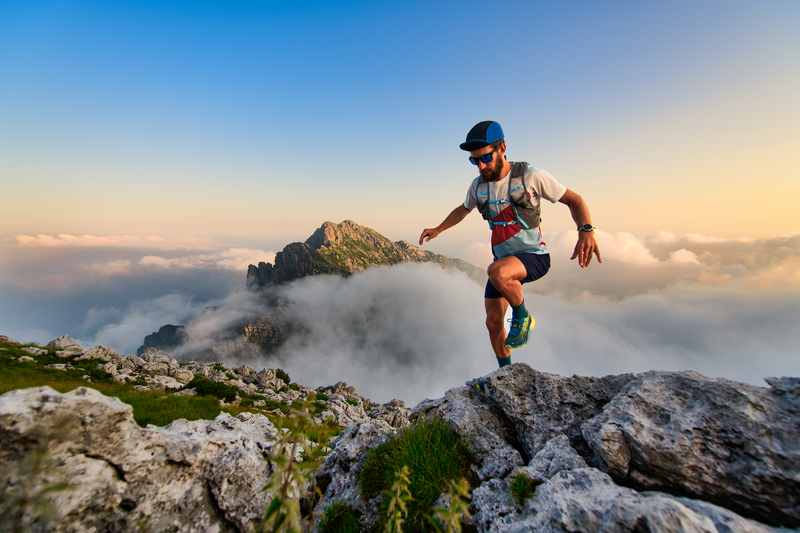
95% of researchers rate our articles as excellent or good
Learn more about the work of our research integrity team to safeguard the quality of each article we publish.
Find out more
MINI REVIEW article
Front. Cell Dev. Biol. , 29 June 2022
Sec. Cell Growth and Division
Volume 10 - 2022 | https://doi.org/10.3389/fcell.2022.941893
This article is part of the Research Topic Editors' Showcase 2021: Insights in Cell Growth and Division View all 11 articles
Adult neural stem and progenitor cells (aNSPCs) persist lifelong in teleost models in diverse stem cell niches of the brain and spinal cord. Fish maintain developmental stem cell populations throughout life, including both neuro-epithelial cells (NECs) and radial-glial cells (RGCs). Within stem cell domains of the brain, RGCs persist in a cycling or quiescent state, whereas NECs continuously divide. Heterogeneous populations of RGCs also sit adjacent the central canal of the spinal cord, showing infrequent proliferative activity under homeostasis. With the rise of the zebrafish (Danio rerio) model to study adult neurogenesis and neuroregeneration in the central nervous system (CNS), it has become evident that aNSPC proliferation is regulated by a wealth of stimuli that may be coupled with biological function. Growing evidence suggests that aNSPCs are sensitive to environmental cues, social interactions, nutrient availability, and neurotrauma for example, and that distinct stem and progenitor cell populations alter their cell cycle activity accordingly. Such stimuli appear to act as triggers to either turn on normally dormant aNSPCs or modulate constitutive rates of niche-specific cell cycle behaviour. Defining the various forms of stimuli that influence RGC and NEC proliferation, and identifying the molecular regulators responsible, will strengthen our understanding of the connection between aNSPC activity and their biological significance. In this review, we aim to bring together the current state of knowledge on aNSPCs from studies investigating the zebrafish CNS, while highlighting emerging cell cycle regulators and outstanding questions that will help to advance this fascinating field of stem cell biology.
Teleost fishes serve as exceptional models to study the cell cycle dynamics and function of adult neural stem and progenitor cells (aNSPCs) throughout the central nervous system (CNS). The lifelong presence of proliferating aNSPCs across diverse stem cell niches of the brain (Zupanc et al., 2005; Grandel et al., 2006), along with their remarkable neuroregenerative capacity following brain and spinal cord injury (Zupanc and Sirbulescu, 2012) make teleosts extremely attractive to study. These attributes have allowed researchers to take advantage of fish models to study the biological significance of adult neurogenesis (Lindsey and Tropepe, 2006) as well as the process of timely brain and spinal cord repair (Becker and Becker, 2008). Adult neurogenesis is defined as a lineage-directed process that commences with dividing aNSPCs that generate daughter cells fated towards a neuronal phenotype. A major difference between constitutive adult neurogenesis and regenerative neurogenesis that occurs after CNS damage, is that the latter largely relies on the activation of normally quiescent aNSPCs to re-enter the cell cycle. A fundamental question under homeostasis and following injury is what suite of factors are responsible for controlling aNSPC activity.
Distinguished as the most diverse vertebrate class and having adapted to nearly every aquatic environment (Kotrschal and Palzenberger, 1992), teleosts are excellent examples to explore how differences in habitat, environment, social interactions, and neurotrauma can impinge upon aNSPC function. The zebrafish (Danio rerio) has become one of the most popular laboratory models to study these factors in the brain and spinal cord. The social nature and complex behaviours displayed by this species (Kalueff et al., 2013), in combination with the multitude of molecular tools and small size of the CNS for imaging, has set the zebrafish apart to study neurogenesis and neurorepair. Unlike amniotes (Kriegstein and Alvarez-Buylla, 2009), fish retain developmental stem cell populations over ontogeny, including radial-glial cells (RGCs; aka ependymoglia in the spinal cord) in a quiescent or cycling state, and constitutively proliferating neuro-epithelial cells (NECs). These cells are distributed across diverse integrative and sensory niches of the brain and spinal cord (Lindsey et al., 2018). Therefore, this model offers the opportunity to uncover how unique biological contexts can stimulate aNSPC phenotypes (Figure 1), and help reveal the mechanisms regulating cell cycle activity.
FIGURE 1. The many regulators of niche-specific aNSPCs in the adult zebrafish CNS. Mid-sagittal view of the zebrafish brain and spinal cord displaying major aNSPC niches and the array of homeostatic, injury-induced, or neurodegenerative regulators that may control their cell cycle dynamics. OB, olfactory bulbs; Tel, telencephalon; Dm, medial dorsal pallium; Dl, lateral dorsal pallium; Vv, ventral ventricular zone; TeO, optic tectum; PoA, preoptic area; H, hypothalamus; CCe, corpus cerebelli; LX/LVII, vagal and facial lobes; SC, spinal cord. Dark blue indicates approximate location of brain ventricles and the spinal cord central canal.
