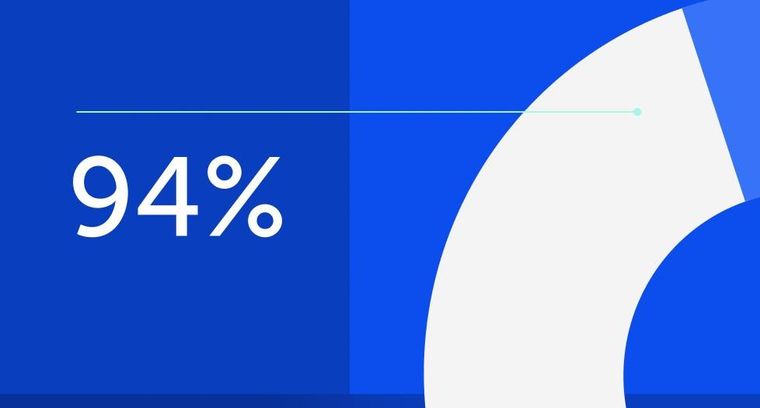
94% of researchers rate our articles as excellent or good
Learn more about the work of our research integrity team to safeguard the quality of each article we publish.
Find out more
MINI REVIEW article
Front. Cell Dev. Biol., 06 September 2022
Sec. Stem Cell Research
Volume 10 - 2022 | https://doi.org/10.3389/fcell.2022.982199
This article is part of the Research TopicChondrogenic Potentials, Protocols and Mechanisms of Mesenchymal Progenitor CellsView all 5 articles
There is an unmet need for novel and efficacious therapeutics for regenerating injured articular cartilage in progressive osteoarthritis (OA) and/or trauma. Mesenchymal stem cells (MSCs) are particularly promising for their chondrogenic differentiation, local healing environment modulation, and tissue- and organism-specific activity; however, despite early in vivo success, MSCs require further investigation in highly-translatable models prior to disseminated clinical usage. Large animal models, such as canine, porcine, ruminant, and equine models, are particularly valuable for studying allogenic and xenogenic human MSCs in a human-like osteochondral microenvironment, and thus play a critical role in identifying promising approaches for subsequent clinical investigation. In this mini-review, we focus on [1] considerations for MSC-harnessing studies in each large animal model, [2] source tissues and organisms of MSCs for large animal studies, and [3] tissue engineering strategies for optimizing MSC-based cartilage regeneration in large animal models, with a focus on research published within the last 5 years. We also highlight the dearth of standard assessments and protocols regarding several crucial aspects of MSC-harnessing cartilage regeneration in large animal models, and call for further research to maximize the translatability of future MSC findings.
Articular cartilage injury secondary to trauma and aging affects over 300 million people (Safiri et al., 2020) and poses significant health burdens at the individual and socioeconomic level (Hunter et al., 2014; Katz et al., 2021). Injury ranges from acute defects, which can accelerate osteoarthritis (OA) if untreated, to diffuse cartilage loss in end-stage disease (Chen et al., 2017). Moreover, cartilage possesses particularly poor intrinsic healing capacity due to it’s avascularity, complex matrix architecture, and limited chondrocyte replication (Grassel and Aszodi 2019).
There exists an unmet need for novel and efficacious treatments for articular cartilage injury. Osteochondral grafting (OCG) and autologous chondrocyte implantation (ACI) restore the articular surface in focal defects, but suffer from donor site morbidity, limited tissue availability, and poor efficacy for diffuse cartilage loss (Zhang et al., 2021). Likewise, microfracture insufficiently recruits host growth factors and stem cells, promoting fibrocartilage formation with poor biomechanics (Mustapich et al., 2020). Total joint arthroplasty is indicated for end-stage OA in large joints, which alleviates pain but introduces potential complications including implant infection, postoperative stiffness and pain, and need for revision surgery (Heo et al., 2020). Therefore, no existing treatments effectively reconstitute the osteochondral unit.
Among emerging therapies, mesenchymal stem cells (MSCs) demonstrate promising potential for in vivo cartilage regeneration (Zhang et al., 2019). However, clinical translation has been limited despite multiple ongoing clinical trials for intra-articular MSC injection (Hernigou et al., 2021; Lamo-Espinosa et al., 2021). Arguably, the disconnected and inconsistent results between preclinical small and large animal studies may contribute substantially to the limited translation in current practice. Therefore, in this mini-review we discuss [1] highly clinically-relevant large animal models of cartilage regeneration, [2] source tissues and organisms for MSCs in large animal studies, and [3] tissue engineering strategies for optimizing MSC-based cartilage regeneration in large animals, focusing on research published within the last 5 years. We extracted relevant literature from Pubmed using the terms “mesenchymal stem cell,” “articular cartilage regeneration,” and either “pig,” “sheep,” “goat,” “dog,” or “horse.”
Unlike the small joint size, thin articular cartilage, and robust cartilaginous defect regeneration of small animals (i.e., rodents, rabbits) (Libbin and Rivera 1989; Moran et al., 2016), large animal models are particularly useful for studying and identifying proposed treatments for further clinical investigation. Focal defects model isolated injury and regeneration, involve the full-thickness chondral layer or entire osteochondral unit on load- or non-load-bearing surfaces, and unlike in clinical disease, possess well-defined margins and cover limited areas (Cook et al., 2014). Alternatively, diffuse cartilage degeneration akin to OA is induced via articular surface surgical manipulation/injury (Zhang B. Y. et al., 2018), anterior cruciate ligament (ACL) and/or medial meniscus resection followed by weight-bearing (Ude et al., 2014), and chemical treatment (Uilenreef et al., 2019). Spontaneous OA is also studied in non-human primates (McCoy 2015) and long-lived companion animals including canines and equines, which can develop OA in their natural life course (Sasaki et al., 2019) (Table 1). Selectively-bred genetic OA is typically limited to small animal models (Chen et al., 2017).
TABLE 1. Advantages, disadvantages, and logistics of large animal models for articular cartilage injury and regeneration with MSC treatment, with parameters of relevant studies published within the last 5 years.
Domestic canines are unique, relatively long-lived models that suffer from similar spontaneous OA and poor cartilage healing as humans. Canines also handle postoperative exercise and loading regimens particularly well (Chu et al., 2010). Different breeds exhibit varying biomechanics, load patterns, and skeletal maturity ages based on size, which may complicate translation. Uniquely, the canine’s role as a family pet also presents ethical issues and limits the extent of post-treatment analysis, although arthroscopies can still rapidly enable articular surface evaluation (Chu et al., 2010).
Anatomically, canine cartilage is thinner than in humans and only relatively small defects, most commonly 4 mm, are created which limits comparability (Ahern et al., 2009). Like most quadrupeds, the canine knee joint also exhibits greater flexion and decreased extension than in humans, and possesses a quadrupedal-specific long digital extensor tendon which supplements joint stability (Proffen et al., 2012).
