- 1Institute of Geriatrics, Xiyuan Hospital, China Academy of Chinese Medical Sciences, Beijing, China
- 2Beijing University of Chinese Medicine, Beijing, China
- 3Wangjing Hospital, China Academy of Chinese Medical Sciences, Beijing, China
Synaptic dysfunction is closely related to Alzheimer’s disease (AD) which is also recognized as synaptic disorder. β-amyloid (Aβ) is one of the main pathogenic factors in AD, which disrupts synaptic plasticity and mediates the synaptic toxicity through different mechanisms. Aβ disrupts glutamate receptors, such as NMDA and AMPA receptors, which mediates calcium dyshomeostasis and damages synapse plasticity characterized by long-term potentiation (LTP) suppression and long-term depression (LTD) enhancement. As Aβ stimulates and Ca2+ influx, microglial cells and astrocyte can be activated and release cytokines, which reduces glutamate uptake and further impair synapse function. Besides, extracellular glutamate accumulation induced by Aβ mediates synapse toxicity resulting from reduced glutamate receptors and glutamate spillovers. Aβ also mediates synaptic dysfunction by acting on various signaling pathways and molecular targets, disrupting mitochondria and energy metabolism. In addition, Aβ overdeposition aggravates the toxic damage of hyperphosphorylated tau to synapses. Synaptic dysfunction plays a critical role in cognitive impairment of AD. The review addresses the possible mechanisms by which Aβ mediates AD-related synaptic impairment from distant perspectives.
Introduction
AD is currently considered as a disease of synaptic failure (Babri et al., 2012), which is closely related to β-amyloid (Aβ) deposition and tau hyperphosphorylation (Kopeikina et al., 2012; Breijyeh and Karaman, 2020, Author Anonymous, 2020). Synapses are the locus where information is transferred from a pre-to a postsynaptic neuron (Bellot et al., 2014), which is largely mediated by neurotransmitters that are released by the presynaptic axon terminals and then bind to receptors on the postsynaptic dendritic spines (Raven et al., 2018). The regulation and alteration of synaptic function are generally recognized as synaptic plasticity. Once synaptic plasticity impaired, the synaptic connections and neural communication were disrupted, ultimately causing brain dysfunction at large. For example, the processing and storage of information result from external stimuli were dysfunctional (Zucker and Regehr, 2002; Cooke and Bliss, 2006; Raven et al., 2018). Long-term potentiation (LTP) and long-term depression (LTD) are the two manifestations of synaptic plasticity (Yuste and Bonhoeffer, 2001; De Roo et al., 2008), which are recognized as the biological basis of learning and memory activities at the cellular level (Selkoe, 2002; Yun et al., 2006). LTP and LTD are mainly regulated by N-methyl-D-aspartate receptors (NMDARs) and α-amino-3-hydroxy-5-methyl-4-isoxazolepropionic acid receptor (AMPARs). NMDARs and AMPARs are cationic channels gated by the neurotransmitter glutamate that plays a critical role in excitatory synaptic transmission and synaptic plasticity in the central nervous system (CNS). Under physiological conditions, NMDARs and AMPARs are relevant for LTP and LTD, both of them constitute molecular key mechanisms of hippocampal learning and memory (Cooke and Bliss, 2006). Aβ, a potent neurotoxic peptide, can activate NMDARs to increase excessive inflow of calcium ions (Ca2+) and trigger the internalization of NMDARs and AMPARs to suppress LTP and facilitates LTD (O'Riordan et al., 2018; Shankar et al., 2008; Findley et al., 2019), ultimately leading to synapse toxicity and learning-memory deficits seen in AD (Wei J. et al., 2010; Jo et al., 2011; Bergström et al., 2016). One study demonstrated that abnormal synaptic transmission and impaired long-term potentiation (LTP) were often well associated with Aβ plaque formation in the transgenic mouse (Roberson et al., 2011). Similarly, it is reported that Aβ and hyperphosphorylated tau play a synergistic role in NMDAR-mediated synaptotoxicity, the excessive formation of reactive oxygen species (ROS) and oxidative stress (De Felice et al., 2007; Bezprozvanny and Mattson, 2008; Kamat et al., 2016). Recent studies proved that NMDAR activation, excessive Ca2+ influxes, and free radical generation are closely related to synaptic dysfunction and tau phosphorylation (Kamat et al., 2013; Rai et al., 2013). As Aβ is an important participant in the pathogenesis of AD, which damages the synaptic plasticity and mediates the synaptic excitotoxicity via different mechanisms (Babri et al., 2012). However, the series of events that impair the synaptic function induced by Aβ still under debate. Therefore, in this review, we elaborated the role of Aβ in the synaptic dysfunction related to AD, hoping to find new therapeutic targets for AD through a further insights into the pathological mechanism of AD.
Aβ can disrupt glutamate receptors to damage synapse plasticity
Aβ is one of the main pathological factors of AD (Knobloch et al., 2007; Tu et al., 2014; Herrup, 2015). Aβ can not only damage synaptic function via its own internalization and intracellular accumulation but also disrupt synaptic glutamatergic receptors, NMDA and AMPA receptors, which mediate synaptic plasticity impairment in the early stage of AD (Ripoli et al., 2014). Aβ oligomers can activate NMDA and AMPA receptors to disrupt calcium homeostasis, which interference with the main forms of synaptic plasticity, LTP and LTD (Takahashi et al., 2002; Tu et al., 2014) (Figure 1). Studies indicated that Aβ oligomers could directly or indirectly act on NMDA and AMPA receptors, dysregulate their activity, disrupt calcium influx, which resulted in LTP suppression and LTD enhancement (Shankar et al., 2007; Shankar et al., 2008; Tu et al., 2014). Many synaptic membrane receptors are the major sites of Aβ toxicity, especially mGluR and NMDARs (Ittner and Götz, 2011; Yang et al., 2018). The dysregulation of NMDARs induced by Aβ is more prominent in synaptic plasticity damage. Excessive or inappropriate activation of NMDARs mediated by Aβ can inhibit LTP (Coan et al., 1989). However, synaptic and extrasynaptic NMDARs activation have different consequences on synaptic plasticity, gene regulation and neuronal death (Léveillé et al., 2008; Li et al., 2011). Aβ oligomers can not only downregulate the synaptic NMDARs function via increasing the endocytosis of NMDARs, but also activate extrasynaptic NMDARs to inhibit LTP (Hu et al., 2017). The activation of synaptic NMDARs reduces Aβ generation, while the activated extrasynaptic NMDARs increases Aβ production. One study showed that soluble Aβ1-42, a toxic peptide, which significantly increased the extrasynaptic NMDA response and Ca2+ inflow through extrasynaptic NR2B-containing NMDARs (Zhou et al., 2010; Yang et al., 2018), ultimately impaired spatial cognitive function and inhibited LTP (Zhou et al., 2010). Extrasynaptic NR2B-containing NMDA receptor might be a target which mediates the early neuronal dysfunction induced by soluble Aβ1-42 (Sinor et al., 2000). Aβ1-42 can accelerate the phosphorylation of GluN2B subunits tyrosine and trigger an ifenprodil-sensitive transient activation of Akt (Abbott et al., 2008). Akt Activation mediates the phosphorylation of glycogen synthase kinase-3β (GSK-3β), which obviously interferes with the induction of synaptic plasticity, including LTP (Figure 1) (Hooper et al., 2007; Peineau et al., 2007). However, LTP suppression mediated by soluble Aβ1-42 was mainly attributed to the overactivation of extrasynaptic NR2B-containing NMDA receptors, which further disrupted calcium homeostasis and damaged synaptic plasticity (Li et al., 2011). Besides, another study showed that LTP inhibition was more affected by Ca2+ influx induced by the activation of GluN2A-rather than GluN2B-containing NMDARs (Izumi et al., 2006; Hu et al., 2009). Aβ may act on multiple subunits of NMDARs, including NR2A and NR2B. Furthermore, NMDARs overactivation and LTP suppression induced by Aβ may be a vicious circle, which exacerbated the damage of synaptic plasticity.
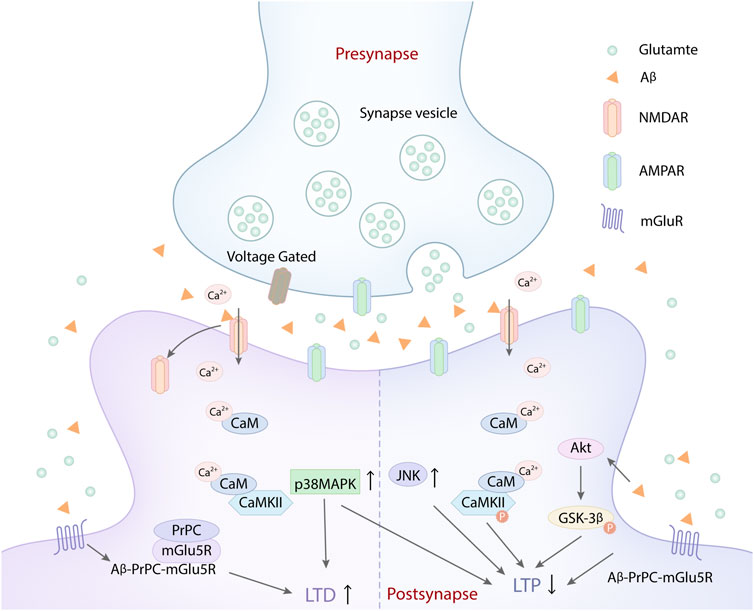
FIGURE 1. Aβ disrupts synapse plasticity by acting on glutamate receptors. Aβ activated NMDARs and increased Ca2+ influx, which leaded to intracellular calcium overload and suppressed the LTP. Besides, the glutamate released from synapse vesicle in presynapse also increased the activation of NMDARs and AMPARs, which aggravated the intracellular Ca2+ overload, further inhibiting the LTP. Aβ peptide triggered the rapid activation of Ca2+ and CaMKІІ. The aberrant activation of CaMKІІ causes deficits in LTP, leading to synaptic loss via the destabilization of AMPARs. Similarly, Aβ could selectively impair glutamatergic synaptic transmission, LTP and LTD expression by activating p38 MAPK and JNK. At the same time, the aberrant activation of Akt induced byAβ can promote the phosphorylation of GSK-3β, which also inhibited LTP. In addition, Aβ assemblies acted on cellular prion protein (PrPC) and mGlu5R, which induced the formation of the complex of Aβ-PrPC-mGlu5R triad at the postsynaptic density. The complex formation caused the mGlu5R-dependent LTD enhancement and NMDAR-dependent LTP inhibition, which exacerbated the damage of synaptic plasticity.
In addition, one study demonstrated that oligomeric and synaptotoxic forms of the Aβ peptide could trigger the rapid activation of Ca2+ and calmodulin-dependent protein kinase ІІ (CaMKІІ) (Figure 1). The aberrant activation of CaMKІІ causes deficits in LTP, leading to synaptic loss via the destabilization of AMPARs (Takumi et al., 1999). It is well known that the phosphorylation of GluR1-containing AMPARs at a CaMKІІ-dependent site could facilitate LTP generation (Lisman et al., 2002). Aβ-induced synaptic dysfunction is associated with the removal of synaptic AMPARs (Zhao et al., 2004; Gu et al., 2009). Therefore, oligomeric Aβ can impair synapse plasticity by disrupting glutamatergic receptors and calcium homeostasis (Reinders et al., 2016; Arbel-Ornath et al., 2017; Liu et al., 2019). However, the effect of oligomeric Aβ on glutamatergic receptors may be bidirectional. Similarly, the effect of Aβ on synaptic plasticity was concentration-dependent (Falcicchia et al., 2020). At physiological levels, Aβ selectively enhances NMDAR-mediated currents and synaptic transmission (Kelly and Ferreira, 2006; Domingues et al., 2007). Brief periods of high synaptic activity open NMDARs, increasing postsynaptic AMPARs, spine growth, and LTP of synaptic transmission (Hayashi et al., 2000; Kopec et al., 2006). While increased Aβ application promotes endocytosis of NMDARs in cortical neurons and produces a rapid and persistent depression of NMDA-evoked currents in cortical neurons via activating nAChRs (Snyder et al., 2005). Under pathological condition, high concentrations of Aβ can enhance the activation of NMDARs (Kawamoto et al., 2008) and cause NMDAR agonist-induced delayed cognitive dysfunction (Nakamura et al., 2006). Low concentration of Aβ can selectively impair glutamatergic synaptic transmission, LTP and LTD expression by activating p38 MAPK and JNK (Origlia et al., 2008; Origlia et al., 2010; Falcicchia et al., 2020) (Figure 1). Thus it can be seen that increased Aβ production increases NMDAR activation, and increased NMDAR activation in turn increases Aβ production within limits. Once the homeostatic balance between NMDA activation and Aβ production is broken, NMDAR overactivation or Aβ deposition both can damage synaptic plasticity, ultimately leading to cognitive impairment in AD (Parihar and Brewer, 2010). Additionally, low-dose Aβ can accelerate the generation of LTD mediated by metabotropic glutamate-5 receptors (mGlu5Rs). mGlu5Rs is a primary glutamate receptor subtype, which also participates in LTP inhibition induced by Aβ (Hu et al., 2014). Besides, certain synaptotoxic Aβ assemblies act on cellular prion protein (PrPC), which is involved in LTD generation mediated by Aβ (Laurén et al., 2009; Nicoll et al., 2013). Aβ, PrPC and mGlu5R can form the complex of Aβ-PrPC-mGlu5R triad at the postsynaptic density. Aβ-PrPC-mGlu5R triad not only enhances mGlu5R-dependent LTD, but also inhibits NMDAR-dependent LTP (Figure 1), which exacerbates the damage of synaptic plasticity (Um et al., 2013). Therefore, targeting Aβ-PrPC-mGlu5R triad may provide a new direction for preventing synaptic plasticity damage in early AD.
