- Department of Pharmacology, UT Southwestern Medical Center, Dallas, TX, United States
Metastasis is the major cause of mortality in cancer patients. Analyses of mouse models and patient data have implicated the protein kinase WNK1 as one of a handful of genes uniquely linked to a subset of invasive cancers. WNK1 signaling pathways are widely implicated in the regulation of ion co-transporters and in controlling cell responses to osmotic stress. In this review we will discuss its actions in tumor malignancy in human cancers and present evidence for its function in invasion, migration, angiogenesis and mesenchymal transition.
Introduction
WNK (With-No-Lysine) kinases were originally identified in a screen for novel members of the MAP kinase kinase (MAP2K) family (Xu et al., 2000; Verissimo and Jordan, 2001). The four WNK family members are characterized by an atypical placement of the catalytic lysine. These serine-threonine kinases participate in several functionally distinct signaling cascades controlling cellular responses to osmotic stress (Xu et al., 2005b; Kahle et al., 2005; Lenertz et al., 2005; Moriguchi et al., 2005; Anselmo et al., 2006; Gagnon et al., 2006; Vitari et al., 2006; Zagorska et al., 2007; Richardson et al., 2008; Roy et al., 2015). Many putative WNK substrates have been identified; the best-known are the related kinases OSR1 (oxidative stress responsive kinase1) and SPAK (STE20/SPS1-related proline/alanine-rich kinase) (Chen et al., 2004; Moriguchi et al., 2005; Vitari et al., 2005; Anselmo et al., 2006; Cope et al., 2006; Vitari et al., 2006; Richardson and Alessi, 2008). OSR1 and SPAK regulate ion co-transporters and will be discussed further below (Moriguchi et al., 2005; Vitari et al., 2005; Anselmo et al., 2006; Roy et al., 2015). In recent years, there is growing evidence that WNK1 is a critical kinase involved in various types of cancer (Chen et al., 2017; Gallolu Kankanamalage et al., 2018; Shahi Thakuri et al., 2020), but the exact mechanisms by which WNK1 modulates tumor progression are not well understood. Here we summarize the structure and turnover of WNK1 protein and evidence for its actions in tumor malignancy in human cancers.
Overview of With-No-Lysine kinases Structure and Expression
In humans, the four WNK kinases, WNK1-4, range in size from 2833 amino acids (the longest isoform of WNK1) to 1243 amino acids (WNK4) (Verissimo and Jordan, 2001; Wilson et al., 2001; Delaloy et al., 2003; Vidal-Petiot et al., 2012). The four WNK family members share high homology in their kinase domains with 85–90% sequence identity, two autoinhibitory-like domains, a coiled-coil domain, and proline-rich (PXXP) motifs for protein-protein interactions (Xu et al., 2000; Verissimo and Jordan, 2001; Wilson et al., 2001; Xu et al., 2005a; Huang et al., 2007; Wang et al., 2008). Crystal structures of the kinase domains and autoinhibitory domains of multiple WNKs have been determined and some structures with small molecules bound are available (Min et al., 2004; Xu et al., 2005a; Moon et al., 2013). Outside of these regions, WNKs show low sequence identity, which is assumed to confer functional diversity for each of the WNK family members. Further, the abundance of low complexity sequence outside of the kinase domain makes structure determination challenging. Alphafold2 did not provide much greater clarity on the balance of the WNK1 molecule, for example, (Jumper et al., 2021; Varadi et al., 2021).
WNK1 and WNK4 have been extensively studied because they are responsible for pseudohypoaldosteronism type II (PHA II), a genetic disease characterized by hyperkalemic hypertension (Wilson et al., 2001; Susa et al., 2014). In contrast to other members of the WNK family which display a more tissue-restricted pattern of expression, WNK1 is widely and highly expressed in most animal tissues and cell types (Xu et al., 2000; Wilson et al., 2001; O'reilly et al., 2003; Vitari et al., 2005). The sequence of WNK1 is conserved across species (human WNK1 > 85% identical with mouse, pig 86%, rat 76%, and bovine 68%) and is recognizable in plants and many unicellular eukaryotes. The kinase activity of WNK1 is regulated via autophosphorylation on activation loop residues Ser382 and Ser378 (Xu et al., 2002). Details of mechanisms controlling autophosphorylation remain scant; however, importantly, chloride ion binding in the active site prevents autophosphorylation (Piala et al., 2014). This finding is relevant to WNK function as central regulators of ion homeostasis.
With-No-Lysine kinases1 Turnover and Ion Homeostasis
Due to its importance for cellular homeostasis (Shekarabi et al., 2017), the abundance of WNK1 protein is tightly controlled by several layers of negative regulation (Mccormick and Ellison, 2011; Li et al., 2014; Roy et al., 2015; Dbouk et al., 2016). The set point in the total amount of WNK1 protein in a cell has a substantial impact on control of its downstream targets. All WNK family members share the highly conserved acidic degron motif near their autoinhibitory domain as a signal for destruction, which plays a critical role in the ubiquitin-dependent proteolytic process (Schumacher et al., 2014; Chen et al., 2022). The initial finding showed that the adaptor protein kelch-like family member 3 (KLHL3)-cullin3 (CUL3) E3 ligase complex is associated with WNK1 and WNK4 (Shibata et al., 2013). KLHL3 as a substrate adaptor binds to the conserved degron motif just C-terminal to the kinase domain in these WNKs to facilitate the recruitment of the CUL3 E3 ubiquitin ligase, thereby promoting ubiquitination and degradation (Shibata et al., 2013; Schumacher et al., 2014). Perturbations in this interaction have great effects on WNK-mediated electrolyte homeostasis, which was demonstrated by the finding that mutations in KLHL3-CUL3 also cause PHA II (Ohta et al., 2013; Mccormick et al., 2014; Lin et al., 2019).
Other ubiquitination pathways have also been connected to WNK1 turnover. The HECT-type ubiquitin ligase NEDD4-2 (also called NEDD4L) can bind to PY motifs in WNK1 for facilitating its ubiquitin-dependent proteasomal degradation (Heise et al., 2010; Roy et al., 2015). This is supported by the finding that WNK1 abundance was increased and OSR1/SPAK activation were observed in mice lacking NEDD4-2 (Roy et al., 2015; Al-Qusairi et al., 2017). We recently showed that WNK1 is degraded not only by the ubiquitin-proteasome pathway, but also by the lysosomal pathway (Jung et al., 2022). Non-lysosomal cysteine proteases calpain and caspase-3 were also able to influence WNK1 abundance. This study identified UBR5 (ubiquitin protein ligase E3 component N-recognin 5) as a previously unknown regulator of WNK1 turnover that mediates lysosomal degradation of WNK1 protein (Jung et al., 2022). Though UBR5 inhibition only modestly elevated WNK1 protein, that change caused a significant increase in phosphorylation of OSR1/SPAK, indicating that even small changes in WNK1 protein will have a significant impact on cellular processes. It is therefore likely that multiple degradative pathways in cells participate in the modulation of cellular WNK1 protein amount. WNK1 itself can also act as an adaptor for endosomal trafficking. WNK1 is thought to be crucial for glucose transporter GLUT1 and GLUT4 endosomal trafficking through regulating the Rab GTPase-activating protein AS160 (Akt substrate of 160 kDa) (Mendes et al., 2010; Tan et al., 2012; Kim et al., 2018; Henriques et al., 2020). WNK1 interacts with the endocytic scaffold protein intersectin which is involved in clathrin-mediated endocytosis that impacts recycling of ROMK (renal outer medullary potassium channel) (He et al., 2007; Wang et al., 2008). Moreover, we previously found that WNK1 negatively regulates autophagic degradation pathways through inhibition of class III phosphatidylinositol 3-kinase (PI3KC3) (Gallolu Kankanamalage et al., 2016). These results imply that WNK1 protein is degraded by multiple proteolytic pathways, whereas it is also a critical modulator of endocytic degradation. The mechanisms underlying the feedback between WNK1 turnover and its function as a trafficking regulator remain to be determined.
With-No-Lysine kinases1, Angiogenesis, and Cell Junction Regulation
Phenotypes identified in mouse knockout studies demonstrated that WNK1 is required for angiogenesis. Angiogenesis is a highly regulated process that is turned on transiently during development, reproduction, and wound repair, which involves formation of new capillaries through sprouting or by splitting off from the original vessel (intussusception) (Folkman and Shing, 1992; Otrock et al., 2007). Homozygous disruption of the WNK1 gene results in a lethal developmental failure in mice around embryonic day E12, due to impaired angiogenesis (Xie et al., 2009; Xie et al., 2009). The phenotype of the WNK1 global knockout mouse mimics the defects caused by endothelial-specific ablation of WNK1 and is rescued either by endothelial-specific expression of WNK1 (Xie et al., 2009) or an activated form of OSR1 (Xie et al., 2013). Additionally, depletion of WNK1 and OSR1 decreased in vitro vascular cord formation and cell migration in endothelial cells (Dbouk et al., 2014; Jaykumar et al., 2022), indicating a crucial role of WNK1-activated OSR1 signaling in angiogenesis, and vascular remodeling.