Allogenic and xenogenic human MSCs yield promising cartilage regeneration results in canines. Intra-articular injection of human umbilical cord matrix-derived MSCs (umMSCs) increased regenerated cartilage thickness and improved articular surface appearance on magnetic resonance imaging (MRI) (Zhang B. Y. et al., 2018). Adipose tissue-derived MSCs (aMSCs) and umMSCs injections also trended towards suppressed blood/synovial inflammatory markers, including interleukin-6 and tumor necrosis factor-alpha (Botto et al., 2022). Moreover, intra-articular knee injection of 10 million allogenic bone marrow-derived MSCs (bMSCs) and hyaluronic acid (HA) encouraged cartilaginous tissue formation in chondral defects on gross and histologic analysis, compared to HA or saline alone (Li et al., 2018).
Miniature porcine breeds are among the most commonly-studied large animals, due to their similar joint size, loading mechanics, weight, intrinsically-poor cartilage regenerative ability, collagen fiber arrangement, bone apposition rate, and trabecular thickness as humans (Proffen et al., 2012; Takroni et al., 2016). Minipigs are also commonly used to evaluate inflammation and toxicity of implanted osteochondral biomaterials (Cone et al., 2017). At 1–2 mm thick, minipig cartilage is thinner than in humans; nevertheless, larger 6–8 mm diameter osteochondral defects can be created (Chu et al., 2010). Disadvantageously, minipigs cannot participate in many exercise or weight-bearing regimens. Like other large animals, only skeletally-mature minipigs exhibit diminished intrinsic cartilaginous repair, which extends maintenance duration and overall cost.
Numerous studies support that autologous, allogenic, and xenogenic human MSC administration bolsters osteochondral reconstitution in pigs. Porcine aMSCs seeded onto decellularized cartilage extracellular matrix (DCECM) performed similarly to chondrocytes regarding histological chondral defect regeneration (Lu L. et al., 2021). Scaffold-free implantation of porcine bMSCs and aMSCs at 5–30 million cells per defect, respectively, also improved cartilage histological and MRI score compared to nontreatment (Yamasaki et al., 2019; Theruvath et al., 2021). Likewise, autologous porcine synovial tissue-derived MSCs (sMSCs) aggregates, at 400,000 cells/defect, bolstered histology and macroscopic scores in femoral condyle after 12 weeks (Kondo et al., 2019).
Regarding xenogenic studies, human bMSCs seeded and chondrogenically-induced on collagen scaffold encouraged cartilaginous tissue formation 5 months after bone plug co-implantation into osteoarthritic pigs (Tseng et al., 2018). Moreover, 5 million human umMSCs suspended in HA hydrogel enhanced gross and histological cartilage scoring compared with nontreatment, in porcine full-thickness trochlear defects (Wu et al., 2019). Such studies suggest comparable therapeutic efficacy of human-derived and porcine MSCs in minipigs.
Ruminants are popular and accessible models for osteochondral defect studies, as they exhibit poor spontaneous regeneration and are cheaper and easier to handle than other large animals. Cyclic loading, biomechanics, and contact pressure during ruminant gait is comparable to that of humans (Moran et al., 2016). Biomechanically, goat knees experience higher peak pressures than in human tissues, which contributes to comparatively poor cartilage repair (Patil et al., 2014). Caprine defects up to 6 mm are reported, although cartilage thickness varies significantly across breed size/sex with upper limits of 1.5–2.0 mm. Ovine models possess a cartilage thickness of 0.4–1.7 mm, with defects up to 7 mm reported (Cook et al., 2014).
Numerous studies report that allogenic/xenogeneic MSCs promote osteochondral regeneration in ruminants. Allogenic bMSCs or aMSCs seeded onto polycaprolactone, collagen, alginate, and/or tantalum scaffolds improve cartilaginous tissue deposition and histologic scores compared to untreated defects in sheep (Vahedi et al., 2019; Favreau et al., 2020) and goats (Wei et al., 2019), with comparable histological scoring to autograft reported (Keller et al., 2019). Injection of aMSCs in sheep similarly suppressed synovial fluid inflammatory factors and bolstered histological, macroscopic, and MRI scores (Feng et al., 2018; Lv et al., 2018; Veronesi et al., 2022).
Regarding human-derived MSC studies, 1 million umMSCs seeded on DCECM scaffold yielded improved tissue elasticity modulus, collagen II content, and MRI evaluation in goat defects compared to microfracture (Zhang Y. et al., 2018). Intra-articular injection of 25 million human umbilical cord blood-derived MSCs (ubMSCs) and DCECM in OA-induced goats also improved gross articular appearance and radiographic scoring after 6 months (Kim et al., 2022). Additionally, 10 million total human umMSCs and goat chondrocytes in DCECM scaffold encouraged cartilaginous tissue formation with superior glycosaminoglycan content, Young’s modulus, and surrounding tissue integration in caprine chondral defects after 9 months, compared to scaffold alone or nontreatment (Zhang et al., 2020). Importantly, umMSCs persisted in regenerated tissue after 9 months, suggesting sustained chondrogenic differentiation and minimal immunogenicity.
Notably, ruminants rarely develop spontaneous OA and require meniscal removal or disruption—ACL transection alone insufficiently induces significant OA, unlike in other large animals (McCoy 2015). Exercise and weight-bearing protocols are also difficult to implement (Chu et al., 2010), although caprine OA was successfully reported following meniscal manipulation and running regimens (Al Faqeh et al., 2012).
Equine models are uniquely useful yet challenging for cartilage injury investigation. Horses spontaneously develop chondral defects and age/trauma-induced OA secondary to high athletic and lifestyle demands, and thus provide a uniquely-representative model of osteochondral injury for humans (McIlwraith et al., 2012). Surgical OA models can also be generated via osteochondral defects, commonly within the carpal joint (McCoy 2015). Equine articular cartilage possesses minimal intrinsic repair capability, with cartilage thickness ranging between 1.5–2 mm and study defect sizes between 6–20 mm (McIlwraith et al., 2012). Horse stifle structure and bone mineral density are also comparable to that of humans (Ahern et al., 2009). Importantly, equine cartilage experiences significantly greater loading forces compared to humans (Murray et al., 2001), which can induce long-term healing failure and is difficult to prevent (McCoy 2015). Horses are also especially costly to maintain due to specialized housing and care.
