- 1Department of Functional Neurobiology, Tokyo University of Pharmacy and Life Sciences, Hachioji, Japan
- 2Department of Occupational Therapy, Faculty of Rehabilitation, Niigata University of Health and Welfare, Niigata, Japan
In vertebrates, a high density of voltage-gated Na+ channel at nodes of Ranvier and of voltage-gated K+ channel at juxtaparanodes is necessary for rapid propagation of action potential, that is, for saltatory conduction in myelinated axons. Myelin loops attach to the axonal membrane and form paranodal axoglial junctions (PNJs) at paranodes adjacent to nodes of Ranvier. There is growing evidence that the PNJs contribute to axonal homeostasis in addition to their roles as lateral fences that restrict the location of nodal axolemmal proteins for effective saltatory conduction. Perturbations of PNJs, as in specific PNJ protein knockouts as well as in myelin lipid deficient mice, result in internodal axonal alterations, even if their internodal myelin is preserved. Here we review studies showing that PNJs play crucial roles in the myelinated axonal homeostasis. The present evidence points to two functions in particular: 1) PNJs facilitate axonal transport of membranous organelles as well as cytoskeletal proteins; and 2) they regulate the axonal distribution of type 1 inositol 1,4,5-trisphosphate receptor (IP3R1) in cerebellar Purkinje axons. Myelinated axonal homeostasis depends among others on the state of PNJs, and consequently, a better understanding of this dependency may contribute to the clarification of CNS disease mechanisms and the development of novel therapies.
Introduction
Myelin is a membrane structure that developed in the evolution of vertebrates (Boullerne, 2016; Salzer and Zalc, 2016). In the developmental stage of the central nervous system, axons become myelinated through wrapping by oligodendrocytes. The resulting myelinated axons have distinct specialized regions: nodes of Ranvier, paranodes, juxtaparanodes, and internodes (Figure 1). At the nodes of Ranvier there is a high density of voltage-gated Na+ channels (Nav1.2 and Nav1.6) that conduct inward depolarizing currents, whereas juxtaparanodal K+ channels (Kv1.1 and Kv1.2) maintain electrical stability and polarization (Ritchie and Rogart, 1977; Caldwell et al., 2000). As a result, rapid conduction of nerve impulses, known as saltatory conduction, is achieved by action potentials leaping along myelinated nerves via the nodes of Ranvier (Tasaki, 1939; Huxley and Stampfli, 1949). A recent study by Kanda et al. showed that two-pore-domain potassium (K2P) channels, TREK-1 and TRAAK, are also clustered at the nodes of Ranvier and that these are required for rapid action potential regeneration (Kanda et al., 2019). At the paranodes adjacent to the nodes of Ranvier, there are paranodal axoglial junctions (PNJs) that are characterized by intermittent densities associated with the outer leaflet of the axolemma, called transverse bands (Peters, 1966; Rosenbluth, 1976), and myelin loops that attach to the axonal membrane (Rosenbluth, 1999). Three paranode-specific cell adhesion molecules are primarily required for the formation of PNJs: contactin (Rios et al., 2000; Bhat et al., 2001; Boyle et al., 2001) and contactin-associated protein (Einheber et al., 1997; Peles et al., 1997) on the side of the axonal membrane, and Neurofascin-155 on the side of the glial membrane (Tait et al., 2000; Sherman et al., 2005). Autoantibodies against Neurofascin-155 and contactin have been found in patients with neuropathy (Labasque et al., 2014; Koike et al., 2017; Vallat et al., 2017). Koike and colleagues demonstrated that these antibodies induced paranodal axoglial detachment, resulting in aberrant nerve conduction and axonal damage (Koike et al., 2017). Anti-Neurofascin-155 antibody is also present in patients with combined central and peripheral demyelination (CCPD; Kawamura et al., 2013; Kira, 2021).
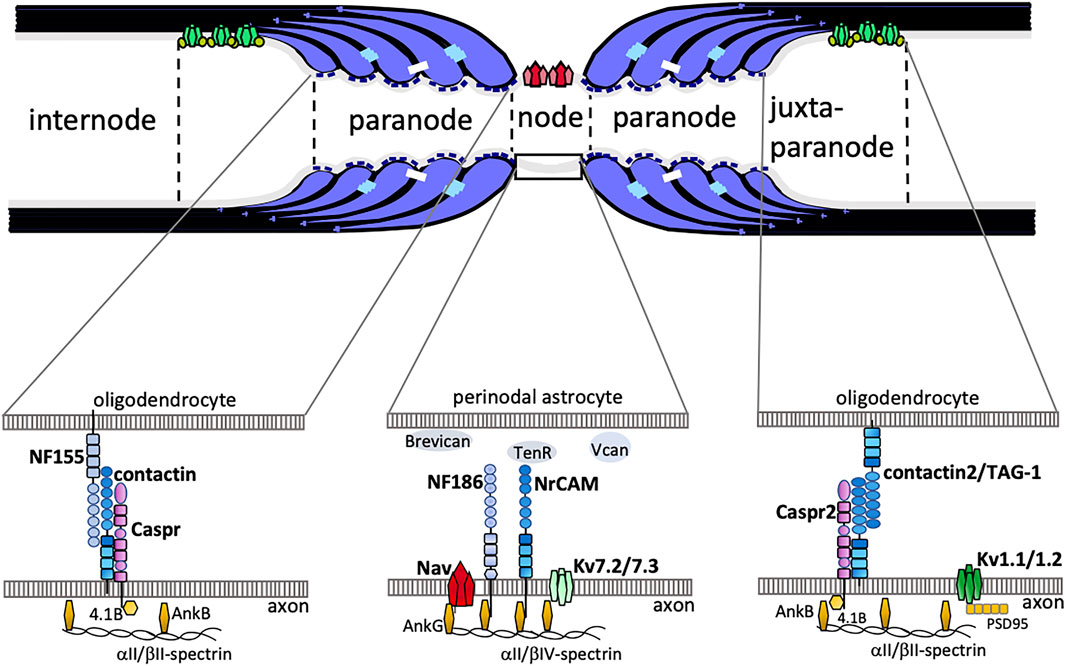
FIGURE 1. The schematic drawing shows junctional complexes between oligodendrocytes and axons in myelinated axons. The nodal axolemma with voltage-gated Na+ channels (Nav) has concentrations of neurofascin 186 (NF186) and neuronal cell adhesion molecule (NrCAM) belonging to the L1-family of CAMs at the node of Ranvier. The cytoplasmic region of axonal NF186 and NrCAM binds ankyrinG (AnkG), which anchors the nodal complex to βIV-spectrin and to the actin cytoskeleton. AnkG enables the clustering of Nav and Kv7.2/7.3 channels. Extracellular matrices such as Brevican, Versican (Vcan), and TenascinR (TenR) are surrounding the nodes. At the paranode, the cis-complex of Caspr and contactin interacts in trans with NF155 on the paranodal myelin loop. This complex is stabilized by protein 4.1B which co-localizes with AnkB, aII/bII-spectrin. At the juxtaparanode, Shaker-type voltage gated K+ channels Kv1.1/1.2 form clusters. A complex of contactin2 (also known as TAG-1) and Caspr2 is implicated in the formation of juxtaparanodes.
