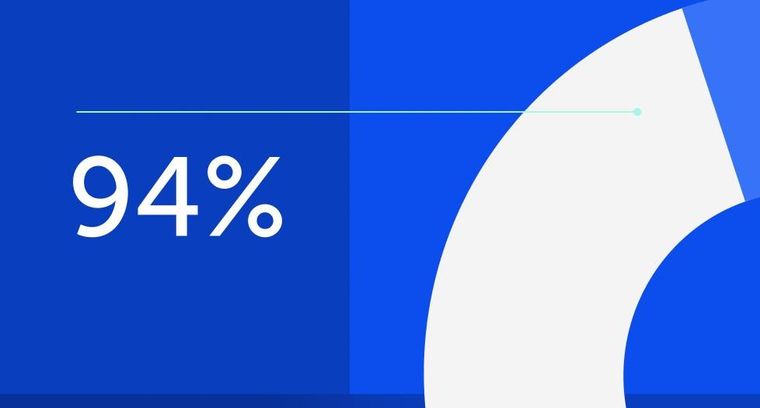
94% of researchers rate our articles as excellent or good
Learn more about the work of our research integrity team to safeguard the quality of each article we publish.
Find out more
REVIEW article
Front. Cell Dev. Biol., 02 September 2022
Sec. Molecular and Cellular Pathology
Volume 10 - 2022 | https://doi.org/10.3389/fcell.2022.948395
This article is part of the Research TopicCellular Reprogramming in Development, Disease, and Design of Cell-Replacement TherapiesView all 5 articles
The differentiation of human pluripotent stem cells (hPSCs) towards organoids is one of the biggest scientific advances in regenerative medicine. Kidney organoids have not only laid the groundwork for various organ-like tissue systems but also provided insights into kidney embryonic development. Thus, several protocols for the differentiation of renal progenitors or mature cell types have been established. Insights into the interplay of developmental pathways in nephrogenesis and determination of different cell fates have enabled the in vitro recapitulation of nephrogenesis. Here we first provide an overview of kidney morphogenesis and patterning in the mouse model in order to dissect signalling pathways that are key to define culture conditions sustaining renal differentiation from hPSCs. Secondly, we also highlight how genome editing approaches have provided insights on the specific role of different genes and molecular pathways during renal differentiation from hPSCs. Based on this knowledge we further review how CRISPR/Cas9 technology has enabled the recapitulation and correction of cellular phenotypes associated with human renal disease. Last, we also revise how the field has positively benefited from emerging technologies as single cell RNA sequencing and discuss current limitations on kidney organoid technology that will take advantage from bioengineering solutions to help standardizing the use of this model systems to study kidney development and disease.
Kidney diseases are world-wide a leading cause for mortality and health care costs. Chronic kidney disease (CKD) -defined as the chronic decline of glomerular filtration rate-is a risk factor for premature death (Webster et al., 2017) There are rarely therapies for underlying causes of CKD and it’s end stage renal failure (ESRD) can only be cured by renal replacement therapy (RRT) or transplantation (Wouters et al., 2015). However, long-term RRT itself poses a health risk while access to kidney transplantation is severely restricted because of the significant shortage of kidney donors. Thus, there is an urgent need for improved public awareness, prevention strategies, early detection, education, and development of therapies for CKD in clinical practice (Zoccali et al., 2018).
However, modelling kidney (patho)- physiology and function in the animal model had been hampered for a long time by the fact that most in vivo models do not fully and reliably recapitulate the human kidney (Kumar et al., 2014). Also, in vitro models lack reliability and feasibility because primary cells from patients with a lot of common renal diseases are difficult to obtain and culture long-term. Kidney-derived cell lines don’t display all characteristics of their original cell or maintain specific features only a short amount of time and therefore provide limited insights of an organ consisting of 26 distinct cells with various functions from pH regulation, excretion, erythropoiesis or blood pressure regulation (Baer et al., 1999; Al-Awqati and Oliver, 2002; Jenkinson et al., 2012). Therefore, the possibility to direct human pluripotent stem cells (hPSCs) towards a renal lineage opens exciting opportunities to develop new in vitro models of the kidney.
The mammalian kidney, the metanephros, is formed of complex epithelial tubules, the nephrons. Each single nephron connects with the collecting duct network through which the urinary filtrate passes to exit the kidney and move to the bladder. In humans, each of our kidneys consists of up to 2 million nephrons (Bertram et al., 2011), whereas in the mouse, this number is around 15,000 per each organ (Merlet-Benichou et al., 1999). As in humans nephron formation is exhausted by birth a lower number in nephrons due to hypertension or smoking during pregnancy has been shown to be associated with a later risk to develop CKD (Hinchliffe et al., 1991). Hence, there is no postnatal stem cell able to replace or generate de novo lost nephrons in humans.
Interestingly, it has been described that the adults of simple vertebrates like elasmobranch (Elger et al., 2003) and teleost fishes (Reimschuessel and Williams, 1995), as well as amphibians (Gray, 1930) and reptiles (Solomon, 1985) have the ability to regenerate entire nephrons but this ability seems to have been reduced to only the repair of nephron parts in adult mammals during the evolution (Romagnani et al., 2013). Due to the existence of such regenerative potential across the animal phylia, it has been postulated that the possibility to emulate such mechanisms in the mammalian kidney would represent an alternative strategy to promote kidney regeneration with no need of cell replacement therapies. In this regard, the regeneration after renal injury in different experimental animal models, and even humans, has been of great interest in the field (Ho et al., 2008; Shi et al., 2008; Soufi et al., 2012).
Potentially replacing damaged cells by nephron progenitors has emerged as an alternative strategy in regenerative medicine. Nephron progenitor cells (NPCs) can be isolated from the mouse embryonic kidney and further identification of cell culture conditions for their expansion in vitro raises the prospect of nephrogenesis in vitro (Brown et al., 2015). Nevertheless, access to NPCs from human embryonic kidneys is not affordable and raises important ethical concerns. An alternative approach lies in the identification of human cell sources with renal differentiation potential. Although for a long time not clear if a hierarchical relationship between NPC and mature renal cells do exist, this strategy laid the groundwork for the successful in vitro recapitulation of nephrogenesis. In this regard, hPSCs due to their capacity to differentiate into all three embryonic germ layers represent a unique cell source to virtually generate any cell type of our body. Profiting from this characteristic both human embryonic stem cells (hESCs) (Thomson et al., 1998) and human induced pluripotent stem cells (hiPSCs) (Takahashi et al., 2007) have been used to mimic early steps of human tissue specification and differentiation. This has led to the establishment of cell culture procedures which recapitulate developmental pathways by culturing hPSCs in cell culture media containing soluble factors or chemical compounds emulating the biochemical signalling that hPSCs encounter during tissue development. Initial approaches have largely been relying on the use of other external stimuli as extracellular matrix proteins promoting differentiation or the use of supportive cells as producers of paracrine signalling. However, this turned out to be neither cost effective nor feasible regarding prospectives of regenerative medicine like developing cell-based therapies or patient-derived tissues to regenerate a damaged organ.
In general, the hPSCs field has profited from the extensive studies of mouse kidney development and mouse hPSCs differentiation in 2D conditions. These approaches were also performed through the generation of hPSCs aggregate-like structures named embryoid bodies (EBs). For instance, this knowledge was key for the establishment of the first renal-like cells using mouse embryonic stem cells (Kim and Dressler, 2005; Bruce et al., 2007; Vigneau et al., 2007; Morizane et al., 2013; Taguchi et al., 2014). Later, other approaches did take advantage of 2D monolayer cultures from both human and mouse PSCs to derive renal progenitor cells that showed renal differentiation potential in vivo or ex vivo (Song et al., 2012; Narayanan et al., 2013). Building upon these findings, the work of different research groups, including Izpisúa Belmonte (Xia et al., 2013), Bonventre (Morizane et al., 2015) or Little (Takasato et al., 2015), proved the possibility to generate three dimensional (3D) cultures that mimic the embryonic kidney in terms of cellular composition and a wide variety of cell identities capable to functionally respond to external insults. In this context, a recent study has revealed that kidney organoids can faithfully model renal intrinsic repair upon toxin administration as well as a transition to an incomplete repair upon repetitive and a more aggressive insult, which mimics an in vivo kidney’s capacity faithfully (Gupta et al., 2022). Later on, the use of microfluidic platforms has allowed for the differentiation with generation of single renal cell types (Musah et al., 2017) or the vascularization of kidney organoids (Homan et al., 2019).
A more recent advance in the field is the combination of organoid technology with gene editing. This approach enables the generation of patient-derived hPSCs or genetically manipulated hPSCs carrying a disease-related mutation to further explore how genetic alterations impact kidney organoid phenotypes and function. Recent findings show that combining both kidney organoids and CRISPR/Cas9 enable the recapitulation of important physiopathological mechanisms such as cyst formation in renal polycystic disease (Xia et al., 2013; Freedman et al., 2015) or identification of the central role of podocyte microvilli in kidney dysfunction (Kim et al., 2017).
Based on these findings this review aims to provide a comprehensive overview on the latest advances in kidney organoid derivation to further discuss how the application of genome editing in these model systems is opening new possibilities to model kidney disease. We first provide an overview of the establishment of the first protocols for kidney organoid derivation. Next, we revise the different works using CRISPR/Cas9 technology to model renal disease and finally we discuss the utility of genome editing in the field of kidney organoid engineering to understand kidney development and disease. We will also provide an outlook on how the application of bioengineering is expected to improve our understanding of controlling and guiding kidney organoid maturation and function.
The adult kidney is composed of specialized cell types, including epithelial, endothelial, and stromal components. Both the collecting duct system and the nephron epithelium of the kidney represent the major epithelial component of this organ. These cell types emerge soon during development from a common lineage, the intermediate mesoderm (IM) which derives from the primitive streak (PS). The IM appears soon after gastrulation, in humans, by embryonic day 22 (E8.0 in mice) and is so-called due to its specific location along the mediolateral axis of the embryo between the axial (or somitic mesoderm) and the lateral plate mesoderm (Little and McMahon, 2012).
During embryonic kidney development, two different progenitor populations are derived from the IM: the ureteric bud (UB) and the metanephric mesenchyme (MM) (Little et al., 2007). In mice, the IM patterns into anterior-posterior axis from embryonic day (E) 8.5 to E9.5. At this stage, the cells from the UB lineage are derived from the anterior part of the IM (at 8.5E), whereas the MM develops from the posterior IM (at E9.5). Later on, a subset of the MM condenses around each UB tip to form the cap mesenchyme (CM), which refers to the multipotent population of progenitor cells that will form the nephrons (the filtering units of the mature kidney). From the UB the collecting duct system, which includes the collecting duct, the renal pelvis, ureter, and bladder trigone, will form. Interestingly, the pioneering work from Grobstein showed that the formation of nephrons requires a primary induction event from the UB to the CM (Grobstein, 1953; Auerbach and Grobstein, 1958). This inductive signal triggers mesenchymal to epithelial transition (MET) within the CM generating the renal vesicles (RV). The proximal portion of the RV elongates forming a comma-shaped body while distal region fuses with the adjacent ureteric tip generating a renal connecting tubule. The comma-shaped body undergoes further morphological changes to acquire an S shape. The S-shaped (SS) nephron is a transitional nephron stage with additional level of patterning which includes proximal, medial, and distal segments. Both, the distal and medial segments of the S-shaped body give rise to the epithelial tubule which is finally compartmentalized into the proximal tubule, the Loop of Henle, and the distal tubule. The proximal segment of the S-shaped body give rise to both parietal (Bowman’s capsule) and visceral (podocyte) epithelial layers that, upon invasion of endothelial and mesangial cells, generates the mature glomerulus. This cell population expresses a subset of transcription factors, such as SIX2, PAX2, and Sall1 that are essential for both, maintenance of their multipotent progenitor fate and organization of their later differentiation (Torres et al., 1995; Nishinakamura et al., 2001; Osafune et al., 2006; Kobayashi et al., 2008).
