- Division of Neurogeriatrics, Center for Alzheimer Research, Department of Neurobiology, Care Science and Society (NVS), Karolinska Institutet, Stockholm, Sweden
Alzheimer’s disease (AD) is the most common neurodegenerative disease affecting a growing number of elderly individuals. No disease-modifying drugs have yet been identified despite over 30 years of research on the topic, showing the need for further research on this multifactorial disease. In addition to the accumulation of amyloid β-peptide (Aβ) and hyperphosphorylated tau (p-tau), several other alterations have been associated with AD such as calcium (Ca2+) signaling, glucose-, fatty acid-, cholesterol-, and phospholipid metabolism, inflammation, and mitochondrial dysfunction. Interestingly, all these processes have been associated with the mitochondria–endoplasmic reticulum (ER) contact site (MERCS) signaling hub. We and others have hypothesized that the dysregulated MERCS function may be one of the main pathogenic pathways driving AD pathology. Due to the variety of biological processes overseen at the MERCS, we believe that they constitute unique therapeutic targets to boost the neuronal function and recover neuronal homeostasis. Thus, developing molecules with the capacity to correct and/or modulate the MERCS interplay can unleash unique therapeutic opportunities for AD. The potential pharmacological intervention using MERCS modulators in different models of AD is currently under investigation. Here, we survey small molecules with the potential to modulate MERCS structures and functions and restore neuronal homeostasis in AD. We will focus on recently reported examples and provide an overview of the current challenges and future perspectives to develop MERCS modulators in the context of translational research.
1 Background
Alzheimer’s disease (AD) is a neurodegenerative disorder primarily associated with dementia and pathological features such as extensive neuronal and synaptic loss, accumulation of plaques consisting of amyloid β-peptide (Aβ), and intracellular neurofibrillary tangles (NFTs) formed by hyperphosphorylated tau (p-tau) protein (Braak and Braak, 1991). AD is classified as familial AD (fAD) and sporadic AD (sAD). Most AD cases are sporadic and still lack a clear genetic or environmental component. fAD accounts for less than 1% of diagnosed cases and is caused by the inheritance of autosomal mutations in three genes: amyloid precursor protein (APP), and presenilin (PS) 1 and 2. The amyloid hypothesis has been dominating the field, which assumes that increased proteolytic cleavage of APP and decreased clearance of Aβ, result in several downstream effects leading to tau phosphorylation and aggregation, synapse dysfunction, and neuronal death. However, this theory fails to explain several features of the disorder, thoroughly reviewed in Morris et al. (2014). When the role of Aβ on neurotoxicity is undeniable, AD research is shifting toward considering the disorder as a multifactorial disease, characterized by different types of microdamage and different factors that lead to cognitive decline (Sasaguri et al., 2017).
The drug development pipeline to find a cure or treatment for AD has continuously been challenged and has focused mainly on the amyloid deposition aspects of the disease. Recent evidence suggests that before cognitive decline commences, Aβ accumulation initiates, peaks, and decelerates. Therefore, by the time cognitive impairment is clinically observed, Aβ accumulation has already plateaued (Hardy and Selkoe, 2002; Jack et al., 2010; Karran et al., 2011; Selkoe and Hardy, 2016). Indeed, compounds (e.g., bapineuzumab, solanezumab, and gantenerumab) used in clinical trials to either reduce Aβ42 production (e.g., ClinicalTrials.gov identifiers: NCT00470418, NCT01303744, NCT01421056, and NCT00606164) or prevent Aβ aggregation (e.g., NCT00568776 and NCT00934050) have been unsuccessful in slowing down or preventing the pathophysiology of AD (Aisen et al., 2011; Mehta et al., 2017). Although recently the FDA approved immunoglobulin gamma 1 (IgG1) monoclonal antibody aducanumab (brand name Aduhelm) for treatment of certain subgroups of AD patients, a comprehensive analysis of these results is still pending to fully access its neuroprotective effects. The European Medicines Agency (EMA) has recommended that Aduhelm be denied marketing authorization due to its uncertain therapeutic potential and safety concerns (Mahase, 2021). Failures in AD drug development so far might be related to 1) drugs being tested mainly in the late symptomatic stages of AD once irreversible damage has occurred, 2) drugs target advanced stages of disease pathways rather than early modifiable ones, 3) overreliance on the drug development pipeline on fAD mechanisms and amyloid hypothesis, and 4) lack of drive to explore other pathological targets.
Among these underexplored pathological targets in AD drug discovery, reduced glucose metabolism has been reported decades before clinical symptoms manifested (Minoshima et al., 1997; Mosconi, 2005), and multiple studies have revealed mitochondrial dysfunction as an upstream event of Aβ accumulation (Reddy et al., 2004; Lee et al., 2006; Swerdlow, 2012). Indeed, electron transport chain (ETC) inhibition results in upregulated tau phosphorylation and amylogenic pathway activation (Lovell et al., 2004; Leuner et al., 2012; Esteras et al., 2017). Furthermore, introducing mitochondrial DNA of sAD subjects in mitochondrial DNA-depleted cells increases apoptotic mechanisms such as cytochrome c release and caspase-3 activation (Khan et al., 2000), abnormal intracellular Ca2+ signaling (Sheehan et al., 1997), and oversecretion of Aβ peptides (Khan et al., 2000). Furthermore, decreased mitochondrial protein expression of ETC complexes in the absence of Aβ and tau pathology has been reported in sAD human iPSC-derived neuronal cell models (Birnbaum et al., 2018). Altogether, in the context of clinical trials, these studies suggest that metabolic dysfunctions may precede amyloid pathology, and early treatment in the prodromal stage may increase the likelihood of slowing down AD progression. Drug delivery strategies that work directly or indirectly on mitochondria have been established and studied in neurodegenerative disorders (Cenini and Voos, 2019). Several classes of drugs, with different modes of action, have been tested to tackle mitochondrial dysfunction in AD including antioxidants, compounds targeting mitochondria membrane potential (ΔΨm), and inhibiting mitochondria permeability transition pore (mPTP) opening, as well as natural compounds from the flavonoid family. When mitotherapeutics are gaining momentum, only 10 phase III clinical trials incorporating new drugs for the treatment of mitochondrial illnesses are currently registered, and only one of these 10 trials has been completed (Weissig, 2020). Regarding AD, currently, 12% of drugs under clinical trials in phase III have been shown to impact on metabolism and bioenergetics, showing the promising potential of targeting mitochondria in AD (Cummings et al., 2021).
The cellular pathways regulating mitochondrial function and neuropathological AD-related processes converge at mitochondria–endoplasmic reticulum (ER) contact sites (MERCS), intracellular microdomains which have been receiving increased attention from the scientific community. We and others have hypothesized that upregulated MERCS structures and functions may be one of the main events driving AD pathology (Hedskog et al., 2013; Area-Gomez and Schon, 2017), and it is interesting to note that many dysfunctional mechanisms observed in AD are mediated at this signaling platform. In this review, we summarize up-to-date knowledge on MERCS physiology and its alterations in AD, provide a survey of small molecules with the capacity to modulate MERCS structures and functions to restore neuronal homeostasis, and address new directions for the development of small molecules targeting MERCS. We focus on recently reported cases of pharmaceutical agents proven to influence MERCS structures or functions and provide an overview of the current challenges and future perspectives to develop MERCS modulators in the context of translational research.
2 Mitochondria–Endoplasmic Reticulum Contact Sites
2.1 Contactology: a New Field for Organelle Interaction
The interaction of different intracellular organelles has been established as a fascinating new subject in cellular biology, and a growing number of cellular physiological and pathological mechanisms have been attributed to these contacts. Csordás, Weaver, and Hajnóczky introduced the name “contactology” to describe this new field of study (Csordás et al., 2018). Membrane contact sites are intracellular regions where the membranes of two organelles are closely juxtaposed. There are some characteristics that all contact sites present including tethering regulators of proximity between two membranes, a distinct lipidome/proteome from the rest of the membranes, and no fusion events between the two membranes (Scorrano et al., 2019). Contacts between different organelles have been described; nevertheless, all best characterized organelle contacts involve interaction with the ER. Contacts between the ER and the plasma membrane, the Golgi apparatus, the vacuole/lysosome, and mitochondria mediate Ca2+ homeostasis and lipid shuttling. Additionally, ER interactions with the vacuole/lysosome and mitochondria have been shown to mediate microautophagy and autophagosome formation, respectively (Holthuis and Ungermann, 2013).
2.2 Mitochondria–Endoplasmic Reticulum Contact Sites
Mitochondria need to communicate with other organelles, particularly the ER, in order to regulate their functions. Mitochondria-associated ER membranes (MAMs) are lipid raft-like domains in the ER that can interact with OMM. The MAM is an insoluble lipid raft that differs biochemically from the pure ER and pure mitochondria. MERCS are formed when approximately 20% of the mitochondrial surface is closely apposed to MAM (10 to 30 nm distance) (Csordás and Hajnóczky, 2001; Csordás et al., 2006; Paillusson et al., 2016). MERCS were first observed on electron micrographs of the rat liver in the 1950s (Bernhard and Rouiller, 1956). These subcellular regions drew additional attention when Jean Vance identified subcellular fractions enriched in the MAM in the early 1990s, then known as fraction X (Vance, 1990). Since then, the contactology field has advanced significantly, and considerable efforts have been made by the scientific community to characterize the mechanisms that connect the ER to mitochondria.
2.3 Linking the Two Membranes: Mitochondria–Endoplasmic Reticulum Contact Site Tethers and Contact Regulators
Generally, 10–30 nm distance between the ER and mitochondria allows for protein-mediated tethers to be formed. The presence of protein bridges between the two structures has been confirmed by the ability of proteolytic agents to untether the two organelles (Csordás et al., 2006). In yeast, the tethering structure linking the two membranes has been thoroughly characterized by containing OMM proteins Mdm34 and Mdm10, cytosolic protein Mdm12, and ER transmembrane protein Mmm1 forming together as the ER–mitochondria encounter structure (ERMES) complex (Puri et al., 2019). So far, no ERMES homologs in mammals have been identified. The mammalian proteins involved in MERCS juxtaposition have been identified upon manipulation of the examined proteins by analyzing proximity and MERCS functional alteration, such as Ca2+ shuttling between the ER and mitochondria (Herrera-Cruz and Simmen, 2017). The mammalian proteins that have thus far been discovered are summarized in this study.
Phosphofurin acid cluster sorting protein 2 (PACS2) was identified as the first mediator of the ER to mitochondria juxtaposition in mammalian cells. Considering PACS2 is a cytoplasmic protein rather than a MERCS tether; it may indirectly couple the ER to the mitochondria through upstream pathways. Nonetheless, knocking down this protein reduces MERCS and Ca2+ shuttling from the ER to the mitochondria and has been used in earlier studies in the field to modulate MERCS structures and functions (Simmen et al., 2005).
Homodimeric and heterodimeric interactions between mitofusin 1 (Mfn1) and mitofusin 2 (Mfn2), proteins involved in mitochondrial fusion, were first described as a scaffolding bridge between the ER and mitochondria (De Brito and Scorrano, 2008). Studies have shown that deleting the Mfn2 gene or conditionally knocking down Mfn2 reduces the mitochondria–ER interaction in a variety of models including in cell lines (Alford et al., 2012; Sugiura et al., 2013), neurons (Schneeberger et al., 2013), and astrocytes in vivo (Gӧbel et al., 2020). Several investigations, however, have suggested that Mfn2 knockdown promotes MERCS apposition and Ca2+ shuttling, implying that this protein acts as a negative regulator of contact formation (Cosson et al., 2012; Filadi et al., 2015; Leal et al., 2016; Cieri et al., 2018a; Dentoni et al., 2022). These differences may be attributed to different cell models and culturing methods employed, variable compensatory mechanisms from Mfn2 knockout or knockdown, and methods used to measure the ER to mitochondria proximity, which have been thoroughly reviewed by Filadi et al. (2018a). Regardless of its role, Mfn2 manipulation has been used and characterized as a method to modify ER–mitochondria proximity.
Another pair of proteins working as bona fide tethers are the OMM protein tyrosine–phosphatase-interacting protein 51 (PTPIP51) and the ER protein vesicle-associated membrane protein-associated protein B (VAPB). Indeed, knocking down either protein reduces apposition between the two organelles, while overexpression has the opposite effect (De Vos et al., 2012; Stoica et al., 2014). In particular, knocking down either protein prevents the ER to mitochondria Ca2+ shuttling, whereas overexpression increases the functional coupling between the two organelles (Stoica et al., 2014; Puri et al., 2019).
PDZD8, an ER transmembrane protein, has been identified through a modeling screening approach, whereby the synaptotagmin-like mitochondrial-lipid-binding (SMP) domain homology between ERMES proteins and mammalian proteins was assessed. Indeed, the PDZD8 SMP domain shows substantial homology to the ERMES ER protein Mmm1. PDZD8 knockout or knockdown revealed substantial downregulation of MERCS apposition and Ca2+ shuttling in cell lines and neurons, shaping dendritic Ca2+ fluxes. PDZD8 mitochondrial tethering counterpart remains undetected (Hirabayashi et al., 2017).