Although a detailed mechanistic understanding of the effects of WNK1 on angiogenesis is lacking, decreased expression of WNK1 in cultured endothelial cells caused reduced expression of a number of factors that promote angiogenesis including Slug (SNAI2), vascular endothelial growth factor A (VEGF-A), and matrix metallo-proteinases (MMPs) (Dbouk et al., 2014). During vein graft remodeling, Slug mediates endothelial-mesenchymal transition (also called Endo-MT) via SMAD2/3-mediated transforming growth factor-β (TGF-β) signaling (Cooley et al., 2014; Welch-Reardon et al., 2014; Mahmoud et al., 2017), a widely known inducer of this transition (Nakajima et al., 2000; Pardali et al., 2017). We previously showed that WNK1 interacts with, and phosphorylates SMAD2 and regulates its function (Lee et al., 2007; Li et al., 2020). WNK1 signaling takes part in the regulation of TGF-β/SMAD-dependent Endo-MT for promoting onset of cell sprouting, migration and vascular remodeling. These and other findings indicate an effect of the WNK1 cascade on induction of a mesenchymal phenotype essential for endothelial wound healing (Yoshimatsu and Watabe, 2011; Welch-Reardon et al., 2014).
Partial Endo-MT is characterized by transient loss of an endothelial phenotype and acquisition of mesenchymal characteristics such as loss of cell-cell junctions, polarity and gain of motility to promote angiogenic sprouting and cell migration (Otrock et al., 2007; Welch-Reardon et al., 2015). TGF-β initiates cytoskeletal turnover and a drastic down-regulation and disintegration of tight junctions to promote migration in endothelial cells. Upon TGF-β stimulation, the TGF-β receptor type II redistributes into tight junctions which leads to their dissolution (Barrios-Rodiles, 2005; Ozdamar et al., 2005). Because OSR1 is a mediator of WNK1 action and because OSR1 was found to be a component of the TGF-β interactome (Barrios-Rodiles, 2005), we explored the possibility that WNK1 regulates OSR1 to influence endothelial tight junction turnover. We found that OSR1 is involved in WNK1-mediated regulation of turnover of tight junctions and adherens junctions, in part, through its interaction with occludin via a TGF-β-sensitive process. While occludin is not necessary for the formation of tight junctions, occludin is vital to tight junction integrity (Rao, 2009; Cummins, 2011). Interestingly, occludin is also important for directional migration of epithelial cells (Du et al., 2010). Control of both tight junction integrity and directional migration of endothelial cells are central to angiogenesis (Liu et al., 2016). One mechanism underlying the importance of WNK1/OSR1 to angiogenesis is the capacity to target occludin turnover. This same capacity may also contribute to effects of the WNK1 pathway on endothelial-mesenchymal transition. Furthermore, recent studies have shown that occludin is also involved in endothelial neovascularization and angiogenesis (Liu et al., 2016). In addition to effects on occludin, we identified other events that underlie WNK1-mediated control of angiogenesis including stabilization of TGF-β-regulated components AXL (a TAM receptor tyrosine kinase), ALK1 (a TGF-β receptor), SMAD2/3, RhoA, and VE-cadherin (Jaykumar et al., 2022).
Adherens junctions are required for endothelial cell stabilization and homeostasis because they promote contact inhibition of growth and decrease cell responsiveness to apoptotic stimuli. Adherens junctions in endothelial cells primarily consist of VE-cadherin (Dejana, 2004; Rudini et al., 2008). VE-cadherin is also known to be an endothelial cell-specific regulator of TGF-β/SMAD signaling (Rudini et al., 2008). TGF-β induces TGF-βRII association with VE-cadherin and this clustering promotes TGF-β signaling, which, in turn, destabilizes cell-cell junctions (Ma et al., 2020). Moreover, VE-cadherin is essential for TGF-β-induced endothelial cell migration (Rudini et al., 2008). VE-cadherin is a positive regulator of TGF-β-induced SMAD2/3 phosphorylation. TGF-β stimulation induces association of VE-cadherin with TGF-βRII/TGF-βRI and therefore it participates in maximal activation of the TGF-β pathway (Rudini et al., 2008). OSR1 was also shown to phosphorylate the SMAD2/3 linker region to promote TGF-β signaling (Li et al., 2020). We found that WNK1 inhibition leads to decreased localization of VE-cadherin at cell-cell junctions only in the presence of TGF-β, indicating that WNK1 actions are context dependent (Jaykumar et al., 2022).
With-No-Lysine kinases1 and Cancer Prognosis
For the last two decades, the majority of studies on WNK1 have focused on their roles in hypertension and kidney function. Although multiple studies revealed that WNK1 is involved in major cancer-related signaling pathways such as PI3K-AKT, TGF-β and NF-κB (Xu et al., 2005c; Jiang et al., 2005; Lee et al., 2007; Yan et al., 2008), little is known regarding how WNK1 contributes to cancer progression. Recently, there has been growing interest in involvement of WNK1 in cancers. In fact, large-scale cancer database analysis from the cBioPortal for Cancer Genomics revealed that a high level of WNK1 expression has been observed in various tumor types including prostate, ovarian, testis and breast cancers (The cBioPortal for Cancer Genomics; http://cbioportal.org). As noted above, upregulation of WNK1 protein can result in increased activation of its downstream pathways and potential cancer-promoting actions. Utilizing transposon-mediated insertional mutagenesis for identifying candidate BC driver genes, WNK1 was identified as one of a handful of driver genes in high-risk invasive breast cancer (Chen et al., 2017).
Paralleling findings in endothelial cells, WNK1 has been implicated in migration with epithelial-mesenchymal (EMT) features through knockdown studies in multiple cancer types, and also as a contributor to stem-like properties in metastatic breast cancers (Shyamasundar et al., 2016; Hung et al., 2017; Pio et al., 2017). Mechanistic information has suggested links to expression of EMT factors Slug and Snail, microRNA networks, changes in expression of cell surface proteins, altered vesicle trafficking, and effects on actin polymerization (Shyamasundar et al., 2016; Hung et al., 2017; Tanaka and Siemann, 2019). One study showed that WNK protects the interaction between β-Catenin and the glucose-induced degradation deficient (GID) complex, which includes an E3 ubiquitin ligase targeting β-Catenin, and that WNK regulates β-Catenin levels. Furthermore, WNK inhibitors induced β-Catenin degradation and suppressed xenograft tumor development in mice (Sato et al., 2020). Elevated WNK1 mRNA and protein have been detected in hepatocellular carcinoma (HCC) and significantly correlated with poor prognosis, highlighting the clinical significance of WNK1 in HCC progression (Ho et al., 2020). Knockdown and inhibition of WNK1 also decreased tumor-induced ectopic vessel formation and inhibited tumor proliferation in two zebrafish models transplanted with intestinal and hepatocellular carcinomas (Sie et al., 2020). Endothelial-specific overexpression of WNK1 enhanced tumorigenesis in transgenic carcinogenic zebrafish, supporting endothelial cell-autonomous effect of WNK1 in tumor production (Sie et al., 2020). In addition, WNK1 fusion to B4GALNT3 is a potential oncogenic driver in a patient with papillary thyroid carcinoma, which is the most common type of thyroid cancer (Costa et al., 2015). Another newly identified gene fusion WNK1-ROS1 (c-Ros oncogene-1) has been described as a novel driver of lung adenocarcinoma (Liu et al., 2019). WNK1 has been shown to promote tumorigenesis in cancers through its ability to promote cell proliferation via anti-apoptotic and pro-survival functions (Tu et al., 2011; Fulford et al., 2016; Wang et al., 2017). Importantly, the WNK1 signaling axis contributes to pathologic features of malignancies such as enhanced invasion, migration, adhesion and tumorigenicity, as shown in Figure 1. Thus, interfering with WNK1 expression may have therapeutic value in human cancers. In the following sections, we discuss different mechanisms by which WNK1 has been reported to support tumor malignancy.
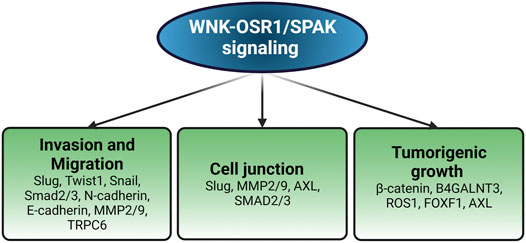
FIGURE 1. Pathological impacts of WNK1 in cancer. WNK1 regulates the expression of various molecules involved in different aspects of oncogenesis. Created with BioRender.com.
With-No-Lysine kinases1 and Epithelial–Mesenchymal Transition
The major characteristic of malignant tumors is a diffuse invasion into adjacent normal tissue and migration to a significant distance from the primary tumor area, a fundamental step in metastasis (Van Zijl et al., 2011; Clark and Vignjevic, 2015; Lambert et al., 2017). This characteristic is one of the most difficult challenges to target therapeutically in human cancers. Therefore, a clear understanding of the cellular and molecular mechanisms underlying the malignant behavior is crucial for the development of specific therapeutic strategies.