Allogenic MSCs yield mixed results in equine osteochondral defects. Scaffold-free implantation of 50 million sMSCs into femoral condyle osteochondral defects bolstered gross, MRI, and histological scores of cartilage regeneration (Murata et al., 2022). However, polycaprolactone and HA scaffolds containing bMSCs and cartilage progenitor cells yielded poor neocartilage formation, hypothesized to stem from early implanted cell loss (Mancini et al., 2020). Insufficient MSC numbers and off-target differentiation may also hinder regeneration; implantation of autologous bone marrow concentrate (containing non-expanded bMSCs) and thrombin into full-thickness trochlear defects yielded fibrocartilaginous tissue after 1 year, comparable to microfracture (Chu et al., 2018). Future equine studies may elucidate mechanisms of treatment failure including local inflammation, pathologic scaffold degradation, and MSC dedifferentiation (Mancini et al., 2020).
MSCs are multipotent, self-renewing progenitor cells isolatable from various tissues and studied for cartilage regeneration in vitro (Fulber et al., 2016; Sasaki et al., 2018), in the aforementioned models, and in clinical trials (Matas et al., 2019; Dilogo et al., 2020). Attractively, MSCs can proliferate and differentiate into chondrocytes under endogenous/exogenous signals including matrix, growth factors, proteins, drugs, and mechanical stimuli (Le et al., 2020). MSCs also secrete a secretome of bioactive molecules, i.e., growth factors, prostaglandins, and extracellular vesicles, which modulate the local niche to attenuate inflammation and promote host cell migration, proliferation, differentiation, and matrix deposition (Maumus et al., 2018).
MSCderived exosomes—a subtype of extracellular vesicles—are particularly promising for cell-free cartilage therapeutics. Exosomes are increasingly considered the primary secretory mechanism by which MSCs modulate local healing (Toh et al., 2017), and mediate intercellular communication by exhibiting target-specific paracrine effects on recipient cells. Exosome contents include proteins, nucleic acids, lipids, and other biomolecules encapsulated within phospholipid bilayer and surface ligands (Bao and He 2021). Importantly, exosomes avoid potential shortcomings of direct MSC transplantation, include dedifferentiation, immunogenicity, and batch heterogeneity (Bao and He 2021).
The different MSC tissue sources studied in the aforementioned models (Table 1) affect the proliferation, cartilaginous matrix deposition, chondrogenic differentiation, and overall therapeutic behavior of isolated MSCs (Yaneselli et al., 2018; Gugjoo et al., 2019). bMSCs are among the earliest and most-studied MSC populations in large animal and clinical studies (Lo Monaco et al., 2018). Equine bMSCs exhibit greater chondrogenic potential than aMSCs, while human bMSCs exhibit less calcification potential than sMSCs (Sasaki et al., 2018). However, bone marrow extraction is invasive and complicated by low bMSC density (∼7800 MSCs/ml in humans) (Hernigou et al., 2021). Therefore, aMSCs (particularly from joint-associated adipose) are increasingly popular for cartilage regeneration due to highly-available and easily-accessible tissue, rapid procurement, and chondrogenic potential (Zhang et al., 2019; De Francesco et al., 2021). Ovine aMSCs proliferated faster than bMSCs but expressed lower cartilage-specific gene levels for collagen II, SOX9, and aggrecan in vitro, despite both MSC types performing similarly in osteoarthritic ovine knees (Ude et al., 2014). Canine infrapatellar fat aMSCs also exhibited greater proliferation and colony expansion in vitro than those from subcutaneous fat (Sasaki et al., 2018). Moreover, equine intra-articular fat aMSCs displayed greater chondrogenic potential than those from non-joint-associated adipose (Gugjoo et al., 2019).
MSCs are exciting for cartilage regeneration due to their significant chondrogenic potential reported by numerous studies. sMSCs are isolatable from synovial membrane or fluid, although equine studies demonstrated superior chondrogenic potential of synovial fluid sMSCs in vitro (To et al., 2019). MSCs from subtypes of porcine synovium (fibrous vs. adipose) also varied in growth factor signaling and membrane receptors (Siengdee et al., 2020). Compared with bMSCs and aMSCs, canine sMSCs exhibited superior proliferation, matrix deposition, and rates of stem cell marker CD90 positivity (Sasaki et al., 2018), while porcine sMSCs displayed greater chondrogenic potential (Nakamura et al., 2012). A notable disadvantage is limited synovium and cell quantity—autologous sMSCs are typically expanded prior to reimplantation (To et al., 2019).
Xenogenic human umbilical cord-derived MSCs provide valuable opportunities to study human MSC behavior within large animal osteochondral microenvironments. MSCs are extracted from umbilical cord matrix and blood (Rakic et al., 2018) and demonstrate marked advantages of phenotypic homogeneity, minimal immunogenicity, and tissue availability (Zhang Y. et al., 2018). Simple/noninvasive MSC procurement also circumvents autologous cell extraction procedures and avoids associated donor site morbidity. Promising early findings in multiple large animal models suggest that human umbilical cord MSCs may perform similarly in a clinical setting (Zhang B. Y. et al., 2018; Wu et al., 2019; Kim et al., 2022).
Finally, the choice of autogenic, allogenic, or xenogenic MSCs shapes the conclusions and translatability of large animal studies. Autologous and allogenic mammalian MSCs facilitate proof-of-concept studies, but may yield poor results upon clinical translation due to human and inter-animal/species differences in cell behavior (Siengdee et al., 2020). Subsequently, xenogenic human MSC delivery in large animals helps elucidate human-specific cellular behavior in vivo and hint at future translational efficacy, although immunogenicity, interaction with animal host cells, and poor model representation of real-world pathology may still alter outcomes (Lo Monaco et al., 2018; Zayed et al., 2018).
Tissue engineering strategies facilitate MSC delivery and modulate MSC activity, and typically categorize into three-dimensional scaffolds, bioactive dissolved molecules, direct cellular modification, defect targeting systems (Go et al., 2021) and extracellular vesicles (Figure 1). Several large animal studies report that biphasic osteochondral scaffolds simultaneously promote bone and cartilage regeneration (Zhang et al., 2017; Cunniffe et al., 2019). However, others report mixed results hypothesized to stem from local inflammation and residual byproducts from biphasic construct degradation (Wang et al., 2018; Bothe et al., 2019; Mancini et al., 2020). Alternatively, three-dimensional-printed MSC constructs (Di Bella et al., 2018; Yamasaki et al., 2019) or cell-suspension hydrogels (Levato et al., 2017; Rathan et al., 2019) enable resurfacing of diffusely-osteoarthritic articular surfaces with irregular borders. Bioactive molecules encompass growth factors [bone morphogenic protein-2, transforming growth factor-beta, fibroblast growth factor-2 (Desance et al., 2018), and NEL-like molecule-1 (Li et al., 2016)], matrix molecules including DCECM (Kim et al., 2019; Lu Y. et al., 2021), and ions which recapitulate the native chondrogenic niche and bolster MSC chondrogenic differentiation, proliferation, and matrix expression.
