by Shikaya Y, Takase Y, Tadokoro R, Nakamura R, Inaba M and Takahashi Y (2022). Front. Cell Dev. Biol. 10:827079. doi: 10.3389/fcell.2022.827079
In their recent article, Shikaya et al. (2022) reported on the existence of preferential sites of nucleation of contractile waves along the chicken embryonic day 12 midgut, termed sites of “origin of peristaltic waves” (OPW). Histological analysis by the authors did not reveal any peculiarities of these sites compared to other locations along the gut. Applying tetrodotoxin or ablating neural crest cells did not suppress the OPWs, but led to a shift in the position of the OPWs along the gut tract. From these observations, the authors suggested that the enteric nervous system may be involved in the motility at earlier stages than previously reported (E16) (Chevalier et al., 2019). Here, I show by simulation that OPWs can emerge spontaneously in smooth muscle tissue that has a high probability of spontaneous depolarization and an associated refractory period, without need for pacemaker or neurogenic activity. The simulations additionally demonstrates that pacemaking by Interstitial Cells of Cajal requires a developmental decrease of the spontaneous depolarization probability of the smooth muscle syncytium.
Localized above-threshold depolarization of the smooth muscle leads to the nucleation of two contractile waves propagating symmetrically away from the point of depolarization, at a constant speed of about 30 μm/s at E8-E12 (Chevalier et al., 2020; Shikaya et al., 2022), and that further depolarizes the tissue in its wake. Smooth muscle can only spontaneously depolarize again after a certain refractory period, which is typically on the order of 10–60 s for chicken embryonic intestinal smooth muscle. The simple idea behind the simulations I present here is that the most probable site for the nucleation of a second wave is the site of the first nucleation, because it is the point where most time has elapsed since it last depolarized. To implement this idea, I put together a Matlab program for a discretized gut (N = 200 sites), with the following rules:
• At each time-step, each site can self-depolarize with a probability
• If self-depolarized, a site gives rise to 2 contractile (depolarizing) waves propagating symmetrically away from the point of depolarization, at a constant speed of 30 μm/s.
• A site can only depolarize after a refractory time
• Colliding waves annihilate, as is observed experimentally.
The simulation was run over 500 time steps, and parameters p and τ were explored to reproduce the experimentally observed frequencies of contractiles waves
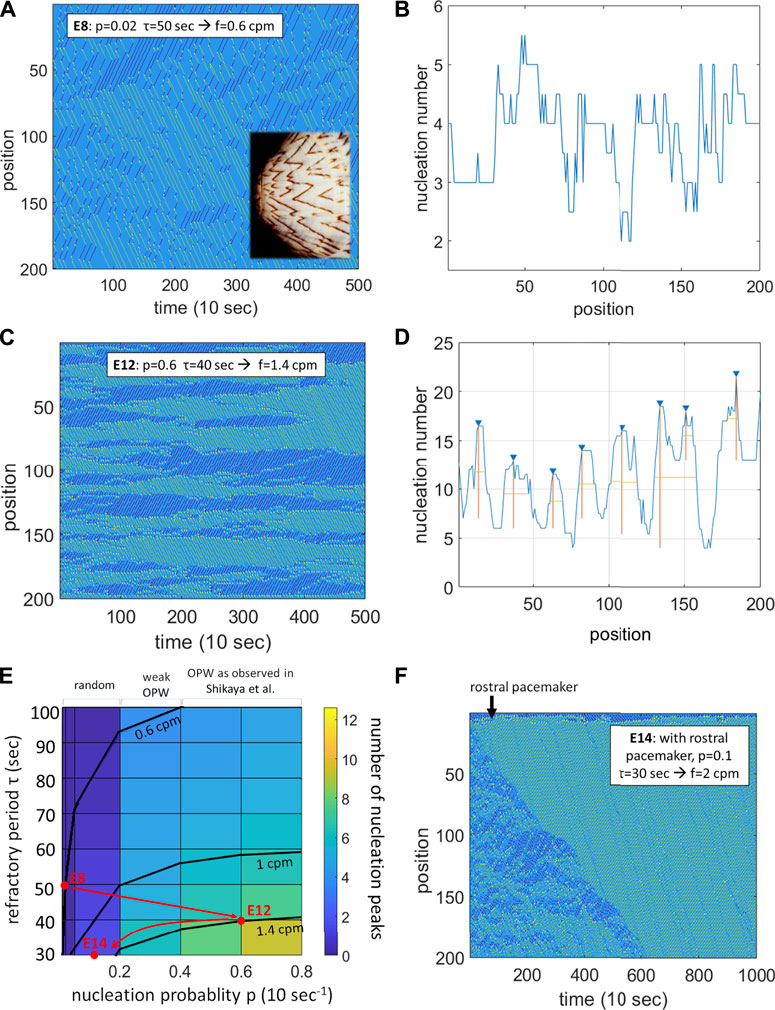
FIGURE 1. A probabilistic refractory period model of the intestinal smooth muscle syncitium reproduces the contractile wave patterns observed in chicken at E8, E12 and E14. (A) Spatiotemporal map for p = 0.02, τ = 50 s, yielding f = 0.6 cpm. Rostro-caudal waves in green, caudo-rostral waves in dark blue, nucleation sites in yellow. Inset: the wave pattern is reminiscent of the pigmentation of some seashells (Meinhardt and Klingler, 1987). (B) Homogeneous distribution of nucleation sites along the gut corresponding to (A). (C) Spatiotemporal map for p = 0.4, τ = 40 s, yielding f = 1.4 cpm. (D) Peaked distribution of nucleation sites (OPWs) along the gut corresponding to (C). (E) Color-coded map of the average number of nucleation sites (OPWs) as a function of the refractory period τ and of the nucleation probability p. Frequency isolines at 0.6, 1 and 1.4 cpm are shown. Red dots: parameters used in (A,C,F) with the suggested developmental trajectory. (F) Spatiotemporal map for p = 0.1, τ = 30 s with an additional rostral pacemaker
