- 1Department of Biodiversity and Evolutionary Biology, Museo Nacional de Ciencias Naturales, CSIC, Madrid, Spain
- 2Centro de Biología Celular y Molecular, Facultad de Ciencias Exactas, Físicas y Naturales (FCEFyN—UNC), Universidad Nacional de Córdoba, Córdoba, Argentina
- 3Instituto de Investigaciones Biológicas y Tecnológicas, Consejo Nacional de Investigaciones Científicas y Técnicas (IIByT—CONICET, UNC), Córdoba, Argentina
- 4Department of Structural and Chemical Biology, Centro de Investigaciones Biológicas Margarita Salas, CSIC, Madrid, Spain
In mammals, sperm acquire fertilization ability after a series of physiological and biochemical changes, collectively known as capacitation, that occur inside the female reproductive tract. In addition to other requirements, sperm bioenergetic metabolism has been identified as a fundamental component in the acquisition of capacitation. Mammalian sperm produce ATP through two main metabolic processes, oxidative phosphorylation (OXPHOS) and aerobic glycolysis that are localized to two different flagellar compartments, the midpiece, and the principal piece, respectively. In mouse sperm, the occurrence of many events associated with capacitation relies on the activity of these two energy-producing pathways, leading to the hypothesis that some of these events may impose changes in sperm energetic demands. In the present study, we used extracellular flux analysis to evaluate changes in glycolytic and respiratory parameters of murine sperm that occur as a consequence of capacitation. Furthermore, we examined whether these variations affect sperm ATP sustainability. Our results show that capacitation promotes a shift in the usage ratio of the two main metabolic pathways, from oxidative to glycolytic. However, this metabolic rewiring does not seem to affect the rate at which the sperm consume ATP. We conclude that the probable function of the metabolic switch is to increase the ATP supply in the distal flagellar regions, thus sustaining the energetic demands that arise from capacitation.
Introduction
After ejaculation, mammalian sperm must spend a species-specific amount of time in the female reproductive tract in order to acquire the ability to fertilize the ova. During this period, sperm undergo a series of structural, biochemical, physiological, and behavioral changes that have been collectively termed “capacitation” (Austin, 1951; Chang, 1951; De Jonge, 2017). As a consequence of capacitation, sperm become able to orient their movement in response to chemical cues originating in the cumulus-oocyte complex (chemotaxis) (Giojalas et al., 2015; Guidobaldi et al., 2017), and undergo the acrosome reaction, which allows them to penetrate the oocyte vestments and fuse with the oolema (Buffone, 2016; Stival et al., 2016; Nixon and Bromfield, 2018). In addition to these modifications, capacitation is associated with a change in sperm movement pattern, termed “hyperactivation” (Yanagimachi, 1970). Hyperactivated movement is characterized by highly vigorous, asymmetric flagellar beating with increased amplitude (Yanagimachi, 1970; Suarez, 2008; Chang and Suarez, 2011; Goodson et al., 2011), which results in the loss of linear movement in aqueous media but increases progression efficiency in viscous media (Perez-Cerezales et al., 2016).
At the molecular level, sperm capacitation constitutes a highly complex process that includes modifications in the majority of cell components (Gervasi and Visconti, 2016). Capacitation involves hyperpolarization (Zeng et al., 1996; Fraser, 2010) and alterations in the architecture (Harrison, 1996; Visconti et al., 1999; Gadella and Harrison, 2000; Cuasnicú et al., 2016) and composition of the plasma membrane (Zarintash and Cross, 1996; Gadella and Harrison, 2000; Escoffier et al., 2012), increase in the intracellular Ca2+ concentration (Bailey and Storey, 1994; Arnoult et al., 1999), and elevation of intracellular pH (Zeng et al., 1996). This process also involves the activation of lipid signalling pathways (Roldan and Vazquez, 1996), and a soluble adenylyl cyclase with the subsequent increase in the levels of cAMP (Okamura et al., 1985; Garty et al., 1988; Visconti et al., 1990; Fraser, 2010), which in turn activates the cAMP-dependent protein kinase A (PKA) pathway (Harrison and Miller, 2000; Turner, 2006; Fraser, 2010), leading to a substantial increase in the phosphorylation of tyrosine and serine-threonine residues throughout the cell (Visconti et al., 1995a; Harrison, 2004; Visconti, 2009; Fraser, 2010; Krapf et al., 2010).
Previous studies on in vitro sperm capacitation have identified a series of medium components that are key for this process (see (Visconti, 2009; Fraser, 2010; Gervasi and Visconti, 2016) for reviews on the subject). Ions such as HCO3- (Lee and Storey, 1986; Shi and Roldan, 1995; Gadella and Harrison, 2000; Demarco et al., 2003; Harrison and Gadella, 2005) and Ca2+ (Fraser, 1977; Fraser, 1982; Navarrete et al., 2015) are necessary for the activation of the phosphorylation pathways, and a cholesterol acceptor (usually serum albumin) to facilitate the efflux of cholesterol from the membrane (Demarco et al., 2003). More recently, sperm bioenergetic metabolism has been identified as an additional central component in the acquisition of the capacitated status since a number of studies have identified the activity of particular metabolic pathways as necessary for sperm capacitation (see review in (Ferramosca and Zara, 2014)).
In order to sustain flagellar motility, mammalian sperm produce ATP by means of two main metabolic pathways, oxidative phosphorylation (OXPHOS) and aerobic glycolysis (Ford, 2006; Ruiz-Pesini et al., 2007; Storey, 2008), which occur in different cellular compartments. While OXPHOS takes place in the mitochondria located in the midpiece (i.e., the section of the flagellum most proximate to the head), glycolysis takes place in the principal piece, where glycolytic enzymes are associated with the fibrous sheath that surrounds the axoneme (Ford, 2006; Ruiz-Pesini et al., 2007; Storey, 2008; Cummins, 2009). In the laboratory mouse, the activity of both metabolic routes is necessary to maintain vigorous motility (Travis et al., 2001; Narisawa et al., 2002; Goodson et al., 2012; Odet et al., 2013; Takei et al., 2014) since sperm motility decreases to its eventual cessation under inhibition of glycolytic enzymes or absence of glycolytic substrates (Ferramosca et al., 2012; Odet et al., 2013), and alterations of mitochondrial functions are associated to male infertility as a consequence of impaired sperm motility (Narisawa et al., 2002).
Metabolic requirements for sperm capacitation in mammals seem to be species-specific (Cummins, 2009; Ferramosca and Zara, 2014). Numerous studies have revealed that an active glycolytic pathway is necessary for the normal occurrence of mouse sperm capacitation and its associated processes, including fertilization (Hoppe, 1976; Fraser and Quinn, 1981; Cooper, 1984; Urner and Sakkas, 1996; Travis et al., 2001; Urner et al., 2001; Mukai and Okuno, 2004; Travis et al., 2004; Goodson et al., 2012), and mutations that impair the catalytic function of key glycolytic enzymes (Miki et al., 2004; Odet et al., 2008; Danshina et al., 2010; Odet et al., 2011; Odet et al., 2013; Tang et al., 2013) have been found to promote disruptions in sperm capacitation, hyperactivation, and fertilizing ability (Miki et al., 2004; Danshina et al., 2010). These findings support the idea that the physiological events associated with capacitation, such as active ion transport, activation of multiple intracellular signaling pathways, extensive protein phosphorylation, and the vigorous flagellar beating typical of hyperactivation, may impose changes in sperm energetic demand (Travis et al., 2004; Ishijima, 2011).
A previous study from our laboratory (Tourmente et al., 2015a) examined the metabolism of sperm cells in the house mouse and other closely related species in survival (i.e., non-capacitating) conditions using extracellular flux analysis (Agilent-Seahorse XF24). This study revealed that these cells engaged in glycolysis and OXPHOS when glucose was the only substrate in the medium, and that the ratio of usage of each metabolic pathway was species-specific. However, until recently, there was little to no evidence regarding the changes in metabolic rate experienced by mouse sperm during the capacitation process. Two recent studies revealed that mouse sperm increase their glucose uptake rate when exposed to capacitating medium (Hidalgo et al., 2020), or during chemically induced capacitation (Balbach et al., 2020), in response to elevated intracellular cAMP and Ca2+ concentrations (Hidalgo et al., 2020). Furthermore, they showed the elevation of substrate uptake was associated with its utilization to fuel the increased activities of both glycolytic and respiratory pathways (Balbach et al., 2020). Nonetheless, these results only allowed for a limited analysis of the metabolic processes involved in the change from a non-capacitated to a capacitated status, since 1) capacitation was achieved via chemical stimulation with db-cAMP and IBMX in the absence of NaHCO3, and 2) basal respiration and glycolysis rates were not calculated by using combinations of pathway inhibitors and, thus, phenomena such as non-mitochondrial oxygen consumption, proton leakage rate, and non-glycolytic acidification were not contemplated. Finally, there is evidence showing that an increase in mitochondrial membrane potential, which occurs only in capacitated sperm (Yang et al., 2020; Giaccagli et al., 2021), is necessary for the acquisition of the hyperactivated movement pattern. In all, this evidence supports the notion that the bioenergetics of murine sperm capacitation involves changes in both glycolytic and respiratory pathways.
The majority of studies on the relationship between sperm bioenergetics and capacitation have focused on the variations that occur in ATP production mechanisms. It is intuitive to think that higher cellular ATP content would be the immediate consequence of higher ATP production rates. Nonetheless, in order to maintain cellular homeostasis and engage in any metabolic process (capacitation included), there is a need for an equilibrium between ATP synthesis and hydrolysis. Thus, the ratio between ATP production and consumption determines the level of metabolic intensity sperm may achieve and its ability to maintain adequate motility. Comparative studies in muroid rodents showed that the species with high sperm metabolic rates (ATP production) (Tourmente et al., 2015a) can sustain higher ATP consumption rates (Tourmente et al., 2019), and consequently, faster swimming velocities for a longer time (Tourmente et al., 2015b). On the other hand, sperm from other Mus species have been shown to experience a decline in ATP content when incubated under capacitating conditions (Sansegundo et al., 2022), suggesting that the events involved in capacitation may impose ATP demands that even the enhanced metabolic rate of capacitated sperm might not be able to sustain for a long time.
In the present study, we used extracellular flux analysis (hereafter EFA) to evaluate which changes in the glycolytic and respiratory parameters of murine sperm occur as a consequence of capacitation. Furthermore, we examined the variations in ATP sustainability that may be imposed by capacitation. We show that capacitation promotes a shift in the usage ratio of the main metabolic pathways, from oxidative to glycolytic, probably as a manner to maintain local ATP concentration in the distal flagellar regions.
Materials and methods
Reagents
Unless stated otherwise, reagents were purchased from Merck (Madrid, Spain).
Animals, sperm collection, and incubation
B6D2F1 adult male mice (3–4 months old) were purchased from Envigo (Barcelona, Spain). The animals were kept in the facilities of the Museo Nacional de Ciencias Naturales (Madrid) in individual cages under controlled temperature (20–24°C) on a 14/10 h light/darkness photoperiod, and supplied with water and food ad libitum. Care and maintenance of the mice used in this study followed the Royal Decree on Protection of Experimental Animals RD53/2013 and the European Union Regulation 2010/63, and had the approval of CSIC’s ethics committee and the Comunidad de Madrid (28079-47-A). Mice were sacrificed by cervical dislocation, which is considered a humane method by Spanish and European regulations. No procedures other than sacrifice for the collection of gametes were performed.