The morphological structure of the nodes of Ranvier and their adjacent paranodes suggests that nodal formation and maturation depend on the state of the PNJs. In fact, it has been reported that nodal Na+ channel clustering depends on paranodal axoglial contact in the developing CNS (Rasband et al., 1999). These paranode-dependent clusterings of nodal Na+ channels require axonal βII spectrin, which is concentrated at the paranodes (Ogawa et al., 2006; Amor et al., 2017). Zhang et al. also reported that the accumulation of Neurofascin-186, a key adhesion molecule of nodes, is regulated by PNJs (Zhang et al., 2020). Additionally, PNJs are required for the maintenance of Na+ channel clustering and nodal maturation (Ishibashi et al., 2002; Ishibashi et al., 2003; Rasband et al., 2003). More recently, Elazar et al. demonstrated that coordinated action of internodal (Cadm4 and MAG) and paranodal (Caspr) cell adhesion molecules is required for accurate myelination (Elazar et al., 2019a; Elazar et al., 2019b). Hence, the PNJs play several pivotal roles—among others, they promote node formation and are anchored to the axonal cytoskeleton, thus serving as a fence that limits the lateral movement of axolemmal proteins and keeps them in place (Rasband et al., 1999; Amor et al., 2014, 2017; Brivio et al., 2017). Furthermore, there is growing evidence that the PNJs contribute to the maintenance of functional, myelinated axons and of axonal homeostasis (Pillai et al., 2009; Rosenbluth, 2009; Ohno et al., 2011; Ishibashi et al., 2015). Numerous reviews (Rosenbluth, 2009; Faivre-Sarrailh et al., 2013; Rosenbluth et al., 2013; Arancibia-Carcamo and Attwell, 2014; Susuki et al., 2016; Faivre-Sarrailh, 2020) have discussed the identities and roles of the ever-increasing number of molecules associated with the adhesion molecules at the paranodes, as well as voltage gated Na+ channels at the nodes and voltage gated K+ channels at the juxtaparanodes. This review focuses on the significance of PNJs in the facilitation of axonal transport and in cerebellar Purkinje axonal homeostasis.
PNJs: A Key Facilitator in Axonal Transport
Neurons are highly polarized cells with elongated axons that can reach a considerable distance from the perikaryon. In the axon, membranous organelles, molecular complexes, and cytoskeletal components of the axoplasm (such as microtubules and neurofilament) are transported by either fast or slow transport from one end of the axon to the other (Grafstein and Forman, 1980; Hirokawa, 1997). It is, therefore, essential for axonal polarity and axonal stabilization to maintain accurate axonal transport (Sleigh et al., 2019; Guillaud et al., 2020). Axonal mitochondrial dynamics have been studied especially intensively under both physiological and pathological conditions (Andrew et al., 2006; Kiryu-Seo et al., 2010; Chiu, 2011; Zambonin et al., 2011; Zhang et al., 2012; Itoh et al., 2013; Ohno et al., 2014; Sorbara et al., 2014; Joshi et al., 2015; Joshi et al., 2019; Kiryu-Seo and Kiyama, 2019).
Impairment of axonal transport precedes the axonal degeneration observed in demyelinating diseases, such as multiple sclerosis (MS) and amyotrophic lateral sclerosis (ALS), and is also a common hallmark in various neurodegenerative diseases (Coleman, 2005; Morfini et al., 2009; Sorbara et al., 2014; Sleigh et al., 2019; Guillaud et al., 2020). Altered axonal transport and focal axonal swelling formation with mitochondrial alterations have been reported in several knockout mice ablating specific myelin proteins (Griffiths et al., 1998; Lappe-Siefke 2003; Edgar et al., 2004), in dysmyelinating mouse Shiverer (Joshi et al., 2015; Joshi et al., 2019), and in experimental autoimmune encephalomyelitis (EAE) which is the most commonly used experimental model for MS (Nikić et al., 2011). Superb in vivo imaging studies demonstrated that abnormal mitochondria and transport deficits were already present in myelinated axons in an acute EAE model (Nikić et al., 2011; Sorbara et al., 2014). They also demonstrated that the initial swellings of the axon often occur at putative nodes of Ranvier (Nikić et al., 2011).
Similar impairment of axonal transport has been reported in knockout (KO) mice ablating PNJ proteins Caspr (Einheber et al., 1997; Bhat et al., 2001; Einheber et al., 2006) and glia-specific Neurofascin-155 (Pillai et al., 2009), in Caspr mutant mouse shambling (Takagishi et al., 2016), in mice ablating cytoskeletal scaffolding proteins Band 4.1B and Whirlin (Saifetiarova and Bhat, 2019), and in mice with defective synthesis of the myelin lipids ceramide galactosyltransferase (CGT)-null (Garcia-Fresco et al., 2006) and cerebroside sulfotransferase (CST)-null (Honke et al., 2002; Ishibashi et al., 2015). Furthermore, ether lipids-deficient mice (Teigler et al., 2009) and Purkinje cell-specific Ugcg KO mice (Watanabe et al., 2010) that displayed disrupted PNJs also showed axonal swellings. The most noticeable common finding in these PNJ-disrupted mouse models is that the focal impaired axonal transport indicated by axonal swellings has already become apparent in axons with intact compact myelin sheaths at early developmental stages (Pillai et al., 2009; Teigler et al., 2009; Ishibashi et al., 2015). Significantly, in case of CST-null mice, it has been shown that swelling occurs even if the transverse bands at the paranodes are only partially disrupted (Honke et al., 2002; Marcus et al., 2006).
