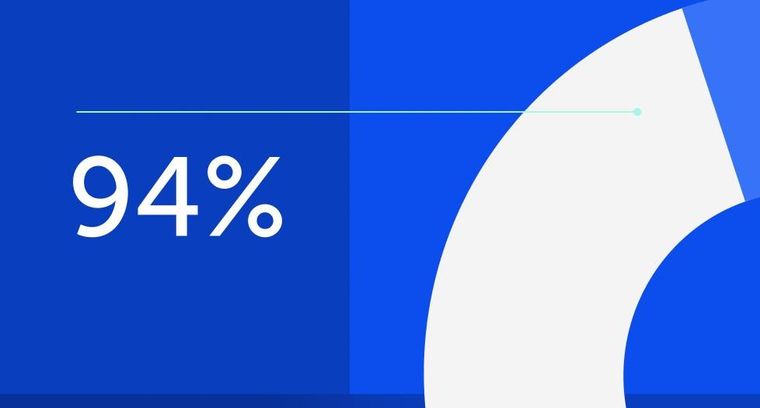
94% of researchers rate our articles as excellent or good
Learn more about the work of our research integrity team to safeguard the quality of each article we publish.
Find out more
REVIEW article
Front. Cell Dev. Biol., 07 September 2022
Sec. Cell Adhesion and Migration
Volume 10 - 2022 | https://doi.org/10.3389/fcell.2022.954099
This article is part of the Research TopicFrom Mechanosensing to Signaling and the Cell Response: The Ion Channel ForceView all 5 articles
Physical cues have emerged as critical influencers of cell function during physiological processes, like development and organogenesis, and throughout pathological abnormalities, including cancer progression and fibrosis. While ion channels have been implicated in maintaining cellular homeostasis, their cell surface localization often places them among the first few molecules to sense external cues. Mechanosensitive ion channels (MICs) are especially important transducers of physical stimuli into biochemical signals. In this review, we describe how physical cues in the tumor microenvironment are sensed by MICs and contribute to cancer metastasis. First, we highlight mechanical perturbations, by both solid and fluid surroundings typically found in the tumor microenvironment and during critical stages of cancer cell dissemination from the primary tumor. Next, we describe how Piezo1/2 and transient receptor potential (TRP) channels respond to these physical cues to regulate cancer cell behavior during different stages of metastasis. We conclude by proposing alternative mechanisms of MIC activation that work in tandem with cytoskeletal components and other ion channels to bestow cells with the capacity to sense, respond and navigate through the surrounding microenvironment. Collectively, this review provides a perspective for devising treatment strategies against cancer by targeting MICs that sense aberrant physical characteristics during metastasis, the most lethal aspect of cancer.
Cells possess complex mechanisms to tightly regulate the molecular machinery involved in proliferation, migration and apoptosis. In contrast, cancer cells frequently lack critical checkpoints and/or homeostatic mechanisms, resulting in sustained proliferation and/or decreased apoptosis, which lead to the formation of primary tumors and enhanced invasion into the surrounding stroma, thereby often culminating in the formation of metastases (Hanahan and Weinberg, 2011). Traditionally, genetic modifications and biochemical factors have been implicated in transforming resident cells into metastatic cancer cells. More recently, however, physical cues have emerged as critical mediators of this transformation (Emon et al., 2018).
The extracellular matrix (ECM) provides biochemical and biophysical cues which can influence cancer progression and metastasis. The ECM is an intricate three-dimensional (3D) network composed of proteoglycans and fibrous proteins, such as collagens, elastins, fibronectins and laminins, which provides structural support to tissues (Winkler et al., 2020). These fibers provide critical attachment points for cells to facilitate the proper development and organization of tissues (Rozario and DeSimone, 2010). Additionally, the ECM provides docking sites for cytokines, growth factors, and other bioactive molecules which promote the growth, differentiation and maintenance of cells by engaging intracellular signaling pathways (Taipale and Keski-Oja, 1997; Schonherr and Hausser, 2000). Aberrant changes in ECM structure and organization can perturb tissue homeostasis by promoting abnormal cell proliferation, elevated contractility, and transformation due to transcriptional changes (Wozniak et al., 2003; Paszek et al., 2005; Ondeck et al., 2019; Stowers et al., 2019). As tumor cells proliferate, the surrounding ECM undergoes further architectural changes which impact cellular physiology, resulting in a sustained feedforward loop that contributes to disease progression (Bonnans et al., 2014; Winkler et al., 2020).
Cancer cells in the primary tumor experience mechanical compression, hydraulic pressure, fluid viscosity, substrate stiffness and viscoelasticity (Wisdom et al., 2018; Nia et al., 2020; Park et al., 2020) (Table 1). As cells disseminate from the primary tumor and invade into the local tissue microenvironment, they encounter additional mechanical forces due to the organization and structure of the ECM. Cancer cells must navigate a variety of topographical features, such as ECM fibers, small pores and channel-like tracks with prescribed physical properties to reach the vasculature (Paul et al., 2017). Fiber architecture and cross-linking density regulate ECM porosity, stiffness and viscoelasticity (Yamada and Sixt, 2019; Chaudhuri et al., 2020). Extracellular fluid, which hydrates the ECM, also exerts osmotic pressure and hydraulic resistance (Table 1) due to hydrostatic pressure and fluid viscosity on migrating cells (Li et al., 2020a). Once cancer cells enter the vasculature, they must withstand shear stress caused by blood flow to disseminate throughout the body (Shen and Kang, 2020). Thus, cancer cells must sense, integrate and interpret a multitude of physical forces during each step of the metastatic cascade to successfully colonize distant organs/tissues (Figure 1).
FIGURE 1. Schematic depicting the contribution of various physical cues during different steps of the metastatic cascade. In the primary tumor, cancer cells experience mechanical compression and hydraulic pressure as well as different levels of substrate stiffness, viscoelasticity and extracellular fluid viscosity. Following primary tumor cell dissemination, invading cancer cells encounter substrate stiffness and viscoelasticity, mechanical compression, fluid viscosity, solid barriers, confined tracks, and other topographies within the local tissue microenvironment. During intravasation, cancer cells experience shear stress caused by blood flow and continue to be exposed to this physical cue while in circulation. The viscosity of blood is also elevated relative to interstitial fluids, which potentially impacts cell behavior. As cancer cells arrest to the vascular endothelium and extravasate out of the bloodstream, they experience shear stress from the blood flow. Taken together, cancer cells are exposed to many different physical forces during the metastatic cascade which they must sense, integrate and interpret to engage appropriate cellular mechanisms for efficient dissemination. Schematic created with BioRender.com.
Mechanosensitive ion channels (MICs), or stretch-activated ion channels, are a family of pore-forming proteins that play an important role in sensing and transducing physical stimuli. Their cell surface localization often places them among the first few molecules to sense external physical cues and initiate intracellular biochemical cascades in a process known as mechanotransduction (Patel et al., 2010; Ranade et al., 2015; Canales Coutiño and Mayor, 2021). To sense complex physical cues and changes within the microenvironment, MICs facilitate Ca2+ entry into cells through a mechanism known as channel gating (Table 2). This process requires a threshold level of membrane tension to initiate a conformational change from a closed to open state. The gating process can also depend on transmembrane voltage, which is regulated by ion channels (Yellin et al., 2018; Li et al., 2020a). Mechanosensitive gating mechanisms can be broadly categorized into 2 types: 1) direct mechanosensing, which is regulated by physical changes in the cell membrane, and 2) indirect mechanosensing, which is regulated by intracellular signaling cascades (Patel et al., 2010; Ranade et al., 2015; Petho et al., 2019).
Compressive forces in the primary tumor, fluid shear stress in the blood vessels, and other mechanical stimuli that cause local changes in cell membrane curvature, composition and tension can directly open MICs such as Piezo1/2, transient receptor potential cation channel subfamily C member 1 (TRPC1), and transient receptor potential cation channel subfamily V member 4 (TRPV4) (Petho et al., 2019). Alternatively, mechanosensitive molecules on the plasma membrane can indirectly trigger MIC activation through a multi-step process involving several intermediate signaling molecules. This mechanism typically relies on the ability of G-protein coupled receptors to detect changes in the extracellular environment and induce a conformational change in MICs, such as transient receptor potential cation channel subfamily M member 7 (TRPM7) and TRPV4 (Petho et al., 2019). Self-generated forces arising from actomyosin contractility can also lead to the activation of MICs in migrating cells (Lee et al., 2021). For example, tension in actin cytoskeletal scaffolds and enhanced signaling through integrins (cell-matrix interactions) and cadherins (cell-cell interactions) can indirectly influence the gating of MICs (Petho et al., 2019).
While the role of MICs in nerves and sensory cells involved in sight, smell, taste and touch is well-characterized (Ranade et al., 2015), their expression and function in cancer progression has recently received attention (Adapala et al., 2016; Dombroski et al., 2021; Karki and Tojkander, 2021; Yankaskas et al., 2021). Given that cancer cells are subject to a multitude of forces that continuously change with the local microenvironment, it is important to understand how MICs regulate cell behavior during each step of the metastatic cascade. Here, we provide a comprehensive overview of physical cues that cancer cells experience and how MICs allow cells to sense and interpret these forces. In particular, we discuss the roles of Piezo1/2, TRPC1, TRPC5, TRPM2, TRPM4, TRPM7, TRPV2, and TRPV4 in mechanosensing and cancer metastasis.
Piezo1 (Fam38A) and Piezo2 (Fam38B) are mechanically-activated cation channels located in the plasma membrane of vertebrates (Coste et al., 2010). Structurally, they are pore-forming membrane proteins with numerous transmembrane regions that are directly activated by mechanical stimuli applied to the cell membrane like other MICs (Table 2); however, they do not share sequence homology with any known ion channels or receptors. Compared to other MICs, which can be activated by both mechanical and chemical stimuli, Piezo1/2 are the only channels primarily gated by mechanical stimuli (De Felice and Alaimo, 2020), including compression, shear stress, membrane stretching, poking, and suction (Table 1).
Since their discovery in 2010, the physiological roles of Piezo channels have been extensively studied. Piezo1 was initially identified in mouse neuroblastoma cells as a critical mediator of mechanically-activated currents, but subsequently found to be expressed in the bladder, colon, lungs, kidneys and skin (Coste et al., 2010). Piezo1 plays essential regulatory roles in bone anabolism (Li et al., 2019a), iron metabolism (Ma et al., 2021), innate immunity (Solis et al., 2019), vascular development (Ranade et al., 2014a) and erythrocyte volume homeostasis (Cahalan et al., 2015; Cinar et al., 2015). Piezo1 is also required for neurogenesis (Pathak et al., 2014), cell migration (Hung et al., 2016; Mousawi et al., 2020) and axonal pathfinding and growth (Koser et al., 2016). Additionally, Piezo1 controls epithelial cell numbers by inducing cell division in sparse environments and cell extrusion in crowded environments (Gudipaty et al., 2017), and regulates endosomal trafficking for efficient cytokinetic abscission of nascent daughter cells (Carrillo-Garcia et al., 2021). Consequently, Piezo1 expression is required for embryonic development (Ranade et al., 2014a).
Piezo2, which shares ∼42% sequence homology with Piezo1, was identified in dorsal root and trigeminal ganglia sensory neurons, where it facilitates a subset of kinetically distinct mechanically-activated currents involved in somatosensation and proprioception (Coste et al., 2010; Chesler et al., 2016). Piezo2 is also expressed in the bladder, colon, lungs, kidneys and skin (Coste et al., 2010). Like Piezo1, Piezo2 participates in a diverse set of mechanisms, including Merkel cell sensitivity to light touch, itch, mechanical pain, proprioception, baroreflex, breathing and bladder control (Szczot et al., 2021). Piezo2 is primarily involved in mechanosensation; however, global knockout of gene expression results in perinatal lethality (Ranade et al., 2014b) like Piezo1 (Ranade et al., 2014a). Thus, there are likely many other functions of Piezo2 that remain to be identified.
A growing body of evidence indicates that the expression and function of Piezo1/2 are altered in cancer. Piezo1 is abnormally expressed in tissues typically exposed to high levels of mechanical stress. Specifically, breast (Li et al., 2015), colorectal (Sun et al., 2020), gastric (Wang et al., 2021) and prostate (Han et al., 2019) cancer exhibit elevated levels of expression, as well as gliomas (Zhou et al., 2020) and oral squamous cell carcinoma (OSCC) (Hasegawa et al., 2021). Piezo2 is also upregulated in breast cancer (Pardo-Pastor et al., 2018) and gliomas (Yang et al., 2016).
The TRP superfamily of MICs consists of 7 different subfamilies based on sequence homology, including TRPA, TRPC, TRPM, TRPML, TRPN, TRPP, and TRPV, all of which are expressed in mammalian cells except for TRPN (Montell, 2005). TRP channels are primarily localized to the plasma membrane and endoplasmic reticulum and can be activated by various stimuli such as heat, tension, pH, osmolarity and pressure (Table 1) (Pedersen and Nilius, 2007; Patel et al., 2010). Most TRP channels function as tetramers with six putative transmembrane segments (S1-S6) and a pore-forming re-entrant loop between S5 and S6. Except for TRPM4 and TRPM5, most TRPs are also non-selective cation channels that permit Na+, K+, Ca2+ and/or Mg2+ influx to varying degrees depending on their specific structure. Increased cation entry can affect cellular function directly or by enhancing intracellular signaling cascades which lead to the downstream release of calcium (Pedersen and Nilius, 2007; Petho et al., 2019). Importantly, TRP channels also regulate the proliferation, differentiation, migration, invasion and chemoresistance of cancer cells by sensing osmotic perturbations, shear forces and hydrostatic pressure (Clapham, 2003; Liedtke et al., 2003; Gomis et al., 2008; Mendoza et al., 2010; Shen et al., 2015; Zhao et al., 2019; Yankaskas et al., 2021; Zhao et al., 2021) (Table 1). Several members of the TRP superfamily also participate in actin remodeling and focal adhesion dynamics in response to mechanical stimuli (Kobayashi and Sokabe, 2010; Shen et al., 2015; Zhao et al., 2019). TRP channels implicated in mechanotransduction mechanisms that regulate cancer cell migration, invasion and metastasis include, among others, TRPC1, TRPC5, TRPM2, TRPM7, TRPM4, TRPV2 and TRPV4 (Table 3) (Patel et al., 2010; Canales et al., 2019).
Cancer arises from defects in regulatory circuits that control normal cell processes. Several lines of evidence suggest that tumor cells acquire a self-sufficiency in growth signals, an insensitivity to growth suppression, limitless replicative potential, sustained angiogenesis and the ability to evade apoptosis (Hanahan and Weinberg, 2011). These characteristics enable tumor cells at the primary site to outgrow and dominate their local tissue environment.
Cells encounter a variety of physical forces within the primary tumor, including mechanical compression, hydraulic pressure and fluid viscosity (Figure 1; Table 1). As described earlier, MICs are a diverse group of ion channels that allow cells to sense, interpret and respond to a multitude of mechanical stimuli (Ranade et al., 2015). Thus, a detailed understanding of these forces is required to contextualize how MICs may be involved in the first few steps of the tumor cell transformation and the initiation of cancer metastasis.
As murine or human cancer cells proliferate in the mammary fat pad of mice, the total tumor volume expands and deforms the surrounding tissue, leading to the accumulation of compressive stress upwards of 150 mm Hg (20 kPa) (Stylianopoulos et al., 2012; Jain et al., 2014). Increasing tumor volume also generates mechanical compression in the tumor (Figure 1; Table 1). The accumulation of compressive stress is evident from force balance and the observed stress relaxation of tumor halves when excised tumors are cut along their longest axis; without the application of any external load, “cutting” releases internal stress, resulting in simultaneous bulging at the center and retraction at the boundary of the tumor, which are indicative of compression in the intratumoral region and radial stress at the periphery, respectively (Stylianopoulos et al., 2012).
Compressive stress in the tumor interior can influence the shape of the tumor mass and alter cancer cell biology, resulting in enhanced invasiveness (Nia et al., 2020). Stress levels equivalent to those encountered in the native breast tumor microenvironment drive cancer cells towards a more invasive phenotype (Tse et al., 2012) by altering gene expression profiles that remodel the ECM and tumor vessels (Demou, 2010; Rivron et al., 2012), thereby facilitating tumor dissemination out of a primary tumor mass. For example, compression can enhance breast cancer cell migration by inducing the formation of new adhesion contacts with the substrate via localized fibronectin secretion (Tse et al., 2012), and increase invasion by engaging matrix metalloproteinases (MMPs) (Luo et al., 2022). In particular, mechanical compression of breast cancer cells stimulates Ca2+ influx through Piezo1, leading to Src/ERK pathway activation, invadopodia formation, increased matrix degradation and enhanced cell invasion (Luo et al., 2022). The localization and function of Piezo1 is dependent on the integrity of caveolae in the cell membrane as knockdown of Cav-1 abrogates Ca2+ influx and cell invasion (Luo et al., 2022). High compressive stress inside spheroid models of breast cancer also promotes an invasive phenotype by facilitating water flow across cell boundaries from the tumor core to the periphery and invasive front via gap junctions (Han et al., 2020). Interestingly, this pattern of cell volume regulation is preserved in tissue samples from human patients; namely, cells positioned progressively further from the tumor core exhibit greater volumes (Han et al., 2020). Of note, high stress can induce growth arrest and apoptosis of murine mammary carcinoma cells in the interior of tumor spheroids, while anisotropic forces can pattern tumor volume increase in the direction of least stress (Cheng et al., 2009).
The hydraulic environment around tumors is largely determined by the composition of fluids combined with the structure and organization of blood and lymphatic vessels (Koumoutsakos et al., 2013). Dynamic ECM deformation enhances microvascular network formation in vitro by altering gene expression (Ruehle et al., 2020). Compressive stresses can also pinch blood and lymphatic vessels at the tumor interior and transform the cross-sectional area of such vessels at the peritumoral region into elliptical shapes. The compression of leaky blood vessels, which are frequently associated with cancer progression (Hashizume et al., 2000), results in elevated interstitial fluid pressure (IFP) (Table 1) (Cheng et al., 2009) by increasing fluid flux from the vasculature into interstitial spaces (Figure 1). Concurrently, compression of lymphatic vessels results in improper drainage of excessive interstitial fluids, which further increases IFP (Jain and Baxter, 1988; Baxter and Jain, 1989). Overall, the haphazard and leaky tumor vasculature contributes to higher IFP, which can drive local breast cancer cell dissemination and metastasis (Haessler et al., 2012; Li et al., 2020a).
Elevated IFP at the tip of three-dimensional (3D) aggregates of human breast cancer (MDA-MB-231) cells increases invasion by enhancing the expression of genes associated with epithelial-to-mesenchymal transition (EMT), namely Snail and vimentin (Piotrowski-Daspit et al., 2016). Interestingly, increased IFP at the invasive tips also enhances the expression of the epithelial marker E-cadherin (Piotrowski-Daspit et al., 2016), whose role in breast cancer progression is debated, yet has been demonstrated to support metastasis (Kowalski et al., 2003; Padmanaban et al., 2019). Elevated IFP also increases the volume, motility and invasiveness of human lung cancer (CL1-5 and A549) cells due to ERK1/2 pathway activation (Kao et al., 2017). Hydrostatic pressure initiates a cascade of caveolin-1, Akt1/2, ERK1/2 and cortactin phosphorylation which enhances filopodia formation and drives the increased expression of aquaporin 1 (AQP1), Snail and vinculin (Kao et al., 2017). Importantly, hydrostatic pressure does not significantly enhance the volume, motility or proliferation of normal lung epithelial (BEAS-2B) cells due to negligible changes in the expression of AQP1 and the phosphorylation status of caveolin-1 and ERK1/2 (Kao et al., 2017).
The viscosity of extracellular fluids also constitutes an important physical parameter that varies throughout the human body during both healthy and diseased states (Figure 1; Table 1). The fluids surrounding primary tumors have elevated viscosity due to deregulated blood circulation (Sun et al., 2007) and increased amounts of ECM degradation (Ellis, 2001). Additionally, resident epithelial and cancer cells secrete macromolecules, such as mucins, which further increase fluid viscosity (Ellis, 2001). Magnetic resonance elastography studies of patient samples reveal local upregulation of shear viscosity at tumor sites, with malignant breast tumor masses displaying 3-fold greater shear viscosity than benign tumors (Sinkus et al., 2005; Kumar et al., 2018). Although magnetic resonance electrograms measure the material properties of bulk tumor masses, a recent development in molecular rotor-based fluorescent sensors has enabled the measurement of fluid viscosities in vivo (Yin et al., 2021). Importantly, in vivo imaging of a viscosity-activated fluorescent probe in mice bearing murine breast tumors confirmed the existence of significantly elevated fluid viscosity in tumors compared to native tissues (Yin et al., 2021). Unfortunately, it remains elusive how cells in the primary tumor respond to physiologically-relevant values of viscosities. Further work is required to elucidate the impact of extracellular fluid viscosity on cancer cell behavior and uncover the molecular mechanisms that are engaged by this physical cue.
ECM structure surrounding primary tumor sites is often modified compared to native tissues (Winkler et al., 2020) resulting in unique topographies which cells can use to invade out of the primary tumor region. Cancer cells can remodel the ECM by promoting the secretion and deposition of additional matrix components; altering the organization of fibers through proteolytic cleavage and chemical modifications; and applying mechanical forces on existing fibers (Winkler et al., 2020). In turn, cells can sense and respond to material properties and the architecture of ECM fibers (Sharma et al., 2013; Doyle et al., 2015; Mukherjee et al., 2019). Increased collagen deposition, alignment and cross-linking affect the stiffness (Micalet et al., 2021) and viscoelasticity (Mierke, 2021) of the local microenvironment and establish migration paths for cells to escape the primary tumor (Figure 1).
ECM deposition, modification and organization during cancer progression contribute to increased matrix stiffness or elasticity (Figure 1; Table 1). In the case of breast cancer, shear wave elastography reveals that tumor stiffness increases with tumor grade and disease progression; benign lesions measure ∼45 ± 40 kPa whereas malignant lesions can reach ∼147 ± 40 kPa (Athanasiou et al., 2010; Bae et al., 2017). Nanoscale atomic force microscopy indentation of breast tissue samples also demonstrates a dramatic stiffening of invasive ductal carcinomas (>5 kPa) compared to normal tissue (∼0.4 kPa) (Paszek et al., 2005). Additionally, stromal stiffness at the invasive edge of tumors is 4-fold greater than non-invasive regions (Paszek et al., 2005).