Another type of precursor cell present in the MM are the stromal progenitor cells. These arise surrounding the UB tips and developing nephrons. This cell population is critical for the regulation of the NPCs and UB development. Together with NPCs, stromal cells secrete glial-cell-derived neurotrophic factor (GDNF) in the MM to promote UB branching (Magella et al., 2018). Additionally, stromal cells produce retinoic acid which in turn upregulates expression of RET in the UB and therefore contribute reciprocally to UB branching (Rosselot et al., 2010). Another function of stromal cells during kidney embryonic development is to control NPCs expansion via FAT4 protocadherin expression which binds to DCHS1/2 cadherin-related protein in MM to restrict progenitor self-renewal (Bagherie-Lachidan et al., 2015; Mao et al., 2015). Finally, stromal progenitor cells give rise to all the cell types that comprise the mural cell layer of renal blood and lymphoid vessels along with other relevant cell types such as glomerular mesangial cells or pericytes. The renal interstitium consists of all these cells. It`s function, apart from providing structural support to kidney through extracellular matrix (ECM) production, is to fulfill important endocrine functions with the identification of interstitial renin- and erythropoietin-producing cells (Zeisberg and Kalluri, 2015). All these findings identify NPCs, stromal progenitor cells and UB cells, as the essential precursors that develop into functional components of the mammalian kidney. Physical and chemical crosstalk between these cell lineages is critical for appropriate kidney development and homeostasis. Thus, isolation and expansion of these precursor cells and their derived mature cells is crucial to stablish in vitro models of kidney development, physiology, and disease.
Findings on the genetic mechanisms and cell processes that shape kidney development in animal models such as chick and mouse have been crucial when providing a correct understanding of early kidney embryogenesis in humans.
Recently, the field has been able to investigate human embryonic kidney samples at different stages of development. These pioneering works shed light on differences between human and mouse kidneys (Lindström et al., 2018b). With the advance of powerful tools such as single-cell RNA-sequencing it is now possible to perform a thorough characterization of the identity of the multiple cell types encountered in the human kidney as well as the proportion of a specific cell population with respect to the others. When generating this data sets from mouse and kidney organs at different stages during embryonic development the resolution of differentiation state and spatial distribution at single cell level may help to improve our understanding on kidney development. To date different reports have defined culture conditions for the isolation and expansion of embryonic kidney cells (reviewed in (Shankland et al., 2007; Romagnani and Anders, 2013; Romagnani et al., 2013).
An alternative strategy for the generation of unlimited quantities of kidney-related cell types is the differentiation of hPSCs. In the last few years independent research groups have described the possibility of generating different kidney populations from hPSCs. Several procedures have been published using different sources of techniques of differentiation of hPSCs to renal cell types. They all have in common the idea to break down organogenesis to different decision points which pattern the kidney within an embryo. Insights in kidney development provide the ground to reach a desired renal cell by forcing the phenotype-specific gene expression profiles chronologically on it. Nephrogenesis, when translated to in vitro studies to differentiate hPSCs towards the renal lineage, has been to date divided in three consecutive steps: the induction of IM-like cells, the specification of a transitory MM giving rise to the formation of RV structures, and finally nephron formation in the absence of instructive morphogens/cytokines proving the autonomous responses of these culture model systems (Figure 1).
FIGURE 1. Schematic stepwise description of the differentiation of kidney organoids from hPSCs. After treatment of hPSCs with factors and small molecules according to chosen protocol posterior primitive streak is induced. Further treatment with renal inductive signals leads to intermediate mesoderm (IM) commitment. Specifically, in our protocol, this step is characterized by the formation of a tight monolayer of IM committed cells on Day 4 of differentiation. IM-comitted cells are treated with a short pulse of CHIR, then aggregated to spheroids and further stimulated with FGF9 signalling until Day 11 to induce the formation of renal vesicels (RVs), which are visible at the borders of the organoid (yellow arrows). Upon removal of factors, RVs develop into tubular and glomerular structures (red arrow heads) to generate kidney organoids by Day 20 of differentiation. Scale bars, 100 µm.
The aim of initial studies was to differentiate towards mature renal cell types because mature cells of the adult kidney are well characterized and the primary target of many kidney diseases. In this regard, Song and colleagues were the first ones to describe the derivation of podocyte progenitor cells from hiPSCs. In their work the authors showed that hiPSCS-derived podocytes were efficiently integrated into mouse metanephric tissues (Song et al., 2012). Other protocols on the development of renal cell types were also described by Narayanan and colleagues who described the generation of renal epithelial cells from hESCs. The differentiated stem cells exhibited markers characteristic of renal proximal tubular cells and their precursors generating tubular structures in vitro and in vivo (Narayanan et al., 2013). However, these studies didn’t yield efficiently cells of the renal lineage that could be used for further applications. Still, these studies were among the first ones to apply and establish a validation scheme of kidney development markers which proved that recapitulation of in vivo nephrogenesis in vitro might be possible by treatment of hSPCs with growth factors.
Soon after those first two studies the field started to explore the possibility to derive renal progenitor cells from hPSCs as a new approach to understand common and divergent developmental processes guiding kidney development between mice and human species. Furthermore, they aimed to generate cell sources with the potential to model these processes in the human background. In an early study Mae and colleagues tried for the first time to differentiate monolayers of hESCs towards IM using a reporter hiPSC line in which green fluorescent protein (GFP) was targeted into Odd-skipped related 1 (OSR-1, a gene transiently expressed in the IM during mouse embryogenesis). With this approach the authors showed that using both EB and monolayer culture methods, a sequence of growth factor combinations as GSK-3β inhibitor CHIR99021 (CHIR), Activin A, and BMP7 in the presence of fetal bovine serum led to PS formation and the generation of an OSR1+ IM population characterized for expression of kidney developmental markers, including PAX2, WT1, OSR1, EYA1, LHX1, and CD24 over 8 days of differentiation (Mae et al., 2013).
Later studies by Bonventre and Little laboratories generated PS and IM relying on Wnt signalling to generate PS and highlighting the role of fibroblast growth factor (FGF) signalling to induce the formation of IM and MMcell populations from hPSCs, respectively (Lam et al., 2014; Takasato et al., 2014).
By the same time, the Izpisua Belmonte laboratory demonstrated the possibility to generate, for the first time, UB progenitors from both hESCs and from hiPSCs derived from patients affected by polycystic kidney disease (PKD). Making use of a two-step protocol the authors first induced mesodermal specification by bone morphogenetic protein 4 (BMP4) and FGF2. Then IM anteriorization was induced exposing the cells to retinoic acid, activin A and BMP2. After only 4 days in culture UB progenitor cells expressed HOXB7, RET and GFRA1. Moreover, when UB-like-hiPSC-derived cells were co-cultured with dissociated E11.5 mouse metanephric cells, UB-like-hiPSC-derived cells only integrated into cytokeratin 8 positive (+) UB-like structures, suggesting, for the first time, the induction of UB lineage-committed IM cells ex vivo (Xia et al., 2013). In the same manner, Taguchi and colleagues reported on the derivation of both mouse ESCs- and hiPSCs-which showed the ability to reconstitute 3D nephron-like structures (including both glomerulus- and renal tubule-like structures in vitro). These co-cultures made use of embryonic spinal cords as an external inducing source for kidney differentiation ex vivo (Taguchi et al., 2014). In regard to functional usage Imberti and colleagues demonstrated for the first time that hiPSC-derived renal progenitors robustly engrafted into damaged tubuli restoring renal function (Imberti et al., 2015). In their work, the authors generated NPCs using retinoic acid, RhoA and PI3K inhibitors and activin A to induce IM generation. IM-committed hiPSCs were treated with FGF2, BMP7 and GDNF for 13 additional days to generate MM-derived hiPSCs. Along this same line, another work from the same laboratory, showed that kidney stem cells derived from hPSC can be induced to form spheroids mirroring tissue-specific epithelial physiology. However, although these studies yielded SIX2+ NPCs cells with a higher efficiency than the work from Mae and colleagues, the necessity for agents as spinal cord precluded the generation of hPSCs-derived NPCs on a larger scale.
Other limitations at that point were highlighted by Morizane and collaborators who argued that the still not satisfying feasibility and efficiency to generate hPSCs-derived renal cells was caused by the fact that most of the initial procedures did not properly distinguish anterior from posterior IM at early steps of directed differentiation (Morizane et al., 2015). These findings and observations altogether laid the groundwork for the generation of the so-called kidney organoids. To date, various well characterized protocols to generate kidney organoids are available. The common theme used in these approaches is the activation of canonical Wnt signalling via CHIR in undifferentiated hPSCs to promote the formation of PS and IM derivatives from which the kidney derives (Takasato et al., 2014; Morizane et al., 2015; Garreta et al., 2019).
Morizane and colleagues reconsidered the application of BMP4, which was used in previous renal differentiation protocols to induce the anterior-posterior patterning of the PS. In this study, the authors hypothesized that posterior IM cells arise from the late-stage PS rather than from posterior PS, and highlighted the importance of recapitulating the timing of migration of mesodermal precursors out of the PS, a process that defines mesodermal anterior-posterior patterning during development. Following this rationale, they tested several doses and duration of CHIR treatments in order to effectively differentiate hPSC into late-PS cells to further increase the efficiency of IM formation (Morizane et al., 2015). This led to the now general notion that for optimal differentiation the duration of Wnt signalling determines whether anterior IM-derived UB or posterior IM-derived MM becomes the most dominant population as anteriorization of PS favors UB formation (Taguchi et al., 2014). This hypothesis was confirmed by Takasato and colleagues. In their study a shorter CHIR treatment generated predominantly anterior IM whereas longer exposure induced posterior IM (Takasato et al., 2015). Takasto and colleagues generated kidney organoids containing individual nephrons (∼100 nephrons/organoid) that further segmented into distal and proximal tubules, early loops of Henle and glomeruli containing podocytes elaborating foot processes. In this work the authors performed bulk RNA sequencing (RNA seq) for transcriptomically profile the extent of kidney organoid differentiation also comparing the matureness of the generated organoids with human embryonic kidneys and demonstrated that upon 25 days of differentiation kidney organoids transcriptomically resembled the first trimester gestational kidney (Takasato et al., 2015).
Morizane and colleagues reported that 3D culture conditions proved to be advantageous in order to generate tubular structures at a higher efficiency (Morizane et al., 2015). Furthermore organoid podocytes are being shown to express a range of proteins required for glomerular function (e.g., NEPHRIN, PODOCIN, PODOCALYXIN, SYNAPTOPODIN) which are nearly absent in conventional 2D podocyte cell lines (Yousef Yengej et al., 2020). In our own study we also observed that forcing cell-to-cell and cell-to-extracellular matrix interactions through the aggregation of NPC-derived hPSCs early during the time course of organoid formation results in the development of kidney organoids which upon 16 days in culture transcriptomically matched the second trimester human gestational kidney (Garreta et al., 2019) (Figure 2). Characterization of organoids via single cell RNA-sequencing (scRNAseq) confirmed the presence of developing podocytes, parietal epithelial cells, proximal tubules, loops of Henle, distal tubules, collecting ducts, and interstitial and endothelial cells. Kidney organoids reveal a great deal (20%) of off-target cells, mainly neural. Mostly, the common renal differentiation protocols generate kidneys matching second or trimester fetal kidneys (Combes et al., 2019; Subramanian et al., 2019).