The IP3R-Grp75-VDAC bridge is a complex constituted by the inositol 1,4,5-triphosphate receptor (IP3R), glucose-regulated protein 75 (Grp75), and VDAC (voltage-dependent anion channel). Grp75 allows for close apposition of IP3R, a key ER Ca2+ release channel, to the OMM and VDAC (Szabadkai et al., 2006), hence constituting the main route of Ca2+ transfer from the ER to mitochondria. Previous research has shown that deleting IP3Rs has no effect on the distance between the two organelles (Csordas et al., 2006); this suggests that their apposition to VDAC is purely functional in mediating Ca2+ transfer. However, in recent investigations on IP3R-deficient (triple knockout (KO)) DT40 cells, where individual IP3R isoforms were recovered, each IP3R isoform restored Ca2+ shuttling and ER–mitochondrial proximity in triple KO cells, indicating a structural involvement in MERCS juxtaposition (Bartok et al., 2019).
Other less studied tethering proteins have been identified in mammalian cells, including B-cell receptor-associated protein 31 (BAP31) and Fis1 (Iwasawa et al., 2011), FATE1 (Doghman-Bouguerra et al., 2016), transglutaminase type 2 (TG2) (D’Eletto et al., 2018), and FK506-binding protein 8 (FKBP8) (Kwak et al., 2020).
Although several approaches have been employed to evaluate tethering mechanisms regulating the ER to mitochondria juxtaposition, only a few studies have looked directly at the proteome of these structures. To date, three proteomic investigations on mouse brain fractions enriched in the MERCS have been conducted, and between 1000 and 2400 proteins have been discovered (Poston et al., 2013; Ma et al., 2017; Wang et al., 2018). A comparison of previously described datasets identified 648 common proteins present in mouse brain MERCS-enriched fractions, including MERCS functional and structural tethering proteins such as PACS, Mfn2, VAPB, IP3R1, and all VDAC isoforms (Magalhães Rebelo et al., 2020). Interestingly, when comparing the proteome of the MERCS-enriched fraction from the brain to the proteome from other tissues and cells, such as the testis, liver, and NG108-15 cell line, only 18 proteins were shown to be common within these databases (Magalhães Rebelo et al., 2020), suggesting a tissue-specific enrichment of proteins at the MERCS (Leal and Martins, 2021).
2.4 Mitochondria–Endoplasmic Reticulum Contact Sites’ Functions: a Physiological Subcellular Hub
Several proteins have been identified at MERCS acting as functional rather than structural mediators of juxtaposition between the two membranes. These proteins play diverse physiological roles in this subcellular domain (Figure 1).
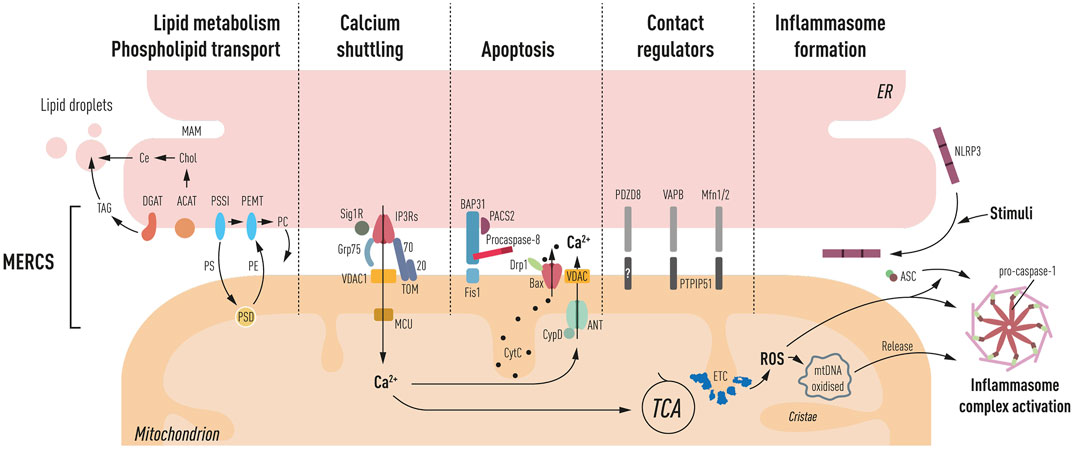
FIGURE 1. Schematic representation of processes ascribed at MERCS: lipid metabolism and phospholipid transport. PS, phosphatidylserine; PE, phosphatidylethanolamine; PC, phosphatidylcholine; shuttling occurs through MAM, OMM, and IMM triple contact sites, and PSSI, PSD, and PEMT mediate their synthesis, respectively. Furthermore, the synthesis of cholesteryl esters (Ce) through ACAT and triglycerides (TAG) through DGAT occurs at the MAM, which is the main component of lipid droplets, generated close to the ER and mitochondria interface. Ca2+ shuttling between the ER and mitochondria is mediated by IP3Rs. Grp75 mediating close juxtaposition of IP3Rs to VDAC in the OMM and MCU in IMM facilitates Ca2+ entry into the matrix leading to TCA cycle activation. Sig1R and TOM70 mediate the IP3R activity. Apoptosis PACS-2, a multifunctional sorting protein, by localizing to the MAM regulates BAP31 interaction with Fis1, mediating a platform for procaspase-8 activation. Increased Ca2+ in the matrix can lead to activation of mPTP (CyD, ANT, and VDAC). Bax has also been located at MERCS, and upon apoptosis induction, it leads to the formation of pores and cytochrome c (CytC) release. Contact regulators’ several proteins have been shown to have a role in mediating ER to mitochondria apposition including PDZD8, VAPB–PTPIP51, and Mfn2, a negative regulator of MERCS. Inflammasome formation NLRP3 under resting conditions is located in the ER membrane, upon inflammatory stimuli recruits ASC and redistributes at the MAM. Mitochondrial ROS production and oxidized DNA release induce the activation of the autophagosome complex, consisting of NLRP3, ASC, and pro-caspase-1 propagating the inflammatory response.
2.4.1 Endoplasmic Reticulum to Mitochondria Ca2+ Shuttling
MERCS play an important role in the ER to mitochondria Ca2+ shuttling, generating high Ca2+ hotspots (Ca2+>10 µM) at the interface between the two membranes, allowing the activation of low-affinity calcium transporters MCU while avoiding an overall cellular rise in Ca2+ (Rizzuto et al., 1993). Mitochondrial Ca2+ import is carried out through the IP3R-Grp75-VDAC bridge and plays a central bioenergetic function by activating tricarboxylic acid (TCA) cycle dehydrogenases and boosting ATP production (Jouaville et al., 1999; Cardenas et al., 2010a) (depicted in Figure 1), which seems to be the preferential method for boosting metabolism compared to the cytosolic Ca2+ influx (Rohács et al., 1997). The ryanodine receptor (RyR) and IP3R are the main Ca2+ extrusion channels in the ER. RyR has also been found at MERCS and, like IP3R, it regulates Ca2+ shuttling to mitochondria; however, most research on RyR has been conducted in muscle cells (Csordás and Hajnóczky, 2001). This ion’s under- or over-shuttling, as well as the activation of Ca2+ channels, can have negative consequences for cellular physiology. Reduced Ca2+ shuttling can induce a bioenergetic crisis, whereas pathologically elevated Ca2+ levels can promote mitophagy and death (Rowland and Voeltz, 2012). Ca2+ responses can be modulated by several proteins in this subcellular area. MERCS-resident protein sigma-1 receptor (Sig1R) modulates the IP3R activity through ankyrin 2 (Ank2) interaction, stabilizing IP3Rs during conditions of high calcium transfer (Hayashi and Su, 2007). Importantly, in Sig1R knockout motor neurons, there is a significant reduction of connections between the two organelles, and whether this is dependent on its interaction with IP3Rs remains unknown (Bernard-Marissal et al., 2015). Upon ER Ca2+ depletion and ER stress, Sig1R increases Ca2+ shuttling to mitochondria resulting in enhanced ATP (Hayashi and Su, 2007). Furthermore, we discovered that TOM70 operates as an ER–Ca2+ regulator by interacting with IP3R3, facilitating Ca2+ shuttling at MERCS, and influencing bioenergetics, cell proliferation, and autophagy (Filadi et al., 2018c). Other proteins involved in the stabilization of the IP3R-Grp75-VDAC bridge include Tespa-1 (Matsuzaki et al., 2013), transglutaminase type 2 (TGM2) (D’Eletto et al., 2018), and DJ-1 (Liu et al., 2019). The sacro/endoplasmic reticulum (SR/ER) Ca2+ ATPase (SERCA), which replenishes intracellular ER Ca2+ stores, has also been shown to control ER–mitochondria Ca2+ transfer by attenuating mitochondrial Ca2+ uptake during continuous Ca2+ release at MERCS (Csordás and Hajnóczky, 2001). ER Ca2+ modulators found at MERCS controlling the SERCA activity include calnexin (CNX) (Roderick et al., 2000), calreticulin (John et al., 1998), ERp57 (Li and Camacho, 2004), and the thioredoxin-related transmembrane protein (TMX1) (Raturi et al., 2016).
2.4.2 Phospholipid Shuttling, Cholesterol, and Lipid Metabolism
MAM is a lipid raft domain enriched in cholesterol and sphingolipids, which facilitates the stabilization of specific proteins enriched in these membranes (Vance, 2014). MERCS also play a role in the phospholipid inter-organelle transport by enabling their exchange between the two organelles (Vance, 1990). Indeed, phosphatidylserine (PS) is synthesized at the MAM and then transported to the mitochondria OMM where it is converted into phosphatidylethanolamine (PE) (Vance, 1990) through a triple contact site formed with the ER, outer mitochondrial membrane (OMM), and inner mitochondrial membrane (IMM) (Ardail et al., 1993). PE can remain in the IMM or be transported back to the ER (Vance, 1991). Next, PE can be further modified in the ER to phosphatidylcholine (PC) (depicted in Figure 1). Furthermore, MERCS play a vital role in the metabolism of other lipids, including cholesterol and triglycerides (Vance, 2014). The major components of lipid droplets are triglycerides and sterol esters (Guo et al., 2009), which have been demonstrated to form near the ER–mitochondria contact interface (Perreault et al., 2009). Accordingly, lipid droplet formation has been used as an indirect way to assess phospholipid-related MERCS functions (Area-Gomez et al., 2012; Del Prete et al., 2017). Recently, MERCS tethering protein PTPIP51 has been shown to work as a phospholipid transfer protein. PTPIP51 depletion reduced levels of mitochondrial cardiolipin in cells, a mitochondrial lipid almost exclusively found within the IMM (Yeo et al., 2021).
2.4.3 Initiation of Autophagosome Formation
Autophagosome membrane formation has been identified as another physiological function attributed to MERCS (Hamasaki et al., 2013; Garofalo et al., 2016). MERCS tethering protein VAPB or PTPIP51 overexpression increases ER–mitochondria contacts, impairing autophagosome formation, while downregulation of these proteins decreases contacts, upregulating autophagosome formation (Gomez-Suaga et al., 2017). It is interesting to note that in MERCS where autophagosomes are generated, the distance between the two membranes is further spaced out (50 nm), compared with MERCS mediating Ca2+ and lipid transfer (10–30 nm), to adapt to the growing autophagosome membrane formation (Giacomello and Pellegrini, 2016). Thus, MERCS appears to be an active membrane assembly area due to its phospholipid exchange and synthesis of autophagosome membranes.
2.4.4 Apoptosis and Mitochondrial Fission
Although increased apposition between the two organelles is fundamental for bioenergetics, sustained increased connectivity makes mitochondria prone to Ca2+ overloading, ensuing mPTP activation (Csordas et al., 2006). Furthermore, ER tethering protein BAP31 interacts with its mitochondrial partner Fis1, which together create a platform for the activation of procaspase 8 (Iwasawa et al., 2011); accordingly, depletion of Fis1 has been shown to delay apoptosis (Lee et al., 2004).
Drp1 mediates mitochondrial division by being recruited at MERCS (Friedman et al., 2011a) via the ER-resident inverted formin 2 (INF2) (Korobova et al., 2013). This process requires polymerization of actin near constriction sites and the ER to mitochondria Ca2+ shuttling (Chakrabarti et al., 2017). Interestingly, Drp1 is recruited to the mitochondria by the apoptotic mediator Bax during apoptosis at MERCS (Wasiak et al., 2007). Bax and Drp1 colocalization at MERCS coordinate the activation of mitochondria outer membrane permeabilization (MOMP), hence mechanistically linking mitochondria fragmentation to apoptosis.
2.4.5 ROS Production and Inflammasome Formation
Communication between the ER and mitochondria is also important for redox signaling between the two organelles. Ca2+ shuttling at MERCS can boost mitochondrial-derived ROS generation, resulting in the generation of MERCS ROS nanodomains (Booth et al., 2016). Indeed, ROS can influence the activity of ER Ca2+ channels such as RyR and IP3Rs (Bogeski et al., 2011), thus establishing a bidirectional communication at MERCS.
Moreover, ROS production at MERCS has been shown to induce the assembly and activation of the inflammasome (Zhou et al., 2011), a protein complex formed by nucleotide-binding oligomerization domain (NOD)-like receptor protein 3 (NLRP3), adapter protein apoptosis-associated speck-like protein containing a CARD (ASC), and caspase-1 which results in cleavage and secretion of cytokines, propagating the inflammatory response (Yabal et al., 2019). NLRP3 is activated by a variety of stimuli including ion flow disruption across the plasma membrane, mitochondrial ROS and oxidized mitochondrial DNA (mtDNA) release, lysosomal membrane disruption, and bacterial or viral infection (Missiroli et al., 2018). Interestingly, NLRP3 and ASC have been shown to translocate to MERCS upon triggering stimuli, activating caspase-1 (Zhou et al., 2011; Namgaladze et al., 2019) (depicted in Figure 1). Furthermore, evidence points to Ca2+ shuttling between the ER and mitochondria being important for the activation of inflammasomes (Murakami et al., 2012). IP3R inhibition resulted in decreased ROS, mtDNA release, and secretion of cytokines (Triantafilou et al., 2013). From the aforementioned evidence, the NLRP3 inflammasome’s presence at MERCS is strategically placed to detect stress-related mitochondrial signals. The mechanism underlying the ER to mitochondria apposition influencing inflammasome formation and activation remains unexplored.