Aβ can destroy glutamate cycle to induce synapse toxicity
Aβ oligomers can induce extracellular glutamate accumulation, which destroys the glutamate cycle and results in the synapse toxicity (Li and Selkoe, 2020). The extracellular glutamate accumulation mainly blames on Aβ-induced dysregulation of two mechanisms. On the one hand, Aβ can reduce neuronal glutamate uptake by triggering the rapid glutamate transporter mislocalization and internalization in astrocytes (Matos et al., 2008; Li et al., 2009; Matos et al., 2012; Lanznaster et al., 2017), which decreases glutamate clearance (Scimemi et al., 2013; Yang et al., 2018). On the other hand, Aβ can increase the glutamate spillover through α7 nicotinic acetylcholine receptors (a7nAChR) (Kabogo et al., 2010; Hascup and Hascup, 2016), which excessively activates extrasynaptic NMDARs, subsequently resulting in neurotoxicity (Varga et al., 2014). Studies have demonstrated that Aβ oligomers could facilitate the release of glutamate by activating astrocytes through a7nAChR, thereby enhancing the activation of extracsynaptic NMDARs (Talantova et al., 2013) (Figure 2). Similarly, the aggregation of extracellular glutamate induced by Aβ is recognized as a detrimental upstream factor, which can also cause the overactivation of extrasynaptic NMDARs, ultimately leading to LTP impairment, LTD enhancement, and synapse loss (Huang et al., 2018). In addition, Aβ oligomers can combine with the fibronectin repeats domain of EphB2 and trigger EphB2 degradation in the proteasome. EphB2 can regulate the synaptic localization of NMDARs, which mediates tyrosine phosphorylation of GluN2B at Y1472 and stabilizes NMDARs on the cell surface and thereby enhances the response of NMDARs. GluN2B phosphorylation at Y1472 is important for GluN1/GluN2B trafficking to the cell member, which plays an important role in NMDA receptor-dependent synaptic plasticity (Salter and Kalia, 2004; Shi et al., 2016). Aβ oligomers can inhibit GluN2B phosphorylation and the surface expression of GluN2B via meidating EphB2 degradation in the proteasome (Hu et al., 2017). At the same time, Aβ oligomers can accelerate NMDARs endocytosis result from reducing GluN2B phosphorylation. It can also activate extrasynaptic NMDARs to inhibit LTP (Hu et al., 2017). Besides, Aβ can decrease the surface expression of GluN1 in cortical neurons by activating a7nAChR and the tyrosine phosphatase STEP (Snyder et al., 2005).
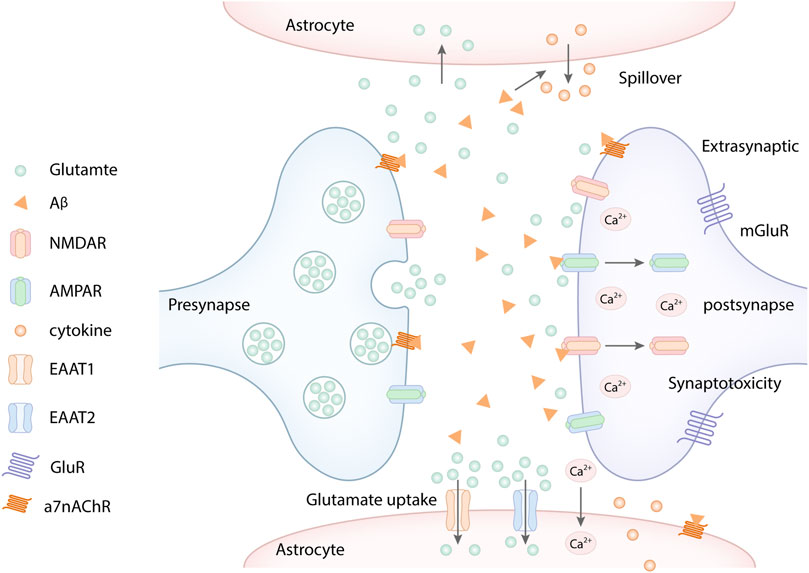
FIGURE 2. Aβ promotes the extracellular glutamate aggregation. Aβ increased the glutamate spillover through α7 nicotinic acetylcholine receptors (a7nAChR), which excessively activates extrasynaptic NMDARs, subsequently resulting in Ca2+ influx. Similarly, Aβ overexpression mediated the endocytosis of NMDA and AMPA receptors, which further increased the extracellular glutamate aggregation. In addition, Aβ reduced neuronal glutamate uptake by decreasing glutamate transporter EAAT1 and EAAT2 in astrocytes, which decreases glutamate clearance. Besides, Aβ oligomers can activate astrocytes, which released pro-inflammatory cytokines and inhibited the ability of glutamate uptake of astrocytes.
Some studies have proved that oligomeric and synaptotoxic forms of the Aβ peptide induced aberrant activation of CaMKII, which leaded to deficits in LTP, ultimately resulting in synaptic loss via the destabilization of AMPARs (Lisman et al., 2002). It is well known that excitatory synapses contain AMPA and NMDA ionotropic glutamate receptors as well as metabotropic type glutamate receptors (mGluRs) positioned on dendritic spines (Snyder et al., 2005; Hsieh et al., 2006). Aβ-induced synaptic dysfunction plays a critical role in the synaptic removal of AMPARs (Gu et al., 2009). In addition, Aβ overexpression can mediate the endocytosis of NMDA and AMPA receptors (Figure 2), which inhibits NMDA and AMPA receptor-mediated synaptic transmission, leading to synapse toxicity (Snyder et al., 2005; Hsieh et al., 2006; Yang et al., 2018). One study showed that Aβ1-42 preferentially binds to glutamatergic neurons expressing NR1 or NR2B-containing NMDA receptors compared with other subunits (Lacor et al., 2007). The decreased NMDARs and AMPARs induced the glutamate aggregation, which in turn increased the endocytosis and decreased surface expression of NR1 and NR2B (Snyder et al., 2005; Findley et al., 2019). Similarly, Aβ can activate the apoptotic effector component caspase-3, which is required in AMPAR removal and consequent LTD induction (Li et al., 2010). Reduced glutamate receptors further increase extra-synaptic glutamatergic accumulation, subsequently result in Aβ-induced synaptotoxic effects (Snyder et al., 2005). Aβ disrupts the glutamatergic transmission system by significantly decreasing the levels of AMPARs and NMDARs at the neuronal plasma membrane (Roselli et al., 2005; Lacor et al., 2007; De Felice et al., 2009). In a word, Aβ oligomers can aggravate the glutamate excitotoxicity by damaging glutamate transporters resulting from interrupting glutamate receptors, including NMADRs, AMPARs, and metabotropic glutamate receptors (Li and Selkoe, 2020).
Moreover, Aβ oligomers can aslo inhibit the astrocytic glutamate uptake by decreasing astrocytic glutamate transporters expression, causing extracellular glutamate aggregation, ultimately leading to a serious of glutamate toxicity cascades (Fernández-Tomé et al., 2004; Matos et al., 2008; Tong et al., 2017; Huang et al., 2018). Besides, Aβ oligomers can activate glial cells, which can release pro-inflammatory cytokines in pathological conditions. The pro-inflammatory cytokines can inhibit the ability of glial glutamate uptake and impair glutamate transporters (Carmen et al., 2009; Dumont et al., 2014; Tong et al., 2017). Consistent with these results, both glutamate transporters EAAT1 and EAAT2 which play an important role in the glutamate uptake in glial cells are decreased in the hippocampus of AD patients (Jacob et al., 2007). In a word, Aβ can disrupt Glu-recycling at the synapse by increasing glutamate spillover or decreasing glutamate transporters (Varga et al., 2015) (Figure 2). Taken together, Aβ can disrupt Glu-recycling at the synapse by increasing glutamate spillover, decreasing glutamate uptake, and impairing glutamate transporters, causing the accumulation of excessive glutamate in the extrasynaptic space, which in turn aberrantly activates eNMDARs and induces synaptic dysfunction.
Aβ can disturb molecular signaling pathway to damage synapse
Aβ oligomers not only act directly or indirectly on glutamate receptors, but also interfere with synapse-related signaling pathways and molecular targets to impair synaptic function. Studies showed that Aβ-induced dsyregulation of NMDARs inhibits the Wnt/β-catenin signaling pathway (Inestrosa et al., 2007; Magdesian et al., 2008). Under normal physiologic condition, the activation of Wnt signaling pathway can facilitate GSK-3β inactivation, elevate intracellular β-catenin, and promote Wnt target gene transcription, which are conducive to dendrite development, synapse formation, glutamate receptor insertion, and synaptic plasticity in the postsynaptic region (Beaumont et al., 2007; Cerpa et al., 2011; Inestrosa and Varela-Nallar, 2014). Some studies indicated that the activation of Wnt-5a signaling pathway protected PSD-95 from Aβ-induced synaptic toxicity and activated downstream proteins such as PKC, CaMKII, and JNK (Kühl et al., 2000; Dinamarca et al., 2008; Killick et al., 2014). Among of them, the Wnt-5a/JNK pathway modulates PSD-95 (Farías et al., 2009; Varela-Nallar, et al., 2010); the Wnt-5a/PKC pathway directly adjusts the localization of NR1 on the postsynaptic membrane (Frozza et al., 2009; Cerpa et al., 2011). At the same time, alteration of CaMKII activity triggers the incorporation of NMDARs in the synapse (Kühl et al., 2000). Therefore, the loss of Wnt signaling is closely related to the neurodegeneration and synaptic impairment induced by Aβ in AD (Liu et al., 2014). Aβ-induced neurotoxicity causes GSK-3β activation and decreases β-catenin (Chen et al., 2000; Magdesian et al., 2008), which cause reduced expression of Wnt-target gene and inhibition of Wnt signaling pathway (Cerpa et al., 2010; Cerpa et al., 2011). One study proved that the GSK-3β expression was up-regulated and the β-catenin was down-regulated in the hippocampus of AD patients (Chen et al., 2000). Besides, Aβ-induced GSK-3β overactivation increases the endocytosis/internalization of NMDARs and AMPARs, which disrupts the glutamatergic transmission and damage the synaptic function (Chen et al., 2007; Wei W. et al., 2010; Deng et al., 2014). Moreover, Aβ oligomers also attributes to the reduction and degradation of post-synaptic density-95 (PSD-95), which is a vital protein to sustain synaptic plasticity and the stabilization of AMPAR and NMDAR at synapses (Roselli et al., 2005). Once NMDAR and AMPAR becomes dysfunctional, it can lead to neuronal Ca2+ overload, oxidative stress (De Felice et al., 2007), and inhibition of the Wnt/β-catenin signaling pathway (Inestrosa et al., 2007; Parihar and Brewer, 2010) (Table 1).
