- 1Department of Molecular and Cell Biology, University of Connecticut, Storrs, CT, United States
- 2Institute for Systems Genomics, University of Connecticut, Storrs, CT, United States
- 3Department of Genetics and Genome Sciences, University of Connecticut Health Center, Farmington, CT, United States
Genome organization includes contacts both within a single chromosome and between distinct chromosomes. Thus, regulatory organization in the nucleus may include interplay of these two types of chromosomal interactions with genome activity. Emerging advances in omics and single-cell imaging technologies have allowed new insights into chromosomal contacts, including those of homologs and sister chromatids, and their significance to genome function. In this review, we highlight recent studies in this field and discuss their impact on understanding the principles of chromosome organization and associated functional implications in diverse cellular processes. Specifically, we describe the contributions of intra-chromosomal, inter-homolog, and inter-sister chromatid contacts to genome organization and gene expression.
Introduction
The genome in eukaryotes is packaged and regulated intricately within the nucleus throughout development. The path from zygote to fully developed multicellular organism includes extensive genome remodeling to achieve diverse cell types. Thus, development represents a powerful system to investigate the processes that lead to varying cellular identities. These fascinating processes include changes at the level of 3D genome structure, epigenetic landscape, and transcription. However, the impact of these changes and their potential interplay remain a topic of active debate. Emerging technologies for high-throughput imaging and mapping of chromosomal contacts have enabled new insights into the relationship between genome morphology, function, and development. Such technological advances have been highlighted in previous reviews (Davies et al., 2017; Kempfer and Pombo, 2020; Hickey et al., 2021; Jerkovic and Cavalli, 2021; Rao et al., 2021). Here, we will discuss recent studies on how interactions between different chromosomes (inter-chromosomal) and those within individual chromosomes (intra-chromosomal) may bear functional significance in the regulation of various cell fates during development.
The interplay between chromosome structure and function
Genome structure is highly organized at several levels of complexity. For instance, the formation of chromatin loops through extrusion can demarcate domains (also called topologically associating domains; TADs), and thereby, partition chromosomes (Rao et al., 2014; Sanborn et al., 2015; Fudenberg et al., 2016). Such domains represent regions of high contact frequency within insulated chromatin neighborhoods separated by boundary regions of low contact frequency (Dixon et al., 2012; Nora et al., 2012; Sexton et al., 2012; Rao et al., 2014). Furthermore, nuclear organization can be spatially segregated into two compartment types associated with either open or closed chromatin (Lieberman-Aiden et al., 2009). More globally, individual chromosomes can occupy distinct territories with a propensity to intermingle with neighboring chromosomes (Cremer and Cremer, 2001; Bolzer et al., 2005; Branco and Pombo, 2006).
Despite an emerging detailed genome structure, it is still unclear whether genome structure is a mere reflection of genome function or if regulation of gene expression is driven by genome organization, ultimately leading to cellular identities [recently reviewed in (van Steensel and Furlong, 2019; Ghavi-Helm, 2020; McCord et al., 2020; Oudelaar and Higgs, 2021)]. Specifically, the impact of chromosomal disruptions on chromatin organization and function seems to vary. For instance, structural disruptions at some individual loci result in a dramatic impact on gene regulation and disease (Spielmann et al., 2018; Ghavi-Helm, 2020; Oudelaar and Higgs, 2021). In contrast, other global and local chromosomal rearrangements do not appear to lead to major alterations in gene expression (Spielmann et al., 2018; Akdemir et al., 2020; Ghavi-Helm, 2020; Oudelaar and Higgs, 2021). Moreover, depletion of regulators such as cohesin and CTCF, both implicated in genome architecture, does not have a strong impact on gene expression (Nora et al., 2017; Rao et al., 2017; Schwarzer et al., 2017). These opposing findings have implications for our understanding of how certain factors act on distinct regulatory elements such as enhancers and promoters to orchestrate cell type-specific gene expression. Some mechanisms suggest chromosome looping may mediate enhancer-promoter contacts and could be correlated with gene activity (Palstra et al., 2003; Vernimmen et al., 2007; Rao et al., 2014; Bonev et al., 2017; Freire-Pritchett et al., 2017; Ghavi-Helm, 2020; Oudelaar et al., 2020; Oudelaar and Higgs, 2021; Reed et al., 2022). Interestingly, a recent study suggests that distinct regulatory sequences, termed tethering elements, could mediate distal enhancer-promoter contacts and determine activation dynamics (Batut et al., 2022). Such promoter-proximal tethering elements are also implicated in co-regulation of distant genes that have mutually shared enhancers (Levo et al., 2022). Conversely, direct contacts between enhancers and promoters may not be required to facilitate gene expression (Alexander et al., 2019; Benabdallah et al., 2019; Heist et al., 2019). Moreover, chromatin contacts do not seem to alter between different embryonic cell types regardless of changes in gene expression (Espinola et al., 2021; Ing-Simmons et al., 2021). Chromosome looping could also be involved in the formation of insulated chromatin domains within individual chromosomes (Dowen et al., 2014; Rao et al., 2014; Sanborn et al., 2015; Fudenberg et al., 2016; Hnisz et al., 2016; Ji et al., 2016). These insulated domains may facilitate enhancer-promoter contacts within domains and could prevent improper contacts between nearby neighboring domains (Dowen et al., 2014; Hnisz et al., 2016; Ji et al., 2016; Spielmann et al., 2018; Batut et al., 2022; Zuin et al., 2022). As promoters and enhancers drive gene expression in development, the generation of such insulated chromatin domains may be key for proper regulatory interactions.
Functional implications of inter-chromosomal contacts
In addition to the spatial organization of individual chromosomes, positioning and interactions between different chromosomes have been gaining increasing attention due to their potential role in multiple cellular processes such as translocations, gene regulation, DNA repair, and evolution. For instance, in various systems, the levels of inter-chromosomal interactions relate to the frequencies of chromosomal translocations (Bickmore and Teague, 2002; Hlatky et al., 2002; Holley et al., 2002; Roix et al., 2003; Arsuaga et al., 2004; Branco and Pombo, 2006; Klein et al., 2011; Engreitz et al., 2012; Evdokimova et al., 2012; Zhang et al., 2012; Roukos et al., 2013; Canela et al., 2017; Rosin et al., 2019). These interactions are regulated by condensin II complex during interphase (Rosin et al., 2018). Consequently, condensin II knockdown results in increased translocation events in the presence of DNA damage (Rosin et al., 2019). Thus, proper intermingling of chromosome territories may be important in securing genome integrity from aberrant translocations. Appearance of elevated translocation events may have significant implications for diseases (Bickmore and Teague, 2002; Roix et al., 2003; Branco and Pombo, 2006; Klein et al., 2011; Engreitz et al., 2012; Evdokimova et al., 2012; Zhang et al., 2012; Roukos et al., 2013; Canela et al., 2017). Moreover, an increase of inter-chromosomal contacts among smaller chromosomes compared to larger ones across multiple vertebrate species may have indications for recombination rates and chromosome evolution (Tanabe et al., 2002; Lieberman-Aiden et al., 2009; Perry et al., 2021; Marlétaz et al., 2022).
Interactions between different chromosomes have also been implicated in gene regulation and chromatin segregation. Active regions associated with open chromatin and gene expression may be in close spatial proximity even if located on different chromosomes (Osborne et al., 2004; Branco and Pombo, 2006; Spilianakis and Flavell, 2006; Zhao et al., 2006; Apostolou and Thanos, 2008; Schoenfelder et al., 2010; Markenscoff-Papadimitriou et al., 2014; Maass et al., 2019; Monahan et al., 2019). For example, monoallelic olfactory receptor expression involves inter-chromosomal interactions between the chosen allele and a collection of intergenic enhancers bound by transcription factors (Markenscoff-Papadimitriou et al., 2014; Monahan et al., 2019). Such specific multi-chromosomal interactions during differentiation could drive the diversity of cellular identities. On the other hand, regions associated with repressed chromatin can also interact. For instance, inter-chromosomal interactions have been implicated in constitutive and facultative heterochromatin formation including telomere and centromere clustering (Dernburg et al., 1996; Mayer et al., 2000; Dimitri, 2004; Bantignies et al., 2011; Clowney et al., 2012; Sexton et al., 2012; Li et al., 2015; Stadler et al., 2017; AlHaj Abed et al., 2019; Erceg et al., 2019).
The concentration of RNAs and proteins with their functionally related genomic loci in the nucleus has gained increasing attention (Bouwman et al., 2022). Specifically, phase separation and multivalent interactions have been implicated in the formation of active and inactive hubs [reviewed in Sabari et al. (2020)]. Complementarily, recent technological advances including ligation-independent approaches have provided insight into multi-way and inter-chromosomal interactions as well as integration of transcript and protein information (Kempfer and Pombo, 2020; Jerkovic and Cavalli, 2021; Winick-Ng et al., 2021). For instance, split-pool recognition of interactions by tag extension (SPRITE) and its derivatives extensively map inter-chromosomal hubs associated with both gene activation and silencing around distinct nuclear bodies (Quinodoz et al., 2018; Quinodoz et al., 2021; Arrastia et al., 2022). In the case of transcription, inhibition of nascent RNAs may affect RNA processing hubs (Quinodoz et al., 2021). Similarly, knockdown of satellite RNAs could impact pericentromeric regions, namely the assembly of a heterochromatic chromocenter (Quinodoz et al., 2021) as previously observed during early development (Casanova et al., 2013). Thus, inter-chromosomal hubs may be another possibility of how gene expression is mediated through a high concentration of non-coding RNAs and/or transcription factors (Figure 1A). Such functional inter-chromosomal hubs could facilitate sharing of spatially clustered resources to selectively promote specific cellular processes.
