- 1Department of Immunology & Microbiology, University of Colorado School of Medicine, Aurora, CO, United States
- 2Veterans Affairs Eastern Colorado Health Care System, Denver, CO, United States
The metal ion manganese (Mn2+) is equally coveted by hosts and bacterial pathogens. The host restricts Mn2+ in the gastrointestinal tract and Salmonella-containing vacuoles, as part of a process generally known as nutritional immunity. Salmonella enterica serovar Typhimurium counteract Mn2+ limitation using a plethora of metal importers, whose expression is under elaborate transcriptional and posttranscriptional control. Mn2+ serves as cofactor for a variety of enzymes involved in antioxidant defense or central metabolism. Because of its thermodynamic stability and low reactivity, bacterial pathogens may favor Mn2+-cofactored metalloenzymes during periods of oxidative stress. This divalent metal catalyzes metabolic flow through lower glycolysis, reductive tricarboxylic acid and the pentose phosphate pathway, thereby providing energetic, redox and biosynthetic outputs associated with the resistance of Salmonella to reactive oxygen species generated in the respiratory burst of professional phagocytic cells. Combined, the oxyradical-detoxifying properties of Mn2+ together with the ability of this divalent metal cation to support central metabolism help Salmonella colonize the mammalian gut and establish systemic infections.
Introduction
A rich microbiome, cellular and abiotic mucosal barriers, as well as cellular and humoral effectors of the innate and adaptive immune system may limit the colonization, growth and spread of pathogenic bacteria in mammalian hosts (Rosales and Uribe-Querol, 2017; Levinson et al., 2018; Uribe-Querol and Rosales, 2020). Hosts use metal transporters, calprotectins, siderocalins and a variety of other metal-sequestering proteins to limit the availability of Mg2+, Fe2+, Mn2+ and Zn2+ ions from bacteria, a phenomenon known as nutritional immunity (Bellamy, 2003; Loomis et al., 2014; Hennigar and McClung, 2016; Wang et al., 2018; Cunrath and Bumann, 2019; Monteith and Skaar, 2021). Bacteria display sophisticated transport systems that counteract metal restrictions imposed by hosts (Porcheron et al., 2013; Chandrangsu et al., 2017). Among the bioactive metal ions, Mn2+ plays a salient role in bacterial physiology and the adaptation of prokaryotic cells to stress (Papp-Wallace and Maguire, 2006). Mn2+ is a cofactor of enzymes involved in carbon and nucleotide metabolism, DNA replication, and protein translation (Lovley and Phillips, 1988; Martin and Imlay, 2011; Culotta and Daly, 2013; Torrents, 2014; Kaur et al., 2017; Tong et al., 2017; Daniel et al., 2018; Li and Yang, 2018; Hutfilz et al., 2019). Mn2+ is also a cofactor of superoxide dismutase (SOD) and catalase (KatN) family members, and this divalent metal is utilized by carbon utilization enzymes such as phosphoglycerate mutase (PGM) and fructose-1,6-bisphosphate phosphatase, or envelope stress phosphatases (Shi et al., 2001; Miriyala et al., 2012; Whittaker, 2012; Radin et al., 2019). The MntR repressor coordinates a Mn2+ ion and the ferric uptake regulator (Fur) can bind to Fe2+ or Mn2+ (Hiemstra et al., 1999; Kehres et al., 2002a; Ikeda et al., 2005; Waters, 2020).
Salmonella enterica serovar Typhimurium is a facultative intracellular pathogen associated with intestinal and invasive diseases in humans and animals (Galan, 2021). The success of Salmonella as a pathogen can be directly ascribed to its capacity to overcome colonization resistance by resident gut microbiota, invade epithelial cells, and establish intracellular infections within host enterocytes and macrophages (Winter and Bäumler, 2011; Lorkowski et al., 2014; Lhocine et al., 2015; Aljahdali et al., 2020; Jiang et al., 2021; Powers et al., 2021). During their associations with host cells, Salmonella are exposed to acid pH, oxidative and nitrosative stress, and nutritional deprivation (Fang et al., 2016). A variety of adaptive mechanisms are used by Salmonella to fight the hostile host environment (Bernal-Bayard and Ramos-Morales, 2018; Tanner and Kingsley, 2018; Gogoi et al., 2019), and a few reviews have examined the contribution of Mn2+ in Salmonella pathogenesis (Kehres and Maguire, 2003; Papp-Wallace and Maguire, 2006; Osman and Cavet, 2011). Despite these advances, many unanswered questions and occasional contradictory findings warrant a review of our current understanding of Mn2+-mediated stress responses in Salmonella pathogenesis. In this review, we discuss the adaptive mechanisms that allow Salmonella to compete with the host for Mn2+, and present ways by which this divalent metal contributes to metabolic programs associated with resistance of Salmonella to oxidative and nitrosative stress.
Manganese Limitation in the Host
Hosts sequester transition metals in their fight against pathogenic organisms (Hennigar and McClung, 2016). Metal sequestration is mediated by calprotectin, lipochalin 2, metallothioneins, ferritin and transport systems including NRAMP1. Mn2+ limitation is mostly mediated by calprotectin and NRAMP1.
Calprotectin
The host protein calprotectin, a member of the calcium-binding S100 family, limits Mn2+ from extracellular Salmonella. Human calprotectin is a heterooligomer with a dedicated Zn2+-binding site-1 and a versatile Mn2+-, Fe2+-, Zn2+- and Ni2+-binding site-2 (Hayden et al., 2013; Zygiel and Nolan, 2018). Calprotectin is secreted by infiltrating neutrophils at sites of inflammation, reaching extracellular concentrations of about 40 μM, but is also expressed by epithelial cells and keratinocytes (Johne et al., 1997; Zygiel and Nolan, 2018; Jukic et al., 2021). Calprotectin limits metal bioavailability from bacteria, thus inhibiting bacterial growth, and its expression in epithelial cells diminishes binding of Salmonella to host cells (Nisapakultorn et al., 2001). Infection of gut mucosa by Salmonella attracts neutrophils, which secrete calprotectin in extracellular traps (Urban et al., 2009; Patel and McCormick, 2014). Fecal calprotectin levels in diarrheic children correlate with the severity of bacterial infection, and the concentration of calprotectin is increased in plasma during acute salmonellosis (Chen et al., 2012; De Jong et al., 2015). The acidic pH typical of infectious sites disrupts the tetramerization of calprotectin, not only attenuating its capacity to bind Mn2+ but also impairing its growth-inhibiting properties (Menkin, 1956; Rosen and Nolan, 2020). Although calprotectin inhibits Salmonella growth in vitro, its effectiveness in the intestinal lumen is severely limited by Salmonella’s Zn2+ and Mn2+ metal transporters (Liu et al., 2012; De Jong et al., 2015; Diaz-Ochoa et al., 2016).
NRAMP1
The integral membrane protein NRAMP1, which is also known as SLC11A1, transports divalent transition metals such as Fe2+, Mn2+, Co2+ and Mg2+ (Canonne-Hergaux et al., 1999; Forbes and Gros, 2003; Cellier et al., 2007; Cunrath and Bumann, 2019). The expression of NRAMP1 is restricted to lysosomal compartments of monocytes and macrophages, whereas the highly homologous NRAMP2 protein is widely distributed on most cells (Vidal et al., 1995; Gunshin et al., 1997). The expression of NRAMP1 is maximal at late stages in the maturation of phagosomes (Gruenheid et al., 1997). NRAMP1 can import or efflux metals into the phagosome (Atkinson and Barton, 1999; Kuhn et al., 1999; Zwilling et al., 1999; Jabado et al., 2000), although recent structural studies have suggested a strong unidirectional efflux movement under physiological conditions (Bozzi and Gaudet, 2021). The directionality of NRAMP1-mediated ion transport is dependent on pH (Goswami et al., 2001). Although the affinity of human or mouse NRAMP1 to Mn2+ has not been determined, a homologous transporter from Arabidopsis has a Km of 28 nM, (i.e., about 4-fold higher than the affinity of the Salmonella transporters MntH and SitABCD for Mn2+) (Kehres et al., 2000; Kehres et al., 2002b; Cailliatte et al., 2010).
Nutritional immunity imposed by NRAMP1 is a significant defense determinant against Salmonella as vividly illustrated by the high resistance of Sv129S6 or C3H/HeN mice carrying an intact nramp1 locus and the propensity of BALB/c or C57BL/6 mice bearing the mutant allele nramp1G169D to develop severe Salmonella infections (Brown et al., 2013; Wendy P.; Loomis et al., 2014). Recent work argued that NRAMP1 contributes to Salmonella pathogenesis by depriving phagosomes of Mg2+ (Cunrath and Bumann, 2019). However, the latter model appears to be in conflict with the observation that the attenuation of Salmonella bearing mutations in the Mn2+ transport system SitABCD or the Mn2+-dependent SpoT enzyme are contingent on the expression of a functional NRAMP1 (see below). More investigations are needed to definitively identify the metal specificity of NRAMP1 in different tissues and different times in the course of the Salmonella infection.
Mn2+ Import Promotes Salmonella Pathogenesis
Entry of Mn2+ into the periplasmic space is mostly mediated by non-specific porins on the outer membrane, whereas transporters in the cytoplasmic membrane actively influx this divalent metal cation into the bacterial cytoplasm (Figure 1A). MntH, a proton-dependent NRAMP1 homolog, actively imports Mn2+ with a Km of 0.1 μM (Kehres et al., 2000). Salmonella upregulate MntH expression in response to both low Mn2+ concentrations and oxidative stress (Kehres et al., 2002a; Cunrath and Palmer, 2021). MntH mutant Salmonella grow poorly in mouse macrophages, but replicate rather well in mice (Boyer et al., 2002; Zaharik et al., 2004; Diaz-Ochoa et al., 2016). The slight attenuation of mntH deficient Salmonella suggests the existence of redundant transport systems. Genetic analyses revealed the presence of a Mn2+ responsive element in the promoter of the sitABCD operon that is encoded within the Salmonella pathogenicity island-1 gene cluster (Janakiraman and Slauch, 2000; Kehres et al., 2002b). The SitABCD transporter is a typical ABC transporter consisting of a periplasmic-binding protein SitA, an ATP-binding protein SitB and two integral membrane permeases, SitC and SitD. Initially, the SitABCD transporter was thought to function as a Fe2+ transport system, as this locus is regulated by Fur under iron-limiting conditions (Janakiraman and Slauch, 2000; Ikeda et al., 2005). However, metal ion uptake studies have shown that SitABCD mediates influx of Mn2+ with an apparent affinity of 0.1 μM, transporting Fe2+ with 30–100 times lower efficiency (Kehres et al., 2002b). A third transporter with broad cation specificity, ZupT, has also been implicated in Mn2+ transport in Salmonella during nitrosative stress (Yousuf et al., 2020).
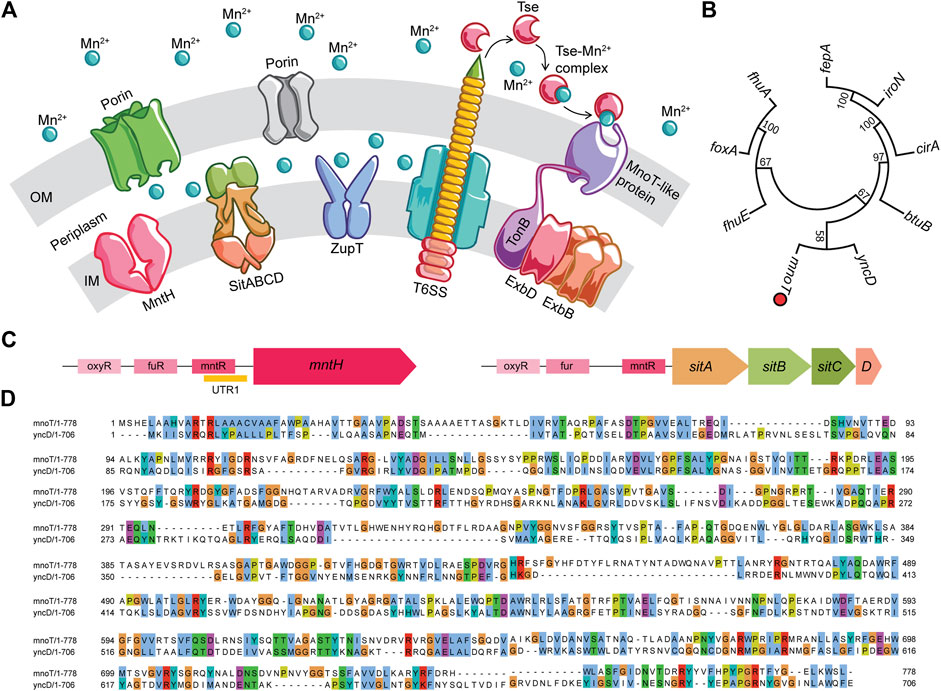
FIGURE 1. Manganese transport systems in Salmonella. (A) While the outer membrane is permeable to Mn2+ ions, the inner membrane imports this metal ion via specific and non-specific transporters like MntH, SitABCD and ZupT. A type VI secretion system dependent Mn2+ acquisition mechanism observed in pathogens such as Burkholderia, Vibrio, and Yersinia is proposed. (B) Neighbor-joining tree of MnoT-like proteins identified in Salmonella genome by Pattern Hit Initiated BLAST. (C) Genetic organization of mnTH and sitABCD operons with regulatory elements in the promoter regions. (D) Clustal alignment of Burkholderia MnoT (WP_171466016) and Salmonella YncD (ACY88391.1) proteins by Clustal Omega aligner. The alignment results were extracted and reformatted in MView command line utility. All protein identities were normalized by aligned length and the residues are colored using the default built-in colormap.