In addition, the IKK/NF-κB signaling pathway also plays a critical role in Aβ-mediated hippocampal LTP impairment. Aβ might alter IKK/NF-κB activity via interacting with tumor necrosis factor receptor (TNFR). Aβ can motivate pro-inflammatory cytokine like TNF-α or interleukins to impair memory via directly combining with TNF-α receptors or activating microglial cell (Wang et al., 2005; Heppner et al., 2015) (Table 1). Microglia activated by Aβ and TNF-α can induce neuronal cell death (Floden et al., 2005). Besides, TNF-α activates dsRNA-dependent protein kinase (PKR), which mediate neuroinflammation and memory impairment induced by Aβ oligomers. Similarly, overmuch TNF-α also cause the abnormal trafficking of AMPARs, which leads to glutamate excitotoxicity (Leonoudakis et al., 2004). TNF-α acts through TNFR and mediates cellular functions primarily through NF-κB and IKK (Sakurai et al., 2003; Kwon et al., 2004; Luo et al., 2005; Rossol et al., 2007). The activation of NF-κB accelerates pro-inflammatory genes transcription and triggers the expression of Amyloid precursor protein (APP) gene, which results in Aβ overdeposition and synaptic loss (Valerio et al., 2006). Therefore, oligomeric Aβ might suppress hippocampal LTP via acting on TNF-α receptor and subsequently activating IKK and NF-κB signaling, ultimately forming a vicious cycle of synaptic damage (Samidurai et al., 2018). In addition, the inhibitory effects of oligomeric Aβ on hippocampal LTP involves apoptotic proteins (caspases) and reactive oxygen species (ROS) production (Ivins et al., 1999; Wang et al., 2004a). Oligomeric Aβ can intervenes in hippocampal LTP by activating caspase-3, AKT8 virus oncogene cellular homolog (Akt), and GSK-3β pathway (Jo et al., 2011).
Besides, soluble Aβ can aggravate memory disorder resulting from interfering with the Janus kinase 2 (JAK2)/Signal transducer and activator of transcription 3 (STAT3) axis and cholinergic dysfunction (Chiba et al., 2009). Kinases JNK, CDK-5, p25, and p38 MAPK are also involved in LTP inhibition mediated by Aβ (Wang et al., 2004b; Danysz and Parsons, 2012). p25 is a molecule that can induce the hallmark early Alzheimer-like synaptic pathology. Recent studies showed that stimulation of CDK-5 and JNK signaling by overproduction of p25 can rapidly inhibit NMDA and AMPA responses, reduce synapse density via the removal of newly delivered synaptic AMPARs, which altered synaptic transmission (Chergui et al., 2004; Peng et al., 2013). The up-regulation of JNK/Aβ induced synaptic depressions, which has the same result as suppressing and eliminating synapses (Zhu et al., 2005; Sheng et al., 2016) (Table 1). Besides, Aβ can also mediate synaptic depression on account of mGluRs activation, which provoked a series of downstream molecular events including MAPK and calcineurin, ultimately caused AMPARs internalization and synapse collapse (Wang, Rowan et al. (2004a)). In brief, Aβ can interfere with distant molecular signaling pathways to destroy synaptic transmission and plasticity.
Aβ can damage mitochondria and energy metabolism
Mitochondria provide the necessary ATP for the survival and optimal function of neurons, and mitochondrial dysfunction is closely related to aging and neurodegenerative diseases (Scheibye-Knudsen, et al., 2015; Kerr, et al., 2017). In AD, mitochondrial dysfunction has been widely recognized as a common pathological hallmark (Silva et al., 2011; Sorrentino, et al., 2017). The overexpression of APP and Aβ deposition can cause mitochondrial malfunctioning, such as mitochondrial fragmentation and abnormal distribution, which results in abnormal mitochondrial dynamics and ATP synthesis (von Bernhardi and Eugenín, 2012). Excessive mitochondrial fission and increased ROS production results in the progression of extensive macromolecular oxidative damage and amyloid lesions caused by ROS (Yu et al., 2006; Lakatos et al., 2010). Moreover, the interaction between intracellular Aβ and 3-hydroxyacyl-CoA dehydrogenase type-2 (HSD17B10, also known as ABAD) further lead to mitochondrial dysfunction and ROS leakage (Lustbader et al., 2004). Meanwhile, Aβ can interact with dynamin-1-like protein Drp1 (DNM1L), which can also promote excessive mitochondrial fragmentation and neuronal damage (Manczak et al., 2011). The mitochondria impairment alters the mitochondrial membrane potential, dysregulates mitochondrial calcium levels, and increases mitochondrial O2- (Chung et al., 2001). In addition, mitochondrial dysfunction-induced energy deficiency and Aβ1-42 oligomers cause intracellular Ca2+ imbalance and 5′AMP-activated protein kinase (AMPK) activation, resulting in synaptotoxicity and memory loss (Mairet-Coello, et al., 2013; Kerr, et al., 2017). The dysregulation of Ca2+ homeostasis further causes mitochondrial dysfunction, impairments in synaptic transmission and plasticity, and oxidative stress, which leads to the age-related cognitive impairment (Kang et al., 2011). Moreover, the axonal transport of mitochondria play a key role in neuronal function, Aβ1-42 and phosphorylated tau mediate defects in axonal transport, leading to synapse starvation, ATP depletion, and ultimately neurodegeneration (Vossel, et al., 2010; Fang, et al., 2019). Therefore, there may be a series of complex relationships between mitochondrial dysfunction and AD, suggesting that targeting defective mitochondria may be an important approach for AD treatment.
Aβ and tau have synergistic toxic effects on synapses
Aβ and tau are two main pathogenic factors of AD. Aβ and hyperphosphorylated tau have synergistic effect on impairing synapse function. Under physiologic condition, tau proteins increase in the PSD-enriched fraction accompanied by enrichment in PSD-95 and GluA1, which are requisite for synaptic potentiation related to LTP (Ehlers, 2003; Steiner et al., 2008; Kessels and Malinow, 2009). However, hyperphosphorylated tau disrupts NMDARs and AMPARs trafficking and anchoring (Hoover et al., 2010; Ittner et al., 2010; Miller et al., 2014), which can reduce synaptic responses and inhibit the expression of AMPARs and NMDARs in dendritic spines (Hoover et al., 2010; Crimins et al., 2012; Liao et al., 2014). Recent studies found that reduced NMDA receptor suppressed field excitatory postsynaptic potential (fEPSP) in hippocampal slices of tau transgenic mice (Burnouf et al., 2013). At the same time, tau hyperphosphorylation can decrease the internalization of AMPARs mediated by calcineurin, which reduces postsynaptic transmission at a very early stage of pathology (Miller et al., 2014; Müller-Thomsen et al., 2020). Excessive deposition of Aβ can promote tau hyperphosphorylation and activate GSK-3β, which exacerbates the toxic effect of phosphorylated tau on synapse, ultimately resulting in forming neurofibrillary tangles (NFTs) and loss of neurons and synapses (Wang and Mandelkow, 2016). Hyperphosphorylated tau proteins mediated by Aβ are more prone to hydrolysis or misfolding into tau oligomers. Extracellular tau oligomers acts on the postsynaptic regions, which increases Ca2+ influx and damages LTP (Usenovic et al., 2015; Fá et al., 2016). Moreover, tau oligomers also seed the misfolding and aggregation of cellular monomeric tau in a prion-like manner, which cause the diffusion of tau toxicity among synapses, eventually leading to synaptic dysfunction and the progression of AD lesions (Sanders et al., 2014; Comerota et al., 2019). In addition, a synaptic weaken stimulus mediates Ca2+ influx mediates through NMDAR and then activates the serine/threonine kinase GSK-3β. Activated GSK-3β triggers the short-lived phosphorylation of tau (Ser396), which paly a vital role in subsequent AMPAR internalization (Regan et al., 2017). Moreover, tau transports the tyrosine kinase Fyn to dendritic spines and interacts with postsynaptic targeting of Fyn kinase as well as its substrates PSD-95 and NR2B subunit (Ittner et al., 2010; Frandemiche et al., 2014) (Figure 3), which mediates downstream excitotoxic effects of phosphorylated tau (Ittner et al., 2010; Tracy et al., 2016; Regan et al., 2017). One study indicated that reduced tau could ameliorate Aβ-induced toxicity and excitotoxicity in vivo (Roberson et al., 2007). The result manifests that the synaptic toxicity of Aβ was dependent on tau to some extent (Biundo et al., 2018). Besides, tau phosphorylation is also regulated by the activities of protein phosphatase-2A (PP-2A), which is decreased in AD (Gong et al., 1993; Gong et al., 2000). Aβ can inhibit the activation of kinase PP2A (Chan and Sucher, 2001; Chohan and Iqbal, 2006). Similarly, the overexpression of GSK-3β induced by Aβ also inhibits PP2A, which may appear a negative feedback mechanism for GSK-3β activity (Liu et al., 2008). PP2A inactivity is involved in hyperphosphorylation of tau and facilitates phosphorylation of extracellular receptor kinase (ERK) 1/2 (Schulz et al., 2014). Therefore, It can be assumed that decreased PP2A activity may induce the activation of ERK1/2 and several other kinases and the abnormal hyperphosphorylation of tau. Additionally, ERKs can be activated by mitogens, c-Jun N-terminal kinase (JNK)/SAPK, and p38 mitogen-activated protein kinase (MAPK), which belong to stress-activated kinases (Tibbles and Woodgett, 1999). The activities of these enzymes can be triggered by a mass of exogenous and endogenous stress-inducing stimuli, such as ROS and oxidative stress. The kinases that phosphorylate tau can be activated by NMDA-induced oxidative stress including hyperactivation of CDK5 signaling pathway, MAPK, and several stress-activated protein kinases (Fuentes et al., 2012). Tau phosphorylation mediated by these kinases impaired microtubule assembly and induced the formation of paired helical filament (PHF) (Evans et al., 2000). Aβ activates GSK-3β, which induces oxidative stress, hyperphosphorylation of tau, NFT formation, neuronal death, and synaptic loss that can induce memory deficits. Furthermore, Aβ can activate calcineurin (CaN) to inhibit NMDAR function and impair LTP (Figure 3). Activated CaN increases tau phosphorylation, neurodegeneration, tangle formation and, subsequently, synapse dysfunction ((Ermak et al., 2001; Cook et al., 2005).
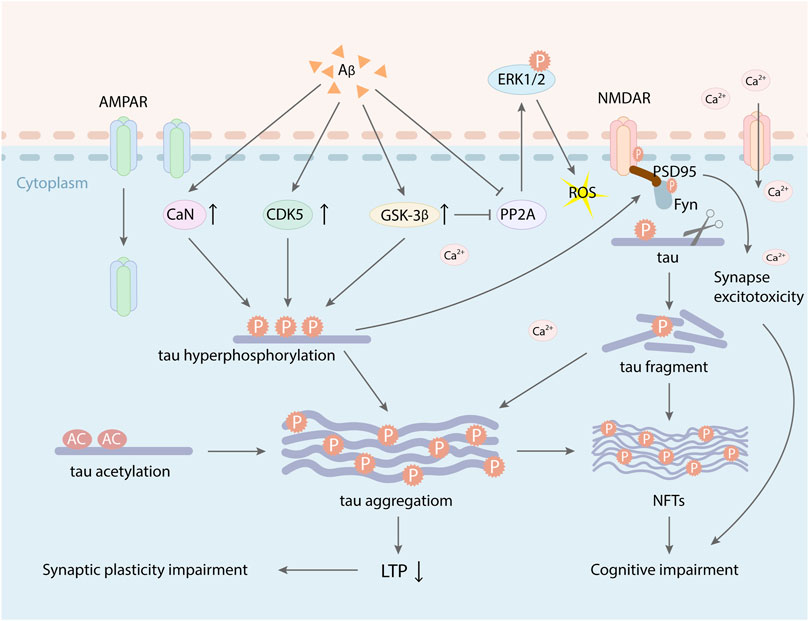
FIGURE 3. Aβ and tau have synergistic toxic effects on synapse plasticity. Excessive deposition of Aβ increased the activity of GSK-3β, CDK-5, and CaN, which promoted tau hyperphosphorylation and the formation of NFTs, leading to LTP suppression and cognitive impairment. Aberrant phosphorylation of tau protein can mediate Fyn facilitation to dendrites and make Fyn to interact with the PSD-95/NMDAR complex, which induced the synapse toxicity. At the same time, activated GSK-3β and tau hyperphosphorylation also induced AMPAR internalization. PP2A inactivity involved in hyperphosphorylation of tau and facilitated phosphorylation of extracellular receptor kinase (ERK) 1/2, which caused ROS and oxidative stress. Besides, Aβ induced tau acetylation, which increased tau aggregation and also inhibited the expression of LTP.