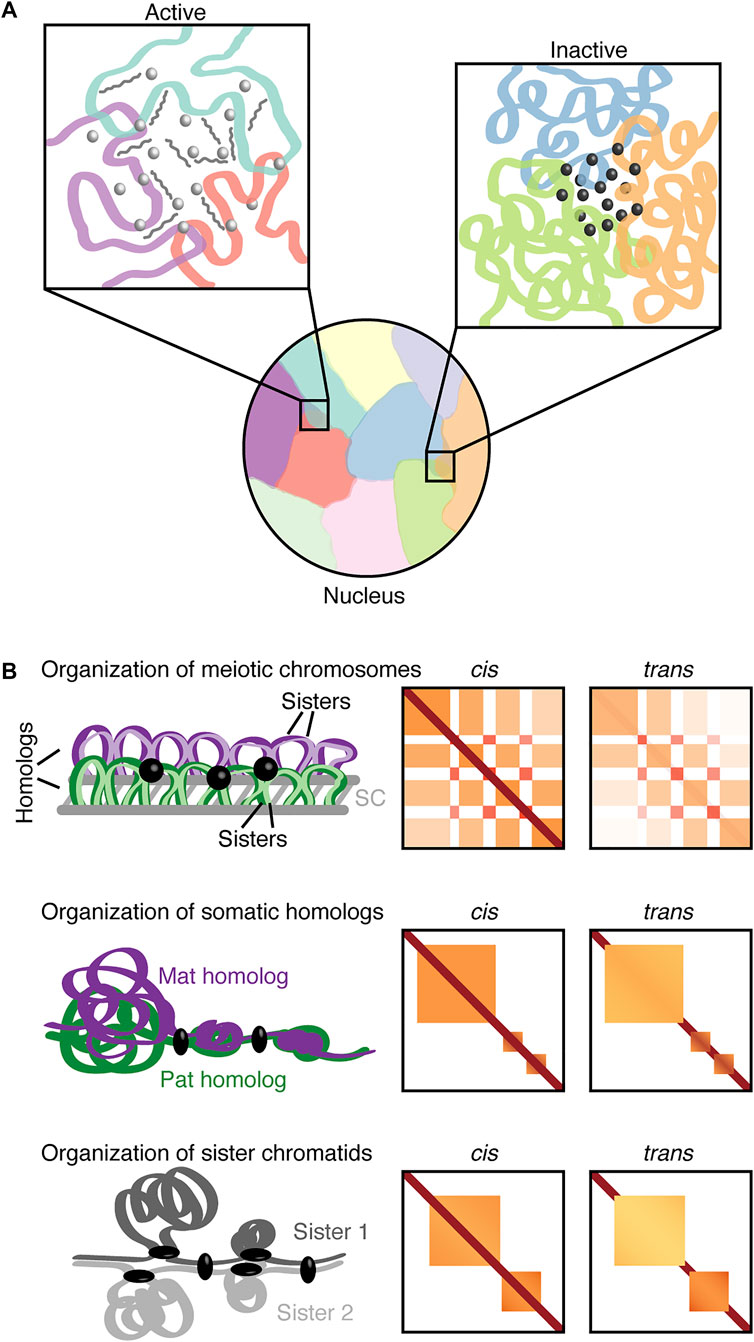
FIGURE 1. Nuclear inter-chromosomal interactions. (A) Chromosomes can occupy discrete territories with a tendency for neighboring chromosomes to intermingle. The left inset depicts an inter-chromosomal hub associated with open chromatin and active transcription with transcription factors (light gray spheres) and RNAs (medium gray). The right inset depicts an inter-chromosomal hub of heterochromatin with associated regulatory factors (dark gray spheres). (B) Schematic organization of mammalian meiotic chromosomes (pachynema; top panel), Drosophila somatic homologs (middle panel), and sister chromatids (bottom panel) with corresponding representations of cis and trans Hi-C contact maps. The meiotic trans contact map depicts inter-homolog contacts. Purple, maternal homolog (Mat); green, paternal homolog (Pat); respective shades of the homolog colors (top panel) or two shades of gray (bottom panel), sister chromatids; gray lines, synaptonemal complex (SC); black sphere, transcription-related clustering; black ellipsoid, regulators (insulators or architectural proteins).
3D chromosome organization in meiotic events
A specific type of interchromosomal interactions in diploid organisms may involve homologous maternal and paternal chromosomes with nearly identical sequences (Figure 1B). Such interactions between pairs of homologs are termed homolog pairing. In meiosis, the juxtaposition between paired homologous chromosomes is facilitated by a proteinaceous structure called the synaptonemal complex. This event together with the formation of DNA double-strand breaks is implicated in proper interhomolog contacts and the promotion of meiotic recombination [reviewed in Zickler and Kleckner (1999), Handel and Schimenti (2010), Keeney et al. (2014)]. Meiotic recombination followed by chromosome segregation mediates the generation of diverse, functional haploid gametes during gametogenesis. Defects in meiotic events may lead to mis-segregation and aneuploidy, thus, impacting fertility and developmental disorders (Hassold et al., 2007; Handel and Schimenti, 2010).
Recent technological advances in contact mapping approaches have allowed for in-depth investigations of the meiotic chromosome organization (Muller et al., 2018; Alavattam et al., 2019; Patel et al., 2019; Schalbetter et al., 2019; Wang et al., 2019). These approaches reveal that during mammalian meiotic prophase domains tend to dissolve, while compartments related to transcription are readily visible (Alavattam et al., 2019; Patel et al., 2019; Wang et al., 2019). Specifically, the gene-rich, transcriptionally active A compartments may form strong inter-chromosomal contacts (Alavattam et al., 2019). Furthermore, haplotype-specific Hi-C has revealed that compartments and clustered transcription-related interactions also occur between paired homologs (Patel et al., 2019). Conversely, meiotic chromosomes condense into arrays of chromatin loops to facilitate effective chromosome segregation (Muller et al., 2018; Alavattam et al., 2019; Patel et al., 2019; Schalbetter et al., 2019; Wang et al., 2019). Such observations indicate that the balance between the compaction of meiotic chromosomes, homolog pairing, and transcription may be critical for development.
While compartments are associated with homolog pairing, the compartment type is also related to meiotic recombination (Patel et al., 2019). In particular, meiotic DNA double-strand break hotspots correlate with the gene-rich A compartments (Patel et al., 2019). In contrast to autosomal recombination, the sex chromosomes X and Y can only pair, synapse, and recombine in the small pseudoautosomal region. The remaining unsynapsed parts of X and Y chromosomes are subject to meiotic sex chromosome inactivation (MSCI) (McKee and Handel, 1993; Handel, 2004; Turner, 2007). Chromosomal contact maps reveal reorganization of the X chromosome from zygonema into pachynema, including depletion of compartments and transcription-related clustering (Alavattam et al., 2019; Patel et al., 2019; Wang et al., 2019). However, the meiotic compaction of X chromosome through chromatin loop arrays is retained (Patel et al., 2019; Wang et al., 2019). Notably, this X chromosome organization in male meiosis is distinct from the inactive X chromosome organization in female X-chromosome inactivation (XCI) (Nora et al., 2012; Rao et al., 2014; Deng et al., 2015; Minajigi et al., 2015; Darrow et al., 2016; Giorgetti et al., 2016; Bonora et al., 2018). This difference may be potentially related to different underlying mechanisms, such as DNA damage response in MSCI or non-coding transcript Xist in XCI (Ichijima et al., 2012; Loda et al., 2022).
The detailed structure of meiotic chromosomes may vary between species as checkerboard patterns on heatmaps have not yet been observed in yeast (Muller et al., 2018; Schalbetter et al., 2019). However, the principal chromatin organization of loop arrays emanating from a proteinaceous axis is preserved across species (Muller et al., 2018; Alavattam et al., 2019; Patel et al., 2019; Schalbetter et al., 2019; Wang et al., 2019). The observation that defects in the synaptonemal complex may impact chromosome compaction in different systems (Schalbetter et al., 2019; Wang et al., 2019) further supports the notion that the fundamental global organization of meiotic chromosomes is largely conserved.
Varying structures and related roles of somatic homolog pairing
While the role of meiotic homolog pairing and its association with recombination is well studied (Zickler and Kleckner, 1999; Handel and Schimenti, 2010; Keeney et al., 2014; Patel et al., 2019), the precise role of somatic homolog pairing is still elusive. Homolog proximity was first noted more than a century ago (Stevens, 1908) and the potential influence between maternal and paternal homologs was hypothesized. Several decades later this communication was observed through interallelic complementation at the Bithorax complex (Lewis, 1954). Since then, this phenomenon, termed transvection, which involves pairing-dependent interallelic complementation, has been observed at multiple individual loci (Pirrotta, 1999; Wu and Morris, 1999; Duncan, 2002; Kennison and Southworth, 2002; McKee, 2004; Apte and Meller, 2012; Kassis, 2012; Blick et al., 2016; Joyce et al., 2016; Fukaya and Levine, 2017; Lim et al., 2018; Tian et al., 2019; Galouzis and Prud’homme, 2021). Homolog pairing can drive or silence gene expression through various regulatory elements including Polycomb response elements (PREs), insulators, enhancers, and promoters (Kassis et al., 1991; Fauvarque and Dura, 1993; Kassis, 1994; Gindhart and Kaufman, 1995; Kapoun and Kaufman, 1995; Geyer, 1997; Sigrist and Pirrotta, 1997; Fujioka et al., 1999; Muller et al., 1999; Zhou et al., 1999; Shimell et al., 2000; Duncan, 2002; Kennison and Southworth, 2002; Bantignies et al., 2003; Kravchenko et al., 2005; Vazquez et al., 2006; Fujioka et al., 2009; Li et al., 2011; Kassis, 2012; Blick et al., 2016; Fujioka et al., 2016; Joyce et al., 2016; Fukaya and Levine, 2017; Lim et al., 2018; Piwko et al., 2019; Galouzis and Prud’homme, 2021). Firstly, several specific factors were suggested to regulate pairing (Fritsch et al., 2006; Williams et al., 2007; Hartl et al., 2008), then comprehensive global screens were conducted to identify more factors implicated in somatic pairing (Bateman and Wu, 2008; Bateman et al., 2012b; Joyce et al., 2012). The identification of over one hundred factors that enhance or antagonize pairing indicates a delicate balance between pairing and anti-pairing of homologous chromosomes (Joyce et al., 2012; Joyce et al., 2016). These factors are implicated in key cellular processes such as mitotic cell division, DNA replication, and chromosome organization (Joyce et al., 2012). Interestingly, no zygotic product is required for pairing initiation in embryos (Bateman and Wu, 2008). Furthermore, over 90% of the identified factors are conserved from Drosophila to human (Joyce et al., 2012). In addition to extensive pairing in Dipteran insects such as Drosophila, pairing can also occur transiently at specific loci in mammals [reviewed in Apte and Meller (2012), Joyce et al. (2016)]. Such mammalian pairing has been observed in V(D)J recombination, DNA repair, imprinting, and XCI. In the latter case, pairing seems not to have a main impact on Xist regulation in vitro; whether pairing could influence XCI during development at other stages is still unclear (Barakat et al., 2014; Pollex and Heard, 2019; Loda et al., 2022).
