- Academy of Medical Engineering and Translational Medicine, Tianjin University, Tianjin, China
Revealing the mechanisms of neural development and the pathogenesis of neural diseases are one of the most challenging missions in life science. Pluripotent stem cells derived brain organoids mimic the development, maturation, signal generation, and function of human brains, providing unique advantage for neurology. Single-cell RNA sequencing (scRNA-Seq) and multielectrode array independently revealed the similarity between brain organoids and immature human brain at early developmental stages, in the context of gene transcription and dynamic network of neuronal signals. Brain organoids provided the unique opportunity to investigate the underlying mechanism of neural differentiation, senescence, and pathogenesis. In this review, we summarized the latest knowledge and technology in the brain organoid field, the current and potential applications in disease models and pre-clinic studies, with emphasizing the importance of transcriptional and epigenetic analysis.
Introduction
The human brain uses 100 billion neurons and 100 trillion connections between neurons to receive signals, generates consciousness, and sends orders to target organs, which makes it the almost perfect information processing system. All of the neurons derived from neural stem cells. The development of human brain is dependent on the information encoded in genomic sequence and regulated by epigenetic modification (Barkovich et al., 2012). Because of the critical ethical concerns, there are lots of untouchable field in the molecular mechanisms of human brain. Brain organoids can mimic the cell composition, hierarchical structure, electronical network, and disorders of human brain. From the perspective of transcriptome and epigenome atlas, significant similarity was observed between iPSCs (induced pluripotent stem cells) derived brain organoids and human brain (Amiri et al., 2018) (Figure 1). Reintroduction of a mutated gene in brain organoids provides not only the opportunity to study the function of a specific gene in the context of neural development, but also valuable disease models for neural diseases, which includes autism spectrum disorders, schizophrenia, and others.
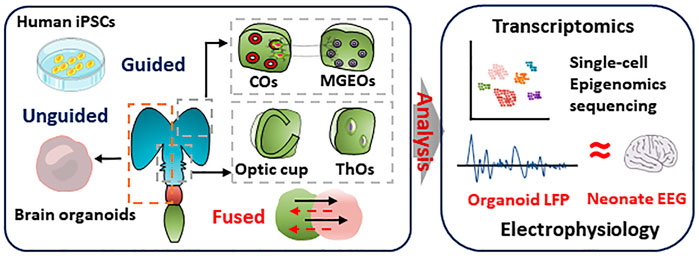
FIGURE 1. Genetic and epigenetic regulation in the brain organoids. Top, genetics in brain organoids. iPSCs carrying ASD or SCZ pathogenic genes are derived from patients or gene editing. The mutation of ASD or SCZ are referring to the commonly mutated genes in GWAS databases, which are shown in middle. The ASD organoids showed macrocephaly, microcephaly phenotypes, and over-produced GABAergic neurons. The SCZ-organoids characterized abnormal proliferation of progenitor, reduced mature neurons, and disruption of synaptic function. Bottom, epigenetics in brain organoids. Combined with single-cell sequencing, ATACseq and CHIP-seq analyze epigenetics during brain organoids and human brain development, including DNA methylation, histone variants, non-coding RNA, and chromatin accessibility. Epigenetics can regulate timing during development. Epigenetic regulation is deeply influenced by environmental factors. Exposure to toxic substances, viruses, alcohol, and stress may have epigenetic effects on fetal brain development. Brain organoids infected with Zika virus during early development showed epigenetic abnormalities, impaired progenitor proliferation as well as a distinct microcephaly phenotype.
Genetic Factors of Neural Development and Diseases: from Human Brain to Brain Organoids
Brain Organoids Provide Unique Advantage in Hominin Evolution Studies
There are many hominin lineage branches in the evolution path of humans. They have similar but slightly different genomic sequences. The fossil record provides the genetic sequences of Neanderthals and Denisovans (Green et al., 2010; Meyer et al., 2012). An interesting idea is investigating the contribution of genetic sequence difference in human brain evolution among different hominin lineages. It is not possible to directly compare the living cells and tissue between hominins and humans. Using iPSCs and CRISPR-Cas9 technology, hominin genes were introduced into human cells. Brain organoids were generated from iPSCs possess hominin genes. The differences in synaptogenesis, neural network, and electrophysical traits are observed between brain organoids with modern and archaic genes, which provide critical evidence to study the evolution of humans (Trujillo et al., 2021).