Collagen is the most abundant ECM component deposited during breast cancer progression (Schedin and Keely, 2011; Naba et al., 2014). Increased stromal collagen in mice promotes tumor initiation, cancer cell invasion and lung metastasis (Provenzano et al., 2008). Accordingly, elevated collagen is a risk factor for breast cancer (Li et al., 2005). Increased collagen crosslinking and stiffening of the ECM promote focal adhesion formation and enhanced phosphoinositide 3-kinase (PI3K) activity in human mammary epithelial cells. Along these lines, inhibition of lysyl oxidase (LOX)-mediated collagen crosslinking decreases focal adhesions, reduces PI3K activity, and impedes tumor progression in mice (Levental et al., 2009). Deregulated ECM architecture also affects the progression of many other cancer types, including colon (Birk et al., 2014), prostate (Kapinas et al., 2012) and ovarian (Nadiarnykh et al., 2010) cancer. It is important to note that the stiffness of the local microenvironment varies considerably due to tumor heterogeneity (Plodinec et al., 2012). Tissue stiffness also varies widely throughout the body (Handorf et al., 2015). Thus, cancer cells must constantly sense and adapt to local changes in stiffness as they disseminate from the primary tumor and migrate towards the vasculature (Figure 1).
Cells can sense the rigidity and topography (Table 1) of the underlying ECM through cell-matrix adhesions (Box 1) (Geiger et al., 2009), which connect the cellular cytoskeleton to the local microenvironment. More recently, the role of MICs in substrate stiffness sensing has also emerged. For example, primary mouse chondrocytes cultured on polydimethylsiloxane (PDMS) exhibit elevated Ca2+ signaling with increasing stiffness (2–197 kPa); TRPV4 preferentially mediates Ca2+ influx on stiffer (197 and 78 kPa) substrates, whereas Piezo1/2 facilitates Ca2+ influx on softer (54 and 2 kPa) substrates (Du et al., 2021). In the context of prostate cancer, TRPV4 expression in murine tumor endothelial cells regulates Ca2+ influx and Rho activity in response to substrate stiffness (Adapala et al., 2016). Endothelial cells with diminished levels of TRPV4 exhibit reduced mechanosensitivity, which increases cell migration on stiffer substrates and decreases VE-cadherin at cell-cell junctions, leading to abnormal angiogenesis, enhanced tumor growth, and enhanced lung metastasis (Adapala et al., 2016; Cappelli et al., 2019).
Box 1 | Cell-matrix adhesions are important structures that facilitate cancer cell migration and mechanosensing. Adhesions are composed of integrins and cytoplasmic proteins that form a <200 nm plaque containing at least 156 components with 690 interactions (Zaidel-Bar et al., 2007a; Kuo et al., 2011). There are four main classes of adhesions which are distinguished by their size, protein composition and lifetime: nascent adhesions, focal complexes, focal adhesions and fibrillar adhesions (Wolfenson et al., 2013). Nascent adhesions at the leading edge of migratory cells are <1 μm in diameter, primarily composed of integrins, talin and paxillin, and have a lifespan <60 s (Laukaitis et al., 2001; Zaidel-Bar et al., 2003; Wolfenson et al., 2013). Nascent adhesions mature into focal complexes upon vinculin recruitment and attachment to the actin cytoskeleton (DePasquale and Izzard, 1987). Mechanical force promotes the recruitment of more scaffold proteins and strengthening of the adhesion-actin cytoskeleton link, leading to the formation of focal adhesions (Galbraith et al., 2002; Choi et al., 2008). Focal adhesions are larger than focal complexes (1 μm wide × 3–5 μm long), contain zyxin, and have a considerably longer lifetime (>8.5 min) (Zaidel-Bar et al., 2003; Zaidel-Bar et al., 2007b). Continued force application transitions focal adhesions into fibrillar adhesions, which are significantly longer, contain tensin, and have a very long lifetime (∼42 min) (Pankov et al., 2000; Zaidel-Bar et al., 2007b). Cells typically form adhesions along ECM bundles of collagen and fibronectin (Harunaga and Yamada, 2011; Doyle et al., 2015). Nascent adhesions are initially formed at the leading edge of cells in a force- and stiffness-independent fashion (Nayal et al., 2006; Choi et al., 2008). Many of these small adhesions quickly decay while a few continue to increase in size and move towards the cell interior (Parsons et al., 2010). Substrate stiffness increases the fraction of adhesions that mature into large complexes (Discher et al., 2005) by unmasking additional binding sites on adhesion proteins (Horton et al., 2016; del Rio et al., 2009). Adhesions on stiff matrices also experience stronger forces from actomyosin contraction, and generate stronger frictional forces on the actin cytoskeleton (Walcott and Sun, 2010). For this reason, collagen crosslinking and matrix stiffening promote adhesion formation and maturation (Levental et al., 2009; Provenzano et al., 2009; Walcott et al., 2011). Increased ECM stiffness also induces EMT (Wei et al., 2015), which is associated with tumor cell dissemination, immune evasion and chemoresistance (Aiello and Kang, 2019). Mesenchymal cell migration is highly dependent on adhesion dynamics. Accordingly, upregulation of proteins that enhance actin cytoskeleton and adhesion dynamics is often observed in invasive and metastatic cancer cells, while inhibition of these mediators is beneficial in blocking cell migration (Yamaguchi and Condeelis, 2007; Wendt and Schiemann, 2009). Force fluctuations within adhesions are converted into biochemical signals through a focal adhesion kinase (FAK)/phosphopaxillin/vinculin signaling pathway (Plotnikov et al., 2012). Other studies have also identified FAK (Klein et al., 2009; Wang et al., 2019), vinculin (Plotnikov et al., 2012; Stutchbury et al., 2017), talin (del Rio et al., 2009; Austen et al., 2015; Elosegui-Artola et al., 2016) and zyxin (Yoshigi et al., 2005; Hirata et al., 2008) as mechanosensitive proteins required for substrate stiffness sensing. These proteins allow cells to rapidly respond to changes in substrate stiffness (Pelham and Wang, 1997; Lo et al., 2000) and migrate towards stiffer areas almost immediately after substrate stretching or compression (Lo et al., 2000) through a process known as durotaxis (DuChez et al., 2019). Mathematical models of steady-state cell speed suggest cells migrate optimally within a narrow range of stiffnesses, with soft or very stiff substrates both resulting in less cell migration (Pelham and Wang, 1997; Dokukina and Gracheva, 2010). In agreement with this hypothesis, glioma (U251) cells exhibit preferential migration towards substrates with a stiffness of 10 kPa from both softer and stiffer regions (Bangasser et al., 2017). Small adhesions formed on soft substrates fail to support traction forces required for cell migration. Conversely, stiffer substrates induce cell spreading, adhesion formation, and adhesion maturation, which collectively result in slower migration (Discher et al., 2005).
It has been postulated that local stretching of the plasma membrane near focal adhesions (Box 1) activates MICs, which ultimately control cell migration by regulating actomyosin contractility and the fate of focal adhesions (Kobayashi and Sokabe, 2010). Ca2+ flickers have been observed near adhesions in migrating human embryonic lung fibroblasts (Wei et al., 2009). Rapid local application of an RGD sequence recognized by integrins enhances flicker activity. In contrast, TRPM7 knockdown and myosin inhibition prevent flicker production (Wei et al., 2009). Ca2+ entry through membrane-associated channels impacts adhesion dynamics by activating calpain, a cysteine protease (Khorchid and Ikura, 2002) that cleaves several adhesion components, including integrin (Du et al., 1995), focal adhesion kinase (FAK) (Chan et al., 2010) and talin (Franco et al., 2004). In fact, calpain-mediated proteolysis of talin is a rate-limiting step during adhesion turnover (Franco et al., 2004); calpain inhibition decreases integrin release from the cell membrane, leading to a reduction in cell speed (Huttenlocher et al., 1997). Accordingly, human glioma (U87), breast cancer (T47D) and embryonic kidney (HEK) cells expressing a defective form of TRPV4 exhibit long protrusions with large, elongated adhesions that prevent migration (Mrkonjić et al., 2015). In contrast, cells overexpressing wildtype TRPV4 possess very few and small adhesions (Mrkonjić et al., 2015). TRPV4 inhibition in human mesenchymal stem cells also decreases tensile forces across vinculin and dramatically blocks collagen fibril assembly in vitro (Gilchrist et al., 2019). Moreover, TRPV4 activation in human breast cancer (4T07) cells enhances Akt and FAK phosphorylation and transendothelial migration (Lee et al., 2017a). Piezo1 has also been shown to enhance the growth of human glioblastoma stem cells and promote tumor development by activating integrin/FAK signaling (Chen et al., 2018). Piezo1 knockdown cells fail to assemble focal adhesion structures which halts a positive feedback loop that otherwise allows glioma cells to remodel the local ECM, increase tissue stiffness, and further enhance Piezo1 activity (Chen et al., 2018). As such, doxycycline-mediated shRNA knockdown of Piezo1 suppresses tumor growth and prolongs the survival of mice (Chen et al., 2018). Interestingly, TRPV4 expression in normal mouse primary epidermal keratinocytes has been shown to regulate transforming growth factor β (TGFβ)-induced EMT and nuclear translocation of Transcriptional coactivator with PDZ-binding motif (TAZ) in response to matrix stiffness (Sharma et al., 2019); however, these findings remain to be investigated in cancerous cells.
While stiffness is an important prognostic factor that enhances metastatic phenotypes, it is important to note that most biological tissues and ECMs are not purely elastic (Chaudhuri et al., 2020). Tissues exhibit a time-dependent mechanical response and dissipate a fraction of energy it took to deform them, a property known as viscoelasticity (Table 1). Soft tissues, such as brain, liver and lung, generally exhibit viscous moduli that are 10%–20% of their elastic moduli (Chaudhuri et al., 2020). Even hard tissues, such as bone and cartilage, display viscoelastic behaviors with viscous moduli that are about 10% of their elastic moduli (Chaudhuri et al., 2020). Thus, most biological tissues undergo permanent deformation following the application of external force. Importantly, magnetic resonance elastography (Sinkus et al., 2007) and ultrasonic strain imaging (Bayat et al., 2018) and elastography (Kumar et al., 2018) reveal that malignant lesions are more viscoelastic than benign lesions.
Malignant tumors have increased collagen density (Li et al., 2005) with collagen fibers that are structurally different from those of normal ECM stroma (Provenzano et al., 2006). Elevated collagen concentrations, the elongation of individual fibers, and the formation of new crosslinks substantially increase plasticity (Figure 1) (Ban et al., 2018). Viscoelastic 2D substrates enhance the migration of human fibrosarcoma (HT-1080), breast carcinoma (MDA-MB-231), and mammary epithelial cells by promoting the formation of filopodial protrusions and nascent adhesions; fascin1, formin and myosin-X facilitate filopodia formation, while integrin β1, Arp2/3, Rac1 and myosin participate in adhesion-based motility (Adebowale et al., 2021). Importantly, MDA-MB-231 cells in viscoelastic 3D matrices with a stiffness of ∼1.8 kPa can extend invadopodia and mechanically enlarge the gel pores to enhance migration through the ECM (Wisdom et al., 2018). Human chondrocytes are able to sense changes in substrate viscoelasticity via TRPV4, which in turn regulates the phosphorylation of glycogen synthase kinase 3β (GSK3β) (Agarwal et al., 2021). However, the molecular mechanisms through which cancer cells sense substrate viscoelasticity remain unknown and the effect of viscoelasticity on cancer cell metastasis requires further investigation. Nevertheless, these results implicate viscoelasticity as an important physical cue that affects cell migration and invasion.
Cells can align to nano- and micro-scale topographical features of the substrate and migrate along them using contact guidance (Table 1) (Martinez et al., 2009; Paul et al., 2016). Second harmonic generation (SHG) imaging of human breast cancer biopsies and mouse tumors shows that collagen fibers are increasingly aligned in invasive versus benign stages of cancer and in more aggressive tumors (Provenzano et al., 2006; Conklin et al., 2011). Intravital imaging also demonstrates that breast cancer cell invasion out of the primary tumor is predominantly oriented along aligned collagen fibers (Condeelis and Segall, 2003; Provenzano et al., 2006). Remarkably, primary tumor explants from mice cultured in a randomly organized collagen matrix can realign collagen fibers to promote outward migration of individual cancer cells along radially oriented fibers (Provenzano et al., 2006). Consequently, collagen fiber alignment relative to the breast tumor interface is an independent prognostic marker for disease progression (Li et al., 2005; Zunder et al., 2020) and survival (Paul et al., 2016).
Cancerous and non-cancerous cells can wrap around suspended fibers that are 0.1–1 µm in diameter and alter their protrusion dynamics depending upon the fiber curvature (Mukherjee et al., 2019). For example, human glioblastoma (U251) cells seeded on suspended nanofibers migrate faster and more persistently on 1D geometries than on 2D orthogonal fibers, and cells on closely spaced parallel fibers achieve even faster speeds than on single fibers (Estabridis et al., 2018). These three migratory behaviors are successfully recapitulated by a mathematical model that uses motor-clutch based force transmission (Chan and Odde, 2008), highlighting the role of topographical cues during glioblastoma cell migration (Estabridis et al., 2018). The stiffness and length of fibers also affects the migration speed and phenotype of human glioma (DBTRG-05MG) cells (Sharma et al., 2013). Motile cells can also align parallel to ridges on a 2D surface. Interestingly, using laminin-coated ridges with sub-micron features, the migratory phenotype of 14 patient-derived glioblastoma samples in response to platelet-derived growth factor (PDGF) could predict the tumor recurrence in the clinic (Smith et al., 2016).
Besides the direct sensing of native topographical cues by cancer cells, cancer associated fibroblasts (CAFs), which are abundantly present in the tumor microenvironment, alter the ECM architecture by producing collagen, fibronectin, and many other matrix components (Winkler et al., 2020). CAFs can also secrete LOX (Karagiannis et al., 2012; Liu et al., 2019a) and interact with collagen-rich ECM through focal adhesions to align fibers via RhoA-mediated activation of myosin light chain activity (Gaggioli et al., 2007; Goetz et al., 2011). Taken together, deregulated ECM architecture provides distinct topographical cues that facilitate tumor cell invasion into the surrounding tissue. Thus, contact guidance along ECM fibers and topographical features act as clinically relevant physical cue of cancer progression.
The ECM contains pores of varying sizes from 1 to 20 µm in diameter and narrow tube-like tracks that are 3–30 µm in width and up to 600 µm long, due to the organization and cross-linking of matrix components (Wolf et al., 2009). Cancer cells can physically widen or enzymatically degrade the surrounding ECM to create their own migration tracks (Bremer et al., 2001; Fisher et al., 2009; Wisdom et al., 2018), follow paths created by other cancer cells and CAFs (Gaggioli et al., 2007; Patsialou et al., 2013), or move through pre-existing channel-like tracks present in the native environment (Friedl and Alexander, 2011; Weigelin et al., 2012) (Figure 1).
Cells typically adapt to the local environment and migrate through the ECM by selecting the path of least resistance (Renkawitz et al., 2019; Yamada and Sixt, 2019; Zhao et al., 2019). To migrate through small channels and openings efficiently, cells rely on mechanosensitive mechanisms that detect changes in confinement and modulate their migration strategy accordingly (Hung et al., 2013). Using microfabricated PDMS devices and substrate printing methods, it was shown that confinement increases Ca2+ influx in Chinese Hamster Ovary (CHO) cells via the stretch-activated cation channel Piezo1 to reduce the activity of cyclic AMP (cAMP)-dependent protein kinase A (PKA) near the plasma membrane (Hung et al., 2016). Similarly, Ca2+ influx through Piezo2 was shown to facilitate efficient migration of brain metastatic MDA-MB-231 cells through narrow channels by modulating the activity of RhoA and the formation and orientation of stress fibers and focal adhesions (Pardo-Pastor et al., 2018). One possible mechanism linking Piezo2 and RhoA activation involves Fyn kinase recruitment and calpain activation at the leading edge of migrating cells (Pardo-Pastor et al., 2018). The coordinated activity of these proteins is known to regulate focal adhesion dynamics and stress fiber formation (Pardo-Pastor et al., 2018).
Remarkably, cells can squeeze through confining pores narrower than their resting dimensions by distorting the shape of their cell body and organelles; however, as the largest and stiffest cellular component, the nucleus determines the smallest pore size cells can pass through without degrading the surrounding matrix (Friedl et al., 2011; Wolf et al., 2013). Indeed, MMP-independent migration linearly decreases with pore size until the nucleus deforms down to ∼10% of its original cross section, at which point cells are no longer able to traverse the physical constraint (7 μm2 for tumor cells, 4 μm2 for T cells and 2 μm2 for neutrophils) (Wolf et al., 2013). Cells with reduced lamin-A expression possess more malleable nuclei and migrate through pores more quickly; however, the chance of apoptosis is increased due to nuclear envelope (NE) rupture which promotes DNA damage (Harada et al., 2014; Denais et al., 2016; Raab et al., 2016; Mistriotis et al., 2019). DNA damage repair pathways are rapidly recruited following NE rupture to mitigate apoptosis; inhibiting their activation substantially increases cell death after nuclear rupture (Denais et al., 2016; Raab et al., 2016). Even in the absence of NE rupture, mechanical deformation of the nucleus is sufficient to cause DNA damage (Shah et al., 2021). Thus, there is a delicate balance between enhanced cell migration and DNA damage in confinement.
The nucleus can help cells probe paths of different cross-sectional area and direct cell entry into channels with the least resistance by serving as a mechanical gauge (Renkawitz et al., 2019). Cells can measure the degree of spatial confinement via Ca2+ release from internal stores. Vertical compression of the nucleus in human cervical cancer (HeLa-Kyoto) cells triggers unfolding of the inner nuclear membrane and the activation of cytosolic phospholipase A2 (cPLA2), which increases lipid arachidonic acid (ARA) production, Ca2+ release from internal stores, and myosin II activity (Lomakin et al., 2020; Venturini et al., 2020). Such mechanotransduction allowed cells to rapidly adapt (<1 min) their behavior to changing tissue environments, yet the responses were stable over time (>60 min) (Lomakin et al., 2020; Venturini et al., 2020). Given that MICs, such as Piezo1 (Gudipaty et al., 2017) can be found on the nuclear membrane, it will be interesting to investigate how the nucleus and Ca2+ permeable MICs potentially act as a comprehensive mechanosensory module.
As cancer cells migrate out of the primary tumor and begin to overcome the multitude of obstacles posed by the ECM, they are also subjected to a variety of physical forces imposed by the extracellular fluid (Figure 1). For example, cells uptake and discharge water during confined cell migration and/or displace a column of fluid ahead of them, which generates hydraulic resistance (Table 1) (Stroka et al., 2014a; Li et al., 2020a). Neutrophil-like cells are capable of sensing small changes in hydraulic pressure on the order of ∼1 Pa and display bias towards the path of lower hydraulic resistance when presented with multiple confining paths (Prentice-Mott et al., 2013). Similarly, cancer cells choose the path of least resistance during confined migration (Zhao et al., 2019). In particular, hydraulic pressure triggers Ca2+ influx through TRPM7 in HT1080 and MDA-MB-231 cells to generate a thick cortical actin meshwork with an elevated density of myosin-IIA, which directs cell entry into channels of less resistance (Zhao et al., 2019). CRISPR/Cas9 knockout of TRPM7 blinds cells to hydraulic resistance and causes them to choose migratory paths based on cross-sectional area (Zhao et al., 2019). TRPM7 not only influences the directional navigation of cancer cells but also provides cells with plasticity to counter elevated hydraulic resistances (Zhao et al., 2021). Elevated hydraulic resistance induces a shift in cell migration phenotype from amoeboid to mesenchymal (Zhao et al., 2021), which relies on the formation of actin-rich lamellipodia and integrin-based cell-matrix adhesions rather than membrane blebs (Friedl and Wolf, 2010; Pankova et al., 2010). This transition occurs via intricate modulation of actomyosin turnover machinery initiated by TRPM7-mediated calcium signaling (Zhao et al., 2021), and promotes faster cell migration through confinement (Wisniewski et al., 2020; Zhao et al., 2021). Elevated hydraulic resistance is not only encountered during cell migration through confined spaces with stiff channel walls; different ECM architectures can also influence the resistance faced by cells in 3D (Maity et al., 2019). In fact, collagen or ECM permeability can play a major role in dictating the hydraulic resistance experienced by a cell (Maity et al., 2019). Taken together, the hydraulic pressure and resistance of static fluids significantly influences the detachment of cancer cells from the primary tumor and the migratory path taken by cells as they traverse the ECM.
Tumor sites possess increased fluid resistance and likely contain interstitial fluids with elevated viscosities (Jain et al., 2014); however, the contribution of these forces towards cancer cell dissemination and metastasis has largely been overlooked. Most cell migration studies to date have been exclusively performed using media which has a viscosity close to that of water (0.77 cP at 37°C). In contrast, the viscosity of interstitial fluid can reach 3.5 cP (Wells and Merrill, 1961; Yao et al., 2013; Gonzalez-Molina et al., 2018), while whole blood has a viscosity of 4–6 cP, which can be further elevated in pathological conditions (Rosenson et al., 1996). Several clinical studies demonstrate that fluid viscosity is correlated with negative outcomes in cancer patients. Elevated hematocrit levels increase the viscosity of whole blood which enhances metastasis in melanoma patients (Dintenfass, 1977). Similarly, high whole blood viscosity is associated with advanced stages of head and neck carcinoma (Khan et al., 1995). Increased whole blood viscosity is also associated with extrahepatic metastasis and reduced survival of patients with hepatocellular carcinoma (Han et al., 2021). Moreover, elevated plasma viscosity has been reported as an independent prognostic marker for the overall survival of breast cancer patients (Han et al., 2021). Thus, the role of physiologically relevant extracellular fluid viscosity in cancer cell migration and metastasis should be urgently investigated. Of note, supraphysiological viscosities (40–64.7 cP) induce increased motility and cell spread area in hepatic carcinoma cells (Gonzalez-Molina et al., 2018). However, it is unknown how cells sense and respond to fluid viscosities typically encountered in vivo.