FIGURE 2. hPSC-derived kidney organoids display renal features that partially resemble those in human fetal kidneys. Histological analysis of kidney organoid (A) and 22 weeks of gestation human fetal kidney (B). Higher magnifications of the boxed areas show details of glomeruli (G) and tubule (T) structures. Scale bars, 200 and 50 µm (higher magnifications). Immunohistochemistry of kidney organoid (C) and 22 weeks of gestation human fetal kidney (D) for the detection of the basement membrane protein Laminin and the podocyte marker Nephrin. Cell nuclei are counterstained with DAPI. Higher magnifications of the boxed areas show details of glomerulus found in kidney organoid compared to human fetal kidney. Interestingly, nephrin+ podocyte-like cells in kidney organoids are aligned on the basement membrane (laminin+) following a similar distribution as in the human fetal kidney. Scale bars, 100 and 25 µm (higher magnifications).
Stromal populations have been identified in kidney organoids by immunofluorescence as well. The expression of FOXD1 and MEIS1 indicated the presence of interstitial cells in kidney organoids (Takasato et al., 2014). Although not much attention is paid to stromal cells in the MM-derived organoids, Taguchi and colleagues identified them to be crucial for enhancing branching morphogenesis of the ureteric tree when incorporating mouse embryonic interstitium together with induced UBs and progenitors (Taguchi et al., 2014). Again, scRNAseq analysis highlighted underrepresented or missing cell types as mesangial cells, immune cells, glomerular endothelium, principal and intercalated cells (Combes et al., 2019; Yousef Yengej et al., 2020).
Besides all the advances mentioned above, the kidney organoid field is already facing important drawbacks as organoids lack a nervous or immune system, a progenitor niche, and proper vascularization. Also, the assembly of UB and epithelial progenitors to generate a more developed model still needs to be achieved. As described earlier reciprocal interactions between the UB and the surrounding SIX2+ CM, however are essential to nephrogenesis. In this regard, the majority of procedures to derive kidney organoids from hPSCs first differentiate to kidney progenitors through the formation of NPCs that are then induced to epithelialize using a pulse of GSK3 inhibitor CHIR. Importantly, this single epithelialization induction differs from the process of differentiation in the native developing kidney, where cells at numerous stages of differentiation co-exist within the organ as recently described by the MacMahon laboratory (Lindström et al., 2018a). Following this logic, the laboratory of Oxburgh has recently shown that the asynchronous mixing of hPSCs-derived NPCs with epithelializing nephrons over time results in the generation of heterochronic organoids in where the proximal and distal nephron components preferentially derive from different cell populations. Furthermore, the resulting organoids were well vascularized when engrafted under the kidney capsule (Kumar Gupta et al., 2020).
Addressing the lack of vascularization is essential for several reasons. One is that renal diseases in the adult are mainly caused or aggravated by the immune system and widely based on damages of the vascular system (Tecklenborg et al., 2018). Kidney organoids lack an immune system and only contain an endothelial cell population instead of a fully developed vascular system, which might also be the reason why maturation cannot be pushed further and only leads to fibrosis. The improvement of vascularization of kidney organoids is studied more than the immune system, probably due to the notion that a vascular system is the key to more mature organoids by triggering cell communication. It is hypothesized that the lack of oxygen in the inner parts of the organoid are the main reason to why maturation stops at some point (Wörsdörfer and Ergün, 2021). We addressed the vascularization problem in our study by implanting organoids into chick immune-deficient chorio-allantoic membrane (CAM), a highly vascularized extraembryonic tissue previously used to grow tumoral cells or engrafting biomaterials. In our study, we took advantage of this system to enhance the proper organization of endogenous kidney organoid endothelial cells also exploiting the CAM assay as in ovo bioreactor to grow kidney organoids on demand. Our approach after 3 days of organoid implantation resulted in the invasion of multiple blood vessels from the CAM throughout kidney organoids. The circulation of chick blood within kidney organoids was clearly observed after 5 days of implantation, Overall, compared with in vitro counterparts, CAM-implanted kidney organoids exhibited glomeruli with an enlarged Bowman’s space and tubule-like structures with enlarged lumens (Garreta et al., 2019).
An alternative approach to provide a vascular component to hPSCs-derived organoids is to transplant them under the kidney capsule of immunodeficient mice profiting from the high vascularization capacity of this tissue. Two studies showed a maturation in transplanted podocytes and vascularization of glomeruli by the host respectively (Sharmin et al., 2016; Van Den Berg et al., 2018). Also it seems that fluid flow is contributing to an improvement of vascularization as it stimulates endothelial cells to invade podocyte populations (Musah et al., 2017). A recent study has explored this approach through the design of a 3D printed chamber at the millimeter scale to culture hPSC-derived kidney organoids under constant fluid flow that sufficed for the expansion of endothelial progenitors within the organoids and a higher degree of vascularization compared to the static culture conditions (Homan et al., 2019). This opens future perspectives in the bioengineering field to use chips, scaffold and other devices to culture organoids on in order to mimic filtration and tissue interface and recapitulate renal cell injury and proteinuria (Begum, 2019).
Recent studies also focused on the generation of kidney organoids with enhanced endothelial cell compartment such as reported from the Xia laboratory. They generated vascularized organoids by modulating Wnt signalling allowing to further control the relative proportion of proximal versus distal nephron segments for the production of vascular endothelial growth factor A (VEGFA), the major factor responsible for the maintenance of the glomerular vasculature (Eremina et al., 2003; Sison et al., 2010). More importantly, in this work the authors made use of single-cell RNA sequencing (scRNAseq) to further identify a subset of NPCs as a potential source of renal vasculature which was further supported by the revelation of Six1+CD31+ (SALL1+CD31+) cells (Low et al., 2019). In this regard, recent work from MacMahon laboratory has shown on the utility of single-nucleus droplet-based sequencing of the human fetal kidney for the identification of nephron, interstitial, and vascular cell types that together generate the renal corpuscles identifying factors predicting precursors or mature podocytes which express FBLN2, BMP4, or NTN4, in conjunction with recruitment, differentiation, and modelling of vascular and mesangial cell types into a functional filter (Kim et al., 2019). In vitro studies using primary cells from fetal kidneys proved that these factors exhibit angiogenic or mesangial recruiting potential also exerting inductive properties consistent with a key organizing role for podocyte precursors in kidney development. It will be interesting to challenge developing hPSCs kidney organoid to boost these processes.
Another technical challenge, regardless of the approach used to induce vascularization, is to recapitulate embryonic branching morphogenesis in kidney organoids. As predicted from mice (Costantini and Kopan, 2010), recent analysis in the human fetal kidney would suggest that the collecting duct tips should be marked by RET expression (Lindström et al., 2018b; Menon et al., 2018; Hochane et al., 2019). Nonetheless, there is no study showing WNT9B expression in kidney organoids nor any evidence for the existence of ureteric epithelium. In this regard, the Melissa Little group referred to the GATA3+CDH1+ structures encountered in kidney organoids as collecting duct (Takasato et al., 2015), however, in that same work RNA seq analysis did not reveal WNT9B or RET expression. Recently, the same group has referred to those structures as connecting segment (Little and Combes, 2019), which refers to the region of the nephron which bridges to the collecting duct epithelium (Little et al., 2007). Nonetheless, the same group did not find that this presumptive connective segment derives from SIX2+ cells as happens in mouse (Georgas et al., 2011) suggesting that this epithelium may arise from an ureteric epithelium during the time course of organoid formation. Therefore, the work from the Nishinakamura laboratory has been the only one providing a comprehensive overview on the distinct origins and developmental processes of the ureteric bud (Taguchi and Nishinakamura, 2017) taking advantage of the Hoxb7-GFP transgenic mouse line to establish a kidney reconstruction assay by modifying previously reported methods (Grobstein, 1953; Auerbach and Grobstein, 1958; Ganeva et al., 2011). Through the reaggregation of E11.5 MMs, including NPs and SPs with a wolffian duct (WD) or UB from E9.5, E10.5, and E11.5 the authors were able to show that UB or WD from the E11.5 embryo would robustly branch in front of WD from E10.5 and E9.5. Importantly, the authors employed the best culture condition supporting WD acquired branching capacity to define the biochemical cocktail inducing the maturation of WD progenitors into UB-like cells. This information allowed for the derivation of novel culture procedures for the differentiation of mouse ESCs into induced UB, which effectively reconstructed the higher-order structure of the embryonic kidney by their assembly with NPCs and isolated renal embryonic stromal cells. Then the authors translated these findings to applying the different procedures using hiPSCs. All in all, the work of Nishinakamura showed that reassembled organoids developed the inherent architectures of the embryonic kidney, including the peripheral progenitor niche and internally differentiated nephrons that were interconnected by a ramified ureteric epithelium. Thus, exploiting the selective induction on hPSCs together with the reassembly of the different cell types will be a powerful approach to recapitulate organotypic architecture in hPSC-derived organoids and to further assess the role of human UB branching epithelium as an organizer of tissue geometry and cell viability during human embryonic kidney development. A summary of recent published reports on the generation of hPSC-derived kidney progenitors and kidney organoids is compiled in Table 1.
TABLE 1. Major approaches and protocols for directed differentiation of hPSCs into renal progenitor cells and kidney organoids.
Genetic lineage tracing using transgenic mice with fluorescent reporter genes have significantly contributed to our knowledge of mammalian kidney development and physiology (Humphreys and DiRocco, 2014). In those studies, fluorescence labelling allowed for the identification and real time monitoring of specific renal cell types during development or disease progression in vivo (Boyle et al., 2008; Zhang et al., 2020). Although this approach is not affordable in the human context it is possible to generate kidney organoids from hPSCs reporter lines to address similar questions so far addressed in the mice system. To date, accumulative findings on the generation of kidney organoids highlight the utility of this cell cultures as in vitro models to study mammalian kidney development, multicellular organization and physiological function. In this context, the possibility to perform functional genetic studies help to elucidate molecular mechanisms underlying organ development and disease. Nowadays, the use of targeted nucleases for genome editing facilitates our capacity to manipulate the genome of hPSCs and exploit these cellular platforms to virtually any desired cell type, including organoids. Among current genome editing technologies, this review focus on the utility of the CRISPR/Cas9 system in kidney organoids as an amenable system on which kidney differentiation and maturation can be monitored in living cells using fluorescently tagged kidney lineage markers. Indeed, in the near future our reliance on these cellular models may not only be restricted in their utility in differentiation studies but also help us to study the impact of renal cell de-differentiation under disease contexts (i.e., podocyte de-differentiation is a hallmark of CKD, as well as the de-differentiation of tubular epithelial cells upon acute injury, among others).
In the recent years the work of Boreström and colleagues exploited CRISPR/Cas9 to generate three kidney-specific reporter cell lines (Boreström et al., 2018). These include the derivation of two single reporters (SIX2- GFP and NPHS1-GFP), and one dual reporter cell line (SIX2-GFP/NPHS1-mKate). Making use of SIX2-GFP and NPHS1-GFP reporter cell lines the authors were able to monitor the emergence and maturation of kidney progenitor cells and podocytes, respectively. The percentage of fluorescent positive cells was used as a quantitative readout to redefine existing procedures for kidney organoid derivation. Furthermore, the authors made use of the NPHS1-GFP reporter cell line as a surrogate of podocyte health by assessing the impact of several insults on podocyte de-differentiation (measuring GFP loss). On balance, this panel of hPSCs reporter lines allowed the authors to establish an approach to monitor kidney differentiation, glomerular maturation, and podocyte performance in living cells.
