- Department of Neurology, Boston University School of Medicine, Boston, MA, United States
Skeletal muscle is essential to physical activity and energy metabolism. Maintaining intact functions of skeletal muscle is crucial to health and wellbeing. Evolutionarily, skeletal muscle has developed a remarkable capacity to maintain homeostasis and to regenerate after injury, which indispensably relies on the resident muscle stem cells, satellite cells. Satellite cells are largely quiescent in the homeostatic steady state. They are activated in response to muscle injury. Activated satellite cells proliferate and differentiate into myoblasts. Myoblasts fuse to form myotubes which further grow and differentiate into mature myofibers. This process is tightly regulated by muscle microenvironment that consists of multiple cellular and molecular components, including macrophages. Present in both homeostatic and injured muscles, macrophages contain heterogeneous functional subtypes that play diverse roles in maintaining homeostasis and promoting injury repair. The spatial-temporal presence of different functional subtypes of macrophages and their interactions with myogenic cells are vital to the proper regeneration of skeletal muscle after injury. However, this well-coordinated process is often disrupted in a chronic muscle disease, such as muscular dystrophy, leading to asynchronous activation and differentiation of satellite cells and aberrant muscle regeneration. Understanding the precise cellular and molecular processes regulating interactions between macrophages and myogenic cells is critical to the development of therapeutic manipulation of macrophages to promote injury repair. Here, we review the current knowledge of the many roles played by macrophages in the regulation of myogenic cells in homeostatic, regenerating, and dystrophic skeletal muscles.
1 Introduction
Skeletal muscle injury can be acute or chronic depending on etiologies. Acute injury is commonly caused by trauma, ischemia, freeze, or myotoxin exposure. Chronic injury is usually associated with a disease process, such as muscular dystrophy, inflammatory myopathy, or infectious myopathy. Skeletal muscle injury repair is a complex process, consisting of muscle inflammation, regeneration, revascularization, and extracellular matrix (ECM) remodeling (Bentzinger et al., 2013a; Yin et al., 2013; Dumont et al., 2015a). As a tissue that constantly encounters mechanical stretch, skeletal muscle suffers a high rate of micro-injury in the normal steady state. To cope with this challenge, skeletal muscle has evolutionarily developed a remarkable regenerative capacity, which involves activation, proliferation, differentiation, and growth of myogenic cells (Bentzinger et al., 2013a; Yin et al., 2013; Dumont et al., 2015a). Acutely injured skeletal muscle repairs well if the injury is not large or repeated. The injury repair process, however, requires an adequate inflammatory response which is initiated by transient neutrophil infiltration followed shortly by massive macrophage infiltration. Infiltrating macrophages not only phagocytose damaged tissue debris but also produce cytokines and growth factors to interact with myogenic, fibrogenic, and angiogenic cells to support skeletal muscle injury repair (Tidball and Villalta, 2010; Tidball, 2011; Muñoz-Cánoves and Serrano, 2015; Dort et al., 2019). While essential to the acute skeletal muscle injury repair, infiltrating macrophages contribute to muscle pathology in chronic injury associated with muscular dystrophy. With such diverse roles, macrophages have become a central topic of research in the field of skeletal muscle injury repair.
Macrophages are heterogeneous and multi-functional cells that are critical to tissue functions in both steady state and disease state. Although classically identified as innate immune cells, functioning in the activation and resolution of tissue inflammation, it is now clear that macrophages play important roles in a much wider range of biological processes, such as tissue remodeling during organogenesis, tissue homeostasis, injury repair, and immune response to pathogens (Hashimoto et al., 2013; Wynn et al., 2013; Kierdorf et al., 2015; Ginhoux et al., 2016; Wynn and Vannella, 2016). In the normal steady state, resident macrophages maintain tissue homeostasis via surveillance of local tissue environment and response to physiological and pathological changes. In a disease state, macrophages exert pro-inflammatory, anti-inflammatory, pro-fibrotic, or pro-regenerative functions depending on the tissue environment and macrophage origin. They are critically involved in a variety of disease processes, such as chronic tissue inflammation, tumor growth and metastasis, and tissue fibrosis (McNelis and Olefsky, 2014; Noy and Pollard, 2014; Ginhoux et al., 2016; Wynn and Vannella, 2016; Vannella and Wynn, 2017). Such diverse capabilities of macrophages are rooted in their diverse origins and high plasticity when responding to environmental changes. In this article, we will review the multiple origins and many roles of macrophages in skeletal muscle homeostasis, regeneration following acute injury, and degeneration, regeneration, and fibrosis in muscular dystrophy.
2 Skeletal Muscle-Resident Macrophages Arise From Multiple Origins and Appear Active in Maintaining Tissue Homeostasis and Promoting Muscle Growth and Regeneration
Tissue macrophages consist of two classes: resident macrophages and infiltrating macrophages. In adult mammals, while resident macrophages are present in all tissues, infiltrating macrophages are found in a diseased tissue, such as injured tissue. Unlike infiltrating macrophages which are all derived from blood monocytes originating from bone marrow hematopoietic stem cells (HSCs), tissue-resident macrophages arise from multiple origins during embryonic and adult hematopoiesis.
Macrophages reside in homeostatic tissues including skeletal muscle (Wang et al., 2020). Most of the tissue-resident macrophage populations in the steady state are established prenatally by two embryonic progenitors: primitive yolk sac macrophages and fetal liver monocytes (aka fetal monocytes) (Ginhoux et al., 2010; Schulz et al., 2012; Hashimoto et al., 2013; Ginhoux and Jung, 2014; Gomez Perdiguero et al., 2015; Hoeffel et al., 2015; Hoeffel and Ginhoux, 2015; Mass et al., 2016; Hoeffel and Ginhoux, 2018). Primitive yolk sac macrophages originate from early erythro-myeloid progenitors (EMPs) which emerge in yolk sac at embryonic day 7 (E7) in mice. They differentiate into primitive macrophages and migrate to embryonic tissues from E9.5. Fetal monocytes mainly arise from late EMPs that emerge in yolk sac at E8.5. They migrate into fetal liver and differentiate into fetal monocytes at E12.5. Fetal monocytes seed all other embryonic tissues except for brain (Hoeffel et al., 2015). Within individual tissues, primitive macrophages and fetal monocytes are induced by local tissue environment to differentiate into tissue-specific resident macrophages, expressing tissue-specific transcription factors and displaying tissue-specific functions. They persist into adulthood through proliferative self-renewal. Pre-hematopoietic stem cells (HSCs) first appear at aorta-gonad-mesonephros (AGM) at E9.5 and then seed fetal liver around E10.5, where they differentiate into mature HSCs (Hoeffel and Ginhoux, 2015). HSCs also contribute to fetal monocytes at the later stage of embryonic development (Gomez Perdiguero et al., 2015; Wang et al., 2020). Mature HSCs migrate into nascent bone marrow at the late embryonic stage and give rise to blood monocytes (adult monocytes) after birth. Adult monocytes are recruited by many tissues to replenish resident macrophages (Tamoutounour et al., 2013; Bain et al., 2014; Epelman et al., 2014; Bain et al., 2016; Scott et al., 2016), including by skeletal muscle (Wang et al., 2020) but not the brain (Hoeffel et al., 2015). Depending on the origin and tissue environment, resident macrophages display high plasticity in their function and activation.
Resident macrophages in the steady-state skeletal muscle have been identified and studied in mice (Wang et al., 2020). CD45+F4/80+CD64+ resident macrophages are found in interstitial tissues of skeletal muscle, expressing a low level of Ly6C and a high level of CD163 and CD206. They arise from both embryonic hematopoietic progenitors, including yolk sac primitive macrophages and fetal liver monocytes, and adult bone marrow HSCs. The transcriptome of resident macrophages in skeletal muscle is highly distinctive from that in other tissues. Skeletal muscle-resident macrophages express a specific set of transcription factor genes, including Maf, Mef2c, and Tcf4 (Wang et al., 2020). They appear active in maintaining tissue homeostasis and promoting muscle growth and regeneration based on their differentially expressed genes. Functionally diverse subsets correlating to their origins are identified within skeletal muscle-resident macrophages. While the CCR2+MHCIIhiLyve1low macrophages are mainly derived from adult blood monocytes and are more active antigen-presenting cells, the CCR2-MHCIIlowLyve1hi macrophages arise from both embryonic and adult progenitors and are more active phagocytes. Both subsets may play roles in maintaining skeletal muscle homeostasis. Interestingly, skeletal muscle-resident macrophages also have muscle type-specific features, as they express a higher level of stress response genes in respiratory muscle than in limb muscle (Wang et al., 2020).
Functional study of skeletal muscle-resident macrophages is limited. A recent study showed that in multiple tissues, including skeletal muscle, tissue-resident macrophages can rapidly cloak tissue microlesions through sensing damage-associated alarmins. The cloaking prevents chemoattractant signaling-mediated neutrophil swarms and subsequent inflammatory tissue damage. As a result, tissue microlesions heal without inflammation (Uderhardt et al., 2019). In the steady-state skeletal muscle, the cloaking by resident macrophages prevents complete death of myofibers with microlesions and maintains their structural integrity (Uderhardt et al., 2019). The findings support the important homeostatic function of resident macrophages in the steady-state skeletal muscle. The interactions between resident macrophages and myogenic cells in the steady-state and the roles of resident macrophages in skeletal muscle development and postnatal growth remain largely unknown and need to be determined.
3 Infiltrating Macrophages are Essential to Skeletal Muscle Regeneration Following Acute Injury
3.1 Skeletal Muscle Regenerates Well Following Acute Injury
Skeletal muscle has an excellent regenerative capacity. Unless caused by a repeated or a large volumetric muscle loss injury (Dadgar et al., 2014; Corona et al., 2015), acutely injured skeletal muscle regenerates well. Acute skeletal muscle injury can be caused by many etiologies, including intense exercise, trauma, ischemia, freeze, and myotoxin exposure. To study acute skeletal muscle injury repair, several animal models have been developed and used, including those with acute injuries caused by mechanical damage, intramuscular toxin or heavy metal salt injection, muscle freeze, and muscle ischemia induced by artery ligation. The technical merits of different injury models are reviewed by Baghdadi and Tajbakhsh (2018). Studies with these models have revealed a similar muscle repair process with small differences. Figure 1A illustrates the time course of murine skeletal muscle repair following acute injury induced by barium chloride. Massive muscle fiber necrosis is observed at day 1 post-injury, accompanied by inflammatory cell infiltration. The inflammation peaks at day 3. Small, central-nucleated myoblasts and multi-nucleated myotubes emerge around day 5. At day 7, infiltrating inflammatory cells drop significantly in number, and necrotic fibers are largely replaced by regenerating fibers. Extracellular matrix deposition is increased (transient fibrosis) at this stage to provide structural support to the injury repair. Inflammation and transient fibrosis resolve by day 14, and muscle fibers reach the size comparable to un-injured muscle.
