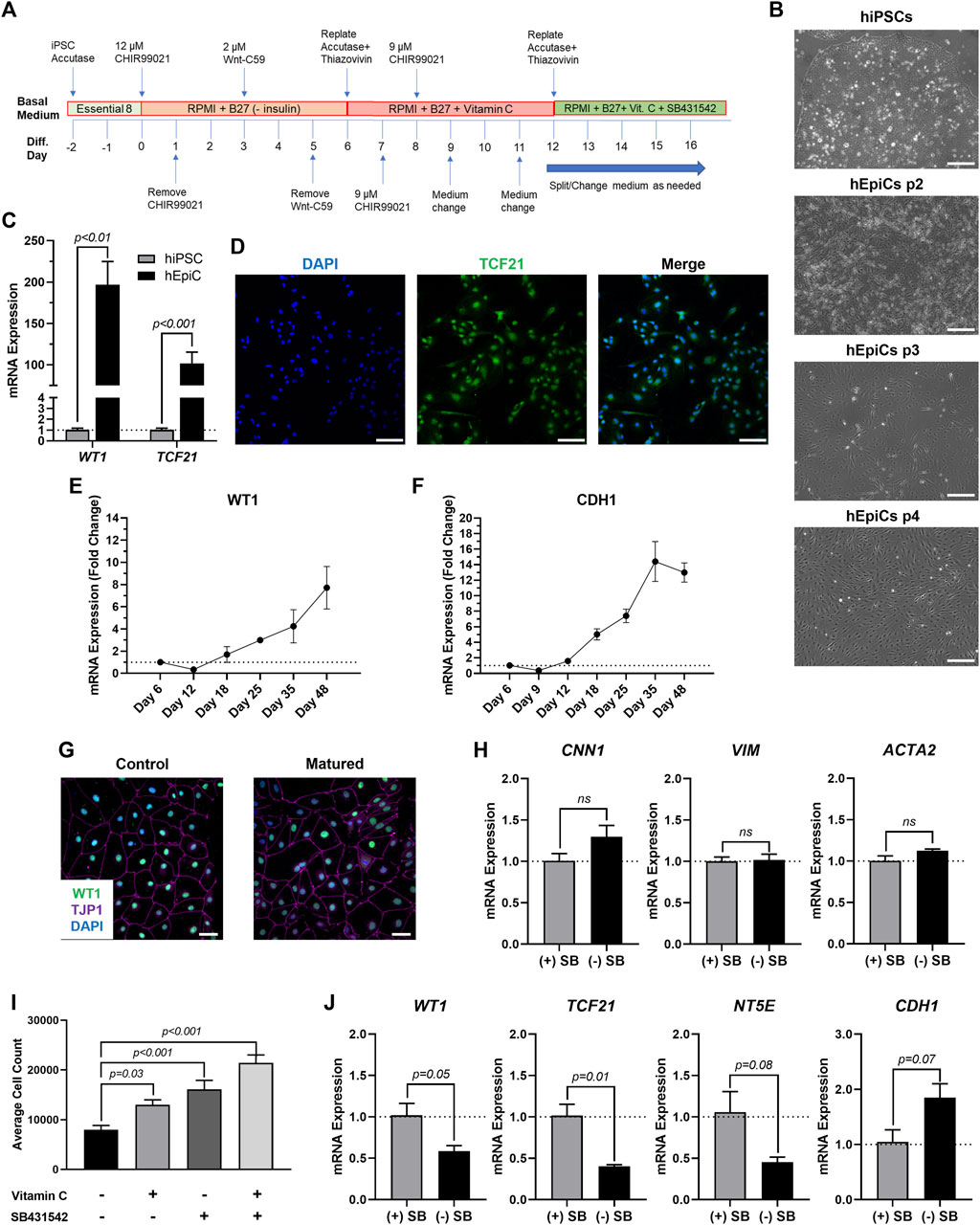
FIGURE 1. Differentiation of Mature-Like Epicardial Cell Cultures from hiPSCs. (A) Schematic of protocol used for hEpiC differentiation. (B) Brightfield images of hEpiCs through four passages showing the gradual accumulation of the classic epithelial cell cobblestone morphology; scale bar: 500 µm. (C) qRT-PCR data for epicardial markers WT1 and TCF21 in hiPSCs and hEpiCs; n = 4-6 per cell line. (D) Confocal immunofluorescent images for TCF21 (green) and DAPI (blue) showing nearly 100% epicardial differentiation efficiency from hiPSCs; scale bar: 100 µm. (E,F) Time course qRT-PCR data for WT1 (E) and CDH1 (F) throughout hEpiC differentiation, suggesting an increase in epicardial nature over time; n = 3 per time point. (G) Confocal immunofluorescent images showing robust expression of epithelial markers after removal of Vitamin C and SB431542 from the culture media. Epicardial cells are labeled with WT1 (green), epithelial membranes with TJP1 (magenta), nuclei with DAPI (blue); scale bar: 50 µm. (H) qRT-PCR data for hEpiCs in the presence or absence of SB431542, showing no change in smooth muscle or fibroblast differentiation; n = 3 per condition. (I) Absolute counts of DAPI-labeled nuclei after exposure to different combinations of Vitamin C and SB431542; n > 20 per condition. (J) qRT-PCR data for hEpiCs in the presence or absence of SB431542 (SB), showing an increase in maturity when this compound is removed from the media; n = 3 per condition.
Oxytocin induces human epicardial cell activation to a progenitor-like state
Because the brain is critical for maintenance of body homeostasis (Guyenet & Bayliss, 2015; Dampney, 2016; Morrison, 2016; Roh et al., 2016) and damage to the hypothalamus inhibits limb regeneration in vertebrates (Zhang et al., 2018), we hypothesized that the brain neuroendocrine system could also play a role in the process of heart regeneration. To identify potential neuroendocrine hormones involved in epicardial activation and possibly heart regeneration, we screened fifteen endogenous candidates in hEpiCs meeting the following two main criteria: 1) being involved in the physiological response to injury and 2) being produced and secreted by neuroendocrine tissue (see Supplementary Table S1). We identified 15 candidates and screened each of them for their proliferative effects by adding them to mature hEpiCs in culture. After 3 days, the percentage of phosphorylated histone H3 (H3P) positive cells was measured as a putative marker of proliferation using a high-content imaging microscope. Oxytocin (OXT), a neuroendocrine peptide released by the neurohypophysis, had the strongest proliferative effects (∼3-fold versus control) (Figure 2A) and was selected for further downstream analysis (Figure 2B). We then exposed hEpiCs to different OXT concentrations in the physiological range and assessed cellular proliferation levels by two alternative methods, Ki67 staining and automated direct cell number counts using a nuclear dye (DAPI), as epicardial cells are mononucleated. We found that the percentage of hEpiCs double positive for both WT1 and Ki67 increased by ∼50% 3 days after addition of 100 nM OXT (Figure 2C,D). Automated direct cell counting using DAPI staining produced comparable results and indicated that the absolute number of epicardial cells doubled with oxytocin exposure (Figure 2E). We compared the effect of OXT to that of thymosin β4, a short peptide previously shown to elicit strong epicardial activation in vitro and in vivo in mice (Smart et al., 2007, 2011; Wang et al., 2021). Administration of 100 nM OXT caused a significantly more potent proliferative effect than thymosin β4 (Figure 2F). OXT induced hEpiC epithelial-to-mesenchymal transition (EMT), as determined by an increase in gene expression for EMT markers WT1, TCF21, and SNAI1 (Figure 2G). Taken together, these data suggest that oxytocin induces epicardial cell activation to a progenitor-like state.
