- 1Department of Cardiology, China-Japan Union Hospital of Jilin University, Changchun, China
- 2Department of Medical Oncology, Jilin Provincial Cancer Hospital, Changchun, China
Cardiac fibrosis results from both the differentiation of cardiac fibroblasts and excessive accumulation of extracellular matrix (ECM), leading to myocardial stiffness and reduced compliance of the ventricular wall. The conversion of cardiac fibroblasts to myofibroblasts is the most important initiating step in the process of this pathological cardiac remodeling. It occurs during the progression of many cardiovascular diseases, adversely influencing both the clinical course and outcome of the disease. The pathogenesis is complex and there is no effective treatment. Exosomes are extracellular vesicles that mediate intercellular communication through delivering specific cargoes of functional nucleic acids and proteins derived from particular cell types. Recent studies have found that exosomes play an important role in the diagnosis and treatment of cardiac fibrosis, and is a potential biotherapeutics and drug delivery vectors for the treatment of cardiac fibrosis. The present review aimed to summarize the current knowledge of exosome-related mechanisms underlying cardiac fibrosis and to suggest potential therapy that could be used to treat the condition.
Introduction
Cardiac fibrosis is a pathological process occurring in almost all cardiovascular disorders and results from fibroblast activation and the deposition of excess extracellular matrix (ECM) (Kong et al., 2014; Ma et al., 2018). Cardiac fibrosis leads to increased stiffness and reduced flexibility of the myocardium with varying degrees of myocardial systolic or diastolic dysfunction, resulting in heart failure and death (Li et al., 2018; Maruyama & Imanaka-Yoshida, 2022). Although numerous pathogenic factors have been identified, the specific mechanisms underlying its development are unclear and require further investigation. Exosomes are small vesicles (30–150 nm in size) secreted by cells that play an essential role in intercellular communication (Zaborowski et al., 2015; Jiang et al., 2021), mediated by a class of proteins and nucleic acids carried within the exosomes that are transported from cell to cell (Zamani et al., 2019). Under disease conditions, fibroblasts, cardiomyocytes, macrophages, lymphocytes, and vascular endothelial cells communicate with each other through exosomes and their cargoes, ultimately leading to myocardial fibrosis (Jiang et al., 2021). In recent years, although exosomes have been reported to be involved in the diagnosis and treatment of a variety of diseases, their role in myocardial fibrosis is less well understood. Therefore, this review summarizes recent research on exosomes in relation to cardiac fibrosis, discussing the possible mechanisms through which exosomes influence the condition and the potential applications of this knowledge in the management of cardiovascular disease.
Structure and Function of Exosomes
Exosomes have a circular single-membrane structure and range between 30 and 150 nm in size. They are formed by a series of regulated processes such as endocytosis-fusion-exocytosis (Raposo & Stoorvogel, 2013; Zaborowski et al., 2015; Nguyen et al., 2016; Jiang et al., 2021). Early endosomes are formed by invagination of the cell membrane and subsequently mature into multivesicular bodies (MVBs) (Raposo & Stoorvogel, 2013; Yáñez-Mó et al., 2015). Exosomes are formed by MVB fusion with the cytoplasmic membrane and exosomal into the extracellular space, with the remaining MVBs recycled by the trans-Golgi network (TGN)or sent to lysosomes for degradation (Simons & Raposo, 2009; Théry et al., 2009; Bebelman et al., 2018; Doyle & Wang, 2019) (Figure 1). Exosomes were initially described in sheep reticulocytes in 1983 (Bobrie et al., 2011; Zhang & Yu, 2019) with the term “exosome” coined by Johnstone in 1987 (Viaud et al., 2008; Zhang & Yu, 2019). All mammalian cells have been found to secrete exosomes, and exosomes are commonly found in various body fluids, comprising cerebrospinal fluid, blood, and saliva (Pisitkun et al., 2004; Caby et al., 2005; Akers et al., 2013; Hornick et al., 2015; Backes et al., 2016; Kim et al., 2020). Although initially exosomes were believed to transport waste material from cells (Hessvik & Llorente, 2018), they have subsequently been shown to play important roles in a variety of biological and pathological processes. Exosomes are not only involved in intercellular material transport and information transfer, regulating cellular physiological activities but are also involved in all aspects of the body’s immune response, antigen presentation, cell migration, cell differentiation, and tumor invasion (Kalluri, 2016; Lindenbergh & Stoorvogel, 2018; Mashouri et al., 2019; Zhang & Yu, 2019; Daassi et al., 2020; Shen et al., 2020; Sung et al., 2021). Exosomes contain a wide variety of cargoes that are responsible for mediating intercellular communication and performing biological functions.
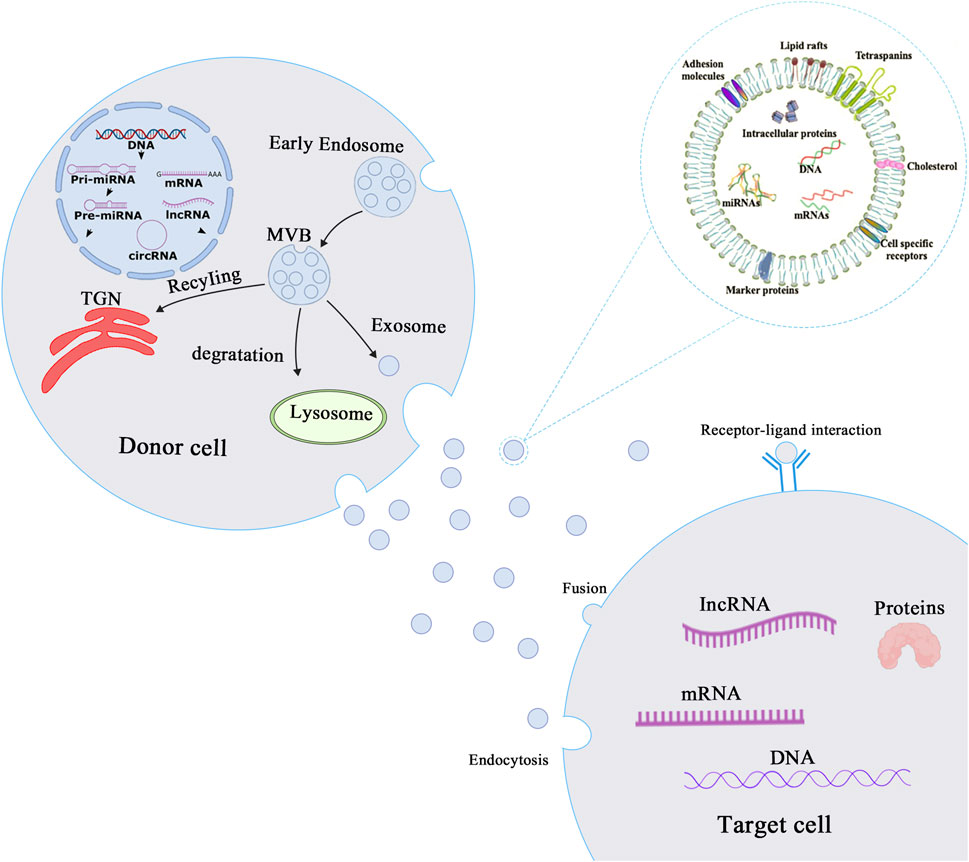
FIGURE 1. Biogenesis and mechanisms of action of exosomes. Biogenesis and mechanism of action of exosomes. The process of exosome production starts with the invagination of the cell membrane to form early endosomes, which bud inward to form intracellular multivesicular bodies (MVBs), and finally the outer membrane of MVB fuses with the cell membrane to release exosomes into the extracellular matrix. Exosomes then play a role in mediating intercellular communication through endocytosis, fusion or receptor-ligand interaction mechanisms.
After uptake by target cells, mRNAs from the exosomal cargo can be translated into proteins, and mRNA expression can be regulated by microRNAs (miRNAs) at the post-transcriptional level (Figure 1) (Thum & Condorelli, 2015; Zhang et al., 2015; Piccoli et al., 2016; Yang et al., 2020). In addition, both exosomal cargoes and membranes contain numerous biologically active proteins, such as Rab proteins, annexins (regulating exosomal membrane exchange and fusion) (Monastyrskaya, 2018), heat shock proteins (HSP60, HSP70, HSP90), tetraspanin transmembrane proteins (CD9, CD63, and CD81), metabolic enzymes (such as GAPDH, enolase, and antioxidant enzymes), and adhesion factors (MFGE8, integrins) (Klinkert & Echard, 2016; Gao et al., 2019). With the advancement of research on exosomes and the application of advanced experimental techniques, exosomes are expected to become early diagnostic markers for several diseases and to be used as carriers of genes and drugs.