3 Mitochondria–Endoplasmic Reticulum Contact Sites and Neurodegeneration
MERCS are involved in a wide range of cellular mechanisms, as mentioned in the previous section. It is not surprising, then, that MERCS dysfunction has been linked to several neurodegenerative disorders. Although it is uncertain whether MERCS play an active function in disease development, numerous pieces of evidence suggest that MERCS and pathologically associated proteins found at MERCS are important players in various neurodegenerative disorders (Paillusson et al., 2016). In amyotrophic lateral sclerosis (ALS), a neuromuscular neurodegenerative disorder that causes gradual loss of motor neurons, overexpression of TDP-43, one of the thought initiators of disease, dampens the ER to mitochondria apposition via the VAPB-PTPIP51 tethering complex (Stoica et al., 2014). Dopaminergic neuronal loss and cytosolic α-synuclein aggregates have been detected in Parkinson’s disease (PD) patients’ brains. α-synuclein has been localized at MERCS, and mutant forms of this protein disrupt the ER to mitochondria apposition (Paillusson et al., 2017). Huntington’s disease (HD) is an autosomal dominant neurodegenerative condition in which mutant Huntingtin protein (Htt) accumulates in the nucleus. Htt disrupts ER–mitochondrial interactions, resulting in the dampening of Ca2+ signaling, in the striatum of HD mutant mice (Cherubini et al., 2020).
3.1 Mitochondria–Endoplasmic Reticulum Contact Sites in Alzheimer’s Disease Pathology
The scientific community has given special attention to MERCS in the context of AD pathogenesis, with evidence pointing to the increased ER to mitochondria apposition as a key factor in AD pathogenesis (Area-Gomez et al., 2018). Area-Gomez et al. (2009) pioneered the discovery of the presence of APP, as well as the γ-secretase components PS1 and PS2, in the MERCS-enriched fraction of mouse brains. Furthermore, studies on SH-SY5Y cells carrying PS2 FAD mutations (T122R) showed for the first time increased ER to mitochondria apposition and Ca2+ shuttling (Zampese et al., 2011). Indeed, PS2 was shown to interact with the negative regulator of MERCS, Mfn2 (Filadi et al., 2016). An increase in the MERCS number was observed in fAD fibroblasts and sAD fibroblasts (Area-Gomez et al., 2012), mouse and rat primary neurons treated with Aβ (Hedskog et al., 2013; Calvo-Rodríguez et al., 2016; Calvo-Rodriguez et al., 2019), SH-SY5Y cells overexpressing APP (Del Prete et al., 2017), and the hippocampus of APP knock-in AppNL-F and AppNL-G-F mice (Leal et al., 2020). We have also recently demonstrated that inhibiting oAβ selectively, using the Aβ-neutralizing scFvA13 antibody, reversed the increase in MERCS number, implying that oAβ peptides have a direct and specific effect on contact dynamics (Leal et al., 2020). Further evidence has been emerging connecting MERCS and AD. For example, membrane cellular fractionation experiments on adult mouse brains reveal that Aβ is present in the MERCS-enriched fraction along with the APP-processing machinery including β-secretase and active γ-secretase (Schreiner et al., 2015; Del Prete et al., 2017). The unprocessed APP fragment C99 has been localized at the MAM and shown to increase the MERCS activity and apposition (Pera et al., 2017). Not only are these components present at MERCS but also MERCS themselves are capable of modulating Aβ production. MFN KO cells and conditional knockdown of contact regulator Mfn2 was accompanied by decreased γ-secretase activity (Area-Gomez et al., 2012) and Aβ production (Leal et al., 2016). The exact mechanism linking MERCS and Aβ production is currently unknown.
To date, limited studies have analyzed the role of tau in ER–mitochondria contacts. Tau has been localized at both the mitochondria and ER (Perreault et al., 2009; Cieri et al., 2018a). Mice overexpressing P301L tau showed upregulated juxtaposition between the two organelles in spinal cord motor neurons (Perreault et al., 2009). One study showed that the truncated caspase 3-cleaved 2N4RΔC20 tau protein induced fibrillation and seeding of wild-type (WT) tau, leading to the increased ER to mitochondria proximity (Cieri et al., 2018b). Whether tau affects MERCS apposition itself remains elusive; target validation studies should address this important question.
So far only two studies have reported the decreased ER to mitochondria proximity in AD models. Lower MERCS apposition has been shown in transgenic rats overexpressing APP at a 10-nm distance using a FRET system of proximity detection, while MERCS length investigated using the EM was also reported; however, number of contacts or distance of contacts were not investigated using the EM (Martino Adami et al., 2019). Accordingly, in the postmortem sAD brain cortex using a proximity ligation assay (PLA), fewer VAPB-PTPIP51 appositions were detected in early to mid-Braak stages (Lau et al., 2020a). These conflicting results might be due to less sensitive techniques, which measure indirect ER to mitochondria apposition, compared with transmission electron microscopy (TEM) studies.
Regardless of these discrepancies, it appears that MERCS play a substantial role in the progression of AD pathology, with most studies reporting increased ER to mitochondria contacts as a pathological phenotype in AD models.
3.2 Increased Contacts in Alzheimer’s Disease—Beneficial or Harmful?
MERCS role in AD pathogenesis is a relatively recent field, and although extensive research has been carried out in the last decade to understand the basic mechanism and nature of MERCS, several questions remain withstanding. Of relevance, the increased proximity between the ER and mitochondria in AD has been shown to have both beneficial and deleterious effects in different models. Indeed, it is important to note that MERCS are not static entities; they are dynamic structures that change depending on the bioenergetic needs of the cell (Sood et al., 2014). We believe that increased MERCS in AD might be an early compensatory mechanism to offset changes in the cellular environment and increase ATP production to sustain its compromised function. This hypothesis agrees with a recent study showing that increasing the ER to mitochondria apposition restores climbing ability and increased lifespan in Αβ42 overexpressing flies (Garrido-Maraver et al., 2020). Furthermore, we have, in recent studies, found that increased mitochondrial activity precedes amyloid deposition in AppNL-G-F mice (Naia et al., unpublished) and in AppNL-F embryonically derived primary neurons (Dentoni et al., unpublished), suggesting that increased MERCS and hypermetabolism are an early feature of the disease. However, on the other hand, a sustained increase in MERCS can have deleterious effects on the cell, eventually leading to apoptosis (Csordas et al., 2006). Increased retention of Ca2+ was detected in AD models implying that high and sustained levels of Ca2+ can induce mitochondrial matrix overload and Ca2+-activated mitochondrial permeability transition pore opening, resulting in age-associated cognitive decline in AD models (Jadiya et al., 2019). Furthermore, a recent preprint has shown that artificially increasing MERCS block mitochondrial movement in axons, resulting in deformed neuromuscular junctions in flies (Hewitt et al., 2020). From this evidence, we believe that when an early upregulation of MERCS might be necessary to boost bioenergetics, as pathology progresses, this compensatory mechanism might lead to apoptosis due to overactive Ca2+ shuttling. Therefore, it is fundamental to assess how modulation of contacts at specific timepoints of pathogenies could be beneficial for the disease. Due to the conflicting evidence in the literature reported, it would be important to test strategies that both increase and decrease contacts and observe how this relates to changes in the pathogenesis of the disorder, its progression, and neuroprotective properties of specific MERCS modulators. Indeed, it might be necessary to use strategies to increase MERCS early while pharmacological blockage of the ER to mitochondria apposition might be necessary for patients with advanced AD.
4 Use of Small Molecules to Modulate Mitochondria–Endoplasmic Reticulum Contact Sites’ Function and Restore Neuronal Homeostasis in Alzheimer’s Disease
Developing molecules to correct and/or modulate the mitochondria–ER interplay can unleash unique therapeutic opportunities in AD. Recently, MERCS modulators have been categorized based on either their interaction with MERC-resident proteins, protein expression levels, or upstream signaling cascades (Magalhães Rebelo et al., 2020). For this review, we have categorized MERCS modulators slightly different based on 1) their interaction with tethering or contact regulators, 2) interaction with functional modulators at MERCS, and 3) upstream signaling cascades. We believe that there is an opportunity of targeting MERCS with different small molecules to modulate its morphology and/or functions. In doing so, we could stimulate downstream cascades that can either promote cellular homeostasis or sensitize to current AD therapies or help restore cellular homeostasis in the prodromal stage of AD. In the following section, we surveyed small molecules with MERCS modulatory potential both structurally and functionally (MERCS modulators), aiming to restore neuronal homeostasis in AD, summarized in Figure 2. In addition, we collected the molecular structure, associated MERCS molecular target, and its observed effect on MERCS from different publications (Table 1). Although some molecules have not yet been tested in AD models, we believe their proven MERCS role or their interaction with MERCS components has the potential to restore neuronal homeostasis. We set a stringent criterion to select molecules with proven MERCS modulatory effect or molecules having an extremely likely effect on this subcellular region, due to their interaction with key tethering proteins at MERCS. Indeed, analyzing the literature on the subject, several molecules have been shown to alter levels of tethering and non-tethering proteins at MERCS; however, whether changes in these proteins translate to changes in structures or functions remain to be debated.
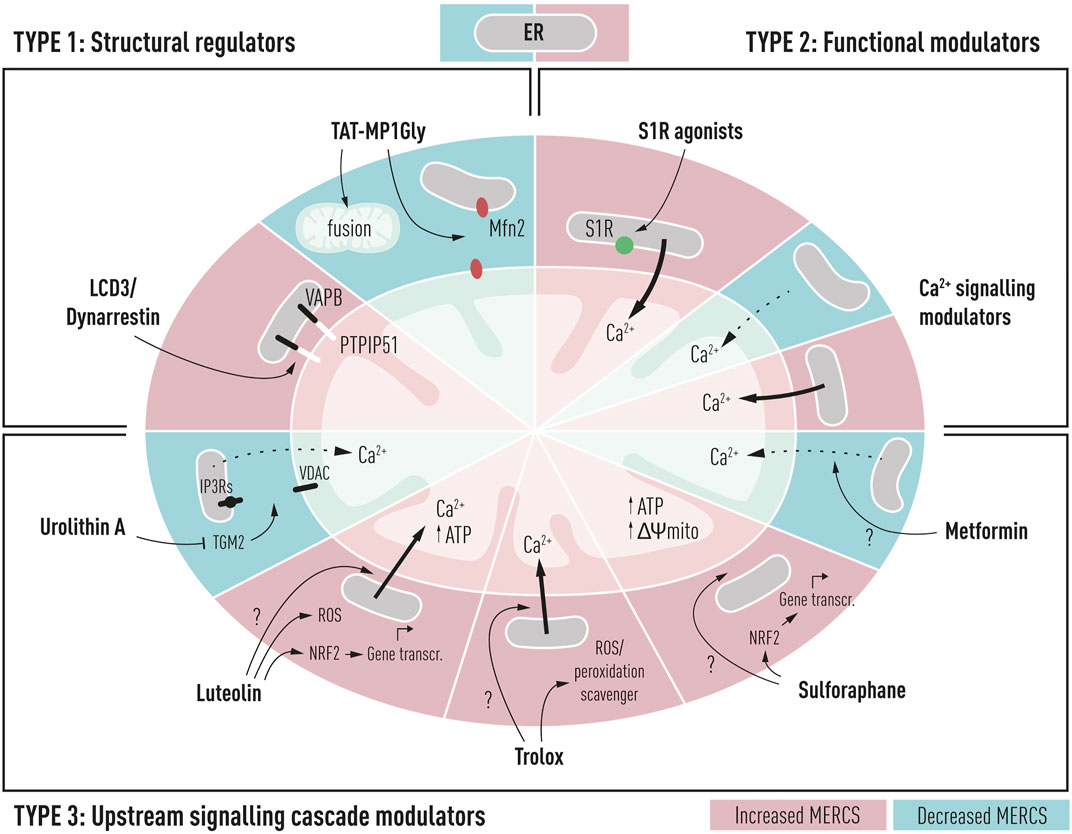
FIGURE 2. Schematic representation of potential drug targets for the modulation of mitochondria–ER contacts in Alzheimer’s disease and their cellular and mitochondrial effects. TYPE 1: structural regulators such as LCD3/Dynarrestin and TAT-MP1Gly can interact directly with structural regulators of contacts such as PTPIP51 and Mfn2 resulting in increased or decreased proximity between the two membranes. TYPE 2: functional modulators could be used to alter the ER to mitochondria functional proximity, through modulation of Ca2+ shuttling between the ER and mitochondria; such compounds include sigma-1 receptor agonists (S1R) and Ca2+ signaling modulators targeting IP3Rs, VDAC, or MCU complex. TYPE 3: upstream signaling cascade modulators alter proximity between two membranes, but their specific target is unknown. These compounds could affect upstream signaling cascades, leading to the alteration of MERCS proximity. These compounds include metformin, sulforaphane, Trolox, luteolin, and urolithin A.