Despite the implications of pairing in a plethora of cellular processes, the detailed structure of homolog pairing, and the global extent of its functional impact on gene regulation have been long-standing questions. Recent applications of advanced imaging technologies including sequential hybridization and super-resolution microscopy have revealed intricate structures of pairing. Specifically, Drosophila cell lines and embryos may include tightly paired regions and well-separated chromatin domains at a few homologous loci (Cattoni et al., 2017; Szabo et al., 2018; Cardozo Gizzi et al., 2019; Mateo et al., 2019). Alternatively, complementary strategies to microscopy, such as Hi-C-based approaches can reveal global and local pairing. For instance, Hi-C reads mapping to the same restriction fragments may facilitate the detection of short-range contacts between homologous chromosomes or sister chromatids (Rowley et al., 2019). Such an approach supports the enrichment of short-range chromosome pairing in active regions (Rowley et al., 2019). On the other hand, simulations that combined Hi-C with lamina-DamID suggest relationships between pairing strength and chromatin states (Li et al., 2017). Despite these predicted relationships, the challenge in distinguishing the homologous maternal and paternal chromosomes hampered the ability to elucidate pairing. Recent studies in Drosophila used haplotype-resolved Hi-C and developed a computational method, Ohm, to accurately distinguish trans contacts between homologous chromosomes from cis contacts within an individual homolog (AlHaj Abed et al., 2019; Erceg et al., 2019). Ohm also allowed for in-depth investigations of pairing ranging from kilobase to megabase scales. Together these studies (AlHaj Abed et al., 2019; Erceg et al., 2019) reveal that pairing is highly structured genome-wide with compartments, domains, and interaction peaks occurring between homologs. Pairing is also remarkably variable and composed of at least two modes; tightly paired regions with small domains alternating with domain boundaries and loosely paired regions with large single domains. Loose pairing is mainly associated with low gene expression and B compartments, while tight pairing may be associated with both lowly and highly transcribed genes, and largely A compartments (AlHaj Abed et al., 2019). Interestingly, most of the previously investigated transvection-related loci and the binding of insulator and architectural proteins (AlHaj Abed et al., 2019; Rowley et al., 2019) coincide with tightly paired regions (AlHaj Abed et al., 2019). Hence, varying structures of homolog pairing including tight and loose pairing in somatic cells can bear functional significance to gene expression. These observations provide unprecedented global connections of pairing structure with gene regulation.
Homolog pairing during early development and differentiation
Since pairing is important for the regulation of gene expression, somatic pairing could be a key step in mediating the acquisition of cellular identities during development. For instance, pairing levels increase dramatically in development (Hiraoka et al., 1993; Fung et al., 1998; Gemkow et al., 1998; Joyce et al., 2013; Erceg et al., 2019). Specifically, this may indicate the role of somatic homolog pairing in the growth and development of organisms. Pairing levels are globally correlated with nascent gene expression and binding of RNA Pol II during zygotic genome activation (Erceg et al., 2019), a key event when the embryonic genome is activated. Depletion of the pioneer factor Zelda, which mediates chromatin accessibility in early embryogenesis, affects local levels of pairing (Erceg et al., 2019). Thus, establishment of homolog pairing is closely intertwined with genome activation and the opening of chromatin, where bringing homologs together may facilitate the formation of functionally compartmentalized inter-chromosomal hubs with concentrated regulatory elements and factors (Strom et al., 2017; Lim et al., 2018; Erceg et al., 2019). On the other hand, spatial segregation of hubs as well as the Rabl orientation of polarized centromeres and telomeres may reduce homolog search space and facilitate pairing (Hiraoka et al., 1993; Fung et al., 1998; Gemkow et al., 1998; Erceg et al., 2019; Child et al., 2021).
In addition to somatic pairing in embryogenesis, pairing can also occur during Drosophila germline stem-cell differentiation preceding meiosis in the adult gonads (McKee, 2004; Christophorou et al., 2013; Joyce et al., 2013; Rubin et al., 2021; Antel et al., 2022). Interestingly, centromere pairing in differentiating mitotic cells prior to meiosis is dependent on the synaptonemal complex components, suggesting that pre-meiotic pairing may not be similar to somatic embryonic pairing (Christophorou et al., 2013; Joyce et al., 2013; Rubin et al., 2021). Differentiating cells also have decreasing levels of Stat92E expression, a factor that plays a role in maintaining stem cell identity (Sheng et al., 2009). The Stat92E locus has tight pairing interactions in germline stem cells (Antel et al., 2022). However, in differentiating gonialblasts the pairing immediately changes to loose, indicating that pairing may act as a “switch.” This “switch” may be dependent on cell specificity and could regulate transcription for a specific locus. Disturbances of the Stat92E pairing status can have an influential impact on its own gene expression, and consequently the differentiation of Drosophila germline (Antel et al., 2022). Another example suggests that changes in pairing levels of Oct4 alleles in the mouse stem cell system are associated with a reduction in Oct4 expression during the transition from pluripotent to differentiated state (Hogan et al., 2015). Together, these observations indicate a potential role of pairing in gene regulation during stem-cell differentiation (Hogan et al., 2015; Antel et al., 2022). In addition, pairing levels (Hiraoka et al., 1993; Dernburg et al., 1996; Fung et al., 1998; Gemkow et al., 1998; Joyce et al., 2013; Erceg et al., 2019) and transvection (Kassis et al., 1991; Bateman et al., 2012a; Mellert and Truman, 2012; Blick et al., 2016) can also vary in different cell types, including during development, where pairing levels may impact the effectiveness of the related transvection in the corresponding tissue (Viets et al., 2019). Thus, variation in levels of homolog pairing may facilitate cell type-specific gene regulation (Figure 2).
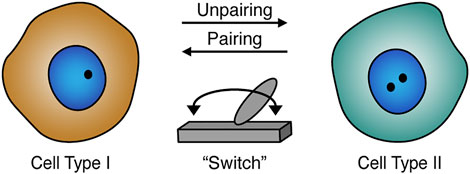
FIGURE 2. Homolog pairing role during development and differentiation. Homolog pairing levels may act as a potential “switch” (gray) that could play a role in cell type-specific gene regulation. The pairing status can also be related to cell differentiation including the formation or maintenance of cellular identities. Schematic of paired (one black dot) or unpaired (two black dots) homologs in the nucleus (blue).
Characteristics of sister chromatid organization
During DNA replication each homolog is replicated to generate a set of sister chromatids (Onn et al., 2008; Yatskevich et al., 2019), which adds another opportunity for interchromosomal interactions (Figure 1B). In Drosophila mechanisms behind homolog pairing, including those mediated by condensin II, could contribute to sister chromatid contacts (Senaratne et al., 2016). Interestingly, super-resolution imaging revealed that contacts between sister chromatids can manifest as distinct chromatin domains similar to homologs (Szabo et al., 2018). Moreover, live-cell and FISH imaging suggest that the separation of sister chromatids is associated with nuclear positioning, chromatin state, and replication timing (Stanyte et al., 2018). However, the global organization of sister chromatids remained largely elusive as sequence identity between sister chromatids presented a challenge for their distinction using typical sequencing-based methods. Recent studies have overcome this challenge by utilizing nucleotide analogs and then either chemical conversion to generate point mutations (Mitter et al., 2020) or Hoechst/ultraviolet treatment to degrade nucleotide-analog-incorporated strand (Oomen et al., 2020) followed by high-throughput sequencing. These approaches enabled detailed inspection of both cis interactions within individual sister chromatids and trans interactions between sister chromatids. Interestingly, in yeast, sister chromatids are precisely aligned at centromeres but display less aligned pairing along chromosome arms (Oomen et al., 2020). Meanwhile, in humans, the trans sister chromatid interactions are highly enhanced at domain boundaries (Mitter et al., 2020). In addition, the presence of trans interactions in domains varies depending on domain size. In smaller domains, which are associated with the Polycomb-repressive chromatin mark H3K27me3, sister chromatids are highly paired, whereas larger unpaired domains generally lack trans contacts and exhibit loose connections (Mitter et al., 2020). These trans sister chromatid interactions at domain boundaries and domains seem reminiscent of tightly and loosely paired regions observed in somatic homolog pairing (AlHaj Abed et al., 2019; Erceg et al., 2019). Nevertheless, distinct pools of cohesin complexes are implicated in global cohesion of aligned sister chromatids and local structuring of domains and boundaries during loop formation (Mitter et al., 2020; Oomen et al., 2020). Surprisingly, components of the cohesin complex are not identified in the screen for factors implicated in somatic homolog pairing (Joyce et al., 2012). These observations suggest that some of the underlying mechanisms for the pairing of sister chromatids and somatic homolog pairing may also differ.
Taken together, elucidating 3D organization of sister chromatids is a key step in understanding the role of sister chromatids in DNA repair, gene expression, and the cell cycle (Mitter et al., 2020; Oomen et al., 2020). More broadly, knowledge of principles of inter-chromosomal organization, including interactions between sister chromatids and those between homologs, will greatly enhance our fundamental understanding of their potential functional implications in diverse cellular processes.