Pathogenesis of Cardiac Fibrosis
Cardiac fibrosis is well-defined through remodeling of the myocardial interstitium, with enhanced numbers of myofibroblasts and collagen accumulation. This leads to abnormalities in cardiac function and metabolism and is commonly seen at the end stages of several cardiovascular diseases (Pathak et al., 2005; Frangogiannis, 2019, 2021). Cardiac fibrosis results in myocardial stiffness and reduced compliance of the ventricular wall, which severely affects the systolic and diastolic functions of the heart (Porter & Turner, 2009; Park et al., 2019). Cardiac fibrosis is usually attributed to one of two types: acute (or reparative) and chronic (or reactive) fibrosis. Since the adult mammalian heart has limited regenerating ability, the death of a significant proportion of cardiomyocytes causes reparative fibrosis. Dead myocardium activates the reparative process and is replaced by collagen-based scar after an acute myocardial infarction, which is necessary to safeguard the structural integrity of the ventricles and prevent cardiac rupture (Talman & Ruskoaho, 2016). In the absence of infarction, pathophysiologic factors such as pressure overload, volume overload, metabolic dysfunction, and aging can facilitate interstitial and perivascular fibrosis, and these changes are known as reactive fibrosis (Frangogiannis, 2019). Left ventricular pressure overload typically occurs as a result of systemic hypertension or aortic valve dysfunction, resulting in progressive interstitial and perivascular reactive fibrosis of the myocardium with a substantial proportion of noncollagenous matrix. Aging and abnormalities of glycolipid metabolism can also cause an interstitial myocardial fibrotic response, which is characterized by increased cardiac stiffness while the ejection fraction remains unaltered (Cavalera et al., 2014; Nattel, 2017; Bai et al., 2019). Cardiac fibroblasts are the major matrix-producing cells and represent one of the largest cell populations in normal mammalian hearts. They are closely involved in the maintenance of normal cardiac function as well as cardiac remodeling in pathological states (Camelliti et al., 2005; Tallquist, 2020). Fibroblasts in the fibrotic heart are a highly heterogeneous population of cells, and this heterogeneity is caused by their different origins. Resident fibroblasts are by far the most important source of myofibroblasts. In addition, myofibroblasts are also differentiated from endothelial cells, bone marrow-derived mesenchymal stem cells, and inflammatory cells (Figure 2) (Li et al., 2018). Although the conversion of cardiac fibroblasts into myofibroblasts is the most important initial step in all myocardial fibrosis, different etiologies trigger different molecular patterns of fibroblast activation that affect the pathological outcome of cardiac fibrosis.
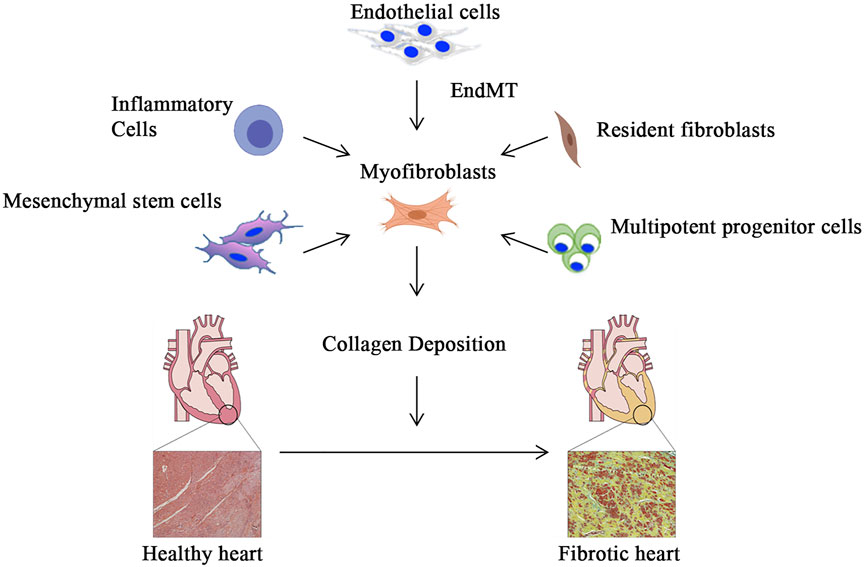
FIGURE 2. Myofibroblast activation and myocardial fibrosis. In addition to differentiation from resident fibroblasts, myofibroblasts can be derived from inflammatory cells, bone marrow-derived mesenchymal stem cells and pluripotent progenitor cells, and endothelial cells that undergo endothelial-mesenchymal transition. Subsequently, activated myofibroblasts secrete extracellular matrix, which gradually leads to the development of myocardial fibrosis.
The pathogenesis of myocardial fibrosis is complex and remains unclear. Fibrogenic signaling cascades are triggered by fibrogenic growth factors (including PDGFs and TGF-β), cytokines (such as TNFα, IL-1, IL-6, and IL-10), and neurohumoral pathway components that bind to cell surface receptors, activating downstream signaling cascades (Kong et al., 2014). Current research has revealed a close connection between the well-studied TGF-β/Smad signaling pathway and cardiac fibrosis that affects both the secretion and degradation of ECM components and myofibroblast differentiation (Khalil et al., 2017). The involvement of the innate immune system in the regulation of heart functioning and remodeling is well-documented (Baci et al., 2020). NLRP3 inflammasome activation and the levels of pro-inflammatory cytokine, comprising those of TNF-α, IL-1, and IL-6, have been found to be significantly elevated in several pathological cardiac conditions linked with fibrosis (Bracey et al., 2015; Siamwala et al., 2020). Louwe et al. demonstrated that transplantation of Nlrp3−/− bone marrow in mice after myocardial infarction attenuated cardiac remodeling compared with wild-type bone marrow, suggesting that the absence of the NLRP3 inflammasome in hematopoietic cells reduces adverse remodeling (Louwe et al., 2020). Members of the endothelin family of peptides are important regulators of cardiovascular function as well as the maintenance of basal vascular tone and cardiovascular system homeostasis (Barton & Yanagisawa, 2019; Miyauchi & Sakai, 2019). Recent studies have shown that ET-1 promotes fibroblast activation and collagen production and is an effective mediator of pro-fibrosis (Phosri et al., 2017; Zhang et al., 2021).
Exosomes in Cardiac Fibrosis
There is mounting evidence of the involvement of exosomal intercellular communication in cardiac fibrosis (Qin et al., 2020; Jiang et al., 2021). Exosomes generated by different cells under the same disease condition or by the same cells under different disease conditions have positive or negative effects on fibroblast activation and cardiac fibrosis, a reflection of exosomal heterogeneity (Dutka et al., 2019; Hohn et al., 2021). Exosomal cargoes include proteins, lipids, mRNAs, miRNAs, and lncRNAs that are involved in paracrine signaling, underlying their significance in the regulation of cardiac fibrosis (Ranjan et al., 2019; Wei et al., 2021).