Blood and lymphatic vessels in the human body provide a conduit for migrating tumor cells to travel throughout the body and colonize distant organs and tissues (Figure 1). To successfully form metastatic colonies, cancer cells must first intravasate through the endothelial lining of the bloodstream. In vitro studies reveal that MDA-MB-231 cells can create disruptions at least 20 µm in width to cross tissue-engineering microvessels (Wong and Searson, 2017). Disruption of endothelial cell-cell junctions is believed to be a key mediator of intravasation (Chiang et al., 2016); however, cancer cells can also enter the vasculature through pre-existing openings. For example, human breast cancer (MDA-MB-435) cells in zebrafish can intravasate into the bloodstream where it is actively being remodeled, but not at intact vessels (Stoletov et al., 2007). Murine mammary carcinoma models frequently display intercellular holes in tumor-associated vasculature (Hashizume et al., 2000). Tumor vasculature is predominantly leaky due to proteolytic degradation, remodeling/angiogenesis, paracrine signaling (such as the secretion of tumor necrosis factor (TNF)α) and hypoxia which disrupt the normal function of endothelial cells (Chiang et al., 2016). For this reason, intravasation typically occurs in the vicinity of the tumor where angiogenesis-induced capillary sprouts grow (Chiang et al., 2016). As mentioned previously, TRPV4 downregulation in murine endothelial cells also destabilizes tumor vessel integrity due to abnormal stiffness sensing and reduced VE-cadherin expression at cell-cell contacts (Adapala et al., 2016; Cappelli et al., 2019).
Once cells enter the vasculature, they must withstand shear stress (Table 1) at the vessel wall (Figure 1). Due to the nature of fluid flow in conduits with a cylindrical cross section, shear stress is maximal at the blood vessel walls and ranges from 1 to 4 dyn/cm2 in venous circulation and 4–30 dyn/cm2 in arterial circulation (Turitto, 1982) with notable variations between different organs within the body. For example, turbulent blood flow (Table 1) in the heart can raise shear stress levels to as high as 1,000 dyn/cm2 (Moose et al., 2020). Shear stress is detrimental to the survival of cancer cells and leads to “metastatic inefficiency” (Weiss, 1990; Chiang et al., 2016). Using microfluidic devices that apply physiologically relevant levels of shear stress (0.5–5 dyn/cm2), it was recently shown that normal human dermal fibroblasts avoid exposure to regions of fluid flow, while HT-1080 cells readily enter such regions (Yankaskas et al., 2021). This finding is consistent with prior computational modeling showing that tumor cell intravasation predominantly occurs at sites of low shear stress (0.2–6 dyn/cm2) (Stapor et al., 2011). The behavior of normal fibroblasts is driven by elevated expression of TRPM7, which senses and activates RhoA-driven myosin-II contractility at the cell edge exposed to shear stress, and causes the reversal of migration direction via a calmodulin/IQGAP1/Cdc42 pathway (Yankaskas et al., 2021). As such, overexpression of TRPM7 in fibrosarcoma cells restores sensitivity to fluid shear stress, which markedly reduces their ability to intravasate in vivo (Yankaskas et al., 2021). Taken together, the integrity of the endothelial barrier and the level of shear stress generated by blood flow regulates the location and extent of intravasation.
Fluid flow in the circulatory system facilitates the transport of cancer cells throughout the body; however, cells must withstand shear stress for distant colonization (Table 1). Most circulating tumor cells (CTCs) that enter the circulation undergo apoptosis due to shear stress. Indeed, isolated CTCs shed from human colon adenocarcinoma (LS174T) cells and its highly metastatic subline (LS LiM 6) display a high degree of apoptosis compared to the native tumor population in mice (Swartz et al., 1999). Breast and prostate cancer patients also exhibit many apoptotic cells in their CTC population (Larson et al., 2004; Kallergi et al., 2013). Nevertheless, transformed cells are more resistant to fluid shear stress than their non-transformed counterparts (Barnes et al., 2012). Cancer cells can employ several strategies to cope with high variations in shear stress. For example, human prostate cancer (PC-3) cells become significantly stiffer upon exposure to shear stress compared to untransformed prostate epithelial (PrEC LH) cells, indicating a higher level of mechanical adaptability in cancer cells (Chivukula et al., 2015). PC-3, MDA-MB-231 and urinary bladder cancer (TCCSUP) cells can also activate RhoA signaling to protect themselves from shear-induced damage by restructuring the actin cytoskeleton using formin (Moose et al., 2020). Resistance to fluid shear stress is dependent on actomyosin contractility. Consequently, pre-treating PC-3 cells with myosin-II inhibitor delays metastasis formation in mice (Moose et al., 2020).
Blood flow velocity can also affect the ability of CTCs to arrest and extravasate out of the vasculature (Table 1) by controlling the effectiveness and availability of ligand-mediated adhesion to the endothelial lumen. Blood flow velocities below 400–600 μm/s allow CTCs to arrest and adhere to endothelial cells in zebrafish embryos and mouse brain capillaries (Follain et al., 2018). Early arrest is mediated by weak adhesions composed of CD44 and integrins ɑvβ3 which are subsequently stabilized by integrins ɑ5β1 that bind fibronectin (Osmani et al., 2019). Of note, CD44 is a functional P-selectin ligand (Napier et al., 2007; Konstantopoulos and Thomas, 2009), which can also bind to E-selectin (and L-selectin) (Hanley et al., 2005; Hanley et al., 2006; Thomas et al., 2008), thereby mediating tumor cell tethering to endothelial cells under flow. Interestingly, a certain level of blood flow is required to promote the extravasation of CTCs, as reducing blood flow with lidocaine significantly decreases the percentage of cells that exit the vasculature in zebrafish embryos (Follain et al., 2018). This phenomenon is primarily driven by endothelial cells, which increase fibronectin deposition on their luminal surface in response to shear stress (Osmani et al., 2019). Similarly, in vitro studies using transendothelial assays show that hemodynamic shear stress increases the extravasation of MDA-MB-231 cells by inducing intracellular reactive oxygen species (ROS) production and ERK1/2 pathway activation (Ma et al., 2017). Accordingly, antioxidant suppression of ROS reduces tumor cell extravasation in vitro and in zebrafish (Ma et al., 2017). In a separate study, metastatic PC-3 cells were seeded on the luminal surface of PDMS tubes with a stiffness of 17 MPa and subjected to wall shear stress of 0.05 dyn/cm2 (Lee et al., 2017b) to mimic the stiffness of vascular walls and shear stress within interstitial or initial lymphatics (Swartz and Fleury, 2007; Resto et al., 2008). Fluid shear stress in this setting promoted an invasive phenotype characterized by filopodia (Lee et al., 2017b). Cells exposed to shear stress also migrated faster due to upregulated ROCK/LIMK/cofilin signaling, which induced Yes-associated protein (YAP)-mediated mechanotransduction and gene signature changes (Lee et al., 2017b). A recent study using a high-resolution humanoid blood flow model found that blood flow alone can predict to a high degree the distribution of metastatic site for a given primary tumor (Font-Clos et al., 2020). Thus, cells which can successfully withstand shear stresses found within the vasculature constitute a critical nucleator of secondary metastatic colonies.
MICs play a key role in sensing various mechanical stimuli in the primary tumor, local microenvironment and the vasculature due to direct mechanosensing in many cancer cell types (Table 3). In addition to the mechanotransduction pathways described above, MICs have been reported to regulate numerous other intracellular biochemical pathways that influence cancer progression. While it is currently unknown how these pathways are linked to mechanical forces, it is evident that MICs play a diverse role in promoting metastatic phenotypes. In the following section, we provide an overview of how Piezo1/2, TRPC1, TRPC5, TRPM2, TRPM4, TRPM7, TRPV2 and TRPV4 affect cancer cell behavior. Channel characteristics and mechanisms of activation are summarized in Table 2.
Piezo1 overexpression is associated with poor outcomes in breast and colon cancer patients due to enhanced cell migration and invasion, respectively (Tables 2, 3) (Li et al., 2015; Sun et al., 2020). In particular, Piezo1 promotes the proliferation and migration of human colorectal cancer (SW-480 and HCT-116) cells via mitochondrial calcium uniporter (MCU), hypoxia-inducible factor (HIF)-1ɑ and vascular endothelial growth factor (VEGF) (Sun et al., 2020). Gastric cancer patients with Piezo1 upregulation also exhibit increased distant metastasis due to enhanced cell proliferation and motility (Yang et al., 2014; Zhang et al., 2018; Wang et al., 2021). Piezo1 enhances the migration of human gastric cancer cells by modulating the activity of Rho GTPase family members (Zhang et al., 2018), altering the expression of integrin subunits via Trefoil factor family 1 (TFF1) (Yang et al., 2014), and inducing the expression EMT-associated genes via HIF-1ɑ (Wang et al., 2021). Conversely, Piezo1 knockdown decreases the migration, proliferation and metastasis of human gastric cancer cells by reducing mitochondrial membrane potential and the expression of p53, p21, several cyclin dependent kinases, and VEGF (Wang et al., 2021).
Immunohistochemistry analysis of malignant and benign prostate cancer tissues shows that Piezo1 is elevated with disease progression (Han et al., 2019). Overexpression of Piezo1 promotes tumorigenesis by enhancing Akt/mTOR pathway activation. In particular, Piezo1 promotes cell cycle progression via cyclin D1 and cyclin dependent kinase 4 (Han et al., 2019). Accordingly, Piezo1 downregulation in human prostate cancer (DU145) cells reduces proliferation and migration in vitro and tumor growth in vivo (Han et al., 2019).
RNA sequencing and microarray analyses reveal that Piezo1 expression is correlated with higher grades of glioma and worse clinical outcome (Zhou et al., 2020). Gene ontology analysis shows that high levels of Piezo1 are correlated with tumor microenvironment-related genes that encode proteins involved in ECM organization, angiogenesis and cell migration, including MMPs, mitogen-activated protein kinase (MAPK) family members and PI3K family members (Zhou et al., 2020). Piezo2 is also a crucial regulator of glioma cell growth, migration and invasion. Piezo2 promotes a favorable tumor microenvironment with increased vascular density and leakage by inducing Ca2+-dependent Wnt/β-catenin signaling in endothelial cells (Yang et al., 2016). Interestingly, Piezo2 knockdown in murine glioma (GL261) cells is sufficient to abrogate these environmental changes, suggesting a potential cross-talk between glioma and endothelial cells that supports tumor growth and reduces apoptosis (Yang et al., 2016).
Piezo1 also plays a key role in the pathogenesis of OSCC. Immunohistochemistry analysis of surgical specimens shows that Piezo1 expression in OSCC tissues is elevated compared to normal tissues (Hasegawa et al., 2021). Increased YAP activity in human OSCC enhances Piezo1 mRNA transcript levels (Hasegawa et al., 2021). Piezo1 overexpression subsequently activates ERK1/2 and p38 MAPK pathways through increased Ca2+ influx, leading to the enhanced migration and proliferation of OSCC cells (Hasegawa et al., 2021).
Interestingly, both Piezo1 and Piezo2 are downregulated in non-small cell lung cancer (NSCLC) tumor tissue compared to matched adjacent normal tissue (Huang et al., 2019). Accordingly, higher mRNA expression of Piezo channels is correlated with better overall survival, especially for patients with lung adenocarcinoma (Huang et al., 2019). Loss of Piezo1 reduces focal adhesion formation and calpain activity, switching normal human bronchial epithelial (16HBE) cells to an ameboid mode of migration (McHugh et al., 2012). Actin cytoskeletal re-arrangement and increased expression of tensin 4 in Piezo1 knockdown cells also results in faster 2D migration speeds and enhanced 3D invasion (McHugh et al., 2012). Additionally, Piezo1 promotes anchorage independent growth leading to formation of more colonies on soft agar (McHugh et al., 2012).
TRPC1 is a crucial MIC involved in the regulation of cancer cell migration and metastasis (Canales Coutiño and Mayor, 2021). TRPC1 mediates mechanosensation in response to plasma membrane tension changes, such as pressure and fluid flow (Table 3); however, it is activated through intracellular signaling pathways (Table 2). TRPC1 plays a key role in cell polarity and directed cell migration via Ca2+ signaling events in the lamellipodium (Fabian et al., 2008). TRPC1 silencing in transformed renal epithelial cells causes a partial loss in cell polarity which impairs the speed and directionality of migrating cells (Fabian et al., 2008). Reduced expression of TRPC1 in CNE2 nasopharyngeal tumor cells also inhibits cell migration and invasion through transwell inserts with 8 μm pores (He et al., 2012). Additionally, TRPC1 knockdown in breast cancer cells reduces ERK1/2 phosphorylation and cell cycle progression (El Hiani et al., 2009; Faouzi et al., 2016). TRPC1 contributes to hypoxia-induced EMT by regulating EGFR phosphorylation, Akt activation, and the expression of HIF-1ɑ, Snai1 and Twist1 (Azimi et al., 2017). Consequently, TRPC1 overexpression is associated with more aggressive breast cancer subtypes and poorer patient outcomes (Azimi et al., 2017). TRPC1 overexpression is also correlated with colorectal cancer progression and poor prognosis (Sun et al., 2021). TRPC1 increases store-operated Ca2+ entry in several human colon carcinoma cells through Orai1 and STIM1, leading to enhanced cell proliferation and invasion (Sobradillo et al., 2014). TRPC1 also increases: 1) PI3K/Akt signaling through direct interaction with calmodulin, 2) the expression of CyclinB1 and CDK1 which promote cell-cycle progression through G2, and 3) the expression of mesenchymal markers, such as N-cadherin, Snai1 and Slug (Sun et al., 2021). As a result, colorectal cancer cells expressing TRPC1 proliferate, migrate, invade and metastasize significantly more than cells with TRPC1 knockdown (Sun et al., 2021). Lastly, TRPC1 is overexpressed in human pancreatic cancer cells, where it mediates TGFβ-induced cell migration by increasing intracellular Ca2+ release from the endoplasmic reticulum (Dong et al., 2010), and NSCLC cells, where it contributes to disease progression by upregulating HIF-1ɑ through store-operated Ca2+ entry (Jiang et al., 2013; Wang et al., 2018a).
TRPC5 is another TRP family member that enhances cancer progression (Table 2). Previous studies demonstrate that TRPC5 channels respond to hypoosmotic stimulation and pressure-induced membrane stretch (Table 3), and this mechanosensitivity depends on actin filaments (Gomis et al., 2008; Shen et al., 2015). TRPC5 promotes the initiation, progression and metastasis of colon cancer by inducing EMT via HIF1ɑ and Twist1 (Chen et al., 2017a). Colon cancer cells with TRPC5 overexpression exhibit increased intracellular Ca2+, which promotes increased migration, invasion and proliferation (Chen et al., 2017a). TRPC5 also regulates the chemoresistance of colon cancer cells by enhancing Wnt/β-catenin signaling, which increases the expression of P-glycoprotein (Wang et al., 2015), an ABC drug efflux transporter, and glucose transporter 1 (GLUT1) (Wang et al., 2017). Patients with poorly differentiated tumors possess CTCs with high levels of TRPC5 expression (Cai et al., 2021). Consequently, TRPC5 overexpression is associated with poorer patient outcomes (Chen et al., 2017a; Cai et al., 2021). TRPC5 also plays an important role in breast cancer chemoresistance. Breast cancer cells resistant to doxorubicin express high levels of TRPC5, which enhances the expression of P-glycoprotein via the Ca2+-dependent transcription factor NFATc3 (Ma et al., 2012). Interestingly, breast cancer patients contain circulating exosomes with TRPC5 in their peripheral blood (Ma et al., 2014). Ca2+ influx via TRPC5 enhances the release of these exosomes, which can enter other breast cancer cells and confer resistance (Ma et al., 2014). Additionally, TRPC5 enhances chemoresistance by increasing autophagy via calmodulin-dependent protein kinase kinase β (CAMKKβ), AMP-activated kinase ɑ (AMPKɑ) and mTOR (Zhang et al., 2017). Accordingly, breast cancer patients exhibit markedly increased levels of TRPC5 and the autophagy marker LC3 after chemotherapy (Zhang et al., 2017). Remarkably, lentiviral injection of an shRNA against TRPC5 at tumor sites reverses chemoresistance to doxorubicin and paclitaxel resulting in tumor regression (Ma et al., 2012). Thus, TRPC5 may be an important clinical target for the treatment of breast cancer. Epidermal growth factor signaling via PI3K, Rac1 and phosphatidylinositol 4-phosphase 5-kinase (PIP(5)Kɑ) enhances TRPC5 trafficking to the plasma membrane in human embryonic kidney (HEK-293) cells (Bezzerides et al., 2004). This pathway may participate in the translocation and release of TRPC5 exosomes in breast cancer, however further investigation is required.
TRPM2 also enhances cancer cell migration and metastasis (Table 2). TRPM2 can be activated by temperature (Tan and McNaughton, 2016), oxidative stress (Perraud et al., 2005), and other stimuli that increase NAD+-related metabolites (Table 3) (Huang et al., 2018). H2O2-mediated activation of TRPM2 in cervical cancer (HeLa) and prostate cancer (PC-3) cells induces actin cytoskeleton remodeling, focal adhesion disassembly, and enhanced cell migration (Li et al., 2016). Interestingly, this phenotype is mediated by intracellular release of Zn2+ rather than Ca2+ (Li et al., 2016). Overexpression of TRPM2 is also associated with poor outcomes in pancreatic cancer patients due to PKC/MEK pathway activation, which results in increased cell proliferation, migration and invasion (Lin et al., 2021). Furthermore, TRPM2 enhances the migration, invasion and tumorigenesis of gastric cancer cells by decreasing PTEN activity and increasing Akt signaling (Almasi et al., 2019). These changes downregulate E-cadherin expression and upregulate EMT-related genes Twist and Zeb1 (Almasi et al., 2019). Additionally, TRPM2 reduces apoptosis and enhances mitochondrial metabolism, which increases cell proliferation, autophagy and mitophagy through a c-Jun N-terminal kinase (JNK)-dependent signaling pathway (Almasi et al., 2018). Due to its effects on autophagy, TRPM2 downregulation sensitizes gastric cancer cells to paclitaxel and doxorubicin treatment (Almasi et al., 2018).
TRPM4 is another TRPM channel subfamily member associated with cell migration and cancer metastasis. Although TRPM4 itself is not a stretch-activated Ca2+ channel (Table 2), it may work in tandem with other MICs to facilitate mechanosensing (Table 3). For example, TRPM4 acts together with Piezo1 to sense pressure overload in left ventricular hypertrophy (Yu et al., 2022). In mouse embryonic fibroblasts, TRPM4 localizes to adhesions and regulates cell spreading, migration and invasion by altering focal adhesion and actin cytoskeleton dynamics (Cáceres et al., 2015). Interestingly, TRPM4 mediates FAK and Rac GTPase activation following serum-induced increases in intracellular Ca2+ (Cáceres et al., 2015). One possible explanation is that TRPM4 facilitates localized Na+ influx, which causes membrane depolarization and leads to the activation of voltage-dependent Ca2+ channels (Cáceres et al., 2015). TRPM4 also regulates the migration and invasion of prostate cancer (PC-3) cells by altering the expression of genes involved in EMT, including the repression of E-cadherin and upregulation of Snai1, MMP-9, N-cadherin and vimentin (Sagredo et al., 2019). Accordingly, TRPM4 overexpression is correlated with higher grade prostate cancer (Sagredo et al., 2019). Similarly, knockout of TRPM4, which is overexpressed in human colorectal tumors and correlates with late-stage metastatic cancer, hinders cell migration, invasion, proliferation and viability (Kappel et al., 2019). In contrast, increased expression of TRPM4 in endometrial carcinoma cells is associated with a more favorable prognosis (Li et al., 2020b). TRPM4 downregulation due to estrogen decreases p53 levels, promotes PI3K/Akt/mTOR pathway activation, and increases EMT progression (Li et al., 2020b). Consequently, TRPM4 knockdown increases the migratory ability of endometrial carcinoma (AN3 CA) cells.
TRPM7 is a divalent cation channel with inherent serine/threonine kinase activity (Table 2) that is ubiquitously expressed in the human body and involved in many (patho) physiological processes. Previous studies indicate that TRPM7 is activated by hydraulic pressure (Zhao et al., 2019; Zhao et al., 2021), shear stress (Yankaskas et al., 2021) and cell swelling (Numata et al., 2007) (Table 3). TRPM7 can mediate breast cancer cell migration and invasion through Src and MAPK signaling pathways, without the involvement of Akt (Meng et al., 2013). In addition, TRPM7 regulates cell-cell adhesions, cell-matrix adhesions and cell migration speeds by regulating myosin II-based contractility (Middelbeek et al., 2012). For these reasons, TRPM7 expression is functionally required to form metastases in mouse xenograft models and predicts poor outcome in breast cancer patients (Middelbeek et al., 2012). In ovarian cancer, TRPM7 promotes the phosphorylation of Akt, Src and p38 (Wang et al., 2014) and induces EMT via Twist1 expression (Liu et al., 2019b). Conversely, TRPM7 depletion inhibits cell proliferation, colony formation, migration, invasion and metastasis (Wang et al., 2014; Liu et al., 2019b). As a result, TRPM7 overexpression is associated with poor prognosis in ovarian cancer patients as well (Wang et al., 2014). In prostate cancer, cholesterol stimulates Ca2+ influx through TRPM7, which enhances cell proliferation and migration via ERK and Akt phosphorylation, reduces E-cadherin expression, and increases calpain activity (Sun et al., 2014a). Accordingly, TRPM7 knockdown reverses EMT, leading to the downregulation of MMPs and upregulation of E-cadherin (Chen et al., 2017b). Androgen-independent prostate cancer cells also undergo EMT in response to hypoxia via a TRPM7/HIF-1ɑ/Annexin A1 signaling pathway (Yang et al., 2020a). In contrast, TRPM7 knockdown suppresses cell migration, invasion and EMT by enhancing RACK1-mediated degradation of HIF-1α (Yang et al., 2020a). Consequently, TRPM7 overexpression is associated with poor survival in prostate cancer patients (Yang et al., 2020a). TRPM7 expression is also associated with advanced colorectal cancer due to EMT (Su et al., 2019). TRPM7 downregulation reverses EMT, which promotes apoptosis and reduces cell proliferation, migration, and invasion (Su et al., 2019). On the other hand, TRPM7 enhances the invasion of pancreatic adenocarcinoma cells through an HSP90ɑ/uPA/MMP-2 proteolytic axis (Rybarczyk et al., 2017). This axis may enhance cell migration in response to elastin-derived peptides that are released following ECM degradation (Lefebvre et al., 2020). As a result, TRPM7 is inversely correlated with the survival of pancreatic cancer patients (Rybarczyk et al., 2017). TRPM7 is also overexpressed in bladder cancer and promotes cell migration, invasion and proliferation which results in poor patient outcomes (Gao et al., 2017). Finally, sustained Ca2+ influx through TRPM7 enhances the migratory ability of human nasopharyngeal carcinoma cells by stimulating ryanodine receptors to release additional Ca2+ from intracellular stores (Chen et al., 2010).