4.1 Structural Regulators
4.1.1 LDC3/Dynarrestin
LDC3/Dynarrestin is a small aminothiazole inhibitor of cytoplasmic dynein 1 and 2 (Höing et al., 2018). The molecule interferes with the Hedgehog pathway by the inhibition of dynein, affecting the endosome movement and mitosis. PTPIP51 can activate the MAPK pathway via Raf1 coupling. One study has shown that the knockdown of PTPIP51 abolishes the MAPK stimulating effect of LDC3/Dynarrestin normally induced by the PTPIP51/14-3-3/Raf1 interactome (Brobeil et al., 2017).
4.1.1.1 Mitochondria–Endoplasmic Reticulum Contact Sites’ Modulation
In a recent small high-throughput screen, LCD3/Dynarrestin was shown to possess a high affinity to PTPIP51 (Dietel et al., 2019). PTPIP51 acts as a linker to bind to VAPB and regulate Ca2+ homeostasis and autophagy, Figure 1 (Stoica et al., 2014; Gomez-Suaga et al., 2017). In the original study, LCD3/Dynarrestin increased the PTPIP51 interaction with VAPB, having its highest effect at high concentrations of 5 and 50 µM for 24–48 h, hinting to a role of this molecule in modulating MERCS. Whether this molecule affects the MERCS ultrastructure and functions is not known; however, an MTT assay was performed to assess the mitochondrial metabolic activity upon treatment. No changes were seen in the MTT assay up to 5 µM concentration, while at 50 µM of LCD3/Dynarrestin for 24 h impaired the mitochondrial metabolic activity (Dietel et al., 2019).
4.1.1.2 Therapeutic Potential in Alzheimer’s Disease and Clinical Trial
Modulation of PTPIP51 with LCD3/Dynarrestin could modify related signaling pathways in AD and create a “cascade-like” Ca2+ homeostasis, due to its potential in modulating the ER to mitochondria apposition. The increased interaction between PTPIP51 and VAPB is thought to result in a more stable ER–mitochondria juxtaposition. Although increased interaction between the ER and mitochondria has been observed in AD, in early AD postmortem brains, lower levels of VAPB and decreased proximity ligation assay immunoreactivity between VAPB and PTPIP51 have also been reported (Lau et al., 2020b). As a result, this molecule may have a beneficial effect in stabilizing such couple interactions or further supporting metabolism through ER to mitochondria Ca2+ shuttling. However, in the original study, the authors also argue that LCD3/Dynarrestin regulates this interaction for longer incubation times and at high concentrations, suggesting that the increased PTPIP51 and VAPB interaction may be caused by secondary mechanisms (Dietel et al., 2019). Future studies on this molecule shall shed light on these unanswered questions.
4.1.2 TAT-MP1Gly
TAT-MP1Gly is a cell-permeant peptide, based on the residues 367–384 of Mfn2 and the residues 47–57 of the HIV transactivator protein, capable of modifying Mfn2 structural conformation and thought to promote Mfn2-dependent mitochondrial fusion (Franco et al., 2016a).
4.1.2.1 Mitochondria–Endoplasmic Reticulum Contact Sites’ Modulation
Charcot–Marie–Tooth disease type 2A (CMT2A) is a hereditary neurological disorder characterized by motor and sensory neuropathy of the peripheral nervous system, leading to foot and lower leg muscle weakness and paralysis. Mfn2 autosomal dominant mutations cause pathological mitochondrial fragmentation in CMT2A. TAT-MP1Gly treatment for 24 h reversed mitochondrial fragmentation, clumping, and mitochondrial depolarization in vitro from mouse neurons expressing either the artificial GTPase-deficient Mfn2K109A mutant or the naturally occurring human Charcot–Marie–Tooth disease type 2A (CMT2A) GTPase mutant, Mfn2T105M (Franco et al., 2016b). Several lines of evidence point to impaired ER–mitochondria tethering both in mouse and human fibroblasts as a pathological feature of Mfn2-mediated CMT2A, resulting in decreased ER to mitochondria apposition and Ca2+ shuttling (Bernard-Marissal et al., 2019).
4.2.2.2 Therapeutic Potential in Alzheimer’s Disease and Clinical Trial
In AD, Mfn2 levels have been shown to be downregulated both in vitro, in vivo, and in postmortem brains (Wang et al., 2009; Leal et al., 2020). Functionally, AD mitochondria appear smaller and fragmented likely due to Mfn2 loss of function (Wang et al., 2009). Mfn2 has also been implicated in MERCS dynamics in vitro in AD (Leal et al., 2020). Similar to CMT2A, AD is characterized by the widespread fragmented mitochondria, and enhancing the Mfn2 function might have a positive effect on mitochondrial dynamics (Wang et al., 2020). As Mfn2 plays an important role either as a tethering or negative regulator of contacts, conformational changes in Mfn2 could likely correct changes in MERCS and restore mitochondrial dynamics in AD models. No studies so far have assessed the MERCS-dependent role of these peptides in AD or in CM2A. We believe that Mfn2 modulation could have benefits for AD by both reestablishing mitochondrial dynamics and either directly or indirectly restoring MERCS homeostasis. As we believe Mfn2 plays as a negative regulator at MERCS, these peptides might enhance the Mfn2 spacer activity at MERCS, normalizing its physiology.
4.2 Functional Modulators
4.2.1 Sigma-1 Receptor Agonists
The sigma-1 receptor (S1R) is enriched at MERCS, where it stabilizes IP3R, regulating the IP3R-mediated ER to mitochondrial Ca2+ transfer (Figure 1) (Hayashi and Su, 2007). Several S1R agonists have been tested and shown to have positive effects on neurodegenerative disorders including pridopidine (Ryskamp D. A. et al., 2019).
4.2.1.1 Mitochondria–Endoplasmic Reticulum Contact Sites’ Modulation
In HD models where ER to mitochondria apposition is decreased, pridopidine promotes normal IP3R-dependent Ca2+ release, as well as activation of critical Ca2+-regulating genes (Eddings et al., 2019). Furthermore, pridopidine treatment restored MERCS connectivity and improved co-localization of IP3R3 and its chaperone S1R within mitochondria in striatal neurons isolated from YAC128 mice. By affecting the MERCS signaling platform, mitochondrial respiration, dynamics, and motility were recovered in pridopidine-treated YAC128 neurons (Naia et al., 2021a). In AD models such as PS1-KI mice, administration of pridopidine stabilized mushroom spines in vivo and in vitro through a Ca2+-ER-dependent mechanism (Ryskamp et al., 2017), suggesting the mechanistic involvement of MERCS; however, no study in AD has made the connection between MERCS dynamics and pridopidine treatment. Although it is known that pridopidine affects MERCS-resident S1R, whether this agonism influences direct or indirect MERCS through indirect modulation of Ca2+ signaling remains unexplored.
4.2.1.2 Therapeutic Potential in Alzheimer’s Disease and Clinical Trials
Interestingly, S1R polymorphisms have been found to affect the likelihood of acquiring AD, which working synergistically with apolipoprotein E (APOE) increases the risk of AD (Huang et al., 2011). An early postmortem investigation found that the S1R was reduced in the CA1 region of the hippocampus in AD patients. Positron emission tomography (PET) using a radioligand for the receptor was used confirming its depletion in the early stages of AD (Mishina et al., 2008). Among other functions, S1R has been reported to take part in neuromodulation and neuroplasticity, stressing its potential role in affecting memory and cognition (reviewed in (Maurice et al., 2006; Kourrich et al., 2012). Concordantly, different S1R modulators such as pridopidine, N-n-propyl-3-(3-hydroxyphenyl) piperidine (3-PPP), and AF710B (ANAVEX 3–71) displayed neuroprotective properties in animal models of AD, reviewed in Ryskamp D. et al. (2019). Several ongoing trials for HD such as PROOF-HD (Phase 3) (Reilmann et al., 2021) and HEALEY ALS (Phase 2–3) (Paganoni et al., 2022) have been spurred by promising results of pridopidine in vitro and in vivo. AD pridopidine itself is not currently being tested; nonetheless, other S1R agonists including blarcamesine (phase II/III) (Hampel et al., 2020), edonerpic (phase II) (Schneider et al., 2019), dextromethorphan formulations AVP-786 (phase III) (Cummings et al., 2015), AVP-923 (phase IV) (Fralick et al., 2019), and AXS-05 (phase II-III) (Wilkinson and Sanacora, 2019) are undergoing clinical trial validation. In summary, these studies suggest that pridopidine and other S1R agonists could act as disease-modifying and neuroprotective agents in AD by stimulating MERCS-associated functions. In the context of AD, we believe such molecules could be used in the early stages to sustain MERCS-mediated bioenergetics and ATP production.
4.2.2 Ca2+ Signaling Modulators
The functional bridge shuttling Ca2+ between the ER and mitochondria consisting of IP3R-Grp75-VDAC and the mitochondrial Ca2+ uniporter (MCU) is essential for the regulation of Ca2+ dynamics and associated MERCS function including bioenergetics (Rizzuto and Pozzan, 2006), autophagy (Gomez-Suaga et al., 2017), mitochondrial dynamics (Friedman et al., 2011b), and vesicle release (Dentoni et al., 2022).
4.2.2.1 Mitochondria–Endoplasmic Reticulum Contact Sites’ Modulation and Therapeutic Potential in Alzheimer’s Disease and Clinical Trials
Several studies have shown potential neuroprotective effects of blockage of MERCS-mediated Ca2+ shuttling. Indeed, blocking IP3Rs and MCU has been the most used strategies to influence Ca2+ in this nanodomain (Gomez-Suaga et al., 2017; Naia et al., 2021a). This has been thoroughly reviewed in several publications (Magalhães Rebelo et al., 2020; Leal and Martins, 2021; Rodríguez et al., 2022). Briefly, various strategies can be implemented targeting the Ca2+ shuttling bridge at MERCS:
•IP3Rs: fAD PS1 mutations have been shown to potentiate the IP3R-mediated Ca2+ release (Leissring et al., 2001). In fAD PS1 mice, knockdown of IP3R1 normalized disrupted Ca2+ signaling, alleviating mutant PS-linked fAD pathogenesis (Shilling et al., 2014). IP3R hyperactivity in AD suggests that inhibiting the IP3R function might have neuroprotective effects. IP3R inhibitors such as xestospongin B and C are shown to modulate MERCS-mediated functions such as autophagy (Gomez-Suaga et al., 2017), vesicle release (Dentoni et al., 2022), bioenergetics, and Ca2+ homeostasis (Cardenas et al., 2010b).
•VDAC: VDAC is a critical protein in mitochondria-mediated apoptosis and acts as a mitochondrial gatekeeper, controlling metabolic dynamics between the mitochondria and the cytoplasm (Shoshan-Barmatz et al., 2018). Its interaction with AD hallmarks such as Aβ (Smilansky et al., 2015), phopsho-tau (Manczak and Reddy, 2012), and γ-secretase (Hur et al., 2012) modifies its properties. Indeed, the Aβ interaction with VDAC resulted in a two-fold increase in channel conductance, resulting in mitochondrial dysfunction and apoptosis (Smilansky et al., 2015). Several classes of molecules have been shown to influence VDAC opening directly or through its interactions such as AKOS-022, hesperidin, and VBIT-3/4 reviewed in Magrì et al. (2018).
•MCU: MCU has been widely recognized as a potential target for AD. Aβ load has been shown to increase cytosolic Ca2+, resulting in mitochondrial Ca2+ overload in APP/PS1 mouse models. MCU has a pivotal role in this pathway, as inhibiting MCU with Ru360 blocks mitochondrial Ca2+ overload (Calvo-Rodriguez et al., 2020). Excessive Ca2+ uptake by mitochondria can cause mPTP opening, caspase activation, and neuronal cell death (Hurst et al., 2017). Drugs such as the recently identified cell-permeable MCUi4 and MCUi11 could be used to avoid increased mitochondrial Ca2+ matrix accumulation (Di Marco et al., 2020). Decreased activation of MCU and its components, however, have been shown to enhance neuronal damage in mouse neurons and fibroblasts (Verma et al., 2017). A recent review has thoroughly assessed how MERC-dependent MCU activation could be neuroprotective in models of neurodegeneration, highlighting molecules such as kaempferol as potential agents to prevent neurodegeneration (Rodríguez et al., 2022).
Developing compounds that target this MERCS Ca2+ axis may be challenging due to the multifunctional role of Ca2+ in the cells and the plethora of mechanisms it is involved in. What is challenging about these strategies is to find a balance between enough ER to mitochondria Ca2+ shuttling to sustain bioenergetics and not too much interaction that would have deleterious effects such as ROS production and induction of apoptosis.
4.3 Upstream Signaling Cascade Modulators
4.3.1 Metformin
Metformin is an antihyperglycemic drug reducing the glucose output and increasing the insulin-mediated glucose uptake. The molecular targets of metformin include the mitochondrial complex I and other enzymes modulated by the altered energy metabolism, including AMPK signaling, reviewed in Pernicova and Korbonits (2014).
4.3.1.1 Mitochondria–Endoplasmic Reticulum Contact Sites’ Modulation
Studies on the role of metformin in modulating the ER to mitochondria juxtaposition in neurons are scarce. In cardiomyocytes, metformin was shown to have a cardioprotective effect at MERCS. In a recent study, the number of MERCS calculated as the proximity between IP3R1 and VDAC1 was upregulated in Duchenne muscular dystrophy (DMD) cardiomyocytes. IP3R1, as well as MCU and its regulatory subunit, MICU1, expression was upregulated in the DMD heart. In this mouse model, increased MERCS was also associated with an increase in the mitochondrial Ca2+ uptake and complex I dysfunction and ROS production (Angebault et al., 2020), also reported in AD. Treatment of DMD mice for 1 month with metformin improved complex I-driven respiration, normalizing MERCS number, decreasing MICU1 expression, and mitochondrial Ca2+ concentration (Angebault et al., 2020). Whether these effects on MERCS are direct or indirect remains unknown; target validation analysis could shed light on metformin-dependent pathways and mechanisms.