Low Mn2+ concentrations activate the expression of MntH and SitABCD (Kehres and Maguire, 2003; Chandrangsu et al., 2017; Bosma et al., 2021). The mntH gene is repressed by both MntR and Fur in response to high Mn2+ and iron replete conditions, respectively (Kehres et al., 2000; Kehres et al., 2002a; Troxell et al., 2011; Powers et al., 2021). Expression of mntH is also induced by peroxide via H2O2-sensing OxyR regulatory protein (Kehres et al., 2002a) (Figure 1C). The persistence of Mn2+-dependent repression of mntH in ΔmntR Salmonella points to the existence of MntR-independent control. Analysis of the mntH 5′ UTR identified an Mn2+-sensing riboswitch that forms a Rho-independent terminator (Shi et al., 2014; Scull et al., 2020) (Figure 1C). The presence of several transcriptional breakpoints suggests dynamic expression of MntH in diverse host niches. MntR, Fur and OxyR elements are also present in the sitABCD promoter (Ikeda et al., 2005). The sitABCD operon does not contain, however, a riboswitch-like sequence at the 5′ UTR. Despite the similarities in transcriptional control and their identical affinity for Mn2+, MntH and SitABCD have specialized functions. MntH primarily transports Mn2+ at acidic pH, whereas SitABCD preferentially works at alkaline pH (Kehres et al., 2002b), suggesting that Salmonella may preferentially utilize MntH or SitABCD at different anatomical locations or times during infection (Ikeda et al., 2005).
mntH mutant Salmonella display little attenuation, whereas sitABCD mutants are attenuated in systemic models of infection (Janakiraman and Slauch, 2000; Boyer et al., 2002; Zaharik et al., 2004). Attenuation of sitABCD mutant Salmonella is contingent on the presence of host NRAMP1 (W. P. Loomis et al., 2014; Zaharik et al., 2004). Additionally, the non-specific transporter ZupT also competes with the host NRAMP1 for Mn2+ metal ions in phagosomes, contributing to Salmonella virulence (Karlinsey et al., 2010). By competing for Mn2+ with host cell calprotectin, MntH and SitABCD Mn2+ transport systems help Salmonella overgrow commensals in the inflamed gut (Diaz-Ochoa et al., 2016). Acquisition of Mn2+ also powers detoxification of ROS produced by inflammatory neutrophils in the gut mucosa (Diaz-Ochoa et al., 2016). The role of MntH and SitABCD may not be limited to extracellular bacteria, as genes encoding these Mn2+ transporters are transcribed in Salmonella residing in the cytosol of epithelial cells, and mntH and sitA Salmonella mutants grow poorly in the cytosol of epithelial cells (Powers et al., 2021). The acquisition of Mn2+ by cytosolic Salmonella may counteract oxidative stress, while facilitating utilization of sugars (Powers et al., 2021).
Salmonella deficient of MntH, SitA and ZupT transporters still acquire trace levels of Mn2+ in vitro, indicating the presence of other import systems (Karlinsey et al., 2010; Yousuf et al., 2020). The search for other modes of Mn2+ import into Salmonella is still underway, and examples from other bacteria may lead to the discovery of novel Mn2+ uptake systems in Salmonella. Burkholderia pseudomallei import Mn2+ via a type VI secretion system (T6SS) and the TonB cell envelope protein (Si et al., 2017; DeShazer, 2019). B. pseudomallei undergoing oxidative stress secrete the Mn2+-binding T6SS effector TseM, and low Mn2+ concentrations induce expression of the Mn2+ specific, TonB-dependent MnoT integral protein (Figure 1A). TseM scavenges extracellular Mn2+ and actively shuttles the metal via MnoT. Salmonella T6SS is activated by oxidative stress and our bioinformatics analysis has revealed a conserved locus in the Salmonella genome with high similarity to Burkholderia MnoT (Figures 1B,D) (Kroger et al., 2013). Additional T6SS substrates with Mn2+-scavenging capacities are still being uncovered, including the TssS micropeptide from Yersinia pseudotuberculosis that chelates Mn2+ and sabotages bacterial clearance by inhibiting STING-mediated innate immune response (Zhu et al., 2021).
Mn2+ Export in Salmonella Pathogenesis
Paradoxically, excessive Mn2+ evokes oxidative stress in E. coli, affecting protein stability, interfering with envelope biogenesis, disrupting iron homeostasis and diminishing both tricarboxylic acid cycle and electron transport chain functions (Kaur et al., 2017). Bacteria excrete excessive Mn2+ using both the LysE superfamily MntP protein, and the cation diffuser facilitator (CDF) family member MntE (Martin et al., 2015). The Xanthomonas MntP homolog has two DUF204 domains that are conserved in Salmonella MntP protein (Li et al., 2011). MntP is regulated at transcriptional and posttranscriptional levels via MntR, mismetallated Fur and a yybP-ykoY riboswitch (Dambach et al., 2015; Bosma et al., 2021). The expression of the small RNA rybA, which encodes the small protein MntS, is repressed by MntR (Waters et al., 2011; Martin et al., 2015). The accumulation of apo-MntR in Mn2+ starving cells activates production of MntS, which represses MntP efflux activity and thus enlarges the intracytoplasmic pool of Mn2+ (Martin et al., 2015). On the other hand, the yybP-ykoY riboswitch directly binds to Mn2+, stabilizing a secondary structure that prevents sequestration of the mntP ribosome-binding site during translation (Dambach et al., 2015). MntP mediates efflux of Mn2+ ions in Salmonella following nitrosative stress (Ouyang et al., 2022).
The second efflux pump MntE is widely distributed in Gram-positive bacteria (Chandrangsu et al., 2017; Lam et al., 2020). Streptococci bearing mutations in mntE harbor excessive intracellular Mn2+ and experience attenuation of virulence (Rosch et al., 2009). Our bioinformatic analysis shows that Salmonella FieF (Yiip) protein belonging to CDF family has around 26%–64% sequence similarity with Streptococcus MntE, although it mediates zinc and iron export (Grass et al., 2005; Huang et al., 2017).
Mn2+ Helps Salmonella Adapt to Oxidative Stress
Salmonella are exposed to ROS generated in the innate host response. Sulfur-containing cysteine and methionine amino acids are primary targets of H2O2 (Bin et al., 2017). In addition, ROS carbonylate arginine, lysine, proline and threonine residues, and oxidize metal prosthetic groups and histidine residues (Ortiz de Orue Lucana et al., 2012; Chang et al., 2020). Salmonella have evolved diverse mechanisms to counter ROS generation, prevent formation of hydroxy radicals, inhibit delivery of ROS into Salmonella-containing vesicles, detoxify and scavenge ROS, or repair the resultant protein and DNA modifications (Buchmeier et al., 1995; De Groote et al., 1997; Vazquez-Torres et al., 2000a; Vazquez-Torres et al., 2000b; Gallois et al., 2001; Vazquez-Torres and Fang, 2001; Waterman and Holden, 2003; Halsey et al., 2004; Aussel et al., 2011; Bogomolnaya et al., 2013; Song et al., 2013; Rhen, 2019; Bogomolnaya et al., 2020; Shome et al., 2020). Of particular interest to this review, Mn2+ protects Salmonella from ROS-mediated cytotoxicity by serving as a cofactor for SOD and KatN enzymes, replacing Fe2+ in the active sites of mononuclear iron-containing enzymes, and acting as a nonproteinaceous antioxidant (Culotta and Daly, 2013; Imlay, 2014; Ighodaro and Akinloye, 2018).
Manganese-Based Detoxification of ROS
Salmonella confronts exogenous ROS generated by either host NADPH oxidase in phagocytes or dual oxidase 2 in epithelial cells (Vazquez-Torres and Fang, 2001; Behnsen et al., 2014). Superoxide anion (O2.-) formed by the vectorial transfer of electrons from flavoproteins and semiquinones to molecular oxygen is also a source of endogenous oxidative stress (Imlay, 2013). SODs and catalases expressed basally scavenge endogenously produced O2•- and H2O2, which accumulate at steady-intracellular concentrations of ∼0.2 and ∼50 nM, respectively (Imlay, 2013). Cytoplasmic membranes are semipermeable to exogenous H2O2, but at neutral pH prevent entry of O2•- (Bienert et al., 2006; Imlay, 2019). However, the HO2• acid conjugate readily reaches the bacterial cytoplasm (Imlay, 2019). Salmonella synthesizes two structurally distinct classes of SOD enzymes that catalyze the disproportionation of O2•- to O2 and H2O2 (Perry et al., 2010; Rhen, 2019). Both Mn and Fe-dependent SODs (SodA and SodB, respectively) are cytoplasmic, whereas Cu,Zn-dependent SodC-I and SodC-II are periplasmic (Tsolis et al., 1995; Canvin et al., 1996; Taylor et al., 2009; Perry et al., 2010; Bismuth et al., 2021). Cu,Zn-SOD protect periplasmic or inner membrane targets from O2•- toxicity, and limit peroxynitrite formation from the reaction of O2•- and nitric oxide (NO•) (De Groote et al., 1997; Gort et al., 1999). Mutants devoid of cytoplasmic Mn-SOD and Fe-SOD are auxotrophic for branched chain amino acids, sulfur-containing amino acids, and aromatic amino acids, and, due to defects in aconitase and fumarase, can only grow on fermentable carbon sources (Carlioz and Touati, 1986; Imlay and Fridovich, 1992). Salmonella lacking Mn-SOD are susceptible to early killing by J774 macrophages but are virulent in an acute mouse model of Salmonella infection, likely reflecting the existence of redundant antioxidant systems (Tsolis et al., 1995). However, a Salmonella strain deficient in Mn-SOD is at a competitive disadvantage in the gut because of the Mn2+ limitation imposed by calprotectin (Diaz-Ochoa et al., 2016). Collectively, these investigations indicate that the role played by Mn-SOD in Salmonella pathogenesis is tissue specific.
Salmonella degrade H2O2 with the aid of the catalase activity of KatG, KatE, and KatN, of which KatN uses Mn2+ as cofactor. H2O2 induces transcription of katG in an OxyR-dependent manner, whereas katE and katN are members of the RpoS regulon (Buchmeier et al., 1995; Ibanez-Ruiz et al., 2000; Seaver and Imlay, 2001; Pardo-Este et al., 2018). Under oxidative stress and Mn2+ deplete conditions, KatN seems to be dispensable for Salmonella growth, likely reflecting redundancy of multiple peroxide degrading enzymes such as the alkyl/thiol hydroperoxide reductases AhpC and TsaA as well as peroxiredoxin Tpx (Hebrard et al., 2009; Horst et al., 2010; Diaz-Ochoa et al., 2016). Independently, H2O2-induced protein damage can be effectively repaired by thioredoxin and glutathione systems (Agbor et al., 2014; Song et al., 2016). The redundancy of antioxidant defenses in Salmonella attest to the tremendous selective pressure this intracellular pathogen faces during the respiratory burst of professional phagocytes.
