- Institute of Biomedical Engineering and Health Sciences, School of Pharmacy and School of Medicine, Changzhou University, Changzhou, Jiangsu, China
Cell–cell mechanical communications at a large spatial scale (above hundreds of micrometers) have been increasingly recognized in recent decade, which shows importance in tissue-level assembly and morphodynamics. The involved mechanosensing mechanism and resulted physiological functions are still to be fully understood. Recent work showed that traction force sensation in the matrix induces cell communications for self-assembly. Here, based on the experimental model of cell directional migration on Matrigel hydrogel, containing 0.5 mg/ml type I collagen, we studied the mechano-responsive pathways for cell distant communications. Airway smooth muscle (ASM) cells assembled network structure on the hydrogel, whereas stayed isolated individually when cultured on glass without force transmission. Cell directional migration, or network assembly was significantly attenuated by inhibited actomyosin activity, or inhibition of inositol 1,4,5-trisphosphate receptor (IP3R) calcium channel or SERCA pump on endoplasmic reticulum (ER) membrane, or L-type calcium channel on the plasma membrane. Inhibition of integrin β1 with siRNA knockdown reduced cell directional migration and branching assembly, whereas inhibition of cell junctional N-cadherin with siRNA had little effect on distant attractions but blocked branching assembly. Our work demonstrated that the endoplasmic reticulum calcium channels and integrin are mechanosensing signals for cell mechanical communications regulated by actomyosin activity, while N-cadherin is responsible for traction force-induced cell stable connections in the assembly.
Introduction
Cell–cell mechanical communications in distance have been much documented in recent decade, which has shown remote mechanosensitive responses among cells (Alisafaei et al., 2021; Long et al., 2022). In tracking the development of this discovery back, biologists had observed the outgrowth of separated neuronal tissues toward each other in early last century (Weiss, 1929; Katzberg, 1959). In 1981, Harris et al. reported that traction force from fibroblast tissue explants induced fibrillary morphogenesis of type I collagen (COL), in which separation reached a distance of 1.5–4 cm (Harris et al., 1981; Stopak and Harris, 1982). The work is followed with a mechanistic study on the collagen fiber self-organization driven by cell and matrix movements (Sawhney and Howard, 2002). Over 10 years ago, single-cell studies reported mechanical interactions within neighboring cells (Reinhart-King et al., 2008; Winer et al., 2009). Later, studies established long-range force-induced communications among mammary cell clusters, which causes COL fiber remodeling and helps assembly of tissue patterns (Guo et al., 2012; Brownfield et al., 2013; Shi et al., 2014).
In recent decade, cell long-range mechanical communications have been shown in different scenarios. Cardiomyocytes can reserve a beating frequency induced by a mechanical probe nearby or achieve synchronized beating (Nitsan et al., 2016; Sapir and Tzlil, 2017). Fibroblast cells show an ability to remotely mechanosense the lateral boundaries when seeding on floating collagen gel (Mohammadi et al., 2014). Collective migration of epithelial sheet is promoted by long-range intercellular force transmission on the gradient-stiffness substrate (Sunyer et al., 2016). Fibroblasts can collectively align through long-range interactions arising from modification of underlying matrix (Li et al., 2017). Long-range mechanotransduction via collagen fibers enables myofibroblast–fibroblast interactions during fibrosis expansion (Liu et al., 2020). Fibroblasts display remote attraction of macrophages on collagen hydrogel via distant force transmission (Pakshir et al., 2019). Neural crest cell group achieves collective migration in a supracellular fashion with persistent contractility at the rear of the cluster, and self-generates a stiffness gradient within the tissue in vivo (Shellard et al., 2018; Shellard and Mayor, 2021). These studies have demonstrated long-range mechanical communications as a common feature of different cells.
At the same time, the relevant mechanism of long-range force transmission and COL fibrillary modeling have been investigated based on experimental data and computational simulations (Ma et al., 2013; Wang et al., 2014; Baker et al., 2015). Clinical biopsy check and in vitro assays both show cells aligning collagen fibers to guide metastatic migrations of breast cancer cells (Han et al., 2016). The property of non-linear elastic extracellular matrix (ECM) from tissue can facilitate distant force transmission (Sopher et al., 2018), and cell-generated stresses are capable of buckling COL filaments in ECM at large spatial extent (Han et al., 2018; Mann et al., 2019). Our recent data have shown that motile cells can actively recruit soluble environmental COL to assemble filaments for tissue-level assembly (Wang et al., 2020).
These observations also lead to an interesting topic on how cells mechanosense each other in distance or at large spatial scales. Some progresses have emerged in recent years, for example, mechanosensitive integrin signals and ion channels in cells are necessary components in mechanical communications (Pakshir et al., 2019; Liu et al., 2020). Another mechanistic advancement is the demonstration that cells are capable of rapidly sensing traction force in the matrix or substrate deformation, which results in directional migration and tissue-level assembly (Zarkoob et al., 2018; Ouyang et al., 2020). It has also been proven that cells can directly sense the mechanical force from the substrate besides conventional stiffness (Panzetta et al., 2019). From computational simulation along with experimental verification, COL fiber bundles re-organized by cell contraction bear the major tensile force in the ECM, which can be transmitted to distant cells (Fan et al., 2021). During migration of epithelial cell sheet, there is also long-range force transmission through cell–cell junctions, and ERK signal wave is activated in cells to promote the migration direction (Sunyer et al., 2016; Hino et al., 2020). Cell mechanical communications also play a role in tissue pattern formation (Guo et al., 2012). Our work has shown that cells can form stable connections by mechanical interactions on the elastic ECM, but not on coated glass, and the assembled connections rely on cell contraction force by the ECM substrate (Ouyang et al., 2020). The cellular mechanism for distant mechanical communications and resulted cell connections still remains largely to be elucidated.
In this work, we applied the experimental model of mechanical communication from our recent study (Ouyang et al., 2020) and investigated the mechanosensitive pathways in regulating the mechanical attractions and cell connection stability. Cell mechanotransduction has been shown involving a bunch of molecules such as the typical membrane receptors integrins and cadherins and ion channels (Martino et al., 2018). Here, we screened the roles of integrin, cadherin, and calcium channels in cell distant mechanical communications and branching assembly. In regards, specifically, cells communicate with the microenviromental matrix largely through ligations between integrins and matrix proteins (Li et al., 2016; Sun et al., 2016); cadherins located at the cell–cell junctions are mechanosensitive molecules between cells (Leckband and de Rooij, 2014); membrane calcium channels have been demonstrated critical for cells in transducing external mechanics (Kim et al., 2015; Fang et al., 2022). Through experimental characterizations, our work demonstrated the importance of calcium channels on the ER membranes and integrin signal along with actomyosin contraction in cell distant mechanical attractions, and N-cadherin in stabilizing cell–cell connections.