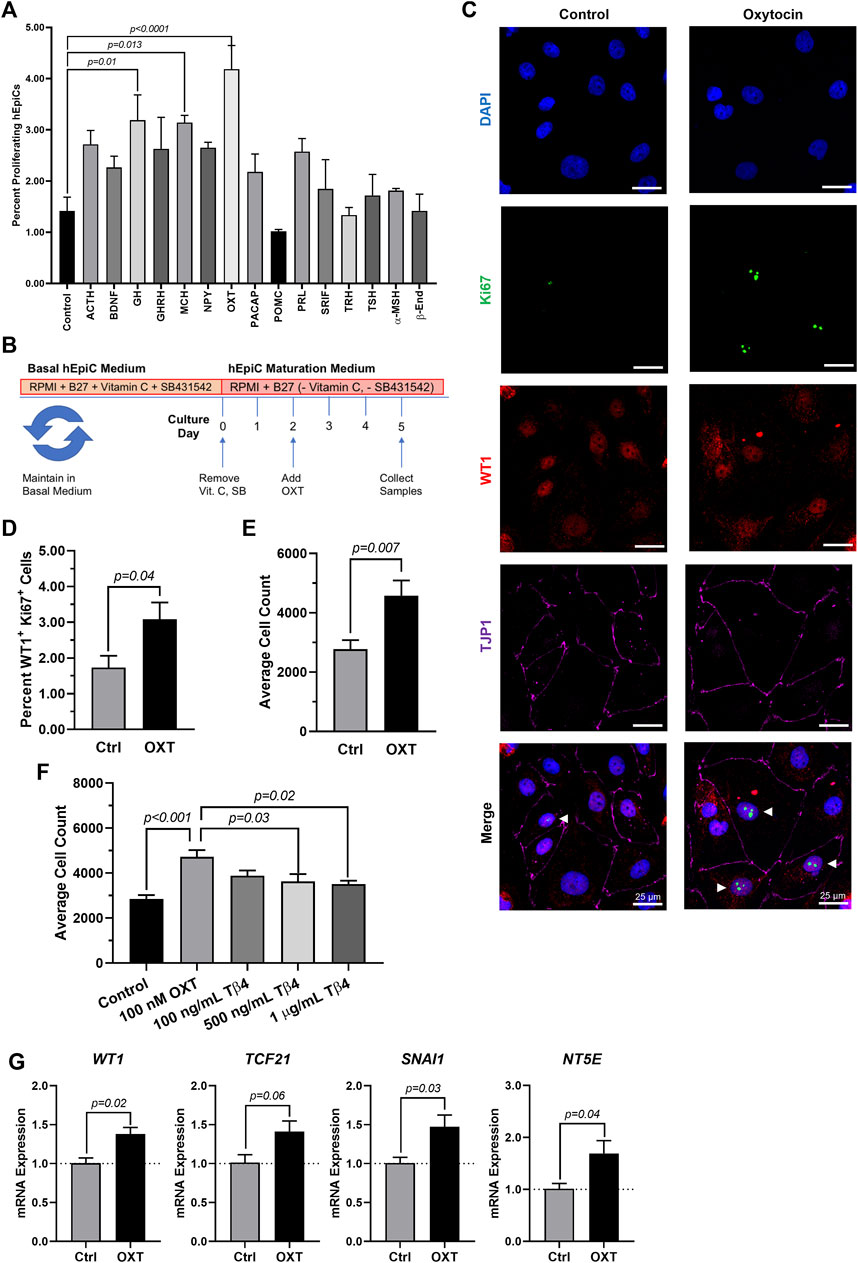
FIGURE 2. Oxytocin Induces Proliferation and Activation of hiPSC-Derived Epicardial Cells. (A) Screening results for 15 candidate neuroendocrine peptides, shown as the percentage of hEpiCs expressing H3P after exposure to each compound; n = 3 per condition. (B) Experimental timeline used to quantify OXT-induced epicardial activation. (C,D) Confocal immunofluorescent images (C) and quantification (D) of proliferating hEpiCs after 3 days OXT exposure. In (C), epicardial cells are labeled with WT1 (red), epithelial membranes are labeled with TJP1 (magenta), proliferating cells (arrowheads) are labeled with Ki67 (green), nuclei are labeled with DAPI (blue); n = 6 images per condition, scale bar: 25 µm. (E,F) Absolute counts of DAPI-labeled epicardial cell nuclei after 3 days exposure to OXT (E) or thymosin β4 (F), a compound previously shown to induce epicardial activation; n ≥ 6 per condition. (G) qRT-PCR data for epicardial cells exposed to OXT, showing an increase in EpiPC (WT1, TCF21), EMT (SNAI1), and mesenchymal (NT5E) markers; n = 4 per condition.
Oxytocin exerts its pro-regenerative effects through the oxytocin receptor (OXTR)
We sought to gain some mechanistic insight as to how OXT causes its effects in vitro. There is only one oxytocin receptor in humans (OXTR), and it is a G-protein coupled receptor (GPCR) (Gimpl & Fahrenholz, 2001). We first conducted a dose-response experiment and quantified epicardial cell proliferation at each concentration. OXT had a concentration-dependent effect on hEpiC proliferation, with a maximal response (>2-fold increase in epicardial cell counts) occurring at 100 nM and an EC50 at ∼300 p.m. (Figure 3A and Supplementary Figure 1A), consistent with a GPCR receptor-mediated response. Gene expression analysis showed that OXTR expression increased 20 to 30-fold in hEpiCs compared to undifferentiated hiPSCs (Figure 3B). Immunostaining and confocal imaging in both cell types confirmed that OXTR is present on the cell membrane and in the cytoplasm only in hEpiCs (Figure 3C). Epicardial cells did not express any detectable levels of OXT as assessed by qRT-PCR. In addition to binding its own receptor, oxytocin can also bind to the arginine vasopressin (AVP) receptors in humans, albeit with lower affinity than to OXTR (Gimpl & Fahrenholz, 2001), however hEpiCs also did not express any of the three AVP receptor isoforms. These data led us to believe that oxytocin acts through OXTR to induce epicardial activation. We created an OXTR knockdown hiPSC line using an shRNA-lentivirus targeting OXTR (Supplementary Figure 1B). This procedure resulted in a >90% knockdown in OXTR expression compared to scrambled epicardial cells (Supplementary Figure 1C) and did not affect the ability of hiPSCs to differentiate into hEpiCs (Supplementary Figure 1D). Exposure to 100 nM OXT led to strong hEpiC activation in scrambled cells, with an even greater response to LIT-001, a recently characterized and very potent non-peptide OXTR agonist (Frantz et al., 2018; Hilfiger et al., 2020) (Supplementary Figure 1E). These findings were confirmed when we stained the cells for proliferation marker Ki67. We observed a ∼40% increase in proliferating hEpiCs in the scrambled line when challenged with 100 nM OXT, but no response in the shOXTR line (Figures 3D,E). We next carried out qRT-PCR gene expression analyses to determine if EMT was also affected by OXTR knockdown. We observed a 2 to 4-fold increase in hEpiC activation (WT1, TCF21, and SNAI1 marker expression) in scrambled cells, but these effects were entirely prevented in OXTR knocked-down hEpiCs (Figure 3F). We concluded that oxytocin acts through the oxytocin receptor to induce human epicardial cell activation in vitro.
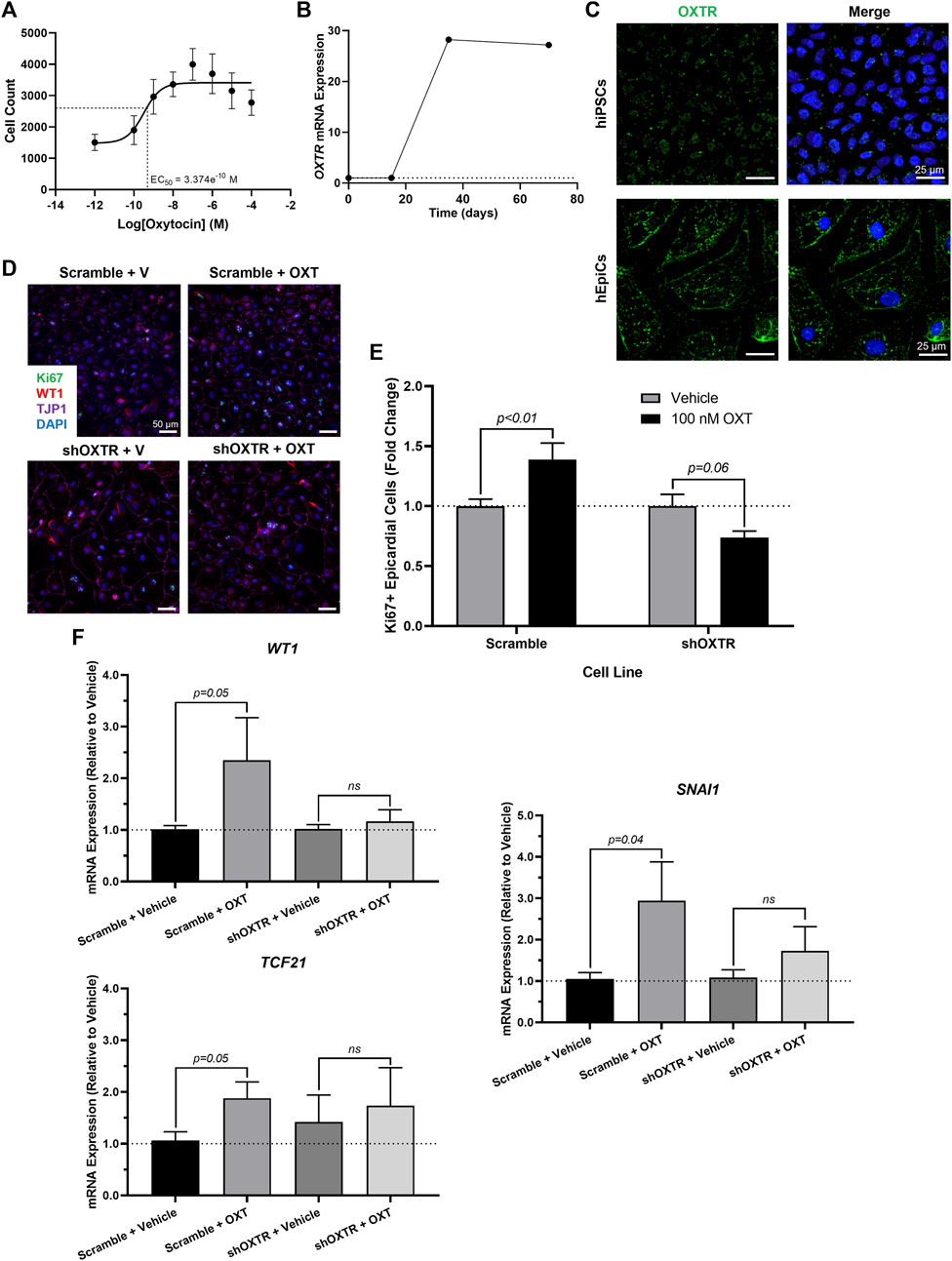
FIGURE 3. OXTR Knockdown Decreases Proliferation and Activation of hiPSC-Derived Epicardial Cells. (A) Dose-response data for hEpiCs exposed to different concentrations of OXT over the course of 3 days, expressed as number of nuclei at each concentration; n = 10 per concentration. (B) Time course qRT-PCR data for OXTR in hEpiCs, dashed line corresponds to expression in undifferentiated hiPSCs. (C) Confocal immunofluorescent images for OXTR (green) and DAPI (blue) showing oxytocin receptor expression on the cell membrane and in the peri-nuclear region only in hEpiCs; scale bar: 25 µm. (D,E) Confocal immunofluorescent images (D) and quantification (E) of proliferating hEpiCs in both cell lines in the presence and absence of OXT at day 25 of differentiation. In (D), epicardial cells are labeled with WT1 (red), epithelial membranes are labeled with TJP1 (magenta), proliferating cells are labeled with Ki67 (green), nuclei are labeled with DAPI (blue); n = 10 images per condition, scale bar: 50 µm. (F) qRT-PCR data for scrambled and shOXTR hEpiCs, showing an increase in epicardial activation in control cells that is prevented after OXTR knockdown; n = 6 per condition, V: Vehicle.