Multiple studies have implicated WNKs in cell migration in wound healing assays (Adams et al., 2017; Desjardins et al., 2019; Kim et al., 2019; Liu et al., 2020). A direct relationship between WNK1 and cell invasion in three-dimensional assays was described in human umbilical vein endothelial cells (HUVECs) (Dbouk et al., 2014). In that study, sprouting and invasion into the surrounding matrix were significantly diminished by WNK1 knockdown in spheroid sprouting assays. The impaired invasive phenotype of WNK1-depleted cancer cells was linked to downregulation of the EMT-associated transcription factor Slug which was partially rescued by overexpressing the WNK1-regulated kinases OSR1 or SPAK (Jaykumar et al., 2021). Consistent with this, it has recently been proposed that OSR1 depletion in MDA-MB-231 cells displays a decrease in expression of EMT transcription factors Twist1, Snail, and Slug at mRNA and protein levels, resulting in reduced invasion of breast cancer cells in vitro and in vivo (Li et al., 2021). As expected, OSR1 overexpression elevated levels of EMT transcription factors (Li et al., 2021). The pathological importance of complete or partial EMT has become clear from numerous studies and the molecular mechanisms governing malignant phenotypes of cancer cells such as invasion and metastasis formation are becoming clearer (Dang et al., 2015; Brabletz et al., 2018; Ramesh et al., 2020; Ribatti et al., 2020). Inhibition of WNK signaling with WNK463, an allosteric inhibitor of WNK kinase activity (Yamada et al., 2016; Zhang et al., 2016), significantly decreased expression of the EMT-related factor N-cadherin in breast cancer cells (Jaykumar et al., 2021). This mesenchymal marker is often highly expressed in cancers and has been shown to be associated with poor prognosis (Mariotti et al., 2007; Mrozik et al., 2018). Depletion of WNK1 in MDA-MB-231 cells significantly attenuated invasive potential in three-dimensional collagen matrices, and a similar result was also observed in cells treated with WNK463 (Jaykumar et al., 2021). In another study in MDA-MB-231 cells, miRNA-93 was reported to suppress WNK1 expression, resulting in a decrease in the invasiveness of the cells through upregulation of epithelial markers CLDN1, CLDN3 and CDH1 (Shyamasundar et al., 2016). Additionally, it was shown that overexpression of WNK1 in hepatocellular carcinoma enhanced the expression of MMP-2 and MMP-9, which are EMT-related proteolytic enzymes that can promote degradation of the extracellular matrix, EMT, and invasion (Dong et al., 2020). Thus, diverse studies place WNK1 as a regulator of EMT during the invasion of different types of cancer cells.
Evidence generated from several studies suggests that WNK1 is a key in cancer cell migration via regulation of EMT. Since the initial finding through in vitro scratch wound healing assays that knockdown of WNK1 reduced migration of mouse neural progenitor cell line C17.2 accompanied by morphological changes (Sun et al., 2006), numerous studies have identified WNK1 regulation of the migratory phenotype in cancer cells. In non-small cell lung cancer cell lines, WNK1 silencing leads to decreased EMT molecules N-cadherin and Snail, and increased E-cadherin, resulting in reduced migration of CL1-5 and H1299 cells (Hung et al., 2017). Also, work from our lab has shown that WNK1 inhibition in five different breast cancer cell lines MDA-MB-231, BT-549, BT-20, HCC1569, and HCC1419 significantly reduced the migratory capacity concomitantly with the reduction in N-cadherin protein expression (Jaykumar et al., 2021).
With-No-Lysine kinases, Ion Cotransporters and Migration
As discussed above, the WNK-OSR1/SPAK signaling pathway is key in the regulation of electrolyte homeostasis through regulating ion co-transporters of the SCL12A family. These include the Na+K+2Cl− co-transporters 1 and 2 (NKCC1 and NKCC2), the Na+Cl− co-transporter (NCC), and multiple K+Cl− co-transporters (KCC) that modulate the flux of ions across the cell membrane (Gamba, 2005; Flatman, 2008; Richardson and Alessi, 2008). WNK1 has also been demonstrated to regulate cell surface expression of ion channels including the epithelial Na+ channel (ENaC) and renal outer medullary K+ channel (ROMK, Kir 1.1) (Xu et al., 2005b; Cope et al., 2006; Lazrak et al., 2006). We recently showed that Kir2.1 and Kir2.3 channels harbor an alternative SPAK/OSR1 binding motifs (RxFxV), leading to their plasma membrane localization (Taylor et al., 2018). Alterations of electrolyte homeostasis are frequently observed in cancer patients (Miltiadous et al., 2008; Rosner and Dalkin, 2014; Li et al., 2020; Bennet et al., 2021).
These ion transporters and channels have frequently been implicated in the regulation of migration and invasion (Cuddapah and Sontheimer, 2011; Schwab and Stock, 2014; Stock and Schwab, 2015; Martial, 2016). WNK1 knockdown in primary glioma cells results in attenuated phosphorylation of NKCC1, the ubiquitously expressed ion co-transporter, in response to the chemotherapeutic drug temozolomide, reducing glioma cell migration (Garzon-Muvdi et al., 2012). In a further study, Köchl et al. described the mechanistic connection between WNK1 and NKCC1 on migration of T cells in vitro and in vivo. In that study, WNK1 was shown to negatively regulate integrin-mediated adhesion through inhibition of the small GTPase RAP1, and positively regulate migration through promoting OSR1/SPAK-mediated NKCC1 activation, proposed to account for cell volume changes required for cell migration (Kochl et al., 2016). The importance of NKCC1 in cancer cell invasion and migration is supported by studies carried out in numerous systems. In glioblastoma cells, NKCC1 silencing impedes invasion and migration, accompanied by inhibition of MMP-2 and MMP-9 (Sun et al., 2020). The invasive ability of hepatocellular carcinoma was regulated by NKCC1 (Zhou et al., 2017). We found that depletion of OSR1 also reduced migration of breast cancer cells; however, inhibition of NKCC1 was not as effective as OSR1 depletion in slowing migration (Jaykumar et al., 2021). Finally, NKCC1 was reported to facilitate EMT of human gastric cancer cells through the MAPK-JNK signaling pathway, resulting in enhanced invasion and migration (Wang et al., 2021). WNK1 regulation of calcium homeostasis has been previously described (Lee et al., 2004; Xu et al., 2005a; Liu et al., 2015). A recent study has hinted that actions of WNK1 on calcium homeostasis may participate in cancer progression. WNK1 affects TRPC6 (canonical transient receptor potential channel)-mediated Ca2+ influx, leading to migration in clear-cell renal-cell carcinoma (Kim et al., 2019). These results highlight the functional importance of ion homeostasis regulated by WNK1 in cancer cell invasion and migration.
With-No-Lysine kinases1 and AXL
Phenotypic plasticity in several cancers is dependent on the expression of the receptor tyrosine kinase AXL. In normal mammary epithelial cells, AXL is a driver of stemness (Fujino et al., 2017; Engelsen et al., 2020). Interestingly, several characteristics of epithelial-mesenchymal transition overlap with those in endothelial cells (Welch-Reardon et al., 2015). Endothelial-expressed AXL is known to modulate angiogenesis (Gallicchio et al., 2005; Sacha et al., 2005; Li et al., 2009). The catalytic activity of AXL induces endothelial tube formation in vitro and knockdown of AXL in breast cancer cells and in endothelial cell co-culture impairs this process (Sacha et al., 2005; Li et al., 2009). AXL was identified as a downstream effector of TGF-β and modulates expression of TGF-β/SMAD-dependent target genes involved in cell migration in hepatocellular carcinoma (Bauer et al., 2012; Lee et al., 2014; Reichl et al., 2015). Moreover, inhibition of AXL decreases autocrine TGF-β signaling in hepatocellular carcinoma and impairs secretion of pro-angiogenic factors in breast cancer cells which in turn affects the function of endothelial cells in co-culture and in vivo (Reichl et al., 2015; Tanaka and Siemann, 2019). Paracrine angiogenic factors have also been shown to be expressed in endothelial cells, suggestive of an autocrine signaling loop (Lee et al., 2007). In view of these observations, we investigated the potential of an AXL and WNK1 signaling collaboration in endothelial cells to regulate endothelial cell migration and tube formation. We found that inhibiting WNK1 decreased expression of the tyrosine kinase AXL, apparently not due to a change in AXL mRNA (Jaykumar et al., 2021).
AXL expression is associated with metastasis and poor prognosis in a variety of tumor types including breast cancer (Oh et al., 2011; Dang et al., 2015; Garrido-Castro et al., 2019). BGB324 is a first-in-class AXL inhibitor, currently in phase II clinical trials, exhibiting promising therapeutic characteristics. It displays AXL-selective antitumor and antimetastatic activity in murine models of breast cancer (Garrido-Castro et al., 2019). AXL inhibition in tumor cells decreases the secretion of pro-angiogenic factors such as endothelin and VEGF-A and impairs functional properties of endothelial cells in vivo, suggesting its important role in the initiation of tumor angiogenesis (Tanaka and Siemann, 2019). In our recent study, we found elevated phospho-OSR1 in bone metastatic cells, suggesting that increased WNK activity may be a feature of breast cancers that can metastasize to multiple sites. We showed that inhibition of WNK1 reduces tumor volume and dispersion of metastatic cells in a mouse xenograft model of metastatic breast cancer, in part, via a network involving Slug and AXL (Jaykumar et al., 2021). Interestingly, we previously reported that mRNAs encoding these factors were also decreased upon knockdown of WNK1 in endothelial cells (Dbouk et al., 2014). Perhaps WNK1 knockdown diminishes these mRNAs in part via suppression of AXL (Dang et al., 2015). The connection revealed here between AXL and WNK1 raises the possibility that WNK1 may be a therapeutic option in other AXL-dependent tumor types as well. We also found that inhibition of WNK1 via WNK463, had a more pronounced effect than the AXL inhibitor to attenuate migration in MDA-MB-231 cells. Yet, the combination of AXL and WNK inhibitors was more effective at reducing migration than inhibition of either alone (Jaykumar et al., 2021). This observation warrants future studies examining the combination of AXL and WNK inhibitors on tumor progression and metastasis in animal models, which could potentially inform future clinical trials (Figure 2).