After sacrifice, mice were dissected and their caudae epididymides excised. The blood vessels, fat, and surrounding connective tissue were removed from the caudae. Each cauda epididymis was placed in a 35 mm Petri dish containing 0.4 ml of one of two variants of culture medium pre-warmed to 37°C. One cauda epididymis was placed in non-capacitating medium under air and the other one was placed in capacitating medium under 5% CO2/air. The compositions of both media were based on a Hepes-buffered modified Tyrode’s medium (Shi and Roldan, 1995), supplemented with albumin, lactate, and pyruvate (pH = 7.4, osmolality = 295 mOsm kg−1). The composition of the non-capacitating medium was: 132 mM NaCl, 2.68 mM KCl, 0.49 mM MgCl2.6H2O, 0.36 mM NaH2PO4.2H2O, 5.56 mM glucose, 20 mM HEPES, 1.80 mM CaCl2, 0.02 mM phenol red, 0.09 mM kanamycin, 4 mg ml−1 fatty acid-free BSA, 20 mM Na lactate, and 0.5 mM Na pyruvate. The capacitating medium differed from the non-capacitated medium in that 15 mM NaHCO3 was added and NaCl adjusted to maintain the osmolality.
Three to five incisions were performed in the distal region of the caudae, and sperm were allowed to swim out for 5 min (Sansegundo et al., 2022). For most experiments (see below), sperm from two mice were used in each experiment. One epididymis from each of two males was placed in a Petri dish (total of two per dish) and the sperm of both was mixed during swim out. After swim out, the epididymal tissue was discarded and the sperm suspension was transferred to a plastic tube under a suitable atmosphere. Sperm concentrations were estimated for each sperm suspension by using a modified Neubauer chamber and adjusted to 100 × 106 sperm ml−1 with the corresponding medium. Concentration-adjusted sperm suspensions were then incubated for 1 h at 37°C under suitable atmospheres since, after such incubation time, the mouse sperm population tends to reach the peak of capacitated cells (Sansegundo et al., 2022). In all procedures, large-bore pipette tips were used to minimize mechanical damage to spermatozoa.
In the case of sperm ATP consumption measurements, only one male was used in each experiment. Thus, sperm from one cauda epididymis was collected in each medium. In this case, the sperm concentration of each suspension was adjusted to 20 × 106 sperm ml−1 prior to the 1-h incubation time.
Sperm capacitation
Sperm capacitation status was assessed at the end of the 1 h incubation period, and prior to extracellular flux analysis. Capacitation was assessed using chlortetracycline (CTC, C4881) [73] combined with a vital stain (Ward and Storey, 1984; Sansegundo et al., 2022). Briefly, after a 1 h incubation under different conditions, 100 μl of the sperm suspension were mixed with 50 μl of 6 μg/ml Hoechst 33258 bisbenzamide (B2883) and incubated for 1 min in the dark. Subsequently, the samples were centrifuged for 2 min at 100 ×g, the supernatant was discarded, the pellet resuspended in 100 μl of medium and fixed with an equal volume of 2% glutaraldehyde-0.165 M sodium cacodylate solution. Immediately before evaluation, an aliquot of 20 μl of sperm suspension was added to 20 μl of 250 μM CTC solution and incubated in the dark for 3 min. Spermatozoa were observed at 1,000× magnification under fluorescence and phase-contrast microscopy simultaneously (E-600 microscope, Nikon). Pre-fixation viability of the spermatozoa was assessed using a Nikon UV-2A 330-nm filter and fluorescence emission via a DM 400 dichroic mirror. Only the cells that excluded the Hoechst stain were considered viable. CTC staining patterns were observed using a Nikon BV-2A 405-nm filter and fluorescence emission with a DM 455 dichroic mirror, and the following patterns were distinguished (Sansegundo et al., 2022): 1) F (non-capacitated sperm): the head of spermatozoa was uniformly stained with CTC, 2) B (capacitated sperm): the post-acrosomal region of the head was not stained with CTC, and 3) AR (without the sperm acrosome): the head of the sperm cell showed little or no CTC staining. Capacitation status was evaluated in 100 viable spermatozoa with intact acrosomes per sample and expressed as the percentage of sperm showing staining pattern “B.”
Assessment of sperm metabolic rates
An XFp extracellular flux analyzer (Agilent Seahorse, Santa Clara, CA) was used to measure oxygen consumption rate (OCR) and extracellular acidification rate (ECAR) in real-time. In this system, OCR indicates the level of respiratory activity in a population of cells, and ECAR, an estimate of the rate of lactate release to the medium, is used as a proxy for aerobic glycolytic activity. After 1 h incubation in differential conditions (i.e., capacitation period), the cells were transferred to an 8-well XFp plastic microplate. As a substantial difference from the original method developed by Tourmente et al. (Tourmente et al., 2015a), sperm were not attached to the bottom of the well. Thus, measurements were obtained from a free-swimming sperm population. This required an increase in the number of sperm added to each well in order to ensure that the 2 µl closed micro-chamber, formed between the sensor and the bottom of the plate when the instrument is measuring, contained enough cells to detect a reliable signal. Three wells were seeded with 80 µl of sperm suspension (approximately 8 × 106 sperm) incubated under non-capacitating conditions, other three wells were seeded with sperm suspension incubated under capacitating conditions, and two wells were left without cells to perform background corrections. The plate was centrifuged for 1 min at x 1,300 g in each direction to temporarily set the cells at the bottom of the well. The supernatant was removed from each well and 200 µl of unbuffered assay medium was added to the 8 wells of the plate, thus resuspending the cells. Since the presence of a pH buffer in the medium would impede an accurate measurement of ECAR, an unbuffered assay medium was used for the measurements and dilution of the metabolic modulators. In this medium, Hepes and NaHCO3 were replaced by NaCl to preserve osmolality, and pH was adjusted to 7.4 at 37°C. After medium replacement, the plate with the sperm was placed in the XFp, and a 12 min equilibration step was allowed before measurements.
OCR and ECAR were measured at 37°C and the measurement cycle consisted of 2 min of mixing, 1 min of waiting, and 3 min of measuring. In the first set of experiments (n = 4), we assessed whether stable measurements of sperm metabolism could be obtained from mouse sperm samples before the addition of any compound. Thus, OCR and ECAR were recorded for 48 min (8 cycles). Subsequently, 1 µM rotenone and 1µM antimycin A (inhibitors of the mitochondrial respiratory chain complexes I and III respectively) were added to the wells, and both metabolic variables were monitored for an additional 12 min (10 cycles, 60 min). Oxygen levels showed a marked descent during the measurement phase that fully recovered during the mixing and waiting phases (Supplementary Figure S1), indicating that the sperm concentration used in the experiment did not lead to hypoxia.
For the second set of experiments, we measured sperm metabolism both before and after the addition of metabolically active compounds, in order to calculate a range of metabolic parameters. OCR and ECAR were measured for 36 min (6 cycles) to establish a baseline value (hereafter “basal”). Subsequently, one of three metabolic modulators was added to the wells, and sperm metabolism was recorded for 3 additional cycles (18 min). Metabolic modulators were: 1) 5 μM oligomycin A, an inhibitor of the mitochondrial ATP synthase, 2) 1 µM carbonyl cyanide p-trifluoro-methoxyphenylhydrazone (FCCP), an uncoupler of mitochondrial OXPHOS, and 3) 50 mM 2-deoxy-d-glucose (2DOG), a glucose analog that competitively inhibits the first step of glycolysis. Finally, 1 µM rotenone and 1 µM antimycin A were added to the wells for two final measurements (11 cycles, 66 min). Oligomycin and FCCP were used in four separate experiments, while 2DOG was used in three separate experiments (total n = 11 for the second set). For all experiments, the first two measurements were discarded since, according to our previous experience, these tend to show a higher degree of instability. Thus, the first OCR and ECAR values considered for this study were taken 12 min after the beginning of the measurements.
At the end of the experiment, the sperm suspension in each well was mixed by pipetting, and 10 µl was collected to estimate sperm concentration using a modified Neubauer chamber. Sperm numbers in the wells were calculated and the OCR and ECAR values for each well were normalized by the number of sperm present in the 2 µl measurement volume (reported as amol of O2 min−1 sperm−1, and nano-pH min−1 sperm−1, respectively).
Calculation of metabolic parameters
The addition of the metabolic modulators used in the second set of EFAs allowed us to calculate several metabolic parameters (Supplementary Table S1) that have more informative value than the raw normalized measurements. They also allowed for a more accurate comparison between the two incubation conditions (Marinangeli et al., 2018; Zhao et al., 2020). Metabolic parameters were calculated for each well as the average value of the measurements taken for that well in condition A minus the average value of the measurements taken for that well in condition B (see Supplementary Table S1 for a description of the conditions and Supplementary Figure S2 for a graphical representation). Values corresponding to the basal state (no additions) were averaged across measurement cycles 3-6, values corresponding to oligomycin and 2DOG additions were averaged across cycles 7-9, and values corresponding to A + R additions were averaged across cycles 10-11. Exceptionally, for the calculation of the stimulating effects of FCCP and Oligomycin on OCR and ECAR respectively, the highest value among cycles 7-9 was used instead of the average.
In order to facilitate comparisons with previous and future studies, we also calculated the response of OCR and ECAR to metabolic modulators as a percentage of the averaged baseline values (measures 3-6) for each well. In the case of OCR, both the baseline and the stimulated/inhibited values were first corrected by subtracting the average of the measures after the addition of A + R (cycles 10-11).
Sperm ATP content
A separate set of experiments (n = 5) was performed in order to compare sperm ATP consumption rates between sperm incubated in the different conditions. After 50 min of incubation under capacitating or non-capacitating conditions, each sperm suspension was divided into two aliquots. The first sperm aliquot (hereafter “control”) was used to monitor basal ATP content throughout the consumption experiment. Sperm ATP content was assessed in this aliquot at 55 and 70 min of incubation. A combination of inhibitors of OXPHOS (1 μM antimycin A, 1 μM rotenone, and 5 μM oligomycin) and glycolysis (50 mM 2DOG) were diluted in the corresponding medium and added to the second aliquot (hereafter “inhibition” treatment) after 60 min of incubation in order to fully inhibit both ATP production pathways, thus making sperm able to consume ATP without producing it (Tourmente et al., 2019). Sperm ATP content was assessed in the inhibition treatment immediately after the addition of inhibitors and after 2, 4, 6, 8, and 10 min. Although the addition of rotenone and antimycin A would suffice to stop OXPHOS-derived ATP production, oligomycin was used to prevent an artefactual ATP depletion due to the reverse action of the mitochondrial ATP synthase [55]. Since OXPHOS inhibitors were dissolved in DMSO prior to the addition to the sperm suspension, an equivalent concentration (0.7% v/v) of this solvent was added to the control treatment before the first ATP content assessment.