Oxytocin acts through the TGF-β signaling pathway to induce epicardial activation
To obtain additional clues related to the potential mechanism of action driving the effects of oxytocin, we performed RNA-seq on hEpiCs in control and OXT-treated conditions as described above. RNA was collected for transcriptomic differential gene expression analysis after 3 days of 100 nM OXT exposure. We found that oxytocin induced significant widespread gene expression changes (Figure 4A). Computational analysis using gene ontology identified upregulated and downregulated clusters consistent with our previous observations on the effects of oxytocin (induction of a progenitor-like state, increased proliferation, EMT) (Figure 4B,C). Of particular interest was the upregulation of TGF-β/BMP pathway biological processes, as well as developmental ones (Figure 4B). Among downregulated processes, a series of metabolic functions well-ascribed to the epicardium were identified, consistent with our proposed model of mature epicardial cells becoming epicardial progenitors upon OXT treatment. Leading genes driving the TGF-β pathway activation were ligands LEFTY2, GDF15, and INHBB, and the BMP pathway activator TMEM100 (Figure 4D). Overall, these data suggest that oxytocin, through the activation of OXTR, can promote the expression of proteins involved in TGF-β and BMP signaling in the epicardium, leading to increased epicardial progenitor cell mobilization.
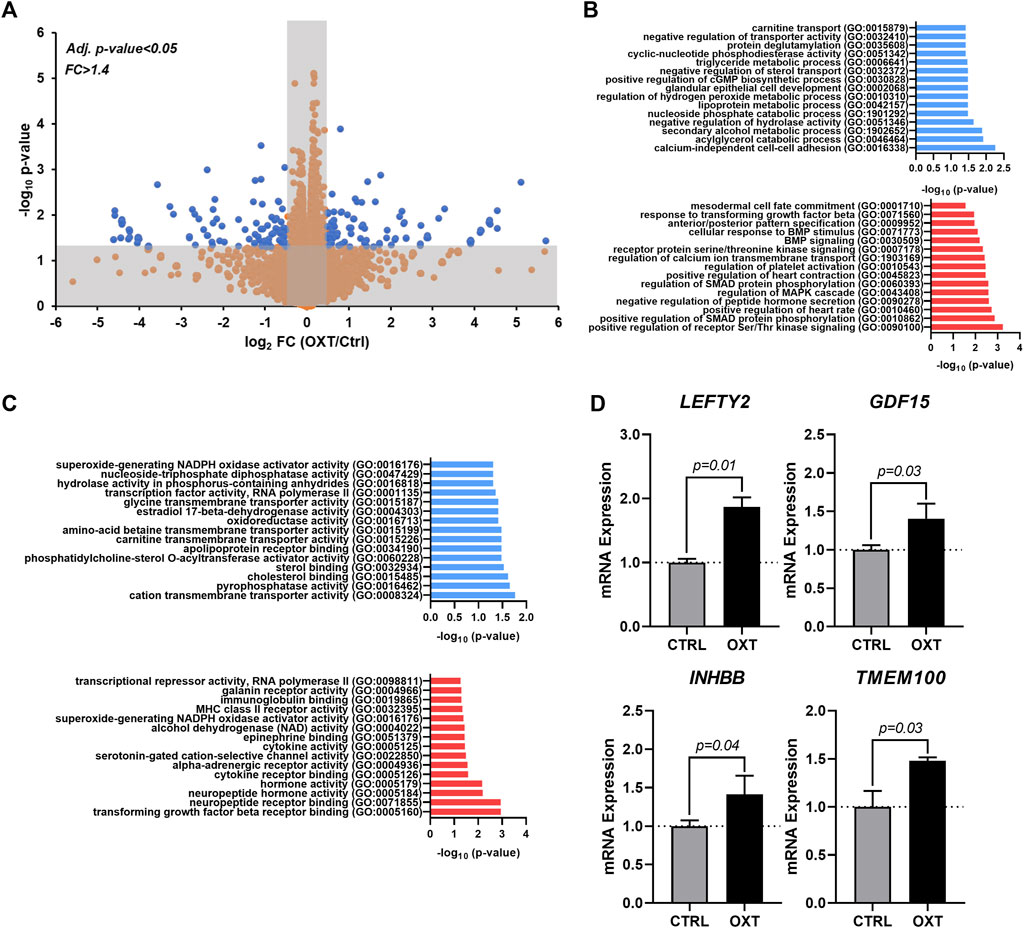
FIGURE 4. Transcriptome Analysis of Oxytocin-Treated Human Epicardial Cells. (A) Volcano plot showing differentially expressed genes between control and OXT-treated hEpiCs, as determined by RNA sequencing; Blue dots correspond to genes with a fold change ≥1.4 and a p-value ≤ 0.05. (B,C) Gene ontology analysis showing upregulated and downregulated biological processes (B) and molecular functions (C) after oxytocin exposure in hEpiCs. (D) Relative mRNA expression of ligands for the TGF-β pathway and activators of BMP signaling in control and OXT-treated hEpiCs; n = 3 per condition.
Oxytocin signaling plays a role in the formation and migration of the proepicardial organ (PEO) during heart development
Our data suggest that OXT might play an important and overlooked role in epicardial function in adults. We hypothesized that this may be reflected during heart development too. We therefore sought to determine if oxtr (the zebrafish ortholog to mammalian OXTR) inhibition would have deleterious effects on epicardium formation during heart development. The epicardium derives from an extra-cardiac cell cluster originating in the splanchnic mesoderm called the proepicardial organ (PEO). Once the PEO forms, it contributes new cells to the developing heart by migration into the epicardial and subepicardial spaces. These cells undergo EMT to form EpiPCs, which go on to differentiate into mature epicardial cells and other cardiac cell types (Simões & Riley, 2018; Quijada et al., 2020). We bred double transgenic myl7-DsRed2, tcf21-nls-eGFP zebrafish embryos with a dsRed2 reporter in cardiomyocytes and an enhanced green fluorescent protein (EGFP) tag attached to a nuclear localization signal for tcf21, a well-established EpiPC marker (Kikuchi et al., 2010; Wang et al., 2011). This method allowed us to visualize the development of the epicardium and myocardium in real time via fluorescence microscopy. We dissolved different concentrations of L-368,899, a non-peptide OXTR antagonist, into zebrafish embryo medium and imaged epicardium formation at different time points (3, 5, and 7 dpf), quantifying the number of tcf21 positive (green) cells covering the ventricular myocardium (red). L-368,899 induced a statistically significant decrease in the number of epicardial cells covering the ventricle shortly after PEO formation, at 3 dpf (Figures 5A,B). As time passed, this difference intensified and manifested as a delay in epicardial formation at 5 and 7 dpf (Figures 5C–F). We also found that oxtr inhibition adversely affected formation of the atrial epicardium, as tcf21+ nuclei counts on the atrium were modestly (∼15–30%) lower after drug treatment too (Supplementary Figures 2A,B). We confirmed the above findings by repeating this experiment with atosiban, a competitive OXTR antagonist (Supplementary Figures 2C,D). We concluded that oxytocin receptor inhibition significantly disrupts the formation of the epicardium in zebrafish. This delay could be due to defects in proepicardial cell migration to the surface of the developing heart, as the proepicardial cluster appears farther away from the myocardium in atosiban-treated embryos than in control embryos (Supplementary Figures 2C,D). Alternatively, it could be due to a defect in epicardial cell proliferation, as both migration and proliferation of epicardial progenitors seem to be affected by OXT.
