- 1Reproductive and Developmental Biology Laboratory, National Institute of Environmental Health Sciences, Research Triangle Park, NC, United States
- 2Université Paris-Saclay, UVSQ, INRAE, BREED, Jouy en Josas, France
Differentiation of the bipotential gonadal primordium into ovaries and testes is a common process among vertebrate species. While vertebrate ovaries eventually share the same functions of producing oocytes and estrogens, ovarian differentiation relies on different morphogenetic, cellular, and molecular cues depending on species. The aim of this review is to highlight the conserved and divergent features of ovarian differentiation through an evolutionary perspective. From teleosts to mammals, each clade or species has a different story to tell. For this purpose, this review focuses on three specific aspects of ovarian differentiation: ovarian morphogenesis, the evolution of the role of estrogens on ovarian differentiation and the molecular pathways involved in granulosa cell determination and maintenance.
Introduction
In vertebrate species, ovaries arise from the gonadal primordium, a structure that has the bipotential capacity to differentiate into either ovaries or testes during embryogenesis. This dual developmental fate relies on the ability of gonadal cells to differentially respond to genetic or environmental cues that will dictate their fate toward ovarian or testis identity. Among the gonadal somatic cells, the supporting cells are considered the orchestrator of gonad differentiation. These supporting cells are usually the first gonadal cell-type to initiate their male or female fate and become Sertoli or granulosa cells respectively (Sinclair et al., 1990). For instance, in most mammals, the XY supporting cells first express the Y-linked sex-determining gene SRY, which activates the Sertoli-cell differentiation program that drives testis differentiation (Koopman et al., 1991; Albrecht and Eicher, 2001; Sekido and Lovell-Badge, 2008). In the absence of the Y-chromosome or of SRY, supporting cells differentiate into granulosa cells, tipping the gonadal fate toward ovaries. For this reason, ovarian differentiation has been considered a default process. However, genetic evidence from mice, humans, and other species have revealed that ovarian differentiation is in fact an active process that involves unique morphogenetic changes and activation/repression of specific genetic programs. Beyond mammals, bipotential gonads of all vertebrate species face this critical fate decision to differentiate into an ovary or a testis. Gonadal development and ovarian differentiation vary among vertebrate species. From different chromosomic systems (XX/XY vs. ZZ/ZW vs. polygenic), different sex determining triggers (SRY in mammals, DMRT1/DMY in chicken and medaka, AMHY in tilapia) (Nagahama et al., 2021), different cell origins, to divergent ovarian morphology, each clade or species adapts its unique molecular and cellular mechanisms. Despite this divergence, there is a large conservation of key genetic players and cell types involved in ovarian differentiation throughout vertebrate evolution.
In this review, we intend to generate a comparative view of ovarian differentiation from three main vertebrate clades: fish, birds, and mammals. We focus on three aspects of ovarian differentiation: 1) ovarian morphogenesis and origins of granulosa cells; 2) the evolutionary role of estrogens in ovarian differentiation; and 3) the genetic pathways that regulate granulosa cell/ovarian differentiation.
Ovary morphogenesis and origins of granulosa cells
In vertebrates, the ovary develops from the gonadal primordium that eventually form a structure composed of follicles, the functional units of the ovary. Follicles are composed of three key cell populations: an oocyte, surrounded by supporting granulosa cells, themselves surrounded by steroidogenic theca cells. Precursors of these cell populations are suspected to be present in the gonadal primordium. The primordium arises from the convergence of primordial germ cells, along with coordinated events of epithelial-mesenchymal transition (EMT) from the coelomic epithelium/lateral plate mesoderm and cell migration from the mesonephric/pronephric region. Morphogenesis of the ovary has been studied in various species and the origin of ovarian granulosa cells has been a long ongoing debate. It was already speculated in the 1960s/70s that mammalian granulosa cells could arise from either two waves of recruitment from the ovarian surface epithelium to form medullary and cortical cords; or from the mesenchyme; or even from cells of mesonephric origin, the “rete ovarii” (for review, see Byskov, 1986). While vertebrate ovaries eventually share the same functions of producing oocytes and reproductive hormones, the ovarian morphogenesis varies from species to species.
Fish
Teleosts are the largest infraclass of the Actinopterygii, the ray-finned fishes. They present a variety of sex determination strategies that range from gonochorism, when the bipotential gonads directly differentiate into either an ovary or a testis, to sequential hermaphroditism, when a species switches sex later in life. Such diversity is caused by the capacity of the gonad to follow various genetic and/or environmental cues in a species-specific manner. Consequently, the timing and process of ovarian morphogenesis differ greatly from one fish species to another.
The medaka (Oryzias latipes) is one of the most-studied fish species regarding sex-determination and differentiation. It is a gonochoristic species, and the bipotential gonads differentiate into an ovary or a testis based on the presence of XX or XY sex chromosomes with the Y-linked sex determining gene dmy/dmrt1by (Matsuda et al., 2002; Nanda et al., 2002). Gonadal ridges form between the hindgut and nephric ducts around 3 days post-fertilization (dpf) (Figure 1A). Two somatic cell gonadal precursors originate from the lateral plate mesoderm: cells expressing ftz-f1, ortholog of Nr5a1, located laterally to the hindgut, and cells expressing sox9b, located more dorsally between the hindgut and nephric duct (Nakamura et al., 2006). The migrating primordial germ cells (PGCs), sox9b+ cells and ftz-f1+ cells meet dorsally around stage 33 (around 4.4 dpf) to form a single gonadal primordium (Figure 1A). The sox9b+ cells correspond to supporting cell progenitors that surround germ cells to form bipotential “units” common to both male and female gonads (Nakamura et al., 2008; Nishimura et al., 2016). As sox9b+ cells surround PGCs, they start expressing ftz-f1 (Nishimura and Tanaka, 2014). These sox9b+ bipotential supporting cells form interwoven threadlike cords that surround nests of germline stem cells to form germinal cradles. These cradles, along with epithelial cells, constitute the multilayered germinal epithelium that contribute to egg production throughout adult life (Nakamura et al., 2010). The first sexually dimorphic difference is the presence of more germ cells in female gonads than the male gonads 1 day before hatching at stage 38 (Satoh and Egami, 1972), followed by meiosis initiation 1-day post-hatching (dph) (Nakamura et al., 2006). After initiation of ovarian differentiation at hatching, diplotene oocytes individually surrounded by sox9b+ supporting cells exit the cradles for the stroma. These supporting cells further differentiate into granulosa cells by downregulating sox9b expression and activating foxl2 (Nakamoto et al., 2006). Meanwhile, around 5 dph, cyp19a1a becomes expressed in stroma cells located in the ventral region of the differentiating ovary (Figure 1A) (Nakamura et al., 2009). Once in the stroma compartment, granulosa cells recruit two types of steroidogenic stroma cells, cyp19a1a+ cells and cyp17a1+ cells, to form the outer theca layer of the follicles (Nakamura et al., 2009). As the gonad differentiates into an ovary, it is composed of three main structures: 1) the stroma, located in the ventral side where follicles will grow; 2) the germinal epithelium containing the germinal cradles; and 3) the ovarian cavity, formed around 30 dph, into which mature oocytes are ovulated (Figure 1A).
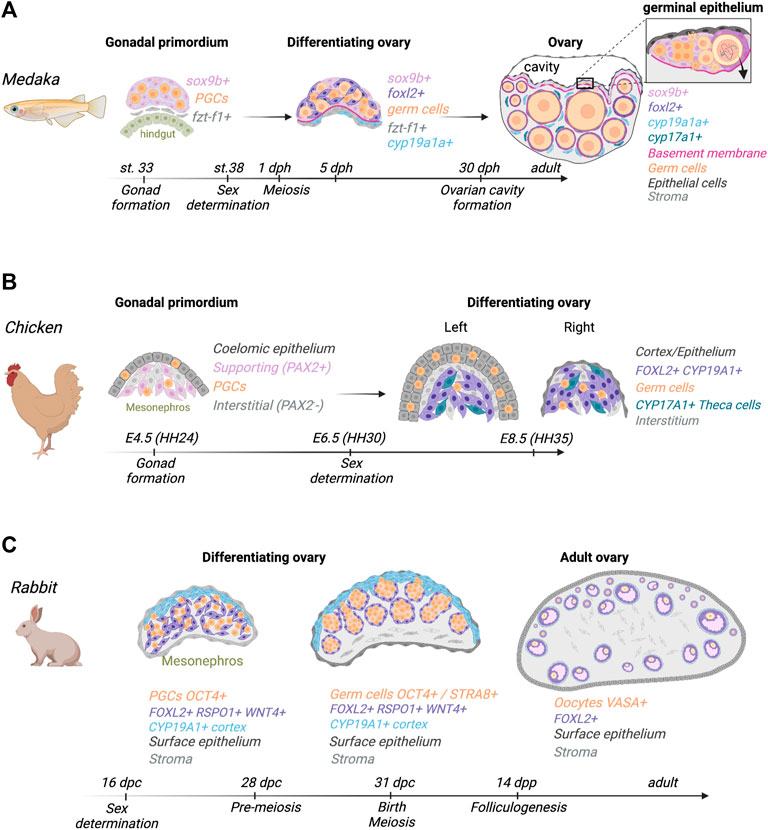
FIGURE 1. Comparative ovarian morphogenesis in medaka, chicken, and rabbit. (A) In medaka, the gonadal primordium is composed of three cell populations: primordial germ cells (PGCs), ftz-f1+ cells, and sox9b+ supporting cell precursors. Sex determination is initiated just before hatching, at stage 38. sox9b+ cells surround PGCs to give rise to the germinal cradles that later form a multilayered germinal epithelium along with epithelial cells. At hatching, a basement membrane is formed at the ventral side. Meiosis is initiated 1-day post-hatching (dph), and foxl2 becomes expressed in supporting cells surrounding diplotene oocytes that exit cradles. Around 5 dph, cyp19a1+ becomes expressed in stroma cells in the ventral region. As follicles grow in the stroma, foxl2+ granulosa cells recruit cyp19a1a+ cells and cyp17a1+ theca cells. Ovarian cavity is formed around 30 dph. Adult ovaries still contain a germinal epithelium (inset) composed of undifferentiated sox9+ supporting cells, and germline stem cells that synchronously divide, enter meiosis and either die or exit the cradle to form follicles in the stroma. (B) In chickens, the gonadal primordium consists of two main compartments, the coelomic epithelium and the underlying mesenchyme. In the left ovary, the coelomic epithelium gives rise to the cortex and interstitial cells. FOXL2+/CYP19A1+ pre-granulosa cells and CYP17A1+ steroidogenic cells derive from a PAX2+ mesenchymal population. Germ cells that migrate into the gonad from the bloodstream accumulate in the cortical region of the left ovary. The right ovary does not form a cortex and germ cells remain in the medulla. (C) In rabbits, ovarian cell differentiation starts at 18 dpc and is associated with an upregulation of FOXL2, WNT4 and RSPO1 in somatic cells. At this stage, proliferating cells in the coelomic epithelium start to express CYP19A1. At 30 dpc, somatic cells expressing FOXL2, WNT4 and RSPO1 surround OCT4/STRA8 positive germ cells and form ovigerous cords. The expression of STRA8 signals meiosis initiation. At 14 dpp, the formation of the first primordial follicles is initiated. Then, growing follicles are composed of FOXL2+ granulosa cells enclosing VASA+ oocytes.
On the other hand, the zebrafish (Danio rerio) has a different strategy of sex determination and gonad differentiation from the medaka. While the zebrafish is widely used as a model organism to study developmental processes, sex determination and gonadal differentiation are still poorly understood and varies between wild (ZZ/ZW system) vs laboratory (lack sex chromosomes) strains (for review, see Kossack and Draper, 2019). Zebrafish presents a juvenile hermaphroditism, as males and females first develop transient juvenile ovaries containing oocytes during larval life (∼13–25 dpf) (Takahashi, 1977). Gonadal development starts with the appearance of an elongated gonadal primordium around 5 dpf (Braat et al., 1999). Around 8–10 dpf, at least two populations of gonadal somatic cells co-exist: an outer layer of epithelial fgf24+ cells that become located to the dorsal edge of the gonad; and an inner layer of fgf24-/etv4+ cells in closer contact with germ cells and whose development appears to rely on the outer fgf24+ cell population (Leerberg et al., 2017). A subset of germ cells become oogonia and initiate meiosis in all gonads (Tong et al., 2010). At 12 dpf, the fgf24- cells start to separate into three distinct sub-populations: etv4+ cells, cyp19+ cells likely representing steroidogenic cell precursors (Dranow et al., 2016) and amh+ cells likely representing supporting cell precursors (Rodríguez-Marí et al., 2005). A few cells expressing gsdf, a marker specific of supporting cell lineage (Gautier et al., 2011; Yan et al., 2017), are detected as early as 8 dpf and appear to originate from the dorsal epithelium to eventually surrounds germ cells (Song et al., 2021). Gonadal differentiation is initiated around 20–25 dpf. The first clear morphological change between sexes is the degeneration of meiotic oocytes in presumptive males (Uchida et al., 2002). During this period (20–25 dpf), expression of the granulosa cell marker wnt4a is maintained in supporting cells of the presumptive ovary whereas it is lost in the presumptive testis (Kossack et al., 2019). On the other hand, the Sertoli-cell marker sox9a is lost in the presumptive ovary but maintained in the presumptive testis (Rodríguez-Marí et al., 2005). cyp19a1a only becomes expressed in granulosa cells later, when granulosa cells surround oocytes that are past mid-Stage II (Dranow et al., 2016). Similar to medaka and other fish species, zebrafish ovary contains a germinal epithelium at the surface of the ovary, composed of germline stem cell (GSC) surrounded by bipotential supporting cells (Beer and Draper, 2013), that contribute to continuous production of follicles throughout life, ovary regeneration and gonad plasticity (Cao et al., 2019).
Avians
In birds, the gonadal primordium first appears in the ventromedial surface of the mesonephros around embryonic day 4.5 (E4.5) (Figure 1B) (Rodemer et al., 1986). Lineage tracing experiments in the chicken embryo evidenced that the coelomic epithelium contributes to the gonadal epithelial and interstitial cells, but not to the supporting cells, as opposed to mammals (Sekido and Lovell-Badge, 2007; Estermann et al., 2020). Instead, the supporting cell population derives from the mesonephric mesoderm, which itself is of intermediate mesodermal origin. More specifically, these supporting cells derive from a PAX2/DMRT1/WNT4/OSR1 positive mesenchymal population (Figure 1B) (Estermann et al., 2020). It is worth noting that both OSR1 and PAX2 are some of the earliest intermediate mesoderm markers (James et al., 2006), supporting the intermediate mesoderm origin of avian supporting cells. Moreover, PAX2-positive progenitor cells were identified in the mesenchymal region of undifferentiated quail (Galloanserae), zebrafinch (Neoaves) and emu (Palaeognathe) gonads (Estermann et al., 2021b). This suggests that the mesenchymal origin of supporting cells is conserved among all bird clades.
Undifferentiated avian gonads exhibit a left/right asymmetry, impacting later ovarian development. This asymmetry is the result of increased proliferation in the left gonadal epithelium, rather than an increase in epithelial apoptosis in the right gonad (Ishimaru et al., 2008). RALDH2, the enzyme responsible for retinoic acid synthesis, is expressed asymmetrically in the right epithelium of the undifferentiated chicken gonads at E5-5.5. Retinoic acid suppresses ERα and NR5A1 expression, resulting in the downregulation of cyclin D1, one of the main proliferation regulators. In the left gonad, the expression of PITX2 inhibits RALDH2 expression, upregulating NR5A1, ERα, cyclin D1 and consequently stimulating cell proliferation (Guioli and Lovell-Badge, 2007; Ishimaru et al., 2008; Rodríguez-León et al., 2008). Ovarian sex determination occurs at E6.5-E7. During ovarian differentiation, the left ovary eventually becomes enlarged and a thick multi-layered cortex forms, surrounding the underlying medulla (Figure 1B) (Guioli and Lovell-Badge, 2007). This becomes morphologically evident at E8.5. Estrogens, synthesized in the ovarian medulla, play a crucial role in the cortical formation through ERα signaling (Lambeth et al., 2013; Guioli et al., 2020). On the contrary, the epithelium of the right ovary does not form a cortex and regresses over time (Figure 1B). Despite lacking a cortex, the right gonad remains as a steroidogenic organ, being able to produce estrogens during embryonic development (Guioli et al., 2020). Most proliferating primordial germ cells (PGCs) in the developing left ovary are located in the cortical region (Figure 1B). Around E10.5, RALDH2 starts expressing in the ovarian left cortex, whereas CYP26B1 expression is restricted to the juxtacortical medulla (Smith et al., 2008a). This results in higher retinoic acid levels in the left cortex. Around E15.5, these germ cells enter meiosis and later arrest at prophase I. In the right gonad, the PGCs undergo some proliferation but do not enter meiosis and later die (Ukeshima, 1996). Development of the functional left ovary is completed after hatching with the formation of follicles in the cortex, harboring the oocytes.
The medullary region of the fetal ovary comprises three main cell populations: the FOXL2+/CYP19A1+ pre-granulosa cells, the steroidogenic theca cells, and the interstitial/stromal cells (Figure 1B) (Estermann et al., 2020). Between the cortex and the medulla of the left ovary, an accumulation of interstitial cells forms a compact structure called the juxtacortical medulla (Estermann et al., 2021a). This structure derives from the gonadal epithelium by EMT. The functional significance of this structure remains unclear, but it is the site of expression of the retinoic acid degrading enzyme CYP26B1 later in development and might be implicated in meiosis (Smith et al., 2008a).
After sex determination, pre-granulosa cells and germ cells are located into two distinctive compartments in the ovary. Germ cells are in the cortical and/or juxtacortical medulla (JCM) region, whereas the pre-granulosa cells are in the medulla. Granulosa and germ cells must associate to form the ovarian follicles. In E14.5 chicken ovaries, FOXL2+/ERα- cells accumulate in the cortical or juxtacortical medulla region of the ovary (Major et al., 2019). The origin of these cells is currently unknown. One of the possibilities is that the medullary pre-granulosa cells migrate into the JCM/cortical region, guided by epithelial or oocyte-secreted factors. On the other hand, these FOXL2+ cells could derive from cortical cells through EMT, downregulate ERα and upregulate FOXL2 along the process. If that is the case, the medullary pre-granulosa cells could function as a source of steroids due to the presence of androgenic theca cells and estrogenic pre-granulosa cells. It is unclear if CYP19A1 is expressed in these cortical/juxtacortical FOXL2+ cells.
Mammals
Mouse
The development of the mouse ovary starts during the first half of fetal development at E9.5-11. Similar to other mammal species, bipotential gonad emerges from the thickening of the coelomic epithelium of the intermediate mesoderm (Moritz and Wintour, 1999; Bunce et al., 2021). The bipotential gonad is composed of primordial germ cells and somatic precursor cells, including supporting cells and interstitial cells that can follow either a testis or an ovary fate (Stevant et al., 2019). In the XX gonad, supporting cells are responsible for the initiation of ovarian determination by differentiating into pre-granulosa cells. Pre-granulosa cells arise from two timely waves of differentiation (Mork et al., 2012; Zheng et al., 2014; Niu and Spradling, 2020). The first wave of pre-granulosa cells arises from the Runx1+ bipotential supporting cells. As sex determination is initiated, Runx1 expression is maintained in XX gonads whereas repressed in XY gonads (Nicol et al., 2019; Stevant et al., 2019). These Runx1+ supporting cells start expressing Foxl2 around E12 and give rise to medullary pre-granulosa cells. Starting around E12.5, a second wave of pre-granulosa cell population arises from Lgr5+ cells of the ovarian surface epithelium that ingress into the ovary (Gustin et al., 2016; Niu and Spradling, 2020; Fukuda et al., 2021). These cells give rise to cortical pre-granulosa cells, which eventually lose Lgr5 expression and upregulate Foxl2 shortly after birth (Rastetter et al., 2014; Feng et al., 2016; Stevant et al., 2019; Cai et al., 2020; Niu and Spradling, 2020). The medullary granulosa cells are responsible for the first wave of folliculogenesis after puberty, whereas the cortical granulosa cells are involved in the second wave of folliculogenesis during the adult life (Mork et al., 2012; Zheng et al., 2014; Niu and Spradling, 2020). Primordial germ cells (PGCs) reach the gonad between E10 and 11.5 days, when sex determination of the somatic cells is initiated. The commitment of PGCs into male or female gametes depends on their somatic environment. The female germ cells proliferate until they initiate meiosis. Meiosis begins at E12.5 in a few cysts in the antero-medial region and radiates outward (Soygur et al., 2021), followed by an anterior to posterior initiation wave at E13.5 (Menke et al., 2003). At this stage, female PGCs are surrounded by a layer of pre-granulosa cells to form ovigerous cords. Around birth, granulosa cells break down the ovigerous cords by enclosing single oocytes, leading to the formation of primordial follicles. Finally, theca cells are recruited postnatally from both the ovarian stroma and the mesonephros to surround developing follicles (Liu et al., 2015).
Rabbit
In rabbits, the gestation lasts 31 days and sex determination happens at 16 dpc. The bipotential gonads appear around 14 dpc (Mario et al., 2018) (Figure 1C). The first event of somatic cell differentiation starts at 16 dpc (Mario et al., 2018) and is associated with a regression of the mesonephros between 16 dpc to 25 dpc (Bernier and Beaumont, 1964). Ovarian commitment is initiated at 18 dpc with the expression of FOXL2, RSPO1 and WNT4 (Daniel-Carlier et al., 2013). At 23 dpc, the gonadal and mesonephric tissues are separated by connective tissue that is supposed to prevent the migration of cells and other substances (Hayashi et al., 2000). At 30 dpc, the ovigerous cords and the surrounding stromal tissue are compactly arranged in the ovarian cortex, whereas in the medullary region, the stromal tissue is loosely arranged, and the ovigerous cords are easily discerned and maintain continuity with the surface epithelium (Diaz-Hernandez et al., 2019). In parallel, the germ cells express the pre-meiotic marker STRA8 and enter a pre-meiotic phase from 24 to 28 dpc. Then, meiosis occurs asynchronously during the 15 days following birth and germ cells remain arrested in prophase I of meiosis. At this stage, the rupture of the ovigerous nests followed by the formation of the first primordial follicles is initiated at the interface between the cortex and medulla of the ovary.