Cambialistic Enzymes
Because of the high binding affinity and ready availability in anoxic environments of the primitive Earth, Fe2+ was incorporated as cofactor of many primordial metabolic enzymes (Imlay, 2014). However, Fe2+ bound to a polypeptide can reduce H2O2, generating reactive hydroxyl and ferryl radicals in situ (Touati, 2000). This feature predisposes the Feα in [4Fe-4S] clusters of dehydratases and mononuclear Fe2+ to H2O2 attack, and Fe2+-mediated reduction of H2O2 in proximity to DNA inflicts genotoxicity (Winterbourn, 1995; Henle et al., 1999; Park and Imlay, 2003; Anjem and Imlay, 2012). Iron and manganese exist in two interchangeable redox forms, 2+ and 3+. Due to the symmetry of half-filled d5 electron shells, Mn2+ and Fe3+ (3d5) are more thermodynamically stable than Mn3+ (3d4) and Fe2+ (3d6) (Lingappa et al., 2019). The Mn3+/Mn2+ and Fe3+/Fe2+ redox couples have potentials of 1.51 and 0.77 V, respectively. Therefore, Mn2+ is less likely to donate electrons than Fe2+ (Guillemet-Fritsch et al., 2005). It is for this reason that, under most biological conditions, Mn2+ is less reactive than Fe2+ (Nealson and Myers, 1992). The thermodynamic stability of Mn2+, lower reactivity, and identical coordination geometry favor the mismetallation of Fe2+ by Mn2+. Replacement of Fe2+ with Mn2+ prevents oxidative damage of metalloenzymes (Emerson et al., 2008; Puri et al., 2010; Hood and Skaar, 2012). Accordingly, members of the Enterobacteriaceae shift from an iron- to a manganese-centric metabolism following oxidative stress (Anjem et al., 2009; Aguirre and Culotta, 2012). Examples of cambialistic enzymes include Rpe in the pentose phosphate pathway as discussed below. Incorporation of Mn2+ in place of Fe2+ allows metabolic flow during exposure to oxidative stress.
Mn2+-Dependent Nonproteinaceous Antioxidants
Manganese ions render bacteria resistant to oxidative stress, even in the absence of Mn-SOD (Horsburgh et al., 2002). This protection may be mediated by the Mn2+-dependent degradation of O2•- and H2O2 (Archibald and Fridovich, 1981, 1982; Berlett et al., 1990; Yocum and Pecoraro, 1999). Mn2+ reacts with O2•- to form transient MnO2+, which converts to manganous phosphate, H2O2 and H2O (Barnese et al., 2012). In turn, Mn2+ disproportionates H2O2 to H2O and O2 (Stadtman et al., 1990).
Mn2+-Driven Central Metabolism in Salmonella Virulence
Growth of Salmonella in host cells relies on a versatile metabolism. Relevant to this review, Mn2+ impacts glycolysis, reductive TCA and the pentose phosphate pathway in Salmonella sustaining oxidative stress.
Metabolism of Mn2+ in Glycolysis and Reductive TCA During Oxidative Stress
The electron transport chain is a source of ATP and a dominant pathway for balancing NADH/NAD+ redox. The oxidative inhibition of NDH-I NADH dehydrogenase in Salmonella undergoing oxidative stress decreases the energetic and redox outputs of the respiratory chain (Husain et al., 2008; Chakraborty et al., 2020). Thus, Salmonella experiencing oxidative stress favor glycolysis and fermentation (Figure 2A) to rescue ATP homeostasis and to balance redox. Glycolysis and associated fermentation generate ATP via substrate-level phosphorylation, produce intermediates for a variety of biosynthetic pathways, and balance redox (Figure 2A). Glycolysis is indispensable for the successful survival of Salmonella in host cells and is an essential component in resistance of Salmonella to the phagocyte NADPH oxidase (NOX2) (Bowden et al., 2009; Paterson et al., 2009; Götz and Goebel, 2010; Fitzsimmons L. et al., 2018; Chakraborty et al., 2020). Salmonella activate overflow metabolism in macrophages, partially to utilize the glycolytic products 3-phosphoglycerate (3PG) and 2-phosphoglycerate (2PG) as carbon sources (Jiang et al., 2021). Phosphoglycerate mutase (PGM) plays a unique role in controlling the overflow metabolism that mitigates oxidative stress in Salmonella. PGM, the third enzyme in the payoff phase of glycolysis, converts 3PG to 2PG. Many bacteria encode two analogous PGM enzymes with no sequence or structural similarity (Foster et al., 2010; Radin et al., 2019). The dPGM isoform utilizes the cofactor 2,3-bisphosphoglycerate, whereas the iPGM isoform requires Mn2+. While dPGM functions as a dimer or trimer, iPGM is active as a monomer (Foster et al., 2010). These Non-homologous I Sofunctional Enzymes (NISE) evolved independently to undertake the crucial metabolic conversion 3PG and 2PG, and bacteria may have accrued them via lateral gene transfer or non-orthologous gene displacement (Omelchenko et al., 2010). The genome of Salmonella enterica encodes two Mn2+-dependent iPGMs (GpmB and GpmI) and one Mn2+-independent dPGM (GpmA) (Figures 2B,C). The two Mn2+-dependent iPGMs are unique in sequence and structure. Our bioinformatic analysis revealed that Salmonella GpmB, which is structurally similar to Bacillus stearothermophilus phosphatase, PhoE, is conserved among major Enterobacteriaceae members, while two-domain monomer GpmI has around 50% sequence similarity with Staphylococcus aureus orthologue (Figure 2C), suggesting a common evolutionary origin (Figure 2B). Strikingly, Salmonella exposed to ROS produced in inflammation preferentially utilize the Mn2+-independent GpmA isoform over the Mn2+-cofactored GpmB enzyme (Chakraborty et al., 2020). The preferential utilization of the Mn2+-independent GpmA by Salmonella during resistance to NADPH oxidase-mediated host defense may be explained by the high demand for Mn2+ during periods of oxidative stress as hinted by the negative selection of mntH mutants after H2O2 treatment (Chakraborty et al., 2020). In addition to the constraints imposed by Mn2+ limitation, the NOX2-dependent acidification of the cytoplasm of intracellular Salmonella may also explain the preferential utilization of the acid phosphatase family member GpmA over its alkaline GpmB counterpart.
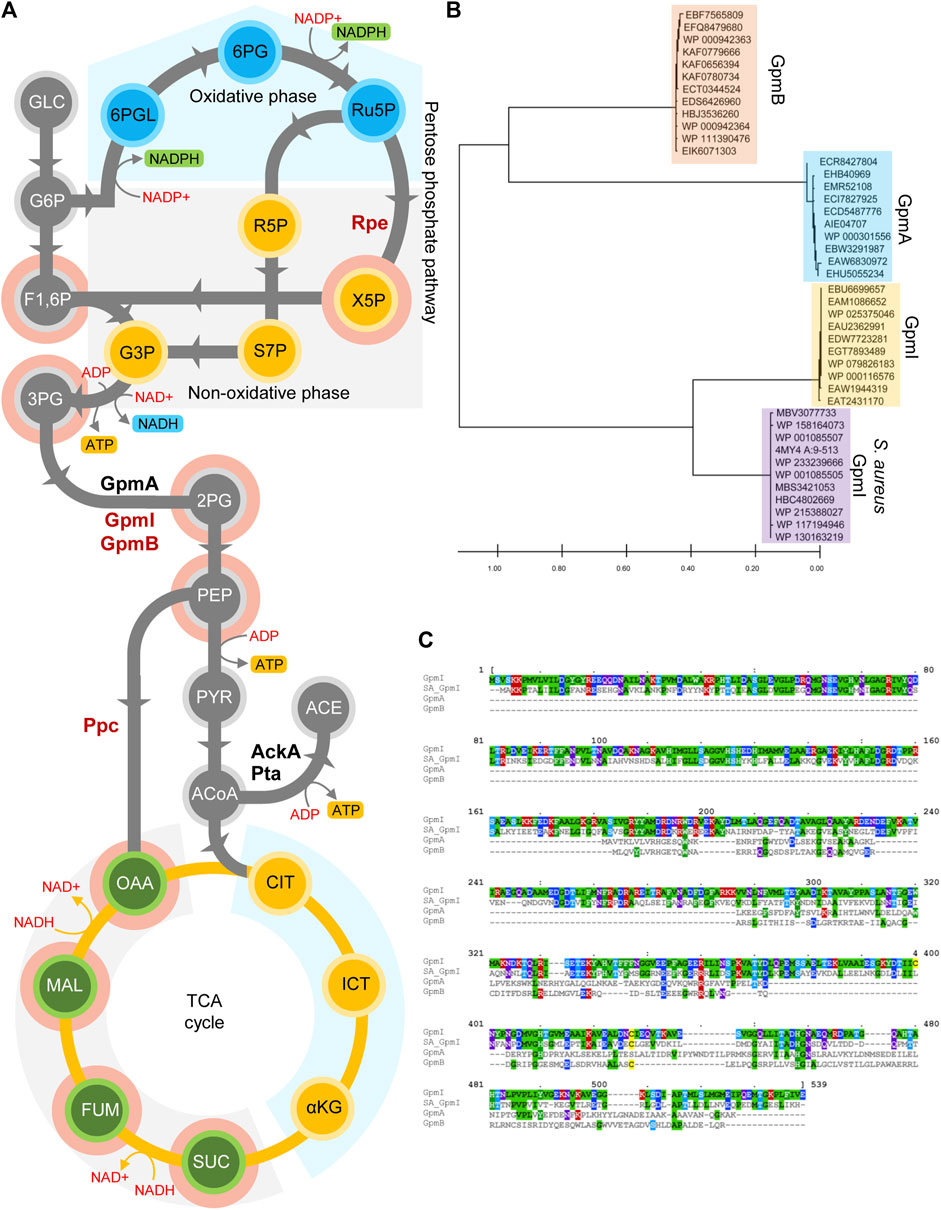
FIGURE 2. Manganese dependent metabolic adaptations in Salmonella. (A) Schematic representation of central metabolites and enzymes involved in glycolysis and TCA cycle. Enzymes in red are Mn2+ dependent. Glycolytic conversion of 3PG to 2PG is catalyzed by GpmA, a Mn2+-independent protein or its non-homologous isofunctional Mn2+-dependent GpmB and GpmI. During oxidative stress induced Mn2+ limitation, Salmonella utilizes the GpmA isoform to synthesise 2PG. The Mn2+-dependent phosphoenolpyruvate carboxylase (Ppc) shunts PEP into the reductive TCA cycle. Ribulose-5-PO4, 3-epimerase (Rpe), which catalyzes the conversion of ribulose-5PO4 to xylulose-5-PO4, is mismetallated during oxidative stress. As a result, Salmonella metabolism shifts into the production of reductive intermediates of the TCA cycle by Ppc and compromised non-oxidative phase of pentose phosphate pathway. (B) Phylogenetic analysis of Salmonella GpmA, B and I enzymes reveal that GpmI is similar to S. aureus GpmI. Differences between sequences are estimated by the scale shown at the bottom of the panel. (C) Clustal alignment of Salmonella GpmA (ACY87394.1), GpmB (ACY91834.1) and GpmI (ACY90848.1) with S. aureus GpmI (WP_001085507). Same scheme as in Figure 1D was followed to represent the alignment.
A knowledge-based and mathematical model of carbon flux in Salmonella revealed the importance of anaplerotic reactions around phosphoenolpyruvate (PEP) to oxaloacetate (OAA) conversion (Thiele et al., 2011; Dandekar et al., 2012). When modeled with glucose as sole C-source, the Mn2+-dependent PEP carboxylase enzyme (Ppc) seemed essential for fluxing glycolytic substrates into the reductive TCA cycle (Matsumura et al., 1999). Salmonella deficient of Ppc is virulent in BALB/c mouse model of infection (Tchawa Yimga et al., 2006). However, the combination of mutations in Ppc, acetate kinase (AckA) and phosphotransacetylase (Pta) results in a dramatic attenuation of Salmonella virulence (Chakraborty et al., 2020). Thus, generation of ATP via substrate-level phosphorylation together with the balancing of redox in the reductive TCA that is facilitated by fluxing PEP to oxaloacetate by the Mn2+-dependent Ppc contribute to Salmonella pathogenesis.