TRPV2 was initially characterized as a heat-gated ion channel (Caterina et al., 1999; Liu and Qin, 2016); however, it can also respond to membrane stretch in neurons (Table 2) (Mihara et al., 2010; Shibasaki et al., 2010). In addition, TRPV2 function and expression are linked to cell migration and cancer metastasis (Table 3). TRPV2 enhances the migration, invasion proliferation and survival of esophageal squamous cell carcinoma cells (Kudou et al., 2019). Accordingly, TRPV2 expression is associated with poor patient prognosis (Kudou et al., 2019). In addition, TRPV2 increases gastric cancer cell migration and invasion through the TGFβ signaling pathway (Kato et al., 2022). In contrast, TRPV2 knockdown reduces the expression of MMP-2, MMP-9 and integrin alpha V (Kato et al., 2022). As a result, TRPV2 expression is associated with lymphatic invasion, venous invasion and poor prognosis in gastric cancer patients (Kato et al., 2022). Interestingly, several studies indicate that TRPV2 facilitates the uptake of chemotherapy drugs. Moreover, cannabidiol can be used to trigger TRPV2 to enhance the uptake of chemotherapy drugs via increased Ca2+ influx. In particular, cannabidiol has been shown to sensitize breast cancer (Elbaz et al., 2018), endometrial cancer (Marinelli et al., 2020) and glioblastoma (Nabissi et al., 2013) cells to chemotherapeutic agents.
TRPV4 is another TRPV channel subfamily member with well-characterized roles in cell migration and cancer metastasis. Like many other TRP channels, TRPV4 is a polymodal protein (Table 2) that can be activated by cell swelling, shear stress, moderate temperatures (∼27°C), hypoosmotic conditions and chemical agonists (Clapham, 2003; Liedtke et al., 2003; Mendoza et al., 2010) (Table 3). TRPV4 regulates the migration of several cell types by modulating focal adhesion dynamics (Mrkonjić et al., 2015). Ca2+ influx through TRPV4 at focal adhesion sites activates calpain, which promotes focal adhesion disassembly and efficient retraction of the trailing edge (Mrkonjić et al., 2015). In contrast, overexpressing TRPV4 mutants that lack the phosphoinositide-binding site (121AAWAA) or a functional pore (TRPV4-M680D) reduces calpain activity, increases focal adhesion size, and promotes the formation of a tail, which anchors the cell to the substrate (Mrkonjić et al., 2015). TRPV4 overexpression is correlated with significantly poorer overall survival and disease-free survival in breast cancer patients (Lee et al., 2017a). Ca2+ influx through TRPV4 enhances Akt and FAK phosphorylation, reduces E-cadherin and β-catenin expression, and alters the expression of many other proteins involved cytoskeleton and ECM remodeling (Lee et al., 2017a). Indeed, TRPV4 overexpression promotes actin reorganization, cell blebbing and cell softness (Lee et al., 2016). These changes increase transendothelial migration and lung metastasis of breast cancer cells (Lee et al., 2016; Lee et al., 2017a). TRPV4 also accelerates glioma cell migration and invasion through Akt phosphorylation and Rac1 activation (Ou-Yang et al., 2018). Moreover, TRPV4 can promote the formation of filopodia through Cdc42 and N-wasp (Yang et al., 2020b). TRPV4 inhibition reduces tumor growth, decreases invasion into the surrounding brain tissue, and significantly prolongs the survival time of mice (Yang et al., 2020b). Accordingly, TRPV4 overexpression is associated with poor prognosis in glioma patients (Ou-Yang et al., 2018; Yang et al., 2020b). In line with these studies, TRPV4 overexpression increases the migration and invasion of colon cancer cells by activating Akt (Zhang et al., 2021). Silencing or inhibiting TRPV4 suppresses invasiveness by abrogating ZEB1-mediated EMT (Zhang et al., 2021). Finally, TRPV4-mediated Ca2+ influx in endometrial cancer cells enhances cell migration via a RhoA/ROCK1/LIMK/cofilin pathway that remodels the actin cytoskeleton and reinforces focal adhesions (Li et al., 2020c).
In the last two decades, physical cues have been identified as crucial regulators of cancer progression (Wirtz et al., 2011; Nia et al., 2020). More recently, the role of extracellular fluids in regulating cell behavior has also emerged (Follain et al., 2020). Cells encounter both solid and fluid interfaces in vivo (Figure 1) and thus it is crucial to understand how cells interpret physical cues to better control metastatic spread. MICs play an important role in sensing and transducing physical stimuli, such as compression, membrane tension, heat, pressure, hydraulic resistance, shear stress, substrate stiffness and viscoelasticity (Table 1), which allows cells to adapt to the local microenvironment. While the role of MICs in normal cells is well known (Ranade et al., 2015), their expression and function in cancer cells has recently received attention (Adapala et al., 2016; Dombroski et al., 2021; Karki and Tojkander, 2021; Yankaskas et al., 2021). Of particular interest is how MICs allow cancer cells to sense, integrate and interpret physical cues encountered during metastasis. Here, we described physical cues encountered at the primary tumor site followed by those encountered during invasion and inside the vasculature. We provided an overview of Piezo1/2, TRPC1, TRPC5, TRPM2, TRPM4, TRPM7, TRPV2 and TRPV4 (Table 2) and showed that these channels participate in numerous biochemical pathways that enhance metastatic phenotypes, such as cell migration, invasion and metastasis (Table 3).
The classical view of mechanosensing implicates focal adhesions (Box 1) as the primary sensors involved in perceiving and transmitting physical cues from the substrate to the cellular cytoskeleton (Albiges-Rizo et al., 2009; Geiger et al., 2009; Plotnikov et al., 2012; Horton et al., 2016; Stutchbury et al., 2017). However, accumulating evidence demonstrates that the dynamics of these critical structures are strongly influenced by the activity of MICs. For example, Piezo1 can localize to focal adhesions and regulate their assembly via FAK (Chen et al., 2018). Piezo1 can also activate Rho GTPase family members, alter the expression of integrin subunits, and induce EMT (Zhang et al., 2018; Wang et al., 2021). Similarly, Piezo2 can regulate focal adhesion dynamics via Fyn kinase and calpain activity and promote stress fiber assembly through RhoA/mDia (Pardo-Pastor et al., 2018). TRP channels have also been shown to regulate focal adhesion dynamics by activating calpain, FAK and PI3K/Akt signaling (Sun et al., 2014a; Wang et al., 2014; Cáceres et al., 2015; Mrkonjić et al., 2015; Lee et al., 2017a; Azimi et al., 2017; Ou-Yang et al., 2018; Almasi et al., 2019; Li et al., 2020b; Sun et al., 2021; Zhang et al., 2021). For instance, TRPV4 knockdown reduces calpain activity, which prevents focal adhesion disassembly and leads to the formation of large adhesions that anchor the cell (Mrkonjić et al., 2015). Moreover, TRP channels can regulate actin dynamics and stimulate cellular contractility by activating Rac, RhoA/myosin-II, RhoA/ROCK1/LIMK/cofilin or calmodulin/IQGAP/Cdc42 (Adapala et al., 2016; Li et al., 2020c; Yankaskas et al., 2021). Additionally, TRPC1, TRPC5, TRPM2, TRPM4, TRPM7, TRPV2 and TRPV4 can independently induce EMT in cancer cells (Chen et al., 2017a; Azimi et al., 2017; Chen et al., 2017b; Almasi et al., 2019; Liu et al., 2019b; Kudou et al., 2019; Sagredo et al., 2019; Su et al., 2019; Yang et al., 2020a; Li et al., 2020b; Zhang et al., 2021; Kato et al., 2022).
Cells on 2D substrates can freely spread out and migrate without any physical perturbations to their plasma membrane. On the other hand, cells migrating through 3D ECM frequently encounter obstacles that deform the plasma membrane, causing local changes in tension. Additionally, as we described in this article, tumor cells continually experience forces arising from bodily fluids at different locations in the metastatic cascade which cannot be directly detected by focal adhesion complexes. Thus MICs, together with focal adhesion components, can facilitate effective cell migration/invasion in response to changing mechanical cues. At the same time, traction forces generated by actomyosin contractility may also cause local changes in membrane tension surrounding adhesions (Ellefsen et al., 2019). These changes may induce MIC channel opening, which subsequently regulates adhesion strength and actomyosin contractility. Therefore, there is likely a dynamic crosstalk between adhesions and MICs that requires further investigation.
MICs may also regulate the assembly of specialized invasive structures known as invadopodia (Box 2), which contain a perpendicular alignment of actin filaments with respect to the underlying ECM. These structures allow cells to exert protrusive forces on the matrix as MMPs enzymatically degrade fibers (Albiges-Rizo et al., 2009). Focal adhesions and invadopodia share similar scaffolding and signaling components, albeit in a different arrangement (Revach et al., 2020). Given that Ca2+ influx through MICs regulates focal adhesion formation, it is plausible that MICs may regulate invadopodia formation. Indeed, Piezo1 and Piezo2 have recently been shown to enhance invadopodia formation in breast cancer cells (Pardo-Pastor et al., 2018; Luo et al., 2022). Alternatively, MIC crosstalk with intracellular store-operated Ca2+ (SOC) channels may control invadopodia formation. In melanoma cells, the SOC channel Orai1 localizes to invadopodia and spontaneously mediates discrete Ca2+ transients (Lu et al., 2019). Ca2+ entry through Orai1/STIM1 initiates calmodulin binding to the autoinhibitory domain of Pyk2, which allows Pyk2 to activate Src (Lu et al., 2019). Overexpression of a dominant-negative Orai1 mutant (Orai1-E106A) or STIM1 knockout largely abolishes invadopodial Ca2+ signals (Lu et al., 2019). Store-operated Ca2+ entry blockade also inhibits invadopodia formation and ECM degradation (Sun et al., 2014b). Accordingly, STIM1 knockdown significantly reduces the metastatic ability of melanoma cells (Sun et al., 2014b). In contrast, TRPM7 knockout fails to affect the frequency, amplitude or duration of Ca2+ signals in invadopodia (Lu et al., 2019). By responding to substrate stiffness, MICs, such as Piezo1/2 and TRPV4, may also regulate invadopodia formation in response to matrix rigidity. At low matrix stiffness (0.165 kPa), cells use membrane blebs to invade in a protease-independent manner (Aung et al., 2014). In contrast, higher matrix stiffness induces the formation of invadopodia to facilitate protease-dependent invasion (Aung et al., 2014). Several studies demonstrate that invadopodia form optimally in response to certain substrate stiffnesses. For example, breast cancer cells form more invadopodia as substrate stiffness increases from 1.1 to 28 kPa but exhibit less invadopodia on 3.1 and 5.6 MPa substrates (Aung et al., 2014; Dalaka et al., 2020). Similarly, head and neck squamous cell carcinoma cells degrade ECM more effectively on 22.7 kPa compared to 1.0 kPa (Jerrell and Parekh, 2014). Thus, it would be interesting to determine whether MIC function is required for invadopodia formation in response to these stiffnesses.
Box 2 | Invadopodia are important structures that facilitate cancer cell invasion. Invadopodia are dynamic structures with a typical lifetime of 5–15 min on 2D substrates (Eddy et al., 2017). Current models of formation suggest that cofilin, Arp2/3, N-WASP and cortactin are involved in precursor core initiation (Eddy et al., 2017). Tks5 and SHIP2 are subsequently recruited to stabilize the precursor core to the plasma membrane (Eddy et al., 2017). Finally, Nck1 and Cdc42 activate the N-WASP-Arp2/3 complex to nucleate actin polymerization; Cdc42 and RhoA also recruit membrane tethered matrix metalloproteinase (MT1-MMP) to the plasma membrane (Eddy et al., 2017). Invadopodia allow cancer cells to breach basement membranes, intravasate into and extravasate out of the bloodstream, and establish metastatic colonies (Leong et al., 2014; Harney et al., 2015; Eddy et al., 2017). Accordingly, knockdown of critical invadopodia components, such as Tks5, cortactin, and LPP, reduces cancer cell proliferation, invasion and metastasis (Blouw et al., 2015; Ngan et al., 2017).
Changes in membrane tension are thought to activate MICs and trigger Ca2+ current across the cell surface (Matthews et al., 2006). Indeed, each of the mechanical forces described above enhance intracellular Ca2+ levels, which stimulate intracellular signaling pathways involved in cell migration and invasion. In addition to direct mechanical activation, the activity of MICs may be influenced by ion channels and transporters that affect plasma membrane tension (Box 3). For example, breast cancer cells can migrate through tightly confined channels by preferentially polarizing ion transporters and water channels in a process known as the Osmotic Engine Model (OEM) (Stroka et al., 2014a; Stroka et al., 2014b; Li and Sun, 2018; Li et al., 2019b). Na+/H+ exchanger isoform-1 (NHE1) and aquaporin 5 (AQP5) polarization to the leading edge of cells facilitates water influx at the cell front, which promotes forward movement of the leading-edge membrane (Stroka et al., 2014a). In contrast, hypotonic shock at the cell front reverses the direction of cell migration by forcing cells to expel water at their “old” leading edge (Stroka et al., 2014a). Such expansion-contraction events can accentuate or attenuate membrane tension in migrating cells, which may trigger MIC activation and lead to a feedback loop between volume regulatory ion channels and MICs. TRP Ca2+ currents are also affected by changes in the transmembrane voltage (Zheng, 2013), which may be altered due to ion fluxes through other ion channels/transporters. Thus, it is expected that MICs should have a broad role in cell mechanosensation as well as sensing of cell electrical activity.
Box 3 | Ion channels that affect membrane tension may influence MIC activation. Cells possess feedback mechanisms that actively maintain membrane tension at a homeostatic level. In the unperturbed state, cell membrane tension arises from balancing osmotic and hydraulic pressure differences across the cell surface (Li et al., 2020a). From the Young-Laplace relation, the hydraulic pressure difference across the cell surface at mechanical equilibrium, ΔP, is balanced by tension in the cell surface, i.e.,
Taken together, MICs bestow cancer cells with the capacity to sense and respond to complex physical cues encountered during metastasis. As tumor cells migrate through the tissue microenvironments, they rely on mechanotransduction pathways engaged by MICs to choose the most efficient migratory route and adjust cellular functions accordingly. Interestingly, the expression level of MICs may be stage dependent, as demonstrated by TRPM7. On one hand, TRPM7 overexpression enhances cancer cell migration via myosin II-based contractility (Middelbeek et al., 2012; Meng et al., 2013), however, TRPM7 downregulation is required during intravasation to de-sensitize cells to shear stress (Yankaskas et al., 2021). Stage-dependent expression may allow cancer cells to dynamically refine their mechanical properties, which is required to successfully complete all steps of the metastatic cascade (Gensbittel et al., 2021). Thus, a detailed understanding and characterization of physical forces and associated MICs will provide opportunities to improve the treatment of metastatic cancer.
KB, AK and YZ have contributed equally to this work and share first authorship. KB, AK, YZ and KK conceptualized and wrote the manuscript. KK supervised the study. All authors read and edited the manuscript.
This work was supported, in part, by R01 CA254193 (KK and SXS), R01 CA257647 (KK), R01 GM134542 (SS and KK), R01 GM142175 (KK) and postdoctoral fellowships from the Fonds de recherche du Québec—Nature et technologies and the Natural Sciences and Engineering Research Council of Canada (AK).
The authors declare that the research was conducted in the absence of any commercial or financial relationships that could be construed as a potential conflict of interest.
All claims expressed in this article are solely those of the authors and do not necessarily represent those of their affiliated organizations, or those of the publisher, the editors and the reviewers. Any product that may be evaluated in this article, or claim that may be made by its manufacturer, is not guaranteed or endorsed by the publisher.
Adapala, R. K., Thoppil, R. J., Ghosh, K., Cappelli, H. C., Dudley, A. C., Paruchuri, S., et al. (2016). Activation of mechanosensitive ion channel TRPV4 normalizes tumor vasculature and improves cancer therapy. Oncogene 35, 314–322. doi:10.1038/onc.2015.83
Adebowale, K., Gong, Z., Hou, J. C., Wisdom, K. M., Garbett, D., Lee, H. P., et al. (2021). Enhanced substrate stress relaxation promotes filopodia-mediated cell migration. Nat. Mat. 20, 1290–1299. doi:10.1038/s41563-021-00981-w
Agarwal, P., Lee, H. P., Smeriglio, P., Grandi, F., Goodman, S., Chaudhuri, O., et al. (2021). A dysfunctional TRPV4-GSK3β pathway prevents osteoarthritic chondrocytes from sensing changes in extracellular matrix viscoelasticity. Nat. Biomed. Eng. 5, 1472–1484. doi:10.1038/s41551-021-00691-3
Aiello, N. M., and Kang, Y. (2019). Context-dependent EMT programs in cancer metastasis. J. Exp. Med. 216, 1016–1026. doi:10.1084/jem.20181827
Albiges-Rizo, C., Destaing, O., Fourcade, B., Planus, E., and Block, M. R. (2009). Actin machinery and mechanosensitivity in invadopodia, podosomes and focal adhesions. J. Cell Sci. 122, 3037–3049. doi:10.1242/jcs.052704
Almasi, S., Kennedy, B. E., El-Aghil, M., Sterea, A. M., Gujar, S., Partida-Sanchez, S., et al. (2018). TRPM2 channel-mediated regulation of autophagy maintains mitochondrial function and promotes gastric cancer cell survival via the JNK-signaling pathway. J. Biol. Chem. 293, 3637–3650. doi:10.1074/jbc.M117.817635
Almasi, S., Sterea, A. M., Fernando, W., Clements, D. R., Marcato, P., Hoskin, D. W., et al. (2019). TRPM2 ion channel promotes gastric cancer migration, invasion and tumor growth through the AKT signaling pathway. Sci. Rep. 9, 4182. doi:10.1038/s41598-019-40330-1
Athanasiou, A., Tardivon, A., Tanter, M., Sigal-Zafrani, B., Bercoff, J., Deffieux, T., et al. (2010). Breast lesions: Quantitative elastography with supersonic shear imaging-preliminary results. Radiology 256, 297–303. doi:10.1148/radiol.10090385
Aung, A., Seo, Y. N., Lu, S., Wang, Y., Jamora, C., del Alamo, J. C., et al. (2014). 3D traction stresses activate protease-dependent invasion of cancer cells. Biophys. J. 107, 2528–2537. doi:10.1016/j.bpj.2014.07.078
Austen, K., Ringer, P., Mehlich, A., Chrostek-Grashoff, A., Kluger, C., Klingner, C., et al. (2015). Extracellular rigidity sensing by talin isoform-specific mechanical linkages. Nat. Cell Biol. 17, 1597–1606. doi:10.1038/ncb3268
Azimi, I., Milevskiy, M. J. G., Kaemmerer, E., Turner, D., Yapa, K., Brown, M. A., et al. (2017). TRPC1 is a differential regulator of hypoxia-mediated events and Akt signalling in PTEN-deficient breast cancer cells. J. Cell Sci. 130, 2292–2305. doi:10.1242/jcs.196659
Bae, J. S., Chang, J. M., Lee, S. H., Shin, S. U., and Moon, W. K. (2017). Prediction of invasive breast cancer using shear-wave elastography in patients with biopsy-confirmed ductal carcinoma in situ. Eur. Radiol. 27, 7–15. doi:10.1007/s00330-016-4359-6
Ban, E., Franklin, J. M., Nam, S., Smith, L. R., Wang, H., Wells, R. G., et al. (2018). Mechanisms of plastic deformation in collagen networks induced by cellular forces. Biophys. J. 114, 450–461. doi:10.1016/j.bpj.2017.11.3739
Bangasser, B. L., Shamsan, G. A., Chan, C. E., Opoku, K. N., Tuzel, E., Schlichtmann, B. W., et al. (2017). Shifting the optimal stiffness for cell migration. Nat. Commun. 8, 15313. doi:10.1038/ncomms15313
Barnes, J. M., Nauseef, J. T., and Henry, M. D. (2012). Resistance to fluid shear stress is a conserved biophysical property of malignant cells. PLoS One 7, e50973. doi:10.1371/journal.pone.0050973
Baudel, M., Shi, J., Large, W. A., and Albert, A. P. (2020). Insights into activation mechanisms of store-operated TRPC1 channels in vascular smooth muscle. Cells 9, E179. doi:10.3390/cells9010179
Baxter, L. T., and Jain, R. K. (1989). Transport of fluid and macromolecules in tumors. I. Role of interstitial pressure and convection. Microvasc. Res. 37, 77–104. doi:10.1016/0026-2862(89)90074-5
Bayat, M., Nabavizadeh, A., Kumar, V., Gregory, A., Insana, M., Alizad, A., et al. (2018). Automated in vivo sub-hertz analysis of viscoelasticity (SAVE) for evaluation of breast lesions. IEEE Trans. Biomed. Eng. 65, 2237–2247. doi:10.1109/TBME.2017.2787679
Bezzerides, V. J., Ramsey, I. S., Kotecha, S., Greka, A., and Clapham, D. E. (2004). Rapid vesicular translocation and insertion of TRP channels. Nat. Cell Biol. 6, 709–720. doi:10.1038/ncb1150
Birk, J. W., Tadros, M., Moezardalan, K., Nadyarnykh, O., Forouhar, F., Anderson, J., et al. (2014). Second harmonic generation imaging distinguishes both high-grade dysplasia and cancer from normal colonic mucosa. Dig. Dis. Sci. 59, 1529–1534. doi:10.1007/s10620-014-3121-7
Blair, N. T., Kaczmarek, J. S., and Clapham, D. E. (2009). Intracellular calcium strongly potentiates agonist-activated TRPC5 channels. J. Gen. Physiol. 133, 525–546. doi:10.1085/jgp.200810153
Blouw, B., Patel, M., Iizuka, S., Abdullah, C., You, W. K., Huang, X., et al. (2015). The invadopodia scaffold protein Tks5 is required for the growth of human breast cancer cells in vitro and in vivo. PLoS One 10, e0121003. doi:10.1371/journal.pone.0121003
Bonnans, C., Chou, J., and Werb, Z. (2014). Remodelling the extracellular matrix in development and disease. Nat. Rev. Mol. Cell Biol. 15, 786–801. doi:10.1038/nrm3904
Bremer, C., Tung, C. H., and Weissleder, R. (2001). In vivo molecular target assessment of matrix metalloproteinase inhibition. Nat. Med. 7, 743–748. doi:10.1038/89126
Cáceres, M., Ortiz, L., Recabarren, T., Romero, A., Colombo, A., Leiva-Salcedo, E., et al. (2015). TRPM4 is a novel component of the adhesome required for focal adhesion disassembly, migration and contractility. PLoS One 10, e0130540. doi:10.1371/journal.pone.0130540