Brain Organoids Reveal the Genetic Mechanism of Neural Diseases
Brain organoids were used as disease models in multiple neural disorders, which are mainly caused by abnormal neural cell survival, proliferation, and differentiation. This is reviewed in some other reviews (Sidhaye and Knoblich 2021). For emphasizing the advantage of brain organoids in stem cell biology and neurology, we choose autism spectrum disorder (ASD) and schizophrenia to illustrate the unique role brain organoids played in revealing the pathogenesis mechanisms and discovering potential therapeutic strategies. Based on the genetic information, cortex structural, and functional regions, the correlation between DNA sequences and cortical surface area was established (Chen et al., 2012), which emphasizes the importance of genome in human brain development and function. Taking the advantage of whole gene sequencing, increasing disease-associated genes were identified.
The mutation of some genes was considered to contribute to the initiation of ASD, which includes but not limited to CAPRIN1, AFF2(Jiang et al., 2013). Another different kind of genetic change, copy number variation, was also linked to ASD (Levy et al., 2011) (Figure 2). Investigation revealed that deletion and duplication of genes have less impact on females than males. More researches are needed to answer if environmental factors played a role in the unequal frequency between different genders.
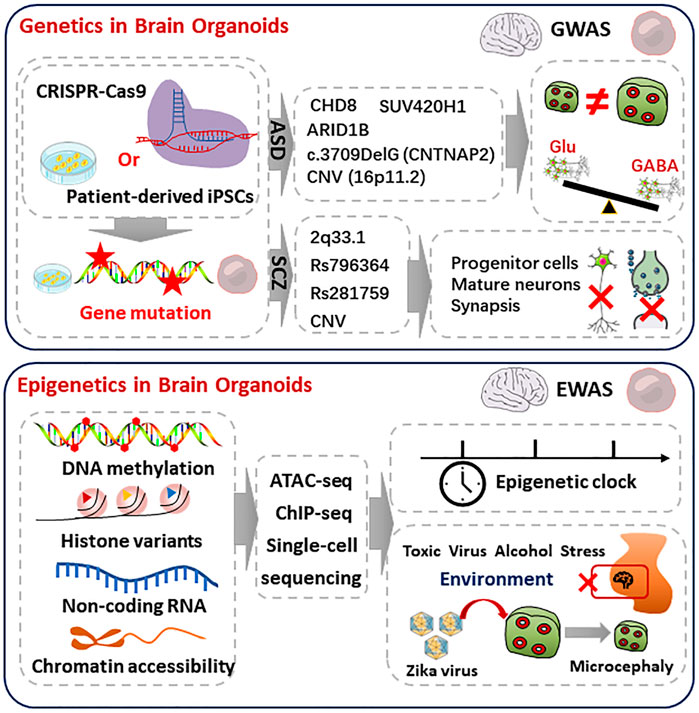
FIGURE 2. Reality of brain organoids in simulating human brain. Brain organoids and region-specific brain organoids were induced by undirected or directed differentiation, respectively. Single-cell sequencing and transcriptome analysis was performed to characterize the similarity of cellular composition and gene expression in brain organoids and human brain. Multi-electrode arrays detected the electrophysiological signals of cortical brain organoids that is similar to EEG signals in preterm infants
Besides mutation in the coding regions, more ASD-associated changes were observed in the non-coding region of genome. These regions not only include expression regulating factors, but also hold a number of regulations of splicing differences (Walker et al., 2019). Genetic sequencing of patient samples established the correlation between genes and neural diseases. However, it is hard to reveal the mechanisms by which the roles of disease-causing genes played in regulating neuron function and neural network, based on the data directly derived from patient samples. Based on scRNA-seq (Single-cell RNA sequencing) analysis, remarkable similarity was observed between in vitro human brain organoids and human fetal cortical tissues. Using the transcriptome-based unbiased clustering, neural progenitor cells and diverse differentiated cells were identified in brain organoids, which recapitulates the hierarchies, organization, and developmental trajectories of human fetal neocortex (Camp et al., 2015). However, no technique or evidence is available to investigate whether advanced neural functions exist in brain organoids.