MiRNAs are a highly conserved subset of non-coding RNAs with lengths between 18 and 22 nucleotides. miRNAs interact with the 3’ UTR regions of their target mRNAs to modulate the expression of the latter (Bartel, 2004). (Yang et al., 2019)discovered upregulation of the expression of miR-208a in cardiomyocytes of rats after myocardial infarction; the miRNA was delivered to the fibroblasts by exosomes, leading to fibroblast proliferation and cardiac fibrosis (Yang et al., 2018). Furthermore, the application of an miR-208a antagomir was able to reverse this pro-fibrotic effect and ameliorate cardiac functions in post-myocardial-infarction rats. Previous investigations have demonstrated that the levels of miR-217 expression are enhanced in the hearts of cases suffering from chronic heart failure (Li et al., 2016). However, the role of miR-217 in myocardial fibrosis is unclear. Recently, Nie et al. (2018) found that cardiomyocyte-derived miR-217 was delivered by exosomes to act through a paracrine mechanism in fibroblasts, exacerbating pressure overload-induced myocardial fibrosis and cardiac dysfunction. In rat and mouse models of ischemic heart failure, miR-30d expression is elevated in cardiomyocytes and targets integrin α5 in fibroblasts via a paracrine mechanism, thus inhibiting fibroblast proliferation and fibrosis (Li et al., 2021). Song et al. found that direct injection of miR-21-rich exosomes into the myocardial infarct area of mice reduced scar formation in the infarct area and significantly improved cardiac function at 4 weeks (Song et al., 2019). Qiao et al. (2019) found that the impaired ability of exosomes derived from cardiac stromal cells of heart failure patients to promote endothelial tube formation and cardiomyocyte proliferation was attributed to dysregulated miR-21-5p expression. In contrast, stem cell-derived exosomes enriched in miR-21-5p improved cardiomyocyte function and attenuated myocardial fibrosis (Qiao et al., 2019). Moreover, Kang et al. found that miR-21 within human peripheral blood derived-exosomes increased cardiac fibrosis (Kang et al., 2019). Furthermore, miR-155, which is abundant in macrophage-derived exosomes, can aggravate cardiac fibrosis and inflammation by promoting the abnormal proliferation and differentiation of fibroblasts (Wang et al., 2017). The presence of increased numbers of CD4+ T cells in the heart is correlated with pathological cardiac remodeling and dysfunction (Savvatis et al., 2014). Cai et al. (2020) observed that exosomes originating from activated CD4+T cells accelerated post-ischemic cardiac fibrosis through the activation of myofibroblasts by exosome-derived miR-142-3p-WNT signaling. Recent literature suggests that exosomes are also able to modulate fibroblast function and reverse the pathological changes of myocardial fibrosis (Zhang et al., 2016; Yang et al., 2017). Exosomes produced by miR-126-overexpressing adipose-derived stem cells (ADSCs) were found to alleviate acute myocardial ischemic injury through the improvement of cardiac inflammation and fibrosis, suggesting that this could be a possible target for treating cardiac fibrosis (Luo et al., 2017). In recent years, several miRs (Table 1) that modulate fibroblast proliferation, differentiation, and cardiac fibrosis have been identified, and targeting these miRs in the exosome may help to diminish fibrosis and ameliorate cardiac functions.
Exosomal proteins have been shown to play a role in myocardial fibrosis regulation. Heat shock protein 90 (HSP90) in myocyte-derived exosomes was found to trigger STAT-3 signaling in cardiac fibroblasts, leading to excessive collagen synthesis and cardiac dysfunction during cardiac hypertrophy (Datta et al., 2017). Diabetic cardiomyocytes are more likely than their normal counterparts to release potentially damaging exosomes containing low amounts of HSP20. A study by Wang et al. (2016) reported that raised levels of HSP20 in exosomes in an HSP20-overexpressing transgenic mouse model significantly alleviated cardiac dysfunction and fibrosis resulting from streptozotocin treatment. Exosome-derived HSP70 levels are negatively correlated with aging, and downregulation of exosomal surface HSP70 levels may contribute to cardiac fibrosis (Yang et al., 2018). The signaling pathway of WNT performs a significant task in the development of cardiac fibrosis. Exosomes comprising WNT proteins are able to activate fibrosis-promoting WNT pathways in cardiac fibroblasts, suggesting a new strategy for the dissemination of fibrosis-promoting signals throughout the heart (Działo et al., 2019).
Exosomes in the Diagnosis of Cardiac Fibrosis
Cardiac fibrosis results in structural and compliance alterations in the heart, ultimately leading to heart dysfunction and death (Kong et al., 2014; Sohns & Marrouche, 2020). Early identification and diagnosis of cardiac fibrosis are necessary to improve disease management and the prognosis and survival of patients. The diagnosis and classification of cardiac fibrosis rely largely on invasive tests, such as tissue biopsies, which are difficult to perform and put patients at risk, making the diagnosis of cardiac fibrosis very difficult. Fortunately, recent investigations have illustrated the significance of exosomal cargoes, especially miRNAs, for cardiac fibrosis and these can be used to diagnose myocardial fibrosis (Olson, 2014; Saadatpour et al., 2016; Poller et al., 2018). Exosomes represent novel biomarkers carrying disease-specific cargoes, are simple to obtain, and are stable in body fluids (Wang C. et al, 2021). Exosome-derived miRNAs should theoretically be better biomarkers than circulating miRNAs in plasma/serum as may be isolated from specific cell types, ensuring sensitivity and specificity (Jansen et al., 2014; Raju et al., 2020). A study by Wang et al. (2018a) reported that circulating exosomal miRNA-425 and miRNA-744 function as novel biomarkers that can predict cardiac fibrosis progression and heart dysfunction in patients with heart failure. Liu et al. (2019) found that miR-92a-3p within endothelial cell-derived exosomes was linked with atherogenesis (Liu et al., 2019) while Li et al. (2021) demonstrated that reduced expression of miR-30d in heart-derived exosomes was strongly related to deleterious cardiac remodeling and the expression of fibrosis- and inflammation-related genes in both rodent models and human subjects (Li et al., 2021). Jansen found that miR-126 and miR-199a in exosomes were found to be associated with cardiovascular events in stable coronary artery heart disease, rather than free miR-126 and miR-199a in plasma (Jansen et al., 2014). The increased levels of miR-1, and miR-133a were associated with acute MI, unstable angina pectoris, or Takotsubo cardiomyopathy (Barile et al., 2017). However, extensive studies in large groups of human patients are required before any final conclusions can be reached.
Additionally, exosomal proteins may also be associated with cardiac fibrosis. Overexpression of HIF-1α in exosomes from mesenchymal stem cells reduced cardiac fibrosis through the promotion of neovessel formation in rats after myocardial infarction (Sun et al., 2020). (Yang et al., 2019) found that HSP70 on the surface of serum exosomes was negatively associated with aging and was implicated in cardiac fibrosis, suggesting that it may be a promising diagnostic target for aging-related myocardial fibrosis (Yang et al., 2018). Therefore, exosomes are potentially effective diagnostic markers for the diagnosis and etiological classification of the early stages of cardiac fibrosis.
Exosomes as Targets for the Treatment of Cardiac Fibrosis
Exosomes have been recently investigated as potential biotherapeutics and drug delivery vectors for the treatment of cardiac fibrosis. Many reports have suggested that exosomes can function as therapeutic agents and drug delivery systems (El-Andaloussi et al., 2012; Arrighetti et al., 2019; Shao J. et al, 2020; Liang et al., 2021). As exosomes carry specific cargoes of functional nucleic acids and proteins derived from particular cell types, it is likely that they may be used as therapeutic drugs. Milano et al. found that miR-146a-5 is significantly enriched in exosomes derived from resident cardiac mesenchymal progenitor cells, and intravenous administration of these exosomes could attenuate doxorubicin/trastuzumab-induced cardiotoxicity and alleviate cardiac fibrosis (Milano et al., 2020). Exosomes from cardiosphere-derived cells enriched with miR-92a attenuated myocardial fibrosis and improved survival in mice with myocardial infarction (Ibrahim et al., 2019). Previous studies have identified that miR-26a regulates extracellular matrix production and overexpression of miR-26a can inhibits cardiac fibrosis caused by diseases such as myocardial infarction and chronic kidney disease (CKD) (Wei et al., 2013; Wang et al., 2019; Chiang et al., 2020). Wang et al. (2019) engineered an exosome vector containing Lamp2b (an exosomal membrane protein gene fused with a muscle-specific surface peptide that targets muscle delivery) and transfected it into muscle satellite cells overexpressing miR-26a to generate miR-26a-enriched exosomes, which were subsequently injected into mouse tibialis anterior muscle. The results showed that miR-26a inhibited myocardial fibrotic lesions by reducing ECM production through inhibition of Fox01, which provides a possible therapeutic strategy for the use of exosome delivery of miR-26a for the treatment of CKD complications. In addition, Yao et al. (2021) advanced a minimally invasive exosome spray (EXOS) on the basis of the mesenchymal stem cell exosomes and biomaterials that can be used to reduce cardiac fibrosis in a mouse model of acute myocardial infarction.
Furthermore, exosomes can also act as drug carriers, thus enabling more efficient delivery of drugs to target cells. The natural compound curcumin has both anti-inflammatory and anti-fibrotic properties (He et al., 2015; Yu et al., 2019; Zhao et al., 2021). Sun et al. used exosomes for the targeted delivery of curcumin to inflammatory cells, significantly reducing inflammatory factor levels (Sun et al., 2010). In addition, they also demonstrated that curcumin delivered by exosomes had higher concentrations in the blood. With the improvement of technological tools, molecular cloning and lentiviral packaging techniques have been applied to the treatment of myocardial fibrosis. Wang et al. (2018b) designed engineered exosomes containing an ischemic myocardium-targeting peptide, which significantly reduced inflammation and fibrosis in the ischemic heart region in a mouse model of myocardial infarction. Plant-derived exosome-like nanoparticles (ELNs) have been shown to also prevent and treat a variety of diseases, with functions closely related to the plant of origin (Teng et al., 2018; Cong et al., 2022). ELNs have lipid membrane structures that can also be used as natural or engineered carriers for drug loading and delivery (Karamanidou & Tsouknidas, 2021). Moreover, ELNs are cost-effective and easy to obtain, and have great potential for development and application. In brief, the above studies provide robust evidence for the use of exosomes as antifibrotic therapy.