4.3.1.2 Therapeutic Potential in Alzheimer’s Disease and Clinical Trials
Insulin resistance and diabetes are becoming well recognized as risk factors in the development of dementia and AD (Burillo et al., 2021). Metformin’s potential by acting on mitochondrial metabolism and insulin signaling is the basis for its use for neurodegenerative disorders and AD. Metformin use is linked to a decreased risk of cognitive impairment in T2DM patients (Teng et al., 2001). Studies show that diabetics taking metformin had a decreased likelihood of developing AD than diabetics taking other glucose-lowering drugs (Chin-Hsiao, 2019). Metformin is currently being used as a neuroprotective agent in MCI patients in clinical trials in phases II and III (NCT0498666). By decreasing the ER to mitochondria apposition, these drugs could be used in later stages of the disease to prevent excessive Ca2+ shuttling, hence counteracting negative effects of overtly active MERCS such as ROS production and apoptosis initiation. Combined with MERCS off-target effects, metformin has the potential and is currently being considered as a powerful neuroprotector in AD.
4.3.2 Sulforaphane
Sulforaphane (SFN) is a small lipophilic isothiocyanate abundantly found in green vegetables such as broccoli, with higher bioavailability in the bloodstream than other phytochemicals (Hanlon et al., 2008). It has been showed that SFN can reduce glucose production and improve glucose control in patients with type 2 diabetes (Axelsson et al., 2017). Previous studies have showed that SFN induces nuclear translocation of the nuclear factor erythroid 2-related factor 2 (Nrf2) and binding to G6pc and PEPCK to suppress gluconeogenesis (Heiss et al., 2013). Since activation of Nrf2 protects against oxidative stress and supports the structural and functional integrity of mitochondria (Dinkova-Kostova and Abramov, 2015).
4.3.2.1 Mitochondria–Endoplasmic Reticulum Contact Sites’ Modulation
No studies so far have analyzed the role of this molecule in MERCS modulation in neuronal models. However, SFN has been shown to have MERCS-positive effects in hepatocytes. Indeed, MERCS have been shown to be intercellular hubs for hepatic metabolism. In insulin resistance models, studies have shown MERCS to be either downregulated or upregulated (Arruda et al., 2014; Tubbs et al., 2014). Regarding MERCS modulation, SFN improved disrupted ER–mitochondria interactions in primary mouse hepatocytes isolated from mice with genetically induced obesity, resulting in increased IP3R1–VDAC proximity, increased MERCS protein levels, and decreased ER stress (Tubbs et al., 2014). In nonalcoholic fatty liver disease (NHFLD) mouse models where the ER to mitochondria apposition is shown to be upregulated, SFN maintained Ca2+ homeostasis at MERCS by regulating the Ca2+ flux via Ca2+ channels IP3R and MCU. When MERCS numbers were not actively assessed post-treatment in this study, ΔΨm and ATP synthesis were upregulated post-SFN treatment (Tian et al., 2021).
4.3.2.2 Therapeutic Potential in Alzheimer’s Disease and Clinical Trials
Recently, the potential of SFN as a neuroprotective agent has been summarized showing its potential as a small-molecule modulator in different animal models of AD (Kim, 2021). The SFN neuroprotective potential has been considered for the treatment of prodromal and mild AD, which is currently recruiting (NCT04213391). Other analogs of SFN, such as Avmacol under clinical investigation, have been shown to modulate the symptoms of autism (Bent et al., 2018). Nevertheless, the mechanism in which SFN improves ER–mitochondria interactions is an open field that requires further investigation. We speculate on the use of SFN or its analogs could activate the Nrf2 pathway, improve ER–mitochondria interactions, activate the transcriptional regulation of cytoprotective genes, and regulate concomitant pathways such as inflammation, mitochondrial dynamics, and cellular metabolism.
4.3.3 Trolox
Trolox (6-hydroxy2,5,7,8-tetramethylchroman-2-carboxylic acid) is a small water-soluble vitamin E-derived antioxidant. As a lipid peroxidation scavenger, Trolox aids in the stabilization of cellular membranes (Lúcio et al., 2009).
4.3.3.1 Mitochondria–Endoplasmic Reticulum Contact Sites’ Modulation
Several lines of evidence point to Trolox as a potential MERCS modulator. Earlier studies have shown that Trolox treatment increases the protein levels of Mfn2 in Chinese hamster ovary (CHO) cells (Distelmaier et al., 2012). Another study showed chronic Trolox treatment to increase mitochondrial complex I activity, reduce ROS levels, and restore cytosolic Ca2+ ATP handling and ΔΨm in primary skin fibroblasts isolated from children with NADH deficiency (Koopman et al., 2008). A study showing the direct role of this drug on MERCS reported that in the neuroblastoma cell model of Friedreich ataxia (FRDA), Trolox could restore MERCS and increase Ca2+ shuttling between the ER and mitochondria (Rodríguez et al., 2020). Friedreich ataxia (FRDA) is a neurodegenerative disorder leading to dorsal root ganglia degeneration, caused by mutations of the FXN gene, which encodes frataxin (FXN), a mitochondrial protein found in the IMM. Modulation of MERCS through Marf knockdown (Mfn2 D. melanogaster homolog) has been shown to be beneficial in frataxin-deficient glial cells, likely through increased ER to mitochondria apposition which was not evaluated in this study (Edenharter et al., 2018). Similarly, Trolox restoration of Ca2+ levels through the ER to mitochondria increased juxtaposition was sufficient to reverse the defects caused by frataxin deficiency in D. melanogaster (Rodríguez et al., 2020).
4.3.3.2 Therapeutic Potential in Alzheimer’s Disease and Clinical Trial
The efficacy of vitamin E and its derivatives, such as Trolox, as a potential treatment intervention for AD has been tested in several randomized studies (Regner-Nelke et al., 2021). Trolox can also protect against neurotoxicity caused by Aβ and inhibit glycogen synthase kinase 3 (GSK 3), a protein in neurofibrillary tangle development (Muñoz et al., 2002; Radesäter et al., 2003). Despite this, evidence for vitamin E’s therapeutic effectiveness in the treatment of AD remains unclear. Despite strong evidence of increased ROS in AD etiology and lower circulating vitamin E levels, clinical trials examining vitamin E as a treatment have yet to show conclusive results (Regner-Nelke et al., 2021). When no clinical studies have been conducted with Trolox specifically for AD, studies have shown that a combination of donepezil and Trolox has been tested as multifunctional agents for AD. These compounds had moderate to good inhibitory actions against acetylcholinesterase (AChE) and monoamine oxidase B (MAO-B), as well as significant antioxidant properties (Cai et al., 2017). These compounds have shown promising improvements in cognition and spatial memory in vivo. The additional effects of Trolox on MERCS could stabilize and promote bioenergetics mechanism, decreasing ROS production by effectively coupling respiration and resulting in an overall improvement in the AD phenotype.
4.3.4 Luteolin
Luteolin is a natural flavone that has been found to have potent antioxidant and anti-inflammatory activities (Nabavi et al., 2015). One of the most common protective mechanisms of luteolin is to increase the binding of Nrf2 to the antioxidant responsive element (ARE) (Wruck et al., 2007; Lin et al., 2010). The Nrf2–ARE pathway is a mechanism of defense which reduces oxidative stress and neuroinflammation promoting neuronal homeostasis. In AD, it has been shown that the Nrf2–ARE pathway is disrupted mainly due to decreased Nrf2 expression (Ramsey et al., 2007).
4.3.4.1 Mitochondria–Endoplasmic Reticulum Contact Sites’ Modulation
In addition to luteolin’s well-known properties, we recently reported a new mechanism for luteolin-induced neuroprotection through MERCS. Our laboratory established a neuronal cell-based high-throughput screen to search for mitotherapeutics and identified luteolin as one hit. Low micromolar concentrations of luteolin increased bioenergetics in primary mouse neurons and isolated mouse brain mitochondria and crude synaptosomal preparations containing MERCS (Naia et al., 2021b). We identified ER to mitochondrial Ca2+ shuttling as a mechanism promoted by luteolin, increasing the Krebs cycle activity and consequently mitochondrial respiration and ATP production. Luteolin enhanced MERCS through an IP3Rs-dependent mechanism as inhibition of IP3Rs through xestospongin C treatment hindered luteolin-induced ATP production. Luteolin administration also partially recovered mitochondrial and locomotory activities in C. elegans and primary neuron models expressing the mutant huntingtin (Naia et al., 2021b).
4.3.4.2 Therapeutic Potential in Alzheimer’s Disease and Clinical Trial
Several in vitro and in vivo studies have reported positive effects of luteolin on reducing ROS production (Dragicevic et al., 2011), restoring ATP levels (Dragicevic et al., 2011), increasing synaptic marker expression (Xu et al., 2013), reducing pathological hallmarks of AD and decreasing Aβ production and tau phosphorylation (Sawmiller et al., 2014), and restoring cognitive functions (Wang et al., 2016). Interestingly, luteolin was also reported to increase the sacro/endoplasmic reticulum Ca2+ ATPase 2a (SERCA2a) activity, which in turn influences Aβ generation (Green et al., 2008; Zheng et al., 2015), pointing to its Ca2+-modifying properties in AD. Luteolin has been used in clinical phase 2 trials as a dietary supplement for 4 months with positive outcomes increasing behavioral and social interactions in subjects with autism spectrum disorder (ASD) (Taliou et al., 2013). A recent clinical trial assessing the effect of luteolin on memory in healthy subjects was started in January 2020 but halted due to the COVID-19 pandemic (https://clinicaltrials.gov/, NCT04468854); hence, this compound is currently being considered for the treatment of neurodegenerative disorders in humans.
4.3.5 Urolithin A
Urolithin A is a gut microbial product, metabolized from ingested ellagitannins and ellagic acid (D’Amico et al., 2021). Surprisingly, the conversion of dietary precursors to urolithin A does not occur equally in all individuals, depending on specific gut microbiota composition (Cortés-Martín et al., 2020). Due to its anti-inflammatory and neuroprotective characteristics in preclinical AD models, PD models, diabetes mellitus, ischemic neuronal injury, and normal aging, urolithin A has received considerable attention recently (D'Amico et al., 2021).
4.3.5.1 Mitochondria-Endoplasmic Reticulum Contact Sites’ Modulation
The role of urolithin A in modulating MERCS was unknown until very recently. In a hyperglycemia cell model, characterized by increased ER to mitochondria Ca2+ shuttling and increased mtROS production and Aβ production, transglutaminase 2 (TGM2) increased MERCS by stabilizing the IP3R–Grp75–VDAC interaction (previously discussed in Section 2.2 ER to mitochondria Ca2+ shuttling). Indeed, TGM2 knockdown reverted pathology in cells under hyperglycemic stress (Lee et al., 2021). Similar to TGM2 knockdown, urolithin A reduced TGM2-dependent MERCS formation and ROS and Aβ production by affecting the AIP–AhR transcription complex, resulting in reduced TGM2 levels (Lee et al., 2021).
4.3.5.2 Therapeutic Potential in Alzheimer’s Disease and Clinical Trial
From the aforementioned data, Urolithin A has emerged as a promising candidate to disengage stable MERCS in conditions in which the ER to mitochondria apposition is upregulated such as in AD and diabetes, a major risk factor for developing dementia. In AD animal models such as APP/PS1 mice, urolithin A treatment improved cognition and enhanced neurogenesis (Gong et al., 2019). Furthermore, urolithin A attenuated Aβ deposition, microgliosis, and astrocytosis in the cortex and hippocampus (Fang et al., 2019; Gong et al., 2019). Urolithin A is capable of persisting in the plasma and crossing BBB, making it an ideal neuroactive compound (Gasperotti et al., 2015; Kujawska et al., 2020). Clinical trials on this compound are limited; however, some are currently ongoing to assess the bioavailability of urolithin A in patients’ blood (NCT04985630), its protective role in prostate cancer (NCT03535675), and to boost muscle endurance (NCT04783207).
5 Current Challenges and Future Perspectives to Develop Mitochondria–Endoplasmic Reticulum Contact Sites’ Function and Modulators
Experimental efforts spanning over the past decade have revealed MERCS as a new player in AD pathogenesis. We believe that the therapeutic potential of MERCS modulators requires further translational research to overcome several challenges.
One of the most ambitious challenges regarding pharmaceutical modulation of MERCS is the difficulty of finding the precise conditions in which small-molecule modulators “fine-tune” MERCS to achieve neuronal balance. For example, the “fine-tuning” of MERCS to increase the Ca2+ flux and promote Krebs cycle activity would promote neuronal homeostasis. However, excessive Ca2+ flux can activate ROS production and apoptosis pathways, leading to cell death (Csordas et al., 2006). Therefore, increasing many or decreasing very few MERCS-associated functions can create an adverse effect and be deleterious for neuronal homeostasis. Finding MERCS modulators that could sense the bioenergetics needs of the cell, such as MERCS do under physiological conditions, would be fundamental to reestablish neuronal homeostasis. As previously mentioned, understanding whether decreased or increased ER to mitochondria apposition is beneficial in AD models and at which stage of the pathology, together with each strategy’s influence on downstream pathological effects, would need to be set as a priority to progress in research on MERCS pharmaceutical modulators for neurodegeneration, especially AD.