Impairments in social communication and behavior patterns are the main clinical signs of ASD. The abnormal development of neural system of ASD starts from embryonic stage, which usually shows symptoms in childhood. Although genetic mutations are considered to be the principle causing of ASD, only 10% ASD patients were identified by known genetic condition. More investigations are needed to establish the clear linkage between inherited material and ASD. Thanks to stem cell and genome editing technology, patient-derived brain organoids provided the unique opportunity to investigate the mechanisms by which disease-driving genes impair the neurons. By comparing organoids derived from iPSCs of autism patients and their unaffected family members, differences in cell proliferation, synaptogenesis, and GABA/glutamate neuron differentiation were observed, although there is no significant neuronal organization and excitability. To explore the mechanism by which genes with different expression contribute to the ASD-associated phenotypes, FOXG1 was selected and its expression in brain organoids was regulated by lentiviruses carrying short hairpin RNAs, which is almost not possible in patients or patient-derived tissues. Attenuated FOXG1 expression restored the GABAergic neuronal differentiation to the normal level. These data revealed that FOXG1 might be an important driver of ASD neural phenotypes (Mariani et al., 2015).
Homozygous loss-of-function mutation in contactin-associated-protein-like 2 (CNTNAP2) causes a special kind of ASD, with the symptoms of early-onset epilepsy and increased head circumference (Strauss et al., 2006). Although knockouting Caspr2 causes some neural dysfunction, it also misses some critical disease-related symptoms, such as hyperproliferation of neural cells (Poliak et al., 2003). Increased proliferation and total number were observed in the brain organoids derived from ASD patients who harbored CNTNAP2 mutation, which provided the possible mechanism of increased head circumference of the patients. ScRNA-seq of organoids revealed that CNTNAP2 dominantly expressed in PFC-excitatory neurons and the targets genes regulated by CNTNAP2 mainly enriched in ASD-associated genes (de Jong et al., 2021). These studies emphasized the unique advantage of brain organoids in recapturing the phenotypes which are exclusively present in human neural system. Organoids helped neurologists uncover the role that inherited material plays in ASD. However, there is few investigations focusing on gene-environment interactions. More studies or new methods are needed to explore deeper mechanism of ASD from environmental factors perspectives.
One percent of human population suffers from schizophrenia, which is a chronic mental disorder with major signs of psychosis and cognitive impairment (Plooster et al., 2022). Most of the schizophrenia associated loci are protein-coding genes, which include involved not only glutamatergic neurotransmission and synaptic plasticity gene, but also the target of antipsychotic drugs. Besides brain tissue, schizophrenia linked genome association enriched in B lymphocytes as well, the antibody-producing cells (Schizophrenia Working Group of the Psychiatric Genomics 2014). This observation might explain the relationship between autoimmune diseases and schizophrenia, and the mechanism of some self-antibodies were found in schizophrenia patients with much higher proportion than healthy individuals (Benros et al., 2012).
Impaired neural differentiation and disrupted neuron quantity were observed in schizophrenia patient-derived organoids, compared with the organoids derived from healthy donors. Compared to organoids derived from healthy donors, there are more apoptosis, neural progenitors, in the ventricular zone of schizophrenia patients derived organoids (Notaras et al., 2021a). This is one of the unique advantages of 3D organoids, as brain structures usually do not exist in 2D in vitro neural culture.
Schizophrenia-derived iPSCs and the derived brain organoids provide novel in vitro model to investigate the psychiatric disorder from the perspective of etiology, mechanism, and therapy. Hundreds of different expression genes between schizophrenia patients and healthy donors derived organoids were identified by total RNA sequencing. Almost quarter of these genes are overlapped with schizophrenia associated genes discovered by GWAS (Kathuria et al., 2020). They enriched in neurodevelopment, metabolism, neural function, and immune responses. Although the genes which differently expressed in schizophrenia organoids enriched in immune response as well, few researches investigated the brain organoids from immunological perspectives. Schizophrenia organoids were also subjected to quantitative proteomic analysis. Similar defects were observed in axon and neuronal differentiation at protein level (Notaras et al., 2021b), which is consistent with transcriptome and genome researches.
Epigenetic Modification of Human Brain and Brain Organoids
Epigenome is a reversible change of inherited materials. Epigenetic change does not alter a DNA sequence. It changes and tunes the transcription of DNA sequence. Epigenomics mainly encompasses DNA methylation, histone modification, and noncoding RNA mediated transcriptional regulation. Environment, age, behavior, and diseases can cause epigenetic changes (Figure 2). Epigenetic regulation plays a critical role in tissue development, homeostasis, pathogenesis, and senescence (Vezzani et al., 2022).