Conclusion
Cardiac fibrosis is a hallmark of cardiac remodeling and a cause of clinical disease. The search for ways to slow, stop, or even reverse the progression of myocardial fibrosis has generated widespread interest. Cardiac fibrosis occurs frequently in the course of several cardiovascular diseases and can result in heart failure and death. Over the past few years, many extensive and in-depth studies of exosomes have provided important new insights that have led to a clearer understanding of both exosomal structures and actions. There is mounting evidence that exosomes play significant roles in cardiac fibrosis, and they can thus be useful as biomarkers and drug delivery vehicles for the diagnosis and treatment, respectively, of cardiac fibrosis. Although no exosome-based treatment for cardiac fibrosis has been clinically realized at this stage, the application of exosomes has already displayed a strong therapeutic potential, and thus further research is needed in the future. In addition, several unanswered questions remain to be addressed, such as 1) How to efficiently get samples with the required cargo? 2) What factors influence exosome biogenesis in cardiac fibrosis? 3) What are the long-term effects and possible concomitant adverse effects of exosome therapy for cardiac fibrosis?
Author Contributions
JF wrote the article and generated the figures. MR revised the article. YH designed the conception and figures. All the authors read and approved the submitted version.
Conflict of Interest
The authors declare that the research was conducted in the absence of any commercial or financial relationships that could be construed as a potential conflict of interest.
Publisher’s Note
All claims expressed in this article are solely those of the authors and do not necessarily represent those of their affiliated organizations, or those of the publisher, the editors and the reviewers. Any product that may be evaluated in this article, or claim that may be made by its manufacturer, is not guaranteed or endorsed by the publisher.
References
Akers, J. C., Ramakrishnan, V., Kim, R., Skog, J., Nakano, I., Pingle, S., et al. (2013). MiR-21 in the Extracellular Vesicles (EVs) of Cerebrospinal Fluid (CSF): a Platform for Glioblastoma Biomarker Development. PLoS One 8 (10), e78115. doi:10.1371/journal.pone.0078115
Arrighetti, N., Corbo, C., Evangelopoulos, M., Pastò, A., Zuco, V., and Tasciotti, E. (2019). Exosome-like Nanovectors for Drug Delivery in Cancer. Cmc 26 (33), 6132–6148. doi:10.2174/0929867325666180831150259
Baci, D., Bosi, A., Parisi, L., Buono, G., Mortara, L., Ambrosio, G., et al. (2020). Innate Immunity Effector Cells as Inflammatory Drivers of Cardiac Fibrosis. Int. J. Mol. Sci. 21 (19), 7165. doi:10.3390/ijms21197165
Backes, C., Meese, E., and Keller, A. (2016). Specific miRNA Disease Biomarkers in Blood, Serum and Plasma: Challenges and Prospects. Mol. Diagn Ther. 20 (6), 509–518. doi:10.1007/s40291-016-0221-4
Bai, B., Yang, W., Fu, Y., Foon, H. L., Tay, W. T., Yang, K., et al. (2019). Seipin Knockout Mice Develop Heart Failure With Preserved Ejection Fraction. JACC Basic Transl. Sci. 4 (8), 924–937. doi:10.1016/j.jacbts.2019.07.008
Barile, L., Moccetti, T., Marbán, E., and Vassalli, G. (2017). Roles of Exosomes in Cardioprotection. Eur. Heart J. 38 (18), 1372–1379. doi:10.1093/eurheartj/ehw304
Barton, M., and Yanagisawa, M. (2019). Endothelin: 30 Years From Discovery to Therapy. Hypertension 74 (6), 1232–1265. doi:10.1161/hypertensionaha.119.12105
Bebelman, M. P., Smit, M. J., Pegtel, D. M., and Baglio, S. R. (2018). Biogenesis and Function of Extracellular Vesicles in Cancer. Pharmacol. Ther. 188, 1–11. doi:10.1016/j.pharmthera.2018.02.013
Bier, A., Berenstein, P., Kronfeld, N., Morgoulis, D., Ziv-Av, A., Goldstein, H., et al. (2018). Placenta-derived Mesenchymal Stromal Cells and Their Exosomes Exert Therapeutic Effects in Duchenne Muscular Dystrophy. Biomaterials 174, 67–78. doi:10.1016/j.biomaterials.2018.04.055
Bobrie, A., Colombo, M., Raposo, G., and Théry, C. (2011). Exosome Secretion: Molecular Mechanisms and Roles in Immune Responses. Traffic 12 (12), 1659–1668. doi:10.1111/j.1600-0854.2011.01225.x
Bracey, N. A., Duff, H. J., and Muruve, D. A. (2015). Hierarchical Regulation of Wound Healing by NOD-like Receptors in Cardiovascular Disease. Antioxidants Redox Signal. 22 (13), 1176–1187. doi:10.1089/ars.2014.6125
Caby, M.-P., Lankar, D., Vincendeau-Scherrer, C., Raposo, G., and Bonnerot, C. (2005). Exosomal-like Vesicles Are Present in Human Blood Plasma. Int. Immunol. 17 (7), 879–887. doi:10.1093/intimm/dxh267
Cai, L., Chao, G., Li, W., Zhu, J., Li, F., Qi, B., et al. (2020). Activated CD4+ T Cells-Derived Exosomal miR-142-3p Boosts Post-ischemic Ventricular Remodeling by Activating Myofibroblast. Aging 12 (8), 7380–7396. doi:10.18632/aging.103084
Camelliti, P., Borg, T., and Kohl, P. (2005). Structural and Functional Characterisation of Cardiac Fibroblasts. Cardiovasc. Res. 65 (1), 40–51. doi:10.1016/j.cardiores.2004.08.020
Cavalera, M., Wang, J., and Frangogiannis, N. G. (2014). Obesity, Metabolic Dysfunction, and Cardiac Fibrosis: Pathophysiological Pathways, Molecular Mechanisms, and Therapeutic Opportunities. Transl. Res. 164 (4), 323–335. doi:10.1016/j.trsl.2014.05.001
Chaturvedi, P., Kalani, A., Medina, I., Familtseva, A., and Tyagi, S. C. (2015). Cardiosome Mediated Regulation of MMP9 in Diabetic Heart: Role of Mir29b and Mir455 in Exercise. J. Cell. Mol. Med. 19 (9), 2153–2161. doi:10.1111/jcmm.12589
Chiang, M. H., Liang, C. J., Lin, L. C., Yang, Y. F., Huang, C. C., Chen, Y. H., et al. (2020). miR‐26a Attenuates Cardiac Apoptosis and Fibrosis by Targeting Ataxia-Telangiectasia Mutated in Myocardial Infarction. J. Cell Physiol. 235 (9), 6085–6102. doi:10.1002/jcp.29537
Cong, M., Tan, S., Li, S., Gao, L., Huang, L., Zhang, H.-G., et al. (2022). Technology Insight: Plant-Derived Vesicles-How Far from the Clinical Biotherapeutics and Therapeutic Drug Carriers? Adv. Drug Deliv. Rev. 182, 114108. doi:10.1016/j.addr.2021.114108
Daassi, D., Mahoney, K. M., and Freeman, G. J. (2020). The Importance of Exosomal PDL1 in Tumour Immune Evasion. Nat. Rev. Immunol. 20 (4), 209–215. doi:10.1038/s41577-019-0264-y
Datta, R., Bansal, T., Rana, S., Datta, K., Datta Chaudhuri, R., Chawla-Sarkar, M., et al. (2017). Myocyte-Derived Hsp90 Modulates Collagen Upregulation via Biphasic Activation of STAT-3 in Fibroblasts during Cardiac Hypertrophy. Mol. Cell Biol. 37 (6), e00611–16. doi:10.1128/MCB.00611-16
Dong, J., Zhu, W., and Wan, D. (2021). Downregulation of microRNA-21-5p from Macrophages-Derived Exosomes Represses Ventricular Remodeling after Myocardial Infarction via Inhibiting Tissue Inhibitors of Metalloproteinase 3. Int. Immunopharmacol. 96, 107611. doi:10.1016/j.intimp.2021.107611
