- 1Institute of Biology, Westlake Institute for Advanced Study, Hangzhou, China
- 2Laboratory of Cell Fate Control, School of Life Sciences, Westlake University, Hangzhou, China
- 3College of Life Sciences, Zhejiang University, Hangzhou, China
Traditional pharmaceuticals in the forms of small chemical compounds or macromolecules such as proteins or RNAs have provided lifesaving solutions to many acute and chronic conditions to date. However, there are still many unmet medical needs, especially those of degenerative nature. The advent of cell-based therapy holds the promise to meet these challenges. In this review, we highlight a relatively new paradigm for generating or regenerating functional cells for replacement therapy against conditions such as type I diabetes, myocardial infarction, neurodegenerative diseases and liver fibrosis. We focus on the latest progresses in cellular reprogramming for generating diverse functional cell types. We will also discuss the mechanisms involved and conclude with likely general principles underlying reprogramming.
Introduction
Cellular reprogramming refers to a process of cell fate transition, i.e., converting one type of cells to another. The field has a long and rich history starting from the pioneering work of Gurdon in the 1950s. The initial success in frog cloning highlighted the potential of reprogramming fully differentiated nuclei back to totipotent state that can give rise to a new frog (Gurdon, 1962). Three decades later, MyoD was used to convert the mouse embryonic fibroblasts (MEFs) into myoblasts in 1987, marking the first transcription factor or TF to convert cell fate (Davis et al., 1987). In 2006, Takahashi and Yamanaka used 4 TFs, Oct4, Sox2, Klf4 and Myc to convert MEFs to induced pluripotent stem cells (iPSCs) (Takahashi and Yamanaka, 2006; Takahashi et al., 2007). In addition, numerous functional cell types have been reported through reprogramming, including neurons (Vierbuchen et al., 2010; Barker et al., 2018), myoblasts (Davis et al., 1987), cardiomyocytes (Ieda et al., 2010; Qian et al., 2012), hepatocytes (Huang et al., 2011; Sekiya and Suzuki, 2011) and pancreatic β-cells (Zhou et al., 2008). Together, these studies not only demonstrated the feasibility of generating desired cell types from somatic cells, but also provided a rational system to analyze cell fate control.
Despite tremendous advances so far, concerns such as tumorigenicity and efficiency have hampered efforts to implement reprogramming technologies towards human therapies. Alternative approaches such as direct programming in vivo or transdifferentiation can provide more appropriate microenvironment with adequate and suitable biochemical and biophysical conditions to make induced mature cells involved into the surrounding tissues and replenish the loss of functional cells in the injured organs (Wang H. et al., 2021). Nevertheless, there are challenges ahead that require more careful investigations, leading to better solutions to issues such as low efficiencies, lack of standard, as well as safe and specific delivery (Fu and Srivastava, 2015; Gascon et al., 2017; Barker et al., 2018; Wang H. et al., 2021). In recent years, the field has achieved substantial progresses in solving these problems. Here we review these novel and promising advances by different cell types and summarize the mechanism and general principles underlying the process of reprogramming for generation for functional cells.
Skeletal Myogenesis
MYOD was the first TF known to mediate lineage fate conversion, although earlier hints were reported on myoblast generation by treatment with DNA methylation inhibitor 5-Azacytidine that can target the locus of MyoD and activate the expression (Constantinides et al., 1977; Davis et al., 1987). Since then, MYOD has become the most used factor for inducing the reprogramming of skeletal muscle lineage (Chal and Pourquie, 2017). Furthermore, MYOD has been used to induce the direct differentiation of human embryonic stem cells (hESCs) and human-induced pluripotent stem cells (hiPSCs) to myogenic cells, which can be utilized to transplant to alleviate the symptoms of mouse muscle diseases like Duchenne or Miyoshi muscular dystrophies (Goudenege et al., 2012; Saho et al., 2016; Young et al., 2016). The combination of MYOD overexpression and treatment with three chemical molecules CHIR99021, Forskolin and RepSOX could convert mouse fibroblasts into expandable induced PAX7+ myogenic progenitor cells (iMPCs) (Bar-Nur et al., 2018). Mechanically, the characteristics of fibroblasts cell fate would be lost gradually, which is prior to the acquirement of stem cell properties during the converting process where Tet regulated DNA demethylation plays a critical role (Yagi et al., 2021).
Interestingly, alternative factors such as transcription factors PAX3/PAX7 have been shown to induce myogenic differentiation in mouse and human pluripotent stem cells (Darabi et al., 2008; Darabi et al., 2012; Quattrocelli et al., 2015; Carrio et al., 2016). Compared with MYOD triggered transdifferentiation, the PAX3/PAX7 approach takes advantage of embryonic body (EB) differentiation followed with sorting by cell surface markers like PDGFRα (CD140a) or CD56 to enrich myogenic cells (Chal and Pourquie, 2017). While the in vitro induced myogenic progenitors by conditional expression of Pax3/Pax7 were immature and resemble embryonic/fetal myoblasts, these cells are functionally mature and have long-term regenerative capability upon transplantation (Incitti et al., 2019).
In addition to transcription factors, bioactive nanomaterials have also been utilized to promote the myogenic differentiation. Monodispersed gold and gold-silver nanoparticles (AuNPs and Au-AgNPs) can be used for the attachment and proliferation of myoblasts and facilitate the myogenic differentiation of myoblasts through activating p38α mitogen-activated protein kinase pathway and enhancing the expression of myogenic genes MyoD, MyoG and Tnnt-1 (Ge et al., 2018). Polypyrrole (PPy) is another frequently used biomaterials that has been reported to promote the myogenic differentiation in vitro (Li et al., 2019). However, the material has poor degradability and low solubility, limiting its use in vivo for regenerative medicine (Li et al., 2019). Recently, Zhou et al. designed an injectable multifunctional polypyrrole@polydopamine (PPy@PDA) crosslinked nanocomposite hydrogel to overcome the drawbacks mentioned above, and they constructed the nanocomposite-crosslinked Pluronic F-127 (F127)-polycitrate matrix (FPCP) to accomplish the enhancement of skeletal muscle repair and regeneration in vivo (Zhou et al., 2021). The combination of advanced materials as well as engineered cell types may ultimately provide the solution to myogenic therapies in patients.
Islet β-cells
The pancreatic islet is a specialized organ with both endocrine (islets of Langerhans) and exocrine (acinar cells and ductal cells) functions (Zhou and Melton, 2018). Exocrine cells, which make up the majority of islets, have strong regenerative capacity whereby the exocrine pancreas could restore rapidly when acute pancreatitis occurs. On the other hand, the endocrine cells, only accounting for the 5% of pancreatic islet, have little capability of regeneration (Zhou and Melton, 2018). Islet β-cells, as one of pancreatic endocrine cell types, can secrete insulin especially and are selectively destroyed in type I diabetes. Replenishing these patients’ pancreatic cells with functional β-cells through a regenerative medicine approach has great potential as a therapeutic alternative to the traditional therapy of lifelong insulin injections.
In vivo transdifferentiation is a promising strategy whereby the initial cell source is abundant, and the immune rejection could be avoided. In 2008, the first documented in vivo direct reprogramming was performed of β-cells regeneration converted from pancreatic exocrine cells (acinar cells) via the combinatory expression of three transcription factors Ngn3, Pdx1 and MafA (Zhou et al., 2008; Li et al., 2014). Further investigation found this combination could also induce the gastrointestinal epithelial cells into endocrine β-like cells (Chen Y.-J. et al., 2014). Similarly, cells of the antral stomach also tend to complete this conversion (Ariyachet et al., 2016). Only the combination of Pdx1 and MafA delivered by adeno-associated virus (AAVs) through pancreatic duct could reprogram alpha cells into beta cells in mouse and human cells (Xiao et al., 2018; Furuyama et al., 2019). Moreover, by taking advantages of the close developmental relationship, ectopic expression of Tgif2 can convert hepatic cell fate to that of pancreatic progenitors (Cerda-Esteban et al., 2017). Unexpectedly, depletion of Foxo1 in Neurog3 (+) enteroendocrine progenitor cells generated mature β-like cells with insulin and C-peptide secreting capacity in response to glucose and sulfonylureas (Talchai et al., 2012). Additionally, the inactivation of Fbw7 could convert pancreatic ductal cells into α, δ, and β cells (Sancho et al., 2014) (Table 1).