On top of protein-coding genes, non-coding regions of human genome are also found to be critical in human cortical neurogenesis (de la Torre-Ubieta et al., 2018).
Epigenetic and gene expression between human and non-human primate brains are extremely distinct due to evolution of species, which are expected to interpret the epigenetic changes in human-specific sequences (Mendizabal et al., 2016). The interplay of the activation and inhibition of multiple developmental signaling pathways in brain development was tightly controlled by epigenetics (Imamura et al., 2014). Whole-genome single-base analysis revealed that dynamic genome non-CG methylation occurred in the brain development of fetal to adult, which characterized constant changes at different developmental stages and specifically accumulated in neurons or glial cells, respectively (Lister et al., 2013). The cell-type-specific 3D epigenomes revealed new laws of cis-acting element regulation of gene expression during cell fate determination, was expected to provide new reference data for brain development and neuropsychiatric disorders (Song et al., 2020). The epigenetics of iPSC-derived neurons has been extensively studied, which was limited by the lack of complex networks (Vieira et al., 2019).
Based on histone mark (methylation and acetylation) ChIP-seq brain organoids at different developmental stages, dynamic epigenetic traits were observed along with organoids maturation. After compared with brain tissue data from the PsychENCODE developmental dataset, similar enhancer active models were observed between brain organoids and human brain development. This implied that brain organoids might be a good tool to recapitulate neural system development. As epigenetic modification is considered as reversible, brain organoids are also a valuable platform to investigate and evaluate potential therapies.
Epigenome-wide association studies (EWAS) (Verma, 2016) is an important method to study the variation in epigenetic level, mainly DNA methylation. In a study focusing on AD, EWAS revealed that ankyrin 1 (ANK1) is one of the AD-associated genes with abnormal epigenetic modification. The hypermethylation of ANK1 was observed in AD manifested region of brain, the entorhinal cortex, not in the regions which were protected from AD-related neurodegeneration (cerebellum), nor peripheral blood (Lunnon et al., 2014). Increased evidence suggested that epigenetic change played an important role in the development of schizophrenia. However, genetic studies dominate this niche, outnumbering epigenetic studies. More investigation, especially fundamental basic studies, about the contribution of epigenetic factors is needed to clarify the development of schizophrenia and find potential interventions for mental illness (Francisco et al., 2022). Brain organoid recaptured early non-CG methylation (an epigenetic modification suppressing gene expression) of super-enhancers and demethylation profiles of fatal human brain (Luo et al., 2016). This supports the possibility of using organoids to model epigenetic change in human brain development and neural disorders.
The opening or closing of genes anomalously controlled by epigenetics could lead to abnormal gene expression and neurodevelopmental disorders. Especially, environmental factors cannot be ignored. Prenatal exposure to risk factors such as alcohol, heavy metals, toxic substances, as well as maternal immune activation may induce epigenetic changes (Portales-Casamar et al., 2016). Epigenetic changes in brain organoids infected with the Zika virus were analyzed by whole-genome bisulfite sequencing (WGBS) beyond ethical and species-specific limitations. The results showed that Zika virus was able to alter DNA methylation at specific gene loci and infected-brain organoids displayed a microcephaly phenotype (Janssens et al., 2018). Epigenetic editing regulates target gene expression from the transcription without modification of gene sequences (Holtzman and Gersbach 2018). Epigenetic alterations have a potential of reshaping the mechanism between chromatin state, gene regulation, and cellular phenotype (Jurkowski et al., 2015). Epigenetic editing based on brain organoids is expected to reveal disease mechanisms and treatment techniques in vitro. Based on CRISPR-cas9 system, precise genetic editing was achieved and was used in brain organoids to investigating gene function in neural development and their contribution in neural diseases. However, epigenetic editing tools usually act globally. Site specific epigenetic editing may not only improve current understanding about the role of epigenetic marks played in neural diseases, but also provide novel opportunity to develop next generation therapeutic strategies targeting epigenetic factors.