Cahalan, S. M., Lukacs, V., Ranade, S. S., Chien, S., Bandell, M., and Patapoutian, A. (2015). Piezo1 links mechanical forces to red blood cell volume. Elife 4, e07370. doi:10.7554/eLife.07370
Cai, D., Li, N., Jin, L., Qi, X., Hua, D., and Wang, T. (2021). High CTC-TRPC5 expression significantly associated with poor prognosis in radical resected colorectal cancer patients. Front. Mol. Biosci. 8, 727864. doi:10.3389/fmolb.2021.727864
Canales Coutiño, B., and Mayor, R. (2021). Mechanosensitive ion channels in cell migration. Cells Dev. 166, 203683. doi:10.1016/j.cdev.2021.203683
Canales, J., Morales, D., Blanco, C., Rivas, J., Díaz, N., Angelopoulos, I., et al. (2019). A TR(i)P to cell migration: New roles of TRP channels in mechanotransduction and cancer. Front. Physiol. 10, 757. doi:10.3389/fphys.2019.00757
Cappelli, H. C., Kanugula, A. K., Adapala, R. K., Amin, V., Sharma, P., Midha, P., et al. (2019). Mechanosensitive TRPV4 channels stabilize VE-cadherin junctions to regulate tumor vascular integrity and metastasis. Cancer Lett. 442, 15–20. doi:10.1016/j.canlet.2018.07.042
Carrillo-Garcia, J., Herrera-Fernández, V., Serra, S. A., Rubio-Moscardo, F., Vogel-Gonzalez, M., Doñate-Macian, P., et al. (2021). The mechanosensitive Piezo1 channel controls endosome trafficking for an efficient cytokinetic abscission. Sci. Adv. 7, eabi7785. doi:10.1126/sciadv.abi7785
Caterina, M. J., Rosen, T. A., Tominaga, M., Brake, A. J., and Julius, D. (1999). A capsaicin-receptor homologue with a high threshold for noxious heat. Nature 398, 436–441. doi:10.1038/18906
Chan, C. E., and Odde, D. J. (2008). Traction dynamics of filopodia on compliant substrates. Science 322, 1687–1691. doi:10.1126/science.1163595
Chan, K. T., Bennin, D. A., and Huttenlocher, A. (2010). Regulation of adhesion dynamics by calpain-mediated proteolysis of focal adhesion kinase (FAK). J. Biol. Chem. 285, 11418–11426. doi:10.1074/jbc.M109.090746
Chaudhuri, O., Cooper-White, J., Janmey, P. A., Mooney, D. J., and Shenoy, V. B. (2020). Effects of extracellular matrix viscoelasticity on cellular behaviour. Nature 584, 535–546. doi:10.1038/s41586-020-2612-2
Chen, J. P., Luan, Y., You, C. X., Chen, X. H., Luo, R. C., and Li, R. (2010). TRPM7 regulates the migration of human nasopharyngeal carcinoma cell by mediating Ca(2+) influx. Cell Calcium 47, 425–432. doi:10.1016/j.ceca.2010.03.003
Chen, L., Cao, R., Wang, G., Yuan, L., Qian, G., Guo, Z., et al. (2017). Downregulation of TRPM7 suppressed migration and invasion by regulating epithelial-mesenchymal transition in prostate cancer cells. Med. Oncol. 34, 127. doi:10.1007/s12032-017-0987-1
Chen, X., Wanggou, S., Bodalia, A., Zhu, M., Dong, W., Fan, J. J., et al. (2018). A feedforward mechanism mediated by mechanosensitive ion channel PIEZO1 and tissue mechanics promotes glioma aggression. Neuron 100, 799–815. doi:10.1016/j.neuron.2018.09.046
Chen, Z., Zhu, Y., Dong, Y., Zhang, P., Han, X., Jin, J., et al. (2017). Overexpression of TrpC5 promotes tumor metastasis via the HIF-1α-Twist signaling pathway in colon cancer. Clin. Sci. 131, 2439–2450. doi:10.1042/CS20171069
Cheng, G., Tse, J., Jain, R. K., and Munn, L. L. (2009). Micro-environmental mechanical stress controls tumor spheroid size and morphology by suppressing proliferation and inducing apoptosis in cancer cells. PLoS one 4, e4632. doi:10.1371/journal.pone.0004632
Cheng, K. T., Liu, X., Ong, H. L., Swaim, W., and Ambudkar, I. S. (2011). Local Ca²+ entry via Orai1 regulates plasma membrane recruitment of TRPC1 and controls cytosolic Ca²+ signals required for specific cell functions. PLoS Biol. 9, e1001025. doi:10.1371/journal.pbio.1001025
Chesler, A. T., Szczot, M., Bharucha-Goebel, D., Ceko, M., Donkervoort, S., Laubacher, C., et al. (2016). The role of PIEZO2 in human mechanosensation. N. Engl. J. Med. 375, 1355–1364. doi:10.1056/NEJMoa1602812
Chiang, S. P., Cabrera, R. M., and Segall, J. E. (2016). Tumor cell intravasation. Am. J. Physiol. Cell Physiol. 311, C1–C14. doi:10.1152/ajpcell.00238.2015
Chivukula, V. K., Krog, B. L., Nauseef, J. T., Henry, M. D., and Vigmostad, S. C. (2015). Alterations in cancer cell mechanical properties after fluid shear stress exposure: A micropipette aspiration study. Cell Health cytoskelet. 7, 25–35. doi:10.2147/CHC.S71852
Choi, C. K., Vicente-Manzanares, M., Zareno, J., Whitmore, L. A., Mogilner, A., and Horwitz, A. R. (2008). Actin and alpha-actinin orchestrate the assembly and maturation of nascent adhesions in a myosin II motor-independent manner. Nat. Cell Biol. 10, 1039–1050. doi:10.1038/ncb1763
Cinar, E., Zhou, S., DeCourcey, J., Wang, Y., Waugh, R. E., and Wan, J. (2015). Piezo1 regulates mechanotransductive release of ATP from human RBCs. Proc. Natl. Acad. Sci. U. S. A. 112, 11783–11788. doi:10.1073/pnas.1507309112
Clapham, D. E. (2003). TRP channels as cellular sensors. Nature 426, 517–524. doi:10.1038/nature02196
Condeelis, J., and Segall, J. E. (2003). Intravital imaging of cell movement in tumours. Nat. Rev. Cancer 3, 921–930. doi:10.1038/nrc1231
Conklin, M. W., Eickhoff, J. C., Riching, K. M., Pehlke, C. A., Eliceiri, K. W., Provenzano, P. P., et al. (2011). Aligned collagen is a prognostic signature for survival in human breast carcinoma. Am. J. Pathol. 178, 1221–1232. doi:10.1016/j.ajpath.2010.11.076
Coste, B., Mathur, J., Schmidt, M., Earley, T. J., Ranade, S., Petrus, M. J., et al. (2010). Piezo1 and Piezo2 are essential components of distinct mechanically activated cation channels. Science 330, 55–60. doi:10.1126/science.1193270
Coste, B., Xiao, B. L., Santos, J. S., Syeda, R., Grandl, J., Spencer, K. S., et al. (2012). Piezo proteins are pore-forming subunits of mechanically activated channels. Nature 483, 176–181. doi:10.1038/nature10812
Cox, C. D., Bae, C., Ziegler, L., Hartley, S., Nikolova-Krstevski, V., Rohde, P. R., et al. (2016). Removal of the mechanoprotective influence of the cytoskeleton reveals PIEZO1 is gated by bilayer tension. Nat. Commun. 7, 10366. doi:10.1038/ncomms10366
Dalaka, E., Kronenberg, N. M., Liehm, P., Segall, J. E., Prystowsky, M. B., and Gather, M. C. (2020). Direct measurement of vertical forces shows correlation between mechanical activity and proteolytic ability of invadopodia. Sci. Adv. 6, eaax6912. doi:10.1126/sciadv.aax6912
De Felice, D., and Alaimo, A. (2020). Mechanosensitive Piezo channels in cancer: Focus on altered calcium signaling in cancer cells and in tumor progression. Cancers (Basel) 12, E1780. doi:10.3390/cancers12071780
del Rio, A., Perez-Jimenez, R., Liu, R., Roca-Cusachs, P., Fernandez, J. M., and Sheetz, M. P. (2009). Stretching single talin rod molecules activates vinculin binding. Science 323, 638–641. doi:10.1126/science.1162912
Demou, Z. N. (2010). Gene expression profiles in 3D tumor analogs indicate compressive strain differentially enhances metastatic potential. Ann. Biomed. Eng. 38, 3509–3520. doi:10.1007/s10439-010-0097-0
Denais, C. M., Gilbert, R. M., Isermann, P., McGregor, A. L., te Lindert, M., Weigelin, B., et al. (2016). Nuclear envelope rupture and repair during cancer cell migration. Science 352, 353–358. doi:10.1126/science.aad7297
Deng, Z., Paknejad, N., Maksaev, G., Sala-Rabanal, M., Nichols, C. G., Hite, R. K., et al. (2018). Cryo-EM and X-ray structures of TRPV4 reveal insight into ion permeation and gating mechanisms. Nat. Struct. Mol. Biol. 25, 252–260. doi:10.1038/s41594-018-0037-5
DePasquale, J. A., and Izzard, C. S. (1987). Evidence for an actin-containing cytoplasmic precursor of the focal contact and the timing of incorporation of vinculin at the focal contact. J. Cell Biol. 105, 2803–2809. doi:10.1083/jcb.105.6.2803
Dintenfass, L. (1977). Some aspects of haemorrheology of metastasis in malignant melanoma. Haematologia 11, 301–307.
Discher, D. E., Janmey, P., and Wang, Y. L. (2005). Tissue cells feel and respond to the stiffness of their substrate. Science 310, 1139–1143. doi:10.1126/science.1116995
Dokukina, I. V., and Gracheva, M. E. (2010). A model of fibroblast motility on substrates with different rigidities. Biophys. J. 98, 2794–2803. doi:10.1016/j.bpj.2010.03.026
Dombroski, J. A., Hope, J. M., Sarna, N. S., and King, M. R. (2021). Channeling the force: Piezo1 mechanotransduction in cancer metastasis. Cells 10, 2815. doi:10.3390/cells10112815
Dong, H., Shim, K. N., Li, J. M., Estrema, C., Ornelas, T. A., Nguyen, F., et al. (2010). Molecular mechanisms underlying Ca2+-mediated motility of human pancreatic duct cells. Am. J. Physiol. Cell Physiol. 299, C1493–C1503. doi:10.1152/ajpcell.00242.2010
Doyle, A. D., Carvajal, N., Jin, A., Matsumoto, K., and Yamada, K. M. (2015). Local 3D matrix microenvironment regulates cell migration through spatiotemporal dynamics of contractility-dependent adhesions. Nat. Commun. 6, 8720. doi:10.1038/ncomms9720
Du, G., Chen, W., Li, L., and Zhang, Q. (2021). The potential role of mechanosensitive ion channels in substrate stiffness-regulated Ca(2+) response in chondrocytes. Connect. Tissue Res. 1, 1–10.
Du, X., Saido, T. C., Tsubuki, S., Indig, F. E., Williams, M. J., and Ginsberg, M. H. (1995). Calpain cleavage of the cytoplasmic domain of the integrin beta 3 subunit. J. Biol. Chem. 270, 26146–26151. doi:10.1074/jbc.270.44.26146
Duan, J., Li, Z., Li, J., Hulse, R. E., Santa-Cruz, A., Valinsky, W. C., et al. (2018). Structure of the mammalian TRPM7, a magnesium channel required during embryonic development. Proc. Natl. Acad. Sci. U. S. A. 115, E8201–E8210. doi:10.1073/pnas.1810719115
Duan, J., Li, Z., Li, J., Santa-Cruz, A., Sanchez-Martinez, S., Zhang, J., et al. (2018). Structure of full-length human TRPM4. Proc. Natl. Acad. Sci. U. S. A. 115, 2377–2382. doi:10.1073/pnas.1722038115
DuChez, B. J., Doyle, A. D., Dimitriadis, E. K., and Yamada, K. M. (2019). Durotaxis by human cancer cells. Biophys. J. 116, 670–683. doi:10.1016/j.bpj.2019.01.009
Eddy, R. J., Weidmann, M. D., Sharma, V. P., and Condeelis, J. S. (2017). Tumor cell invadopodia: Invasive protrusions that orchestrate metastasis. Trends Cell Biol. 27, 595–607. doi:10.1016/j.tcb.2017.03.003
El Hiani, Y., Ahidouch, A., Lehen'kyi, V., Hague, F., Gouilleux, F., Mentaverri, R., et al. (2009). Extracellular signal-regulated kinases 1 and 2 and TRPC1 channels are required for calcium-sensing receptor-stimulated MCF-7 breast cancer cell proliferation. Cell. Physiol. biochem. 23, 335–346. doi:10.1159/000218179
Elbaz, M., Ahirwar, D., Xiaoli, Z., Zhou, X., Lustberg, M., Nasser, M. W., et al. (2018). TRPV2 is a novel biomarker and therapeutic target in triple negative breast cancer. Oncotarget 9, 33459–33470. doi:10.18632/oncotarget.9663
Ellefsen, K. L., Holt, J. R., Chang, A. C., Nourse, J. L., Arulmoli, J., Mekhdjian, A. H., et al. (2019). Myosin-II mediated traction forces evoke localized Piezo1-dependent Ca(2+) flickers. Commun. Biol. 2, 298. doi:10.1038/s42003-019-0514-3
Ellis, R. J. (2001). Macromolecular crowding: Obvious but underappreciated. Trends biochem. Sci. 26, 597–604. doi:10.1016/s0968-0004(01)01938-7
Elosegui-Artola, A., Oria, R., Chen, Y., Kosmalska, A., Pérez-González, C., Castro, N., et al. (2016). Mechanical regulation of a molecular clutch defines force transmission and transduction in response to matrix rigidity. Nat. Cell Biol. 18, 540–548. doi:10.1038/ncb3336
Emon, B., Bauer, J., Jain, Y., Jung, B., and Saif, T. (2018). Biophysics of tumor microenvironment and cancer metastasis - a mini review. Comput. Struct. Biotechnol. J. 16, 279–287. doi:10.1016/j.csbj.2018.07.003
Estabridis, H. M., Jana, A., Nain, A., and Odde, D. J. (2018). Cell migration in 1D and 2D nanofiber microenvironments. Ann. Biomed. Eng. 46, 392–403. doi:10.1007/s10439-017-1958-6
Etem, E., Ceylan, G. G., Özaydın, S., Ceylan, C., Özercan, I., and Kuloğlu, T. (2018). The increased expression of Piezo1 and Piezo2 ion channels in human and mouse bladder carcinoma. Adv. Clin. Exp. Med. 27, 1025–1031. doi:10.17219/acem/71080
Fabian, A., Fortmann, T., Dieterich, P., Riethmüller, C., Schön, P., Mally, S., et al. (2008). TRPC1 channels regulate directionality of migrating cells. Pflugers Arch. 457, 475–484. doi:10.1007/s00424-008-0515-4
Faouzi, M., Hague, F., Geerts, D., Ay, A. S., Potier-Cartereau, M., Ahidouch, A., et al. (2016). Functional cooperation between KCa3.1 and TRPC1 channels in human breast cancer: Role in cell proliferation and patient prognosis. Oncotarget 7, 36419–36435. doi:10.18632/oncotarget.9261
Fisher, K. E., Sacharidou, A., Stratman, A. N., Mayo, A. M., Fisher, S. B., Mahan, R. D., et al. (2009). MT1-MMP- and Cdc42-dependent signaling co-regulate cell invasion and tunnel formation in 3D collagen matrices. J. Cell Sci. 122, 4558–4569. doi:10.1242/jcs.050724
Fleig, A., and Chubanov, V. (2014). Handb. Exp. Pharmacol. 222, 521–546. doi:10.1007/978-3-642-54215-2_21
Follain, G., Herrmann, D., Harlepp, S., Hyenne, V., Osmani, N., Warren, S. C., et al. (2020). Fluids and their mechanics in tumour transit: Shaping metastasis. Nat. Rev. Cancer 20, 107–124. doi:10.1038/s41568-019-0221-x
Follain, G., Osmani, N., Azevedo, A. S., Allio, G., Mercier, L., Karreman, M. A., et al. (2018). Hemodynamic forces tune the arrest, adhesion, and extravasation of circulating tumor cells. Dev. Cell 45, 33–52. doi:10.1016/j.devcel.2018.02.015
Font-Clos, F., Zapperi, S., and La Porta, C. A. M. (2020). Blood flow contributions to cancer metastasis. iScience 23, 101073. doi:10.1016/j.isci.2020.101073
Franco, S. J., Rodgers, M. A., Perrin, B. J., Han, J., Bennin, D. A., Critchley, D. R., et al. (2004). Calpain-mediated proteolysis of talin regulates adhesion dynamics. Nat. Cell Biol. 6, 977–983. doi:10.1038/ncb1175
Friedl, P., and Alexander, S. (2011). Cancer invasion and the microenvironment: Plasticity and reciprocity. Cell 147, 992–1009. doi:10.1016/j.cell.2011.11.016
Friedl, P., Wolf, K., and Lammerding, J. (2011). Nuclear mechanics during cell migration. Curr. Opin. Cell Biol. 23, 55–64. doi:10.1016/j.ceb.2010.10.015
Friedl, P., and Wolf, K. (2010). Plasticity of cell migration: A multiscale tuning model. J. Cell Biol. 188, 11–19. doi:10.1083/jcb.200909003
Gaggioli, C., Hooper, S., Hidalgo-Carcedo, C., Grosse, R., Marshall, J. F., Harrington, K., et al. (2007). Fibroblast-led collective invasion of carcinoma cells with differing roles for RhoGTPases in leading and following cells. Nat. Cell Biol. 9, 1392–1400. doi:10.1038/ncb1658
Galbraith, C. G., Yamada, K. M., and Sheetz, M. P. (2002). The relationship between force and focal complex development. J. Cell Biol. 159, 695–705. doi:10.1083/jcb.200204153
Gao, S. L., Kong, C. Z., Zhang, Z., Li, Z. L., Bi, J. B., and Liu, X. K. (2017). TRPM7 is overexpressed in bladder cancer and promotes proliferation, migration, invasion and tumor growth. Oncol. Rep. 38, 1967–1976. doi:10.3892/or.2017.5883
Garcia-Elias, A., Mrkonjic, S., Pardo-Pastor, C., Inada, H., Hellmich, U. A., Rubio-Moscardo, F., et al. (2013). Phosphatidylinositol-4, 5-biphosphate-dependent rearrangement of TRPV4 cytosolic tails enables channel activation by physiological stimuli. Proc. Natl. Acad. Sci. U. S. A. 110, 9553–9558. doi:10.1073/pnas.1220231110
Ge, J., Li, W., Zhao, Q., Li, N., Chen, M., Zhi, P., et al. (2015). Architecture of the mammalian mechanosensitive Piezo1 channel. Nature 527, 64–69. doi:10.1038/nature15247
Geiger, B., Spatz, J. P., and Bershadsky, A. D. (2009). Environmental sensing through focal adhesions. Nat. Rev. Mol. Cell Biol. 10, 21–33. doi:10.1038/nrm2593
Gensbittel, V., Krater, M., Harlepp, S., Busnelli, I., Guck, J., and Goetz, J. G. (2021). Mechanical adaptability of tumor cells in metastasis. Dev. Cell 56, 164–179. doi:10.1016/j.devcel.2020.10.011
Gilchrist, C. L., Leddy, H. A., Kaye, L., Case, N. D., Rothenberg, K. E., Little, D., et al. (2019). TRPV4-mediated calcium signaling in mesenchymal stem cells regulates aligned collagen matrix formation and vinculin tension. Proc. Natl. Acad. Sci. U. S. A. 116, 1992–1997. doi:10.1073/pnas.1811095116
Goetz, J. G., Minguet, S., Navarro-Lerida, I., Lazcano, J. J., Samaniego, R., Calvo, E., et al. (2011). Biomechanical remodeling of the microenvironment by stromal caveolin-1 favors tumor invasion and metastasis. Cell 146, 148–163. doi:10.1016/j.cell.2011.05.040
Gomis, A., Soriano, S., Belmonte, C., and Viana, F. (2008). Hypoosmotic- and pressure-induced membrane stretch activate TRPC5 channels. J. Physiol. 586, 5633–5649. doi:10.1113/jphysiol.2008.161257
Gonzalez-Molina, J., Zhang, X., Borghesan, M., Mendonca da Silva, J., Awan, M., Fuller, B., et al. (2018). Extracellular fluid viscosity enhances liver cancer cell mechanosensing and migration. Biomaterials 177, 113–124. doi:10.1016/j.biomaterials.2018.05.058
Gudipaty, S. A., Lindblom, J., Loftus, P. D., Redd, M. J., Edes, K., Davey, C. F., et al. (2017). Mechanical stretch triggers rapid epithelial cell division through Piezo1. Nature 543, 118–121. doi:10.1038/nature21407
Guo, J., She, J., Zeng, W., Chen, Q., Bai, X. C., and Jiang, Y. (2017). Structures of the calcium-activated, non-selective cation channel TRPM4. Nature 552, 205–209. doi:10.1038/nature24997
Haessler, U., Teo, J. C., Foretay, D., Renaud, P., and Swartz, M. A. (2012). Migration dynamics of breast cancer cells in a tunable 3D interstitial flow chamber. Integr. Biol. 4, 401–409. doi:10.1039/c1ib00128k
Han, J. W., Sung, P. S., Jang, J. W., Choi, J. Y., and Yoon, S. K. (2021). Whole blood viscosity is associated with extrahepatic metastases and survival in patients with hepatocellular carcinoma. PLoS One 16, e0260311. doi:10.1371/journal.pone.0260311
Han, Y., Liu, C., Zhang, D., Men, H., Huo, L., Geng, Q., et al. (2019). Mechanosensitive ion channel Piezo1 promotes prostate cancer development through the activation of the Akt/mTOR pathway and acceleration of cell cycle. Int. J. Oncol. 55, 629–644. doi:10.3892/ijo.2019.4839
Han, Y. L., Pegoraro, A. F., Li, H., Li, K., Yuan, Y., Xu, G., et al. (2020). Cell swelling, softening and invasion in a three-dimensional breast cancer model. Nat. Phys. 16, 101–108. doi:10.1038/s41567-019-0680-8
Hanahan, D., and Weinberg, R. A. (2011). Hallmarks of cancer: The next generation. Cell 144, 646–674. doi:10.1016/j.cell.2011.02.013
Handorf, A. M., Zhou, Y., Halanski, M. A., and Li, W. J. (2015). Tissue stiffness dictates development, homeostasis, and disease progression. Organogenesis 11, 1–15. doi:10.1080/15476278.2015.1019687
Hanley, W. D., Burdick, M. M., Konstantopoulos, K., and Sackstein, R. (2005). CD44 on LS174T colon carcinoma cells possesses E-selectin ligand activity. Cancer Res. 65, 5812–5817. doi:10.1158/0008-5472.CAN-04-4557
Hanley, W. D., Napier, S. L., Burdick, M. M., Schnaar, R. L., Sackstein, R., and Konstantopoulos, K. (2006). Variant isoforms of CD44 are P- and L-selectin ligands on colon carcinoma cells. FASEB J. 20, 337–339. doi:10.1096/fj.05-4574fje
Harada, T., Swift, J., Irianto, J., Shin, J. W., Spinler, K. R., Athirasala, A., et al. (2014). Nuclear lamin stiffness is a barrier to 3D migration, but softness can limit survival. J. Cell Biol. 204, 669–682. doi:10.1083/jcb.201308029
Harney, A. S., Arwert, E. N., Entenberg, D., Wang, Y., Guo, P., Qian, B. Z., et al. (2015). Real-time imaging reveals local, transient vascular permeability, and tumor cell intravasation stimulated by TIE2hi macrophage-derived VEGFA. Cancer Discov. 5, 932–943. doi:10.1158/2159-8290.CD-15-0012
Harunaga, J. S., and Yamada, K. M. (2011). Cell-matrix adhesions in 3D. Matrix Biol. 30, 363–368. doi:10.1016/j.matbio.2011.06.001
Hasegawa, K., Fujii, S., Matsumoto, S., Tajiri, Y., Kikuchi, A., and Kiyoshima, T. (2021). YAP signaling induces PIEZO1 to promote oral squamous cell carcinoma cell proliferation. J. Pathol. 253, 80–93. doi:10.1002/path.5553
Hashizume, H., Baluk, P., Morikawa, S., McLean, J. W., Thurston, G., Roberge, S., et al. (2000). Openings between defective endothelial cells explain tumor vessel leakiness. Am. J. Pathol. 156, 1363–1380. doi:10.1016/S0002-9440(10)65006-7
He, B., Liu, F., Ruan, J., Li, A., Chen, J., Li, R., et al. (2012). Silencing TRPC1 expression inhibits invasion of CNE2 nasopharyngeal tumor cells. Oncol. Rep. 27, 1548–1554. doi:10.3892/or.2012.1695
Hirata, H., Tatsumi, H., and Sokabe, M. (2008). Mechanical forces facilitate actin polymerization at focal adhesions in a zyxin-dependent manner. J. Cell Sci. 121, 2795–2804. doi:10.1242/jcs.030320
Horton, E. R., Astudillo, P., Humphries, M. J., and Humphries, J. D. (2016). Mechanosensitivity of integrin adhesion complexes: Role of the consensus adhesome. Exp. Cell Res. 343, 7–13. doi:10.1016/j.yexcr.2015.10.025
Huang, Y., Winkler, P. A., Sun, W., Lü, W., and Du, J. (2018). Architecture of the TRPM2 channel and its activation mechanism by ADP-ribose and calcium. Nature 562, 145–149. doi:10.1038/s41586-018-0558-4
Huang, Z., Sun, Z., Zhang, X., Niu, K., Wang, Y., Zheng, J., et al. (2019). Loss of stretch-activated channels, PIEZOs, accelerates non-small cell lung cancer progression and cell migration. Biosci. Rep. 39, BSR20181679. doi:10.1042/BSR20181679
Hung, W. C., Chen, S. H., Paul, C. D., Stroka, K. M., Lo, Y. C., Yang, J. T., et al. (2013). Distinct signaling mechanisms regulate migration in unconfined versus confined spaces. J. Cell Biol. 202, 807–824. doi:10.1083/jcb.201302132
Hung, W. C., Yang, J. R., Yankaskas, C. L., Wong, B. S., Wu, P. H., Pardo-Pastor, C., et al. (2016). Confinement sensing and signal optimization via Piezo1/PKA and myosin II pathways. Cell Rep. 15, 1430–1441. doi:10.1016/j.celrep.2016.04.035
Huttenlocher, A., Palecek, S. P., Lu, Q., Zhang, W., Mellgren, R. L., Lauffenburger, D. A., et al. (1997). Regulation of cell migration by the calcium-dependent protease calpain. J. Biol. Chem. 272, 32719–32722. doi:10.1074/jbc.272.52.32719
Huynh, K. W., Cohen, M. R., Jiang, J., Samanta, A., Lodowski, D. T., Zhou, Z. H., et al. (2016). Structure of the full-length TRPV2 channel by cryo-EM. Nat. Commun. 7, 11130. doi:10.1038/ncomms11130
Jain, R. K., and Baxter, L. T. (1988). Mechanisms of heterogeneous distribution of monoclonal antibodies and other macromolecules in tumors: Significance of elevated interstitial pressure. Cancer Res. 48, 7022–7032.