FIGURE 1. MSC-harnessing strategies for articular cartilage regeneration in large animal models. Different types of MSCs—autologous, allogenic and xenogenic—were first obtained from adipose tissue (aMSC), bone marrow (bMSC), synovium (sMSC), and human umbilical cords (umMSC & ubMSC). Subsequently, MSCs were pretreated with and/or delivered through (1) 3-D scaffolds, (2) bioactive dissolved molecules, (3) direct cellular modifications, (4) defect targeting systems, and (5) cell-free MSC-derived exosomes, for enhancing cartilage regeneration and/or modulating inflammation. Currently, the two major routes of MSC administration in preclinical large animal studies are intra-articular injection and local implantation within chondral/osteochondral defects. Figure created with BioRender.com.
Regarding exosomes, intra-articular injection of 1 mg human MSC exosomes and HA improved cartilage Young’s modulus, stiffness, and MRI/histological scores in porcine femoral condyles (Zhang et al., 2022). Preliminary comparisons, albeit in rodents, also suggest that specific dosages of MSCs and MSC-derived exosomes demonstrate similar cartilage regenerative efficacy (Kim et al., 2020). Although the mechanism is incompletely understood, exosome molecules, i.e., micro ribonucleic acids and enzymes are proposed to upregulate chondrocyte proliferation and chondrogenesis (Toh et al., 2017; Kim et al., 2020). MSC exosomes also reportedly attenuate cartilage injury by inhibiting pathologic inflammation, chondrocyte apoptosis, and macrophage activity (Kim et al., 2020). Nevertheless, exosomes can exhibit nonspecific effects—intra-articular injection of 1 mg human embryonic stem cell exosomes after bone marrow stimulation upregulated bone deposition in porcine chondral defects, with impaired cartilage formation on histology (Hede et al., 2021).
There is a pressing need to develop novel and efficacious therapies for regenerating chondral/osteochondral defects in OA. MSCs are among the most promising substitutes for native chondrocytes, as they can exhibit chondrogenic differentiation and local microenvironment modulation. MSC activity also varies with origin tissue and organism, presenting critical considerations when developing regenerative strategies and interpreting preclinical findings. Comparatively, sMSCs may hold particular promise for future cartilage therapeutic development due to superior chondrogenic potential and clinical accessibility. MSC-derived exosomes may also offer similar efficacy while avoiding direct MSC transplantation.
Given the biomechanical/anatomical differences and potent healing capacity of small animals, preclinical studies must utilize large animal models to adequately develop translatable therapeutics. Notably, most MSC-based studies on cartilage regeneration were conducted in ruminant and porcine models (Table 1; Figure 1) because of anatomical/biomechanical similarity to human tissue, cost-effectiveness, and extensive post-treatment analysis options. Nevertheless, canines and equines also offer unique advantages regarding spontaneous OA modelling and subsequent clinical translatability. Moreover, tremendous advances in chondrogenic MSC delivery systems include architecturally- and mechanically-biomimetic scaffolds (Levato et al., 2017; Rathan et al., 2019) and MSC genetic editing (Sun et al., 2020) (Figure 1).
Despite the limited scope of this mini-review, we determined that there is no clear consensus or standard regarding critical aspects of MSC therapy for large animal cartilage regeneration, particularly regarding effective MSC dosages for implantation or injection in differing species (Table 1) (Li et al., 2018; Kim et al., 2022). Other unstandardized aspects include methodology for MSC chondrogenic induction and/or expansion, surgical protocols for OCG or ACI, and therapeutic benchmarks/controls for cartilage regeneration efficacy in various species. As the field advances, it is paramount to establish comparability between studies and holistically evaluate translatability of MSC-therapeutic findings, especially in large animal models, to maximize clinical relevancy and impact.
TL, PH, and CX collected and interrelated the data for the work. TL, PH, and CX interpreted the data, as well as drafted and revised the manuscript. TL, CX, and KK drafted and revised the supplemental table and figures. AJ and JE critically-reviewed and revised the manuscript. QY and XZ established the concept, designed the work, provided financial support, and critically-reviewed, revised, and approved the manuscript.
This study was supported by the NIH/NIDCR R01DE029353 and Science and Technology Planning Project of Jiangsu Province (BK20200001).
XZ is a founder of Bone Biologics Inc./Bone Biologics Corp., which sublicenses Nell-1 patents from the UC Regents, which also hold equity in the company.
The remaining authors declare that the research was conducted in the absence of any commercial or financial relationships that could be construed as a potential conflict of interest.
All claims expressed in this article are solely those of the authors and do not necessarily represent those of their affiliated organizations, or those of the publisher, the editors and the reviewers. Any product that may be evaluated in this article, or claim that may be made by its manufacturer, is not guaranteed or endorsed by the publisher.
ACI, autologous chondrocyte implantation; ACL, anterior cruciate ligament; aMSCs, adipose tissue-derived mesenchymal stem cells; bMSCs, bone marrow-derived mesenchymal stem cells; DCECM, decellularized cartilage extracellular matrix; HA, hyaluronic acid; MSCs, mesenchymal stem cells; MRI, magnetic resonance imaging; OA, osteoarthritis; OCG, osteochondral grafting; sMSCs, synovial tissue-derived mesenchymal stem cells; ubMSCs, umbilical cord blood-derived mesenchymal stem cells; umMSCs, umbilical cord matrix-derived mesenchymal stem cells.