Ruminants
In farm animal species, ovaries can be identified at 20–23 dpc in sheep, 25 dpc in goat and 32 dpc in cow (Mauleon, 1976; McNatty et al., 1995; Pailhoux et al., 2002). Before sex determination, cells from the mesonephros migrate and populate the gonadal primordium (Zamboni et al., 1979; Pailhoux et al., 2002; Kenngott et al., 2013). In cows, cells from the surface epithelium, named gonadal ridge epithelial-like cells (GREL) give rise to pre-granulosa cells (Hummitzsch et al., 2013). A similar cell population is suspected to exist in the sheep ovary (Juengel et al., 2002). Primordial germ cells are observed at the genital ridge at 25 dpc in goat (Pailhoux et al., 2002) from 21 dpc in sheep (Ledda et al., 2010) and 31 dpc in cow (Wrobel and Suss, 1998). Ovarian morphological differentiation and cortical development is first apparent at 29 dpc in sheep, 34–36 dpc in goat and 42 dpc in cow. Proliferating germ cells and pre-granulosa form cord-like structures named ovigerous nests (Juengel et al., 2002; Pannetier et al., 2012; Hummitzsch et al., 2013). Around 55 dpc in goat and sheep and 75 dpc in cow, female germ cells enter meiosis while mesonephric-derived somatic cells colonize the genital ridge. Contrary to mice, primordial follicles form during gestation, around 75 dpc in sheep, 90 dpc in goat and 130 dpc in cow (Erickson, 1966; Pailhoux et al., 2002; Sawyer et al., 2002).
Human
The human gonadal primordium first becomes discernible around 4 weeks post-conception (wpc), and PGCs reach the genital ridges between 4 and 6 wpc. The gonadal primordium is composed of a proliferating coelomic epithelium and an underlying compartment containing mesenchymal cells, blood vessels and mesonephric cells (Byskov, 1986). Sex determination is initiated just before 6 wpc, when XY gonads start expressing SRY in the supporting cell precursors (Mamsen et al., 2017). In the ovary, the cortex further develops, and poorly defined ovarian cords start to form around 8 wpc with connections to the ovarian surface epithelium. These cords are composed of germ cell clusters surrounded by a single layer of flattened supporting cells. Human granulosa cells are suspected to arise, at least in part, from the surface epithelium, similar to findings from the mouse, cow, and sheep. Indeed, electron microscopy revealed that the extension of human ovarian cords correlates with ingrowths of proliferating surface epithelium (Motta and Makabe, 1982). Around 11 wpc, some germ cells initiate meiosis (Gondos et al., 1986). Following meiosis entry, waves of germ cell apoptosis occur throughout the rest of gestation. Germ cell cyst breakdown starts at mid-gestation, around 20 wpc in the human ovary.
Evolutionary perspectives
From fish to human, ovaries share the same purpose of producing oocytes and reproductive hormones. However, some differences during their morphogenesis leads to species-specific properties. A major difference during vertebrate evolution is the retention of germline stem cell (GSC) surrounded by bipotential supporting cells in fish. This germinal epithelium has the capacity to produce unlimited oocytes throughout life and to regenerate the ovary. It is also likely responsible for gonadal plasticity leading to fully functional sex reversal in adult fish upon various genetic or environmental cues. Birds and mammals have lost this capacity to regenerate the ovary. Instead of a germinal epithelium, they form an ovarian cortex containing the stock of follicles that will be used later in life.
Ovarian morphogenesis is conserved among mammals, with the formation of ovigerous cords that eventually break down to form follicles. A key difference among mammals is the timing of meiosis initiation and follicle formation. Meiosis is only initiated after birth in rabbit, contrary to mice and other mammals. Similarly, follicle formation happens postnatally in mouse and rabbit and during gestation in larger mammals. The evolutionary and functional meaning of these differences may be related to the extension of gestation period in larger mammals.
In all clades, ovaries are composed of three main cell populations: germ cells, supporting cells and mesenchymal/steroidogenic cells. It has become clear that in mammals, granulosa cells arise at least in part from the ovarian surface epithelium. On the other hand, in chicken, granulosa cells derive from mesonephric mesoderm. Lineage tracing experiments helped tackling the question of origins of somatic cell populations. Unfortunately, it remains difficult to compare these findings between species as genes used for cell lineage tracing, such as fgf24 or gsdf, were lost during vertebrate evolution (Jovelin et al., 2010; Hsu and Chung, 2021). With more scRNA-seq datasets becoming available for differentiating ovaries from various species, it would provide the opportunity to not only compare the cell populations identified, but an evolutionary analysis of gonadal cell lineages. Such comparisons will help decipher convergent and divergent origins of ovarian cell populations among vertebrates.
Finally, most birds present an intriguing feature during ovarian morphogenesis: they only keep one functional ovary. In chickens, despite developing both left and right gonads during early embryogenesis, only the left one remains as a fully functional ovary whereas the right ovary regresses. It is still unknown what the evolutionary mechanism is for developing one main ovary. Fossilized remains of birds from the early Cretaceous period suggest this trait was acquired early in birds’ evolution (Zheng et al., 2013).
Role of estrogens in ovarian differentiation
The role of estrogens in ovarian differentiation has been studied for many decades in various vertebrate species. Estrogens represent the main hormones produced by the ovary. They are produced through the conversion of androgens by the enzyme CYP19A1, also known as aromatase. By binding to their receptors (ERα and β), estrogens regulate gene expression and thus ovarian differentiation and/or development. The use of aromatase inhibitors, exogenous estrogens and transgenic models were key to elucidate the role of estrogens in ovarian differentiation in a wide range of organisms.
Fish
In many fish species, manipulations of estrogen signaling at specific windows of development lead to fully functional sex-reversal (for review, see Guiguen et al., 2010; Li et al., 2019) (Figure 2A). With the teleost-specific whole genome duplication (Meyer and Van de Peer, 2005), cyp19a1 is present in two sub-functionalized copies: cyp19a1a is specifically expressed in the ovary, while cyp19a1b is expressed in the brain and occasionally in the ovary (Chang et al., 2005). cyp19a1a is expressed before histological differentiation of the gonads in various fish species (Vizziano et al., 2007; Dranow et al., 2016). Teleosts have at least three estrogen receptors (esr1, esr2a and esr2b) that are expressed in both male and female developing gonads, explaining their sensitivity to the feminizing effects of estrogens.
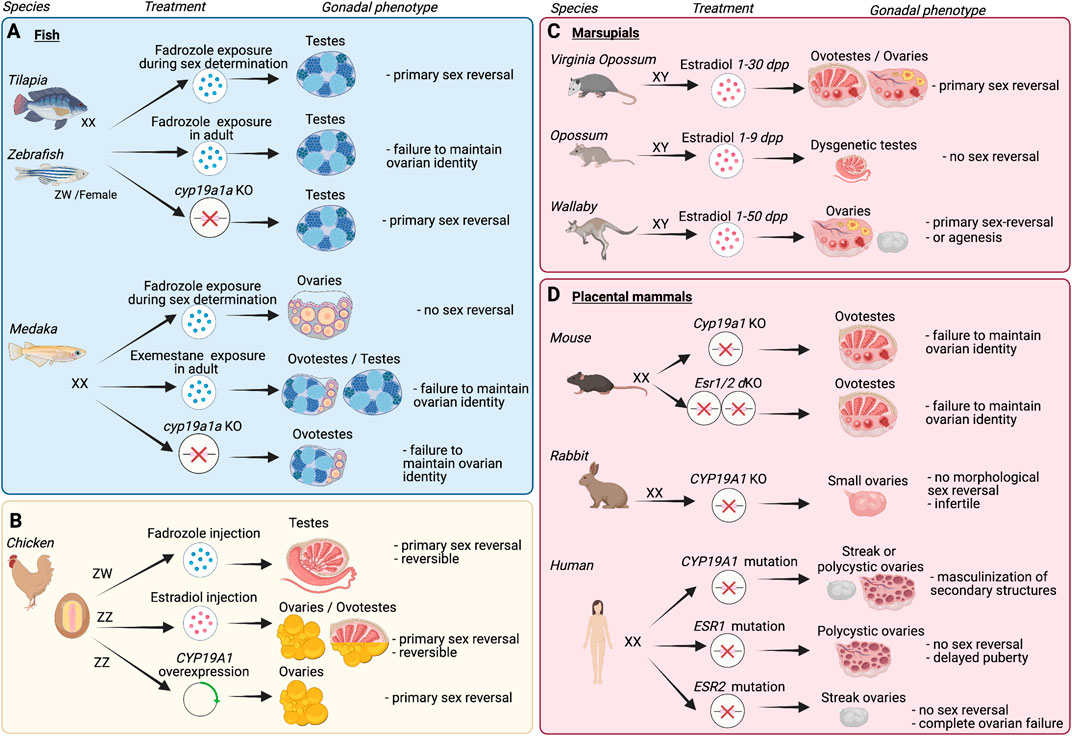
FIGURE 2. Effects of manipulating estrogen signaling on the gonadal fate in fish, chickens, and mammals. (A) In fish: In female tilapia and zebrafish, both exposure to aromatase inhibitor fadrozole during sex determination and knockout of cyp19a1a cause primary ovary-to-testis sex reversal, while long-term fadrozole treatment of mature females leads to ovary-to-testis sex reversal. Therefore, cyp19a1a is involved in both primary ovarian differentiation and maintenance of ovarian identity. In medaka, exposure to fadrozole during sex determination has no effect on ovarian differentiation, while both cyp19a1a KO and treatment of adult females with aromatase inhibitor exemestane eventually masculinize the ovaries. This indicates that cyp19a1a is only involved in the maintenance of medaka ovarian identity. (B) In chickens: treatment with aromatase inhibitor fadrozole in ZW female embryos results in transient testis development. On the other hand, estradiol injection to ZZ male embryos results in ovary or ovotestis differentiation. Moreover, ovarian development is induced in male embryos overexpressing CYP19A1. (C,D) In mammals: (C) In marsupials. Effects of estradiol treatment on XY males varies from species to species. Newborn XY Virginia opossum exposed to estradiol for 30 days post-partum (dpp) develops ovaries or ovotestes, whereas newborn XY gray short-tailed opossum exposed for 9 dpp develops dysgenetic testes. Newborn XY wallaby exposed for 50 dpp develops ovaries or gonadal agenesis if born prematurely. (D) In placental mammals: In the mouse, Cyp19a1 knockout or Esr1/Esr2 double knockout leads to postnatal transdifferentiation of granulosa cells into Sertoli-like cells. This indicates a role in the maintenance of ovarian identity rather than in primary ovarian differentiation. In the rabbit, CYP19A1 knockout does not impair primary ovarian differentiation but prevents the proper development of ovaries, resulting in small ovaries and infertility. In humans, mutations in CYP19A1 or ESR1 or ESR2 genes do not impact primary ovarian differentiation but cause either streak ovaries or polycystic ovaries.
In tilapia, treatment of XX larvae with the non-steroidal aromatase inhibitor fadrozole during sex determination causes masculinization of the gonads (Kwon et al., 2000). Meanwhile, long-term treatment of adult females with fadrozole transforms the ovaries into functional testes (Paul-Prasanth et al., 2013; Sun et al., 2014). Furthermore, cyp19a1a knockout causes primary ovary-to-testis sex reversal, with upregulation of Sertoli gene dmrt1 at the time of sex determination (Zhang et al., 2017). The transcription factor Dmrt1 binds cyp19a1a promoter and represses its expression (Wang et al., 2010). These observations support the role of cyp19a1a and estrogens in both primary ovarian determination and maintenance of ovarian identity in adulthood. Loss of either of the three estrogen receptors does not lead to masculinization, suggesting some functional redundancy between estrogen receptors (Yan et al., 2019).
In zebrafish, exposure to fadrozole during sex determination causes testis differentiation (Fenske and Segner, 2004). Loss of cyp19a1a also leads to testis development (Lau et al., 2016; Yin et al., 2017). This sex-reversal phenotype in the cyp19a1a knockout model is rescued by additional loss of dmrt1, suggesting that estrogen signaling controls ovarian differentiation by repressing dmrt1 in zebrafish (Wu et al., 2020). Long-term treatment of mature females with fadrozole results in ovary-to-testis sex-reversal, indicating that cyp19a1a is also required for the maintenance of ovarian identity (Takatsu et al., 2013). Similar to tilapia, none of the three estrogen receptors single KO results in ovary-to-testis sex-reversal (Lu et al., 2017). Nonetheless, in the absence of all three receptors, ovaries eventually transdifferentiate into ovo-testes or testes. While the triple estrogen receptor KO leads to an all-male phenotype, it does not fully recapitulate the cyp19a1 KO phenotype, which prevents primary ovarian differentiation. It remains unclear how estrogens drive early ovarian differentiation independently of estrogen receptors in zebrafish.
In medaka, brief exposure to estrogens just 1 day post-fertilization causes functional testis-to-ovary sex reversal, similar to other fish species (Kobayashi and Iwamatsu, 2005). Yet, intrinsic estrogen production is not critical for primary ovarian differentiation. Indeed, cyp19a1a expression only appears in the ovarian interstitium several days after sex determination is initiated (Suzuki et al., 2004). Exposure to fadrozole during gonad differentiation after hatching does not affect ovarian development (Suzuki et al., 2004). Meanwhile, long-term exposure to the aromatase inhibitor exemestane in adult females causes functional ovary-to-testis sex reversal (Paul-Prasanth et al., 2013). Loss of cyp19a1 in medaka does not impair initial ovarian differentiation, but later leads to ovary degeneration and partial ovary-to-testis sex reversal after puberty (Nakamoto et al., 2018). Therefore, in medaka, endogenous estrogens are only necessary for the maintenance of ovarian identity. Similar to tilapia and zebrafish, none of the three estrogen receptors single KO cause ovary-to-testis sex reversal (Kayo et al., 2019), but the effects of combined loss remain to be determined.
Avians
In avian species like chicken, endogenous estrogens have a central role in ovarian development (Smith et al., 1997; Brunström et al., 2009). Unlike mammals, the estrogen-synthesizing enzyme CYP19A1 is expressed in female gonads at the onset of ovarian differentiation (Figure 1B) (Elbrecht and Smith, 1992; Andrews et al., 1997; Nomura et al., 1999). This results in elevated ovarian and systemic estrogen levels in females from the time of sex determination (George and Wilson, 1982; Tanabe et al., 1986; Elbrecht and Smith, 1992). CYP19A1 is detected in pre-granulosa cells and in a subset of embryonic theca cells (Estermann et al., 2020). Steroidogenic theca cells are responsible for the synthesis of androgens, which then are converted into estrogens by CYP19A1. Chicken embryonic ovaries contain more CYP17A1+ steroidogenic cells than testes (Estermann et al., 2020). This likely reflects the higher demand of androgens in the ovary to be converted into estrogens. In chickens, embryonic steroidogenic theca cells were found to derive from the female supporting cell lineage. A subset of FOXL2+/CYP19A1+ pre-granulosa cells in the medulla upregulates the androgen-producing enzyme CYP17A1 and become transitioning/intermediate cells, expressing both theca and granulosa cell markers (Estermann et al., 2020). Then, pre-granulosa cell markers are downregulated whereas other steroidogenic markers are upregulated, completing the differentiation towards theca cells (Estermann et al., 2020). It is still unknown if these embryonic theca cells become the adult theca cells, or if they have additional origins as in mice (Liu et al., 2015).
Estrogen is required for ovarian cortex formation and pre-granulosa differentiation. In the left chicken ovary, the epithelium becomes multilayered, forming the ovarian cortex that harbors meiotic germ cells. The chromosomal composition (ZZ or ZW) does not contribute to cortex formation (Guioli et al., 2020). Instead, estrogens, synthesized locally by CYP19A1 in the medulla, are required to induce cortex formation through ERα signaling. When ERα is knocked down, the cortex fails to form in the ovary (Elbrecht and Smith, 1992; Guioli et al., 2020).
Treatment with aromatase inhibitors fadrozole or letrozole in ZW chicken embryos inhibits estrogen synthesis, upregulate DMRT1, resulting in ovary-to-testis sex reversal at E6.5 (Figure 2B) (Smith et al., 2003; Trukhina et al., 2014). This sex reversal is not permanent as ZW embryos treated with fadrozole at E3.5 eventually upregulate CYP19A1 and revert to ovotestis, (Figure 2B) (Nishikimi et al., 2000; Vaillant et al., 2001; Estermann et al., 2021a). Conversely, exposure of genetically male (ZZ) chicken embryos to exogenous 17β-estradiol results in ovarian development, that reverses back to testis upon estradiol decay (Figure 2B) (Bannister et al., 2011; Guioli et al., 2020; Shioda et al., 2021). Moreover, constitutive CYP19A1 over-expression in ZZ embryos leads to complete ovary formation (Figure 2B) (Lambeth et al., 2016). Altogether, these experiments implicate the formative role of estrogen in inducing the program for ovary formation.
Mammals
Marsupials
Marsupials constitute a clade of non-placental mammalians that includes opossums, wallabies, kangaroos, koalas, wombats, Tasmanian devils, and bandicoots. Marsupial embryonic development is characterized by a premature birth. These immature newborns crawl up into their mothers’ pouch (marsupium), attach themselves to a teat, and continue their development (Mahadevaiah et al., 2020; Smith and Keyte, 2020). Gonadal sex determination in marsupials occurs after birth, while they are growing in the pouch (Renfree and Short, 1988; Baker et al., 1993; Renfree et al., 1996). This external development makes them a remarkable model to study embryonic gonadal differentiation, compared to the in-utero development of eutherian mammals.
Although estrogens are not synthesized in early embryonic ovaries, marsupial sex determination is sensitive to exogenous estrogens (George et al., 1985; Renfree et al., 1992) (Figure 2C). This could be attributed to the expression of both estrogen receptors α and β in undifferentiated gonads and in differentiated supporting and germ cells (Calatayud et al., 2010). In vitro and in vivo analysis in different marsupial species demonstrate that estrogens have a role in inhibiting testicular development or inducing ovarian development. In the Virginia opossum (Didelphis virginiana), exposure of XY embryos to estradiol dipropionate for 30 days post-partum (or dpp) leads to formation ovotestes or a complete testis-to-ovary sex reversal (Figure 2C) (Burns, 1955). In the gray short-tailed opossum (Monodelphis domestica), estradiol benzoate treatment to XY embryos from days 1–9 post-partum result in dysgenetic testes, but not testis-to-ovary sex reversal (Figure 2C) (Fadem and Tesoriero, 1986). In the tammar wallaby (Macropus eugenii), estradiol benzoate treatment to XY embryos at birth for 25 days result in ovotestis development at day 25 post-partum (Shaw et al., 1988), followed by complete testis-to-ovary sex reversal at 50 dpp (Coveney et al., 2001) (Figure 2C). In prematurely born XY embryos, estradiol benzoate treatment caused gonadal agenesis. In vitro culture of tammar wallaby gonads was used to study the molecular mechanisms responsible of estrogen mediated gonadal sex reversal (Calatayud et al., 2010; Pask et al., 2010). In cultured XY gonads, estrogens reduce SRY and AMH expression and induce FOXL2 and WNT4 expression. While SOX9 expression was not downregulated, estrogen treatment inhibits SOX9 translocation into the nucleus, consequently preventing activation of the testis differentiation pathway (Pask et al., 2010).
Mouse
In the mouse, neither estrogen receptors (Esr1/2) nor Cyp19a1 are expressed in the fetal ovary during sex determination at E10-11.5. ERα (encoded by Esr1) and ERβ (encoded by Esr2) become detected in the fetal ovary later on, around E14.5-E15.5 (Lemmen et al., 1999; Chen et al., 2009). In the neonatal ovary, ERα is expressed in somatic cells and ERβ is detected in oocytes (Chen et al., 2009). In the adult ovary, ERα is mainly expressed in theca cells and ERβ in granulosa cells (Jefferson et al., 2000). There are some discrepancies regarding whether Cyp19a1 is expressed in the fetal ovary after sex determination. Some studies show that Cyp19a1 expression is only detected close to birth (Greco and Payne, 1994) whereas others detect CYP19A1 protein as early as E13.5 (Dutta et al., 2014). Inactivation of Cyp19a1 does not impact early ovarian differentiation but the females are infertile (Fisher et al., 1998) (Figure 2D). Further analyses revealed that Cyp19a1 KO mouse ovaries present some testis-like structures with Sertoli-like cells after postnatal follicle formation, and this masculinization could be reversed by exposure to estrogens (Britt et al., 2001; Britt et al., 2002; Britt et al., 2004). Similarly, while single loss of Esr1 or Esr2 does not impact ovarian identity, their combined loss causes postnatal transdifferentiation of granulosa cells into SOX9+ Sertoli-like cells (Couse et al., 1999). Estrogen receptors cooperate with FOXL2 to maintain the identity of ovarian granulosa cells through repression of SOX9 (Uhlenhaut et al., 2009; Georges et al., 2014). In addition, FOXL2 ChIP-seq revealed that Esr2 is a direct target of FOXL2 in adult granulosa cells, and that FOXL2 positively regulates Esr2 (Georges et al., 2014). Altogether, these results indicate that estrogen signaling is not necessary for ovarian determination but is required for the maintenance of granulosa cell identity in mice.