Materials and methods
Cell culture and reagents
Primary airway smooth muscle (ASM) cells were originated from 6–8-week-old Sprague–Dawley rats, as described previously (approved by the Ethics Committee of Changzhou University on Studies Ethics, Grant No. NSFC 11532003) (Wang et al., 2016). ASM cells were cultured in low-glucose DMEM (Invitrogen) supplemented with 10% FBS and penicillin/streptomycin antibiotics. The cells were maintained in the humidified culture incubator, containing 5% CO2 at 37°C. The cells applied in the experiments were generally within 10 passages during regular culture.
Matrigel was purchased from BD Biotechnology, and type I collagen was from Advanced Biomatrix. The chemical reagents 2-amino-ethoxydiphenyl borate (2-APB, #D9754-10G), nifedipine (#N7634-1G), cytochalasin D (1 µM), blebbistatin (20 µM), and ML-7 (20 µM) were purchased from Sigma-Aldrich, and thapsigargin (TG, #ab 120286) from Abcam. ON-TARGETplus SMARTpool N-cadherin siRNA (N-cadherin siRNA, #M-091851-01-0005) was purchased from Horizon Discovery. Integrin β1 (ITGB1) siRNA (#AM16708) was purchased from Thermo Fisher Scientific.
Preparation of hydrogel in the polydimethylsiloxane (PDMS) mold
The preparation processes of PDMS mold and cell network culture have been introduced in our recent work (Ouyang et al., 2020). Briefly, a thin layer of PDMS (∼600 μm in thickness) was generated by mixture of the two liquid components (10:1) from the Sylgard 184 kit (Dow Corning). The PDMS sheet was cut into circular pieces, on which one or more holes with 0.6 cm in diameter were created by a mechanical puncher. The PDMS mold was sterilized and attached onto the glass-bottom dish (NEST). Matrigel containing 0.5 mg/ml COL was added into the PDMS mold on ice, and then placed into the incubator to solidify at 37°C for 30 min. To seed cells, about 30 μL of cell suspension was added on top of the hydrogel and stayed for 30 min in the incubator before the addition of more culture medium.
siRNA transfection and inhibitor applications
ASM cells were cultured to 40% of confluency in 6-well plates, and then transfected with 25 nM Integrin β1 or N-cadherin siRNA, or control siRNA (final siRNA concentration in medium) by using Lipofectamine 3,000 transfection kit (#L3000-008, Thermo). After 12 h, the medium was replaced with 10% FBS DMEM, and 72 h after transfection, the cells were ready for experiments.
After the cells were planted on the hydrogel in the glass-bottom dish placed into a 6-well plate container (Zeiss), 2 ml regular culture medium was added containing appropriate concentration of inhibitor. The experimental concentrations of 2-APB, nifedipine, and thapsigargin were 100 μM, 10 μM, and 10 μM, respectively.
Measurements of siRNA transfection efficiency by Q-PCR
The efficiency of siRNA transfection was assessed with mRNA expression by real-time quantitative PCR (Q-PCR) assay, which was described in our recent work (Fang et al., 2022). The sequences of associated Q-PCR primers for rat ITGB1 were GAATGGAGTGAATGGGACAGGAG (ITGB1 forward), CAGATGAACTGAAGGACCACCTC (ITGB1 reverse), the control GAPDH primers AGGTCGGTGTGAACGGATTTG (forward), and GGGGTCGTTGATGGCAACA (reverse) (Luo et al., 2018), and for rat N-cadherin primers GTTTACAGCGCAGTCTTACCGAAG (forward) and ATTTCTTCCCTTTCATGTGGTTCC (reverse). PowerUp SYBR Green Master Mix (#A25742, Applied Biosystems, CA) was used for PCR amplification.
Time-lapse microscopy imaging
As described recently (Ouyang et al., 2020), the epi-microscopy system (Zeiss) was equipped with the X–Y–Z control stage for multi-position function, fine auto-focusing for time-lapse imaging, and temperature (37°C)-CO2 (5%) chamber to maintain cell culture conditions. Most of the imaging experiments on hydrogel were visualized with ×20 objective, and the interval time was generally set as 30 min. The imaging durations were generally within 18–24 h. During the fluorescence imaging of cells stained with WGA, the excitation light from the lamp was reduced to 1/8 of the full power, and interval time was set as 1 h to minimize the photobleaching.
Trajectory analysis of cell movements
To track cell movements, the stacked images were input into ImageJ, and the distance in pixels was transduced to length in µm on the images by the “Set Scale” function. Then by using the “Manual tracking” function from the plugin list (Plugins ̶>Tracking ̶>Manual tracking ̶>Add track), the time-sequence positions (x, y) and moving distances were generated automatically by continuously clicking the target cells through the first to last frames. The acquired digital file was further input into MATLAB software to calibrate the move distances and generate the map of trajectories. The movement quantifications were generally from imaging experiments within the time ranges of 18–24 h. The migration velocities and speeds of the cells were calibrated manually based on the move distances, displacements, and time durations.
GraphPad and Origin2020 were applied for statistical analysis and generation of data graphs. The values on the graphs represent the mean ± S.D. (standard derivation) from their groups (in scattering dots). *, **, ***, and **** indicate p < 0.05, 0.01, 0.001, and 0.0001, respectively, for significant difference between each two groups from Student’s t-test.
Results
Characterization of cell directional migrations during mechanical communications
Recent studies including our work have further established cell–cell distant communications using traction force sensation. To investigate the responsible cellular mechanosensitive pathways, we utilized the experimental model of cell directional migration induced by mechanical interactions. Basically, the cells were seeded on Matrigel hydrogel, containing 0.5 mg/ml type I collagen (COL) within the PDMS mold, and ASM cells formed branching networks in 1 day (Supplementary Figure S1). The boundary stiffness of the PDMS mold within the regular scale did not show obvious impact on the branching assembly in this experiment (Supplementary Figure S2).
To characterize the directional migrations, time-lapse imaging was taken every 0.5 h for 18 h, and cell positions were tracked on the hydrogel or glass for comparison. ASM cells were assembled into the network structure on the hydrogel in 18 h whereas stayed individually on the glass, and cell trajectory analysis showed efficient directional movements on the hydrogel, but in random movements with far less reached spatial ranges on the glass (Figure 1A; Supplementary Movie S1). Statistical quantifications of move velocity and speed confirmed quicker migrations of ASM cells on the hydrogel than on the glass (Figure 1B). The cells displayed elongated branching structures on the hydrogel with averaged ∼400 μm in length within the image windows (Figure 1C). The move speed, which reflects the direct linear distance between the initial and final positions in 18 h, shows multiple times difference between the two conditions. The quicker movement on the hydrogel may reflect a promoting role by the cell–cell mechanical interactions.