Pentose-Phosphate Pathway
The mononuclear iron in ribulose-5-PO4, 3-epimerase (Rpe) in the pentose-phosphate pathway can be poisoned by submicromolar H2O2 concentrations (Sobota and Imlay, 2011). The inactive form of Rpe, however, can rapidly metallate with Mn2+ ions and revert to its active form (Sobota and Imlay, 2011). Metallation of Rpe with oxidative stress-resistant Mn2+ may allow for carbon flow through the pentose phosphate pathway, thereby generating NADPH reducing power that is needed to maintain antioxidant defenses such as glutathione or thioredoxin reductase (Song et al., 2016).
Mn2+-Based Antinitrosative Defenses
Reactive nitrogen species synthesized by inducible nitric oxide (NO) synthase are bacteriostatic against Salmonella (Vazquez-Torres et al., 2000a; Thiele et al., 2011; Henard and Vázquez-Torres, 2012; Fitzsimmons L. F. et al., 2018). RNS modify biomolecules containing radicals, heme prosthetic groups, mononuclear iron, [Fe-S] clusters or redox active thiols in cysteine residues (Mikkelsen and Wardman, 2003; Forman et al., 2004; Poole, 2005; Husain et al., 2008; Pearce et al., 2009; Crawford et al., 2016; Jones-Carson et al., 2016; Jones-Carson et al., 2020). Salmonella mutants lacking Mn2+ transporters are more sensitive to RNS (Frawley et al., 2018; Yousuf et al., 2020; Ouyang et al., 2022). The intracellular concentrations of Mn2+ increase in Salmonella undergoing nitrosative stress, likely reflecting the upregulation of Mn2+ importers (Richardson et al., 2011; Yousuf et al., 2020). Regulation of Mn2+ transport systems is under the control of the transcription factor DksA (Crawford et al., 2016). Maintaining the homeostasis of intracellular Mn2+ in Salmonella after exposure to NO also involves the MntP and FieF efflux pumps (Ouyang et al., 2022). It remains unknown if these Mn2+ efflux systems contribute to the antinitrosative defenses of Salmonella.
Transient drops in intracellular amino acids during NO stress induce RelA-catalyzed synthesis of the (p)ppGpp alarmone, and the hydrolytic activity of SpoT is essential for reestablishing ppGpp homeostasis (Richardson et al., 2011; Fitzsimmons L. F. et al., 2018). A Mn2+ ion is coordinated by at least two carboxylates from aspartate and glutamate residues in the hydrolase domain of SpoT (Hogg et al., 2004). Histidine along with H2O molecules coordinate the rest of the four electrons of Mn2+. Studies in E. coli and Streptococcus revealed that ppGpp hydrolysis is strictly dependent on Mn2+ ions (Heinemeyer et al., 1978; Mechold et al., 1996). The nucleotidyltransferase domain (cd05399) of SpoT has a metal binding region dominated by aspartate and glutamate residues. Interestingly, the aspartate and glutamic acid residues of CD05399 are conserved in the synthetase domain rather than the hydrolase domain of SpoT, raising the possibility that Mn2+ may catalyze ppGpp synthesis rather than hydrolysis in Salmonella’s SpoT proteins. However, studies in Streptococcus revealed that intramolecular signal transmission between the two domains in the presence of ppGpp creates an allosteric shift in the synthetase domain, resulting in coordination of Mn2+ by an additional aspartate (Hogg et al., 2004). The additional coordination suppresses synthetase enzymatic activity. Interestingly, intracellular Salmonella lacking the non-catalytic regulatory C-terminal domain of SpoT (i.e., SpoT-ΔCTD) transcribe abnormally low levels of the Mn2+ importer SitABCD in macrophages (Fitzsimmons et al., 2020). SpoT-ΔCTD-expressing Salmonella are attenuated in NRAMP1+ C3H/HeN mice but not in NRAMP1- C57BL/6 mice, suggesting that the SpoT-dependent regulation of SitABCD combats the Mn2+ restrictions associated with a functional NRAMP1 transporter (Fitzsimmons et al., 2020).
Conclusion
Mn2+ homeostasis plays a poorly understood, but vital role in Salmonella pathogenesis. The elaborate transcriptional and posttranscriptional regulation of expression of diverse Mn2+ uptake and efflux systems help Salmonella navigate different metal-restricted anatomical sites in the vertebrate host. Historically, Mn2+ has been recognized as a cofactor of critical antioxidant defenses of Salmonella. Mn2+-dependent SOD protects Salmonella from oxidative stress; a function for Mn2+-dependent catalase in Salmonella virulence remains to be determined. Recent investigations have revealed the intricate relations between Mn2+ homeostasis, central metabolism, and antioxidant defenses of Salmonella. Salmonella rely on Mn2+ independent glycolysis during their adaptations to oxidative killing, but use Mn2+ to power anapleurotic pentose phosphate pathway reactions involved in redox balance necessary for central metabolism and synthesis of reductive power that fuels classical antioxidant defenses, such as glutathione reductase. Many unanswered questions still exist about the involvement of Mn2+ in the adaptations that promote Salmonella pathogenesis, providing a myriad of opportunities for future research.
Author Contributions
SRU and AV-T conceived the structure of this review and wrote the manuscript. SRU elaborated figures. All authors revised the manuscript and approved the final version.
Funding
These studies were supported by a VA Merit Grant BX0002073, and NIH grants R01AI54959 and R01AI136520.
Conflict of Interest
The authors declare that the research was conducted in the absence of any commercial or financial relationships that could be construed as a potential conflict of interest.
Publisher’s Note
All claims expressed in this article are solely those of the authors and do not necessarily represent those of their affiliated organizations, or those of the publisher, the editors and the reviewers. Any product that may be evaluated in this article, or claim that may be made by its manufacturer, is not guaranteed or endorsed by the publisher.
References
Agbor, T. A., Demma, Z., Mrsny, R. J., Castillo, A., Boll, E. J., and McCormick, B. A. (2014). The Oxido‐reductase Enzyme Glutathione Peroxidase 4 (GPX4) governsSalmonella Typhimurium‐induced Neutrophil Transepithelial Migration. Cell Microbiol. 16 (9), 1339–1353. doi:10.1111/cmi.12290
Aguirre, J. D., and Culotta, V. C. (2012). Battles with Iron: Manganese in Oxidative Stress Protection. J. Biol. Chem. 287 (17), 13541–13548. doi:10.1074/jbc.R111.312181
Aljahdali, N. H., Sanad, Y. M., Han, J., and Foley, S. L. (2020). Current Knowledge and Perspectives of Potential Impacts of Salmonella enterica on the Profile of the Gut Microbiota. BMC Microbiol. 20 (1), 353. doi:10.1186/s12866-020-02008-x
Anjem, A., and Imlay, J. A. (2012). Mononuclear Iron Enzymes Are Primary Targets of Hydrogen Peroxide Stress. J. Biol. Chem. 287 (19), 15544–15556. doi:10.1074/jbc.M111.330365
Anjem, A., Varghese, S., and Imlay, J. A. (2009). Manganese Import Is a Key Element of the OxyR Response to Hydrogen Peroxide inEscherichia Coli. Mol. Microbiol. 72 (4), 844–858. doi:10.1111/j.1365-2958.2009.06699.x
Archibald, F. S., and Fridovich, I. (1981). Manganese and Defenses against Oxygen Toxicity in Lactobacillus Plantarum. J. Bacteriol. 145 (1), 442–451. doi:10.1128/jb.145.1.442-451.1981
Archibald, F. S., and Fridovich, I. (1982). The Scavenging of Superoxide Radical by Manganous Complexes: In Vitro. Archives Biochem. Biophysics 214 (2), 452–463. doi:10.1016/0003-9861(82)90049-2
Atkinson, P. G., and Barton, C. H. (1999). High Level Expression ofNramp1G169in RAW264.7 Cell Transfectants: Analysis of Intracellular Iron Transport. Immunology 96 (4), 656–662. doi:10.1046/j.1365-2567.1999.00672.x
Aussel, L., Zhao, W., Hébrard, M., Guilhon, A.-A., Viala, J. P. M., Henri, S., et al. (2011). Salmonella Detoxifying Enzymes Are Sufficient to Cope with the Host Oxidative Burst. Mol. Microbiol. 80 (3), 628–640. doi:10.1111/j.1365-2958.2011.07611.x
Barnese, K., Gralla, E. B., Valentine, J. S., and Cabelli, D. E. (2012). Biologically Relevant Mechanism for Catalytic Superoxide Removal by Simple Manganese Compounds. Proc. Natl. Acad. Sci. U.S.A. 109 (18), 6892–6897. doi:10.1073/pnas.1203051109
Behnsen, J., Jellbauer, S., Wong, C. P., Edwards, R. A., George, M. D., Ouyang, W., et al. (2014). The Cytokine IL-22 Promotes Pathogen Colonization by Suppressing Related Commensal Bacteria. Immunity 40 (2), 262–273. doi:10.1016/j.immuni.2014.01.003
Bellamy, R. (2003). “The NRAMP Family: Co-evolution of a Host/pathogen Defence System,” in Bacterial Evasion of Host Immune Responses. Editors B. Henderson, and P. C. F. Oyston (Cambridge University Press), 39–52. doi:10.1017/CBO9780511546266.004
Berlett, B. S., Chock, P. B., Yim, M. B., and Stadtman, E. R. (1990). Manganese(II) Catalyzes the Bicarbonate-dependent Oxidation of Amino Acids by Hydrogen Peroxide and the Amino Acid-Facilitated Dismutation of Hydrogen Peroxide. Proc. Natl. Acad. Sci. U.S.A. 87 (1), 389–393. doi:10.1073/pnas.87.1.389
Bernal-Bayard, J., and Ramos-Morales, F. (2018). Molecular Mechanisms Used by Salmonella to Evade the Immune System. Curr. Issues Mol. Biol. 25, 133–168. doi:10.21775/cimb.025.133
Bienert, G. P., Schjoerring, J. K., and Jahn, T. P. (2006). Membrane Transport of Hydrogen Peroxide. Biochimica Biophysica Acta (BBA) - Biomembr. 1758 (8), 994–1003. doi:10.1016/j.bbamem.2006.02.015
Bin, P., Huang, R., and Zhou, X. (2017). Oxidation Resistance of the Sulfur Amino Acids: Methionine and Cysteine. BioMed Res. Int. 2017, 1–6. doi:10.1155/2017/9584932
Bismuth, H. D., Brasseur, G., Ezraty, B., and Aussel, L. (2021). Bacterial Genetic Approach to the Study of Reactive Oxygen Species Production in Galleria Mellonella during Salmonella Infection. Front. Cell. Infect. Microbiol. 11, 640112. doi:10.3389/fcimb.2021.640112