Rabbit
In rabbits, CYP19A1 expression in the ovary and the capacity of fetal and neonatal granulosa cells to synthesize steroid hormones has been well described (Gondos, 1969; Erickson et al., 1974; George and Wilson, 1980; Gondos et al., 1983). CYP19A1 expression starts at 16 dpc and reaches its peak at 20 dpc. At this stage, CYP19A1, ESR1 and FOXL2 are located in distinct cell populations. CYP19A1 and ESR1 are expressed in the coelomic epithelium and contribute to cortex development whereas FOXL2 is expressed in the supporting cells located in the medulla (Jolivet et al., 2022). Concomitantly, intracytoplasmic lipid droplets, which provide the main source of cholesterol for steroid synthesis, accumulate in granulosa cells at 19 dpc (Gondos et al., 1983). Based on these observations, it has been hypothesized that this estrogen surge plays a role during rabbit ovary differentiation. However, loss of CYP19A1 in the XX rabbit embryo has no impact on fetal ovary formation (Figure 2D), although ovaries are smaller and eventually contain a reduced follicular reserve (Jolivet et al., 2022). In the CYP19A1 KO adult ovary, DMRT1 expression is upregulated in some granulosa cells while SOX9 expression is absent, and no structural masculinization of the ovary is observed. Estrogens appear to be dispensable for ovary formation in XX rabbit, but they play an important role in the establishment of the ovarian reserve by maintaining granulosa cell and germ cell proliferation.
Human
Transcriptomic analyses of human gonads during sex determination showed that both ESR1 and ESR2 are expressed in the gonads (Mamsen et al., 2017; Lecluze et al., 2020). There are however discrepancies whether their expression is stronger in the ovary than in the testis (Lecluze et al., 2020) or not different between sexes (Mamsen et al., 2017). During the second trimester, CYP19A1 protein is sporadically detected in the fetal ovary at 12 weeks of gestation and becomes strongly expressed by 19 weeks. CYP19A1 is detected in somatic cells surrounding oocyte nests and in granulosa cells of primordial follicles in the fetal ovary (Fowler et al., 2011). Patients with mutations in CYP19A1 gene do not develop ovary-to-testis sex reversal (Figure 2D), but present ambiguous external genitalia at birth (Shozu et al., 1991; Mazen et al., 2017; Hathi et al., 2022). These patients tend to have either streak or polycystic ovaries (Mazen et al., 2017; Praveen et al., 2020). ESR1 or ESR2 mutations do not cause ovary-to-testis sex reversal or ambiguous external genitalia at birth, but ovaries present a polycystic or streak phenotype respectively (Quaynor et al., 2013; Bernard et al., 2017; Lang-Muritano et al., 2018). Such difference in ovarian phenotype suggests a more prominent role for ESR2 than for ESR1 during early ovarian development. Overall, these studies indicate that endogenous estrogens and their actions are not required for ovarian determination in humans, but mis-regulation of estrogen signaling has dramatic effects on ovarian development/function and physiology in adulthood.
Evolutionary perspectives
From fish to human, estrogen signaling appears to be less and less involved in primary ovarian determination but remains critical for proper ovarian development and functions. In eutherian mammals, sex determination became de-coupled from the feminizing effects of estrogens as found in fish, birds, and other vertebrate species. This could be an evolutionary response to the maternal estrogens that pass through the placenta. In marsupials, gonadal sex determination occurs after birth, not being influenced by the maternal estrogens. Despite this, they are susceptible to external estrogens, a potential link to a more ancestral state that was lost in eutherian mammal evolution.
Estrogen receptors are expressed in ovarian embryonic somatic cells of mice, humans, goats, sheep, and marsupials, indicating that regulation of some elements of the estrogen signaling machinery remain conserved throughout vertebrate ovary differentiation. One key difference arises from the timing and location of CYP19A1 expression. In fish and chicken, CYP19A1 is not exclusively expressed in granulosa cells, but is detected in some stromal steroidogenic cells during early ovarian differentiation. These non-granulosa CYP19A1+ cells are not found in mammalian embryonic ovaries. This raises the question whether this unique population is responsible for estrogens action in ovarian determination, and the loss of this cell population contributed to the decoupling of estrogen involvement in ovarian determination in mammals. In both chicken and rabbit, estrogen signaling is required for cortex development in the developing ovary, contributing to cell proliferation and expansion of the cortex. It would be interesting to determine if this function is also conserved in other vertebrate species.
Altogether, these findings provide a potential explanation for the major shift in vertebrate sex determination mechanisms from an estrogen dependence on ovarian differentiation to a strictly genetic regulation.
Genes and pathways controlling granulosa cell differentiation and ovarian identity
During sex differentiation, the gonad develops into an ovary or a testis, depending on the signaling pathways activated or repressed in the supporting cells. While the master sex determining gene varies among vertebrate species, the genes or pathways involved in pre-granulosa and ovarian differentiation are relatively conserved. FOXL2 is one of the most conserved pro-ovarian genes in vertebrates and beyond. Independent of FOXL2, the WNT4/β-catenin pathway is also important for ovarian development in vertebrate species. Genome editing technologies such as CRISPR/Cas9 enabled the generation of knockout models in more and more non-model vertebrate species. Comparison of knockout models for these main pathways in various species helps determining the evolution of the role of these pathways during vertebrate ovarian differentiation. In addition, in recent years, omics technologies led to the identification of novel candidate genes involved in ovarian differentiation. The following sections compare and contrast the genes and pathways that control granulosa cell differentiation and ovarian identity.
Fish
Compared to tetrapods, teleosts generally have two copies of each gene due to the teleost-specific whole genome duplication (Meyer and Van de Peer, 2005). This potentially results in sub-functionalization or neo-functionalization of genes and impacts genes associated with ovarian differentiation.
foxl2 gonadal expression has been analyzed in more than two dozen fish species, revealing a higher expression in the embryonic ovaries vs testes in almost all species studied (for review, see Bertho et al., 2016). In medaka (Figure 3A), foxl2 expression is initiated after ovarian differentiation has started (Nakamoto et al., 2006). foxl2 functions in medaka ovarian differentiation remain to be determined. Foxl2 protein is detected in the nuclei of granulosa cells, as well as in a subpopulation of Cyp19a1a+ stromal/theca cells (Nakamoto et al., 2006; Herpin et al., 2013). This expression of foxl2 in some steroidogenic cells has been observed in other fish species such as tilapia (Wang et al., 2007) and gibel carp (Gan et al., 2021). In tilapia (Figure 3B), loss of foxl2 causes complete ovary-to-testis sex-reversal (Li et al., 2013; Zhang et al., 2017). The role of the transcription factor Foxl2 in fish ovarian differentiation seems to rely on its capacity to directly induce cyp19a1a expression (Wang et al., 2007; Yamaguchi et al., 2007; Bertho et al., 2018; Gan et al., 2021; Yan et al., 2021), and/or to repress the pro-Sertoli gene dmrt1 (Fan et al., 2019). Loss of foxl2 in tilapia results in absence of cyp19a1 expression and upregulation of dmrt1 at time of sex determination (Zhang et al., 2017). In zebrafish (Figure 3C), foxl2a and foxl2b are both expressed in granulosa cells, but they present some sub-functionalization in the ovary (Yang et al., 2017). Single loss of foxl2a or foxl2b does not impair initial ovarian differentiation, but respectively leads to premature ovarian failure or partial sex-reversal in adult females. Their combined loss causes upregulation of Sertoli genes dmrt1 and sox9a and complete ovary-to-testis sex reversal weeks after gonad differentiation. This phenotype suggests a cooperative role of these two sub-functionalized foxl2 variants in maintaining ovarian identity in zebrafish, with a predominant role by foxl2b.
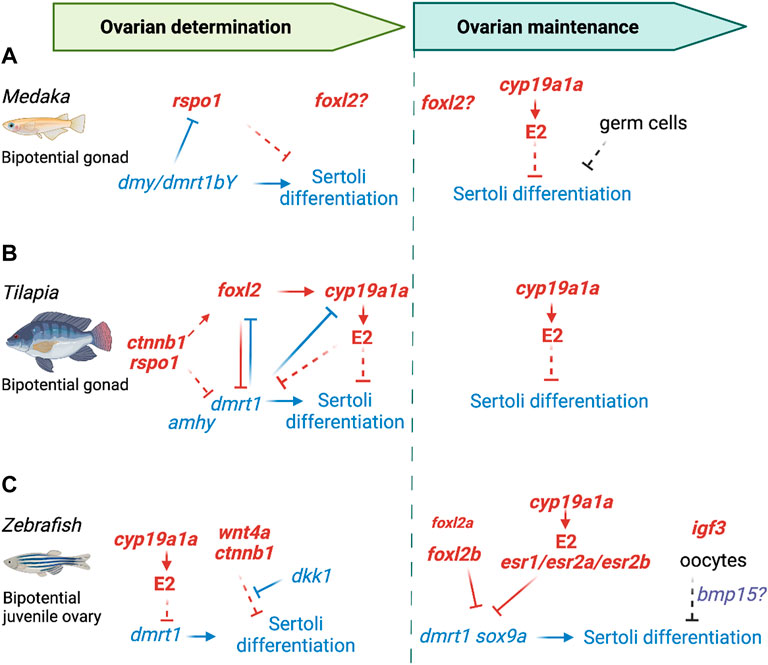
FIGURE 3. Genetic regulation of pre-granulosa cell differentiation in fish. (A) In medaka, in the absence of the Y-linked sex determining gene dmy, pro-ovarian genes (rspo1 and later foxl2) are upregulated. rspo1, which is directly repressed by Dmy, is sufficient to fully drive ovarian differentiation in XY gonads. The functional role of foxl2 remains to be determined. cyp19a1 is only involved in the maintenance of ovarian differentiation. Loss of germ cells prevents the maintenance of granulosa cell fate. (B) In tilapia, in the absence of the Y-linked sex determining gene amhy, pro-ovarian genes are quickly upregulated. Repression of Wnt/β-Catenin pathway through either rspo1 KO or exposure to inhibitors leads to repression of foxl2 and upregulation of dmrt1, causing Sertoli cell differentiation. Loss of foxl2 results in absence of cyp19a1 expression and upregulation of dmrt1 at time of sex determination. There is mutual antagonism between pro-testis gene dmrt1 and pro-ovary genes foxl2/cyp19a1. cyp19a1 is also involved in the maintenance of ovarian identity. (C) In zebrafish, all larvae first develop a bipotential juvenile ovary. Loss of cyp19a1a leads to upregulation of dmrt1 and Sertoli cell differentiation. Repression of the Wnt/β-Catenin pathway, through either wnt4a KO or overexpression of inhibitor Dkk1 causes Sertoli cell differentiation. Combined loss of either foxl2a/foxl2b, or the three estrogen receptors impairs the maintenance of granulosa cell identity, resulting in complete ovary-to-testis sex reversal. Oocytes are required for the maintenance of granulosa cell fate. Loss of genes involved in PGC/oocyte development, such as igf3, results in ovary-to-testis sex-reversal. Bmp15 is suspected to be the oocyte secreted factor that directly acts on supporting cells.
The role of the Rspo1/Wnt/β-catenin pathway in ovarian differentiation has been investigated in various fish species. R-spondin 1 (Rspo1) is a secreted factor that potentiates the canonical Wnt/β-catenin pathway. Expression of rspo1 is higher in the differentiating ovary than in the testis in several fish species (Zhang et al., 2011; Zhou et al., 2012; Liu et al., 2018). In medaka, rspo1 is detected at the onset of sex determination, around hatching, earlier than foxl2. At 10 dph, rspo1 is detected in ovarian germ cells and surrounding somatic cells (Zhou et al., 2012). Ectopic expression of rspo1 in XY medaka causes complete testis-to-ovary sex reversal and the development of fertile females (Zhou et al., 2016). Medaka Y-linked sex determining factor Dmy/Dmrt1bY, and its homolog Dmrt1a, both bind to rspo1 promoter and repress its expression in vitro (Zhou et al., 2016). In the Nile tilapia (Oreochromis niloticus), a gonochoristic species with a XX/XY sex determination system (Li et al., 2015), rspo1 is expressed in germ cells in both sexes and becomes more expressed in the ovary before meiosis initiation. Reduction of rspo1 expression causes both defects in ovarian and testis development in tilapia (Wu et al., 2016). Beside rspo1, other members of the Wnt/β-catenin pathway are more expressed in the differentiating ovary. In medaka, both wnt4b and ctnnb1 present a sexually dimorphic expression (Zhou et al., 2016). In zebrafish, wnt4a becomes specifically expressed in somatic cells surrounding larger oocytes. Both wnt4a KO (Kossack et al., 2019) and Wnt antagonist dkk1 overexpression cause testis development (Sreenivasan et al., 2014), confirming a key role of the Wnt/β-catenin pathway in ovarian differentiation in zebrafish. Different chemical inhibitors of the Wnt/β-catenin pathway have been used in other fish species, resulting in downregulation of pro-ovarian genes such as cyp19a1a or foxl2 in tongue sole (Zhu et al., 2017) and carp (Wu et al., 2019) or upregulation of pro-testis gene dmrt1 in tilapia (Wu et al., 2016). This suggests a conservation of the Wnt/β-catenin pathway functions in ovarian differentiation in teleosts, although each actor of the pathway may not play the same part, depending on the species. This also raises the question whether other Wnt ligands could be involved in ovarian differentiation.
Granulosa cell differentiation or the maintenance of their identity is also influenced by signals from the germ cells in fish. Beyond their capacity to intrinsically determine their female fate through foxl2l (formerly foxl3) (Nishimura et al., 2015; Dai et al., 2021), fish germ cells have a feminizing effect on the surrounding somatic environment. While loss of germ cells has no impact on ovarian differentiation in mice (Maatouk et al., 2012), absence of germ cells causes gonad masculinization in medaka (Kurokawa et al., 2007; Tanaka et al., 2008; Nishimura et al., 2018), zebrafish (Slanchev and Stebler, 2005; Siegfried and Nüsslein-Volhard, 2008) and hermaphroditic rice field eel (Hou et al., 2022). In XX medaka, in the absence of germ cells, supporting cells initiate their differentiation into granulosa cells, but they eventually start expressing Sertoli-specific genes (Kurokawa et al., 2007; Nishimura et al., 2018). Therefore, germ cells are required for the maintenance of granulosa cell differentiation rather than their initial determination. It remains unclear how germ cells influence their somatic environment. In zebrafish, whose gonads first form transient juvenile ovaries, it is hypothesized that oocytes secrete factors that prevent somatic cell masculinization. Dimorphic proliferation of PGCs is observed at 14 dpf, a week before gonad differentiation (Tzung et al., 2015). Reduction in germ cell number, whether it happens at larval or adult stage, causes ovary-to-testis sex reversal (Dranow et al., 2013; Dai et al., 2015; Tzung et al., 2015). In zebrafish, multiple ovary-to-testis sex reversal phenotypes are indirectly caused by the knockout of genes involved in PGCs migration, such as adamts9 or prmt5 (Carter et al., 2019; Zhu et al., 2019; Carver et al., 2021), in oocyte development, such as bmp15 and gdf9 (Dranow et al., 2016; Chen et al., 2017), nobox (Qin et al., 2022), igf3 (Xie et al., 2021), RNA-binding proteins rbpms2a/b and ddx5 (Kaufman et al., 2018; Sone et al., 2020), or genes from the Fanconi Anemia/BRCA DNA repair pathway (Rodríguez-Marí et al., 2010; Ramanagoudr-Bhojappa et al., 2018). Among them, Bmp15 is suspected to be the oocyte secreted factor that directly acts on supporting cells through binding to its receptor Bmpr2a/b at their surface (Dranow et al., 2016). Finally, loss of nr0b1 (or dax1) leads to a skewed sex-ratio toward male, likely as a result of a significant decrease in germ cell numbers, although a role directly on somatic cells cannot be excluded (Chen et al., 2016).
Avians
In chicken gonads, undifferentiated male and female supporting cells have similar transcriptomes to the differentiated pre-granulosa cells (Estermann et al., 2020), suggesting that progenitors of the supporting cells are primed to a ovarian (pre-granulosa) fate. Pre-granulosa showed a continuous differentiation process from the undifferentiated supporting cells at E4.5 to differentiated pre-granulosa cells at E10.5 (Estermann et al., 2020). On the contrary, Sertoli cell differentiation is characterized by a rapid transcriptional change in male, resulting in a clear lineage separation. A similar phenomenon was also described in mice (Stevant et al., 2019). As mentioned before, chicken undifferentiated supporting cells express four key markers, PAX2, OSR1, WNT4 and DMRT1 (Estermann et al., 2020) (Figure 4A). PAX2 expression is downregulated at the onset of sex differentiation in both males and females (E6.5) (Estermann et al., 2020; Estermann et al., 2021b), a pattern also identified in other bird species, including emu, quail, and zebra finch, precisely predicting the onset of sex determination in all the analyzed avian species (Estermann et al., 2021b).
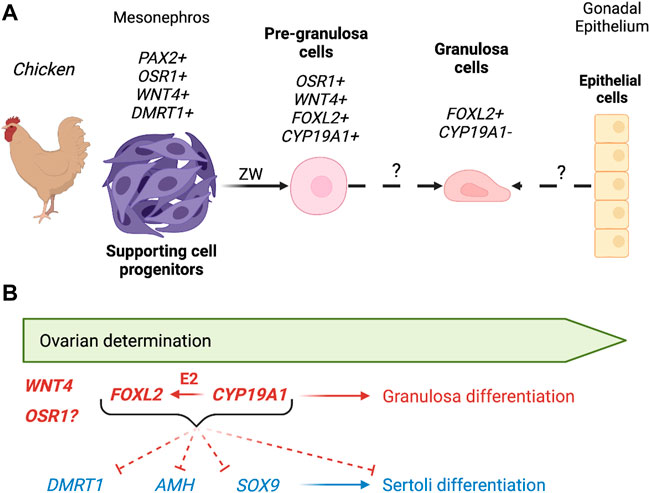
FIGURE 4. Genetic regulation of pre-granulosa cell differentiation in birds. (A) In chickens, the pre-granulosa cells derive from a PAX2/OSR1/WNT4/DMRT1 positive mesenchymal cell population. During sex differentiation, they downregulate DMRT1 and PAX2 and start upregulating key pre-granulosa cell genes CYP19A1 and FOXL2. It is still unknown if these medullary pre-granulosa cells give rise to the granulosa cells in the adult ovary follicles. (B) Although WNT4 and OSR1 were identified as pre-granulosa cell genes, their functions have not been analyzed yet. FOXL2 and CYP19A1 downregulate Sertoli cell genes DMRT1, AMH and SOX9, inhibiting Sertoli cell differentiation and inducing pre-granulosa cell program. CYP19A1 is also known to regulate FOXL2 expression. Red: pro-ovarian genes/pathways; Blue: pro-testis genes/pathways; Plain arrow: direct effect; Dotted arrow: indirect effect.
DMRT1 is the Z-linked testis-determining gene in birds (Smith et al., 2009; Lambeth et al., 2014; Ioannidis et al., 2021; Lee et al., 2021). Due to the lack of chromosome dosage compensation in birds, DMRT1 is expressed twice as high in males (ZZ) than females (ZW). The higher level of DMRT1 triggers the supporting cell differentiation into Sertoli cells by upregulating Sertoli markers AMH and SOX9 and downregulating WNT4 and OSR1 and PAX2 (Lambeth et al., 2014; Estermann et al., 2020). In females, a single dose of DMRT1 is not sufficient to trigger Sertoli differentiation (Ioannidis et al., 2021), which leads to maintenance of pre-granulosa cell genes such as WNT4 and OSR1, the downregulation of the undifferentiated marker PAX2, and upregulation of key granulosa genes FOXL2 and CYP19A1 (Figure 4A) (Major et al., 2019; Estermann et al., 2020). FOXL2 is the first to be activated in pre-granulosa cells upon sex determination in chickens (Loffler et al., 2003; Major et al., 2019). FOXL2 overexpression in male chicken gonads results in downregulation of DMRT1, SOX9 and AMH (Figure 4B) (Major et al., 2019). Conversely, FOXL2 knockdown in females results in upregulation of SOX9. Surprisingly, and unlike several fish species, CYP19A1 expression is not altered by FOXL2 knockdown or over-expression in chicken. CYP19A1 overexpression in male chicken embryos induces ovarian differentiation, inhibiting DMRT1, SOX9 and AMH and upregulating FOXL2 (Figure 4B) (Lambeth et al., 2013). This demonstrates the importance of estrogens during ovarian sex determination. It is still unclear if estrogens synthesized by CYP19A1 activate FOXL2 gene expression through ERα signaling or if it’s an indirect effect of the downregulation of repressive male genes (Figure 4B).
In chickens, WNT4 is expressed in undifferentiated supporting cells, but is downregulated in males after the onset of sex determination (Smith et al., 2008b). Both β-catenin and RSPO1 are expressed in the cortical region of the ovary (Smith et al., 2008b; Ayers et al., 2013). The role of these genes in the chicken ovary determination has not been explored but based on their expression pattern they might play a role in development of ovarian cortex.
Odd-Skipped Related Transcription Factor 1 (OSR1) was not previously associated with mammalian sex determination or supporting cell differentiation. OSR1 is expressed in differentiated chicken pre-granulosa cells, colocalizing with FOXL2 (Estermann et al., 2020). OSR1 is also significantly enriched in Muscovy duck embryonic ovaries (Bai et al., 2020), and in snapping turtle (Chelydra serpentina) embryonic gonads at the female-producing temperature (Rhen et al., 2021). In Xenopus and zebrafish, Osr1 was shown to control kidney development, but its contribution to gonadal development remains unexplored (Tena et al., 2007). In mice, lineage tracing experiments show that all gonadal somatic cells derive from a Osr1+ intermediate mesoderm/lateral plate mesoderm population from E8.5 to E9.5 (Sasaki et al., 2021). Most of the Osr1−/− mice die at early embryonic stages (E11.5-E12.5), and they lack intermediate mesoderm derivatives such as adrenal glands, metanephros and gonads (Wang et al., 2005). Therefore, Osr1 is required for gonad formation in mice, but it remains unclear whether Osr1 plays a role in ovarian differentiation.