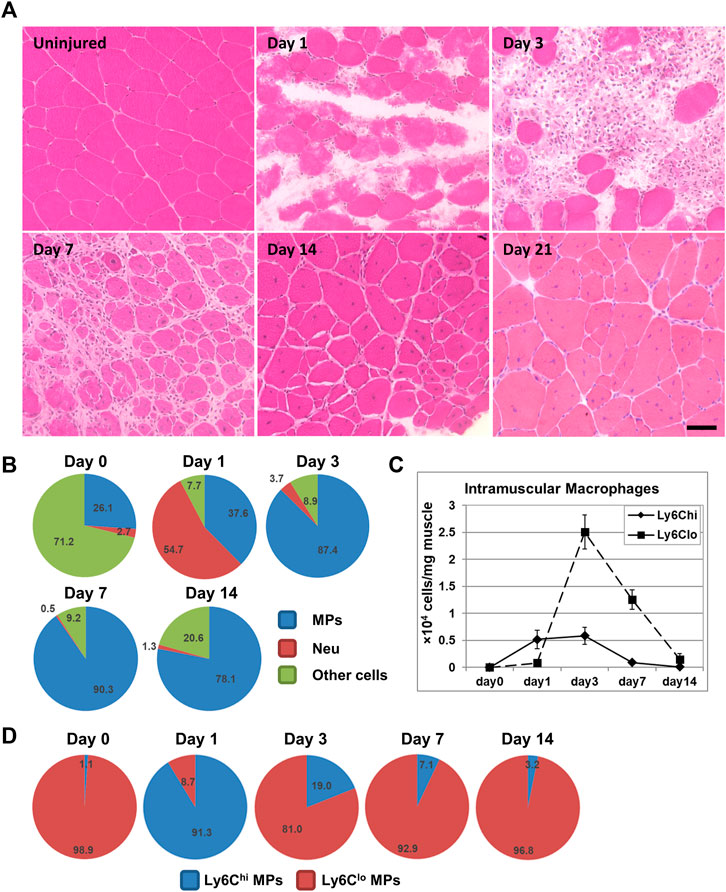
FIGURE 1. (A) H&E staining illustrating the normal acute muscle injury repair process following intramuscular BaCl2 injection into mouse quadriceps muscle. Bar = 50 μm. (B) Pie chart showing percentages of macrophages (MPs), neutrophils (Neu), and other cells among CD45+ cells in BaCl2-injured quadriceps muscle at different days post injury. (C) Line graph showing densities of intramuscular Ly6Chi and Ly6Clo macrophages in BaCl2-injured quadriceps at different days post injury. (D) Pie charts showing percentages of Ly6Chi and Ly6Clo subpopulations among macrophages in BaCl2-injured quadriceps at different days post injury.
3.2 Myogenic Cell Homeostasis, Activation, and Differentiation During Skeletal Muscle Regeneration
Regeneration of injured skeletal muscle relies on muscle-resident stem cells, satellite cells, as depletion of satellite cells completely abolishes skeletal muscle regeneration (Murphy et al., 2011). In the homeostatic healthy muscle, satellite cells are quiescent and in close association with myofibers, residing between sarcolemma of muscle fibers and basal lamina that surrounds fibers (Mauro, 1961). Quiescent satellite cells are characterized by the expression of paired box 7 (Pax7) (Seale et al., 2000) and forkhead box (FOXO) transcription factors (García-Prat et al., 2020). Upon injury, satellite cells undergo activation and differentiation to regenerate muscle. This process is regulated by a distinct set of transcription factors termed myogenic regulatory factors (MRFs) which include MYOD, MYF5, MRF4 (MYF6), and myogenin (MYOG) (Segalés et al., 2015; Hernández-Hernández et al., 2017; Massenet et al., 2021). Myf5 and Myod1 are transcribed in quiescent satellite cells, but the subsequent protein translation is prevented by posttranscriptional regulation (Beauchamp et al., 2000; Crist et al., 2012; van Velthoven et al., 2017; Yue et al., 2020). Upon muscle injury, the injured muscle microenvironment releases signals to activate satellite cells to allow protein translation of Myf5 and Myod1 mRNAs (Crist et al., 2012; Yue et al., 2020) and loss of FOXO expression (García-Prat et al., 2020). Activated satellite cells can generate both Pax7+MYF5+ and Pax7+MYF5− cells through apical-basal asymmetric division, of which the Pax7+MYF5+ cells exhibit precocious differentiation, while the Pax7+Myf5− cells contribute to the satellite cell reservoir (Kuang et al., 2007). Activated Pax7+MYF5+MYOD+ satellite cells, which are also called myoblasts, expand through symmetric division. Terminal differentiation of myoblasts, symbolled by upregulation of MYOG and MYF6/MRF4 and loss of PAX7 and MYF5 expression, generates myocytes and ultimately myofibers through fusion (Bentzinger et al., 2013a; Yin et al., 2013; Dumont et al., 2015a; Hernández-Hernández et al., 2017; Massenet et al., 2021).
The dynamic balance among quiescence, activation, and differentiation of satellite cells is vital to the maintenance of stem cell pool in healthy muscle and the successful regeneration in injured muscle. It is tightly regulated during skeletal muscle regeneration following injury (Dumont et al., 2015b). Infiltrating macrophages contribute to the signals required for satellite cell activation and differentiation.
3.3 Macrophages are the Predominant Inflammatory Cells in Acutely Injured Muscle, and They Differentiate From Circulation-Derived Inflammatory Monocytes
Although multiple immune cells are involved in the inflammatory response induced by acute muscle injury, neutrophils and macrophages are the predominant ones (Figure 1B) (Tidball and Villalta, 2010; Tidball, 2011; Yin et al., 2013). Neutrophils are the earliest inflammatory cells that infiltrate injured muscle. The neutrophil infiltration starts within 2 h post-injury, and the number peaks around 24 h post-injury (Tidball and Villalta, 2010). Neutrophils phagocytose damaged muscle debris and release reactive oxygen species (ROS), protease, and inflammatory cytokines to promote inflammation (Tidball, 2011; Wang et al., 2018). Depleting neutrophils during acute skeletal muscle injury impairs phagocytosis of necrotic tissue and delays regeneration (Teixeira et al., 2003; Toumi et al., 2006). Ly6Chi monocyte/macrophage infiltration starts shortly after the neutrophil infiltration, and the number peaks 1–3 days after injury (Figures 1B–D) (Arnold et al., 2007). The total number of macrophages and the number of Ly6Clo macrophages peak at day 3 post-injury (Figures 1B–D) (Arnold et al., 2007; Wang et al., 2018). Inflammation resolution is complete by day 14 (Arnold et al., 2007; Wang et al., 2018).
Infiltrating macrophages are derived from blood inflammatory monocytes (Arnold et al., 2007; Shi and Pamer, 2011). Blood monocytes consist of two principal subsets: Ly6ChiCCR2+CX3CR1lo and Ly6CloCCR2−CX3CR1hi cells in mice, distinguished by the expression of cell surface markers Ly6C, C-C motif chemokine receptor 2 (CCR2), and CX3C chemokine receptor 1 (CX3CR1) (Geissmann et al., 2003). Tissue recruitment of cells from blood circulation requires the chemokine system, with tissue cells expressing chemokine ligands to chemoattract blood cells that express corresponding chemokine receptors. The Ly6ChiCCR2+CX3CR1lo cells are inflammatory monocytes, which rapidly enter tissues upon injury or infection and differentiate into inflammatory macrophages (Geissmann et al., 2003). It has been shown that spleen is also a reservoir of the Ly6Chi inflammatory monocytes which can be deployed into inflamed tissues, including skeletal muscle (Swirski et al., 2009; Rizzo et al., 2020). The recruitment of Ly6Chi inflammatory monocytes by acutely injured muscle requires CCR2 expression by monocytes and CC chemokine ligand 2 (CCL2) expression by both muscle resident cells and infiltrating macrophages (Sun et al., 2009; Lu et al., 2011a; Lu et al., 2011b). CCL2 is the main ligand of CCR2. Deficiency in CCR2 or CCL2 diminishes macrophage infiltration in several acute skeletal muscle injury models (Arnold et al., 2007; Contreras-Shannon et al., 2007; Sun et al., 2009; Lu et al., 2011a; Lu et al., 2011b). The Ly6CloCCR2−CX3CR1hi cells are patrolling monocytes, which patrol the vascular endothelial surface and may enter tissue via CX3CR1/CX3CL1 to contribute to tissue-resident macrophages (Auffray et al., 2007). It has been shown that Ly6Clo monocytes are not recruited by acutely injured muscle during injury repair (Varga et al., 2013). However, while most of the intramuscular macrophages at day 1 are Ly6Chi, the majority at day 3 are Ly6Clo (Figures 1C,D). The accumulation of Ly6Clo macrophages is resulted from Ly6Chi-to-Ly6Clo switch, as Ly6Chi inflammatory macrophages switch into Ly6Clo macrophages after phagocytosing necrotic muscle debris (Arnold et al., 2007). Ly6C is not expressed in human cells. The CD14hiCD16low and CD14lowCD16hi monocytes in humans correspond to the Ly6Chi and Ly6Clo monocytes in mice, respectively (Ziegler-Heitbrock et al., 2010).
3.4 Macrophages Undergo Phenotype Changes With Time to Support Acute Skeletal Muscle Injury Repair
Macrophages have been historically classified into M1 (classically activated) and M2 (alternatively activated) subsets, mainly based on in vitro studies and in vivo studies of parasite infections (Martinez and Gordon, 2014). M1 and M2 macrophages are different in their activation stimuli, cell surface markers, arginine metabolism, and cytokine production profiles (Martinez et al., 2006; Martinez and Gordon, 2014). While M1 macrophages, activated by IFN-γ ± LPS, are pro-inflammatory, M2 macrophages, activated by Il-4 ± IL-13, can be anti-inflammatory, pro-regenerative, and/or pro-fibrotic. Following this bipolar macrophage activation model, the Ly6Chi and Ly6Clo macrophages in injured skeletal muscle have once been considered M1 and M2 macrophages, respectively, based on the findings that the Ly6Chi macrophages express more pro-inflammatory genes, while the Ly6Clo macrophages express more anti-inflammatory genes (Arnold et al., 2007; Ruffell et al., 2009; Perdiguero et al., 2011; Wang et al., 2014). However, there has been growing evidence demonstrating that the M1/M2 paradigm of macrophage activation is over-simplistic and cannot mimic complex in vivo settings, in which the macrophage activation status can be influenced by many other co-existing cell types. In vivo, M1 and M2 stimuli often co-exist, macrophages can display mixed M1/M2 phenotypes, and they may not expand clonally to maintain phenotype (Martinez and Gordon, 2014; Murray et al., 2014; Ransohoff, 2016). The phenotype of in vivo macrophages may be M1-like or M2-like but not strictly M1 or M2. Gene expression profiles of macrophages in acutely injured skeletal muscle indicate that the Ly6Chi macrophages at an early stage of inflammation (day 1–2 post-injury) are not strictly M1, and the Ly6Clo macrophages at the late stage of inflammation are not strictly M2 (Novak et al., 2014; Varga et al., 2016a; Wang et al., 2018). One study showed that although the Ly6Chi-to-Ly6Clo switch of macrophages was accompanied by downregulation of M1 genes (tnfa, il1b, and il6) and upregulation of M2 genes (cd206, tgfb1, and igf1), the Ly6Chi macrophages at day 1 co-expressed a high level of both M1 (tnfa, il1b, and il6) and M2 genes (arginase 1, ym1, and il10), and the Ly6Chi and Ly6Clo macrophages at day 3 expressed a similar level of many M1 and M2 genes (Wang et al., 2018). A more profound transcriptome analysis revealed four macrophage activation statuses, specifying the sequential changes of macrophages during acute muscle injury repair: 1) infiltrating Ly6Chi macrophages expressing acute-phase proteins and exhibiting an inflammatory profile; 2) metabolic changes in macrophages characterized by decreased glycolysis and increased tricarboxylic acid cycle/oxidative pathways; 3) Ly6Clo macrophages actively proliferating; 4) restorative Ly6Clo macrophages featuring secretion of molecules for intercellular communication (Varga et al., 2016a). Both studies suggest that the changes in macrophage phenotype in injured muscle is driven by the changes in muscle microenvironment with time (Varga et al., 2016a; Wang et al., 2018). The macrophages at the early stage of inflammation display a more “pro-inflammatory” phenotype, while the macrophages at the later stage of inflammation display a more “anti-inflammatory” and “pro-regenerative” phenotype. This is further supported by the changes in macrophage-produced lipid mediators from pro-inflammatory lipids at the early-stage to pro-resolving lipids at the late-stage (Scher and Pillinger, 2005; Giannakis et al., 2019).