Concluding remarks
Broadly, variability can occur at any level of genome organization as suggested by single-cell omics and microscopy studies (Finn and Misteli, 2019). Single-cell approaches can highlight complex variability that could be missed by population-based approaches. Variability in the genome organization and gene expression of individual cells may provide plasticity in response to various stimuli (Finn and Misteli, 2019). To capture this plasticity, it is important to distinguish not only a handful of genes, but the dynamics of hundreds to thousands of genomic regions integrated with transcriptional activity. This facilitates investigations of individual cell fates within tissue microenvironments in response to developmental cues and disease progression. High-throughput imaging technologies have shown significant strides in bridging the gap from single cells to multicellular tissues using spatial genomics and transcriptomics (Kempfer and Pombo, 2020; Jerkovic and Cavalli, 2021; Rao et al., 2021; Zhao et al., 2022). Spatial-based DNA and RNA approaches integrated with burgeoning multiplexed protein imaging (Hickey et al., 2021; Takei et al., 2021; Ben-Chetrit et al., 2022; Liu et al., 2022; Vickovic et al., 2022) will enhance the understanding of how the dynamics of genome organization and function contribute to cellular identities.
Author contributions
KF, RR, and JE wrote and edited the manuscript.
Funding
RR was supported by a Summer Undergraduate Research Fund (SURF) award. Work in JE’s laboratory was supported by the University of Connecticut.
Acknowledgments
We apologize to the authors whose work could not be included due to space limitations.
Conflict of interest
The authors declare that the research was conducted in the absence of any commercial or financial relationships that could be construed as a potential conflict of interest.
Publisher’s note
All claims expressed in this article are solely those of the authors and do not necessarily represent those of their affiliated organizations, or those of the publisher, the editors and the reviewers. Any product that may be evaluated in this article, or claim that may be made by its manufacturer, is not guaranteed or endorsed by the publisher.
References
Akdemir, K. C., Le, V. T., Chandran, S., Li, Y., Verhaak, R. G., Beroukhim, R., et al. (2020). Disruption of chromatin folding domains by somatic genomic rearrangements in human cancer. Nat. Genet. 52 (3), 294–305. doi:10.1038/s41588-019-0564-y
Alavattam, K. G., Maezawa, S., Sakashita, A., Khoury, H., Barski, A., Kaplan, N., et al. (2019). Attenuated chromatin compartmentalization in meiosis and its maturation in sperm development. Nat. Struct. Mol. Biol. 26 (3), 175–184. doi:10.1038/s41594-019-0189-y
Alexander, J. M., Guan, J., Li, B., Maliskova, L., Song, M., Shen, Y., et al. (2019). Live-cell imaging reveals enhancer-dependent Sox2 transcription in the absence of enhancer proximity. Elife 8, e41769. doi:10.7554/eLife.41769
AlHaj Abed, J., Erceg, J., Goloborodko, A., Nguyen, S. C., McCole, R. B., Saylor, W., et al. (2019). Highly structured homolog pairing reflects functional organization of the Drosophila genome. Nat. Commun. 10 (1), 4485. doi:10.1038/s41467-019-12208-3
Antel, M., Raj, R., Masoud, M. Y. G., Pan, Z., Li, S., Mellone, B. G., et al. (2022). Interchromosomal interaction of homologous Stat92E alleles regulates transcriptional switch during stem-cell differentiation. Nat. Commun. 13 (1), 3981. doi:10.1038/s41467-022-31737-y
Apostolou, E., and Thanos, D. (2008). Virus Infection Induces NF-kappaB-dependent interchromosomal associations mediating monoallelic IFN-beta gene expression. Cell 134 (1), 85–96. doi:10.1016/j.cell.2008.05.052
Apte, M. S., and Meller, V. H. (2012). Homologue pairing in flies and mammals: Gene regulation when two are involved. Genet. Res. Int. 2012, 430587. doi:10.1155/2012/430587
Arrastia, M. V., Jachowicz, J. W., Ollikainen, N., Curtis, M. S., Lai, C., Quinodoz, S. A., et al. (2022). Single-cell measurement of higher-order 3D genome organization with scSPRITE. Nat. Biotechnol. 40 (1), 64–73. doi:10.1038/s41587-021-00998-1
Arsuaga, J., Greulich-Bode, K. M., Vazquez, M., Bruckner, M., Hahnfeldt, P., Brenner, D. J., et al. (2004). Chromosome spatial clustering inferred from radiogenic aberrations. Int. J. Radiat. Biol. 80 (7), 507–515. doi:10.1080/09553000410001723857
Bantignies, F., Grimaud, C., Lavrov, S., Gabut, M., and Cavalli, G. (2003). Inheritance of Polycomb-dependent chromosomal interactions in Drosophila. Genes Dev. 17 (19), 2406–2420. doi:10.1101/gad.269503
Bantignies, F., Roure, V., Comet, I., Leblanc, B., Schuettengruber, B., Bonnet, J., et al. (2011). Polycomb-dependent regulatory contacts between distant Hox loci in Drosophila. Cell 144 (2), 214–226. doi:10.1016/j.cell.2010.12.026
Barakat, T. S., Loos, F., van Staveren, S., Myronova, E., Ghazvini, M., Grootegoed, J. A., et al. (2014). The trans-activator RNF12 and cis-acting elements effectuate X chromosome inactivation independent of X-pairing. Mol. Cell 53 (6), 965–978. doi:10.1016/j.molcel.2014.02.006
Bateman, J. R., Johnson, J. E., and Locke, M. N. (2012a). Comparing enhancer action in cis and in trans. Genetics 191 (4), 1143–1155. doi:10.1534/genetics.112.140954
Bateman, J. R., Larschan, E., D'Souza, R., Marshall, L. S., Dempsey, K. E., Johnson, J. E., et al. (2012b). A genome-wide screen identifies genes that affect somatic homolog pairing in Drosophila. G3 (Bethesda) 2 (7), 731–740. doi:10.1534/g3.112.002840
Bateman, J. R., and Wu, C. T. (2008). A genomewide survey argues that every zygotic gene product is dispensable for the initiation of somatic homolog pairing in Drosophila. Genetics 180 (3), 1329–1342. doi:10.1534/genetics.108.094862
Batut, P. J., Bing, X. Y., Sisco, Z., Raimundo, J., Levo, M., and Levine, M. S. (2022). Genome organization controls transcriptional dynamics during development. Science 375 (6580), 566–570. doi:10.1126/science.abi7178
Ben-Chetrit, N., Niu, X., Swett, A. D., Sotelo, J., Jiao, M. S., Roelli, P., et al. (2022). Integrated protein and transcriptome high-throughput spatial profiling. bioRxiv. [Preprint]. doi:10.1101/2022.03.15.484516
Benabdallah, N. S., Williamson, I., Illingworth, R. S., Kane, L., Boyle, S., Sengupta, D., et al. (2019). Decreased enhancer-promoter proximity accompanying enhancer activation. Mol. Cell 76 (3), 473–484. doi:10.1016/j.molcel.2019.07.038
Bickmore, W. A., and Teague, P. (2002). Influences of chromosome size, gene density and nuclear position on the frequency of constitutional translocations in the human population. Chromosome Res. 10 (8), 707–715. doi:10.1023/a:1021589031769
Blick, A. J., Mayer-Hirshfeld, I., Malibiran, B. R., Cooper, M. A., Martino, P. A., Johnson, J. E., et al. (2016). The capacity to act in trans varies among Drosophila enhancers. Genetics 203 (1), 203–218. doi:10.1534/genetics.115.185645
Bolzer, A., Kreth, G., Solovei, I., Koehler, D., Saracoglu, K., Fauth, C., et al. (2005). Three-dimensional maps of all chromosomes in human male fibroblast nuclei and prometaphase rosettes. PLoS Biol. 3 (5), e157. doi:10.1371/journal.pbio.0030157
Bonev, B., Mendelson Cohen, N., Szabo, Q., Fritsch, L., Papadopoulos, G. L., Lubling, Y., et al. (2017). Multiscale 3D genome rewiring during mouse neural development. Cell 171 (3), 557–572. e524. doi:10.1016/j.cell.2017.09.043
Bonora, G., Deng, X., Fang, H., Ramani, V., Qiu, R., Berletch, J. B., et al. (2018). Orientation-dependent Dxz4 contacts shape the 3D structure of the inactive X chromosome. Nat. Commun. 9 (1), 1445. doi:10.1038/s41467-018-03694-y
Bouwman, B. A. M., Crosetto, N., and Bienko, M. (2022). RNA gradients: Shapers of 3D genome architecture. Curr. Opin. Cell Biol. 74, 7–12. doi:10.1016/j.ceb.2021.12.001
Branco, M. R., and Pombo, A. (2006). Intermingling of chromosome territories in interphase suggests role in translocations and transcription-dependent associations. PLoS Biol. 4 (5), e138. doi:10.1371/journal.pbio.0040138
Canela, A., Maman, Y., Jung, S., Wong, N., Callen, E., Day, A., et al. (2017). Genome organization drives chromosome fragility. Cell 170 (3), 507–521. e518. doi:10.1016/j.cell.2017.06.034
Cardozo Gizzi, A. M., Cattoni, D. I., Fiche, J. B., Espinola, S. M., Gurgo, J., Messina, O., et al. (2019). Microscopy-based chromosome conformation capture enables simultaneous visualization of genome organization and transcription in intact organisms. Mol. Cell 74 (1), 212–222. e215. doi:10.1016/j.molcel.2019.01.011