Mammals
Mouse
Advances in transcriptomics technologies enabled the identification of genetic programs controlling ovarian differentiation, from microarrays on purified cell populations (Nef et al., 2005; Bouma et al., 2010; Jameson et al., 2012), to bulk RNA-seq (Zhao et al., 2018), and now single-cell RNA-seq (Stevant et al., 2019; Niu and Spradling, 2020). At time of sex determination, supporting cell precursors are already primed toward granulosa cell fate (Jameson et al., 2012), similar to findings in chicken (Estermann et al., 2020). This female bias was further confirmed by enrichment in open chromatin near granulosa-promoting genes in both XX and XY E10.5 supporting progenitors (Garcia-Moreno et al., 2019). As gonads differentiate into ovaries, a robust female genetic program is established through timely activation or maintenance of pro-ovarian genes and repression of pro-testis genes (Nef et al., 2005; Jameson et al., 2012; Munger et al., 2013). This differentiation is associated with an increase in active enhancers located near granulosa-promoting genes. These enhancers are enriched for TCF and FOX binding motifs, suggesting activity of the WNT/β-catenin and FOXL2 respectively (Garcia-Moreno et al., 2019).
Before sex determination, Rspo1 and Wnt4 are expressed similarly in XX and XY supporting cell precursors (Stevant et al., 2019), and the canonical β-catenin pathway is active in the coelomic epithelium (Usongo and Farookhi, 2012). Wnt4 and Rspo1 are required for the proliferation of supporting cell precursors in both XY and XX gonads (Chassot et al., 2012). Then, as sex determination is initiated, Wnt4 and Rspo1 expression and cortical β-catenin pathway activity are only maintained in XX gonads (Vainio et al., 1999; Parma et al., 2006; Usongo and Farookhi, 2012). Deletion of any of the RSPO1/WNT/β-catenin pathway factors Wnt4, Rspo1, Ctnnb1, or RSPO1 putative receptor Lgr4 results in partial ovary-to-testis sex reversal. These knockout models share similar phenotypes, with appearance of Sertoli-like cells, ectopic steroidogenic cells, and testis-specific coelomic vessel (Vainio et al., 1999; Chassot et al., 2008; Manuylov et al., 2008; Liu et al., 2009; Koizumi et al., 2015). A mutually antagonistic relationship exists between RSPO1/WNT/β-catenin pathway and pro-Sertoli genes such as Sox9 and Fgf9 (Kim et al., 2006; Lavery et al., 2012; Nicol and Yao, 2015; Tang et al., 2020). In absence of Wnt4, granulosa cells exit mitotic arrest, prematurely differentiate, and eventually transdifferentiate into Sox9+ Sertoli-like cells in the perinatal ovary (Maatouk et al., 2013). Stabilization of β-catenin in XX Rspo1 KO ovary rescues the ovary-to-testis sex reversal (Chassot et al., 2008), confirming that Rspo1 acts through the WNT/β-catenin pathway. Overexpression of β-Catenin in XY mice is sufficient to induce testis-to-ovary sex reversal (Maatouk et al., 2008). However, this is not the case when Wnt4 or Rspo1 are overexpressed in XY gonads, suggesting that additional factors are missing to efficiently stabilize β-Catenin signaling and drive granulosa cell differentiation in the XY gonads (Jordan et al., 2003; Buscara et al., 2009).
FOXL2 is a conserved transcription factor expressed in pre-granulosa cells from E12 to mature granulosa cells in adult ovaries (Schmidt et al., 2004). In the absence of Foxl2, XX embryos develop morphologically normal ovaries; then, granulosa cells gradually transdifferentiate into DMRT1+ Sertoli cells postnatally (Schmidt et al., 2004; Uda et al., 2004; Ottolenghi et al., 2005; Garcia-Ortiz et al., 2009; Nicol et al., 2019). In addition, conditional deletion of Foxl2 in adult ovarian granulosa cells induces a reprogramming into Sertoli-like cells, appearance of Leydig-like cells and testosterone production (Uhlenhaut et al., 2009). Therefore, while FOXL2 is not necessary for the initiation of the pre-granulosa cell program, it plays an important role in maintaining its identity. FOXL2 cooperates with Estrogen receptors to maintain granulosa cell identity in adult ovaries. Indeed, a similar transdifferentiation phenotype is observed in adult XX mice lacking both Esr1/Esr2 (Couse et al., 1999), or lacking both Esr1 and one Foxl2 allele in granulosa cells (Uhlenhaut et al., 2009). FOXL2 directly controls Esr2 expression and cooperates with estrogen receptors to repress Sox9 transcription in adult granulosa cells (Uhlenhaut et al., 2009; Georges et al., 2014). On the other hand, ectopic expression of Foxl2 in XY somatic cells causes partial testis-to-ovary sex reversal (Ottolenghi et al., 2007; Garcia-Ortiz et al., 2009; Nicol et al., 2018). FOXL2 plays complementary roles with the WNT/β-catenin pathway during ovarian differentiation. Indeed, Wnt4/Foxl2 and Rspo1/Foxl2 compound knockout XX mice develop a more pronounced ovary-to-testis sex reversal phenotype than Wnt4, Rspo1 or Foxl2 single knockout XX mice (Ottolenghi et al., 2005; Auguste et al., 2011).
Additional genes are involved in ovarian differentiation, such as the transcription factors FOG2 and GATA4 (Manuylov et al., 2008). The identification of RUNX1, a transcription factor involved in cell-fate determination, further highlights the complexity of the ovarian pathway. RUNX1 is expressed in the bipotential supporting cell lineage and then detected in granulosa cells and ovarian surface epithelium (Nef et al., 2005; Nicol et al., 2019). RUNX1 plays redundant roles with FOXL2 during ovarian differentiation. Ablation of Runx1 alone in somatic cells impacts common sets of genes without affecting granulosa cell identity in the fetal ovary. Runx1/Foxl2 double knockout causes masculinization of the supporting cells in the fetal ovary (Nicol et al., 2019). RUNX1 chromatin occupancy partially overlaps with FOXL2, suggesting that RUNX1 and FOXL2 share common direct target genes during ovarian differentiation.
Overall, these models reveal the multilayered regulations of ovarian differentiation, with synergistic and complementary roles between RSPO1/WNT/β-catenin, FOG2/GATA4, FOXL2 and RUNX1 to drive and maintain granulosa cell identity.
Rabbit
In the rabbit gonads, expression of FOXL2, RSPO1 and WNT4 marks the commitment toward an ovarian fate (Daniel-Carlier et al., 2013). WNT4 and RSPO1 are expressed in both XX and XY undifferentiated gonads. As sex determination is initiated at 16 dpc, WNT4 and RSPO1 are upregulated in XX gonads, reaching a peak of expression at 24 dpc and 7 dpp respectively, to eventually decrease to minimal levels in adult ovaries. FOXL2 expression is first detected between 16 and 18 dpc and rises gradually to reach a maximum that is sustained in adults (Daniel-Carlier et al., 2013). FOXL2 protein is detected in granulosa cells at 18 dpp and RSPO1 in the germ cell cytoplasm in the cortical zone at 4 dpp. To date, no knockout models are available to study the role of the WNT4, RSPO1 and FOXL2 in the rabbit ovary. In the XX CYP19A1 KO fetal ovary, the expression of both FOXL2 and RSPO1 is decreased at 22 dpc while WNT4 expression is increased, suggesting that WNT/β-catenin signaling is stimulated in KO ovaries and may contribute to absence of sex reversal (Figure 5B) (Jolivet et al., 2022).
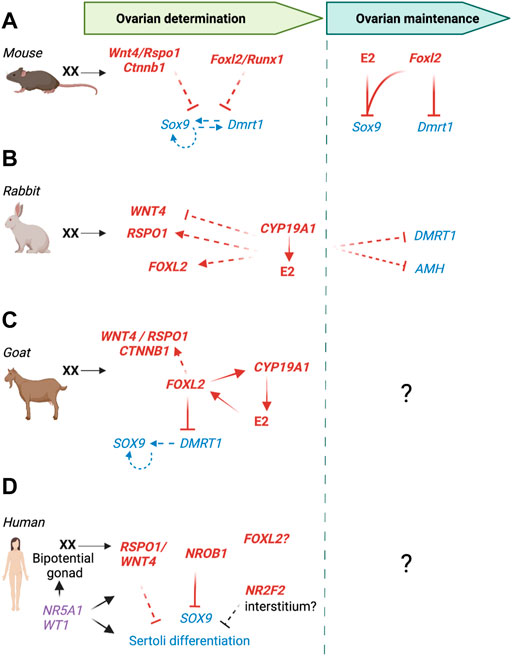
FIGURE 5. Genetic regulation of pre-granulosa cell differentiation in mammals. (A) In the mouse, RSPO1/WNT4/β-catenin pathway and Foxl2/Runx1 tilt the balance towards the female side by silencing the expression of Sox9, Dmrt1, and downstream pro-Sertoli genes. Mutation in RSPO1/WNT4/β-catenin pathway or Foxl2 causes partial ovary-to-testis sex reversal, whereas the combined loss of Foxl2 with either Runx1 or Wnt4 or Rspo1 leads to a more severe ovary-to-testis sex reversal. FOXL2 is also essential for the maintenance of granulosa cell identity in adult ovaries. The joint action of FOXL2 and estrogens (E2) enables the maintenance of ovarian identity. Loss of Foxl2 or estrogen receptors Esr1/Esr2 leads to an upregulation of SOX9 and DMRT1, and transdifferentiation of granulosa into Sertoli-like cells. (B) In the rabbit, CYP19A1 is not involved in primary sex determination, but plays a crucial role in early ovarian development. Loss of CYP19A1 reduces the expression of FOXL2 and RSPO1 in fetal ovaries and upregulates the expression of DMRT1 and AMH in some adult granulosa cells from large antral follicles, leading to ovarian defects without any sign of morphological gonad masculinization. (C) In the goat, FOXL2 is a crucial factor in early ovarian differentiation. FOXL2 directly controls CYP19A1 FOXL2 loss of function causes downregulation of the WNT/β-catenin pathway, upregulation of DMRT1 followed by SOX9, leading to complete ovary-to-testis sex reversal. (D) In humans, RSPO1 is one of the earliest sexually dimorphic pro-ovarian genes during gonad differentiation. Mutations in WNT4 or RSPO1 causes XX ovotesticular development while duplication of the genomic region containing both WNT4 and RSPO1 causes XY gonadal dysgenesis. Duplication of NR0B1 (DAX1) causes XY male-to-female sex reversal with gonad dysgenesis. Mutations of NR5A1 or WT1 cause both XX and XY DSDs, likely due to their role in bipotential gonad formation. Mutation or deletion of a portion of NR2F2 causes XX testis development. As NR2F2 expression is detected in interstitium, it is unclear how this gene impacts granulosa cell identity. It remains unclear whether FOXL2 is involved in ovarian determination as heterozygous mutations cause folliculogenesis defects rather than gonad masculinization. Red: pro-ovarian genes/pathways; Blue: pro-testis genes/pathways; Plain arrow: direct effect; Dotted arrow: indirect effect.
Goat
In goats, a naturally occurring deletion 280 kb upstream of the FOXL2 gene is responsible for the polled intersex phenotype (PIS), causing ovary-to-testis sex reversal in XX homozygotes (Pailhoux et al., 2001). The PIS phenotype is associated with the loss of expression of FOXL2 and three long non-coding RNAs (Pailhoux et al., 2001; Beysen et al., 2005). Generation of a goat FOXL2 KO model demonstrated that loss of FOXL2 expression alone is responsible for PIS and XX ovary-to-testis sex reversal phenotype (Boulanger et al., 2014). In both XX PIS mutant and XX FOXL2 KO gonads, expression of pro-ovarian genes RSPO1, RSPO2, WNT4, CYP19 and FST is downregulated while the expression of pro-testis genes SOX9, AMH, DMRT1 and CYP17 is upregulated (Figure 5C) (Boulanger et al., 2014; Elzaiat et al., 2014).
During goat ovarian differentiation, granulosa cells in the ovarian medulla co-express FOXL2 and CYP19A1, while RSPO1 is expressed in the ovarian cortex (Pannetier et al., 2006; Kocer et al., 2008). In vitro studies showed that FOXL2 could regulate CYP19A1 promoter activity. DMRT1 up-regulation in XX PIS mutant gonads precedes SOX9 up-regulation, suggesting a critical role of DMRT1 for testis differentiation in goats (Figure 5C) (Elzaiat et al., 2014). RUNX1 is highly expressed in XX gonads during sex differentiation, but its function in goat ovary remains to be determined (Nicol et al., 2019). Hence, ovarian differentiation in goats is mainly mediated by FOXL2 and partially regulated by the RSPO1/WNT4 pathway. FOXL2 prevents activation of the pro-testis program through antagonizing of DMRT1 (Figure 5C).
Human
In humans, the identification of genes involved in granulosa cell differentiation and ovarian development derives from analyses of individuals presenting Differences of Sex Development (DSD) (Figure 5D), combined with knowledge acquired from genetically modified mouse models. DSDs are congenital conditions in which the development of chromosomal, gonadal, or anatomical sex is atypical. Analyses of SRY-negative cases of XX testicular DSD and XY feminization or dysgenesis, led to the identification of key pro-ovarian genes. Duplication of the X-linked gene NR0B1 (also called DAX1) causes 46, XY male-to-female sex reversal with gonad dysgenesis (Bardoni et al., 1994; García-Acero et al., 2019). In the mouse, overexpression of Nr0b1 in XY gonads inhibits NR5A1 activation of Sox9 enhancer, resulting in ovotestis development (Ludbrook et al., 2012). Duplication of a genomic region containing both WNT4 and RSPO1 genes results in XY gonadal dysgenesis (Jordan et al., 2001). WNT4 missense mutation causes embryonic lethal SERKAL syndrome with 46, XX (ovo)testicular DSD (Mandel et al., 2008). Homozygous mutations for RSPO1 are associated with XX DSD and testicular or ovotesticular development (Parma et al., 2006; Tomaselli et al., 2011; Naasse et al., 2017; Tallapaka et al., 2018). These findings confirm the key role that WNT4 and RSPO1 play in granulosa cell differentiation and early ovarian differentiation in humans. Further analyses revealed that RSPO1 is upregulated in the ovary between 6 and 9 wpc, whereas the expression of WNT4 and CTNNB1 is not significantly different between ovary and testis at this stage (Tomaselli et al., 2011).
In humans, contrary to other vertebrate species, no FOXL2 mutations are linked to 46, XX (ovo)testicular DSD. Heterozygous mutations of FOXL2 are associated with the autosomal dominant Blepharophimosis Ptosis Epicanthus Inversus syndrome (BPES), which causes early premature ovarian insufficiency in BPES type I (Crisponi et al., 2001). This raises the question whether FOXL2 still acts in early granulosa cell differentiation/ovarian determination in humans, or if its role is limited to later granulosa cell function in folliculogenesis.
NR5A1, encoding for Steroidogenic Factor 1 (SF-1), is probably one of the most complex genes studied in DSDs, associated with a wide spectrum of DSD cases ranging from XY gonadal dysgenesis to male infertility, as well as primary ovarian insufficiency in women (Domenice et al., 2016). Multiple cases of 46, XX (ovo)testicular DSD were linked to a heterozygous missense mutation p.R92W in the DNA binding domain of NR5A1 (Bashamboo et al., 2016; Baetens et al., 2017). Remarkably, some XX carriers were asymptomatic and fertile, whereas in one family, this same mutation also led to XY DSD in a sibling. It remains unclear how the phenotype of this point mutation is so variable. Generation of a mouse model carrying this mutation caused XY dysgenesis, but not XX sex-reversal, suggesting some differences in p.R92W mutated NR5A1 activity between human and mouse (Miyado et al., 2016). This capacity to cause both XX and XY DSD is likely due to NR5A1 involvement in early gonadogenesis and establishment of bipotential supporting cells (Hanley et al., 1999). Like NR5A1, the gene WT1 is involved in bipotential gonad formation and mutations were previously only associated with XY DSD. However, several pathogenic variants of WT1 have been associated with testis differentiation in XX individuals (Gomes et al., 2019; Eozenou et al., 2020; Sirokha et al., 2021; Ferrari et al., 2022). For both NR5A1 and WT1 mutations, it is suspected that these pathogenic variants impair or modify the capacity of NR5A1 or WT1 to physically interact with β-catenin, therefore compromising ovarian differentiation (Bashamboo et al., 2016; Eozenou et al., 2020).
Heterozygous mutation or genomic deletion of NR2F2 (or COUP-TFII) cause testis development in XX individuals (Bashamboo et al., 2018; Carvalheira et al., 2019). These genetic defects were also associated with BPES, a syndrome usually associated with FOXL2 mutations. NR2F2 protein is detected exclusively in interstitial cells of the human ovary at 9 wpc and not in FOXL2+ granulosa cells. It is unclear how NR2F2 impacts granulosa cell differentiation and how its loss of function results in testis tissue development. NR2F2 may act indirectly from the stroma compartment. Another possibility is that NR2F2 is expressed early on in supporting cell precursors before becoming exclusively expressed in the interstitial cells, and its loss impacts supporting cell capacity to differentiate into granulosa cells. Again, the DSD cases of NR2F2 mutations highlight the differences in early gonad differentiation between the mouse and human, as loss of Nr2f2 in the mouse ovary is not associated with ovary-to-testis sex reversal (Zhao et al., 2017).
Many cases of SRY-negative XX ovotesticular DSD remain unsolved. Mutations may be located in non-coding genomic regions that control the expression of known pro-ovarian genes. For instance, rare single nucleotide variations in putative enhancers for WNT4 and RSPO1 were found in some patients with impaired ovarian development (Nakagawa et al., 2022). The functional significance of these putative enhancers and their mutations require additional experiments for confirmation.
Transcriptomic analyses of human fetal ovaries by microarray (Mamsen et al., 2017) and bulk RNA-seq (Lecluze et al., 2020) provided new insights into the dynamics of expression of the genes identified as involved in XX DSD. Some notable differences with the mouse model were identified: for instance, expression of NR5A1 is maintained longer in the human fetal ovary during early sex-differentiation (Mamsen et al., 2017; Lecluze et al., 2020) whereas it is quickly decreased in the mouse fetal ovary at the onset of sex determination (Ikeda et al., 1994). This difference in NR5A1 expression dynamics may explain the difference in phenotype with the NR5A1 p.R92W missense mutation that results in XX testicular DSD in humans but not in the mouse (Miyado et al., 2016). Another discrepancy between mouse and human implicates WNT4: while Wnt4 expression is sexually dimorphic during sex determination in the mouse, human WNT4 expression is not significantly different between sexes, despite its involvement in 46, XX testicular DSD (Mamsen et al., 2017). On the other hand, RSPO1 and AMHR2, LGR5, and RUNX1 are among the earliest pro-ovarian genes expressed in human fetal ovaries with strong sexual dimorphism around 6 wpc/early 7 wpc (Lecluze et al., 2020). Meanwhile, FOXL2 becomes enriched in the human fetal ovary after 7 wpc, a bit later than these genes (Lecluze et al., 2020). This may explain why mutations in FOXL2 gene does not lead to ovary masculinization in humans but instead to a folliculogenesis phenotype.
Evolutionary perspectives
Altogether, although some factors like the RSPO1/WNT/β-Catenin pathway and FOXL2 have a broadly conserved role in ovarian differentiation, some divergence led to clade-specific, and even species-specific sub-functionalization. Among the conserved pathways, the RSPO1/WNT/β-catenin signaling is a strong driver of ovarian differentiation from fish to human. In mammals, this pathway seems to have taken the front seat, as the strongest ovary-to-testis sex-reversal phenotype from a single gene deletion is caused by interrupting this pathway in mice. Similarly in humans, rare cases of XY DSD caused by a genomic duplication involve the locus encompassing RSPO1 and WNT4. While FOXL2 expression in the ovary is highly conserved throughout vertebrate species, its functions in either primary sex determination, ovarian maintenance or folliculogenesis has evolved within vertebrate clades, and even within species from the same clade. This phenomenon may be related to the evolution of DMRT1 functions in testis determination and maintenance, as FOXL2/DMRT1 directly antagonize each other. Another possibility is that FOXL2 functions evolved with the role of estrogens in ovarian differentiation through FOXL2’s capacity to directly control CYP19A1 expression and cooperate with estrogen receptors. Indeed, FOXL2 seems to play a more prominent role in ovarian differentiation of species that rely on estrogen signaling. Tilapia is a good example as foxl2 and cyp19a1a KO both lead to identical phenotypes. In most species, FOXL2 and CYP19A1 are expressed in the same cell populations, whether it is granulosa cells, or steroidogenic cells in fish ovaries, supporting the hypothesis that FOXL2 directly controls CYP19A1 expression. An exception to the rule is the rabbit, as CYP19A1 and FOXL2 are expressed in the cortex and medulla, respectively, in the fetal ovary. This suggests that FOXL2 functions are decoupled from CYP19A1 activation in the rabbit fetal ovary and raises the question of what molecular pathways control CYP19A1 expression in the rabbit ovarian cortex. Therefore, it is still unclear how the mode of action of FOXL2 has evolved depending on the species.
Similar to FOXL2, the role of other genes in ovarian differentiation has evolved within the same clades. For instance, comparison of some human DSD with mouse models for an identical mutation led to surprisingly different phenotypes. These differences may be caused by difference in timing of morphogenesis between the species, changing the influence some genes like NR5A1 or NR2F2 can have on ovarian differentiation.
Finally, one clear evolutionary difference in vertebrate ovarian differentiation is the impact germ cells have on ovarian identity. While germ cell numbers influence the fate of gonadal somatic cells and the maintenance of granulosa cell identity in fish, it is not the case in mice (Maatouk et al., 2012). In fish, ovarian germ cells can also autonomously control their own fate through foxl2l, a gene closely related to FOXL2. During evolution, this gene was lost in terrestrial vertebrates, and germ cell fate became exclusively dependent on supporting cell fate. It is unclear whether the presence of this foxl2l gene also influences the impact of germ cells on supporting cell identity. While germ cells do not influence granulosa cell determination in mice, differentiation of Lgr5+ pre-granulosa cells into Foxl2+ granulosa cells is delayed in mutant ovaries lacking germ cell-specific genes Nanos3 or Figla (Fukuda et al., 2021). Therefore, the key role of supporting/germ cell communication in ovarian determination has been lost during vertebrate evolution, but it remains critical for proper cortical granulosa cell differentiation in mice.
