- Division of Pharmacology, Graduate School of Medical and Dental Sciences, Niigata University, Niigata, Japan
Fetal nuchal edema, a subcutaneous accumulation of extracellular fluid in the fetal neck, is detected as increased nuchal translucency (NT) by ultrasonography in the first trimester of pregnancy. It has been demonstrated that increased NT is associated with chromosomal anomalies and genetic syndromes accompanied with fetal malformations such as defective lymphatic vascular development, cardiac anomalies, anemia, and a wide range of other fetal anomalies. However, in many clinical cases of increased NT, causative genes, pathogenesis and prognosis have not been elucidated in humans. On the other hand, a large number of gene mutations have been reported to induce fetal nuchal edema in mouse models. Here, we review the relationship between the gene mutants causing fetal nuchal edema with defective lymphatic vascular development, cardiac anomalies, anemia and blood vascular endothelial barrier anomalies in mice. Moreover, we discuss how studies using gene mutant mouse models will be useful in developing diagnostic method and predicting prognosis.
Introduction
In the first trimester of pregnancy, fetal nuchal edema, a subcutaneous accumulation of extracellular fluid in the fetal neck, is visualized by ultrasonography as increased nuchal translucency (NT) (Nicolaides et al., 1992). It has been demonstrated that increased NT is associated with chromosomal anomalies such as trisomy 21 (Down syndrome), trisomy 18 and trisomy 13 (Nicolaides et al., 1992; Snijders et al., 1998). In addition, increased NT in fetuses with normal karyotype is associated with defective lymphatic vascular development, cardiac anomalies, hereditary anemia, and a wide range of other fetal anomalies, including skeletal defects, central nervous system defects and diaphragmatic hernia (Tercanli et al., 2001; Souka et al., 2002; Atzei et al., 2005; Souka et al., 2005). Enhanced blood vascular permeability may also be associated with increased NT as seen in subcutaneous edema in adults (Claesson-Welsh et al., 2021). The causative genes have been identified in some but not many clinical cases of increased NT. Many cases of increased NT disappear during the second trimester and the majority of fetuses with increased NT are born normally (Yoshida et al., 2008). Therefore, increased NT is considered to be a transient physiological finding, and the prognosis of fetal nuchal edema has not been sufficiently investigated. However, fetuses with increased NT sometimes have edema worsened to develop hydrops fetalis (Tahmasebpour et al., 2012). It would be desirable to develop the clinical diagnosis to distinguish between cases that ends in temporary changes and life-threatening problems. For this purpose, it is necessary to identify additional causative genes and to understand the relationship between gene mutations, pathogenesis and prognosis.
Compared to studies in humans, a larger number of gene mutations have been reported to induce fetal nuchal edema in mouse models. Therefore, a better understanding of the relationship between causative gene mutations and fetal phenotypes in mutant mice may provide new strategies for treatment of human clinical cases. Here, we review the relationship between the gene mutations causing fetal nuchal edema with defective lymphatic vascular development, cardiac anomalies, anemia and changes in blood vascular endothelial barrier in mice (Figure 1). Moreover, we discuss how studies using gene mutant mice will be useful in developing diagnostic method and predicting prognosis.
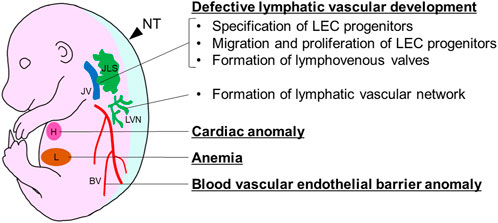
FIGURE 1. Developmental anomalies causing fetal nuchal edema in mice. BV, blood vessel; H, heart; JLS, jugular lymph sac; JV, jugular vein; L, liver; LVN, lymphatic vascular network; NT, nuchal translucency.
Defective lymphatic vascular development
Lymphatic vessels form a network throughout the body and play important roles in tissue fluid homeostasis by collecting excess interstitial fluid and returning it to the blood circulation (Tammela and Alitalo, 2010; Yang and Oliver, 2014; Mäkinen et al., 2021). Therefore, lymphatic vascular dysfunction is a major cause of edema (Souka et al., 2005). Here, we describe stepwise processes of lymphatic vascular development by pointing out gene mutations causing fetal nuchal edema in mice (Table 1).
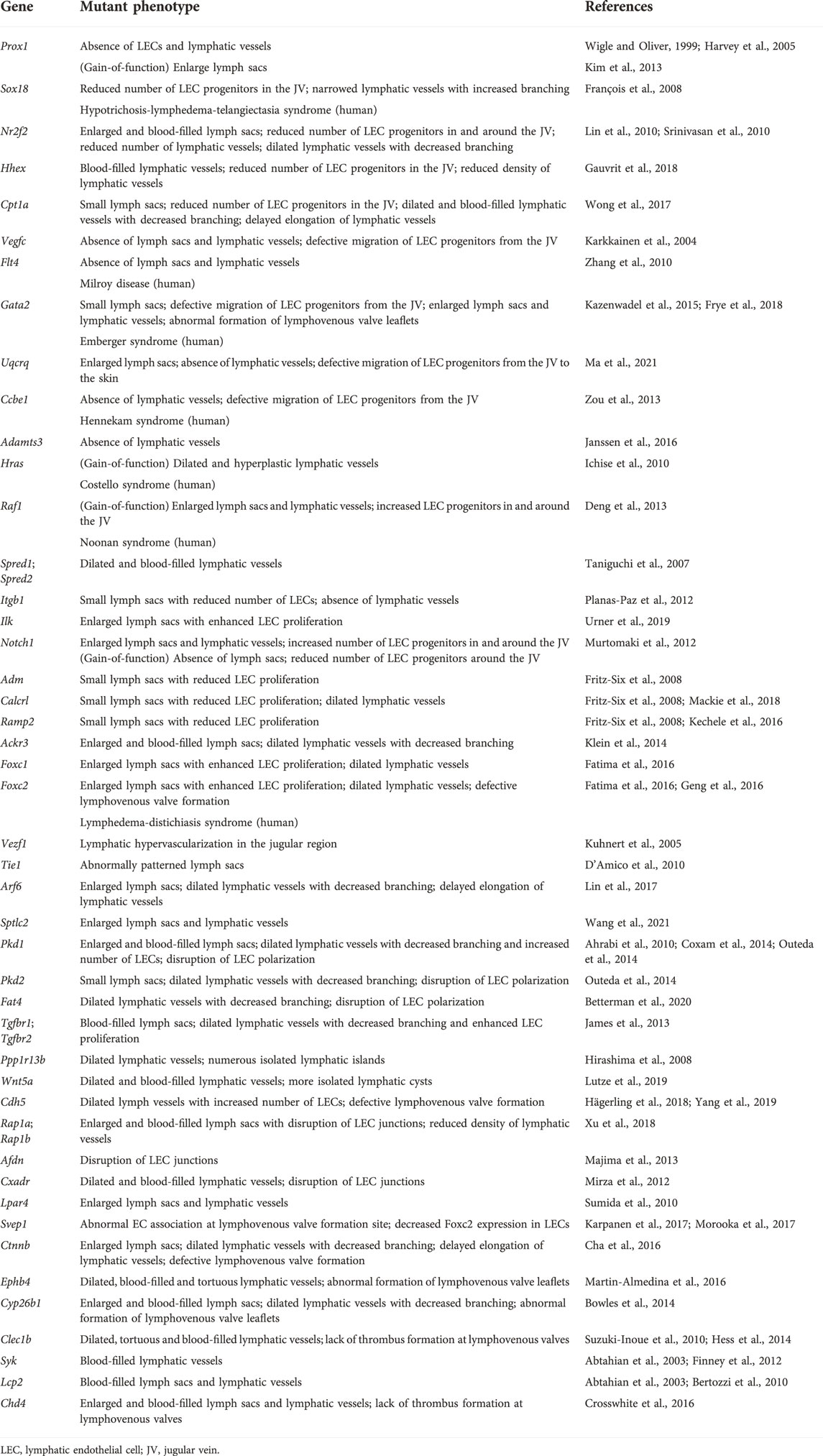
TABLE 1. List of genes and mutant phenotypes related to fetal nuchal edema with defective lymphatic vascular development in mice (loss-of-function mutations if not stated).
Specification of lymphatic endothelial cell progenitors
It is the first step in lymphatic vascular development that the specification of lymphatic endothelial cell (LEC) progenitors from a subpopulation of ECs in the jugular vein (JV) around embryonic day (E) 9.5 (Wigle and Oliver, 1999; Srinivasan et al., 2007; Tammela and Alitalo, 2010; Yang and Oliver, 2014). Gene mutations which impair specification of LEC progenitors cause fetal nuchal edema with hypoplastic lymphatic vessels in mice. Prospero-related homeobox 1 (Prox1) gene encodes a master transcriptional regulator to induce LEC progenitor differentiation and maintain LEC identity (Hong et al., 2002; Tammela and Alitalo, 2010) (Figure 2). Both loss- and gain- of function of Prox1 induce fetal nuchal edema, indicating that appropriate regulation of Prox1 expression is essential for maintenance of lymphatic vascular morphology and fluid homeostasis (Wigle and Oliver, 1999; Harvey et al., 2005; Kim et al., 2013). Prox1 expression is regulated by several transcription factors such as SRY-box transcription factor 18 (Sox18), chicken ovalbumin upstream promoter transcription factor 2 (Coup-TFII) and hematopoietically-expressed homeobox (Hhex) (François et al., 2008; Lin et al., 2010; Srinivasan et al., 2010; Gauvrit et al., 2018). EC-specific deletion of Sox18, Nr2f2, or Hhex gene in mice reduces LEC progenitors and induces fetal nuchal edema (François et al., 2008; Lin et al., 2010; Srinivasan et al., 2010; Gauvrit et al., 2018). Carnitine palmitoyl transferase 1a (Cpt1a) is an enzyme implicated in fatty acid β-oxidation-dependent synthesis of acetyl coenzyme A required for histone acetylation in the regulatory region of Prox1-target genes (Wong et al., 2017; Schlaepfer and Joshi, 2020). LEC-specific deletion of Cpt1a in mice induces fetal nuchal edema (Wong et al., 2017). Wingless type MMTV integration site family, member 5b (Wnt5b) upregulates Prox1 expression in zebrafish and human embryonic stem cell-derived angioblasts (Nicenboim et al., 2015). It will be intriguing whether Wnt5b mutation induces fetal nuchal edema in mice.