3.5 Macrophages Play Essential Roles in Supporting Acute Skeletal Muscle Injury Repair
An adequate inflammatory response predominated by macrophage infiltration is essential to acute skeletal muscle injury repair. The absence of macrophage infiltration or disruption of macrophage functions leads to profound impairment of muscle regeneration and development of muscle fibrosis (Arnold et al., 2007; Contreras-Shannon et al., 2007; Sun et al., 2009; Lu et al., 2011a; Lu et al., 2011b; Wang et al., 2014; Dort et al., 2019). Macrophages regulate not only inflammation but also the other aspects of injury repair, including muscle regeneration, ECM remodeling, and angiogenesis.
3.5.1 Inflammation
Macrophages are both effectors and regulators of the inflammatory response after acute skeletal muscle injury. Ly6Chi monocytes/macrophages massively infiltrate into injured muscle shortly after an injury occurs, and they produce a relatively high level of pro-inflammatory cytokines such as TNF-α (Arnold et al., 2007; Shi and Pamer, 2011; Wang et al., 2018). Pro-inflammatory cytokines can promote inflammation by increasing tissue damage and amplifying inflammatory cell recruitment (Arnold et al., 2007; Shi and Pamer, 2011). Pro-inflammatory macrophages are also known as active phagocytes, as they phagocytose and clear damaged tissue debris for muscle injury repair (Tidball and Villalta, 2010; Tidball, 2011). When macrophage infiltration is diminished due to CCR2 deficiency, the clearance of necrotic muscle fibers is protracted (Lu et al., 2011b). The necrotic fibers eventually disappear more likely by necrotic fiber autolysis than by macrophage phagocytosis in this setting. Phagocytosis of dead cells has been shown essential to the pro-to anti-inflammatory phenotype switch in macrophages (Arnold et al., 2007; Johann et al., 2007; Xiao et al., 2008; Mounier et al., 2013; Zhang et al., 2019; Saclier et al., 2020). The anti-inflammatory macrophages contribute, in part, to inflammation resolution. They express a variety of anti-inflammatory cytokines, such as IL-4, TGF-β1, and IGF-1 (Serhan and Savill, 2005; Arnold et al., 2007; Ruffell et al., 2009; Perdiguero et al., 2011; Mounier et al., 2013; Wang et al., 2014; Wynn and Vannella, 2016; Vannella and Wynn, 2017; Wang et al., 2018), as well as pro-resolving lipids (Scher and Pillinger, 2005; Giannakis et al., 2019). These anti-inflammatory molecules counteract pro-inflammatory signals, reduce ROS production, block neutrophil recruitment, and promote neutrophil apoptosis and clearance by macrophages (Serhan and Savill, 2005). Prolonged presence of neutrophils was observed in injured muscle when macrophages were depleted (Dumont and Frenette, 2010). Therefore, macrophages play essential roles in both initiation and resolution of inflammation during acute skeletal muscle injury repair.
3.5.2 Muscle Regeneration
Macrophages interact with myogenic cells to regulated muscle regeneration following acute injury. In a study using in vitro engineered model of rat adult skeletal muscle repair, incorporation of macrophages was required to stimulate satellite cell-mediated myogenesis (Juhas et al., 2018). Implantation of macrophages within engineered tissues in a mouse dorsal window-chamber model augmented muscle regeneration and contractile function (Juhas et al., 2018). Meanwhile, macrophages of different activation status have been shown to differentially regulate myogenic cell activation, proliferation, and differentiation. While pro-inflammatory macrophages promote myoblast proliferation but inhibit myoblast fusion and differentiation, anti-inflammatory macrophages inhibit myoblast proliferation but promote myotube formation and differentiation (Arnold et al., 2007; Bencze et al., 2012; Saclier et al., 2013; Hsieh et al., 2018). The differential regulation is mediated, at least in part, by paracrine cytokines and growth factors released by macrophages. Fibronectin, an ECM component that is highly expressed by day 1 pro-inflammatory macrophages (Wang et al., 2018), can activate satellite cells (Bentzinger et al., 2013b). Pro-inflammatory macrophages also produce a high level of IL-6 (Zhang et al., 2013), TNF-α (Li, 2002), PGE2 (Ho et al., 2017), and A Disintegrin-Like and Metalloproteinase with Thrombospondin Type 1 Motif (ADAMTS1) (Du et al., 2017) that can stimulate satellite cell proliferation. On the other hand, molecules that are highly expressed by day 3 anti-inflammatory macrophages, including IL-4 (Horsley et al., 2003), IGF-1 (Dumont and Frenette, 2010; Lu et al., 2011b; Tonkin et al., 2015), and GDF-3 (Varga et al., 2016b), can stimulate myoblast differentiation and myofiber growth. The increase in glutamine synthesis in macrophages during pro-to anti-inflammatory phenotype transition can also boost satellite cell activation and muscle regeneration (Shang et al., 2020). Therefore, the pro-to anti-inflammatory macrophage phenotype switch is likely important for the sequential activation, proliferation, differentiation, and growth of myogenic elements to complete muscle regeneration for injury repair. The critical role of the spatiotemporal presence of pro- and anti-inflammatory macrophages in acutely injured muscle has been corroborated by in vivo studies showing that targeting signaling molecules that regulate the pro-to anti-inflammatory macrophage phenotype switch, including IGF-1 (Tonkin et al., 2015), Meteorin-like (Metrnl) (Baht et al., 2020), AMP-activated protein kinase-1 (AMPKα1) (Mounier et al., 2013; McArthur et al., 2020), Nuclear Factor IX (Nfix) (Saclier et al., 2020), CCAAT/enhancer binding protein-β (C/EBPβ) (Ruffell et al., 2009), and peroxisome proliferator-activated receptor-γ (PPARγ) (Varga et al., 2016b), impaired myofiber growth without affecting clearance of necrotic tissue. Direct physical contact of macrophages with myogenic cells also appears important for myogenesis, as in vitro co-culture experiments showed that physical contact of macrophages with myogenic cells prevented apoptosis of myogenic cells (Chazaud et al., 2003; Sonnet et al., 2006). Both paracrine and direct physical contact require close proximity between macrophages and myogenic cells, which has been observed in vivo (Saclier et al., 2013; Ceafalan et al., 2018). In regenerating muscle, pro-inflammatory macrophages are in close proximity to proliferating satellite cells, while anti-inflammatory macrophages are close to the area containing differentiated myoblasts (Saclier et al., 2013).
3.5.3 Extracellular Matrix remodeling
A well-regulated ECM remodeling is important to providing structural support for skeletal muscle injury repair. The ECM components, collagen 6a (Col6a) (Urciuolo et al., 2013) and fibronectin (Bentzinger et al., 2013b), were also important to satellite cell activation. Fibro/adipogenic progenitors (FAPs), the effector cells of ECM remodeling, not only produce ECM proteins but also support satellite cell activation and differentiation to facilitate muscle regeneration (Joe et al., 2010; Uezumi et al., 2010; Murphy et al., 2011; Uezumi et al., 2014). When the regenerative process is impaired; however, FAPs drive fibro-fatty replacement and fail to support satellite cell activation (Uezumi et al., 2014). Therefore, the FAP activity and ECM remodeling must be properly regulated. Macrophages regulate the accumulation and activation of FAPs during acute skeletal muscle injury repair. Pro-inflammatory macrophages limit the accumulation of FAPs by secreting TNF-α to induce FAP apoptosis (Lemos et al., 2015). Anti-inflammatory macrophages, on the other hand, can promote activation of fibrogenic cells by producing a high level of pro-fibrotic factors, including TGF-β1, PDGFα, and PDGFβ (Wang et al., 2016). Anti-inflammatory macrophages, therefore, may contribute to the transient fibrosis during muscle injury repair. The importance of macrophage regulation of FAP activity and ECM remodeling is supported by the findings that depleting macrophages or blocking macrophage recruitment leads to muscle fibrosis (Lu et al., 2011a; Lemos et al., 2015).
3.5.4 Angiogenesis
Revascularization to restore blood supply is vital for tissue injury repair. The exact regulatory roles played by macrophage functional subtypes in this process remain elusive (Rahat et al., 2014). There are mixed reports of the roles of infiltrating macrophages in angiogenesis or vascular remodeling during acute skeletal muscle injury repair, which could be due to the different injury models used in these studies. One study showed that the diminished macrophage infiltration caused by CCR2 deficiency delayed VEGF production and angiogenesis during the repair of cardiotoxin-injured muscle (Ochoa et al., 2007). But another study using the BaCl2 injury model showed that blocking macrophage recruitment did not affect endomysial capillary density (Lu et al., 2011b). It has also been shown in the cardiotoxin injury model that macrophage depletion caused a significant endothelial-to-mesenchymal transition of the endothelial-derived progenitors, compromised blood vessel formation, and increased collagen deposition (Zordan et al., 2014). In addition, restorative macrophages have been shown to stimulate interaction between angiogenic cells and myogenic cells via oncostain M production to couple angiogenesis and myogenesis during muscle regeneration (Latroche et al., 2017). The roles of macrophages in angiogenesis during acute skeletal muscle injury repair need to be further elucidated.
In summary, adequate macrophage infiltration is essential to acute skeletal muscle injury repair. Infiltrating macrophages actively interact with myogenic cells to regulate their activation, proliferation, differentiation, and growth for proper muscle regeneration. The sequential presence of pro- and anti-inflammatory macrophages is crucial to the tightly-regulated, satellite cell-mediated regenerative process. Insufficient macrophage infiltration or disrupted pro-to anti-inflammatory macrophage transition impairs muscle regeneration.