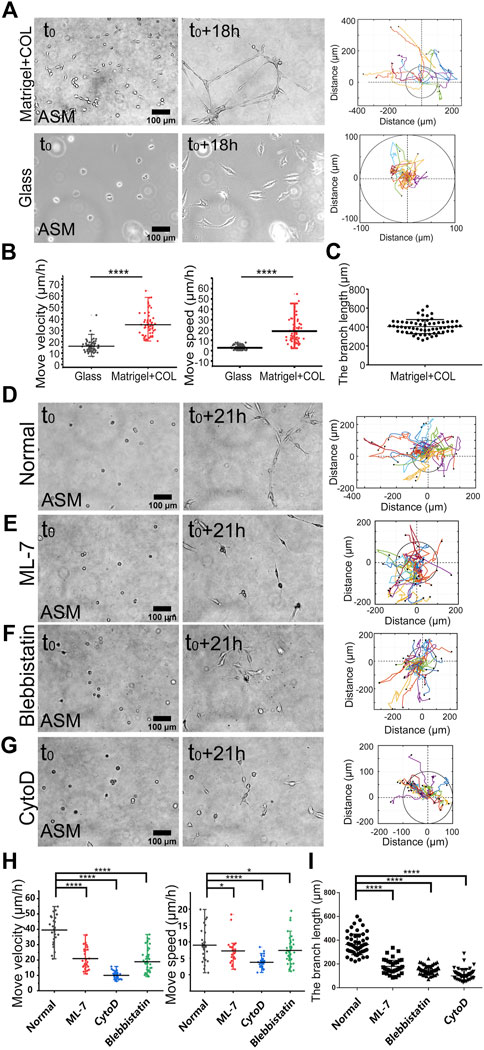
FIGURE 1. Actomyosin contraction force-mediated cell directional migrations during network assembly on the hydrogel. The hydrogel refers to 3D Matrigel containing 0.5 mg/ml COL in this work. The trajectory analysis of cell movements was performed by ImageJ as described in the Methods. (A) Time-lapse imaging of ASM cells on the hydrogel and glass, and the trajectory analysis of cell movements. The images were taken with ×20 objective for 18 h. The indicated circles present the size of 200 µm in diameter. (B) Statistical quantifications of cell move velocity and speed (mean ± S.D.) on the hydrogel and glass in 18 h. Move velocity (μm/h): 16.0 ± 5.2, n = 72 on glass; 34.9 ± 10.4, n = 60 on hydrogel, and move speed (μm/h): 2.6 ± 2.0, n = 45 on glass; 14.5 ± 13.5, n = 32 on hydrogel. (C) Measured branch length on the hydrogel within the imaging windows. (D–G) Representative cell images at 0 and 21 h, and trajectory analysis under control condition (DMSO) (D), or incubation with ML-7 (20 μM) (E), blebbistatin (25 μM) (F), and CytoD (2 μM) (G). (H,I) Statistical quantifications of cell move velocity and speed within 21 h (H) and the branching lengths (I) under the indicated conditions of (D–G). Cell sample size n = 29 (DMSO), 28 (ML-7), 31 (CytoD), and 47 (blebbistatin), respectively. Statistical comparisons were conducted by Student’s t-test analysis between each two groups, and ∗, ∗∗, ∗∗∗, and ∗∗∗∗indicate p < 0.05, 0.01, 0.001, and 0.0001 for significant difference, respectively, and so on through the manuscript.
Our previous work demonstrated that cell mutual attraction and migration rely on sensation of traction force on the hydrogel, which is derived from cell contraction (Ouyang et al., 2020). Here, we tried to verify the directionally migrating model in cell contraction force-dependent manner. By inhibition of actomyosin contraction with myosin II light chain kinase (MLCK) inhibitor ML-7 or myosin II ATPase activity inhibitor blebbistatin, or disruption of actin cytoskeleton with cytochalasin D (CytoD), cell migrations were significantly attenuated (Figures 2D–G; Supplementary Movie S2), which was further verified by statistical quantifications (Figure 2H). Particularly without integrity of actin cytoskeleton, cell directional migration was inhibited the most (Figures 2D,E). Due to cell migrating not always straight forward within 10–20 h period, the quantified speed of cell movements was generally smaller than the velocity. Consequentially from the attenuated migrations, the branching assembly was reduced under inhibited actomyosin contraction (Figure 2I). It is seen that branching assembly relies on or is resulted from directional migration. Hence, we applied the migration model to investigate the mechanosensitive pathways for cell–cell mechanical communications.