Conclusion and perspectives
From fish species with an incredible range of sex determination systems and gonadal plasticity, to chicken with left-right asymmetrical ovarian development, to placental mammals whose gonad differentiation occurs in the environment of the womb and maternal hormones, evolution of ovarian differentiation reveals both relatively conserved and unique features within vertebrate clades and species. This demonstrates that evolution of ovarian differentiation is non-linear, and some unique evolutionary events occurred independently among species. Of course, this review on vertebrate ovarian differentiation does not intend to cover all vertebrate species, and other vertebrates such as amphibians, lizards or turtles have their own story to tell.
Intriguing new potential players in ovarian differentiation require further study in different vertebrate clades. For instance, while RUNX1 plays complementary roles with FOXL2 in maintaining fetal granulosa cell identity in mice, its ovarian function in other vertebrate species remains to be determined. The potential roles of OSR1, a gene enriched in avian pre-granulosa cells, and NR2F2, a gene linked to ovary-to-testis sex reversal in humans, need to be examined in other species. Beyond granulosa cells, the functions of other ovarian cell populations such as NR2F2+ interstitium or germ cells cannot be ignored. For instance, Lhx2 gene in germ cells is involved in repression of endothelial cell migration in the mouse developing ovary (Singh et al., 2022).
The emerging single-cell sequencing technologies are becoming a game changer in the study of developmental processes, providing single-cell resolution of tissue composition and cell lineage differentiation trajectories. Single-cell RNA-seq methods have already been used to study gonad differentiation in various species (Estermann and Smith, 2020) and more studies come out regularly. In addition, the use of related techniques such as spatial transcriptomics and single-cell ATAC-seq will allow the building of a comprehensive developmental cell atlas of gonad differentiation and further improve the comparative analyses of granulosa cell origins and differentiation kinetics within vertebrate clades. For instance, the Human Gonad Development Atlas, a part of the Human Developmental Cell Atlas (HDCA), is aimed to create a comprehensive map of cells during human fetal development using 2D/3D imaging as well as single cell multiomics (Haniffa et al., 2021; Garcia-Alonso et al., 2022). Beyond gene expression, identifying non-coding regulatory elements, enhancers with potential granulosa cell signatures and cell-specific chromatin dynamics associated with different stages of ovarian differentiation will further improve our knowledge of ovarian differentiation and maintenance of its identity.
Author contributions
BN, ME, HY, and NM conceived and wrote the review. All authors approved the submitted version.
Funding
This work was supported by the Intramural Research Program (ES102965 to HY) of the NIH, National Institute of Environmental Health Sciences, and by ANR grants (ANR-19-CE14-0012-RNA-SEX and ANR-20-CE14-0022-ARDIGERM to NM).
Acknowledgments
Figures were created with BioRender.com.
Conflict of interest
The authors declare that the research was conducted in the absence of any commercial or financial relationships that could be construed as a potential conflict of interest.
Publisher’s note
All claims expressed in this article are solely those of the authors and do not necessarily represent those of their affiliated organizations, or those of the publisher, the editors and the reviewers. Any product that may be evaluated in this article, or claim that may be made by its manufacturer, is not guaranteed or endorsed by the publisher.
References
Albrecht, K. H., and Eicher, E. M. (2001). Evidence that Sry is expressed in pre-Sertoli cells and Sertoli and granulosa cells have a common precursor. Dev. Biol. 240 (1), 92–107. doi:10.1006/dbio.2001.0438
Andrews, J. E., Smith, C. A., and Sinclair, A. H. (1997). Sites of estrogen receptor and aromatase expression in the chicken embryo. Gen. Comp. Endocrinol. 108 (2), 182–190. doi:10.1006/gcen.1997.6978
Auguste, A., Chassot, A. A., Gregoire, E. P., Renault, L., Pannetier, M., Treier, M., et al. (2011). Loss of R-spondin1 and Foxl2 amplifies female-to-male sex reversal in XX mice. Sex. Dev. 5 (6), 304–317. doi:10.1159/000334517
Ayers, K. L., Sinclair, A. H., and Smith, C. A. (2013). The molecular genetics of ovarian differentiation in the avian model. Sex. Dev. 7 (1-3), 80–94. doi:10.1159/000342358
Baetens, D., Stoop, H., Peelman, F., Todeschini, A. L., Rosseel, T., Coppieters, F., et al. (2017). NR5A1 is a novel disease gene for 46, XX testicular and ovotesticular disorders of sex development. Genet. Med. 19 (4), 367–376. doi:10.1038/gim.2016.118
Bai, D. P., Chen, Y., Hu, Y. Q., He, W. F., Shi, Y. Z., Fan, Q. M., et al. (2020). Transcriptome analysis of genes related to gonad differentiation and development in Muscovy ducks. BMC Genomics 21 (1), 438. doi:10.1186/s12864-020-06852-z
Baker, P. J., Moore, H. D., Burgess, A. M., and Mittwoch, U. (1993). Gonadal sex differentiation in embryos and neonates of the marsupial, Monodelphis domestica: Arrest of testis development in postterm embryos. J. Anat. 182 (2), 267–273.
Bannister, S. C., Smith, C. A., Roeszler, K. N., Doran, T. J., Sinclair, A. H., and Tizard, M. L. (2011). Manipulation of estrogen synthesis alters MIR202* expression in embryonic chicken gonads. Biol. Reprod. 85 (1), 22–30. doi:10.1095/biolreprod.110.088476
Bardoni, B., Zanaria, E., Guioli, S., Floridia, G., Worley, K. C., Tonini, G., et al. (1994). A dosage sensitive locus at chromosome Xp21 is involved in male to female sex reversal. Nat. Genet. 7 (4), 497–501. doi:10.1038/ng0894-497
Bashamboo, A., Donohoue, P. A., Vilain, E., Rojo, S., Calvel, P., Seneviratne, S. N., et al. (2016). A recurrent p.Arg92Trp variant in steroidogenic factor-1 (NR5A1) can act as a molecular switch in human sex development. Hum. Mol. Genet. 25 (16), 3446–3453. doi:10.1093/hmg/ddw186
Bashamboo, A., Eozenou, C., Jorgensen, A., Bignon-Topalovic, J., Siffroi, J. P., Hyon, C., et al. (2018). Loss of function of the nuclear receptor NR2F2, encoding COUP-TF2, causes testis development and cardiac defects in 46, XX children. Am. J. Hum. Genet. 102 (3), 487–493. doi:10.1016/j.ajhg.2018.01.021
Beer, R. L., and Draper, B. W. (2013). nanos3 maintains germline stem cells and expression of the conserved germline stem cell gene nanos2 in the zebrafish ovary. Dev. Biol. 374 (2), 308–318. doi:10.1016/j.ydbio.2012.12.003
Bernard, V., Kherra, S., Francou, B., Fagart, J., Viengchareun, S., Guéchot, J., et al. (2017). Familial multiplicity of estrogen insensitivity associated with a loss-of-function ESR1 mutation. J. Clin. Endocrinol. Metab. 102 (1), 93–99. doi:10.1210/jc.2016-2749
Bernier, N., and Beaumont, A. (1964). [Structure and regression of the mesonephros of the rabbit fetus]. C. R. Seances Soc. Biol. Fil. 158, 2227–2230.
Bertho, S., Herpin, A., Branthonne, A., Jouanno, E., Yano, A., Nicol, B., et al. (2018). The unusual rainbow trout sex determination gene hijacked the canonical vertebrate gonadal differentiation pathway. Proc. Natl. Acad. Sci. U. S. A. 115 (50), 12781–12786. doi:10.1073/pnas.1803826115
Bertho, S., Pasquier, J., Pan, Q., Le Trionnaire, G., Bobe, J., Postlethwait, J. H., et al. (2016). Foxl2 and its relatives are evolutionary conserved players in gonadal sex differentiation. Sex. Dev. 10 (3), 111–129. doi:10.1159/000447611
Beysen, D., Raes, J., Leroy, B. P., Lucassen, A., Yates, J. R., Clayton-Smith, J., et al. (2005). Deletions involving long-range conserved nongenic sequences upstream and downstream of FOXL2 as a novel disease-causing mechanism in blepharophimosis syndrome. Am. J. Hum. Genet. 77 (2), 205–218. doi:10.1086/432083
Boulanger, L., Pannetier, M., Gall, L., Allais-Bonnet, A., Elzaiat, M., Le Bourhis, D., et al. (2014). FOXL2 is a female sex-determining gene in the goat. Curr. Biol. 24 (4), 404–408. doi:10.1016/j.cub.2013.12.039
Bouma, G. J., Hudson, Q. J., Washburn, L. L., and Eicher, E. M. (2010). New candidate genes identified for controlling mouse gonadal sex determination and the early stages of granulosa and Sertoli cell differentiation. Biol. Reprod. 82 (2), 380–389. doi:10.1095/biolreprod.109.079822
Braat, A. K., Zandbergen, T., van de Water, S., Goos, H. J., and Zivkovic, D. (1999). Characterization of zebrafish primordial germ cells: Morphology and early distribution of vasa RNA. Dev. Dyn. 216 (2), 153–167. doi:10.1002/(SICI)1097-0177(199910)216:2<153::AID-DVDY6>3.0.CO;2-1
Britt, K. L., Drummond, A. E., Dyson, M., Wreford, N. G., Jones, M. E., Simpson, E. R., et al. (2001). The ovarian phenotype of the aromatase knockout (ArKO) mouse. J. Steroid Biochem. Mol. Biol. 79 (1-5), 181–185. doi:10.1016/s0960-0760(01)00158-3
Britt, K. L., Kerr, J., O'Donnell, L., Jones, M. E., Drummond, A. E., Davis, S. R., et al. (2002). Estrogen regulates development of the somatic cell phenotype in the eutherian ovary. FASEB J. 16 (11), 1389–1397. doi:10.1096/fj.01-0992com
Britt, K. L., Stanton, P. G., Misso, M., Simpson, E. R., and Findlay, J. K. (2004). The effects of estrogen on the expression of genes underlying the differentiation of somatic cells in the murine gonad. Endocrinology 145 (8), 3950–3960. doi:10.1210/en.2003-1628
Brunström, B., Axelsson, J., Mattsson, A., and Halldin, K. (2009). Effects of estrogens on sex differentiation in Japanese quail and chicken. Gen. Comp. Endocrinol. 163 (1-2), 97–103. doi:10.1016/j.ygcen.2009.01.006
Bunce, C., McKey, J., and Capel, B. (2021). Concerted morphogenesis of genital ridges and nephric ducts in the mouse captured through whole-embryo imaging. Development 148 (18), dev199208. doi:10.1242/dev.199208
Burns, R. K. (1955). Experimental reversal of sex in the gon ads of the opossum Didelphis virginiana. Proc. Natl. Acad. Sci. U. S. A. 41 (9), 669–676. doi:10.1073/pnas.41.9.669
Buscara, L., Montazer-Torbati, F., Chadi, S., Auguste, A., Laubier, J., Chassot, A. A., et al. (2009). Goat RSPO1 over-expression rescues sex-reversal in Rspo1-knockout XX mice but does not perturb testis differentiation in XY or sex-reversed XX mice. Transgenic Res. 18 (4), 649–654. doi:10.1007/s11248-009-9247-2
Byskov, A. G. (1986). Differentiation of mammalian embryonic gonad. Physiol. Rev. 66 (1), 71–117. doi:10.1152/physrev.1986.66.1.71
Cai, H., Liu, B., Wang, H., Sun, G., Feng, L., Chen, Z., et al. (2020). SP1 governs primordial folliculogenesis by regulating pregranulosa cell development in mice. J. Mol. Cell Biol. 12 (3), 230–244. doi:10.1093/jmcb/mjz059
Calatayud, N. E., Pask, A. J., Shaw, G., Richings, N. M., Osborn, S., and Renfree, M. B. (2010). Ontogeny of the oestrogen receptors ESR1 and ESR2 during gonadal development in the tammar wallaby, Macropus eugenii. Reproduction 139 (3), 599–611. doi:10.1530/rep-09-0305
Cao, Z., Mao, X., and Luo, L. (2019). Germline stem cells drive ovary regeneration in zebrafish. Cell Rep. 26 (7), 1709–1717. doi:10.1016/j.celrep.2019.01.061
Carter, N. J., Roach, Z. A., Byrnes, M. M., and Zhu, Y. (2019). Adamts9 is necessary for ovarian development in zebrafish. Gen. Comp. Endocrinol. 277, 130–140. doi:10.1016/j.ygcen.2019.04.003
Carvalheira, G., Malinverni, A. M., Moysés-Oliveira, M., Ueta, R., Cardili, L., Monteagudo, P., et al. (2019). The natural history of a man with ovotesticular 46, XX DSD caused by a novel 3-mb 15q26.2 deletion containing NR2F2 gene. J. Endocr. Soc. 3 (11), 2107–2113. doi:10.1210/js.2019-00241
Carver, J. J., He, Y., and Zhu, Y. (2021). Delay in primordial germ cell migration in adamts9 knockout zebrafish. Sci. Rep. 11 (1), 8545. doi:10.1038/s41598-021-88024-x
Chang, X., Kobayashi, T., Senthilkumaran, B., Kobayashi-Kajura, H., Sudhakumari, C. C., and Nagahama, Y. (2005). Two types of aromatase with different encoding genes, tissue distribution and developmental expression in Nile tilapia (Oreochromis niloticus). Gen. Comp. Endocrinol. 141 (2), 101–115. doi:10.1016/j.ygcen.2004.11.020
Chassot, A. A., Bradford, S. T., Auguste, A., Gregoire, E. P., Pailhoux, E., de Rooij, D. G., et al. (2012). WNT4 and RSPO1 together are required for cell proliferation in the early mouse gonad. Development 139 (23), 4461–4472. doi:10.1242/dev.078972
Chassot, A. A., Ranc, F., Gregoire, E. P., Roepers-Gajadien, H. L., Taketo, M. M., Camerino, G., et al. (2008). Activation of beta-catenin signaling by Rspo1 controls differentiation of the mammalian ovary. Hum. Mol. Genet. 17 (9), 1264–1277. doi:10.1093/hmg/ddn016
Chen, S., Zhang, H., Wang, F., Zhang, W., and Peng, G. (2016). nr0b1 (DAX1) mutation in zebrafish causes female-to-male sex reversal through abnormal gonadal proliferation and differentiation. Mol. Cell. Endocrinol. 433, 105–116. doi:10.1016/j.mce.2016.06.005
Chen, W., Liu, L., and Ge, W. (2017). Expression analysis of growth differentiation factor 9 (Gdf9/gdf9), anti-müllerian hormone (Amh/amh) and aromatase (Cyp19a1a/cyp19a1a) during gonadal differentiation of the zebrafish, Danio rerio. Biol. Reprod. 96 (2), 401–413. doi:10.1095/biolreprod.116.144964
Chen, Y., Breen, K., and Pepling, M. E. (2009). Estrogen can signal through multiple pathways to regulate oocyte cyst breakdown and primordial follicle assembly in the neonatal mouse ovary. J. Endocrinol. 202 (3), 407–417. doi:10.1677/joe-09-0109
Couse, J. F., Hewitt, S. C., Bunch, D. O., Sar, M., Walker, V. R., Davis, B. J., et al. (1999). Postnatal sex reversal of the ovaries in mice lacking estrogen receptors alpha and beta. Science 286 (5448), 2328–2331. doi:10.1126/science.286.5448.2328
Coveney, D., Shaw, G., and Renfree, M. B. (2001). Estrogen-induced gonadal sex reversal in the tammar wallaby. Biol. Reprod. 65 (2), 613–621. doi:10.1095/biolreprod65.2.613
Crisponi, L., Deiana, M., Loi, A., Chiappe, F., Uda, M., Amati, P., et al. (2001). The putative forkhead transcription factor FOXL2 is mutated in blepharophimosis/ptosis/epicanthus inversus syndrome. Nat. Genet. 27 (2), 159–166. doi:10.1038/84781
Dai, S., Qi, S., Wei, X., Liu, X., Li, Y., Zhou, X., et al. (2021). Germline sexual fate is determined by the antagonistic action of dmrt1 and foxl3/foxl2 in tilapia. Development 148 (8), dev199380. doi:10.1242/dev.199380
Dai, X., Jin, X., Chen, X., He, J., and Yin, Z. (2015). Sufficient numbers of early germ cells are essential for female sex development in zebrafish. PLoS One 10 (2), e0117824. doi:10.1371/journal.pone.0117824
Daniel-Carlier, N., Harscoet, E., Thepot, D., Auguste, A., Pailhoux, E., and Jolivet, G. (2013). Gonad differentiation in the rabbit: Evidence of species-specific features. PLoS One 8 (4), e60451. doi:10.1371/journal.pone.0060451
Diaz-Hernandez, V., Caldelas, I., and Merchant-Larios, H. (2019). Gene expression in the supporting cells at the onset of meiosis in rabbit gonads. Sex. Dev. 13 (3), 125–136. doi:10.1159/000502193
Domenice, S., Machado, A. Z., Ferreira, F. M., Ferraz-de-Souza, B., Lerario, A. M., Lin, L., et al. (2016). Wide spectrum of NR5A1-related phenotypes in 46, XY and 46, XX individuals. Birth Defects Res. C Embryo Today 108 (4), 309–320. doi:10.1002/bdrc.21145
Dranow, D. B., Hu, K., Bird, A. M., Lawry, S. T., Adams, M. T., Sanchez, A., et al. (2016). Bmp15 is an oocyte-produced signal required for maintenance of the adult female sexual phenotype in zebrafish. PLoS Genet. 12 (9), e1006323. doi:10.1371/journal.pgen.1006323
Dranow, D. B., Tucker, R. P., and Draper, B. W. (2013). Germ cells are required to maintain a stable sexual phenotype in adult zebrafish. Dev. Biol. 376 (1), 43–50. doi:10.1016/j.ydbio.2013.01.016
Dutta, S., Mark-Kappeler, C. J., Hoyer, P. B., and Pepling, M. E. (2014). The steroid hormone environment during primordial follicle formation in perinatal mouse ovaries. Biol. Reprod. 91 (3), 68. doi:10.1095/biolreprod.114.119214
Elbrecht, A., and Smith, R. G. (1992). Aromatase enzyme activity and sex determination in chickens. Science 255 (5043), 467–470. doi:10.1126/science.1734525
Elzaiat, M., Jouneau, L., Thepot, D., Klopp, C., Allais-Bonnet, A., Cabau, C., et al. (2014). High-throughput sequencing analyses of XX genital ridges lacking FOXL2 reveal DMRT1 up-regulation before SOX9 expression during the sex-reversal process in goats. Biol. Reprod. 91 (6), 153. doi:10.1095/biolreprod.114.122796
Eozenou, C., Gonen, N., Touzon, M. S., Jorgensen, A., Yatsenko, S. A., Fusee, L., et al. (2020). Testis formation in XX individuals resulting from novel pathogenic variants in Wilms' tumor 1 (WT1) gene. Proc. Natl. Acad. Sci. U. S. A. 117 (24), 13680–13688. doi:10.1073/pnas.1921676117
Erickson, B. H. (1966). Development and radio-response of the prenatal bovine ovary. Reproduction 11 (1), 97–105. doi:10.1530/jrf.0.0110097
Erickson, G. F., Challis, J. R., and Ryan, K. J. (1974). A developmental study on the capacity of rabbit granulosa cells to respond to trophic hormones and secrete progesterone in vitro. Dev. Biol. 40 (2), 208–224. doi:10.1016/0012-1606(74)90124-9
Estermann, M. A., Hirst, C. E., Major, A. T., and Smith, C. A. (2021a). The homeobox gene TGIF1 is required for chicken ovarian cortical development and generation of the juxtacortical medulla. Development 148 (16), dev199646. doi:10.1242/dev.199646
Estermann, M. A., Mariette, M. M., Moreau, J. L. M., Combes, A. N., and Smith, C. A. (2021b). PAX2 (+) mesenchymal origin of gonadal supporting cells is conserved in birds. Front. Cell Dev. Biol. 9, 735203. doi:10.3389/fcell.2021.735203
Estermann, M. A., and Smith, C. A. (2020). Applying single-cell analysis to gonadogenesis and DSDs (Disorders/Differences of sex development). Int. J. Mol. Sci. 21 (18), E6614. doi:10.3390/ijms21186614
Estermann, M. A., Williams, S., Hirst, C. E., Roly, Z. Y., Serralbo, O., Adhikari, D., et al. (2020). Insights into gonadal sex differentiation provided by single-cell transcriptomics in the chicken embryo. Cell Rep. 31 (1), 107491. doi:10.1016/j.celrep.2020.03.055
Fadem, B. H., and Tesoriero, J. V. (1986). Inhibition of testicular development and feminization of the male genitalia by neonatal estrogen treatment in a marsupial. Biol. Reprod. 34 (4), 771–776. doi:10.1095/biolreprod34.4.771
Fan, Z., Zou, Y., Liang, D., Tan, X., Jiao, S., Wu, Z., et al. (2019). Roles of forkhead box protein L2 (foxl2) during gonad differentiation and maintenance in a fish, the olive flounder (Paralichthys olivaceus). Reprod. Fertil. Dev. 31 (11), 1742–1752. doi:10.1071/rd18233
Feng, L., Wang, Y., Cai, H., Sun, G., Niu, W., Xin, Q., et al. (2016). ADAM10-Notch signaling governs the recruitment of ovarian pregranulosa cells and controls folliculogenesis in mice. J. Cell Sci. 129 (11), 2202–2212. doi:10.1242/jcs.184267
Fenske, M., and Segner, H. (2004). Aromatase modulation alters gonadal differentiation in developing zebrafish (Danio rerio). Aquat. Toxicol. 67 (2), 105–126. doi:10.1016/j.aquatox.2003.10.008
Ferrari, M. T. M., Watanabe, A., da Silva, T. E., Gomes, N. L., Batista, R. L., Nishi, M. Y., et al. (2022). WT1 pathogenic variants are associated with a broad spectrum of differences in sex development phenotypes and heterogeneous progression of renal disease. Sex. Dev. 16 (1), 46–54. doi:10.1159/000517373
Fisher, C. R., Graves, K. H., Parlow, A. F., and Simpson, E. R. (1998). Characterization of mice deficient in aromatase (ArKO) because of targeted disruption of the cyp19 gene. Proc. Natl. Acad. Sci. U. S. A. 95 (12), 6965–6970. doi:10.1073/pnas.95.12.6965
Fowler, P. A., Anderson, R. A., Saunders, P. T., Kinnell, H., Mason, J. I., Evans, D. B., et al. (2011). Development of steroid signaling pathways during primordial follicle formation in the human fetal ovary. J. Clin. Endocrinol. Metab. 96 (6), 1754–1762. doi:10.1210/jc.2010-2618
Fukuda, K., Muraoka, M., Kato, Y., and Saga, Y. (2021). Decoding the transcriptome of pre-granulosa cells during the formation of primordial follicles in the mouse. Biol. Reprod. 105 (1), 179–191. doi:10.1093/biolre/ioab065