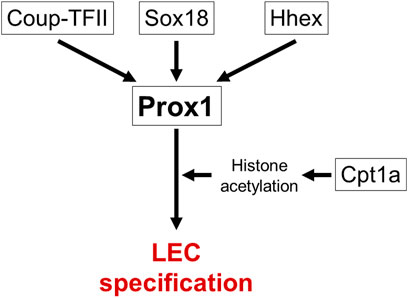
FIGURE 2. Transcriptional networks in specification of LEC progenitors. Prox1 is a master transcriptional regulator gene of LEC specification. Several transcription factors (Sox18, Coup-TFII, Hhex) and Cpt1a-modulated histone acetylation regulate Prox1 expression. Causative genes for fetal nuchal edema in mice are surrounded by square.
Migration and proliferation of lymphatic endothelial cell progenitors
Differentiated LEC progenitors migrate away from the JV toward the neighboring mesenchymal tissues to form early lymph sacs (Wigle and Oliver, 1999; Tammela and Alitalo, 2010; Yang et al., 2012; Yang and Oliver, 2014). Gene mutations which impair migration and proliferation of LEC progenitors cause fetal nuchal edema with hypoplastic lymphatic vessels in mice. Vascular endothelial growth factor receptor 3 (VEGFR3/Flt4) is induced in LEC progenitors with elevated Prox1 expression (Karkkainen et al., 2004). VEGF-C upregulates Prox1 expression levels via Prox1-VEGFR3 feedback loop, which maintains the number of LEC progenitors (Srinivasan et al., 2014). Migration and proliferation of LEC progenitors are promoted by intracellular signaling via VEGFR3 and its ligand VEGF-C produced by mesenchymal cells (Karkkainen et al., 2004) (Figure 3). Vegfc knockout mice exhibit fetal nuchal edema with hypoplastic lymphatic vessels due to impaired migration of LEC progenitors from JV (Karkkainen et al., 2004). Chy mice, which carries a heterozygous inactivating point mutation in tyrosine kinase domain of VEGFR3 induced by ethylnitrosourea, exhibit fetal nuchal edema with lack of lymphatic vessels (Zhang et al., 2010). Flt4 expression levels are also important for lymphatic vascular development. GATA2 regulates VEGFR3 expression in LECs by directly binding to the intronic enhancer region of the FLT4 gene (Frye et al., 2018). Mitochondrial respiratory chain complex III QPC subunit (Uqcrq) is required for the maintenance of epigenetic modifications at the FLT4 promoter (Ma et al., 2021). Deletion of Gata2 or Uqcrq in mice induces fetal nuchal edema with defective migration of LEC progenitors from the JV (Frye et al., 2018; Ma et al., 2021). VEGF-C, originally generated as a prepropeptide, requires proteolysis to produce active form that allows binding to VEGFR3 (Joukov et al., 1997). Collagen and calcium-binding EGF domain-containing protein 1 (Ccbe1) and a disintegrin and metalloproteinase with thrombospondin motifs 3 (Adamts3) play a critical role in proteolytic cleavage of pro-VEGF-C (Jeltsch et al., 2014). Deletion of Ccbe1 or Adamts3 in mice induces fetal nuchal edema with lack of lymphatic vessels (Zou et al., 2013; Janssen et al., 2016). Binding of VEGF-C to VEGFR3 results in tyrosine phosphorylation, which in turn activates Ras/Raf/extracellular signal regulated kinase (ERK) signaling and eventually promotes LEC migration and sprouting (Mäkinen et al., 2021). In humans, gain-of-function mutations in Ras pathway-related genes including HRAS, RAF1, PTPN11, KRAS, SOS1 and RIT1 are actually identified in Noonan syndrome and related disorder (Costello syndrome) patients associated with increased NT (Croonen et al., 2013; Sleutjes et al., 2022). Noonan syndrome is a multiple malformation syndrome with characteristic facies, congenital heart disease, and short stature, and presents with prenatal and postnatal lymphedema (Noonan, 2006; Sleutjes et al., 2022). Some of these gene mutations have been shown to cause fetal nuchal edema in mouse models. EC-specific overexpression of Hras in mice induces fetal nuchal edema with dilated and hyperplastic lymphatic vessels (Ichise et al., 2010). Raf1 is phosphorylated at Ser259 downstream of phosphatidylinositol 3 kinase (PI3K)/Akt signaling and is inhibited under normal condition in ECs (Ren et al., 2010). Thus, substitution of this Serine to Phenylalanine (S259F) or to Threonine (S259T) results in gain-of-function mutation of Raf1 (Pandit et al., 2007; Ko et al., 2008). EC-specific RAF1S259A expression in mice causes fetal nuchal edema with increased number of LEC progenitors around the JV (Deng et al., 2013). Raf activity is also negatively regulated by Sprouty-related Ena/VASP homology1-domain containing (Spred) (Wakioka et al., 2001). Spred1 and Spread2 double knockout mice exhibit fetal nuchal edema with blood-filled lymphatic vessels (Taniguchi et al., 2007). VEGFR3 signaling is also activated by β1 integrin in a VEGF-C-independent manner (Planas-Paz et al., 2012). It has been proposed that β1 integrin interaction to VEGFR3 is inhibited by integrin-linked kinase (Ilk), but upon mechanical stretch, the complex of β1 integrin and Ilk is transiently disrupted, which in turn promotes VEGFR3 phosphorylation and subsequent LEC proliferation (Urner et al., 2019). EC-specific deletion of Itgb1 in mice induces fetal nuchal edema with hypoplastic lymph sacs and lymphatic vessels and reduced LEC proliferation (Planas-Paz et al., 2012). LEC-specific deletion of Ilk in mice induces fetal nuchal edema with enhanced LEC proliferation (Urner et al., 2019). Notch1 is also suggested as an inhibitory molecule for VEGFR3 signaling and LEC migration (Zheng et al., 2011; Choi et al., 2017). Loss-of-function mutation of Notch1 in LECs induces fetal nuchal edema with increased number of LEC progenitors around the JV (Murtomaki et al., 2012). On the other hand, gain-of-function mutation of Notch1 in LECs induces fetal nuchal edema with lymph sac hypoplasia and downregulation of Coup-TFII and VEGFR3 (Murtomaki et al., 2012). It is interesting to note that fetal nuchal edema is not caused by only hypoplasia but also hyperplasia of lymphatic vessels in mice.
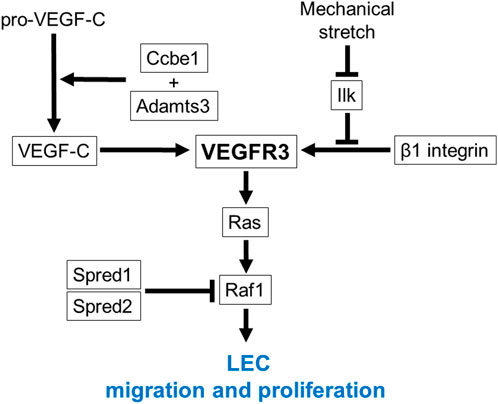
FIGURE 3. VEGFR3 signaling in migration and proliferation of LEC progenitors. VEGFR3 functions as a main signaling receptor for migration and proliferation of LEC progenitors. Ccbe1 and Adamts3 cleave pro-VEGF-C and produce active form that allows binding to VEGFR3. Binding of VEGF-C to VEGFR3 results in tyrosine phosphorylation, which in turn activates Ras/Raf signaling. Raf activity is negatively regulated by Spred1 and Spred2. VEGFR3 signaling is also regulated by β1 integrin in a VEGF-C independent manner. β1 integrin interaction to VEGFR3 is inhibited by Ilk, but upon mechanical stretch, the complex of β1 integrin and Ilk is transiently disrupted, which in turn promotes VEGFR3 phosphorylation. Causative genes for fetal nuchal edema in mice are surrounded by square.