Ahern, B. J., Parvizi, J., Boston, R., and Schaer, T. P. (2009). Preclinical animal models in single site cartilage defect testing: A systematic review. Osteoarthr. Cartil. 17 (6), 705–713. doi:10.1016/j.joca.2008.11.008
Al Faqeh, H., Nor Hamdan, B. M., Chen, H. C., Aminuddin, B. S., and Ruszymah, B. H. (2012). The potential of intra-articular injection of chondrogenic-induced bone marrow stem cells to retard the progression of osteoarthritis in a sheep model. Exp. Gerontol. 47 (6), 458–464. doi:10.1016/j.exger.2012.03.018
Bao, C., and He, C. (2021). The role and therapeutic potential of MSC-derived exosomes in osteoarthritis. Arch. Biochem. Biophys. 710, 109002. doi:10.1016/j.abb.2021.109002
Bothe, F., Deubel, A. K., Hesse, E., Lotz, B., Groll, J., Werner, C., et al. (2019). Treatment of focal cartilage defects in minipigs with zonal chondrocyte/mesenchymal progenitor cell constructs. Int. J. Mol. Sci. 20 (3), E653. doi:10.3390/ijms20030653
Botto, R., Riccio, V., Galosi, L., Rossi, G., Vincenzetti, S., Tambella, A. M., et al. (2022). Effects of intra-articular autologous adipose micrograft for the treatment of osteoarthritis in dogs: A prospective, randomized, controlled study. Animals. 12 (14), 1844. doi:10.3390/ani12141844
Chen, D., Shen, J., Zhao, W., Wang, T., Han, L., Hamilton, J. L., et al. (2017). Osteoarthritis: Toward a comprehensive understanding of pathological mechanism. Bone Res. 5, 16044. doi:10.1038/boneres.2016.44
Chu, C. R., Fortier, L. A., Williams, A., Payne, K. A., McCarrel, T. M., Bowers, M. E., et al. (2018). Minimally manipulated bone marrow concentrate compared with microfracture treatment of full-thickness chondral defects A one-year study in an equine model. J. Bone Jt. Surg. Am. 100 (2), 138–146. doi:10.2106/Jbjs.17.00132
Chu, C. R., Szczodry, M., and Bruno, S. (2010). Animal models for cartilage regeneration and repair. Tissue Eng. Part B Rev. 16 (1), 105–115. doi:10.1089/ten.TEB.2009.0452
Cone, S. G., Warren, P. B., and Fisher, M. B. (2017). Rise of the pigs: Utilization of the porcine model to study musculoskeletal biomechanics and tissue engineering during skeletal growth. Tissue Eng. Part C Methods 23 (11), 763–780. doi:10.1089/ten.TEC.2017.0227
Cook, J. L., Hung, C. T., Kuroki, K., Stoker, A. M., Cook, C. R., Pfeiffer, F. M., et al. (2014). Animal models of cartilage repair. Bone Jt. Res. 3 (4), 89–94. doi:10.1302/2046-3758.34.2000238
Cunniffe, G. M., Diaz-Payno, P. J., Sheehy, E. J., Critchley, S. E., Almeida, H. V., Pitacco, P., et al. (2019). Tissue-specific extracellular matrix scaffolds for the regeneration of spatially complex musculoskeletal tissues. Biomaterials 188, 63–73. doi:10.1016/j.biomaterials.2018.09.044
De Francesco, F., Riccio, V., Biswas, R., Busato, A., Di Bella, C., Serri, E., et al. (2021). In vitro characterization of canine microfragmented adipose tissue non-enzymatically extracted from the thigh and lumbar regions. Animals. 11 (11), 3231. doi:10.3390/ani11113231
Desance, M., Contentin, R., Bertoni, L., Gomez-Leduc, T., Branly, T., Jacquet, S., et al. (2018). Chondrogenic differentiation of defined equine mesenchymal stem cells derived from umbilical cord blood for use in cartilage repair therapy. Int. J. Mol. Sci. 19 (2), E537. doi:10.3390/ijms19020537
Di Bella, C., Duchi, S., O'Connell, C. D., Blanchard, R., Augustine, C., Yue, Z., et al. (2018). In situ handheld three-dimensional bioprinting for cartilage regeneration. J. Tissue Eng. Regen. Med. 12 (3), 611–621. doi:10.1002/term.2476
Dilogo, I. H., Canintika, A. F., Hanitya, A. L., Pawitan, J. A., Liem, I. K., and Pandelaki, J. (2020). Umbilical cord-derived mesenchymal stem cells for treating osteoarthritis of the knee: A single-arm, open-label study. Eur. J. Orthop. Surg. Traumatol. 30 (5), 799–807. doi:10.1007/s00590-020-02630-5
Favreau, H., Pijnenburg, L., Seitlinger, J., Fioretti, F., Keller, L., Scipioni, D., et al. (2020). Osteochondral repair combining therapeutics implant with mesenchymal stem cells spheroids. Nanomedicine 29, 102253. doi:10.1016/j.nano.2020.102253
Feng, C., Luo, X., He, N., Xia, H., Lv, X., Zhang, X., et al. (2018). Efficacy and persistence of allogeneic adipose-derived mesenchymal stem cells combined with hyaluronic acid in osteoarthritis after intra-articular injection in a sheep model. Tissue Eng. Part A 24 (3-4), 219–233. doi:10.1089/ten.TEA.2017.0039
Fulber, J., Maria, D. A., da Silva, L. C., Massoco, C. O., Agreste, F., and Baccarin, R. Y. (2016). Comparative study of equine mesenchymal stem cells from healthy and injured synovial tissues: An in vitro assessment. Stem Cell. Res. Ther. 7, 35. doi:10.1186/s13287-016-0294-3
Go, G., Yoo, A., Kim, S., Seon, J. K., Kim, C. S., Park, J. O., et al. (2021). Magnetization-switchable implant system to target delivery of stem cell-loaded bioactive polymeric microcarriers. Adv. Healthc. Mat. 10 (19), e2100068. doi:10.1002/adhm.202100068
Grassel, S., and Aszodi, A. (2019). Osteoarthritis and cartilage regeneration: Focus on pathophysiology and molecular mechanisms. Int. J. Mol. Sci. 20 (24), E6156. doi:10.3390/ijms20246156
Gugjoo, M. B., AmarpalFazili, M. U. R., Gayas, M. A., Ahmad, R. A., and Dhama, K. (2019). Animal mesenchymal stem cell research in cartilage regenerative medicine - a review. Vet. Q. 39 (1), 95–120. doi:10.1080/01652176.2019.1643051
Hede, K. T. C., Christensen, B. B., Olesen, M. L., Thomsen, J. S., Foldager, C. B., Toh, W. S., et al. (2021). Mesenchymal stem cell extracellular vesicles as adjuvant to bone marrow stimulation in chondral defect repair in a minipig model. Cartilage 13(2_Suppl), 254s–266s. doi:10.1177/19476035211029707
Heo, S. M., Harris, I., Naylor, J., and Lewin, A. M. (2020). Complications to 6 months following total hip or knee arthroplasty: Observations from an Australian clinical outcomes registry. BMC Musculoskelet. Disord. 21 (1), 602. doi:10.1186/s12891-020-03612-8