In a more extensive approach Howden and colleagues targeted the SIX2 locus of hiPSCs to generate both reporter and lineage tracer cell lines (Howden et al., 2019). In line with similar lineage tracing studies in mice, the panel of CRISPR engineered hiPSCs was used to perform fate-mapping studies during kidney organoid differentiation. Importantly, the authors took advantage of these cell lines to underscore if the SIX2 progeny contributes to nephrogenesis in developing hPSCs-kidney organoids. To achieve this CRISPR/Cas9 was used to generate reporter lines by inserting an EGFP expression cassette at the SIX2 locus of hiPSCs which upon characterization were differentiated into kidney organoids. By monitoring EGFP fluorescence expression during differentiation the authors were able to dissect the emergence of the EGFP+/SIX2+ cell population soon after kidney organoid formation and to demonstrate the presence of SIX2+ cells until later stages of differentiation. Then, CRISPR/Cas9 was used to generate a lineage tracer line by inserting both a Cre recombinase gene and a dual-fluorescence expression cassette that included a loxP-flanked EGFP gene adjacent to a mCherry gene. These constructs were inserted into the SIX2 and GAPDH loci of hiPSCs, respectively. Monitoring mCherry and EGFP fluorescence during kidney organoid differentiation allowed to determine how the mCherry+/SIX2+ cell population gives rise to nephron epithelial cell types but not to presumptive ureteric epithelium (EGFP+) as previously reported in mice (Georgas et al., 2011). Interestingly, this study showed that some mCherry+/SIX2+ cells can give rise to a subset of the renal stroma, which points out a possible species divergence compared to mice. Finally, the authors made use of a CRISPR/Cas9 knock-in approach to generate an inducible lineage tracer line by inserting a tamoxifen-inducible version of the Cre recombinase (CreERT2). By controlling the labelling of SIX2+ cells at different time points during organoid differentiation the authors ascertain whether the SIX2 lineage contributes to nephrogenesis. This approach allowed the authors to induce SIX2 expression at later time-points during differentiation, further showing the absence of mCherry + cells into nephron developed structures but their detection in the surrounding interstitium. Then the authors made use of CHIR at later stages of kidney organoid differentiation and treated cells with tamoxifen further showing the presence of mCherry + cells into nephron structures. Based on these results the authors concluded, that in contrast to kidney development in vivo, kidney organoids lack a self-renewal progenitor niche with active nephron-forming capacity.
Later on, the same group reported the application of CRISPR/Cas9 to develop a wide panel of reporter lines in a more throughput manner (Vanslambrouck et al., 2019). Towards this aim the authors used a single step protocol of simultaneous somatic reprogramming using episomal vectors together with CRISPR/Cas9 mediated genome editing for the generation of gene edited hiPSCs (Howden et al., 2018). Interestingly, the authors utilized a variant of Cas9 in order to minimize unwanted mutations derived from NHEJ DNA repair. This variant consisted of a Cas9 fused to a peptide derived from the human Geminin protein which mediates degradation of the chimeric protein (Cas9-Gem) during the G1 phase of the cell cycle at which cell stage NHEJ predominates over DSB. Thus, the absence of Cas9-Gem prevents from undesired mutations. Using this elegant approach, the authors expanded their previous reported panel of reporter cell lines up to ten different hiPSCs reporter lines that were further differentiated into kidney organoids. In this same study the authors further explored SIX2 positive presumptive NPCs to ascertain on the role of this transient cell population in kidney organoids. In order to further characterize, visualize, and isolate this SIX2+ cells, additional hiPSC reporter lines were generated by targeting the nephron progenitor marker CITED1. This way the authors generated new single CITED1mCherry and double SIX2EGFP:CITED1mCherry hiPSC reporter lines. Remarkably, CITED1+/mCherry + cells were detected in higher amounts upon posterior primitive streak induction in agreement with the observed CITED1 expression pattern in developing mice (Boyle et al., 2007). In the same work the authors also developed single reporter hiPSCs to further explore on the contribution of differentiated organoid cell types in vivo, including proximal tubular cells and podocytes, further showing correct engraftment of reporter cells into corresponding nephron compartments upon kidney organoid transplantation.
Another challenge in kidney organoid differentiation is the maintenance of a ureteric tip environment for NPCs survival and self-renewal. In vivo UB branches into a contiguous ureteric epithelium to which distal nephrons are connected. To allow further characterization of this ureteric and late distal epithelium within kidney organoids, in this same work the authors generated both a single GATA3mCherry hiPSC reporter line and double GATA3mCherry/MAFBmTagBFP2 reporter lines. Consistent with previous data, during differentiation GATA3+mCherry + cells were found into interstitial and nonepithelial organoids compartments that were frequently associated with NPHS1+ podocytes of the glomeruli. Furthermore, live imaging during kidney differentiation of the double reporter line evidenced coincidence of both GATA3+Cherry+ and MAFB + BFP2+ populations within a given organoid and dichotomy in onset of its labeled gene expression. These data provide novel insights into the debate around whether both human iPSC-derived NPCs and UB progenitors can be simultaneously generated in a single differentiation despite their divergent lineage origin.
On balance, the use of reporter hPSCs for the generation of kidney organoids allows to dissect and monitor human kidney morphogenesis in real time. The convergence of hPSCs reporter cell lines together with kidney organoid technology is expected to improve existing differentiation protocols with respect to cell type maturation and minimization of off-target populations. In this regard recent findings from the Greka laboratory (Subramanian et al., 2019) recently show on the impact of kidney organoid transplantation as a new approach to diminish the presence of off-target cells using scRNAseq. It will be interesting to further exploit these same approaches making use of hPSCs reporter cell lines and using strategies for further hPSCs lineage tracing to further ascertain the transcriptomic profile of the derived cells. The convergence of these methods may help to categorize organoids protocols with regards to the production of specific cell types and to predict fidelity and reproducibility.
Another application of CRISPR gene editing in kidney organoids is to interrogate and dissect human lineage relationships in vitro during kidney disease. This approach allows to perform functional experiments to either validate already known kidney disease related genes but also to identify new genes and cellular pathways malfunctioning during kidney disease. Specifically, by using CRISPR one can introduce mutations at candidate genes and, consequently, generate mutated hPSCs to be compared versus untargeted hPSCs controls that will share the same genetic backgrounds. In this manner, by differentiating mutant and wild type counterparts towards kidney organoids is not possible to study phenotypic and molecular differences arising from the genetic disorder.
Following this rationale Freedman and colleagues pioneered the application of CRISPR to introduce loss-of-function mutations into polycystin 1 (PKD1), polycystin 2 (PKD2) and podocalyxin (PODXL) genes. PKD1 and PKD2 genes were targeted to model polycystic kidney disease (PKD), which cause autosomal polycystic kidney disease, a condition which leads to end-stage renal failure due to expansion of fluid-filled cysts in the kidney. The same group targeted also the PODXL gene to investigate its possible implication into diseases which are based on a massive loss of protein due to defects in the kidney basal membrane as focal segmental glomerulosclerosis or congenital nephrotic syndrome (Freedman et al., 2015). In this initial study, although only a low percentage of PKD mutant organoids formed cysts and the disease mechanism still needs to be clarified, they proved that a functional model can be achieved this way. The authors furthermore generated a CRISPR/Cas9 engineered iPS PODXL knock-out line, which revealed that PODXL defective organoids exhibited an impairment of junction organization between podocytes-like cells.
Interestingly, a follow up study by the same group made use of kidney organoids derived from PKD1 and PKD2 mutant hPSCs to identify modulators of early PKD cystogenesis (Cruz et al., 2017). To achieve this, the authors first performed time lapse imaging and showed how cysts emerge from tubular segments and rapidly expand by partial detachment from the mutant organoids. To further prove that the loss of adherent forces was related to cyst outgrowth, organoids were cultured in suspension. The removal of adherent cues dramatically increased cyst formation rates up to 10-fold compared to standard used adherent culture systems. Importantly, cyst formation rate was assessed upon several months in culture demonstrating that CRISPR-mutant organoids showed cysts which expanded to 1-centimetre diameter compared to wild type isogenic controls. These observations recapitulated phenotypic features of early stage PKD-cysts which showed a lining epithelium with overlapped expression of proximal and distal tubular markers except for some patches with stromal cells. Furthermore, PKD-cyst showed absence of podocytes and marginal collagen deposition in line with observations in prenatal PKD-cysts. Further gene expression profiling of cyst lining cells showed overexpression of gene sets related with cell cycle progression. The authors concluded that organoid cysts result from hyperproliferative kidney tubular epithelial cells (KTECs) as previously shown in mouse and human PKD-cyst samples. Importantly, KTECs were able to proliferate and migrate as monolayer explants in the absence of stroma when kidney organoids were cultured over an ECM. Further protein expression analysis in KTECs outgrowths and undifferentiated hPSCs revealed a strong down regulation of PC1 protein expression in PC2 defective cells. Conversely, PC2 expression levels were unchanged in PC1 defective cells. Decrease in PC1 protein expression was reproduced by knocking down PC2 protein in control hPSCs. These results indicated that PC2 was required for human PC1 expression in contrast to previous studies in mice. To prove the implication of PC1 in adhesion and ECM remodelling, kidney organoids derived from PKD1 mutants and wildtype isogenic lines were embedded into collagen droplets and cultured in suspension. Only wildtype organoids were able to noticeably compress droplets in contrast to PKD1 mutants. This approach demonstrated that kidney organoid epithelia can remodel ECM microenvironment with apparent dependence on PC1. Biochemical stimuli which is characteristic of PKD were also challenged in this model system. Specifically, treatment with cAMP signalling agonists induced cyst formation in both PKD mutants and wildtype derived organoids. Later, the same group proved the possibility to scale up the production of CRISPR engineered organoids in a high throughput format (HTS) (Czerniecki et al., 2018). Organoids also reproduced cystic swelling response to cAMP signalling agonist treatment, demonstrating the feasibility of this format for screening applications. As a proof of concept, the authors performed a small-scale screen with eight candidate factors and an inhibitor of non-muscle myosin II (NMII as a potent inducer of cystogenesis). These results suggest on the role of polycystins maintaining cytoskeletal stability in tubules through actomyosin activation.
One major limitation of the discussed studies is the usage of protocols that differentiate towards the MM and consecutive nephron organoids. However, in patients with ADPKD, cysts are known to originate primarily from collecting duct cells which are not present in kidney organoids with mostly nephron-like structures (Devuyst et al., 1996). In this context, the Nishinkamura laboratory recently reported the use of PKD1 mutant hiPSCs to generate UB organoids with cyst forming capacity providing a robust in vitro ADPKD model (Kuraoka et al., 2020). They electrocorporated a designed sgRNA vector targeting the exon 15 of PKD, Cas9 expression vector and targeting vector into a human iPSCS line and conducted a puromycin selection for PKD−/− clones. After differentiation of the mutant cell line and wildtype control towards nephron organoids they showed that, upon stimulation of cAMP signalling with forskolin, the PKD−/− -derived kidney organoids developed more severe cysts than the wildtype control. Interestingly, the cysts appeared mainly in LTL positive (proximal tubule) cells and WT1+ glomerular parietal epithelial cells independently of the genotype, consistent with data in mice ADPKD model (Ahrabi et al., 2010). Next the mutant PKD−/− hiPSC cell line and the wildtype control line were induced to form UB organoids. Upon treatment with forskolin, PKD mutant UB organoids developed pronounced cysts. Importantly, cysts did not develop in wildtype control UB organoids in contrast to wildtype nephron organoids that showed moderate cyst formation. In addition, they found that arginine vasopressin receptor 1A gene (AVPR1A) was functionally expressed in UB organoids. Treatment of UB organoids with the natural cAMP ligand vasopressin resulted in efficient cyst formation in PKD−/− but neither in PKD1+/− nor in wild-type UB or in nephron organoids. Moreover, they validated these findings using iPSCs derived from a patient with a heterozygous mutation in the PKD1 gene, showing that UB organoids derived from the patient iPSCs evidenced robust cyst formation upon forskolin stimulation.