Migrated LECs begin to form lymph sacs (Tammela and Alitalo, 2010; Yang et al., 2012; Yang and Oliver, 2014). Enlarged jugular lymph sacs are detected in some fetuses exhibiting increased NT (Bekker et al., 2005). Gene mutations which impair lymph sac formation cause fetal nuchal edema in mice. Adrenomedullin (Adm)-calcitonin receptor-like receptor (Calcrl) axis promotes lymph sac formation through enhanced LEC proliferation (Fritz-Six et al., 2008). Receptor activity-modifying proteins (Ramps) regulate the interaction between Adm and Calcrl (McLatchie et al., 1998). Knockout mice for Adm, Calcr1 or Ramp2 exhibit fetal nuchal edema with hypoplastic lymph sacs (Fritz-Six et al., 2008; Kechele et al., 2016; Mackie et al., 2018). C-X-C chemokine receptor type 7 (Cxcr7/Ackr3) is a decoy receptor for Adm and modulates Adm-mediated lymphatic vascular development. Deletion of Ackr3 in mice induces fetal nuchal edema with enlarged lymph sacs (Klein et al., 2014). Forkhead box (Fox) transcription factor Foxc1 and Foxc2 regulate lymph sac development by modulating LEC proliferation. LEC-specific deletion of Foxc1 or Foxc2 in mice induces fetal nuchal edema with enlarged lymph sacs (Fatima et al., 2016). In addition, knockout mice for the Vezf1 gene encoding vascular endothelial zinc finger 1, a transcriptional regulatory protein, exhibit fetal nuchal edema with lymphatic hypervascularization in the jugular lymph sac region (Kuhnert et al., 2005). Knockout mice for Tie1, a receptor tyrosine kinase that regulates migration and proliferation in ECs, exhibit fetal nuchal edema with abnormally patterned lymph sacs (D’Amico et al., 2010).
Formation of lymphatic vascular network
As lymph sacs are formed, LECs continue to proliferate and migrate, forming lymphatic vascular network throughout the developing embryo (Tammela and Alitalo, 2010; Yang and Oliver, 2014). Gene mutations which impair the formation of lymphatic vascular network cause fetal nuchal edema in mice. ADP-ribosylation factor 6 (Arf6) is a small GTPase which regulates internalization of β1 integrin in LECs. LEC-specific deletion of Arf6 in mice induces fetal nuchal edema with suppressed elongation of dermal lymphatic vessels to the dorsal midline (Lin et al., 2017). Serine palmitoyltransferase long chain base subunit 2 (Sptlc2), the rate-limiting enzyme of sphingolipid biosynthesis, increases Coup-TFII transcriptional activity. EC-specific deletion of Sptlc2 in mice induces fetal nuchal edema with dilated dermal lymphatic vessels (Wang et al., 2021). Polycystin is a cell surface receptor involved in cell-cell and cell-matrix interactions and is encoded by PKD1 and PKD2, the genes responsible for autosomal dominant polycystic kidney disease (Delmas, 2004). FAT4 is atypical cadherin which is expressed on the cell surface of plasma membrane (Katoh, 2012). Polycystin and FAT4 regulate polarity in LECs and deletion of Pkd1, Pkd2 or Fat4 in mice induces fetal nuchal edema with dilatation and decreased vascular branching of dermal lymphatic vessels (Ahrabi et al., 2010; Coxam et al., 2014; Outeda et al., 2014; Betterman et al., 2020). Transforming growth factor-β is widely known to play an important role in multiple cellular process (Barnard et al., 1990), and EC-specific deletion for its receptor Tgfbr1 or Tgfbr2 induces fetal nuchal edema with dilated dermal lymphatic vessels with decreased vascular branching (James et al., 2013). Furthermore, we previously reported that knockout mice for Ppp1r13b, which encodes apoptosis stimulating protein of p53 (Aspp1), exhibit fetal nuchal edema with numerous isolated lymphatic islands as well as disorganized lymphatic vessels in embryonic skin (Hirashima et al., 2008). Deletion of Wnt5a, a member of the WNT family in mice also induces fetal nuchal edema with isolated lymphatic cysts in embryonic skin (Lutze et al., 2019).
Gene mutations which affect LEC junctions cause fetal nuchal edema in mice. VE-cadherin is a representative endothelial cell-cell adhesion molecule responsible for vascular integrity (Zhang et al., 2020). LEC-specific knockout mice for Cdh5 gene encoding VE-cadherin exhibit fetal nuchal edema with dilated lymphatic vessels (Hägerling et al., 2018). LEC-specific knockout mice for Rap1a/b exhibit fetal nuchal edema with enlarged and blood-filled lymph sacs accompanied by destabilization of VE-cadherin-mediated LEC junctions (Xu et al., 2018). Afadin (Afdn) is a membrane scaffold protein with filamentous-actin binding activity and is colocalized with VE-cadherin at LEC junctions. EC-specific deletion of Afdn in mice induces fetal nuchal edema with reduced VE-cadherin expression and numerous punctures in lymphatic endothelium (Majima et al., 2013). The coxsackie- and adenovirus receptor (CAR) is a cell adhesion molecule localized at LEC junctions. Deletion of the Cxadr gene encoding CAR in mice induces fetal nuchal edema with enlarged blood-filled lymphatic vessels (Mirza et al., 2012).
Formation of lymphovenous valves
The downstream end of the thoracic duct is connected to the vein at the jugular venous angle, which allows the lymph flows into the blood circulation. Lymphovenous valves formed at the connection prevent the influx of blood cells into the lymphatic vessels (Yang and Oliver, 2014). Gene mutations which impair formation of lymphovenous valves cause fetal nuchal edema in mice. Lymphovenous valve formation begins with the differentiation of a partial venous ECs into lymphovenous valve ECs (LVV-ECs) (Geng et al., 2016). LVV-ECs highly expresses Gata2 and Foxc2, which play critical roles in formation of lymphovenous valves (Yang and Oliver, 2014; Geng et al., 2016). LEC-specific deletion of Gata2 in mice induces fetal nuchal edema with blood-filled lymph sacs due to abnormal formation of lymphovenous valve leaflets (Kazenwadel et al., 2015). Approximately 50% of heterozygous knockout mice for Foxc2 exhibit severe fetal nuchal edema with lack of lymphovenous valves and enlarged lymph sacs (Geng et al., 2016). The similar phenotypes are reported in knockout mice for the Lpa4 gene encoding lysophosphatidic acid receptor 4 (Sumida et al., 2010). Extracellular matrix protein Svep1 regulates Foxc2 expression in LECs. LEC-specific deletion of Svep1 in mice induces severe fetal nuchal edema (Karpanen et al., 2017; Morooka et al., 2017). VE-cadherin encoded by the Cdh5 gene is the mechanotransduction protein which senses shear stress and elevates FOXC2 expression (Yang et al., 2019). β-catenin (Ctnnb), an intracellular protein that colocalizes with VE-cadherin, mediates shear stress-induced upregulation of FOXC2 (Cha et al., 2016). LEC-specific deletion of Cdh5 or Ctnnb in mice induces fetal nuchal edema with lack of lymphovenous valves (Cha et al., 2016; Yang et al., 2019). Intracellular signaling mediated by the receptor tyrosine kinase EphB4 and its ligand EphrinB2 is also required for formation of lymphovenous valves. LEC-specific knockout mice for Ephb4 exhibit fetal nuchal edema with blood-filled lymphatic vessels due to hypoplasia of lymphovenous valve leaflets (Martin-Almedina et al., 2016). Retinoic acid is a regulator of lymphovenous valve formation. Deletion of cytochrome P450 26B1 (Cyp26b1), which catalyzes the degradation of retinoic acid, induces fetal nuchal edema with blood-filled lymph sacs due to expanded size of lymphovenous valve leaflets (Bowles et al., 2014).
LECs at lymphovenous valves can come in direct contact with blood cells if influx of blood cells into lymphatic vessels occurs. LEC-mediated platelet activation and thrombus formation at lymphovenous valves has been implicated in lymph-blood partitioning (Welsh et al., 2016). Gene mutations which impair platelet activation cause fetal nuchal edema in mice. Podoplanin expressed on LECs activates the C-type lectin-like receptor 2 (Clec2/Clec1b) in platelets, leading to platelet activation via Syk-Slp76/Lcp2 signaling (Welsh et al., 2016). Knockout mice for Clec1b, Syk or Lcp2 encoding these signaling molecules exhibit fetal nuchal edema with blood-filled lymphatic vessels (Abtahian et al., 2003; Bertozzi et al., 2010; Suzuki-Inoue et al., 2010; Finney et al., 2012; Hess et al., 2014). Besides, LEC-specific deletion of chromodomain helicase DNA binding protein 4 (Chd4), chromatin remodeling enzyme induces fetal nuchal edema with blood-filled lymphatic vessels due to lack of thrombus formation at lymphovenous valves by increased plasmin activity (Crosswhite et al., 2016).
Cardiac anomaly
Cardiac anomalies or reduction in cardiac contractility decreases cardiac output, which causes an elevation in venous pressure and a subsequent elevation in capillary hydrostatic pressure. Edema occurs when fluid leakage by high capillary hydrostatic pressure exceeds the ability of the lymphatic system to return fluid to the blood circulation (Cho and Atwood, 2002). The prevalence of cardiac anomalies correlates with NT thickness in fetuses (Atzei et al., 2005; Souka et al., 2005). Gene mutations which induce cardiac anomalies cause fetal nuchal edema (Table 2).