4 Macrophages Play Pleiotropic Roles in Chronically Injured Skeletal Muscles in Muscular Dystrophy
Chronically injured skeletal muscle features chronic inflammation, with continuous Ly6Chi monocyte and macrophage infiltration and Ly6Chi-to-Ly6Clo switch. This creates an asynchronous regenerative environment that interrupts the spatiotemporal presence of pro- and anti-inflammatory macrophages, leading to dysregulated muscle regeneration. This hypothesis is supported by a study utilizing a simplified model of repeated muscle injury (Dadgar et al., 2014). In this study, skeletal muscle injury was induced twice, separated by 4 or 10 days. Concurrent accumulation of both pro- and anti-inflammation macrophages was observed in injured muscle, along with the development of persistent inflammation and fibrosis and the impairment of muscle regeneration. The asynchronous microenvironment in chronically injured muscle is much more complex than this simplified model, which may drive macrophages to play very different roles. The most studied muscle disease caused by chronic injury is Duchene Muscular Dystrophy (DMD). DMD is a genetic disease caused by a defective dystrophin gene on the X chromosome, which leads to muscle membrane instability, muscle necrosis, secondary muscle inflammation and fibrosis, muscle weakness, and premature death (Hoffman et al., 1987; Emery, 1993). The most commonly used animal model for studying DMD is mdx mice.
4.1 Chronic Inflammation in Mdx Mice Is Predominated by Macrophage Infiltration
Mdx or mdx5cv mice display a mild phenotype compared to DMD patients. But they do show persistent inflammation and progressive fibrosis in the diaphragm (Stedman et al., 1991; Goldspink et al., 1994; Hartel et al., 2001; Beastrom et al., 2011). Muscle inflammation in the mdx mice starts around age 3 weeks, persists into 2–3 months, and then subsides spontaneously in the limb muscles but the not the diaphragm. Progressive fibrosis mainly occurs in the diaphragm, which impairs respiratory function, resembling dystrophic muscles in human DMD patients (Stedman et al., 1991; Dupont-Versteegden and McCarter, 1992; Zhou et al., 2006; Huang et al., 2011). Muscle inflammation in mdx mice is also predominated by macrophage infiltration (Zhou et al., 2006) (Figure 2).
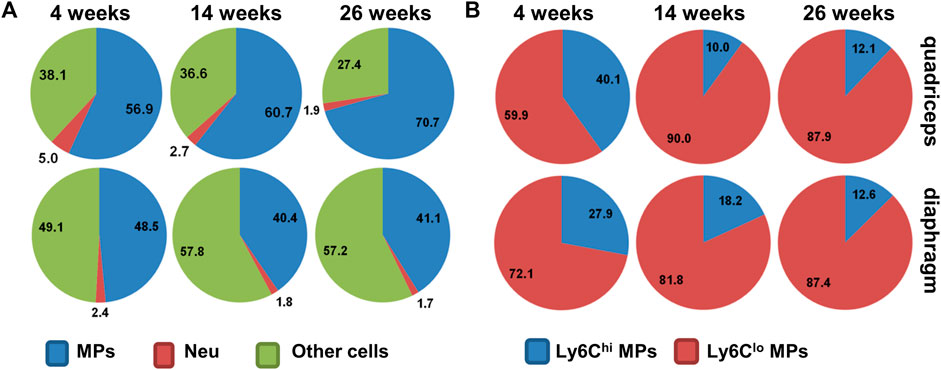
FIGURE 2. (A) Pie chart showing percentages of macrophages (MPs), neutrophils (Neu), and other cells among intramuscular CD45+ cells in mdx5cv mice at different ages. (B) Pie chart showing percentages of Ly6Chi and Ly6Clo subpopulations among intramuscular macrophages in mdx5cv mice at different ages.
4.2 Macrophages Play Pleiotropic Roles in Dystrophic Muscles of Mdx Mice
Like in acute injury, muscle recruitment of Ly6Chi inflammatory monocytes/macrophages in mdx is also mediated by CCR2, and intramuscular Ly6Chi-to-Ly6Clo macrophage switch also occurs (Mojumdar et al., 2014; Zhao et al., 2017). Correspondingly, macrophages in the mdx leg muscles are more pro-inflammatory at 4 weeks while more pro-regenerative at 12 weeks (Villalta et al., 2009). Macrophages in mdx muscles appear pathogenic in general, as blocking Ly6Chi inflammatory monocyte/macrophage infiltration via removal of splenic source of Ly6Chi monocytes by splenectomy or via genetic ablation or pharmacological inhibition of CCR2 reduced muscle damage and fibrosis and improved muscle function in both leg and diaphragm muscles before 3 months of age (Mojumdar et al., 2014; Zhao et al., 2017; Rizzo et al., 2020). Similarly, toll-like receptor 4 (TRL4) deficiency in mdx mice, which also reduced macrophage infiltration at 6 and 12 weeks of age, decreased muscle fibrosis (Giordano et al., 2015). Macrophages may influence muscle inflammation, necrosis, regeneration, and fibrosis by producing pro-inflammatory, anti-inflammatory, pro-regenerative, and pro-fibrotic cytokines and growth factors, such as iNOS, TNF-α, IL-1β, IL-6, IL-10, IGF-1, TGF-β, and osteopontin (Zhou et al., 2006; Villalta et al., 2009; Tidball and Villalta, 2010; Villalta et al., 2011; Lemos et al., 2015; Capote et al., 2016; Ji et al., 2020).
In mdx limb muscles, the pro-inflammatory macrophages appear to contribute to muscle damage, as depleting macrophages by an F4/80 neutralizing antibody reduced leg muscle necrosis at 4 weeks of age (Wehling et al., 2001). The anti-inflammatory macrophages appear more pro-regenerative than pro-degenerative, which may contribute to the remarkable spontaneous improvement of limb muscle pathology after 3 months of age (Zhou et al., 2006; Beastrom et al., 2011). This hypothesis is supported by a study showing that depletion of macrophages locally in mdx leg muscles from 10 to 12 weeks of age exacerbated dystrophic changes with decreased myofiber formation and increased fat deposition and fibrosis (Madaro et al., 2019). Macrophage depletion impaired proliferation and differentiation of myogenic progenitors and caused adipogenic conversion of satellite cells (Madaro et al., 2019). The Ly6Chi macrophages in the mdx leg muscles at 8–10 weeks of age, however, contribute to fibrosis (Juban et al., 2018). They produce latent TGF-β1 due to a high level of latent-TGF-β-binding protein 4 (LTBP4) synthesis, and the latent TGF-β1 is subsequently activated by FAP-derived TGF-β-activating enzymes to promote fibrosis (Juban et al., 2018). Activation of AMPK, which promotes the pro-inflammatory to anti-inflammatory phenotype switch of macrophages (Mounier et al., 2013; McArthur et al., 2020), downregulated LTBP4 expression and TGF-β1 production, leading to decreased fibrosis and improved muscle function (Juban et al., 2018).
Macrophages in the mdx diaphragm might be different from those in the mdx limb muscles, as the diaphragm undergoes persistent inflammation and progressive fibrosis, while the limb muscles do not (Stedman et al., 1991; Dupont-Versteegden and McCarter, 1992; Zhou et al., 2006; Huang et al., 2011). One study showed that intramuscular fibrocytes, a subset of collagen-producing Ly6Clo macrophages, were more pro-inflammatory and pro-fibrotic in the mdx5cv diaphragm than in the mdx5cv quadriceps at 14 weeks of age (Wang et al., 2016). But the comprehensive comparison of macrophages between diaphragm and limb muscles is still lacking.
4.3 Blocking Monocyte/Macrophage Recruitment by Targeting CCR2 Signaling Provides Transient Benefits in Mdx Mice, Potentiating a Role of Skeletal Muscle-Resident Macrophages
Since macrophages contribute to muscular dystrophy pathology, blocking their recruitment becomes a potential strategy to ameliorate the disease. Blocking macrophage infiltration by genetic ablation or pharmacological inhibition of CCR2 indeed improved muscle pathology and function in the mdx diaphragm at early stages (6 and 12 weeks) (Mojumdar et al., 2014). However, the beneficial effects are transient and lost at late stages. CCR2 deficiency in mdx5cv mice reduced diaphragm muscle damage and fibrosis and improved diaphragm muscle regeneration and function at 14 weeks but not 6 months (Zhao et al., 2017). Analysis of macrophage recruitment revealed that CCR2 deficiency diminished intramuscular Ly6Chi macrophages at all stages, but reduced Ly6Clo macrophages only at the early stages (4 and 9 weeks) but not the late stages (14 weeks or 6 months) (Zhao et al., 2017). The recovery of Ly6Clo macrophages and the concurrent progression of diaphragm muscle dystrophy at the later stages suggest that the Ly6Clo macrophages are also pathogenic. Therefore, targeting Ly6Chi macrophages alone is not sufficient, and the Ly6Clo macrophages must also be targeted simultaneously. To achieve this, one question must be answered first: where do these Ly6Clo macrophages originate from in the absence of CCR2?
In the absence of CCR2, intramuscular Ly6Clo macrophages may originate from Ly6Clo monocyte recruitment and/or resident macrophage expansion. Since the chemotaxis of Ly6Clo blood monocytes requires CX3CR1 (Charo and Ransohoff, 2006), and the development of Ly6Clo blood monocyte requires Nur77 (Hanna et al., 2011), additional targeting of these two molecules in the mdx/Ccr2−/−mice would help answer whether Ly6Clo blood monocytes are recruited in the mdx/Ccr2−/− mice, and whether this recruitment contributes to the recovery of Ly6Clo macrophages. Lineage tracing would help determine whether resident macrophage expansion occurs, and whether resident macrophages also regulate muscular dystrophy.
5 Resident Macrophages May Play Active Roles in Skeletal Muscle Injury Repair
Following the identification of skeletal muscle-resident macrophages in the steady state, one question arises as to what roles these cells play during injury repair. One early study in rats suggests that muscle resident macrophages do not phagocytose degenerating muscle fibers; they instead act as sentinels activated by damage-associated molecular patterns (DAMPs) during injury to facilitate the recruitment of circulating leukocytes (McLennan, 1993). However, this is contradicted by a recent study showing that resident macrophages in skeletal muscle sense and cloak tissue microlesions to prevent excessive tissue damage under physiological and disease conditions (Uderhardt et al., 2019). Early depletion of resident macrophages in mdx mice leads to premature onset of muscle disease featured by increased neutrophil infiltrates (Uderhardt et al., 2019), suggesting that resident macrophages may protect muscle from inflammatory damage at the early stage in this chronic disease model. The contradictory roles might be partially attributed to the existence of different subsets of muscle resident macrophages. Studies of resident macrophages in heart seem to support this hypothesis: following myocardial injury, the CCR2+ subset promotes recruitment of neutrophils (Li et al., 2016) and monocytes (Bajpai et al., 2019), while the CCR2- subset inhibits monocytes recruitment (Bajpai et al., 2019). A subset-specific analysis is yet to be done in acutely and chronically injured skeletal muscle.