The spatiotemporal diagram changes drastically at E14 as pacemaker interstitial cells of Cajal start being active (Chevalier et al., 2020), with waves becoming more unidirectional and regular in time. I modeled this by implementing an oscillatory probability of nucleation at the rostral end
Author contributions
The author confirms being the sole contributor of this work and has approved it for publication.
Funding
Writing of this article was supported by the IdEx Université de Paris ANR-18-IDEX-0001 grant, by the Agence Nationale de la Recherche ANR GASTROMOVE-ANR-19-CE30-0016-01 grant, and by the CNRS PEPS INSIS “COXHAM” grant.
Conflict of interest
The author declares that the research was conducted in the absence of any commercial or financial relationships that could be construed as a potential conflict of interest.
Publisher’s note
All claims expressed in this article are solely those of the authors and do not necessarily represent those of their affiliated organizations, or those of the publisher, the editors and the reviewers. Any product that may be evaluated in this article, or claim that may be made by its manufacturer, is not guaranteed or endorsed by the publisher.
References
Chevalier, N. R., Ammouche, Y., Gomis, A., Teyssaire, C., de Santa Barbara, P., and Faure, S. (2020). Shifting into high gear: How interstitial cells of cajal change the motility pattern of the developing intestine. Am. J. Physiol. Gastrointest. Liver Physiol. 319 (4), G519–G528. doi:10.1152/ajpgi.00112.2020
Chevalier, N. R., Dacher, N., Jacques, C., Langlois, L., Guedj, C., and Faklaris, O. (2019). Embryogenesis of the peristaltic reflex. J. Physiol. 597, 2785–2801. doi:10.1113/JP277746
Chevalier, N. R., Fleury, V., Dufour, S., Proux-Gillardeaux, V., and Asnacios, A. (2017). Emergence and development of gut motility in the chicken embryo. Plos One 12, e0172511. doi:10.1371/journal.pone.0172511
Meinhardt, H., and Klingler, M. (1987). A model for pattern formation on the shells of molluscs. J. Theor. Biol. 126, 63–89. doi:10.1016/s0022-5193(87)80101-7
Shikaya, Y., Takase, Y., Tadokoro, R., Nakamura, R., Inaba, M., and Takahashi, Y. (2022). Distribution map of peristaltic waves in the chicken embryonic gut reveals importance of enteric nervous system and inter-region Cross Talks along the gut Axis. Front. Cell Dev. Biol. 10, 827079–827113. doi:10.3389/fcell.2022.827079
Keywords: chicken, gut, peristalsis, myogenic, refractory period
Citation: Chevalier NR (2022) Commentary: Localization of contractile wave nucleation sites as an emerging phenomenon of stochastic myogenic gut motility. Front. Cell Dev. Biol. 10:979646. doi: 10.3389/fcell.2022.979646
Received: 27 June 2022; Accepted: 22 July 2022;
Published: 29 August 2022.
Edited by:
Shinji Takada, Graduate University for Advanced Studies (Sokendai), JapanReviewed by:
Yoshiko Takahashi, Kyoto University, JapanCopyright © 2022 Chevalier. This is an open-access article distributed under the terms of the Creative Commons Attribution License (CC BY). The use, distribution or reproduction in other forums is permitted, provided the original author(s) and the copyright owner(s) are credited and that the original publication in this journal is cited, in accordance with accepted academic practice. No use, distribution or reproduction is permitted which does not comply with these terms.
*Correspondence: Nicolas R. Chevalier, bmljb2xhcy5jaGV2YWxpZXJAdS1wYXJpcy5mcg==