Jain, R. K., Martin, J. D., and Stylianopoulos, T. (2014). The role of mechanical forces in tumor growth and therapy. Annu. Rev. Biomed. Eng. 16, 321–346. doi:10.1146/annurev-bioeng-071813-105259
Jerrell, R. J., and Parekh, A. (2014). Cellular traction stresses mediate extracellular matrix degradation by invadopodia. Acta Biomater. 10, 1886–1896. doi:10.1016/j.actbio.2013.12.058
Jiang, H. N., Zeng, B., Zhang, Y., Daskoulidou, N., Fan, H., Qu, J. M., et al. (2013). Involvement of TRPC channels in lung cancer cell differentiation and the correlation analysis in human non-small cell lung cancer. PLoS One 8, e67637. doi:10.1371/journal.pone.0067637
Jiang, L., Zhao, Y. D., and Chen, W. X. (2017). The function of the novel mechanical activated ion channel Piezo1 in the human osteosarcoma cells. Med. Sci. Monit. 23, 5070–5082. doi:10.12659/msm.906959
Kallergi, G., Konstantinidis, G., Markomanolaki, H., Papadaki, M. A., Mavroudis, D., Stournaras, C., et al. (2013). Apoptotic circulating tumor cells in early and metastatic breast cancer patients. Mol. Cancer Ther. 12, 1886–1895. doi:10.1158/1535-7163.MCT-12-1167
Kao, Y. C., Jheng, J. R., Pan, H. J., Liao, W. Y., Lee, C. H., and Kuo, P. L. (2017). Elevated hydrostatic pressure enhances the motility and enlarges the size of the lung cancer cells through aquaporin upregulation mediated by caveolin-1 and ERK1/2 signaling. Oncogene 36, 863–874. doi:10.1038/onc.2016.255
Kapinas, K., Lowther, K. M., Kessler, C. B., Tilbury, K., Lieberman, J. R., Tirnauer, J. S., et al. (2012). Bone matrix osteonectin limits prostate cancer cell growth and survival. Matrix Biol. 31, 299–307. doi:10.1016/j.matbio.2012.03.002
Kappel, S., Stokłosa, P., Hauert, B., Ross-Kaschitza, D., Borgström, A., Baur, R., et al. (2019). TRPM4 is highly expressed in human colorectal tumor buds and contributes to proliferation, cell cycle, and invasion of colorectal cancer cells. Mol. Oncol. 13, 2393–2405. doi:10.1002/1878-0261.12566
Karagiannis, G. S., Poutahidis, T., Erdman, S. E., Kirsch, R., Riddell, R. H., and Diamandis, E. P. (2012). Cancer-associated fibroblasts drive the progression of metastasis through both paracrine and mechanical pressure on cancer tissue. Mol. Cancer Res. 10, 1403–1418. doi:10.1158/1541-7786.MCR-12-0307
Karki, T., and Tojkander, S. (2021). TRPV protein family-from mechanosensing to cancer invasion. Biomolecules 11, 1019. doi:10.3390/biom11071019
Kato, S., Shiozaki, A., Kudou, M., Shimizu, H., Kosuga, T., Ohashi, T., et al. (2022). TRPV2 promotes cell migration and invasion in gastric cancer via the transforming growth factor-β signaling pathway. Ann. Surg. Oncol. 29, 2944–2956. doi:10.1245/s10434-021-11132-5
Khan, M., Puniyani, R., Huilgol, N., Hussain, M., and Ranade, G. (1995). Hemorheological profiles in cancer patients. Clin. Hemorheol. Microcirc. 15, 37–44. doi:10.3233/ch-1995-15105
Khorchid, A., and Ikura, M. (2002). How calpain is activated by calcium. Nat. Struct. Biol. 9, 239–241. doi:10.1038/nsb0402-239
Klein, E. A., Yin, L., Kothapalli, D., Castagnino, P., Byfield, F. J., Xu, T., et al. (2009). Cell-cycle control by physiological matrix elasticity and in vivo tissue stiffening. Curr. Biol. 19, 1511–1518. doi:10.1016/j.cub.2009.07.069
Kobayashi, T., and Sokabe, M. (2010). Sensing substrate rigidity by mechanosensitive ion channels with stress fibers and focal adhesions. Curr. Opin. Cell Biol. 22, 669–676. doi:10.1016/j.ceb.2010.08.023
Konstantopoulos, K., and Thomas, S. N. (2009). Cancer cells in transit: The vascular interactions of tumor cells. Annu. Rev. Biomed. Eng. 11, 177–202. doi:10.1146/annurev-bioeng-061008-124949
Koser, D. E., Thompson, A. J., Foster, S. K., Dwivedy, A., Pillai, E. K., Sheridan, G. K., et al. (2016). Mechanosensing is critical for axon growth in the developing brain. Nat. Neurosci. 19, 1592–1598. doi:10.1038/nn.4394
Koumoutsakos, P., Pivkin, I., and Milde, F. (2013). The fluid mechanics of cancer and its therapy. Annu. Rev. Fluid Mech. 45, 325–355. doi:10.1146/annurev-fluid-120710-101102
Kowalski, P. J., Rubin, M. A., and Kleer, C. G. (2003). E-cadherin expression in primary carcinomas of the breast and its distant metastases. Breast Cancer Res. 5, R217–R222. doi:10.1186/bcr651
Krapivinsky, G., Krapivinsky, L., Manasian, Y., and Clapham, D. E. (2014). The TRPM7 chanzyme is cleaved to release a chromatin-modifying kinase. Cell 157, 1061–1072. doi:10.1016/j.cell.2014.03.046
Kudou, M., Shiozaki, A., Yamazato, Y., Katsurahara, K., Kosuga, T., Shoda, K., et al. (2019). The expression and role of TRPV2 in esophageal squamous cell carcinoma. Sci. Rep. 9, 16055. doi:10.1038/s41598-019-52227-0
Kumar, V., Denis, M., Gregory, A., Bayat, M., Mehrmohammadi, M., Fazzio, R., et al. (2018). Viscoelastic parameters as discriminators of breast masses: Initial human study results. PLoS One 13, e0205717. doi:10.1371/journal.pone.0205717
Kuo, J. C., Han, X., Hsiao, C. T., Yates, J. R., and Waterman, C. M. (2011). Analysis of the myosin-II-responsive focal adhesion proteome reveals a role for beta-Pix in negative regulation of focal adhesion maturation. Nat. Cell Biol. 13, 383–393. doi:10.1038/ncb2216
Larson, C. J., Moreno, J. G., Pienta, K. J., Gross, S., Repollet, M., O'hara, S. M., et al. (2004). Apoptosis of circulating tumor cells in prostate cancer patients. Cytom. A 62, 46–53. doi:10.1002/cyto.a.20073
Laukaitis, C. M., Webb, D. J., Donais, K., and Horwitz, A. F. (2001). Differential dynamics of alpha 5 integrin, paxillin, and alpha-actinin during formation and disassembly of adhesions in migrating cells. J. Cell Biol. 153, 1427–1440. doi:10.1083/jcb.153.7.1427
Lee, H. J., Diaz, M. F., Price, K. M., Ozuna, J. A., Zhang, S., Sevick-Muraca, E. M., et al. (2017). Fluid shear stress activates YAP1 to promote cancer cell motility. Nat. Commun. 8, 14122. doi:10.1038/ncomms14122
Lee, H. P., Alisafaei, F., Adebawale, K., Chang, J., Shenoy, V. B., and Chaudhuri, O. (2021). The nuclear piston activates mechanosensitive ion channels to generate cell migration paths in confining microenvironments. Sci. Adv. 7, eabd4058. doi:10.1126/sciadv.abd4058
Lee, W. H., Choong, L. Y., Jin, T. H., Mon, N. N., Chong, S., Liew, C. S., et al. (2017). TRPV4 plays a role in breast cancer cell migration via Ca2+-dependent activation of AKT and downregulation of E-cadherin cell cortex protein. Oncogenesis 6, e338. doi:10.1038/oncsis.2017.39
Lee, W. H., Choong, L. Y., Mon, N. N., Lu, S., Lin, Q., Pang, B., et al. (2016). TRPV4 regulates breast cancer cell extravasation, stiffness and actin cortex. Sci. Rep. 6, 27903. doi:10.1038/srep27903
Lefebvre, T., Rybarczyk, P., Bretaudeau, C., Vanlaeys, A., Cousin, R., Brassart-Pasco, S., et al. (2020). TRPM7/RPSA complex regulates pancreatic cancer cell migration. Front. Cell Dev. Biol. 8, 549. doi:10.3389/fcell.2020.00549
Leong, H. S., Robertson, A. E., Stoletov, K., Leith, S. J., Chin, C. A., Chien, A. E., et al. (2014). Invadopodia are required for cancer cell extravasation and are a therapeutic target for metastasis. Cell Rep. 8, 1558–1570. doi:10.1016/j.celrep.2014.07.050
Levental, K. R., Yu, H., Kass, L., Lakins, J. N., Egeblad, M., Erler, J. T., et al. (2009). Matrix crosslinking forces tumor progression by enhancing integrin signaling. Cell 139, 891–906. doi:10.1016/j.cell.2009.10.027
Li, C., Rezania, S., Kammerer, S., Sokolowski, A., Devaney, T., Gorischek, A., et al. (2015). Piezo1 forms mechanosensitive ion channels in the human MCF-7 breast cancer cell line. Sci. Rep. 5, 8364. doi:10.1038/srep08364
Li, F., Abuarab, N., and Sivaprasadarao, A. (2016). Reciprocal regulation of actin cytoskeleton remodelling and cell migration by Ca2+ and Zn2+: Role of TRPM2 channels. J. Cell Sci. 129, 2016–2029. doi:10.1242/jcs.179796
Li, N., He, Y., Yang, G., Yu, Q., and Li, M. (2019). Role of TRPC1 channels in pressure-mediated activation of airway remodeling. Respir. Res. 20, 91. doi:10.1186/s12931-019-1050-x
Li, T., Sun, L., Miller, N., Nicklee, T., Woo, J., Hulse-Smith, L., et al. (2005). The association of measured breast tissue characteristics with mammographic density and other risk factors for breast cancer. Cancer Epidemiol. Biomarkers Prev. 14, 343–349. doi:10.1158/1055-9965.EPI-04-0490
Li, X. C., Cheng, Y., Yang, X., Zhou, J. Y., Dong, Y. Y., Shen, B. Q., et al. (2020). Decreased expression of TRPM4 is associated with unfavorable prognosis and aggressive progression of endometrial carcinoma. Am. J. Transl. Res. 12, 3926–3939.
Li, X., Cheng, Y., Wang, Z., Zhou, J., Jia, Y., He, X., et al. (2020). Calcium and TRPV4 promote metastasis by regulating cytoskeleton through the RhoA/ROCK1 pathway in endometrial cancer. Cell Death Dis. 11, 1009. doi:10.1038/s41419-020-03181-7
Li, X., Han, L., Nookaew, I., Mannen, E., Silva, M. J., Almeida, M., et al. (2019). Stimulation of Piezo1 by mechanical signals promotes bone anabolism. Elife 8, e49631. doi:10.7554/eLife.49631
Li, Y., Konstantopoulos, K., Zhao, R., Mori, Y., and Sun, S. X. (2020). The importance of water and hydraulic pressure in cell dynamics. J. Cell Sci. 133, jcs240341. doi:10.1242/jcs.240341
Li, Y., and Sun, S. X. (2018). Transition from actin-driven to water-driven cell migration depends on external hydraulic resistance. Biophys. J. 114, 2965–2973. doi:10.1016/j.bpj.2018.04.045
Li, Y., Yao, L., Mori, Y., and Sun, S. X. (2019). On the energy efficiency of cell migration in diverse physical environments. Proc. Natl. Acad. Sci. U. S. A. 116, 23894–23900. doi:10.1073/pnas.1907625116
Liao, M., Cao, E., Julius, D., and Cheng, Y. (2013). Structure of the TRPV1 ion channel determined by electron cryo-microscopy. Nature 504, 107–112. doi:10.1038/nature12822
Liedtke, W., Tobin, D. M., Bargmann, C. I., and Friedman, J. M. (2003). Mammalian TRPV4 (VR-OAC) directs behavioral responses to osmotic and mechanical stimuli in Caenorhabditis elegans. Proc. Natl. Acad. Sci. U. S. A. 100 (2), 14531–14536. doi:10.1073/pnas.2235619100
Lin, R., Bao, X., Wang, H., Zhu, S., Liu, Z., Chen, Q., et al. (2021). TRPM2 promotes pancreatic cancer by PKC/MAPK pathway. Cell Death Dis. 12, 585. doi:10.1038/s41419-021-03856-9
Lin, R., Wang, Y., Chen, Q., Liu, Z., Xiao, S., Wang, B., et al. (2018). TRPM2 promotes the proliferation and invasion of pancreatic ductal adenocarcinoma. Mol. Med. Rep. 17, 7537–7544. doi:10.3892/mmr.2018.8816
Lin, Y. C., Guo, Y. R., Miyagi, A., Levring, J., MacKinnon, R., and Scheuring, S. (2019). Force-induced conformational changes in PIEZO1. Nature 573, 230–234. doi:10.1038/s41586-019-1499-2
Liu, B., and Qin, F. (2016). Use dependence of heat sensitivity of vanilloid receptor TRPV2. Biophys. J. 110, 1523–1537. doi:10.1016/j.bpj.2016.03.005
Liu, L., Wu, N., Wang, Y., Zhang, X., Xia, B., Tang, J., et al. (2019). TRPM7 promotes the epithelial-mesenchymal transition in ovarian cancer through the calcium-related PI3K/AKT oncogenic signaling. J. Exp. Clin. Cancer Res. 38, 106. doi:10.1186/s13046-019-1061-y
Liu, Q., and Wang, X. (2013). Effect of TRPV2 cation channels on the proliferation, migration and invasion of 5637 bladder cancer cells. Exp. Ther. Med. 6, 1277–1282. doi:10.3892/etm.2013.1301
Liu, T., Zhou, L., Li, D., Andl, T., and Zhang, Y. (2019). Cancer-associated fibroblasts build and secure the tumor microenvironment. Front. Cell Dev. Biol. 7, 60. doi:10.3389/fcell.2019.00060
Lo, C. M., Wang, H. B., Dembo, M., and Wang, Y. L. (2000). Cell movement is guided by the rigidity of the substrate. Biophys. J. 79, 144–152. doi:10.1016/S0006-3495(00)76279-5
Lomakin, A. J., Cattin, C. J., Cuvelier, D., Alraies, Z., Molina, M., Nader, G. P. F., et al. (2020). The nucleus acts as a ruler tailoring cell responses to spatial constraints. Science 370, eaba2894. doi:10.1126/science.aba2894
Lu, F., Sun, J., Zheng, Q., Li, J., Hu, Y., Yu, P., et al. (2019). Imaging elemental events of store-operated Ca(2+) entry in invading cancer cells with plasmalemmal targeted sensors. J. Cell Sci. 132, jcs224923. doi:10.1242/jcs.224923
Luo, M., Cai, G., Ho, K. K. Y., Wen, K., Tong, Z., Deng, L., et al. (2022). Compression enhances invasive phenotype and matrix degradation of breast Cancer cells via Piezo1 activation. BMC Mol. Cell Biol. 23, 1. doi:10.1186/s12860-021-00401-6
Ma, S., Dubin, A. E., Zhang, Y., Mousavi, S. A. R., Wang, Y., Coombs, A. M., et al. (2021). A role of PIEZO1 in iron metabolism in mice and humans. Cell 184, 969–982. e13. doi:10.1016/j.cell.2021.01.024
Ma, S., Fu, A., Chiew, G. G., and Luo, K. Q. (2017). Hemodynamic shear stress stimulates migration and extravasation of tumor cells by elevating cellular oxidative level. Cancer Lett. 388, 239–248. doi:10.1016/j.canlet.2016.12.001
Ma, X., Cai, Y., He, D., Zou, C., Zhang, P., Lo, C. Y., et al. (2012). Transient receptor potential channel TRPC5 is essential for P-glycoprotein induction in drug-resistant cancer cells. Proc. Natl. Acad. Sci. U. S. A. 109, 16282–16287. doi:10.1073/pnas.1202989109
Ma, X., Chen, Z., Hua, D., He, D., Wang, L., Zhang, P., et al. (2014). Essential role for TrpC5-containing extracellular vesicles in breast cancer with chemotherapeutic resistance. Proc. Natl. Acad. Sci. U. S. A. 111, 6389–6394. doi:10.1073/pnas.1400272111
Maity, D., Li, Y., Chen, Y., and Sun, S. X. (2019). Response of collagen matrices under pressure and hydraulic resistance in hydrogels. Soft Matter 15, 2617–2626. doi:10.1039/c8sm02143k
Marinelli, O., Morelli, M. B., Annibali, D., Aguzzi, C., Zeppa, L., Tuyaerts, S., et al. (2020). The effects of cannabidiol and prognostic role of TRPV2 in human endometrial cancer. Int. J. Mol. Sci. 21, E5409. doi:10.3390/ijms21155409
Martinez, E., Engel, E., Planell, J. A., and Samitier, J. (2009). Effects of artificial micro- and nano-structured surfaces on cell behaviour. Ann. Anat. 191, 126–135. doi:10.1016/j.aanat.2008.05.006
Matthews, B. D., Overby, D. R., Mannix, R., and Ingber, D. E. (2006). Cellular adaptation to mechanical stress: Role of integrins, Rho, cytoskeletal tension and mechanosensitive ion channels. J. Cell Sci. 119, 508–518. doi:10.1242/jcs.02760
McHugh, B. J., Murdoch, A., Haslett, C., and Sethi, T. (2012). Loss of the integrin-activating transmembrane protein Fam38A (Piezo1) promotes a switch to a reduced integrin-dependent mode of cell migration. PLoS One 7, e40346. doi:10.1371/journal.pone.0040346
Mendoza, S. A., Fang, J., Gutterman, D. D., Wilcox, D. A., Bubolz, A. H., Li, R., et al. (2010). TRPV4-mediated endothelial Ca2+ influx and vasodilation in response to shear stress. Am. J. Physiol. Heart Circ. Physiol. 298, H466–H476. doi:10.1152/ajpheart.00854.2009
Meng, X., Cai, C., Wu, J., Cai, S., Ye, C., Chen, H., et al. (2013). TRPM7 mediates breast cancer cell migration and invasion through the MAPK pathway. Cancer Lett. 333, 96–102. doi:10.1016/j.canlet.2013.01.031
Micalet, A., Moeendarbary, E., and Cheema, U. (2021). 3D in vitro models for investigating the role of stiffness in cancer invasion. ACS Biomater. Sci. Eng. 1, 0c01530. doi:10.1021/acsbiomaterials.0c01530
Middelbeek, J., Kuipers, A. J., Henneman, L., Visser, D., Eidhof, I., van Horssen, R., et al. (2012). TRPM7 is required for breast tumor cell metastasis. Cancer Res. 72, 4250–4261. doi:10.1158/0008-5472.CAN-11-3863
Mierke, C. T. (2021). Viscoelasticity acts as a marker for tumor extracellular matrix characteristics. Front. Cell Dev. Biol. 9, 785138. doi:10.3389/fcell.2021.785138
Mihara, H., Boudaka, A., Shibasaki, K., Yamanaka, A., Sugiyama, T., and Tominaga, M. (2010). Involvement of TRPV2 activation in intestinal movement through nitric oxide production in mice. J. Neurosci. 30, 16536–16544. doi:10.1523/JNEUROSCI.4426-10.2010
Mistriotis, P., Wisniewski, E. O., Bera, K., Keys, J., Li, Y., Tuntithavornwat, S., et al. (2019). Confinement hinders motility by inducing RhoA-mediated nuclear influx, volume expansion, and blebbing. J. Cell Biol. 218, 4093–4111. doi:10.1083/jcb.201902057
Montell, C. (2005). The TRP superfamily of cation channels. Sci. STKE 2005, re3. doi:10.1126/stke.2722005re3
Moose, D. L., Krog, B. L., Kim, T. H., Zhao, L., Williams-Perez, S., Burke, G., et al. (2020). Cancer cells resist mechanical destruction in circulation via RhoA/actomyosin-dependent mechano-adaptation. Cell Rep. 30, 3864–3874. e6. doi:10.1016/j.celrep.2020.02.080
Morita, H., Honda, A., Inoue, R., Ito, Y., Abe, K., Nelson, M. T., et al. (2007). Membrane stretch-induced activation of a TRPM4-like nonselective cation channel in cerebral artery myocytes. J. Pharmacol. Sci. 103, 417–426. doi:10.1254/jphs.fp0061332
Moroni, M., Servin-Vences, M. R., Fleischer, R., Sánchez-Carranza, O., and Lewin, G. R. (2018). Voltage gating of mechanosensitive PIEZO channels. Nat. Commun. 9, 1096. doi:10.1038/s41467-018-03502-7
Mousawi, F., Peng, H., Li, J., Ponnambalam, S., Roger, S., Zhao, H., et al. (2020). Chemical activation of the Piezo1 channel drives mesenchymal stem cell migration via inducing ATP release and activation of P2 receptor purinergic signaling. Stem Cells 38, 410–421. doi:10.1002/stem.3114
Mrkonjić, S., Garcia-Elias, A., Pardo-Pastor, C., Bazellières, E., Trepat, X., Vriens, J., et al. (2015). TRPV4 participates in the establishment of trailing adhesions and directional persistence of migrating cells. Pflugers Arch. 467, 2107–2119. doi:10.1007/s00424-014-1679-8
Mukherjee, A., Behkam, B., and Nain, A. S. (2019). Cancer cells sense fibers by coiling on them in a curvature-dependent manner. iScience 19, 905–915. doi:10.1016/j.isci.2019.08.023
Naba, A., Clauser, K. R., Lamar, J. M., Carr, S. A., and Hynes, R. O. (2014). Extracellular matrix signatures of human mammary carcinoma identify novel metastasis promoters. Elife 3, e01308. doi:10.7554/eLife.01308
Nabissi, M., Morelli, M. B., Santoni, M., and Santoni, G. (2013). Triggering of the TRPV2 channel by cannabidiol sensitizes glioblastoma cells to cytotoxic chemotherapeutic agents. Carcinogenesis 34, 48–57. doi:10.1093/carcin/bgs328
Nadiarnykh, O., LaComb, R. B., Brewer, M. A., and Campagnola, P. J. (2010). Alterations of the extracellular matrix in ovarian cancer studied by Second Harmonic Generation imaging microscopy. BMC Cancer 10, 94. doi:10.1186/1471-2407-10-94
Napier, S. L., Healy, Z. R., Schnaar, R. L., and Konstantopoulos, K. (2007). Selectin ligand expression regulates the initial vascular interactions of colon carcinoma cells: The roles of CD44v and alternative sialofucosylated selectin ligands. J. Biol. Chem. 282, 3433–3441. doi:10.1074/jbc.M607219200
Nayal, A., Webb, D. J., Brown, C. M., Schaefer, E. M., Vicente-Manzanares, M., and Horwitz, A. R. (2006). Paxillin phosphorylation at Ser273 localizes a GIT1-PIX-PAK complex and regulates adhesion and protrusion dynamics. J. Cell Biol. 173, 587–589. doi:10.1083/jcb.200509075