Hernigou, P., Delambre, J., Quiennec, S., and Poignard, A. (2021). Human bone marrow mesenchymal stem cell injection in subchondral lesions of knee osteoarthritis: A prospective randomized study versus contralateral arthroplasty at a mean fifteen year follow-up. Int. Orthop. 45 (2), 365–373. doi:10.1007/s00264-020-04571-4
Hunter, D. J., Schofield, D., and Callander, E. (2014). The individual and socioeconomic impact of osteoarthritis. Nat. Rev. Rheumatol. 10 (7), 437–441. doi:10.1038/nrrheum.2014.44
Katz, J. N., Arant, K. R., and Loeser, R. F. (2021). Diagnosis and treatment of hip and knee osteoarthritis: A review. JAMA 325 (6), 568–578. doi:10.1001/jama.2020.22171
Keller, L., Pijnenburg, L., Idoux-Gillet, Y., Bornert, F., Benameur, L., Tabrizian, M., et al. (2019). Preclinical safety study of a combined therapeutic bone wound dressing for osteoarticular regeneration. Nat. Commun. 10 (1), 2156. doi:10.1038/s41467-019-10165-5
Kim, M., Ahn, J., Lee, J., Song, S., Lee, S., Lee, S., et al. (2022). Combined mesenchymal stem cells and cartilage acellular matrix injection therapy for osteoarthritis in goats. Tissue Eng. Regen. Med. 19 (1), 177–187. doi:10.1007/s13770-021-00407-3
Kim, Y. G., Choi, J., and Kim, K. (2020). Mesenchymal stem cell-derived exosomes for effective cartilage tissue repair and treatment of osteoarthritis. Biotechnol. J. 15 (12), e2000082. doi:10.1002/biot.202000082
Kim, Y. S., Majid, M., Melchiorri, A. J., and Mikos, A. G. (2019). Applications of decellularized extracellular matrix in bone and cartilage tissue engineering. Bioeng. Transl. Med. 4 (1), 83–95. doi:10.1002/btm2.10110
Kondo, S., Nakagawa, Y., Mizuno, M., Katagiri, K., Tsuji, K., Kiuchi, S., et al. (2019). Transplantation of aggregates of autologous synovial mesenchymal stem cells for treatment of cartilage defects in the femoral condyle and the femoral groove in microminipigs. Am. J. Sports Med. 47 (10), 2338–2347. doi:10.1177/0363546519859855
Lamo-Espinosa, J. M., Prosper, F., Blanco, J. F., Sanchez-Guijo, F., Alberca, M., Garcia, V., et al. (2021). Long-term efficacy of autologous bone marrow mesenchymal stromal cells for treatment of knee osteoarthritis. J. Transl. Med. 19 (1), 506. ARTN 506. doi:10.1186/s12967-021-03160-2
Le, H., Xu, W., Zhuang, X., Chang, F., Wang, Y., and Ding, J. (2020). Mesenchymal stem cells for cartilage regeneration. J. Tissue Eng. 11, 2041731420943839. doi:10.1177/2041731420943839
Levato, R., Webb, W. R., Otto, I. A., Mensinga, A., Zhang, Y., van Rijen, M., et al. (2017). The bio in the ink: Cartilage regeneration with bioprintable hydrogels and articular cartilage-derived progenitor cells. Acta Biomater. 61, 41–53. doi:10.1016/j.actbio.2017.08.005
Li, C. S., Zhang, X., Peault, B., Jiang, J., Ting, K., Soo, C., et al. (2016). Accelerated chondrogenic differentiation of human perivascular stem cells with NELL-1. Tissue Eng. Part A 22 (3-4), 272–285. doi:10.1089/ten.TEA.2015.0250
Li, L., Duan, X., Fan, Z. X., Chen, L., Xing, F., Xu, Z., et al. (2018). Mesenchymal stem cells in combination with hyaluronic acid for articular cartilage defects. Sci. Rep. 8, 9900. ARTN 9900. doi:10.1038/s41598-018-27737-y
Libbin, R. M., and Rivera, M. E. (1989). Regeneration of growth plate cartilage induced in the neonatal rat hindlimb by reamputation. J. Orthop. Res. 7 (5), 674–682. doi:10.1002/jor.1100070507
Lo Monaco, M., Merckx, G., Ratajczak, J., Gervois, P., Hilkens, P., Clegg, P., et al. (2018). Stem cells for cartilage repair: Preclinical studies and insights in translational animal models and outcome measures. Stem Cells Int. 2018, 9079538. doi:10.1155/2018/9079538
Lu, L., Shang, X. F., Liu, B., Chen, W. J., Zhang, Y., Liu, S. Y., et al. (2021a). Repair of articular cartilage defect using adipose-derived stem cell-loaded scaffold derived from native cartilage extracellular matrix. J. Cell. Physiol. 236 (6), 4244–4257. doi:10.1002/jcp.30020
Lu, Y., Wang, Y., Zhang, H., Tang, Z., Cui, X., Li, X., et al. (2021b). Solubilized cartilage ECM facilitates the recruitment and chondrogenesis of endogenous BMSCs in collagen scaffolds for enhancing microfracture treatment. ACS Appl. Mat. Interfaces 13 (21), 24553–24564. doi:10.1021/acsami.1c07530
Lv, X., He, J., Zhang, X., Luo, X., He, N., Sun, Z., et al. (2018). Comparative efficacy of autologous stromal vascular fraction and autologous adipose-derived mesenchymal stem cells combined with hyaluronic acid for the treatment of sheep osteoarthritis. Cell. Transpl. 27 (7), 1111–1125. doi:10.1177/0963689718773333
Mancini, I. A. D., Schmidt, S., Brommer, H., Pouran, B., Schafer, S., Tessmar, J., et al. (2020). A composite hydrogel-3D printed thermoplast osteochondral anchor as example for a zonal approach to cartilage repair: In vivo performance in a long-term equine model. Biofabrication 12 (3), 035028. doi:10.1088/1758-5090/ab94ce
Matas, J., Orrego, M., Amenabar, D., Infante, C., Tapia-Limonchi, R., Cadiz, M. I., et al. (2019). Umbilical cord-derived mesenchymal stromal cells (MSCs) for knee osteoarthritis: Repeated MSC dosing is superior to a single MSC dose and to hyaluronic acid in a controlled randomized phase I/II trial. Stem Cells Transl. Med. 8 (3), 215–224. doi:10.1002/sctm.18-0053
Maumus, M., Pers, Y. M., Ruiz, M., Jorgensen, C., and Noel, D. (2018). Mesenchymal stem cells and regenerative medicine: Future perspectives in osteoarthritis. Med. Sci. 34 (12), 1092–1099. doi:10.1051/medsci/2018294
McCoy, A. M. (2015). Animal models of osteoarthritis: Comparisons and key considerations. Vet. Pathol. 52 (5), 803–818. doi:10.1177/0300985815588611
McIlwraith, C. W., Frisbie, D. D., and Kawcak, C. E. (2012). The horse as a model of naturally occurring osteoarthritis. Bone Jt. Res. 1 (11), 297–309. doi:10.1302/2046-3758.111.2000132
Moran, C. J., Ramesh, A., Brama, P. A., O'Byrne, J. M., O'Brien, F. J., and Levingstone, T. J. (2016). The benefits and limitations of animal models for translational research in cartilage repair. J. Exp. Orthop. 3 (1), 1. doi:10.1186/s40634-015-0037-x