Here, we provide an overview of the recent factors understood to regulate aNSPC cell cycle dynamics in teleosts, focusing on studies from the zebrafish model (summarized in Table 1). A key element of this review is to synthesize our knowledge of how day-to-day environmental stimuli can modulate constitutive rates of cell proliferation; an area poorly investigated in fish. We conclude by discussing key outstanding questions and available techniques to move these forward, to yield novel insight regarding the activity of aNSPCs under physiological and pathological conditions.
Zebrafish are highly social species in the wild and under laboratory conditions (Suriyampola et al., 2016). Social interactions can take the form of predator-prey encounters, mating opportunities, conspecific relationships, and the formation of social hierarchies. This daily social plasticity commonly involves one or more chemosensory or visual cues, as well as the possibility of changes in swimming performance. This suggests that a broad range of adult niches could be implicated in aNSPC dynamics, including those processing sensory input and potentially even the spinal cord where additional motor neurons may be required to accommodate increased swimming. A consequence of these interactions is their effect on the physiology of the animal, such as the stress axis, and how this information acts to control aNSPCs behaviour.
In thinking how social behaviours modulate aNSPC activity, a key question is what constitutes a physiological stressful event. Zebrafish RGCs are known to possess the glucocorticoid receptor Nr3c1 (Nelson et al., 2019), making them sensitive to changes in circulating cortisol. Higher cortisol levels have been identified in group-housed compared to individually-housed zebrafish (Onarheim et al., 2022), arguing against the idea that isolation consistently increases stress in social animals. One hypothesis may be that group composition, including sex ratios, fish size, and potential for hierarchies, are potential drivers of stress levels. Cues in the environment of isolated fish further appear to play a role in regulating cortisol levels. This has been illustrated by zebrafish having higher cortisol in an enriched-isolated context compared to a barren-isolated context (von Krogh et al., 2010). Work by Lindsey and Tropepe (2014) rather found that cortisol levels strongly correlated with social context, with the effect on aNSPC proliferation or neuronal differentiation being niche-specific. Social novelty and isolation revealed lower cortisol levels than grouped animals, with aNSPCs in sensory niches having the largest reduction in cell cycle activity. This work highlights the importance of pre-existing social experiences in shaping the future stress response and probability of stimulating niche-specific aNSPCs.
A small number of studies have also centered around the role of social hierarchies in driving aNSPC behaviour. One interesting report focused on sex-specific differences. In this study, the authors illustrated that subordinate males displayed reduced cell proliferation and neurogenesis in the dorsal telencephalon, along with increased cortisol levels (Tea et al., 2019). In females, however, no change was observed in dominant or subordinate animals when compared to group-housed controls. This suggests the possibility that hormonal differences may play a part in the cellular activity of aNSPCs. This finding aligns with earlier work studying socially suppressed subordinate male cichlids, where cell proliferation in the brain was lowest compared with dominant animals (Maruska et al., 2012). Notably, this study showed that if males were given the opportunity to rise in rank, the proportion of dividing aNSPCs increased in parallel. In the zebrafish, we now understand that changes in social status are closely correlated with gene expression patterns involved in neural plasticity in a niche-specific manner (Teles et al., 2016).
In the past few years, newer studies have emerged addressing the role of hormones and diet in regulating aNSPC activity in the zebrafish. This recent focus could provide valuable insight towards the importance of sex-specific differences, reproductive cycles, seasonality, food availability and nutrient composition in teleost fish. Several of these factors could also be increasingly important to consider during the design phase of experiments aimed at studying adult neurogenic plasticity.
Interestingly, in zebrafish only RGCs and not NECs are known to express the estrogen-synthesizing enzyme, aromatase-B (Pellegrini et al., 2016). Upon administration of estrogen to female zebrafish a reduction in cell proliferation in multiple brain regions has been reported, with the greatest impact in the dorsal/ventral telencephalon, preoptic area, hypothalamus, and cerebellum (Makantasi and Dermon, 2014). Within the niche of the preoptic area, animals treated with gonadotropin releasing hormone, but not testosterone produce an increase in cell division in non-RGC stem cell populations (Ceriani and Whitlock, 2021). Alternatively, inhibiting aNSPCs residing in the hypothalamus using the serotonin blocker tryptophan hydroxylase attenuates aNSPC proliferation, illustrating the dependency of stem cells in this domain on serotonin (Pérez et al., 2013).
How zebrafish diet or feeding regime relates to proliferative behaviour of aNSPCs is poorly characterized to date. Currently, studies have taken the form of either exposure to a high fat diet or starvation. Chronic hyperglycemia in adult zebrafish, for instance, was reported to impair homeostatic neurogenesis in the telencephalon, midbrain, and cerebellum, while also having a pro-inflammatory and oxidative stress effect (Dorsemans et al., 2017; Stankiewicz et al., 2017; Ghaddar et al., 2021). Conversely, 10 weeks of reduced food intake appears to be insufficient to alter aNSPC activity in the forebrain (Arslan-Ergul et al., 2016), but a 5 week starvation is adequate to decrease aNSPC proliferation in the optic tectum (Benítez-Santana et al., 2016). Additional niche-wide studies focusing on food type, nutrient composition, and feeding frequency undoubtedly would be beneficial to determine if distinct aNSPC populations are differentially affected and how this relates to changes in cell metabolism.
Sensory input is a potent regulator of animal behaviour. In an aquatic environment teleosts receive this information from visual cues, olfactory and taste cues (i.e., chemosensory), as well as lateral line input (i.e., mechanosensory). A unique feature of zebrafish is that aNSPCs exist in neurogenic niches within primary processing sensory structures of the mature brain, including the forebrain olfactory bulbs (smell), midbrain optic tectum (vision), and hindbrain vagal/facial lobes (taste; Zupanc et al., 2005). These sensory domains offer the ability to study a range of modality-specific cues and their effect on aNSPC lineage activity. With several teleost models having conserved sensory niches, including the zebrafish, medaka, brown ghost knifefish, goldfish, and killifish (Ganz and Brand, 2016), this field is wide open to compare the functional role of aNSPCs using species-specific biologically relevant forms of sensory stimuli.