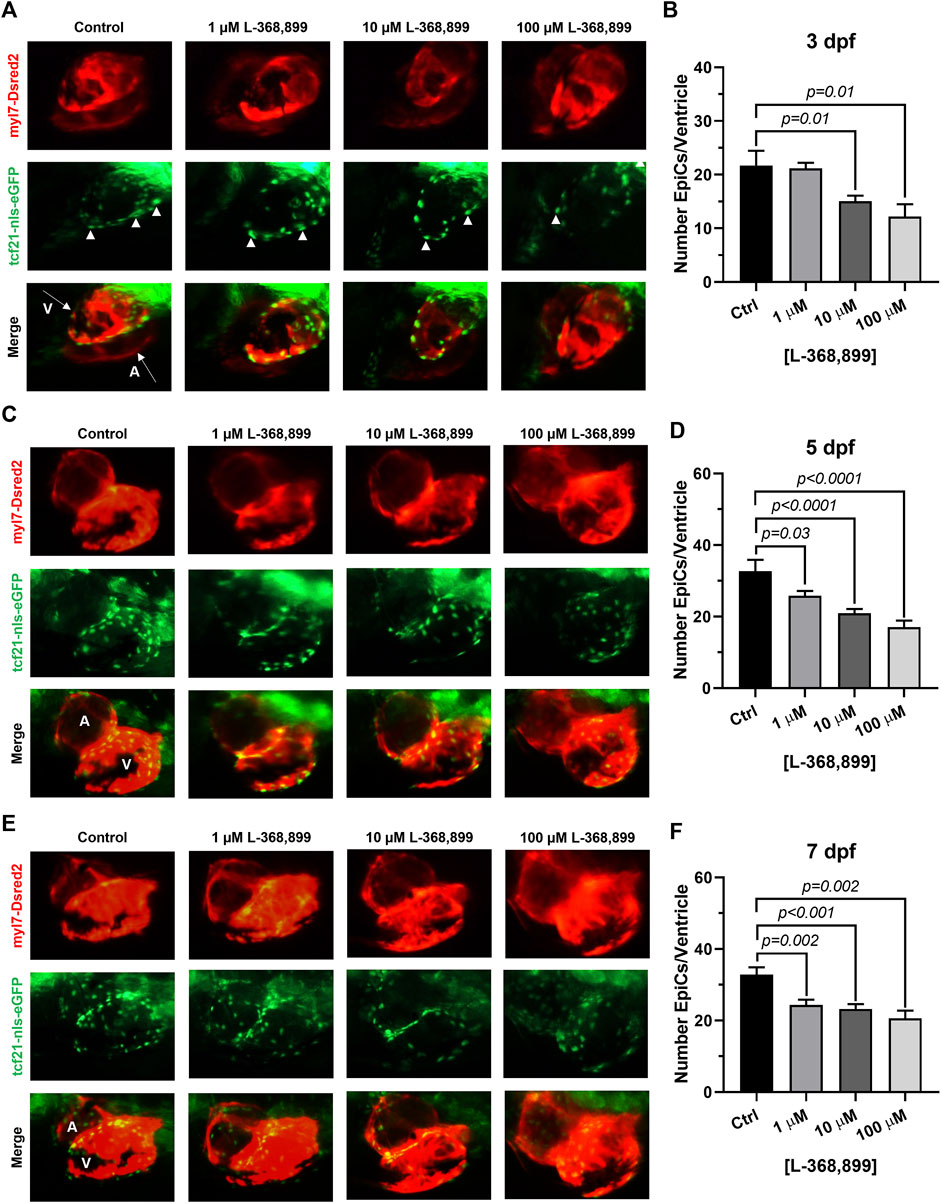
FIGURE 5. Oxytocin Signaling is Necessary for Epicardium Development in Zebrafish. (A–F) Fluorescent images (A,C,E) and epicardial cell counts per ventricle (B,D,F) of developing zebrafish embryos at 3, 5, and 7 dpf treated with different concentrations of L-368,899. In (A) (C), and (E) proepicardial and epicardial cell nuclei are labeled with GFP (tcf21-nls-eGFP, arrowheads), myocardium is labeled with DsRed2 (red); n ≥ 5 embryos per condition for each time point; (A) Atrium, dpf: Days Post-Fertilization, V: Ventricle.
Oxytocin signaling is necessary for heart regeneration in vivo
The zebrafish is a powerful naturally regenerating organism popular for heart regeneration studies (Poss et al., 2002; Jopling et al., 2010; Aguirre et al., 2014). We decided to test if the role OXT plays in epicardial activation was conserved during zebrafish heart regeneration using a well-established cardiac cryoinjury model (González-Rosa et al., 2011; González-Rosa & Mercader, 2012) (Figure 6A). After cryoinjury, we observed an 18-fold increase in oxt mRNA levels in zebrafish brains at 3 dpi (days-post injury) and lasting until at least 7 dpi (Figure 6B), suggesting that a burst of oxytocin is released from the brain into the bloodstream after cardiac injury over a sustained period of time. To determine the functional significance of this oxytocin burst, we injected the specific OXTR inhibitor L-368,899 directly into the thoracic cavity of the fish right after the cryoinjury procedure and every other day thereafter. The size of the injured myocardial area was assessed by Masson’s trichrome staining after injury in control and L-368,899 treated hearts. The differences in scar size between control and treated animals increased as time passed after cryoinjury. Inhibitor-treated animals displayed a modest increase in injured myocardium (∼1.25-1.5 fold) at 3 and 7 dpi and a ∼2-fold statistically significant increase at 14 dpi (Figures 6C,D). In addition, injured hearts treated with L-368,899 had more myocardial fibrosis accumulation than control hearts at 14 dpi (Figure 6E). Taken together, these data suggest that oxytocin signaling is necessary for proper heart regeneration. Two typical physiological responses observed in zebrafish heart regeneration are cardiomyocyte proliferation and endothelial revascularization adjacent to the injured area (González-Rosa et al., 2017; Sanz-Morejón & Mercader, 2020). Therefore, we collected control and treated hearts at 3 dpi and co-stained for proliferating cell nuclear antigen (PCNA), a commonly used cell proliferation marker in zebrafish heart regeneration studies (Jopling et al., 2010; Aguirre et al., 2014) and TNNT2, a specific cardiomyocyte marker. We observed a significant >2-fold decrease in cardiomyocyte proliferation at the infarct border zone after L-368,899 administration (Figures 6F,G). We also conducted cryoinjuries in fli1-eGFP zebrafish, a transgenic strain that specifically labels endothelial and endocardial cells with GFP (Lawson & Weinstein, 2002). We found that GFP fluorescence in the cryoinjured area was >1.5-fold higher in control fish compared to treated animals, suggesting that reendothelialization and vascular regrowth in the wound were also slowed by oxtr inhibition (Figures 6H,I). The deleterious effects of L-368,899 on regeneration may therefore be due to a global response in multiple cardiac cell types.
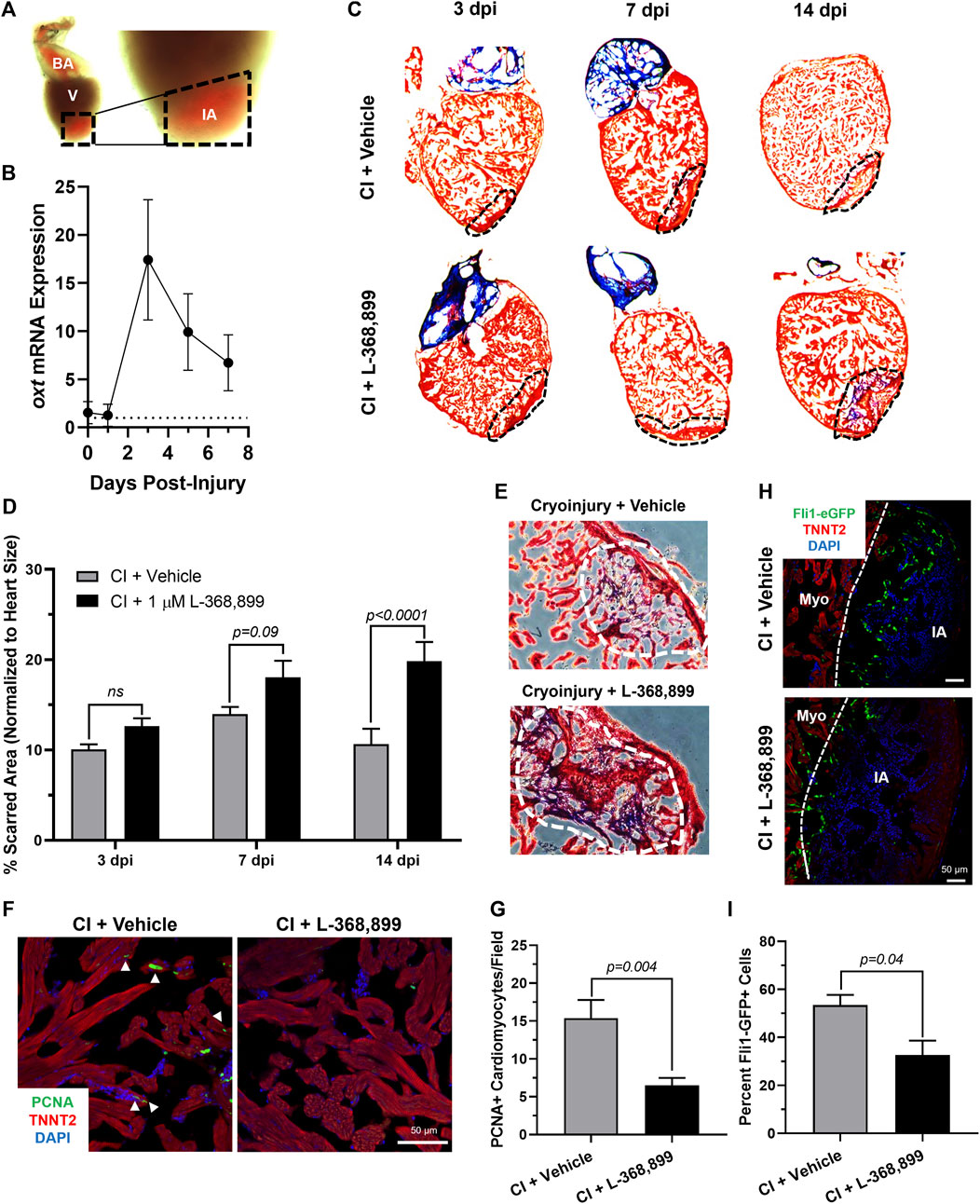
FIGURE 6. Oxytocin Signaling is Necessary for Proper Heart Regeneration in Zebrafish. (A) Example of a freshly cryoinjured zebrafish heart, dashed lines demarcate injured area. (B) Time course qRT-PCR data for oxt in zebrafish brains after cardiac cryoinjury; n = 3 per time point. (C,D) Masson’s trichrome staining (C) and quantification (D) of cryoinjured zebrafish hearts at 3, 7, and 14 dpi treated with and without 1 µM L-368,899. In (C), myocardium is stained red, collagen is stained blue, dashed lines demarcate injured area, which is quantified in (D); n = 3–5 hearts per time point. (E) Representative images of cryoinjured zebrafish myocardium (red) at 14 dpi showing more fibrosis accumulation (blue) after OXTR inhibition. Dashed lines demarcate fibrotic area. (F–G) Confocal immunofluorescent images (F) and quantification (G) of proliferating cardiomyocytes in cryoinjured zebrafish hearts at 3 dpi adjacent to injured areas. In (F), cardiomyocytes are labeled with TNNT2 (red), proliferating cells (arrowheads) with PCNA (green), nuclei with DAPI (blue); n = 8 images per condition, scale bar: 50 µm. (H–I) Confocal immunofluorescent images (H) and quantification (I) of fli1-eGFP transgenic zebrafish at 3 dpi showing endothelial revascularization in cryoinjured and L-368,899-treated hearts. In (H), endothelial and endocardial cells are labeled with GFP (green), cardiomyocytes with TNNT2 (red), nuclei with DAPI (blue); n = 3,4 images per condition, scale bar: 50 μm; BA: Bulbus Arteriosus, CI: Cryoinjury, CM: Cardiomyocyte, dpi: Days Post-Injury, IA: Injured Area, Myo: Myocardium, V: Ventricle.