Conclusion
Brain organoid shows extraordinary potential in neurology. The differentiation of stem cells in brain organoids is still a self-organization process. Although different brain regions are generated by regionally specific culture system, many human brain structures do not exist in brain organoids. Few neural development and diseases can be recapitulated in brain organoids. Revolution or professional cell culture devices are needed to improve the cellular and structure diversity of brain organoids.
Functional maturation of a brain organoid needs more than 3 months. Reliable electrical signals usually merge arise 6-month differentiation. This extremely long in vitro culture makes brain organoids to be vulnerable to contamination of infection and external environment. It also increases the organoid-to-organoid variability, which seriously impaired the comparability of data generated from different laboratories. Although significant similarity was observed between human brain and brain organoids, there is still nonnegligible brain-organoids variation, in the perspective of electrical function, cellular diversity, and structural integrity. Electrical activity is one of the most critical neural functions of brain organoids. Traditional MEA can not measure the inside electrical signal of 3D brain organoids. Although few studies use 3D electrodes analyzing the deep signal of brain organoids, the number of electrodes is much smaller than MEA. Besides transcriptomic similarity, brain organoids show action potentials and electrophysiological responses, the signature characteristics of human brain neural network (Logan et al., 2020). Some computational toolkits, such as VoxHunt, were developed to anchor the organoid data to brain references, which accelerated and significantly improve the accuracy in organoids analysis (Fleck et al., 2021). Although there are still some technical obstacles. Brain organoids provide a unique and unreplaceable opportunity to build up, investigate, and redesign the neuron, the neural diseases, and the neural system. It will also be a benefit to developing novel therapeutic strategies.
Author Contributions
Y-WW: writing- reviewing and editing, conceptualization. NH: data curation, writing- original draft preparation. X-HL: supervision, conceptualization and project administration. All authors listed have made a substantial, direct, and intellectual contribution to the work and approved it for publication.
Funding
This work was supported by the National Key Research and Development Plan of China (2021YFF1200800), the National Nature Scientific Fund of China (82171861).
Conflict of Interest
The authors declare that the research was conducted in the absence of any commercial or financial relationships that could be construed as a potential conflict of interest.
Publisher’s Note
All claims expressed in this article are solely those of the authors and do not necessarily represent those of their affiliated organizations, or those of the publisher, the editors and the reviewers. Any product that may be evaluated in this article, or claim that may be made by its manufacturer, is not guaranteed or endorsed by the publisher.
Abbreviations
COs, cortical brain organoids; MGEOs, medial ganglion protrusion brain organoids; ThOs, thalamic organoids; iPSCs, induced pluripotent stem cells; GWAS, Genome-wide association study; EWAS, epigenome-wide association studies; ASD, autistic spectrum disorders; SCZ, schizophrenia; CNV, Copy number variations; ATAC-seq, Assay for Transposase-Accessible Chromatin with high throughput sequencing; CHIP-seq, Chromatin Immunoprecipitation Sequencing
References