Sperm ATP content was measured using a luciferase-based ATP bioluminescent assay kit (Roche, ATP Bioluminescence Assay Kit HS II) based on the protocol of Tourmente et al. (Tourmente et al., 2019). Sperm suspension was diluted 1:10 in the corresponding medium, and a 100 μl aliquot of diluted sperm suspension was mixed with 100 μl of Cell Lysis Reagent and frozen in liquid N2. At the end of the experiment, all the aliquots were thawed at room temperature for 5 min. The resulting cell lysate was centrifuged at 12,000 × g for 2 min, and the supernatant was recovered and frozen in liquid N2. Bioluminescence was measured in triplicate in 96-well plates using a luminometer (Biotek Synergy, Biotek Instruments Inc.). Auto injectors were used to add 50 μl of luciferase reagent to 50 μl of sample in each well, and, following a 1 s delay, light emission was measured over a 5 s integration period. Standard curves were constructed using ATP standards diluted with the corresponding media and Cell Lysis Reagent in a proportion equivalent to that of the samples. ATP content was expressed as amol sperm−1.
Statistical analyses
All analyses were conducted using R version 4.1.3 (R Foundation for Statistical Computing, Vienna, Austria), with α = 0.05. To assess whether incubation conditions affected the percentage of capacitated sperm, we applied a generalized linear mixed effect model (GLMM, mixed function, afex package) with binomial distributions and “logit” link function, including incubation conditions as a fixed factor with 2 levels (non-capacitating vs capacitating), and experiment as random factor. In the first set of EFAs the stability of the OCR and ECAR measures during the assay was assessed via linear mixed effect models (LMM, mixed function, afex package) using time as a fixed factor with 8 levels (one per measurement cycle), and experiment as random factor. In the second set of EFAs, we analyzed the effect of incubation conditions and metabolic modulators on OCR and ECAR expressed as percentages relative to the baseline using LMMs with incubation medium and treatment (basal, oligomycin, FCCP, 2DOG, and A + R) as fixed factors, and experiment as random factor. The inclusion of the experiment as a random factor allowed for the consideration of each well as an individual value in the statistical analyses, thus decreasing the impact of outliers and increasing the statistical powers of the tests by controlling for the between-experiment variability (Yepez et al., 2018). The values of the metabolic parameters calculated after EFAs were compared between incubation conditions using LMMs with incubation medium as a fixed factor and experiment as a random factor.
Non-linear regression models (ngls function, nlme package) were used to estimate sperm ATP consumption rates for sperm incubated in capacitating and non-capacitating conditions and subsequently treated with inhibitors. First, a general regression curve (ATP = a x eb x time), based on the values of ATP content at the six sampling time points, was estimated for each condition using the pooled measurements of the four experiments. The parameter “b” was defined as the ATP consumption rate, that is, the exponential rate of decline in ATP content as a function of time. In addition, a second ATP consumption rate was estimated for each individual sample and the “b” exponents of the curves were compared between conditions by means of an LMM with experiment as random factor.
All variables were log10-transformed for statistical purposes in all analyses with the exception of the values expressed as percentages, and the estimated ATP consumption rates. Significant differences between levels of fixed factors were analyzed via post-hoc estimated marginal means tests (pairwise function of the emmeans package).
Results
After 1 h of incubation post collection, approximately 50% of the live sperm incubated in capacitating conditions presented the pattern “B” of CTC staining. On the other hand, only 13% of the live sperm showed such pattern when incubated under non-capacitating conditions, confirming that incubation in capacitating medium promoted a significant increase in the percentage of capacitated sperm in the samples (mean ± SE: 51.5 ± 1.59 vs. 13.17 ± 1.59; GLMM: Χ2 = 428.52, p < 0.001; Figure 1).
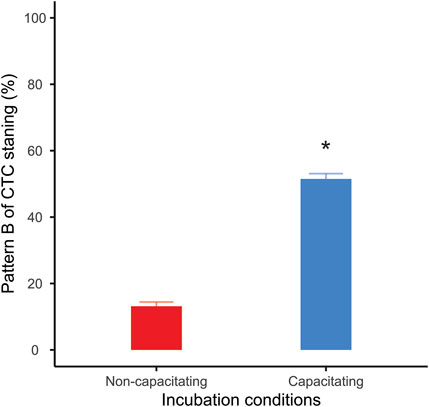
FIGURE 1. Percentage of live capacitated sperm (CTC pattern “B”) after one hour of incubation. Bars correspond to means +standard error. Red bar: sperm incubated in non-capacitating conditions; blue bar: sperm incubated in capacitating conditions. Asterisk indicates significant differences (p < 0.05) in percentages between incubation media.
The first set of EFAs showed that experimental time had no significant effects on OCR values before A + R addition in both incubation conditions (non-capacitating: LMM Χ2 = 4.13, p = 0.531; capacitating: LMM Χ2 = 2.56, p = 0.768); Supplementary Figures S3A,C). Similarly, experimental time did not have a significant effect on ECAR for any of the two incubation conditions (non-capacitating: LMM Χ2 = 1.59, p = 0.903; capacitating: LMM Χ2 = 8.66, p = 0.123; Supplementary Figures S3B,D). These results indicate that the modification applied to the EFA technique allowed us to obtain stable OCR and ECAR values for at least 54 min after introducing the samples into the XFp analyzer.
The OCR and ECAR profiles for the second set of EFAs in both incubation conditions are shown in Figure 2. In both non-capacitating and capacitating sperm, cells responded as expected to the injection of metabolically active compounds (Figure 2; Table 1). Thus, OCR significantly increased when exposed to the uncoupler FCCP, decreased when exposed to the ATP synthase inhibitor oligomycin, and further diminished when exposed to antimycin and rotenone (Figures 2A,B; Table 1). Furthermore, sperm OCR was reduced when exposed to the glycolytic inhibitor 2DOG. ECAR values decreased as expected in response to the addition of 2DOG in both incubation conditions, and increased when respiration was inhibited by the addition of oligomycin (Figures 2C,D; Table 1).
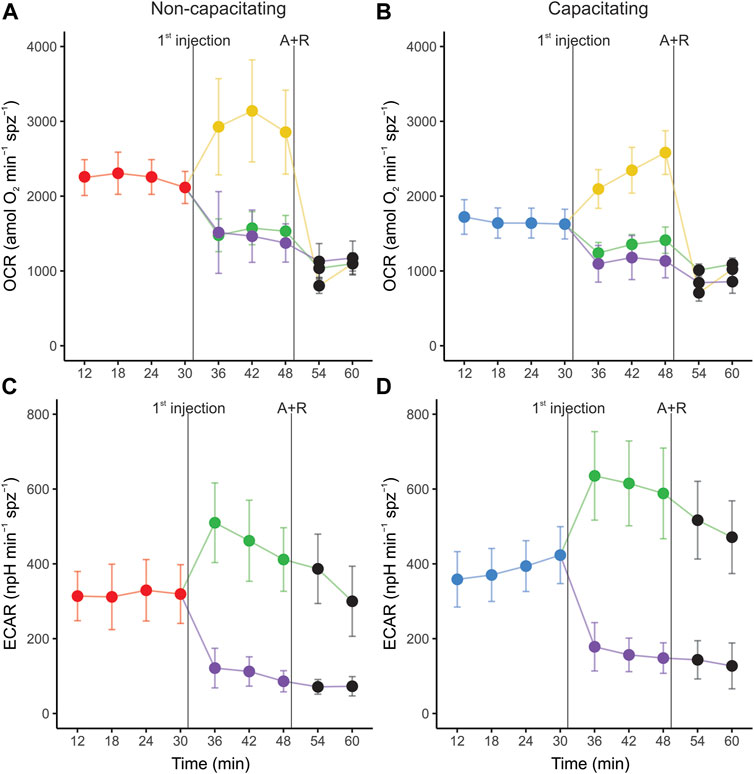
FIGURE 2. Real-time measurements of oxygen consumption rate (OCR) (A,C) and extracellular acidification rate (ECAR) (B,D) in mouse sperm before and after treatment with metabolic modulators. Sperm were incubated for 1 h in non-capacitating (A,C) and capacitating (B,D) conditions prior to extracellular flux analysis. Values have been normalized by sperm numbers inside each well. Symbols and whiskers correspond to means ± standard error. Time = 0 was defined as the start of the first measurement cycle; measurement cycles 3 to 11 are reported. The line labeled as “1st injection” marks the addition of either 5 µM oligomycin, 1 µM FCCP, or 50 mM 2DOG; the line labeled “A + R” marks the addition of 1 µM antimycin A+ 1 µM rotenone. Red and blue symbols: values before any addition (basal state) in non-capacitating and capacitating conditions respectively; green symbols: after oligomycin injection; yellow symbols: after FFCP injection; purple symbols: after 2DOG injection; black symbols: after A + R injection.
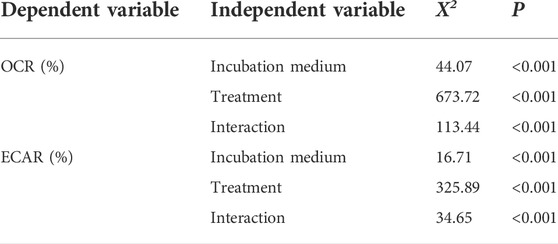
TABLE 1. Effect of incubation conditions and metabolic modulators on mouse sperm OCR and ECAR. Independent variables were tested as percentages relative to the basal state. Χ2 and p values were estimated using LMMs and likelihood ratio tests. Incubation medium (non-capacitating or capacitating) and treatment (basal, 5 µM oligomycin, 1 µM FCCP, 50 mM 2DOG, and 1 µM antimycin +1 µM rotenone) were used as fixed factors and experiment as random factor. Significant differences (p < 0.05) are shown in boldface.
Sperm populations incubated under both conditions showed qualitatively similar responses to metabolic modulators when OCR and ECAR were expressed as percentages relative to the basal state. Exposure to oligomycin caused a reduction of approximately 71% in OCR (Figures 3A, 4A, Table 1). The addition of 2DOG promoted a decline in OCR that was similar to that produced by exposure to oligomycin (∼70%) (Figures 3C, 4A; Table 1) and a decrease of approximately 60% in ECAR (Figures 3E, 4B; Table 1). On the other hand, some treatments elicited different responses in non-capacitating and capacitating sperm. The increase in OCR associated to the addition of FCCP was significantly higher in sperm incubated under capacitating conditions (319 % vs. 192%) (Figures 3B, 4A; Table 1). Also, the rise in ECAR as a response to oligomycin in sperm incubated under capacitating conditions surpassed that of sperm incubated in non-capacitating conditions (90 % vs. 60%) (Figures 3D, 4B; Table 1).