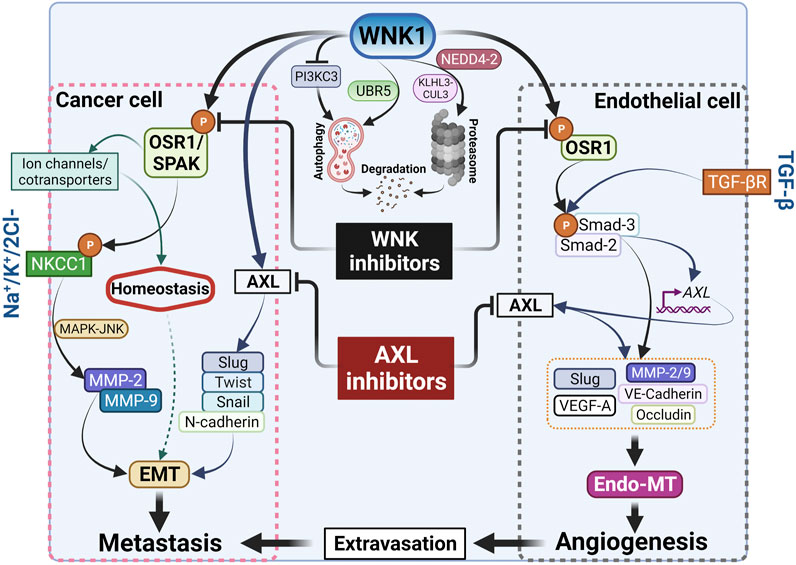
FIGURE 2. Cancer metastasis and angiogenesis by WNK1. WNK1 is a key in the metastasis cascade via regulation of EMT-effectors and -transcription factors. Created with BioRender.com.
Conclusion
In summary, we briefly highlight four points that may be considered in future analyses of the actions of WNK1 and other WNK family members in cancers. It should be clear that WNK1 has related actions in angiogenesis and cancer. How many of these related actions are shared by other WNK family members is not clear. The control WNK1 exerts over ion transport is a significant factor in its actions in cancers but may be overshadowed by its potential impact on cell phenotype. In both endothelial cells and cancer cells, WNK1 has the capacity to induce migration and enable transition towards a mesenchymal phenotype (Figure 2). This occurs in certain contexts, e.g., in endothelial cells during wound repair, but what other triggers exist and what contexts determine when these actions of WNK1 will overtake its homeostatic functions are not established or yet recognized. We close with the question why and when does WNK1 switch from being a homeostatic housekeeper to instead promoting EMT.
Author Contributions
All authors listed have made a substantial, direct, and intellectual contribution to the work and approved it for publication.
Funding
Work by the authors that is reviewed here was supported by CPRIT training grant RP210041 to J-UJ, NIH R01 HL147661 and I1243 from Welch Foundation to MC.
Conflict of Interest
The authors declare that the research was conducted in the absence of any commercial or financial relationships that could be construed as a potential conflict of interest.
Publisher’s Note
All claims expressed in this article are solely those of the authors and do not necessarily represent those of their affiliated organizations, or those of the publisher, the editors and the reviewers. Any product that may be evaluated in this article, or claim that may be made by its manufacturer, is not guaranteed or endorsed by the publisher.
Acknowledgments
We thank members of the Cobb laboratory and Gray Pearson (Georgetown University) for discussing this work and Dionne Ware for administrative support.
References
Adams, N. R., Vasquez, Y. M., Mo, Q., Gibbons, W., Kovanci, E., and Demayo, F. J. (2017). WNK Lysine Deficient Protein Kinase 1 Regulates Human Endometrial Stromal Cell Decidualization, Proliferation, and Migration in Part through Mitogen-Activated Protein Kinase 7. Biol. Reprod. 97, 400–412. doi:10.1093/biolre/iox108
Al-Qusairi, L., Basquin, D., Roy, A., Rajaram, R. D., Maillard, M. P., Subramanya, A. R., et al. (2017). Renal Tubular Ubiquitin-Protein Ligase NEDD4-2 Is Required for Renal Adaptation during Long-Term Potassium Depletion. J. Am. Soc. Nephrol. 28, 2431–2442. doi:10.1681/asn.2016070732
Anselmo, A. N., Earnest, S., Chen, W., Juang, Y.-C., Kim, S. C., Zhao, Y., et al. (2006). WNK1 and OSR1 Regulate the Na + , K + , 2Cl − Cotransporter in HeLa Cells. Proc. Natl. Acad. Sci. U.S.A. 103, 10883–10888. doi:10.1073/pnas.0604607103
Barrios-Rodiles, M., Brown, K. R., Ozdamar, B., Bose, R., Liu, Z., Donovan, R. S., et al. (2005). High-Throughput Mapping of a Dynamic Signaling Network in Mammalian Cells. Science 307, 1621–1625. doi:10.1126/science.1105776
Bauer, T., Zagórska, A., Jurkin, J., Yasmin, N., Köffel, R., Richter, S., et al. (2012). Identification of Axl as a Downstream Effector of TGF-Β1 during Langerhans Cell Differentiation and Epidermal Homeostasis. J. Exp. Med. 209, 2033–2047. doi:10.1084/jem.20120493
Bennet, D., Khorsandian, Y., Pelusi, J., Mirabella, A., Pirrotte, P., and Zenhausern, F. (2021). Molecular and Physical Technologies for Monitoring Fluid and Electrolyte Imbalance: A Focus on Cancer Population. Clin. Transl. Med. 11, e461. doi:10.1002/ctm2.461
Brabletz, T., Kalluri, R., Nieto, M. A., and Weinberg, R. A. (2018). EMT in Cancer. Nat. Rev. Cancer 18, 128–134. doi:10.1038/nrc.2017.118
Chen, W., Yazicioglu, M., and Cobb, M. H. (2004). Characterization of OSR1, a Member of the Mammalian Ste20p/germinal Center Kinase Subfamily. J. Biol. Chem. 279, 11129–11136. doi:10.1074/jbc.m313562200
Chen, L., Jenjaroenpun, P., Pillai, A. M., Ivshina, A. V., Ow, G. S., Efthimios, M., et al. (2017). Transposon Insertional Mutagenesis in Mice Identifies Human Breast Cancer Susceptibility Genes and Signatures for Stratification. Proc. Natl. Acad. Sci. U. S. A. 114, E2215–E2224. doi:10.1073/pnas.1701512114
Chen, Z., Zhang, J., Murillo-De-Ozores, A. R., Castañeda-Bueno, M., D'amico, F., Heilig, R., et al. (2022). Sequence and Structural Variations Determining the Recruitment of WNK Kinases to the KLHL3 E3 Ligase. Biochem. J. 479, 661–675. doi:10.1042/bcj20220019
Clark, A. G., and Vignjevic, D. M. (2015). Modes of Cancer Cell Invasion and the Role of the Microenvironment. Curr. Opin. Cell. Biol. 36, 13–22. doi:10.1016/j.ceb.2015.06.004
Cooley, B. C., Nevado, J., Mellad, J., Yang, D., St Hilaire, C., Negro, A., et al. (2014). TGF-β Signaling Mediates Endothelial-To-Mesenchymal Transition (EndMT) during Vein Graft Remodeling. Sci. Transl. Med. 6, 227ra34. doi:10.1126/scitranslmed.3006927
Cope, G., Murthy, M., Golbang, A. P., Hamad, A., Liu, C.-H., Cuthbert, A. W., et al. (2006). WNK1 Affects Surface Expression of the ROMK Potassium Channel Independent of WNK4. J. Am. Soc. Nephrol. 17, 1867–1874. doi:10.1681/asn.2005111224
Costa, V., Esposito, R., Ziviello, C., Sepe, R., Bim, L. V., Cacciola, N. A., et al. (2015). New Somatic Mutations andWNK1-B4GALNT3gene Fusion in Papillary Thyroid Carcinoma. Oncotarget 6, 11242–11251. doi:10.18632/oncotarget.3593
Cuddapah, V. A., and Sontheimer, H. (2011). Ion Channels and Tranporters in Cancer. 2. Ion Channels and the Control of Cancer Cell Migration. Am. J. Physiology-Cell Physiology 301, C541–C549. doi:10.1152/ajpcell.00102.2011
Cummins, P. M. (2012). Occludin: One Protein, Many Forms. Mol. Cell. Biol. 32, 242–250. doi:10.1128/MCB.06029-11
Dang, T. T., Esparza, M. A., Maine, E. A., Westcott, J. M., and Pearson, G. W. (2015). ΔNp63α Promotes Breast Cancer Cell Motility through the Selective Activation of Components of the Epithelial-To-Mesenchymal Transition Program. Cancer Res. 75, 3925–3935. doi:10.1158/0008-5472.can-14-3363