Doyle, L. M., and Wang, M. Z. (2019). Overview of Extracellular Vesicles, Their Origin, Composition, Purpose, and Methods for Exosome Isolation and Analysis. Cells 8 (7), 727. doi:10.3390/cells8070727
Dutka, M., Bobiński, R., and Korbecki, J. (2019). The Relevance of microRNA in Post-infarction Left Ventricular Remodelling and Heart Failure. Heart Fail Rev. 24 (4), 575–586. doi:10.1007/s10741-019-09770-9
Działo, E., Rudnik, M., Koning, R. I., Czepiel, M., Tkacz, K., Baj-Krzyworzeka, M., et al. (2019). WNT3a and WNT5a Transported by Exosomes Activate WNT Signaling Pathways in Human Cardiac Fibroblasts. Int. J. Mol. Sci. 20 (6), 1436. doi:10.3390/ijms20061436
El-Andaloussi, S., Lee, Y., Lakhal-Littleton, S., Li, J., Seow, Y., Gardiner, C., et al. (2012). Exosome-mediated Delivery of siRNA In Vitro and In Vivo. Nat. Protoc. 7 (12), 2112–2126. doi:10.1038/nprot.2012.131
Feng, Y., Huang, W., Wani, M., Yu, X., and Ashraf, M. (2014). Ischemic Preconditioning Potentiates the Protective Effect of Stem Cells through Secretion of Exosomes by Targeting Mecp2 via miR-22. PLoS One 9 (2), e88685. doi:10.1371/journal.pone.0088685
Frangogiannis, N. G. (2021). Cardiac Fibrosis. Cardiovasc Res. 117 (6), 1450–1488. doi:10.1093/cvr/cvaa324
Frangogiannis, N. G. (2019). Cardiac Fibrosis: Cell Biological Mechanisms, Molecular Pathways and Therapeutic Opportunities. Mol. Aspects Med. 65, 70–99. doi:10.1016/j.mam.2018.07.001
Gao, X.-F., Wang, Z.-M., Wang, F., Gu, Y., Zhang, J.-J., and Chen, S.-L. (2019). Exosomes in Coronary Artery Disease. Int. J. Biol. Sci. 15 (11), 2461–2470. doi:10.7150/ijbs.36427
Gollmann-Tepeköylü, C., Pölzl, L., Graber, M., Hirsch, J., Nägele, F., Lobenwein, D., et al. (2020). miR-19a-3p Containing Exosomes Improve Function of Ischaemic Myocardium upon Shock Wave Therapy. Cardiovasc Res. 116 (6), 1226–1236.
He, Y., Yue, Y., Zheng, X., Zhang, K., Chen, S., and Du, Z. (2015). Curcumin, Inflammation, and Chronic Diseases: How Are They Linked? Molecules 20 (5), 9183–9213. doi:10.3390/molecules20059183
Hessvik, N. P., and Llorente, A. (2018). Current Knowledge on Exosome Biogenesis and Release. Cell. Mol. Life Sci. 75 (2), 193–208. doi:10.1007/s00018-017-2595-9
Hohn, J., Tan, W., Carver, A., Barrett, H., and Carver, W. (2021). Roles of Exosomes in Cardiac Fibroblast Activation and Fibrosis. Cells 10 (11), 2933. doi:10.3390/cells10112933
Hornick, N. I., Huan, J., Doron, B., Goloviznina, N. A., Lapidus, J., Chang, B. H., et al. (2015). Serum Exosome MicroRNA as a Minimally-Invasive Early Biomarker of AML. Sci. Rep. 5, 11295. doi:10.1038/srep11295
Huang, Y., Chen, L., Feng, Z., Chen, W., Yan, S., Yang, R., et al. (2021). EPC-Derived Exosomal miR-1246 and miR-1290 Regulate Phenotypic Changes of Fibroblasts to Endothelial Cells to Exert Protective Effects on Myocardial Infarction by Targeting ELF5 and SP1. Front. Cell Dev. Biol. 9, 647763. doi:10.3389/fcell.2021.647763
Ibrahim, A. G. E., Li, C., Rogers, R., Fournier, M., Li, L., Vaturi, S. D., et al. (2019). Augmenting Canonical Wnt Signalling in Therapeutically Inert Cells Converts Them into Therapeutically Potent Exosome Factories. Nat. Biomed. Eng. 3 (9), 695–705. doi:10.1038/s41551-019-0448-6
Izarra, A., Moscoso, I., Levent, E., Cañón, S., Cerrada, I., Díez-Juan, A., et al. (2014). miR-133a Enhances the Protective Capacity of Cardiac Progenitors Cells after Myocardial Infarction. Stem Cell Rep. 3 (6), 1029–1042. doi:10.1016/j.stemcr.2014.10.010
Jansen, F., Yang, X., Proebsting, S., Hoelscher, M., Przybilla, D., Baumann, K., et al. (2014). MicroRNA Expression in Circulating Microvesicles Predicts Cardiovascular Events in Patients with Coronary Artery Disease. J. Am. Heart Assoc. 3 (6), e001249. doi:10.1161/JAHA.114.001249
Jiang, W., Xiong, Y., Li, X., and Yang, Y. (2021). Cardiac Fibrosis: Cellular Effectors, Molecular Pathways, and Exosomal Roles. Front. Cardiovasc. Med. 8, 715258. doi:10.3389/fcvm.2021.715258
Kalluri, R. (2016). The Biology and Function of Exosomes in Cancer. J. Clin. Invest 126 (4), 1208–1215. doi:10.1172/jci81135
Kang, J. Y., Park, H., Kim, H., Mun, D., Park, H., Yun, N., et al. (2019). [Corrigendum] Human Peripheral Blood-derived E-xosomes for microRNA D-elivery. Int. J. Mol. Med. 44 (6), 358–2328. doi:10.3892/ijmm.2019.4202
Karamanidou, T., and Tsouknidas, A. (2021). Plant-Derived Extracellular Vesicles as Therapeutic Nanocarriers. Int. J. Mol. Sci. 23 (1), 191. doi:10.3390/ijms23010191
Ke, X., Yang, R., Wu, F., Wang, X., Liang, J., Hu, X., et al. (2021). Exosomal miR-218-5p/miR-363-3p from Endothelial Progenitor Cells Ameliorate Myocardial Infarction by Targeting the P53/JMY Signaling Pathway. Oxid. Med. Cell Longev. 2021, 5529430. doi:10.1155/2021/5529430
Khalil, H., Kanisicak, O., Prasad, V., Correll, R. N., Fu, X., Schips, T., et al. (2017). Fibroblast-specific TGF-β-Smad2/3 Signaling Underlies Cardiac Fibrosis. J. Clin. Invest 127 (10), 3770–3783. doi:10.1172/jci94753
Khan, M., Nickoloff, E., Abramova, T., Johnson, J., Verma, S. K., Krishnamurthy, P., et al. (2015). Embryonic Stem Cell-Derived Exosomes Promote Endogenous Repair Mechanisms and Enhance Cardiac Function Following Myocardial Infarction. Circ. Res. 117 (1), 52–64. doi:10.1161/circresaha.117.305990
Kim, K. U., Kim, W. H., Jeong, C. H., Yi, D. Y., and Min, H. (2020). More Than Nutrition: Therapeutic Potential of Breast Milk-Derived Exosomes in Cancer. Int. J. Mol. Sci. 21 (19), 7327. doi:10.3390/ijms21197327
Klinkert, K., and Echard, A. (2016). Rab35 GTPase: A Central Regulator of Phosphoinositides and F-Actin in Endocytic Recycling and Beyond. Traffic 17 (10), 1063–1077. doi:10.1111/tra.12422
Kong, P., Christia, P., and Frangogiannis, N. G. (2014). The Pathogenesis of Cardiac Fibrosis. Cell. Mol. Life Sci. 71 (4), 549–574. doi:10.1007/s00018-013-1349-6
Li, H., Fan, J., Yin, Z., Wang, F., Chen, C., and Wang, D. W. (2016). Identification of Cardiac-Related Circulating microRNA Profile in Human Chronic Heart Failure. Oncotarget 7 (1), 33–45. doi:10.18632/oncotarget.6631
Li, J., Salvador, A. M., Li, G., Valkov, N., Ziegler, O., Yeri, A., et al. (2021). Mir-30d Regulates Cardiac Remodeling by Intracellular and Paracrine Signaling. Circ. Res. 128 (1), e1–e23. doi:10.1161/CIRCRESAHA.120.317244
Li, L., Zhao, Q., and Kong, W. (2018). Extracellular Matrix Remodeling and Cardiac Fibrosis. Matrix Biol. 68-69, 490–506. doi:10.1016/j.matbio.2018.01.013
Liang, Y., Duan, L., Lu, J., and Xia, J. (2021). Engineering Exosomes for Targeted Drug Delivery. Theranostics 11 (7), 3183–3195. doi:10.7150/thno.52570