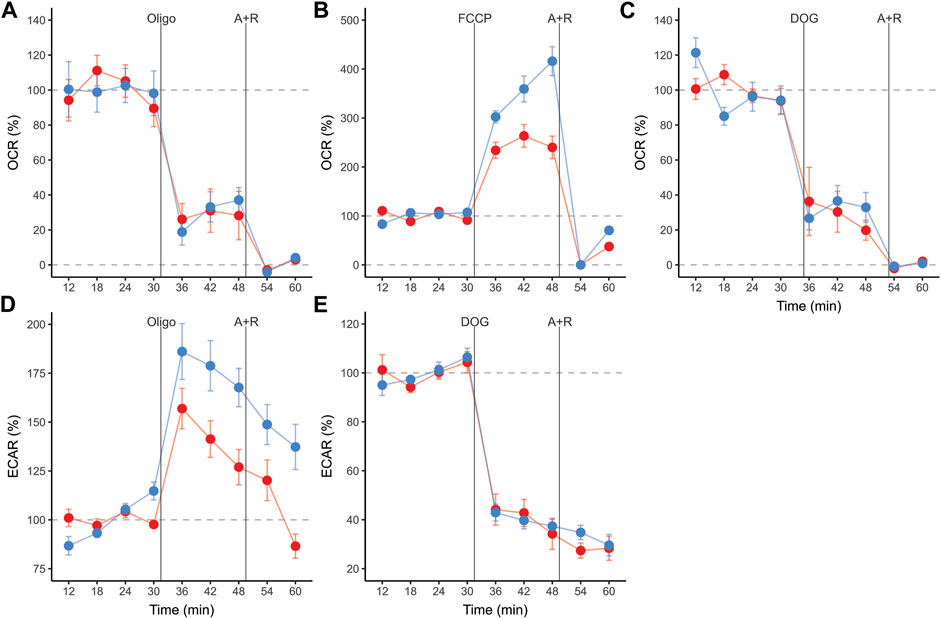
FIGURE 3. Real-time measurements of oxygen consumption rate (OCR) (A,B,C), and extracellular acidification rate (ECAR) (D,E) in mouse sperm before and after treatment with metabolic modulators. Values are expressed as percentages relative to the basal state (100%), which was defined as the mean of the four measurement cycles before any addition and is represented by the horizontal dashed line. Sperm were incubated for 1 h in non-capacitating (red symbols) and capacitating (blue symbols) conditions prior to extracellular flux analysis. Symbols and whiskers correspond to means ± standard error. Time = 0 was defined as the start of the first measurement cycle; measurement cycles 3 to 11 are reported. Dashed grey lines indicate 100 % and 0% levels. The first vertical line marks the addition of either 5 µM oligomycin, 1 µM FCCP, or 50 mM 2DOG; the line labeled “A + R” marks the addition of 1 µM antimycin A+ 1 µM rotenone.
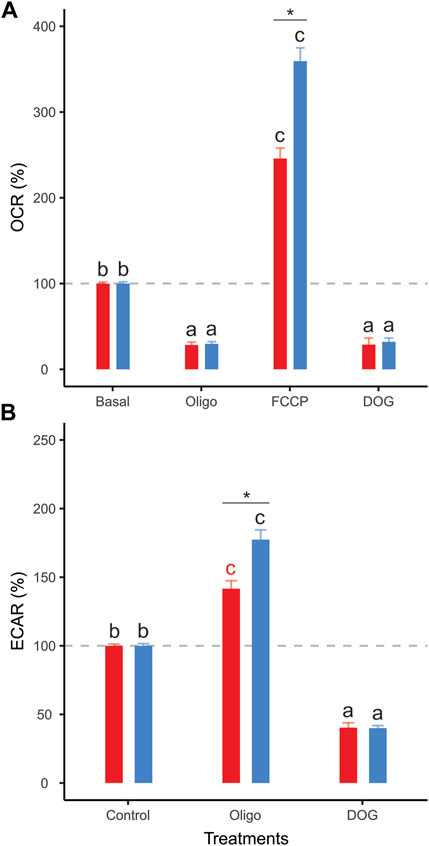
FIGURE 4. Effect of metabolic modulators on mouse sperm OCR (A), ECAR (B) expressed as percentages relative to the basal state. Sperm were incubated in non-capacitating (red bars) and capacitating (blue bars) conditions prior to extracellular flux analysis. Bars correspond to means +standard error. Treatments represent sperm metabolic rates before any addition (Basal) or after the addition of 5 µM oligomycin (Oligo); 1 µM FCCP (FCCP); 50 mM 2DOG (DOG); or 1 µM antimycin A+ 1 µM rotenone (A + R). Dashed grey lines indicate 100% level. Different letters indicate significant differences (p < 0.05) between treatments for the same incubation condition in a post-hoc marginal means test. Asterisks indicate significant differences (p < 0.05) between incubation conditions for the same treatment in a post-hoc marginal means test.
Clear differences between incubation conditions emerged when the OCR and ECAR measurements were used to calculate and compare a range of metabolic parameters (Supplementary Table S1). Sperm incubated under non-capacitating conditions presented higher values in nearly all parameters associated with respiratory metabolism (basal respiration rate, proton leak, respiratory ATP production, and maximum respiration rate) (Figures 5A–D), with the exception of spare respiratory capacity, which did not show any significant differences between incubation conditions (Figure 5E). It should be noted that FCCP-stimulated OCR did not stabilize within the three measurements taken in sperm incubated in capacitating conditions. Thus, there is the possibility that maximum respiration rate and spare respiratory capacity are underestimated in these cells. Conversely, the two parameters associated with aerobic glycolysis (basal glycolysis rate and glycolytic reserve) showed higher values in sperm incubated under capacitating conditions (Figures 5G,H). The OCR/ECAR ratio supported this trend since it was significantly higher in the sperm incubated in non-capacitated conditions (Figure 5F). In all, these results confirm the occurrence of a switch in the usage ratio of the two energy supply pathways as a consequence of sperm capacitation.
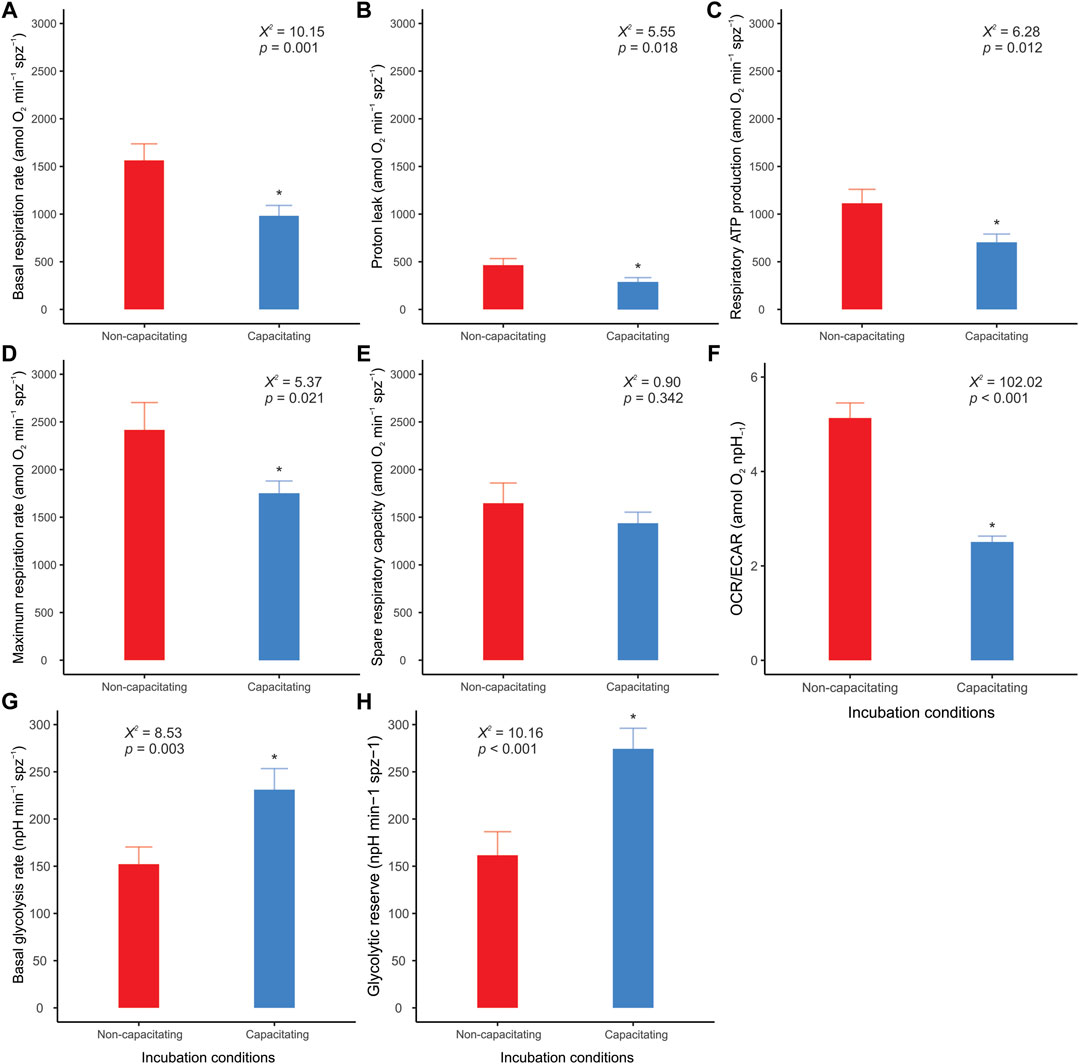
FIGURE 5. Effect of incubation conditions on mouse sperm metabolic parameters and OCR/ECAR ratio. Sperm were incubated in non-capacitating (red bars) and capacitating (blue bars) conditions prior to extracellular flux analysis. (A) Basal respiration rate; (B) Proton leak; (C) Respiratory ATP production; (D) Maximum respiration rate; (E) Spare respiratory capacity; (F) OCR/ECAR ratio of basal state (before any additions); (G) Basal glycolysis rate; (H) Glycolytic reserve. Bars correspond to means + standard error. Asterisks indicate significant differences (p < 0.05) between sperm incubated in non-capacitating and capacitating conditions. X2 and p values were estimated using LMMs and likelihood ratio tests.
Incubation conditions did not produce significant variations in the ATP content of sperm that had not been treated with metabolic inhibitors (LMM Χ2 = 0.45, p = 0.503). However, these cells showed a slight (∼20%) but significant reduction in ATP content throughout the experimental time (Figure 6A) (LMM Χ2 = 5.60, p = 0.018). Conversely, sperm incubated in the presence of inhibitors showed a severe and sustained decrease in ATP content over time in both incubation conditions (Figure 7A). Non-linear regression analyses performed by pooling the measurements of the five individuals yielded ATP consumption curves that significantly adjusted to the “ATP = a x eb x time” model in both incubation conditions (non-capacitating: ATP = 37.09 x e−0.21 x time, Fb = 50.25, pb < 0.001; capacitating: ATP = 30.50 x e−0.19 x time, Fb = 32.33, pb < 0.001) (Figure 6B). In order to compare ATP consumption between incubation conditions, we estimated ATP consumption curves for each individual in each condition and compared the “b” exponents of the curve. There were no significant differences (LMM Χ2 = 0.15, p = 0.712) between the values of the exponents from curves estimated for non-capacitating (b = −0.20 ± 0.02) and capacitating conditions (b = −0.19 ± 0.03).
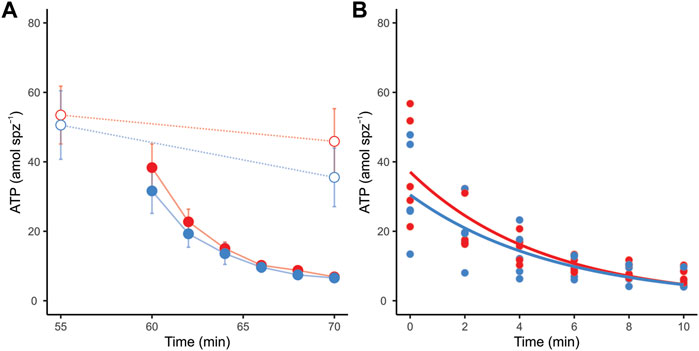
FIGURE 6. ATP consumption in mouse sperm in non-capacitating (red symbols and lines) and capacitating (blue symbols and lines) conditions. (A) ATP content in response to the addition of metabolic inhibitors to sperm in both incubation conditions. Dotted lines and open symbols represent control conditions (no inhibitors added); continuous lines and filled symbols represent metabolically inhibited sperm (a combination of 5 µM oligomycin, 50 mM 2DOG, 1 µM antimycin A, and 1 µM rotenone was added). (B) Lines represent estimated sperm ATP consumption curves of metabolically inhibited sperm in non-capacitating (ATP = 37.09 x e−0.21 x time) and capacitating (ATP = 30.50 x e−0.19 x time) conditions. Symbols represent individual measurements.