Dbouk, H. A., Weil, L. M., Perera, G. K. S., Dellinger, M. T., Pearson, G., Brekken, R. A., et al. (2014). Actions of the Protein Kinase WNK1 on Endothelial Cells Are Differentially Mediated by its Substrate Kinases OSR1 and SPAK. Proc. Natl. Acad. Sci. U.S.A. 111 (45), 15999–16004. doi:10.1073/pnas.1419057111
Dbouk, H. A., Huang, C.-L., and Cobb, M. H. (2016). Hypertension: the Missing WNKs. Am. J. Physiology-Renal Physiology 311, F16–F27. doi:10.1152/ajprenal.00358.2015
Dejana, E. (2004). Endothelial Cell-Cell Junctions: Happy Together. Nat. Rev. Mol. Cell. Biol. 5, 261–270. doi:10.1038/nrm1357
Delaloy, C., Lu, J., Houot, A.-M., Disse-Nicodeme, S., Gasc, J.-M., Corvol, P., et al. (2003). Multiple Promoters in the WNK1 Gene: One Controls Expression of a Kidney-specific Kinase-Defective Isoform. Mol. Cell. Biol. 23, 9208–9221. doi:10.1128/mcb.23.24.9208-9221.2003
Desjardins, P., Couture, C., Germain, L., and Guérin, S. L. (2019). Contribution of the WNK1 Kinase to Corneal Wound Healing Using the Tissue‐engineered Human Cornea as an In Vitro Model. J. Tissue Eng. Regen. Med. 13, 1595–1608. doi:10.1002/term.2912
Dong, H., Jian, P., Yu, M., and Wang, L. (2020). Silencing of Long Noncoding RNA LEF1‐AS1 Prevents the Progression of Hepatocellular Carcinoma via the Crosstalk with microRNA‐136‐5p/WNK1. J. Cell. Physiology 235, 6548–6562. doi:10.1002/jcp.29503
Du, D., Xu, F., Yu, L., Zhang, C., Lu, X., Yuan, H., et al. (2010). The Tight Junction Protein, Occludin, Regulates the Directional Migration of Epithelial Cells. Dev. Cell. 18, 52–63. doi:10.1016/j.devcel.2009.12.008
Engelsen, A. S. T., Wnuk-Lipinska, K., Bougnaud, S., Pelissier Vatter, F. A., Tiron, C., Villadsen, R., et al. (2020). AXL Is a Driver of Stemness in Normal Mammary Gland and Breast Cancer. iScience 23, 101649. doi:10.1016/j.isci.2020.101649
Flatman, P. W. (2008). Cotransporters, WNKs and Hypertension: an Update. Curr. Opin. Nephrol. Hypertens. 17, 186–192. doi:10.1097/mnh.0b013e3282f5244e
Folkman, J., and Shing, Y. (1992). Angiogenesis. J. Biol. Chem. 267, 10931–10934. doi:10.1016/s0021-9258(19)49853-0
Fujino, N., Kubo, H., and Maciewicz, R. A. (2017). Phenotypic Screening Identifies Axl Kinase as a Negative Regulator of an Alveolar Epithelial Cell Phenotype. Lab. Invest. 97, 1047–1062. doi:10.1038/labinvest.2017.52
Fulford, L., Milewski, D., Ustiyan, V., Ravishankar, N., Cai, Y., Le, T., et al. (2016). The Transcription Factor FOXF1 Promotes Prostate Cancer by Stimulating the Mitogen-Activated Protein Kinase ERK5. Sci. Signal 9, ra48. doi:10.1126/scisignal.aad5582
Gagnon, K. B. E., England, R., and Delpire, E. (2006). Volume Sensitivity of Cation-Cl−cotransporters Is Modulated by the Interaction of Two Kinases: Ste20-Related Proline-Alanine-Rich Kinase and WNK4. Am. J. Physiology-Cell Physiology 290, C134–C142. doi:10.1152/ajpcell.00037.2005
Gallicchio, M., Mitola, S., Valdembri, D., Fantozzi, R., Varnum, B., Avanzi, G. C., et al. (2005). Inhibition of Vascular Endothelial Growth Factor Receptor 2-mediated Endothelial Cell Activation by Axl Tyrosine Kinase Receptor. Blood 105, 1970–1976. doi:10.1182/blood-2004-04-1469
Gallolu Kankanamalage, S., Karra, A. S., and Cobb, M. H. (2018). WNK Pathways in Cancer Signaling Networks. Cell. Commun. Signal 16, 72. doi:10.1186/s12964-018-0287-1
Gallolu Kankanamalage, S., Lee, A.-Y., Wichaidit, C., Lorente-Rodriguez, A., Shah, A. M., Stippec, S., et al. (2016). Multistep Regulation of Autophagy by WNK1. Proc. Natl. Acad. Sci. U.S.A. 113, 14342–14347. doi:10.1073/pnas.1617649113
Gamba, G. (2005). Molecular Physiology and Pathophysiology of Electroneutral Cation-Chloride Cotransporters. Physiol. Rev. 85, 423–493. doi:10.1152/physrev.00011.2004
Garrido-Castro, A. C., Lin, N. U., and Polyak, K. (2019). Insights into Molecular Classifications of Triple-Negative Breast Cancer: Improving Patient Selection for Treatment. Cancer Discov. 9, 176–198. doi:10.1158/2159-8290.cd-18-1177
Garzon-Muvdi, T., Schiapparelli, P., Ap Rhys, C., Guerrero-Cazares, H., Smith, C., Kim, D.-H., et al. (2012). Regulation of Brain Tumor Dispersal by NKCC1 through a Novel Role in Focal Adhesion Regulation. PLoS Biol. 10, e1001320. doi:10.1371/journal.pbio.1001320
He, G., Wang, H.-R., Huang, S.-K., and Huang, C.-L. (2007). Intersectin Links WNK Kinases to Endocytosis of ROMK1. J. Clin. Invest. 117, 1078–1087. doi:10.1172/jci30087
Heise, C. J., Xu, B.-e., Deaton, S. L., Cha, S.-K., Cheng, C.-J., Earnest, S., et al. (2010). Serum and Glucocorticoid-Induced Kinase (SGK) 1 and the Epithelial Sodium Channel Are Regulated by Multiple with No Lysine (WNK) Family Members. J. Biol. Chem. 285, 25161–25167. doi:10.1074/jbc.m110.103432
Henriques, A. F. A., Matos, P., Carvalho, A. S., Azkargorta, M., Elortza, F., Matthiesen, R., et al. (2020). WNK1 Phosphorylation Sites in TBC1D1 and TBC1D4 Modulate Cell Surface Expression of GLUT1. Archives Biochem. Biophysics 679, 108223. doi:10.1016/j.abb.2019.108223
Ho, Y.-J., Chang, J., Yeh, K.-T., Gong, Z., Lin, Y.-M., and Lu, J.-W. (2020). Prognostic and Clinical Implications of WNK Lysine Deficient Protein Kinase 1 Expression in Patients with Hepatocellular Carcinoma. Vivo 34, 2631–2640. doi:10.21873/invivo.12081
Holland, S. J., Powell, M. J., Franci, C., Chan, E. W., Friera, A. M., Atchison, R. E., et al. (2005). Multiple Roles for the Receptor Tyrosine Kinase Axl in Tumor Formation. Cancer Res. 65, 9294–9303. doi:10.1158/0008-5472.CAN-05-0993
Huang, C.-L., Cha, S.-K., Wang, H.-R., Xie, J., and Cobb, M. H. (2007). WNKs: Protein Kinases with a Unique Kinase Domain. Exp. Mol. Med. 39, 565–573. doi:10.1038/emm.2007.62
Hung, J.-Y., Yen, M.-C., Jian, S.-F., Wu, C.-Y., Chang, W.-A., Liu, K.-T., et al. (2017). Secreted Protein Acidic and Rich in Cysteine (SPARC) Induces Cell Migration and Epithelial Mesenchymal Transition through WNK1/snail in Non-small Cell Lung Cancer. Oncotarget 8, 63691–63702. doi:10.18632/oncotarget.19475
Jaykumar, A. B., Jung, J.-U., Parida, P. K., Dang, T. T., Wichaidit, C., Kannangara, A. R., et al. (2021). WNK1 Enhances Migration and Invasion in Breast Cancer Models. Mol. Cancer Ther. 20, 1800–1808. doi:10.1158/1535-7163.mct-21-0174
Jaykumar, A. B., Plumber, S., Barry, D. M., Binns, D., Wichaidit, C., Grzemska, M., et al. (2022). WNK1 Collaborates with TGF-β in Endothelial Cell Junction Turnover and Angiogenesis. Proc. Natl. Acad. Sci. U. S. A. (In Press).