Next, we explored how blocking oxt affected epicardial progenitor cell activation in vivo. Because one hallmark of epicardial activation after cardiac injury in vivo is upregulation of WT1 levels (Smits et al., 2018; Quijada et al., 2020), we next collected cryoinjured hearts to quantify its expression. We found that wt1b increased significantly after cryoinjury, with peak mRNA levels at 3 dpi (Figure 7A) and expression patterns very consistent with the brain release of oxt (Figure 6B). In addition, immunofluorescence using transgenic tcf21-nls-eGFP fish showed an expansion of epicardial progenitors within the outer layers of the heart, but these effects were abolished in L-368,899 treated animals (Figures 7B–D). EpiPC migration towards the injury site was also prevented after oxtr inhibition, as control fish displayed ∼2-fold more h3p+/wt1b + nuclei in the subepicardial space (Supplementary Figures 3A,B). We also found that L-368,899 administration resulted in significantly lower expression of wt1b and tcf21 compared to control hearts (Figure 7E) and decreased expression of EMT markers, as revealed by snai1a and snai2 mRNA levels (50% reduction) (Figure 7F). Thus, expansion of the subepicardial area and migration of WT1+ EpiPCs into the myocardium, two important features of zebrafish heart regeneration (González-Rosa et al., 2017; Smits et al., 2018; Quijada et al., 2020; Sanz-Morejón & Mercader, 2020), were gravely impaired upon blocking of oxytocin signaling. We concluded that oxtr inhibition prevents robust activation of the epicardium and its progenitor cell populations after injury, leading to an impaired regenerative response.
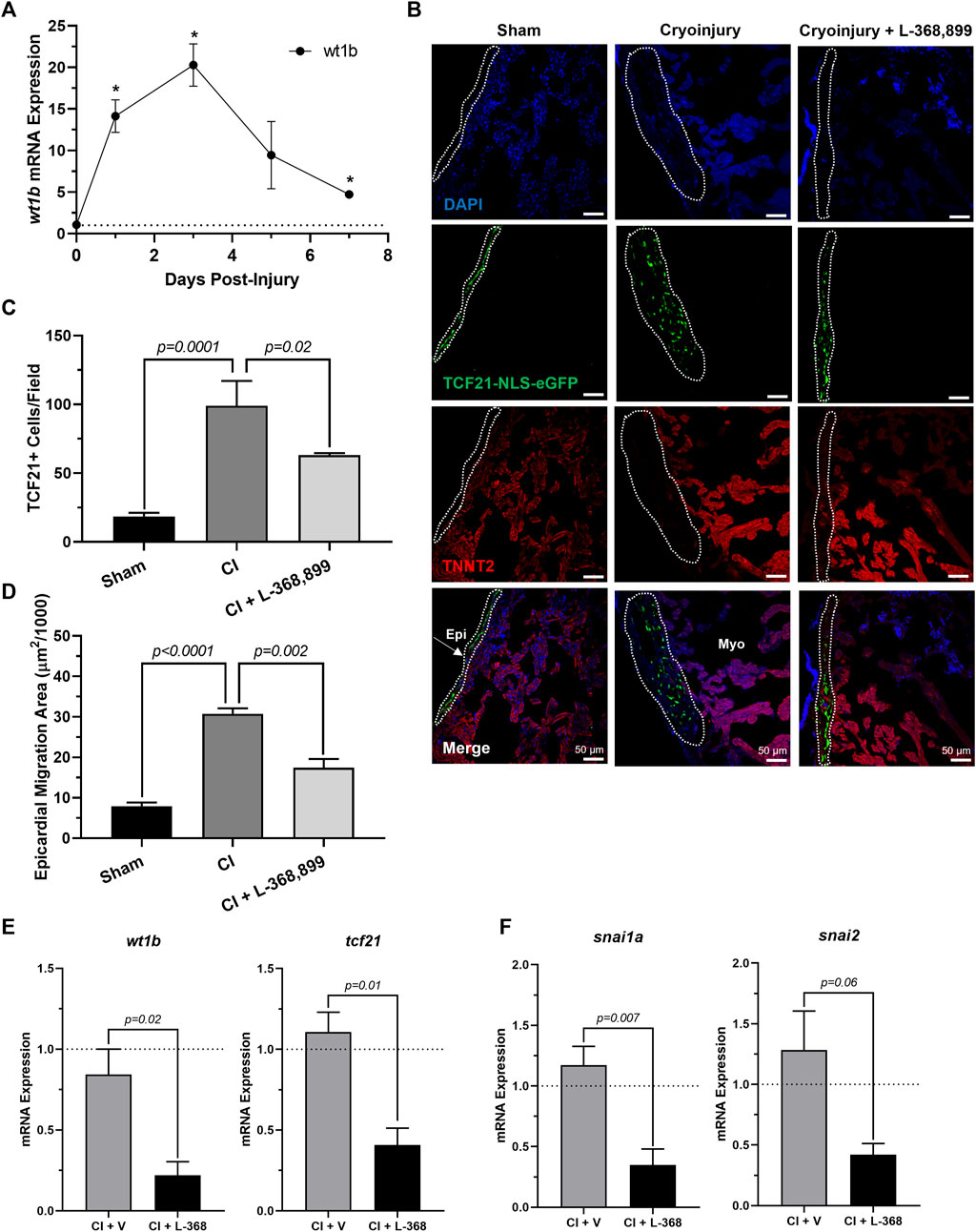
FIGURE 7. Inhibition of Oxytocin Signaling Prevents Epicardial Activation After Zebrafish Cardiac Injury. (A) Time course qRT-PCR data for wt1b in zebrafish hearts after cardiac cryoinjury; *p < 0.05 versus sham operated heart; n = 3 per time point. (B–D) Confocal immunofluorescent images (B) and quantification (C–D) of tcf21-nls-eGFP transgenic zebrafish at 3 dpi showing epicardial activation and expansion in sham, cryoinjured, and L-368,899-treated hearts. In (B), epicardial cell nuclei are labeled with GFP (green), cardiomyocytes are labeled with TNNT2 (red), nuclei are labeled with DAPI (blue); n = 3–5 images per condition, scale bar: 50 µm. (E–F) qRT-PCR data for wt1b and tcf21 (E) and snai1a and snai2 (F) in cryoinjured zebrafish hearts at 3 dpi treated with and without 1 µM L-368,899 (L-368); n = 3–5 per condition; CI: Cryoinjury, dpi: Days Post-Injury, Epi: Epicardium, Myo: Myocardium, V: Vehicle.
Discussion
Oxytocin is a hypothalamic neuroendocrine hormone best known for its functions in parturition, lactation, and social bonding. However, it also plays other less known physiological roles, including in the cardiovascular system, where it lowers blood pressure, induces negative inotropic and chronotropic effects, and serves as an anti-inflammatory and antioxidant (Gutkowska et al., 2014; Jankowski et al., 2020). OXT also has cardioprotective benefits in heart failure models (Garrott et al., 2017; Dyavanapalli et al., 2020) and after experimental myocardial infarction (Jankowski et al., 2010; Khori et al., 2021), however, it has not yet been linked to heart regeneration. Many of the above actions are carried out by OXT-mediated release of atrial natriuretic peptide (ANP) and nitric oxide (NO) (Jankowski et al., 2020), which have well-characterized cardioprotective effects (Jones & Bolli, 2006; Nishikimi et al., 2006) and may themselves contribute to cardiac regeneration (Kook et al., 2003; Rochon et al., 2020). Notably, OXT also induces differentiation of cardiomyocytes from embryonic stem cells (Paquin et al., 2002). Recent studies show that damage to neuroendocrine structures, such as the hypothalamus, strongly inhibits endogenous regenerative processes in vertebrates (Zhang et al., 2018), suggesting that critical pro-regenerative factors are released from this brain region. In addition, acute MI activates OXT-releasing neurons in the paraventricular nucleus of the rat hypothalamus (Roy et al., 2019). The above studies, combined with ample evidence to suggest that epicardial activation can be primed by specific signaling factors, such as thymosin β4 (Smart et al., 2007, 2011), suggest that oxytocin may be the critical factor in achieving sufficient EpiPC activation and differentiation to regenerate the lost myocardium after cardiac injury.