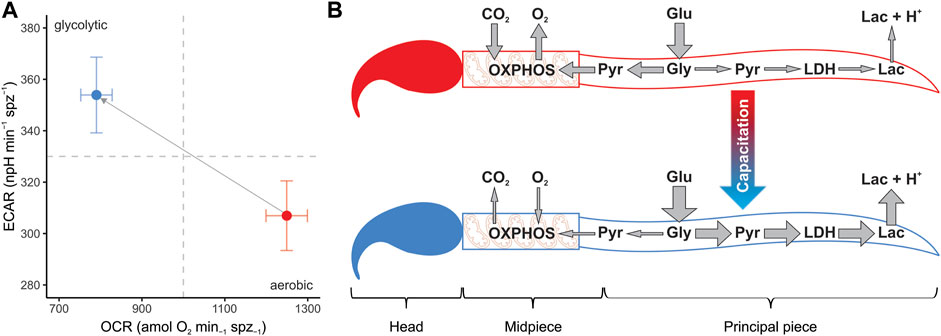
FIGURE 7. Changes in metabolic phenotype promoted by sperm capacitation in murine sperm. (A) Metabolic phenotype plot of mouse sperm depicting the usage of the two main metabolic pathways (OXPHOS and anaerobic glycolysis). Symbols and whiskers correspond to means ± standard error. Red symbols: non-capacitating conditions. Blue symbols: capacitating conditions. Dashed grey lines indicate the midpoint in the measurement range for both variables. (B) Graphical depiction of conclusions. Capacitation promotes a metabolic shift in the mouse sperm, increasing the uptake of glucose and altering the proportion of pyruvate that enters each metabolic pathway. A greater proportion of pyruvate is reduced into lactate by the enzyme lactate dehydrogenase and transported to the extracellular environment along with protons, while a relatively minor proportion is transported to the mitochondria in the midpiece, thereby entering the OXPHOS pathway. Lac: lactate. LDH: lactate dehydrogenase. Pyr: pyruvate. Gly: glycolysis. Glu: glucose.
Discussion
The results of our study revealed that the development of capacitation in mouse sperm is associated with changes in the energy metabolism of these cells. In the present study, sperm capacitation was achieved through the incubation of spermatozoa for 1 h in a medium containing BSA, Ca2+, and NaHCO3. Sperm capacitation was confirmed by CTC staining in live cells. These incubation period and medium components necessary for murine sperm capacitation have been described in previous studies (Shi and Roldan, 1995; Visconti et al., 1995a; Visconti et al., 1995b; Sansegundo et al., 2022), and the changes in CTC staining patterns have been shown to strongly correlate to markers of sperm capacitation, such as progesterone-induced acrosome reaction (Gomendio et al., 2006) and hyperactivated movement (Sansegundo et al., 2022). Thus, we can confidently infer that the observed rise in CTC pattern “B" after incubation in capacitating conditions was indicative of an increased proportion of capacitated cells within the sample. Moreover, sperm populations incubated under capacitating conditions are a mix of capacitated and non-capacitated cells and, thus, the metabolic variations observed as a consequence of sperm capacitation are most likely an underestimation since they are attenuated by the presence of non-capacitated sperm.
In the present study, the increase in the proportion of capacitated cells in the sperm populations was associated with a change in their metabolic phenotype (Figure 7A). Parameters associated with respiratory metabolism (basal respiration, proton leak, respiratory ATP production, and maximal respiration) presented higher values in sperm populations with a low percentage of capacitated cells. Conversely, sperm populations enriched in capacitated sperm relied more on glycolysis, as evidenced by their higher basal glycolysis and glycolytic reserve values. This change in metabolic profile is summarized in the OCR/ECAR ratio, which decreases by 50% after incubation under capacitating conditions. It should be borne in mind that the magnitude of this metabolic switch may be underestimated by the nature of the measuring system (i.e., using ECAR as a proxy of the rate of lactate formation) since HCO3− formation, derived from the production of CO2 via OXPHOS, will contribute to total ECAR in variable proportions depending on cell type and substrate (Mookerjee et al., 2015). In our study, the decrease in sperm O2 consumption (OXPHOS) associated with capacitation leads to a stoichiometric reduction in CO2 production. Thus, the increase in ECAR from lactate excretion (glycolysis), which results from the metabolic switch associated with capacitation, is partially attenuated because the CO2 contribution has diminished.
Sperm incubated in both media (capacitating and non-capacitating), reacted as expected to the addition of metabolic modulators. While OCR decreased in response to oligomycin or antimycin plus rotenone and increased in response to FCCP, ECAR was reduced when sperm were exposed to 2DOG. These results allowed us to validate the robustness of the technique, and to calculate, for the first time in mouse sperm, a range of relevant metabolic parameters that allow for a comparison between studies and species. Importantly, this is, to the best of our knowledge, the first study to achieve robust metabolic measurements using XF EFA technology in freely moving sperm, since previous work using these techniques necessitated the use of attached cells (Tourmente et al., 2015a; Magdanz et al., 2019; Balbach et al., 2020).
Whereas the metabolism of mouse sperm responded similarly to metabolic modulation regardless of incubation conditions, there were quantitative differences in the level of these responses associated with capacitation. The increased elevation of glycolytic rate by capacitated sperm in response to OXPHOS inhibition (glycolytic reserve) was caused by a more pronounced reaction from a higher basal glycolytic rate. On the other hand, the proportionally higher stimulation of the respiratory chain exhibited by sperm in capacitating medium was a consequence of their decreased basal respiration rate. This evidence further supports the notion of a metabolic rewiring of capacitated spermatozoa in favor of aerobic glycolysis over OXPHOS.
A recent EFA study on mouse sperm (Balbach et al., 2020) revealed an increase in rates of OXPHOS and aerobic glycolysis that were both concomitant with the emergence of capacitation markers, and with an increase in glucose uptake. Although at first hand, the observations in this earlier study (Balbach et al., 2020) seem contradictory to our results, there are important differences between the experimental designs of both studies that make comparisons difficult. The measurements in Balbach et al. (Balbach et al., 2020) were obtained by subjecting sperm to capacitating conditions within the extracellular flux analyzer, with capacitation being stimulated pharmacologically via the addition of a cAMP analog (db-cAMP) and a phosphodiesterase inhibitor (IBMX) in the absence of NaHCO3. In our case, sperm were preincubated for 1 h under capacitating conditions (including NaHCO3) before placing them in the analyzer, and extracellular flux measurements were performed after sperm capacitation had occurred in a high proportion of cells in the population. Although pharmacological elevation of cAMP levels is sufficient to promote the protein phosphorylation events associated with capacitation in mouse sperm (Visconti et al., 1995a; Visconti et al., 1995b; Balbach et al., 2020), the absence of HCO3− might affect other capacitation-related processes that depend on the concentration of this ion (Zeng et al., 1996; Demarco et al., 2003; Guerrero et al., 2011; Nishigaki et al., 2014), such as membrane hyperpolarization, intracellular pH increase, and hyperactivation, and could impact the activity of metabolic pathways (Kamp et al., 2003; Mannowetz et al., 2012; Shahrokhi et al., 2020). In addition, the effects of sperm capacitation on sperm metabolism found in the present study are smaller in magnitude than those reported by Balbach et al. (2020). The changes in metabolic activity following pharmacologically-stimulated capacitation ranged between 5 and 7-fold (for OCR) and 4-5-fold (for ECAR) in their study. In the present study, incubation under capacitating conditions resulted in a decrease of 37% in basal respiration rate and an increase of 52% in basal glycolysis rate. Finally, comparison between treatments in the previous study is problematic since phenomena such as non-mitochondrial oxygen consumption, and non-glycolytic acidification were not estimated by subtracting values after inhibition from basal measurements.
As an alternative to a general increase in sperm metabolism linked to capacitation (Balbach et al., 2020), our results suggest that sperm capacitation promotes a change in the usage ratio of the two main metabolic pathways, from a highly oxidative metabolic phenotype in non-capacitated sperm to a predominantly glycolytic one in capacitated sperm (Figure 7B). Since oxidative phosphorylation yields more ATP per molecule of glucose consumed than aerobic glycolysis, the evidence does not support the hypothesis that sperm would be expected to reorient their metabolism to pathways that produce ATP more efficiently in response to increased ATP demands associated with capacitation (Travis et al., 2004; Ishijima, 2011). Nonetheless, some caveats could be raised.
Recent studies have found that mouse sperm capacitation is associated with increased glucose uptake (Hidalgo et al., 2020) and that active glycolysis is crucial for the maintenance of ATP concentration and bending capacity in the distal end of the flagellum (Takei et al., 2014). This suggests that 1) ATP produced in the mitochondria does not diffuse sufficiently fast in order to reach the end of the flagellum, and 2) local ATP provision is a crucial physiological variable for the motility of these cells. According to our results, capacitated mouse sperm do not appear to engage in increased ATP consumption and are able to maintain ATP levels similar to those of non-capacitated cells. Considering that aerobic glycolysis occurs along most of the principal piece, a high glycolytic rate would provide a metabolic alternative to ensure sufficient ATP supply if physiological events associated with sperm capacitation (ion transport, protein phosphorylation, or hyperactivated movement) resulted in modifications of the local ATP demands in specific flagellar regions. Local ATP generation by mechanisms other than OXPHOS is relatively common in cellular structures with high energetic demands whose dimensions or structure make them unable to accommodate mitochondria (Villar et al., 2021). In addition, it should also be borne in mind that EFA and ATP consumption analyses were performed in sperm populations with a high proportion of capacitated sperm (Sansegundo et al., 2022) and, thus, early processes that take place during the acquisition of capacitation, and that may affect energy metabolism, have been excluded from this study.
In coincidence with previous results (Balbach et al., 2020), inhibition of glycolysis resulted in a decrease of OXPHOS rates even when the culture medium was supplemented with respiratory substrates, thus indicating that the substrate used to fuel the Krebs cycle in the mitochondria of these cells is necessarily of endogenous origin. In support of this interpretation, earlier studies revealed that in a medium containing glucose, the monocarboxylate transporters expressed in the flagellar membranes of mouse sperm (MCT2) are predominantly used to export lactate instead of importing respiratory substrates (Garcia et al., 1995; Boussouar et al., 2003; Mannowetz et al., 2012). In all, the alteration of the OCR/ECAR ratio promoted by capacitation might be simply regarded as an increase in the proportion of the pyruvate that, after its generation in the principal piece, is exported as lactate instead of being transported to the mitochondria to be oxidized (see Figure 7B for a graphical conclusion). While the diversion of potential OXPHOS substrates to an excretion pathway might appear as a waste of energetic resources for the cell, the reduction of pyruvate by lactate dehydrogenase (LDH) to produce lactate involves the regeneration of the NAD+ pool (from oxidized NADH), a crucial requirement for the maintenance of high glycolytic rates (Yeung et al., 2019; Luengo et al., 2021).