Jiang, Z. Y., Zhou, Q. L., Holik, J., Patel, S., Leszyk, J., Coleman, K., et al. (2005). Identification of WNK1 as a Substrate of Akt/protein Kinase B and a Negative Regulator of Insulin-Stimulated Mitogenesis in 3T3-L1 Cells. J. Biol. Chem. 280, 21622–21628. doi:10.1074/jbc.m414464200
Jumper, J., Evans, R., Pritzel, A., Green, T., Figurnov, M., Ronneberger, O., et al. (2021). Highly Accurate Protein Structure Prediction with AlphaFold. Nature 596, 583–589. doi:10.1038/s41586-021-03819-2
Jung, J. U., Ghosh, A., Earnest, S., Deaton, S. L., and Cobb, M. H. (2022). UBR5 Is a Novel Regulator of WNK1 Stability. Am. J. Physiol. Cell. Physiol. 322, C1176–C1186. doi:10.1152/ajpcell.00417.2021
Kahle, K. T., Rinehart, J., De Los Heros, P., Louvi, A., Meade, P., Vazquez, N., et al. (2005). WNK3 Modulates Transport of Cl - in and Out of Cells: Implications for Control of Cell Volume and Neuronal Excitability. Proc. Natl. Acad. Sci. U.S.A. 102, 16783–16788. doi:10.1073/pnas.0508307102
Kim, J. H., Kim, H., Hwang, K. H., Chang, J. S., Park, K. S., Cha, S. K., et al. (2018). WNK1 Kinase Is Essential for Insulin‐stimulated GLUT4 Trafficking in Skeletal Muscle. FEBS Open Bio 8, 1866–1874. doi:10.1002/2211-5463.12528
Kim, J. H., Hwang, K. H., Eom, M., Kim, M., Park, E. Y., Jeong, Y., et al. (2019). WNK1 Promotes Renal Tumor Progression by Activating TRPC6‐NFAT Pathway. FASEB J. 33, 8588–8599. doi:10.1096/fj.201802019rr
Köchl, R., Thelen, F., Vanes, L., Brazão, T. F., Fountain, K., Xie, J., et al. (2016). WNK1 Kinase Balances T Cell Adhesion versus Migration In Vivo. Nat. Immunol. 17, 1075–1083. doi:10.1038/ni.3495
Lambert, A. W., Pattabiraman, D. R., and Weinberg, R. A. (2017). Emerging Biological Principles of Metastasis. Cell. 168, 670–691. doi:10.1016/j.cell.2016.11.037
Lazrak, A., Liu, Z., and Huang, C.-L. (2006). Antagonistic Regulation of ROMK by Long and Kidney-specific WNK1 Isoforms. Proc. Natl. Acad. Sci. U.S.A. 103, 1615–1620. doi:10.1073/pnas.0510609103
Lee, B.-H., Min, X., Heise, C. J., Xu, B.-e., Chen, S., Shu, H., et al. (2004). WNK1 Phosphorylates Synaptotagmin 2 and Modulates its Membrane Binding. Mol. Cell. 15, 741–751. doi:10.1016/j.molcel.2004.07.018
Lee, B.-H., Chen, W., Stippec, S., and Cobb, M. H. (2007). Biological Cross-Talk between WNK1 and the Transforming Growth Factor β-Smad Signaling Pathway. J. Biol. Chem. 282, 17985–17996. doi:10.1074/jbc.m702664200
Lee, H.-J., Jeng, Y.-M., Chen, Y.-L., Chung, L., and Yuan, R.-H. (2014). Gas6/Axl Pathway Promotes Tumor Invasion through the Transcriptional Activation of Slug in Hepatocellular Carcinoma. Carcinogenesis 35, 769–775. doi:10.1093/carcin/bgt372
Lenertz, L. Y., Lee, B.-H., Min, X., Xu, B.-e., Wedin, K., Earnest, S., et al. (2005). Properties of WNK1 and Implications for Other Family Members. J. Biol. Chem. 280, 26653–26658. doi:10.1074/jbc.m502598200
Li, Y., Ye, X., Tan, C., Hongo, J.-A., Zha, J., Liu, J., et al. (2009). Axl as a Potential Therapeutic Target in Cancer: Role of Axl in Tumor Growth, Metastasis and Angiogenesis. Oncogene 28, 3442–3455. doi:10.1038/onc.2009.212
Li, C., Feng, M., Shi, Z., Hao, Q., Song, X., Wang, W., et al. (2014). Structural and Biochemical Insights into the Activation Mechanisms of Germinal Center Kinase OSR1. J. Biol. Chem. 289, 35969–35978. doi:10.1074/jbc.m114.592097
Li, Y., Chen, X., Shen, Z., Wang, Y., Hu, J., Xu, J., et al. (2020). Electrolyte and Acid-Base Disorders in Cancer Patients and its Impact on Clinical Outcomes: Evidence from a Real-World Study in China. Ren. Fail. 42, 234–243. doi:10.1080/0886022x.2020.1735417
Li, Y., Li, L., Qin, J., Wu, J., Dai, X., and Xu, J. (2021). OSR1 Phosphorylates the Smad2/3 Linker Region and Induces TGF-Β1 Autocrine to Promote EMT and Metastasis in Breast Cancer. Oncogene 40, 68–84. doi:10.1038/s41388-020-01499-2
Lin, C.-M., Cheng, C.-J., Yang, S.-S., Tseng, M.-H., Yen, M.-T., Sung, C.-C., et al. (2019). Generation and Analysis of a Mouse Model of Pseudohypoaldosteronism Type II Caused by KLHL3 Mutation in BTB Domain. FASEB J. 33, 1051–1061. doi:10.1096/fj.201801023r
Liu, X., Dreffs, A., Díaz-Coránguez, M., Runkle, E. A., Gardner, T. W., Chiodo, V. A., et al. (2016). Occludin S490 Phosphorylation Regulates Vascular Endothelial Growth Factor-Induced Retinal Neovascularization. Am. J. Pathology 186, 2486–2499. doi:10.1016/j.ajpath.2016.04.018
Liu, Y., Liu, T., Li, N., Wang, T., Pu, Y., and Lin, R. (2019). Identification of a Novel WNK1-ROS1 Fusion in a Lung Adenocarcinoma Sensitive to Crizotinib. Lung Cancer 129, 92–94. doi:10.1016/j.lungcan.2018.12.011
Liu, Y., Song, X., Shi, Y., Shi, Z., Niu, W., Feng, X., et al. (2015). WNK1 Activates Large-Conductance Ca2+-Activated K+Channels through Modulation of ERK1/2 Signaling. J. Am. Soc. Nephrol. 26, 844–854. doi:10.1681/asn.2014020186
Liu, Z., Yoon, J., Wichaidit, C., Jaykumar, A. B., Dbouk, H. A., Embry, A. E., et al. (2020). Control of Podocyte and Glomerular Capillary Wall Structure and Elasticity by WNK1 Kinase. Front. Cell. Dev. Biol. 8, 618898. doi:10.3389/fcell.2020.618898
Ma, J., Sanchez-Duffhues, G., Goumans, M.-J., and Ten Dijke, P. (2020). TGF-β-Induced Endothelial to Mesenchymal Transition in Disease and Tissue Engineering. Front. Cell. Dev. Biol. 8, 260. doi:10.3389/fcell.2020.00260
Mahmoud, M. M., Serbanovic-Canic, J., Feng, S., Souilhol, C., Xing, R., Hsiao, S., et al. (2017). Shear Stress Induces Endothelial-To-Mesenchymal Transition via the Transcription Factor Snail. Sci. Rep. 7, 3375. doi:10.1038/s41598-017-03532-z
Mariotti, A., Perotti, A., Sessa, C., and Rüegg, C. (2007). N-cadherin as a Therapeutic Target in Cancer. Expert Opin. Investigational Drugs 16, 451–465. doi:10.1517/13543784.16.4.451
Martial, S. (2016). Involvement of Ion Channels and Transporters in Carcinoma Angiogenesis and Metastasis. Am. J. Physiology-Cell Physiology 310, C710–C727. doi:10.1152/ajpcell.00218.2015
Mccormick, J. A., and Ellison, D. H. (2011). The WNKs: Atypical Protein Kinases with Pleiotropic Actions. Physiol. Rev. 91, 177–219. doi:10.1152/physrev.00017.2010
Mccormick, J. A., Yang, C.-L., Zhang, C., Davidge, B., Blankenstein, K. I., Terker, A. S., et al. (2014). Hyperkalemic Hypertension-Associated Cullin 3 Promotes WNK Signaling by Degrading KLHL3. J. Clin. Invest. 124, 4723–4736. doi:10.1172/jci76126
Mendes, A. I., Matos, P., Moniz, S., and Jordan, P. (2010). Protein Kinase WNK1 Promotes Cell Surface Expression of Glucose Transporter GLUT1 by Regulating a Tre-2/usp6-BUB2-Cdc16 Domain Family Member 4 (TBC1D4)-Rab8A Complex. J. Biol. Chem. 285, 39117–39126. doi:10.1074/jbc.m110.159418
Miltiadous, G., Christidis, D., Kalogirou, M., and Elisaf, M. (2008). Causes and Mechanisms of Acid-Base and Electrolyte Abnormalities in Cancer Patients. Eur. J. Intern. Med. 19, 1–7. doi:10.1016/j.ejim.2007.04.016
Min, X., Lee, B.-H., Cobb, M. H., and Goldsmith, E. J. (2004). Crystal Structure of the Kinase Domain of WNK1, a Kinase that Causes a Hereditary Form of Hypertension. Structure 12, 1303–1311. doi:10.1016/j.str.2004.04.014
Moon, T. M., Correa, F., Kinch, L. N., Piala, A. T., Gardner, K. H., and Goldsmith, E. J. (2013). Solution Structure of the WNK1 Autoinhibitory Domain, a WNK-specific PF2 Domain. J. Mol. Biol. 425, 1245–1252. doi:10.1016/j.jmb.2013.01.031
Moriguchi, T., Urushiyama, S., Hisamoto, N., Iemura, S.-i., Uchida, S., Natsume, T., et al. (2005). WNK1 Regulates Phosphorylation of Cation-Chloride-Coupled Cotransporters via the STE20-Related Kinases, SPAK and OSR1. J. Biol. Chem. 280, 42685–42693. doi:10.1074/jbc.m510042200
Mrozik, K. M., Blaschuk, O. W., Cheong, C. M., Zannettino, A. C. W., and Vandyke, K. (2018). N-cadherin in Cancer Metastasis, its Emerging Role in Haematological Malignancies and Potential as a Therapeutic Target in Cancer. BMC Cancer 18, 939. doi:10.1186/s12885-018-4845-0
Nakajima, Y., Yamagishi, T., Hokari, S., and Nakamura, H. (2000). Mechanisms Involved in Valvuloseptal Endocardial Cushion Formation in Early Cardiogenesis: Roles of Transforming Growth Factor (TGF)-β and Bone Morphogenetic Protein (BMP). Anat. Rec. 258, 119–127. doi:10.1002/(sici)1097-0185(20000201)258:2<119:aid-ar1>3.0.co;2-u
O'reilly, M., Marshall, E., Speirs, H. J., and Brown, R. W. (2003). WNK1, a Gene within a Novel Blood Pressure Control Pathway, Tissue-Specifically Generates Radically Different Isoforms with and without a Kinase Domain. J. Am. Soc. Nephrol. 14, 2447–2456. doi:10.1097/01.asn.0000089830.97681.3b
Oh, J.-E., Kim, R. H., Shin, K.-H., Park, N.-H., and Kang, M. K. (2011). ΔNp63α Protein Triggers Epithelial-Mesenchymal Transition and Confers Stem Cell Properties in Normal Human Keratinocytes. J. Biol. Chem. 286 (44), 38757–38767. doi:10.1074/jbc.m111.244939
Ohta, A., Schumacher, F.-R., Mehellou, Y., Johnson, C., Knebel, A., Macartney, T. J., et al. (2013). The CUL3-KLHL3 E3 Ligase Complex Mutated in Gordon's Hypertension Syndrome Interacts with and Ubiquitylates WNK Isoforms: Disease-Causing Mutations in KLHL3 and WNK4 Disrupt Interaction. Biochem. J. 451, 111–122. doi:10.1042/bj20121903
Otrock, Z., Mahfouz, R., Makarem, J., and Shamseddine, A. (2007). Understanding the Biology of Angiogenesis: Review of the Most Important Molecular Mechanisms. Blood Cells, Mol. Dis. 39, 212–220. doi:10.1016/j.bcmd.2007.04.001
Ozdamar, B., Bose, R., Barrios-Rodiles, M., Wang, H.-R., Zhang, Y., and Wrana, J. L. (2005). Regulation of the Polarity Protein Par6 by TGFß Receptors Controls Epithelial Cell Plasticity. Science 307, 1603–1609. doi:10.1126/science.1105718
Pardali, E., Sanchez-Duffhues, G., Gomez-Puerto, M., and Ten Dijke, P. (2017). TGF-β-Induced Endothelial-Mesenchymal Transition in Fibrotic Diseases. Int. J. Mol. Sci. 18, 2157. doi:10.3390/ijms18102157
Piala, A. T., Moon, T. M., Akella, R., He, H., Cobb, M. H., and Goldsmith, E. J. (2014). Chloride Sensing by WNK1 Involves Inhibition of Autophosphorylation. Sci Signal 7, ra41.