Although we do not know whether the formation of PNJs is intact at early stages in acute mouse models of MS and in acute human MS lesions (Nikić et al., 2011), the observation that the initial disruption of the axon often occurs close to nodes of Ranvier seems to imply that the state of PNJs is a cause of focal axonal damage. At the paranode, the cytoplasmic portion of Caspr is associated with axonal actin cytoskeleton via scaffolding proteins Band 4.1B, Whirlin, αII and βII spectrin, and ankyrin B (Garcia-Fresco et al., 2006; Ogawa et al., 2006; Saifetiarova and Bhat, 2019). Therefore, even partial alteration of PNJs may disturb axonal cytoskeletal functions, resulting in impaired axonal transport. Taken together, the evidence suggests that the structure of PNJs is one of the most important influences on axonal transport and plays a key role as a facilitator for the transport.
The State of PNJs and Purkinje Axonal Homeostasis
Focal axonal swellings arise in several regions of the central nervous system (CNS) in a number of neurodegenerative disorders and in traumatic brain injury (Trapp et al., 1998; Nikić et al., 2011; Sorbara et al., 2014; Jin et al., 2015; Maia et al., 2015). In these cases, the axonal swellings on cerebellar Purkinje cells—known as torpedoes—are associated with Purkinje cell loss (Louis et al., 2009, 2014; Maia et al., 2015; Ljungberg., 2016; Gionco et al., 2021). Although there are many causes of Purkinje axonal swellings, there are common morphological features in Purkinje axons in the mouse models with perturbations of PNJs, described in the following.
The Purkinje axonal swellings are usually covered with PLP-positive compact myelin in CST-null mice (Ishibashi et al., 2015). Similar observations have been made with electron microscopy (EM) analysis in other mouse models, including deletion of CGT (Garcia-Fresco et al., 2006), ether lipids (Teigler et al., 2009), glia-specific Neurofascin-155 (Pillai et al., 2007; Pillai et al., 2009), cytoskeletal scaffolding proteins Band 4.1B and Whirlin (Saifetiarova and Bhat, 2019), and Caspr mutant mouse shambling (Takagishi et al., 2016), suggesting that these swellings are formed in the internodal axon. In CST-null mice, the swellings along Purkinje axons became prominent only after myelin formation, and their number and size progressively increased with age (Ishibashi et al., 2015). Initially, the swellings were small and characterized by Calbindin-positive axoplasm, but gradually, axonal cytoskeletal neurofilament accumulations were observed within the swellings (Ishibashi et al., 2015). These axonal swelling features are also reported in mice ablating cytoskeletal scaffolding proteins Band 4.1B and Whirlin (Saifetiarova and Bhat, 2019). In CST-null, with the increasing size of the swellings, the accumulation of mitochondrial COXIV became prominent, followed by the detection of neurofilaments and amyloid precursor protein (APP), which is a marker for axonal damage (Ishibashi et al., 2015). The mitochondrial accumulation revealed by cytochrome c staining, as well as neurofilaments in the axonal swellings, are also reported in Caspr-null and CGT-null mice (Garcia-Fresco et al., 2006) and in Purkinje cell-specific Ugcg KO mice (Watanabe et al., 2010). These findings seem to indicate a process of deterioration of focal axonal transport and suggest that the focal axoplasm alteration occurs before destabilization of the axonal cytoskeleton in disrupted PNJs Purkinje axons.
Then, what could be the cause of Purkinje axonal swellings in disrupted PNJs? The most intriguing common features in Purkinje axonal swellings in mice with disrupted PNJs are accumulations of smooth endoplasmic reticulum (sER) and focal accumulations of type 1 inositol 1,4,5-trisphosphate receptor (IP3R1). In CST-null, EM analysis showed numerous membranous organelles, dense bodies, and tubular structures closely resembling sER in the Purkinje axonal swellings (Ishibashi et al., 2015). Similar accumulations of sER were reported in the Purkinje axonal swellings in other mutant mice with disrupted PNJs (Garcia-Fresco et al., 2006; Pillai et al., 2007; Teigler et al., 2009; Takagishi et al., 2016; Saifetiarova and Bhat, 2019), and an in vitro overexpression study of IP3R1 reported similar stacks of membranous sER (Takei et al., 1994). Furthermore, IP3R1 is especially abundant in Purkinje neurons and localizes to the sER (Satoh et al., 1990; Mikoshiba, 2007). All of these findings suggest that IP3R1 overexpression causes these sER accumulations.
Further supporting this suggestion, in CST-null mice, IP3R1-positive focal accumulations are the earliest finding in the Purkinje axonal swellings (Ishibashi et al., 2015), and IP3R1 had accumulated even in small-sized swellings that still lacked (observed) neurofilament accumulation. In the developmental stage, IP3R1-positive axonal swellings start appearing by 12 days of age, just after myelination in CST-null mice, and these IP3R1-positive axonal swellings always occur at the myelinated internode and not at the disrupted paranodal regions (Ishibashi et al., 2015). The accumulation of IP3R1 in the Purkinje axonal swellings was also reported in ether lipid-deficient mice (Teigler et al., 2009) and in Caspr mutant mouse shambling (Takagishi et al., 2016), suggesting that this is a common feature in myelinated axons with PNJs disruption. Although the molecular mechanism responsible for the focal IP3R1 accumulation and sER accumulation in the PNJ-disrupted Purkinje cells still need to be established, the formation of axonal swellings in disrupted PNJ Purkinje cells by accumulating IP3R1-rich sER may be the result of paranodal disorganization causing impaired axonal transport and disturbance of Ca2+ homeostasis. Therefore, the formation of a robust fence at the paranode appears to be critical for an appropriate distribution of IP3R1 in Ca2+ homeostasis in myelinated nerves.
Discussion
It is well known that an increase in neuronal Ca2+ is involved in the triggering of neuronal death. Ca2+ release by IP3Rs on internal stores, such as ER and mitochondria, plays an essential role in several neurological disorders (Chiu, 2011; Barsukova et al., 2012; Takada et al., 2017), but details of these processes remain elusive. Accumulation not just of sER has been repeatedly observed in the Purkinje axonal swellings of mice with disrupted PNJs, but also of mitochondria. Because an increase in the concentration of cytosolic Ca2+ has been shown to lead to arrested movement of ER and mitochondria (Wang et al., 2000; Brough et al., 2005), the focal activation of IP3 signaling may induce alteration of motility of mitochondria as well as ER in the PNJ-disrupted Purkinje axons. Structural interactions between ER and mitochondria in mitochondria-associated ER membranes (MAMs) are crucial for Ca2+ transfer between ER and mitochondria (Hayashi et al., 2009; Paillusson et al., 2016). In MAMs, IP3R and voltage-dependent anion channel (VDAC) interact via glucose-regulated protein 75-kDa (GRP75), allowing a direct transfer of Ca2+ into the mitochondria (Rapizzi et al., 2002; Szabadkai et al., 2006; Paillusson et al., 2016). This suggests the involvement of a local calcium dysregulation through IP3R1 in the occurrence of Purkinje axonal swellings in the disrupted PNJ Purkinje cells.