Casanova, M., Pasternak, M., El Marjou, F., Le Baccon, P., Probst, A. V., and Almouzni, G. (2013). Heterochromatin reorganization during early mouse development requires a single-stranded noncoding transcript. Cell Rep. 4 (6), 1156–1167. doi:10.1016/j.celrep.2013.08.015
Cattoni, D. I., Cardozo Gizzi, A. M., Georgieva, M., Di Stefano, M., Valeri, A., Chamousset, D., et al. (2017). Single-cell absolute contact probability detection reveals chromosomes are organized by multiple low-frequency yet specific interactions. Nat. Commun. 8 (1), 1753. doi:10.1038/s41467-017-01962-x
Child, M. B. t., Bateman, J. R., Jahangiri, A., Reimer, A., Lammers, N. C., Sabouni, N., et al. (2021). Live imaging and biophysical modeling support a button-based mechanism of somatic homolog pairing in Drosophila. Elife 10, e64412. doi:10.7554/eLife.64412
Christophorou, N., Rubin, T., and Huynh, J. R. (2013). Synaptonemal complex components promote centromere pairing in pre-meiotic germ cells. PLoS Genet. 9 (12), e1004012. doi:10.1371/journal.pgen.1004012
Clowney, E. J., LeGros, M. A., Mosley, C. P., Clowney, F. G., Markenskoff-Papadimitriou, E. C., Myllys, M., et al. (2012). Nuclear aggregation of olfactory receptor genes governs their monogenic expression. Cell 151 (4), 724–737. doi:10.1016/j.cell.2012.09.043
Cremer, T., and Cremer, C. (2001). Chromosome territories, nuclear architecture and gene regulation in mammalian cells. Nat. Rev. Genet. 2 (4), 292–301. doi:10.1038/35066075
Darrow, E. M., Huntley, M. H., Dudchenko, O., Stamenova, E. K., Durand, N. C., Sun, Z., et al. (2016). Deletion of DXZ4 on the human inactive X chromosome alters higher-order genome architecture. Proc. Natl. Acad. Sci. U. S. A. 113 (31), E4504–E4512. doi:10.1073/pnas.1609643113
Davies, J. O., Oudelaar, A. M., Higgs, D. R., and Hughes, J. R. (2017). How best to identify chromosomal interactions: A comparison of approaches. Nat. Methods 14 (2), 125–134. doi:10.1038/nmeth.4146
Deng, X., Ma, W., Ramani, V., Hill, A., Yang, F., Ay, F., et al. (2015). Bipartite structure of the inactive mouse X chromosome. Genome Biol. 16, 152. doi:10.1186/s13059-015-0728-8
Dernburg, A. F., Broman, K. W., Fung, J. C., Marshall, W. F., Philips, J., Agard, D. A., et al. (1996). Perturbation of nuclear architecture by long-distance chromosome interactions. Cell 85 (5), 745–759. doi:10.1016/s0092-8674(00)81240-4
Dimitri, P. (2004). Fluorescent in situ hybridization with transposable element probes to mitotic chromosomal heterochromatin of Drosophila. Methods Mol. Biol. 260, 29–39. doi:10.1385/1-59259-755-6:029
Dixon, J. R., Selvaraj, S., Yue, F., Kim, A., Li, Y., Shen, Y., et al. (2012). Topological domains in mammalian genomes identified by analysis of chromatin interactions. Nature 485 (7398), 376–380. nature11082 [pii]. doi:10.1038/nature11082
Dowen, J. M., Fan, Z. P., Hnisz, D., Ren, G., Abraham, B. J., Zhang, L. N., et al. (2014). Control of cell identity genes occurs in insulated neighborhoods in mammalian chromosomes. Cell 159 (2), 374–387. doi:10.1016/j.cell.2014.09.030
Duncan, I. W. (2002). Transvection effects in Drosophila. Annu. Rev. Genet. 36, 521–556. doi:10.1146/annurev.genet.36.060402.100441
Engreitz, J. M., Agarwala, V., and Mirny, L. A. (2012). Three-dimensional genome architecture influences partner selection for chromosomal translocations in human disease. PLoS One 7 (9), e44196. doi:10.1371/journal.pone.0044196
Erceg, J., AlHaj Abed, J., Goloborodko, A., Lajoie, B. R., Fudenberg, G., Abdennur, N., et al. (2019). The genome-wide multi-layered architecture of chromosome pairing in early Drosophila embryos. Nat. Commun. 10 (1), 4486. doi:10.1038/s41467-019-12211-8
Espinola, S. M., Gotz, M., Bellec, M., Messina, O., Fiche, J. B., Houbron, C., et al. (2021). Cis-regulatory chromatin loops arise before TADs and gene activation, and are independent of cell fate during early Drosophila development. Nat. Genet. 53 (4), 477–486. doi:10.1038/s41588-021-00816-z
Evdokimova, V., Gandhi, M., Rayapureddi, J., Stringer, J. R., and Nikiforov, Y. E. (2012). Formation of carcinogenic chromosomal rearrangements in human thyroid cells after induction of double-strand DNA breaks by restriction endonucleases. Endocr. Relat. Cancer 19 (3), 271–281. doi:10.1530/ERC-11-0314
Fauvarque, M. O., and Dura, J. M. (1993). Polyhomeotic regulatory sequences induce developmental regulator-dependent variegation and targeted P-element insertions in Drosophila. Genes Dev. 7 (8), 1508–1520. doi:10.1101/gad.7.8.1508
Finn, E. H., and Misteli, T. (2019). Molecular basis and biological function of variability in spatial genome organization. Science 365 (6457), eaaw9498. doi:10.1126/science.aaw9498
Freire-Pritchett, P., Schoenfelder, S., Varnai, C., Wingett, S. W., Cairns, J., Collier, A. J., et al. (2017). Global reorganisation of cis-regulatory units upon lineage commitment of human embryonic stem cells. Elife 6, e21926. doi:10.7554/eLife.21926
Fritsch, C., Ploeger, G., and Arndt-Jovin, D. J. (2006). Drosophila under the lens: Imaging from chromosomes to whole embryos. Chromosome Res. 14 (4), 451–464. doi:10.1007/s10577-006-1068-z
Fudenberg, G., Imakaev, M., Lu, C., Goloborodko, A., Abdennur, N., and Mirny, L. A. (2016). formation of chromosomal domains by loop extrusion. Cell Rep. 15 (9), 2038–2049. doi:10.1016/j.celrep.2016.04.085
Fujioka, M., Emi-Sarker, Y., Yusibova, G. L., Goto, T., and Jaynes, J. B. (1999). Analysis of an even-skipped rescue transgene reveals both composite and discrete neuronal and early blastoderm enhancers, and multi-stripe positioning by gap gene repressor gradients. Development 126 (11), 2527–2538. doi:10.1242/dev.126.11.2527
Fujioka, M., Mistry, H., Schedl, P., and Jaynes, J. B. (2016). Determinants of chromosome architecture: Insulator pairing in cis and in trans. PLoS Genet. 12 (2), e1005889. doi:10.1371/journal.pgen.1005889
Fujioka, M., Wu, X., and Jaynes, J. B. (2009). A chromatin insulator mediates transgene homing and very long-range enhancer-promoter communication. Development 136 (18), 3077–3087. doi:10.1242/dev.036467
Fukaya, T., and Levine, M. (2017). Transvection. Curr. Biol. 27 (19), R1047–R1049. doi:10.1016/j.cub.2017.08.001
Fung, J. C., Marshall, W. F., Dernburg, A., Agard, D. A., and Sedat, J. W. (1998). Homologous chromosome pairing in Drosophila melanogaster proceeds through multiple independent initiations. J. Cell Biol. 141 (1), 5–20. doi:10.1083/jcb.141.1.5
Galouzis, C. C., and Prud'homme, B. (2021). Transvection regulates the sex-biased expression of a fly X-linked gene. Science 371 (6527), 396–400. doi:10.1126/science.abc2745
Gemkow, M. J., Verveer, P. J., and Arndt-Jovin, D. J. (1998). Homologous association of the bithorax-complex during embryogenesis: Consequences for transvection in Drosophila melanogaster. Development 125 (22), 4541–4552. doi:10.1242/dev.125.22.4541
Geyer, P. K. (1997). The role of insulator elements in defining domains of gene expression. Curr. Opin. Genet. Dev. 7 (2), 242–248. doi:10.1016/s0959-437x(97)80134-7
Ghavi-Helm, Y. (2020). Functional consequences of chromosomal rearrangements on gene expression: Not so deleterious after all? J. Mol. Biol. 432 (3), 665–675. doi:10.1016/j.jmb.2019.09.010
Gindhart, J. G., and Kaufman, T. C. (1995). Identification of Polycomb and trithorax group responsive elements in the regulatory region of the Drosophila homeotic gene Sex combs reduced. Genetics 139 (2), 797–814. doi:10.1093/genetics/139.2.797
Giorgetti, L., Lajoie, B. R., Carter, A. C., Attia, M., Zhan, Y., Xu, J., et al. (2016). Structural organization of the inactive X chromosome in the mouse. Nature 535 (7613), 575–579. doi:10.1038/nature18589
Handel, M. A., and Schimenti, J. C. (2010). Genetics of mammalian meiosis: Regulation, dynamics and impact on fertility. Nat. Rev. Genet. 11 (2), 124–136. doi:10.1038/nrg2723
Handel, M. A. (2004). The XY body: A specialized meiotic chromatin domain. Exp. Cell Res. 296 (1), 57–63. doi:10.1016/j.yexcr.2004.03.008
Hartl, T. A., Smith, H. F., and Bosco, G. (2008). Chromosome alignment and transvection are antagonized by condensin II. Science 322 (5906), 1384–1387. doi:10.1126/science.1164216