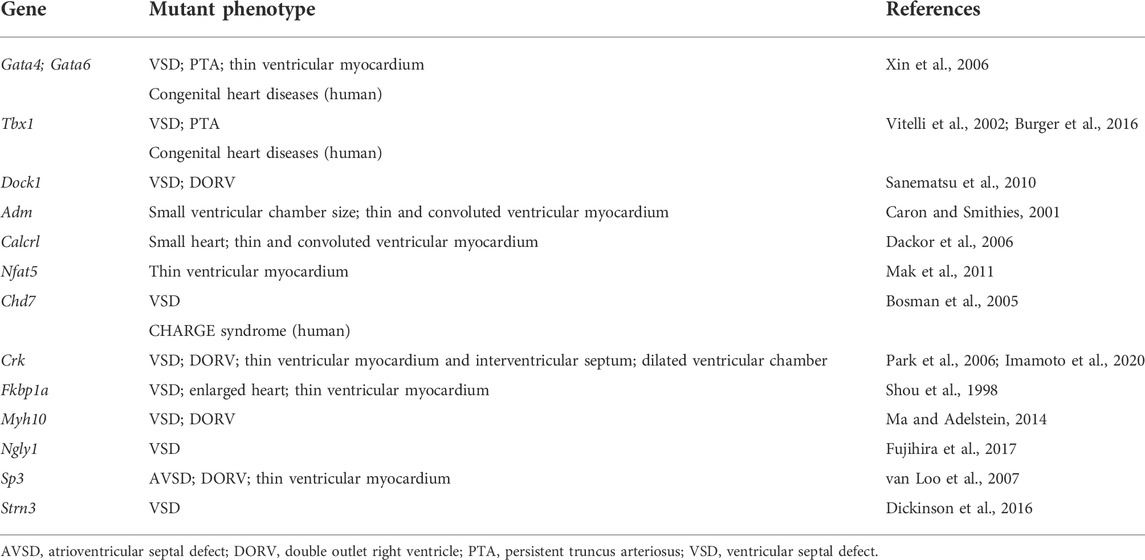
TABLE 2. List of genes and mutant phenotypes related to fetal nuchal edema with cardiac anomalies in mice (loss-of-function mutations).
Congenital heart disease is the leading cause of early postnatal mortality (Soares, 2018). Therefore, in fetuses with increased NT due to cardiac anomalies, it may have a significant impact on prenatal as well as postnatal pathophysiology. Early diagnosis of the presence or absence of cardiac anomalies in fetuses with increased NT is very important.
As described in several review articles on cardiac development, the mature four-chambered heart is formed from a liner heart tube through a complex series of events, including rightward looping, cushion formation, cardiac chamber septation and outflow tract septation (Srivastava and Olson, 2000; Paige et al., 2015; Desgrange et al., 2018). A number of gene mutations have been reported to cause cardiac anomalies in mice (Bruneau, 2002; Desgrange et al., 2018), but only a few of them have been implicated in fetal nuchal edema. Gene mutations which impair these developmental processes cause fetal nuchal edema in mice. GATA4 regulates all the processes of cardiac development (Paige et al., 2015). T-box transcription factor 1 (TBX1) regulates the later process such as chamber septation (Paige et al., 2015). Knockout mice for Tbx1 or double heterozygous knockout mice for Gata4 and Gata6 exhibit fetal nuchal edema with ventricular septal defect (VSD) and persistent truncus arteriosus (PTA) (Vitelli et al., 2002; Xin et al., 2006; Burger et al., 2016). Loss-of-function mutation in the Dock1 gene encoding an atypical Rac activator causes fetal nuchal edema with VSD and double outlet right ventricle (DORV) (Sanematsu et al., 2010). Several other genes have been reported to be associated with cardiac anomalies including VSD and DORV (Table 2).
Reduction in cardiac contractility is associated with myocardial wall thinning (Maciver, 2011). Gene mutations which impair cardiomyocyte proliferation even with normal compartmentalization of four chambers and the great arteries cause fetal nuchal edema in mice. Deletion of Adm, Calcrl or nuclear factor of activated T-cells 5 (Nfat5) in mice reduces cardiomyocyte proliferation and induces thinning of compact zone of ventricular myocardium (Caron and Smithies, 2001; Dackor et al., 2006; Mak et al., 2011).
Despite the high prevalence of cardiac anomalies in patients with increased NT, much fewer number of gene mutations responsible for cardiac anomalies have been reported, compared to those for defective lymphatic vascular development. Kalisch-Smith et al. (2021) reported that mice fed with iron-deficient diets exhibit fetal nuchal edema with VSD and DORV. Taken together, a significant percentage of cardiac anomaly cases may be due to chromosomal abnormalities or non-genetic factors such as nutritional condition of embryos.
Others
Anemia
Anemia in human fetuses has been implicated in increased NT (Tercanli et al., 2001; Souka et al., 2005). Blood type incompatibility between a mother and fetus is known to cause hemolysis, jaundice, and severe anemia in the fetus (Prefumo et al., 2019). Severe anemia induces high-output heart failure, leading to severe edema called as hydrops fetalis (Tongsong et al., 2010). Human parvovirus B19 infection causes increased NT with anemia by inducing apoptosis of erythroid precursors in liver (Poole et al., 2004; Giorgio et al., 2010). Gene mutation which induces anemia causes fetal nuchal edema in mice (Table 3).

TABLE 3. List of genes and mutant phenotypes related to fetal nuchal edema with anemia or blood vascular endothelial barrier anomalies in mice (loss-of-function mutations if not stated).
Serine/threonine kinase 40 (Stk40) knockout mice exhibit fetal nuchal edema with reduced hematocrits and hemoglobin levels (Wang et al., 2017). In this mutant, a primary defect appears enhanced cell apoptosis in the liver, the hematopoietic organ during fetal life (Lewis et al., 2021). Thus, gene mutations affecting hepatocyte proliferation and function during development can be a possible cause of nuchal edema.
Blood vascular endothelial barrier anomaly
Fetal nuchal edema is remarkably observed after E13.5 in mice (D’Amico et al., 2010; Lin et al., 2017), while defective blood vascular development often results in embryonic lethality before E12.5 (Shalaby et al., 1995; Jeansson et al., 2011). Therefore, it is unlikely that the gene mutations that causes major defects in blood vascular development are associated with fetal nuchal edema. On the other hand, gene mutations which impair blood vascular endothelial barrier cause fetal nuchal edema in mice (Table 3). We previously reported that heterozygosity in mice for the Flt1 gene encoding VEGFR1, a decoy receptor for VEGF-A, causes fetal nuchal edema. This mouse model exhibits an enhanced phosphorylation of VEGFR2, the main signaling receptor for VEGF-A, and an increased vascular permeability without affecting vascular morphology (Otowa et al., 2016). EC-specific overexpression of Myc in mice causes fetal nuchal edema by inducing the apoptosis of blood vascular ECs (BECs) and disruption of vascular endothelial barrier (Kokai et al., 2009). Enabled/vasodilator (Ena/VASP), expresses both at focal adhesions and at cell-cell junctions, contributes to stabilization of cell adhesion. Ena/VASP-deficient mice (triple knockout mice for Enah, Vasp and Evl) exhibit fetal nuchal edema with gap formation between BECs in venules (Furman et al., 2007). These reports in mice indicate that increased fluid leakage from blood vessels due to disruption of vascular integrity may also be a cause of increased NT in human cases.
Conclusion
In this review, we described a number of gene mutations, which cause defective lymphatic vascular development, cardiac anomalies, anemia and blood vascular endothelial barrier anomalies, are associated with fetal nuchal edema. Therefore, the gene mutations which induce increased NT are expected to be diverse and vary for individual cases. In the field of oncology, gene panel testing is performed for comprehensive evaluation of numerous cancer-related gene mutations, leading to appropriate treatment for individual patients (Reid and Pal, 2020; Pereira et al., 2021). Although gene panel testing may also be useful for the diagnosis of increased NT, further identification of the causative genes is required to achieve it. Recently, studies of exome sequencing data analysis have been performed for diagnostic approach to human fetuses with increased NT, resulting in the listing of some candidate genes (Mellis et al., 2022; Pauta et al., 2022). Studies using gene mutant mice could be very useful to examine whether these candidate genes are causative genes for fetal nuchal edema, leading to the identification of novel causative genes and the realization of gene panel testing.
Although increased NT is considered to be a transient physiological finding (Yoshida et al., 2008), lymphatic dysfunction and heart defects described in this review are supposed to remain after birth. Even a subtle change in vascular permeability may put an impact on health during the long lifespan. In fact, Prox1 haploinsufficient mice, which survive to adulthood despite anatomical and functional lymphatic anomalies, exhibit obesity, an underlying risk for metabolic syndrome (Harvey et al., 2005). Prediction of the risk of postnatal disease onset by gene panel testing for fetal nuchal edema is expected to contribute to the prevention of various diseases and may present new possibilities for prenatal diagnosis. Taken together, studies of fetal nuchal edema using gene mutant mice will open up new avenues for the accurate diagnosis and treatment of increased NT in clinical medicine.
Author contributions
AS designed and wrote the manuscript. MH designed and revised the manuscript.
Funding
This work was supported in part by Grant-in-Aid for Research Activity Start-up (JSPS KAKENHI Grant Number JP21K20615).
Conflict of interest
The authors declare that the research was conducted in the absence of any commercial or financial relationships that could be construed as a potential conflict of interest.
Publisher’s note
All claims expressed in this article are solely those of the authors and do not necessarily represent those of their affiliated organizations, or those of the publisher, the editors and the reviewers. Any product that may be evaluated in this article, or claim that may be made by its manufacturer, is not guaranteed or endorsed by the publisher.