Gan, R. H., Wang, Y., Li, Z., Yu, Z. X., Li, X. Y., Tong, J. F., et al. (2021). Functional divergence of multiple duplicated Foxl2 homeologs and alleles in a recurrent polyploid fish. Mol. Biol. Evol. 38 (5), 1995–2013. doi:10.1093/molbev/msab002
García-Acero, M., Molina, M., Moreno, O., Ramirez, A., Forero, C., Céspedes, C., et al. (2019). Gene dosage of DAX-1, determining in sexual differentiation: Duplication of DAX-1 in two sisters with gonadal dysgenesis. Mol. Biol. Rep. 46 (3), 2971–2978. doi:10.1007/s11033-019-04758-y
Garcia-Alonso, L., Lorenzi, V., Mazzeo, C. I., Alves-Lopes, J. P., Roberts, K., and Sancho-Serra, C., et al. (2022). Single-cell roadmap of human gonadal development. Nature 607 (7919), 540–547. doi:10.1038/s41586-022-04918-4
Garcia-Moreno, S. A., Futtner, C. R., Salamone, I. M., Gonen, N., Lovell-Badge, R., and Maatouk, D. M. (2019). Gonadal supporting cells acquire sex-specific chromatin landscapes during mammalian sex determination. Dev. Biol. 446 (2), 168–179. doi:10.1016/j.ydbio.2018.12.023
Garcia-Ortiz, J. E., Pelosi, E., Omari, S., Nedorezov, T., Piao, Y., Karmazin, J., et al. (2009). Foxl2 functions in sex determination and histogenesis throughout mouse ovary development. BMC Dev. Biol. 9, 36. doi:10.1186/1471-213X-9-36
Gautier, A., Sohm, F., Joly, J. S., Le Gac, F., and Lareyre, J. J. (2011). The proximal promoter region of the zebrafish gsdf gene is sufficient to mimic the spatio-temporal expression pattern of the endogenous gene in Sertoli and granulosa cells. Biol. Reprod. 85 (6), 1240–1251. doi:10.1095/biolreprod.111.091892
George, F. W., Hodgins, M. B., and Wilson, J. D. (1985). The synthesis and metabolism of gonadal steroids in pouch young of the opossum, Didelphis virginiana. Endocrinology 116 (3), 1145–1150. doi:10.1210/endo-116-3-1145
George, F. W., and Wilson, J. D. (1982). Developmental pattern of increased aromatase activity in the Sebright bantam chicken. Endocrinology 110 (4), 1203–1207. doi:10.1210/endo-110-4-1203
George, F. W., and Wilson, J. D. (1980). Endocrine differentiation of the fetal rabbit ovary in culture. Nature 283 (5750), 861–863. doi:10.1038/283861a0
Georges, A., L'Hote, D., Todeschini, A. L., Auguste, A., Legois, B., Zider, A., et al. (2014). The transcription factor FOXL2 mobilizes estrogen signaling to maintain the identity of ovarian granulosa cells. Elife 3. doi:10.7554/eLife.04207
Gomes, N. L., de Paula, L. C. P., Silva, J. M., Silva, T. E., Lerário, A. M., Nishi, M. Y., et al. (2019). A 46, XX testicular disorder of sex development caused by a Wilms' tumour Factor-1 (WT1) pathogenic variant. Clin. Genet. 95 (1), 172–176. doi:10.1111/cge.13459
Gondos, B., George, F. W., and Wilson, J. D. (1983). Granulosa cell differentiation and estrogen synthesis in the fetal rabbit ovary. Biol. Reprod. 29 (3), 791–798. doi:10.1095/biolreprod29.3.791
Gondos, B. (1969). The ultrastructure of granulose cells in the newborn rabbit ovary. Anat. Rec. 165 (1), 67–77. doi:10.1002/ar.1091650108
Gondos, B., Westergaard, L., and Byskov, A. G. (1986). Initiation of oogenesis in the human fetal ovary: Ultrastructural and squash preparation study. Am. J. Obstet. Gynecol. 155 (1), 189–195. doi:10.1016/0002-9378(86)90109-2
Greco, T. L., and Payne, A. H. (1994). Ontogeny of expression of the genes for steroidogenic enzymes P450 side-chain cleavage, 3 beta-hydroxysteroid dehydrogenase, P450 17 alpha-hydroxylase/C17-20 lyase, and P450 aromatase in fetal mouse gonads. Endocrinology 135 (1), 262–268. doi:10.1210/endo.135.1.8013361
Guiguen, Y., Fostier, A., Piferrer, F., and Chang, C. F. (2010). Ovarian aromatase and estrogens: A pivotal role for gonadal sex differentiation and sex change in fish. Gen. Comp. Endocrinol. 165 (3), 352–366. doi:10.1016/j.ygcen.2009.03.002
Guioli, S., and Lovell-Badge, R. (2007). PITX2 controls asymmetric gonadal development in both sexes of the chick and can rescue the degeneration of the right ovary. Development 134 (23), 4199–4208. doi:10.1242/dev.010249
Guioli, S., Zhao, D., Nandi, S., Clinton, M., and Lovell-Badge, R. (2020). Oestrogen in the chick embryo can induce chromosomally male ZZ left gonad epithelial cells to form an ovarian cortex that can support oogenesis. Development 147 (4), dev181693. doi:10.1242/dev.181693
Gustin, S. E., Hogg, K., Stringer, J. M., Rastetter, R. H., Pelosi, E., Miles, D. C., et al. (2016). WNT/β-catenin and p27/FOXL2 differentially regulate supporting cell proliferation in the developing ovary. Dev. Biol. 412 (2), 250–260. doi:10.1016/j.ydbio.2016.02.024
Haniffa, M., Taylor, D., Linnarsson, S., Aronow, B. J., Bader, G. D., Barker, R. A., et al. (2021). A roadmap for the human developmental cell atlas. Nature 597 (7875), 196–205. doi:10.1038/s41586-021-03620-1
Hanley, N. A., Ball, S. G., Clement-Jones, M., Hagan, D. M., Strachan, T., Lindsay, S., et al. (1999). Expression of steroidogenic factor 1 and Wilms' tumour 1 during early human gonadal development and sex determination. Mech. Dev. 87 (1-2), 175–180. doi:10.1016/s0925-4773(99)00123-9
Hathi, D., Goswami, S., Sengupta, N., and Baidya, A. (2022). A novel homozygous CYP19A1 gene mutation causing aromatase deficiency. Cureus 14 (2), e22059. doi:10.7759/cureus.22059
Hayashi, T., Kageyama, Y., Ishizaka, K., Kihara, K., and Oshima, H. (2000). Sexual dimorphism in the regulation of meiotic process in the rabbit. Biol. Reprod. 62 (6), 1722–1727. doi:10.1095/biolreprod62.6.1722
Herpin, A., Adolfi, M. C., Nicol, B., Hinzmann, M., Schmidt, C., Klughammer, J., et al. (2013). Divergent expression regulation of gonad development genes in medaka shows incomplete conservation of the downstream regulatory network of vertebrate sex determination. Mol. Biol. Evol. 30 (10), 2328–2346. doi:10.1093/molbev/mst130
Hou, M., Feng, K., Luo, H., Jiang, Y., Xu, W., Li, Y., et al. (2022). Complete depletion of primordial germ cells results in masculinization of Monopterus albus, a protogynous hermaphroditic fish. Mar. Biotechnol. 24, 320–334. doi:10.1007/s10126-022-10106-2
Hsu, C.-w., and Chung, B.-c. (2021). Evolution, expression, and function of gonadal somatic cell-derived factor. Front. Cell Dev. Biol. 9, 684352. doi:10.3389/fcell.2021.684352
Hummitzsch, K., Irving-Rodgers, H. F., Hatzirodos, N., Bonner, W., Sabatier, L., Reinhardt, D. P., et al. (2013). A new model of development of the mammalian ovary and follicles. PLoS One 8 (2), e55578. doi:10.1371/journal.pone.0055578
Ikeda, Y., Shen, W. H., Ingraham, H. A., and Parker, K. L. (1994). Developmental expression of mouse steroidogenic factor-1, an essential regulator of the steroid hydroxylases. Mol. Endocrinol. 8 (5), 654–662. doi:10.1210/mend.8.5.8058073
Ioannidis, J., Taylor, G., Zhao, D., Liu, L., Idoko-Akoh, A., Gong, D., et al. (2021). Primary sex determination in birds depends on DMRT1 dosage, but gonadal sex does not determine adult secondary sex characteristics. Proc. Natl. Acad. Sci. U. S. A. 118 (10), e2020909118. doi:10.1073/pnas.2020909118
Ishimaru, Y., Komatsu, T., Kasahara, M., Katoh-Fukui, Y., Ogawa, H., Toyama, Y., et al. (2008). Mechanism of asymmetric ovarian development in chick embryos. Development 135 (4), 677–685. doi:10.1242/dev.012856
James, R. G., Kamei, C. N., Wang, Q., Jiang, R., and Schultheiss, T. M. (2006). Odd-skipped related 1 is required for development of the metanephric kidney and regulates formation and differentiation of kidney precursor cells. Development 133 (15), 2995–3004. doi:10.1242/dev.02442
Jameson, S. A., Natarajan, A., Cool, J., DeFalco, T., Maatouk, D. M., Mork, L., et al. (2012). Temporal transcriptional profiling of somatic and germ cells reveals biased lineage priming of sexual fate in the fetal mouse gonad. PLoS Genet. 8 (3), e1002575. doi:10.1371/journal.pgen.1002575
Jefferson, W. N., Couse, J. F., Banks, E. P., Korach, K. S., and Newbold, R. R. (2000). Expression of estrogen receptor beta is developmentally regulated in reproductive tissues of male and female mice. Biol. Reprod. 62 (2), 310–317. doi:10.1095/biolreprod62.2.310
Jolivet, G., Daniel-Carlier, N., Harscoet, E., Airaud, E., Dewaele, A., Pierson, C., et al. (2022). Fetal estrogens are not involved in sex determination but critical for early ovarian differentiation in rabbits. Endocrinology 163 (1), bqab210. doi:10.1210/endocr/bqab210
Jordan, B. K., Mohammed, M., Ching, S. T., Délot, E., Chen, X. N., Dewing, P., et al. (2001). Up-regulation of WNT-4 signaling and dosage-sensitive sex reversal in humans. Am. J. Hum. Genet. 68 (5), 1102–1109. doi:10.1086/320125
Jordan, B. K., Shen, J. H., Olaso, R., Ingraham, H. A., and Vilain, E. (2003). Wnt4 overexpression disrupts normal testicular vasculature and inhibits testosterone synthesis by repressing steroidogenic factor 1/beta-catenin synergy. Proc. Natl. Acad. Sci. U. S. A. 100 (19), 10866–10871. doi:10.1073/pnas.1834480100
Jovelin, R., Yan, Y. L., He, X., Catchen, J., Amores, A., Canestro, C., et al. (2010). Evolution of developmental regulation in the vertebrate FgfD subfamily. J. Exp. Zool. B Mol. Dev. Evol. 314 (1), 33–56. doi:10.1002/jez.b.21307
Juengel, J. L., Sawyer, H. R., Smith, P. R., Quirke, L. D., Heath, D. A., Lun, S., et al. (2002). Origins of follicular cells and ontogeny of steroidogenesis in ovine fetal ovaries. Mol. Cell. Endocrinol. 191 (1), 1–10. doi:10.1016/s0303-7207(02)00045-x
Kaufman, O. H., Lee, K., Martin, M., Rothhämel, S., and Marlow, F. L. (2018). rbpms2 functions in Balbiani body architecture and ovary fate. PLoS Genet. 14 (7), e1007489. doi:10.1371/journal.pgen.1007489
Kayo, D., Zempo, B., Tomihara, S., Oka, Y., and Kanda, S. (2019). Gene knockout analysis reveals essentiality of estrogen receptor β1 (Esr2a) for female reproduction in medaka. Sci. Rep. 9 (1), 8868. doi:10.1038/s41598-019-45373-y
Kenngott, R. A., Vermehren, M., Ebach, K., and Sinowatz, F. (2013). The role of ovarian surface epithelium in folliculogenesis during fetal development of the bovine ovary: A histological and immunohistochemical study. Sex. Dev. 7 (4), 180–195. doi:10.1159/000348881
Kim, Y., Kobayashi, A., Sekido, R., DiNapoli, L., Brennan, J., Chaboissier, M. C., et al. (2006). Fgf9 and Wnt4 act as antagonistic signals to regulate mammalian sex determination. PLoS Biol. 4 (6), e187. doi:10.1371/journal.pbio.0040187
Kobayashi, H., and Iwamatsu, T. (2005). Sex reversal in the medaka Oryzias latipes by brief exposure of early embryos to estradiol-17beta. Zool. Sci. 22 (10), 1163–1167. doi:10.2108/zsj.22.1163
Kocer, A., Pinheiro, I., Pannetier, M., Renault, L., Parma, P., Radi, O., et al. (2008). R-spondin1 and FOXL2 act into two distinct cellular types during goat ovarian differentiation. BMC Dev. Biol. 8, 36. doi:10.1186/1471-213X-8-36
Koizumi, M., Oyama, K., Yamakami, Y., Kida, T., Satoh, R., Kato, S., et al. (2015). Lgr4 controls specialization of female gonads in mice. Biol. Reprod. 93 (4), 90. doi:10.1095/biolreprod.114.123638
Koopman, P., Gubbay, J., Vivian, N., Goodfellow, P., and Lovell-Badge, R. (1991). Male development of chromosomally female mice transgenic for Sry. Nature 351 (6322), 117–121. doi:10.1038/351117a0
Kossack, M. E., and Draper, B. W. (2019). Genetic regulation of sex determination and maintenance in zebrafish (Danio rerio). Curr. Top. Dev. Biol. 134, 119–149. doi:10.1016/bs.ctdb.2019.02.004
Kossack, M. E., High, S. K., Hopton, R. E., Yan, Y. L., Postlethwait, J. H., and Draper, B. W. (2019). Female sex development and reproductive duct formation depend on Wnt4a in zebrafish. Genetics 211 (1), 219–233. doi:10.1534/genetics.118.301620
Kurokawa, H., Saito, D., Nakamura, S., Katoh-Fukui, Y., Ohta, K., Baba, T., et al. (2007). Germ cells are essential for sexual dimorphism in the medaka gonad. Proc. Natl. Acad. Sci. U. S. A. 104 (43), 16958–16963. doi:10.1073/pnas.0609932104
Kwon, J. Y., Haghpanah, V., Kogson-Hurtado, L. M., McAndrew, B. J., and Penman, D. J. (2000). Masculinization of genetic female nile tilapia (Oreochromis niloticus) by dietary administration of an aromatase inhibitor during sexual differentiation. J. Exp. Zool. 287 (1), 46–53. doi:10.1002/1097-010x(20000615)287:1<46::aid-jez6>3.0.co;2-x
Lambeth, L. S., Cummins, D. M., Doran, T. J., Sinclair, A. H., and Smith, C. A. (2013). Overexpression of aromatase alone is sufficient for ovarian development in genetically male chicken embryos. PLoS One 8 (6), e68362. doi:10.1371/journal.pone.0068362
Lambeth, L. S., Morris, K. R., Wise, T. G., Cummins, D. M., O'Neil, T. E., Cao, Y., et al. (2016). Transgenic chickens overexpressing aromatase have high estrogen levels but maintain a predominantly male phenotype. Endocrinology 157 (1), 83–90. doi:10.1210/en.2015-1697
Lambeth, L. S., Raymond, C. S., Roeszler, K. N., Kuroiwa, A., Nakata, T., Zarkower, D., et al. (2014). Over-expression of DMRT1 induces the male pathway in embryonic chicken gonads. Dev. Biol. 389 (2), 160–172. doi:10.1016/j.ydbio.2014.02.012
Lang-Muritano, M., Sproll, P., Wyss, S., Kolly, A., Hürlimann, R., Konrad, D., et al. (2018). Early-onset complete ovarian failure and lack of puberty in a woman with mutated estrogen receptor β (ESR2). J. Clin. Endocrinol. Metab. 103 (10), 3748–3756. doi:10.1210/jc.2018-00769
Lau, E. S., Zhang, Z., Qin, M., and Ge, W. (2016). Knockout of zebrafish ovarian aromatase gene (cyp19a1a) by TALEN and CRISPR/Cas9 leads to all-male offspring due to failed ovarian differentiation. Sci. Rep. 6, 37357. doi:10.1038/srep37357
Lavery, R., Chassot, A. A., Pauper, E., Gregoire, E. P., Klopfenstein, M., de Rooij, D. G., et al. (2012). Testicular differentiation occurs in absence of R-spondin1 and Sox9 in mouse sex reversals. PLoS Genet. 8 (12), e1003170. doi:10.1371/journal.pgen.1003170
Lee, H. J., Seo, M., Choi, H. J., Rengaraj, D., Jung, K. M., and Park, J. S. (2021). DMRT1 gene disruption alone induces incomplete gonad feminization in chicken. Faseb J. 35 (9), e21876. doi:10.1096/fj.202100902R
Lecluze, E., Rolland, A. D., Filis, P., Evrard, B., Leverrier-Penna, S., Maamar, M. B., et al. (2020). Dynamics of the transcriptional landscape during human fetal testis and ovary development. Hum. Reprod. 35 (5), 1099–1119. doi:10.1093/humrep/deaa041
Ledda, S., Bogliolo, L., Bebbere, D., Ariu, F., and Pirino, S. (2010). Characterization, isolation and culture of primordial germ cells in domestic animals: Recent progress and insights from the ovine species. Theriogenology 74 (4), 534–543. doi:10.1016/j.theriogenology.2010.05.011
Leerberg, D. M., Sano, K., and Draper, B. W. (2017). Fibroblast growth factor signaling is required for early somatic gonad development in zebrafish. PLoS Genet. 13 (9), e1006993. doi:10.1371/journal.pgen.1006993
Lemmen, J. G., Broekhof, J. L., Kuiper, G. G., Gustafsson, J. A., van der Saag, P. T., and van der Burg, B. (1999). Expression of estrogen receptor alpha and beta during mouse embryogenesis. Mech. Dev. 81 (1-2), 163–167. doi:10.1016/s0925-4773(98)00223-8
Li, M. H., Yang, H. H., Li, M. R., Sun, Y. L., Jiang, X. L., Xie, Q. P., et al. (2013). Antagonistic roles of Dmrt1 and Foxl2 in sex differentiation via estrogen production in tilapia as demonstrated by TALENs. Endocrinology 154 (12), 4814–4825. doi:10.1210/en.2013-1451
Li, M., Sun, L., and Wang, D. (2019). Roles of estrogens in fish sexual plasticity and sex differentiation. Gen. Comp. Endocrinol. 277, 9–16. doi:10.1016/j.ygcen.2018.11.015
Li, M., Sun, Y., Zhao, J., Shi, H., Zeng, S., Ye, K., et al. (2015). A tandem duplicate of anti-müllerian hormone with a missense SNP on the Y chromosome is essential for male sex determination in nile Tilapia, Oreochromis niloticus. PLoS Genet. 11 (11), e1005678. doi:10.1371/journal.pgen.1005678
Liu, C. F., Bingham, N., Parker, K., and Yao, H. H. (2009). Sex-specific roles of beta-catenin in mouse gonadal development. Hum. Mol. Genet. 18 (3), 405–417. doi:10.1093/hmg/ddn362
Liu, C., Peng, J., Matzuk, M. M., and Yao, H. H. (2015). Lineage specification of ovarian theca cells requires multicellular interactions via oocyte and granulosa cells. Nat. Commun. 6, 6934. doi:10.1038/ncomms7934
Liu, J., Liu, T., Niu, J., Wu, X., Zhai, J., Zhang, Q., et al. (2018). Expression pattern and functional analysis of R-spondin1 in tongue sole Cynoglossus semilaevis. Gene 642, 453–460. doi:10.1016/j.gene.2017.11.047
Loffler, K. A., Zarkower, D., and Koopman, P. (2003). Etiology of ovarian failure in blepharophimosis ptosis epicanthus inversus syndrome: FOXL2 is a conserved, early-acting gene in vertebrate ovarian development. Endocrinology 144 (7), 3237–3243. doi:10.1210/en.2002-0095
Lu, H., Cui, Y., Jiang, L., and Ge, W. (2017). Functional analysis of nuclear estrogen receptors in zebrafish reproduction by genome editing approach. Endocrinology 158 (7), 2292–2308. doi:10.1210/en.2017-00215
Ludbrook, L. M., Bernard, P., Bagheri-Fam, S., Ryan, J., Sekido, R., Wilhelm, D., et al. (2012). Excess DAX1 leads to XY ovotesticular disorder of sex development (DSD) in mice by inhibiting steroidogenic factor-1 (SF1) activation of the testis enhancer of SRY-box-9 (Sox9). Endocrinology 153 (4), 1948–1958. doi:10.1210/en.2011-1428
Maatouk, D. M., DiNapoli, L., Alvers, A., Parker, K. L., Taketo, M. M., and Capel, B. (2008). Stabilization of beta-catenin in XY gonads causes male-to-female sex-reversal. Hum. Mol. Genet. 17 (19), 2949–2955. doi:10.1093/hmg/ddn193
Maatouk, D. M., Mork, L., Chassot, A. A., Chaboissier, M. C., and Capel, B. (2013). Disruption of mitotic arrest precedes precocious differentiation and transdifferentiation of pregranulosa cells in the perinatal Wnt4 mutant ovary. Dev. Biol. 383 (2), 295–306. doi:10.1016/j.ydbio.2013.08.026
Maatouk, D. M., Mork, L., Hinson, A., Kobayashi, A., McMahon, A. P., and Capel, B. (2012). Germ cells are not required to establish the female pathway in mouse fetal gonads. PLoS One 7 (10), e47238. doi:10.1371/journal.pone.0047238
Mahadevaiah, S. K., Sangrithi, M. N., Hirota, T., and Turner, J. M. A. (2020). A single-cell transcriptome atlas of marsupial embryogenesis and X inactivation. Nature 586 (7830), 612–617. doi:10.1038/s41586-020-2629-6
Major, A. T., Ayers, K., Chue, J., Roeszler, K., and Smith, C. (2019). FOXL2 antagonises the male developmental pathway in embryonic chicken gonads. J. Endocrinol. 243, 211–228. doi:10.1530/joe-19-0277
Mamsen, L. S., Ernst, E. H., Borup, R., Larsen, A., Olesen, R. H., Ernst, E., et al. (2017). Temporal expression pattern of genes during the period of sex differentiation in human embryonic gonads. Sci. Rep. 7 (1), 15961. doi:10.1038/s41598-017-15931-3
Mandel, H., Shemer, R., Borochowitz, Z. U., Okopnik, M., Knopf, C., Indelman, M., et al. (2008). SERKAL syndrome: An autosomal-recessive disorder caused by a loss-of-function mutation in WNT4. Am. J. Hum. Genet. 82 (1), 39–47. doi:10.1016/j.ajhg.2007.08.005
Manuylov, N. L., Smagulova, F. O., Leach, L., and Tevosian, S. G. (2008). Ovarian development in mice requires the GATA4-FOG2 transcription complex. Development 135 (22), 3731–3743. doi:10.1242/dev.024653
Mario, L. C., Borghesi, J., de Almeida da Anunciacao, A. R., de Carvalho Figueiredo Miranda, C. M., César dos Santos, A., Oliveira Favaron, P., et al. (2018). Sexual determination and differentiation during embryonic and fetal development of New Zealand rabbit females. Int. J. Morphol. 36 (2), 677–686. doi:10.4067/s0717-95022018000200677
Matsuda, M., Nagahama, Y., Shinomiya, A., Sato, T., Matsuda, C., Kobayashi, T., et al. (2002). DMY is a Y-specific DM-domain gene required for male development in the medaka fish. Nature 417 (6888), 559–563. doi:10.1038/nature751
Mazen, I., McElreavey, K., Elaidy, A., Kamel, A. K., and Abdel-Hamid, M. S. (2017). Aromatase deficiency due to a homozygous CYP19A1 mutation in a 46, XX Egyptian patient with ambiguous genitalia. Sex. Dev. 11 (5-6), 275–279. doi:10.1159/000485278
McNatty, K. P., Smith, P., Hudson, N. L., Heath, D. A., Tisdall, D. J., O, W. S., et al. (1995). Development of the sheep ovary during fetal and early neonatal life and the effect of fecundity genes. J. Reprod. Fertil. Suppl. 49, 123–135.