Despite considerable advances in understanding many mitochondrial and MERCS processes in the pathogenesis of AD, precise mechanistic knowledge on MERCS physiology is lacking. Perhaps the best example of our lack of understanding of MERCS is the limited information we have on tethers and their role in different diseases. Although VAPB and PTPIP51 have been shown to be related to ALS (Stoica et al., 2014), such a direct connection has not been observed in AD. Indeed, a pressing issue in the field is to understand whether different tethers may be selectively affected in neurodegenerative disorders, generating a specific phenotypic “signature” of the disease. A limitation to current studies assessing proximity between selective pairs of tethers such as VAPB–PTPIP51 and IP3R–VDAC using the proximity ligation assay is that it could lead to misinterpretation of data; when a specific couple of tethers could be decreased, other tethering proteins could be upregulated leading to an overall increase in contact formation, which can only be assessed by structural apposition between the ER and mitochondria using split-green fluorescent protein (GFP)-based contact site sensors (SPLICS) or transmission electron microscopy (TEM). In fact, although VAPB levels and VAPB–PTPT51 proximity have been shown to be downregulated in sAD brains, we have recent data showing in AppNL-F embryonic primary cortical neurons that while VAPB levels were decreased in these cells, overall MERCS structural apposition, measured through TEM, and functional apposition were upregulated (Dentoni et al., unpublished), hence hinting to several tethers playing a role in overall organelle juxtaposition in AD models.
Another concern that might arise from targeting specific molecules to ER–mitochondria tethers is that MERCS-related proteins are not exclusively located in these subcellular hubs of the cells. MERCS-enriched proteins are often also present in the bulk ER and mitochondria, playing a variety of physiological functions. Therefore, targeting a specific protein at MERCS might have off-target effects that need to be thoroughly investigated.
While tethers and regulators of tethering have received much attention in the MERCS field, methods altering the ER and mitochondria function through modulation of functional apposition between the two membranes should also be considered. Recently, we have shown that TOM70 depletion inhibits the IP3-linked ER to mitochondria Ca2+ transport independently from the interaction between the two membranes, in which MERCS structural characteristics remain unchanged upon TOM70 depletion (Filadi et al., 2018b). Indeed, TOM70 knockdown resulted in a decrease in co-localization between IP3Rs and mitochondria suggesting that TOM70 through its interaction with IP3Rs favors these channels’ functional recruitment close to mitochondria (Filadi et al., 2018b). Similarly, targeting IP3Rs and their interactors and stabilizers could prove an essential strategy to guarantee favorable ER to mitochondria apposition.
6 Conclusion
As our understanding of MERCS proteome and pathways are still incomplete, new methods aimed at systematically understanding the MERCS function may uncover novel avenues for therapeutic modulation in AD. Recently, methods to isolate, such as physical and biochemical characterization and detection of MERCS, with different reporters have been described elsewhere (Giamogante et al., 2020; Wilson and Metzakopian, 2021). These methods to identify (i.e., ContactID and SpliTurboID) and characterize MERCS morphology (i.e., EM and TEM), dynamics (i.e., FRET, PLA, and SPLICS), and function (i.e., cellular respirometry, quantification of ATP, Ca2+, Δψm, ROS, and lipid levels) can be incorporated to pooled or arrayed high-throughput screenings to allow for novel recognition of drug candidates.
Although different open questions regarding the MERCS ultrastructure and composition remain to be further explored, we expect that as the local proteome at MERCS is deciphered and better models to study AD pathology emerge, we can develop small-molecule modulators to remodel the MERCS ultrastructure and function to restore the neuronal function in AD. We speculate future possibilities of using MERCS modulators in combination with disease-modifying treatments to decelerate or prevent disease progression even decades before clinical AD symptoms appear. In summary, these observations indicate that a complete understanding of pharmacodynamics and pharmacokinetic properties of MERCS modulators and refined mechanistic knowledge of MERCS-associated processes constitute instrumental processes for developing novel MERCS modulators for clinical therapeutic use in AD.
Author Contributions
All authors listed have made a substantial, direct, and intellectual contribution to the work and approved it for publication.
Funding
This study was funded by the Swedish Research Council (No 2018–03102), the Swedish Alzheimer Foundation (Alzheimerfonden), the Gamla Tjänarinnor Foundation, and the Gun och Bertil Stohnes Foundation.
Conflict of Interest
The authors declare that the research was conducted in the absence of any commercial or financial relationships that could be construed as a potential conflict of interest.
Publisher’s Note
All claims expressed in this article are solely those of the authors and do not necessarily represent those of their affiliated organizations, or those of the publisher, the editors, and the reviewers. Any product that may be evaluated in this article, or claim that may be made by its manufacturer, is not guaranteed or endorsed by the publisher.
Abbreviations
AD, Alzheimer’s disease; MERCS, mitochondria–endoplasmic reticulum contact sites; ER, endoplasmic reticulum; MAMs, mitochondria-associated endoplasmic reticulum membranes; OMM, outer mitochondrial membrane; IMM, inner mitochondrial membrane; PS, phosphatidylserine; PE, phosphatidylethanolamine; PC, phosphatidylcholine; Cer, ceramides.
References
Aisen, P. S., Gauthier, S., Ferris, S. H., Saumier, D., Haine, D., Garceau, D., et al. (2011). Tramiprosate in mild-to-moderate Alzheimer's disease - a randomized, double-blind, placebo-controlled, multi-centre study (the alphase study). Arch. Med. Sci. 7, 102–111. doi:10.5114/aoms.2011.20612
Alford, S. C., Ding, Y., Simmen, T., and Campbell, R. E. (2012). Dimerization-dependent green and yellow fluorescent proteins. ACS Synth. Biol. 1, 569–575. doi:10.1021/sb300050j
Angebault, C., Panel, M., Lacôte, M., Rieusset, J., Lacampagne, A., and Fauconnier, J. (2020). Metformin reverses the enhanced myocardial SR/ER-Mitochondria interaction and impaired complex I-driven respiration in dystrophin-deficient mice. Front. Cell. Dev. Biol. 8, 609493. doi:10.3389/fcell.2020.609493
Ardail, D., Gasnier, F., Lerme, F., Simonot, C., Louisot, P., and Gateauroesch, O. (1993). Involvement of mitochondrial contact sites in the subcellular compartmentalization of phospholipid biosynthetic-enzymes. J. Biol. Chem. 268, 25985–25992. doi:10.1016/s0021-9258(19)74483-4
Area-Gomez, E., De Groof, A., Bonilla, E., Montesinos, J., Tanji, K., Boldogh, I., et al. (2018). A key role for MAM in mediating mitochondrial dysfunction in Alzheimer disease. Cell. Death Dis. 9, 335. doi:10.1038/s41419-017-0215-0
Area-Gomez, E., De Groof, A. J., Boldogh, I., Bird, T. D., Gibson, G. E., Koehler, C. M., et al. (2009). Presenilins are enriched in endoplasmic reticulum membranes associated with mitochondria. Am. J. Pathol. 175, 1810–1816. doi:10.2353/ajpath.2009.090219
Area-Gomez, E., Del Carmen Lara Castillo, M., Tambini, M. D., Guardia-Laguarta, C., De Groof, A. J., Madra, M., et al. (2012). Upregulated function of mitochondria-associated ER membranes in Alzheimer disease. EMBO J. 31, 4106–4123. doi:10.1038/emboj.2012.202
Area-Gomez, E., and Schon, E. A. (2017). On the pathogenesis of Alzheimer's disease: The MAM hypothesis. FASEB J. 31, 864–867. doi:10.1096/fj.201601309
Arruda, A. P., Pers, B. M., Parlakgül, G., Güney, E., Inouye, K., and Hotamisligil, G. S. (2014). Chronic enrichment of hepatic endoplasmic reticulum–mitochondria contact leads to mitochondrial dysfunction in obesity. Nat. Med. 20, 1427–1435. doi:10.1038/nm.3735
Axelsson, A. S., Tubbs, E., Mecham, B., Chacko, S., Nenonen, H. A., Tang, Y., et al. (2017). Sulforaphane reduces hepatic glucose production and improves glucose control in patients with type 2 diabetes. Sci. Transl. Med. 9, eaah4477. doi:10.1126/scitranslmed.aah4477
Bartok, A., Weaver, D., Golenár, T., Nichtova, Z., Katona, M., Bánsághi, S., et al. (2019). IP3 receptor isoforms differently regulate ER-mitochondrial contacts and local calcium transfer. Nat. Commun. 10, 3726. doi:10.1038/s41467-019-11646-3
Bent, S., Lawton, B., Warren, T., Widjaja, F., Dang, K., Fahey, J. W., et al. (2018). Identification of urinary metabolites that correlate with clinical improvements in children with autism treated with sulforaphane from broccoli. Mol. Autism 9, 35. doi:10.1186/s13229-018-0218-4
Bernard-Marissal, N., Médard, J.-J., Azzedine, H., and Chrast, R. (2015). Dysfunction in endoplasmic reticulum-mitochondria crosstalk underlies SIGMAR1 loss of function mediated motor neuron degeneration. Brain. 138, 875–890. doi:10.1093/brain/awv008
Bernard-Marissal, N., Van Hameren, G., Juneja, M., Pellegrino, C., Louhivuori, L., Bartesaghi, L., et al. (2019). Altered interplay between endoplasmic reticulum and mitochondria in Charcot–Marie–Tooth type 2A neuropathy. Proc. Natl. Acad. Sci. U. S. A. 116, 2328–2337. doi:10.1073/pnas.1810932116
Bernhard, W., and Rouiller, C. (1956). Close topographical relationship between mitochondria and ergastoplasm of liver cells in a definite phase of cellular activity. J. Biophys. Biochem. Cytol. 2 (4), 73–78. doi:10.1083/jcb.2.4.73
Birnbaum, J. H., Wanner, D., Gietl, A. F., Saake, A., Kündig, T. M., Hock, C., et al. (2018). Oxidative stress and altered mitochondrial protein expression in the absence of amyloid-β and tau pathology in iPSC-derived neurons from sporadic Alzheimer's disease patients. Stem Cell. Res. 27, 121–130. doi:10.1016/j.scr.2018.01.019
Bogeski, I., Gulaboski, R., Kappl, R., Mirceski, V., Stefova, M., Petreska, J., et al. (2011). Calcium binding and transport by coenzyme Q. J. Am. Chem. Soc. 133, 9293–9303. doi:10.1021/ja110190t
Booth, D. M., Enyedi, B., Geiszt, M., Varnai, P., and Hajnoczky, G. (2016). Redox nanodomains are induced by and control calcium signaling at the ER-mitochondrial interface. Mol. Cell. 63, 240–248. doi:10.1016/j.molcel.2016.05.040
Braak, H., and Braak, E. (1991). Neuropathological stageing of Alzheimer-related changes. Acta Neuropathol. 82, 239–259. doi:10.1007/BF00308809
Brobeil, A., Chehab, R., Dietel, E., Gattenlöhner, S., and Wimmer, M. (2017). Altered protein interactions of the endogenous interactome of PTPIP51 towards MAPK signaling. Biomolecules 7, 55. doi:10.3390/biom7030055
Burillo, J., Marqués, P., Jiménez, B., González-Blanco, C., Benito, M., and Guillén, C. (2021). Insulin resistance and diabetes mellitus in Alzheimer's disease. Cells 10, 1236. doi:10.3390/cells10051236
Cai, P., Fang, S.-Q., Yang, X.-L., Wu, J.-J., Liu, Q.-H., Hong, H., et al. (2017). Rational design and multibiological profiling of novel donepezil–trolox hybrids against alzheimer’s disease, with cholinergic, antioxidant, neuroprotective, and cognition enhancing properties. ACS Chem. Neurosci. 8, 2496–2511. doi:10.1021/acschemneuro.7b00257
Calvo-Rodriguez, M., Hernando-Perez, E., Nunez, L., and Villalobos, C. (2019). Amyloid beta oligomers increase ER-mitochondria Ca(2+) cross talk in young hippocampal neurons and exacerbate aging-induced intracellular Ca(2+) remodeling. Front. Cell. Neurosci. 13, 22. doi:10.3389/fncel.2019.00022
Calvo-Rodriguez, M., Hou, S. S., Snyder, A. C., Kharitonova, E. K., Russ, A. N., Das, S., et al. (2020). Increased mitochondrial calcium levels associated with neuronal death in a mouse model of Alzheimer’s disease. Nat. Commun. 11, 2146. doi:10.1038/s41467-020-16074-2
Calvo-RodríguezGARCÍA-Durillo, M. M., Villalobos, C., and Núñez, L. (2016). Aging enables Ca 2+ overload and apoptosis induced by amyloid-β oligomers in rat hippocampal neurons: Neuroprotection by non-steroidal anti-inflammatory drugs and R-flurbiprofen in aging neurons. J. Alzheimer's Dis. 54, 207–221. doi:10.3233/JAD-151189
Cardenas, C., Miller, R. A., Smith, I., Bui, T., Molgo, J., Muller, M., et al. (2010a). Essential regulation of cell bioenergetics by constitutive InsP3 receptor Ca2+ transfer to mitochondria. Cell. 142, 270–283. doi:10.1016/j.cell.2010.06.007
Cárdenas, C., Miller, R. A., Smith, I., Bui, T., Molgó, J., Müller, M., et al. (2010b). Essential regulation of cell bioenergetics by constitutive InsP3 receptor Ca2+ transfer to mitochondria. Cell. 142, 270–283. doi:10.1016/j.cell.2010.06.007
Cenini, G., and Voos, W. (2019). Mitochondria as potential targets in alzheimer disease therapy: An update. Front. Pharmacol. 10, 902. doi:10.3389/fphar.2019.00902
Chakrabarti, R., Ji, W.-K., Stan, R. V., De Juan Sanz, J., Ryan, T. A., and Higgs, H. N. (2017). INF2-mediated actin polymerization at the ER stimulates mitochondrial calcium uptake, inner membrane constriction, and division. J. Cell. Biol. 217, 251–268. doi:10.1083/jcb.201709111