Amiri, A., Coppola, G., Scuderi, S., Wu, F., Roychowdhury, T., Liu, F., et al. (2018). Transcriptome and Epigenome Landscape of Human Cortical Development Modeled in Organoids. Science 362, eaat6720. doi:10.1126/science.aat6720
Barkovich, A. J., Guerrini, R., Kuzniecky, R. I., Jackson, G. D., and Dobyns, W. B. (2012). A Developmental and Genetic Classification for Malformations of Cortical Development: Update 2012. Brain 135, 1348–1369. doi:10.1093/brain/aws019
Benros, M. E., Mortensen, P. B., and Eaton, W. W. (2012). Autoimmune Diseases and Infections as Risk Factors for Schizophrenia. Ann. N. Y. Acad. Sci. 1262, 56–66. doi:10.1111/j.1749-6632.2012.06638.x
Camp, J. G., Badsha, F., Florio, M., Kanton, S., Gerber, T., Wilsch-Bräuninger, M., et al. (2015). Human Cerebral Organoids Recapitulate Gene Expression Programs of Fetal Neocortex Development. Proc. Natl. Acad. Sci. U.S.A. 112, 15672–15677. doi:10.1073/pnas.1520760112
Chen, C.-H., Gutierrez, E. D., Thompson, W., Panizzon, M. S., Jernigan, T. L., Eyler, L. T., et al. (2012). Hierarchical Genetic Organization of Human Cortical Surface Area. Science 335, 1634–1636. doi:10.1126/science.1215330
de Jong, J. O., Llapashtica, C., Genestine, M., Strauss, K., Provenzano, F., Sun, Y., et al. (2021). Cortical Overgrowth in a Preclinical Forebrain Organoid Model of CNTNAP2-Associated Autism Spectrum Disorder. Nat. Commun. 12, 4087. doi:10.1038/s41467-021-24358-4
Fleck, J. S., Sanchís-Calleja, F., He, Z., Santel, M., Boyle, M. J., Camp, J. G., et al. (2021). Resolving Organoid Brain Region Identities by Mapping Single-Cell Genomic Data to Reference Atlases. Cell. Stem Cell. 28, 1148–1159. doi:10.1016/j.stem.2021.02.015
Green, R. E., Krause, J., Briggs, A. W., Maricic, T., Stenzel, U., Kircher, M., et al. (2010). A Draft Sequence of the Neandertal Genome. Science 328, 710–722. doi:10.1126/science.1188021
Holtzman, L., and Gersbach, C. A. (2018). Editing the Epigenome: Reshaping the Genomic Landscape. Annu. Rev. Genom. Hum. Genet. 19, 43–71. doi:10.1146/annurev-genom-083117-021632
Imamura, T., Uesaka, M., and Nakashima, K. (2014). Epigenetic Setting and Reprogramming for Neural Cell Fate Determination and Differentiation. Phil. Trans. R. Soc. B 369, 20130511. doi:10.1098/rstb.2013.0511
Janssens, S., Schotsaert, M., Karnik, R., Balasubramaniam, V., Dejosez, M., Meissner, A., et al. (2018). Zika Virus Alters DNA Methylation of Neural Genes in an Organoid Model of the Developing Human Brain. mSystems 3, e00219–17. doi:10.1128/mSystems.00219-17
Jiang, Y.-h., Yuen, R. K. C., Jin, X., Wang, M., Chen, N., Wu, X., et al. (2013). Detection of Clinically Relevant Genetic Variants in Autism Spectrum Disorder by Whole-Genome Sequencing. Am. J. Hum. Genet. 93, 249–263. doi:10.1016/j.ajhg.2013.06.012
Jurkowski, T. P., Ravichandran, M., and Stepper, P. (2015). Synthetic Epigenetics-Towards Intelligent Control of Epigenetic States and Cell Identity. Clin. Epigenet 7, 18. doi:10.1186/s13148-015-0044-x
Kathuria, A., Lopez-Lengowski, K., Jagtap, S. S., McPhie, D., Perlis, R. H., Cohen, B. M., et al. (2020). Transcriptomic Landscape and Functional Characterization of Induced Pluripotent Stem Cell-Derived Cerebral Organoids in Schizophrenia. JAMA Psychiatry 77, 745–754. doi:10.1001/jamapsychiatry.2020.0196
Levy, D., Ronemus, M., Yamrom, B., Lee, Y.-h., Leotta, A., Kendall, J., et al. (2011). Rare De Novo and Transmitted Copy-Number Variation in Autistic Spectrum Disorders. Neuron 70, 886–897. doi:10.1016/j.neuron.2011.05.015
Lister, R., Mukamel, E. A., Nery, J. R., Urich, M., Puddifoot, C. A., Johnson, N. D., et al. (2013). Global Epigenomic Reconfiguration during Mammalian Brain Development. Science 341, 1237905. doi:10.1126/science.1237905