Menke, D. B., Koubova, J., and Page, D. C. (2003). Sexual differentiation of germ cells in XX mouse gonads occurs in an anterior-to-posterior wave. Dev. Biol. 262 (2), 303–312. doi:10.1016/s0012-1606(03)00391-9
Meyer, A., and Van de Peer, Y. (2005). From 2R to 3R: Evidence for a fish-specific genome duplication (FSGD). Bioessays 27 (9), 937–945. doi:10.1002/bies.20293
Miyado, M., Inui, M., Igarashi, M., Katoh-Fukui, Y., Takasawa, K., Hakoda, A., et al. (2016). The p.R92W variant of nr5a1/nr5a1 induces testicular development of 46, XX gonads in humans, but not in mice: Phenotypic comparison of human patients and mutation-induced mice. Biol. Sex. Differ. 7, 56. doi:10.1186/s13293-016-0114-6
Moritz, K. M., and Wintour, E. M. (1999). Functional development of the meso- and metanephros. Pediatr. Nephrol. 13 (2), 171–178. doi:10.1007/s004670050587
Mork, L., Maatouk, D. M., McMahon, J. A., Guo, J. J., Zhang, P., McMahon, A. P., et al. (2012). Temporal differences in granulosa cell specification in the ovary reflect distinct follicle fates in mice. Biol. Reprod. 86 (2), 37. doi:10.1095/biolreprod.111.095208
Motta, P. M., and Makabe, S. (1982). Development of the ovarian surface and associated germ cells in the human fetus. A correlated study by scanning and transmission electron microscopy. Cell Tissue Res. 226 (3), 493–510. doi:10.1007/bf00214779
Munger, S. C., Natarajan, A., Looger, L. L., Ohler, U., and Capel, B. (2013). Fine time course expression analysis identifies cascades of activation and repression and maps a putative regulator of mammalian sex determination. PLoS Genet. 9 (7), e1003630. doi:10.1371/journal.pgen.1003630
Naasse, Y., Bakhchane, A., Charoute, H., Jennane, F., Bignon-Topalovic, J., Malki, A., et al. (2017). A novel homozygous missense mutation in the FU-CRD2 domain of the R-spondin1 gene associated with familial 46, XX DSD. Sex. Dev. 11 (5-6), 269–274. doi:10.1159/000485393
Nagahama, Y., Chakraborty, T., Paul-Prasanth, B., Ohta, K., and Nakamura, M. (2021). Sex determination, gonadal sex differentiation, and plasticity in vertebrate species. Physiol. Rev. 101 (3), 1237–1308. doi:10.1152/physrev.00044.2019
Nakagawa, R., Takasawa, K., Gau, M., Tsuji-Hosokawa, A., Kawaji, H., Murakawa, Y., et al. (2022). Two ovarian candidate enhancers, identified by time series enhancer RNA analyses, harbor rare genetic variations identified in ovarian insufficiency. Hum. Mol. Genet. 31, 2223–2235. doi:10.1093/hmg/ddac023
Nakamoto, M., Matsuda, M., Wang, D. S., Nagahama, Y., and Shibata, N. (2006). Molecular cloning and analysis of gonadal expression of Foxl2 in the medaka, Oryzias latipes. Biochem. Biophys. Res. Commun. 344 (1), 353–361. doi:10.1016/j.bbrc.2006.03.137
Nakamoto, M., Shibata, Y., Ohno, K., Usami, T., Kamei, Y., Taniguchi, Y., et al. (2018). Ovarian aromatase loss-of-function mutant medaka undergo ovary degeneration and partial female-to-male sex reversal after puberty. Mol. Cell. Endocrinol. 460, 104–122. doi:10.1016/j.mce.2017.07.013
Nakamura, S., Aoki, Y., Saito, D., Kuroki, Y., Fujiyama, A., Naruse, K., et al. (2008). Sox9b/sox9a2-EGFP transgenic medaka reveals the morphological reorganization of the gonads and a common precursor of both the female and male supporting cells. Mol. Reprod. Dev. 75 (3), 472–476. doi:10.1002/mrd.20764
Nakamura, S., Kobayashi, D., Aoki, Y., Yokoi, H., Ebe, Y., Wittbrodt, J., et al. (2006). Identification and lineage tracing of two populations of somatic gonadal precursors in medaka embryos. Dev. Biol. 295 (2), 678–688. doi:10.1016/j.ydbio.2006.03.052
Nakamura, S., Kobayashi, K., Nishimura, T., Higashijima, S., and Tanaka, M. (2010). Identification of germline stem cells in the ovary of the teleost medaka. Science 328 (5985), 1561–1563. doi:10.1126/science.1185473
Nakamura, S., Kurokawa, H., Asakawa, S., Shimizu, N., and Tanaka, M. (2009). Two distinct types of theca cells in the medaka gonad: Germ cell-dependent maintenance of cyp19a1-expressing theca cells. Dev. Dyn. 238 (10), 2652–2657. doi:10.1002/dvdy.22068
Nanda, I., Kondo, M., Hornung, U., Asakawa, S., Winkler, C., Shimizu, A., et al. (2002). A duplicated copy of DMRT1 in the sex-determining region of the Y chromosome of the medaka, Oryzias latipes. Proc. Natl. Acad. Sci. U. S. A. 99 (18), 11778–11783. doi:10.1073/pnas.182314699
Nef, S., Schaad, O., Stallings, N. R., Cederroth, C. R., Pitetti, J. L., Schaer, G., et al. (2005). Gene expression during sex determination reveals a robust female genetic program at the onset of ovarian development. Dev. Biol. 287 (2), 361–377. doi:10.1016/j.ydbio.2005.09.008
Nicol, B., Grimm, S. A., Chalmel, F., Lecluze, E., Pannetier, M., Pailhoux, E., et al. (2019). RUNX1 maintains the identity of the fetal ovary through an interplay with FOXL2. Nat. Commun. 10 (1), 5116. doi:10.1038/s41467-019-13060-1
Nicol, B., Grimm, S. A., Gruzdev, A., Scott, G. J., Ray, M. K., and Yao, H. H. (2018). Genome-wide identification of FOXL2 binding and characterization of FOXL2 feminizing action in the fetal gonads. Hum. Mol. Genet. 27 (24), 4273–4287. doi:10.1093/hmg/ddy312
Nicol, B., and Yao, H. H. (2015). Gonadal identity in the absence of pro-testis factor SOX9 and pro-ovary factor beta-catenin in mice. Biol. Reprod. 93 (2), 35. doi:10.1095/biolreprod.115.131276
Nishikimi, H., Kansaku, N., Saito, N., Usami, M., Ohno, Y., and Shimada, K. (2000). Sex differentiation and mRNA expression of P450c17, P450arom and AMH in gonads of the chicken. Mol. Reprod. Dev. 55 (1), 20–30. doi:10.1002/(SICI)1098-2795(200001)55:1<20::AID-MRD4>3.0.CO;2-E
Nishimura, T., Nakamura, S., and Tanaka, M. (2016). A structurally and functionally common unit in testes and ovaries of medaka (Oryzias latipes), a teleost fish. Sex. Dev. 10 (3), 159–165. doi:10.1159/000447313
Nishimura, T., Sato, T., Yamamoto, Y., Watakabe, I., Ohkawa, Y., Suyama, M., et al. (2015). Sex determination. foxl3 is a germ cell-intrinsic factor involved in sperm-egg fate decision in medaka. Science 349 (6245), 328–331. doi:10.1126/science.aaa2657
Nishimura, T., and Tanaka, M. (2014). Gonadal development in fish. Sex. Dev. 8 (5), 252–261. doi:10.1159/000364924
Nishimura, T., Yamada, K., Fujimori, C., Kikuchi, M., Kawasaki, T., Siegfried, K. R., et al. (2018). Germ cells in the teleost fish medaka have an inherent feminizing effect. PLoS Genet. 14 (3), e1007259. doi:10.1371/journal.pgen.1007259
Niu, W., and Spradling, A. C. (2020). Two distinct pathways of pregranulosa cell differentiation support follicle formation in the mouse ovary. Proc. Natl. Acad. Sci. U. S. A. 117 (33), 20015–20026. doi:10.1073/pnas.2005570117
Nomura, O., Nakabayashi, O., Nishimori, K., Yasue, H., and Mizuno, S. (1999). Expression of five steroidogenic genes including aromatase gene at early developmental stages of chicken male and female embryos. J. Steroid Biochem. Mol. Biol. 71 (3-4), 103–109. doi:10.1016/s0960-0760(99)00127-2
Ottolenghi, C., Omari, S., Garcia-Ortiz, J. E., Uda, M., Crisponi, L., Forabosco, A., et al. (2005). Foxl2 is required for commitment to ovary differentiation. Hum. Mol. Genet. 14 (14), 2053–2062. doi:10.1093/hmg/ddi210
Ottolenghi, C., Pelosi, E., Tran, J., Colombino, M., Douglass, E., Nedorezov, T., et al. (2007). Loss of Wnt4 and Foxl2 leads to female-to-male sex reversal extending to germ cells. Hum. Mol. Genet. 16 (23), 2795–2804. doi:10.1093/hmg/ddm235
Pailhoux, E., Vigier, B., Chaffaux, S., Servel, N., Taourit, S., Furet, J. P., et al. (2001). A 11.7-kb deletion triggers intersexuality and polledness in goats. Nat. Genet. 29 (4), 453–458. doi:10.1038/ng769
Pailhoux, E., Vigier, B., Vaiman, D., Servel, N., Chaffaux, S., Cribiu, E. P., et al. (2002). Ontogenesis of female-to-male sex-reversal in XX polled goats. Dev. Dyn. 224 (1), 39–50. doi:10.1002/dvdy.10083
Pannetier, M., Elzaiat, M., Thépot, D., and Pailhoux, E. (2012). Telling the story of XX sex reversal in the goat: Highlighting the sex-crossroad in domestic mammals. Sex. Dev. 6 (1-3), 33–45. doi:10.1159/000334056
Pannetier, M., Fabre, S., Batista, F., Kocer, A., Renault, L., Jolivet, G., et al. (2006). FOXL2 activates P450 aromatase gene transcription: Towards a better characterization of the early steps of mammalian ovarian development. J. Mol. Endocrinol. 36 (3), 399–413. doi:10.1677/jme.1.01947
Parma, P., Radi, O., Vidal, V., Chaboissier, M. C., Dellambra, E., Valentini, S., et al. (2006). R-spondin1 is essential in sex determination, skin differentiation and malignancy. Nat. Genet. 38 (11), 1304–1309. doi:10.1038/ng1907
Pask, A. J., Calatayud, N. E., Shaw, G., Wood, W. M., and Renfree, M. B. (2010). Oestrogen blocks the nuclear entry of SOX9 in the developing gonad of a marsupial mammal. BMC Biol. 8, 113. doi:10.1186/1741-7007-8-113
Paul-Prasanth, B., Bhandari, R. K., Kobayashi, T., Horiguchi, R., Kobayashi, Y., Nakamoto, M., et al. (2013). Estrogen oversees the maintenance of the female genetic program in terminally differentiated gonochorists. Sci. Rep. 3, 2862. doi:10.1038/srep02862
Praveen, V. P., Ladjouze, A., Sauter, K. S., Pulickal, A., Katharopoulos, E., Trippel, M., et al. (2020). Novel CYP19A1 mutations extend the genotype-phenotype correlation and reveal the impact on ovarian function. J. Endocr. Soc. 4 (4), bvaa030. doi:10.1210/jendso/bvaa030
Qin, M., Xie, Q., Wu, K., Zhou, X., and Ge, W. (2022). Loss of Nobox prevents ovarian differentiation from juvenile ovaries in zebrafish. Biol. Reprod. 106, 1254–1266. doi:10.1093/biolre/ioac036
Quaynor, S. D., Stradtman, E. W., Kim, H. G., Shen, Y., Chorich, L. P., Schreihofer, D. A., et al. (2013). Delayed puberty and estrogen resistance in a woman with estrogen receptor α variant. N. Engl. J. Med. 369 (2), 164–171. doi:10.1056/NEJMoa1303611
Ramanagoudr-Bhojappa, R., Carrington, B., Ramaswami, M., Bishop, K., Robbins, G. M., Jones, M., et al. (2018). Multiplexed CRISPR/Cas9-mediated knockout of 19 Fanconi anemia pathway genes in zebrafish revealed their roles in growth, sexual development and fertility. PLoS Genet. 14 (12), e1007821. doi:10.1371/journal.pgen.1007821
Rastetter, R. H., Bernard, P., Palmer, J. S., Chassot, A. A., Chen, H., Western, P. S., et al. (2014). Marker genes identify three somatic cell types in the fetal mouse ovary. Dev. Biol. 394 (2), 242–252. doi:10.1016/j.ydbio.2014.08.013
Renfree, M. B., O, W. S., Short, R. V., and Shaw, G. (1996). Sexual differentiation of the urogenital system of the fetal and neonatal tammar wallaby, Macropus eugenii. Anat. Embryol. 194 (2), 111–134. doi:10.1007/bf00195006
Renfree, M. B., and Short, R. V. (1988). Sex determination in marsupials: Evidence for a marsupial-eutherian dichotomy. Philos. Trans. R. Soc. Lond. B Biol. Sci. 322 (1208), 41–53. doi:10.1098/rstb.1988.0112
Renfree, M. B., Wilson, J. D., Short, R. V., Shaw, G., and George, F. W. (1992). Steroid hormone content of the gonads of the tammar wallaby during sexual differentiation. Biol. Reprod. 47 (4), 644–647. doi:10.1095/biolreprod47.4.644
Rhen, T., Even, Z., Brenner, A., Lodewyk, A., Das, D., Singh, S., et al. (2021). Evolutionary turnover in wnt gene expression but conservation of wnt signaling during ovary determination in a TSD reptile. Sex. Dev. 15 (1-3), 47–68. doi:10.1159/000516973
Rodemer, E. S., Ihmer, A., and Wartenberg, H. (1986). Gonadal development of the chick embryo following microsurgically caused agenesis of the mesonephros and using interspecific quail-chick chimaeras. Development 98, 269–285. doi:10.1242/dev.98.1.269
Rodríguez-León, J., Rodríguez Esteban, C., Martí, M., Santiago-Josefat, B., Dubova, I., Rubiralta, X., et al. (2008). Pitx2 regulates gonad morphogenesis. Proc. Natl. Acad. Sci. U. S. A. 105 (32), 11242–11247. doi:10.1073/pnas.0804904105
Rodríguez-Marí, A., Cañestro, C., Bremiller, R. A., Nguyen-Johnson, A., Asakawa, K., Kawakami, K., et al. (2010). Sex reversal in zebrafish fancl mutants is caused by Tp53-mediated germ cell apoptosis. PLoS Genet. 6 (7), e1001034. doi:10.1371/journal.pgen.1001034
Rodríguez-Marí, A., Yan, Y. L., Bremiller, R. A., Wilson, C., Cañestro, C., and Postlethwait, J. H. (2005). Characterization and expression pattern of zebrafish Anti-Müllerian hormone (Amh) relative to sox9a, sox9b, and cyp19a1a, during gonad development. Gene Expr. Patterns 5 (5), 655–667. doi:10.1016/j.modgep.2005.02.008
Sasaki, K., Oguchi, A., Cheng, K., Murakawa, Y., Okamoto, I., Ohta, H., et al. (2021). The embryonic ontogeny of the gonadal somatic cells in mice and monkeys. Cell Rep. 35 (5), 109075. doi:10.1016/j.celrep.2021.109075
Satoh, N., and Egami, N. (1972). Sex differentiation of germ cells in the teleost, Oryzias latipes, during normal embryonic development. Development 28 (2), 385–395. doi:10.1242/dev.28.2.385
Sawyer, H. R., Smith, P., Heath, D. A., Juengel, J. L., Wakefield, S. J., and McNatty, K. P. (2002). Formation of ovarian follicles during fetal development in sheep. Biol. Reprod. 66 (4), 1134–1150. doi:10.1095/biolreprod66.4.1134
Schmidt, D., Ovitt, C. E., Anlag, K., Fehsenfeld, S., Gredsted, L., Treier, A. C., et al. (2004). The murine winged-helix transcription factor Foxl2 is required for granulosa cell differentiation and ovary maintenance. Development 131 (4), 933–942. doi:10.1242/dev.00969
Sekido, R., and Lovell-Badge, R. (2007). Mechanisms of gonadal morphogenesis are not conserved between chick and mouse. Dev. Biol. 302 (1), 132–142. doi:10.1016/j.ydbio.2006.09.007
Sekido, R., and Lovell-Badge, R. (2008). Sex determination involves synergistic action of SRY and SF1 on a specific Sox9 enhancer. Nature 453 (7197), 930–934. doi:10.1038/nature06944
Shaw, G., Renfree, M. B., Short, R. V., and O, W. S. (1988). Experimental manipulation of sexual differentiation in wallaby pouch young treated with exogenous steroids. Development 104 (4), 689–701. doi:10.1242/dev.104.4.689
Shioda, K., Odajima, J., Kobayashi, M., Kobayashi, M., Cordazzo, B., Isselbacher, K. J., et al. (2021). Transcriptomic and epigenetic preservation of genetic sex identity in estrogen-feminized male chicken embryonic gonads. Endocrinology 162 (1), bqaa208. doi:10.1210/endocr/bqaa208
Shozu, M., Akasofu, K., Harada, T., and Kubota, Y. (1991). A new cause of female pseudohermaphroditism: Placental aromatase deficiency. J. Clin. Endocrinol. Metab. 72 (3), 560–566. doi:10.1210/jcem-72-3-560
Siegfried, K. R., and Nüsslein-Volhard, C. (2008). Germ line control of female sex determination in zebrafish. Dev. Biol. 324 (2), 277–287. doi:10.1016/j.ydbio.2008.09.025
Sinclair, A. H., Berta, P., Palmer, M. S., Hawkins, J. R., Griffiths, B. L., Smith, M. J., et al. (1990). A gene from the human sex-determining region encodes a protein with homology to a conserved DNA-binding motif. Nature 346 (6281), 240–244. doi:10.1038/346240a0
Singh, N., Singh, D., Bhide, A., Sharma, R., Sahoo, S., Jolly, M. K., et al. (2022). Lhx2 in germ cells suppresses endothelial cell migration in the developing ovary. Exp. Cell Res. 415 (1), 113108. doi:10.1016/j.yexcr.2022.113108
Sirokha, D., Gorodna, O., Vitrenko, Y., Zelinska, N., Ploski, R., Nef, S., et al. (2021). A novel WT1 mutation identified in a 46, XX testicular/ovotesticular DSD patient results in the retention of intron 9. Biol. (Basel) 10 (12), 1248. doi:10.3390/biology10121248
Slanchev, K., Stebler, J., de la Cueva-Mendez, G., and Raz, E. (2005). Development without germ cells: The role of the germ line in zebrafish sex differentiation. Proc. Natl. Acad. Sci. U. S. A. 102 (11), 4074–4079. doi:10.1073/pnas.0407475102(
Smith, C. A., Andrews, J. E., and Sinclair, A. H. (1997). Gonadal sex differentiation in chicken embryos: Expression of estrogen receptor and aromatase genes. J. Steroid Biochem. Mol. Biol. 60 (5-6), 295–302. doi:10.1016/s0960-0760(96)00196-3
Smith, C. A., Katz, M., and Sinclair, A. H. (2003). DMRT1 is upregulated in the gonads during female-to-male sex reversal in ZW chicken embryos. Biol. Reprod. 68 (2), 560–570. doi:10.1095/biolreprod.102.007294
Smith, C. A., Roeszler, K. N., Bowles, J., Koopman, P., and Sinclair, A. H. (2008a). Onset of meiosis in the chicken embryo; evidence of a role for retinoic acid. BMC Dev. Biol. 8, 85. doi:10.1186/1471-213x-8-85
Smith, C. A., Roeszler, K. N., Ohnesorg, T., Cummins, D. M., Farlie, P. G., Doran, T. J., et al. (2009). The avian Z-linked gene DMRT1 is required for male sex determination in the chicken. Nature 461 (7261), 267–271. doi:10.1038/nature08298
Smith, C. A., Shoemaker, C. M., Roeszler, K. N., Queen, J., Crews, D., and Sinclair, A. H. (2008b). Cloning and expression of R-Spondin1 in different vertebrates suggests a conserved role in ovarian development. BMC Dev. Biol. 8, 72. doi:10.1186/1471-213x-8-72
Smith, K. K., and Keyte, A. L. (2020). Adaptations of the marsupial newborn: Birth as an extreme environment. Anat. Rec. 303 (2), 235–249. doi:10.1002/ar.24049
Sone, R., Taimatsu, K., Ohga, R., Nishimura, T., Tanaka, M., and Kawahara, A. (2020). Critical roles of the ddx5 gene in zebrafish sex differentiation and oocyte maturation. Sci. Rep. 10 (1), 14157. doi:10.1038/s41598-020-71143-2
Song, Y., Hu, W., and Ge, W. (2021). Establishment of transgenic zebrafish (Danio rerio) models expressing fluorescence proteins in the oocytes and somatic supporting cells. Gen. Comp. Endocrinol. 314, 113907. doi:10.1016/j.ygcen.2021.113907
Soygur, B., Jaszczak, R. G., Fries, A., Nguyen, D. H., Malki, S., Hu, G., et al. (2021). Intercellular bridges coordinate the transition from pluripotency to meiosis in mouse fetal oocytes. Sci. Adv. 7 (15), eabc6747. doi:10.1126/sciadv.abc6747
Sreenivasan, R., Jiang, J., Wang, X., Bártfai, R., Kwan, H. Y., Christoffels, A., et al. (2014). Gonad differentiation in zebrafish is regulated by the canonical Wnt signaling pathway. Biol. Reprod. 90 (2), 45. doi:10.1095/biolreprod.113.110874
Stevant, I., Kuhne, F., Greenfield, A., Chaboissier, M. C., Dermitzakis, E. T., and Nef, S. (2019). Dissecting cell lineage specification and sex fate determination in gonadal somatic cells using single-cell transcriptomics. Cell Rep. 26 (12), 3272–3283. e3273. doi:10.1016/j.celrep.2019.02.069
Sun, L. N., Jiang, X. L., Xie, Q. P., Yuan, J., Huang, B. F., Tao, W. J., et al. (2014). Transdifferentiation of differentiated ovary into functional testis by long-term treatment of aromatase inhibitor in Nile tilapia. Endocrinology 155 (4), 1476–1488. doi:10.1210/en.2013-1959
Suzuki, A., Tanaka, M., Shibata, N., and Nagahama, Y. (2004). Expression of aromatase mRNA and effects of aromatase inhibitor during ovarian development in the medaka, Oryzias latipes. J. Exp. Zool. A Comp. Exp. Biol. 301 (3), 266–273. doi:10.1002/jez.a.20027
Takahashi, H. (1977). Juvenile hermaphroditism in the zebrafish, brachydanio rerio. Bull. Fac. Fish. Hokkaido Univ. 28 (2), 57–65.