Studies applying forms of environmental enrichment or selective visual cues have shown a strong link between sensory input and aNSPC activity. A pointed example of how the mere opportunity for visual stimuli can induce changes in long-term aNSPC activity comes from recent work by Dunlap et al. (2021). Here, socially acclimated zebrafish that were first isolated, before being exposed to conspecifics in an adjacent tank chamber, was sufficient alone to enhance forebrain aNSPC activity. The above finding is supported by an early study exposing zebrafish to an enriched environment adorned with aquatic plants and gravel, or devoid of such items, showing a general increase in proliferation of forebrain aNSPCs with enrichment (von Krogh et al., 2010). An outstanding question is what effect does visual enrichment have on parent or progenitor NECs along the tectal marginal zone (Lindsey et al., 2018a), that would be predicted to be modulated.
Visual and chemosensory assays have also been employed to more directly test the effect of modality-specific sensory input on the activity of aNSPCs in corresponding sensory niches. Exposing zebrafish to monochromatic light has been demonstrated to decrease the proportion of cycling aNSPCs in the tectal sensory niche (Lindsey et al., 2014). Experiments using larval zebrafish also show that visual restriction impedes neurogenesis and functional integration into the optic tectum, correlating with reduced BDNF production (Boulanger-Weill et al., 2017; Hall and Tropepe, 2018a). In contrast, a 7-day treatment using a chemosensory assay resulted in an increase in neuronal survival but limited effect on RGC proliferation in the bulbs and vagal lobe (Lindsey et al., 2014). A major finding from this work was that modality-specific sensory input triggered the relevant sensory processing niche, and not those coding alternative modalities.
Taking advantage of sensory niches in the adult zebrafish brain further offers the chance to study the functional significance of aNSPCs for learning. Using sensory paradigms to explore the impact of task complexity on endogenous neurogenesis arising from sensory zones would provide new insight not possible in mammals. For instance, building on previous olfactory learning paradigms (Braubach et al., 2009), researchers can now ask how olfactory learning might modulate aNSPC activity along the Rostral Migratory Stream (RMS; Adolf et al., 2006; Kishimoto et al., 2012) as compared to resident RGC behaviour directly in the bulbs. This would provide valuable comparative data with mammals who maintain a lifelong RMS (Bond et al., 2015). In addition, since learning implicitly involved a memory component, with the dorsal lateral niche of the telencephalon homologous to the mammalian hippocampus (Ganz et al., 2015), the combined activity of aNSPCs in sensory and cognitive niches can be examined.
Modulation of spinal cord RGCs for the most part has not been explored as this population resides mainly in a quiescent state. Recently, one of the first studies of its kind has shown that zebrafish subjected to increased swimming using a swim tunnel was sufficient to enhance cell cycle proliferation of normally dormant spinal cord ependymoglia, and the generation of newborn motor neurons (Chang et al., 2021). Applying the same paradigm after spinal cord injury showed a similar trend, proposing that increased swimming exercise modulates neurogenesis. Conversely, experiments performing movement restraint in larval zebrafish have illustrated a decrease in neural stem and progenitor proliferation in the developing forebrain, though how robust this effect is across the neuro-axis remains unknown (Hall and Tropepe, 2018b). Together, these studies provide early support for the role of “exercise” in modulating baseline levels of aNSPCs in the brain and spinal cord of teleost models.
Much effort has been placed on understanding the behaviour of RGCs and NECs following CNS damage compared to homeostatic modulation. More recently, this has also included examination of aNSPCs in a neurodegenerative context. Common to brain and spinal cord injury is the involvement of quiescent RGCs that are awakened following insult to re-enter the cell cycle and repopulate lost neuronal subtypes. In some instances, constitutively active NECs have been shown to additionally contribute to repair. Growing evidence suggests that aNSPCs retain diverse regenerative capacities that differ by stem cell niche in the adult CNS (Lindsey et al., 2018a), but their role in neurodegenerative diseases is only now beginning to be uncovered. This implies the need to better understand the balance between the factors comprising the damaged stem cell niche, and the intrinsic regenerative programs of distinct aNSPC populations. With many excellent reviews already published on this topic (Alunni and Bally-Cuif, 2016; Alunni et al., 2020), here we strive to provide a brief synopsis of the newest developments in this area to contrast with the regulators of aNSPCs observed under physiological conditions.
In the forebrain and spinal cord, RGCs drive the repair process. Accordingly, dorsal forebrain stab injury leads to the activation of RGCs, in addition to oligodendrocyte precursor cells (OPCs), that serve to replenish lost neurons and oligodendrocytes, respectively (Kroehne et al., 2011; Baumgart et al., 2012; Kishimoto et al., 2012; Barbosa et al., 2015; Sanchez-Gonzales et al., 2022). Most recently, transcriptomic analysis has provided insight regarding early proliferative signatures of RGCs and OPCs in the telencephalon and spinal cord after injury (Tsata et al., 2020; Demirci et al., 2022; Sanchez-Gonzales et al., 2022), adding to our existing knowledge of the role of cxcr5 and Gata3 during neurorepair (Kizil et al., 2012a; 2012b). In the dorsal forebrain, genes regulating stem cell quiescence under homeostasis, such as mdka, have also recently been shown to continue to be expressed following brain injury in non-reactive, RGC populations, suggesting a potential mechanism to prevent NSPC pool exhaustion (Lübke et al., 2022). This finding will add valuable information to our deep-rooted understanding of the role of Notch signalling in maintaining the quiescent state of NSPCs (Chapouton et al., 2010; Anand and Mondal, 2017). In the spinal cord, upon injury ependymoglia enter the cell cycle to produce motor neurons for functional swimming recovery (Reimer et al., 2009). In both the forebrain and spinal cord, numerous developmental regulatory pathways, such as Wnt, Notch, Shh, and ID1/BMP, are recapitulated following injury (Cardozo et al., 2017; Alunni et al., 2020; Zhang et al., 2021). Additionally, studies in the adult forebrain (Kyritsis et al., 2012) and larval spinal cord (Tsarouchas et al., 2018) confirm that activation of the immune response post-injury is critical to induce RGCs to regenerate. The rapid repair offered by the larval zebrafish following spinal cord injury has also gained traction as an efficient model for drug screening to identify therapeutics to test in mammals to improve spinal cord repair (Chapela et al., 2019; Nelson and Granato, 2022). Such studies equally afford the opportunity to examine how NSPC proliferative activity and intrinsic signaling pathways are modulated with application of these small molecules in an organism capable of successful spinal cord regeneration. Comparing the interaction between the immune response and RGCs or OPCs of the mature brain and spinal cord would be of great interest to identify CNS-wide themes in neuroregeneration. Unfortunately, detailed studies investigating the immune response following spinal cord injury in juvenile and adult stages are still lacking.