Following acute skeletal muscle injury, macrophage infiltration is required for the injury repair, suggesting that resident macrophages fail to compensate for the pro-regenerative functions of the inflammatory macrophages (Lu et al., 2011b). During chronic injury in the mdx5cv/Ccr2−/− mice, however, macrophage-mediated inflammation is only compromised at the early stages but recovers at the later stages (Zhao et al., 2017), suggesting that resident macrophage expansion may occur with time to compensate for the lack of recruited inflammatory macrophages. Whether this is true requires further studies. Despite of the scattered evidence, the roles of resident macrophages and their functional subsets in both acute and chronic skeletal muscle injuries are largely unexplored. Studies to specifically target resident macrophages are to be conducted to understand the functions of these cells in muscle inflammation, fibrosis, and regeneration.
6 Conclusion
Recent years have seen increasing evidence that macrophages actively regulate diverse physiological and pathological processes. This extraordinary ability relies on their high plasticity in response to tissue environmental changes. Following acute skeletal muscle injury, infiltrating macrophages respond to the changes in intramuscular microenvironment, switching their functional phenotypes in a spatiotemporal manner to promote injury repair. The sequential presence of differentially activated macrophages has been proven vital for well-coordinated, satellite cell-mediated muscle regeneration. In a chronic disease such as DMD, the spatiotemporal activation of macrophages is disrupted, and some macrophages become detrimental, resulting in aberrant muscle regeneration and contributing to the disease progression. Macrophage manipulation, either by blocking their accumulation or by modulating their function, will be likely beneficial to the treatment. To this end, further understanding of the origins, functions, and activation mechanisms of macrophages is required. Advanced technologies, such as lineage tracing and single-cell transcriptome analysis, will continue to help generate valuable insights. It is worth noting that most of the knowledge reviewed here is from animal studies. The knowledge of macrophage contribution to the homeostasis and injury repair in human skeletal muscle is still lacking. Nevertheless, the knowledge gained from animal studies is instructive, which may facilitate future studies of human skeletal muscle. This line of research may eventually develop macrophage-based therapies to promote skeletal muscle injury repair.
Author Contributions
XW: drafted the manuscript and prepared the figures LZ: drafted the manuscript.
Funding
The work was supported by the National Institute of Arthritis And Musculoskeletal and Skin Diseases of the National Institutes of Health under Award Number 1R01AR074428 (LZ). The content is solely the responsibility of the authors and does not necessarily represent the official views of the National Institutes of Health.
Conflict of Interest
The authors declare that the research was conducted in the absence of any commercial or financial relationships that could be construed as a potential conflict of interest.
Publisher’s Note
All claims expressed in this article are solely those of the authors and do not necessarily represent those of their affiliated organizations, or those of the publisher, the editors and the reviewers. Any product that may be evaluated in this article, or claim that may be made by its manufacturer, is not guaranteed or endorsed by the publisher.
References
Arnold, L., Henry, A., Poron, F., Baba-Amer, Y., van Rooijen, N., Plonquet, A., et al. (2007). Inflammatory Monocytes Recruited after Skeletal Muscle Injury Switch into Antiinflammatory Macrophages to Support Myogenesis. J. Exp. Med. 204 (5), 1057–1069. doi:10.1084/jem.20070075
Auffray, C., Fogg, D., Garfa, M., Elain, G., Join-Lambert, O., Kayal, S., et al. (2007). Monitoring of Blood Vessels and Tissues by a Population of Monocytes with Patrolling Behavior. Science 317 (5838), 666–670. doi:10.1126/science.1142883
Baghdadi, M. B., and Tajbakhsh, S. (2018). Regulation and Phylogeny of Skeletal Muscle Regeneration. Dev. Biol. 433 (2), 200–209. doi:10.1016/j.ydbio.2017.07.026
Baht, G. S., Bareja, A., Lee, D. E., Rao, R. R., Huang, R., Huebner, J. L., et al. (2020). Meteorin-like Facilitates Skeletal Muscle Repair through a Stat3/IGF-1 Mechanism. Nat. Metab. 2 (3), 278–289. doi:10.1038/s42255-020-0184-y
Bain, C. C., Bravo-Blas, A., Scott, C. L., Gomez Perdiguero, E., Geissmann, F., Henri, S., et al. (2014). Constant Replenishment from Circulating Monocytes Maintains the Macrophage Pool in the Intestine of Adult Mice. Nat. Immunol. 15 (10), 929–937. doi:10.1038/ni.2967
Bain, C. C., Hawley, C. A., Garner, H., Scott, C. L., Schridde, A., Steers, N. J., et al. (2016). Long-lived Self-Renewing Bone Marrow-Derived Macrophages Displace Embryo-Derived Cells to Inhabit Adult Serous Cavities. Nat. Commun. 7, ncomms11852. doi:10.1038/ncomms11852
Bajpai, G., Bredemeyer, A., Li, W., Zaitsev, K., Koenig, A. L., Lokshina, I., et al. (2019). Tissue Resident CCR2− and CCR2+ Cardiac Macrophages Differentially Orchestrate Monocyte Recruitment and Fate Specification Following Myocardial Injury. Circ. Res. 124 (2), 263–278. doi:10.1161/circresaha.118.314028
Beastrom, N., Lu, H., Macke, A., Canan, B. D., Johnson, E. K., Penton, C. M., et al. (2011). mdx((5)cv) Mdx Mice Manifest More Severe Muscle Dysfunction and Diaphragm Force Deficits Than Do Mdx Mice. Am. J. pathology 179 (5), 2464–2474. doi:10.1016/j.ajpath.2011.07.009
Beauchamp, J. R., Heslop, L., Yu, D. S. W., Tajbakhsh, S., Kelly, R. G., Wernig, A., et al. (2000). Expression of CD34 and Myf5 Defines the Majority of Quiescent Adult Skeletal Muscle Satellite Cells. J. Cell Biol. 151 (6), 1221–1234. doi:10.1083/jcb.151.6.1221
Bencze, M., Negroni, E., Vallese, D., Yacoub-Youssef, H., Chaouch, S., Wolff, A., et al. (2012). Proinflammatory Macrophages Enhance the Regenerative Capacity of Human Myoblasts by Modifying Their Kinetics of Proliferation and Differentiation. Mol. Ther. 20 (11), 2168–2179. doi:10.1038/mt.2012.189
Bentzinger, C. F., Wang, Y. X., Dumont, N. A., and Rudnicki, M. A. (2013a). Cellular Dynamics in the Muscle Satellite Cell Niche. EMBO Rep. 14 (12), 1062–1072. doi:10.1038/embor.2013.182
Bentzinger, C. F., Wang, Y. X., von Maltzahn, J., Soleimani, V. D., Yin, H., and Rudnicki, M. A. (2013b). Fibronectin Regulates Wnt7a Signaling and Satellite Cell Expansion. Cell Stem Cell 12 (1), 75–87. doi:10.1016/j.stem.2012.09.015
Capote, J., Kramerova, I., Martinez, L., Vetrone, S., Barton, E. R., Sweeney, H. L., et al. (2016). Osteopontin Ablation Ameliorates Muscular Dystrophy by Shifting Macrophages to a Pro-regenerative Phenotype. J. Cell Biol. 213 (2), 275–288. doi:10.1083/jcb.201510086
Ceafalan, L. C., Fertig, T. E., Popescu, A. C., Popescu, B. O., Hinescu, M. E., and Gherghiceanu, M. (2018). Skeletal Muscle Regeneration Involves Macrophage-Myoblast Bonding. Cell Adhesion Migr. 12 (3), 228–235. doi:10.1080/19336918.2017.1346774
Charo, I. F., and Ransohoff, R. M. (2006). The Many Roles of Chemokines and Chemokine Receptors in Inflammation. N. Engl. J. Med. 354 (6), 610–621. doi:10.1056/nejmra052723
Chazaud, B., Sonnet, C., Lafuste, P., Bassez, G., Rimaniol, A.-C., Poron, F., et al. (2003). Satellite Cells Attract Monocytes and Use Macrophages as a Support to Escape Apoptosis and Enhance Muscle Growth. J. Cell Biol. 163 (5), 1133–1143. doi:10.1083/jcb.200212046
Contreras-Shannon, V., Ochoa, O., Reyes-Reyna, S. M., Sun, D., Michalek, J. E., Kuziel, W. A., et al. (2007). Fat Accumulation with Altered Inflammation and Regeneration in Skeletal Muscle of CCR2−/− Mice Following Ischemic Injury. Am. J. Physiology-Cell Physiology 292 (2), C953–C967. doi:10.1152/ajpcell.00154.2006
Corona, B. T., Rivera, J. C., Owens, J. G., Wenke, J. C., and Rathbone, C. R. (2015). Volumetric Muscle Loss Leads to Permanent Disability Following Extremity Trauma. J. Rehabil. Res. Dev. 52 (7), 785–792. doi:10.1682/jrrd.2014.07.0165
Crist, C. G., Montarras, D., and Buckingham, M. (2012). Muscle Satellite Cells Are Primed for Myogenesis but Maintain Quiescence with Sequestration of Myf5 mRNA Targeted by microRNA-31 in mRNP Granules. Cell Stem Cell 11 (1), 118–126. doi:10.1016/j.stem.2012.03.011
Dadgar, S., Wang, Z., Johnston, H., Kesari, A., Nagaraju, K., Chen, Y. W., et al. (2014). Asynchronous Remodeling is a Driver of Failed Regeneration in Duchenne Muscular Dystrophy. J. Cell Biol. 207 (1), 139–158. doi:10.1083/jcb.201402079