Bogomolnaya, L. M., Andrews, K. D., Talamantes, M., Maple, A., Ragoza, Y., Vazquez-Torres, A., et al. (2013). The ABC-type Efflux Pump MacAB Protects Salmonella enterica Serovar Typhimurium from Oxidative Stress. mBio 4 (6), e00630–00613. doi:10.1128/mBio.00630-13
Bogomolnaya, L. M., Tilvawala, R., Elfenbein, J. R., Cirillo, J. D., and Andrews-Polymenis, H. L. (2020). Linearized Siderophore Products Secreted via MacAB Efflux Pump Protect Salmonella enterica Serovar Typhimurium from Oxidative Stress. mBio 11 (3). doi:10.1128/mBio.00528-20
Bosma, E. F., Rau, M. H., van Gijtenbeek, L. A., and Siedler, S. (2021). Regulation and Distinct Physiological Roles of Manganese in Bacteria. FEMS Microbiol. Rev. 45 (6). doi:10.1093/femsre/fuab028
Bowden, S. D., Rowley, G., Hinton, J. C. D., and Thompson, A. (2009). Glucose and Glycolysis Are Required for the Successful Infection of Macrophages and Mice by Salmonella enterica Serovar Typhimurium. Infect. Immun. 77 (7), 3117–3126. doi:10.1128/IAI.00093-09
Boyer, E., Bergevin, I., Malo, D., Gros, P., and Cellier, M. F. M. (2002). Acquisition of Mn(II) in Addition to Fe(II) Is Required for Full Virulence of Salmonella enterica Serovar Typhimurium. Infect. Immun. 70 (11), 6032–6042. doi:10.1128/IAI.70.11.6032-6042.2002
Bozzi, A. T., and Gaudet, R. (2021). Molecular Mechanism of Nramp-Family Transition Metal Transport. J. Mol. Biol. 433 (16), 166991. doi:10.1016/j.jmb.2021.166991
Brown, D. E., Libby, S. J., Moreland, S. M., McCoy, M. W., Brabb, T., Stepanek, A., et al. (2013). Salmonella enterica Causes More Severe Inflammatory Disease in C57/BL6 Nramp1G169Mice Than Sv129S6 Mice. Vet. Pathol. 50 (5), 867–876. doi:10.1177/0300985813478213
Buchmeier, N. A., Libby, S. J., Xu, Y., Loewen, P. C., Switala, J., Guiney, D. G., et al. (1995). DNA Repair Is More Important Than Catalase for Salmonella Virulence in Mice. J. Clin. Invest. 95 (3), 1047–1053. doi:10.1172/jci117750
Cailliatte, R., Schikora, A., Briat, J.-F., Mari, S., and Curie, C. (2010). High-Affinity Manganese Uptake by the Metal Transporter NRAMP1 Is Essential for Arabidopsis Growth in Low Manganese Conditions. Plant cell 22 (3), 904–917. doi:10.1105/tpc.109.073023
Canonne-Hergaux, F., Gruenheid, S., Govoni, G., and Gros, P. (1999). The Nramp1 Protein and its Role in Resistance to Infection and Macrophage Function. Proc. Assoc. Am. Phys. 111 (4), 283–289. doi:10.1046/j.1525-1381.1999.99236.x
Canvin, J., Langford, P. R., Wilks, K. E., and Kroll, J. S. (1996). Identification ofsodCencoding Periplasmic [Cu,Zn]-Superoxide Dismutase inSalmonella. FEMS Microbiol. Lett. 136 (2), 215–220. doi:10.1111/j.1574-6968.1996.tb08052.x
Carlioz, A., and Touati, D. (1986). Isolation of Superoxide Dismutase Mutants in Escherichia coli: Is Superoxide Dismutase Necessary for Aerobic Life? EMBO J. 5 (3), 623–630. doi:10.1002/j.1460-2075.1986.tb04256.x
Cellier, M. F., Courville, P., and Campion, C. (2007). Nramp1 Phagocyte Intracellular Metal Withdrawal Defense. Microbes Infect. 9 (14-15), 1662–1670. doi:10.1016/j.micinf.2007.09.006
Chakraborty, S., Liu, L., Fitzsimmons, L., Porwollik, S., Kim, J.-S., Desai, P., et al. (2020). Glycolytic Reprograming in Salmonella Counters NOX2-Mediated Dissipation of ΔpH. Nat. Commun. 11 (1), 1783. doi:10.1038/s41467-020-15604-2
Chandrangsu, P., Rensing, C., and Helmann, J. D. (2017). Metal Homeostasis and Resistance in Bacteria. Nat. Rev. Microbiol. 15 (6), 338–350. doi:10.1038/nrmicro.2017.15
Chang, R. L., Stanley, J. A., Robinson, M. C., Sher, J. W., Li, Z., Chan, Y. A., et al. (2020). Protein Structure, Amino Acid Composition and Sequence Determine Proteome Vulnerability to Oxidation‐induced Damage. EMBO J. 39 (23), e104523. doi:10.15252/embj.2020104523
Chen, C.-C., Huang, J.-L., Chang, C.-J., and Kong, M.-S. (2012). Fecal Calprotectin as a Correlative Marker in Clinical Severity of Infectious Diarrhea and Usefulness in Evaluating Bacterial or Viral Pathogens in Children. J. Pediatr. Gastroenterol. Nutr. 55 (5), 541–547. doi:10.1097/MPG.0b013e318262a718
Crawford, M. A., Henard, C. A., Tapscott, T., Porwollik, S., McClelland, M., and Vázquez-Torres, A. (2016). DksA-Dependent Transcriptional Regulation in Salmonella Experiencing Nitrosative Stress. Front. Microbiol. 7, 444. doi:10.3389/fmicb.2016.00444
Culotta, V. C., and Daly, M. J. (2013). Manganese Complexes: Diverse Metabolic Routes to Oxidative Stress Resistance in Prokaryotes and Yeast. Antioxidants Redox Signal. 19 (9), 933–944. doi:10.1089/ars.2012.5093
Cunrath, O., and Bumann, D. (2019). Host Resistance Factor SLC11A1 Restricts Salmonella Growth through Magnesium Deprivation. Science, 366(6468), 995–999. doi:10.1126/science.aax7898
Cunrath, O., and Palmer, J. D. (2021). An Overview of Salmonella enterica Metal Homeostasis Pathways during Infection. Microlife 2, uqab001. doi:10.1093/femsml/uqab001
Dambach, M., Sandoval, M., Updegrove, T. B., Anantharaman, V., Aravind, L., Waters, L. S., et al. (2015). The Ubiquitous yybP-ykoY Riboswitch Is a Manganese-Responsive Regulatory Element. Mol. Cell 57 (6), 1099–1109. doi:10.1016/j.molcel.2015.01.035
Dandekar, T., Astrid, F., Jasmin, P., and Hensel, M. (2012). Salmonella enterica: a Surprisingly Well-Adapted Intracellular Lifestyle. Front. Microbio. 3, 164. doi:10.3389/fmicb.2012.00164
Daniel, J., Abraham, L., Martin, A., Pablo, X., and Reyes, S. (2018). Rv2477c Is an Antibiotic-Sensitive Manganese-dependent ABC-F ATPase in Mycobacterium tuberculosis. Biochem. Biophysical Res. Commun. 495 (1), 35–40. doi:10.1016/j.bbrc.2017.10.168
De Groote, M. A., Ochsner, U. A., Shiloh, M. U., Nathan, C., McCord, J. M., Dinauer, M. C., et al. (1997). Periplasmic Superoxide Dismutase Protects Salmonella from Products of Phagocyte NADPH-Oxidase and Nitric Oxide Synthase. Proc. Natl. Acad. Sci. U.S.A. 94 (25), 13997–14001. doi:10.1073/pnas.94.25.13997
De Jong, H. K., Achouiti, A., Koh, G. C. K. W., Parry, C. M., Baker, S., Faiz, M. A., et al. (2015). Expression and Function of S100A8/A9 (Calprotectin) in Human Typhoid Fever and the Murine Salmonella Model. PLoS Negl. Trop. Dis. 9 (4), e0003663. doi:10.1371/journal.pntd.0003663
DeShazer, D. (2019). A Novel Contact-independent T6SS that Maintains Redox Homeostasis via Zn2+ and Mn2+ Acquisition Is Conserved in the Burkholderia Pseudomallei Complex. Microbiol. Res. 226, 48–54. doi:10.1016/j.micres.2019.05.007
Diaz-Ochoa, V. E., Lam, D., Lee, C. S., Klaus, S., Behnsen, J., Liu, J. Z., et al. (2016). Salmonella Mitigates Oxidative Stress and Thrives in the Inflamed Gut by Evading Calprotectin-Mediated Manganese Sequestration. Cell Host Microbe 19 (6), 814–825. doi:10.1016/j.chom.2016.05.005
Emerson, J. P., Kovaleva, E. G., Farquhar, E. R., Lipscomb, J. D., and Que, L. (2008). Swapping Metals in Fe- and Mn-dependent Dioxygenases: Evidence for Oxygen Activation without a Change in Metal Redox State. Proc. Natl. Acad. Sci. U.S.A. 105 (21), 7347–7352. doi:10.1073/pnas.0711179105
Fang, F. C., Frawley, E. R., Tapscott, T., and Vázquez-Torres, A. (2016). Bacterial Stress Responses during Host Infection. Cell Host Microbe 20 (2), 133–143. doi:10.1016/j.chom.2016.07.009
Fitzsimmons, L. F., Liu, L., Kant, S., Kim, J.-S., Till, J. K., Jones-Carson, J., et al. (2020). SpoT Induces Intracellular Salmonella Virulence Programs in the Phagosome. mBio 11 (1). doi:10.1128/mBio.03397-19
Fitzsimmons, L. F., Liu, L., Kim, J.-S., Jones-Carson, J., and Vázquez-Torres, A. (2018a). Salmonella Reprograms Nucleotide Metabolism in its Adaptation to Nitrosative Stress. mBio 9 (1). doi:10.1128/mBio.00211-18
Fitzsimmons, L., Liu, L., Porwollik, S., Chakraborty, S., Desai, P., Tapscott, T., et al. (2018b). Zinc-dependent Substrate-Level Phosphorylation Powers Salmonella Growth under Nitrosative Stress of the Innate Host Response. PLoS Pathog. 14 (10), e1007388. doi:10.1371/journal.ppat.1007388
Forbes, J. R., and Gros, P. (2003). Iron, Manganese, and Cobalt Transport by Nramp1 (Slc11a1) and Nramp2 (Slc11a2) Expressed at the Plasma Membrane. Blood 102 (5), 1884–1892. doi:10.1182/blood-2003-02-0425
Forman, H. J., Fukuto, J. M., and Torres, M. (2004). Redox Signaling: Thiol Chemistry Defines Which Reactive Oxygen and Nitrogen Species Can Act as Second Messengers. Am. J. Physiology-Cell Physiology 287 (2), C246–C256. doi:10.1152/ajpcell.00516.2003
Foster, J. M., Davis, P. J., Raverdy, S., Sibley, M. H., Raleigh, E. A., Kumar, S., et al. (2010). Evolution of Bacterial Phosphoglycerate Mutases: Non-homologous Isofunctional Enzymes Undergoing Gene Losses, Gains and Lateral Transfers. PLoS One 5 (10), e13576. doi:10.1371/journal.pone.0013576
Frawley, E. R., Karlinsey, J. E., Singhal, A., Libby, S. J., Doulias, P.-T., Ischiropoulos, H., et al. (2018). Nitric Oxide Disrupts Zinc Homeostasis in Salmonella enterica Serovar Typhimurium. mBio 9 (4). doi:10.1128/mBio.01040-18
Galán, J. E. (2021). Salmonella Typhimurium and Inflammation: a Pathogen-Centric Affair. Nat. Rev. Microbiol. 19 (11), 716–725. doi:10.1038/s41579-021-00561-4
Gallois, A., Klein, J. R., Allen, L.-A. H., Jones, B. D., and Nauseef, W. M. (2001). SalmonellaPathogenicity Island 2-Encoded Type III Secretion System Mediates Exclusion of NADPH Oxidase Assembly from the Phagosomal Membrane. J. Immunol. 166 (9), 5741–5748. doi:10.4049/jimmunol.166.9.5741
Gogoi, M., Shreenivas, M. M., and Chakravortty, D. (2019). Hoodwinking the Big-Eater to Prosper: The Salmonella-Macrophage Paradigm. J. Innate Immun. 11 (3), 289–299. doi:10.1159/000490953
Gort, A. S., Ferber, D. M., and Imlay, J. A. (1999). The Regulation and Role of the Periplasmic Copper, Zinc Superoxide Dismutase of Escherichia coli. Mol. Microbiol. 32 (1), 179–191. doi:10.1046/j.1365-2958.1999.01343.x
Goswami, T., Bhattacharjee, A., Babal, P., Searle, S., Moore, E., Li, M., et al. (2001). Natural-resistance-associated Macrophage Protein 1 Is an H+/bivalent Cation Antiporter. Biochem. J. 354 (Pt 3), 511–519. doi:10.1042/0264-6021:3540511