Recent publications have shown that mouse sperm increase their mitochondrial membrane potential (MMP) during incubation under capacitating conditions (Yang et al., 2020; Ferreira et al., 2021; Giaccagli et al., 2021) and that this event appears to be necessary to achieve hyperactivated motility (Ferreira et al., 2021; Giaccagli et al., 2021). These findings might appear to contradict our results since they were interpreted as a sign of higher OXPHOS in capacitated cells. In other words, high MMP was associated with elevated mitochondrial ATP synthesis. However, H+ reentry through the ATP synthase during ADP phosphorylation causes a drop in MMP with the concomitant compensatory increase in oxygen consumption, thus, higher ATP synthesis rates result in lower MMP (Brand and Nicholls, 2011). In bioenergetic terms, the transition from state 3 (active ATP synthesis) to state 4 (e.g., oligomycin inhibited ATP synthesis) would in turn lead to MMP hyperpolarization (Brand and Nicholls, 2011; Sanderson et al., 2013; Zorova et al., 2018). Thus, the increased MMP exhibited by capacitated mouse sperm when compared to non-capacitated (Yang et al., 2020; Giaccagli et al., 2021) cells would be consistent with the decrease in the oxygen consumption rates observed in our study.
Our study also revealed that inhibition of mitochondrial ATP production with oligomycin resulted in increased lactate excretion rates. This phenomenon could be due to the conversion to lactate of the excess pyruvate that remains in the cytosol (via LDH) since its usage in the Krebs cycle would be diminished as a consequence of ATPase inhibition. Alternatively, there is evidence showing that a decrease in ATP concentration produced by OXPHOS inhibition leads to the activation of the AMP protein kinase (AMPK) which, among other effects, promotes an increase in catabolic processes (i.e., glycolysis) (Martin-Hidalgo et al., 2018). Moreover, mouse sperm have been revealed to increase their glucose uptake rates under physiological conditions (Hidalgo et al., 2020). Thus, mouse sperm would be capable of shifting the burden of ATP supply to glycolysis when respiration is insufficient to meet the energetic demands of the cell. Various degrees of metabolic flexibility are observed in cell types that are frequently subjected to unpredictable environments (Mookerjee et al., 2016; Bekebrede et al., 2021). In the case of mouse sperm, this would represent a significant evolutionary advantage since it would allow them to sustain an adequate ATP supply during variations in availability and concentration of metabolic substrates that are known to exist within the female reproductive tract (Gardner and Leese, 1990; Harris et al., 2005). On the other hand, mouse sperm seem unable to perform the reverse compensation (i.e., increasing respiratory rate when glycolytic ATP production is unavailable) since the inhibition of glycolysis by 2DOG produces a decrease in OXPHOS of a similar level to that caused by oligomycin. The inability of mouse sperm to utilize exogenous pyruvate and their dependence on glycolysis to provide endogenous respiratory substrates has been previously reported (Balbach et al., 2020), and it seems to be at odds with the significant presence of pyruvate and lactate in the mouse uterine, oviductal, and follicular fluids (Gardner and Leese, 1990; Harris et al., 2005).
In conclusion, our study has revealed that mouse sperm exhibit a shift in the usage ratio of the main metabolic pathways, from oxidative to glycolytic, as a consequence of capacitation. These changes do not seem to affect intracellular ATP concentration and ATP consumption rates. Thus, the probable function of this metabolic switch is not to increase overall ATP production, but to sustain ATP levels while ensuring the local provision of ATP to flagellar regions with specific ATP demands that arise from capacitation. The molecular mechanisms that govern and regulate these phenomena are still poorly understood, and further research is needed to address these issues.
Data availability statement
The raw data supporting the conclusions of this article will be made available by the authors, without undue reservation.
Ethics statement
The animal study was reviewed and approved by National Museum of Natural Sciences, Spanish Research Council and Madrid Regional Government.
Author contributions
ERRS, ER, MT, and ES conceived the study. ES and MT performed experiments. MT, ES, and ERRS analyzed data. MT, ES, ER, and ERRS wrote the paper. All authors have read and agreed to the published version of the manuscript.
Funding
This work was supported by the Spanish Ministry of Science and Innovation (projects CGL 2016-80577-P and PID 2019-108649GB-I00). ES was funded by an FPI studentship and MT held a “Juan de la Cierva” postdoctoral fellowship, both from the Ministry of Science and Innovation.
Acknowledgments
We are grateful to Juan Antonio Rielo for supervising animal facilities and Esperanza Navarro for animal care at the Museo Nacional de Ciencias Naturales in Madrid.
Conflict of interest
The authors declare that the research was conducted in the absence of any commercial or financial relationships that could be construed as a potential conflict of interest.
Publisher’s note
All claims expressed in this article are solely those of the authors and do not necessarily represent those of their affiliated organizations, or those of the publisher, the editors and the reviewers. Any product that may be evaluated in this article, or claim that may be made by its manufacturer, is not guaranteed or endorsed by the publisher.
Supplementary material
The Supplementary Material for this article can be found online at: https://www.frontiersin.org/articles/10.3389/fcell.2022.950979/full#supplementary-material
References
Arnoult, C., Kazam, I. G., Visconti, P. E., Kopf, G. S., Villaz, M., and Florman, H. M. (1999). Control of the low voltage-activated calcium channel of mouse sperm by egg Zp3 and by membrane hyperpolarization during capacitation. Proc. Natl. Acad. Sci. U. S. A. 96, 6757–6762. doi:10.1073/pnas.96.12.6757
Austin, C. R. (1951). Observations on the penetration of the sperm in the mammalian egg. Aust. J. Sci. Res. B 4, 581–596. doi:10.1071/bi9510581
Bailey, J. L., and Storey, B. T. (1994). Calcium influx into mouse spermatozoa activated by solubilized mouse zona pellucida, monitored with the calcium fluorescent indicator, Fluo-3. Inhibition of the influx by three inhibitors of the zona pellucida induced acrosome reaction: Tyrphostin a48, pertussis toxin, and 3-quinuclidinyl benzilate. Mol. Reprod. Dev. 39, 297–308. doi:10.1002/mrd.1080390307
Balbach, M., Gervasi, M. G., Hidalgo, D. M., Visconti, P. E., Levin, L. R., and Buck, J. (2020). Metabolic changes in mouse sperm during capacitation. Biol. Reprod. 103, 791–801. doi:10.1093/biolre/ioaa114
Bekebrede, A. F., Keijer, J., Gerrits, W. J. J., and De Boer, V. C. J. (2021). Mitochondrial and glycolytic extracellular flux analysis optimization for isolated pig intestinal epithelial cells. Sci. Rep. 11, 19961. doi:10.1038/s41598-021-99460-0
Boussouar, F., Mauduit, C., Tabone, E., Pellerin, L., Magistretti, P. J., and Benahmed, M. (2003). Developmental and hormonal regulation of the monocarboxylate transporter 2 (mct2) expression in the mouse germ cells. Biol. Reprod. 69, 1069–1078. doi:10.1095/biolreprod.102.010074
Brand, M. D., and Nicholls, D. G. (2011). Assessing mitochondrial dysfunction in cells. Biochem. J. 435, 297–312. doi:10.1042/BJ20110162
Buffone, M. G. (2016). Sperm acrosome biogenesis and function during fertilization. New York, USA: Springer. doi:10.1007/978-3-319-30567-7
Chang, H., and Suarez, S. S. (2011). Two distinct Ca(2+) signaling pathways modulate sperm flagellar beating patterns in mice. Biol. Reprod. 85, 296–305. doi:10.1095/biolreprod.110.089789
Chang, M. C. (1951). Fertilizing capacity of spermatozoa deposited into the fallopian tubes. Nature 168, 697–698. doi:10.1038/168697b0
Cooper, T. G. (1984). The onset and maintenance of hyperactivated motility of spermatozoa from the mouse. Gamete Res. 9, 55–74. doi:10.1002/mrd.1120090106
Cuasnicú, P., Da Ros, V., Weigel Muñoz, M., and Cohen, D. J. (2016). “Acrosome reaction as a preparation for gamete fusion,” in Sperm acrosome biogenesis and function during fertilization. Editor M. G. Buffone (Cham, Switzerland: Springer International Publishing), 159–172. doi:10.1007/978-3-319-30567-7
Cummins, J. (2009). “Sperm motility and energetics,” in Sperm Biology. Editors T. R. Birkhead, D. J. Hosken, and S. Pitnick (San Diego, CA: Academic Press), 185–206. doi:10.1016/b978-0-12-372568-4.00005-7
Danshina, P. V., Geyer, C. B., Dai, Q., Goulding, E. H., Willis, W. D., Kitto, G. B., et al. (2010). Phosphoglycerate kinase 2 (Pgk2) is essential for sperm function and male fertility in mice. Biol. Reprod. 82, 136–145. doi:10.1095/biolreprod.109.079699
De Jonge, C. (2017). Biological basis for human capacitation-revisited. Hum. Reprod. Update 23, 289–299. doi:10.1093/humupd/dmw048
Demarco, I. A., Espinosa, F., Edwards, J., Sosnik, J., De La Vega-Beltran, J. L., Hockensmith, J. W., et al. (2003). Involvement of a Na+/HCO-3 cotransporter in mouse sperm capacitation. J. Biol. Chem. 278, 7001–7009. doi:10.1074/jbc.M206284200
Escoffier, J., Krapf, D., Navarrete, F., Darszon, A., and Visconti, P. E. (2012). Flow cytometry analysis reveals a decrease in intracellular sodium during sperm capacitation. J. Cell Sci. 125, 473–485. doi:10.1242/jcs.093344
Ferramosca, A., Provenzano, S. P., Coppola, L., and Zara, V. (2012). Mitochondrial respiratory efficiency is positively correlated with human sperm motility. Urology 79, 809–814. doi:10.1016/j.urology.2011.12.042