To date, there is no evidence of brain-derived hormones directly regulating regeneration after cardiac injury. However, studies show that both the sympathetic (White et al., 2015) and parasympathetic (Mahmoud et al., 2015) branches of the autonomic nervous system are important for proper heart regeneration in neonatal mice and zebrafish. It is reasonable to infer that the central nervous system may play a role in these processes as well, especially since several brain-induced peripheral hormones, such as estrogen (Xu et al., 2020), thyroid hormone (Hirose et al., 2019), and cortisol (Sallin & Jaźwińska, 2016), have been implicated in regulating heart regeneration. Here, we have taken these findings one step further and shown that OXT induces a pro-regenerative phenotype in vitro and in vivo by activating the epicardium. When hiPSC-derived epicardial cells were exposed to OXT, they increased their proliferation rates (Figures 2C–E) and adopted a more dedifferentiated gene expression profile (Figure 2G), two hallmarks of epicardial activation. Notably, oxytocin induced a greater proliferative response than thymosin β4 (Figure 2F) on hEpiCs, however it is possible that the state of our in vitro maturated epicardial cells is not identical to that of adult epicardial cells from hearts, the setting in which thymosin has shown more promising activity in the past We found that OXT acted through its receptor to exert its effects as the increases in hEpiC proliferation and activation that were seen in scrambled cells were completely abolished in OXTR knockdown epicardial cells (Figures 3D–F). Although OXT is also able to bind to AVP receptors with lower affinity (Gimpl & Fahrenholz, 2001), it does not appear as if this signaling pathway plays a role in our model, as hEpiCs do not express these receptors. Despite our findings, a more thorough characterization of the OXT mechanism of action is necessary to identify more specific pathways and effectors. Additionally, further characterization of the hEpiC maturation model is needed (e.g. other epithelial adhesion proteins, longer term studies), as this could be a useful tool for future studies into epicardial biology. To further demonstrate the importance of oxytocin signaling to heart regeneration, we extended our experiments to zebrafish, one of the most powerful naturally regenerating animal models known (Poss et al., 2002; Jopling et al., 2010; Beffagna, 2019). Several seminal studies of the last ∼20 years have shown that the epicardium plays a crucial role in zebrafish heart regeneration (Lepilina et al., 2006; Kikuchi et al., 2011b; Cao et al., 2016; Cao & Poss, 2018), therefore it is the perfect model system with which to expand our studies in vivo. We demonstrated that pharmacological inhibition of OXT signaling significantly slowed cardiac regeneration after cryoinjury, as seen by increased fibrosis accumulation and myocardial injury area when zebrafish were treated with L-368,899 (Figures 6C–E). In addition, this compound decreased activation and proliferation of CMs as well as revascularization of the wound (Figures 6F–I). OXTR inhibition also prevented the proliferative response of epicardial progenitor cells that is normally seen after cryoinjury (Figures 7B,C) and decreased the cardiac expression of several genes involved in EpiPC activation and EMT (Figures 7E,F). It is certainly possible that the anti-proliferative effects L-368,899 treatment has on the epicardium ultimately cause the effects in the other cell types, possibly through indirect pathways related to epicardial secretion of pro-regenerative molecules (Lavine et al., 2005; Li et al., 2011). However, as described above, oxytocin plays numerous roles in the heart, and global inhibition of its receptor would likely result in several cardiac phenotypes. To truly demonstrate the extent that the anti-regenerative effects seen are due to epicardial cell activity, an epicardium-specific oxtr knockout would be required. To that end, future studies will utilize more advanced genetic zebrafish models to further explore the interaction of EpiPCs with CMs, endothelial cells, and other cardiac cells, such as cardiac fibroblasts and immune cells, during heart regeneration.
Our hypothesis is that oxytocin is released from the brain into the bloodstream after cardiac injury to facilitate epicardial activation and heart regeneration. We showed that oxt mRNA levels increased ∼20-fold in zebrafish brains 3 days after cardiac cryoinjury (Figure 6B), with a corresponding increase seen in wt1b expression in the injured hearts (Figure 7A). These results suggest that this newly synthesized oxytocin travels to the heart to induce epicardial activation, a phenomenon that has not been described previously. While these qRT-PCR data are encouraging correlational findings, it is possible that this increase in epicardial activation could be due partly to local effects, as the heart is able to produce some OXT on its own, albeit at several-fold lower levels than the hypothalamus (Jankowski et al., 1998; Gutkowska et al., 2000). Therefore, subsequent regeneration may be driven by oxytocin derived not only from the brain but from the heart as well. Indeed, heart regeneration is a coordinated effort that involves communication between multiple organs (Filosa & Sawamiphak, 2021), and our studies strongly indicate that the brain plays a central role in this network.
In conclusion, we show here that oxytocin is an important activator of EpiPCs and is necessary for heart regeneration and proper epicardium development. We demonstrate that OXT induces reprogramming of human epicardial cells into an epicardial progenitor state by activating the OXTR. These effects are mediated through the TGF-β pathway and its associated downstream effectors. Overall, our results establish a previously uncharacterized link between OXT release after cardiac injury and heart regeneration and provide evidence to support the notion that cardiac regeneration is, at least partially, under neuroendocrine control. These findings offer exciting translational potential, as oxytocin or one of its agonists could be used in the clinic to aid in the recovery from severe cardiac events, such as an MI, and prevent progression to heart failure in the future.
Data availability statement
The datasets presented in this study can be found in online repositories. The names of the repository/repositories and accession number(s) can be found below: https://www.ncbi.nlm.nih.gov/geo/, GSE199427.
Ethics statement
The animal study was reviewed and approved by MSU IACUC under protocol number PROTO202100340.
Author contributions
AW and AA designed all experiments and conceptualized the work. AW performed all experiments, acquired images, and analyzed data. AH performed cell culture, zebrafish husbandry, and experiments. YL-I acquired in vitro data and confocal images. MD and AM acquired and analyzed molecular biology data. MV performed zebrafish experiments. AW prepared all figures, and AW and AA wrote the manuscript. AA supervised all the work.
Funding
Work in the Aguirre laboratory is supported by the National Heart, Lung, and Blood Institute of the National Institutes of Health under award numbers K01HL135464 and R01HL151505, by the American Heart Association under award number 19IPLOI34660342, and by the Spectrum-MSU Foundation.
Acknowledgments
We wish to thank the MSU Genomics Core for RNA sequencing support and Dr. William Jackson at the MSU Department of Pharmacology/Toxicology for access to confocal microscopes. We also want to thank all members of the Aguirre lab for helpful comments and discussions.
Conflict of interest
The authors declare that the research was conducted in the absence of any commercial or financial relationships that could be construed as a potential conflict of interest.
Publisher’s note
All claims expressed in this article are solely those of the authors and do not necessarily represent those of their affiliated organizations, or those of the publisher, the editors and the reviewers. Any product that may be evaluated in this article, or claim that may be made by its manufacturer, is not guaranteed or endorsed by the publisher.