Götz, A., and Goebel, W. (2010). Glucose and Glucose 6-phosphate as Carbon Sources in Extra- and Intracellular Growth of Enteroinvasive Escherichia coli and Salmonella enterica. Microbiol. Read. 156 (Pt 4), 1176–1187. doi:10.1099/mic.0.034744-0
Grass, G., Otto, M., Fricke, B., Haney, C. J., Rensing, C., Nies, D. H., et al. (2005). FieF (YiiP) from Escherichia coli Mediates Decreased Cellular Accumulation of Iron and Relieves Iron Stress. Arch. Microbiol. 183 (1), 9–18. doi:10.1007/s00203-004-0739-4
Gruenheid, S., Pinner, E., Desjardins, M., and Gros, P. (1997). Natural Resistance to Infection with Intracellular Pathogens: The Nramp1 Protein Is Recruited to the Membrane of the Phagosome. J. Exp. Med. 185 (4), 717–730. doi:10.1084/jem.185.4.717
Guillemet-Fritsch, S., Navrotsky, A., Tailhades, P., Coradin, H., and Wang, M. (2005). Thermochemistry of Iron Manganese Oxide Spinels. J. Solid State Chem. 178, 106–113. doi:10.1016/j.jssc.2004.10.031
Gunshin, H., Mackenzie, B., Berger, U. V., Gunshin, Y., Romero, M. F., Boron, W. F., et al. (1997). Cloning and Characterization of a Mammalian Proton-Coupled Metal-Ion Transporter. Nature 388 (6641), 482–488. doi:10.1038/41343
Halsey, T. A., Vazquez-Torres, A., Gravdahl, D. J., Fang, F. C., and Libby, S. J. (2004). The Ferritin-like Dps Protein Is Required for Salmonella enterica Serovar Typhimurium Oxidative Stress Resistance and Virulence. Infect. Immun. 72 (2), 1155–1158. doi:10.1128/iai.72.2.1155-1158.2004
Hayden, J. A., Brophy, M. B., Cunden, L. S., and Nolan, E. M. (2013). High-Affinity Manganese Coordination by Human Calprotectin Is Calcium-dependent and Requires the Histidine-Rich Site Formed at the Dimer Interface. J. Am. Chem. Soc. 135 (2), 775–787. doi:10.1021/ja3096416
Heinemeyer, E.-A., Geis, M., and Richter, D. (1978). Degradation of Guanosine 3'-diphosphate 5'-diphosphate In Vitro by the spoT Gene Product of Escherichia coli. Eur. J. Biochem. 89 (1), 125–131. doi:10.1111/j.1432-1033.1978.tb20904.x
Henard, C. A., and Vázquez-Torres, A. (2012). DksA-dependent Resistance of Salmonella enterica Serovar Typhimurium against the Antimicrobial Activity of Inducible Nitric Oxide Synthase. Infect. Immun. 80 (4), 1373–1380. doi:10.1128/iai.06316-11
Henle, E. S., Han, Z., Tang, N., Rai, P., Luo, Y., and Linn, S. (1999). Sequence-specific DNA Cleavage by Fe2+-Mediated Fenton Reactions Has Possible Biological Implications. J. Biol. Chem. 274 (2), 962–971. doi:10.1074/jbc.274.2.962
Hennigar, S. R., and McClung, J. P. (2016). Nutritional Immunity. Am. J. Lifestyle Med. 10 (3), 170–173. doi:10.1177/1559827616629117
Hébrard, M., Viala, J. P. M., Méresse, S., Barras, F., and Aussel, L. (2009). Redundant Hydrogen Peroxide Scavengers Contribute to Salmonella Virulence and Oxidative Stress Resistance. J. Bacteriol. 191 (14), 4605–4614. doi:10.1128/JB.00144-09
Hiemstra, P. S., van den Barselaar, M. T., Roest, M., Nibbering, P. H., and van Furth, R. (1999). Ubiquicidin, a Novel Murine Microbicidal Protein Present in the Cytosolic Fraction of Macrophages. J. Leukoc. Biol. 66 (3), 423–428. doi:10.1002/jlb.66.3.423
Hogg, T., Mechold, U., Malke, H., Cashel, M., and Hilgenfeld, R. (2004). Conformational Antagonism between Opposing Active Sites in a Bifunctional RelA/SpoT Homolog Modulates (p)ppGpp Metabolism during the Stringent Response. Cell 117 (1), 57–68. doi:10.1016/s0092-8674(04)00260-0
Hood, M. I., and Skaar, E. P. (2012). Nutritional Immunity: Transition Metals at the Pathogen-Host Interface. Nat. Rev. Microbiol. 10 (8), 525–537. doi:10.1038/nrmicro2836
Horsburgh, M. J., Wharton, S. J., Karavolos, M., and Foster, S. J. (2002). Manganese: Elemental Defence for a Life with Oxygen. Trends Microbiol. 10 (11), 496–501. doi:10.1016/s0966-842x(02)02462-9
Horst, S. A., Jaeger, T., Denkel, L. A., Rouf, S. F., Rhen, M., and Bange, F.-C. (2010). Thiol Peroxidase Protects Salmonella enterica from Hydrogen Peroxide Stress In Vitro and Facilitates Intracellular Growth. J. Bacteriol. 192 (11), 2929–2932. doi:10.1128/jb.01652-09
Huang, K., Wang, D., Frederiksen, R. F., Rensing, C., Olsen, J. E., and Fresno, A. H. (2017). Investigation of the Role of Genes Encoding Zinc Exporters zntA, zitB, and fieF during Salmonella Typhimurium Infection. Front. Microbiol. 8, 2656. doi:10.3389/fmicb.2017.02656
Husain, M., Bourret, T. J., McCollister, B. D., Jones-Carson, J., Laughlin, J., and Vázquez-Torres, A. (2008). Nitric Oxide Evokes an Adaptive Response to Oxidative Stress by Arresting Respiration. J. Biol. Chem. 283 (12), 7682–7689. doi:10.1074/jbc.M708845200
Hutfilz, C. R., Wang, N. E., Hoff, C. A., Lee, J. A., Hackert, B. J., Courcelle, J., et al. (2019). Manganese Is Required for the Rapid Recovery of DNA Synthesis Following Oxidative Challenge in Escherichia coli. J. Bacteriol. 201 (24). doi:10.1128/JB.00426-19
Ibanez-Ruiz, M., Robbe-Saule, V., Hermant, D., Labrude, S., and Norel, F. (2000). Identification of RpoS (ς S )-Regulated Genes in Salmonella enterica Serovar Typhimurium. J. Bacteriol. 182 (20), 5749–5756. doi:10.1128/JB.182.20.5749-5756.2000
Ighodaro, O. M., and Akinloye, O. A. (2018). First Line Defence Antioxidants-Superoxide Dismutase (SOD), Catalase (CAT) and Glutathione Peroxidase (GPX): Their Fundamental Role in the Entire Antioxidant Defence Grid. Alexandria J. Med. 54 (4), 287–293. doi:10.1016/j.ajme.2017.09.001
Ikeda, J. S., Janakiraman, A., Kehres, D. G., Maguire, M. E., and Slauch, J. M. (2005). Transcriptional Regulation of sitABCD of Salmonella enterica Serovar Typhimurium by MntR and Fur. J. Bacteriol. 187 (3), 912–922. doi:10.1128/JB.187.3.912-922.2005
Imlay, J. A., and Fridovich, I. (1992). Suppression of Oxidative Envelope Damage by Pseudoreversion of a Superoxide Dismutase-Deficient Mutant of Escherichia coli. J. Bacteriol. 174 (3), 953–961. doi:10.1128/jb.174.3.953-961.1992
Imlay, J. A. (2014). The Mismetallation of Enzymes during Oxidative Stress. J. Biol. Chem. 289 (41), 28121–28128. doi:10.1074/jbc.R114.588814
Imlay, J. A. (2013). The Molecular Mechanisms and Physiological Consequences of Oxidative Stress: Lessons from a Model Bacterium. Nat. Rev. Microbiol. 11 (7), 443–454. doi:10.1038/nrmicro3032
Imlay, J. A. (2019). Where in the World Do Bacteria Experience Oxidative Stress? Environ. Microbiol. 21 (2), 521–530. doi:10.1111/1462-2920.14445
Jabado, N., Jankowski, A., Dougaparsad, S., Picard, V., Grinstein, S., and Gros, P. (2000). Natural Resistance to Intracellular Infections. J. Exp. Med. 192 (9), 1237–1248. doi:10.1084/jem.192.9.1237
Janakiraman, A., and Slauch, J. M. (2000). The Putative Iron Transport System SitABCD Encoded on SPI1 Is Required for Full Virulence of Salmonella typhimurium. Mol. Microbiol. 35 (5), 1146–1155. doi:10.1046/j.1365-2958.2000.01783.x
Jiang, L., Wang, P., Song, X., Zhang, H., Ma, S., Wang, J., et al. (2021). Salmonella Typhimurium Reprograms Macrophage Metabolism via T3SS Effector SopE2 to Promote Intracellular Replication and Virulence. Nat. Commun. 12 (1), 879. doi:10.1038/s41467-021-21186-4
Johne, B., Fagerhol, M. K., Lyberg, T., Prydz, H., Brandtzaeg, P., Naess-Andresen, C. F., et al. (1997). Functional and Clinical Aspects of the Myelomonocyte Protein Calprotectin. Mol. Pathol. 50 (3), 113–123. doi:10.1136/mp.50.3.113
Jones-Carson, J., Husain, M., Liu, L., Orlicky, D. J., and Vázquez-Torres, A. (2016). Cytochrome Bd -Dependent Bioenergetics and Antinitrosative Defenses in Salmonella Pathogenesis. mBio 7 (6). doi:10.1128/mBio.02052-16
Jones-Carson, J., Yahashiri, A., Kim, J.-S., Liu, L., Fitzsimmons, L. F., Weiss, D. S., et al. (2020). Nitric Oxide Disrupts Bacterial Cytokinesis by Poisoning Purine Metabolism. Sci. Adv. 6 (9), eaaz0260. doi:10.1126/sciadv.aaz0260
Jukic, A., Bakiri, L., Wagner, E. F., Tilg, H., and Adolph, T. E. (2021). Calprotectin: from Biomarker to Biological Function. Gut 70 (10), 1978–1988. doi:10.1136/gutjnl-2021-324855
Karlinsey, J. E., Maguire, M. E., Becker, L. A., Crouch, M.-L. V., and Fang, F. C. (2010). The Phage Shock Protein PspA Facilitates Divalent Metal Transport and Is Required for Virulence of Salmonella enterica Sv. Typhimurium. Mol. Microbiol. 78 (3), 669–685. doi:10.1111/j.1365-2958.2010.07357.x
Kaur, G., Kumar, V., Arora, A., Tomar, A., AshishSur, R., Sur, R., et al. (2017). Affected Energy Metabolism under Manganese Stress Governs Cellular Toxicity. Sci. Rep. 7 (1), 11645. doi:10.1038/s41598-017-12004-3
Kehres, D. G., and Maguire, M. E. (2003). Emerging Themes in Manganese Transport, Biochemistry and Pathogenesis in Bacteria. FEMS Microbiol. Rev. 27 (2-3), 263–290. doi:10.1016/s0168-6445(03)00052-4
Kehres, D. G., Janakiraman, A., Slauch, J. M., and Maguire, M. E. (2002a). Regulation of Salmonella enterica Serovar Typhimurium mntH Transcription by H 2 O 2 , Fe 2+ , and Mn 2+. J. Bacteriol. 184 (12), 3151–3158. doi:10.1128/JB.184.12.3151-3158.2002
Kehres, D. G., Janakiraman, A., Slauch, J. M., and Maguire, M. E. (2002b). SitABCD Is the Alkaline Mn 2+ Transporter of Salmonella enterica Serovar Typhimurium. J. Bacteriol. 184 (12), 3159–3166. doi:10.1128/jb.184.12.3159-3166.2002
Kehres, D. G., Zaharik, M. L., Finlay, B. B., and Maguire, M. E. (2000). The NRAMP Proteins of Salmonella typhimurium and Escherichia coli Are Selective Manganese Transporters Involved in the Response to Reactive Oxygen. Mol. Microbiol., 36(5), 1085–1100. doi:10.1046/j.1365-2958.2000.01922.x
Kröger, C., Colgan, A., Srikumar, S., Händler, K., Sivasankaran, S. K., Hammarlöf, D. L., et al. (2013). An Infection-Relevant Transcriptomic Compendium for Salmonella enterica Serovar Typhimurium. Cell Host Microbe 14 (6), 683–695. doi:10.1016/j.chom.2013.11.010