Studies demonstrated that the accumulation and mislocalization of hyperphosphorylated tau in the somatodendritic compartment of neurons in AD interfered with glutamate receptor trafficking and synaptic function (Ballatore et al., 2007; Hoover et al., 2010). Tau protein also combines with a postsynaptic protein complex including PSD-95, the scaffold for synaptic NMDARs which can adjust synaptic plasticity (El-Husseini et al., 2000). Endogenous tau protein is typically present in the post-synaptic dendrites, where it interacts with the PSD-95/NMDAR complex (Mondragón-Rodríguez et al., 2012). Treatment with human tau into the presynaptic terminal disrupts synaptic transmission, which may be closely related to blocking proper docking of synaptic vesicles (Moreno et al., 2011). Some studies also reported that tau toxicity on synaptic plasticity was involved in the acetylation of two lysines on tau, K274, and K281, which were related to AD. Acetylation decreased tau degradation (Min et al., 2010), inhibited tau binding to microtubule (Cohen, et al., 2011; Sohn et al., 2016), and increased tau aggregation (Cohen, et al., 2011; Min et al., 2015). Acetylated tau could inhibit the expression of LTP and lead to dysmnesia in AD (Figure 3). At the same time, acetylated tau could also disrupt the postsynaptic localization of memory-related KIBRA, which restrained the recruitment of postsynaptic AMPARs and damaged synaptic plasticity (Zempel et al., 2010; de Calignon et al., 2012; Tracy et al., 2016; Tracy and Gan, 2017). Therefore, tau may play an important role in regulating synaptic function and targeting neurotransmitter receptors to the synapse (Ittner and Götz, 2011; Kamat et al., 2016), while Aβ may be a synergist in this event.
Conclusion and future directions
Synaptic impairment is an early lesion of AD. Aβ, as one of major pathogenic factors in AD, is involved in the early synaptic damage. On the one hand, Aβ can disrupts extrapsynaptic/synaptic NMDARs to mediate Ca2+ influx, which leads to intracellular calcium overload and synaptic damage. Excessive Aβ deposition promotes NMDARs activation, which in turn increases Aβ production to a certain extent. Both can damage synaptic plasticity, which results in cognitive deficit of AD (Parihar and Brewer, 2010). On the other hand, Aβ can induce the internalization or removel of extrapsynaptic NMDARs and AMPARs by distant mechanisms, which results in extracellular accumulation of glutamate. The damage of synaptic plasticity is mainly blamed on calcium dyshomeostasis caused by NMDAR overactivation. The neuroexcitatory toxicity is mainly blamed on glutamate aggregation induced by reduced glutamate receptors and the dysfunction of glial cells induced by Aβ. NMDARs can not only improve learning and memory via sustaining LTP, but also cause synaptic plasticity damage and excitotoxicity through excitatory amino acid toxicity induced by NMDARs, resulting in learning and memory impairment, which is associated with Ca2+ disorders mediated by NR2B subunit (Martel et al., 2009). Besides, excessive NMDAR activation leads to excessive Ca2+ influx, which triggers a series of toxic reaction and activates diverse degradation enzymes, such as phospholipase C, CAMKII, PKC, NO synthase. As a result, they destroy neuronal lipid membrane and opalase bone frame, which disrupts the synaptic transmission, eventually developing learning and memory deficits (Biondi et al., 2010). In a word, Aβ-induced excessive Ca2+ influx, extracellular glutamate excitotoxicity, and increased internalization of NMDARs can suppress LTP, ultimately results in neuronal damage and learning-memory impairment in AD (Shankar et al., 2007; Talantova et al., 2013; Findley et al., 2019). In addition, the overexpression of APP and Aβ deposition can cause mitochondrial malfunctioning and destroy energy metabolism. Therefore, reducing Aβ deposition and extrasynaptic NMDARs overactivation may be a worthy consideration for preventing synaptic impairment in AD (Rammes et al., 2011). Meanwhile, targeting defective mitochondria may be an important approach for AD treatment. The current drug memantine which inhibits extrasynaptic NMDAR activity can be considered. Memantine is a non-competitive NMDAR antagonist, which can ameliorated Aβ-induced dysfunction of GluN2B-containing NMDARs trafficking by inhibiting the phosphorylation and surface expression of GluN2B, and prevent the downregulation of ERK/CREB signaling to alleviate neurotoxicity. Excitotoxicity refers to the sustained stimulation of excitatory amino acid receptors mainly involving NMDARs, which mediated a series of toxic impairment to neurons (Shin and Linden, 2005; Nicholls et al., 2007). The series of toxic impairment derived from excitotoxicity is mainly due to the upregulation of detrimental signaling pathways, disrupted Ca2+ homeostasis, and ROS/reactive nitrogen species (RNS) with further oxidative/nitrosative stress ultimately leading to cell death (Rai et al., 2013). Collective information suggests that NMDAR-mediated oxidative stress and neuronal apoptosis directly or indirectly influences synapse function (Kamat et al., 2016). The production of Aβ also requires activation of extrasynaptic NMDARs (probably GluN2B-enriched NMDARs) (Bordji et al., 2010). In conclusion, the glutamate-induced excitotoxicity and synaptic dysfunction could be excellent targets for the therapy of AD (Kamat et al., 2016). In addition, Aβ promotes tau phosphorylation in different manners, which further mediates the synaptic toxicity. Thus, blocking the targets of synergistic effect of Aβ and tau needs to be further explored, which may provide a new insight to prevent AD.
Author contributions
HZ wrote the manuscript. XJ and HZ drew the figures and designed the table in this manuscript. HL assisted in ideas and modification of the manuscript. LM and WW assisted in the manuscript writing. ZL, SC, JW, and JS helped revise the manuscript. All authors contributed to the article and approved the submitted version.
Funding
This review was funded by the National Natural Science Foundation of China (No. 81873350), the CACMS Innovation Fund (Nos CI 2021A01401 and CI 2021A01404), the Fundamental Research Funds for the Central public welfare research institutes (No. ZZ15-YQ-015), and the Innovation Team and Talents Cultivation Program of National Administration of Traditional Chinese Medicine (No. ZYYCXTD-C-202007).
Conflict of interest
The authors declare that the research was conducted in the absence of any commercial or financial relationships that could be construed as a potential conflict of interest.
Publisher’s note
All claims expressed in this article are solely those of the authors and do not necessarily represent those of their affiliated organizations, or those of the publisher, the editors and the reviewers. Any product that may be evaluated in this article, or claim that may be made by its manufacturer, is not guaranteed or endorsed by the publisher.
References
Abbott, J., Howlett, D., Francis, P., and Williams, R. (2008). Abeta(1-42) modulation of Akt phosphorylation via alpha7 nAChR and NMDA receptors. Neurobiol. Aging 29, 992–1001. doi:10.1016/j.neurobiolaging.2007.01.003
Arbel-Ornath, M., Hudry, E., Boivin, J., Hashimoto, T., Takeda, S., Kuchibhotla, K., et al. (2017). Soluble oligomeric amyloid-β induces calcium dyshomeostasis that precedes synapse loss in the living mouse brain. Mol. Neurodegener. 12, 27. doi:10.1186/s13024-017-0169-9
Author Anonymous, (2020). 2020 Alzheimer's disease facts and figures. Alzheimer's dementia J. Alzheimer's Assoc. 16, (3):391–460. doi:10.1002/alz.12068
Babri, S., Amani, M., Mohaddes, G., Alihemmati, A., and Ebrahimi, H. (2012). Effect of aggregated β-amyloid (1-42) on synaptic plasticity of hippocampal dentate gyrus granule cells in vivo. Bioimpacts. 2, 189–194. doi:10.5681/bi.2012.022
Ballatore, C., Lee, V., and Trojanowski, J. (2007). Tau-mediated neurodegeneration in Alzheimer's disease and related disorders. Nat. Rev. Neurosci. 8, 663–672. doi:10.1038/nrn2194
Beaumont, V., Thompson, S., Choudhry, F., Nuthall, H., Glantschnig, H., Lipfert, L., et al. (2007). Evidence for an enhancement of excitatory transmission in adult CNS by Wnt signaling pathway modulation. Mol. Cell. Neurosci. 35, 513–524. doi:10.1016/j.mcn.2007.03.004
Bellot, A., Guivernau, B., Tajes, M., Bosch-Morató, M., Valls-Comamala, V., and Muñoz, F. (2014). The structure and function of actin cytoskeleton in mature glutamatergic dendritic spines. Brain Res. 1573, 1–16. doi:10.1016/j.brainres.2014.05.024
Bergström, P., Agholme, L., Nazir, F., Satir, T., Toombs, J., Wellington, H., et al. (2016). Amyloid precursor protein expression and processing are differentially regulated during cortical neuron differentiation. Sci. Rep. 6, 29200. doi:10.1038/srep29200
Bezprozvanny, I., and Mattson, M. (2008). Neuronal calcium mishandling and the pathogenesis of Alzheimer's disease. Trends Neurosci. 31, 454–463. doi:10.1016/j.tins.2008.06.005
Biondi, O., Branchu, J., Sanchez, G., Lancelin, C., Deforges, S., Lopes, P., et al. (2010). In vivo NMDA receptor activation accelerates motor unit maturation, protects spinal motor neurons, and enhances SMN2 gene expression in severe spinal muscular atrophy mice. J. Neurosci. 30, 11288–11299. doi:10.1523/jneurosci.1764-10.2010
Biundo, F., Del Prete, D., Zhang, H., Arancio, O., and D'Adamio, L. (2018). A role for tau in learning, memory and synaptic plasticity. Sci. Rep. 8, 3184. doi:10.1038/s41598-018-21596-3
Bordji, K., Becerril-Ortega, J., Nicole, O., and Buisson, A. (2010). Activation of extrasynaptic, but not synaptic, NMDA receptors modifies amyloid precursor protein expression pattern and increases amyloid-ß production. J. Neurosci. 30, 15927–15942. doi:10.1523/jneurosci.3021-10.2010