Cherubini, M., Lopez-Molina, L., and Gines, S. (2020). Mitochondrial fission in Huntington's disease mouse striatum disrupts ER-mitochondria contacts leading to disturbances in Ca2+ efflux and Reactive Oxygen Species (ROS) homeostasis. Neurobiol. Dis. 136, 104741. doi:10.1016/j.nbd.2020.104741
Chin-Hsiao, T. (2019). Metformin and the risk of dementia in type 2 diabetes patients. Aging Dis. 10, 37–48. doi:10.14336/AD.2017.1202
Cieri, D., Vicario, M., Giacomello, M., Vallese, F., Filadi, R., Wagner, T., et al. (2018a). Splics: A split green fluorescent protein-based contact site sensor for narrow and wide heterotypic organelle juxtaposition. Cell. Death Differ. 25, 1131–1145. doi:10.1038/s41418-017-0033-z
Cieri, D., Vicario, M., Vallese, F., D'Orsi, B., Berto, P., Grinzato, A., et al. (2018b). Tau localises within mitochondrial sub-compartments and its caspase cleavage affects ER-mitochondria interactions and cellular Ca(2+) handling. Biochim. Biophys. Acta. Mol. Basis Dis. 1864, 3247–3256. doi:10.1016/j.bbadis.2018.07.011
Cortés-Martín, A., Selma, M. V., Tomás-Barberán, F. A., González-Sarrías, A., and Espín, J. C. (2020). Where to look into the puzzle of polyphenols and health? The postbiotics and gut microbiota associated with human metabotypes. Mol. Nutr. Food Res. 64, 1900952. doi:10.1002/mnfr.201900952
Cosson, P., Marchetti, A., Ravazzola, M., and Orci, L. (2012). Mitofusin-2 independent juxtaposition of endoplasmic reticulum and mitochondria: An ultrastructural study. PLoS One 7, e46293. doi:10.1371/journal.pone.0046293
Csordás, G., and Hajnóczky, G. (2001). Sorting of calcium signals at the junctions of endoplasmic reticulum and mitochondria. Cell. Calcium 29, 249–262. doi:10.1054/ceca.2000.0191
Csordas, G., Renken, C., Varnai, P., Walter, L., Weaver, D., Buttle, K. F., et al. (2006). Structural and functional features and significance of the physical linkage between ER and mitochondria. J. Cell. Biol. 174, 915–921. doi:10.1083/jcb.200604016
Csordás, G., Renken, C., Várnai, P., Walter, L., Weaver, D., Buttle, K. F., et al. (2006). Structural and functional features and significance of the physical linkage between ER and mitochondria. J. Cell. Biol. 174, 915–921. doi:10.1083/jcb.200604016
Csordás, G., Weaver, D., and Hajnóczky, G. (2018). Endoplasmic reticulum–mitochondrial contactology: Structure and signaling functions. Trends Cell. Biol. 28, 523–540. doi:10.1016/j.tcb.2018.02.009
Cummings, J., Lee, G., Zhong, K., Fonseca, J., and Taghva, K. (2021). Alzheimer's disease drug development pipeline: 2021. Alzheimers Dement. 7, e12179. doi:10.1002/trc2.12179
Cummings, J. L., Lyketsos, C. G., Peskind, E. R., Porsteinsson, A. P., Mintzer, J. E., Scharre, D. W., et al. (2015). Effect of dextromethorphan-quinidine on agitation in patients with alzheimer disease dementia: A randomized clinical trial. Jama 314, 1242–1254. doi:10.1001/jama.2015.10214
D'amico, D., Andreux, P. A., Valdés, P., Singh, A., Rinsch, C., and Auwerx, J. (2021). Impact of the natural compound Urolithin A on health, disease, and aging. Trends Mol. Med. 27, 687–699. doi:10.1016/j.molmed.2021.04.009
De Brito, O. M., and Scorrano, L. (2008). Mitofusin 2 tethers endoplasmic reticulum to mitochondria. Nature 456, 605–610. doi:10.1038/nature07534
De Vos, K. J., Morotz, G. M., Stoica, R., Tudor, E. L., Lau, K. F., Ackerley, S., et al. (2012). VAPB interacts with the mitochondrial protein PTPIP51 to regulate calcium homeostasis. Hum. Mol. Genet. 21, 1299–1311. doi:10.1093/hmg/ddr559
Del Prete, D., Suski, J. M., Oules, B., Debayle, D., Gay, A. S., Lacas-Gervais, S., et al. (2017). Localization and processing of the amyloid-beta protein precursor in mitochondria-associated membranes. J. Alzheimers Dis. 55, 1549–1570. doi:10.3233/JAD-160953
D’eletto, M., Rossin, F., Occhigrossi, L., Farrace, M. G., Faccenda, D., Desai, R., et al. (2018). Transglutaminase type 2 regulates ER-mitochondria contact sites by interacting with GRP75. Cell. Rep. 25, 3573–3581. e4. doi:10.1016/j.celrep.2018.11.094
Dentoni, G., Naia, L., and Ankarcrona, M. (2022). Mitochondria-endoplasmic reticulum interplay regulates exo-cytosis in human neuroblastoma cells. Cells 11, 514. doi:10.3390/cells11030514
Di Marco, G., Vallese, F., Jourde, B., Bergsdorf, C., Sturlese, M., De Mario, A., et al. (2020). A high-throughput screening identifies MICU1 targeting compounds. Cell. Rep. 30, 2321–2331.e6. e6. doi:10.1016/j.celrep.2020.01.081
Dietel, E., Brobeil, A., Delventhal, L., Tag, C., Gattenlöhner, S., and Wimmer, M. (2019). Crosstalks of the PTPIP51 interactome revealed in Her2 amplified breast cancer cells by the novel small molecule LDC3/Dynarrestin. PloS one 14, e0216642. doi:10.1371/journal.pone.0216642
Dinkova-Kostova, A. T., and Abramov, A. Y. (2015). The emerging role of Nrf2 in mitochondrial function. Free Radic. Biol. Med. 88, 179–188. doi:10.1016/j.freeradbiomed.2015.04.036
Distelmaier, F., Valsecchi, F., Forkink, M., Van Emst-De Vries, S., Swarts, H. G., Rodenburg, R. J. T., et al. (2012). Trolox-sensitive reactive oxygen species regulate mitochondrial morphology, oxidative phosphorylation and cytosolic calcium handling in healthy cells. Antioxid. Redox Signal. 17 (12), 1657–1669. doi:10.1089/ars.2011.4294
Doghman-Bouguerra, M., Granatiero, V., Sbiera, S., Sbiera, I., Lacas-Gervais, S., Brau, F., et al. (2016). FATE1 antagonizes calcium- and drug-induced apoptosis by uncoupling ER and mitochondria. EMBO Rep. 17, 1264–1280. doi:10.15252/embr.201541504
Dragicevic, N., Smith, A., Lin, X., Yuan, F., Copes, N., Delic, V., et al. (2011). Green tea epigallocatechin-3-gallate (EGCG) and other flavonoids reduce Alzheimer's amyloid-induced mitochondrial dysfunction. J. Alzheimers Dis. 26, 507–521. doi:10.3233/JAD-2011-101629
Eddings, C. R., Arbez, N., Akimov, S., Geva, M., Hayden, M. R., and Ross, C. A. (2019). Pridopidine protects neurons from mutant-huntingtin toxicity via the sigma-1 receptor. Neurobiol. Dis. 129, 118–129. doi:10.1016/j.nbd.2019.05.009
Edenharter, O., Schneuwly, S., and Navarro, J. A. (2018). Mitofusin-dependent ER stress triggers glial dysfunction and nervous system degeneration in a Drosophila model of friedreich's ataxia. Front. Mol. Neurosci. 11, 38. doi:10.3389/fnmol.2018.00038
Esteras, N., Rohrer, J. D., Hardy, J., Wray, S., and Abramov, A. Y. (2017). Mitochondrial hyperpolarization in iPSC-derived neurons from patients of FTDP-17 with 10+16 MAPT mutation leads to oxidative stress and neurodegeneration. Redox Biol. 12, 410–422. doi:10.1016/j.redox.2017.03.008
Fang, E. F., Hou, Y., Palikaras, K., Adriaanse, B. A., Kerr, J. S., Yang, B., et al. (2019). Mitophagy inhibits amyloid-β and tau pathology and reverses cognitive deficits in models of Alzheimer’s disease. Nat. Neurosci. 22, 401–412. doi:10.1038/s41593-018-0332-9
Filadi, R., Greotti, E., and Pizzo, P. (2018a). Highlighting the endoplasmic reticulum-mitochondria connection: Focus on Mitofusin 2. Pharmacol. Res. 128, 42–51. doi:10.1016/j.phrs.2018.01.003
Filadi, R., Greotti, E., Turacchio, G., Luini, A., Pozzan, T., and Pizzo, P. (2015). Mitofusin 2 ablation increases endoplasmic reticulum-mitochondria coupling. Proc. Natl. Acad. Sci. U. S. A. 112, E2174–E2181. doi:10.1073/pnas.1504880112
Filadi, R., Greotti, E., Turacchio, G., Luini, A., Pozzan, T., and Pizzo, P. (2016). Presenilin 2 modulates endoplasmic reticulum-mitochondria coupling by tuning the antagonistic effect of mitofusin 2. Cell. Rep. 15 (10), 2226–2238. doi:10.1016/j.celrep.2016.05.013
Filadi, R., Leal, N. S., Schreiner, B., Rossi, A., Dentoni, G., Pinho, C. M., et al. (2018b). TOM70 sustains cell bioenergetics by promoting IP3R3-mediated ER to mitochondria Ca 2+ transfer. Curr. Biol. 28, 369–382.e6. doi:10.1016/j.cub.2017.12.047
Filadi, R., Leal, N. S., Schreiner, B., Rossi, A., Dentoni, G., Pinho, C. M., et al. (2018c). TOM70 sustains cell bioenergetics by promoting IP3R3-mediated ER to mitochondria Ca(2+) transfer. Curr. Biol. 28, 369–382. e6. doi:10.1016/j.cub.2017.12.047
Fralick, M., Sacks, C. A., and Kesselheim, A. S. (2019). Assessment of use of combined dextromethorphan and quinidine in patients with dementia or Parkinson disease after US food and drug administration approval for pseudobulbar affect. JAMA Intern. Med. 179, 224–230. doi:10.1001/jamainternmed.2018.6112
Franco, A., Kitsis, R. N., Fleischer, J. A., Gavathiotis, E., Kornfeld, O. S., Gong, G., et al. (2016a). Correcting mitochondrial fusion by manipulating mitofusin conformations. Nature 540, 74–79. doi:10.1038/nature20156
Franco, A., Kitsis, R. N., Fleischer, J. A., Gavathiotis, E., Kornfeld, O. S., Gong, G., et al. II (2016b). Correcting mitochondrial fusion by manipulating mitofusin conformations. Nature 540, 74–79. doi:10.1038/nature20156
Friedman, J. R., Lackner, L. L., West, M., Dibenedetto, J. R., Nunnari, J., and Voeltz, G. K. (2011a). ER tubules mark sites of mitochondrial division. Science 334, 358–362. doi:10.1126/science.1207385
Friedman, J. R., Lackner, L. L., West, M., Dibenedetto, J. R., Nunnari, J., Voeltz, G. K., et al. (2011b). ER tubules mark sites of mitochondrial division. Science 334, 358–362. doi:10.1126/science.1207385
Garofalo, T., Matarrese, P., Manganelli, V., Marconi, M., Tinari, A., Gambardella, L., et al. (2016). Evidence for the involvement of lipid rafts localized at the ER-mitochondria associated membranes in autophagosome formation. Autophagy 12, 917–935. doi:10.1080/15548627.2016.1160971
Garrido-Maraver, J., Loh, S. H. Y., and Martins, L. M. (2020). Forcing contacts between mitochondria and the endoplasmic reticulum extends lifespan in a Drosophila model of Alzheimer's disease. Biol. Open 9, bio047530. doi:10.1242/bio.047530
Gasperotti, M., Passamonti, S., Tramer, F., Masuero, D., Guella, G., Mattivi, F., et al. (2015). Fate of microbial metabolites of dietary polyphenols in rats: Is the brain their target destination? ACS Chem. Neurosci. 6, 1341–1352. doi:10.1021/acschemneuro.5b00051
Giacomello, M., and Pellegrini, L. (2016). The coming of age of the mitochondria–ER contact: A matter of thickness. Cell. Death Differ. 23, 1417–1427. doi:10.1038/cdd.2016.52
Giamogante, F., Barazzuol, L., Brini, M., and Calì, T. (2020). ER-mitochondria contact sites reporters: Strengths and weaknesses of the available approaches. Int. J. Mol. Sci. 21, 8157. doi:10.3390/ijms21218157
Gӧbel, J., Engelhardt, E., Pelzer, P., Sakthivelu, V., Jahn, H. M., Jevtic, M., et al. (2020). Mitochondria-endoplasmic reticulum contacts in reactive astrocytes promote vascular remodeling. Cell. Metab. 31, 791–808. e8. doi:10.1016/j.cmet.2020.03.005
Gomez-Suaga, P., Paillusson, S., Stoica, R., Noble, W., Hanger, D. P., and Miller, C. C. J. (2017). The ER-mitochondria tethering complex VAPB-PTPIP51 regulates autophagy. Curr. Biol. 27, 371–385. doi:10.1016/j.cub.2016.12.038
Gong, Z., Huang, J., Xu, B., Ou, Z., Zhang, L., Lin, X., et al. (2019). Urolithin A attenuates memory impairment and neuroinflammation in APP/PS1 mice. J. Neuroinflammation 16, 62. doi:10.1186/s12974-019-1450-3
Green, K. N., Demuro, A., Akbari, Y., Hitt, B. D., Smith, I. F., Parker, I., et al. (2008). SERCA pump activity is physiologically regulated by presenilin and regulates amyloid β production. J. Cell. Biol. 181, 1107–1116. doi:10.1083/jcb.200706171
Guo, Y., Cordes, K. R., Farese, R. V., and Walther, T. C. (2009). Lipid droplets at a glance. J. Cell. Sci. 122, 749–752. doi:10.1242/jcs.037630
Hamasaki, M., Furuta, N., Matsuda, A., Nezu, A., Yamamoto, A., Fujita, N., et al. (2013). Autophagosomes form at ER-mitochondria contact sites. Nature 495, 389–393. doi:10.1038/nature11910
Hampel, H., Williams, C., Etcheto, A., Goodsaid, F., Parmentier, F., Sallantin, J., et al. (2020). A precision medicine framework using artificial intelligence for the identification and confirmation of genomic biomarkers of response to an Alzheimer's disease therapy: Analysis of the blarcamesine (ANAVEX2-73) Phase 2a clinical study. Alzheimers Dement. 6, e12013. doi:10.1002/trc2.12013
Hanlon, N., Coldham, N., Gielbert, A., Kuhnert, N., Sauer, M. J., King, L. J., et al. (2008). Absolute bioavailability and dose-dependent pharmacokinetic behaviour of dietary doses of the chemopreventive isothiocyanate sulforaphane in rat. Br. J. Nutr. 99, 559–564. doi:10.1017/S0007114507824093
Hardy, J., and Selkoe, D. J. (2002). The amyloid hypothesis of Alzheimer's disease: Progress and problems on the road to therapeutics. Science 297, 353–356. doi:10.1126/science.1072994
Hayashi, T., and Su, T. P. (2007). Sigma-1 receptor chaperones at the ER- mitochondrion interface regulate Ca2+ signaling and cell survival. Cell. 131, 596–610. doi:10.1016/j.cell.2007.08.036
Hedskog, L., Pinho, C. M., Filadi, R., Ronnback, A., Hertwig, L., Wiehager, B., et al. (2013). Modulation of the endoplasmic reticulum-mitochondria interface in Alzheimer's disease and related models. Proc. Natl. Acad. Sci. U. S. A. 110, 7916–7921. doi:10.1073/pnas.1300677110
Heiss, E. H., Schachner, D., Zimmermann, K., and Dirsch, V. M. (2013). Glucose availability is a decisive factor for Nrf2-mediated gene expression. Redox Biol. 1, 359–365. doi:10.1016/j.redox.2013.06.001
Herrera-Cruz, M. S., and Simmen, T. (2017). Of yeast, mice and men: MAMs come in two flavors. Biol. Direct 12, 3. doi:10.1186/s13062-017-0174-5
Hewitt, V. L., Miller-Fleming, L., Andreazza, S., Mattedi, F., Prudent, J., Polleux, F., et al. (2020). Decreasing pdzd8-mediated mitochondrial-ER contacts in neurons improves fitness by increasing mitophagy. bioRxiv. 2020.11.14.382861-2020.11.14.382861.