Pio, G. M., Xia, Y., Piaseczny, M. M., Chu, J. E., and Allan, A. L. (2017). Soluble Bone-Derived Osteopontin Promotes Migration and Stem-like Behavior of Breast Cancer Cells. PLoS ONE 12, e0177640. doi:10.1371/journal.pone.0177640
Ramesh, V., Brabletz, T., and Ceppi, P. (2020). Targeting EMT in Cancer with Repurposed Metabolic Inhibitors. Trends Cancer 6, 942–950. doi:10.1016/j.trecan.2020.06.005
Rao, R. (2009). Occludin Phosphorylation in Regulation of Epithelial Tight Junctions. Ann. N. Y. Acad. Sci. 1165 (1), 62–68. doi:10.1111/j.1749-6632.2009.04054.x
Reichl, P., Dengler, M., vanZijl, F., Huber, H., Führlinger, G., Reichel, C., et al. (2015). Axl Activates Autocrine Transforming Growth Factor‐β Signaling in Hepatocellular Carcinoma. Hepatology 61, 930–941. doi:10.1002/hep.27492
Ribatti, D., Tamma, R., and Annese, T. (2020). Epithelial-Mesenchymal Transition in Cancer: A Historical Overview. Transl. Oncol. 13, 100773. doi:10.1016/j.tranon.2020.100773
Richardson, C., and Alessi, D. R. (2008). The Regulation of Salt Transport and Blood Pressure by the WNK-SPAK/OSR1 Signalling Pathway. J. Cell. Sci. 121, 3293–3304. doi:10.1242/jcs.029223
Richardson, C., Rafiqi, F. H., Karlsson, H. K. R., Moleleki, N., Vandewalle, A., Campbell, D. G., et al. (2008). Activation of the Thiazide-Sensitive Na+-Cl- Cotransporter by the WNK-Regulated Kinases SPAK and OSR1. J. Cell. Sci. 121, 675–684. doi:10.1242/jcs.025312
Rosner, M. H., and Dalkin, A. C. (2014). Electrolyte Disorders Associated with Cancer. Adv. Chronic Kidney Dis. 21, 7–17. doi:10.1053/j.ackd.2013.05.005
Roy, A., Al-Qusairi, L., Donnelly, B. F., Ronzaud, C., Marciszyn, A. L., Gong, F., et al. (2015). Alternatively Spliced Proline-Rich Cassettes Link WNK1 to Aldosterone Action. J. Clin. Invest. 125, 3433–3448. doi:10.1172/jci75245
Rudini, N., Felici, A., Giampietro, C., Lampugnani, M., Corada, M., Swirsding, K., et al. (2008). VE-cadherin Is a Critical Endothelial Regulator of TGF-β Signalling. EMBO J. 27, 993–1004. doi:10.1038/emboj.2008.46
Sato, A., Shimizu, M., Goto, T., Masuno, H., Kagechika, H., Tanaka, N., et al. (2020). WNK Regulates Wnt Signalling and β-Catenin Levels by Interfering with the Interaction between β-Catenin and GID. Commun. Biol. 3, 666. doi:10.1038/s42003-020-01386-2
Schumacher, F.-R., Sorrell, F. J., Alessi, D. R., Bullock, A. N., and Kurz, T. (2014). Structural and Biochemical Characterization of the KLHL3-WNK Kinase Interaction Important in Blood Pressure Regulation. Biochem. J. 460, 237–246. doi:10.1042/bj20140153
Schwab, A., and Stock, C. (2014). Ion Channels and Transporters in Tumour Cell Migration and Invasion. Phil. Trans. R. Soc. B 369, 20130102. doi:10.1098/rstb.2013.0102
Shahi Thakuri, P., Gupta, M., Singh, S., Joshi, R., Glasgow, E., Lekan, A., et al. (2020). Phytochemicals Inhibit Migration of Triple Negative Breast Cancer Cells by Targeting Kinase Signaling. BMC Cancer 20, 4. doi:10.1186/s12885-019-6479-2
Shekarabi, M., Zhang, J., Khanna, A. R., Ellison, D. H., Delpire, E., and Kahle, K. T. (2017). WNK Kinase Signaling in Ion Homeostasis and Human Disease. Cell. Metab. 25, 285–299. doi:10.1016/j.cmet.2017.01.007
Shibata, S., Zhang, J., Puthumana, J., Stone, K. L., and Lifton, R. P. (2013). Kelch-like 3 and Cullin 3 Regulate Electrolyte Homeostasis via Ubiquitination and Degradation of WNK4. Proc. Natl. Acad. Sci. U.S.A. 110, 7838–7843. doi:10.1073/pnas.1304592110
Shyamasundar, S., Lim, J. P., and Bay, B. H. (2016). miR-93 Inhibits the Invasive Potential of Triple-Negative Breast Cancer Cells In Vitro via Protein Kinase WNK1. Int. J. Oncol. 49, 2629–2636. doi:10.3892/ijo.2016.3761
Sie, Z.-L., Li, R.-Y., Sampurna, B. P., Hsu, P.-J., Liu, S.-C., Wang, H.-D., et al. (2020). WNK1 Kinase Stimulates Angiogenesis to Promote Tumor Growth and Metastasis. Cancers 12, 575. doi:10.3390/cancers12030575
Stock, C., and Schwab, A. (2015). Ion Channels and Transporters in Metastasis. Biochimica Biophysica Acta (BBA) - Biomembr. 1848, 2638–2646. doi:10.1016/j.bbamem.2014.11.012
Sun, H., Long, S., Wu, B., Liu, J., and Li, G. (2020). NKCC1 Involvement in the Epithelial-To-Mesenchymal Transition Is a Prognostic Biomarker in Gliomas. PeerJ 8, e8787. doi:10.7717/peerj.8787
Sun, X., Gao, L., Yu, R. K., and Zeng, G. (2006). Down-regulation of WNK1 Protein Kinase in Neural Progenitor Cells Suppresses Cell Proliferation and Migration. J. Neurochem. 99, 1114–1121. doi:10.1111/j.1471-4159.2006.04159.x
Susa, K., Sohara, E., Rai, T., Zeniya, M., Mori, Y., Mori, T., et al. (2014). Impaired Degradation of WNK1 and WNK4 Kinases Causes PHAII in Mutant KLHL3 Knock-In Mice. Hum. Mol. Genet. 23, 5052–5060. doi:10.1093/hmg/ddu217
Tan, S.-X., Ng, Y., Burchfield, J. G., Ramm, G., Lambright, D. G., Stöckli, J., et al. (2012). The Rab GTPase-Activating Protein TBC1D4/AS160 Contains an Atypical Phosphotyrosine-Binding Domain that Interacts with Plasma Membrane Phospholipids to Facilitate GLUT4 Trafficking in Adipocytes. Mol. Cell. Biol. 32, 4946–4959. doi:10.1128/mcb.00761-12
Tanaka, M., and Siemann, D. W. (2019). Axl Signaling Is an Important Mediator of Tumor Angiogenesis. Oncotarget 10, 2887–2898. doi:10.18632/oncotarget.26882
Taylor, C. A., An, S.-W., Kankanamalage, S. G., Stippec, S., Earnest, S., Trivedi, A. T., et al. (2018). OSR1 Regulates a Subset of Inward Rectifier Potassium Channels via a Binding Motif Variant. Proc. Natl. Acad. Sci. U.S.A. 115, 3840–3845. doi:10.1073/pnas.1802339115
Tu, S.-w., Bugde, A., Luby-Phelps, K., and Cobb, M. H. (2011). WNK1 Is Required for Mitosis and Abscission. Proc. Natl. Acad. Sci. U.S.A. 108, 1385–1390. doi:10.1073/pnas.1018567108
Van Zijl, F., Krupitza, G., and Mikulits, W. (2011). Initial Steps of Metastasis: Cell Invasion and Endothelial Transmigration. Mutat. Research/Reviews Mutat. Res. 728, 23–34. doi:10.1016/j.mrrev.2011.05.002
Varadi, M., Anyango, S., Deshpande, M., Nair, S., Natassia, C., Yordanova, G., et al. (2021). AlphaFold Protein Structure Database: Massively Expanding the Structural Coverage of Protein-Sequence Space with High-Accuracy Models. Nucleic Acids Res. 50, D439–D444. doi:10.1093/nar/gkab1061