However, one of the challenges in this field is that the newly induced β-cells cannot persist for long in type I diabetes due to autoimmunity (Pipeleers et al., 2002; Zaret and Grompe, 2008). Moreover, it has been reported that β-cells could be reactivated through the reversal of autoimmunity (Tang et al., 2020). Therefore, the combination of anti-autoimmune therapy would have a positive effect on the regeneration of β-cells (Wherrett et al., 2011; Bluestone et al., 2015; Wang et al., 2018; Tang et al., 2020). Alternatively, some porous biomaterials, like alginate derivatives, could be utilized as immune barrier to prevent these cells from the attack of autoimmunity (Vegas et al., 2016). Intriguingly, gene therapy through ectopic expression of Pdx1 and MafA by AAVs could obtain insulin-producing cells with the resistance of autoimmunity in the non-obese diabetic (NOD) mouse (Xiao et al., 2018).
Overall, these diverse approaches all show promises in generating functional cells, thus, providing solid foundations for future pre- and clinical development for curing type I and II diabetes with cell reprogramming.
Cardiomyocytes
Heart failure is one of the most serious diseases that threaten human life in the modern era, affecting more than 14 million people worldwide (Laflamme and Murry, 2011; Qian et al., 2012; Arrigo et al., 2020; Roger, 2021; Savarese et al., 2022). About 1/4 cardiomyocytes (CMs) dysfunction a few hours after a myocardial infarction in the human left ventricle, but the left ventricle resident cardiomyocytes have little capability of proliferation after birth (Murry et al., 2006). Even with the aging process, the left and right ventricular myocardium have been estimated to lose 38 million and 14 million myocyte nuclei/year irreversibly, along with the enlargement of the remaining cardiomyocytes (Olivetti et al., 1991). Therefore, it is critical to obtain the abundant functional cardiomyocytes capable of repairing the injured or aging heart.
Several approaches have been reported. First, combinatorial expression of two cardiac transcription factors, Gata4 and Tbx5 and the subunit of BAF chromatin remodeling complexes, Smarcd3, has been reported to convert mouse mesoderm into cardiomyocytes efficiently (Takeuchi and Bruneau, 2009). Secondly, Gata4, Tbx5 and Mef2c can induce the direct reprogramming of postnatal cardiac or dermal fibroblasts into cardiomyocyte-like cells in a complementary approach (Ieda et al., 2010). Remarkably, this combination of the three transcription factors (Gata4, Tbx5 and Mef2c) with or without the fourth factor Hand2 can convert the postnatal murine cardiac fibroblasts into induced cardiomyocytes in the mouse model of myocardial infarction for the first time (Qian et al., 2012; Song et al., 2012). Mechanically, Mef2c and Tbx5 serve to open the compacted chromatin and the factors behave through context-specific cooperative mechanisms to guide cardiac reprogramming (Liu et al., 2020). Thirdly, miRNAs (miRNAs 1,133, 208, and 499) can convert fibroblasts to cardiomyocytes in vitro and in vivo (Jayawardena et al., 2012). Fourthly, a combination of microRNA mimic miR-208b-3p with the treatment of ascorbic acid and bone morphogenetic protein 4 (BMP4) can trigger the formation of a tissue-like structure containing three distinct cell types, cardiomyocytes, endothelial cells and smooth muscle cells from mouse tail-tip fibroblasts and this structure could be transplanted for the restoration of the infarcted hearts (Cho et al., 2021). Finally, nine chemicals (9C) can induce human fibroblasts to generate cardiomyocyte-like cells in vivo of mouse heart infarction model (Cao et al., 2016) (Table 2).
There were discrepancies associated with some of the above mentioned methods. For instance, while the three transcription factors Gata4, Mef2 and Tbx5 (GMT) can realize a robust transdifferentiation to cardiomyocytes in mouse, the same combination fails to perform the corresponding reprogramming in human (Yamakawa and Ieda, 2021). In the later research, Rie Wada and others achieved this purpose of transdifferentiation from human fibroblasts by addition of two cardiac-specific genes MESP1 and MYOCD (GMTMM) (Wada et al., 2013). Although other combinations, like six factors (Gata4, Tbx5, Hand2, Myocd, miR-1, and miR-133) (Nam et al., 2013) and seven factors (Gata4, Mef2c, Tbx5, Mesp1, Myocd, Esrrg, and Zfpm2) (Fu et al., 2013) have also been reported to complete this transition, almost all documented cases have lower efficiency of conversion comparing with that in mouse and lack functional beating property (Yamakawa and Ieda, 2021) (Table 2). These studies highlight the need to standardize protocols and criteria used in evaluating efficacy.
In addition to the transdifferentiation from cardiac fibroblasts into cardiomyocytes to alleviate the symptom of heat failure, enhancing the capability of myocardial regeneration was considered another promising strategy. Through the transient ectopic heart-specific expression of Oct4, Sox2, Klf4, and c-Myc (OSKM), the adult mouse cardiomyocytes could be reset into a kind of fetal state whereby nondividing cardiomyocytes regain their regenerative capacity (Chen et al., 2021). Notably, the long-term expression of OSKM tends to lead to tumor formation (Chen et al., 2021).
A newly isolated culture of expandable cardiovascular progenitor cells (CPCs) was reported with promising characteristics for regenerative medicine (Lalit et al., 2016; Zhang et al., 2016; Wang et al., 2022). These multipotent stem cells exist exclusively in the embryonic development and could subsequently generate heart tissue (Laugwitz et al., 2005). Recently, CPCs were reported to be obtained by treatment with six small molecules from mouse or human fibroblasts and cultured in chemical define and xeno-free conditions (Wang et al., 2022), which provides a new and abundant cell source for cardiac cell therapy and enhances the clinical application prospects of CPCs.
Neurons
Neural degenerative diseases such as Parkinson’s disease and Alzheimer disease remain untreatable. Major efforts in regenerative medicine are directed towards these two degenerative diseases. Due to limited capability of self-renewal in the adult central nervous system and the highly organized complicated neural circuits make the neurodegeneration or nerve injuries one of the most intractable diseases to cure.
The non-neuronal macroglia include NG2 glia and astrocytes. NG2 cells are the progenitors of myelinating oligodendrocytes with capability of self-renewal and proliferation (Kang et al., 2010). Astrocytes are widespread in the central nervous system and contain neural differentiation potential under pathological conditions (Magnusson et al., 2014). These characteristics make glial cells the ideal cell sources to replenish the loss functional neurons in neural degenerative diseases or neural injuries (Wang H. et al., 2021). Nevertheless, it has proved to be difficult for traditional strategies to replace the compensatory glial cells or scars with functional neurons (Barker et al., 2018). Recently, the approaches of direct reprogramming of resident glial cells to functional neurons in vivo have shed light on the repair of the diseased nervous system.
Since 2013, several groups have proved the feasibility that the glial cells can be converted to functional new neurons through ectopic expression of neural master gene(s) by different adeno-associated virus (AAV) serotypes or retrovirus, independently (Guo et al., 2014; Matsuda et al., 2019; Chen et al., 2020; Zhou et al., 2020). Single factors including NEUROD1 (Guo et al., 2014; Chen et al., 2020), SOX2 (Niu et al., 2013; Tai et al., 2021) or ASCL1 (Liu Y. et al., 2015) is enough to accomplish this kind of transition. It is worth noting that SOX2 seems to induce the glial cells into a progenitor condition (Niu et al., 2013; Tai et al., 2021). Furthermore, this genetic method of transformation can be substituted by sequential exposure to a cocktail of small molecules in vitro (Zhang et al., 2015). Intriguingly, knocking down only one single RNA-binding protein, polypyrimidine tract-binding protein 1 (Ptbp1), which has been reported to induce the transdifferentiation of fibroblasts into neurons in vitro previously (Xue et al., 2013), can perform a robust conversion of astrocytes into functional dopaminergic neurons to alleviate motor defects in a Parkinson’s disease mouse model by CRISPR system CasRx (Zhou et al., 2020) or antisense oligonucleotides (ASOs) (Qian et al., 2020). Additionally, this approach can also alleviate the eyesight of retinal injury mouse model by reprogramming Müller glia into retinal ganglion cells (RGCs) (Zhou et al., 2020). If true, the small molecules targeting Ptbp1 may also be utilized in the design of therapeutics for neurodegeneration or nerve injuries (Table 3).