A study by Yin and others revealed the importance of mitochondria-ER associations in Ca2+ homeostasis in myelinated axons (Yin et al., 2016), and another study by Thai and others suggests that the interactions between mitochondria and ER are enhanced in chronically demyelinated axons and maintained during axonal degeneration in hereditary myelin disease (Thai et al., 2018). To improve understanding of the relation between myelination and MAM formation and/or ER distribution in axonal Ca2+ homeostasis, it needs to be established whether elevated Ca2+ concentration occurs in the Purkinje axonal swellings of PNJ-disrupted mice. Although further experiments are needed to reveal the mechanism of accumulation of IP3R1, the findings from the mice with disrupted PNJs suggest that the formation of a robust fence at the paranode is critical for an appropriate distribution of IP3R1 in Ca2+ homeostasis in myelinated nerves.
The focal axonal swellings in Purkinje cells are found in various pathological conditions as well as during development. A study by Lang-Ouellette and others recently reported that Purkinje axonal swellings in healthy young mice enhance action potential fidelity and cerebellar function (Ljungberg et al., 2016; Lans-Quellette et al., 2021). However, the density of intracellular organelles in the axonal swellings did not change compared to control axons (Lans-Quellette et al., 2021), suggesting that the Purkinje axonal swellings in healthy development and disease models differ in their subcellular composition.
There are many kinds of KO and mutant mice that show disrupted PNJs, and the severity of the junctional disruption in paranodes is diverse. Therefore, such mice will be a useful tool to identify the molecular mechanisms through which myelin interacts with the axon to maintain Ca2+ homeostasis and to protect it from degeneration.
Author Contributions
TI wrote the manuscript and HB helped manuscript amendment. All authors reviewed the manuscript.
Funding
JSPS KAKENHI Grant Number 20K06863.
Conflict of Interest
The authors declare that the research was conducted in the absence of any commercial or financial relationships that could be construed as a potential conflict of interest.
Publisher’s Note
All claims expressed in this article are solely those of the authors and do not necessarily represent those of their affiliated organizations, or those of the publisher, the editors and the reviewers. Any product that may be evaluated in this article, or claim that may be made by its manufacturer, is not guaranteed or endorsed by the publisher.
References
Amor, V., Feinberg, K., Eshed-Eisenbach, Y., Vainshtein, A., Frechter, S., Grumet, M., et al. (2014). Long-term Maintenance of Na+ Channels at Nodes of Ranvier Depends on Glial Contact Mediated by Gliomedin and NrCAM. J. Neurosci. 34 (15), 5089–5098. doi:10.1523/JNEUROSCI.4752-13.2014
Amor, V., Zhang, C., Vainshtein, A., Zhang, A., Zollinger, D. R., Eshed-Eisenbach, Y., et al. (2017). The Paranodal Cytoskeleton Clusters Na+ Channels at Nodes of Ranvier. Elife 6, e21392. doi:10.7554/eLife.21392
Andrews, H., White, K., Thomson, C., Edgar, J., Bates, D., Griffiths, I., et al. (2006). Increased Axonal Mitochondrial Activity as an Adaptation to Myelin Deficiency in the Shiverer Mouse. Neurosci. Res. 83 (8), 1533–1539. doi:10.1002/jnr.20842
Arancibia-Carcamo, I. L., and Attwell, D. (2014). The Node of Ranvier in CNS Pathology. Acta Neuropathol. 128 (2), 161–175. doi:10.1007/s00401-014-1305-z
Barsukova, A. G., Forte, M., and Bourdette, D. J. (2012). Focal Increases of Axoplasmic Ca2+, Aggregation of Sodium-Calcium Exchanger, N-type Ca2+ Channel, and Actin Define the Sites of Spheroids in Axons Undergoing Oxidative Stress. J. Neurosci. 32 (35), 12028–12037. doi:10.1523/JNEUROSCI.0408-12.2012
Bhat, M. A., Rios, J. C., Lu, Y., Garcia-Fresco, G. P., Ching, W., St Martin, M., et al. (2001). Axo-glia Interactions and the Domain Organization of Myelinated Axons Requires Neurexin IV/Caspr/Paranodin. Neuron 30 (2), 369–383. doi:10.1016/s0896-6273(01)00294-x
Boullerne, A. I. (2016). The History of Myelin. Exp. Neurol. 283 (Pt B), 431–445. doi:10.1016/j.expneurol.2016.06.005
Boyle, M. E., Berglund, E. O., Murai, K. K., Weber, L., Peles, E., and Ranscht, B. (2001). Contactin Orchestrates Assembly of the Septate-like Junctions at the Paranode in Myelinated Peripheral Nerve. Neuron 30 (2), 385–397. doi:10.1016/s0896-6273(01)00296-3
Brivio, V., Faivre-Sarrailh, C., Peles, E., Sherman, D. L., and Brophy, P. J. (2017). Assembly of CNS Nodes of Ranvier in Myelinated Nerves Is Promoted by the Axon Cytoskeleton. Curr. Biol. 27, 1068–1073. doi:10.1016/j.cub.2017.01.025
Brough, D., Schell, M. J., and Irvine, R. F. (2005). Agonist-induced Regulation of Mitochondrial and Endoplasmic Reticulum Motility. Biochem. J. 392 (Pt 2), 291–297. doi:10.1042/BJ20050738
Caldwell, J. H., Schaller, K. L., Lasher, R. S., Peles, E., and Levinson, S. R. (2000). Sodium Channel Na(v)1.6 Is Localized at Nodes of Ranvier, Dendrites, and Synapses. Proc. Natl. Acad. Sci. U. S. A. 97 (10), 5616–5620. doi:10.1073/pnas.090034797