Supplementary material
The Supplementary Material for this article can be found online at: https://www.frontiersin.org/articles/10.3389/fcell.2022.985298/full#supplementary-material
References
Aguirre, A., Montserrat, N., Zacchigna, S., Nivet, E., Hishida, T., Krause, M. N., et al. (2014). In vivo activation of a conserved microRNA program induces mammalian heart regeneration. Cell Stem Cell 15 (5), 589–604. doi:10.1016/j.stem.2014.10.003
Bao, X., Lian, X., Hacker, T. A., Schmuck, E. G., Qian, T., Bhute, V. J., et al. (2016). Long-term self-renewing human epicardial cells generated from pluripotent stem cells under defined xeno-free conditions. Nat. Biomed. Eng. 1, 0003–0012. doi:10.1038/s41551-016-0003
Bao, X., Lian, X., Qian, T., Bhute, V. J., Han, T., and Palecek, S. P. (2017). Directed differentiation and long-term maintenance of epicardial cells derived from human pluripotent stem cells under fully defined conditions. Nat. Protoc. 12 (9), 1890–1900. doi:10.1038/nprot.2017.080
Beffagna, G. (2019). Zebrafish as a Smart model to understand regeneration after heart injury: How fish could help humans. Front. Cardiovasc. Med. 6, 107–108. doi:10.3389/fcvm.2019.00107
Bergmann, O., Bhardwaj, R. D., Bernard, S., Zdunek, S., Barnabé-Heider, F., Walsh, S., et al. (2009). Evidence for cardiomyocyte renewal in humans. Science 324 (5923), 98–102. doi:10.1126/science.1164680
Bise, T., and Jaźwińska, A. (2019). Intrathoracic injection for the study of adult zebrafish heart. J. Vis. Exp. 147, 1–10. doi:10.3791/59724
Cai, C.-L., Martin, J. C., Sun, Y., Cui, L., Wang, L., Ouyang, J., et al. (2008). A myocardial lineage derives from Tbx18 epicardial cells. Nature 454 (7200), 104–108. doi:10.1038/nature06969
Cao, J., Navis, A., Cox, B. D., Dickson, A. L., Gemberling, M., Karra, R., et al. (2016). Single epicardial cell transcriptome sequencing identifies caveolin 1 as an essential factor in zebrafish heart regeneration. Development 143, 232–243. doi:10.1242/dev.130534
Cao, J., and Poss, K. D. (2018). The epicardium as a hub for heart regeneration. Nat. Rev. Cardiol. 15 (10), 631–647. doi:10.1038/s41569-018-0046-4
Christoffels, V. M., Grieskamp, T., Norden, J., Mommersteeg, M. T. M., Rudat, C., and Kispert, A. (2009). Tbx18 and the fate of epicardial progenitors. Nature 458 (7240), E8–E9. doi:10.1038/nature07916
Dampney, R. A. L. (2016). Central neural control of the cardiovascular system: Current perspectives. Adv. Physiol. Educ. 40 (3), 283–296. doi:10.1152/advan.00027.2016
Dyavanapalli, J., Rodriguez, J., Rocha dos Santos, C., Escobar, J. B., Dwyer, M. K., Schloen, J., et al. (2020). Activation of oxytocin neurons improves cardiac function in a pressure-overload model of heart failure. JACC. Basic Transl. Sci. 5 (5), 484–497. doi:10.1016/j.jacbts.2020.03.007
Eroglu, E., Yen, C. Y. T., Tsoi, Y. L., Witman, N., Elewa, A., Joven Araus, A., et al. (2022). Epicardium-derived cells organize through tight junctions to replenish cardiac muscle in salamanders. Nat. Cell Biol. 24 (5), 645–658. doi:10.1038/s41556-022-00902-2
Fan, D., Takawale, A., Lee, J., and Kassiri, Z. (2012). Cardiac fibroblasts, fibrosis and extracellular matrix remodeling in heart disease. Fibrogenes. Tissue Repair 5, 15–13. doi:10.1186/1755-1536-5-15
Filosa, A., and Sawamiphak, S. (2021). Heart development and regeneration-a multi-organ effort. FEBS J. 1–18. doi:10.1111/febs.16319
Frantz, M. C., Pellissier, L. P., Pflimlin, E., Loison, S., Gandiá, J., Marsol, C., et al. (2018). LIT-001, the first nonpeptide oxytocin receptor agonist that improves social interaction in a mouse model of autism. J. Med. Chem. 61 (19), 8670–8692. doi:10.1021/acs.jmedchem.8b00697
Garrott, K., Dyavanapalli, J., Cauley, E., Dwyer, M. K., Kuzmiak-Glancy, S., Wang, X., et al. (2017). Chronic activation of hypothalamic oxytocin neurons improves cardiac function during left ventricular hypertrophy-induced heart failure. Cardiovasc. Res. 113 (11), 1318–1328. doi:10.1093/cvr/cvx084
Gimpl, G., and Fahrenholz, F. (2001). The oxytocin receptor system: Structure, function, and regulation. Physiol. Rev. 81 (2), 629–683. doi:10.1152/physrev.2001.81.2.629
González-Rosa, J. M., Burns, C. E., and Burns, C. G. (2017). Zebrafish heart regeneration: 15 years of discoveries. Regeneration 4 (3), 105–123. doi:10.1002/reg2.83
González-Rosa, J. M., Martín, V., Peralta, M., Torres, M., and Mercader, N. (2011). Extensive scar formation and regression during heart regeneration after cryoinjury in zebrafish. Development 138 (9), 1663–1674. doi:10.1242/dev.060897
González-Rosa, J. M., and Mercader, N. (2012). Cryoinjury as a myocardial infarction model for the study of cardiac regeneration in the zebrafish. Nat. Protoc. 7 (4), 782–788. doi:10.1038/nprot.2012.025
Gordan, R., Gwathmey, J. K., and Xie, L.-H. (2015). Autonomic and endocrine control of cardiovascular function. World J. Cardiol. 7 (4), 204–214. doi:10.4330/wjc.v7.i4.204
Gutkowska, J., Jankowski, M., and Antunes-Rodrigues, J. (2014). The role of oxytocin in cardiovascular regulation. Braz. J. Med. Biol. Res. 47 (3), 206–214. doi:10.1590/1414-431X20133309
Gutkowska, J., Jankowski, M., Mukaddam-Daher, S., and McCann, S. M. (2000). Oxytocin is a cardiovascular hormone. Braz. J. Med. Biol. Res. 33 (6), 625–633. doi:10.1590/S0100-879X2000000600003
Guyenet, P. G., and Bayliss, D. A. (2015). Neural control of breathing and CO2 homeostasis. Neuron 87 (5), 946–961. doi:10.1016/j.neuron.2015.08.001
Hilfiger, L., Zhao, Q., Kerspern, D., Inquimbert, P., Andry, V., Goumon, Y., et al. (2020). A nonpeptide oxytocin receptor agonist for a durable relief of inflammatory pain. Sci. Rep. 10 (1), 3017–3110. doi:10.1038/s41598-020-59929-w
Hirose, K., Payumo, A. Y., Cutie, S., Hoang, A., Zhang, H., Guyot, R., et al. (2019). Evidence for hormonal control of heart regenerative capacity during endothermy acquisition. Science 364 (6436), 184–188. doi:10.1126/science.aar2038
Jankowski, M., Bissonauth, V., Gao, L., Gangal, M., Wang, D., Danalache, B., et al. (2010). Anti-inflammatory effect of oxytocin in rat myocardial infarction. Basic Res. Cardiol. 105 (2), 205–218. doi:10.1007/s00395-009-0076-5
Jankowski, M., Broderick, T. L., and Gutkowska, J. (2020). The role of oxytocin in cardiovascular protection. Front. Psychol. 11, 2139–2217. doi:10.3389/fpsyg.2020.02139
Jankowski, M., Hajjar, F., Al Kawas, S., Mukaddam-Daher, S., Hoffman, G., Mccann, S. M., et al. (1998). Rat heart: A site of oxytocin production and action. Proc. Natl. Acad. Sci. U. S. A. 95 (24), 14558–14563. doi:10.1073/pnas.95.24.14558
Jones, S. P., and Bolli, R. (2006). The ubiquitous role of nitric oxide in cardioprotection. J. Mol. Cell. Cardiol. 40 (1), 16–23. doi:10.1016/j.yjmcc.2005.09.011
Jopling, C., Sleep, E., Raya, M., Marti, M., Raya, A., and Izpisua Belmonte, J. C. (2010). Zebrafish heart regeneration occurs by cardiomyocyte dedifferentiation and proliferation. Nature 176 (12), 606–609. doi:10.1038/nature08899
Khori, V., Mohammad Zadeh, F., Tavakoli-Far, B., Alizadeh, A. M., Khalighfard, S., Ghandian Zanjan, M., et al. (2021). Role of oxytocin and c-Myc pathway in cardiac remodeling in neonatal rats undergoing cardiac apical resection. Eur. J. Pharmacol. 908, 174348–174357. doi:10.1016/j.ejphar.2021.174348
Kikuchi, K., Gupta, V., Wang, J., Holdway, J. E., Wills, A. A., Fang, Y., et al. (2011a). Tcf21+ epicardial cells adopt non-myocardial fates during zebrafish heart development and regeneration. Development 138 (14), 2895–2902. doi:10.1242/dev.067041
Kikuchi, K., Holdway, J. E., Major, R. J., Blum, N., Dahn, R. D., Begemann, G., et al. (2011b). Retinoic acid production by endocardium and epicardium is an injury response essential for zebrafish heart regeneration. Dev. Cell 20 (3), 397–404. doi:10.1016/j.devcel.2011.01.010
Kikuchi, K., Holdway, J. E., Werdich, A. A., Anderson, R. M., Fang, Y., Egnaczyk, G. F., et al. (2010). Primary contribution to zebrafish heart regeneration by gata4+ cardiomyocytes. Nature 464 (7288), 601–605. doi:10.1038/nature08804
Kook, H., Itoh, H., Choi, B. S., Sawada, N., Doi, K., Hwang, T. J., et al. (2003). Physiological concentration of atrial natriuretic peptide induces endothelial regeneration in vitro. Am. J. Physiol. Heart Circ. Physiol. 284 (4), 1388–1397. doi:10.1152/ajpheart.00414.2002
Laflamme, M. A., and Murry, C. E. (2011). Heart regeneration. Nature 473 (7347), 326–335. doi:10.1038/nature10147
Lavine, K. J., Yu, K., White, A. C., Zhang, X., Smith, C., Partanen, J., et al. (2005). Endocardial and epicardial derived FGF signals regulate myocardial proliferation and differentiation in vivo. Dev. Cell 8 (1), 85–95. doi:10.1016/j.devcel.2004.12.002
Lawson, N. D., and Weinstein, B. M. (2002). In vivo imaging of embryonic vascular development using transgenic zebrafish. Dev. Biol. 248 (2), 307–318. doi:10.1006/dbio.2002.0711