A small number of studies have also underscored the contribution of constitutively cycling NECs following brain injury. In the optic tectum, quiescent RGCs reside along the roof of the tectal ventricle whereas a pool of NECs sit at the midline tectal marginal zone, continuously furnishing the optic tectum with a small number of newborn neurons (Ito et al., 2010). Following tectal injury, it was reported that NECs amplify their cell cycle rates and neuronal output, while progeny of activated RGCs produce only newborn RGCs (Lindsey et al., 2019). Tectal injuries more proximal to the NEC population and in younger adult animals nevertheless showed evidence that RGCs could generate newborn neurons (Shimizu et al., 2018), suggesting that adult age appears to play a role in the regenerative potential of aNSPCs (Edelmann et al., 2013). This and more recent work has implicated Wnt and IL-6/STAT3 pathways in regulating RGC activity after injury in the tectum (Shimizu et al., 2018; 2021). In the adult cerebellum, NECs also appear to be the main contributor to restorative neurogenesis (Kaslin et al., 2017). However, in juvenile fish, RGCs of the cerebellum play a more prominent role in the regenerative process alongside NECs, giving rise to neuronal phenotypes similar to constitutive neurogenesis. Collectively, work on RGCs and NECs provide growing evidence that the regenerative process in zebrafish is accomplished by a combination of reactivated RGCs and constitutively proliferating NECs that are influenced by cellular senescence.
Models of Alzheimer’s and Parkinson’s disease have also been established in the zebrafish, permitting studies of aNSPC activity during the process of neurodegeneration. Alzheimer’s models along with single-cell transcriptomics have illustrated neurodegenerative specific regulation of aNSPCs. These studies show that induced inflammation leads to a cascade of events, initiated by the activation of the IL4/STAT6 pathway. Subsequent downregulation of serotonin metabolism and promotion of BDNF in turn increase aNSPCs proliferation in a subtype and niche-specific manner (Bhattarai et al., 2016; Cosacak et al., 2019; Bhattarai et al., 2020). Parallel studies in older adult zebrafish showed similar levels of aNSPC proliferation, but a diminished immune response and fewer newborn neurons (Bhattarai et al., 2017). Modelling Parkinson’s disease in the zebrafish, reports have shown that ablation of dopaminergic neurons leads to inflammation and RGC progenitor activity in the diencephalon to repopulate newborn dopaminergic neurons (Caldwell et al., 2019). Inducible transgenic lines have also come available to temporally ablate dopaminergic neurons (Godoy et al., 2015), providing a valuable tool to better interrogate this disease. While still new to the field of neurodegeneration, the zebrafish model offers many excellent advantages to elucidate the response of aNSPCs following Alzheimer’s or Parkinson’s disease that have yet to be capitalized upon.
The zebrafish is a tractable model to probe the mechanisms regulating aNSPC function. Still many outstanding questions persist. First, many niches of the brain are composed of populations of both quiescent and actively cycling RGCs. Adult neurogenesis by definition focuses on constitutively proliferating cells, whereas CNS regenerative studies concentrate primarily on the reactivation of quiescent aNSPCs. Whether certain forms of stimuli under homeostasis are sufficient to push quiescent RGCs into a cycling state, or a subpopulation of normally dividing RGCs of the forebrain might contribute to regeneration, is unclear. Equally unknown is whether RGCs or NECs can undergo shifts in their multipotency to generate specific neuronal lineages under different contexts, and if so, what are the intrinsic programs or changes in the microenvironment responsible for this cellular plasticity? Second, without knowledge of the molecular signature of similar aNSPCs found in different adult niches, it is unclear whether their gene expression profiles align with their varied response to environmental or injury-induced cues. Many cell autonomous features also remain to be understood, including cell metabolism (Santoro, 2014), senescence (Da Silva-Álvarez et al., 2020), and the role of the unfolded protein response (Clark et al., 2020). Considering the stem cell niche, most of our knowledge is limited to the role of the immune response post-injury in activating aNSPC populations. Studying the involvement of neighboring cells, the extracellular matrix (Kim et al., 2018), and vasculature (Chen et al., 2019) is paramount to gain broader knowledge of niche-specific regulation of aNSPCs for successful neurorepair.
Methods to study the activity of zebrafish aNSPCs are extensive. These include the use of thymidine analogues such as BrdU and EdU, that label cells undergoing DNA synthesis (Cavanagh et al., 2011), as well as the endogenous cell cycle marker PCNA (Schönenberger et al., 2015). The dual Fucci line further allows changes in cell cycle phases to be fluorescently monitored (Bouldin and Kimelman, 2014); a generally underutilized tool thus far. More recent tamoxifen-inducible Cre-lox systems in zebrafish have allowed temporal colour-switching of aNSPCs to monitor population dynamics (Mosimann et al., 2011). Above all, the zebrabow system permits the most advanced multi-colour labeling to faithfully track stem cell lineages arising from parent stem cells (Pan et al., 2013). This suite of tools provides the opportunity to gain deeper insight towards aNSPC context-dependent activity. Combining these techniques with the multiple imaging approaches in the zebrafish, including live in vivo studies, 3-dimensional imaging of the CNS (Lindsey et al., 2018b), and advanced electron microscopy (Oorschot et al., 2021), the next decade promises to hold many exciting discoveries regarding teleost aNSPC activity, regulation, and biological significance.
AC, LT, and BWL equally contributed to the conceptualization, writing, editing, and figure/table design of the manuscript.
This work is supported by a Natural Sciences and Engineering Research Council of Canada (NSERC) Discovery Grant, and a Research Manitoba New Investigator Operating Grant, awarded to BWL.
The authors declare that the research was conducted in the absence of any commercial or financial relationships that could be construed as a potential conflict of interest.
All claims expressed in this article are solely those of the authors and do not necessarily represent those of their affiliated organizations, or those of the publisher, the editors and the reviewers. Any product that may be evaluated in this article, or claim that may be made by its manufacturer, is not guaranteed or endorsed by the publisher.
We thank Masozi Palata and Suhaila Selamat for their helpful comments in reviewing and improving the quality of this manuscript.