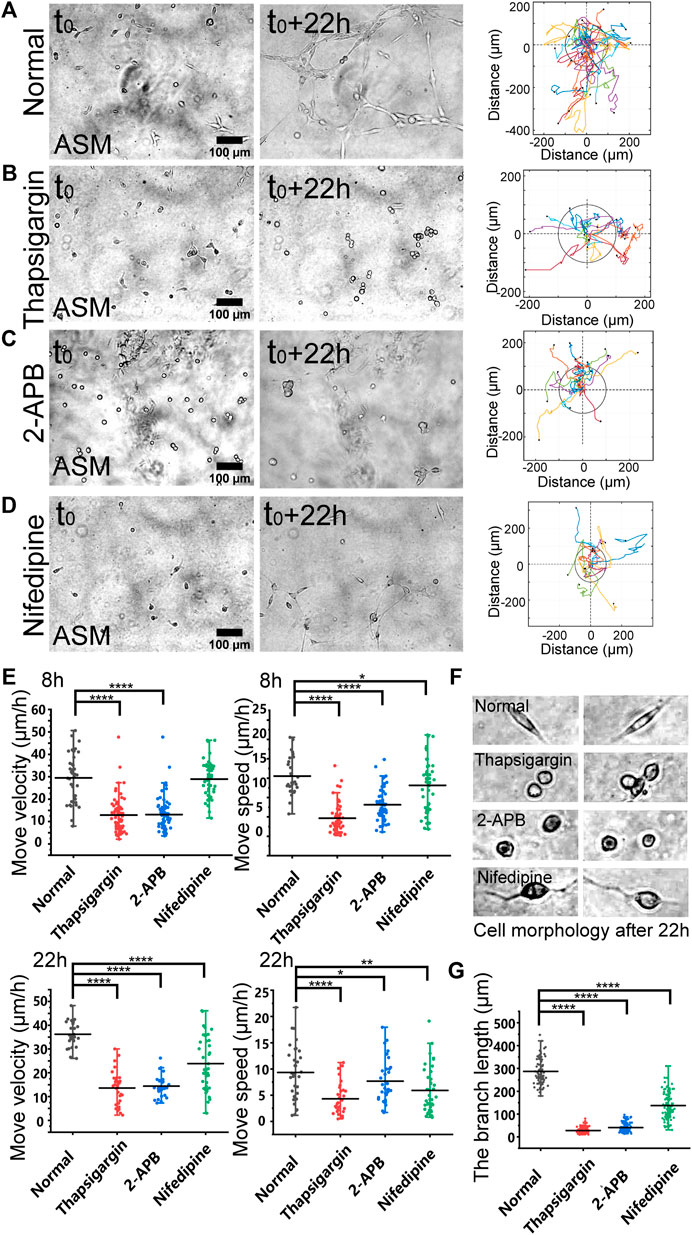
FIGURE 2. Regulations by membrane calcium channels in cell migration and branching assembly. ASM cells were seeded on the hydrogel with or without calcium channel inhibitor in the culture medium. Time-lapse imaging was taken every 0.5 h for 22 h, followed with trajectory analysis and branching quantification. (A–D) Cell images at 0 and 22 h, and trajectory analysis of cell movements under the control condition (DMSO) (A), or with thapsigargin (10 µM) (B), 2-APB (100 µM) (C), or nifedipine (10 µM) (D) in the medium. (E) Statistical quantifications of cell move velocity and speed with or without the calcium channel inhibitors when culturing on the hydrogel. The sample size n = 46, 55, 34, 36 (velocity) and 56, 55, 34, 35 (speed) for 8 h (top panel), and n = 29, 28, 31, 37 (velocity) and 32, 31, 34, 40 (speed) for 22 h (bottom panel), respectively. In considering that at later time, the cells might have formed branching or clusters and stopped directional migration, so quantifications were performed at 8 and 22 h, respectively. (F) Representative cell morphologies with or without the calcium inhibitors. (G) Statistical quantifications of the branching lengths with or without the calcium channel inhibitors when culturing on the hydrogel for 22 h (n = 81, 112, 112, 102, respectively).
Calcium channels regulate mechanosensation-induced cell directional migration
Based on the migration model (Figure 1), we further investigated the cellular mechanosensing pathways. Calcium channels on the cellular membranes show mechanical sensitivity (Kim et al., 2015). By selective inhibition of inositol 1,4,5-trisphosphate receptor (IP3R) calcium channel with 2-APB or the SERCA (sarcoendoplasmic reticulum calcium ATPase) pump with thapsigargin on the endoplasmic reticulum (ER) membrane, the cells showed attenuated directional migrations, and significantly inhibited branching formations (Figures 2A–C; Supplementary Movie S3). Inhibition of L-type calcium channel with nifedipine on the plasma membrane also resulted in downregulated cell migration and branching assembly (Figure 2D; Supplementary Movie S3). Statistical quantifications confirmed reduced migration after inhibition of these calcium channels, but less reduced migration in the early 8 h with nifedipine treatment in comparison to the control group (Figure 2E), suggesting early time mechanosensation less dependent on these calcium channels. Morphologically, the cells displayed round shapes with IP3R or SERCA inhibition, and long membrane protrusions with L-type calcium channel inhibition, in comparison to the normal elongated cell body culturing on the hydrogel (Figure 3F). Particularly, inhibitions of the calcium channels and pump resulted in losing branching assembly (Figure 3G). These data indicate that membrane calcium channels were mechanosensitive components during the cell–cell mechanical interactions.
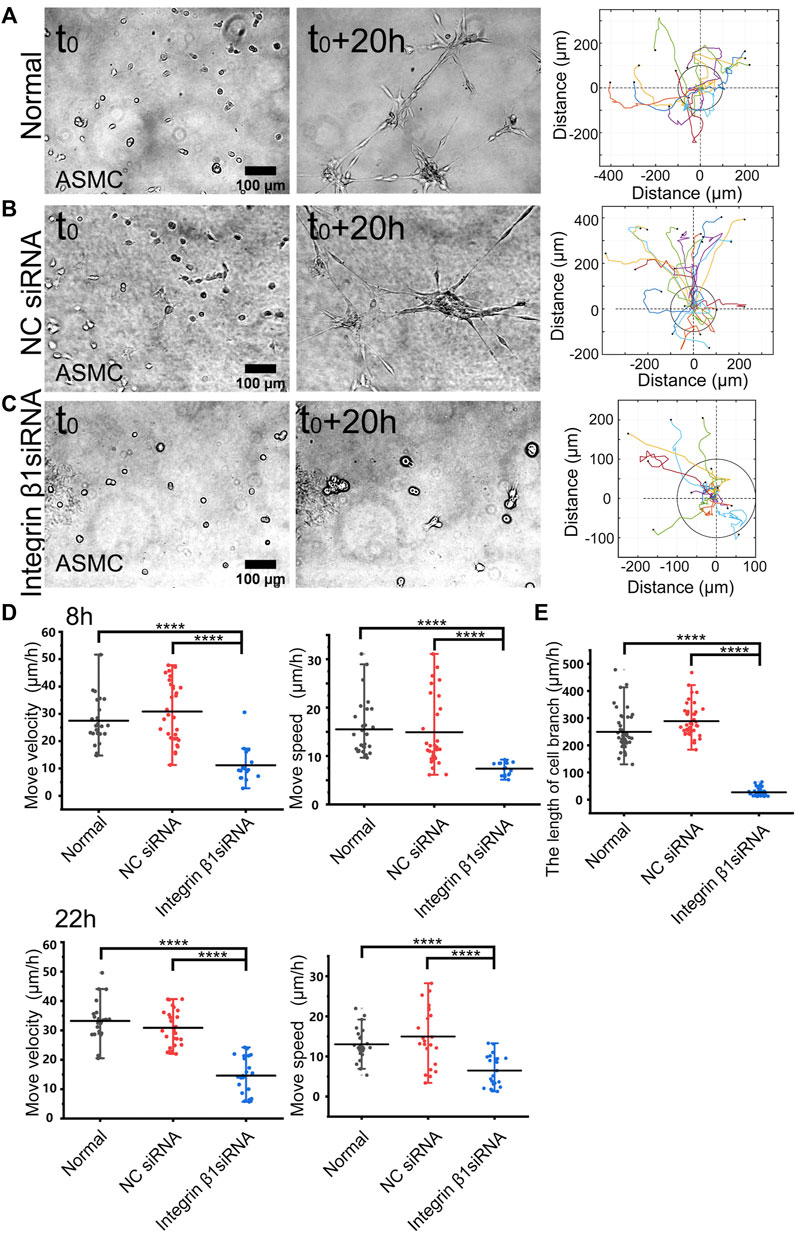
FIGURE 3. Role of integrin β1 in cell directional migration. ASM cells were transfected with control or ITGB1 siRNA, and after 72 h, the cells were seeded on the hydrogel for time-lapse imaging. (A–C) Cell branching assembly and trajectory analysis in ASM cells under normal condition (A), or transfected with control siRNA (B) or ITGB1 siRNA (C). (D,E) Statistical quantifications of cell move velocity and speed in 8 h (top panel, n = 23, 34, 16, respectively) and 22 h (bottom panel, n = ∼23, ∼20, 22, respectively) (D), and assembled branching lengths (n = 44, 44, 48, respectively) (E) under the indicated conditions.