Breijyeh, Z., and Karaman, R. (2020). Comprehensive Review on Alzheimer's Disease: Causes and Treatment. Mol. (Basel, Switz. 25, 5789. doi:10.3390/molecules25245789
Burnouf, S., Martire, A., Derisbourg, M., Laurent, C., Belarbi, K., Leboucher, A., et al. (2013). NMDA receptor dysfunction contributes to impaired brain-derived neurotrophic factor-induced facilitation of hippocampal synaptic transmission in a Tau transgenic model. Aging Cell 12, 11–23. doi:10.1111/acel.12018
Carmen, J., Rothstein, J., and Kerr, D. (2009). Tumor necrosis factor-alpha modulates glutamate transport in the CNS and is a critical determinant of outcome from viral encephalomyelitis. Brain Res. 1263, 143–154. doi:10.1016/j.brainres.2009.01.040
Cerpa, W., Farías, G., Godoy, J., Fuenzalida, M., Bonansco, C., and Inestrosa, N. (2010). Wnt-5a occludes Abeta oligomer-induced depression of glutamatergic transmission in hippocampal neurons. Mol. Neurodegener. 5, 3. doi:10.1186/1750-1326-5-3
Cerpa, W., Gambrill, A., Inestrosa, N., and Barria, A. (2011). Regulation of NMDA-receptor synaptic transmission by Wnt signaling. J. Neurosci. 31, 9466–9471. doi:10.1523/jneurosci.6311-10.2011
Chan, S., and Sucher, N. (2001). An NMDA receptor signaling complex with protein phosphatase 2A. J. Neurosci. 21, 7985–7992. doi:10.1523/jneurosci.21-20-07985.2001
Chen, R., Ding, W., and McCormick, F. (2000). Wnt signaling to beta-catenin involves two interactive components. Glycogen synthase kinase-3beta inhibition and activation of protein kinase C. J. Biol. Chem. 275, 17894–17899. doi:10.1074/jbc.M905336199
Chen, P., Gu, Z., Liu, W., and Yan, Z. (2007). Glycogen synthase kinase 3 regulates N-methyl-D-aspartate receptor channel trafficking and function in cortical neurons. Mol. Pharmacol. 72, 40–51. doi:10.1124/mol.107.034942
Chergui, K., Svenningsson, P., and Greengard, P. (2004). Cyclin-dependent kinase 5 regulates dopaminergic and glutamatergic transmission in the striatum. Proc. Natl. Acad. Sci. U. S. A. 101, 2191–2196. doi:10.1073/pnas.0308652100
Chiba, T., Yamada, M., Sasabe, J., Terashita, K., Shimoda, M., Matsuoka, M., et al. (2009). Amyloid-beta causes memory impairment by disturbing the JAK2/STAT3 axis in hippocampal neurons. Mol. Psychiatry 14, 206–222. doi:10.1038/mp.2008.105
Chohan, M., and Iqbal, K. (2006). From tau to toxicity: Emerging roles of NMDA receptor in Alzheimer's disease. J. Alzheimers Dis. 10, 81–87. doi:10.3233/jad-2006-10112
Chung, C., Song, Y., Kim, I., Yoon, W., Ryu, B., Jo, D., et al. (2001). Proapoptotic effects of tau cleavage product generated by caspase-3. Neurobiol. Dis. 8, 162–172. doi:10.1006/nbdi.2000.0335
Coan, E., Irving, A., and Collingridge, G. (1989). Low-frequency activation of the NMDA receptor system can prevent the induction of LTP. Neurosci. Lett. 105, 205–210. doi:10.1016/0304-3940(89)90038-4
Cohen, T., Guo, J., Hurtado, D., Kwong, L., Mills, I., Trojanowski, J., et al. (2011). The acetylation of tau inhibits its function and promotes pathological tau aggregation. Nat. Commun. 2, 252. doi:10.1038/ncomms1255
Comerota, M., Tumurbaatar, B., Krishnan, B., Kayed, R., and Taglialatela, G. (2019). Near infrared light treatment reduces synaptic levels of toxic tau oligomers in two transgenic mouse models of human tauopathies. Mol. Neurobiol. 56, 3341–3355. doi:10.1007/s12035-018-1248-9
Cook, C., Hejna, M., Magnuson, D., and Lee, J. (2005). Expression of calcipressin1, an inhibitor of the phosphatase calcineurin, is altered with aging and Alzheimer's disease. J. Alzheimers Dis. 8, 63–73. doi:10.3233/jad-2005-8108
Cooke, S., and Bliss, T. (2006). Plasticity in the human central nervous system. Brain. 129, 1659–1673. doi:10.1093/brain/awl082
Crimins, J., Rocher, A., and Luebke, J. (2012). Electrophysiological changes precede morphological changes to frontal cortical pyramidal neurons in the rTg4510 mouse model of progressive tauopathy. Acta Neuropathol. 124, 777–795. doi:10.1007/s00401-012-1038-9
Danysz, W., and Parsons, C. (2012). Alzheimer's disease, β-amyloid, glutamate, NMDA receptors and memantine--searching for the connections. Br. J. Pharmacol. 167, 324–352. doi:10.1111/j.1476-5381.2012.02057.x
de Calignon, A., Polydoro, M., Suárez-Calvet, M., William, C., Adamowicz, D., Kopeikina, K., et al. (2012). Propagation of tau pathology in a model of early Alzheimer's disease. Neuron 73, 685–697. doi:10.1016/j.neuron.2011.11.033
De Felice, F., Velasco, P., Lambert, M., Viola, K., Fernandez, S., Ferreira, S., et al. (2007). Abeta oligomers induce neuronal oxidative stress through an N-methyl-D-aspartate receptor-dependent mechanism that is blocked by the Alzheimer drug memantine. J. Biol. Chem. 282, 11590–11601. doi:10.1074/jbc.M607483200
De Felice, F., Vieira, M., Bomfim, T., Decker, H., Velasco, P., Lambert, M., et al. (2009). Protection of synapses against alzheimer's-linked toxins: Insulin signaling prevents the pathogenic binding of abeta oligomers. Proc. Natl. Acad. Sci. U. S. A. 106, 1971–1976. doi:10.1073/pnas.0809158106
De Roo, M., Klauser, P., Garcia, P., Poglia, L., and Muller, D. (2008). Spine dynamics and synapse remodeling during LTP and memory processes. Prog. Brain Res. 169, 199–207. doi:10.1016/s0079-6123(07)00011-8
Deng, Y., Xiong, Z., Chen, P., Wei, J., Chen, S., and Yan, Z. (2014). β-amyloid impairs the regulation of N-methyl-D-aspartate receptors by glycogen synthase kinase 3. Neurobiol. Aging 35, 449–459. doi:10.1016/j.neurobiolaging.2013.08.031
Dinamarca, M., Colombres, M., Cerpa, W., Bonansco, C., and Inestrosa, N. (2008). Beta-amyloid oligomers affect the structure and function of the postsynaptic region: Role of the wnt signaling pathway. Neurodegener. Dis. 5, 149–152. doi:10.1159/000113687
Domingues, A., Almeida, S., da Cruz e Silva, E., Oliveira, C., and Rego, A. (2007). Toxicity of beta-amyloid in HEK293 cells expressing NR1/NR2A or NR1/NR2B N-methyl-D-aspartate receptor subunits. Neurochem. Int. 50, 872–880. doi:10.1016/j.neuint.2007.03.001
Dumont, A., Goursaud, S., Desmet, N., and Hermans, E. (2014). Differential regulation of glutamate transporter subtypes by pro-inflammatory cytokine TNF-α in cortical astrocytes from a rat model of amyotrophic lateral sclerosis. PloS one 9, e97649. doi:10.1371/journal.pone.0097649
Ehlers, M. (2003). Activity level controls postsynaptic composition and signaling via the ubiquitin-proteasome system. Nat. Neurosci. 6, 231–242. doi:10.1038/nn1013
El-Husseini, A., Schnell, E., Chetkovich, D., Nicoll, R., and Bredt, D. (2000). PSD-95 involvement in maturation of excitatory synapses. Sci. (New York, N.Y.) 290, 1364–1368. doi:10.1126/science.290.5495.1364
Ermak, G., Morgan, T., and Davies, K. (2001). Chronic overexpression of the calcineurin inhibitory gene DSCR1 (Adapt78) is associated with Alzheimer's disease. J. Biol. Chem. 276, 38787–38794. doi:10.1074/jbc.M102829200
Evans, D., Rank, K., Bhattacharya, K., Thomsen, D., Gurney, M., and Sharma, S. (2000). Tau phosphorylation at serine 396 and serine 404 by human recombinant tau protein kinase II inhibits tau's ability to promote microtubule assembly. J. Biol. Chem. 275, 24977–24983. doi:10.1074/jbc.M000808200
Fá, M., Puzzo, D., Piacentini, R., Staniszewski, A., Zhang, H., Baltrons, M., et al. (2016). Extracellular tau oligomers produce an immediate impairment of LTP and memory. Sci. Rep. 6, 19393. doi:10.1038/srep19393
Falcicchia, C., Tozzi, F., Arancio, O., Watterson, D., and Origlia, N. (2020). Involvement of p38 MAPK in synaptic function and dysfunction. Int. J. Mol. Sci. 21, E5624. doi:10.3390/ijms21165624
Fang, E., Hou, Y., Palikaras, K., Adriaanse, B., Kerr, J., Yang, B., et al. (2019). Mitophagy inhibits amyloid-β and tau pathology and reverses cognitive deficits in models of Alzheimer's disease. Nat. Neurosci. 22, 401–412. doi:10.1038/s41593-018-0332-9
Farías, G., Alfaro, I., Cerpa, W., Grabowski, C., Godoy, J., Bonansco, C., et al. (2009). Wnt-5a/JNK signaling promotes the clustering of PSD-95 in hippocampal neurons. J. Biol. Chem. 284, 15857–15866. doi:10.1074/jbc.M808986200
Fernández-Tomé, P., Brera, B., Arévalo, M., and de Ceballos, M. (2004). Beta-amyloid25-35 inhibits glutamate uptake in cultured neurons and astrocytes: Modulation of uptake as a survival mechanism. Neurobiol. Dis. 15, 580–589. doi:10.1016/j.nbd.2003.12.006
Findley, C., Bartke, A., Hascup, K., and Hascup, E. (2019). Amyloid beta-related alterations to glutamate signaling dynamics during Alzheimer's disease progression. ASN neuro 11, 1759091419855541. doi:10.1177/1759091419855541
Floden, A., Li, S., and Combs, C. (2005). Beta-amyloid-stimulated microglia induce neuron death via synergistic stimulation of tumor necrosis factor alpha and NMDA receptors. J. Neurosci. 25, 2566–2575. doi:10.1523/jneurosci.4998-04.2005
Frandemiche, M., De Seranno, S., Rush, T., Borel, E., Elie, A., Arnal, I., et al. (2014). Activity-dependent tau protein translocation to excitatory synapse is disrupted by exposure to amyloid-beta oligomers. J. Neurosci. 34, 6084–6097. doi:10.1523/jneurosci.4261-13.2014
Frozza, R., Horn, A., Hoppe, J., Simão, F., Gerhardt, D., Comiran, R., et al. (2009). A comparative study of beta-amyloid peptides Abeta1-42 and Abeta25-35 toxicity in organotypic hippocampal slice cultures. Neurochem. Res. 34, 295–303. doi:10.1007/s11064-008-9776-8
Fuentes, F., Zimmer, D., Atienza, M., Schottenfeld, J., Penkala, I., Bale, T., et al. (2012). Protein tyrosine phosphatase PTP1B is involved in hippocampal synapse formation and learning. PloS one 7, e41536. doi:10.1371/journal.pone.0041536
Gong, C., Singh, T., Grundke-Iqbal, I., and Iqbal, K. (1993). Phosphoprotein phosphatase activities in Alzheimer disease brain. J. Neurochem. 61, 921–927. doi:10.1111/j.1471-4159.1993.tb03603.x