To overcome issues related to kidney organoid inter and intra batch variability Low and colleagues reported an approach in where the CRISPR/Cas9 system was used not only to model a disease but also to correct a patient-specific PKD1 mutation in patient derived hiPSCs (Low et al., 2019). In line with previous studies, subsequent differentiation of corrected and non-corrected PKD1 hiPSCs lines towards kidney organoids showed a low incidence of spontaneous cyst formation in patient derived kidney organoids. As in previous studies, intracellular levels of cAMP were stimulated to boost cyst incidence. After treatment, kidney organoids derived from PKD1 hiPSCs lines showed a severe increase in cystogenesis, while CRISPR-corrected isogenic controls showed marginal cyst formation. Remarkably, kidney organoids derived from PKD1 hiPSCs lines exhibited phenotypic features observed in PKD patients, such as proximal to distal enlargement of the tubule lumen, reduction in the expression of segment-specific markers, distorted glomeruli squeezed between cysts, and unfunctional proximal tubular cells at the cyst lining. Furthermore, the authors assessed the effect of two chemical compounds as blockers of cystogenesis in kidney organoids derived from PKD1 hiPSCs lines. Altogether, these results set the basis for the utilization of kidney organoids as a preclinical model for drug screening applications. In contrast with previous studies, this approach allowed for personalized studies because both mutant hiPSCs and CRISPR corrected isogenic controls comprise patient specific genetic backgrounds. In the same manner, the Freedman group also studied on the impact of the knock-out of PODXL in podocyte differentiation and function through the generation of kidney organoids from CRISPR gene-edited hPSCs lacking PODXL (PODXL−/−) (Kim et al., 2017). Using Transmission Electron Microscopy (TEM) the authors showed that podocytes derived from PODXL−/−organoids exhibited a drastic reduction in microvilli compared to wildtype controls. Consequently, PODXL defective podocytes reduced their lateral interspaces by increasing the formation of lateral cell-cell junctions. In contrast, confocal imaging of control podocytes showed extended lateral interspaces with apical microvilli expressing PODXL. Defects of human mutant podocytes in vitro were phenocopied in PODXL deficient prenatal mice. Furthermore, Podxl−/− mice die of anuria shortly after birth. Altogether these findings demonstrated the central role of PODXL in the formation of microvilli in podocytes. hPSCs allowed the characterization of PODXL mediated cell interactions at the biophysical and transcriptomic level. On one hand, when dissociated with optical tweezers, control hPSCs exhibited an anti-adhesive effect increasing cell to cell separation while PODXL−/−cells remained in closer distance. This effect was explained by the presence of electrostatic repulsion forces between negative PODOCALYXIN charges presented in adjacent cell membranes of PODXL expressing cells. On the other hand, comparative transcriptomic analysis by RNA-Seq identified that PODXL−/− hPSCs upregulated the expression of genes related with cell adhesion concomitantly with a downregulation in the expression of genes involved in microvillus formation. Altogether, these results proposed a model in which PODOCALYXIN induces apical-to-basal junctional migration by its progressive localization at apical and lateral cell membranes of podocytes in where PODOCALYXIN induces microvilli formation. Electrostatic repulsion between apical microvilli limits cell-cell contact to the basal membrane of podocytes and generates lateral interspaces between them for proper glomerular filtration. This model predicts that loss-of-function mutations in human PODXL may cause embryonic or perinatal lethality due to kidney failure, as podocyte microvilli are critical for urine production in mammals. In line with this expectation, only few studies reported PODOCALYXIN mutations in living patients with kidney disease. To date only few heterozygous non-synonymous or nonsense mutations have been identified in a reduced number of patients with focal and segmental glomerulosclerosis. Remarkably, only one study reports a neonatal patient with biallelic nonsense mutations in PODXL. Unfortunately, the newborn early died afterbirth (∼4 months) presenting severe nephrotic syndrome and omphalocele. These features are similar to main defects described in Podxl-deficient mice. On balance, this work showed that CRISPR/Cas9 engineered organoids offer a predictive power to discover novel mechanisms explaining disease gestation and progression. A summary of recent published reports on the use of CRISPR/Cas9 technology to model renal disease is compiled in Table 2.
TABLE 2. Published work about disease modelling combining kidney organoids and CRISPR genome editing.
The Little group demonstrated what powerful tool the combination of CRISPR/Cas9 and organoids technology can be in order to reach important perspectives of regenerative medicine as a possible therapy of genetic disorders (Forbes et al., 2018). They reprogrammed cells from a patient with nephronophtisis ciliopathy with a IFT140 mutation to hPSCs and corrected that mutation in one step. Patient-derived cells were reprogrammed with seven transcription factors in a vector–free manner. Simultaneously IFT140 gene was targeted by a EGFP reporter and homologous recombination facilitated by using in vitro transcribed mRNA encoding Cas9, a plasmid encoding a gene specific short-guide RNA and a donor template encoding EGFP reporter and a puromycin resistance gene flanked by homology arms specific to sequences upstream and downstream of the IFT140 start codon. This protocol had been developed by the lab earlier and is supposedly highly efficient. Proband-derived iPCS as well as corrected iPSCs were differentiated to kidney organoids. The former differentiated to organoids with shorter, club-shaped cilia which is in line with findings in mouse models (Miller KA et al., 2013). Tubules showed a phenotype that is characteristic in nephronophthisis. This study did not only demonstrate the successful correction of a mutation associated with nephronophthisis but also unveiled pathogenic pathways not previously described in IFT140-deficient disease models. Therefore, not only differences in between species regarding disorders of the cilia was overcome which had hampered the quest to a deeper understanding. The same group went further on to target PKHD1 gene which causes autosomal-recessive polycystic kidney disease in order to demonstrate that distal nephron epithelium that is part of the nephrons of their organoids is indeed functional. A GATA3+/EPCAM+ positive population in their organoids transcriptionally matches a distal/connecting segment the most. This population was propagated by culturing in a specific ureteric endothelium (UE) favoring medium or conditions, respectively. They exploited the established protocols to generate reporter cell lines as described earlier and targeted PKHD1 in a GATA3 mCherry reporter cell line. CrRNAs were designed to bind within the PKHD1 gene in order to reach a homozygous deletion and generation of a premature stop codon within the exon 4 of the PKHD1 coding region. PKHD1null cells were differentiated, purified by FACS and propagated in UE favoring conditions. Upon transition to stalk medium containing aldosterone, vasopressin, FGF2 and retinoic acid the organoids displayed large cyst-like structures. Interestingly, these cysts could also be induced by forskolin (Howden et al., 2021). Here, disease modelling and establishment of UE culturing profit one another. Interestingly, this approach does question the paradigm that they and Morizane et al. had earlier established as in that duration of Wnt signalling will decide on lineage fate exclusively either towards MM or UB (Takasato et al., 2015 and Morizane et al., 2014).
Waehle et al. generated a (Wilms Tumor 1) WT1 knock-out (KO) cell line by inducing hPSCS harbouring a doxycycline-inducible Cas9 protein with lentiviruses driving expression of a WT1 specific guide RNA and a red-fluorescent protein. They induced gene editing at different time points (prior to differentiation, during nephrogenesis) following the Morizane protocol to differentiate hPSCS to organoids. WT1 is a tumor suppressor gene and its homozygous loss is associated with Wilms Tumor, the most common kidney cancer in children. (Hendry and Little, 2012). WT1 continues to be expressed in the NPs as well as the developing nephrons (Pelletier et al., 1991). Studying WT1 not only is promising in leading to deeper insights in Wilms Tumor, but it is also a marker of the MM during nephrogenesis and therefore helpful to determine its function in organ development better. WT1 KO organoids showed to be smaller compared to the wildtype which is caused by WT1 inducing an overgrowth of NPCs at the expense of tubular/glomerular differentiation. RNA-seq revealed that NPC markers are induced and decline in control organoids while they persist in WT1 KO organoids as well as mesenchymal to epithelial transition genes were perturbed in KO organoids. Furthermore, KO line-derived organoids transcriptionally and phenotypically resembled a subset of WT1 patients and remained in a pre-epithelialized state. Although protocols and procedures used in this study are established, the successful match and capacity of KO organoids to recapitulate phenotypic and genetic changes of WT1 in humans despite the earlier shortcomings of the organoid technology is exciting.
Taken together, the multiple procedures described for the recapitulation of early stages of kidney development using hPSCs are showing the production of complex 3D culture systems to model human kidney development and disease. These last years have shown the amenability of hPSCs for further transgenesis taking advantage of the CRISPR/Cas9 system, including targeting fluorescent reporters to identify lineage-specific cell types within the organoid, and correlate and trace these processes during differentiation. Furthermore, it is also possible to introduce fluorescent reporters under the endogenous regulation of a renal cell type-specific gene, avoiding undesired responses due to the genetic context and thus provide novel approaches to understand the embryonic origin of a desired cell type. In the next years, these approaches may shed light into some of the questions discussed in the present review, such as the developmental origin of UB cells or endothelial cells in human kidney embryogenesis compared to other model systems as mice. Other advantages of CRISPR stands on the possibility to target Cas9 into any of the well-known safe harbour loci of hPSCs (to date AVVS1, ROSA26 or CLYBL) for further applications which may include the generation of multiple knock-out, the introduction of single nucleotide alterations, as well as generation of inducible knock-out during hPSC differentiation (Gonzalez et al., 2014). Such approach may also facilitate the generation of more complex genomic modifications, such as the generation of reporter alleles via HDR-mediated gene targeting using long donor DNA templates encoding protein tags or fluorescent proteins. On the other hand the combination of deactivated Cas9 (dCas9) fused to functional transcriptional repressors (Krüppel-associated box -KRAB) (Gilbert et al., 2013) or activators [as the tripartite activator VP64-p65-Rta (VPR) module] (Chavez et al., 2015) may open new venues for the simultaneous activation or repression of endogenous coding and noncoding sequences and thus expand our armamentarium when modelling complex renal disorders arising as secondary complications from other major complications (including hypertension, diabetes, among others).
The lessons learnt from these last years, show that there are still important drawbacks that need to be overcome when envisioning kidney organoids as faithful models to target CKD. In this regard, the lack of a vascular component and the immatureness of these tissue surrogates represent major issues that preclude the immediate application of these model systems in renal disease modelling. In this regard, technologies including scRNA-seq are helping the field to categorize the existence and maturation of the organoid cell types and to match their transcriptomic status to that found in the native tissue (including both embryonic and adult kidney counterparts). Exploiting scRNAseq has also being crucial when reference laboratories in the field of kidney development and disease (i.e., Humphreys, Little and McMahon, among others) have established quantitative comparisons between protocols, batches, and pluripotent cell lines providing important information on how to improve protocol’s reproducibility and quality (Wu et al., 2018; Phipson et al., 2019; Subramanian et al., 2019). In the same line, transcriptomic data sets have also been used to reconstruct lineage trajectories to promote maturation of desired kidney cell types or inhibit differentiation of undesired off-target cell types. In the next years the convergence of scRNAseq and kidney organoid technology is expected to provide crucial information on how to externally guide kidney differentiation in a predictable manner and further exploit these cell culture platforms to model renal disease and perform drug screening.
Hopefully, CRISPR-editing in hPSCs derived kidney organoids and further generation of renal-reporter cell lines will continue to expand our understanding on kidney development and disease. Further approaches from the bioengineering field will also increase our capacities to develop complex 3D organoids for applications in renal disease modelling. All these advances together with current efforts in the generation of new Cas9 variants with reduced off-target effects together with high-stringency criteria for sgRNA design will have a strong impact for transitioning from basic research to precise medicine application exploiting hPSC-derived kidney organoid models.