Integrin β1 mediates cell directional migration and branching assembly
Integrin β1 forms heterodimers with variable integrin α subunits (α1-11 or αv) (Cai et al., 2021), so we tried to inhibit β1 subunit and checked the role in mechanosensation-induced cell directional migration. After transfected with integrin β1 (ITGB1) siRNA (the efficiency shown in Supplementary Figure S3A), cell directional migration was significantly reduced in comparison with normal condition or control siRNA (Figures 3A–C; Supplementary Movie S4). Particularly, the branching structure could not be assembled in ASM cells transfected with ITGB1 siRNA (Figure 3C). As shown by further quantifications, ITGB1 siRNA transfection resulted in inhibited cell directional migration (Figure 3D) and nearly blocked branching assembly (Figure 3E). These results indicate that integrin α(x) β1 is the mechanosensitive component for the cell–cell mutual distant interactions.
N-cadherin regulates cell branching formation
N-cadherin is one mechanosensitive molecule on the plasma membrane and regulates cell–cell junctional connections (Leckband and de Rooij, 2014). We further investigated the role of N-cadherin in cell migration and branching assembly during the cell–cell mechanical interactions. ASM cells displayed directional migration and branching assembly under normal condition or with control siRNA and showed regular migration and formed cell clusters within 13 h instead of branching structures with N-cadherin siRNA (Figures 4A–C; Supplementary Movie S4). Statistical quantifications demonstrated that ASM cells maintained regular directional migrations after N-cadherin siRNA transfection (the efficiency shown in Supplementary Figure S3B) but failed in assembly of branching structures (Figure 4D). From the quantifications (Figure 4D), the cells seemed moving more with N-cadherin siRNA, which might be due to contraction of the cell groups, whereas the cells were more stabilized in the branches under the control conditions. These results indicate that N-cadherin was not necessary for cell distant mechanosensation but essential for cell–cell junctional connections to form stable branching structures.
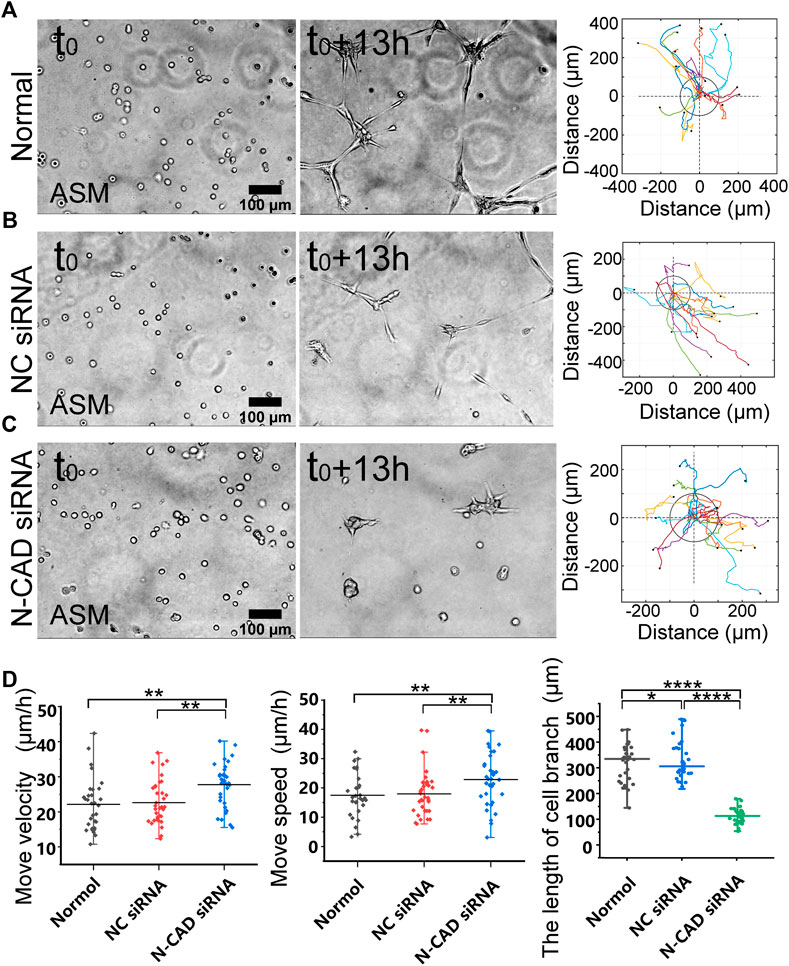
FIGURE 4. N-cadherin in regulating cell branching formation. ASM cells were transfected with N-cadherin (N-CAD) or control siRNA, and after 72 h, the cells were seeded on the hydrogel for time-lapse imaging. (A–C) ASM cells branch assembly and trajectory analysis under normal condition (A), or transfected with control siRNA (B) or N-CAD siRNA (C). Cells transfected with N-CAD siRNA formed clusters without further migration later, and the first 13-h images were analyzed. (D) Statistical quantifications of ASM cell move velocity and speed (n = 33, 34, 37, respectively) and lengths of assembled branches (n = 29, 29, 38, respectively) under the indicated conditions of (A–C).
Discussion
Cell–cell mechanical communications in long or large spatial scale have been increasingly recognized in the past decade. It has been further revealed recently that cells are able to communicate via traction force mechanosensation through the matrix substrates. The mechano-responsive mechanism is still to be investigated. In this work, we tried to study the primary mechanosensing components for cell–cell distant mechanical interactions. Based on the experimental model from our recent work (Ouyang et al., 2020), we characterized cell directional migration and network assembly to measure cell–cell mechanical attractions, which was applied to identify cellular mechanosensitive components.