Liao, Z., Chen, Y., Duan, C., Zhu, K., Huang, R., Zhao, H., et al. (2021). Cardiac Telocytes Inhibit Cardiac Microvascular Endothelial Cell Apoptosis through Exosomal miRNA-21-5p-Targeted Cdip1 Silencing to Improve Angiogenesis Following Myocardial Infarction. Theranostics 11 (1), 268–291. doi:10.7150/thno.47021
Lindenbergh, M. F. S., and Stoorvogel, W. (2018). Antigen Presentation by Extracellular Vesicles from Professional Antigen-Presenting Cells. Annu. Rev. Immunol. 36, 435–459. doi:10.1146/annurev-immunol-041015-055700
Liu, W., Zhang, H., Mai, J., Chen, Z., Huang, T., Wang, S., et al. (2018). Distinct Anti-Fibrotic Effects of Exosomes Derived from Endothelial Colony-Forming Cells Cultured Under Normoxia and Hypoxia. Med. Sci. Monit. 24, 6187–6199. doi:10.12659/msm.911306
Liu, Y., Li, Q., Hosen, M. R., Zietzer, A., Flender, A., Levermann, P., et al. (2019). Atherosclerotic Conditions Promote the Packaging of Functional MicroRNA-92a-3p into Endothelial Microvesicles. Circ. Res. 124 (4), 575–587. doi:10.1161/circresaha.118.314010
Louwe, M. C., Olsen, M. B., Kaasbøll, O. J., Yang, K., Fosshaug, L. E., Alfsnes, K., et al. (2020). Absence of NLRP3 Inflammasome in Hematopoietic Cells Reduces Adverse Remodeling After Experimental Myocardial Infarction. JACC Basic Transl. Sci. 5 (12), 1210–1224. doi:10.1016/j.jacbts.2020.09.013
Luo, Q., Guo, D., Liu, G., Chen, G., Hang, M., and Jin, M. (2017). Exosomes from MiR-126-Overexpressing Adscs Are Therapeutic in Relieving Acute Myocardial Ischaemic Injury. Cell Physiol. Biochem. 44 (6), 2105–2116. doi:10.1159/000485949
Ma, Z.-G., Yuan, Y.-P., Wu, H.-M., Zhang, X., and Tang, Q.-Z. (2018). Cardiac Fibrosis: New Insights into the Pathogenesis. Int. J. Biol. Sci. 14 (12), 1645–1657. doi:10.7150/ijbs.28103
Maruyama, K., and Imanaka-Yoshida, K. (2022). The Pathogenesis of Cardiac Fibrosis: A Review of Recent Progress. Int. J. Mol. Sci. 23 (5). doi:10.3390/ijms23052617
Mashouri, L., Yousefi, H., Aref, A. R., Ahadi, A. M., Molaei, F., and Alahari, S. K. (2019). Exosomes: Composition, Biogenesis, and Mechanisms in Cancer Metastasis and Drug Resistance. Mol. Cancer 18 (1), 75. doi:10.1186/s12943-019-0991-5
Milano, G., Biemmi, V., Lazzarini, E., Balbi, C., Ciullo, A., Bolis, S., et al. (2020). Intravenous Administration of Cardiac Progenitor Cell-Derived Exosomes Protects against Doxorubicin/trastuzumab-Induced Cardiac Toxicity. Cardiovasc Res. 116 (2), 383–392. doi:10.1093/cvr/cvz108
Miyauchi, T., and Sakai, S. (2019). Endothelin and the Heart in Health and Diseases. Peptides 111, 77–88. doi:10.1016/j.peptides.2018.10.002
Monastyrskaya, K. (2018). Functional Association between Regulatory RNAs and the Annexins. Int. J. Mol. Sci. 19 (2), 591. doi:10.3390/ijms19020591
Nattel, S. (2017). Molecular and Cellular Mechanisms of Atrial Fibrosis in Atrial Fibrillation. JACC Clin. Electrophysiol. 3 (5), 425–435. doi:10.1016/j.jacep.2017.03.002
Nguyen, H. P. T., Simpson, R. J., Salamonsen, L. A., and Greening, D. W. (2016). Extracellular Vesicles in the Intrauterine Environment: Challenges and Potential Functions. Biol. Reproduction 95 (5), 109. doi:10.1095/biolreprod.116.143503
Nie, X., Fan, J., Li, H., Yin, Z., Zhao, Y., Dai, B., et al. (2018). miR-217 Promotes Cardiac Hypertrophy and Dysfunction by Targeting PTEN. Mol. Ther. - Nucleic Acids 12, 254–266. doi:10.1016/j.omtn.2018.05.013
Olson, E. N. (2014). MicroRNAs as Therapeutic Targets and Biomarkers of Cardiovascular Disease. Sci. Transl. Med. 6 (239), 239ps3. doi:10.1126/scitranslmed.3009008
Pan, J., Alimujiang, M., Chen, Q., Shi, H., and Luo, X. (2019). Exosomes Derived from miR‐146a‐modified Adipose‐derived Stem Cells Attenuate Acute Myocardial Infarction−induced Myocardial Damage via Downregulation of Early Growth Response Factor 1. J Cell. Biochem. 120 (3), 4433–4443. doi:10.1002/jcb.27731
Park, S., Nguyen, N. B., Pezhouman, A., and Ardehali, R. (2019). Cardiac Fibrosis: Potential Therapeutic Targets. Transl. Res. 209, 121–137. doi:10.1016/j.trsl.2019.03.001
Pathak, A., del Monte, F., Zhao, W., Schultz, J.-E., Lorenz, J. N., Bodi, I., et al. (2005). Enhancement of Cardiac Function and Suppression of Heart Failure Progression by Inhibition of Protein Phosphatase 1. Circulation Res. 96 (7), 756–766. doi:10.1161/01.res.0000161256.85833.fa
Phosri, S., Arieyawong, A., Bunrukchai, K., Parichatikanond, W., Nishimura, A., Nishida, M., et al. (2017). Stimulation of Adenosine A2B Receptor Inhibits Endothelin-1-Induced Cardiac Fibroblast Proliferation and α-Smooth Muscle Actin Synthesis Through the cAMP/Epac/PI3K/Akt-Signaling Pathway. Front. Pharmacol. 8, 428. doi:10.3389/fphar.2017.00428
Piccoli, M.-T., Bär, C., and Thum, T. (2016). Non-coding RNAs as Modulators of the Cardiac Fibroblast Phenotype. J. Mol. Cell. Cardiol. 92, 75–81. doi:10.1016/j.yjmcc.2015.12.023
Pisitkun, T., Shen, R.-F., and Knepper, M. A. (2004). Identification and Proteomic Profiling of Exosomes in Human Urine. Proc. Natl. Acad. Sci. U.S.A. 101 (36), 13368–13373. doi:10.1073/pnas.0403453101
Poller, W., Dimmeler, S., Heymans, S., Zeller, T., Haas, J., Karakas, M., et al. (2018). Non-coding RNAs in Cardiovascular Diseases: Diagnostic and Therapeutic Perspectives. Eur. Heart J. 39 (29), 2704–2716. doi:10.1093/eurheartj/ehx165
Porter, K. E., and Turner, N. A. (2009). Cardiac Fibroblasts: at the Heart of Myocardial Remodeling. Pharmacol. Ther. 123 (2), 255–278. doi:10.1016/j.pharmthera.2009.05.002
Qiao, L., Hu, S., Liu, S., Zhang, H., Ma, H., Huang, K., et al. (2019). microRNA-21-5p Dysregulation in Exosomes Derived from Heart Failure Patients Impairs Regenerative Potential. J. Clin. Invest 129 (6), 2237–2250. doi:10.1172/jci123135
Qin, X.-j., Zhang, J.-x., and Wang, R.-l. (2020). Exosomes as Mediators and Biomarkers in Fibrosis. Biomarkers Med. 14 (8), 697–712. doi:10.2217/bmm-2019-0368
Raju, S., Fish, J. E., and Howe, K. L. (2020). MicroRNAs as Sentinels and Protagonists of Carotid Artery Thromboembolism. Clin. Sci. (Lond) 134 (2), 169–192. doi:10.1042/cs20190651
Ranjan, P., Kumari, R., and Verma, S. K. (2019). Cardiac Fibroblasts and Cardiac Fibrosis: Precise Role of Exosomes. Front. Cell Dev. Biol. 7, 318. doi:10.3389/fcell.2019.00318
Raposo, G., and Stoorvogel, W. (2013). Extracellular Vesicles: Exosomes, Microvesicles, and Friends. J. Cell Biol. 200 (4), 373–383. doi:10.1083/jcb.201211138
Saadatpour, L., Fadaee, E., Fadaei, S., Nassiri Mansour, R., Mohammadi, M., Mousavi, S. M., et al. (2016). Glioblastoma: Exosome and microRNA as Novel Diagnosis Biomarkers. Cancer Gene Ther. 23 (12), 415–418. doi:10.1038/cgt.2016.48
Savvatis, K., Pappritz, K., Becher, P. M., Lindner, D., Zietsch, C., Volk, H.-D., et al. (2014). Interleukin-23 Deficiency Leads to Impaired Wound Healing and Adverse Prognosis after Myocardial Infarction. Circ. Heart Fail. 7 (1), 161–171. doi:10.1161/circheartfailure.113.000604