Chiu, S. Y. (2011). Matching Mitochondria to Metabolic Needs at Nodes of Ranvier. Neuroscientist 17 (4), 343–350. doi:10.1177/1073858410393740
Coleman, M. (2005). Axon Degeneration Mechanisms: Commonality amid Diversity. Nat. Rev. Neurosci. 6 (11), 889–898. doi:10.1038/nrn1788
Edgar, J. M., McLaughlin, M., Yool, D., Zhang, S. C., Fowler, J. H., Montague, P., et al. (2004). Oligodendroglial Modulation of Fast Axonal Transport in a Mouse Model of Hereditary Spastic Paraplegia. J. Cell Biol. 166 (1), 121–131. doi:10.1083/jcb.200312012
Einheber, S., Bhat, M. A., and Salzer, J. L. (2006). Disrupted Axo-Glial Junctions Result in Accumulation of Abnormal Mitochondria at Nodes of Ranvier. Neuron Glia Biol. 2 (3), 165–174. doi:10.1017/S1740925X06000275
Einheber, S., Zanazzi, G., Ching, W., Scherer, S., Milner, T. A., Peles, E., et al. (1997). The Axonal Membrane Protein Caspr, a Homologue of Neurexin IV, Is a Component of the Septate-like Paranodal Junctions that Assemble during Myelination. J. Cell Biol. 139 (6), 1495–1506. doi:10.1083/jcb.139.6.1495
Elazar, N., Vainshtein, A., Golan, N., Vijayaragavan, B., Schaeren-Wiemers, N., Eshed-Eisenbach, Y., et al. (2019a). Axoglial Adhesion by Cadm4 Regulates CNS Myelination. Neuron 101 (2), 224–231. e5. doi:10.1016/j.neuron.2018.11.032
Elazar, N., Vainshtein, A., Rechav, K., Tsoory, M., Eshed-Eisenbach, Y., and Peles, E. (2019b). Coordinated Internodal and Paranodal Adhesion Controls Accurate Myelination by Oligodendrocytes. J. Cell Biol. 218 (9), 2887–2895. doi:10.1083/jcb.201906099
Faivre-Sarrailh, C., and Devaux, J. J. (2013). Neuro-glial Interactions at the Nodes of Ranvier: Implication in Health and Diseases. Front. Cell Neurosci. 7, 196. doi:10.3389/fncel.2013.00196
Faivre-Sarrailh, C. (2020). Molecular Organization and Function of Vertebrate Septate-like Junctions. Biochim. Biophys. Acta Biomembr. 1862 (5), 183211. doi:10.1016/j.bbamem.2020.183211
Garcia-Fresco, G. P., Sousa, A. D., Pillai, A. M., Moy, S. S., Crawley, J. N., Tessarollo, L., et al. (2006). Disruption of Axo-Glial Junctions Causes Cytoskeletal Disorganization and Degeneration of Purkinje Neuron Axons. Proc. Natl. Acad. Sci. U. S. A. 103 (13), 5137–5142. doi:10.1073/pnas.0601082103
Gionco, J. T., Hartstone, W. G., Martuscello, R. T., Kuo, S. H., Faust, P. L., and Louis, E. D. (2021). Essential Tremor versus "ET-Plus": A Detailed Postmortem Study of Cerebellar Pathology. Cerebellum 20 (6), 904–912. doi:10.1007/s12311-021-01263-6
Grafstein, B., and Forman, D. S. (1980). Intracellular Transport in Neurons. Physiol. Rev. 60 (4), 1167–1283. doi:10.1152/physrev.1980.60.4.1167
Griffiths, I., Klugmann, M., Anderson, T., Yool, D., Thomson, C., Schwab, M. H., et al. (1998). Axonal Swellings and Degeneration in Mice Lacking the Major Proteolipid of Myelin. Science 280 (5369), 1610–1613. doi:10.1126/science.280.5369.1610
Guillaud, L., El-Agamy, S. E., Otsuki, M., and Terenzio, M. (2020). Anterograde Axonal Transport in Neuronal Homeostasis and Disease. Front. Mol. Neurosci. 13, 556175. doi:10.3389/fnmol.2020.556175
Hayashi, T., Rizzuto, R., Hajnoczky, G., and Su, T. P. (2009). MAM: More Than Just a Housekeeper. Trends. Cell Biol. 19 (2), 81–88. doi:10.1016/j.tcb.2008.12.002
Hirokawa, N. (1997). The Mechanisms of Fast and Slow Transport in Neurons: Identification and Characterization of the New Kinesin Superfamily Motors. Curr. Opin. Neurobiol. 7 (5), 605–614. doi:10.1016/s0959-4388(97)80079-7
Honke, K., Hirahara, Y., Dupree, J., Suzuki, K., Popko, B., Fukushima, K., et al. (2002). Paranodal Junction Formation and Spermatogenesis Require Sulfoglycolipids. Proc. Natl. Acad. Sci. U. S. A. 99 (7), 4227–4232. doi:10.1073/pnas.032068299
Huxley, A. F., and Stampfli, R. (1949). Evidence for Saltatory Conduction in Peripheral Myelinated Nerve Fibers. J. Physiol. 108 (3), 315–339.
Ishibashi, T., Dupree, J. L., Ikenaka, K., Hirahara, Y., Honke, K., Peles, E., et al. (2002). A Myelin Galactolipid, Sulfatide, Is Essential for Maintenance of Ion Channels on Myelinated Axon but Not Essential for Initial Cluster Formation. J. Neurosci. 22 (15), 6507–6514. doi:10.1523/JNEUROSCI.22-15-06507.2002
Ishibashi, T., Ikenaka, K., Shimizu, T., Kagawa, T., and Baba, H. (2003). Initiation of Sodium Channel Clustering at the Node of Ranvier in the Mouse Optic Nerve. Neurochem. Res. 28 (1), 117–125. doi:10.1023/a:1021608514646
Ishibashi, T., Kodama, A., and Baba, H. (2015). Disruption of Paranodal Axo-Glial Interaction And/or Absence of Sulfatide Causes Irregular Type I Inositol 1,4,5-trisphosphate Receptor Deposition in Cerebellar Purkinje Neuron Axons. J. Neurosci. Res. 93 (1), 19–27. doi:10.1002/jnr.23465
Itoh, K., Nakamura, K., Iijima, M., and Sesaki, H. (2013). Mitochondrial Dynamics in Neurodegeneration. Trends Cell Biol. 23 (2), 64–71. doi:10.1016/j.tcb.2012.10.006
Jin, C., Washimi, Y., Yoshida, K., Hashizume, Y., and Yazawa, I. (2015). Characterization of Spheroids in Hereditary Diffuse Leukoencephalopathy with Axonal Spheroids. J. Neurol. Sci. 352 (1-2), 74–78. doi:10.1016/j.jns.2015.03.033