Nevertheless, the promising strategies of glia-to-neuron conversion have also been challenged by the work recently reported (Wang L.-L. et al., 2021; Rao et al., 2021). By rigorous lineage tracing methods and various AAVs, they questioned the capability of NeuroD1 of inducing the reprogramming from astrocytes to functional neurons. Instead, the so-called newborn neurons are the progenies of resident neurons. Furthermore, the astrocytes-to-neurons transition also fails to be detected upon the depletion of PTBP1 (Wang L.-L. et al., 2021). Meanwhile, the NeuroD1-induced microglia-to-neuron conversion is also questioned (Rao et al., 2021).
In summary, despite the reported induced reprogramming from the resident glial cells to functional neurons, this is a promising means to replenish the loss of neurons resulting from the neurodegenerative diseases or nerve injuries. The details underlying this process and the original cell species are still controversial, which is partly on account of the variant experimental conditions, including virus titer, the parameters and window of detection and the disease models to name a few. A broad and comprehensive knowledge will be necessary before we can perform the clinical trials.
Tumor Cells
In theory, it should be possible to apply iPSC technology to tumor cells, to convert them to a benign phenotype, or one sensitive to chemotherapeutic drugs. Indeed, Utikal et al. identified that mouse malignant R545 melanoma cell line could be reprogrammed into iPSCs by the transfection of Yamanaka factors (Utikal et al., 2009). The expression of endogenous Oct4, Klf4, and Myc was activated in these iPSC clones derived from parental R545 cells. Besides, these iPSC lines also showed demethylation of the pluripotency-related gene Oct4 and Nanog promoters and the decrease of tumorigenicity in vivo (Utikal et al., 2009). In 2010, Mioshi et al. reported that gastrointestinal cancer cells, including pancreatic, liver, and colorectal cancer cells, acquired pluripotency so that these cells displayed the potential to form ectoderm, mesoderm, and endoderm morphological patterns after the introduction of Yamanaka factors. Induced cells were less malignant both in vitro and in vivo but showed higher sensitivity to 5-fluorodeoxyuridine than the parental cells (Miyoshi et al., 2010). To explore the effect of oncogene on cancer cell reprogramming, Carette et al. reprogrammed human KBM7 chronic myeloid leukemia cells, which harbored breakpoint-cluster region -Abelson leukemia gene (BCR-ABL) fusion, through the retrovirus-mediated expression of Yamanaka factors. They found that reprogramming activated the expression of pluripotency-related genes and restored the differentiation potency in cancer cell-derived iPSCs. In addition, reprogrammed cells lost dependency on BCR-ABL oncogene signaling and possessed resistance to the inhibitor treatment, leading to a potential approach for leukemia treatment (Carette et al., 2010). Similarly, in the study of Liu et al., human mixed-lineage leukemia-AF9 fusion gene overexpressed acute myeloid leukemia (AML) cells were successfully converted into iPSCs through Yamanaka factors transfection. Reprogramming re-wrote epigenetic patterns so that oncogenic gene MLL-AF9 was silenced (Liu et al., 2014). To investigate whether the acquired benign feature could maintain during terminal differentiation into initial and alternative cell lineages, human osteosarcoma, liposarcoma, and sarcomas were reprogrammed by transfection of OCT4, NANOG, SOX2, LIN28, KLF4, and MYC. Interestingly, each pattern of terminal differentiation of such induced cells could abrogate the tumorigenicity of parental cells (Zhang et al., 2013) (Table 4). Cancer cell reprogramming and subsequent differentiation might be a promising strategy for the treatment of cancer.
Despite of these reported studies, only a minority of cancer cells are amenable to successful reprogramming. One obvious reason is certain mutations and genomic instability might impede cancer cell reprogramming. For instance, it has proved that Notch1 mutation induced T-acute lymphoblastic leukemia could not be converted into the pluripotent state (Liu et al., 2014). Besides, the majority of cancer patients undergo chemo- or radio-therapy before surgical resection. Whether such treatments could prevent the generation of cancer cell-derived iPSCs remains unclear. Therefore, although such iPSC technology is feasible to reverse many types of cancer cells back into a pluripotent state with benign features, the efficiency, safety and universality remains the huge challenge for its clinical application.
Principles of Cell Reprogramming
Chromatin Accessibility Dynamics During Reprogramming
The reprogramming approaches discussed above are quite diverse in terms of factors and cell types involved. One may wish to search for general principles governing these diverse processes. As it takes anywhere between 40 and 200 trillion cells to make a human, making up our brains, muscles, organs and every part of us (Bianconi et al., 2013). Yet, each of these cells share one common genome, thus, inspiring hope that unifying principles can be researched and identified. Now tools are becoming available at a faster pace, this may allow us to interrogate the genome in much greater detail. Indeed, recent cell atlas efforts have shown that each cell type possesses a unique chromatin accessibility pattern (Cusanovich et al., 2018; Cao et al., 2020; Zhang et al., 2021), which may serve as a fingerprint for the identification of cell types undergoing reprogramming or fate transition. To this end, our group have recently made progress in profiling chromatin accessibility dynamics (CAD) during reprogramming (Li D. et al., 2017; Cao et al., 2018). We have provided a general logic for understanding cell fate transition at the chromatin level as shown in Figure 1. If we mark the open chromatin sites by “1” and the opposite sites by “0” during the chromatin accessibility dynamics, every cell type could be labeled by a specific binary chromatin accessibility code.
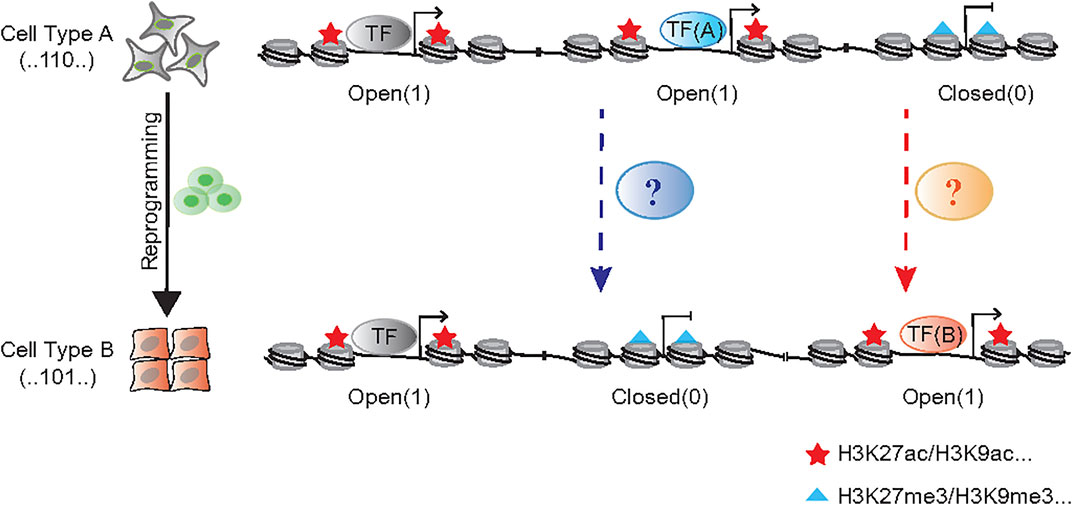
FIGURE 1. The binary chromatin accessibility code during cell reprogramming. The chromatin remodeler complexes, like BAF and NuRD function to open and close chromatin in the process of cell fate transition, respectively. The cellular morphological changes, such as EMT/MET, always accompany with that process.
It would be an intractable task to screen for the appropriate combination of factors from the highly variable gene list to perform the cell reprogramming into the targeted cell type. The analysis of chromatin accessibility dynamics could offer certain clues in that the core factors’ footprints would be uncovered from the dynamic sites. Directly comparing the chromatin accessibility dynamics between mESCs and mouse embryonic fibroblasts (MEFs) revealed that the most enriched binding motifs of transcription factors in the open sites were those of OCT, SOX2 and KLF (Li D. et al., 2017), i.e., the core Yamanaka factors in somatic cells reprogramming (Takahashi and Yamanaka, 2006). Additionally, the highest ranking enriched motifs within closed sites were the AP-1 family which would impede somatic cells reprogramming dramatically, and the AP-1 antagonist JDP2 could even substitute OCT4 to realize this transition (Liu J. et al., 2015). While the motif analysis of chromatin accessibility dynamics is an approach to rationally predict critical factors to perform unimplemented cell reprogramming, it must rely on pre-existing motif databases and the culture medium to capture the cell types of interest. Also, this method cannot be used to predict the essential regulators without DNA binding motif.