NM, EG, and WS draftet and revised article and figures. AM, PP, and DM contributed images and revised article critically.
We are grateful to members of the N. Montserrat laboratory for insightful discussions and critical reading of the manuscript. We would particularly like to acknowledge the patients and the Fetal Tissue Bank of Vall d’Hebron University Hospital Biobank (PT13/0010/0021), part of the Platform ISCIII Biobanks and Biomodels, for its collaboration. W.S. is supported by Deutsche Forschungsgemeinschaft (DFG- SA 4062/1–1). This work has received funding from the European Research Council (ERC) under the European Union’s Horizon 2020 Research and Innovation Programme (CoG-2020_101002478_ENGINORG to N.M., GA- 874827 BRAV3 to N.M., and GA- 964342—ECABOX to N.M. and A.M). This project has received funding from the Innovative Medicines Initiative 2 Joint Undertaking (JU) under grant agreement no. 101005026. The JU receives support from the European Union’s Horizon 2020 research and innovation program and EFPIA.
This research has been supported by EIT Health under grant ID 20366 (R2U-Tox-Assay) to N.M. N.M. is also supported by the MCIN/AEI/10.13039/501100011033 (PID 2020-119929RB-I00) and MCIN/European Union‘‘Next Generation EU/PRTR’’ (PLEC 2021–008127) and the Generalitat de Catalunya and CERCA Programme, and the IBEC Faster Future program (A por la COVID-19). This study has been funded by Instituto de Salud Carlos III (ISCIII) through the Biobanks and Biomodels Platform and co-funded by the European Union (PTC20/00013, and PTC20/00130 to N.M.) and from Instituto de Salud Carlos III and European Union—Next Generation EU, Plan de Recuperación Transformación y Resiliencia (TERAV/ISCIII RD21/0017/0018) to N.M. This project has received funding from Ayudas Fundación BBVA a Equipos de Investigación Científica SARS-CoV-2 years COVID-19 through the project ‘‘Identifying SARS-CoV-2-host cell interactions exploiting CRISPR-Cas9-engineered human organoids: through the development of specific therapies against COVID19″ and Fundacio’ la Marato’ de TV3 (201910–31 and 202125–3) to N.M.
The authors declare that the research was conducted in the absence of any commercial or financial relationships that could be construed as a potential conflict of interest.
All claims expressed in this article are solely those of the authors and do not necessarily represent those of their affiliated organizations, or those of the publisher, the editors and the reviewers. Any product that may be evaluated in this article, or claim that may be made by its manufacturer, is not guaranteed or endorsed by the publisher.
Ahrabi, A. K., Jouret, F., Marbaix, E., Delporte, C., Horie, S., Mulroy, S., et al. (2010). Glomerular and proximal tubule cysts as early manifestations of Pkd1 deletion. Nephrol. Dial. Transpl. 25, 1067–1078. doi:10.1093/ndt/gfp611
Al-Awqati, Q., and Oliver, J. A. (2002). Stem cells in the kidney. Kidney Int. 61, 387–395. doi:10.1046/j.1523-1755.2002.00164.x
Auerbach, R., and Grobstein, C. (1958). Inductive interaction of embryonic tissues after dissociation and reaggregation. Exp. Cell Res. 15, 384–397. doi:10.1016/0014-4827(58)90039-9
Baer, P. C., Tunn, U. W., Nunez, G., Scherberich, J. E., and Geiger, H. (1999). Transdifferentiation of distal but not proximal tubular epithelial cells from human kidney in culture. Exp. Nephrol. 7, 306–313. doi:10.1159/000020618
Bagherie-Lachidan, M., Reginensi, A., Pan, Q., Zaveri, H. P., Scott, D. A., Blencowe, B. J., et al. (2015). Stromal Fat4 acts non-autonomously with Dchs1/2 to restrict the nephron progenitor pool. Dev. Camb. 142, 2564–2573. doi:10.1242/dev.122648
Begum, S. (2019). Engineering renal epithelial cells: Programming and directed differentiation towards glomerular podocyte’s progenitor and mature podocyte. Am. J. Transl. Res. 11, 1102–1115.
Bertram, J. F., Douglas-Denton, R. N., Diouf, B., Hughson, M. D., and Hoy, W. E. (2011). Human nephron number: Implications for health and disease. Pediatr. Nephrol. 26, 1529–1533. doi:10.1007/s00467-011-1843-8
Boreström, C., Jonebring, A., Guo, J., Palmgren, H., Cederblad, L., Forslöw, A., et al. (2018). A CRISP(e)R view on kidney organoids allows generation of an induced pluripotent stem cell–derived kidney model for drug discovery. Kidney Int. 94, 1099–1110. doi:10.1016/j.kint.2018.05.003
Boyle, S., Misfeldt, A., Chandler, K. J., Deal, K. K., Southard-Smith, E. M., Mortlock, D. P., et al. (2008). Fate mapping using Cited1-CreERT2 mice demonstrates that the cap mesenchyme contains self-renewing progenitor cells and gives rise exclusively to nephronic epithelia. Dev. Biol. 313, 234–245. doi:10.1016/j.ydbio.2007.10.014
Boyle, S., Shioda, T., Perantoni, A. O., and de Caestecker, M. (2007). Cited1 and Cited2 are differentially expressed in the developing kidney but are not required for nephrogenesis. Dev. Dyn. 236, 2321–2330. doi:10.1002/dvdy.21242
Brown, A. C., Muthukrishnan, S. D., and Oxburgh, L. (2015). A synthetic niche for nephron progenitor cells. Dev. Cell 34, 229–241. doi:10.1016/j.devcel.2015.06.021
Bruce, S. J., Rea, R. W., Steptoe, A. L., Busslinger, M., Bertram, J. F., Perkins, A. C., et al. (2007). In vitro differentiation of murine embryonic stem cells toward a renal lineage. Differentiation. 75, 337–349. doi:10.1111/j.1432-0436.2006.00149.x
Chavez, A., Scheiman, J., Vora, S., Pruitt, B. W., Tuttle, M., P R Iyer, E., et al. (2015). Highly efficient Cas9-mediated transcriptional programming. Nat. Methods 12, 326–328. doi:10.1038/nmeth.3312
Combes, A. N., Zappia, L., Er, P. X., Oshlack, A., and Little, M. H. (2019). Single-cell analysis reveals congruence between kidney organoids and human fetal kidney. Genome Med. 11, 3. doi:10.1186/s13073-019-0615-0
Costantini, F., and Kopan, R. (2010). Patterning a complex organ: Branching morphogenesis and nephron segmentation in kidney development. Dev. Cell 18, 698–712. doi:10.1016/j.devcel.2010.04.008
Cruz, N. M., Song, X., Czerniecki, S. M., Gulieva, R. E., Churchill, A. J., Kim, Y. K., et al. (2017). Organoid cystogenesis reveals a critical role of microenvironment in human polycystic kidney disease. Nat. Mat. 16, 1112–1119. doi:10.1038/NMAT4994
Czerniecki, S. M., Cruz, N. M., Harder, J. L., Menon, R., Annis, J., Otto, E. A., et al. (2018). High-throughput screening enhances kidney organoid differentiation from human pluripotent stem cells and enables automated multidimensional phenotyping. Cell Stem Cell 22, 929–940. e4. doi:10.1016/j.stem.2018.04.022
Devuyst, O., Burrow, C. R., Smith, B. L., Agre, P., Knepper, M. A., Wilson, P. D., et al. (1996). Expression of aquaporins-1 and -2 during nephrogenesis and in autosomal dominant polycystic kidney disease. Am. J. Physiol. 271, F169–F183. doi:10.1152/ajprenal.1996.271.1.F169
Elger, M., Hentschel, H., Litteral, J., Wellner, M., Kirsch, T., Luft, F. C., et al. (2003). Nephrogenesis is induced by partial nephrectomy in the elasmobranch Leucoraja erinacea. J. Am. Soc. Nephrol. 14, 1506–1518. doi:10.1097/01.ASN.0000067645.49562.09
Eremina, V., Sood, M., Haigh, J., Nagy, A., Lajoie, G., Ferrara, N., et al. (2003). Glomerular-specific alterations of VEGF-A expression lead to distinct congenital and acquired renal diseases. J. Clin. Invest. 111, 707–716. doi:10.1172/JCI17423
Forbes, T. A., Howden, S. E., Lawlor, K., Phipson, B., Maksimovic, J., Hale, L., et al. (2018). Patient-iPSC-Derived kidney organoids show functional validation of a ciliopathic renal phenotype and reveal underlying pathogenetic mechanisms. Am. J. Hum. Genet. 102, 816–831. doi:10.1016/j.ajhg.2018.03.014
Freedman, B. S., Brooks, C. R., Lam, A. Q., Fu, H., Morizane, R., Agrawal, V., et al. (2015). Modelling kidney disease with CRISPR-mutant kidney organoids derived from human pluripotent epiblast spheroids. Nat. Commun. 6, 8715. doi:10.1038/ncomms9715
Ganeva, V., Unbekandt, M., and Davies, J. A. (2011). An improved kidney dissociation and re-aggregation culture system results in nephrons arranged organotypically around a single collecting duct system. Organogenesis 7, 83–87. doi:10.4161/org.7.2.14881
Garreta, E., Prado, P., Tarantino, C., Oria, R., Fanlo, L., Martí, E., et al. (2019). Fine tuning the extracellular environment accelerates the derivation of kidney organoids from human pluripotent stem cells. Nat. Mat. 18, 397–405. doi:10.1038/s41563-019-0287-6
Georgas, K. M., Chiu, H. S., Lesieur, E., Rumballe, B. A., and Little, M. H. (2011). Expression of metanephric nephron-patterning genes in differentiating mesonephric tubules. Dev. Dyn. 240, 1600–1612. doi:10.1002/dvdy.22640
Gilbert, L. A., Larson, M. H., Morsut, L., Liu, Z., Brar, G. A., Torres, S. E., et al. (2013). CRISPR-mediated modular RNA-guided regulation of transcription in eukaryotes. Cell 154, 442–451. doi:10.1016/j.cell.2013.06.044
Gonzalez, F., Zhu, Z., Shi, Z.-D., Lelli, K., Verma, N., Li, Q. V., et al. (2014). An iCRISPR platform for rapid, multiplexable, and inducible genome editing in human pluripotent stem cells. Cell stem cell 15, 215–226. doi:10.1016/j.stem.2014.05.018
Gray, P. (1930). Memoirs: The development of the Amphibian kidney: Part I: The development of the mesonephros of Rana temporaria. J. cell Sci. 2, 507. doi:10.1242/jcs.s2-78.311.445
Grobstein, C. (1953). Inductive epitheliomesenchymal interaction in cultured organ rudiments of the mouse. Sci. (New York, N.Y.) 118, 52–55. doi:10.1126/science.118.3054.52
Gupta, N., Matsumoto, T., Hiratsuka, K., Garcia Saiz, E., Galichon, P., Miyoshi, T., et al. (2022). Modeling injury and repair in kidney organoids reveals that homologous recombination governs tubular intrinsic repair. Sci. Transl. Med. 14, eabj4772. doi:10.1126/scitranslmed.abj4772
Hendry, C. E., and Little, M. H. (2012). Reprogramming the kidney: A novel approach for regeneration. Kidney Int. 82, 138–146. doi:10.1038/ki.2012.68
Hinchliffe, S. A., Sargent, P. H., Howard, C. V., Chan, Y. F., and Velzen, D. V. (1991). Human intrauterine renal growth expressed in absolute number of glomeruli assessed by the disector method and Cavalieri principle. Lab. Invest. 64, 777–784.