Joshi, D. C., Zhang, C. L., Babujee, L., Vevea, J. D., August, B. K., Sheng, Z. H., et al. (2019). Inappropriate Intrusion of an Axonal Mitochondrial Anchor into Dendrites Causes Neurodegeneration. Cell Rep. 29 (3), 685–696. e5. doi:10.1016/j.celrep.2019.09.012
Joshi, D. C., Zhang, C. L., Lin, T. M., Gusain, A., Harris, M. G., Tree, E., et al. (2015). Deletion of Mitochondrial Anchoring Protects Dysmyelinating Shiverer: Implications for Progressive MS. J. Neurosci. 35 (13), 5293–5306. doi:10.1523/JNEUROSCI.3859-14.2015
Kanda, H., Ling, J., Tonomura, S., Noguchi, K., Matalon, S., and Gu, J. G. (2019). TREK-1 and TRAAK Are Principal K + Channels at the Nodes of Ranvier for Rapid Action Potential Conduction on Mammalian Myelinated Afferent Nerves. Neuron 104 (5), 960–971. e7. doi:10.1016/j.neuron.2019.08.042
Kawamura, N., Yamasaki, R., Yonekawa, T., Matsushita, T., Kusunoki, S., Nagayama, S., et al. (2013). Anti-neurofascin Antibody in Patients with Combined Central and Peripheral Demyelination. Neurology 81 (8), 714–722. doi:10.1212/WNL.0b013e3182a1aa9c
Kira, J. I. (2021). Anti-Neurofascin 155 Antibody-Positive Chronic Inflammatory Demyelinating Polyneuropathy/Combined Central and Peripheral Demyelination: Strategies for Diagnosis and Treatment Based on the Disease Mechanism. Front. Neurol. 12, 665136. doi:10.3389/fneur.2021.665136
Kiryu-Seo, S., and Kiyama, H. (2019). Mitochondrial Behavior during Axon Regeneration/degeneration In Vivo. Neurosci. Res. 139, 42–47. doi:10.1016/j.neures.2018.08.014
Kiryu-Seo, S., Ohno, N., Kidd, G. J., Komuro, H., and Trapp, B. D. (2010). Demyelination Increases Axonal Stationary Mitochondrial Size and the Speed of Axonal Mitochondrial Transport. J. Neurosci. 30 (19), 6658–6666. doi:10.1523/JNEUROSCI.5265-09.2010
Koike, H., Kadoya, M., Kaida, K. I., Ikeda, S., Kawagashira, Y., Iijima, M., et al. (2017). Paranodal Dissection in Chronic Inflammatory Demyelinating Polyneuropathy with Anti-neurofascin-155 and Anti-contactin-1 Antibodies. Neurol. Neurosurg. Psychiatry 88 (6), 465–473. doi:10.1136/jnnp-2016-314895
Labasque, M., Hivert, B., Nogales-Gadea, G., Querol, L., Illa, I., and Faivre-Sarrailh, C. (2014). Specific Contactin N-Glycans Are Implicated in Neurofascin Binding and Autoimmune Targeting in Peripheral Neuropathies. J. Biol. Chem. 289 (11), 7907–7918. doi:10.1074/jbc.M113.528489
Lang-Ouellette, D., Gruver, K. M., Smith-Dijak, A., Blot, F. G. C., Stewart, C. A., de Vanssay de Blavous, P., et al. (2021). Purkinje Cell Axonal Swellings Enhance Action Potential Fidelity and Cerebellar Function. Nat. Commun. 12 (1), 4129. doi:10.1038/s41467-021-24390-4
Lappe-Siefke, C., Goebbels, S., Gravel, M., Nicksch, E., Lee, J., Braun, P. E., et al. (2003). Disruption of Cnp1 Uncouples Oligodendroglial Functions in Axonal Support and Myelination. Nat. Genet. 33 (3), 366–374. doi:10.1038/ng1095
Ljungberg, L., Lang-Ouellette, D., Yang, A., Jayabal, S., Quilez, S., and Watt, A. J. (2016). Transient Developmental Purkinje Cell Axonal Torpedoes in Healthy and Ataxic Mouse Cerebellum. Front. Cell Neurosci. 10, 248. doi:10.3389/fncel.2016.00248
Louis, E. D., Faust, P. L., Vonsattel, J. P., Honig, L. S., Rajput, A., Rajput, A., et al. (2009). Torpedoes in Parkinson's Disease, Alzheimer's Disease, Essential Tremor, and Control Brains. Mov. Disord. 24 (11), 1600–1605. doi:10.1002/mds.22567
Louis, E. D., Kuo, S. H., Vonsattel, J. P., and Faust, P. L. (2014). Torpedo Formation and Purkinje Cell Loss: Modeling Their Relationship in Cerebellar Disease. Cerebellum 13 (4), 433–439. doi:10.1007/s12311-014-0556-5
Maia, P. D., Hemphill, M. A., Zehnder, B., Zhang, C., Parker, K. K., and Kutz, J. N. (2015). Diagnostic Tools for Evaluating the Impact of Focal Axonal Swellings Arising in Neurodegenerative Diseases And/or Traumatic Brain Injury. J. Neurosci. Methods 253, 233–243. doi:10.1016/j.jneumeth.2015.06.022
Marcus, J., Honigbaum, S., Shroff, S., Honke, K., Rosenbluth, J., and Dupree, J. L. (2006). Sulfatide Is Essential for the Maintenance of CNS Myelin and Axon Structure. Glia 53 (4), 372–381. doi:10.1002/glia.20292
Mikoshiba, K. (2007). IP3 receptor/Ca2+ Channel: from Discovery to New Signaling Concepts. J. Neurochem. 102 (5), 1426–1446. doi:10.1111/j.1471-4159.2007.04825.x
Morfini, G. A., Burns, M., Binder, L. I., Kanaan, N. M., LaPointe, N., Bosco, D. A., et al. (2009). Axonal Transport Defects in Neurodegenerative Diseases. J. Neurosci. 29 (41), 12776–12786. doi:10.1523/JNEUROSCI.3463-09.2009
Nikić, I., Merkler, D., Sorbara, C., Brinkoetter, M., Kreutzfeldt, M., Bareyre, F. M., et al. (2011). A Reversible Form of Axon Damage in Experimental Autoimmune Encephalomyelitis and Multiple Sclerosis. Nat. Med. 17 (4), 495–499. doi:10.1038/nm.2324
Ogawa, Y., Schafer, D. P., Horresh, I., Bar, V., Hales, K., Yang, Y., et al. (2006). Spectrins and ankyrinB Constitute a Specialized Paranodal Cytoskeleton. J. Neurosci. 26 (19), 5230–5239. doi:10.1523/JNEUROSCI.0425-06.2006