The General Protein Machine During Reprogramming
The process of cellular reprogramming is invariably accompanied by reduction of the characteristics of initial cells and the acquisition of those in targeted cells, which is also revealed by the dynamic changes in genomic accessibility. Pioneer factors serve a pivotal role in facilitating chromatin accessibility when cell fate is converted, such as NEUROD1 in microglia-neuron transition (Matsuda et al., 2019), SOX2 in somatic cell reprogramming (Chen J. et al., 2014), FOXA in fibroblast-hepatocyte transdifferentiation (Huang et al., 2011; Sekiya and Suzuki, 2011). But generally, ATP-dependent chromatin remodeling complex BAF (BRG1/BRM-associated Factor) serves a broader role in exposing DNA that wraps around nucleosomes and opens chromatin, which participates almost all the biological process (Ho and Crabtree, 2010; Hodges et al., 2016; Cenik and Shilatifard, 2021). In addition to the enzyme core subunit BRG1/BRM, the recent works suggest the other accessory compositions are also involved in reprogramming regulation, like DPF2 (Zhang et al., 2019), SS18 (Kuang et al., 2021) and PBRM1 (Sinha et al., 2020). In contrast with the opening of chromatin during reprogramming, it is equally important to close the genomic loci representing the initial cell state. Histone deacetylase 1/2 (HDAC1/2) contained nucleosome remodeling and histone deacetylation (NuRD) complex could be one of the “brake” apparatuses (Li D. et al., 2017) (Figure 1).
The Cell Morphological Changes During Reprogramming
In addition to the chromatin dynamics, cell morphological changes are also a phenomenon that cannot be ignored, which would provide certain guiding significance for the optimization in the process of cellular reprogramming. Mesenchymal-to-epithelial transitions (MET) or the opposite process EMT could provide a kind of that conceptual framework (Li et al., 2010; Zhao et al., 2015; Li Q. et al., 2017; Wang et al., 2022) to investigate the underlying mechanism and improve the strategy for reprogramming.
Chemical Induction of Reprogramming
Cell reprogramming induced by the cocktail of small molecules alone holds huge promise in regenerative medicine in that the chemical compounds are convenient to be synthesized, preserved, and standardized, and the effects are often reversible (Yu et al., 2014; Qin et al., 2017). However, in the absence of directional lineage-specific factors, reprogramming process induced by small molecules tends to be context dependent with one or more intermediate stages whereby the cell fate is highly plastic and has the potential for multidirectional differentiation (Cao et al., 2017; Li X. et al., 2017; Cao et al., 2018; Guan et al., 2022). Unlike the transcription factors that activate downstream functional gene expression directly, small molecules regulate cell fate transition through interfering epigenetic machines, signal pathways, metabolic fluxes, etc., which results in a relatively less efficient and longer process (Yu et al., 2014; Qin et al., 2017).
Conclusion and Perspectives
The successful obtainment of functional cells through cell reprogramming enables cell replacement therapy for regenerative medicine. In the last 4 decades, various cell types with no or little capability of regeneration, including cardiomyocytes, neurons, and Islet β-cells were acquired by direct or trans differentiation in vivo or vitro utilizing transcription factors, cytokines and/or small molecules.
As research on the cell atlas progresses, the increasing cell types are revealed, but only a minority of which we can control cell fate. To date, we still lack enough successful paradigms to draw a general principle/law to instruct the performance of cell reprogramming, especially for small molecules induced process. One of the well-studied barriers preventing the transition between different cell fate is the epigenetic landscape and the future promising work in this field is to figure out whether there are core epigenetic rules to stabilize cell fate and the universal tools to remove these barriers. Another thorny problem is that there are still very few cell types that can be cultured in vitro, which results in the failure to capture the target cell population. In addition to developing more suitable media, 3D cultures and in vivo reprogramming approaches may provide alternative solutions. Of course, the emerging technologies, including Cas9 screening (Black et al., 2020; Kuang et al., 2021), antibody libraries screening (Blanchard et al., 2017), Cas9-derived endogenous genes regulation tools (Grath and Dai, 2019; Russo et al., 2021), computational modelling (Letort et al., 2019; Kang and Li, 2020), etc., will also positively contribute to this field.
Author Contributions
JK and TH wrote the review article, JK and DP revised the manuscript. All authors contributed to the article and approved the submitted version.
Conflict of Interest
The authors declare that the research was conducted in the absence of any commercial or financial relationships that could be construed as a potential conflict of interest.
Publisher’s Note
All claims expressed in this article are solely those of the authors and do not necessarily represent those of their affiliated organizations, or those of the publisher, the editors and the reviewers. Any product that may be evaluated in this article, or claim that may be made by its manufacturer, is not guaranteed or endorsed by the publisher.
References
Ariyachet, C., Tovaglieri, A., Xiang, G., Lu, J., Shah, M. S., Richmond, C. A., et al. (2016). Reprogrammed Stomach Tissue as a Renewable Source of Functional β Cells for Blood Glucose Regulation. Cell. Stem Cell. 18, 410–421. doi:10.1016/j.stem.2016.01.003
Arrigo, M., Jessup, M., Mullens, W., Reza, N., Shah, A. M., Sliwa, K., et al. (2020). Acute Heart Failure. Nat. Rev. Dis. Prim. 6, 16. doi:10.1038/s41572-020-0151-7
Bar-Nur, O., Gerli, M. F. M., Di Stefano, B., Almada, A. E., Galvin, A., Coffey, A., et al. (2018). Direct Reprogramming of Mouse Fibroblasts into Functional Skeletal Muscle Progenitors. Stem Cell. Rep. 10, 1505–1521. doi:10.1016/j.stemcr.2018.04.009
Barker, R. A., Götz, M., and Parmar, M. (2018). New Approaches for Brain Repair-From Rescue to Reprogramming. Nature 557, 329–334. doi:10.1038/s41586-018-0087-1
Bianconi, E., Piovesan, A., Facchin, F., Beraudi, A., Casadei, R., Frabetti, F., et al. (2013). An Estimation of the Number of Cells in the Human Body. Ann. Hum. Biol. 40, 463–471. doi:10.3109/03014460.2013.807878