Shao, J., Zaro, J., and Shen, Y. (2020a). Advances in Exosome-Based Drug Delivery and Tumor Targeting: From Tissue Distribution to Intracellular Fate. Ijn 15, 9355–9371. doi:10.2147/ijn.s281890
Shao, L., Zhang, Y., Pan, X., Liu, B., Liang, C., Zhang, Y., et al. (2020b). Knockout of Beta-2 Microglobulin Enhances Cardiac Repair by Modulating Exosome Imprinting and Inhibiting Stem Cell-Induced Immune Rejection. Cell. Mol. Life Sci. 77 (5), 937–952. doi:10.1007/s00018-019-03220-3
Shen, J., Xing, W., Gong, F., Wang, W., Yan, Y., Zhang, Y., et al. (2019). MiR-150-5p Retards the Progression of Myocardial Fibrosis by Targeting EGR1. Cell Cycle 18 (12), 1335–1348. doi:10.1080/15384101.2019.1617614
Shen, M., Shen, Y., Fan, X., Men, R., Ye, T., and Yang, L. (2020). Roles of Macrophages and Exosomes in Liver Diseases. Front. Med. 7, 583691. doi:10.3389/fmed.2020.583691
Siamwala, J. H., Zhao, A., Barthel, H., Pagano, F. S., Gilbert, R. J., and Rounds, S. (2020). Adaptive and Innate Immune Mechanisms in Cardiac Fibrosis Complicating Pulmonary Arterial Hypertension. Physiol. Rep. 8 (15), e14532. doi:10.14814/phy2.14532
Simons, M., and Raposo, G. (2009). Exosomes - Vesicular Carriers for Intercellular Communication. Curr. Opin. Cell Biol. 21 (4), 575–581. doi:10.1016/j.ceb.2009.03.007
Sohns, C., and Marrouche, N. F. (2020). Atrial Fibrillation and Cardiac Fibrosis. Eur. Heart J. 41 (10), 1123–1131. doi:10.1093/eurheartj/ehz786
Song, Y., Zhang, C., Zhang, J., Jiao, Z., Dong, N., Wang, G., et al. (2019). Localized Injection of miRNA-21-Enriched Extracellular Vesicles Effectively Restores Cardiac Function after Myocardial Infarction. Theranostics 9 (8), 2346–2360. doi:10.7150/thno.29945
Sun, D., Zhuang, X., Xiang, X., Liu, Y., Zhang, S., Liu, C., et al. (2010). A Novel Nanoparticle Drug Delivery System: the Anti-inflammatory Activity of Curcumin Is Enhanced when Encapsulated in Exosomes. Mol. Ther. 18 (9), 1606–1614. doi:10.1038/mt.2010.105
Sun, J., Shen, H., Shao, L., Teng, X., Chen, Y., Liu, X., et al. (2020). HIF-1α Overexpression in Mesenchymal Stem Cell-Derived Exosomes Mediates Cardioprotection in Myocardial Infarction by Enhanced Angiogenesis. Stem Cell Res. Ther. 11 (1), 373. doi:10.1186/s13287-020-01881-7
Sung, B. H., Parent, C. A., and Weaver, A. M. (2021). Extracellular Vesicles: Critical Players during Cell Migration. Dev. Cell 56 (13), 1861–1874. doi:10.1016/j.devcel.2021.03.020
Tallquist, M. D. (2020). Cardiac Fibroblast Diversity. Annu. Rev. Physiol. 82, 63–78. doi:10.1146/annurev-physiol-021119-034527
Talman, V., and Ruskoaho, H. (2016). Cardiac Fibrosis in Myocardial Infarction-From Repair and Remodeling to Regeneration. Cell Tissue Res. 365 (3), 563–581. doi:10.1007/s00441-016-2431-9
Teng, Y., Ren, Y., Sayed, M., Hu, X., Lei, C., Kumar, A., et al. (2018). Plant-Derived Exosomal MicroRNAs Shape the Gut Microbiota. Cell Host Microbe 24 (5), 637–652. e8. doi:10.1016/j.chom.2018.10.001
Théry, C., Ostrowski, M., and Segura, E. (2009). Membrane Vesicles as Conveyors of Immune Responses. Nat. Rev. Immunol. 9 (8), 581–593. doi:10.1038/nri2567
Thum, T., and Condorelli, G. (2015). Long Noncoding RNAs and microRNAs in Cardiovascular Pathophysiology. Circ. Res. 116 (4), 751–762. doi:10.1161/circresaha.116.303549
Tian, C., Gao, L., Zimmerman, M. C., and Zucker, I. H. (2018). Myocardial Infarction-Induced microRNA-Enriched Exosomes Contribute to Cardiac Nrf2 Dysregulation in Chronic Heart Failure. Am. J. Physiology-Heart Circulatory Physiology 314 (5), H928–h939. doi:10.1152/ajpheart.00602.2017
Viaud, S., Ullrich, E., Zitvogel, L., and Chaput, N. (2008). Exosomes for the Treatment of Human Malignancies. Horm. Metab. Res. 40 (2), 82–88. doi:10.1055/s-2007-1022548
Wang, B., Wang, Z.-M., Ji, J.-L., Gan, W., Zhang, A., Shi, H.-J., et al. (2020). Macrophage-Derived Exosomal Mir-155 Regulating Cardiomyocyte Pyroptosis and Hypertrophy in Uremic Cardiomyopathy. JACC Basic Transl. Sci. 5 (2), 148–166. doi:10.1016/j.jacbts.2019.10.011
Wang, B., Zhang, A., Wang, H., Klein, J. D., Tan, L., Wang, Z.-M., et al. (2019). miR-26a Limits Muscle Wasting and Cardiac Fibrosis through Exosome-Mediated microRNA Transfer in Chronic Kidney Disease. Theranostics 9 (7), 1864–1877. doi:10.7150/thno.29579
Wang, C., Li, Z., Liu, Y., and Yuan, L. (2021a). Exosomes in Atherosclerosis: Performers, Bystanders, Biomarkers, and Therapeutic Targets. Theranostics 11 (8), 3996–4010. doi:10.7150/thno.56035
Wang, C., Zhang, C., Liu, L., A, X., Chen, B., Li, Y., et al. (2017). Macrophage-Derived Mir-155-Containing Exosomes Suppress Fibroblast Proliferation and Promote Fibroblast Inflammation during Cardiac Injury. Mol. Ther. 25 (1), 192–204. doi:10.1016/j.ymthe.2016.09.001
Wang, L., Liu, J., Xu, B., Liu, Y.-L., and Liu, Z. (2018a). Reduced Exosome miR-425 and miR-744 in the Plasma Represents the Progression of Fibrosis and Heart Failure. Kaohsiung J. Med. Sci. 34 (11), 626–633. doi:10.1016/j.kjms.2018.05.008
Wang, Q.-G., Cheng, B., He, Y.-Z., Li, L.-J., Ling, Y., Luo, G., et al. (2021b). miR-320a in S-erum E-xosomes P-romotes M-yocardial F-ibroblast P-roliferation via R-egulating the PIK3CA/Akt/mTOR S-ignaling P-athway in HEH2 C-ells. Exp. Ther. Med. 22 (2), 873. doi:10.3892/etm.2021.10305
Wang, X., Chen, Y., Zhao, Z., Meng, Q., Yu, Y., Sun, J., et al. (2018b). Engineered Exosomes With Ischemic Myocardium-Targeting Peptide for Targeted Therapy in Myocardial Infarction. J. Am. Heart Assoc. 7 (15), e008737. doi:10.1161/JAHA.118.008737
Wang, X., Gu, H., Huang, W., Peng, J., Li, Y., Yang, L., et al. (2016). Hsp20-Mediated Activation of Exosome Biogenesis in Cardiomyocytes Improves Cardiac Function and Angiogenesis in Diabetic Mice. Diabetes 65 (10), 3111–3128. doi:10.2337/db15-1563
Wei, C., Kim, I.-K., Kumar, S., Jayasinghe, S., Hong, N., Castoldi, G., et al. (2013). NF-κB Mediated miR-26a Regulation in Cardiac Fibrosis. J. Cell. Physiol. 228 (7), 1433–1442. doi:10.1002/jcp.24296
Wei, H., Chen, Q., Lin, L., Sha, C., Li, T., Liu, Y., et al. (2021). Regulation of Exosome Production and Cargo Sorting. Int. J. Biol. Sci. 17 (1), 163–177. doi:10.7150/ijbs.53671
Yáñez-Mó, M., Siljander, P. R., Andreu, Z., Zavec, A. B., Borràs, F. E., Buzas, E. I., et al. (2015). Biological Properties of Extracellular Vesicles and Their Physiological Functions. J. Extracell. Vesicles 4, 27066. doi:10.3402/jev.v4.27066
Yang, D., Zhang, W., Zhang, H., Zhang, F., Chen, L., Ma, L., et al. (2020). Progress, Opportunity, and Perspective on Exosome Isolation - Efforts for Efficient Exosome-Based Theranostics. Theranostics 10 (8), 3684–3707. doi:10.7150/thno.41580
Yang, J., Li, Y., Xue, F., Liu, W., and Zhang, S. (2017). Exosomes Derived from Cardiac Telocytes Exert Positive Effects on Endothelial Cells. Am. J. Transl. Res. 9 (12), 5375–5387.