ASM cells assembled into network structures within 1 day on the hydrogel, while stayed isolated individually on the glass surface without force transmission (Figure 1A, Supplementary Figure S1; Supplementary Movie S1). Migration quantifications showed much quicker movements on the hydrogel than on the glass, indicating a significant promoting role in driving cell directional migration by cell–cell mechanical interactions (Figure 1B). The integrity of actin cytoskeleton and actomyosin activity are essential for the mechanical interactions (Figures 1D–H; Supplementary Movie S2), verifying the interaction force originated from cellular contraction. Hence, we have validated the experimental model based on migration and branching assembly for cell–cell mechanical communications.
Recent studies reported that Piezo ion channel (one type of mechanosensitive calcium channel) on the plasma membrane plays an important role in cell mechanosensation of force (Pakshir et al., 2019; Liu et al., 2020). Here, we further explored the relevance of calcium channels on plasma and ER membranes during the cell–cell distant mechanical interactions. The experimental data demonstrated that the IP3R calcium channel and SERCA pump on the ER membrane are important for cell directional migration and essential for branching assembly (Figures 2A–E; Supplementary Movie S3). This observation is consistent with the previous reports that the calcium signal from ER storage is mechanosensitive to mechanical stimulation (Kim et al., 2015; Kim et al., 2020), which also supports the cell–cell distant attractions due to mechanical communications.
Integrins are mechanosensitive receptors on the plasma membrane in response to ECM mechanics (Alenghat and Ingber, 2002; Isomursu et al., 2019). Two recent studies have shown that integrin signaling mediates intracellular mechanical interactions (Pakshir et al., 2019; Liu et al., 2020). Here, we tried to validate the role of integrin signaling in this experimental model. Inhibition of integrin β1 with siRNA in ASM cells, which is a representative subunit forming dimer with variable α subunits, led to significantly reduced cell–cell remote attractions as shown by the inhibited directional migrations and branching formations (Figures 3A–E; Supplementary Movie S4). In considering that integrins are basic components in assembling focal adhesions (Kanchanawong et al., 2010), deficiency in integrin may hinder the mechano-response for directional migrations toward the neighboring cells. From these few studies, it is conclusive that integrins are mechanosensitive signals in the cell–cell distant mechanical communications.
There is an interesting observation in our work that ASM cells formed one-to-one connections to assemble a continuous network structure, but no stable connections occurred on the glass without traction force transmission (Supplementary Figure S1; Figures 1A,B) (Ouyang et al., 2020). N-cadherin is one mechanosensitive molecule at cell–cell junctions (Leckband and de Rooij, 2014) and also shows the role in promoting cell directional movements (Choi et al., 2021). In this work, selective knockdown of N-cadherin with siRNA did not have apparent impact on cell–cell remote attraction as measured by the move speeds, but inhibited one-to-one stable cell connections for branching assembly (Figures 4A–D, Supplementary Movie S5). This result implies that N-cadherin is critical for traction force-induced stable cell–cell connections.
From this work, we have not understood the correlations of these few factors during cell–cell mechanical communications. From previously known information and this study, calcium channels mediate actomyosin contraction by calcium signaling, which drives cell migration (Kuo and Ehrlich, 2015); integrin is important in transducing biomechanical signaling at the cell–matrix interface, and promoting cell protrusion at the migratory front (Huttenlocher and Horwitz, 2011); N-cadherin is not directly responsive in this distant mechanical communication, but maintains the branching through cell–cell junctions adjacent with intracellular cytoskeleton. There are cross talks between integrin signaling and cadherin, as both interacting closely with actin cytoskeleton (Weber et al., 2011).
In summary, our work identified the importance of the IP3R calcium channel and SERCA pump on the ER membrane and also verified the integrin signal in the cell–cell distant mechano-attractions, and further demonstrated junctional N-cadherin in stabilizing traction force-induced cell–cell connections. The molecular mechanism or subsequential relations of these components during the mechanosenation need more studies.
Data availability statement
The raw data supporting the conclusion of this article will be made available by the authors, without undue reservation.
Author contributions
MO and LD conceived the project and designed the research; YZ performed the majority of experiments; YZ and MO did the major data analysis and organization; JW and QZ helped with experiments and data analysis; JG and BB joined in project discussion and provided technique supports; LD provided the setups of equipment; and MO, LD, and YZ prepared the manuscript.
Funding
This work was supported financially by the National Natural Science Foundation of China (NSFC 11872129), the Natural Science Foundation of Jiangsu Province (BK20181416), and the Jiangsu Provincial Department of Education (MO); National Natural Science Foundation of China (11532003) (LD); National Natural Science Foundation of China (11902051) (BB).
Conflict of interest
The authors declare that the research was conducted in the absence of any commercial or financial relationships that could be construed as a potential conflict of interest.
Publisher’s note
All claims expressed in this article are solely those of the authors and do not necessarily represent those of their affiliated organizations, or those of the publisher, the editors, and the reviewers. Any product that may be evaluated in this article, or claim that may be made by its manufacturer, is not guaranteed or endorsed by the publisher.