Ho, J., Kar, H. N., Rosen, S., Dostal, A., Gregory, R. I., Kreidberg, J. A., et al. (2008). Podocyte-specific loss of functional microRNAs leads to rapid glomerular and tubular injury. J. Am. Soc. Nephrol. 19, 2069–2075. doi:10.1681/ASN.2008020162
Hochane, M., van den Berg, P. R., Fan, X., Bérenger-Currias, N., Adegeest, E., Bialecka, M., et al. (2019). Single-cell transcriptomics reveals gene expression dynamics of human fetal kidney development. doi:10.1371/journal.pbio.3000152
Homan, K. A., Gupta, N., Kroll, K. T., Kolesky, D. B., Skylar-Scott, M., Miyoshi, T., et al. (2019). Flow-enhanced vascularization and maturation of kidney organoids in vitro. Nat. Methods 16, 255–262. doi:10.1038/s41592-019-0325-y
Howden, S. E., Thomson, J. A., and Little, M. H. (2018). Simultaneous reprogramming and gene editing of human fibroblasts. Nat. Protoc. 13, 875–898. doi:10.1038/nprot.2018.007
Howden, S. E., Vanslambrouck, J. M., Wilson, S. B., Tan, K. S., and Little, M. H. (2019). Reporter-based fate mapping in human kidney organoids confirms nephron lineage relationships and reveals synchronous nephron formation. EMBO Rep. 20, e47483. doi:10.15252/embr.201847483
Humphreys, B. D., and DiRocco, D. P. (2014). Lineage-tracing methods and the kidney. Kidney Int. 86, 481–488. doi:10.1038/ki.2013.368
Imberti, B., Tomasoni, S., Ciampi, O., Pezzotta, A., Derosas, M., Xinaris, C., et al. (2015). Renal progenitors derived from human iPSCs engraft and restore function in a mouse model of acute kidney injury. Sci. Rep. 5, 8826. doi:10.1038/srep08826
Jenkinson, S. E., Chung, G. W., van Loon, E., Bakar, N. S., Dalzell, A. M., Brown, C. D. A., et al. (2012). The limitations of renal epithelial cell line HK-2 as a model of drug transporter expression and function in the proximal tubule. Pflugers Arch. 464, 601–611. doi:10.1007/s00424-012-1163-2
Kim, A. D., Lake, B. B., Chen, S., Wu, Y., Guo, J., Parvez, R. K., et al. (2019). Cellular recruitment by podocyte-derived pro-migratory factors in assembly of the human renal filter. iScience 20, 402–414. doi:10.1016/j.isci.2019.09.029
Kim, D., and Dressler, G. R. (2005). Nephrogenic factors promote differentiation of mouse embryonic stem cells into renal epithelia. J. Am. Soc. Nephrol. 16, 3527–3534. doi:10.1681/ASN.2005050544
Kim, Y. K., Refaeli, I., Brooks, C. R., Jing, P., Gulieva, R. E., Hughes, M. R., et al. (2017). Gene-edited human kidney organoids reveal mechanisms of disease in podocyte development. Stem Cells 35, 2366–2378. doi:10.1002/stem.2707
Kobayashi, A., Valerius, M. T., Mugford, J. W., Carroll, T. J., Self, M., Oliver, G., et al. (2008). Six2 defines and regulates a multipotent self-renewing nephron progenitor population throughout mammalian kidney development. Cell Stem Cell 3, 169–181. doi:10.1016/j.stem.2008.05.020
Kumar Gupta, A., Sarkar, P., Wertheim, J. A., Pan, X., Carroll, T. J., Oxburgh, L., et al. (2020). Asynchronous mixing of kidney progenitor cells potentiates nephrogenesis in organoids. Commun. Biol. 3, 231. doi:10.1038/s42003-020-0948-7
Kumar, S., Liu, J., and McMahon, A. P. (2014). Defining the acute kidney injury and repair transcriptome. Semin. Nephrol. 34, 404–417. doi:10.1016/j.semnephrol.2014.06.007
Kuraoka, S., Tanigawa, S., Taguchi, A., Hotta, A., Nakazato, H., Osafune, K., et al. (2020). PKD1-Dependent renal cystogenesis in human induced pluripotent stem cell-derived ureteric bud/collecting duct organoids. J. Am. Soc. Nephrol. 31, 2355–2371. doi:10.1681/ASN.2020030378
Lam, A. Q., Freedman, B. S., and Bonventre, J. V. (2014). Directed differentiation of pluripotent stem cells to kidney cells. Semin. Nephrol. 34, 445–461. doi:10.1016/j.semnephrol.2014.06.011
Lindström, N. O., De Sena Brandine, G., Tran, T., Ransick, A., Suh, G., Guo, J., et al. (2018a). Progressive recruitment of mesenchymal progenitors reveals a time-dependent process of cell fate acquisition in mouse and human nephrogenesis. Dev. Cell 45, 651–660. e4. doi:10.1016/j.devcel.2018.05.010
Lindström, N. O., Tran, T., Guo, J., Rutledge, E., Parvez, R. K., Thornton, M. E., et al. (2018b). Conserved and divergent molecular and anatomic features of human and mouse nephron patterning. J. Am. Soc. Nephrol. 29, 825–840. doi:10.1681/ASN.2017091036
Little, M. H., Brennan, J., Georgas, K., Davies, J. A., Davidson, D. R., Baldock, R. A., et al. (2007). Corrigendum to “A high-resolution anatomical ontology of the developing murine genitourinary tract” [Gene Expression Patterns 7 (2007) 680–699]. Gene Expr. Patterns 8, 47–50. doi:10.1016/j.modgep.2007.07.003
Little, M. H., and Combes, A. N. (2019). Kidney organoids: Accurate models or fortunate accidents. Genes Dev. 33, 1319–1345. doi:10.1101/gad.329573.119
Little, M. H., and McMahon, A. P. (2012). Mammalian kidney development: Principles, progress, and projections. Cold Spring Harb. Perspect. Biol. 4, a008300. doi:10.1101/cshperspect.a008300
Low, J. H., Li, P., Chew, E. G. Y., Zhou, B., Suzuki, K., Zhang, T., et al. (2019). Generation of human PSC-derived kidney organoids with patterned nephron segments and a de novo vascular network. Cell Stem Cell 1, 373–387. doi:10.1016/j.stem.2019.06.009
Mae, S.-I., Shono, A., Shiota, F., Yasuno, T., Kajiwara, M., Gotoda-Nishimura, N., et al. (2013). Monitoring and robust induction of nephrogenic intermediate mesoderm from human pluripotent stem cells. Nat. Commun. 4, 1367. doi:10.1038/ncomms2378
Magella, B., Adam, M., Potter, A. S., Venkatasubramanian, M., Chetal, K., Hay, S. B., et al. (2018). Cross-platform single cell analysis of kidney development shows stromal cells express Gdnf. Dev. Biol. 434, 36–47. doi:10.1016/j.ydbio.2017.11.006
Mao, Y., Francis-West, P., and Irvine, K. D. (2015). Fat4/Dchs1 signaling between stromal and cap mesenchyme cells influences nephrogenesis and ureteric bud branching. Dev. Camb. Engl. 142, 2574–2585. doi:10.1242/dev.122630
Menon, R., Otto, E. A., Kokoruda, A., Zhou, J., Zhang, Z., Yoon, E., et al. (2018). Single-cell analysis of progenitor cell dynamics and lineage specification in the human fetal kidney. Development 145, dev164038. doi:10.1242/dev.164038
Merlet-Benichou, C., Gilbert, T., Vilar, J., Moreau, E., Freund, N., Lelievre- Pegorier, M., et al. (1999). Nephron number: Variability is the rule: Causes and consequences. Lab. Invest. 79, 515–527.
Morizane, R., Lam, A. Q., Freedman, B. S., Kishi, S., Valerius, M. T., Bonventre, J. V., et al. (2015). Nephron organoids derived from human pluripotent stem cells model kidney development and injury. Nat. Biotechnol. 33, 1193–1200. doi:10.1038/nbt.3392
Morizane, R., Monkawa, T., Fujii, S., Yamaguchi, S., Homma, K., Matsuzaki, Y., et al. (2013). Kidney specific protein-positive cells derived from embryonic stem cells reproduce tubular structures in vitro and differentiate into renal tubular cells. PLoS ONE 8, e64843. doi:10.1371/journal.pone.0064843
Musah, S., Mammoto, A., Ferrante, T. C., Jeanty, S. S. F., Hirano-Kobayashi, M., Mammoto, T., et al. (2017). Mature induced-pluripotent-stem-cell-derived human podocytes reconstitute kidney glomerular-capillary-wall function on a chip. Nat. Biomed. Eng. 1, 0069. doi:10.1038/s41551-017-0069
Narayanan, K., Schumacher, K. M., Tasnim, F., Kandasamy, K., Schumacher, A., Ni, M., et al. (2013). Human embryonic stem cells differentiate into functional renal proximal tubular-like cells. Kidney Int. 83, 593–603. doi:10.1038/ki.2012.442
Nishinakamura, R., Matsumoto, Y., Nakao, K., Nakamura, K., Sato, A., Copeland, N. G., et al. (2001). Murine homolog of SALL1 is essential for ureteric bud invasion in kidney development. Development 128, 3105–3115. doi:10.1242/dev.128.16.3105
Osafune, K., Takasato, M., Kispert, A., Asashima, M., and Nishinakamura, R. (2006). Identification of multipotent progenitors in the embryonic mouse kidney by a novel colony-forming assay. Development 133, 151–161. doi:10.1242/dev.02174
Pelletier, J., Schalling, M., Buckler, A. J., Rogers, A., Haber, D. A., and Housman, D. (1991). Expression of the Wilms’ tumor gene WT1 in the murine urogenital system. Genes Dev. 5, 1345–1356. doi:10.1101/gad.5.8.1345
Phipson, B., Er, P. X., Combes, A. N., Forbes, T. A., Howden, S. E., Zappia, L., et al. (2019). Evaluation of variability in human kidney organoids. Nat. Methods 16, 79–87. doi:10.1038/s41592-018-0253-2
Reimschuessel, R., and Williams, D. (1995). Development of new nephrons in adult kidneys following gentamicin-induced nephrotoxicity. Ren. Fail. 17, 101–106. doi:10.3109/08860229509026246
Romagnani, P., and Anders, H. J. (2013). What can tubular progenitor cultures teach us about kidney regeneration? Kidney Int. 83, 351–353. doi:10.1038/ki.2012.437
Romagnani, P., Lasagni, L., and Remuzzi, G. (2013). Renal progenitors: An evolutionary conserved strategy for kidney regeneration. Nat. Rev. Nephrol. 9, 137–146. doi:10.1038/nrneph.2012.290
Rosselot, C., Spraggon, L., Chia, I., Batourina, E., Riccio, P., Lu, B., et al. (2010). Non-cell-autonomous retinoid signaling is crucial for renal development. Development 137, 283–292. doi:10.1242/dev.040287
Shi, S., Yu, L., Chiu, C., Sun, Y., Chen, J., Khitrov, G., et al. (2008). Podocyte-selective deletion of dicer induces proteinuria and glomerulosclerosis. J. Am. Soc. Nephrol. 19, 2159–2169. doi:10.1681/ASN.2008030312
Shankland, S. J., Pippin, J. W., Reiser, J., and Mundel, P. (2007). Podocytes in culture: Past, present, and future. Kidney Int. 72, 26–36. doi:10.1038/sj.ki.5002291
Sharmin, S., Taguchi, A., Kaku, Y., Yoshimura, Y., Ohmori, T., Sakuma, T., et al. (2016). Human induced pluripotent stem cell-derived podocytes mature into vascularized glomeruli upon experimental transplantation. J. Am. Soc. Nephrol. 27, 1778–1791. doi:10.1681/ASN.2015010096
Sison, K., Eremina, V., Baelde, H., Min, W., Hirashima, M., Fantus, I. G., et al. (2010). Glomerular structure and function require paracrine, not autocrine, VEGF-VEGFR-2 signaling. J. Am. Soc. Nephrol. 21, 1691–1701. doi:10.1681/ASN.2010030295
Solomon, S. E. (1985). The morphology of the kidney of the green turtle (Chelonia mydas L.). J. Anat. 140, 355–369.