Lepilina, A., Coon, A. N., Kikuchi, K., Holdway, J. E., Roberts, R. W., Burns, C. G., et al. (2006). A dynamic epicardial injury response supports progenitor cell activity during zebrafish heart regeneration. Cell 127 (3), 607–619. doi:10.1016/j.cell.2006.08.052
Li, P., Cavallero, S., Gu, Y., Chen, T. H. P., Hughes, J., Hassan, A. B., et al. (2011). IGF signaling directs ventricular cardiomyocyte proliferation during embryonic heart development. Development 138 (9), 1795–1805. doi:10.1242/dev.054338
Mahmoud, A. I., O’Meara, C. C., Gemberling, M., Zhao, L., Bryant, D. M., Zheng, R., et al. (2015). Nerves regulate cardiomyocyte proliferation and heart regeneration. Dev. Cell 34 (4), 387–399. doi:10.1016/j.devcel.2015.06.017
Martínez-Estrada, O. M., Lettice, L. A., Essafi, A., Guadix, J. A., Slight, J., Velecela, V., et al. (2010). Wt1 is required for cardiovascular progenitor cell formation through transcriptional control of Snail and E-cadherin. Nat. Genet. 42 (1), 89–93. doi:10.1038/ng.494
Morrison, S. F. (2016). Central control of body temperature. F1000Res. 5, 880. doi:10.12688/F1000RESEARCH.7958.1
Nishikimi, T., Maeda, N., and Matsuoka, H. (2006). The role of natriuretic peptides in cardioprotection. Cardiovasc. Res. 69 (2), 318–328. doi:10.1016/j.cardiores.2005.10.001
Palma, J.-A., and Benarroch, E. E. (2014). Neural control of the heart: Recent concepts and clinical correlations. Neurology 83 (3), 261–271. doi:10.1212/WNL.0000000000000605
Paquin, J., Danalache, B. A., Jankowski, M., McCann, S. M., and Gutkowska, J. (2002). Oxytocin induces differentiation of P19 embryonic stem cells to cardiomyocytes. Proc. Natl. Acad. Sci. U. S. A. 99 (14), 9550–9555. doi:10.1073/pnas.152302499
Peralta, M., González-Rosa, J. M., Marques, I. J., and Mercader, N. (2014). The epicardium in the embryonic and adult zebrafish. J. Dev. Biol. 2 (2), 101–116. doi:10.3390/jdb2020101
Poss, K. D., Wilson, L. G., and Keating, M. T. (2002). Heart regeneration in zebrafish. Science 298 (5601), 2188–2190. doi:10.1126/science.1077857
Quijada, P., Trembley, M. A., and Small, E. M. (2020). The role of the epicardium during heart development and repair. Circ. Res. 126, 377–394. doi:10.1161/CIRCRESAHA.119.315857
Rao, K. S., and Spees, J. L. (2017). Harnessing epicardial progenitor cells and their derivatives for rescue and repair of cardiac tissue after myocardial infarction. Curr. Mol. Biol. Rep. 3 (3), 149–158. doi:10.1007/s40610-017-0066-6
Rhee, S. S., and Pearce, E. N. (2011). Update: Systemic diseases and the cardiovascular system (II). The endocrine system and the heart: A review. Rev. Esp. Cardiol. 64 (3), 220–231. doi:10.1016/j.recesp.2010.10.015
Riley, P. R. (2012). An epicardial floor plan for building and rebuilding the mammalian heart. Curr. Top. Dev. Biol. 100, 233–251. doi:10.1016/B978-0-12-387786-4.00007-5
Rochon, E. R., Missinato, M. A., Xue, J., Tejero, J., Tsang, M., Gladwin, M. T., et al. (2020). Nitrite improves heart regeneration in zebrafish. Antioxid. Redox Signal. 32 (6), 363–377. doi:10.1089/ars.2018.7687
Roh, E., Song, D. K., and Kim, M. S. (2016). Emerging role of the brain in the homeostatic regulation of energy and glucose metabolism. Exp. Mol. Med. 48 (3), e216–12. doi:10.1038/emm.2016.4
Roy, R. K., Augustine, R. A., Brown, C. H., and Schwenke, D. O. (2019). Acute myocardial infarction activates magnocellular vasopressin and oxytocin neurones. J. Neuroendocrinol. 31 (12), e12808–10. doi:10.1111/jne.12808
Sallin, P., and Jaźwińska, A. (2016). Acute stress is detrimental to heart regeneration in zebrafish. Open Biol. 6, 160012–160015. doi:10.1098/rsob.160012
Sanz-Morejón, A., and Mercader, N. (2020). Recent insights into zebrafish cardiac regeneration. Curr. Opin. Genet. Dev. 64, 37–43. doi:10.1016/j.gde.2020.05.020
Simões, F. C., and Riley, P. R. (2018). The ontogeny, activation and function of the epicardium during heart development and regeneration. Development 145 (7), dev155994–13. doi:10.1242/dev.155994
Smart, N., Bollini, S., Dubé, K. N., Vieira, J. M., Zhou, B., Davidson, S., et al. (2011). De novo cardiomyocytes from within the activated adult heart after injury. Nature 474 (7353), 640–644. doi:10.1038/nature10188
Smart, N., Risebro, C. A., Melville, A. A. D., Moses, K., Schwartz, R. J., Chien, K. R., et al. (2007). Thymosin beta4 induces adult epicardial progenitor mobilization and neovascularization. Nature 445 (7124), 177–182. doi:10.1038/nature05383
Smits, A. M., Dronkers, E., and Goumans, M. J. (2018). The epicardium as a source of multipotent adult cardiac progenitor cells: Their origin, role and fate. Pharmacol. Res. 127, 129–140. doi:10.1016/j.phrs.2017.07.020
Streef, T. J., and Smits, A. M. (2021). Epicardial contribution to the developing and injured heart: Exploring the cellular composition of the epicardium. Front. Cardiovasc. Med. 8, 750243–750316. doi:10.3389/fcvm.2021.750243
Uygur, A., and Lee, R. T. (2016). Mechanisms of cardiac regeneration. Dev. Cell 36 (4), 362–374. doi:10.1016/j.devcel.2016.01.018
Van Wijk, B., Gunst, Q. D., Moorman, A. F. M., and van den Hoff, M. J. B. (2012). Cardiac regeneration from activated epicardium. PLoS ONE 7 (9), e44692–14. doi:10.1371/journal.pone.0044692
Virani, S. S., Alonso, A., Benjamin, E. J., Bittencourt, M. S., Callaway, C. W., Carson, A. P., et al. (2020). Heart disease and stroke statistics—2020 update: A report from the American heart association. Circulation 141, E139–E596. doi:10.1161/CIR.0000000000000757
Wang, J., Panáková, D., Kikuchi, K., Holdway, J. E., Gemberling, M., Burris, J. S., et al. (2011). The regenerative capacity of zebrafish reverses cardiac failure caused by genetic cardiomyocyte depletion. Development 138 (16), 3421–3430. doi:10.1242/dev.068601
Wang, Y. L., Yu, S. N., Shen, H. R., Wang, H. J., Wu, X. P., Wang, Q. L., et al. (2021). Thymosin β4 released from functionalized self-assembling peptide activates epicardium and enhances repair of infarcted myocardium. Theranostics 11 (9), 4262–4280. doi:10.7150/THNO.52309
Wei, K., Serpooshan, V., Hurtado, C., Diez-Cunado, M., Zhao, M., Maruyama, S., et al. (2015). Epicardial FSTL1 reconstitution regenerates the adult mammalian heart. Nature 525 (7570), 479–485. doi:10.1038/nature15372
White, I. A., Gordon, J., Balkan, W., and Hare, J. M. (2015). Sympathetic reinnervation is required for mammalian cardiac regeneration. Circ. Res. 117 (12), 990–994. doi:10.1161/CIRCRESAHA.115.307465
Xu, S., Xie, F., Tian, L., Fallah, S., Babaei, F., Manno, S. H. C., et al. (2020). Estrogen accelerates heart regeneration by promoting the inflammatory response in zebrafish. J. Endocrinol. 245 (1), 39–51. doi:10.1530/JOE-19-0413
Zhang, M., Chen, Y., Xu, H., Yang, L., Yuan, F., Li, L., et al. (2018). Melanocortin receptor 4 signaling regulates vertebrate limb regeneration. Dev. Cell 46 (4), 397–409. doi:10.1016/j.devcel.2018.07.021
Zhou, B., Honor, L. B., He, H., Qing, M., Oh, J. H., Butterfield, C., et al. (2011). Adult mouse epicardium modulates myocardial injury by secreting paracrine factors. J. Clin. Invest. 121 (5), 1894–1904. doi:10.1172/JCI45529
Zhou, B., Honor, L. B., Ma, Q., Oh, J. H., Lin, R. Z., Melero-Martin, J. M., et al. (2012). Thymosin beta 4 treatment after myocardial infarction does not reprogram epicardial cells into cardiomyocytes. J. Mol. Cell. Cardiol. 52, 43–47. doi:10.1016/j.yjmcc.2011.08.020
Keywords: epicardial progenitor cells, oxytocin, cardiac regeneration, induced pluripotent stem cells, epicardium, cardiac development, zebrafish
Citation: Wasserman AH, Huang AR, Lewis-Israeli YR, Dooley MD, Mitchell AL, Venkatesan M and Aguirre A (2022) Oxytocin promotes epicardial cell activation and heart regeneration after cardiac injury. Front. Cell Dev. Biol. 10:985298. doi: 10.3389/fcell.2022.985298
Received: 03 July 2022; Accepted: 31 August 2022;
Published: 30 September 2022.
Edited by:
Alysson Renato Muotri, University of California, San Diego, United StatesReviewed by:
Fabian Zanella, Sana Biotechnology, Inc, United StatesKonstantinos Chatzistergos, Aristotle University of Thessaloniki, Greece
Sam Senyo, Case Western Reserve University, United States
Copyright © 2022 Wasserman, Huang, Lewis-Israeli, Dooley, Mitchell, Venkatesan and Aguirre. This is an open-access article distributed under the terms of the Creative Commons Attribution License (CC BY). The use, distribution or reproduction in other forums is permitted, provided the original author(s) and the copyright owner(s) are credited and that the original publication in this journal is cited, in accordance with accepted academic practice. No use, distribution or reproduction is permitted which does not comply with these terms.
*Correspondence: Aitor Aguirre, aaguirre@msu.edu