Adolf, B., Chapouton, P., Lam, C. S., Topp, S., Tannhäuser, B., Strähle, U., et al. (2006). Conserved and acquired features of adult neurogenesis in the zebrafish telencephalon. Dev. Biol. 295, 278–293. doi:10.1016/j.ydbio.2006.03.023
Alunni, A., and Bally-Cuif, L. (2016). A comparative view of regenerative neurogenesis in vertebrates. Development 143, 741–753. doi:10.1242/dev.122796
Alunni, A., Coolen, M., Foucher, I., and Bally-Cuif, L. (2020). “Chapter 26 - Neurogenesis in zebrafish,” in Patterning and Cell Type Specification in the Developing CNS and PNS. Editors J. Rubenstein, P. Rakic, B. Chen, and K. Y. Kwan. Second Edition (Academic Press), 643–697. doi:10.1016/b978-0-12-814405-3.00026-6
Anand, S. K., and Mondal, A. C. (2017). Cellular and molecular attributes of neural stem cell niches in adult zebrafish brain. Dev. Neurobio. 77 (10), 1188–1205. doi:10.1002/dneu.22508
Arslan-Ergul, A., Erbaba, B., Karoglu, E. T., Halim, D. O., and Adams, M. M. (2016). Short-term dietary restriction in old zebrafish changes cell senescence mechanisms. Neuroscience 334, 64–75. doi:10.1016/j.neuroscience.2016.07.033
Barbosa, J. S., Sanchez-Gonzalez, R., Di Giaimo, R., Baumgart, E. V., Theis, F. J., Gotz, M., et al. (2015). Live imaging of adult neural stem cell behavior in the intact and injured zebrafish brain. Science 348, 789–793. doi:10.1126/science.aaa2729
Baumgart, E. V., Barbosa, J. S., Bally-Cuif, L., Götz, M., and Ninkovic, J. (2012). Stab wound injury of the zebrafish telencephalon: A model for comparative analysis of reactive gliosis. Glia 60, 343–357. doi:10.1002/glia.22269
Becker, C. G., and Becker, T. (2008). Adult zebrafish as a model for successful central nervous system regeneration. Restor. Neurol. Neurosci. 26, 71–80.
Benítez-Santana, T., Simion, M., Corraze, G., Médale, F., and Joly, J.-S. (2016). Effect of nutrient availability on progenitor cells in zebrafish (Danio Rerio). Dev. Neurobiol. 77, 26–38. doi:10.1002/dneu.22406
Bhattarai, P., Thomas, A. K., Cosacak, M. I., Papadimitriou, C., Mashkaryan, V., Froc, C., et al. (2016). IL4/STAT6 Signaling activates neural stem cell proliferation and neurogenesis upon amyloid-β42 aggregation in adult zebrafish brain. Cell Rep. 17, 941–948. doi:10.1016/j.celrep.2016.09.075
Bhattarai, P., Thomas, A. K., Zhang, Y., and Kizil, C. (2017). The effects of aging on Amyloid-β42-induced neurodegeneration and regeneration in adult zebrafish brain. Neurogenesis 4, e1322666. doi:10.1080/23262133.2017.1322666
Bhattarai, P., Cosacak, M. I., Mashkaryan, V., Demir, S., Popova, S. D., Govindarajan, N., et al. (2020). Neuron-glia interaction through Serotonin-BDNF-NGFR axis enables regenerative neurogenesis in Alzheimer's model of adult zebrafish brain. PLoS Biol. 18, e3000585. doi:10.1371/journal.pbio.3000585
Bond, A. M., Ming, G.-L., and Song, H. (2015). Adult mammalian neural stem cells and neurogenesis: five decades later. Cell Stem Cell 17, 385–395. doi:10.1016/j.stem.2015.09.003
Boulanger-Weill, J., Candat, V., Jouary, A., Romano, S. A., Pérez-Schuster, V., and Sumbre, G. (2017). Functional interactions between newborn and mature neurons leading to integration into established neuronal circuits. Curr. Biol. 27, 1707–1720.e5. doi:10.1016/j.cub.2017.05.029
Bouldin, C. M., and Kimelman, D. (2014). Dual fucci: a new transgenic line for studying the cell cycle from embryos to adults. Zebrafish 11, 182–183. doi:10.1089/zeb.2014.0986
Braubach, O. R., Wood, H.-D., Gadbois, S., Fine, A., and Croll, R. P. (2009). Olfactory conditioning in the zebrafish (Danio rerio). Behav. Brain Res. 198, 190–198. doi:10.1016/j.bbr.2008.10.044
Caldwell, L. J., Davies, N. O., Cavone, L., Mysiak, K. S., Semenova, S. A., Panula, P., et al. (2019). Regeneration of dopaminergic neurons in adult zebrafish depends on immune system activation and differs for distinct populations. J. Neurosci. 39, 4694–4713. doi:10.1523/jneurosci.2706-18.2019
Cardozo, M. J., Mysiak, K. S., Becker, T., and Becker, C. G. (2017). Reduce, reuse, recycle - Developmental signals in spinal cord regeneration. Dev. Biol. 432, 53–62. doi:10.1016/j.ydbio.2017.05.011
Cavanagh, B. L., Walker, T., Norazit, A., and Meedeniya, A. C. B. (2011). Thymidine analogues for tracking DNA synthesis. Molecules 16, 7980–7993. doi:10.3390/molecules16097980
Ceriani, R., and Whitlock, K. E. (2021). Gonadotropin releasing hormone (Gnrh) triggers neurogenesis in the hypothalamus of adult zebrafish. Int. J. Mol. Sci. 22, 5926. doi:10.3390/ijms22115926
Chang, W., Pedroni, A., Bertuzzi, M., Kizil, C., Simon, A., and Ampatzis, K. (2021). Locomotion dependent neuron-glia interactions control neurogenesis and regeneration in the adult zebrafish spinal cord. Nat. Commun. 12, 4857. doi:10.1038/s41467-021-25052-1
Chapela, D., Sousa, S., Martins, I., Cristóvão, A. M., Pinto, P., Corte-Real, S., et al. (2019). A zebrafish drug screening platform boosts the discovery of novel therapeutics for spinal cord injury in mammals. Sci. Rep. 9, 10475. doi:10.1038/s41598-019-47006-w
Chapouton, P., Skupien, P., Hesl, B., Coolen, M., Moore, J. C., Madelaine, R., et al. (2010). Notch activity levels control the balance between quiescence and recruitment of adult neural stem cells. J. Neurosci. 30, 7961–7974. doi:10.1523/JNEUROSCI.6170-09.2010