Hassold, T., Hall, H., and Hunt, P. (2007). The origin of human aneuploidy: Where we have been, where we are going. Hum. Mol. Genet. 16 (2), R203–R208. doi:10.1093/hmg/ddm243
Heist, T., Fukaya, T., and Levine, M. (2019). Large distances separate coregulated genes in living Drosophila embryos. Proc. Natl. Acad. Sci. U. S. A. 116 (30), 15062–15067. doi:10.1073/pnas.1908962116
Hickey, J. W., Neumann, E. K., Radtke, A. J., Camarillo, J. M., Beuschel, R. T., Albanese, A., et al. (2021). Spatial mapping of protein composition and tissue organization: A primer for multiplexed antibody-based imaging. Nat. Methods 19, 284–295. doi:10.1038/s41592-021-01316-y
Hiraoka, Y., Dernburg, A. F., Parmelee, S. J., Rykowski, M. C., Agard, D. A., and Sedat, J. W. (1993). The onset of homologous chromosome pairing during Drosophila melanogaster embryogenesis. J. Cell Biol. 120 (3), 591–600. doi:10.1083/jcb.120.3.591
Hlatky, L., Sachs, R. K., Vazquez, M., and Cornforth, M. N. (2002). Radiation-induced chromosome aberrations: Insights gained from biophysical modeling. Bioessays 24 (8), 714–723. doi:10.1002/bies.10126
Hnisz, D., Weintraub, A. S., Day, D. S., Valton, A. L., Bak, R. O., Li, C. H., et al. (2016). Activation of proto-oncogenes by disruption of chromosome neighborhoods. Science 351 (6280), 1454–1458. doi:10.1126/science.aad9024
Hogan, M. S., Parfitt, D. E., Zepeda-Mendoza, C. J., Shen, M. M., and Spector, D. L. (2015). Transient pairing of homologous Oct4 alleles accompanies the onset of embryonic stem cell differentiation. Cell Stem Cell 16 (3), 275–288. doi:10.1016/j.stem.2015.02.001
Holley, W. R., Mian, I. S., Park, S. J., Rydberg, B., and Chatterjee, A. (2002). A model for interphase chromosomes and evaluation of radiation-induced aberrations. Radiat. Res. 158 (5), 568–580. doi:10.1667/0033-7587(2002)158[0568:amfica]2.0.co;2
Ichijima, Y., Sin, H. S., and Namekawa, S. H. (2012). Sex chromosome inactivation in germ cells: Emerging roles of DNA damage response pathways. Cell. Mol. Life Sci. 69 (15), 2559–2572. doi:10.1007/s00018-012-0941-5
Ing-Simmons, E., Vaid, R., Bing, X. Y., Levine, M., Mannervik, M., and Vaquerizas, J. M. (2021). Independence of chromatin conformation and gene regulation during Drosophila dorsoventral patterning. Nat. Genet. 53 (4), 487–499. doi:10.1038/s41588-021-00799-x
Jerkovic, I., and Cavalli, G. (2021). Understanding 3D genome organization by multidisciplinary methods. Nat. Rev. Mol. Cell Biol. 22 (8), 511–528. doi:10.1038/s41580-021-00362-w
Ji, X., Dadon, D. B., Powell, B. E., Fan, Z. P., Borges-Rivera, D., Shachar, S., et al. (2016). 3D chromosome regulatory landscape of human pluripotent cells. Cell Stem Cell 18 (2), 262–275. doi:10.1016/j.stem.2015.11.007
Joyce, E. F., Apostolopoulos, N., Beliveau, B. J., and Wu, C. T. (2013). Germline progenitors escape the widespread phenomenon of homolog pairing during Drosophila development. PLoS Genet. 9 (12), e1004013. doi:10.1371/journal.pgen.1004013
Joyce, E. F., Erceg, J., and Wu, C. T. (2016). Pairing and anti-pairing: A balancing act in the diploid genome. Curr. Opin. Genet. Dev. 37, 119–128. doi:10.1016/j.gde.2016.03.002
Joyce, E. F., Williams, B. R., Xie, T., and Wu, C. T. (2012). Identification of genes that promote or antagonize somatic homolog pairing using a high-throughput FISH-based screen. PLoS Genet. 8 (5), e1002667. doi:10.1371/journal.pgen.1002667
Kapoun, A. M., and Kaufman, T. C. (1995). Regulatory regions of the homeotic gene proboscipedia are sensitive to chromosomal pairing. Genetics 140 (2), 643–658. doi:10.1093/genetics/140.2.643
Kassis, J. A. (2012). Transvection in 2012: Site-specific transgenes reveal a plethora of trans-regulatory effects. Genetics 191 (4), 1037–1039. doi:10.1534/genetics.112.142893
Kassis, J. A. (1994). Unusual properties of regulatory DNA from the Drosophila engrailed gene: Three "pairing-sensitive" sites within a 1.6-kb region. Genetics 136 (3), 1025–1038. doi:10.1093/genetics/136.3.1025
Kassis, J. A., VanSickle, E. P., and Sensabaugh, S. M. (1991). A fragment of engrailed regulatory DNA can mediate transvection of the white gene in Drosophila. Genetics 128 (4), 751–761. doi:10.1093/genetics/128.4.751
Keeney, S., Lange, J., and Mohibullah, N. (2014). Self-organization of meiotic recombination initiation: General principles and molecular pathways. Annu. Rev. Genet. 48, 187–214. doi:10.1146/annurev-genet-120213-092304
Kempfer, R., and Pombo, A. (2020). Methods for mapping 3D chromosome architecture. Nat. Rev. Genet. 21 (4), 207–226. doi:10.1038/s41576-019-0195-2
Kennison, J. A., and Southworth, J. W. (2002). Transvection in Drosophila. Adv. Genet. 46, 399–420. doi:10.1016/s0065-2660(02)46014-2
Klein, I. A., Resch, W., Jankovic, M., Oliveira, T., Yamane, A., Nakahashi, H., et al. (2011). Translocation-capture sequencing reveals the extent and nature of chromosomal rearrangements in B lymphocytes. Cell 147 (1), 95–106. doi:10.1016/j.cell.2011.07.048
Kravchenko, E., Savitskaya, E., Kravchuk, O., Parshikov, A., Georgiev, P., and Savitsky, M. (2005). Pairing between gypsy insulators facilitates the enhancer action in trans throughout the Drosophila genome. Mol. Cell. Biol. 25 (21), 9283–9291. doi:10.1128/MCB.25.21.9283-9291.2005
Levo, M., Raimundo, J., Bing, X. Y., Sisco, Z., Batut, P. J., Ryabichko, S., et al. (2022). Transcriptional coupling of distant regulatory genes in living embryos. Nature 605 (7911), 754–760. doi:10.1038/s41586-022-04680-7
Lewis, E. B. (1954). The theory and application of a new method of detecting chromosomal rearrangements in Drosophila melanogaster. Am. Nat. 88, 225–239. doi:10.1086/281833
Li, H. B., Muller, M., Bahechar, I. A., Kyrchanova, O., Ohno, K., Georgiev, P., et al. (2011). Insulators, not Polycomb response elements, are required for long-range interactions between Polycomb targets in Drosophila melanogaster. Mol. Cell. Biol. 31 (4), 616–625. doi:10.1128/MCB.00849-10
Li, L., Lyu, X., Hou, C., Takenaka, N., Nguyen, H. Q., Ong, C. T., et al. (2015). Widespread rearrangement of 3D chromatin organization underlies polycomb-mediated stress-induced silencing. Mol. Cell 58 (2), 216–231. doi:10.1016/j.molcel.2015.02.023
Li, Q., Tjong, H., Li, X., Gong, K., Zhou, X. J., Chiolo, I., et al. (2017). The three-dimensional genome organization of Drosophila melanogaster through data integration. Genome Biol. 18 (1), 145. doi:10.1186/s13059-017-1264-5
Lieberman-Aiden, E., van Berkum, N. L., Williams, L., Imakaev, M., Ragoczy, T., Telling, A., et al. (2009). Comprehensive mapping of long-range interactions reveals folding principles of the human genome. Science 326 (5950), 289–293. doi:10.1126/science.1181369
Lim, B., Heist, T., Levine, M., and Fukaya, T. (2018). Visualization of transvection in living Drosophila embryos. Mol. Cell 70 (2), 287–296. doi:10.1016/j.molcel.2018.02.029
Liu, Y., DiStasio, M., Su, G., Asashima, H., Enninful, A., Qin, X., et al. (2022). Spatial-CITE-seq: Spatially resolved high-plex protein and whole transcriptome co-mapping. bioRxiv. [Preprint]. doi:10.1101/2022.04.01.486788
Loda, A., Collombet, S., and Heard, E. (2022). Gene regulation in time and space during X-chromosome inactivation. Nat. Rev. Mol. Cell Biol. 23, 231–249. doi:10.1038/s41580-021-00438-7
Maass, P. G., Barutcu, A. R., and Rinn, J. L. (2019). Interchromosomal interactions: A genomic love story of kissing chromosomes. J. Cell Biol. 218 (1), 27–38. doi:10.1083/jcb.201806052
Markenscoff-Papadimitriou, E., Allen, W. E., Colquitt, B. M., Goh, T., Murphy, K. K., Monahan, K., et al. (2014). Enhancer interaction networks as a means for singular olfactory receptor expression. Cell 159 (3), 543–557. doi:10.1016/j.cell.2014.09.033
Marlétaz, F., de la Calle-Mustienes, E., Acemel, R. D., Nakamura, T., Paliou, C., Naranjo, S., et al. (2022). The little skate genome and the evolutionary emergence of wing-like fin appendages. bioRxiv. [Preprint]. doi:10.1101/2022.03.21.485123
Mateo, L. J., Murphy, S. E., Hafner, A., Cinquini, I. S., Walker, C. A., and Boettiger, A. N. (2019). Visualizing DNA folding and RNA in embryos at single-cell resolution. Nature 568 (7750), 49–54. doi:10.1038/s41586-019-1035-4
Mayer, W., Smith, A., Fundele, R., and Haaf, T. (2000). Spatial separation of parental genomes in preimplantation mouse embryos. J. Cell Biol. 148 (4), 629–634. doi:10.1083/jcb.148.4.629