Gong, C., Lidsky, T., Wegiel, J., Zuck, L., Grundke-Iqbal, I., and Iqbal, K. (2000). Phosphorylation of microtubule-associated protein tau is regulated by protein phosphatase 2A in mammalian brain. Implications for neurofibrillary degeneration in Alzheimer's disease. J. Biol. Chem. 275, 5535–5544. doi:10.1074/jbc.275.8.5535
Gu, Z., Liu, W., and Yan, Z. (2009). {beta}-Amyloid impairs AMPA receptor trafficking and function by reducing Ca2+/calmodulin-dependent protein kinase II synaptic distribution. J. Biol. Chem. 284, 10639–10649. doi:10.1074/jbc.M806508200
Hascup, K., and Hascup, E. (2016). Soluble amyloid-β42 stimulates glutamate release through activation of the α7 nicotinic acetylcholine receptor. J. Alzheimers Dis. 53, 337–347. doi:10.3233/jad-160041
Hayashi, Y., Shi, S., Esteban, J., Piccini, A., Poncer, J., and Malinow, R. (2000). Driving AMPA receptors into synapses by LTP and CaMKII: Requirement for GluR1 and PDZ domain interaction. Sci. (New York, N.Y.) 287, 2262–2267. doi:10.1126/science.287.5461.2262
Heppner, F., Ransohoff, R., and Becher, B. (2015). Immune attack: The role of inflammation in alzheimer disease. Nat. Rev. Neurosci. 16, 358–372. doi:10.1038/nrn3880
Herrup, K. (2015). The case for rejecting the amyloid cascade hypothesis. Nat. Neurosci. 18, 794–799. doi:10.1038/nn.4017
Hooper, C., Markevich, V., Plattner, F., Killick, R., Schofield, E., Engel, T., et al. (2007). Glycogen synthase kinase-3 inhibition is integral to long-term potentiation. Eur. J. Neurosci. 25, 81–86. doi:10.1111/j.1460-9568.2006.05245.x
Hoover, B., Reed, M., Su, J., Penrod, R., Kotilinek, L., Grant, M., et al. (2010). Tau mislocalization to dendritic spines mediates synaptic dysfunction independently of neurodegeneration. Neuron 68, 1067–1081. doi:10.1016/j.neuron.2010.11.030
Hsieh, H., Boehm, J., Sato, C., Iwatsubo, T., Tomita, T., Sisodia, S., et al. (2006). AMPAR removal underlies Abeta-induced synaptic depression and dendritic spine loss. Neuron 52, 831–843. doi:10.1016/j.neuron.2006.10.035
Hu, N., Klyubin, I., Anwyl, R., Anwy, R., and Rowan, M. (2009). GluN2B subunit-containing NMDA receptor antagonists prevent Abeta-mediated synaptic plasticity disruption in vivo. Proc. Natl. Acad. Sci. U. S. A. 106, 20504–20509. doi:10.1073/pnas.0908083106
Hu, N., Nicoll, A., Zhang, D., Mably, A., O'Malley, T., Purro, S., et al. (2014). mGlu5 receptors and cellular prion protein mediate amyloid-β-facilitated synaptic long-term depression in vivo. Nat. Commun. 5, 3374. doi:10.1038/ncomms4374
Hu, R., Wei, P., Jin, L., Zheng, T., Chen, W., Liu, X., et al. (2017). Overexpression of EphB2 in hippocampus rescues impaired NMDA receptors trafficking and cognitive dysfunction in Alzheimer model. Cell Death Dis. 8, e2717. doi:10.1038/cddis.2017.140
Huang, S., Tong, H., Lei, M., Zhou, M., Guo, W., Li, G., et al. (2018). Astrocytic glutamatergic transporters are involved in Aβ-induced synaptic dysfunction. Brain Res. 1678, 129–137. doi:10.1016/j.brainres.2017.10.011
Inestrosa, N., and Varela-Nallar, L. (2014). Wnt signaling in the nervous system and in Alzheimer's disease. J. Mol. Cell Biol. 6, 64–74. doi:10.1093/jmcb/mjt051
Inestrosa, N., Varela-Nallar, L., Grabowski, C., and Colombres, M. (2007). Synaptotoxicity in Alzheimer's disease: The wnt signaling pathway as a molecular target. IUBMB life 59, 316–321. doi:10.1080/15216540701242490
Ittner, L., and Götz, J. (2011). Amyloid-β and tau--a toxic pas de deux in Alzheimer's disease. Nat. Rev. Neurosci. 12, 65–72. doi:10.1038/nrn2967
Ittner, L., Ke, Y., Delerue, F., Bi, M., Gladbach, A., van Eersel, J., et al. (2010). Dendritic function of tau mediates amyloid-beta toxicity in Alzheimer's disease mouse models. Cell 142, 387–397. doi:10.1016/j.cell.2010.06.036
Ivins, K., Thornton, P., Rohn, T., and Cotman, C. (1999). Neuronal apoptosis induced by beta-amyloid is mediated by caspase-8. Neurobiol. Dis. 6, 440–449. doi:10.1006/nbdi.1999.0268
Izumi, Y., Auberson, Y., and Zorumski, C. (2006). Zinc modulates bidirectional hippocampal plasticity by effects on NMDA receptors. J. Neurosci. 26, 7181–7188. doi:10.1523/jneurosci.1258-06.2006
Jacob, C., Koutsilieri, E., Bartl, J., Neuen-Jacob, E., Arzberger, T., Zander, N., et al. (2007). Alterations in expression of glutamatergic transporters and receptors in sporadic Alzheimer's disease. J. Alzheimers Dis. 11, 97–116. doi:10.3233/jad-2007-11113
Jo, J., Whitcomb, D., Olsen, K., Kerrigan, T., Lo, S., Bru-Mercier, G., et al. (2011). Aβ(1-42) inhibition of LTP is mediated by a signaling pathway involving caspase-3, Akt1 and GSK-3β. Nat. Neurosci. 14, 545–547. doi:10.1038/nn.2785
Kabogo, D., Rauw, G., Amritraj, A., Baker, G., and Kar, S. (2010). ß-amyloid-related peptides potentiate K+-evoked glutamate release from adult rat hippocampal slices. Neurobiol. Aging 31, 1164–1172. doi:10.1016/j.neurobiolaging.2008.08.009
Kamat, P., Rai, S., Swarnkar, S., Shukla, R., Ali, S., Najmi, A., et al. (2013). Okadaic acid-induced tau phosphorylation in rat brain: Role of NMDA receptor. Neuroscience 238, 97–113. doi:10.1016/j.neuroscience.2013.01.075
Kamat, P., Kalani, A., Rai, S., Swarnkar, S., Tota, S., Nath, C., et al. (2016). Mechanism of oxidative stress and synapse dysfunction in the pathogenesis of Alzheimer's disease: Understanding the therapeutics strategies. Mol. Neurobiol. 53, 648–661. doi:10.1007/s12035-014-9053-6
Kang, D., Roh, S., Woo, J., Liu, T., Bu, J., Jung, A., et al. (2011). The interface between cytoskeletal aberrations and mitochondrial dysfunction in Alzheimer's disease and related disorders. Exp. Neurobiol. 20, 67–80. doi:10.5607/en.2011.20.2.67
Kawamoto, E., Lepsch, L., Boaventura, M., Munhoz, C., Lima, L., Yshii, L., et al. (2008). Amyloid beta-peptide activates nuclear factor-kappaB through an N-methyl-D-aspartate signaling pathway in cultured cerebellar cells. J. Neurosci. Res. 86, 845–860. doi:10.1002/jnr.21548
Kelly, B., and Ferreira, A. (2006). beta-Amyloid-induced dynamin 1 degradation is mediated by N-methyl-D-aspartate receptors in hippocampal neurons. J. Biol. Chem. 281, 28079–28089. doi:10.1074/jbc.M605081200
Kerr, J., Adriaanse, B., Greig, N., Mattson, M., Cader, M., Bohr, V., et al. (2017). Mitophagy and Alzheimer's disease: Cellular and molecular mechanisms. Trends Neurosci. 40, 151–166. doi:10.1016/j.tins.2017.01.002
Kessels, H., and Malinow, R. (2009). Synaptic AMPA receptor plasticity and behavior. Neuron 61, 340–350. doi:10.1016/j.neuron.2009.01.015
Killick, R., Ribe, E., Al-Shawi, R., Malik, B., Hooper, C., Fernandes, C., et al. (2014). Clusterin regulates β-amyloid toxicity via Dickkopf-1-driven induction of the wnt-PCP-JNK pathway. Mol. Psychiatry 19, 88–98. doi:10.1038/mp.2012.163
Knobloch, M., Konietzko, U., Krebs, D., and Nitsch, R. (2007). Intracellular Abeta and cognitive deficits precede beta-amyloid deposition in transgenic arcAbeta mice. Neurobiol. Aging 28, 1297–1306. doi:10.1016/j.neurobiolaging.2006.06.019
Kopec, C., Li, B., Wei, W., Boehm, J., and Malinow, R. (2006). Glutamate receptor exocytosis and spine enlargement during chemically induced long-term potentiation. J. Neurosci. 26, 2000–2009. doi:10.1523/jneurosci.3918-05.2006
Kopeikina, K., Hyman, B., and Spires-Jones, T. (2012). Soluble forms of tau are toxic in Alzheimer's disease. Transl. Neurosci. 3, 223–233. doi:10.2478/s13380-012-0032-y
Kühl, M., Sheldahl, L., Park, M., Miller, J., and Moon, R. (2000). The wnt/Ca2+ pathway: A new vertebrate wnt signaling pathway takes shape. Trends Genet. 16, 279–283. doi:10.1016/s0168-9525(00)02028-x
Kwon, H., Breese, E., Vig-Varga, E., Luo, Y., Lee, Y., Goebl, M., et al. (2004). Tumor necrosis factor alpha induction of NF-kappaB requires the novel coactivator SIMPL. Mol. Cell. Biol. 24, 9317–9326. doi:10.1128/mcb.24.21.9317-9326.2004
Lacor, P., Buniel, M., Furlow, P., Clemente, A., Velasco, P., Wood, M., et al. (2007). Abeta oligomer-induced aberrations in synapse composition, shape, and density provide a molecular basis for loss of connectivity in Alzheimer's disease. J. Neurosci. 27, 796–807. doi:10.1523/jneurosci.3501-06.2007
Lakatos, A., Derbeneva, O., Younes, D., Keator, D., Bakken, T., Lvova, M., et al. (2010). Association between mitochondrial DNA variations and Alzheimer's disease in the ADNI cohort. Neurobiol. Aging 31, 1355–1363. doi:10.1016/j.neurobiolaging.2010.04.031
Lanznaster, D., Mack, J., Coelho, V., Ganzella, M., Almeida, R., Dal-Cim, T., et al. (2017). Guanosine prevents anhedonic-like behavior and impairment in hippocampal glutamate transport following amyloid-β1-40 administration in mice. Mol. Neurobiol. 54, 5482–5496. doi:10.1007/s12035-016-0082-1
Laurén, J., Gimbel, D., Nygaard, H., Gilbert, J., and Strittmatter, S. (2009). Cellular prion protein mediates impairment of synaptic plasticity by amyloid-beta oligomers. Nature 457, 1128–1132. doi:10.1038/nature07761
Leonoudakis, D., Braithwaite, S., Beattie, M., and Beattie, E. (2004). TNFalpha-induced AMPA-receptor trafficking in CNS neurons; relevance to excitotoxicity? Neuron Glia Biol. 1, 263–273. doi:10.1017/s1740925x05000608
Léveillé, F., El Gaamouch, F., Gouix, E., Lecocq, M., Lobner, D., Nicole, O., et al. (2008). Neuronal viability is controlled by a functional relation between synaptic and extrasynaptic NMDA receptors. FASEB J. official Publ. Fed. Am. Soc. Exp. Biol. 22, 4258–4271. doi:10.1096/fj.08-107268
Li, S., and Selkoe, D. (2020). A mechanistic hypothesis for the impairment of synaptic plasticity by soluble Aβ oligomers from Alzheimer's brain. J. Neurochem. 154, 583–597. doi:10.1111/jnc.15007
Li, S., Hong, S., Shepardson, N., Walsh, D., Shankar, G., and Selkoe, D. (2009). Soluble oligomers of amyloid Beta protein facilitate hippocampal long-term depression by disrupting neuronal glutamate uptake. Neuron 62, 788–801. doi:10.1016/j.neuron.2009.05.012
Li, Z., Jo, J., Jia, J., Lo, S., Whitcomb, D., Jiao, S., et al. (2010). Caspase-3 activation via mitochondria is required for long-term depression and AMPA receptor internalization. Cell 141, 859–871. doi:10.1016/j.cell.2010.03.053