References
Abtahian, F., Guerriero, A., Sebzda, E., Lu, M. M., Zhou, R., Mocsai, A., et al. (2003). Regulation of blood and lymphatic vascular separation by signaling proteins SLP-76 and Syk. Science 299, 247–251. doi:10.1126/science.1079477
Ahrabi, A. K., Jouret, F., Marbaix, E., Delporte, C., Horie, S., Mulroy, S., et al. (2010). Glomerular and proximal tubule cysts as early manifestations of Pkd1 deletion. Nephrol. Dial. Transpl. 25, 1067–1078. doi:10.1093/ndt/gfp611
Atzei, A., Gajewska, K., Huggon, I. C., Allan, L., and Nicolaides, K. H. (2005). Relationship between nuchal translucency thickness and prevalence of major cardiac defects in fetuses with normal karyotype. Ultrasound Obstet. Gynecol. 26, 154–157. doi:10.1002/uog.1936
Barnard, J., Lyons, R. M., and Moses, H. L. (1990). The cell biology of transforming growth factor beta. Biochim. Biophys. Acta 1032, 79–87. doi:10.1016/0304-419X(90)90013-Q
Bekker, M. N., Haak, M. C., Rekoert-Hollander, M., Twisk, J., and van Vugt, J. M. G. (2005). Increased nuchal translucency and distended jugular lymphatic sacs on first-trimester ultrasound. Ultrasound Obstet. Gynecol. 25, 239–245. doi:10.1002/uog.1831
Bertozzi, C. C., Schmaier, A. A., Mericko, P., Hess, P. R., Zou, Z., Chen, M., et al. (2010). Platelets regulate lymphatic vascular development through CLEC-2-SLP-76 signaling. Blood 116, 661–670. doi:10.1182/blood-2010-02-270876
Betterman, K. L., Sutton, D. L., Secker, G. A., Kazenwadel, J., Oszmiana, A., Lim, L., et al. (2020). Atypical cadherin FAT4 orchestrates lymphatic endothelial cell polarity in response to flow. J. Clin. Invest. 130, 3315–3328. doi:10.1172/JCI99027
Bosman, E. A., Penn, A. C., Ambrose, J. C., Kettleborough, R., Stemple, D. L., and Steel, K. P. (2005). Multiple mutations in mouse Chd7 provide models for CHARGE syndrome. Hum. Mol. Genet. 14, 3463–3476. doi:10.1093/hmg/ddi375
Bowles, J., Secker, G., Nguyen, C., Kazenwadel, J., Truong, V., Frampton, E., et al. (2014). Control of retinoid levels by CYP26B1 is important for lymphatic vascular development in the mouse embryo. Dev. Biol. 386, 25–33. doi:10.1016/j.ydbio.2013.12.008
Bruneau, B. G. (2002). Mouse models of cardiac chamber formation and congenital heart disease. Trends Genet. 18, S15–S20. doi:10.1016/S0168-9525(02)02686-0
Burger, N. B., Haak, M. C., Kok, E., de Groot, C. J. M., Shou, W., Scambler, P. J., et al. (2016). Cardiac defects, nuchal edema and abnormal lymphatic development are not associated with morphological changes in the ductus venosus. Early Hum. Dev. 101, 39–48. doi:10.1016/j.earlhumdev.2016.05.017
Caron, K. M., and Smithies, O. (2001). Extreme hydrops fetalis and cardiovascular abnormalities in mice lacking a functional adrenomedullin gene. Proc. Natl. Acad. Sci. U. S. A. 98, 615–619. doi:10.1073/pnas.021548898
Cha, B., Geng, X., Mahamud, M. R., Fu, J., Mukherjee, A., Kim, Y., et al. (2016). Mechanotransduction activates canonical Wnt/β-catenin signaling to promote lymphatic vascular patterning and the development of lymphatic and lymphovenous valves. Genes Dev. 30, 1454–1469. doi:10.1101/gad.282400.116
Cho, S., and Atwood, J. E. (2002). Peripheral edema. Am. J. Med. 113, 580–586. doi:10.1016/S0002-9343(02)01322-0
Choi, D., Park, E., Jung, E., Seong, Y. J., Yoo, J., Lee, E., et al. (2017). Laminar flow downregulates Notch activity to promote lymphatic sprouting. J. Clin. Invest. 127, 1225–1240. doi:10.1172/JCI87442
Claesson-Welsh, L., Dejana, E., and McDonald, D. M. (2021). Permeability of the endothelial barrier: Identifying and reconciling controversies. Trends Mol. Med. 27, 314–331. doi:10.1016/j.molmed.2020.11.006
Coxam, B., Sabine, A., Bower, N. I., Smith, K. A., Pichol-Thievend, C., Skoczylas, R., et al. (2014). Pkd1 regulates lymphatic vascular morphogenesis during development. Cell Rep. 7, 623–633. doi:10.1016/j.celrep.2014.03.063
Croonen, E. A., Nillesen, W. M., Stuurman, K. E., Oudesluijs, G., van de Laar, I. M. B. M., Martens, L., et al. (2013). Prenatal diagnostic testing of the Noonan syndrome genes in fetuses with abnormal ultrasound findings. Eur. J. Hum. Genet. 21, 936–942. doi:10.1038/ejhg.2012.285
Crosswhite, P. L., Podsiadlowska, J. J., Curtis, C. D., Gao, S., Xia, L., Srinivasan, R. S., et al. (2016). CHD4-regulated plasmin activation impacts lymphovenous hemostasis and hepatic vascular integrity. J. Clin. Invest. 126, 2254–2266. doi:10.1172/JCI84652
D’Amico, G., Korhonen, E. A., Waltari, M., Saharinen, P., Laakkonen, P., and Alitalo, K. (2010). Loss of endothelial tie1 receptor impairs lymphatic vessel development-brief report. Arterioscler. Thromb. Vasc. Biol. 30, 207–209. doi:10.1161/ATVBAHA.109.196618
Dackor, R. T., Fritz-Six, K., Dunworth, W. P., Gibbons, C. L., Smithies, O., and Caron, K. M. (2006). Hydrops fetalis, cardiovascular defects, and embryonic lethality in mice lacking the calcitonin receptor-like receptor gene. Mol. Cell. Biol. 26, 2511–2518. doi:10.1128/mcb.26.7.2511-2518.2006
Delmas, P. (2004). Polycystins: From mechanosensation to gene regulation. Cell 118, 145–148. doi:10.1016/j.cell.2004.07.007
Deng, Y., Atri, D., Eichmann, A., and Simons, M. (2013). Endothelial ERK signaling controls lymphatic fate specification. J. Clin. Invest. 123, 1202–1215. doi:10.1172/JCI63034
Desgrange, A., le Garrec, J. F., and Meilhac, S. M. (2018). Left-right asymmetry in heart development and disease: Forming the right loop. Development 145, dev162776. doi:10.1242/dev.162776
Dickinson, M. E., Flenniken, A. M., Ji, X., Teboul, L., Wong, M. D., White, J. K., et al. (2016). High-throughput discovery of novel developmental phenotypes. Nature 537, 508–514. doi:10.1038/nature19356
Fatima, A., Wang, Y., Uchida, Y., Norden, P., Liu, T., Culver, A., et al. (2016). Foxc1 and Foxc2 deletion causes abnormal lymphangiogenesis and correlates with ERK hyperactivation. J. Clin. Invest. 126, 2437–2451. doi:10.1172/JCI80465
Finney, B. A., Schweighoffer, E., Navarro-Núñez, L., Bénézech, C., Barone, F., Hughes, C. E., et al. (2012). CLEC-2 and Syk in the megakaryocytic/platelet lineage are essential for development. Blood 119, 1747–1756. doi:10.1182/blood-2011-09-380709
François, M., Caprini, A., Hosking, B., Orsenigo, F., Wilhelm, D., Browne, C., et al. (2008). Sox18 induces development of the lymphatic vasculature in mice. Nature 456, 643–647. doi:10.1038/nature07391
Fritz-Six, K. L., Dunworth, W. P., Li, M., and Caron, K. M. (2008). Adrenomedullin signaling is necessary for murine lymphatic vascular development. J. Clin. Invest. 118, 40–50. doi:10.1172/JCI33302
Frye, M., Taddei, A., Dierkes, C., Martinez-Corral, I., Fielden, M., Ortsäter, H., et al. (2018). Matrix stiffness controls lymphatic vessel formation through regulation of a GATA2-dependent transcriptional program. Nat. Commun. 9, 1511. doi:10.1038/s41467-018-03959-6
Fujihira, H., Masahara-Negishi, Y., Tamura, M., Huang, C., Harada, Y., Wakana, S., et al. (2017). Lethality of mice bearing a knockout of the Ngly1-gene is partially rescued by the additional deletion of the Engase gene. PLoS Genet. 13, e1006696. doi:10.1371/journal.pgen.1006696
Furman, C., Sieminski, A. L., Kwiatkowski, A. V., Rubinson, D. A., Vasile, E., Bronson, R. T., et al. (2007). Ena/VASP is required for endothelial barrier function in vivo. J. Cell Biol. 179, 761–775. doi:10.1083/jcb.200705002
Gauvrit, S., Villasenor, A., Strilic, B., Kitchen, P., Collins, M. M., Marín-Juez, R., et al. (2018). HHEX is a transcriptional regulator of the VEGFC/FLT4/PROX1 signaling axis during vascular development. Nat. Commun. 9, 2704. doi:10.1038/s41467-018-05039-1
Geng, X., Cha, B., Mahamud, M. R., Lim, K. C., Silasi-Mansat, R., Uddin, M. K. M., et al. (2016). Multiple mouse models of primary lymphedema exhibit distinct defects in lymphovenous valve development. Dev. Biol. 409, 218–233. doi:10.1016/j.ydbio.2015.10.022
Giorgio, E., de Oronzo, M. A., Iozza, I., di Natale, A., Cianci, S., Garofalo, G., et al. Parvovirus B19 during pregnancy: A review. J. Prenat. Med. (2010) 4:63–66.