McCord, R. P., Kaplan, N., and Giorgetti, L. (2020). Chromosome conformation capture and beyond: Toward an integrative view of chromosome structure and function. Mol. Cell 77 (4), 688–708. doi:10.1016/j.molcel.2019.12.021
McKee, B. D., and Handel, M. A. (1993). Sex chromosomes, recombination, and chromatin conformation. Chromosoma 102 (2), 71–80. doi:10.1007/BF00356023
McKee, B. D. (2004). Homologous pairing and chromosome dynamics in meiosis and mitosis. Biochim. Biophys. Acta 1677 (1-3), 165–180. doi:10.1016/j.bbaexp.2003.11.017
Mellert, D. J., and Truman, J. W. (2012). Transvection is common throughout the Drosophila genome. Genetics 191 (4), 1129–1141. doi:10.1534/genetics.112.140475
Minajigi, A., Froberg, J., Wei, C., Sunwoo, H., Kesner, B., Colognori, D., et al. (2015). A comprehensive Xist interactome reveals cohesin repulsion and an RNA-directed chromosome conformation. Science 349 (6245). doi:10.1126/science.aab2276
Mitter, M., Gasser, C., Takacs, Z., Langer, C. C. H., Tang, W., Jessberger, G., et al. (2020). Conformation of sister chromatids in the replicated human genome. Nature 586 (7827), 139–144. doi:10.1038/s41586-020-2744-4
Monahan, K., Horta, A., and Lomvardas, S. (2019). LHX2- and LDB1-mediated trans interactions regulate olfactory receptor choice. Nature 565 (7740), 448–453. doi:10.1038/s41586-018-0845-0
Muller, H., Scolari, V. F., Agier, N., Piazza, A., Thierry, A., Mercy, G., et al. (2018). Characterizing meiotic chromosomes' structure and pairing using a designer sequence optimized for Hi-C. Mol. Syst. Biol. 14 (7), e8293. doi:10.15252/msb.20188293
Muller, M., Hagstrom, K., Gyurkovics, H., Pirrotta, V., and Schedl, P. (1999). The mcp element from the Drosophila melanogaster bithorax complex mediates long-distance regulatory interactions. Genetics 153 (3), 1333–1356. doi:10.1093/genetics/153.3.1333
Nora, E. P., Goloborodko, A., Valton, A. L., Gibcus, J. H., Uebersohn, A., Abdennur, N., et al. (2017). Targeted degradation of CTCF decouples local insulation of chromosome domains from genomic compartmentalization. Cell 169 (5), 930–944. doi:10.1016/j.cell.2017.05.004
Nora, E. P., Lajoie, B. R., Schulz, E. G., Giorgetti, L., Okamoto, I., Servant, N., et al. (2012). Spatial partitioning of the regulatory landscape of the X-inactivation centre. Nature 485 (7398), 381–385. nature11049 [pii]. doi:10.1038/nature11049
Onn, I., Heidinger-Pauli, J. M., Guacci, V., Unal, E., and Koshland, D. E. (2008). Sister chromatid cohesion: A simple concept with a complex reality. Annu. Rev. Cell Dev. Biol. 24, 105–129. doi:10.1146/annurev.cellbio.24.110707.175350
Oomen, M. E., Hedger, A. K., Watts, J. K., and Dekker, J. (2020). Detecting chromatin interactions between and along sister chromatids with SisterC. Nat. Methods 17 (10), 1002–1009. doi:10.1038/s41592-020-0930-9
Osborne, C. S., Chakalova, L., Brown, K. E., Carter, D., Horton, A., Debrand, E., et al. (2004). Active genes dynamically colocalize to shared sites of ongoing transcription. Nat. Genet. 36 (10), 1065–1071. doi:10.1038/ng1423
Oudelaar, A. M., Beagrie, R. A., Gosden, M., de Ornellas, S., Georgiades, E., Kerry, J., et al. (2020). Dynamics of the 4D genome during in vivo lineage specification and differentiation. Nat. Commun. 11 (1), 2722. doi:10.1038/s41467-020-16598-7
Oudelaar, A. M., and Higgs, D. R. (2021). The relationship between genome structure and function. Nat. Rev. Genet. 22 (3), 154–168. doi:10.1038/s41576-020-00303-x
Palstra, R. J., Tolhuis, B., Splinter, E., Nijmeijer, R., Grosveld, F., and de Laat, W. (2003). The beta-globin nuclear compartment in development and erythroid differentiation. Nat. Genet. 35 (2), 190–194. doi:10.1038/ng1244
Patel, L., Kang, R., Rosenberg, S. C., Qiu, Y., Raviram, R., Chee, S., et al. (2019). Dynamic reorganization of the genome shapes the recombination landscape in meiotic prophase. Nat. Struct. Mol. Biol. 26 (3), 164–174. doi:10.1038/s41594-019-0187-0
Perry, B. W., Schield, D. R., Adams, R. H., and Castoe, T. A. (2021). Microchromosomes exhibit distinct features of vertebrate chromosome structure and function with underappreciated ramifications for genome evolution. Mol. Biol. Evol. 38 (3), 904–910. doi:10.1093/molbev/msaa253
Pirrotta, V. (1999). Transvection and chromosomal trans-interaction effects. Biochim. Biophys. Acta 1424 (1), M1–M8. doi:10.1016/s0304-419x(99)00019-0
Piwko, P., Vitsaki, I., Livadaras, I., and Delidakis, C. (2019). The role of insulators in transgene transvection in Drosophila. Genetics 212 (2), 489–508. doi:10.1534/genetics.119.302165
Pollex, T., and Heard, E. (2019). Nuclear positioning and pairing of X-chromosome inactivation centers are not primary determinants during initiation of random X-inactivation. Nat. Genet. 51 (2), 285–295. doi:10.1038/s41588-018-0305-7
Quinodoz, S. A., Jachowicz, J. W., Bhat, P., Ollikainen, N., Banerjee, A. K., Goronzy, I. N., et al. (2021). RNA promotes the formation of spatial compartments in the nucleus. Cell 184 (23), 5775–5790.e30. e5730. doi:10.1016/j.cell.2021.10.014
Quinodoz, S. A., Ollikainen, N., Tabak, B., Palla, A., Schmidt, J. M., Detmar, E., et al. (2018). Higher-Order inter-chromosomal hubs shape 3D genome organization in the nucleus. Cell 174 (3), 744–757. doi:10.1016/j.cell.2018.05.024
Rao, A., Barkley, D., Franca, G. S., and Yanai, I. (2021). Exploring tissue architecture using spatial transcriptomics. Nature 596 (7871), 211–220. doi:10.1038/s41586-021-03634-9
Rao, S. S., Huntley, M. H., Durand, N. C., Stamenova, E. K., Bochkov, I. D., Robinson, J. T., et al. (2014). A 3D map of the human genome at kilobase resolution reveals principles of chromatin looping. Cell 159 (7), 1665–1680. doi:10.1016/j.cell.2014.11.021
Rao, S. S. P., Huang, S. C., Glenn St Hilaire, B., Engreitz, J. M., Perez, E. M., Kieffer-Kwon, K. R., et al. (2017). Cohesin loss eliminates all loop domains. Cell 171 (2), 305–320. doi:10.1016/j.cell.2017.09.026
Reed, K. S. M., Davis, E. S., Bond, M. L., Cabrera, A., Thulson, E., Quiroga, I. Y., et al. (2022). Temporal analysis suggests a reciprocal relationship between 3D chromatin structure and transcription. bioRxiv. [Preprint]. doi:10.1101/2022.05.05.490836
Roix, J. J., McQueen, P. G., Munson, P. J., Parada, L. A., and Misteli, T. (2003). Spatial proximity of translocation-prone gene loci in human lymphomas. Nat. Genet. 34 (3), 287–291. doi:10.1038/ng1177
Rosin, L. F., Crocker, O., Isenhart, R. L., Nguyen, S. C., Xu, Z., and Joyce, E. F. (2019). Chromosome territory formation attenuates the translocation potential of cells. Elife 8, e49553. doi:10.7554/eLife.49553
Rosin, L. F., Nguyen, S. C., and Joyce, E. F. (2018). Condensin II drives large-scale folding and spatial partitioning of interphase chromosomes in Drosophila nuclei. PLoS Genet. 14 (7), e1007393. doi:10.1371/journal.pgen.1007393
Roukos, V., Burman, B., and Misteli, T. (2013). The cellular etiology of chromosome translocations. Curr. Opin. Cell Biol. 25 (3), 357–364. doi:10.1016/j.ceb.2013.02.015
Rowley, M. J., Lyu, X., Rana, V., Ando-Kuri, M., Karns, R., Bosco, G., et al. (2019). Condensin II counteracts cohesin and RNA polymerase II in the establishment of 3D chromatin organization. Cell Rep. 26 (11), 2890–2903. doi:10.1016/j.celrep.2019.01.116
Rubin, T., Macaisne, N., Vallés, A. M., Guilleman, C., Gaugué, I., and Huynh, J. R. (2021). Pre-meiotic pairing of homologous chromosomes during Drosophila male meiosis. bioRxiv. [Preprint]. doi:10.1101/2021.12.07.471586
Sabari, B. R., Dall'Agnese, A., and Young, R. A. (2020). Biomolecular condensates in the nucleus. Trends biochem. Sci. 45 (11), 961–977. doi:10.1016/j.tibs.2020.06.007
Sanborn, A. L., Rao, S. S., Huang, S. C., Durand, N. C., Huntley, M. H., Jewett, A. I., et al. (2015). Chromatin extrusion explains key features of loop and domain formation in wild-type and engineered genomes. Proc. Natl. Acad. Sci. U. S. A. 112 (47), E6456–E6465. doi:10.1073/pnas.1518552112
Schalbetter, S. A., Fudenberg, G., Baxter, J., Pollard, K. S., and Neale, M. J. (2019). Principles of meiotic chromosome assembly revealed in S. cerevisiae. Nat. Commun. 10 (1), 4795. doi:10.1038/s41467-019-12629-0
Schoenfelder, S., Sexton, T., Chakalova, L., Cope, N. F., Horton, A., Andrews, S., et al. (2010). Preferential associations between co-regulated genes reveal a transcriptional interactome in erythroid cells. Nat. Genet. 42 (1), 53–61. doi:10.1038/ng.496