Logan, S., Arzua, T., Yan, Y., Jiang, C., Liu, X., Yu, L.-K., et al. (2020). Dynamic Characterization of Structural, Molecular, and Electrophysiological Phenotypes of Human-Induced Pluripotent Stem Cell-Derived Cerebral Organoids, and Comparison with Fetal and Adult Gene Profiles. Cells 9, 1301. doi:10.3390/cells9051301
Lunnon, K., Smith, R., Hannon, E., De Jager, P. L., Srivastava, G., Volta, M., et al. (2014). Methylomic Profiling Implicates Cortical Deregulation of ANK1 in Alzheimer's Disease. Nat. Neurosci. 17, 1164–1170. doi:10.1038/nn.3782
Luo, C., Lancaster, M. A., Castanon, R., Nery, J. R., Knoblich, J. A., and Ecker, J. R. (2016). Cerebral Organoids Recapitulate Epigenomic Signatures of the Human Fetal Brain. Cell. Rep. 17, 3369–3384. doi:10.1016/j.celrep.2016.12.001
Marcelo, B., Francisco, R., Fernando, V., Norma, E., and Madai, M. (2022). Glial Changes in Schizophrenia: Genetic and Epigenetic Approach. Indian J. Psychiatry 64, 3–12. doi:10.4103/indianjpsychiatry.indianjpsychiatry_104_21
Mariani, J., Coppola, G., Zhang, P., Abyzov, A., Provini, L., Tomasini, L., et al. (2015). FOXG1-Dependent Dysregulation of GABA/Glutamate Neuron Differentiation in Autism Spectrum Disorders. Cell. 162, 375–390. doi:10.1016/j.cell.2015.06.034
Mendizabal, I., Shi, L., Keller, T. E., Konopka, G., Preuss, T. M., Hsieh, T.-F., et al. (2016). Comparative Methylome Analyses Identify Epigenetic Regulatory Loci of Human Brain Evolution. Mol. Biol. Evol. 33, 2947–2959. doi:10.1093/molbev/msw176
Meyer, M., Kircher, M., Gansauge, M.-T., Li, H., Racimo, F., Mallick, S., et al. (2012). A High-Coverage Genome Sequence from an Archaic Denisovan Individual. Science 338, 222–226. doi:10.1126/science.1224344
Notaras, M., Lodhi, A., Dündar, F., Collier, P., Sayles, N. M., Tilgner, H., et al. (2021a). Schizophrenia Is Defined by Cell-specific Neuropathology and Multiple Neurodevelopmental Mechanisms in Patient-Derived Cerebral Organoids. Mol. Psychiatry 27, 1416–1434. doi:10.1038/s41380-021-01316-6
Notaras, M., Lodhi, A., Fang, H., Greening, D., and Colak, D. (2021b). The Proteomic Architecture of Schizophrenia iPSC-Derived Cerebral Organoids Reveals Alterations in GWAS and Neuronal Development Factors. Transl. Psychiatry 11, 541. doi:10.1038/s41398-021-01664-5
Paulsen, B., Velasco, S., Kedaigle, A. J., Pigoni, M., Quadrato, G., Deo, A. J., et al. (2022). Autism Genes Converge on Asynchronous Development of Shared Neuron Classes. Nature 602, 268–273. doi:10.1038/s41586-021-04358-6
Plooster, M., Brennwald, P., and Gupton, S. L. (2022). Endosomal Trafficking in Schizophrenia. Curr. Opin. Neurobiol. 74, 102539. doi:10.1016/j.conb.2022.102539
Poliak, S., Salomon, D., Elhanany, H., Sabanay, H., Kiernan, B., Pevny, L., et al. (2003). Juxtaparanodal Clustering of Shaker-like K+ Channels in Myelinated Axons Depends on Caspr2 and TAG-1. J. Cell. Biol. 162, 1149–1160. doi:10.1083/jcb.200305018
Portales-Casamar, E., Lussier, A. A., Jones, M. J., MacIsaac, J. L., Edgar, R. D., Mah, S. M., et al. (2016). DNA Methylation Signature of Human Fetal Alcohol Spectrum Disorder. Epigenetics Chromatin 9, 25. doi:10.1186/s13072-016-0074-4
Qian, X., Su, Y., Adam, C. D., Deutschmann, A. U., Pather, S. R., Goldberg, E. M., et al. (2020). Sliced Human Cortical Organoids for Modeling Distinct Cortical Layer Formation. Cell. Stem Cell. 26, 766–781. doi:10.1016/j.stem.2020.02.002
Schizophrenia Working Group of the Psychiatric Genomics C (2014). Biological Insights from 108 Schizophrenia-Associated Genetic Loci. Nature 511, 421–427. doi:10.1038/nature13595