Chen, J., He, J., Ni, R., Yang, Q., Zhang, Y., and Luo, L. (2019). Cerebrovascular injuries induce lymphatic invasion into brain parenchyma to guide vascular regeneration in zebrafish. Dev. Cell 49, 697–710.e5. doi:10.1016/j.devcel.2019.03.022
Clark, E. M., Nonarath, H. J. T., Bostrom, J. R., and Link, B. A. (2020). Establishment and validation of an endoplasmic reticulum stress reporter to monitor zebrafish ATF6 activity in development and disease. Dis. Model Mech. 13, dmm041426. doi:10.1242/dmm.041426
Cosacak, M. I., Bhattarai, P., Reinhardt, S., Petzold, A., Dahl, A., Zhang, Y., et al. (2019). Single-Cell transcriptomics analyses of neural stem cell heterogeneity and contextual plasticity in a zebrafish brain model of amyloid toxicity. Cell Rep. 27, 1307–1318.e3. doi:10.1016/j.celrep.2019.03.090
Da Silva-Álvarez, S., Guerra-Varela, J., Sobrido-Cameán, D., Quelle, A., Barreiro-Iglesias, A., Sánchez, L., et al. (2020). Cell senescence contributes to tissue regeneration in zebrafish. Aging Cell 19, e13052. doi:10.1111/acel.13052
Demirci, Y., Heger, G., Katkat, E., Papatheodorou, I., Brazma, A., and Ozhan, G. (2022). Brain regeneration resembles brain cancer at its early wound healing stage and diverges from cancer later at its proliferation and differentiation stages. Front. Cell Dev. Biol. 10, 813314. doi:10.3389/fcell.2022.813314
Dorsemans, A.-C., Soulé, S., Weger, M., Bourdon, E., Lefebvre d'Hellencourt, C., Meilhac, O., et al. (2017). Impaired constitutive and regenerative neurogenesis in adult hyperglycemic zebrafish. J. Comp. Neurol. 525, 442–458. doi:10.1002/cne.24065
Dunlap, K. D., Teles, M. C., and Oliveira, R. F. (2021). Social stimuli increase activity of adult-born cells in the telencephalon of zebrafish (Danio rerio). J. Exp. Biol. 224, jeb242253. doi:10.1242/jeb.242253
Edelmann, K., Glashauser, L., Sprungala, S., Hesl, B., Fritschle, M., Ninkovic, J., et al. (2013). Increased radial glia quiescence, decreased reactivation upon injury and unaltered neuroblast behavior underlie decreased neurogenesis in the aging zebrafish telencephalon. J. Comp. Neurol. 521, 3099–3115. doi:10.1002/cne.23347
Ganz, J., and Brand, M. (2016). Adult Neurogenesis in Fish. Cold Spring Harb. Perspect. Biol. 8, a019018. doi:10.1101/cshperspect.a019018
Ganz, J., Kroehne, V., Freudenreich, D., Machate, A., Geffarth, M., Braasch, I., et al. (2015). Subdivisions of the adult zebrafish pallium based on molecular marker analysis. F1000Res 3, 308. doi:10.12688/f1000research.5595.2
Ghaddar, B., Bringart, M., Lefebvre d’Hellencourt, C., Meilhac, O., and Diotel, N. (2021). Deleterious effects of overfeeding on brain homeostasis and plasticity in adult zebrafish. Zebrafish 18, 190–206. doi:10.1089/zeb.2020.1962
Godoy, R., Noble, S., Yoon, K., Anisman, H., and Ekker, M. (2015). Chemogenetic ablation of dopaminergic neurons leads to transient locomotor impairments in zebrafish larvae. J. Neurochem. 135, 249–260. doi:10.1111/jnc.13214
Grandel, H., Kaslin, J., Ganz, J., Wenzel, I., and Brand, M. (2006). Neural stem cells and neurogenesis in the adult zebrafish brain: origin, proliferation dynamics, migration and cell fate. Dev. Biol. 295, 263–277. doi:10.1016/j.ydbio.2006.03.040
Hall, Z. J., and Tropepe, V. (2018a). Visual experience facilitates BDNF-dependent adaptive recruitment of new neurons in the postembryonic optic tectum. J. Neurosci. 38, 2000–2014. doi:10.1523/jneurosci.1962-17.2018
Hall, Z. J., and Tropepe, V. (2018b). Movement maintains forebrain neurogenesis via peripheral neural feedback in larval zebrafish. eLife 7, e31045. doi:10.7554/eLife.31045
Ito, Y., Tanaka, H., Okamoto, H., and Ohshima, T. (2010). Characterization of neural stem cells and their progeny in the adult zebrafish optic tectum. Dev. Biol. 342, 26–38. doi:10.1016/j.ydbio.2010.03.008
Kalueff, A. V., Gebhardt, M., Stewart, A. M., Cachat, J. M., Brimmer, M., Chawla, J. S., et al. (2013). Towards a comprehensive catalog of zebrafish behavior 1.0 and beyond. Zebrafish 10, 70–86. doi:10.1089/zeb.2012.0861
Kaslin, J., Kroehne, V., Ganz, J., Hans, S., and Brand, M. (2017). Distinct roles of neuroepithelial-like and radial glia-like progenitor cells in cerebellar regeneration. Development 144, 1462–1471. doi:10.1242/dev.144907
Kim, S.-M., Long, D. W., Tsang, M. W. K., and Wang, Y. (2018). Zebrafish extracellular matrix improves neuronal viability and network formation in a 3-dimensional culture. Biomaterials 170, 137–146. doi:10.1016/j.biomaterials.2018.04.009
Kishimoto, N., Shimizu, K., and Sawamoto, K. (2012). Neuronal regeneration in a zebrafish model of adult brain injury. Dis. Model Mech. 5, 200–209. doi:10.1242/dmm.007336
Kizil, C., Dudczig, S., Kyritsis, N., Machate, A., Blaesche, J., Kroehne, V., et al. (2012a). The chemokine receptor cxcr5 regulates the regenerative neurogenesis response in the adult zebrafish brain. Neural Dev. 7, 27. doi:10.1186/1749-8104-7-27
Kizil, C., Kyritsis, N., Dudczig, S., Kroehne, V., Freudenreich, D., Kaslin, J., et al. (2012b). Regenerative neurogenesis from neural progenitor cells requires injury-induced expression of Gata3. Dev. Cell 23, 1230–1237. doi:10.1016/j.devcel.2012.10.014
Kotrschal, K., and Palzenberger, M. (1992). Neuroecology of cyprinids: comparative, quantitative histology reveals diverse brain patterns. Environ. Biol. Fish. 33, 135–152. doi:10.1007/bf00002560