Schwarzer, W., Abdennur, N., Goloborodko, A., Pekowska, A., Fudenberg, G., Loe-Mie, Y., et al. (2017). Two independent modes of chromatin organization revealed by cohesin removal. Nature 551 (7678), 51–56. doi:10.1038/nature24281
Senaratne, T. N., Joyce, E. F., Nguyen, S. C., and Wu, C. T. (2016). Investigating the interplay between sister chromatid cohesion and homolog pairing in Drosophila nuclei. PLoS Genet. 12 (8), e1006169. doi:10.1371/journal.pgen.1006169
Sexton, T., Yaffe, E., Kenigsberg, E., Bantignies, F., Leblanc, B., Hoichman, M., et al. (2012). Three-dimensional folding and functional organization principles of the Drosophila genome. Cell 148 (3), 458–472. doi:10.1016/j.cell.2012.01.010
Sheng, X. R., Posenau, T., Gumulak-Smith, J. J., Matunis, E., Van Doren, M., and Wawersik, M. (2009). Jak-STAT regulation of male germline stem cell establishment during Drosophila embryogenesis. Dev. Biol. 334 (2), 335–344. doi:10.1016/j.ydbio.2009.07.031
Shimell, M. J., Peterson, A. J., Burr, J., Simon, J. A., and O'Connor, M. B. (2000). Functional analysis of repressor binding sites in the iab-2 regulatory region of the abdominal-A homeotic gene. Dev. Biol. 218 (1), 38–52. doi:10.1006/dbio.1999.9576
Sigrist, C. J., and Pirrotta, V. (1997). Chromatin insulator elements block the silencing of a target gene by the Drosophila polycomb response element (PRE) but allow trans interactions between PREs on different chromosomes. Genetics 147 (1), 209–221. doi:10.1093/genetics/147.1.209
Spielmann, M., Lupianez, D. G., and Mundlos, S. (2018). Structural variation in the 3D genome. Nat. Rev. Genet. 19 (7), 453–467. doi:10.1038/s41576-018-0007-0
Spilianakis, C. G., and Flavell, R. A. (2006). Molecular biology. Managing associations between different chromosomes. Science 312 (5771), 207–208. doi:10.1126/science.1126689
Stadler, M. R., Haines, J. E., and Eisen, M. B. (2017). Convergence of topological domain boundaries, insulators, and polytene interbands revealed by high-resolution mapping of chromatin contacts in the early Drosophila melanogaster embryo. Elife 6, e29550. doi:10.7554/eLife.29550
Stanyte, R., Nuebler, J., Blaukopf, C., Hoefler, R., Stocsits, R., Peters, J. M., et al. (2018). Dynamics of sister chromatid resolution during cell cycle progression. J. Cell Biol. 217 (6), 1985–2004. doi:10.1083/jcb.201801157
Stevens, N. M. (1908). A study of the germ cells of certain Diptera, with reference to the heterochromosomes and the phenomena of synapsis. J. Exp. Zool. 5, 359–374. doi:10.1002/jez.1400050304
Strom, A. R., Emelyanov, A. V., Mir, M., Fyodorov, D. V., Darzacq, X., and Karpen, G. H. (2017). Phase separation drives heterochromatin domain formation. Nature 547 (7662), 241–245. doi:10.1038/nature22989
Szabo, Q., Jost, D., Chang, J. M., Cattoni, D. I., Papadopoulos, G. L., Bonev, B., et al. (2018). TADs are 3D structural units of higher-order chromosome organization in Drosophila. Sci. Adv. 4 (2), eaar8082. doi:10.1126/sciadv.aar8082
Takei, Y., Yun, J., Zheng, S., Ollikainen, N., Pierson, N., White, J., et al. (2021). Integrated spatial genomics reveals global architecture of single nuclei. Nature 590 (7845), 344–350. doi:10.1038/s41586-020-03126-2
Tanabe, H., Habermann, F. A., Solovei, I., Cremer, M., and Cremer, T. (2002). Non-random radial arrangements of interphase chromosome territories: Evolutionary considerations and functional implications. Mutat. Res. 504 (1-2), 37–45. doi:10.1016/s0027-5107(02)00077-5
Tian, K., Henderson, R. E., Parker, R., Brown, A., Johnson, J. E., and Bateman, J. R. (2019). Two modes of transvection at the eyes absent gene of Drosophila demonstrate plasticity in transcriptional regulatory interactions in cis and in trans. PLoS Genet. 15 (5), e1008152. doi:10.1371/journal.pgen.1008152
Turner, J. M. (2007). Meiotic sex chromosome inactivation. Development 134 (10), 1823–1831. doi:10.1242/dev.000018
van Steensel, B., and Furlong, E. E. M. (2019). The role of transcription in shaping the spatial organization of the genome. Nat. Rev. Mol. Cell Biol. 20 (6), 327–337. doi:10.1038/s41580-019-0114-6
Vazquez, J., Muller, M., Pirrotta, V., and Sedat, J. W. (2006). The Mcp element mediates stable long-range chromosome-chromosome interactions in Drosophila. Mol. Biol. Cell 17 (5), 2158–2165. doi:10.1091/mbc.e06-01-0049
Vernimmen, D., De Gobbi, M., Sloane-Stanley, J. A., Wood, W. G., and Higgs, D. R. (2007). Long-range chromosomal interactions regulate the timing of the transition between poised and active gene expression. EMBO J. 26 (8), 2041–2051. doi:10.1038/sj.emboj.7601654
Vickovic, S., Lotstedt, B., Klughammer, J., Mages, S., Segerstolpe, A., Rozenblatt-Rosen, O., et al. (2022). SM-Omics is an automated platform for high-throughput spatial multi-omics. Nat. Commun. 13 (1), 795. doi:10.1038/s41467-022-28445-y
Viets, K., Sauria, M. E. G., Chernoff, C., Rodriguez Viales, R., Echterling, M., Anderson, C., et al. (2019). Characterization of button loci that promote homologous chromosome pairing and cell-type-specific interchromosomal gene regulation. Dev. Cell 51 (3), 341–356. doi:10.1016/j.devcel.2019.09.007
Wang, Y., Wang, H., Zhang, Y., Du, Z., Si, W., Fan, S., et al. (2019). Reprogramming of meiotic chromatin architecture during spermatogenesis. Mol. Cell 73 (3), 547–561. e546. doi:10.1016/j.molcel.2018.11.019
Williams, B. R., Bateman, J. R., Novikov, N. D., and Wu, C. T. (2007). Disruption of topoisomerase II perturbs pairing in drosophila cell culture. Genetics 177 (1), 31–46. doi:10.1534/genetics.107.076356
Winick-Ng, W., Kukalev, A., Harabula, I., Zea-Redondo, L., Szabo, D., Meijer, M., et al. (2021). Cell-type specialization is encoded by specific chromatin topologies. Nature 599 (7886), 684–691. doi:10.1038/s41586-021-04081-2
Wu, C. T., and Morris, J. R. (1999). Transvection and other homology effects. Curr. Opin. Genet. Dev. 9 (2), 237–246. doi:10.1016/S0959-437X(99)80035-5
Yatskevich, S., Rhodes, J., and Nasmyth, K. (2019). Organization of chromosomal DNA by SMC complexes. Annu. Rev. Genet. 53, 445–482. doi:10.1146/annurev-genet-112618-043633
Zhang, Y., McCord, R. P., Ho, Y. J., Lajoie, B. R., Hildebrand, D. G., Simon, A. C., et al. (2012). Spatial organization of the mouse genome and its role in recurrent chromosomal translocations. Cell 148 (5), 908–921. doi:10.1016/j.cell.2012.02.002
Zhao, T., Chiang, Z. D., Morriss, J. W., LaFave, L. M., Murray, E. M., Del Priore, I., et al. (2022). Spatial genomics enables multi-modal study of clonal heterogeneity in tissues. Nature 601 (7891), 85–91. doi:10.1038/s41586-021-04217-4
Zhao, Z., Tavoosidana, G., Sjolinder, M., Gondor, A., Mariano, P., Wang, S., et al. (2006). Circular chromosome conformation capture (4C) uncovers extensive networks of epigenetically regulated intra- and interchromosomal interactions. Nat. Genet. 38 (11), 1341–1347. doi:10.1038/ng1891
Zhou, J., Ashe, H., Burks, C., and Levine, M. (1999). Characterization of the transvection mediating region of the abdominal-B locus in Drosophila. Development 126 (14), 3057–3065. doi:10.1242/dev.126.14.3057
Zickler, D., and Kleckner, N. (1999). Meiotic chromosomes: Integrating structure and function. Annu. Rev. Genet. 33, 603–754. doi:10.1146/annurev.genet.33.1.603
Keywords: 3D genome organization, gene regulation, intra-chromosomal contacts, inter-chromosomal contacts, meiotic chromosomes, homolog pairing, sister chromatids, development
Citation: Fleck K, Raj R and Erceg J (2022) The 3D genome landscape: Diverse chromosomal interactions and their functional implications. Front. Cell Dev. Biol. 10:968145. doi: 10.3389/fcell.2022.968145
Received: 13 June 2022; Accepted: 19 July 2022;
Published: 11 August 2022.
Edited by:
Claire Rougeulle, UMR7216 Epigénétique et Destin Cellulaire, FranceReviewed by:
Mario Zurita, UNAM Campus Morelos, National Autonomous University of Mexico, MexicoCopyright © 2022 Fleck, Raj and Erceg. This is an open-access article distributed under the terms of the Creative Commons Attribution License (CC BY). The use, distribution or reproduction in other forums is permitted, provided the original author(s) and the copyright owner(s) are credited and that the original publication in this journal is cited, in accordance with accepted academic practice. No use, distribution or reproduction is permitted which does not comply with these terms.
*Correspondence: Jelena Erceg, amVsZW5hLmVyY2VnQHVjb25uLmVkdQ==
†These authors have contributed equally to this work