Dort, J., Fabre, P., Molina, T., and Dumont, N. A. (2019). Macrophages Are Key Regulators of Stem Cells during Skeletal Muscle Regeneration and Diseases. Stem Cells Int. 2019, 4761427. doi:10.1155/2019/4761427
Du, H., Shih, C.-H., Wosczyna, M. N., Mueller, A. A., Cho, J., Aggarwal, A., et al. (2017). Macrophage-released ADAMTS1 Promotes Muscle Stem Cell Activation. Nat. Commun. 8 (1), 669. doi:10.1038/s41467-017-00522-7
Dumont, N. A., Bentzinger, C. F., Sincennes, M. C., and Rudnicki, M. A. (2015a). Satellite Cells and Skeletal Muscle Regeneration. Compr. Physiol. 5 (3), 1027–1059. doi:10.1002/cphy.c140068
Dumont, N. A., Wang, Y. X., and Rudnicki, M. A. (2015b). Intrinsic and Extrinsic Mechanisms Regulating Satellite Cell Function. Development 142 (9), 1572–1581. doi:10.1242/dev.114223
Dumont, N., and Frenette, J. (2010). Macrophages Protect against Muscle Atrophy and Promote Muscle Recovery In Vivo and In Vitro: a Mechanism Partly Dependent on the Insulin-like Growth Factor-1 Signaling Molecule. Am. J. pathology 176 (5), 2228–2235. doi:10.2353/ajpath.2010.090884
Dupont-Versteegden, E. E., and McCarter, R. J. (1992). Differential Expression of Muscular Dystrophy in Diaphragm versus Hindlimb Muscles of Mdx Mice. Muscle Nerve 15 (10), 1105–1110. doi:10.1002/mus.880151008
Epelman, S., Lavine, K. J., Beaudin, A. E., Sojka, D. K., Carrero, J. A., Calderon, B., et al. (2014). Embryonic and Adult-Derived Resident Cardiac Macrophages Are Maintained through Distinct Mechanisms at Steady State and during Inflammation. Immunity 40 (1), 91–104. doi:10.1016/j.immuni.2013.11.019
García-Prat, L., Perdiguero, E., Alonso-Martín, S., Dell’Orso, S., Ravichandran, S., Brooks, S. R., et al. (2020). FoxO Maintains a Genuine Muscle Stem-Cell Quiescent State until Geriatric Age. Nat. Cell Biol. 22 (11), 1307–1318. doi:10.1038/s41556-020-00593-7
Geissmann, F., Jung, S., and Littman, D. R. (2003). Blood Monocytes Consist of Two Principal Subsets with Distinct Migratory Properties. Immunity 19 (1), 71–82. doi:10.1016/s1074-7613(03)00174-2
Giannakis, N., Sansbury, B. E., Patsalos, A., Hays, T. T., Riley, C. O., Han, X., et al. (2019). Dynamic Changes to Lipid Mediators Support Transitions Among Macrophage Subtypes during Muscle Regeneration. Nat. Immunol. 20 (5), 626–636. doi:10.1038/s41590-019-0356-7
Ginhoux, F., Greter, M., Leboeuf, M., Nandi, S., See, P., Gokhan, S., et al. (2010). Fate Mapping Analysis Reveals that Adult Microglia Derive from Primitive Macrophages. Science 330 (6005), 841–845. doi:10.1126/science.1194637
Ginhoux, F., and Jung, S. (2014). Monocytes and Macrophages: Developmental Pathways and Tissue Homeostasis. Nat. Rev. Immunol. 14 (6), 392–404. doi:10.1038/nri3671
Ginhoux, F., Schultze, J. L., Murray, P. J., Ochando, J., and Biswas, S. K. (2016). New Insights into the Multidimensional Concept of Macrophage Ontogeny, Activation and Function. Nat. Immunol. 17 (1), 34–40. doi:10.1038/ni.3324
Giordano, C., Mojumdar, K., Liang, F., Lemaire, C., Li, T., Richardson, J., et al. (2015). Toll-like Receptor 4 Ablation in Mdx Mice Reveals Innate Immunity as a Therapeutic Target in Duchenne Muscular Dystrophy. Hum. Mol. Genet. 24 (8), 2147–2162. doi:10.1093/hmg/ddu735
Goldspink, G., Fernandes, K., Williams, P. E., and Wells, D. J. (1994). Age-related Changes in Collagen Gene Expression in the Muscles of Mdx Dystrophic and Normal Mice. Neuromuscul. Disord. 4 (3), 183–191. doi:10.1016/0960-8966(94)90019-1
Gomez Perdiguero, E., Klapproth, K., Schulz, C., Busch, K., Azzoni, E., Crozet, L., et al. (2015). Tissue-resident Macrophages Originate from Yolk-Sac-Derived Erythro-Myeloid Progenitors. Nature 518 (7540), 547–551. doi:10.1038/nature13989
Hanna, R. N., Carlin, L. M., Hubbeling, H. G., Nackiewicz, D., Green, A. M., Punt, J. A., et al. (2011). The Transcription Factor NR4A1 (Nur77) Controls Bone Marrow Differentiation and the Survival of Ly6C- Monocytes. Nat. Immunol. 12 (8), 778–785. doi:10.1038/ni.2063
Hartel, J. V., Granchelli, J. A., Hudecki, M. S., Pollina, C. M., and Gosselin, L. E. (2001). Impact of Prednisone on TGF-Beta1 and Collagen in Diaphragm Muscle From mdx Mice. Muscle Nerve 24 (3), 428–432. doi:10.1002/1097-4598(200103)24:3<428::aid-mus1018>3.0.co;2-e
Hashimoto, D., Chow, A., Noizat, C., Teo, P., Beasley, M. B., Leboeuf, M., et al. (2013). Tissue-resident Macrophages Self-Maintain Locally throughout Adult Life with Minimal Contribution from Circulating Monocytes. Immunity 38 (4), 792–804. doi:10.1016/j.immuni.2013.04.004
Hernández-Hernández, J. M., García-González, E. G., Brun, C. E., and Rudnicki, M. A. (2017). The Myogenic Regulatory Factors, Determinants of Muscle Development, Cell Identity and Regeneration. Seminars Cell & Dev. Biol. 72, 10–18. doi:10.1016/j.semcdb.2017.11.010
Ho, A. T. V., Palla, A. R., Blake, M. R., Yucel, N. D., Wang, Y. X., Magnusson, K. E. G., et al. (2017). Prostaglandin E2 Is Essential for Efficacious Skeletal Muscle Stem-Cell Function, Augmenting Regeneration and Strength. Proc. Natl. Acad. Sci. U.S.A. 114 (26), 6675–6684. doi:10.1073/pnas.1705420114
Hoeffel, G., and Ginhoux, F. (2018). Fetal Monocytes and the Origins of Tissue-Resident Macrophages. Cell Immunol. 330, 5–15. doi:10.1016/j.cellimm.2018.01.001
Hoeffel, G., Chen, J., Lavin, Y., Low, D., Almeida, F. F., See, P., et al. (2015). C-Myb+ Erythro-Myeloid Progenitor-Derived Fetal Monocytes Give Rise to Adult Tissue-Resident Macrophages. Immunity 42 (4), 665–678. doi:10.1016/j.immuni.2015.03.011
Hoeffel, G., and Ginhoux, F. (2015). Ontogeny of Tissue-Resident Macrophages. Front. Immunol. 6, 486. doi:10.3389/fimmu.2015.00486
Hoffman, E. P., Brown, R. H., and Kunkel, L. M. (1987). Dystrophin: the Protein Product of the Duchenne Muscular Dystrophy Locus. Cell 51 (6), 919–928. doi:10.1016/0092-8674(87)90579-4
Horsley, V., Jansen, K. M., Mills, S. T., and Pavlath, G. K. (2003). IL-4 Acts as a Myoblast Recruitment Factor during Mammalian Muscle Growth. Cell 113 (4), 483–494. doi:10.1016/s0092-8674(03)00319-2
Hsieh, P.-L., Rybalko, V., Baker, A. B., Suggs, L. J., and Farrar, R. P. (2018). Recruitment and Therapeutic Application of Macrophages in Skeletal Muscles after Hind Limb Ischemia. J. Vasc. Surg. 67 (6), 1908–1920. doi:10.1016/j.jvs.2017.04.070
Huang, P., Cheng, G., Lu, H., Aronica, M., Ransohoff, R. M., and Zhou, L. (2011). Impaired Respiratory Function in Mdx and Mdx/utrn(+/−) Mice. Muscle Nerve 43 (2), 263–267. doi:10.1002/mus.21848
Ji, G., Sun, S., Wu, H., Chen, X., Cui, H., Tang, J., et al. (2020). Effect of AAV9-hIGF-1 on Inflammatory Reaction in Mdx Mice and its Mechanism. Am. J. Transl. Res. 12 (8), 4488–4497.
Joe, A. W. B., Yi, L., Natarajan, A., Le Grand, F., So, L., Wang, J., et al. (2010). Muscle Injury Activates Resident Fibro/adipogenic Progenitors that Facilitate Myogenesis. Nat. Cell Biol. 12 (2), 153–163. doi:10.1038/ncb2015
Johann, A. M., Barra, V., Kuhn, A. M., Weigert, A., Knethen, A., and Brüne, B. (2007). Apoptotic Cells Induce Arginase II in Macrophages, Thereby Attenuating NO Production. FASEB J. 21 (11), 2704–2712. doi:10.1096/fj.06-7815com
Juban, G., Saclier, M., Yacoub-Youssef, H., Kernou, A., Arnold, L., Boisson, C., et al. (2018). AMPK Activation Regulates LTBP4-dependent TGF-Β1 Secretion by Pro-inflammatory Macrophages and Controls Fibrosis in Duchenne Muscular Dystrophy. Cell Rep. 25 (8), 2163–2176. doi:10.1016/j.celrep.2018.10.077
Juhas, M., Abutaleb, N., Wang, J. T., Ye, J., Shaikh, Z., Sriworarat, C., et al. (2018). Incorporation of Macrophages into Engineered Skeletal Muscle Enables Enhanced Muscle Regeneration. Nat. Biomed. Eng. 2 (12), 942–954. doi:10.1038/s41551-018-0290-2
Kierdorf, K., Prinz, M., Geissmann, F., and Gomez Perdiguero, E. (2015). Development and Function of Tissue Resident Macrophages in Mice. Seminars Immunol. 27 (6), 369–378. doi:10.1016/j.smim.2016.03.017
Kuang, S., Kuroda, K., Le Grand, F., and Rudnicki, M. A. (2007). Asymmetric Self-Renewal and Commitment of Satellite Stem Cells in Muscle. Cell 129 (5), 999–1010. doi:10.1016/j.cell.2007.03.044
Latroche, C., Weiss-Gayet, M., Muller, L., Gitiaux, C., Leblanc, P., Liot, S., et al. (2017). Coupling between Myogenesis and Angiogenesis during Skeletal Muscle Regeneration Is Stimulated by Restorative Macrophages. Stem Cell Rep. 9 (6), 2018–2033. doi:10.1016/j.stemcr.2017.10.027
Lemos, D. R., Babaeijandaghi, F., Low, M., Chang, C.-K., Lee, S. T., Fiore, D., et al. (2015). Nilotinib Reduces Muscle Fibrosis in Chronic Muscle Injury by Promoting TNF-Mediated Apoptosis of Fibro/adipogenic Progenitors. Nat. Med. 21 (7), 786–794. doi:10.1038/nm.3869
Li, W., Hsiao, H. M., Higashikubo, R., Saunders, B. T., Bharat, A., Goldstein, D. R., et al. (2016). Heart-resident CCR2(+) Macrophages Promote Neutrophil Extravasation through TLR9/MyD88/CXCL5 Signaling. JCI Insight 1 (12). doi:10.1172/jci.insight.87315