Supplementary material
The Supplementary Material for this article can be found online at: https://www.frontiersin.org/articles/10.3389/fcell.2022.942058/full#supplementary-material
References
Alenghat, F. J., and Ingber, D. E. (2002). Mechanotransduction: all signals point to cytoskeleton, matrix, and integrins. Sci. STKE 2002, pe6. doi:10.1126/stke.2002.119.pe6
Alisafaei, F., Chen, X., Leahy, T., Janmey, P. A., and Shenoy, V. B. (2021). Long-range mechanical signaling in biological systems. Soft Matter 17, 241–253. doi:10.1039/d0sm01442g
Baker, B. M., Trappmann, B., Wang, W. Y., Sakar, M. S., Kim, I. L., Shenoy, V. B., et al. (2015). Cell-mediated fibre recruitment drives extracellular matrix mechanosensing in engineered fibrillar microenvironments. Nat. Mat. 14, 1262–1268. doi:10.1038/nmat4444
Brownfield, D. G., Venugopalan, G., Lo, A., Mori, H., Tanner, K., Fletcher, D. A., et al. (2013). Patterned collagen fibers orient branching mammary epithelium through distinct signaling modules. Curr. Biol. 23, 703–709. doi:10.1016/j.cub.2013.03.032
Cai, C., Sun, H., Hu, L., and Fan, Z. (2021). Visualization of integrin molecules by fluorescence imaging and techniques. Biocell 45, 229–257. doi:10.32604/biocell.2021.014338
Choi, S., Yu, J., Kim, W., and Park, K. S. (2021). N-cadherin mediates the migration of bone marrow-derived mesenchymal stem cells toward breast tumor cells. Theranostics 11, 6786–6799. doi:10.7150/thno.59703
Fan, Q., Zheng, Y., Wang, X., Xie, R., Ding, Y., Wang, B., et al. (2021). Dynamically Re-organized collagen fiber bundles transmit mechanical signals and induce strongly correlated cell migration and self-organization. Angew. Chem. Int. Ed. Engl. 60, 11858–11867. doi:10.1002/anie.202016084
Fang, X., Ni, K., Guo, J., Li, Y., Zhou, Y., Sheng, H., et al. (2022). FRET visualization of cyclic stretch-activated ERK via calcium channels mechanosensation while not integrin β1 in airway smooth muscle cells. Front. Cell Dev. Biol. 10, 847852. doi:10.3389/fcell.2022.847852
Guo, C. L., Ouyang, M., Yu, J. Y., Maslov, J., Price, A., and Shen, C. Y. (2012). Long-range mechanical force enables self-assembly of epithelial tubular patterns. Proc. Natl. Acad. Sci. U. S. A. 109, 5576–5582. doi:10.1073/pnas.1114781109
Han, W., Chen, S., Yuan, W., Fan, Q., Tian, J., Wang, X., et al. (2016). Oriented collagen fibers direct tumor cell intravasation. Proc. Natl. Acad. Sci. U. S. A. 113, 11208–11213. doi:10.1073/pnas.1610347113
Han, Y. L., Ronceray, P., Xu, G., Malandrino, A., Kamm, R. D., Lenz, M., et al. (2018). Cell contraction induces long-ranged stress stiffening in the extracellular matrix. Proc. Natl. Acad. Sci. U. S. A. 115, 4075–4080. doi:10.1073/pnas.1722619115
Harris, A. K., Stopak, D., and Wild, P. (1981). Fibroblast traction as a mechanism for collagen morphogenesis. Nature 290, 249–251. doi:10.1038/290249a0
Hino, N., Rossetti, L., Marin-Llaurado, A., Aoki, K., Trepat, X., Matsuda, M., et al. (2020). ERK-mediated mechanochemical waves direct collective cell polarization. Dev. Cell 53, 646–660. doi:10.1016/j.devcel.2020.05.011
Huttenlocher, A., and Horwitz, A. R. (2011). Integrins in cell migration. Cold Spring Harb. Perspect. Biol. 3, a005074. doi:10.1101/cshperspect.a005074
Isomursu, A., Lerche, M., Taskinen, M. E., Ivaska, J., and Peuhu, E. (2019). Integrin signaling and mechanotransduction in regulation of somatic stem cells. Exp. Cell Res. 378, 217–225. doi:10.1016/j.yexcr.2019.01.027
Kanchanawong, P., Shtengel, G., Pasapera, A. M., Ramko, E. B., Davidson, M. W., Hess, H. F., et al. (2010). Nanoscale architecture of integrin-based cell adhesions. Nature 468, 580–584. doi:10.1038/nature09621
Katzberg, A. A. (1959). The mechanism of traction forces in tissue culture. Ann. Surg. 150, 23–28. doi:10.1097/00000658-195907000-00002
Kim, J., Adams, A. A., Gokina, P., Zambrano, B., Jayakumaran, J., Dobrowolski, R., et al. (2020). Mechanical stretch induces myelin protein loss in oligodendrocytes by activating Erk1/2 in a calcium-dependent manner. Glia 68, 2070–2085. doi:10.1002/glia.23827
Kim, T. J., Joo, C., Seong, J., Vafabakhsh, R., Botvinick, E. L., Berns, M. W., et al. (2015). Distinct mechanisms regulating mechanical force-induced Ca(2)(+) signals at the plasma membrane and the ER in human MSCs. Elife 4, e04876. doi:10.7554/eLife.04876
Kuo, I. Y., and Ehrlich, B. E. (2015). Signaling in muscle contraction. Cold Spring Harb. Perspect. Biol. 7, a006023. doi:10.1101/cshperspect.a006023
Leckband, D. E., and de Rooij, J. (2014). Cadherin adhesion and mechanotransduction. Annu. Rev. Cell Dev. Biol. 30, 291–315. doi:10.1146/annurev-cellbio-100913-013212
Li, X., Balagam, R., He, T. F., Lee, P. P., Igoshin, O. A., and Levine, H. (2017). On the mechanism of long-range orientational order of fibroblasts. Proc. Natl. Acad. Sci. U. S. A. 114, 8974–8979. doi:10.1073/pnas.1707210114
Li, Z., Lee, H., and Zhu, C. (2016). Molecular mechanisms of mechanotransduction in integrin-mediated cell-matrix adhesion. Exp. Cell Res. 349, 85–94. doi:10.1016/j.yexcr.2016.10.001
Liu, L., Yu, H., Zhao, H., Wu, Z., Long, Y., Zhang, J., et al. (2020). Matrix-transmitted paratensile signaling enables myofibroblast-fibroblast cross talk in fibrosis expansion. Proc. Natl. Acad. Sci. U. S. A. 117, 10832–10838. doi:10.1073/pnas.1910650117
Long, Y., Niu, Y., Liang, K., and Du, Y. (2022). Mechanical communication in fibrosis progression. Trends Cell Biol. 32, 70–90. doi:10.1016/j.tcb.2021.10.002
Luo, L., Li, E., Zhao, S., Wang, J., Zhu, Z., Liu, Y., et al. (2018). Gasoline exhaust damages spermatogenesis through downregulating α6-integrin and β1-integrin in the rat model. Andrologia 50, e13045. doi:10.1111/and.13045
Ma, X., Schickel, M. E., Stevenson, M. D., Sarang-Sieminski, A. L., Gooch, K. J., Ghadiali, S. N., et al. (2013). Fibers in the extracellular matrix enable long-range stress transmission between cells. Biophys. J. 104, 1410–1418. doi:10.1016/j.bpj.2013.02.017
Mann, A., Sopher, R. S., Goren, S., Shelah, O., Tchaicheeyan, O., and Lesman, A. (2019). Force chains in cell-cell mechanical communication. J. R. Soc. Interface 16, 20190348. doi:10.1098/rsif.2019.0348