Sidhaye, J., and Knoblich, J. A. (2021). Brain Organoids: an Ensemble of Bioassays to Investigate Human Neurodevelopment and Disease. Cell. Death Differ. 28, 52–67. doi:10.1038/s41418-020-0566-4
Singh, K. K., De Rienzo, G., Drane, L., Mao, Y., Flood, Z., Madison, J., et al. (2011). Common DISC1 Polymorphisms Disrupt Wnt/GSK3β Signaling and Brain Development. Neuron 72, 545–558. doi:10.1016/j.neuron.2011.09.030
Song, M., Pebworth, M.-P., Yang, X., Abnousi, A., Fan, C., Wen, J., et al. (2020). Cell-type-specific 3D Epigenomes in the Developing Human Cortex. Nature 587, 644–649. doi:10.1038/s41586-020-2825-4
Stein, J. L., Won, H., Opland, C. K., Liang, D., Lu, D., et al. (2018). The Dynamic Landscape of Open Chromatin during Human Cortical Neurogenesis. Cell. 172, 289–304. doi:10.1016/j.cell.2017.12.014
Strauss, K. A., Puffenberger, E. G., Huentelman, M. J., Gottlieb, S., Dobrin, S. E., Parod, J. M., et al. (2006). Recessive Symptomatic Focal Epilepsy and Mutant Contactin-Associated Protein-like 2. N. Engl. J. Med. 354, 1370–1377. doi:10.1056/NEJMoa052773
Trujillo, C. A., Rice, E. S., Schaefer, N. K., Chaim, I. A., Wheeler, E. C., Madrigal, A. A., et al. (2021). Reintroduction of the Archaic Variant of NOVA1 in Cortical Organoids Alters Neurodevelopment. Science 371, eaax2537. doi:10.1126/science.aax2537
Urresti, J., Zhang, P., Moran-Losada, P., Yu, N. K., Negraes, P. D., Trujillo, C. A., et al. (2021). Cortical Organoids Model Early Brain Development Disrupted by 16p11.2 Copy Number Variants in Autism. Mol. Psychiatry 26, 7560–7580. doi:10.1038/s41380-021-01243-6
Verma, M. (2016). Genome-wide Association Studies and Epigenome-wide Association Studies Go Together in Cancer Control. Future Oncol. 12, 1645–1664. doi:10.2217/fon-2015-0035
Vezzani, B., Carinci, M., Previati, M., Giacovazzi, S., Della Sala, M., Gafà, R., et al. (2022). Epigenetic Regulation: A Link between Inflammation and Carcinogenesis. Cancers 14, 1221. doi:10.3390/cancers14051221
Vieira, M. S., Goulart, V. A. M., Parreira, R. C., Oliveira-Lima, O. C., Glaser, T., Naaldijk, Y. M., et al. (2019). Decoding Epigenetic Cell Signaling in Neuronal Differentiation. Seminars Cell. & Dev. Biol. 95, 12–24. doi:10.1016/j.semcdb.2018.12.006
Walker, R. L., Ramaswami, G., Hartl, C., Mancuso, N., Gandal, M. J., et al. (2019). Genetic Control of Expression and Splicing in Developing Human Brain Informs Disease Mechanisms. Cell. 179, 750–771. doi:10.1016/j.cell.2019.09.021
Wang, P., Mokhtari, R., Pedrosa, E., Kirschenbaum, M., Bayrak, C., Zheng, D., et al. (2017). CRISPR/Cas9-mediated Heterozygous Knockout of the Autism Gene CHD8 and Characterization of its Transcriptional Networks in Cerebral Organoids Derived from iPS Cells. Mol. Autism 8, 11. doi:10.1186/s13229-017-0124-1
Keywords: brain organoids, gene mutation, epigenetics, autistic spectrum disorders, genetics
Citation: Wang Y-W, Hu N and Li X-H (2022) Genetic and Epigenetic Regulation of Brain Organoids. Front. Cell Dev. Biol. 10:948818. doi: 10.3389/fcell.2022.948818
Received: 20 May 2022; Accepted: 09 June 2022;
Published: 01 July 2022.
Edited by:
Nathalie Beaujean, l’alimentation et l’environnement (INRAE), FranceCopyright © 2022 Wang, Hu and Li. This is an open-access article distributed under the terms of the Creative Commons Attribution License (CC BY). The use, distribution or reproduction in other forums is permitted, provided the original author(s) and the copyright owner(s) are credited and that the original publication in this journal is cited, in accordance with accepted academic practice. No use, distribution or reproduction is permitted which does not comply with these terms.
*Correspondence: Xiao-Hong Li, eGhsaTE4QHRqdS5lZHUuY24=
†These authors have contributed equally to this work