Hägerling, R., Hoppe, E., Dierkes, C., Stehling, M., Makinen, T., Butz, S., et al. (2018). Distinct roles of VE-cadherin for development and maintenance of specific lymph vessel beds. EMBO J. 37, e98271. doi:10.15252/embj.201798271
Harvey, N. L., Srinivasan, R. S., Dillard, M. E., Johnson, N. C., Witte, M. H., Boyd, K., et al. (2005). Lymphatic vascular defects promoted by Prox1 haploinsufficiency cause adult-onset obesity. Nat. Genet. 37, 1072–1081. doi:10.1038/ng1642
Hess, P. R., Rawnsley, D. R., Jakus, Z., Yang, Y., Sweet, D. T., Fu, J., et al. (2014). Platelets mediate lymphovenous hemostasis to maintain blood-lymphatic separation throughout life. J. Clin. Invest. 124, 273–284. doi:10.1172/JCI70422
Hirashima, M., Sano, K., Morisada, T., Murakami, K., Rossant, J., and Suda, T. (2008). Lymphatic vessel assembly is impaired in Aspp1-deficient mouse embryos. Dev. Biol. 316, 149–159. doi:10.1016/j.ydbio.2008.01.023
Hong, Y. K., Harvey, N., Noh, Y. H., Schacht, V., Hirakawa, S., Detmar, M., et al. (2002). Prox1 is a master control gene in the program specifying lymphatic endothelial cell fate. Dev. Dyn. 225, 351–357. doi:10.1002/dvdy.10163
Ichise, T., Yoshida, N., and Ichise, H. H. (2010). H-N- and Kras cooperatively regulate lymphatic vessel growth by modulating VEGFR3 expression in lymphatic endothelial cells in mice. Development 137, 1003–1013. doi:10.1242/dev.043489
Imamoto, A., Ki, S., Li, L., Iwamoto, K., Maruthamuthu, V., Devany, J., et al. (2020). Essential role of the Crk family-dosage in DiGeorge-like anomaly and metabolic homeostasis. Life Sci. Alliance 3, e201900635. doi:10.26508/LSA.201900635
James, J. M., Nalbandian, A., and Mukouyama, Y. (2013). TGFβ signaling is required for sprouting lymphangiogenesis during lymphatic network development in the skin. Development 140, 3903–3914. doi:10.1242/dev.095026
Janssen, L., Dupont, L., Bekhouche, M., Noel, A., Leduc, C., Voz, M., et al. (2016). ADAMTS3 activity is mandatory for embryonic lymphangiogenesis and regulates placental angiogenesis. Angiogenesis 19, 53–65. doi:10.1007/s10456-015-9488-z
Jeansson, M., Gawlik, A., Anderson, G., Li, C., Kerjaschki, D., Henkelman, M., et al. (2011). Angiopoietin-1 is essential in mouse vasculature during development and in response to injury. J. Clin. Invest. 121, 2278–2289. doi:10.1172/JCI46322
Jeltsch, M., Jha, S. K., Tvorogov, D., Anisimov, A., Leppänen, V. M., Holopainen, T., et al. (2014). CCBE1 enhances lymphangiogenesis via a disintegrin and metalloprotease with thrombospondin motifs-3-mediated vascular endothelial growth factor-C activation. Circulation 129, 1962–1971. doi:10.1161/CIRCULATIONAHA.113.002779
Joukov, V., Sorsa, T., Kumar, V., Jeltsch, M., Claesson-Welsh, L., Cao, Y., et al. (1997). Proteolytic processing regulates receptor specificity and activity of VEGF-C. EMBO J. 16, 3898–3911. doi:10.1093/emboj/16.13.3898
Kalisch-Smith, J. I., Ved, N., Szumska, D., Munro, J., Troup, M., Harris, S. E., et al. (2021). Maternal iron deficiency perturbs embryonic cardiovascular development in mice. Nat. Commun. 12, 3447. doi:10.1038/s41467-021-23660-5
Karkkainen, M. J., Haiko, P., Sainio, K., Partanen, J., Taipale, J., Petrova, T. V., et al. (2004). Vascular endothelial growth factor C is required for sprouting of the first lymphatic vessels from embryonic veins. Nat. Immunol. 5, 74–80. doi:10.1038/ni1013
Karpanen, T., Padberg, Y., van de Pavert, S. A., Dierkes, C., Morooka, N., Peterson-Maduro, J., et al. (2017). An evolutionarily conserved role for polydom/svep1 during lymphatic vessel formation. Circ. Res. 120, 1263–1275. doi:10.1161/CIRCRESAHA.116.308813
Katoh, M. (2012). Function and cancer genomics of FAT family genes (Review). Int. J. Oncol. 41, 1913–1918. doi:10.3892/ijo.2012.1669
Kazenwadel, J., Betterman, K. L., Chong, C. E., Stokes, P. H., Lee, Y. K., Secker, G. A., et al. (2015). GATA2 is required for lymphatic vessel valve development and maintenance. J. Clin. Invest. 125, 2979–2994. doi:10.1172/JCI78888
Kechele, D. O., Dunworth, W. P., Trincot, C. E., Wetzel-Strong, S. E., Li, M., Ma, H., et al. (2016). Endothelial restoration of receptor activity-modifying protein 2 is sufficient to rescue lethality, but survivors develop dilated cardiomyopathy. Hypertension 68, 667–677. doi:10.1161/HYPERTENSIONAHA.116.07191
Kim, H., Cruz, M., Bourdeau, A., and Dumont, D. J. (2013). Cell-cell interactions influence vascular reprogramming by Prox1 during embryonic development. PLoS ONE 8, e52197. doi:10.1371/journal.pone.0052197
Klein, K. R., Karpinich, N. O., Espenschied, S. T., Willcockson, H. H., Dunworth, W. P., Hoopes, S. L., et al. (2014). Decoy receptor CXCR7 modulates adrenomedullin-mediated cardiac and lymphatic vascular development. Dev. Cell 30, 528–540. doi:10.1016/j.devcel.2014.07.012
Ko, J. M., Kim, J. M., Kim, G. H., and Yoo, H. W. (2008). PTPN11, SOS1, KRAS, and RAF1 gene analysis, and genotype-phenotype correlation in Korean patients with Noonan syndrome. J. Hum. Genet. 53, 999–1006. doi:10.1007/s10038-008-0343-6
Kokai, E., Voss, F., Fleischer, F., Kempe, S., Marinkovic, D., Wolburg, H., et al. (2009). Myc regulates embryonic vascular permeability and remodeling. Circ. Res. 104, 1151–1159. doi:10.1161/CIRCRESAHA.108.191460
Kuhnert, F., Campagnolo, L., Xiong, J. W., Lemons, D., Fitch, M. J., Zou, Z., et al. (2005). Dosage-dependent requirement for mouse Vezf1 in vascular system development. Dev. Biol. 283, 140–156. doi:10.1016/j.ydbio.2005.04.003
Lewis, K., Yoshimoto, M., and Takebe, T. (2021). Fetal liver hematopoiesis: From development to delivery. Stem Cell Res. Ther. 12, 139. doi:10.1186/s13287-021-02189-w
Lin, F. J., Chen, X., Qin, J., Hong, Y. K., Tsai, M. J., and Tsai, S. Y. (2010). Direct transcriptional regulation of neuropilin-2 by COUP-TFII modulates multiple steps in murine lymphatic vessel development. J. Clin. Invest. 120, 1694–1707. doi:10.1172/JCI40101
Lin, Y. C., Ohbayashi, N., Hongu, T., Katagiri, N., Funakoshi, Y., Lee, H., et al. (2017). Arf6 in lymphatic endothelial cells regulates lymphangiogenesis by controlling directional cell migration. Sci. Rep. 7, 11431. doi:10.1038/s41598-017-11240-x
Lutze, G., Haarmann, A., Demanou Toukam, J. A., Buttler, K., Wilting, J., and Becker, J. (2019). Non-canonical WNT-signaling controls differentiation of lymphatics and extension lymphangiogenesis via RAC and JNK signaling. Sci. Rep. 9, 4739. doi:10.1038/s41598-019-41299-7
Ma, X., and Adelstein, R. S. (2014). A point mutation in Myh10 causes major defects in heart development and body wall closure. Circ. Cardiovasc. Genet. 7, 257–265. doi:10.1161/CIRCGENETICS.113.000455
Ma, W., Gil, H. J., Liu, X., Diebold, L. P., Morgan, M. A., Oxendine-Burns, M. J., et al. (2021). Mitochondrial respiration controls the Prox1-Vegfr3 feedback loop during lymphatic endothelial cell fate specification and maintenance. Sci. Adv. 7, eabe7359. doi:10.1126/sciadv.abe7359
Maciver, D. H. (2011). A new method for quantification of left ventricular systolic function using a corrected ejection fraction. Eur. J. Echocardiogr. 12, 228–234. doi:10.1093/ejechocard/jeq185
Mackie, D. I., al Mutairi, F., Davis, R. B., Kechele, D. O., Nielsen, N. R., Snyder, J. C., et al. (2018). hCALCRL mutation causes autosomal recessive nonimmune hydrops fetalis with lymphatic dysplasia. J. Exp. Med. 215, 2339–2353. doi:10.1084/jem.20180528
Majima, T., Takeuchi, K., Sano, K., Hirashima, M., Zankov, D. P., Tanaka-Okamoto, M., et al. (2013). An adaptor molecule afadin regulates lymphangiogenesis by modulating RhoA activity in the developing mouse embryo. PLoS ONE 8, e68134. doi:10.1371/journal.pone.0068134
Mak, M. C., Lam, K. M., Chan, P. K., Lau, Y. B., Tang, W. H., Yeung, P. K. K., et al. (2011). Embryonic lethality in mice lacking the nuclear factor of activated t cells 5 protein due to impaired cardiac development and function. PLoS ONE, 6, e19186. doi:10.1371/journal.pone.0019186
Mäkinen, T., Boon, L. M., Vikkula, M., and Alitalo, K. (2021). Lymphatic malformations: Genetics, mechanisms and therapeutic strategies. Circ. Res. 129, 136–154. doi:10.1161/CIRCRESAHA.121.318142