Black, J. B., McCutcheon, S. R., Dube, S., Barrera, A., Klann, T. S., Rice, G. A., et al. (2020). Master Regulators and Cofactors of Human Neuronal Cell Fate Specification Identified by CRISPR Gene Activation Screens. Cell. Rep. 33, 108460. doi:10.1016/j.celrep.2020.108460
Blanchard, J. W., Xie, J., El-Mecharrafie, N., Gross, S., Lee, S., Lerner, R. A., et al. (2017). Replacing Reprogramming Factors with Antibodies Selected from Combinatorial Antibody Libraries. Nat. Biotechnol. 35, 960–968. doi:10.1038/nbt.3963
Bluestone, J. A., Buckner, J. H., Fitch, M., Gitelman, S. E., Gupta, S., Hellerstein, M. K., et al. (2015). Type 1 Diabetes Immunotherapy Using Polyclonal Regulatory T Cells. Sci. Transl. Med. 7, 315ra189. doi:10.1126/scitranslmed.aad4134
Cao, J., O'Day, D. R., Pliner, H. A., Kingsley, P. D., Deng, M., Daza, R. M., et al. (2020). A Human Cell Atlas of Fetal Gene Expression. Science 370, eaba7721. doi:10.1126/science.aba7721
Cao, N., Huang, Y., Zheng, J., Spencer, C. I., Zhang, Y., Fu, J.-D., et al. (2016). Conversion of Human Fibroblasts into Functional Cardiomyocytes by Small Molecules. Science 352, 1216–1220. doi:10.1126/science.aaf1502
Cao, S., Yu, S., Chen, Y., Wang, X., Zhou, C., Liu, Y., et al. (2017). Chemical Reprogramming of Mouse Embryonic and Adult Fibroblast into Endoderm Lineage. J. Biol. Chem. 292, 19122–19132. doi:10.1074/jbc.m117.812537
Cao, S., Yu, S., Li, D., Ye, J., Yang, X., Li, C., et al. (2018). Chromatin Accessibility Dynamics during Chemical Induction of Pluripotency. Cell. Stem Cell. 22, 529–542. e525. doi:10.1016/j.stem.2018.03.005
Carette, J. E., Pruszak, J., Varadarajan, M., Blomen, V. A., Gokhale, S., Camargo, F. D., et al. (2010). Generation of iPSCs from Cultured Human Malignant Cells. Blood 115, 4039–4042. doi:10.1182/blood-2009-07-231845
Carrió, E., Magli, A., Muñoz, M., Peinado, M. A., Perlingeiro, R., and Suelves, M. (2016). Muscle Cell Identity Requires Pax7-Mediated Lineage-specific DNA Demethylation. BMC Biol. 14, 30. doi:10.1186/s12915-016-0250-9
Cenik, B. K., and Shilatifard, A. (2021). COMPASS and SWI/SNF Complexes in Development and Disease. Nat. Rev. Genet. 22, 38–58. doi:10.1038/s41576-020-0278-0
Cerdá-Esteban, N., Naumann, H., Ruzittu, S., Mah, N., Pongrac, I. M., Cozzitorto, C., et al. (2017). Stepwise Reprogramming of Liver Cells to a Pancreas Progenitor State by the Transcriptional Regulator Tgif2. Nat. Commun. 8, 14127. doi:10.1038/ncomms14127
Chal, J., and Pourquié, O. (2017). Making Muscle: Skeletal Myogenesis In Vivo and In Vitro. Development 144, 2104–2122. doi:10.1242/dev.151035
Chen, J., Zhang, Z., Li, L., Chen, B.-C., Revyakin, A., Hajj, B., et al. (2014a). Single-molecule Dynamics of Enhanceosome Assembly in Embryonic Stem Cells. Cell. 156, 1274–1285. doi:10.1016/j.cell.2014.01.062
Chen, Y.-C., Ma, N.-X., Pei, Z.-F., Wu, Z., Do-Monte, F. H., Keefe, S., et al. (2020). A NeuroD1 AAV-Based Gene Therapy for Functional Brain Repair after Ischemic Injury through In Vivo Astrocyte-To-Neuron Conversion. Mol. Ther. 28, 217–234. doi:10.1016/j.ymthe.2019.09.003
Chen, Y.-J., Finkbeiner, S. R., Weinblatt, D., Emmett, M. J., Tameire, F., Yousefi, M., et al. (2014b). De Novo Formation of Insulin-Producing "Neo-β Cell Islets" from Intestinal Crypts. Cell. Rep. 6, 1046–1058. doi:10.1016/j.celrep.2014.02.013
Chen, Y., Lüttmann, F. F., Schoger, E., Schöler, H. R., Zelarayán, L. C., Kim, K.-P., et al. (2021). Reversible Reprogramming of Cardiomyocytes to a Fetal State Drives Heart Regeneration in Mice. Science 373, 1537–1540. doi:10.1126/science.abg5159
Cho, J., Kim, S., Lee, H., Rah, W., Cho, H. C., Kim, N. K., et al. (2021). Regeneration of Infarcted Mouse Hearts by Cardiovascular Tissue Formed via the Direct Reprogramming of Mouse Fibroblasts. Nat. Biomed. Eng. 5, 880–896. doi:10.1038/s41551-021-00783-0
Constantinides, P. G., Jones, P. A., and Gevers, W. (1977). Functional Striated Muscle Cells from Non-myoblast Precursors Following 5-azacytidine Treatment. Nature 267, 364–366. doi:10.1038/267364a0
Cusanovich, D. A., Hill, A. J., Aghamirzaie, D., Daza, R. M., Pliner, H. A., Berletch, J. B., et al. (2018). A Single-Cell Atlas of In Vivo Mammalian Chromatin Accessibility. Cell. 174, 1309–1324. doi:10.1016/j.cell.2018.06.052
Darabi, R., Arpke, R. W., Irion, S., Dimos, J. T., Grskovic, M., Kyba, M., et al. (2012). Human ES- and iPS-Derived Myogenic Progenitors Restore DYSTROPHIN and Improve Contractility upon Transplantation in Dystrophic Mice. Cell. Stem Cell. 10, 610–619. doi:10.1016/j.stem.2012.02.015
Darabi, R., Gehlbach, K., Bachoo, R. M., Kamath, S., Osawa, M., Kamm, K. E., et al. (2008). Functional Skeletal Muscle Regeneration from Differentiating Embryonic Stem Cells. Nat. Med. 14, 134–143. doi:10.1038/nm1705
Davis, R. L., Weintraub, H., and Lassar, A. B. (1987). Expression of a Single Transfected cDNA Converts Fibroblasts to Myoblasts. Cell. 51, 987–1000. doi:10.1016/0092-8674(87)90585-x
Fu, J.-D., and Srivastava, D. (2015). Direct Reprogramming of Fibroblasts into Cardiomyocytes for Cardiac Regenerative Medicine. Circ. J. 79, 245–254. doi:10.1253/circj.cj-14-1372
Fu, J.-D., Stone, N. R., Liu, L., Spencer, C. I., Qian, L., Hayashi, Y., et al. (2013). Direct Reprogramming of Human Fibroblasts toward a Cardiomyocyte-like State. Stem Cell. Rep. 1, 235–247. doi:10.1016/j.stemcr.2013.07.005
Furuyama, K., Chera, S., van Gurp, L., Oropeza, D., Ghila, L., Damond, N., et al. (2019). Diabetes Relief in Mice by Glucose-Sensing Insulin-Secreting Human α-cells. Nature 567, 43–48. doi:10.1038/s41586-019-0942-8
Gascón, S., Masserdotti, G., Russo, G. L., and Götz, M. (2017). Direct Neuronal Reprogramming: Achievements, Hurdles, and New Roads to Success. Cell. Stem Cell. 21, 18–34. doi:10.1016/j.stem.2017.06.011
Ge, J., Liu, K., Niu, W., Chen, M., Wang, M., Xue, Y., et al. (2018). Gold and Gold-Silver Alloy Nanoparticles Enhance the Myogenic Differentiation of Myoblasts through P38 MAPK Signaling Pathway and Promote In Vivo Skeletal Muscle Regeneration. Biomaterials 175, 19–29. doi:10.1016/j.biomaterials.2018.05.027
Goudenege, S., Lebel, C., Huot, N. B., Dufour, C., Fujii, I., Gekas, J., et al. (2012). Myoblasts Derived from Normal hESCs and Dystrophic hiPSCs Efficiently Fuse with Existing Muscle Fibers Following Transplantation. Mol. Ther. 20, 2153–2167. doi:10.1038/mt.2012.188
Grande, A., Sumiyoshi, K., López-Juárez, A., Howard, J., Sakthivel, B., Aronow, B., et al. (2013). Environmental Impact on Direct Neuronal Reprogramming In Vivo in the Adult Brain. Nat. Commun. 4, 2373. doi:10.1038/ncomms3373