Kriegstein, A., and Alvarez-Buylla, A. (2009). The glial nature of embryonic and adult neural stem cells. Annu. Rev. Neurosci. 32, 149–184. doi:10.1146/annurev.neuro.051508.135600
Kroehne, V., Freudenreich, D., Hans, S., Kaslin, J., and Brand, M. (2011). Regeneration of the adult zebrafish brain from neurogenic radial glia-type progenitors. Development 138, 4831–4841. doi:10.1242/dev.072587
Kyritsis, N., Kizil, C., Zocher, S., Kroehne, V., Kaslin, J., Freudenreich, D., et al. (2012). Acute inflammation initiates the regenerative response in the adult zebrafish brain. Science 338, 1353–1356. doi:10.1126/science.1228773
Lindsey, B. W., and Tropepe, V. (2006). A comparative framework for understanding the biological principles of adult neurogenesis. Prog. Neurobiol. 80, 281–307. doi:10.1016/j.pneurobio.2006.11.007
Lindsey, B. W., and Tropepe, V. (2014). Changes in the social environment induce neurogenic plasticity predominantly in niches residing in sensory structures of the zebrafish brain independently of cortisol levels: social context regulates adult neurogenesis. Dev. Neurobio. 74, 1053–1077. doi:10.1002/dneu.22183
Lindsey, B. W., Di Donato, S., Kaslin, J., and Tropepe, V. (2014). Sensory-specific modulation of adult neurogenesis in sensory structures is associated with the type of stem cell present in the neurogenic niche of the zebrafish brain. Eur. J. Neurosci. 40, 3591–3607. doi:10.1111/ejn.12729
Lindsey, B. W., Hall, Z. J., Heuzé, A., Joly, J.-S., Tropepe, V., and Kaslin, J. (2018a). The role of neuro-epithelial-like and radial-glial stem and progenitor cells in development, plasticity, and repair. Prog. Neurobiol. 170, 99–114. doi:10.1016/j.pneurobio.2018.06.004
Lindsey, B. W., Douek, A. M., Loosli, F., and Kaslin, J. (2018b). A whole brain staining, embedding, and clearing pipeline for adult zebrafish to visualize cell proliferation and morphology in 3-dimensions. Front. Neurosci. 11, 750. doi:10.3389/fnins.2017.00750
Lindsey, B. W., Aitken, G. E., Tang, J. K., Khabooshan, M., Douek, A. M., Vandestadt, C., et al. (2019). Midbrain tectal stem cells display diverse regenerative capacities in zebrafish. Sci. Rep. 9, 4420–20. doi:10.1038/s41598-019-40734-z
Lübke, L., Zhang, G., Strähle, U., and Rastegar, S. (2022). mdka Expression is associated with quiescent neural stem cells during constitutive and reactive neurogenesis in the adult zebrafish telencephalon. Brain Sci. 12, 284. doi:10.3390/brainsci12020284
Makantasi, P., and Dermon, C. R. (2014). Estradiol treatment decreases cell proliferation in the neurogenic zones of adult female zebrafish (Danio rerio) brain. Neuroscience 277, 306–320. doi:10.1016/j.neuroscience.2014.06.071
Maruska, K. P., Carpenter, R. E., and Fernald, R. D. (2012). Characterization of cell proliferation throughout the brain of the African cichlid fish Astatotilapia burtoni and its regulation by social status. J. Comp. Neurol. 520, 3471–3491. doi:10.1002/cne.23100
Mosimann, C., Kaufman, C. K., Li, P., Pugach, E. K., Tamplin, O. J., and Zon, L. I. (2011). Ubiquitous transgene expression and Cre-based recombination driven by the ubiquitin promoter in zebrafish. Development 138, 169–177. doi:10.1242/dev.059345
Nelson, J. C., and Granato, M. (2022). Zebrafish behavior as a gateway to nervous system assembly and plasticity. Development 149, dev177998. doi:10.1242/dev.177998
Nelson, C. M., Lennon, V. A., Lee, H., Krug, R. G., Kamalova, A., Madigan, N. N., et al. (2019). Glucocorticoids target ependymal glia and inhibit repair of the injured spinal cord. Front. Cell Dev. Biol. 7, 56. doi:10.3389/fcell.2019.00056
Onarheim, T., Janczak, A. M., and Nordgreen, J. (2022). The effects of social vs. individual housing of zebrafish on whole-body cortisol and behavior in two tests of anxiety. Front. Vet. Sci. 9, 859848. doi:10.3389/fvets.2022.859848
Oorschot, V., Lindsey, B. W., Kaslin, J., and Ramm, G. (2021). TEM, SEM, and STEM-based immuno-CLEM workflows offer complementary advantages. Sci. Rep. 11, 899. doi:10.1038/s41598-020-79637-9
Pan, Y. A., Freundlich, T., Weissman, T. A., Schoppik, D., Wang, X. C., Zimmerman, S., et al. (2013). Zebrabow: multispectral cell labeling for cell tracing and lineage analysis in zebrafish. Development 140, 2835–2846. doi:10.1242/dev.094631
Pérez, M. R., Pellegrini, E., Cano-Nicolau, J., Gueguen, M. M., Menouer-Le Guillou, D., Merot, Y., et al. (2013). Relationships between radial glial progenitors and 5-HT neurons in the paraventricular organ of adult zebrafish - potential effects of serotonin on adult neurogenesis. Eur. J. Neurosci. 38, 3292–3301. doi:10.1111/ejn.12348
Pellegrini, E., Diotel, N., Vaillant-Capitaine, C., Pérez Maria, R., Gueguen, M.-M., Nasri, A., et al. (2016). Steroid modulation of neurogenesis: Focus on radial glial cells in zebrafish. J. Steroid Biochem. Mol. Biol. 160, 27–36. doi:10.1016/j.jsbmb.2015.06.011
Reimer, M. M., Kuscha, V., Wyatt, C., Sorensen, I., Frank, R. E., Knuwer, M., et al. (2009). Sonic hedgehog is a polarized signal for motor neuron regeneration in adult zebrafish. J. Neurosci. 29, 15073–15082. doi:10.1523/jneurosci.4748-09.2009
Rodriguez Viales, R., Diotel, N., Ferg, M., Armant, O., Eich, J., Alunni, A., et al. (2015). The helix-loop-helix protein id1 controls stem cell proliferation during regenerative neurogenesis in the adult zebrafish telencephalon. Stem Cells 33, 892–903. doi:10.1002/stem.1883