Murata, D., Ishikawa, S., Sunaga, T., Saito, Y., Sogawa, T., Nakayama, K., et al. (2022). Osteochondral regeneration of the femoral medial condyle by using a scaffold-free 3D construct of synovial membrane-derived mesenchymal stem cells in horses. BMC Vet. Res. 18 (1), 53. doi:10.1186/s12917-021-03126-y
Murray, R. C., Vedi, S., Birch, H. L., Lakhani, K. H., and Goodship, A. E. (2001). Subchondral bone thickness, hardness and remodelling are influenced by short-term exercise in a site-specific manner. J. Orthop. Res. 19 (6), 1035–1042. doi:10.1016/S0736-0266(01)00027-4
Mustapich, T., Schwartz, J., Palacios, P., Liang, H., Sgaglione, N., and Grande, D. A. (2020). A novel strategy to enhance microfracture treatment with stromal cell-derived factor-1 in a rat model. Front. Cell. Dev. Biol. 8, 595932. doi:10.3389/fcell.2020.595932
Nakamura, T., Sekiya, I., Muneta, T., Hatsushika, D., Horie, M., Tsuji, K., et al. (2012). Arthroscopic, histological and MRI analyses of cartilage repair after a minimally invasive method of transplantation of allogeneic synovial mesenchymal stromal cells into cartilage defects in pigs. Cytotherapy 14 (3), 327–338. doi:10.3109/14653249.2011.638912
Patil, S., Steklov, N., Song, L., Bae, W. C., and D'Lima, D. D. (2014). Comparative biomechanical analysis of human and caprine knee articular cartilage. Knee 21 (1), 119–125. doi:10.1016/j.knee.2013.03.009
Proffen, B. L., McElfresh, M., Fleming, B. C., and Murray, M. M. (2012). A comparative anatomical study of the human knee and six animal species. Knee 19 (4), 493–499. doi:10.1016/j.knee.2011.07.005
Rakic, R., Bourdon, B., Demoor, M., Maddens, S., Saulnier, N., and Galera, P. (2018). Differences in the intrinsic chondrogenic potential of equine umbilical cord matrix and cord blood mesenchymal stromal/stem cells for cartilage regeneration. Sci. Rep. 8, 13799. ARTN 13799. doi:10.1038/s41598-018-28164-9
Rathan, S., Dejob, L., Schipani, R., Haffner, B., Mobius, M. E., and Kelly, D. J. (2019). Fiber reinforced cartilage ECM functionalized bioinks for functional cartilage tissue engineering. Adv. Healthc. Mat. 8 (7), e1801501. doi:10.1002/adhm.201801501
Safiri, S., Kolahi, A. A., Smith, E., Hill, C., Bettampadi, D., Mansournia, M. A., et al. (2020). Global, regional and national burden of osteoarthritis 1990-2017: A systematic analysis of the global burden of disease study 2017. Ann. Rheum. Dis. 79 (6), 819–828. doi:10.1136/annrheumdis-2019-216515
Sasaki, A., Mizuno, M., Mochizuki, M., and Sekiya, I. (2019). Mesenchymal stem cells for cartilage regeneration in dogs. World J. Stem Cells 11 (5), 254–269. doi:10.4252/wjsc.v11.i5.254
Sasaki, A., Mizuno, M., Ozeki, N., Katano, H., Otabe, K., Tsuji, K., et al. (2018). Canine mesenchymal stem cells from synovium have a higher chondrogenic potential than those from infrapatellar fat pad, adipose tissue, and bone marrow. Plos One 13 (8), e0202922. ARTN e0202922. doi:10.1371/journal.pone.0202922
Siengdee, P., Oster, M., Reyer, H., Viergutz, T., Wimmers, K., and Ponsuksili, S. (2020). Morphological and molecular features of porcine mesenchymal stem cells derived from different types of synovial membrane, and genetic background of cell donors. Front. Cell. Dev. Biol. 8, 601212. doi:10.3389/fcell.2020.601212
Sun, J., Lyu, J., Xing, F., Chen, R., Duan, X., and Xiang, Z. (2020). A biphasic, demineralized, and Decellularized allograft bone-hydrogel scaffold with a cell-based BMP-7 delivery system for osteochondral defect regeneration. J. Biomed. Mat. Res. A 108 (9), 1909–1921. doi:10.1002/jbm.a.36954
Takroni, T., Laouar, L., Adesida, A., Elliott, J. A., and Jomha, N. M. (2016). Anatomical study: Comparing the human, sheep and pig knee meniscus. J. Exp. Orthop. 3 (1), 35. doi:10.1186/s40634-016-0071-3
Theruvath, A. J., Mahmoud, E. E., Wu, W., Nejadnik, H., Kiru, L., Liang, T., et al. (2021). Ascorbic acid and iron supplement treatment improves stem cell-mediated cartilage regeneration in a minipig model. Am. J. Sports Med. 49 (7), 1861–1870. doi:10.1177/03635465211005754
To, K., Zhang, B., Romain, K., Mak, C., and Khan, W. (2019). Synovium-derived mesenchymal stem cell transplantation in cartilage regeneration: A prisma review of in vivo studies. Front. Bioeng. Biotechnol. 7, 314. doi:10.3389/fbioe.2019.00314
Toh, W. S., Lai, R. C., Hui, J. H. P., and Lim, S. K. (2017). MSC exosome as a cell-free MSC therapy for cartilage regeneration: Implications for osteoarthritis treatment. Semin. Cell. Dev. Biol. 67, 56–64. doi:10.1016/j.semcdb.2016.11.008
Tseng, W. J., Huang, S. W., Fang, C. H., Hsu, L. T., Chen, C. Y., Shen, H. H., et al. (2018). Treatment of osteoarthritis with collagen-based scaffold: A porcine animal model with xenograft mesenchymal stem cells. Histol. Histopathol. 33 (12), 1271–1286. doi:10.14670/Hh-18-013
Ude, C. C., Sulaiman, S. B., Min-Hwei, N., Hui-Cheng, C., Ahmad, J., Yahaya, N. M., et al. (2014). Cartilage regeneration by chondrogenic induced adult stem cells in osteoarthritic sheep model. PLoS One 9 (6), e98770. doi:10.1371/journal.pone.0098770
Uilenreef, J., van der Staay, F. J., and Meijer, E. (2019). A monosodium iodoacetate osteoarthritis lameness model in growing pigs. Animals. 9 (7), E405. doi:10.3390/ani9070405
Vahedi, P., Jarolmasjed, S., Shafaei, H., Roshangar, L., Soleimani Rad, J., and Ahmadian, E. (2019). In vivo articular cartilage regeneration through infrapatellar adipose tissue derived stem cell in nanofiber polycaprolactone scaffold. Tissue Cell. 57, 49–56. doi:10.1016/j.tice.2019.02.002
Veronesi, F., Fini, M., Martini, L., Berardinelli, P., Russo, V., Filardo, G., et al. (2022). In vivo model of osteoarthritis to compare allogenic amniotic epithelial stem cells and autologous adipose derived cells. Biol. (Basel) 11 (5), 681. doi:10.3390/biology11050681
Wang, F. Y., Hu, Y. H., He, D. M., Zhou, G. D., and Ellis, E. (2018). Scaffold-free cartilage cell sheet combined with bone-phase BMSCs-scaffold regenerate osteochondral construct in mini-pig model. Am. J. Transl. Res. 10 (10), 2997–3010.