Kuhn, D. E., Baker, B. D., Lafuse, W. P., and Zwilling, B. S. (1999). Differential Iron Transport into Phagosomes Isolated from the RAW264.7 Macrophage Cell Lines Transfected with Nramp1Gly169 or Nramp1Asp169. J. Leukoc. Biol. 66 (1), 113–119. doi:10.1002/jlb.66.1.113
Lam, L. N., Wong, J. J., Chong, K. K. L., and Kline, K. A. (2020). Enterococcus faecalis Manganese Exporter MntE Alleviates Manganese Toxicity and Is Required for Mouse Gastrointestinal Colonization. Infect. Immun. 88 (6). doi:10.1128/IAI.00058-20
Levinson, W., Chin-Hong, P., Joyce, E. A., Nussbaum, J., and Schwartz, B. (2018). “Host Defenses,” in Review of Medical Microbiology & Immunology: A Guide to Clinical Infectious Diseases (McGraw-Hill Education), 15e.accessmedicine.mhmedical.com/content.aspx?aid=1160145266
Lhocine, N., Arena, E. T., Bomme, P., Ubelmann, F., Prévost, M.-C., Robine, S., et al. (2015). Apical Invasion of Intestinal Epithelial Cells by Salmonella typhimurium Requires Villin to Remodel the Brush Border Actin Cytoskeleton. Cell Host Microbe 17 (2), 164–177. doi:10.1016/j.chom.2014.12.003
Li, C., Tao, J., Mao, D., and He, C. (2011). A Novel Manganese Efflux System, YebN, Is Required for Virulence by Xanthomonas Oryzae Pv. Oryzae. PLOS ONE 6 (7), e21983. doi:10.1371/journal.pone.0021983
Li, L., and Yang, X. (2018). The Essential Element Manganese, Oxidative Stress, and Metabolic Diseases: Links and Interactions. Oxidative Med. Cell. Longev. 2018, 1–11. doi:10.1155/2018/7580707
Lingappa, U. F., Monteverde, D. R., Magyar, J. S., Valentine, J. S., and Fischer, W. W. (2019). How Manganese Empowered Life with Dioxygen (And Vice Versa). Free Radic. Biol. Med. 140, 113–125. doi:10.1016/j.freeradbiomed.2019.01.036
Liu, J. Z., Jellbauer, S., Poe, A. J., Ton, V., Pesciaroli, M., Kehl-Fie, T. E., et al. (2012). Zinc Sequestration by the Neutrophil Protein Calprotectin Enhances Salmonella Growth in the Inflamed Gut. Cell Host Microbe 11 (3), 227–239. doi:10.1016/j.chom.2012.01.017
Loomis, W. P., Johnson, M. L., Brasfield, A., Blanc, M.-P., Yi, J., Miller, S. I., et al. (2014). Temporal and Anatomical Host Resistance to Chronic Salmonella Infection Is Quantitatively Dictated by Nramp1 and Influenced by Host Genetic Background. PLOS ONE 9 (10), e111763. doi:10.1371/journal.pone.0111763
Lorkowski, M., Felipe-López, A., Danzer, C. A., Hansmeier, N., and Hensel, M. (2014). Salmonella enterica Invasion of Polarized Epithelial Cells Is a Highly Cooperative Effort. Infect. Immun. 82 (6), 2657–2667. doi:10.1128/IAI.00023-14
Lovley, D. R., and Phillips, E. J. P. (1988). Novel Mode of Microbial Energy Metabolism: Organic Carbon Oxidation Coupled to Dissimilatory Reduction of Iron or Manganese. Appl. Environ. Microbiol. 54 (6), 1472–1480. doi:10.1128/aem.54.6.1472-1480.1988
Martin, J. E., and Imlay, J. A. (2011). The Alternative Aerobic Ribonucleotide Reductase of Escherichia coli, NrdEF, Is a Manganese-dependent Enzyme that Enables Cell Replication during Periods of Iron Starvation. Mol. Microbiol. 80 (2), 319–334. doi:10.1111/j.1365-2958.2011.07593.x
Martin, J. E., Waters, L. S., Storz, G., and Imlay, J. A. (2015). The Escherichia coli Small Protein MntS and Exporter MntP Optimize the Intracellular Concentration of Manganese. PLoS Genet. 11 (3), e1004977. doi:10.1371/journal.pgen.1004977
Matsumura, H., Terada, M., Shirakata, S., Inoue, T., Yoshinaga, T., Izui, K., et al. (1999). Plausible Phosphoenolpyruvate Binding Site Revealed by 2.6 Å Structure of Mn2+-Bound Phosphoenolpyruvate Carboxylase fromEscherichia Coli. FEBS Lett. 458 (2), 93–96. doi:10.1016/s0014-5793(99)01103-5
Mechold, U., Cashel, M., Steiner, K., Gentry, D., and Malke, H. (1996). Functional Analysis of a relA/spoT Gene Homolog from Streptococcus Equisimilis. J. Bacteriol. 178 (5), 1401–1411. doi:10.1128/jb.178.5.1401-1411.1996
Menkin, V. (1956). Biology of Inflammation. Science 123 (3196), 527–534. doi:10.1126/science.123.3196.527
Mikkelsen, R. B., and Wardman, P. (2003). Biological Chemistry of Reactive Oxygen and Nitrogen and Radiation-Induced Signal Transduction Mechanisms. Oncogene 22 (37), 5734–5754. doi:10.1038/sj.onc.1206663
Miriyala, S., Spasojevic, I., Tovmasyan, A., Salvemini, D., Vujaskovic, Z., St. Clair, D., et al. (2012). Manganese Superoxide Dismutase, MnSOD and its Mimics. Biochimica Biophysica Acta (BBA) - Mol. Basis Dis. 1822 (5), 794–814. doi:10.1016/j.bbadis.2011.12.002
Monteith, A. J., and Skaar, E. P. (2021). The Impact of Metal Availability on Immune Function during Infection. Trends Endocrinol. Metabolism 32 (11), 916–928. doi:10.1016/j.tem.2021.08.004
Nealson, K. H., and Myers, C. R. (1992). Microbial Reduction of Manganese and Iron: New Approaches to Carbon Cycling. Appl. Environ. Microbiol. 58 (2), 439–443. doi:10.1128/aem.58.2.439-443.1992
Nisapakultorn, K., Ross, K. F., and Herzberg, M. C. (2001). Calprotectin Expression Inhibits Bacterial Binding to Mucosal Epithelial Cells. Infect. Immun. 69 (6), 3692–3696. doi:10.1128/iai.69.6.3692-3696.2001
Omelchenko, M. V., Galperin, M. Y., Wolf, Y. I., and Koonin, E. V. (2010). Non-homologous Isofunctional Enzymes: a Systematic Analysis of Alternative Solutions in Enzyme Evolution. Biol. Direct 5, 31. doi:10.1186/1745-6150-5-31
Ortiz de Orué Lucana, D., Wedderhoff, I., and Groves, M. R. (2012). ROS-mediated Signalling in Bacteria: Zinc-Containing Cys-X-X-Cys Redox Centres and Iron-Based Oxidative Stress. J. Signal Transduct. 2012, 1–9. doi:10.1155/2012/605905
Osman, D., and Cavet, J. S. (2011). Metal Sensing in Salmonella. Adv. Microb. Physiol. 58, 175–232. doi:10.1016/b978-0-12-381043-4.00005-2
Ouyang, A., Gasner, K. M., Neville, S. L., McDevitt, C. A., and Frawley, E. R. (2022). MntP and YiiP Contribute to Manganese Efflux in Salmonella enterica Serovar Typhimurium under Conditions of Manganese Overload and Nitrosative Stress. Microbiol. Spectr. 10 (1), e0131621. doi:10.1128/spectrum.01316-21
Papp-Wallace, K. M., and Maguire, M. E. (2006). Manganese Transport and the Role of Manganese in Virulence. Annu. Rev. Microbiol. 60, 187–209. doi:10.1146/annurev.micro.60.080805.142149
Pardo-Esté, C., Hidalgo, A. A., Aguirre, C., Briones, A. C., Cabezas, C. E., Castro-Severyn, J., et al. (2018). The ArcAB Two-Component Regulatory System Promotes Resistance to Reactive Oxygen Species and Systemic Infection by Salmonella Typhimurium. PLOS ONE 13 (9), e0203497. doi:10.1371/journal.pone.0203497
Park, S., and Imlay, J. A. (2003). High Levels of Intracellular Cysteine Promote Oxidative DNA Damage by Driving the Fenton Reaction. J. Bacteriol. 185 (6), 1942–1950. doi:10.1128/jb.185.6.1942-1950.2003
Patel, S., and McCormick, B. A. (2014). Mucosal Inflammatory Response to Salmonella typhimurium Infection. Front. Immunol. 5. doi:10.3389/fimmu.2014.00311
Paterson, G. K., Cone, D. B., Northen, H., Peters, S. E., and Maskell, D. J. (2009). Deletion of the Gene Encoding the Glycolytic Enzyme Triosephosphate Isomerase (Tpi) Alters Morphology ofSalmonella Entericaserovar Typhimurium and Decreases Fitness in Mice. FEMS Microbiol. Lett. 294 (1), 45–51. doi:10.1111/j.1574-6968.2009.01553.x
Pearce, L. L., Martinez-Bosch, S., Manzano, E. L., Winnica, D. E., Epperly, M. W., and Peterson, J. (2009). The Resistance of Electron-Transport Chain Fe-S Clusters to Oxidative Damage during the Reaction of Peroxynitrite with Mitochondrial Complex II and Rat-Heart Pericardium. Nitric Oxide 20 (3), 135–142. doi:10.1016/j.niox.2008.12.001
Perry, J. J. P., Shin, D. S., Getzoff, E. D., and Tainer, J. A. (2010). The Structural Biochemistry of the Superoxide Dismutases. Biochimica Biophysica Acta (BBA) - Proteins Proteomics 1804 (2), 245–262. doi:10.1016/j.bbapap.2009.11.004
Poole, R. K. (2005). Nitric Oxide and Nitrosative Stress Tolerance in Bacteria. Biochem. Soc. Trans. 33 (Pt 1), 176–180. doi:10.1042/BST0330176
Porcheron, G., Garénaux, A., Proulx, J., Sabri, M., and Dozois, C. M. (2013). Iron, Copper, Zinc, and Manganese Transport and Regulation in Pathogenic Enterobacteria: Correlations between Strains, Site of Infection and the Relative Importance of the Different Metal Transport Systems for Virulence. Front. Cell. Infect. Microbiol. 3, 90. doi:10.3389/fcimb.2013.00090
Powers, T. R., Haeberle, A. L., Predeus, A. V., Hammarlöf, D. L., Cundiff, J. A., Saldaña-Ahuactzi, Z., et al. (2021). Intracellular Niche-specific Profiling Reveals Transcriptional Adaptations Required for the Cytosolic Lifestyle of Salmonella enterica. PLoS Pathog. 17 (8), e1009280. doi:10.1371/journal.ppat.1009280
Puri, S., Hohle, T. H., and O'Brian, M. R. (2010). Control of Bacterial Iron Homeostasis by Manganese. Proc. Natl. Acad. Sci. U.S.A. 107 (23), 10691–10695. doi:10.1073/pnas.1002342107
Rhen, M. (2019). Salmonella and Reactive Oxygen Species: A Love-Hate Relationship. J. Innate Immun. 11 (3), 216–226. doi:10.1159/000496370
Radin, J. N., Kelliher, J. L., Solórzano, P. K. P., Grim, K. P., Ramezanifard, R., Slauch, J. M., et al. (2019). Metal-independent Variants of Phosphoglycerate Mutase Promote Resistance to Nutritional Immunity and Retention of Glycolysis during Infection. PLoS Pathog. 15 (7), e1007971. doi:10.1371/journal.ppat.1007971
Richardson, A. R., Payne, E. C., Younger, N., Karlinsey, J. E., Thomas, V. C., Becker, L. A., et al. (2011). Multiple Targets of Nitric Oxide in the Tricarboxylic Acid Cycle of Salmonella enterica Serovar Typhimurium. Cell Host Microbe 10 (1), 33–43. doi:10.1016/j.chom.2011.06.004
Rosales, C., and Uribe-Querol, E. (2017). Phagocytosis: A Fundamental Process in Immunity. BioMed Res. Int. 2017, 1–18. doi:10.1155/2017/9042851
Rosch, J. W., Gao, G., Ridout, G., Wang, Y.-D., and Tuomanen, E. I. (2009). Role of the Manganese Efflux System mntE for Signalling and Pathogenesis in Streptococcus Pneumoniae. Mol. Microbiol. 72 (1), 12–25. doi:10.1111/j.1365-2958.2009.06638.x