Ngan, E., Stoletov, K., Smith, H. W., Common, J., Muller, W. J., Lewis, J. D., et al. (2017). LPP is a Src substrate required for invadopodia formation and efficient breast cancer lung metastasis. Nat. Commun. 8, 15059. doi:10.1038/ncomms15059
Nia, H. T., Munn, L. L., and Jain, R. K. (2020). Physical traits of cancer. Science 370, eaaz0868. doi:10.1126/science.aaz0868
Nilius, B., Prenen, J., Tang, J., Wang, C., Owsianik, G., Janssens, A., et al. (2005). Regulation of the Ca2+ sensitivity of the nonselective cation channel TRPM4. J. Biol. Chem. 280, 6423–6433. doi:10.1074/jbc.M411089200
Ningoo, M., Plant, L. D., Greka, A., and Logothetis, D. E. (2021). PIP2 regulation of TRPC5 channel activation and desensitization. J. Biol. Chem. 296, 100726. doi:10.1016/j.jbc.2021.100726
Numata, T., Shimizu, T., and Okada, Y. (2007). TRPM7 is a stretch- and swelling-activated cation channel involved in volume regulation in human epithelial cells. Am. J. Physiol. Cell Physiol. 292, C460–C467. doi:10.1152/ajpcell.00367.2006
Ondeck, M. G., Kumar, A., Placone, J. K., Plunkett, C. M., Matte, B. F., Wong, K. C., et al. (2019). Dynamically stiffened matrix promotes malignant transformation of mammary epithelial cells via collective mechanical signaling. Proc. Natl. Acad. Sci. U. S. A. 116, 3502–3507. doi:10.1073/pnas.1814204116
Osmani, N., Follain, G., García León, M. J., Lefebvre, O., Busnelli, I., Larnicol, A., et al. (2019). Metastatic tumor cells exploit their adhesion repertoire to counteract shear forces during intravascular arrest. Cell Rep. 28, 2491–2500. e5. doi:10.1016/j.celrep.2019.07.102
Ou-Yang, Q., Li, B., Xu, M., and Liang, H. (2018). TRPV4 promotes the migration and invasion of glioma cells via AKT/Rac1 signaling. Biochem. Biophys. Res. Commun. 503, 876–881. doi:10.1016/j.bbrc.2018.06.090
Padmanaban, V., Krol, I., Suhail, Y., Szczerba, B. M., Aceto, N., Bader, J. S., et al. (2019). E-cadherin is required for metastasis in multiple models of breast cancer. Nature 573, 439–444. doi:10.1038/s41586-019-1526-3
Pankov, R., Cukierman, E., Katz, B. Z., Matsumoto, K., Lin, D. C., Lin, S., et al. (2000). Integrin dynamics and matrix assembly: Tensin-dependent translocation of alpha(5)beta(1) integrins promotes early fibronectin fibrillogenesis. J. Cell Biol. 148, 1075–1090. doi:10.1083/jcb.148.5.1075
Pankova, K., Rosel, D., Novotny, M., and Brabek, J. (2010). The molecular mechanisms of transition between mesenchymal and amoeboid invasiveness in tumor cells. Cell. Mol. Life Sci. 67, 63–71. doi:10.1007/s00018-009-0132-1
Pardo-Pastor, C., Rubio-Moscardo, F., Vogel-Gonzalez, M., Serra, S. A., Afthinos, A., Mrkonjic, S., et al. (2018). Piezo2 channel regulates RhoA and actin cytoskeleton to promote cell mechanobiological responses. Proc. Natl. Acad. Sci. U. S. A. 115, 1925–1930. doi:10.1073/pnas.1718177115
Park, S., Jung, W. H., Pittman, M., Chen, J., and Chen, Y. (2020). The effects of stiffness, fluid viscosity, and geometry of microenvironment in homeostasis, aging, and diseases: A brief review. J. Biomech. Eng. 142, 100804. doi:10.1115/1.4048110
Parsons, J. T., Horwitz, A. R., and Schwartz, M. A. (2010). Cell adhesion: Integrating cytoskeletal dynamics and cellular tension. Nat. Rev. Mol. Cell Biol. 11, 633–643. doi:10.1038/nrm2957
Paszek, M. J., Zahir, N., Johnson, K. R., Lakins, J. N., Rozenberg, G. I., Gefen, A., et al. (2005). Tensional homeostasis and the malignant phenotype. Cancer Cell 8, 241–254. doi:10.1016/j.ccr.2005.08.010
Patel, A., Sharif-Naeini, R., Folgering, J. R. H., Bichet, D., Duprat, F., and Honore, E. (2010). Canonical TRP channels and mechanotransduction: From physiology to disease states. Pflugers Arch. 460, 571–581. doi:10.1007/s00424-010-0847-8
Pathak, M. M., Nourse, J. L., Tran, T., Hwe, J., Arulmoli, J., Le, D. T., et al. (2014). Stretch-activated ion channel Piezo1 directs lineage choice in human neural stem cells. Proc. Natl. Acad. Sci. U. S. A. 111, 16148–16153. doi:10.1073/pnas.1409802111
Patsialou, A., Bravo-Cordero, J. J., Wang, Y., Entenberg, D., Liu, H., Clarke, M., et al. (2013). Intravital multiphoton imaging reveals multicellular streaming as a crucial component of in vivo cell migration in human breast tumors. Intravital 2, e25294. doi:10.4161/intv.25294
Paul, C. D., Mistriotis, P., and Konstantopoulos, K. (2017). Cancer cell motility: Lessons from migration in confined spaces. Nat. Rev. Cancer 17, 131–140. doi:10.1038/nrc.2016.123
Paul, C. D., Shea, D. J., Mahoney, M. R., Chai, A., Laney, V., Hung, W. C., et al. (2016). Interplay of the physical microenvironment, contact guidance, and intracellular signaling in cell decision making. FASEB J. 30, 2161–2170. doi:10.1096/fj.201500199R
Pedersen, S. F., and Nilius, B. (2007). Transient receptor potential channels in mechanosensing and cell volume regulation. Methods Enzymol. 428, 183–207. doi:10.1016/S0076-6879(07)28010-3
Pelham, R. J., and Wang, Y. (1997). Cell locomotion and focal adhesions are regulated by substrate flexibility. Proc. Natl. Acad. Sci. U. S. A. 94, 13661–13665. doi:10.1073/pnas.94.25.13661
Perraud, A. L., Takanishi, C. L., Shen, B., Kang, S., Smith, M. K., Schmitz, C., et al. (2005). Accumulation of free ADP-ribose from mitochondria mediates oxidative stress-induced gating of TRPM2 cation channels. J. Biol. Chem. 280, 6138–6148. doi:10.1074/jbc.M411446200
Petho, Z., Najder, K., Bulk, E., and Schwab, A. (2019). Mechanosensitive ion channels push cancer progression. Cell Calcium 80, 79–90. doi:10.1016/j.ceca.2019.03.007
Piotrowski-Daspit, A. S., Tien, J., and Nelson, C. M. (2016). Interstitial fluid pressure regulates collective invasion in engineered human breast tumors via Snail, vimentin, and E-cadherin. Integr. Biol. 8, 319–331. doi:10.1039/c5ib00282f
Plodinec, M., Loparic, M., Monnier, C. A., Obermann, E. C., Zanetti-Dallenbach, R., Oertle, P., et al. (2012). The nanomechanical signature of breast cancer. Nat. Nanotechnol. 7, 757–765. doi:10.1038/nnano.2012.167
Plotnikov, S. V., Pasapera, A. M., Sabass, B., and Waterman, C. M. (2012). Force fluctuations within focal adhesions mediate ECM-rigidity sensing to guide directed cell migration. Cell 151, 1513–1527. doi:10.1016/j.cell.2012.11.034
Prentice-Mott, H. V., Chang, C. H., Mahadevan, L., Mitchison, T. J., Irimia, D., and Shah, J. V. (2013). Biased migration of confined neutrophil-like cells in asymmetric hydraulic environments. Proc. Natl. Acad. Sci. U. S. A. 110, 21006–21011. doi:10.1073/pnas.1317441110
Provenzano, P. P., Eliceiri, K. W., Campbell, J. M., Inman, D. R., White, J. G., and Keely, P. J. (2006). Collagen reorganization at the tumor-stromal interface facilitates local invasion. BMC Med. 4, 38. doi:10.1186/1741-7015-4-38
Provenzano, P. P., Inman, D. R., Eliceiri, K. W., and Keely, P. J. (2009). Matrix density-induced mechanoregulation of breast cell phenotype, signaling and gene expression through a FAK-ERK linkage. Oncogene 28, 4326–4343. doi:10.1038/onc.2009.299
Provenzano, P. P., Inman, D. R., Eliceiri, K. W., Knittel, J. G., Yan, L., Rueden, C. T., et al. (2008). Collagen density promotes mammary tumor initiation and progression. BMC Med. 6, 11. doi:10.1186/1741-7015-6-11
Raab, M., Gentili, M., de Belly, H., Thiam, H. R., Vargas, P., Jimenez, A. J., et al. (2016). ESCRT III repairs nuclear envelope ruptures during cell migration to limit DNA damage and cell death. Science 352, 359–362. doi:10.1126/science.aad7611
Ranade, S. S., Qiu, Z., Woo, S. H., Hur, S. S., Murthy, S. E., Cahalan, S. M., et al. (2014). Piezo1, a mechanically activated ion channel, is required for vascular development in mice. Proc. Natl. Acad. Sci. U. S. A. 111, 10347–10352. doi:10.1073/pnas.1409233111
Ranade, S. S., Syeda, R., and Patapoutian, A. (2015). Mechanically activated ion channels. Neuron 87, 1162–1179. doi:10.1016/j.neuron.2015.08.032
Ranade, S. S., Woo, S. H., Dubin, A. E., Moshourab, R. A., Wetzel, C., Petrus, M., et al. (2014). Piezo2 is the major transducer of mechanical forces for touch sensation in mice. Nature 516, 121–125. doi:10.1038/nature13980
Renkawitz, J., Kopf, A., Stopp, J., de Vries, I., Driscoll, M. K., Merrin, J., et al. (2019). Nuclear positioning facilitates amoeboid migration along the path of least resistance. Nature 568, 546–550. doi:10.1038/s41586-019-1087-5
Resto, V. A., Burdick, M. M., Dagia, N. M., McCammon, S. D., Fennewald, S. M., and Sackstein, R. (2008). L-selectin-mediated lymphocyte-cancer cell interactions under low fluid shear conditions. J. Biol. Chem. 283, 15816–15824. doi:10.1074/jbc.M708899200
Revach, O. Y., Grosheva, I., and Geiger, B. (2020). Biomechanical regulation of focal adhesion and invadopodia formation. J. Cell Sci. 133, jcs244848. doi:10.1242/jcs.244848
Rivron, N. C., Vrij, E. J., Rouwkema, J., Le Gac, S., van den Berg, A., Truckenmüller, R. K., et al. (2012). Tissue deformation spatially modulates VEGF signaling and angiogenesis. Proc. Natl. Acad. Sci. U. S. A. 109, 6886–6891. doi:10.1073/pnas.1201626109
Rosenson, R. S., McCormick, A., and Uretz, E. F. (1996). Distribution of blood viscosity values and biochemical correlates in healthy adults. Clin. Chem. 42, 1189–1195. doi:10.1093/clinchem/42.8.1189
Rozario, T., and DeSimone, D. W. (2010). The extracellular matrix in development and morphogenesis: A dynamic view. Dev. Biol. 341, 126–140. doi:10.1016/j.ydbio.2009.10.026
Ruehle, M. A., Eastburn, E. A., LaBelle, S. A., Krishnan, L., Weiss, J. A., Boerckel, J. D., et al. (2020). Extracellular matrix compression temporally regulates microvascular angiogenesis. Sci. Adv. 6, eabb6351. doi:10.1126/sciadv.abb6351
Rybarczyk, P., Vanlaeys, A., Brassart, B., Dhennin-Duthille, I., Chatelain, D., Sevestre, H., et al. (2017). The transient receptor potential melastatin 7 channel regulates pancreatic cancer cell invasion through the hsp90α/uPA/MMP2 pathway. Neoplasia 19, 288–300. doi:10.1016/j.neo.2017.01.004
Sagredo, A. I., Sagredo, E. A., Pola, V., Echeverría, C., Andaur, R., Michea, L., et al. (2019). TRPM4 channel is involved in regulating epithelial to mesenchymal transition, migration, and invasion of prostate cancer cell lines. J. Cell. Physiol. 234, 2037–2050. doi:10.1002/jcp.27371
Santoni, G., Amantini, C., Maggi, F., Marinelli, O., Santoni, M., Nabissi, M., et al. (2020). The TRPV2 cation channels: From urothelial cancer invasiveness to glioblastoma multiforme interactome signature. Lab. Invest. 100, 186–198. doi:10.1038/s41374-019-0333-7
Saotome, K., Murthy, S. E., Kefauver, J. M., Whitwam, T., Patapoutian, A., and Ward, A. B. (2018). Structure of the mechanically activated ion channel Piezo1. Nature 554, 481–486. doi:10.1038/nature25453
Schedin, P., and Keely, P. J. (2011). Mammary gland ECM remodeling, stiffness, and mechanosignaling in normal development and tumor progression. Cold Spring Harb. Perspect. Biol. 3, a003228. doi:10.1101/cshperspect.a003228
Schonherr, E., and Hausser, H. J. (2000). Extracellular matrix and cytokines: A functional unit. Dev. Immunol. 7, 89–101. doi:10.1155/2000/31748
Sens, P., and Turner, M. S. (2006). Budded membrane microdomains as tension regulators. Phys. Rev. E Stat. Nonlin. Soft Matter Phys. 73, 031918. doi:10.1103/PhysRevE.73.031918
Shah, P., Hobson, C. M., Cheng, S., Colville, M. J., Paszek, M. J., Superfine, R., et al. (2021). Nuclear deformation causes DNA damage by increasing replication stress. Curr. Biol. 31, 753–765 e6. doi:10.1016/j.cub.2020.11.037
Sharma, P., Sheets, K., Elankumaran, S., and Nain, A. S. (2013). The mechanistic influence of aligned nanofibers on cell shape, migration and blebbing dynamics of glioma cells. Integr. Biol. 5, 1036–1044. doi:10.1039/c3ib40073e
Sharma, S., Goswami, R., and Rahaman, S. O. (2019). The TRPV4-TAZ mechanotransduction signaling axis in matrix stiffness- and TGFβ1-induced epithelial-mesenchymal transition. Cell. Mol. Bioeng. 12, 139–152. doi:10.1007/s12195-018-00565-w
Shen, B., Wong, C. O., Lau, O. C., Woo, T., Bai, S., Huang, Y., et al. (2015). Plasma membrane mechanical stress activates TRPC5 channels. PLoS One 10, e0122227. doi:10.1371/journal.pone.0122227
Shen, M., and Kang, Y. (2020). Stresses in the metastatic cascade: Molecular mechanisms and therapeutic opportunities. Genes Dev. 34, 1577–1598. doi:10.1101/gad.343251.120
Shibasaki, K., Murayama, N., Ono, K., Ishizaki, Y., and Tominaga, M. (2010). TRPV2 enhances axon outgrowth through its activation by membrane stretch in developing sensory and motor neurons. J. Neurosci. 30, 4601–4612. doi:10.1523/JNEUROSCI.5830-09.2010
Sinkus, R., Siegmann, K., Xydeas, T., Tanter, M., Claussen, C., and Fink, M. (2007). MR elastography of breast lesions: Understanding the solid/liquid duality can improve the specificity of contrast-enhanced MR mammography. Magn. Reson. Med. 58, 1135–1144. doi:10.1002/mrm.21404
Sinkus, R., Tanter, M., Catheline, S., Lorenzen, J., Kuhl, C., Sondermann, E., et al. (2005). Imaging anisotropic and viscous properties of breast tissue by magnetic resonance-elastography. Magn. Reson. Med. 53, 372–387. doi:10.1002/mrm.20355
Smith, C. L., Kilic, O., Schiapparelli, P., Guerrero-Cazares, H., Kim, D. H., Sedora-Roman, N. I., et al. (2016). Migration phenotype of brain-cancer cells predicts patient outcomes. Cell Rep. 15, 2616–2624. doi:10.1016/j.celrep.2016.05.042
Sobradillo, D., Hernandez-Morales, M., Ubierna, D., Moyer, M. P., Nunez, L., and Villalobos, C. (2014). A reciprocal shift in transient receptor potential channel 1 (TRPC1) and stromal interaction molecule 2 (STIM2) contributes to Ca2+ remodeling and cancer hallmarks in colorectal carcinoma cells. J. Biol. Chem. 289, 28765–28782. doi:10.1074/jbc.M114.581678
Solis, A. G., Bielecki, P., Steach, H. R., Sharma, L., Harman, C. C. D., Yun, S., et al. (2019). Mechanosensation of cyclical force by PIEZO1 is essential for innate immunity. Nature 573, 69–74. doi:10.1038/s41586-019-1485-8
Stapor, P. C., Wang, W., Murfee, W. L., and Khismatullin, D. B. (2011). The distribution of fluid shear stresses in capillary sprouts. Cardiovasc. Eng. Technol. 2, 124–136. doi:10.1007/s13239-011-0041-y
Stoletov, K., Montel, V., Lester, R. D., Gonias, S. L., and Klemke, R. (2007). High-resolution imaging of the dynamic tumor cell vascular interface in transparent zebrafish. Proc. Natl. Acad. Sci. U. S. A. 104, 17406–17411. doi:10.1073/pnas.0703446104
Stowers, R. S., Shcherbina, A., Israeli, J., Gruber, J. J., Chang, J., Nam, S., et al. (2019). Matrix stiffness induces a tumorigenic phenotype in mammary epithelium through changes in chromatin accessibility. Nat. Biomed. Eng. 3, 1009–1019. doi:10.1038/s41551-019-0420-5
Stroka, K. M., Gu, Z., Sun, S. X., and Konstantopoulos, K. (2014). Bioengineering paradigms for cell migration in confined microenvironments. Curr. Opin. Cell Biol. 30, 41–50. doi:10.1016/j.ceb.2014.06.001
Stroka, K. M., Jiang, H., Chen, S. H., Tong, Z., Wirtz, D., Sun, S. X., et al. (2014). Water permeation drives tumor cell migration in confined microenvironments. Cell 157, 611–623. doi:10.1016/j.cell.2014.02.052
Stutchbury, B., Atherton, P., Tsang, R., Wang, D.-Y., and Ballestrem, C. (2017). Distinct focal adhesion protein modules control different aspects of mechanotransduction. J. Cell Sci. 130, 1612–1624. doi:10.1242/jcs.195362
Stylianopoulos, T., Martin, J. D., Chauhan, V. P., Jain, S. R., Diop-Frimpong, B., Bardeesy, N., et al. (2012). Causes, consequences, and remedies for growth-induced solid stress in murine and human tumors. Proc. Natl. Acad. Sci. U. S. A. 109, 15101–15108. doi:10.1073/pnas.1213353109
Su, F., Wang, B. F., Zhang, T., Hou, X. M., and Feng, M. H. (2019). TRPM7 deficiency suppresses cell proliferation, migration, and invasion in human colorectal cancer via regulation of epithelial-mesenchymal transition. Cancer Biomark. 26, 451–460. doi:10.3233/CBM-190666
Sun, C., Jain, R. K., and Munn, L. L. (2007). Non-uniform plasma leakage affects local hematocrit and blood flow: Implications for inflammation and tumor perfusion. Ann. Biomed. Eng. 35, 2121–2129. doi:10.1007/s10439-007-9377-8
Sun, J., Lu, F., He, H., Shen, J., Messina, J., Mathew, R., et al. (2014). STIM1- and Orai1-mediated Ca(2+) oscillation orchestrates invadopodium formation and melanoma invasion. J. Cell Biol. 207, 535–548. doi:10.1083/jcb.201407082
Sun, Y., Li, M., Liu, G., Zhang, X., Zhi, L., Zhao, J., et al. (2020). The function of Piezo1 in colon cancer metastasis and its potential regulatory mechanism. J. Cancer Res. Clin. Oncol. 146, 1139–1152. doi:10.1007/s00432-020-03179-w
Sun, Y., Sukumaran, P., Varma, A., Derry, S., Sahmoun, A. E., and Singh, B. B. (2014). Cholesterol-induced activation of TRPM7 regulates cell proliferation, migration, and viability of human prostate cells. Biochim. Biophys. Acta 1843, 1839–1850. doi:10.1016/j.bbamcr.2014.04.019
Sun, Y., Ye, C., Tian, W., Ye, W., Gao, Y. Y., Feng, Y. D., et al. (2021). TRPC1 promotes the genesis and progression of colorectal cancer via activating CaM-mediated PI3K/AKT signaling axis. Oncogenesis 10, 67. doi:10.1038/s41389-021-00356-5
Swartz, M. A., and Fleury, M. E. (2007). Interstitial flow and its effects in soft tissues. Annu. Rev. Biomed. Eng. 9, 229–256. doi:10.1146/annurev.bioeng.9.060906.151850
Swartz, M. A., Kristensen, C. A., Melder, R. J., Roberge, S., Calautti, E., Fukumura, D., et al. (1999). Cells shed from tumours show reduced clonogenicity, resistance to apoptosis, and in vivo tumorigenicity. Br. J. Cancer 81, 756–759. doi:10.1038/sj.bjc.6690760
Szczot, M., Nickolls, A. R., Lam, R. M., and Chesler, A. T. (2021). The form and function of PIEZO2. Annu. Rev. Biochem. 90, 507–534. doi:10.1146/annurev-biochem-081720-023244
Taipale, J., and Keski-Oja, J. (1997). Growth factors in the extracellular matrix. FASEB J. 11, 51–59. doi:10.1096/fasebj.11.1.9034166
Tan, C. H., and McNaughton, P. A. (2016). The TRPM2 ion channel is required for sensitivity to warmth. Nature 536, 460–463. doi:10.1038/nature19074
Tao, J., and Sun, S. X. (2015). Active biochemical regulation of cell volume and a simple model of cell tension response. Biophys. J. 109, 1541–1550. doi:10.1016/j.bpj.2015.08.025
Thomas, S. N., Zhu, F., Schnaar, R. L., Alves, C. S., and Konstantopoulos, K. (2008). Carcinoembryonic antigen and CD44 variant isoforms cooperate to mediate colon carcinoma cell adhesion to E- and L-selectin in shear flow. J. Biol. Chem. 283, 15647–15655. doi:10.1074/jbc.M800543200
Trebak, M., Lemonnier, L., Smyth, J. T., Vazquez, G., and Putney, J. W. (2007). Phospholipase C-coupled receptors and activation of TRPC channels. Handb. Exp. Pharmacol. 1, 593–614. doi:10.1007/978-3-540-34891-7_35
Tse, J. M., Cheng, G., Tyrrell, J. A., Wilcox-Adelman, S. A., Boucher, Y., Jain, R. K., et al. (2012). Mechanical compression drives cancer cells toward invasive phenotype. Proc. Natl. Acad. Sci. U. S. A. 109, 911–916. doi:10.1073/pnas.1118910109
Turitto, V. T. (1982). Blood viscosity, mass transport, and thrombogenesis. Prog. Hemost. Thromb. 6, 139–177.