Sanchez-Gonzalez, R., Koupourtidou, C., Lepko, T., Zambusi, A., Novoselc, K. T., Durovic, T., et al. (2022). Innate immune pathways prompote oligodendrocyte progenitor cell recruitment to the injury site in adult zebrafish brain. Cells 11, 520. doi:10.3390/cells11030520
Santoro, M. M. (2014). Zebrafish as a model to explore cell metabolism. Trends Endocrinol. Metab. 25, 546–554. doi:10.1016/j.tem.2014.06.003
Schönenberger, F., Deutzmann, A., Ferrando-May, E., and Merhof, D. (2015). Discrimination of cell cycle phases in PCNA-immunolabeled cells. BMC Bioinform. 16, 180. doi:10.1186/s12859-015-0618-9
Shimizu, Y., Ueda, Y., and Ohshima, T. (2018). Wnt signaling regulates proliferation and differentiation of radial glia in regenerative processes after stab injury in the optic tectum of adult zebrafish. Glia 66, 1382–1394. doi:10.1002/glia.23311
Shimizu, Y., Kiyooka, M., and Ohshima, T. (2021). Transcriptome Analyses Reveal IL6/Stat3 Signaling Involvement in Radial Glia Proliferation After Stab Wound Injury in the Adult Zebrafish Optic Tectum. Front. Cell Dev. Biol. 9, 668408. doi:10.3389/fcell.2021.668408
Stankiewicz, A. J., McGowan, E. M., Yu, L., and Zhdanova, I. V. (2017). Impaired sleep, circadian rhythms and neurogenesis in diet-induced premature aging. Int. J. Mol. Sci. 18, E2243. doi:10.3390/ijms18112243
Suriyampola, P. S., Shelton, D. S., Shukla, R., Roy, T., Bhat, A., and Martins, E. P. (2016). Zebrafish Social Behavior in the Wild. Zebrafish 13, 1–8. doi:10.1089/zeb.2015.1159
Tea, J., Alderman, S. L., and Gilmour, K. M. (2019). Social stress increases plasma cortisol and reduces forebrain cell proliferation in subordinate male zebrafish (Danio rerio). J. Exp. Biol. 222, jeb194894. doi:10.1242/jeb.194894
Teles, M. C., Cardoso, S. D., and Oliveira, R. F. (2016). Social plasticity relies on different neuroplasticity mechanisms across the brain social decision-making network in zebrafish. Front. Behav. Neurosci. 10, 16. doi:10.3389/fnbeh.2016.00016
Thompson, W. A., Arnold, V. I., and Vijayan, M. M. (2017). Venlafaxine in embryos stimulates neurogenesis and disrupts larval behavior in zebrafish. Environ. Sci. Technol. 51, 12889–12897. doi:10.1021/acs.est.7b04099
Tsarouchas, T. M., Wehner, D., Cavone, L., Munir, T., Keatinge, M., Lambertus, M., et al. (2018). Dynamic control of proinflammatory cytokines Il-1β and Tnf-α by macrophages in zebrafish spinal cord regeneration. Nat. Commun. 9, 4670. doi:10.1038/s41467-018-07036-w
Tsata, V., Kroehne, V., Wehner, D., Rost, F., Lange, C., Hoppe, C., et al. (2020). Reactive oligodendrocyte progenitor cells (re-)myelinate the regenerating zebrafish spinal cord. Development 147, dev193946. doi:10.1242/dev.193946
von Krogh, K., Sørensen, C., Nilsson, G. E., and Øverli, Ø. (2010). Forebrain cell proliferation, behavior, and physiology of zebrafish, Danio rerio, kept in enriched or barren environments. Physiol. Behav. 101, 32–39. doi:10.1016/j.physbeh.2010.04.003
Zhang, G., Lübke, L., Chen, F., Beil, T., Takamiya, M., Diotel, N., et al. (2021). Neuron-radial glial cell communication via BMP/Id1 signaling is key to long-term maintenance of the regenerative capacity of the adult zebrafish telencephalon. Cells 10, 2794. doi:10.3390/cells10102794
Zupanc, G. K. H., and Sîrbulescu, R. F. (2012). “Teleost Fish as a Model System to Study Successful Regeneration of the Central Nervous System,” in New Perspectives in Regeneration Current Topics in Microbiology and Immunology. Editors E. Heber-Katz, and D. L. Stocum (Berlin, Heidelberg: Springer Berlin Heidelberg), 193–233. doi:10.1007/82_2012_297
Keywords: adult neural stem and progenitor cells, zebrafish, cell proliferation, cell cycle regulation, environmental enrichment, social behaviour, central nervous system repair, neurogenesis
Citation: Caron A, Trzuskot L and Lindsey BW (2022) Uncovering the spectrum of adult zebrafish neural stem cell cycle regulators. Front. Cell Dev. Biol. 10:941893. doi: 10.3389/fcell.2022.941893
Received: 11 May 2022; Accepted: 16 June 2022;
Published: 29 June 2022.
Edited by:
Philipp Kaldis, Lund University, SwedenReviewed by:
Dimitris Beis, Biomedical Research Foundation of the Academy of Athens (BRFAA), GreeceCopyright © 2022 Caron, Trzuskot and Lindsey. This is an open-access article distributed under the terms of the Creative Commons Attribution License (CC BY). The use, distribution or reproduction in other forums is permitted, provided the original author(s) and the copyright owner(s) are credited and that the original publication in this journal is cited, in accordance with accepted academic practice. No use, distribution or reproduction is permitted which does not comply with these terms.
*Correspondence: Benjamin W. Lindsey, QmVuamFtaW4ubGluZHNleUB1bWFuaXRvYmEuY2E=
Disclaimer: All claims expressed in this article are solely those of the authors and do not necessarily represent those of their affiliated organizations, or those of the publisher, the editors and the reviewers. Any product that may be evaluated in this article or claim that may be made by its manufacturer is not guaranteed or endorsed by the publisher.
Research integrity at Frontiers
Learn more about the work of our research integrity team to safeguard the quality of each article we publish.