Rosen, T., and Nolan, E. M. (2020). Metal Sequestration and Antimicrobial Activity of Human Calprotectin Are pH-dependent. Biochemistry 59 (26), 2468–2478. doi:10.1021/acs.biochem.0c00359
Scull, C. E., Dandpat, S. S., Romero, R. A., and Walter, N. G. (2020). Transcriptional Riboswitches Integrate Timescales for Bacterial Gene Expression Control. Front. Mol. Biosci. 7, 607158. doi:10.3389/fmolb.2020.607158
Seaver, L. C., and Imlay, J. A. (2001). Alkyl Hydroperoxide Reductase Is the Primary Scavenger of Endogenous Hydrogen Peroxide in Escherichia coli. J. Bacteriol. 183 (24), 7173–7181. doi:10.1128/JB.183.24.7173-7181.2001
Shi, L., Kehres, D. G., and Maguire, M. E. (2001). The PPP-Family Protein Phosphatases PrpA and PrpB of Salmonella enterica Serovar Typhimurium Possess Distinct Biochemical Properties. J. Bacteriol. 183 (24), 7053–7057. doi:10.1128/JB.183.24.7053-7057.2001
Shi, Y., Zhao, G., and Kong, W. (2014). Genetic Analysis of Riboswitch-Mediated Transcriptional Regulation Responding to Mn2+ in Salmonella. J. Biol. Chem. 289 (16), 11353–11366. doi:10.1074/jbc.M113.517516
Shome, A., Sarkhel, R., Apoorva, S., Nair, S. S., Chauhan, T. K. S., Bhure, S. K., et al. (2020). Role of Protein Repair Enzymes in Oxidative Stress Survival and Virulence of Salmonella. Ann. Microbiol. 70 (1), 55. doi:10.1186/s13213-020-01597-2
Si, M., Zhao, C., Burkinshaw, B., Zhang, B., Wei, D., Wang, Y., et al. (2017). Manganese Scavenging and Oxidative Stress Response Mediated by Type VI Secretion System in Burkholderia Thailandensis. Proc. Natl. Acad. Sci. U.S.A. 114 (11), E2233–E2242. doi:10.1073/pnas.1614902114
Sobota, J. M., and Imlay, J. A. (2011). Iron Enzyme Ribulose-5-Phosphate 3-epimerase in Escherichia coli Is Rapidly Damaged by Hydrogen Peroxide but Can Be Protected by Manganese. Proc. Natl. Acad. Sci. U.S.A. 108 (13), 5402–5407. doi:10.1073/pnas.1100410108
Song, M., Husain, M., Jones-Carson, J., Liu, L., Henard, C. A., and Vázquez-Torres, A. (2013). Low-molecular-weight Thiol-dependent Antioxidant and Antinitrosative Defences inSalmonellapathogenesis. Mol. Microbiol. 87 (3), 609–622. doi:10.1111/mmi.12119
Song, M., Kim, J.-S., Liu, L., Husain, M., and Vázquez-Torres, A. (2016). Antioxidant Defense by Thioredoxin Can Occur Independently of Canonical Thiol-Disulfide Oxidoreductase Enzymatic Activity. Cell Rep. 14 (12), 2901–2911. doi:10.1016/j.celrep.2016.02.066
Stadtman, E. R., Berlett, B. S., and Chock, P. B. (1990). Manganese-dependent Disproportionation of Hydrogen Peroxide in Bicarbonate Buffer. Proc. Natl. Acad. Sci. U.S.A. 87 (1), 384–388. doi:10.1073/pnas.87.1.384
Tanner, J. R., and Kingsley, R. A. (2018). Evolution of Salmonella within Hosts. Trends Microbiol. 26 (12), 986–998. doi:10.1016/j.tim.2018.06.001
Taylor, C. M., Osman, D., and Cavet, J. S. (2009). Differential Expression from Two Iron-Responsive Promoters in Salmonella enterica Serovar Typhimurium Reveals the Presence of Iron in Macrophage-Phagosomes. Microb. Pathog. 46 (2), 114–118. doi:10.1016/j.micpath.2008.11.001
Tchawa Yimga, M., Leatham, M. P., Allen, J. H., Laux, D. C., Conway, T., and Cohen, P. S. (2006). Role of Gluconeogenesis and the Tricarboxylic Acid Cycle in the Virulence of Salmonella enterica Serovar Typhimurium in BALB/c Mice. Infect. Immun. 74 (2), 1130–1140. doi:10.1128/iai.74.2.1130-1140.2006
Thiele, I., Hyduke, D. R., Steeb, B., Fankam, G., Allen, D. K., Bazzani, S., et al. (2011). A Community Effort towards a Knowledge-Base and Mathematical Model of the Human Pathogen Salmonella Typhimurium LT2. BMC Syst. Biol. 5, 8. doi:10.1186/1752-0509-5-8
Tong, Y., Zhai, Q., Wang, G., Zhang, Q., Liu, X., Tian, F., et al. (2017). System-wide Analysis of Manganese Starvation-Induced Metabolism in Key Elements of Lactobacillus Plantarum. RSC Adv. 7 (21), 12959–12968. doi:10.1039/C7RA00072C
Torrents, E. (2014). Ribonucleotide Reductases: Essential Enzymes for Bacterial Life. Front. Cell. Infect. Microbiol. 4, 52. doi:10.3389/fcimb.2014.00052
Touati, D. (2000). Iron and Oxidative Stress in Bacteria. Archives Biochem. Biophysics 373 (1), 1–6. doi:10.1006/abbi.1999.1518
Troxell, B., Fink, R. C., Porwollik, S., McClelland, M., and Hassan, H. M. (2011). The Fur Regulon in Anaerobically Grown Salmonella enterica Sv. Typhimurium: Identification of New Fur Targets. BMC Microbiol. 11, 236. doi:10.1186/1471-2180-11-236
Tsolis, R. M., Bäumler, A. J., and Heffron, F. (1995). Role of Salmonella typhimurium Mn-Superoxide Dismutase (SodA) in Protection against Early Killing by J774 Macrophages. Infect. Immun. 63 (5), 1739–1744. doi:10.1128/iai.63.5.1739-1744.1995
Urban, C. F., Ermert, D., Schmid, M., Abu-Abed, U., Goosmann, C., Nacken, W., et al. (2009). Neutrophil Extracellular Traps Contain Calprotectin, a Cytosolic Protein Complex Involved in Host Defense against Candida Albicans. PLoS Pathog. 5 (10), e1000639. doi:10.1371/journal.ppat.1000639
Uribe-Querol, E., and Rosales, C. (2020). Phagocytosis: Our Current Understanding of a Universal Biological Process. Front. Immunol. 11, 1066. doi:10.3389/fimmu.2020.01066
Vazquez-Torres, A., and Fang, F. C. (2001). Salmonella Evasion of the NADPH Phagocyte Oxidase. Microbes Infect. 3 (14-15), 1313–1320. doi:10.1016/s1286-4579(01)01492-7
Vazquez-Torres, A., Jones-Carson, J., Mastroeni, P., Ischiropoulos, H., and Fang, F. C. (2000a). Antimicrobial Actions of the NADPH Phagocyte Oxidase and Inducible Nitric Oxide Synthase in Experimental Salmonellosis. I. Effects on Microbial Killing by Activated Peritoneal Macrophages In Vitro. J. Exp. Med. 192 (2), 227–236. doi:10.1084/jem.192.2.227
Vazquez-Torres, A., Xu, Y., Jones-Carson, J., Holden, D. W., Lucia, S. M., Dinauer, M. C., et al. (2000b). Salmonella Pathogenicity Island 2-dependent Evasion of the Phagocyte NADPH Oxidase. Science 287 (5458), 1655–1658. doi:10.1126/science.287.5458.1655
Vidal, S., Belouchi, A.-M., Cellier, M., Beatty, B., and Gros, P. (1995). Cloning and Characterization of a Second Human NRAMP Gene on Chromosome 12q13. Mamm. Genome 6 (4), 224–230. doi:10.1007/bf00352405
Wang, S., Song, R., Wang, Z., Jing, Z., Wang, S., and Ma, J. (2018). S100A8/A9 in Inflammation. Front. Immunol. 9, 1298. doi:10.3389/fimmu.2018.01298
Waterman, S. R., and Holden, D. W. (2003). Functions and Effectors of the Salmonella Pathogenicity Island 2 Type III Secretion System. Cell Microbiol. 5 (8), 501–511. doi:10.1046/j.1462-5822.2003.00294.x
Waters, L. S. (2020). Bacterial Manganese Sensing and Homeostasis. Curr. Opin. Chem. Biol. 55, 96–102. doi:10.1016/j.cbpa.2020.01.003
Waters, L. S., Sandoval, M., and Storz, G. (2011). The Escherichia coli MntR Miniregulon Includes Genes Encoding a Small Protein and an Efflux Pump Required for Manganese Homeostasis. J. Bacteriol. 193 (21), 5887–5897. doi:10.1128/JB.05872-11
Whittaker, J. W. (2012). Non-heme Manganese Catalase - the 'other' Catalase. Archives Biochem. Biophysics 525 (2), 111–120. doi:10.1016/j.abb.2011.12.008
Winter, S. E., and Bäumler, A. J. (2011). A Breathtaking Feat. Gut Microbes 2 (1), 58–60. doi:10.4161/gmic.2.1.14911
Winterbourn, C. C. (1995). Toxicity of Iron and Hydrogen Peroxide: the Fenton Reaction. Toxicol. Lett. 82-83, 969–974. doi:10.1016/0378-4274(95)03532-x
Yocum, C. F., and Pecoraro, V. L. (1999). Recent Advances in the Understanding of the Biological Chemistry of Manganese. Curr. Opin. Chem. Biol. 3 (2), 182–187. doi:10.1016/S1367-5931(99)80031-3
Yousuf, S., Karlinsey, J. E., Neville, S. L., McDevitt, C. A., Libby, S. J., Fang, F. C., et al. (2020). Manganese Import Protects Salmonella enterica Serovar Typhimurium against Nitrosative Stress. Metallomics Integr. biometal Sci. 12 (11), 1791–1801. doi:10.1039/d0mt00178c
Zaharik, M. L., Cullen, V. L., Fung, A. M., Libby, S. J., Kujat Choy, S. L., Coburn, B., et al. (2004). The Salmonella enterica Serovar Typhimurium Divalent Cation Transport Systems MntH and SitABCD Are Essential for Virulence in an Nramp1 G169 Murine Typhoid Model. Infect. Immun. 72 (9), 5522–5525. doi:10.1128/iai.72.9.5522-5525.2004
Zhu, L., Xu, L., Wang, C., Li, C., Li, M., Liu, Q., et al. (2021). T6SS Translocates a Micropeptide to Suppress STING-Mediated Innate Immunity by Sequestering Manganese. Proc. Natl. Acad. Sci. U.S.A. 118 (42). doi:10.1073/pnas.2103526118
Zwilling, B. S., Kuhn, D. E., Wikoff, L., Brown, D., and Lafuse, W. (1999). Role of Iron in Nramp1 -Mediated Inhibition of Mycobacterial Growth. Infect. Immun. 67 (3), 1386–1392. doi:10.1128/iai.67.3.1386-1392.1999
Keywords: manganese, Salmonella, virulence, mismetallation, carbon metabolism, central metabolism, oxidative stress, nitrosative stress
Citation: Uppalapati SR and Vazquez-Torres A (2022) Manganese Utilization in Salmonella Pathogenesis: Beyond the Canonical Antioxidant Response. Front. Cell Dev. Biol. 10:924925. doi: 10.3389/fcell.2022.924925
Received: 20 April 2022; Accepted: 22 June 2022;
Published: 12 July 2022.
Edited by:
Mathieu F. Cellier, Université du Québec, CanadaReviewed by:
Erik Thomas Yukl, New Mexico State University, United StatesCélia Valente Romão, Universidade Nova de Lisboa, Portugal
Andrea Battistoni, University of Rome Tor Vergata, Italy
Copyright © 2022 Uppalapati and Vazquez-Torres. This is an open-access article distributed under the terms of the Creative Commons Attribution License (CC BY). The use, distribution or reproduction in other forums is permitted, provided the original author(s) and the copyright owner(s) are credited and that the original publication in this journal is cited, in accordance with accepted academic practice. No use, distribution or reproduction is permitted which does not comply with these terms.
*Correspondence: Siva R. Uppalapati, c2l2YS51cHBhbGFwYXRpQGN1YW5zY2h1dHouZWR1; Andres Vazquez-Torres, YW5kcmVzLnZhenF1ZXotdG9ycmVzQGN1YW5zY2h1dHouZWR1