Li, S., Jin, M., Koeglsperger, T., Shepardson, N., Shankar, G., and Selkoe, D. (2011). Soluble Aβ oligomers inhibit long-term potentiation through a mechanism involving excessive activation of extrasynaptic NR2B-containing NMDA receptors. J. Neurosci. 31, 6627–6638. doi:10.1523/jneurosci.0203-11.2011
Liao, D., Miller, E., and Teravskis, P. (2014). Tau acts as a mediator for Alzheimer's disease-related synaptic deficits. Eur. J. Neurosci. 39, 1202–1213. doi:10.1111/ejn.12504
Lisman, J., Schulman, H., and Cline, H. (2002). The molecular basis of CaMKII function in synaptic and behavioural memory. Nat. Rev. Neurosci. 3, 175–190. doi:10.1038/nrn753
Liu, G., Zhang, Y., Yao, X., Zhang, C., Fang, J., Wang, Q., et al. (2008). Activation of glycogen synthase kinase-3 inhibits protein phosphatase-2A and the underlying mechanisms. Neurobiol. Aging 29, 1348–1358. doi:10.1016/j.neurobiolaging.2007.03.012
Liu, C., Tsai, C., Deak, F., Rogers, J., Penuliar, M., Sung, Y., et al. (2014). Deficiency in LRP6-mediated Wnt signaling contributes to synaptic abnormalities and amyloid pathology in Alzheimer's disease. Neuron 84, 63–77. doi:10.1016/j.neuron.2014.08.048
Liu, Y., Du, T., Zhang, W., Lu, W., Peng, Z., Huang, S., et al. (2019). βModified huang-lian-jie-du decoction ameliorates A synaptotoxicity in a murine model of Alzheimer's disease. Oxid. Med. Cell. Longev. 2019, 8340192. doi:10.1155/2019/8340192
Luo, J., Kamata, H., and Karin, M. (2005). IKK/NF-kappaB signaling: Balancing life and death--a new approach to cancer therapy. J. Clin. Invest. 115, 2625–2632. doi:10.1172/jci26322
Lustbader, J., Cirilli, M., Lin, C., Xu, H., Takuma, K., Wang, N., et al. (2004). ABAD directly links Abeta to mitochondrial toxicity in Alzheimer's disease. Sci. (New York, N.Y.) 304, 448–452. doi:10.1126/science.1091230
Magdesian, M., Carvalho, M., Mendes, F., Saraiva, L., Juliano, M., Juliano, L., et al. (2008). Amyloid-beta binds to the extracellular cysteine-rich domain of Frizzled and inhibits Wnt/beta-catenin signaling. J. Biol. Chem. 283, 9359–9368. doi:10.1074/jbc.M707108200
Mairet-Coello, G., Courchet, J., Pieraut, S., Courchet, V., Maximov, A., and Polleux, F. (2013). The CAMKK2-AMPK kinase pathway mediates the synaptotoxic effects of Aβ oligomers through Tau phosphorylation. Neuron 78, 94–108. doi:10.1016/j.neuron.2013.02.003
Manczak, M., Calkins, M., and Reddy, P. (2011). Impaired mitochondrial dynamics and abnormal interaction of amyloid beta with mitochondrial protein Drp1 in neurons from patients with Alzheimer's disease: implications for neuronal damage. Hum. Mol. Genet. 20, 2495–2509. doi:10.1093/hmg/ddr139
Martel, M., Wyllie, D., and Hardingham, G. (2009). In developing hippocampal neurons, NR2B-containing N-methyl-D-aspartate receptors (NMDARs) can mediate signaling to neuronal survival and synaptic potentiation, as well as neuronal death. Neuroscience 158, 334–343. doi:10.1016/j.neuroscience.2008.01.080
Matos, M., Augusto, E., Oliveira, C., and Agostinho, P. (2008). Amyloid-beta peptide decreases glutamate uptake in cultured astrocytes: Involvement of oxidative stress and mitogen-activated protein kinase cascades. Neuroscience 156, 898–910. doi:10.1016/j.neuroscience.2008.08.022
Matos, M., Augusto, E., Machado, N., dos Santos-Rodrigues, A., Cunha, R., and Agostinho, P. (2012). Astrocytic adenosine A2A receptors control the amyloid-β peptide-induced decrease of glutamate uptake. J. Alzheimers Dis. 31, 555–567. doi:10.3233/jad-2012-120469
Miller, E., Teravskis, P., Dummer, B., Zhao, X., Huganir, R., and Liao, D. (2014). Tau phosphorylation and tau mislocalization mediate soluble Aβ oligomer-induced AMPA glutamate receptor signaling deficits. Eur. J. Neurosci. 39, 1214–1224. doi:10.1111/ejn.12507
Min, S., Cho, S., Zhou, Y., Schroeder, S., Haroutunian, V., Seeley, W., et al. (2010). Acetylation of tau inhibits its degradation and contributes to tauopathy. Neuron 67, 953–966. doi:10.1016/j.neuron.2010.08.044
Min, S., Chen, X., Tracy, T., Li, Y., Zhou, Y., Wang, C., et al. (2015). Critical role of acetylation in tau-mediated neurodegeneration and cognitive deficits. Nat. Med. 21, 1154–1162. doi:10.1038/nm.3951
Mondragón-Rodríguez, S., Trillaud-Doppia, E., Dudilot, A., Bourgeois, C., Lauzon, M., Leclerc, N., et al. (2012). Interaction of endogenous tau protein with synaptic proteins is regulated by N-methyl-D-aspartate receptor-dependent tau phosphorylation. J. Biol. Chem. 287, 32040–32053. doi:10.1074/jbc.M112.401240
Moreno, H., Choi, S., Yu, E., Brusco, J., Avila, J., Moreira, J., et al. (2011). Blocking effects of human tau on squid giant synapse transmission and its prevention by T-817 MA. Front. Synaptic Neurosci. 3, 3. doi:10.3389/fnsyn.2011.00003
Müller-Thomsen, L., Borgmann, D., Morcinek, K., Schröder, S., Dengler, B., Moser, N., et al. (2020). Consequences of hyperphosphorylated tau on the morphology and excitability of hippocampal neurons in aged tau transgenic mice. Neurobiol. Aging 93, 109–123. doi:10.1016/j.neurobiolaging.2020.03.007
Nakamura, S., Murayama, N., Noshita, T., Katsuragi, R., and Ohno, T. (2006). Cognitive dysfunction induced by sequential injection of amyloid-beta and ibotenate into the bilateral hippocampus; protection by memantine and MK-801. Eur. J. Pharmacol. 548, 115–122. doi:10.1016/j.ejphar.2006.07.049
Nicholls, D., Johnson-Cadwell, L., Vesce, S., Jekabsons, M., and Yadava, N. (2007). Bioenergetics of mitochondria in cultured neurons and their role in glutamate excitotoxicity. J. Neurosci. Res. 85, 3206–3212. doi:10.1002/jnr.21290
Nicoll, A., Panico, S., Freir, D., Wright, D., Terry, C., Risse, E., et al. (2013). Amyloid-β nanotubes are associated with prion protein-dependent synaptotoxicity. Nat. Commun. 4, 2416. doi:10.1038/ncomms3416
Origlia, N., Righi, M., Capsoni, S., Cattaneo, A., Fang, F., Stern, D., et al. (2008). Receptor for advanced glycation end product-dependent activation of p38 mitogen-activated protein kinase contributes to amyloid-beta-mediated cortical synaptic dysfunction. J. Neurosci. 28, 3521–3530. doi:10.1523/jneurosci.0204-08.2008
Origlia, N., Bonadonna, C., Rosellini, A., Leznik, E., Arancio, O., Yan, S., et al. (2010). Microglial receptor for advanced glycation end product-dependent signal pathway drives beta-amyloid-induced synaptic depression and long-term depression impairment in entorhinal cortex. J. Neurosci. 30, 11414–11425. doi:10.1523/jneurosci.2127-10.2010
O'Riordan, K., Hu, N., and Rowan, M. (2018). Aß facilitates LTD at schaffer collateral synapses preferentially in the left Hippocampus. Cell Rep. 22, 2053–2065. doi:10.1016/j.celrep.2018.01.085
Parihar, M., and Brewer, G. (2010). Amyloid-β as a modulator of synaptic plasticity. J. Alzheimers Dis. 22, 741–763. doi:10.3233/jad-2010-101020
Peineau, S., Taghibiglou, C., Bradley, C., Wong, T., Liu, L., Lu, J., et al. (2007). LTP inhibits LTD in the hippocampus via regulation of GSK3beta. Neuron 53, 703–717. doi:10.1016/j.neuron.2007.01.029
Peng, Y., Hou, Z., and Yu, X. (2013). The kinase activity of EphA4 mediates homeostatic scaling-down of synaptic strength via activation of Cdk5. Neuropharmacology 65, 232–243. doi:10.1016/j.neuropharm.2012.10.012
Rai, S., Kamat, P., Nath, C., and Shukla, R. (2013). A study on neuroinflammation and NMDA receptor function in STZ (ICV) induced memory impaired rats. J. Neuroimmunol. 254, 1–9. doi:10.1016/j.jneuroim.2012.08.008
Rammes, G., Hasenjäger, A., Sroka-Saidi, K., Deussing, J., and Parsons, C. (2011). Therapeutic significance of NR2B-containing NMDA receptors and mGluR5 metabotropic glutamate receptors in mediating the synaptotoxic effects of β-amyloid oligomers on long-term potentiation (LTP) in murine hippocampal slices. Neuropharmacology 60, 982–990. doi:10.1016/j.neuropharm.2011.01.051
Raven, F., Van der Zee, E., Meerlo, P., and Havekes, R. (2018). The role of sleep in regulating structural plasticity and synaptic strength: Implications for memory and cognitive function. Sleep. Med. Rev. 39, 3–11. doi:10.1016/j.smrv.2017.05.002
Regan, P., Whitcomb, D., and Cho, K. (2017). Physiological and pathophysiological implications of synaptic tau. Neuroscientist. 23, 137–151. doi:10.1177/1073858416633439
Reinders, N., Pao, Y., Renner, M., da Silva-Matos, C., Lodder, T., Malinow, R., et al. (2016). Amyloid-β effects on synapses and memory require AMPA receptor subunit GluA3. Proc. Natl. Acad. Sci. U. S. A. 113, E6526–E6534. doi:10.1073/pnas.1614249113
Ripoli, C., Cocco, S., Li Puma, D., Piacentini, R., Mastrodonato, A., Scala, F., et al. (2014). Intracellular accumulation of amyloid-β (Aβ) protein plays a major role in Aβ-induced alterations of glutamatergic synaptic transmission and plasticity. J. Neurosci. 34, 12893–12903. doi:10.1523/jneurosci.1201-14.2014
Roberson, E., Scearce-Levie, K., Palop, J., Yan, F., Cheng, I., Wu, T., et al. (2007). Reducing endogenous tau ameliorates amyloid beta-induced deficits in an Alzheimer's disease mouse model. Sci. (New York, N.Y.) 316, 750–754. doi:10.1126/science.1141736
Roberson, E., Halabisky, B., Yoo, J., Yao, J., Chin, J., Yan, F., et al. (2011). Amyloid-β/Fyn-induced synaptic, network, and cognitive impairments depend on tau levels in multiple mouse models of Alzheimer's disease. J. Neurosci. 31, 700–711. doi:10.1523/jneurosci.4152-10.2011
Roselli, F., Tirard, M., Lu, J., Hutzler, P., Lamberti, P., Livrea, P., et al. (2005). Soluble beta-amyloid1-40 induces NMDA-dependent degradation of postsynaptic density-95 at glutamatergic synapses. J. Neurosci. 25, 11061–11070. doi:10.1523/jneurosci.3034-05.2005
Rossol, M., Meusch, U., Pierer, M., Kaltenhäuser, S., Häntzschel, H., Hauschildt, S., et al. (2007)., 179. Baltimore, Md, 4239–4248. doi:10.4049/jimmunol.179.6.4239Interaction between transmembrane TNF and TNFR1/2 mediates the activation of monocytes by contact with T cellsJ. Immunol.