Ferramosca, A., and Zara, V. (2014). Bioenergetics of mammalian sperm capacitation. Biomed. Res. Int. 2014, 902953. doi:10.1155/2014/902953
Ferreira, J. J., Cassina, A., Irigoyen, P., Ford, M., Pietroroia, S., Peramsetty, N., et al. (2021). Increased mitochondrial activity upon CatSper channel activation is required for mouse sperm capacitation. Redox Biol. 48, 102176. doi:10.1016/j.redox.2021.102176
Ford, W. C. (2006). Glycolysis and sperm motility: Does a spoonful of sugar help the flagellum go round? Hum. Reprod. Update 12, 269–274. doi:10.1093/humupd/dmi053
Fraser, L. R. (1982). Ca2+ is required for mouse sperm capacitation and fertilization in vitro. J. Androl. 3, 412–419. doi:10.1002/j.1939-4640.1982.tb00712.x
Fraser, L. R. (1977). Motility patterns in mouse spermatozoa before and after capacitation. J. Exp. Zool. 202, 439–444. doi:10.1002/jez.1402020314
Fraser, L. R., and Quinn, P. J. (1981). A glycolytic product is obligatory for initiation of the sperm acrosome reaction and whiplash motility required for fertilization in the mouse. J. Reprod. Fertil. 61, 25–35. doi:10.1530/jrf.0.0610025
Fraser, L. R. (2010). The "switching on" of mammalian spermatozoa: Molecular events involved in promotion and regulation of capacitation. Mol. Reprod. Dev. 77, 197–208. doi:10.1002/mrd.21124
Gadella, B. M., and Harrison, R. A. (2000). The capacitating agent bicarbonate induces protein kinase A-dependent changes in phospholipid transbilayer behavior in the sperm plasma membrane. Development 127, 2407–2420. doi:10.1242/dev.127.11.2407
Garcia, C. K., Brown, M. S., Pathak, R. K., and Goldstein, J. L. (1995). Cdna cloning of MCT2, a second monocarboxylate transporter expressed in different cells than MCT1. J. Biol. Chem. 270, 1843–1849. doi:10.1074/jbc.270.4.1843
Gardner, D. K., and Leese, H. J. (1990). Concentrations of nutrients in mouse oviduct fluid and their effects on embryo development and metabolism in vitro. J. Reprod. Fertil. 88, 361–368. doi:10.1530/jrf.0.0880361
Garty, N. B., Galiani, D., Aharonheim, A., Ho, Y. K., Phillips, D. M., Dekel, N., et al. (1988). G-proteins in mammalian gametes: An immunocytochemical study. J. Cell Sci. 91 (1), 21–31. doi:10.1242/jcs.91.1.21
Gervasi, M. G., and Visconti, P. E. (2016). Chang's meaning of capacitation: A molecular perspective. Mol. Reprod. Dev. 83, 860–874. doi:10.1002/mrd.22663
Giaccagli, M. M., Gomez-Elias, M. D., Herzfeld, J. D., Marin-Briggiler, C. I., Cuasnicu, P. S., Cohen, D. J., et al. (2021). Capacitation-induced mitochondrial activity is required for sperm fertilizing ability in mice by modulating hyperactivation. Front. Cell Dev. Biol. 9, 767161. doi:10.3389/fcell.2021.767161
Giojalas, L. C., Guidobaldi, H. A., Sánchez, R., and Cosson, J. J. (2015). “Sperm chemotaxis in mammals,” in Flagellar mechanics and sperm guidance (PotomacUS: Bentham Science Publishers), 272–307. doi:10.2174/9781681081281115010012
Gomendio, M., Martin-Coello, J., Crespo, C., Magana, C., and Roldan, E. R. (2006). Sperm competition enhances functional capacity of mammalian spermatozoa. Proc. Natl. Acad. Sci. U. S. A. 103, 15113–15117. doi:10.1073/pnas.0605795103
Goodson, S. G., Qiu, Y., Sutton, K. A., Xie, G., Jia, W., and O'brien, D. A. (2012). Metabolic substrates exhibit differential effects on functional parameters of mouse sperm capacitation. Biol. Reprod. 87, 75. doi:10.1095/biolreprod.112.102673
Goodson, S. G., Zhang, Z., Tsuruta, J. K., Wang, W., and O'brien, D. A. (2011). Classification of mouse sperm motility patterns using an automated multiclass support vector machines model. Biol. Reprod. 84, 1207–1215. doi:10.1095/biolreprod.110.088989
Guerrero, A., Carneiro, J., Pimentel, A., Wood, C. D., Corkidi, G., and Darszon, A. (2011). Strategies for locating the female gamete: The importance of measuring sperm trajectories in three spatial dimensions. Mol. Hum. Reprod. 17, 511–523. doi:10.1093/molehr/gar042
Guidobaldi, H. A., Hirohashi, N., Cubilla, M., Buffone, M. G., and Giojalas, L. C. (2017). An intact acrosome is required for the chemotactic response to progesterone in mouse spermatozoa. Mol. Reprod. Dev. 84, 310–315. doi:10.1002/mrd.22782
Harris, S. E., Gopichandran, N., Picton, H. M., Leese, H. J., and Orsi, N. M. (2005). Nutrient concentrations in murine follicular fluid and the female reproductive tract. Theriogenology 64, 992–1006. doi:10.1016/j.theriogenology.2005.01.004
Harrison, R. A. (1996). Capacitation mechanisms, and the role of capacitation as seen in eutherian mammals. Reprod. Fertil. Dev. 8, 581–594. doi:10.1071/rd9960581
Harrison, R. A., and Gadella, B. M. (2005). Bicarbonate-induced membrane processing in sperm capacitation. Theriogenology 63, 342–351. doi:10.1016/j.theriogenology.2004.09.016
Harrison, R. A., and Miller, N. G. (2000). Camp-dependent protein kinase control of plasma membrane lipid architecture in boar sperm. Mol. Reprod. Dev. 55, 220–228. doi:10.1002/(SICI)1098-2795(200002)55:2<220::AID-MRD12>3.0.CO;2-I
Harrison, R. A. (2004). Rapid pkA-catalysed phosphorylation of boar sperm proteins induced by the capacitating agent bicarbonate. Mol. Reprod. Dev. 67, 337–352. doi:10.1002/mrd.20028
Hidalgo, D. M., Romarowski, A., Gervasi, M. G., Navarrete, F., Balbach, M., Salicioni, A. M., et al. (2020). Capacitation increases glucose consumption in murine sperm. Mol. Reprod. Dev. 87, 1037–1047. doi:10.1002/mrd.23421
Hoppe, P. C. (1976). Glucose requirement for mouse sperm capacitation in vitro. Biol. Reprod. 15, 39–45. doi:10.1095/biolreprod15.1.39
Ishijima, S. (2011). Dynamics of flagellar force generated by a hyperactivated spermatozoon. Reproduction 142, 409–415. doi:10.1530/REP-10-0445
Kamp, G., Busselmann, G., Jones, N., Wiesner, B., and Lauterwein, J. (2003). Energy metabolism and intracellular ph in boar spermatozoa. Reproduction 126, 517–525. doi:10.1530/rep.0.1260517
Krapf, D., Arcelay, E., Wertheimer, E. V., Sanjay, A., Pilder, S. H., Salicioni, A. M., et al. (2010). Inhibition of Ser/Thr phosphatases induces capacitation-associated signaling in the presence of Src kinase inhibitors. J. Biol. Chem. 285, 7977–7985. doi:10.1074/jbc.M109.085845
Lee, M. A., and Storey, B. T. (1986). Bicarbonate is essential for fertilization of mouse eggs: Mouse sperm require it to undergo the acrosome reaction. Biol. Reprod. 34, 349–356. doi:10.1095/biolreprod34.2.349
Luengo, A., Li, Z., Gui, D. Y., Sullivan, L. B., Zagorulya, M., Do, B. T., et al. (2021). Increased demand for NAD(+) relative to atp drives aerobic glycolysis. Mol. Cell 81, 691–707.e6. e696. doi:10.1016/j.molcel.2020.12.012
Magdanz, V., Boryshpolets, S., Ridzewski, C., Eckel, B., and Reinhardt, K. (2019). The motility-based swim-up technique separates bull sperm based on differences in metabolic rates and tail length. PLoS One 14, e0223576. doi:10.1371/journal.pone.0223576
Mannowetz, N., Wandernoth, P. M., and Wennemuth, G. (2012). Glucose is a pH-dependent motor for sperm beat frequency during early activation. PLoS One 7, e41030. doi:10.1371/journal.pone.0041030
Marinangeli, C., Didier, S., Ahmed, T., Caillerez, R., Domise, M., Laloux, C., et al. (2018). Amp-activated protein kinase is essential for the maintenance of energy levels during synaptic activation. iScience 9, 1–13. doi:10.1016/j.isci.2018.10.006
Martin-Hidalgo, D., Hurtado De Llera, A., Calle-Guisado, V., Gonzalez-Fernandez, L., Garcia-Marin, L., and Bragado, M. J. (2018). Ampk function in mammalian spermatozoa. Int. J. Mol. Sci. 19, E3293. doi:10.3390/ijms19113293
Miki, K., Qu, W., Goulding, E. H., Willis, W. D., Bunch, D. O., Strader, L. F., et al. (2004). Glyceraldehyde 3-phosphate dehydrogenase-s, a sperm-specific glycolytic enzyme, is required for sperm motility and male fertility. Proc. Natl. Acad. Sci. U. S. A. 101, 16501–16506. doi:10.1073/pnas.0407708101
Mookerjee, S. A., Goncalves, R. L. S., Gerencser, A. A., Nicholls, D. G., and Brand, M. D. (2015). The contributions of respiration and glycolysis to extracellular acid production. Biochim. Biophys. Acta 1847, 171–181. doi:10.1016/j.bbabio.2014.10.005
Mookerjee, S. A., Nicholls, D. G., and Brand, M. D. (2016). Determining maximum glycolytic capacity using extracellular flux measurements. PLoS One 11, e0152016. doi:10.1371/journal.pone.0152016
Mukai, C., and Okuno, M. (2004). Glycolysis plays a major role for adenosine triphosphate supplementation in mouse sperm flagellar movement. Biol. Reprod. 71, 540–547. doi:10.1095/biolreprod.103.026054
Narisawa, S., Hecht, N. B., Goldberg, E., Boatright, K. M., Reed, J. C., and Millan, J. L. (2002). Testis-specific cytochrome c-null mice produce functional sperm but undergo early testicular atrophy. Mol. Cell. Biol. 22, 5554–5562. doi:10.1128/MCB.22.15.5554-5562.2002
Navarrete, F. A., Garcia-Vazquez, F. A., Alvau, A., Escoffier, J., Krapf, D., Sanchez-Cardenas, C., et al. (2015). Biphasic role of calcium in mouse sperm capacitation signaling pathways. J. Cell. Physiol. 230, 1758–1769. doi:10.1002/jcp.24873
Nishigaki, T., Jose, O., Gonzalez-Cota, A. L., Romero, F., Trevino, C. L., and Darszon, A. (2014). Intracellular pH in sperm physiology. Biochem. Biophys. Res. Commun. 450, 1149–1158. doi:10.1016/j.bbrc.2014.05.100
Nixon, B., and Bromfield, E. G. (2018). “Sperm capacitation,” in Encyclopedia of reproduction. Editor M. K. Skinner (Oxford, UK: Academic Press), 272–278. doi:10.1016/b978-0-12-801238-3.64464-1
Odet, F., Duan, C., Willis, W. D., Goulding, E. H., Kung, A., Eddy, E. M., et al. (2008). Expression of the gene for mouse lactate dehydrogenase C (Ldhc) is required for male fertility. Biol. Reprod. 79, 26–34. doi:10.1095/biolreprod.108.068353
Odet, F., Gabel, S. A., Williams, J., London, R. E., Goldberg, E., and Eddy, E. M. (2011). Lactate dehydrogenase C and energy metabolism in mouse sperm. Biol. Reprod. 85, 556–564. doi:10.1095/biolreprod.111.091546