Martino, F., Perestrelo, A. R., Vinarsky, V., Pagliari, S., and Forte, G. (2018). Cellular mechanotransduction: from tension to function. Front. Physiol. 9, 824. doi:10.3389/fphys.2018.00824
Mohammadi, H., Janmey, P. A., and McCulloch, C. A. (2014). Lateral boundary mechanosensing by adherent cells in a collagen gel system. Biomaterials 35, 1138–1149. doi:10.1016/j.biomaterials.2013.10.059
Nitsan, I., Drori, S., Lewis, Y. E., Cohen, S., and Tzlil, S. (2016). Mechanical communication in cardiac cell synchronized beating. Nat. Phys. 12, 472–477. doi:10.1038/nphys3619
Ouyang, M., Qian, Z., Bu, B., Jin, Y., Wang, J., Zhu, Y., et al. (2020). Sensing traction force on the matrix induces cell-cell distant mechanical communications for self-assembly. ACS Biomater. Sci. Eng. 6, 5833–5848. doi:10.1021/acsbiomaterials.0c01035
Pakshir, P., Alizadehgiashi, M., Wong, B., Coelho, N. M., Chen, X., Gong, Z., et al. (2019). Dynamic fibroblast contractions attract remote macrophages in fibrillar collagen matrix. Nat. Commun. 10, 1850. doi:10.1038/s41467-019-09709-6
Panzetta, V., Fusco, S., and Netti, P. A. (2019). Cell mechanosensing is regulated by substrate strain energy rather than stiffness. Proc. Natl. Acad. Sci. U. S. A. 116, 22004–22013. doi:10.1073/pnas.1904660116
Reinhart-King, C. A., Dembo, M., and Hammer, D. A. (2008). Cell-cell mechanical communication through compliant substrates. Biophys. J. 95, 6044–6051. doi:10.1529/biophysj.107.127662
Sapir, L., and Tzlil, S. (2017). Talking over the extracellular matrix: how do cells communicate mechanically? Semin. Cell Dev. Biol. 71, 99–105. doi:10.1016/j.semcdb.2017.06.010
Sawhney, R. K., and Howard, J. (2002). Slow local movements of collagen fibers by fibroblasts drive the rapid global self-organization of collagen gels. J. Cell Biol. 157, 1083–1091. doi:10.1083/jcb.200203069
Shellard, A., and Mayor, R. (2021). Collective durotaxis along a self-generated stiffness gradient in vivo. Nature 600, 690–694. doi:10.1038/s41586-021-04210-x
Shellard, A., Szabo, A., Trepat, X., and Mayor, R. (2018). Supracellular contraction at the rear of neural crest cell groups drives collective chemotaxis. Science 362, 339–343. doi:10.1126/science.aau3301
Shi, Q., Ghosh, R. P., Engelke, H., Rycroft, C. H., Cassereau, L., Sethian, J. A., et al. (2014). Rapid disorganization of mechanically interacting systems of mammary acini. Proc. Natl. Acad. Sci. U. S. A. 111, 658–663. doi:10.1073/pnas.1311312110
Sopher, R. S., Tokash, H., Natan, S., Sharabi, M., Shelah, O., Tchaicheeyan, O., et al. (2018). Nonlinear elasticity of the ECM fibers facilitates efficient intercellular communication. Biophys. J. 115, 1357–1370. doi:10.1016/j.bpj.2018.07.036
Stopak, D., and Harris, A. K. (1982). Connective tissue morphogenesis by fibroblast traction. I. Tissue culture observations. Dev. Biol. 90, 383–398. doi:10.1016/0012-1606(82)90388-8
Sun, Z., Guo, S. S., and Fassler, R. (2016). Integrin-mediated mechanotransduction. J. Cell Biol. 215, 445–456. doi:10.1083/jcb.201609037
Sunyer, R., Conte, V., Escribano, J., Elosegui-Artola, A., Labernadie, A., Valon, L., et al. (2016). Collective cell durotaxis emerges from long-range intercellular force transmission. Science 353, 1157–1161. doi:10.1126/science.aaf7119
Wang, H., Abhilash, A. S., Chen, C. S., Wells, R. G., and Shenoy, V. B. (2014). Long-range force transmission in fibrous matrices enabled by tension-driven alignment of fibers. Biophys. J. 107, 2592–2603. doi:10.1016/j.bpj.2014.09.044
Wang, J., Guo, J., Che, B., Ouyang, M., and Deng, L. (2020). Cell motion-coordinated fibrillar assembly of soluble collagen I to promote MDCK cell branching formation. Biochem. Biophys. Res. Commun. 524, 317–324. doi:10.1016/j.bbrc.2020.01.019
Wang, Y., Lu, Y., Luo, M., Shi, X., Pan, Y., Zeng, H., et al. (2016). Evaluation of pharmacological relaxation effect of the natural product naringin on in vitro cultured airway smooth muscle cells and in vivo ovalbumin-induced asthma Balb/c mice. Biomed. Rep. 5, 715–722. doi:10.3892/br.2016.797
Weber, G. F., Bjerke, M. A., and DeSimone, D. W. (2011). Integrins and cadherins join forces to form adhesive networks. J. Cell Sci. 124, 1183–1193. doi:10.1242/jcs.064618
Weiss, P. (1929). Erzwingung elementarer strukturverschiedenheiten am in vitro wachsenden gewebe: die wirkung mechanischer spannung auf richtung und intensität des ewebewachstums und ihre analyse. Wilhelm Roux Arch. Entwickl. Mech. Org. 116, 438–554. doi:10.1007/bf02145237
Winer, J. P., Oake, S., and Janmey, P. A. (2009). Non-linear elasticity of extracellular matrices enables contractile cells to communicate local position and orientation. PLoS One 4, e6382. doi:10.1371/journal.pone.0006382
Keywords: cell mechanical communications, mechanosensation, calcium channels, integrin, N-cadherin
Citation: Ouyang M, Zhu Y, Wang J, Zhang Q, Hu Y, Bu B, Guo J and Deng L (2022) Mechanical communication-associated cell directional migration and branching connections mediated by calcium channels, integrin β1, and N-cadherin. Front. Cell Dev. Biol. 10:942058. doi: 10.3389/fcell.2022.942058
Received: 12 May 2022; Accepted: 18 July 2022;
Published: 16 August 2022.
Edited by:
Bo Dong, Ocean University of China, ChinaReviewed by:
Nagaraj Balasubramanian, Indian Institute of Science Education and Research, IndiaYiwei Li, Huazhong University of Science and Technology, China
Copyright © 2022 Ouyang, Zhu, Wang, Zhang, Hu, Bu, Guo and Deng. This is an open-access article distributed under the terms of the Creative Commons Attribution License (CC BY). The use, distribution or reproduction in other forums is permitted, provided the original author(s) and the copyright owner(s) are credited and that the original publication in this journal is cited, in accordance with accepted academic practice. No use, distribution or reproduction is permitted which does not comply with these terms.
*Correspondence: Mingxing Ouyang, bXhvdXlhbmdAY2N6dS5lZHUuY24=; Linhong Deng, ZGxoQGNjenUuZWR1LmNu
†These authors have contributed equally to this work and share first authorship