Sakurai, H., Suzuki, S., Kawasaki, N., Nakano, H., Okazaki, T., Chino, A., et al. (2003). Tumor necrosis factor-alpha-induced IKK phosphorylation of NF-kappaB p65 on serine 536 is mediated through the TRAF2, TRAF5, and TAK1 signaling pathway. J. Biol. Chem. 278, 36916–36923. doi:10.1074/jbc.M301598200
Salter, M., and Kalia, L. (2004). Src kinases: A hub for NMDA receptor regulation. Nat. Rev. Neurosci. 5, 317–328. doi:10.1038/nrn1368
Samidurai, M., Ramasamy, V., and Jo, J. (2018). β-amyloid inhibits hippocampal LTP through TNFR/IKK/NF-κB pathway. Neurol. Res. 40, 268–276. doi:10.1080/01616412.2018.1436872
Sanders, D., Kaufman, S., DeVos, S., Sharma, A., Mirbaha, H., Li, A., et al. (2014). Distinct tau prion strains propagate in cells and mice and define different tauopathies. Neuron 82, 1271–1288. doi:10.1016/j.neuron.2014.04.047
Scheibye-Knudsen, M., Fang, E., Croteau, D., Wilson, D., and Bohr, V. (2015). Protecting the mitochondrial powerhouse. Trends Cell Biol. 25, 158–170. doi:10.1016/j.tcb.2014.11.002
Schulz, E., Wenzel, P., Münzel, T., and Daiber, A. (2014). Mitochondrial redox signaling: Interaction of mitochondrial reactive oxygen species with other sources of oxidative stress. Antioxid. Redox Signal. 20, 308–324. doi:10.1089/ars.2012.4609
Scimemi, A., Meabon, J., Woltjer, R., Sullivan, J., Diamond, J., and Cook, D. (2013). Amyloid-β1-42 slows clearance of synaptically released glutamate by mislocalizing astrocytic GLT-1. J. Neurosci. 33, 5312–5318. doi:10.1523/jneurosci.5274-12.2013
Selkoe, D. (2002). Alzheimer's disease is a synaptic failure. Sci. (New York, N.Y.) 298, 789–791. doi:10.1126/science.1074069
Shankar, G., Bloodgood, B., Townsend, M., Walsh, D., Selkoe, D., and Sabatini, B. (2007). Natural oligomers of the Alzheimer amyloid-beta protein induce reversible synapse loss by modulating an NMDA-type glutamate receptor-dependent signaling pathway. J. Neurosci. 27, 2866–2875. doi:10.1523/jneurosci.4970-06.2007
Shankar, G., Li, S., Mehta, T., Garcia-Munoz, A., Shepardson, N., Smith, I., et al. (2008). Amyloid-beta protein dimers isolated directly from Alzheimer's brains impair synaptic plasticity and memory. Nat. Med. 14, 837–842. doi:10.1038/nm1782
Sheng, Y., Zhang, L., Su, S., Tsai, L., and Julius Zhu, J. (2016). Cdk5 is a new rapid synaptic homeostasis regulator capable of initiating the early alzheimer-like pathology, Cereb. cortex, 26, 2937–2951. doi:10.1093/cercor/bhv032
Shi, X., Sun, K., Hu, R., Liu, X., Hu, Q., Sun, X., et al. (2016). Blocking the interaction between EphB2 and ADDLs by a small peptide rescues impaired synaptic plasticity and memory deficits in a mouse model of Alzheimer's disease. J. Neurosci. 36, 11959–11973. doi:10.1523/jneurosci.1327-16.2016
Shin, J., and Linden, D. (2005). An NMDA receptor/nitric oxide cascade is involved in cerebellar LTD but is not localized to the parallel fiber terminal. J. Neurophysiol. 94, 4281–4289. doi:10.1152/jn.00661.2005
Silva, D., Esteves, A., Oliveira, C., and Cardoso, S. (2011). Mitochondria: The common upstream driver of amyloid-β and tau pathology in Alzheimer's disease. Curr. Alzheimer Res. 8, 563–572. doi:10.2174/156720511796391872
Sinor, J., Du, S., Venneti, S., Blitzblau, R., Leszkiewicz, D., Rosenberg, P., et al. (2000). NMDA and glutamate evoke excitotoxicity at distinct cellular locations in rat cortical neurons in vitro. J. Neurosci. 20, 8831–8837. doi:10.1523/jneurosci.20-23-08831.2000
Snyder, E., Nong, Y., Almeida, C., Paul, S., Moran, T., Choi, E., et al. (2005). Regulation of NMDA receptor trafficking by amyloid-beta. Nat. Neurosci. 8, 1051–1058. doi:10.1038/nn1503
Sohn, P., Tracy, T., Son, H., Zhou, Y., Leite, R., Miller, B., et al. (2016). Acetylated tau destabilizes the cytoskeleton in the axon initial segment and is mislocalized to the somatodendritic compartment. Mol. Neurodegener. 11, 47. doi:10.1186/s13024-016-0109-0
Sorrentino, V., Romani, M., Mouchiroud, L., Beck, J., Zhang, H., D'Amico, D., et al. (2017). Enhancing mitochondrial proteostasis reduces amyloid-β proteotoxicity. Nature 552, 187–193. doi:10.1038/nature25143
Steiner, P., Higley, M., Xu, W., Czervionke, B., Malenka, R., and Sabatini, B. (2008). Destabilization of the postsynaptic density by PSD-95 serine 73 phosphorylation inhibits spine growth and synaptic plasticity. Neuron 60, 788–802. doi:10.1016/j.neuron.2008.10.014
Takahashi, R., Milner, T., Li, F., Nam, E., Edgar, M., Yamaguchi, H., et al. (2002). Intraneuronal Alzheimer abeta42 accumulates in multivesicular bodies and is associated with synaptic pathology. Am. J. Pathol. 161, 1869–1879. doi:10.1016/s0002-9440(10)64463-x
Takumi, Y., Ramírez-Leín, V., Laake, P., Rinvik, E., and Ottersen, O. (1999). Different modes of expression of AMPA and NMDA receptors in hippocampal synapses. Nat. Neurosci. 2, 618–624. doi:10.1038/10172
Talantova, M., Sanz-Blasco, S., Zhang, X., Xia, P., Akhtar, M., Okamoto, S., et al. (2013). Aβ induces astrocytic glutamate release, extrasynaptic NMDA receptor activation, and synaptic loss. Proc. Natl. Acad. Sci. U. S. A. 110, E2518–E2527. doi:10.1073/pnas.1306832110
Tibbles, L., and Woodgett, J. (1999). The stress-activated protein kinase pathways. Cell. Mol. Life Sci. 55, 1230–1254. doi:10.1007/s000180050369
Tong, H., Zhang, X., Meng, X., Xu, P., Zou, X., and Qu, S. (2017). Amyloid-beta peptide decreases expression and function of glutamate transporters in nervous system cells. Int. J. Biochem. Cell Biol. 85, 75–84. doi:10.1016/j.biocel.2017.01.017
Tracy, T., and Gan, L. (2017). Acetylated tau in Alzheimer's disease: An instigator of synaptic dysfunction underlying memory loss: Increased levels of acetylated tau blocks the postsynaptic signaling required for plasticity and promotes memory deficits associated with tauopathy. BioEssays 39, 1600224. doi:10.1002/bies.201600224
Tracy, T., Sohn, P., Minami, S., Wang, C., Min, S., Li, Y., et al. (2016). Acetylated tau obstructs KIBRA-mediated signaling in synaptic plasticity and promotes tauopathy-related memory loss. Neuron 90, 245–260. doi:10.1016/j.neuron.2016.03.005
Tu, S., Okamoto, S., Lipton, S., and Xu, H. (2014). Oligomeric Aβ-induced synaptic dysfunction in Alzheimer's disease. Mol. Neurodegener. 9, 48. doi:10.1186/1750-1326-9-48
Um, J., Kaufman, A., Kostylev, M., Heiss, J., Stagi, M., Takahashi, H., et al. (2013). Metabotropic glutamate receptor 5 is a coreceptor for Alzheimer aβ oligomer bound to cellular prion protein. Neuron 79, 887–902. doi:10.1016/j.neuron.2013.06.036
Usenovic, M., Niroomand, S., Drolet, R., Yao, L., Gaspar, R., Hatcher, N., et al. (2015). Internalized tau oligomers cause neurodegeneration by inducing accumulation of pathogenic tau in human neurons derived from induced pluripotent stem cells. J. Neurosci. 35, 14234–14250. doi:10.1523/jneurosci.1523-15.2015
Valerio, A., Boroni, F., Benarese, M., Sarnico, I., Ghisi, V., Bresciani, L., et al. (2006). NF-kappaB pathway: A target for preventing beta-amyloid (Abeta)-induced neuronal damage and Abeta42 production. Eur. J. Neurosci. 23, 1711–1720. doi:10.1111/j.1460-9568.2006.04722.x
Varela-Nallar, L., Alfaro, I., Serrano, F., Parodi, J., and Inestrosa, N. (2010). Wingless-type family member 5A (Wnt-5a) stimulates synaptic differentiation and function of glutamatergic synapses. Proc. Natl. Acad. Sci. U. S. A. 107, 21164–21169. doi:10.1073/pnas.1010011107
Varga, E., Juhász, G., Bozsó, Z., Penke, B., Fülöp, L., and Szegedi, V. (2014). Abeta(1-42) enhances neuronal excitability in the CA1 via NR2B subunit-containing NMDA receptors. Neural Plast. 2014. 584314. doi:10.1155/2014/584314
Varga, E., Juhász, G., Bozsó, Z., Penke, B., Fülöp, L., and Szegedi, V. (2015). Amyloid-β1-42 disrupts synaptic plasticity by altering glutamate recycling at the synapse. J. Alzheimers Dis. 45, 449–456. doi:10.3233/jad-142367
von Bernhardi, R., and Eugenín, J. (2012). Alzheimer's disease: Redox dysregulation as a common denominator for diverse pathogenic mechanisms. Antioxid. Redox Signal. 16, 974–1031. doi:10.1089/ars.2011.4082
Vossel, K., Zhang, K., Brodbeck, J., Daub, A., Sharma, P., Finkbeiner, S., et al. (2010). Tau reduction prevents Abeta-induced defects in axonal transport. Sci. (New York, N.Y.) 330, 198. doi:10.1126/science.1194653
Wang, Y., and Mandelkow, E. (2016). Tau in physiology and pathology. Nat. Rev. Neurosci. 17, 5–21. doi:10.1038/nrn.2015.1
Wang, Q., Rowan, M., and Anwyl, R. (2004a). Beta-amyloid-mediated inhibition of NMDA receptor-dependent long-term potentiation induction involves activation of microglia and stimulation of inducible nitric oxide synthase and superoxide. J. Neurosci. 24, 6049–6056. doi:10.1523/jneurosci.0233-04.2004
Wang, Q., Walsh, D., Rowan, M., Selkoe, D., and Anwyl, R. (2004b). Block of long-term potentiation by naturally secreted and synthetic amyloid beta-peptide in hippocampal slices is mediated via activation of the kinases c-Jun N-terminal kinase, cyclin-dependent kinase 5, and p38 mitogen-activated protein kinase as well as metabotropic glutamate receptor type 5. J. Neurosci. 24, 3370–3378. doi:10.1523/jneurosci.1633-03.2004
Wang, Q., Wu, J., Rowan, M., and Anwyl, R. (2005). Beta-amyloid inhibition of long-term potentiation is mediated via tumor necrosis factor. Eur. J. Neurosci. 22, 2827–2832. doi:10.1111/j.1460-9568.2005.04457.x
Wei, J., Liu, W., and Yan, Z. (2010a). Regulation of AMPA receptor trafficking and function by glycogen synthase kinase 3. J. Biol. Chem. 285, 26369–26376. doi:10.1074/jbc.M110.121376
Wei, W., Nguyen, L., Kessels, H., Hagiwara, H., Sisodia, S., and Malinow, R. (2010b). Amyloid beta from axons and dendrites reduces local spine number and plasticity. Nat. Neurosci. 13, 190–196. doi:10.1038/nn.2476
Yang, Y., Ji, W., Zhu, Z., Wu, Y., Zhang, Z., and Qu, S. (2018). Rhynchophylline suppresses soluble Aβ1-42-induced impairment of spatial cognition function via inhibiting excessive activation of extrasynaptic NR2B-containing NMDA receptors. Neuropharmacology 135, 100–112. doi:10.1016/j.neuropharm.2018.03.007
Yu, T., Robotham, J., and Yoon, Y. (2006). Increased production of reactive oxygen species in hyperglycemic conditions requires dynamic change of mitochondrial morphology. Proc. Natl. Acad. Sci. U. S. A. 103, 2653–2658. doi:10.1073/pnas.0511154103
Yun, S., Gamkrelidze, G., Stine, W., Sullivan, P., Pasternak, J., Ladu, M., et al. (2006). Amyloid-beta1-42 reduces neuronal excitability in mouse dentate gyrus. Neurosci. Lett. 403, 162–165. doi:10.1016/j.neulet.2006.04.065
Yuste, R., and Bonhoeffer, T. (2001). Morphological changes in dendritic spines associated with long-term synaptic plasticity. Annu. Rev. Neurosci. 24, 1071–1089. doi:10.1146/annurev.neuro.24.1.1071
Zempel, H., Thies, E., Mandelkow, E., and Mandelkow, E. (2010). Abeta oligomers cause localized Ca(2+) elevation, missorting of endogenous Tau into dendrites, Tau phosphorylation, and destruction of microtubules and spines. J. Neurosci. 30, 11938–11950. doi:10.1523/jneurosci.2357-10.2010
Zhao, D., Watson, J., and Xie, C. (2004). Amyloid beta prevents activation of calcium/calmodulin-dependent protein kinase II and AMPA receptor phosphorylation during hippocampal long-term potentiation. J. Neurophysiol. 92, 2853–2858. doi:10.1152/jn.00485.2004
Zhou, J., Mo, Z., and Zhou, S. (2010). Rhynchophylline down-regulates NR2B expression in cortex and hippocampal CA1 area of amphetamine-induced conditioned place preference rat. Arch. Pharm. Res. 33, 557–565. doi:10.1007/s12272-010-0410-3
Zhu, Y., Pak, D., Qin, Y., McCormack, S., Kim, M., Baumgart, J., et al. (2005). Rap2-JNK removes synaptic AMPA receptors during depotentiation. Neuron 46, 905–916. doi:10.1016/j.neuron.2005.04.037
Keywords: synaptic dysfunction, Alzheimer’s disease, β-amyloid, glutamate receptors, synaptic plasticity, synapse toxicity
Citation: Zhang H, Jiang X, Ma L, Wei W, Li Z, Chang S, Wen J, Sun J and Li H (2022) Role of Aβ in Alzheimer’s-related synaptic dysfunction. Front. Cell Dev. Biol. 10:964075. doi: 10.3389/fcell.2022.964075
Received: 08 June 2022; Accepted: 01 August 2022;
Published: 26 August 2022.
Edited by:
Yuzuru Imai, Juntendo University, JapanReviewed by:
Nibaldo C. Inestrosa, Pontificia Universidad Católica de Chile, ChileP. Hemachandra Reddy, Texas Tech University Health Sciences Center, United States
Balaji Krishnan, University of Texas Medical Branch at Galveston, United States
Copyright © 2022 Zhang, Jiang, Ma, Wei, Li, Chang, Wen, Sun and Li. This is an open-access article distributed under the terms of the Creative Commons Attribution License (CC BY). The use, distribution or reproduction in other forums is permitted, provided the original author(s) and the copyright owner(s) are credited and that the original publication in this journal is cited, in accordance with accepted academic practice. No use, distribution or reproduction is permitted which does not comply with these terms.
*Correspondence: Hao Li, eHlocGxpaGFvMTk2NUAxMjYuY29t