Grath, A., and Dai, G. (2019). Direct Cell Reprogramming for Tissue Engineering and Regenerative Medicine. J. Biol. Eng. 13, 14. doi:10.1186/s13036-019-0144-9
Guan, J., Wang, G., Wang, J., Zhang, Z., Fu, Y., Cheng, L., et al. (2022). Chemical Reprogramming of Human Somatic Cells to Pluripotent Stem Cells. Nature 605, 325–331. doi:10.1038/s41586-022-04593-5
Guo, Z., Zhang, L., Wu, Z., Chen, Y., Wang, F., and Chen, G. (2014). In Vivo direct Reprogramming of Reactive Glial Cells into Functional Neurons after Brain Injury and in an Alzheimer's Disease Model. Cell. Stem Cell. 14, 188–202. doi:10.1016/j.stem.2013.12.001
Gurdon, J. B. (1962). The Developmental Capacity of Nuclei Taken from Intestinal Epithelium Cells of Feeding Tadpoles. J. Embryol. Exp. Morphol. 10, 622–640. doi:10.1242/dev.10.4.622
Ho, L., and Crabtree, G. R. (2010). Chromatin Remodelling during Development. Nature 463, 474–484. doi:10.1038/nature08911
Hodges, C., Kirkland, J. G., and Crabtree, G. R. (2016). The Many Roles of BAF (mSWI/SNF) and PBAF Complexes in Cancer. Cold Spring Harb. Perspect. Med. 6, a026930. doi:10.1101/cshperspect.a026930
Huang, P., He, Z., Ji, S., Sun, H., Xiang, D., Liu, C., et al. (2011). Induction of Functional Hepatocyte-like Cells from Mouse Fibroblasts by Defined Factors. Nature 475, 386–389. doi:10.1038/nature10116
Ieda, M., Fu, J.-D., Delgado-Olguin, P., Vedantham, V., Hayashi, Y., Bruneau, B. G., et al. (2010). Direct Reprogramming of Fibroblasts into Functional Cardiomyocytes by Defined Factors. Cell. 142, 375–386. doi:10.1016/j.cell.2010.07.002
Incitti, T., Magli, A., Darabi, R., Yuan, C., Lin, K., Arpke, R. W., et al. (2019). Pluripotent Stem Cell-Derived Myogenic Progenitors Remodel Their Molecular Signature upon In Vivo Engraftment. Proc. Natl. Acad. Sci. U.S.A. 116, 4346–4351. doi:10.1073/pnas.1808303116
Jayawardena, T. M., Egemnazarov, B., Finch, E. A., Zhang, L., Payne, J. A., Pandya, K., et al. (2012). MicroRNA-mediated In Vitro and In Vivo Direct Reprogramming of Cardiac Fibroblasts to Cardiomyocytes. Circ. Res. 110, 1465–1473. doi:10.1161/circresaha.112.269035
Kang, S. H., Fukaya, M., Yang, J. K., Rothstein, J. D., and Bergles, D. E. (2010). NG2+ CNS Glial Progenitors Remain Committed to the Oligodendrocyte Lineage in Postnatal Life and Following Neurodegeneration. Neuron 68, 668–681. doi:10.1016/j.neuron.2010.09.009
Kang, X., and Li, C. (2020). Landscape Inferred from Gene Expression Data Governs Pluripotency in Embryonic Stem Cells. Comput. Struct. Biotechnol. J. 18, 366–374. doi:10.1016/j.csbj.2020.02.004
Kuang, J., Zhai, Z., Li, P., Shi, R., Guo, W., Yao, Y., et al. (2021). SS18 Regulates Pluripotent-Somatic Transition through Phase Separation. Nat. Commun. 12, 4090. doi:10.1038/s41467-021-24373-5
Laflamme, M. A., and Murry, C. E. (2011). Heart Regeneration. Nature 473, 326–335. doi:10.1038/nature10147
Lalit, P. A., Salick, M. R., Nelson, D. O., Squirrell, J. M., Shafer, C. M., Patel, N. G., et al. (2016). Lineage Reprogramming of Fibroblasts into Proliferative Induced Cardiac Progenitor Cells by Defined Factors. Cell. Stem Cell. 18, 354–367. doi:10.1016/j.stem.2015.12.001
Laugwitz, K.-L., Moretti, A., Lam, J., Gruber, P., Chen, Y., Woodard, S., et al. (2005). Postnatal Isl1+ Cardioblasts Enter Fully Differentiated Cardiomyocyte Lineages. Nature 433, 647–653. doi:10.1038/nature03215
Letort, G., Montagud, A., Stoll, G., Heiland, R., Barillot, E., Macklin, P., et al. (2019). PhysiBoSS: a Multi-Scale Agent-Based Modelling Framework Integrating Physical Dimension and Cell Signalling. Bioinformatics 35, 1188–1196. doi:10.1093/bioinformatics/bty766
Li, D., Liu, J., Yang, X., Zhou, C., Guo, J., Wu, C., et al. (2017a). Chromatin Accessibility Dynamics during iPSC Reprogramming. Cell. Stem Cell. 21, 819–833. e816. doi:10.1016/j.stem.2017.10.012
Li, Q., Hutchins, A. P., Chen, Y., Li, S., Shan, Y., Liao, B., et al. (2017b). A Sequential EMT-MET Mechanism Drives the Differentiation of Human Embryonic Stem Cells towards Hepatocytes. Nat. Commun. 8, 15166. doi:10.1038/ncomms15166
Li, R., Liang, J., Ni, S., Zhou, T., Qing, X., Li, H., et al. (2010). A Mesenchymal-To-Epithelial Transition Initiates and Is Required for the Nuclear Reprogramming of Mouse Fibroblasts. Cell. Stem Cell. 7, 51–63. doi:10.1016/j.stem.2010.04.014
Li, W., Cavelti-Weder, C., Zhang, Y., Clement, K., Donovan, S., Gonzalez, G., et al. (2014). Long-term Persistence and Development of Induced Pancreatic Beta Cells Generated by Lineage Conversion of Acinar Cells. Nat. Biotechnol. 32, 1223–1230. doi:10.1038/nbt.3082
Li, X., Liu, D., Ma, Y., Du, X., Jing, J., Wang, L., et al. (2017c). Direct Reprogramming of Fibroblasts via a Chemically Induced XEN-like State. Cell. Stem Cell. 21, 264–273. doi:10.1016/j.stem.2017.05.019
Li, Y., Li, N., Ge, J., Xue, Y., Niu, W., Chen, M., et al. (2019). Biodegradable Thermal Imaging-Tracked Ultralong Nanowire-Reinforced Conductive Nanocomposites Elastomers with Intrinsical Efficient Antibacterial and Anticancer Activity for Enhanced Biomedical Application Potential. Biomaterials 201, 68–76. doi:10.1016/j.biomaterials.2019.02.013
Liu, J., Han, Q., Peng, T., Peng, M., Wei, B., Li, D., et al. (2015a). The Oncogene C-Jun Impedes Somatic Cell Reprogramming. Nat. Cell. Biol. 17, 856–867. doi:10.1038/ncb3193
Liu, M., Wang, Y., Nie, Z., Gai, J., Bhat, J. A., Kong, J., et al. (2020). Double Mutation of Two Homologous Genes YL1 and YL2 Results in a Leaf Yellowing Phenotype in Soybean [Glycine Max (L.) Merr]. Plant Mol. Biol. 103, 527–543. doi:10.1007/s11103-020-01008-9
Liu, Y., Cheng, H., Gao, S., Lu, X., He, F., Hu, L., et al. (2014). Reprogramming of MLL-AF9 Leukemia Cells into Pluripotent Stem Cells. Leukemia 28, 1071–1080. doi:10.1038/leu.2013.304
Liu, Y., Miao, Q., Yuan, J., Han, S., Zhang, P., Li, S., et al. (2015b). Ascl1 Converts Dorsal Midbrain Astrocytes into Functional Neurons In Vivo. J. Neurosci. 35, 9336–9355. doi:10.1523/jneurosci.3975-14.2015
Magnusson, J. P., Göritz, C., Tatarishvili, J., Dias, D. O., Smith, E. M. K., Lindvall, O., et al. (2014). A Latent Neurogenic Program in Astrocytes Regulated by Notch Signaling in the Mouse. Science 346, 237–241. doi:10.1126/science.346.6206.237
Mathieu, J., Zhang, Z., Zhou, W., Wang, A. J., Heddleston, J. M., Pinna, C. M. A., et al. (2011). HIF Induces Human Embryonic Stem Cell Markers in Cancer Cells. Cancer Res. 71, 4640–4652. doi:10.1158/0008-5472.can-10-3320
Matsuda, T., Irie, T., Katsurabayashi, S., Hayashi, Y., Nagai, T., Hamazaki, N., et al. (2019). Pioneer Factor NeuroD1 Rearranges Transcriptional and Epigenetic Profiles to Execute Microglia-Neuron Conversion. Neuron 101, 472–485. doi:10.1016/j.neuron.2018.12.010