Li, Y. P. (2002). TNF-Alpha Is a Mitogen in Skeletal Muscle. Am. J. Physiol. Cell Physiol. 285, C370–C376. doi:10.1152/ajpcell.00453.2002
Lu, H., Huang, D., Ransohoff, R. M., and Zhou, L. (2011a). Acute Skeletal Muscle Injury: CCL2 Expression by Both Monocytes and Injured Muscle Is Required for Repair. FASEB J. 25 (10), 3344–3355. doi:10.1096/fj.10-178939
Lu, H., Huang, D., Saederup, N., Charo, I. F., Ransohoff, R. M., and Zhou, L. (2011b). Macrophages Recruited via CCR2 Produce Insulin-like Growth Factor-1 to Repair Acute Skeletal Muscle Injury. FASEB J. 25 (1), 358–369. doi:10.1096/fj.10-171579
Madaro, L., Torcinaro, A., De Bardi, M., Contino, F. F., Pelizzola, M., Diaferia, G. R., et al. (2019). Macrophages Fine Tune Satellite Cell Fate in Dystrophic Skeletal Muscle of Mdx Mice. PLoS Genet. 15 (10), e1008408. doi:10.1371/journal.pgen.1008408
Martinez, F. O., and Gordon, S. (2014). The M1 and M2 Paradigm of Macrophage Activation: Time for Reassessment. F1000Prime Rep. 6, 13. doi:10.12703/P6-13
Martinez, F. O., Gordon, S., Locati, M., and Mantovani, A. (2006). Transcriptional Profiling of the Human Monocyte-To-Macrophage Differentiation and Polarization: New Molecules and Patterns of Gene Expression. J. Immunol. 177 (10), 7303–7311. doi:10.4049/jimmunol.177.10.7303
Mass, E., Ballesteros, I., Farlik, M., Halbritter, F., Günther, P., Crozet, L., et al. (2016). Specification of Tissue-Resident Macrophages during Organogenesis. Science 353 (6304). doi:10.1126/science.aaf4238
Massenet, J., Gardner, E., Chazaud, B., and Dilworth, F. J. (2021). Epigenetic Regulation of Satellite Cell Fate during Skeletal Muscle Regeneration. Skelet. Muscle 11 (1), 4. doi:10.1186/s13395-020-00259-w
Mauro, A. (1961). Satellite Cell of Skeletal Muscle Fibers. J. Biophys. Biochem. Cytol. 9, 493–495. doi:10.1083/jcb.9.2.493
McArthur, S., Juban, G., Gobbetti, T., Desgeorges, T., Theret, M., Gondin, J., et al. (2020). Annexin A1 Drives Macrophage Skewing to Accelerate Muscle Regeneration through AMPK Activation. J. Clin. Invest. 130 (3), 1156–1167. doi:10.1172/jci124635
McLennan, I. S. (1993). Resident Macrophages (ED2- and ED3-Positive) Do Not Phagocytose Degenerating Rat Skeletal Muscle Fibres. Cell Tissue Res. 272, 193–196. doi:10.1007/bf00323586
McNelis, J. C., and Olefsky, J. M. (2014). Macrophages, Immunity, and Metabolic Disease. Immunity 41 (1), 36–48. doi:10.1016/j.immuni.2014.05.010
Mojumdar, K., Liang, F., Giordano, C., Lemaire, C., Danialou, G., Okazaki, T., et al. (2014). Inflammatory Monocytes Promote Progression of Duchenne Muscular Dystrophy and Can Be Therapeutically Targeted via CCR 2. EMBO Mol. Med. 6 (11), 1476–1492. doi:10.15252/emmm.201403967
Mounier, R., Théret, M., Arnold, L., Cuvellier, S., Bultot, L., Göransson, O., et al. (2013). AMPKα1 Regulates Macrophage Skewing at the Time of Resolution of Inflammation during Skeletal Muscle Regeneration. Cell Metab. 18 (2), 251–264. doi:10.1016/j.cmet.2013.06.017
Muñoz-Cánoves, P., and Serrano, A. L. (2015). Macrophages Decide between Regeneration and Fibrosis in Muscle. Trends Endocrinol. Metabolism 26 (9), 449–450. doi:10.1016/j.tem.2015.07.005
Murphy, M. M., Lawson, J. A., Mathew, S. J., Hutcheson, D. A., and Kardon, G. (2011). Satellite Cells, Connective Tissue Fibroblasts and Their Interactions Are Crucial for Muscle Regeneration. Development 138 (17), 3625–3637. doi:10.1242/dev.064162
Murray, P. J., Allen, J. E., Biswas, S. K., Fisher, E. A., Gilroy, D. W., Goerdt, S., et al. (2014). Macrophage Activation and Polarization: Nomenclature and Experimental Guidelines. Immunity 41 (1), 14–20. doi:10.1016/j.immuni.2014.06.008
Novak, M. L., Weinheimer-Haus, E. M., and Koh, T. J. (2014). Macrophage Activation and Skeletal Muscle Healing Following Traumatic Injury. J. Pathol. 232 (3), 344–355. doi:10.1002/path.4301
Noy, R., and Pollard, J. W. (2014). Tumor-associated Macrophages: from Mechanisms to Therapy. Immunity 41 (1), 49–61. doi:10.1016/j.immuni.2014.06.010
Ochoa, O., Sun, D., Reyes-Reyna, S. M., Waite, L. L., Michalek, J. E., McManus, L. M., et al. (2007). Delayed Angiogenesis and VEGF Production in CCR2−/− Mice during Impaired Skeletal Muscle Regeneration. Am. J. Physiology-Regulatory, Integr. Comp. Physiology 293 (2), R651–R661. doi:10.1152/ajpregu.00069.2007
Perdiguero, E., Sousa-Victor, P., Ruiz-Bonilla, V., Jardí, M., Caelles, C., Serrano, A. L., et al. (2011). p38/MKP-1-regulated AKT Coordinates Macrophage Transitions and Resolution of Inflammation during Tissue Repair. J. Cell Biol. 195 (2), 307–322. doi:10.1083/jcb.201104053
Rahat, M. A., Hemmerlein, B., and Iragavarapu-Charyulu, V. (2014). The Regulation of Angiogenesis by Tissue Cell-Macrophage Interactions. Front. Physiol. 5, 262. doi:10.3389/fphys.2014.00262
Ransohoff, R. M. (2016). A Polarizing Question: Do M1 and M2 Microglia Exist? Nat. Neurosci. 19 (8), 987–991. doi:10.1038/nn.4338
Rizzo, G., Di Maggio, R., Benedetti, A., Morroni, J., Bouche, M., and Lozanoska-Ochser, B. (2020). Splenic Ly6Chi Monocytes Are Critical Players in Dystrophic Muscle Injury and Repair. JCI Insight 5 (2), e130807. doi:10.1172/jci.insight.130807
Ruffell, D., Mourkioti, F., Gambardella, A., Kirstetter, P., Lopez, R. G., Rosenthal, N., et al. (2009). A CREB-C/EBPβ Cascade Induces M2 Macrophage-specific Gene Expression and Promotes Muscle Injury Repair. Proc. Natl. Acad. Sci. U.S.A. 106 (41), 17475–17480. doi:10.1073/pnas.0908641106
Saclier, M., Lapi, M., Bonfanti, C., Rossi, G., Antonini, S., and Messina, G. (2020). The Transcription Factor Nfix Requires RhoA-ROCK1 Dependent Phagocytosis to Mediate Macrophage Skewing during Skeletal Muscle Regeneration. Cells 9 (3). doi:10.3390/cells9030708
Saclier, M., Yacoub-Youssef, H., Mackey, A. L., Arnold, L., Ardjoune, H., Magnan, M., et al. (2013). Differentially Activated Macrophages Orchestrate Myogenic Precursor Cell Fate during Human Skeletal Muscle Regeneration. Stem Cells 31 (2), 384–396. doi:10.1002/stem.1288
Scher, J. U., and Pillinger, M. H. (2005). 15d-PGJ2: the Anti-inflammatory Prostaglandin? Clin. Immunol. 114 (2), 100–109. doi:10.1016/j.clim.2004.09.008
Schulz, C., Perdiguero, E. G., Chorro, L., Szabo-Rogers, H., Cagnard, N., Kierdorf, K., et al. (2012). A Lineage of Myeloid Cells Independent of Myb and Hematopoietic Stem Cells. Science 336 (6077), 86–90. doi:10.1126/science.1219179
Scott, C. L., Zheng, F., De Baetselier, P., Martens, L., Saeys, Y., De Prijck, S., et al. (2016). Bone Marrow-Derived Monocytes Give Rise to Self-Renewing and Fully Differentiated Kupffer Cells. Nat. Commun. 7, 10321. doi:10.1038/ncomms10321
Seale, P., Sabourin, L. A., Girgis-Gabardo, A., Mansouri, A., Gruss, P., and Rudnicki, M. A. (2000). Pax7 Is Required for the Specification of Myogenic Satellite Cells. Cell 102 (6), 777–786. doi:10.1016/s0092-8674(00)00066-0
Segalés, J., Perdiguero, E., and Muñoz-Cánoves, P. (2015). Epigenetic Control of Adult Skeletal Muscle Stem Cell Functions. FEBS J. 282 (9), 1571–1588. doi:10.1111/febs.13065
Serhan, C. N., and Savill, J. (2005). Resolution of Inflammation: the Beginning Programs the End. Nat. Immunol. 6 (12), 1191–1197. doi:10.1038/ni1276
Shang, M., Cappellesso, F., Amorim, R., Serneels, J., Virga, F., Eelen, G., et al. (2020). Macrophage-derived Glutamine Boosts Satellite Cells and Muscle Regeneration. Nature 587 (7835), 626–631. doi:10.1038/s41586-020-2857-9
Shi, C., and Pamer, E. G. (2011). Monocyte Recruitment during Infection and Inflammation. Nat. Rev. Immunol. 11 (11), 762–774. doi:10.1038/nri3070
Sonnet, C., Lafuste, P., Arnold, L., Brigitte, M., Poron, F., Authier, F. J., et al. (2006). Human Macrophages Rescue Myoblasts and Myotubes from Apoptosis through a Set of Adhesion Molecular Systems. J. Cell Sci. 119 (Pt 12), 2497–2507. doi:10.1242/jcs.02988
Stedman, H. H., Sweeney, H. L., Shrager, J. B., Maguire, H. C., Panettieri, R. A., Petrof, B., et al. (1991). The Mdx Mouse Diaphragm Reproduces the Degenerative Changes of Duchenne Muscular Dystrophy. Nature 352 (6335), 536–539. doi:10.1038/352536a0
Sun, D., Martinez, C. O., Ochoa, O., Ruiz-Willhite, L., Bonilla, J. R., Centonze, V. E., et al. (2009). Bone Marrow-Derived Cell Regulation of Skeletal Muscle Regeneration. FASEB J. 23 (2), 382–395. doi:10.1096/fj.07-095901
Swirski, F. K., Nahrendorf, M., Etzrodt, M., Wildgruber, M., Cortez-Retamozo, V., Panizzi, P., et al. (2009). Identification of Splenic Reservoir Monocytes and Their Deployment to Inflammatory Sites. Science 325 (5940), 612–616. doi:10.1126/science.1175202
Tamoutounour, S., Guilliams, M., Montanana Sanchis, F., Liu, H., Terhorst, D., Malosse, C., et al. (2013). Origins and Functional Specialization of Macrophages and of Conventional and Monocyte-Derived Dendritic Cells in Mouse Skin. Immunity 39 (5), 925–938. doi:10.1016/j.immuni.2013.10.004