Song, B., Smink, A. M., Jones, C. V., Callaghan, J. M., Firth, S. D., Bernard, C. A., et al. (2012). The directed differentiation of human iPS cells into kidney podocytes. PLoS One 7, e46453. doi:10.1371/journal.pone.0046453
Soufi, A., Donahue, G., and Zaret, K. S. (2012). Facilitators and impediments of the pluripotency reprogramming factors’ initial engagement with the genome. Cell 151, 994–1004. doi:10.1016/j.cell.2012.09.045
Subramanian, A., Sidhom, E. H., Emani, M., Vernon, K., Sahakian, N., Zhou, Y., et al. (2019). Single cell census of human kidney organoids shows reproducibility and diminished off-target cells after transplantation. Nat. Commun. 10, 5462. doi:10.1038/s41467-019-13382-0
Taguchi, A., Kaku, Y., Ohmori, T., Sharmin, S., Ogawa, M., Sasaki, H., et al. (2014). Redefining the in vivo origin of metanephric nephron progenitors enables generation of complex kidney structures from pluripotent stem cells. Cell Stem Cell 14, 53–67. doi:10.1016/j.stem.2013.11.010
Taguchi, A., and Nishinakamura, R. (2017). Higher-order kidney organogenesis from pluripotent stem cells. Cell Stem Cell 21, 730–746. e6. doi:10.1016/j.stem.2017.10.011
Takahashi, K., Tanabe, K., Ohnuki, M., Narita, M., Ichisaka, T., Tomoda, K., et al. (2007). Induction of pluripotent stem cells from adult human fibroblasts by defined factors. Cell 131, 861–872. doi:10.1016/j.cell.2007.11.019
Takasato, M., Er, P. X., Becroft, M., Vanslambrouck, J. M., Stanley, E. G., Elefanty, A. G., et al. (2014). Directing human embryonic stem cell differentiation towards a renal lineage generates a self-organizing kidney. Nat. Cell Biol. 16, 118–126. doi:10.1038/ncb2894
Takasato, M., Er, P. X., Chiu, H. S., Maier, B., Baillie, G. J., Ferguson, C., et al. (2015). Kidney organoids from human iPS cells contain multiple lineages and model human nephrogenesis. Nature 526, 564–568. doi:10.1038/nature15695
Tecklenborg, J., Clayton, D., Siebert, S., and Coley, S. M. (2018). The role of the immune system in kidney disease. Clin. Exp. Immunol. 192, 142–150. doi:10.1111/cei.13119
Thomson, J. A., Itskovitz-eldor, J., Shapiro, S. S., Waknitz, M. A., Swiergiel, J. J., Marshall, V. S., et al. (1998). Embryonic stem cell lines derived from human blastocysts. Science 282, 1145–1147. doi:10.1126/science.282.5391.1145
Torres, M., Gómez-Pardo, E., Dressler, G. R., and Gruss, P. (1995). Pax-2 controls multiple steps of urogenital development. Development 121, 4057–4065. doi:10.1242/dev.121.12.4057
Van Den Berg, C. W., Ritsma, L., Avramut, M. C., Wiersma, L. E., van den Berg, B. M., Leuning, D. G., et al. (2018). Renal subcapsular transplantation of PSC-derived kidney organoids induces neo-vasculogenesis and significant glomerular and tubular maturation in vivo. Stem Cell Rep. 10, 751–765. doi:10.1016/j.stemcr.2018.01.041
Vanslambrouck, J. M., Wilson, S. B., Tan, K. S., Soo, J. Y., Scurr, M., Spijker, H. S., et al. (2019). A toolbox to characterize human induced pluripotent stem cell – derived kidney cell types and organoids. J. Am. Soc. Nephrol. 30 (10), 1811–1823. doi:10.1681/ASN.2019030303
Vigneau, C., Polgar, K., Striker, G., Elliott, J., Hyink, D., Weber, O., et al. (2007). Mouse embryonic stem cell-derived embryoid bodies generate progenitors that integrate long term into renal proximal tubules in vivo. J. Am. Soc. Nephrol. 18, 1709–1720. doi:10.1681/ASN.2006101078
Webster, A. C., Nagler, E. V., Morton, R. L., and Masson, P. (2017). Chronic kidney disease. Lancet 389, 1238–1252. doi:10.1016/S0140-6736(16)32064-5
Wörsdörfer, P., and Ergün, S. (2021). The impact of oxygen availability and multilineage communication on organoid maturation. Antioxid. Redox Signal. 35, 217–233. doi:10.1089/ars.2020.8195
Wouters, O. J., O’Donoghue, D. J., Ritchie, J., Kanavos, P. G., and Narva, A. S. (2015). Early chronic kidney disease: Diagnosis, management and models of care. Nat. Rev. Nephrol. 11, 491–502. doi:10.1038/nrneph.2015.85
Wu, H., Uchimura, K., Donnelly, E. L., Kirita, Y., Morris, S. A., Humphreys, B. D., et al. (2018). Comparative analysis and refinement of human PSC-derived kidney organoid differentiation with single-cell transcriptomics. Cell Stem Cell 23, 869–881. e8. doi:10.1016/j.stem.2018.10.010
Xia, Y., Nivet, E., Sancho-Martinez, I., Gallegos, T., Suzuki, K., Okamura, D., et al. (2013). Directed differentiation of human pluripotent cells to ureteric bud kidney progenitor-like cells. Nat. Cell Biol. 15, 1507–1515. doi:10.1038/ncb2872
Yousef Yengej, F. A., Jansen, J., Rookmaaker, M. B., Verhaar, M. C., and Clevers, H. (2020). Kidney organoids and tubuloids. Cells 9, E1326. doi:10.3390/cells9061326
Zeisberg, M., and Kalluri, R. (2015). Physiology of the renal interstitium. Clin. J. Am. Soc. Nephrol. 10, 1831–1840. doi:10.2215/CJN.00640114
Zhang, K., Chen, S., Sun, H., Wang, L., Li, H., Zhao, J., et al. (2020). In vivo two-photon microscopy reveals the contribution of Sox9+ cell to kidney regeneration in a mouse model with extracellular vesicle treatment. J. Biol. Chem. 295, 12203–12213. doi:10.1074/jbc.RA120.012732
Zoccali, C., Arici, M., Blankestijn, P. J., Bruchfeld, A., Capasso, G., Fliser, D., et al. (2018). The ERA-EDTA today and tomorrow: A progress document by the ERA-EDTA Council. Clin. Kidney J. 11, 437–442. doi:10.1093/ckj/sfy064
Chronic kidney disease Condition characterized by a gradual loss of kidney function over time based on the presence of kidney damage or glomerular filtration rate (GFR <60 ml/min per 1.73 m2) for ≥3 months, and it is classified into five stages based on the level of GFR
Metanephros Phase of kidney development during the fifth week of gestation in which ureteric bud start to develop into collecting duct system
Intermediate mesoderm A region of embryonic mesoderm extending anterior–posterior between the paraxial, or somitic, mesoderm and the lateral plate mesoderm
Collecting duct system Tubes that receive and concentrates urine from the distal convoluted tubule of nephrons and exudes it into the renal pelvis
Ureteric bud An epithelial tube that arises from the nephric duct during embryonic development
Metanephric mesenchyme The region of posterior intermediate mesoderm adjacent to the nephric ducts from where to the epithelial cells of the kidney emerge upon kidney induction
Cap mesenchyme Comprised of cells which epithelialize and sequentially form the pretubular aggregate (PA), renal vesicle (RV), C-, and S-shaped bodies, and finally the mature nephron
Kidney induction The activation of the epithelial-specific program in the metanephric mesenchyme in response to signals that promote ureteric bud invasion and branching to give rise to the renal collecting duct system
Renal vesicle The first polarized epithelial derivative of the induced metanephric mesenchyme that is found abutting the branching tips of the ureteric bud
Nephron progenitor cells Self-renewing cells which are able to give rise to all the cell types of the kidney. They are involved in the homeostasis and repair of the kidney and they have a therapeutic potential for treatment of kidney failure
Stroma progenitor cells Embryonic connective tissue that is derived from the mesoderm and that differentiates into hematopoietic and connective tissue
Human pluripotent stem cells Stem cell that has the potential to differentiate into any of the three germ layers: endoderm, mesoderm, or ectoderm
Human embryonic stem cells Pluripotent stem cells derived from the inner cell mass of a blastocyst, an early-stage pre-implantation embryo
Human induced pluripotent stem cells Type of pluripotent stem cell that can be generated in vitro from a somatic cell
Embryoid bodies Three-dimensional aggregates of pluripotent stem cells
CRISPR/Cas9 It is a prokaryotic immune system that confers resistance to foreign genetic elements such as those present within plasmids and phages and provides a form of acquired immunity. This system has been used as gene editing technique that enables geneticists and medical researchers to edit parts of the genome by removing, adding or altering sections of the DNA sequence
Single cell RNA sequencing Technique which provides the expression profiles of individual cells with optimized next generation sequencing technologies, giving a higher resolution of cellular differences and a better understanding of the function of the cell in its microenvironment
Reporter cell line Engineered cell in which a specific gene is modified in its regulatory sequence in order to measure or identify the expression or being used as selectable markers
Keywords: pluripotent stem cells, CRISPR, nephrogenesis, kidney engineering, kidney organoids
Citation: Safi W, Marco A, Moya D, Prado P, Garreta E and Montserrat N (2022) Assessing kidney development and disease using kidney organoids and CRISPR engineering. Front. Cell Dev. Biol. 10:948395. doi: 10.3389/fcell.2022.948395
Received: 19 May 2022; Accepted: 06 July 2022;
Published: 02 September 2022.
Edited by:
Macarena Perán, University of Jaén, SpainReviewed by:
Ryuji Morizane, Harvard Medical School, United StatesCopyright © 2022 Safi, Marco, Moya, Prado, Garreta and Montserrat. This is an open-access article distributed under the terms of the Creative Commons Attribution License (CC BY). The use, distribution or reproduction in other forums is permitted, provided the original author(s) and the copyright owner(s) are credited and that the original publication in this journal is cited, in accordance with accepted academic practice. No use, distribution or reproduction is permitted which does not comply with these terms.
*Correspondence: Wajima Safi, d3NhZmlAaWJlY2JhcmNlbG9uYS5ldQ==; Elena Garreta, ZWdhcnJldGFAaWJlY2JhcmNlbG9uYS5ldQ==; Nuria Montserrat, bm1vbnRzZXJyYXRAaWJlY2JhcmNlbG9uYS5ldQ==
†These authors have contributed equally to this work
Disclaimer: All claims expressed in this article are solely those of the authors and do not necessarily represent those of their affiliated organizations, or those of the publisher, the editors and the reviewers. Any product that may be evaluated in this article or claim that may be made by its manufacturer is not guaranteed or endorsed by the publisher.
Research integrity at Frontiers
Learn more about the work of our research integrity team to safeguard the quality of each article we publish.