Martin-Almedina, S., Martinez-Corral, I., Holdhus, R., Vicente, A., Fotiou, E., Lin, S., et al. (2016). EPHB4 kinase-inactivating mutations cause autosomal dominant lymphatic-related hydrops fetalis. J. Clin. Invest. 126, 3080–3088. doi:10.1172/JCI85794
McLatchie, L. M., Fraser, N. J., Main, M. J., Wise, A., Brown, J., Thompson, N., et al. (1998). RAMPs regulate the transport and ligand specificity of the calcitonin-receptor-like receptor. Nature 393, 333–339. doi:10.1038/30666
Mellis, R., Eberhardt, R., Hamilton, S., McMullan, D., Kilby, M., Maher, E., et al. (2022). Fetal exome sequencing for isolated increased nuchal translucency: Should we be doing it? BJOG 129, 52–61. doi:10.1111/1471-0528.16869
Mirza, M., Pang, M. F., Zaini, M. A., Haiko, P., Tammela, T., Alitalo, K., et al. (2012). Essential role of the coxsackie - and adenovirus receptor (CAR) in development of the lymphatic system in mice. PLoS ONE 7, e37523. doi:10.1371/journal.pone.0037523
Morooka, N., Futaki, S., Sato-Nishiuchi, R., Nishino, M., Totani, Y., Shimono, C., et al. (2017). Polydom is an extracellular matrix protein involved in lymphatic vessel remodeling. Circ. Res. 120, 1276–1288. doi:10.1161/CIRCRESAHA.116.308825
Murtomaki, A., Uh, M. K., Choi, Y. K., Kitajewski, C., Borisenko, V., Kitajewski, J., et al. (2012). Notch1 functions as a negative regulator of lymphatic endothelial cell differentiation in the venous endothelium. Development 140, 2365–2376. doi:10.1242/dev.083865
Nicenboim, J., Malkinson, G., Lupo, T., Asaf, L., Sela, Y., Mayseless, O., et al. (2015). Lymphatic vessels arise from specialized angioblasts within a venous niche. Nature 522, 56–61. doi:10.1038/nature14425
Nicolaides, K. H., Azar, G., Byrne, D., Mansur, C., and Marks, K. (1992). Fetal nuchal translucency: Ultrasound screening for chromosomal defects in first trimester of pregnancy. BMJ 304, 867–869. doi:10.1136/bmj.304.6831.867
Noonan, J. A. (2006). Noonan syndrome and related disorders: Alterations in growth and puberty. Rev. Endocr. Metab. Disord. 7, 251–255. doi:10.1007/s11154-006-9021-1
Otowa, Y., Moriwaki, K., Sano, K., Shirakabe, M., Yonemura, S., Shibuya, M., et al. (2016). Flt1/VEGFR1 heterozygosity causes transient embryonic edema. Sci. Rep. 6, 27186. doi:10.1038/srep27186
Outeda, P., Huso, D. L., Fisher, S. A., Halushka, M. K., Kim, H., Qian, F., et al. (2014). Polycystin signaling is required for directed endothelial cell migration and lymphatic development. Cell Rep. 7, 634–644. doi:10.1016/j.celrep.2014.03.064
Paige, S. L., Plonowska, K., Xu, A., and Wu, S. M. (2015). Molecular regulation of cardiomyocyte differentiation. Circ. Res. 116, 341–353. doi:10.1161/CIRCRESAHA.116.302752
Pandit, B., Sarkozy, A., Pennacchio, L. A., Carta, C., Oishi, K., Martinelli, S., et al. (2007). Gain-of-function RAF1 mutations cause Noonan and LEOPARD syndromes with hypertrophic cardiomyopathy. Nat. Genet. 39, 1007–1012. doi:10.1038/ng2073
Park, T. J., Boyd, K., and Curran, T. (2006). Cardiovascular and craniofacial defects in Crk-null mice. Mol. Cell. Biol. 26, 6272–6282. doi:10.1128/mcb.00472-06
Pauta, M., Martinez-Portilla, R. J., and Borrell, A. (2022). Diagnostic yield of next-generation sequencing in fetuses with isolated increased nuchal translucency: Systematic review and meta-analysis. Ultrasound Obstet. Gynecol. 59, 26–32. doi:10.1002/uog.23746
Pereira, F., Teixeira, M. R., Dinis Ribeiro, M., and Brandão, C. (2021). Multi-gene panel testing in gastroenterology: Are we ready for the results? GE Port. J. Gastroenterol. 28, 403–409. doi:10.1159/000513966
Planas-Paz, L., Strilić, B., Goedecke, A., Breier, G., Fässler, R., and Lammert, E. (2012). Mechanoinduction of lymph vessel expansion. EMBO J. 31, 788–804. doi:10.1038/emboj.2011.456
Poole, B. D., Karetnyi, Y. V., and Naides, S. J. (2004). Parvovirus B19-induced apoptosis of hepatocytes. J. Virol. 78, 7775–7783. doi:10.1128/jvi.78.14.7775-7783.2004
Prefumo, F., Fichera, A., Fratelli, N., and Sartori, E. (2019). Fetal anemia: Diagnosis and management. Best. Pract. Res. Clin. Obstet. Gynaecol. 58, 2–14. doi:10.1016/j.bpobgyn.2019.01.001
Reid, S., and Pal, T. (2020). Update on multi-gene panel testing and communication of genetic test results. Breast J. 26, 1513–1519. doi:10.1111/tbj.13971
Ren, B., Deng, Y., Mukhopadhyay, A., Lanahan, A. A., Zhuang, Z. W., Moodie, K. L., et al. (2010). ERK1/2-Akt1 crosstalk regulates arteriogenesis in mice and zebrafish. J. Clin. Invest. 120, 1217–1228. doi:10.1172/JCI39837
Sanematsu, F., Hirashima, M., Laurin, M., Takii, R., Nishikimi, A., Kitajima, K., et al. (2010). DOCK180 is a rac activator that regulates cardiovascular development by acting downstream of CXCR4. Circ. Res. 107, 1102–1105. doi:10.1161/CIRCRESAHA.110.223388
Schlaepfer, I. R., and Joshi, M. (2020). CPT1A-mediated fat oxidation, mechanisms, and therapeutic potential. Endocrinology, 161, bqz046. doi:10.1210/endocr/bqz046
Shalaby, F., Rossant, J., Yamaguchi, T. P., Gertsenstein, M., Wu, X. F., Breitman, M. L., et al. (1995). Failure of blood-island formation and vasculogenesis in Flk-1-deficient mice. Nature 376, 62–66. doi:10.1038/376062a0
Shou, W., Aghdasi, B., Armstrong, D. L., Guo, Q., Bao, S., Charng, M-J., et al. (1998). Cardiac defects and altered ryanodine receptor function in mice lacking FKBP12. Nature 391, 489–492. doi:10.1038/35146
Sleutjes, J., Kleimeier, L., Leenders, E., Klein, W., and Draaisma, J. (2022). Lymphatic abnormalities in noonan syndrome spectrum disorders: A systematic review. Mol. Syndromol. 13, 1–11. doi:10.1159/000517605
Snijders, R. J. M., Noble, P., Sebire, N., Souka, A., and Nicolaides, K. H. (1998). UK multicentre project on assessment of risk of trisomy 21 by maternal age and fetal nuchal-translucency thickness at 10-14 weeks of gestation. Fetal Medicine Foundation First Trimester Screening Group. Lancet 352, 343–346. doi:10.1016/S0140-6736(97)11280-6
Soares, A. M. (2018). Mortality for critical congenital heart diseases and associated risk factors in newborns. A cohort study. Arq. Bras. Cardiol. 111, 674–675. doi:10.5935/abc.20180203
Souka, A. P., Krampl, E., Geerts, L., and Nicolaides, K. H. (2002). Congenital lymphedema presenting with increased nuchal translucency at 13 weeks of gestation. Prenat. Diagn. 22, 91–92. doi:10.1002/pd.104
Souka, A. P., von Kaisenberg, C. S., Hyett, J. A., Sonek, J. D., and Nicolaides, K. H. (2005). Increased nuchal translucency with normal karyotype. Am. J. Obstet. Gynecol. 192, 1005–1021. doi:10.1016/j.ajog.2004.12.093
Srinivasan, R. S., Dillard, M. E., Lagutin, O. V., Lin, F. J., Tsai, S., Tsai, M. J., et al. (2007). Lineage tracing demonstrates the venous origin of the mammalian lymphatic vasculature. Genes Dev. 21, 2422–2432. doi:10.1101/gad.1588407
Srinivasan, R. S., Geng, X., Yang, Y., Wang, Y., Mukatira, S., Studer, M., et al. (2010). The nuclear hormone receptor Coup-TFII is required for the initiation and early maintenance of Prox1 expression in lymphatic endothelial cells. Genes Dev. 24, 696–707. doi:10.1101/gad.1859310
Srinivasan, R. S., Escobedo, N., Yang, Y., Interiano, A., Dillard, M. E., Finkelstein, D., et al. (2014). The Prox1–Vegfr3 feedback loop maintains the identity and the number of lymphatic endothelial cell progenitors. Genes Dev. 28, 2175–2187. doi:10.1101/gad.216226.113
Srivastava, D., and Olson, E. N. (2000). A genetic blueprint for cardiac development. Nature 407, 221–226. doi:10.1038/35025190
Sumida, H., Noguchi, K., Kihara, Y., Abe, M., Yanagida, K., Hamano, F., et al. (2010). LPA4 regulates blood and lymphatic vessel formation during mouse embryogenesis. Blood 116, 5060–5070. doi:10.1182/blood-2010-03-272443
Suzuki-Inoue, K., Inoue, O., Ding, G., Nishimura, S., Hokamura, K., Eto, K., et al. (2010). Essential in vivo roles of the C-type lectin receptor CLEC-2: Embryonic/neonatal lethality of CLEC-2-deficient mice by blood/lymphatic misconnections and impaired thrombus formation of CLEC-2-deficient platelets. J. Biol. Chem. 285, 24494–24507. doi:10.1074/jbc.M110.130575
Tahmasebpour, A., Rafiee, N. B., Ghaffari, S., and Jamal, A. (2012). Increased nuchal translucency and pregnancy outcome. Iran. J. Public Health 41, 92–97.