Takatsu, K., Miyaoku, K., Roy, S. R., Murono, Y., Sago, T., Itagaki, H., et al. (2013). Induction of female-to-male sex change in adult zebrafish by aromatase inhibitor treatment. Sci. Rep. 3, 3400. doi:10.1038/srep03400
Tallapaka, K., Venugopal, V., Dalal, A., and Aggarwal, S. (2018). Novel RSPO1 mutation causing 46, XX testicular disorder of sex development with palmoplantar keratoderma: A review of literature and expansion of clinical phenotype. Am. J. Med. Genet. A 176 (4), 1006–1010. doi:10.1002/ajmg.a.38646
Tanabe, Y., Saito, N., and Nakamura, T. (1986). Ontogenetic steroidogenesis by testes, ovary, and adrenals of embryonic and postembryonic chickens (Gallus domesticus). Gen. Comp. Endocrinol. 63 (3), 456–463. doi:10.1016/0016-6480(86)90146-2
Tanaka, M., Saito, D., Morinaga, C., and Kurokawa, H. (2008). Cross talk between germ cells and gonadal somatic cells is critical for sex differentiation of the gonads in the teleost fish, medaka (Oryzias latipes). Dev. Growth Differ. 50 (4), 273–278. doi:10.1111/j.1440-169X.2008.01015.x
Tang, F., Richardson, N., Albina, A., Chaboissier, M. C., and Perea-Gomez, A. (2020). Mouse gonad development in the absence of the pro-ovary factor WNT4 and the pro-testis factor SOX9. Cells 9 (5), E1103. doi:10.3390/cells9051103
Tena, J. J., Neto, A., de la Calle-Mustienes, E., Bras-Pereira, C., Casares, F., and Gómez-Skarmeta, J. L. (2007). Odd-skipped genes encode repressors that control kidney development. Dev. Biol. 301 (2), 518–531. doi:10.1016/j.ydbio.2006.08.063
Tomaselli, S., Megiorni, F., Lin, L., Mazzilli, M. C., Gerrelli, D., Majore, S., et al. (2011). Human RSPO1/R-spondin1 is expressed during early ovary development and augments β-catenin signaling. PLoS One 6 (1), e16366. doi:10.1371/journal.pone.0016366
Tong, S. K., Hsu, H. J., and Chung, B. C. (2010). Zebrafish monosex population reveals female dominance in sex determination and earliest events of gonad differentiation. Dev. Biol. 344 (2), 849–856. doi:10.1016/j.ydbio.2010.05.515
Trukhina, A. V., Lukina, N. A., Wackerov-Kouzova, N. D., Nekrasova, A. A., and Smirnov, A. F. (2014). Sex inversion in domestic chicken (Gallus gallus domesticus) by letrozole and tamoxifen. Cell tissue Biol. 8 (3), 244–252. doi:10.1134/S1990519X14030122
Tzung, K. W., Goto, R., Saju, J. M., Sreenivasan, R., Saito, T., Arai, K., et al. (2015). Early depletion of primordial germ cells in zebrafish promotes testis formation. Stem Cell Rep. 4 (1), 61–73. doi:10.1016/j.stemcr.2014.10.011
Uchida, D., Yamashita, M., Kitano, T., and Iguchi, T. (2002). Oocyte apoptosis during the transition from ovary-like tissue to testes during sex differentiation of juvenile zebrafish. J. Exp. Biol. 205 (6), 711–718. doi:10.1242/jeb.205.6.711
Uda, M., Ottolenghi, C., Crisponi, L., Garcia, J. E., Deiana, M., Kimber, W., et al. (2004). Foxl2 disruption causes mouse ovarian failure by pervasive blockage of follicle development. Hum. Mol. Genet. 13 (11), 1171–1181. doi:10.1093/hmg/ddh124
Uhlenhaut, N. H., Jakob, S., Anlag, K., Eisenberger, T., Sekido, R., Kress, J., et al. (2009). Somatic sex reprogramming of adult ovaries to testes by FOXL2 ablation. Cell 139 (6), 1130–1142. doi:10.1016/j.cell.2009.11.021
Ukeshima, A. (1996). Germ cell death in the degenerating right ovary of the chick embryo. Zool. Sci. 13 (4), 559–563. doi:10.2108/zsj.13.559
Usongo, M., and Farookhi, R. (2012). β-catenin/Tcf-signaling appears to establish the murine ovarian surface epithelium (OSE) and remains active in selected postnatal OSE cells. BMC Dev. Biol. 12, 17. doi:10.1186/1471-213x-12-17
Vaillant, S., Dorizzi, M., Pieau, C., and Richard-Mercier, N. (2001). Sex reversal and aromatase in chicken. J. Exp. Zool. 290 (7), 727–740. doi:10.1002/jez.1123
Vainio, S., Heikkilä, M., Kispert, A., Chin, N., and McMahon, A. P. (1999). Female development in mammals is regulated by Wnt-4 signalling. Nature 397 (6718), 405–409. doi:10.1038/17068
Vizziano, D., Randuineau, G., Baron, D., Cauty, C., and Guiguen, Y. (2007). Characterization of early molecular sex differentiation in rainbow trout, Oncorhynchus mykiss. Dev. Dyn. 236 (8), 2198–2206. doi:10.1002/dvdy.21212
Wang, D. S., Kobayashi, T., Zhou, L. Y., Paul-Prasanth, B., Ijiri, S., Sakai, F., et al. (2007). Foxl2 up-regulates aromatase gene transcription in a female-specific manner by binding to the promoter as well as interacting with ad4 binding protein/steroidogenic factor 1. Mol. Endocrinol. 21 (3), 712–725. doi:10.1210/me.2006-0248
Wang, D. S., Zhou, L. Y., Kobayashi, T., Matsuda, M., Shibata, Y., Sakai, F., et al. (2010). Doublesex- and Mab-3-related transcription factor-1 repression of aromatase transcription, a possible mechanism favoring the male pathway in tilapia. Endocrinology 151 (3), 1331–1340. doi:10.1210/en.2009-0999
Wang, Q., Lan, Y., Cho, E. S., Maltby, K. M., and Jiang, R. (2005). Odd-skipped related 1 (Odd 1) is an essential regulator of heart and urogenital development. Dev. Biol. 288 (2), 582–594. doi:10.1016/j.ydbio.2005.09.024
Wrobel, K. H., and Suss, F. (1998). Identification and temporospatial distribution of bovine primordial germ cells prior to gonadal sexual differentiation. Anat. Embryol. 197 (6), 451–467. doi:10.1007/s004290050156
Wu, K., Song, W., Zhang, Z., and Ge, W. (2020). Disruption of dmrt1 rescues the all-male phenotype of the cyp19a1a mutant in zebrafish - a novel insight into the roles of aromatase/estrogens in gonadal differentiation and early folliculogenesis. Development 147 (4), dev182758. doi:10.1242/dev.182758
Wu, L., Li, Y., Xu, Y., Li, Y., Wang, L., Ma, X., et al. (2019). Cloning and characterization of wnt4a gene in a natural triploid teleost, Qi river crucian carp (Carassius auratus). Gen. Comp. Endocrinol. 277, 104–111. doi:10.1016/j.ygcen.2019.03.016
Wu, L., Yang, P., Luo, F., Wang, D., and Zhou, L. (2016). R-spondin1 signaling pathway is required for both the ovarian and testicular development in a teleosts, Nile tilapia (Oreochromis niloticus). Gen. Comp. Endocrinol. 230-231, 177–185. doi:10.1016/j.ygcen.2016.04.001
Xie, Y., Huang, D., Chu, L., Liu, Y., Sun, X., Li, J., et al. (2021). Igf3 is essential for ovary differentiation in zebrafish. Biol. Reprod. 104 (3), 589–601. doi:10.1093/biolre/ioaa218
Yamaguchi, T., Yamaguchi, S., Hirai, T., and Kitano, T. (2007). Follicle-stimulating hormone signaling and Foxl2 are involved in transcriptional regulation of aromatase gene during gonadal sex differentiation in Japanese flounder, Paralichthys olivaceus. Biochem. Biophys. Res. Commun. 359 (4), 935–940. doi:10.1016/j.bbrc.2007.05.208
Yan, L., Feng, H., Wang, F., Lu, B., Liu, X., Sun, L., et al. (2019). Establishment of three estrogen receptors (esr1, esr2a, esr2b) knockout lines for functional study in Nile tilapia. J. Steroid Biochem. Mol. Biol. 191, 105379. doi:10.1016/j.jsbmb.2019.105379
Yan, T., Lu, H., Sun, C., Peng, Y., Meng, F., Gan, R., et al. (2021). Nr5a homologues in the ricefield eel Monopterus albus: Alternative splicing, tissue-specific expression, and differential roles on the activation of cyp19a1a promoter in vitro. Gen. Comp. Endocrinol. 312, 113871. doi:10.1016/j.ygcen.2021.113871
Yan, Y. L., Desvignes, T., Bremiller, R., Wilson, C., Dillon, D., High, S., et al. (2017). Gonadal soma controls ovarian follicle proliferation through Gsdf in zebrafish. Dev. Dyn. 246 (11), 925–945. doi:10.1002/dvdy.24579
Yang, Y. J., Wang, Y., Li, Z., Zhou, L., and Gui, J. F. (2017). Sequential, divergent, and cooperative requirements of Foxl2a and Foxl2b in ovary development and maintenance of zebrafish. Genetics 205 (4), 1551–1572. doi:10.1534/genetics.116.199133
Yin, Y., Tang, H., Liu, Y., Chen, Y., Li, G., Liu, X., et al. (2017). Targeted disruption of aromatase reveals dual functions of cyp19a1a during sex differentiation in zebrafish. Endocrinology 158 (9), 3030–3041. doi:10.1210/en.2016-1865
Zamboni, L., Bezard, J., and Mauleon, P. (1979). The role of the mesonephros in the development of the sheep fetal ovary. Ann. Biol. Anim. Bioch. Biophys. 19 (4B), 1153–1178. doi:10.1051/rnd:19790801
Zhang, X., Li, M., Ma, H., Liu, X., Shi, H., Li, M., et al. (2017). Mutation of foxl2 or cyp19a1a results in female to male sex reversal in XX nile Tilapia. Endocrinology 158 (8), 2634–2647. doi:10.1210/en.2017-00127
Zhang, Y., Li, F., Sun, D., Liu, J., Liu, N., and Yu, Q. (2011). Molecular analysis shows differential expression of R-spondin1 in zebrafish (Danio rerio) gonads. Mol. Biol. Rep. 38 (1), 275–282. doi:10.1007/s11033-010-0105-3
Zhao, F., Franco, H. L., Rodriguez, K. F., Brown, P. R., Tsai, M. J., Tsai, S. Y., et al. (2017). Elimination of the male reproductive tract in the female embryo is promoted by COUP-TFII in mice. Science 357 (6352), 717–720. doi:10.1126/science.aai9136
Zhao, L., Wang, C., Lehman, M. L., He, M., An, J., Svingen, T., et al. (2018). Transcriptomic analysis of mRNA expression and alternative splicing during mouse sex determination. Mol. Cell. Endocrinol. 478, 84–96. doi:10.1016/j.mce.2018.07.010
Zheng, W., Zhang, H., Gorre, N., Risal, S., Shen, Y., and Liu, K. (2014). Two classes of ovarian primordial follicles exhibit distinct developmental dynamics and physiological functions. Hum. Mol. Genet. 23 (4), 920–928. doi:10.1093/hmg/ddt486
Zheng, X., O'Connor, J., Huchzermeyer, F., Wang, X., Wang, Y., Wang, M., et al. (2013). Preservation of ovarian follicles reveals early evolution of avian reproductive behaviour. Nature 495 (7442), 507–511. doi:10.1038/nature11985
Zhou, L., Charkraborty, T., Yu, X., Wu, L., Liu, G., Mohapatra, S., et al. (2012). R-spondins are involved in the ovarian differentiation in a teleost, medaka (Oryzias latipes). BMC Dev. Biol. 12, 36. doi:10.1186/1471-213x-12-36
Zhou, L., Charkraborty, T., Zhou, Q., Mohapatra, S., Nagahama, Y., and Zhang, Y. (2016). Rspo1-activated signalling molecules are sufficient to induce ovarian differentiation in XY medaka (Oryzias latipes). Sci. Rep. 6, 19543. doi:10.1038/srep19543
Zhu, J., Zhang, D., Liu, X., Yu, G., Cai, X., Xu, C., et al. (2019). Zebrafish prmt5 arginine methyltransferase is essential for germ cell development. Development 146 (20), dev179572. doi:10.1242/dev.179572
Zhu, Y., Hu, Q., Xu, W., Li, H., Guo, H., Meng, L., et al. (2017). Identification and analysis of the β-catenin1 gene in half-smooth tongue sole (Cynoglossus semilaevis). PLoS One 12 (5), e0176122. doi:10.1371/journal.pone.0176122
Glossary
ADAMTS9 A disintegrin and metalloproteinase with thrombospondin motifs 9
AMH Anti-Mullerian hormone
BMP15 Bone morphogenetic protein 15
BMPR2A/B Bone Morphogenetic Protein Receptor Type 2 a/b
BPES Blepharophimosis–ptosis–epicanthus inversus syndrome
COUPTFII Chicken Ovalbumin Upstream Promoter Transcription Factor 2
CTNNB1 Catenin Beta 1
CYP17A1 Cytochrome P450 Family 17 Subfamily A Member 1
CYP19A1 Cytochrome P450, family 19, subfamily A, polypeptide 1, also called aromatase
CYP26B1 Cytochrome P450 Family 26 Subfamily B Member 1
DAX1 Dosage-sensitive sex reversal (DSS), Adrenal hypoplasia congenital critical region on the X chromosome, gene 1
DDX5 DEAD-Box Helicase 5
DKK1 Dickkopf WNT signaling pathway inhibitor 1
DMRT1 Doublesex and mab-3 related, transcription factor 1
Dmy DM-domain gene on the Y chromosome
dpc Days post coitum
dph Day post hatching
DSD Differences or disorders of sex development
E Embryonic day
E2 Estradiol-17b
EMT Epithelial–Mesenchymal Transition
EMX2 Empty Spiracles Homeobox 2
ERα/β Estrogen receptor alpha/beta
ESR1/2 Estrogen receptor 1 / 2
FOXL2 Forkhead box L2
FST Follistatin
GDF9 Growth differentiation factor 9
GSDF Gonadal soma derived growth factor
IGF3 Insulin-like growth factor-3
JCM Juxtacortical medulla
KO Knockout
LGR4 Leucine-rich repeat-containing G-protein coupled receptor 4
LGR5 Leucine-rich repeat-containing G-protein coupled receptor 5
LHX9 LIM Homeobox 9
NOBOX NOBOX oogenesis homeobox
NR2F2 Nuclear Receptor Subfamily 2 Group F Member 2 (COUP-TFII)
NR5A1 Nuclear receptor subfamily 5 Group A Member 1 (SF-1)
NR5A2 Nuclear receptor subfamily 5 Group A Member 2 (LRH-1)
NR0B1 Nuclear Receptor Subfamily 0 Group B Member 1 (DAX1)
OSR1 Odd-Skipped Related Transcription Factor 1
PAX2 Paired box gene 2
PGCs Primordial Germ Cells
PIS Polled Intersex Syndrome
PITX2 Paired Like Homeodomain 2
PRMT5 Protein arginine methyltransferase 5
RALDH2 retinaldehyde dehydrogenase 2 (ALDH1A2)
RBPMS2 RNA-binding protein of multiple splice forms 2
RSPO1 R-spondin 1
RUNX1 Runt-related transcription factor 1
scRNA-seq single-cell RNA sequencing
SERKAL Sex reversal with dysgenesis of kidneys, adrenals, and lungs
SF-1 Steroidogenic factor-1
SOX9 SRY-related HMG box 9
SRY Sex-determining region on the Y chromosome
TALEN Transcription activator-like effector nuclease
TGF β Transforming growth factor beta
TGIF1 TGFB Induced Factor Homeobox 1
WNT4 Wingless-type MMTV integration site family, member 4
wpc Weeks post coitum/conception
WT1 Wilms’ tumor suppressor 1
Keywords: chicken, mammals, granulosa cells, sex determination, ovary, teleost
Citation: Nicol B, Estermann MA, Yao HH-C and Mellouk N (2022) Becoming female: Ovarian differentiation from an evolutionary perspective. Front. Cell Dev. Biol. 10:944776. doi: 10.3389/fcell.2022.944776
Received: 15 May 2022; Accepted: 16 August 2022;
Published: 07 September 2022.
Edited by:
Shao-Chen Sun, Nanjing Agricultural University, ChinaReviewed by:
Ugo Coppola, Heart Institute, Cincinnati Children’s Hospital Medical Center, United StatesSol Sotillos, Spanish National Research Council (CSIC), Spain
Copyright © 2022 Nicol, Estermann, Yao and Mellouk. This is an open-access article distributed under the terms of the Creative Commons Attribution License (CC BY). The use, distribution or reproduction in other forums is permitted, provided the original author(s) and the copyright owner(s) are credited and that the original publication in this journal is cited, in accordance with accepted academic practice. No use, distribution or reproduction is permitted which does not comply with these terms.
*Correspondence: Barbara Nicol, YmFyYmFyYS5uaWNvbEBuaWguZ292