Odet, F., Gabel, S., London, R. E., Goldberg, E., and Eddy, E. M. (2013). Glycolysis and mitochondrial respiration in mouse LdhC-null sperm. Biol. Reprod. 88, 95. doi:10.1095/biolreprod.113.108530
Okamura, N., Tajima, Y., Soejima, A., Masuda, H., and Sugita, Y. (1985). Sodium bicarbonate in seminal plasma stimulates the motility of mammalian spermatozoa through direct activation of adenylate cyclase. J. Biol. Chem. 260, 9699–9705. doi:10.1016/s0021-9258(17)39295-5
Perez-Cerezales, S., Lopez-Cardona, A. P., and Gutierrez-Adan, A. (2016). Progesterone effects on mouse sperm kinetics in conditions of viscosity. Reproduction 151, 501–507. doi:10.1530/REP-15-0582
Roldan, E. R. S., and Vazquez, J. M. (1996). Bicarbonate/CO2 induces rapid activation of phospholipase A2 and renders boar spermatozoa capable of undergoing acrosomal exocytosis in response to progesterone. FEBS Lett. 396, 227–232. doi:10.1016/0014-5793(96)01110-6
Ruiz-Pesini, E., Díez-Sánchez, C., López-Pérez, M. J., and Enríquez, J. A. (2007). The role of the mitochondrion in sperm function: Is there a place for oxidative phosphorylation or is this a purely glycolytic process? Curr. Top. Dev. Biol. 77, 3–19. doi:10.1016/s0070-2153(06)77001-6
Sanderson, T. H., Reynolds, C. A., Kumar, R., Przyklenk, K., and Huttemann, M. (2013). Molecular mechanisms of ischemia-reperfusion injury in brain: Pivotal role of the mitochondrial membrane potential in reactive oxygen species generation. Mol. Neurobiol. 47, 9–23. doi:10.1007/s12035-012-8344-z
Sansegundo, E., Tourmente, M., and Roldan, E. R. S. (2022). Energy metabolism and hyperactivation of spermatozoa from three mouse species under capacitating conditions. Cells 11, 220. doi:10.3390/cells11020220
Shahrokhi, S. Z., Salehi, P., Alyasin, A., Taghiyar, S., and Deemeh, M. R. (2020). Asthenozoospermia: Cellular and molecular contributing factors and treatment strategies. Andrologia 52, e13463. doi:10.1111/and.13463
Shi, Q. X., and Roldan, E. R. (1995). Bicarbonate/CO2 is not required for zona pellucida- or progesterone-induced acrosomal exocytosis of mouse spermatozoa but is essential for capacitation. Biol. Reprod. 52, 540–546. doi:10.1095/biolreprod52.3.540
Stival, C., Puga Molina, L. C., Paudel, B., Buffone, M. G., Visconti, P. E., and Krapf, D. (2016). “Sperm capacitation and acrosome reaction in mammalian sperm,” in Sperm acrosome biogenesis and function during fertilization. Editor M. G. Buffone (Cham, Switzerland: Springer International Publishing), 93–106. doi:10.1007/978-3-319-30567-7_5
Storey, B. T. (2008). Mammalian sperm metabolism: Oxygen and sugar, friend and foe. Int. J. Dev. Biol. 52, 427–437. doi:10.1387/ijdb.072522bs
Suarez, S. S. (2008). Control of hyperactivation in sperm. Hum. Reprod. Update 14, 647–657. doi:10.1093/humupd/dmn029
Takei, G. L., Miyashiro, D., Mukai, C., and Okuno, M. (2014). Glycolysis plays an important role in energy transfer from the base to the distal end of the flagellum in mouse sperm. J. Exp. Biol. 217, 1876–1886. doi:10.1242/jeb.090985
Tang, H., Duan, C., Bleher, R., and Goldberg, E. (2013). Human Lactate dehydrogenase A (LdhA) rescues mouse LdhC-null sperm function. Biol. Reprod. 88, 96. doi:10.1095/biolreprod.112.107011
Tourmente, M., Varea-Sanchez, M., and Roldan, E. R. S. (2019). Faster and more efficient swimming: Energy consumption of murine spermatozoa under sperm competition. Biol. Reprod. 100, 420–428. doi:10.1093/biolre/ioy197
Tourmente, M., Villar-Moya, P., Rial, E., and Roldan, E. R. (2015a). Differences in ATP generation via glycolysis and oxidative phosphorylation and relationships with sperm motility in mouse species. J. Biol. Chem. 290, 20613–20626. doi:10.1074/jbc.M115.664813
Tourmente, M., Villar-Moya, P., Varea-Sanchez, M., Luque-Larena, J. J., Rial, E., and Roldan, E. R. (2015b). Performance of rodent spermatozoa over time is enhanced by increased atp concentrations: The role of sperm competition. Biol. Reprod. 93, 64. doi:10.1095/biolreprod.114.127621
Travis, A. J., Jorgez, C. J., Merdiushev, T., Jones, B. H., Dess, D. M., Diaz-Cueto, L., et al. (2001). Functional relationships between capacitation-dependent cell signaling and compartmentalized metabolic pathways in murine spermatozoa. J. Biol. Chem. 276, 7630–7636. doi:10.1074/jbc.M006217200
Travis, A. J., Tutuncu, L., Jorgez, C. J., Ord, T. S., Jones, B. H., Kopf, G. S., et al. (2004). Requirements for glucose beyond sperm capacitation during in vitro fertilization in the mouse. Biol. Reprod. 71, 139–145. doi:10.1095/biolreprod.103.025809
Turner, R. M. (2006). Moving to the beat: A review of mammalian sperm motility regulation. Reprod. Fertil. Dev. 18, 25–38. doi:10.1071/rd05120
Urner, F., Leppens-Luisier, G., and Sakkas, D. (2001). Protein tyrosine phosphorylation in sperm during gamete interaction in the mouse: The influence of glucose. Biol. Reprod. 64, 1350–1357. doi:10.1095/biolreprod64.5.1350
Urner, F., and Sakkas, D. (1996). Glucose is not essential for the occurrence of sperm binding and zona pellucida-induced acrosome reaction in the mouse. Int. J. Androl. 19, 91–96. doi:10.1111/j.1365-2605.1996.tb00441.x
Villar, P. S., Vergara, C., and Bacigalupo, J. (2021). Energy sources that fuel metabolic processes in protruding finger-like organelles. FEBS J. 288, 3799–3812. doi:10.1111/febs.15620
Visconti, P. E., Bailey, J. L., Moore, G. D., Pan, D., Olds-Clarke, P., and Kopf, G. S. (1995a). Capacitation of mouse spermatozoa. I. Correlation between the capacitation state and protein tyrosine phosphorylation. Development 121, 1129–1137. doi:10.1242/dev.121.4.1129
Visconti, P. E., Moore, G. D., Bailey, J. L., Leclerc, P., Connors, S. A., Pan, D., et al. (1995b). Capacitation of mouse spermatozoa. II. Protein tyrosine phosphorylation and capacitation are regulated by a cAMP-dependent pathway. Development 121, 1139–1150. doi:10.1242/dev.121.4.1139
Visconti, P. E., Muschietti, J. P., Flawia, M. M., and Tezon, J. G. (1990). Bicarbonate dependence of camp accumulation induced by phorbol esters in hamster spermatozoa. Biochim. Biophys. Acta 1054, 231–236. doi:10.1016/0167-4889(90)90246-a
Visconti, P. E., Ning, X., Fornes, M. W., Alvarez, J. G., Stein, P., Connors, S. A., et al. (1999). Cholesterol efflux-mediated signal transduction in mammalian sperm: Cholesterol release signals an increase in protein tyrosine phosphorylation during mouse sperm capacitation. Dev. Biol. 214, 429–443. doi:10.1006/dbio.1999.9428
Visconti, P. E. (2009). Understanding the molecular basis of sperm capacitation through kinase design. Proc. Natl. Acad. Sci. U. S. A. 106, 667–668. doi:10.1073/pnas.0811895106
Ward, C. R., and Storey, B. T. (1984). Determination of the time course of capacitation in mouse spermatozoa using a chlortetracycline fluorescence assay. Dev. Biol. 104, 287–296. doi:10.1016/0012-1606(84)90084-8
Yanagimachi, R. (1970). The movement of golden hamster spermatozoa before and after capacitation. J. Reprod. Fertil. 23, 193–196. doi:10.1530/jrf.0.0230193
Yang, Q., Wen, Y., Wang, L., Peng, Z., Yeerken, R., Zhen, L., et al. (2020). Ca(2+) ionophore a23187 inhibits ATP generation reducing mouse sperm motility and pkA-dependent phosphorylation. Tissue Cell 66, 101381. doi:10.1016/j.tice.2020.101381
Yepez, V. A., Kremer, L. S., Iuso, A., Gusic, M., Kopajtich, R., Konarikova, E., et al. (2018). Ocr-stats: Robust estimation and statistical testing of mitochondrial respiration activities using seahorse xf analyzer. PLoS One 13, e0199938. doi:10.1371/journal.pone.0199938
Yeung, C., Gibson, A. E., Issaq, S. H., Oshima, N., Baumgart, J. T., Edessa, L. D., et al. (2019). Targeting glycolysis through inhibition of lactate dehydrogenase impairs tumor growth in preclinical models of ewing sarcoma. Cancer Res. 79, 5060–5073. doi:10.1158/0008-5472.CAN-19-0217
Zarintash, R. J., and Cross, N. L. (1996). Unesterified cholesterol content of human sperm regulates the response of the acrosome to the agonist, progesterone. Biol. Reprod. 55, 19–24. doi:10.1095/biolreprod55.1.19
Zeng, Y., Oberdorf, J. A., and Florman, H. M. (1996). Ph regulation in mouse sperm: Identification of NA(+)-Cl(-)-and HCO3(-)-dependent and arylaminobenzoate-dependent regulatory mechanisms and characterization of their roles in sperm capacitation. Dev. Biol. 173, 510–520. doi:10.1006/dbio.1996.0044
Zhao, C., Wang, B., Liu, E., and Zhang, Z. (2020). Loss of PTEN expression is associated with PI3K pathway-dependent metabolic reprogramming in hepatocellular carcinoma. Cell Commun. Signal. 18, 131. doi:10.1186/s12964-020-00622-w
Keywords: sperm metabolism, capacitation, mouse, glycolysis, OXPHOS
Citation: Tourmente M, Sansegundo E, Rial E and Roldan ERS (2022) Capacitation promotes a shift in energy metabolism in murine sperm. Front. Cell Dev. Biol. 10:950979. doi: 10.3389/fcell.2022.950979
Received: 23 May 2022; Accepted: 01 August 2022;
Published: 23 August 2022.
Edited by:
Farners Amargant, Northwestern University, United StatesReviewed by:
Haim Breitbart, Bar-Ilan University, IsraelLuis J. Garcia-Marin, Departamento de Fisiología, Facultad de Veterinaria, Universidad de Extremadura, Spain
Dario Krapf, CONICET Instituto de Biología Molecular y Celular de Rosario (IBR), Argentina
Copyright © 2022 Tourmente, Sansegundo, Rial and Roldan. This is an open-access article distributed under the terms of the Creative Commons Attribution License (CC BY). The use, distribution or reproduction in other forums is permitted, provided the original author(s) and the copyright owner(s) are credited and that the original publication in this journal is cited, in accordance with accepted academic practice. No use, distribution or reproduction is permitted which does not comply with these terms.
*Correspondence: Maximiliano Tourmente, bWF4aW1pbGlhbm8udG91cm1lbnRlQHVuYy5lZHUuYXI=; Eduardo R. S. Roldan, cm9sZGFuZUBtbmNuLmNzaWMuZXM=