Wei, X., Liu, B., Liu, G., Yang, F., Cao, F., Dou, X., et al. (2019). Mesenchymal stem cell-loaded porous tantalum integrated with biomimetic 3D collagen-based scaffold to repair large osteochondral defects in goats. Stem Cell. Res. Ther. 10 (1), 72. doi:10.1186/s13287-019-1176-2
Wu, K. C., Chang, Y. H., Liu, H. W., and Ding, D. C. (2019). Transplanting human umbilical cord mesenchymal stem cells and hyaluronate hydrogel repairs cartilage of osteoarthritis in the minipig model. Ci Ji Yi Xue Za Zhi 31 (1), 11–19. doi:10.4103/tcmj.tcmj_87_18
Yamasaki, A., Kunitomi, Y., Murata, D., Sunaga, T., Kuramoto, T., Sogawa, T., et al. (2019). Osteochondral regeneration using constructs of mesenchymal stem cells made by bio three-dimensional printing in mini-pigs. J. Orthop. Res. 37 (6), 1398–1408. doi:10.1002/jor.24206
Yaneselli, K. M., Kuhl, C. P., Terraciano, P. B., de Oliveira, F. S., Pizzato, S. B., Pazza, K., et al. (2018). Comparison of the characteristics of canine adipose tissue-derived mesenchymal stem cells extracted from different sites and at different passage numbers. J. Vet. Sci. 19 (1), 13–20. doi:10.4142/jvs.2018.19.1.13
Zayed, M., Adair, S., Ursini, T., Schumacher, J., Misk, N., and Dhar, M. (2018). Concepts and challenges in the use of mesenchymal stem cells as a treatment for cartilage damage in the horse. Res. Vet. Sci. 118, 317–323. doi:10.1016/j.rvsc.2018.03.011
Zhang, B. Y., Wang, B. Y., Li, S. C., Luo, D. Z., Zhan, X., Chen, S. F., et al. (2018a). Evaluation of the curative effect of umbilical cord mesenchymal stem cell therapy for knee arthritis in dogs using imaging Technology. Stem Cells Int. 2018, 1983025. doi:10.1155/2018/1983025
Zhang, L., Luo, Y., Zhou, X., Fu, S., and Wang, G. (2021). Outcomes from osteochondral autograft transplant or mosaicplasty in 26 patients with type V osteochondral lesions of the talus. Med. Sci. Monit. 27, e930527. doi:10.12659/MSM.930527
Zhang, R., Ma, J., Han, J., Zhang, W., and Ma, J. (2019). Mesenchymal stem cell related therapies for cartilage lesions and osteoarthritis. Am. J. Transl. Res. 11 (10), 6275–6289.
Zhang, S., Wong, K. L., Ren, X., Teo, K. Y. W., Afizah, H., Choo, A. B. H., et al. (2022). Mesenchymal stem cell exosomes promote functional osteochondral repair in a clinically relevant porcine model. Am. J. Sports Med. 50 (3), 788–800. doi:10.1177/03635465211068129
Zhang, T., Zhang, H., Zhang, L., Jia, S., Liu, J., Xiong, Z., et al. (2017). Biomimetic design and fabrication of multilayered osteochondral scaffolds by low-temperature deposition manufacturing and thermal-induced phase-separation techniques. Biofabrication 9 (2), 025021. doi:10.1088/1758-5090/aa7078
Zhang, Y., Hao, C., Guo, W., Peng, X., Wang, M., Yang, Z., et al. (2020). Co-culture of hWJMSCs and pACs in double biomimetic ACECM oriented scaffold enhances mechanical properties and accelerates articular cartilage regeneration in a caprine model. Stem Cell. Res. Ther. 11 (1), 180. doi:10.1186/s13287-020-01670-2
Zhang, Y., Liu, S., Guo, W., Wang, M., Hao, C., Gao, S., et al. (2018b). Human umbilical cord Wharton's jelly mesenchymal stem cells combined with an acellular cartilage extracellular matrix scaffold improve cartilage repair compared with microfracture in a caprine model. Osteoarthr. Cartil. 26 (7), 954–965. doi:10.1016/j.joca.2018.01.019
Keywords: cartilage regeneration, mesenchymal stem cell, large animal, osteochondral defect, tissue engineering, intraarticular injection, cell implantation, osteoarthritis
Citation: Liu TP, Ha P, Xiao CY, Kim SY, Jensen AR, Easley J, Yao Q and Zhang X (2022) Updates on mesenchymal stem cell therapies for articular cartilage regeneration in large animal models. Front. Cell Dev. Biol. 10:982199. doi: 10.3389/fcell.2022.982199
Received: 30 June 2022; Accepted: 10 August 2022;
Published: 06 September 2022.
Edited by:
Francesco De Francesco, Azienda Ospedaliero Universitaria Ospedali Riuniti, ItalyReviewed by:
Wei Seong Toh, National University of Singapore, SingaporeCopyright © 2022 Liu, Ha, Xiao, Kim, Jensen, Easley, Yao and Zhang. This is an open-access article distributed under the terms of the Creative Commons Attribution License (CC BY). The use, distribution or reproduction in other forums is permitted, provided the original author(s) and the copyright owner(s) are credited and that the original publication in this journal is cited, in accordance with accepted academic practice. No use, distribution or reproduction is permitted which does not comply with these terms.
*Correspondence: Qingqiang Yao, eWFvcWluZ3FpYW5nQG5qbXUuZWR1LmNu; Xinli Zhang, eHpoYW5nQGRlbnRpc3RyeS51Y2xhLmVkdQ==
Disclaimer: All claims expressed in this article are solely those of the authors and do not necessarily represent those of their affiliated organizations, or those of the publisher, the editors and the reviewers. Any product that may be evaluated in this article or claim that may be made by its manufacturer is not guaranteed or endorsed by the publisher.
Research integrity at Frontiers
Learn more about the work of our research integrity team to safeguard the quality of each article we publish.