Venturini, V., Pezzano, F., Catala Castro, F., Hakkinen, H. M., Jimenez-Delgado, S., Colomer-Rosell, M., et al. (2020). The nucleus measures shape changes for cellular proprioception to control dynamic cell behavior. Science 370, eaba2644. doi:10.1126/science.aba2644
Voets, T., Prenen, J., Vriens, J., Watanabe, H., Janssens, A., Wissenbach, U., et al. (2002). Molecular determinants of permeation through the cation channel TRPV4. J. Biol. Chem. 277, 33704–33710. doi:10.1074/jbc.M204828200
Walcott, S., Kim, D. H., Wirtz, D., and Sun, S. X. (2011). Nucleation and decay initiation are the stiffness-sensitive phases of focal adhesion maturation. Biophys. J. 101, 2919–2928. doi:10.1016/j.bpj.2011.11.010
Walcott, S., and Sun, S. X. (2010). A mechanical model of actin stress fiber formation and substrate elasticity sensing in adherent cells. Proc. Natl. Acad. Sci. U. S. A. 107, 7757–7762. doi:10.1073/pnas.0912739107
Wang, J., Jiang, J., Yang, X., Zhou, G., Wang, L., and Xiao, B. (2022). Tethering Piezo channels to the actin cytoskeleton for mechanogating via the cadherin-beta-catenin mechanotransduction complex. Cell Rep. 38, 110342. doi:10.1016/j.celrep.2022.110342
Wang, J., Liao, Q. J., Zhang, Y., Zhou, H., Luo, C. H., Tang, J., et al. (2014). TRPM7 is required for ovarian cancer cell growth, migration and invasion. Biochem. Biophys. Res. Commun. 454, 547–553. doi:10.1016/j.bbrc.2014.10.118
Wang, L., Fu, T. M., Zhou, Y., Xia, S., Greka, A., and Wu, H. (2018). Structures and gating mechanism of human TRPM2. Science 362, eaav4809. doi:10.1126/science.aav4809
Wang, T., Chen, Z., Zhu, Y., Pan, Q., Liu, Y., Qi, X., et al. (2015). Inhibition of transient receptor potential channel 5 reverses 5-Fluorouracil resistance in human colorectal cancer cells. J. Biol. Chem. 290, 448–456. doi:10.1074/jbc.M114.590364
Wang, T., Ning, K., Lu, T. X., and Hua, D. (2017). Elevated expression of TrpC5 and GLUT1 is associated with chemoresistance in colorectal cancer. Oncol. Rep. 37, 1059–1065. doi:10.3892/or.2016.5322
Wang, W., Lollis, E. M., Bordeleau, F., and Reinhart-King, C. A. (2019). Matrix stiffness regulates vascular integrity through focal adhesion kinase activity. FASEB J. 33, 1199–1208. doi:10.1096/fj.201800841R
Wang, X., Cheng, G., Miao, Y., Qiu, F., Bai, L., Gao, Z., et al. (2021). Piezo type mechanosensitive ion channel component 1 facilitates gastric cancer omentum metastasis. J. Cell. Mol. Med. 25, 2238–2253. doi:10.1111/jcmm.16217
Wang, Y., He, J., Jiang, H., Zhang, Q., Yang, H., Xu, X., et al. (2018). Nicotine enhances store-operated calcium entry by upregulating HIF-1α and SOCC components in non-small cell lung cancer cells. Oncol. Rep. 40, 2097–2104. doi:10.3892/or.2018.6580
Wei, C., Wang, X., Chen, M., Ouyang, K., Song, L. S., and Cheng, H. (2009). Calcium flickers steer cell migration. Nature 457, 901–905. doi:10.1038/nature07577
Wei, S. C., Fattet, L., Tsai, J. H., Guo, Y., Pai, V. H., Majeski, H. E., et al. (2015). Matrix stiffness drives epithelial-mesenchymal transition and tumour metastasis through a TWIST1-G3BP2 mechanotransduction pathway. Nat. Cell Biol. 17, 678–688. doi:10.1038/ncb3157
Weigelin, B., Bakker, G. J., and Friedl, P. (2012). Intravital third harmonic generation microscopy of collective melanoma cell invasion: Principles of interface guidance and microvesicle dynamics. Intravital 1, 32–43. doi:10.4161/intv.21223
Wells, R. E., and Merrill, E. W. (1961). Shear rate dependence of the viscosity of whole bllod and plasma. Science 133, 763–764. doi:10.1126/science.133.3455.763
Wendt, M. K., and Schiemann, W. P. (2009). Therapeutic targeting of the focal adhesion complex prevents oncogenic TGF-beta signaling and metastasis. Breast Cancer Res. 11, R68. doi:10.1186/bcr2360
Winkler, J., Abisoye-Ogunniyan, A., Metcalf, K. J., and Werb, Z. (2020). Concepts of extracellular matrix remodelling in tumour progression and metastasis. Nat. Commun. 11, 5120. doi:10.1038/s41467-020-18794-x
Wirtz, D., Konstantopoulos, K., and Searson, P. C. (2011). The physics of cancer: The role of physical interactions and mechanical forces in metastasis. Nat. Rev. Cancer 11, 512–522. doi:10.1038/nrc3080
Wisdom, K. M., Adebowale, K., Chang, J., Lee, J. Y., Nam, S., Desai, R., et al. (2018). Matrix mechanical plasticity regulates cancer cell migration through confining microenvironments. Nat. Commun. 9, 4144. doi:10.1038/s41467-018-06641-z
Wisniewski, E. O., Mistriotis, P., Bera, K., Law, R. A., Zhang, J., Nikolic, M., et al. (2020). Dorsoventral polarity directs cell responses to migration track geometries. Sci. Adv. 6, eaba6505. doi:10.1126/sciadv.aba6505
Wolf, K., Alexander, S., Schacht, V., Coussens, L. M., von Andrian, U. H., van Rheenen, J., et al. (2009). Collagen-based cell migration models in vitro and in vivo. Semin. Cell Dev. Biol. 20, 931–941. doi:10.1016/j.semcdb.2009.08.005
Wolf, K., Te Lindert, M., Krause, M., Alexander, S., Te Riet, J., Willis, A. L., et al. (2013). Physical limits of cell migration: Control by ECM space and nuclear deformation and tuning by proteolysis and traction force. J. Cell Biol. 201, 1069–1084. doi:10.1083/jcb.201210152
Wolfenson, H., Lavelin, I., and Geiger, B. (2013). Dynamic regulation of the structure and functions of integrin adhesions. Dev. Cell 24, 447–458. doi:10.1016/j.devcel.2013.02.012
Wong, A. D., and Searson, P. C. (2017). Mitosis-mediated intravasation in a tissue-engineered tumor-microvessel platform. Cancer Res. 77, 6453–6461. doi:10.1158/0008-5472.CAN-16-3279
Woo, S. H., Lukacs, V., de Nooij, J. C., Zaytseva, D., Criddle, C. R., Francisco, A., et al. (2015). Piezo2 is the principal mechanotransduction channel for proprioception. Nat. Neurosci. 18, 1756–1762. doi:10.1038/nn.4162
Wozniak, M. A., Desai, R., Solski, P. A., Der, C. J., and Keely, P. J. (2003). ROCK-generated contractility regulates breast epithelial cell differentiation in response to the physical properties of a three-dimensional collagen matrix. J. Cell Biol. 163, 583–595. doi:10.1083/jcb.200305010
Yamada, K. M., and Sixt, M. (2019). Mechanisms of 3D cell migration. Nat. Rev. Mol. Cell Biol. 20, 738–752. doi:10.1038/s41580-019-0172-9
Yamaguchi, H., and Condeelis, J. (2007). Regulation of the actin cytoskeleton in cancer cell migration and invasion. Biochim. Biophys. Acta 1773, 642–652. doi:10.1016/j.bbamcr.2006.07.001
Yang, F., Cai, J., Zhan, H., Situ, J., Li, W., Mao, Y., et al. (2020). Suppression of TRPM7 inhibited hypoxia-induced migration and invasion of androgen-independent prostate cancer cells by enhancing RACK1-mediated degradation of HIF-1. Oxid. Med. Cell. Longev. 2020, 6724810. doi:10.1155/2020/6724810
Yang, H., Liu, C., Zhou, R. M., Yao, J., Li, X. M., Shen, Y., et al. (2016). Piezo2 protein: A novel regulator of tumor angiogenesis and hyperpermeability. Oncotarget 7, 44630–44643. doi:10.18632/oncotarget.10134
Yang, W., Wu, P. F., Ma, J. X., Liao, M. J., Xu, L. S., and Yi, L. (2020). TRPV4 activates the Cdc42/N-wasp pathway to promote glioblastoma invasion by altering cellular protrusions. Sci. Rep. 10, 14151. doi:10.1038/s41598-020-70822-4
Yang, X. N., Lu, Y. P., Liu, J. J., Huang, J. K., Liu, Y. P., Xiao, C. X., et al. (2014). Piezo1 is as a novel trefoil factor family 1 binding protein that promotes gastric cancer cell mobility in vitro. Dig. Dis. Sci. 59, 1428–1435. doi:10.1007/s10620-014-3044-3
Yankaskas, C. L., Bera, K., Stoletov, K., Serra, S. A., Carrillo-Garcia, J., Tuntithavornwat, S., et al. (2021). The fluid shear stress sensor TRPM7 regulates tumor cell intravasation. Sci. Adv. 7, eabh3457. doi:10.1126/sciadv.abh3457
Yao, W., Shen, Z., and Ding, G. (2013). Simulation of interstitial fluid flow in ligaments: Comparison among Stokes, brinkman and Darcy models. Int. J. Biol. Sci. 9, 1050–1056. doi:10.7150/ijbs.7242
Yellin, F., Li, Y., Sreenivasan, V. K. A., Farrell, B., Johny, M. B., Yue, D., et al. (2018). Electromechanics and volume dynamics in nonexcitable tissue cells. Biophys. J. 114, 2231–2242. doi:10.1016/j.bpj.2018.03.033
Yin, J., Kong, X., and Lin, W. (2021). Noninvasive cancer diagnosis in vivo based on a viscosity-activated near-infrared fluorescent probe. Anal. Chem. 93, 2072–2081. doi:10.1021/acs.analchem.0c03803
Yoshigi, M., Hoffman, L. M., Jensen, C. C., Yost, H. J., and Beckerle, M. C. (2005). Mechanical force mobilizes zyxin from focal adhesions to actin filaments and regulates cytoskeletal reinforcement. J. Cell Biol. 171, 209–215. doi:10.1083/jcb.200505018
Yu, Y., Wu, X., Liu, S., Zhao, H., Li, B., Feng, X., et al. (2021). Piezo1 regulates migration and invasion of breast cancer cells via modulating cell mechanobiological properties. Acta Biochim. Biophys. Sin. 53, 10–18. doi:10.1093/abbs/gmaa112
Yu, Z.-Y., Gong, H., Kesteven, S., Guo, Y., Wu, J., Li, J., et al. (2022). Piezo1 and TRPM4 work in tandem to initiate cardiac hypertrophic signalling in response to pressure overload. Biophysical J. 121, 493a. doi:10.1016/j.bpj.2021.11.321
Yuan, J. P., Zeng, W., Huang, G. N., Worley, P. F., and Muallem, S. (2007). STIM1 heteromultimerizes TRPC channels to determine their function as store-operated channels. Nat. Cell Biol. 9, 636–645. doi:10.1038/ncb1590
Zaidel-Bar, R., Ballestrem, C., Kam, Z., and Geiger, B. (2003). Early molecular events in the assembly of matrix adhesions at the leading edge of migrating cells. J. Cell Sci. 116, 4605–4613. doi:10.1242/jcs.00792
Zaidel-Bar, R., Itzkovitz, S., Ma'ayan, A., Iyengar, R., and Geiger, B. (2007). Functional atlas of the integrin adhesome. Nat. Cell Biol. 9, 858–867. doi:10.1038/ncb0807-858
Zaidel-Bar, R., Milo, R., Kam, Z., and Geiger, B. (2007). A paxillin tyrosine phosphorylation switch regulates the assembly and form of cell-matrix adhesions. J. Cell Sci. 120, 137–148. doi:10.1242/jcs.03314
Zhang, J., Zhou, Y., Huang, T., Wu, F., Liu, L., Kwan, J. S. H., et al. (2018). PIEZO1 functions as a potential oncogene by promoting cell proliferation and migration in gastric carcinogenesis. Mol. Carcinog. 57, 1144–1155. doi:10.1002/mc.22831
Zhang, L. Y., Zhang, Y. Q., Zeng, Y. Z., Zhu, J. L., Chen, H., Wei, X. L., et al. (2020). TRPC1 inhibits the proliferation and migration of estrogen receptor-positive Breast cancer and gives a better prognosis by inhibiting the PI3K/AKT pathway. Breast Cancer Res. Treat. 182, 21–33. doi:10.1007/s10549-020-05673-8
Zhang, P., Liu, X., Li, H., Chen, Z., Yao, X., Jin, J., et al. (2017). TRPC5-induced autophagy promotes drug resistance in breast carcinoma via CaMKKβ/AMPKα/mTOR pathway. Sci. Rep. 7, 3158. doi:10.1038/s41598-017-03230-w
Zhang, P., Xu, J., Zhang, H., and Liu, X. Y. (2021). Identification of TRPV4 as a novel target in invasiveness of colorectal cancer. BMC Cancer 21, 1264. doi:10.1186/s12885-021-08970-7
Zhao, Q., Zhou, H., Chi, S., Wang, Y., Wang, J., Geng, J., et al. (2018). Structure and mechanogating mechanism of the Piezo1 channel. Nature 554, 487–492. doi:10.1038/nature25743
Zhao, R., Afthinos, A., Zhu, T., Mistriotis, P., Li, Y., Serra, S. A., et al. (2019). Cell sensing and decision-making in confinement: The role of TRPM7 in a tug of war between hydraulic pressure and cross-sectional area. Sci. Adv. 5, eaaw7243. doi:10.1126/sciadv.aaw7243
Zhao, R., Cui, S., Ge, Z., Zhang, Y., Bera, K., Zhu, L., et al. (2021). Hydraulic resistance induces cell phenotypic transition in confinement. Sci. Adv. 7, eabg4934. doi:10.1126/sciadv.abg4934
Zheng, J. (2013). Molecular mechanism of TRP channels. Compr. Physiol. 3, 221–242. doi:10.1002/cphy.c120001
Zhou, W., Liu, X., van Wijnbergen, J. W. M., Yuan, L., Liu, Y., Zhang, C., et al. (2020). Identification of PIEZO1 as a potential prognostic marker in gliomas. Sci. Rep. 10, 16121. doi:10.1038/s41598-020-72886-8
Zubcevic, L., Herzik, M. A., Chung, B. C., Liu, Z., Lander, G. C., and Lee, S. Y. (2016). Cryo-electron microscopy structure of the TRPV2 ion channel. Nat. Struct. Mol. Biol. 23, 180–186. doi:10.1038/nsmb.3159
Keywords: mechanosensitive (MS) ion channel, cancer metastasis, physical forces, cell migration, cell cytoskeleton
Citation: Bera K, Kiepas A, Zhang Y, Sun SX and Konstantopoulos K (2022) The interplay between physical cues and mechanosensitive ion channels in cancer metastasis. Front. Cell Dev. Biol. 10:954099. doi: 10.3389/fcell.2022.954099
Received: 27 May 2022; Accepted: 08 August 2022;
Published: 07 September 2022.
Edited by:
Dimitra Gkika, INSERM UMR1277 Hétérogénéité, Plasticité et Résistance aux Thérapies Anticancéreuses (CANTHER), FranceCopyright © 2022 Bera, Kiepas, Zhang, Sun and Konstantopoulos. This is an open-access article distributed under the terms of the Creative Commons Attribution License (CC BY). The use, distribution or reproduction in other forums is permitted, provided the original author(s) and the copyright owner(s) are credited and that the original publication in this journal is cited, in accordance with accepted academic practice. No use, distribution or reproduction is permitted which does not comply with these terms.
*Correspondence: Alexander Kiepas, YWtpZXBhczFAamh1LmVkdQ==; Konstantinos Konstantopoulos, a29uc3RhbnRAamh1LmVkdQ==
†These authors have contributed equally to this work and share first authorship
Disclaimer: All claims expressed in this article are solely those of the authors and do not necessarily represent those of their affiliated organizations, or those of the publisher, the editors and the reviewers. Any product that may be evaluated in this article or claim that may be made by its manufacturer is not guaranteed or endorsed by the publisher.
Research integrity at Frontiers
Learn more about the work of our research integrity team to safeguard the quality of each article we publish.