Veríssimo, F., and Jordan, P. (2001). WNK Kinases, a Novel Protein Kinase Subfamily in Multi-Cellular Organisms. Oncogene 20, 5562–5569. doi:10.1038/sj.onc.1204726
Vidal-Petiot, E., Cheval, L., Faugeroux, J., Malard, T., Doucet, A., Jeunemaitre, X., et al. (2012). A New Methodology for Quantification of Alternatively Spliced Exons Reveals a Highly Tissue-specific Expression Pattern of WNK1 Isoforms. PLoS One 7, e37751. doi:10.1371/journal.pone.0037751
Vitari, A. C., Deak, M., Morrice, N. A., and Alessi, D. R. (2005). The WNK1 and WNK4 Protein Kinases that Are Mutated in Gordon's Hypertension Syndrome Phosphorylate and Activate SPAK and OSR1 Protein Kinases. Biochem. J. 391, 17–24. doi:10.1042/bj20051180
Vitari, A. C., Thastrup, J., Rafiqi, F. H., Deak, M., Morrice, N. A., Karlsson, H. K. R., et al. (2006). Functional Interactions of the SPAK/OSR1 Kinases with Their Upstream Activator WNK1 and Downstream Substrate NKCC1. Biochem. J. 397, 223–231. doi:10.1042/bj20060220
Wang, H.-R., Liu, Z., and Huang, C.-L. (2008). Domains of WNK1 Kinase in the Regulation of ROMK1. Am. J. Physiology-Renal Physiology 295, F438–F445. doi:10.1152/ajprenal.90287.2008
Wang, L., Fan, J., Francis, J. M., Georghiou, G., Hergert, S., Li, S., et al. (2017). Integrated Single-Cell Genetic and Transcriptional Analysis Suggests Novel Drivers of Chronic Lymphocytic Leukemia. Genome Res. 27, 1300–1311. doi:10.1101/gr.217331.116
Wang, J.-f., Zhao, K., Chen, Y.-y., Qiu, Y., Zhu, J.-h., Li, B.-p., et al. (2021). NKCC1 Promotes Proliferation, Invasion and Migration in Human Gastric Cancer Cells via Activation of the MAPK-JNK/EMT Signaling Pathway. J. Cancer 12, 253–263. doi:10.7150/jca.49709
Welch-Reardon, K. M., Ehsan, S. M., Wang, K., Wu, N., Newman, A. C., Romero-Lopez, M., et al. (2014). Angiogenic Sprouting Is Regulated by Endothelial Cell Expression of Slug. J. Cell. Sci. 127, 2017–2028. doi:10.1242/jcs.143420
Welch-Reardon, K. M., Wu, N., and Hughes, C. C. W. (2015). A Role for Partial Endothelial-Mesenchymal Transitions in Angiogenesis? Arterioscler. Thromb. Vasc. Biol. 35, 303–308. doi:10.1161/atvbaha.114.303220
Wilson, F. H., Disse-Nicodème, S., Choate, K. A., Ishikawa, K., Nelson-Williams, C., Desitter, I., et al. (2001). Human Hypertension Caused by Mutations in WNK Kinases. Science 293, 1107–1112. doi:10.1126/science.1062844
Xie, J., Wu, T., Xu, K., Huang, I. K., Cleaver, O., and Huang, C.-L. (2009). Endothelial-Specific Expression of WNK1 Kinase Is Essential for Angiogenesis and Heart Development in Mice. Am. J. Pathology 175, 1315–1327. doi:10.2353/ajpath.2009.090094
Xie, J., Yoon, J., Yang, S.-S., Lin, S.-H., and Huang, C.-L. (2013). WNK1 Protein Kinase Regulates Embryonic Cardiovascular Development through the OSR1 Signaling Cascade. J. Biol. Chem. 288, 8566–8574. doi:10.1074/jbc.m113.451575
Xu, B.-e., English, J. M., Wilsbacher, J. L., Stippec, S., Goldsmith, E. J., and Cobb, M. H. (2000). WNK1, a Novel Mammalian Serine/threonine Protein Kinase Lacking the Catalytic Lysine in Subdomain II. J. Biol. Chem. 275, 16795–16801. doi:10.1074/jbc.275.22.16795
Xu, B.-e., Min, X., Stippec, S., Lee, B.-H., Goldsmith, E. J., and Cobb, M. H. (2002). Regulation of WNK1 by an Autoinhibitory Domain and Autophosphorylation. J. Biol. Chem. 277, 48456–48462. doi:10.1074/jbc.m207917200
Xu, B.-e., Lee, B.-H., Min, X., Lenertz, L., Heise, C. J., Stippec, S., et al. (2005a). WNK1: Analysis of Protein Kinase Structure, Downstream Targets, and Potential Roles in Hypertension. Cell. Res. 15, 6–10. doi:10.1038/sj.cr.7290256
Xu, B.-e., Stippec, S., Chu, P.-Y., Lazrak, A., Li, X.-J., Lee, B.-H., et al. (2005b). WNK1 Activates SGK1 to Regulate the Epithelial Sodium Channel. Proc. Natl. Acad. Sci. U.S.A. 102, 10315–10320. doi:10.1073/pnas.0504422102
Xu, B.-e., Stippec, S., Lazrak, A., Huang, C.-L., and Cobb, M. H. (2005c). WNK1 Activates SGK1 by a Phosphatidylinositol 3-kinase-dependent and Non-catalytic Mechanism. J. Biol. Chem. 280, 34218–34223. doi:10.1074/jbc.m505735200
Yamada, K., Park, H.-M., Rigel, D. F., Dipetrillo, K., Whalen, E. J., Anisowicz, A., et al. (2016). Small-molecule WNK Inhibition Regulates Cardiovascular and Renal Function. Nat. Chem. Biol. 12, 896–898. doi:10.1038/nchembio.2168
Yan, Y., Dalmasso, G., Nguyen, H. T. T., Obertone, T. S., Charrier-Hisamuddin, L., Sitaraman, S. V., et al. (2008). Nuclear Factor-Κb Is a Critical Mediator of Ste20-like Proline-/Alanine-Rich Kinase Regulation in Intestinal Inflammation. Am. J. Pathology 173, 1013–1028. doi:10.2353/ajpath.2008.080339
Yoshimatsu, Y., and Watabe, T. (2011). Roles of TGF-β Signals in Endothelial-Mesenchymal Transition during Cardiac Fibrosis. Int. J. Inflam. 2011, 724080. doi:10.4061/2011/724080
Zagórska, A., Pozo-Guisado, E., Boudeau, J., Vitari, A. C., Rafiqi, F. H., Thastrup, J., et al. (2007). Regulation of Activity and Localization of the WNK1 Protein Kinase by Hyperosmotic Stress. J. Cell. Biol. 176, 89–100. doi:10.1083/jcb.200605093
Zhang, J., Deng, X., and Kahle, K. T. (2016). Leveraging Unique Structural Characteristics of WNK Kinases to Achieve Therapeutic Inhibition. Sci. Signal 9, e3. doi:10.1126/scisignal.aaj2227
Keywords: WNK1, OSR1, SPAK, TGF-β, EMT
Citation: Jung J-U, Jaykumar AB and Cobb MH (2022) WNK1 in Malignant Behaviors: A Potential Target for Cancer?. Front. Cell Dev. Biol. 10:935318. doi: 10.3389/fcell.2022.935318
Received: 03 May 2022; Accepted: 06 June 2022;
Published: 22 June 2022.
Edited by:
Susan Taylor, University of California, San Diego, United StatesReviewed by:
Sei Kuriyama, Akita University, JapanCopyright © 2022 Jung, Jaykumar and Cobb. This is an open-access article distributed under the terms of the Creative Commons Attribution License (CC BY). The use, distribution or reproduction in other forums is permitted, provided the original author(s) and the copyright owner(s) are credited and that the original publication in this journal is cited, in accordance with accepted academic practice. No use, distribution or reproduction is permitted which does not comply with these terms.
*Correspondence: Melanie H. Cobb, TWVsYW5pZS5Db2JiQFVUU291dGh3ZXN0ZXJuLmVkdQ==
†These authors share first authorship