Yang, J., Yu, X., Xue, F., Li, Y., Liu, W., and Zhang, S. (2018). Exosomes Derived from Cardiomyocytes Promote Cardiac Fibrosis via Myocyte-Fibroblast Cross-Talk. Am. J. Transl. Res. 10 (12), 4350–4366.
Yang, J., Yu, X. F., Li, Y. Y., Xue, F. T., and Zhang, S. (2019). Decreased HSP70 Expression on Serum Exosomes Contributes to Cardiac Fibrosis during Senescence. Eur. Rev. Med. Pharmacol. Sci. 23 (9), 3993–4001. doi:10.26355/eurrev_201905_17829
Yao, J., Huang, K., Zhu, D., Chen, T., Jiang, Y., Zhang, J., et al. (2021). A Minimally Invasive Exosome Spray Repairs Heart after Myocardial Infarction. ACS Nano. 15, 11099–11111. doi:10.1021/acsnano.1c00628
Yu, B., Kim, H. W., Gong, M., Wang, J., Millard, R. W., Wang, Y., et al. (2015). Exosomes Secreted from GATA-4 Overexpressing Mesenchymal Stem Cells Serve as a Reservoir of Anti-apoptotic microRNAs for Cardioprotection. Int. J. Cardiol. 182, 349–360. doi:10.1016/j.ijcard.2014.12.043
Yu, Y., Sun, J., Wang, R., Liu, J., Wang, P., and Wang, C. (2019). Curcumin Management of Myocardial Fibrosis and its Mechanisms of Action: A Review. Am. J. Chin. Med. 47 (8), 1675–1710. doi:10.1142/S0192415X19500861
Yuan, J., Liu, H., Gao, W., Zhang, L., Ye, Y., Yuan, L., et al. (2018). MicroRNA-378 Suppresses Myocardial Fibrosis through a Paracrine Mechanism at the Early Stage of Cardiac Hypertrophy Following Mechanical Stress. Theranostics 8 (9), 2565–2582. doi:10.7150/thno.22878
Zaborowski, M. P., Balaj, L., Breakefield, X. O., and Lai, C. P. (2015). Extracellular Vesicles: Composition, Biological Relevance, and Methods of Study. Bioscience 65 (8), 783–797. doi:10.1093/biosci/biv084
Zamani, P., Fereydouni, N., Butler, A. E., Navashenaq, J. G., and Sahebkar, A. (2019). The Therapeutic and Diagnostic Role of Exosomes in Cardiovascular Diseases. Trends Cardiovasc. Med. 29 (6), 313–323. doi:10.1016/j.tcm.2018.10.010
Zhang, J., Li, S., Li, L., Li, M., Guo, C., Yao, J., et al. (2015). Exosome and Exosomal microRNA: Trafficking, Sorting, and Function. Genomics, Proteomics Bioinforma. 13 (1), 17–24. doi:10.1016/j.gpb.2015.02.001
Zhang, L., and Yu, D. (2019). Exosomes in Cancer Development, Metastasis, and Immunity. Biochimica Biophysica Acta (BBA) - Rev. Cancer 1871 (2), 455–468. doi:10.1016/j.bbcan.2019.04.004
Zhang, W., Chang, H., Zhang, H., and Zhang, L. (2017). MiR-30e Attenuates Isoproterenol-induced Cardiac Fibrosis Through Suppressing Snai1/TGF-β Signaling. J. Cardiovasc Pharmacol. 70 (6), 362–368. doi:10.1097/fjc.0000000000000526
Zhang, X., Hu, C., Yuan, Y.-P., Song, P., Kong, C.-Y., Wu, H.-M., et al. (2021). Endothelial ERG Alleviates Cardiac Fibrosis via Blocking Endothelin-1-dependent Paracrine Mechanism. Cell Biol. Toxicol. 37 (6), 873–890. doi:10.1007/s10565-021-09581-5
Zhang, Z., Yang, J., Yan, W., Li, Y., Shen, Z., and Asahara, T. (2016). Pretreatment of Cardiac Stem Cells With Exosomes Derived From Mesenchymal Stem Cells Enhances Myocardial Repair. J. Am. Heart Assoc. 5 (1), e002856. doi:10.1161/JAHA.115.002856
Zhao, J., Chen, Y., Chen, Q., Hong, T., Zhong, Z., He, J., et al. (2021). Curcumin Ameliorates Cardiac Fibrosis by Regulating Macrophage-Fibroblast Crosstalk via IL18-P-SMAD2/3 Signaling Pathway Inhibition. Front. Pharmacol. 12, 784041. doi:10.3389/fphar.2021.662003
Zhu, J., Lu, K., Zhang, N., Zhao, Y., Ma, Q., Shen, J., et al. (2018). Myocardial Reparative Functions of Exosomes from Mesenchymal Stem Cells Are Enhanced by Hypoxia Treatment of the Cells via Transferring microRNA-210 in an nSMase2-dependent Way. Artif. Cells Nanomed Biotechnol. 46 (8), 1659–1670. doi:10.1080/21691401.2017.1388249
Keywords: exosome, cardiac fibrosis, cardiovascular diseases, myofibroblast, miRNAs
Citation: Fan J, Ren M and He Y (2022) Diagnostic and Therapeutic Properties of Exosomes in Cardiac Fibrosis. Front. Cell Dev. Biol. 10:931082. doi: 10.3389/fcell.2022.931082
Received: 28 April 2022; Accepted: 06 June 2022;
Published: 04 July 2022.
Edited by:
Marcio L. Rodrigues, Oswaldo Cruz Foundation (Fiocruz), BrazilReviewed by:
Ian Michael Dixon, University of Manitoba, CanadaFabiana Passaro, University of Naples Federico II, Italy
Copyright © 2022 Fan, Ren and He. This is an open-access article distributed under the terms of the Creative Commons Attribution License (CC BY). The use, distribution or reproduction in other forums is permitted, provided the original author(s) and the copyright owner(s) are credited and that the original publication in this journal is cited, in accordance with accepted academic practice. No use, distribution or reproduction is permitted which does not comply with these terms.
*Correspondence: Yuquan He, aGV5cUBqbHUuZWR1LmNu