Ohno, N., Chiang, H., Mahad, D. J., Kidd, G. J., Liu, L., Ransohoff, R. M., et al. (2014). Mitochondrial Immobilization Mediated by Syntaphilin Facilitates Survival of Demyelinated Axons. Proc. Natl. Acad. Sci. U. S. A. 111 (27), 9953–9958. doi:10.1073/pnas.1401155111
Ohno, N., Kidd, G. J., Mahad, D., Kiryu-Seo, S., Avishai, A., Komuro, H., et al. (2011). Myelination and Axonal Electrical Activity Modulate the Distribution and Motility of Mitochondria at CNS Nodes of Ranvier. J. Neurosci. 31 (20), 7249–7258. doi:10.1523/JNEUROSCI.0095-11.2011
Paillusson, S., Stoica, R., Gomez-Suaga, P., Lau, D. H., Mueller, S., Miller, T., et al. (2016). There's Something Wrong with My MAM; the ER-Mitochondria Axis and Neurodegenerative Diseases. Trends Neurosci. 3939 (3), 146146–157157. doi:10.1016/j.tins.2016.01.008
Peles, E., Nativ, M., Lustig, M., Grumet, M., Schilling, J., Martinez, R., et al. (1997). Identification of a Novel Contactin-Associated Transmembrane Receptor with Multiple Domains Implicated in Protein-Protein Interactions. EMBO J. 16 (5), 978–988. doi:10.1093/emboj/16.5.978
Peters, A. (1966). The Node of Ranvier in the Central Nervous System. Q. J. Exp. Physiol. Cogn. Med. Sci. 51 (3), 229–236. doi:10.1113/expphysiol.1966.sp001852
Pillai, A. M., Garcia-Fresco, G. P., Sousa, A. D., Dupree, J. L., Philpot, B. D., and Bhat, M. A. (2007). No Effect of Genetic Deletion of Contactin-Associated Protein (CASPR) on Axonal Orientation and Synaptic Plasticity. J. Neurosci. Res. 85 (11), 2318–2331. doi:10.1002/jnr.21374
Pillai, A. M., Thaxton, C., Pribisko, A. L., Cheng, J. G., Dupree, J. L., and Bhat, M. A. J. (2009). Spatiotemporal Ablation of Myelinating Glia-specific Neurofascin (Nfasc NF155) in Mice Reveals Gradual Loss of Paranodal Axoglial Junctions and Concomitant Disorganization of Axonal Domains. Neurosci. Res. 87 (8), 1773–1793. doi:10.1002/jnr.22015
Rapizzi, E., Pinton, P., Szabadkai, G., Wieckowski, M. R., Vandecasteele, G., Baird, T. R. A., et al. (2002). Recombinant Expression of the Voltage-dependent Anion Channel Enhances the Transfer of Ca2+ Microdomains to Mitochondria. J. Cell Biol. 159 (4), 613–624. doi:10.1083/jcb.200205091
Rasband, M. N., Peles, E., Trimmer, J. S., Levinson, S. R., Lux, S. E., and Shrager, P. (1999). Dependence of Nodal Sodium Channel Clustering on Paranodal Axoglial Contact in the Developing CNS. J. Neurosci. 19 (17), 7516–7528. doi:10.1523/JNEUROSCI.19-17-07516.1999
Rasband, M. N., Taylor, C. M., and Bansal, R. (2003). Paranodal Transverse Bands Are Required for Maintenance but Not Initiation of Nav1.6 Sodium Channel Clustering in CNS Optic Nerve Axons. Glia 44 (2), 173–182. doi:10.1002/glia.10284
Rios, J. C., Melendez-Vasquez, C. V., Einheber, S., Lustig, M., Grumet, M., Hemperly, J., et al. (2000). Contactin-associated Protein (Caspr) and Contactin Form a Complex that Is Targeted to the Paranodal Junctions during Myelination. J. Neurosci. 20 (22), 8354–8364. doi:10.1523/JNEUROSCI.20-22-08354.2000
Ritchie, J. M., and Rogart, R. B. (1977). Density of Sodium Channels in Mammalian Myelinated Nerve Fibers and Nature of the Axonal Membrane under the Myelin Sheath. Proc. Natl. Acad. Sci. U. S. A. 74 (1), 211–215. doi:10.1073/pnas.74.1.211
Rosenbluth, J., Mierzwa, A., and Shroff, S. (2013). Molecular Architecture of Myelinated Nerve Fibers: Leaky Paranodal Junctions and Paranodal Dysmyelination. Neuroscientist 19 (6), 629–641. doi:10.1177/1073858413504627
Rosenbluth, J. (1999). A Brief History of Myelinated Nerve Fibers: One Hundred and Fifty Years of Controversy. J. Neurocytol. 28 (4-5), 251–262. doi:10.1023/a:1007083409850
Rosenbluth, J. (1976). Intramembranous Particle Distribution at the Node of Ranvier and Adjacent Axolemma in Myelinated Axons of the Frog Brain. J. Neurocytol. 5 (6), 731–745. doi:10.1007/BF01181584
Rosenbluth, J. (2009). Multiple Functions of the Paranodal Junction of Myelinated Nerve Fibers. J. Neurosci. Res. 87 (15), 3250–3258. doi:10.1002/jnr.22013
Saifetiarova, J., and Bhat, M. A. J. (2019). Ablation of Cytoskeletal Scaffolding Proteins, Band 4.1B and Whirlin, Leads to Cerebellar Purkinje Axon Pathology and Motor Dysfunction. Neurosci. Res. 97 (3), 313–331. doi:10.1002/jnr.24352
SalzerZalc, J. L. B. (2016). Myelination. Curr. Biol. 26 (20), R971–R975. doi:10.1016/j.cub.2016.07.074
Satoh, T., Ross, C. A., Villa, A., Supattapone, S., Pozzan, T., Syndey, S. H., et al. (1990). The Inositol 1,4,5-triphosphate Receptor in Cerebellum Purkinje Cells: Quantitative Immunogold Labeling Reveals Concentration in an ER Subcompartment. J. Cell Biol. 111 (2), 615–624. doi:10.1083/jcb.111.2.615
Sherman, D. L., Tait, S., Melrose, S., Johnson, R., Zonta, B., Court, F. A., et al. (2005). Neurofascins Are Required to Establish Axonal Domains for Saltatory Conduction. Neuron 48 (5), 737–742. doi:10.1016/j.neuron.2005.10.019
Sleigh, J. N., Rossor, A. M., Fellows, A. D., Tosolini, A. P., and Schiavo, G. (2019). Axonal Transport and Neurological Disease. Nat. Rev. Neurol. 15 (12), 691–703. doi:10.1038/s41582-019-0257-2