Mattugini, N., Bocchi, R., Scheuss, V., Russo, G. L., Torper, O., Lao, C. L., et al. (2019). Inducing Different Neuronal Subtypes from Astrocytes in the Injured Mouse Cerebral Cortex. Neuron 103, 1086–1095. doi:10.1016/j.neuron.2019.08.009
Miyoshi, N., Ishii, H., Nagai, K.-i., Hoshino, H., Mimori, K., Tanaka, F., et al. (2010). Defined Factors Induce Reprogramming of Gastrointestinal Cancer Cells. Proc. Natl. Acad. Sci. U.S.A. 107, 40–45. doi:10.1073/pnas.0912407107
Murry, C. E., Reinecke, H., and Pabon, L. M. (2006). Regeneration Gaps. J. Am. Coll. Cardiol. 47, 1777–1785. doi:10.1016/j.jacc.2006.02.002
Nam, Y.-J., Song, K., Luo, X., Daniel, E., Lambeth, K., West, K., et al. (2013). Reprogramming of Human Fibroblasts toward a Cardiac Fate. Proc. Natl. Acad. Sci. U.S.A. 110, 5588–5593. doi:10.1073/pnas.1301019110
Niu, W., Zang, T., Zou, Y., Fang, S., Smith, D. K., Bachoo, R., et al. (2013). In Vivo reprogramming of Astrocytes to Neuroblasts in the Adult Brain. Nat. Cell. Biol. 15, 1164–1175. doi:10.1038/ncb2843
Olivetti, G., Melissari, M., Capasso, J. M., and Anversa, P. (1991). Cardiomyopathy of the Aging Human Heart. Myocyte Loss and Reactive Cellular Hypertrophy. Circ. Res. 68, 1560–1568. doi:10.1161/01.res.68.6.1560
Pipeleers, D., Keymeulen, B., Chatenoud, L., Hendrieckx, C., Ling, Z., Mathieu, C., et al. (2002). A View on Beta Cell Transplantation in Diabetes. Ann. N. Y. Acad. Sci. 958, 69–76. doi:10.1111/j.1749-6632.2002.tb02948.x
Qian, H., Kang, X., Hu, J., Zhang, D., Liang, Z., Meng, F., et al. (2020). Reversing a Model of Parkinson's Disease with In Situ Converted Nigral Neurons. Nature 582, 550–556. doi:10.1038/s41586-020-2388-4
Qian, L., Huang, Y., Spencer, C. I., Foley, A., Vedantham, V., Liu, L., et al. (2012). In Vivo reprogramming of Murine Cardiac Fibroblasts into Induced Cardiomyocytes. Nature 485, 593–598. doi:10.1038/nature11044
Qin, H., Zhao, A., and Fu, X. (2017). Small Molecules for Reprogramming and Transdifferentiation. Cell. Mol. Life Sci. 74, 3553–3575. doi:10.1007/s00018-017-2586-x
Quattrocelli, M., Swinnen, M., Giacomazzi, G., Camps, J., Barthélemy, I., Ceccarelli, G., et al. (2015). Mesodermal iPSC-Derived Progenitor Cells Functionally Regenerate Cardiac and Skeletal Muscle. J. Clin. Investig. 125, 4463–4482. doi:10.1172/jci82735
Rao, Y., Du, S., Yang, B., Wang, Y., Li, Y., Li, R., et al. (2021). NeuroD1 Induces Microglial Apoptosis and Cannot Induce Microglia-To-Neuron Cross-Lineage Reprogramming. Neuron 109, 4094–4108. e4095. doi:10.1016/j.neuron.2021.11.008
Rivetti di Val Cervo, P., Romanov, R. A., Spigolon, G., Masini, D., Martín-Montañez, E., Toledo, E. M., et al. (2017). Induction of Functional Dopamine Neurons from Human Astrocytes In Vitro and Mouse Astrocytes in a Parkinson's Disease Model. Nat. Biotechnol. 35, 444–452. doi:10.1038/nbt.3835
Roger, V. L. (2021). Epidemiology of Heart Failure. Circ. Res. 128, 1421–1434. doi:10.1161/circresaha.121.318172
Russo, G. L., Sonsalla, G., Natarajan, P., Breunig, C. T., Bulli, G., Merl-Pham, J., et al. (2021). CRISPR-mediated Induction of Neuron-Enriched Mitochondrial Proteins Boosts Direct Glia-To-Neuron Conversion. Cell. Stem Cell. 28, 524–534. doi:10.1016/j.stem.2020.10.015
Saho, S., Satoh, H., Kondo, E., Inoue, Y., Yamauchi, A., Murata, H., et al. (2016). Active Secretion of Dimerized S100A11 Induced by the Peroxisome in Mesothelioma Cells. Cancer Microenviron. 9, 93–105. doi:10.1007/s12307-016-0185-2
Sancho, R., Gruber, R., Gu, G., and Behrens, A. (2014). Loss of Fbw7 Reprograms Adult Pancreatic Ductal Cells into α, δ, and β Cells. Cell. Stem Cell. 15, 139–153. doi:10.1016/j.stem.2014.06.019
Savarese, G., Stolfo, D., Sinagra, G., and Lund, L. H. (2022). Heart Failure with Mid-range or Mildly Reduced Ejection Fraction. Nat. Rev. Cardiol. 19, 100–116. doi:10.1038/s41569-021-00605-5
Sekiya, S., and Suzuki, A. (2011). Direct Conversion of Mouse Fibroblasts to Hepatocyte-like Cells by Defined Factors. Nature 475, 390–393. doi:10.1038/nature10263
Sinha, S., Biswas, M., Chatterjee, S. S., Kumar, S., and Sengupta, A. (2020). Pbrm1 Steers Mesenchymal Stromal Cell Osteolineage Differentiation by Integrating PBAF-dependent Chromatin Remodeling and BMP/TGF-β Signaling. Cell. Rep. 31, 107570. doi:10.1016/j.celrep.2020.107570
Song, K., Nam, Y.-J., Luo, X., Qi, X., Tan, W., Huang, G. N., et al. (2012). Heart Repair by Reprogramming Non-myocytes with Cardiac Transcription Factors. Nature 485, 599–604. doi:10.1038/nature11139
Tai, W., Wu, W., Wang, L.-L., Ni, H., Chen, C., Yang, J., et al. (2021). In Vivo reprogramming of NG2 Glia Enables Adult Neurogenesis and Functional Recovery Following Spinal Cord Injury. Cell. Stem Cell. 28, 923–937. doi:10.1016/j.stem.2021.02.009
Takahashi, K., Tanabe, K., Ohnuki, M., Narita, M., Ichisaka, T., Tomoda, K., et al. (2007). Induction of Pluripotent Stem Cells from Adult Human Fibroblasts by Defined Factors. Cell. 131, 861–872. doi:10.1016/j.cell.2007.11.019
Takahashi, K., and Yamanaka, S. (2006). Induction of Pluripotent Stem Cells from Mouse Embryonic and Adult Fibroblast Cultures by Defined Factors. Cell. 126, 663–676. doi:10.1016/j.cell.2006.07.024
Takeuchi, J. K., and Bruneau, B. G. (2009). Directed Transdifferentiation of Mouse Mesoderm to Heart Tissue by Defined Factors. Nature 459, 708–711. doi:10.1038/nature08039
Talchai, C., Xuan, S., Kitamura, T., DePinho, R. A., and Accili, D. (2012). Generation of Functional Insulin-Producing Cells in the Gut by Foxo1 Ablation. Nat. Genet. 44, 406–412. S401. doi:10.1038/ng.2215
Tang, S., Zhang, M., Zeng, S., Huang, Y., Qin, M., Nasri, U., et al. (2020). Reversal of Autoimmunity by Mixed Chimerism Enables Reactivation of β Cells and Transdifferentiation of α Cells in Diabetic NOD Mice. Proc. Natl. Acad. Sci. U.S.A. 117, 31219–31230. doi:10.1073/pnas.2012389117
Torper, O., Ottosson, D. R., Pereira, M., Lau, S., Cardoso, T., Grealish, S., et al. (2015). In Vivo Reprogramming of Striatal NG2 Glia into Functional Neurons that Integrate into Local Host Circuitry. Cell. Rep. 12, 474–481. doi:10.1016/j.celrep.2015.06.040
Utikal, J., Maherali, N., Kulalert, W., and Hochedlinger, K. (2009). Sox2 Is Dispensable for the Reprogramming of Melanocytes and Melanoma Cells into Induced Pluripotent Stem Cells. J. Cell. Sci. 122, 3502–3510. doi:10.1242/jcs.054783
Vegas, A. J., Veiseh, O., Gürtler, M., Millman, J. R., Pagliuca, F. W., Bader, A. R., et al. (2016). Long-term Glycemic Control Using Polymer-Encapsulated Human Stem Cell-Derived Beta Cells in Immune-Competent Mice. Nat. Med. 22, 306–311. doi:10.1038/nm.4030