Tammela, T., and Alitalo, K. (2010). Lymphangiogenesis: Molecular mechanisms and future promise. Cell 140, 460–476. doi:10.1016/j.cell.2010.01.045
Taniguchi, K., Kohno, R., Ayada, T., Kato, R., Ichiyama, K., Morisada, T., et al. (2007). Spreds are essential for embryonic lymphangiogenesis by regulating vascular endothelial growth factor receptor 3 signaling. Mol. Cell. Biol. 27, 4541–4550. doi:10.1128/mcb.01600-06
Tercanli, S., Miny, P., Siebert, M. S., Hösli, I., Surbek, D. V., and Holzgreve, W. (2001). Fanconi anemia associated with increased nuchal translucency detected by first-trimester ultrasound. Ultrasound Obstet. Gynecol. 17, 160–162. doi:10.1046/j.1469-0705.2001.00321.x
Tongsong, T., Tongprasert, F., Srisupundit, K., and Luewan, S. (2010). Venous Doppler studies in low-output and high-output hydrops fetalis. Am. J. Obstet. Gynecol. 203, e1–e6. doi:10.1016/j.ajog.2010.06.003
Urner, S., Planas-Paz, L., Hilger, L. S., Henning, C., Branopolski, A., Kelly-Goss, M., et al. (2019). Identification of ILK as a critical regulator of VEGFR 3 signalling and lymphatic vascular growth. EMBO J. 38, e99322. doi:10.15252/embj.201899322
van Loo, P. F., Mahtab, E. A. F., Wisse, L. J., Hou, J., Grosveld, F., Suske, G., et al. (2007). Transcription factor Sp3 knockout mice display serious cardiac malformations. Mol. Cell. Biol. 27, 8571–8582. doi:10.1128/mcb.01350-07
Vitelli, F., Morishima, M., Taddei, I., Lindsay, E., and Baldini, A. (2002). Tbx1 mutation causes multiple cardiovascular defects and disrupts neural crest and cranial nerve migratory pathways. Hum. Mol. Genet. 11, 915–922. doi:10.1093/hmg/11.8.915
Wakioka, T., Sasaki, A., Kato, R., Shouda, T., Matsumoto, A., Miyoshi, K., et al. (2001). Spred is a Sprouty-related suppressor of Ras signalling. Nature 412, 647–651. doi:10.1038/35088082
Wang, L., Yu, H., Cheng, H., He, K. E., Fang, Z., Ge, L., et al. (2017). Deletion of Stk40 impairs definitive erythropoiesis in the mouse fetal liver. Cell Death Dis. 8, e2722. doi:10.1038/cddis.2017.148
Wang, T., Wang, Z., de Fabritus, L., Tao, J., Saied, E. M., Lee, H. J., et al. (2021). 1-deoxysphingolipids bind to COUP-TF to modulate lymphatic and cardiac cell development. Dev. Cell 56, 3128–3145.e15. doi:10.1016/j.devcel.2021.10.018
Welsh, J. D., Kahn, M. L., and Sweet, D. T. (2016). Lymphovenous hemostasis and the role of platelets in regulating lymphatic flow and lymphatic vessel maturation. Blood 128, 1169–1173. doi:10.1182/blood-2016-04-636415
Wigle, J. T., and Oliver, G. (1999). Prox1 function is required for the development of the murine lymphatic system. Cell 98, 769–778. doi:10.1016/S0092-8674(00)81511-1
Wong, B. W., Wang, X., Zecchin, A., Thienpont, B., Cornelissen, I., Kalucka, J., et al. (2017). The role of fatty acid β-oxidation in lymphangiogenesis. Nature 542, 49–54. doi:10.1038/nature21028
Xin, M., Davis, C. A., Molkentin, J. D., Lien, C., Duncan, S. A., Richardson, J. A., et al. (2006). A threshold of GATA4 and GATA6 expression is required for cardiovascular development. Proc. Natl. Acad. Sci. U. S. A. 103, 11189–11194. doi:10.1073/pnas.0604604103
Xu, W., Wittchen, E. S., Hoopes, S. L., Stefanini, L., Burridge, K., and Caron, K. M. (2018). Small GTPase Rap1a/b is required for lymphatic development and adrenomedullin-induced stabilization of lymphatic endothelial junctions. Arterioscler. Thromb. Vasc. Biol. 38, 2410–2422. doi:10.1161/ATVBAHA.118.311645
Yang, Y., and Oliver, G. (2014). Development of the mammalian lymphatic vasculature. J. Clin. Invest. 124, 888–897. doi:10.1172/JCI71609
Yang, Y., García-Verdugo, J. M., Soriano-Navarro, M., Srinivasan, R. S., Scallan, J. P., Singh, M. K., et al. (2012). Lymphatic endothelial progenitors bud from the cardinal vein and intersomitic vessels in mammalian embryos. Blood 120, 2340–2348. doi:10.1182/blood-2012-05-428607
Yang, Y., Cha, B., Motawe, Z. Y., Srinivasan, R. S., and Scallan, J. P. (2019). VE-cadherin is required for lymphatic valve formation and maintenance. Cell Rep. 28, 2397–2412. doi:10.1016/j.celrep.2019.07.072
Yoshida, S., Miura, K., Yamasaki, K., Miura, S., Shimada, T., Tanigawa, T., et al. (2008). Does increased nuchal translucency indicate a fetal abnormality? A retrospective study to clarify the clinical significance of nuchal translucency in Japan. J. Hum. Genet. 53, 688–693. doi:10.1007/s10038-008-0299-6
Zhang, L., Zhou, F., Han, W., Shen, B., Luo, J., Shibuya, M., et al. (2010). VEGFR-3 ligand-binding and kinase activity are required for lymphangiogenesis but not for angiogenesis. Cell Res. 20, 1319–1331. doi:10.1038/cr.2010.116
Zhang, F., Zarkada, G., Yi, S., and Eichmann, A. (2020). Lymphatic endothelial cell junctions: Molecular regulation in physiology and diseases. Front. Physiol. 11, 509. doi:10.3389/fphys.2020.00509
Zheng, W., Tammela, T., Yamamoto, M., Anisimov, A., Holopainen, T, Kaijalainen, S., et al. (2011). Notch restricts lymphatic vessel sprouting induced by vascular endothelial growth factor. Blood 118, 1154–1162. doi:10.1182/blood-2010-11-317800
Keywords: fetal nuchal edema, gene mutations, lymphatic vascular development, cardiac anomaly, mouse embryos
Citation: Sugiyama A and Hirashima M (2022) Fetal nuchal edema and developmental anomalies caused by gene mutations in mice. Front. Cell Dev. Biol. 10:949013. doi: 10.3389/fcell.2022.949013
Received: 20 May 2022; Accepted: 02 August 2022;
Published: 30 August 2022.
Edited by:
Ramani Ramchandran, Medical College of Wisconsin, United StatesReviewed by:
Nathan Weinstein, Institute of Ecology, National Autonomous University of Mexico, MexicoSalvatore Saccone, University of Catania, Italy
Copyright © 2022 Sugiyama and Hirashima. This is an open-access article distributed under the terms of the Creative Commons Attribution License (CC BY). The use, distribution or reproduction in other forums is permitted, provided the original author(s) and the copyright owner(s) are credited and that the original publication in this journal is cited, in accordance with accepted academic practice. No use, distribution or reproduction is permitted which does not comply with these terms.
*Correspondence: Masanori Hirashima, bWFzYW5vcmlAbWVkLm5paWdhdGEtdS5hYy5qcA==