Teixeira, C. F. P., Zamunér, S. R., Zuliani, J. P., Fernandes, C. M., Cruz-Hofling, M. A., Fernandes, I., et al. (2003). Neutrophils Do Not Contribute to Local Tissue Damage, but Play a Key Role in Skeletal Muscle Regeneration, in Mice Injected withBothrops Aspersnake Venom. Muscle Nerve 28 (4), 449–459. doi:10.1002/mus.10453
Tidball, J. G. (2011). Mechanisms of Muscle Injury, Repair, and Regeneration. Compr. Physiol. 1 (4), 2029–2062. doi:10.1002/cphy.c100092
Tidball, J. G., and Villalta, S. A. (2010). Regulatory Interactions between Muscle and the Immune System during Muscle Regeneration. Am. J. Physiology-Regulatory, Integr. Comp. Physiology 298 (5), R1173–R1187. doi:10.1152/ajpregu.00735.2009
Tonkin, J., Temmerman, L., Sampson, R. D., Gallego-Colon, E., Barberi, L., Bilbao, D., et al. (2015). Monocyte/Macrophage-derived IGF-1 Orchestrates Murine Skeletal Muscle Regeneration and Modulates Autocrine Polarization. Mol. Ther. 23 (7), 1189–1200. doi:10.1038/mt.2015.66
Toumi, H., F’guyer, S., and Best, T. M. (2006). The Role of Neutrophils in Injury and Repair Following Muscle Stretch. J. Anat. 208, 459–470. doi:10.1111/j.1469-7580.2006.00543.x
Uderhardt, S., Martins, A. J., Tsang, J. S., Lämmermann, T., and Germain, R. N. (2019). Resident Macrophages Cloak Tissue Microlesions to Prevent Neutrophil-Driven Inflammatory Damage. Cell 177 (3), 541–555. doi:10.1016/j.cell.2019.02.028
Uezumi, A., Fukada, S.-i., Yamamoto, N., Takeda, S. i., and Tsuchida, K. (2010). Mesenchymal Progenitors Distinct from Satellite Cells Contribute to Ectopic Fat Cell Formation in Skeletal Muscle. Nat. Cell Biol. 12 (2), 143–152. doi:10.1038/ncb2014
Uezumi, A., Ikemoto-Uezumi, M., and Tsuchida, K. (2014). Roles of Nonmyogenic Mesenchymal Progenitors in Pathogenesis and Regeneration of Skeletal Muscle. Front. Physiol. 5, 68. doi:10.3389/fphys.2014.00068
Urciuolo, A., Quarta, M., Morbidoni, V., Gattazzo, F., Molon, S., Grumati, P., et al. (2013). Collagen VI Regulates Satellite Cell Self-Renewal and Muscle Regeneration. Nat. Commun. 4, 1964. doi:10.1038/ncomms2964
van Velthoven, C. T. J., de Morree, A., Egner, I. M., Brett, J. O., and Rando, T. A. (2017). Transcriptional Profiling of Quiescent Muscle Stem Cells In Vivo. Cell Rep. 21 (7), 1994–2004. doi:10.1016/j.celrep.2017.10.037
Vannella, K. M., and Wynn, T. A. (2017). Mechanisms of Organ Injury and Repair by Macrophages. Annu. Rev. Physiol. 79, 593–617. doi:10.1146/annurev-physiol-022516-034356
Varga, T., Mounier, R., Gogolak, P., Poliska, S., Chazaud, B., and Nagy, L. (2013). Tissue LyC6- Macrophages Are Generated in the Absence of Circulating LyC6− Monocytes and Nur77 in a Model of Muscle Regeneration. J. Immunol. 191 (11), 5695–5701. doi:10.4049/jimmunol.1301445
Varga, T., Mounier, R., Horvath, A., Cuvellier, S., Dumont, F., Poliska, S., et al. (2016a). Highly Dynamic Transcriptional Signature of Distinct Macrophage Subsets during Sterile Inflammation, Resolution, and Tissue Repair. J. Immunol. 196 (11), 4771–4782. doi:10.4049/jimmunol.1502490
Varga, T., Mounier, R., Patsalos, A., Gogolák, P., Peloquin, M., Horvath, A., et al. (2016b). Macrophage PPARγ, a Lipid Activated Transcription Factor Controls the Growth Factor GDF3 and Skeletal Muscle Regeneration. Immunity 45 (5), 1038–1051. doi:10.1016/j.immuni.2016.10.016
Villalta, S. A., Nguyen, H. X., Deng, B., Gotoh, T., and Tidball, J. G. (2009). Shifts in Macrophage Phenotypes and Macrophage Competition for Arginine Metabolism Affect the Severity of Muscle Pathology in Muscular Dystrophy. Hum. Mol. Genet. 18 (3), 482–496. doi:10.1093/hmg/ddn376
Villalta, S. A., Rinaldi, C., Deng, B., Liu, G., Fedor, B., and Tidball, J. G. (2011). Interleukin-10 Reduces the Pathology of Mdx Muscular Dystrophy by Deactivating M1 Macrophages and Modulating Macrophage Phenotype. Hum. Mol. Genet. 20 (4), 790–805. doi:10.1093/hmg/ddq523
Wang, H., Melton, D. W., Porter, L., Sarwar, Z. U., McManus, L. M., and Shireman, P. K. (2014). Altered Macrophage Phenotype Transition Impairs Skeletal Muscle Regeneration. Am. J. pathology 184 (4), 1167–1184. doi:10.1016/j.ajpath.2013.12.020
Wang, X., Sathe, A. A., Smith, G. R., Ruf-Zamojski, F., Nair, V., Lavine, K. J., et al. (2020). Heterogeneous Origins and Functions of Mouse Skeletal Muscle-Resident Macrophages. Proc. Natl. Acad. Sci. U.S.A. 117 (34), 20729–20740. doi:10.1073/pnas.1915950117
Wang, X., Zhao, W., Ransohoff, R. M., and Zhou, L. (2016). Identification and Function of Fibrocytes in Skeletal Muscle Injury Repair and Muscular Dystrophy. J. Immunol. 197 (12), 4750–4761. doi:10.4049/jimmunol.1601308
Wang, X., Zhao, W., Ransohoff, R. M., and Zhou, L. (2018). Infiltrating Macrophages Are Broadly Activated at the Early Stage to Support Acute Skeletal Muscle Injury Repair. J. Neuroimmunol. 317, 55–66. doi:10.1016/j.jneuroim.2018.01.004
Wehling, M., Spencer, M. J., and Tidball, J. G. (2001). A Nitric Oxide Synthase Transgene Ameliorates Muscular Dystrophy in Mdx Mice. J. Cell Biol. 155 (1), 123–132. doi:10.1083/jcb.200105110
Wynn, T. A., Chawla, A., and Pollard, J. W. (2013). Macrophage Biology in Development, Homeostasis and Disease. Nature 496, 445–455. doi:10.1038/nature12034
Wynn, T. A., and Vannella, K. M. (2016). Macrophages in Tissue Repair, Regeneration, and Fibrosis. Immunity 44 (3), 450–462. doi:10.1016/j.immuni.2016.02.015
Xiao, Y. Q., Freire-de-Lima, C. G., Schiemann, W. P., Bratton, D. L., Vandivier, R. W., and Henson, P. M. (2008). Transcriptional and Translational Regulation of TGF-β Production in Response to Apoptotic Cells. J. Immunol. 181 (5), 3575–3585. doi:10.4049/jimmunol.181.5.3575
Yin, H., Price, F., and Rudnicki, M. A. (2013). Satellite Cells and the Muscle Stem Cell Niche. Physiol. Rev. 93 (1), 23–67. doi:10.1152/physrev.00043.2011
Yue, L., Wan, R., Luan, S., Zeng, W., and Cheung, T. H. (2020). Dek Modulates Global Intron Retention during Muscle Stem Cells Quiescence Exit. Dev. Cell 53 (6), 661–676. doi:10.1016/j.devcel.2020.05.006
Zhang, C., Li, Y., Wu, Y., Wang, L., Wang, X., and Du, J. (2013). Interleukin-6/signal Transducer and Activator of Transcription 3 (STAT3) Pathway Is Essential for Macrophage Infiltration and Myoblast Proliferation during Muscle Regeneration. J. Biol. Chem. 288 (3), 1489–1499. doi:10.1074/jbc.m112.419788
Zhang, J., Qu, C., Li, T., Cui, W., Wang, X., and Du, J. (2019). Phagocytosis Mediated by Scavenger Receptor Class BI Promotes Macrophage Transition during Skeletal Muscle Regeneration. J. Biol. Chem. 294 (43), 15672–15685. doi:10.1074/jbc.ra119.008795
Zhao, W., Wang, X., Ransohoff, R. M., and Zhou, L. (2017). CCR2 Deficiency Does Not Provide Sustained Improvement of Muscular Dystrophy in Mdx 5cv Mice. FASEB J. 31 (1), 35–46. doi:10.1096/fj.201600619r
Zhou, L., Porter, J. D., Cheng, G., Gong, B., Hatala, D. A., Merriam, A. P., et al. (2006). Temporal and Spatial mRNA Expression Patterns of TGF-Β1, 2, 3 and TβRI, II, III in Skeletal Muscles of Mdx Mice. Neuromuscul. Disord. 16 (1), 32–38. doi:10.1016/j.nmd.2005.09.009
Ziegler-Heitbrock, L., Ancuta, P., Crowe, S., Dalod, M., Grau, V., HartLeenen, D. N., et al. (2010). Nomenclature of Monocytes and Dendritic Cells in Blood. Blood 116 (16), e74–e80. doi:10.1182/blood-2010-02-258558
Zordan, P., Rigamonti, E., Freudenberg, K., Conti, V., Azzoni, E., Rovere-Querini, P., et al. (2014). Macrophages Commit Postnatal Endothelium-Derived Progenitors to Angiogenesis and Restrict Endothelial to Mesenchymal Transition during Muscle Regeneration. Cell Death Dis. 5, e1031. doi:10.1038/cddis.2013.558
Keywords: skeletal muscle, macrophage, injury repair, regeneration, satellite cells
Citation: Wang X and Zhou L (2022) The Many Roles of Macrophages in Skeletal Muscle Injury and Repair. Front. Cell Dev. Biol. 10:952249. doi: 10.3389/fcell.2022.952249
Received: 24 May 2022; Accepted: 23 June 2022;
Published: 11 July 2022.
Edited by:
Lorenzo Giordani, INSERM U974 Institut de Myologie, FranceReviewed by:
Biliana Lozanoska-Ochser, Sapienza University of Rome, ItalyMaria A. Mariggiò, University of Studies G. d’Annunzio Chieti and Pescara, Italy
Copyright © 2022 Wang and Zhou. This is an open-access article distributed under the terms of the Creative Commons Attribution License (CC BY). The use, distribution or reproduction in other forums is permitted, provided the original author(s) and the copyright owner(s) are credited and that the original publication in this journal is cited, in accordance with accepted academic practice. No use, distribution or reproduction is permitted which does not comply with these terms.
*Correspondence: Lan Zhou, bGFuemhvdUBidS5lZHU=