Vierbuchen, T., Ostermeier, A., Pang, Z. P., Kokubu, Y., Südhof, T. C., and Wernig, M. (2010). Direct Conversion of Fibroblasts to Functional Neurons by Defined Factors. Nature 463, 1035–1041. doi:10.1038/nature08797
Wada, R., Muraoka, N., Inagawa, K., Yamakawa, H., Miyamoto, K., Sadahiro, T., et al. (2013). Induction of Human Cardiomyocyte-like Cells from Fibroblasts by Defined Factors. Proc. Natl. Acad. Sci. U.S.A. 110, 12667–12672. doi:10.1073/pnas.1304053110
Wang, H., Yang, Y., Liu, J., and Qian, L. (2021a). Direct Cell Reprogramming: Approaches, Mechanisms and Progress. Nat. Rev. Mol. Cell Biol. 22, 410–424. doi:10.1038/s41580-021-00335-z
Wang, J., Gu, S., Liu, F., Chen, Z., Xu, H., Liu, Z., et al. (2022). Reprogramming of Fibroblasts into Expandable Cardiovascular Progenitor Cells via Small Molecules in Xeno-free Conditions. Nat. Biomed. Eng. 6, 403–420. doi:10.1038/s41551-022-00865-7
Wang, L.-L., Serrano, C., Zhong, X., Ma, S., Zou, Y., and Zhang, C.-L. (2021b). Revisiting Astrocyte to Neuron Conversion with Lineage Tracing In Vivo. Cell 184, 5465–5481. doi:10.1016/j.cell.2021.09.005
Wang, Y., Dorrell, C., Naugler, W. E., Heskett, M., Spellman, P., Li, B., et al. (2018). Long-Term Correction of Diabetes in Mice by In Vivo Reprogramming of Pancreatic Ducts. Mol. Ther. 26, 1327–1342. doi:10.1016/j.ymthe.2018.02.014
Wherrett, D. K., Bundy, B., Becker, D. J., DiMeglio, L. A., Gitelman, S. E., Goland, R., et al. (2011). Antigen-based Therapy with Glutamic Acid Decarboxylase (GAD) Vaccine in Patients with Recent-Onset Type 1 Diabetes: a Randomised Double-Blind Trial. Lancet 378, 319–327. doi:10.1016/s0140-6736(11)60895-7
Wu, Z., Parry, M., Hou, X.-Y., Liu, M.-H., Wang, H., Cain, R., et al. (2020). Gene Therapy Conversion of Striatal Astrocytes into GABAergic Neurons in Mouse Models of Huntington's Disease. Nat. Commun. 11, 1105. doi:10.1038/s41467-020-14855-3
Xiao, X., Guo, P., Shiota, C., Zhang, T., Coudriet, G. M., Fischbach, S., et al. (2018). Endogenous Reprogramming of Alpha Cells into Beta Cells, Induced by Viral Gene Therapy, Reverses Autoimmune Diabetes. Cell Stem Cell 22, 78–90. doi:10.1016/j.stem.2017.11.020
Xue, Y., Ouyang, K., Huang, J., Zhou, Y., Ouyang, H., Li, H., et al. (2013). Direct Conversion of Fibroblasts to Neurons by Reprogramming PTB-Regulated microRNA Circuits. Cell 152, 82–96. doi:10.1016/j.cell.2012.11.045
Yagi, M., Ji, F., Charlton, J., Cristea, S., Messemer, K., Horwitz, N., et al. (2021). Dissecting Dual Roles of MyoD during Lineage Conversion to Mature Myocytes and Myogenic Stem Cells. Genes. Dev. 35, 1209–1228. doi:10.1101/gad.348678.121
Yamakawa, H., and Ieda, M. (2021). Cardiac Regeneration by Direct Reprogramming in This Decade and beyond. Inflamm. Regen. 41, 20. doi:10.1186/s41232-021-00168-5
Young, C. S., Hicks, M. R., Ermolova, N. V., Nakano, H., Jan, M., Younesi, S., et al. (2016). A Single CRISPR-Cas9 Deletion Strategy that Targets the Majority of DMD Patients Restores Dystrophin Function in hiPSC-Derived Muscle Cells. Cell. Stem Cell. 18, 533–540. doi:10.1016/j.stem.2016.01.021
Yu, C., Liu, K., Tang, S., and Ding, S. (2014). Chemical Approaches to Cell Reprogramming. Curr. Opin. Genet. Dev. 28, 50–56. doi:10.1016/j.gde.2014.09.006
Zaret, K. S., and Grompe, M. (2008). Generation and Regeneration of Cells of the Liver and Pancreas. Science 322, 1490–1494. doi:10.1126/science.1161431
Zhang, K., Hocker, J. D., Miller, M., Hou, X., Chiou, J., Poirion, O. B., et al. (2021). A Single-Cell Atlas of Chromatin Accessibility in the Human Genome. Cell. 184, 5985–6001. e5919. doi:10.1016/j.cell.2021.10.024
Zhang, L., Yin, J.-C., Yeh, H., Ma, N.-X., Lee, G., Chen, X. A., et al. (2015). Small Molecules Efficiently Reprogram Human Astroglial Cells into Functional Neurons. Cell. Stem Cell. 17, 735–747. doi:10.1016/j.stem.2015.09.012
Zhang, W., Chronis, C., Chen, X., Zhang, H., Spalinskas, R., Pardo, M., et al. (2019). The BAF and PRC2 Complex Subunits Dpf2 and Eed Antagonistically Converge on Tbx3 to Control ESC Differentiation. Cell. Stem Cell. 24, 138–152. e138. doi:10.1016/j.stem.2018.12.001
Zhang, X., Cruz, F. D., Terry, M., Remotti, F., and Matushansky, I. (2013). Terminal Differentiation and Loss of Tumorigenicity of Human Cancers via Pluripotency-Based Reprogramming. Oncogene 32, 2249–2260. doi:10.1038/onc.2012.237
Zhang, Y., Cao, N., Huang, Y., Spencer, C. I., Fu, J.-d., Yu, C., et al. (2016). Expandable Cardiovascular Progenitor Cells Reprogrammed from Fibroblasts. Cell. Stem Cell. 18, 368–381. doi:10.1016/j.stem.2016.02.001
Zhao, Y., Zhao, T., Guan, J., Zhang, X., Fu, Y., Ye, J., et al. (2015). A XEN-like State Bridges Somatic Cells to Pluripotency during Chemical Reprogramming. Cell. 163, 1678–1691. doi:10.1016/j.cell.2015.11.017
Zhou, H., Su, J., Hu, X., Zhou, C., Li, H., Chen, Z., et al. (2020). Glia-to-Neuron Conversion by CRISPR-CasRx Alleviates Symptoms of Neurological Disease in Mice. Cell. 181, 590–603. doi:10.1016/j.cell.2020.03.024
Zhou, L., Ge, J., Wang, M., Chen, M., Cheng, W., Ji, W., et al. (2021). Injectable Muscle-Adhesive Antioxidant Conductive Photothermal Bioactive Nanomatrix for Efficiently Promoting Full-Thickness Skeletal Muscle Regeneration. Bioact. Mater. 6, 1605–1617. doi:10.1016/j.bioactmat.2020.11.005
Zhou, Q., Brown, J., Kanarek, A., Rajagopal, J., and Melton, D. A. (2008). In Vivo reprogramming of Adult Pancreatic Exocrine Cells to β-cells. Nature 455, 627–632. doi:10.1038/nature07314
Keywords: cell reprogramming, functional cells, cell replacement therapy, chromatin accessibility dynamics, transdifferentiation
Citation: Kuang J, Huang T and Pei D (2022) The Art of Reprogramming for Regenerative Medicine. Front. Cell Dev. Biol. 10:927555. doi: 10.3389/fcell.2022.927555
Received: 24 April 2022; Accepted: 08 June 2022;
Published: 30 June 2022.
Edited by:
Cressida Lyon, University of Bath, United KingdomReviewed by:
Jian Yang, Tongji University, ChinaPei-Shan Hou, National Yang Ming Chiao Tung University, Taipei
Copyright © 2022 Kuang, Huang and Pei. This is an open-access article distributed under the terms of the Creative Commons Attribution License (CC BY). The use, distribution or reproduction in other forums is permitted, provided the original author(s) and the copyright owner(s) are credited and that the original publication in this journal is cited, in accordance with accepted academic practice. No use, distribution or reproduction is permitted which does not comply with these terms.
*Correspondence: Duanqing Pei, cGVpZHVhbnFpbmdAd2VzdGxha2UuZWR1LmNu