Sorbara, C. D., Wagner, N. E., Ladwig, A., Nikić, I., Merkler, D., Kleele, T., et al. (2014). Pervasive Axonal Transport Deficits in Multiple Sclerosis Models. Neuron 84 (6), 1183–1190. doi:10.1016/j.neuron.2014.11.006
Susuki, K., Otani, Y., and Rasband, M. N. (2016). Submembranous Cytoskeletons Stabilize Nodes of Ranvier. Exp. Neurol. 283 (Pt B), 446–451. doi:10.1016/j.expneurol.2015.11.012
Szabadkai, G., Bianchi, K., Várnai, P., De Stefani, D., Wieckowski, M. R., Cavagna, D., et al. (2006). Chaperone-mediated Coupling of Endoplasmic Reticulum and Mitochondrial Ca2+ Channels. J. Cell Biol. 175 (6), 901–911. doi:10.1083/jcb.200608073
Tait, S., Gunn-Moore, F., Collinson, J. M., Huang, J., Lubetzki, C., Pedraza, L., et al. (2000). An Oligodendrocyte Cell Adhesion Molecule at the Site of Assembly of the Paranodal Axo-Glial Junction. J. Cell Biol. 150 (3), 657–666. doi:10.1083/jcb.150.3.657
Takada, S. H., Ikebara, J. M., de Sousa, E., Cardoso, D. S., Resende, R. R., Ulrich, H., et al. (2017). Determining the Roles of Inositol Trisphosphate Receptors in Neurodegeneration: Interdisciplinary Perspectives on a Complex Topic. Mol. Neurobiol. 54 (9), 6870–6884. doi:10.1007/s12035-016-0205-8
Takagishi, Y., Katanosaka, K., Mizoguchi, H., and Murata, Y. (2016). Disrupted Axon-Glia Interactions at the Paranode in Myelinated Nerves Cause Axonal Degeneration and Neuronal Cell Death in the Aged Caspr Mutant Mouse Shambling. Neurobiol. Aging. 43, 34–46. doi:10.1016/j.neurobiolaging.2016.03.020
Takei, K., Mignery, G. A., Mugnaini, E., Südhof, T. C., and De Camilli, P. (1994). Inositol 1,4,5-trisphosphate Receptor Causes Formation of ER Cisternal Stacks in Transfected Fibroblasts and in Cerebellar Purkinje Cells. Neuron 12, 327–342. doi:10.1016/0896-6273(94)90275-5
Tasaki, I. (1939). The Electro-Saltatory Transmission of the Nerve Impulse and the Effect of Narcosis upon the Nerve Fiber. Am. J. Physiology. 127, 211–227. doi:10.1152/ajplegacy.1939.127.2.211
Teigler, A., Komljenovic, D., Draguhn, A., Gorgas, K., and Just, W. W. (2009). Defects in Myelination, Paranode Organization and Purkinje Cell Innervation in the Ether Lipid-Deficient Mouse Cerebellum. Hum. Mol. Genet. 18, 1897–1908. doi:10.1093/hmg/ddp110
Thai, T. Q., Nguyen, H. B., Sui, Y., Ikenaka, K., Oda, T., and Ohno, N. (2018). Interactions between Mitochondria and Endoplasmic Reticulum in Demyelinated Axons. Med. Mol. Morphol. 52 (3), 135–146. doi:10.1007/s00795-018-0212-0
Trapp, B. D., Peterson, J., Ransohoff, R. M., Rudick, R., Mörk, S., and Bö, L. (1998). Axonal Transection in the Lesions of Multiple Sclerosis. N. Engl. J. Med. 338 (5), 278–285. doi:10.1056/NEJM199801293380502
Vallat, J. M., Yuki, N., Sekiguchi, K., Kokubun, N., Oka, N., Mathis, S., et al. (2017). Paranodal Lesions in Chronic Inflammatory Demyelinating Polyneuropathy Associated with Anti-neurofascin 155 Antibodies. Neuromuscul. Disord. 27 (3), 290–293. doi:10.1016/j.nmd.2016.10.008
Wang, H. J., Guay, G., Pogan, L., Sauvé, R., and Nabi, I. R. (2000). Calcium Regulates the Association between Mitochondria and a Smooth Subdomain of the Endoplasmic Reticulum. J. Cell Biol. 150 (6), 1489–1498. doi:10.1083/jcb.150.6.1489
Watanabe, S., Endo, S., Oshima, E., Hoshi, T., Higashi, H., Yamada, K., et al. (2010). Glycosphingolipid Synthesis in Cerebellar Purkinje Neurons: Roles in Myelin Formation and Axonal Homeostasis. Glia 58 (10), 1197–1207. doi:10.1002/glia.20999
Yin, X., Kidd, G. J., Ohno, N., Perkins, G. A., Ellisman, M. H., Bastian, C., et al. (2016). Proteolipid Protein-Deficient Myelin Promotes Axonal Mitochondrial Dysfunction via Altered Metabolic Coupling. J. Cell Biol. 215 (4), 531–542. doi:10.1083/jcb.201607099
Zambonin, J. L., Zhao, C., Ohno, N., Campbell, G. R., Engeham, S., Ziabreva, I., et al. (2011). Increased Mitochondrial Content in Remyelinated Axons: Implications for Multiple Sclerosis. Brain 134 (Pt 7), 1901–1913. doi:10.1093/brain/awr110
Zhang, C. L., Rodenkirch, L., Schultz, J. R., and Chiu, S. Y. (2012). A Novel Method to Study the Local Mitochondrial Fusion in Myelinated Axons In Vivo. J. Neurosci. Methods 207 (1), 51–58. doi:10.1016/j.jneumeth.2012.03.013
Keywords: paranodal junction, myelin, Purkinje, calcium, IP3R1
Citation: Ishibashi T and Baba H (2022) Paranodal Axoglial Junctions, an Essential Component in Axonal Homeostasis. Front. Cell Dev. Biol. 10:951809. doi: 10.3389/fcell.2022.951809
Received: 24 May 2022; Accepted: 20 June 2022;
Published: 06 July 2022.
Edited by:
Nobuharu Suzuki, Tokyo Medical and Dental University, JapanReviewed by:
Koike Haruki, Nagoya University, JapanCopyright © 2022 Ishibashi and Baba. This is an open-access article distributed under the terms of the Creative Commons Attribution License (CC BY). The use, distribution or reproduction in other forums is permitted, provided the original author(s) and the copyright owner(s) are credited and that the original publication in this journal is cited, in accordance with accepted academic practice. No use, distribution or reproduction is permitted which does not comply with these terms.
*Correspondence: Tomoko Ishibashi, aXNoaWJhc3RAdG95YWt1LmFjLmpw