Hirabayashi, Y., Kwon, S. K., Paek, H., Pernice, W. M., Paul, M. A., Lee, J., et al. (2017). ER-mitochondria tethering by PDZD8 regulates Ca(2+) dynamics in mammalian neurons. Science 358, 623–630. doi:10.1126/science.aan6009
Höing, S., Yeh, T. Y., Baumann, M., Martinez, N. E., Habenberger, P., Kremer, L., et al. (2018). Dynarrestin, a novel inhibitor of cytoplasmic dynein. Cell. Chem. Biol. 25, 357–369. e6. doi:10.1016/j.chembiol.2017.12.014
Holthuis, J. C., and Ungermann, C. (2013). Cellular microcompartments constitute general suborganellar functional units in cells. Biol. Chem. 394, 151–161. doi:10.1515/hsz-2012-0265
Huang, Y., Zheng, L., Halliday, G., Dobson-Stone, C., Wang, Y., Tang, H. D., et al. (2011). Genetic polymorphisms in sigma-1 receptor and apolipoprotein E interact to influence the severity of Alzheimer's disease. Curr. Alzheimer Res. 8, 765–770. doi:10.2174/156720511797633232
Hur, J.-Y., Teranishi, Y., Kihara, T., Yamamoto, N. G., Inoue, M., Hosia, W., et al. (2012). Identification of novel γ-secretase-associated proteins in detergent-resistant membranes from brain. J. Biol. Chem. 287, 11991–12005. doi:10.1074/jbc.M111.246074
Hurst, S., Hoek, J., and Sheu, S.-S. (2017). Mitochondrial Ca(2+) and regulation of the permeability transition pore. J. Bioenerg. Biomembr. 49, 27–47. doi:10.1007/s10863-016-9672-x
Iwasawa, R., Mahul-Mellier, A. L., Datler, C., Pazarentzos, E., and Grimm, S. (2011). Fis1 and Bap31 bridge the mitochondria-ER interface to establish a platform for apoptosis induction. EMBO J. 30, 556–568. doi:10.1038/emboj.2010.346
Jack, C. R., Knopman, D. S., Jagust, W. J., Shaw, L. M., Aisen, P. S., Weiner, M. W., et al. (2010). Hypothetical model of dynamic biomarkers of the Alzheimer's pathological cascade. Lancet. Neurol. 9, 119–128. doi:10.1016/S1474-4422(09)70299-6
Jadiya, P., Kolmetzky, D. W., Tomar, D., Di Meco, A., Lombardi, A. A., Lambert, J. P., et al. (2019). Impaired mitochondrial calcium efflux contributes to disease progression in models of Alzheimer’s disease. Nat. Commun. 10, 3885. doi:10.1038/s41467-019-11813-6
John, L. M., Lechleiter, J. D., and Camacho, P. (1998). Differential modulation of SERCA2 isoforms by calreticulin. J. Cell. Biol. 142, 963–973. doi:10.1083/jcb.142.4.963
Jouaville, L. S., Pinton, P., Bastianutto, C., Rutter, G. A., and Rizzuto, R. (1999). Regulation of mitochondrial ATP synthesis by calcium: Evidence for a long-term metabolic priming. Proc. Natl. Acad. Sci. U. S. A. 96, 13807–13812. doi:10.1073/pnas.96.24.13807
Karran, E., Mercken, M., and Strooper, B. D. (2011). The amyloid cascade hypothesis for Alzheimer's disease: An appraisal for the development of therapeutics. Nat. Rev. Drug Discov. 10, 698–712. doi:10.1038/nrd3505
Khan, S. M., Cassarino, D. S., Abramova, N. N., Keeney, P. M., Borland, M. K., Trimmer, P. A., et al. (2000). Alzheimer's disease cybrids replicate β-amyloid abnormalities through cell death pathways. Ann. Neurol. 48, 148–155. doi:10.1002/1531-8249(200008)48:2<148::aid-ana3>3.0.co;2-7
Kim, J. (2021). Pre-clinical neuroprotective evidences and plausible mechanisms of sulforaphane in alzheimer’s disease. Int. J. Mol. Sci. 22, 2929. doi:10.3390/ijms22062929
Koopman, W. J. H., Verkaart, S., Van Emst-De Vries, S. E., Grefte, S., Smeitink, J. A. M., Nijtmans, L. G. J., et al. (2008). Corrigendum to "Mitigation of NADH:ubiquinone oxidoreductase deficiency by chronic Trolox treatment" [Biochimica et Biophysica Acta 1777/7-8 (2008) 853-859]. Biochim. Biophys. Acta. Bioenerg. 1859, 1328. doi:10.1016/j.bbabio.2018.06.003
Korobova, F., Ramabhadran, V., and Higgs, H. N. (2013). An actin-dependent step in mitochondrial fission mediated by the ER-associated formin INF2. Science 339, 464–467. doi:10.1126/science.1228360
Kourrich, S., Su, T. P., Fujimoto, M., and Bonci, A. (2012). The sigma-1 receptor: Roles in neuronal plasticity and disease. Trends Neurosci. 35, 762–767. doi:10.1016/j.tins.2012.09.007
Kujawska, M., Jourdes, M., Kurpik, M., Szulc, M., Szaefer, H., Chmielarz, P., et al. (2020). Neuroprotective effects of pomegranate juice against Parkinson’s disease and presence of ellagitannins-derived metabolite—urolithin A—in the brain. Int. J. Mol. Sci. 21, 202. doi:10.3390/ijms21010202
Kwak, C., Shin, S., Park, J. S., Jung, M., My Nhung, T. T., Kang, M. G., et al. (2020). Contact-ID, a tool for profiling organelle contact sites, reveals regulatory proteins of mitochondrial-associated membrane formation. Proc. Natl. Acad. Sci. U. S. A. 117, 12109–12120. doi:10.1073/pnas.1916584117
Lau, D. H. W., Paillusson, S., Hartopp, N., Rupawala, H., Mórotz, G. M., Gomez-Suaga, P., et al. (2020a). Disruption of endoplasmic reticulum-mitochondria tethering proteins in post-mortem Alzheimer's disease brain. Neurobiol. Dis. 143, 105020. doi:10.1016/j.nbd.2020.105020
Lau, D. H. W., Paillusson, S., Hartopp, N., Rupawala, H., Mórotz, G. M., Gomez-Suaga, P., et al. (2020b). Disruption of endoplasmic reticulum-mitochondria tethering proteins in post-mortem Alzheimer's disease brain. Neurobiol. Dis. 143, 105020. doi:10.1016/j.nbd.2020.105020
Leal, N. S., Dentoni, G., Schreiner, B., Naia, L., Piras, A., Graff, C., et al. (2020). Amyloid β-peptide increases mitochondria-endoplasmic reticulum contact altering mitochondrial function and autophagosome formation in alzheimer’s disease-related models. Cells 9, 2552. doi:10.3390/cells9122552
Leal, N. S., and Martins, L. M. (2021). Mind the gap: Mitochondria and the endoplasmic reticulum in neurodegenerative diseases. Biomedicines 9, 227–235. doi:10.3390/biomedicines9020227
Leal, N. S., Schreiner, B., Pinho, C. M., Filadi, R., Wiehager, B., Karlstrom, H., et al. (2016). Mitofusin-2 knockdown increases ER-mitochondria contact and decreases amyloid beta-peptide production. J. Cell. Mol. Med. 20, 1686–1695. doi:10.1111/jcmm.12863
Lee, H. G., Zhu, X., Nunomura, A., Perry, G., and Smith, M. A. (2006). Amyloid beta: The alternate hypothesis. Curr. Alzheimer Res. 3, 75–80. doi:10.2174/156720506775697124
Lee, H. J., Jung, Y. H., Choi, G. E., Kim, J. S., Chae, C. W., Lim, J. R., et al. (2021). Urolithin A suppresses high glucose-induced neuronal amyloidogenesis by modulating TGM2-dependent ER-mitochondria contacts and calcium homeostasis. Cell. Death Differ. 28, 184–202. doi:10.1038/s41418-020-0593-1
Lee, Y. J., Jeong, S. Y., Karbowski, M., Smith, C. L., and Youle, R. J. (2004). Roles of the mammalian mitochondrial fission and fusion mediators Fis1, Drp1, and Opa1 in apoptosis. Mol. Biol. Cell. 15, 5001–5011. doi:10.1091/mbc.e04-04-0294
Leissring, M. A., Laferla, F. M., Callamaras, N., and Parker, I. (2001). Subcellular mechanisms of presenilin-mediated enhancement of calcium signaling. Neurobiol. Dis. 8, 469–478. doi:10.1006/nbdi.2001.0382
Leuner, K., Schütt, T., Kurz, C., Eckert, S. H., Schiller, C., Occhipinti, A., et al. (2012). Mitochondrion-derived reactive oxygen species lead to enhanced amyloid beta formation. Antioxid. Redox Signal. 16, 1421–1433. doi:10.1089/ars.2011.4173
Li, Y., and Camacho, P. (2004). Ca2+-dependent redox modulation of SERCA 2b by ERp57. J. Cell. Biol. 164, 35–46. doi:10.1083/jcb.200307010
Lin, C. W., Wu, M. J., Liu, I. Y. C., Su, J. D., and Yen, J. H. (2010). Neurotrophic and cytoprotective action of luteolin in PC12 cells through ERK-dependent induction of Nrf2-Driven HO-1 expression. J. Agric. Food Chem. 58, 4477–4486. doi:10.1021/jf904061x
Liu, Y., Ma, X., Fujioka, H., Liu, J., Chen, S., and Zhu, X. (2019). DJ-1 regulates the integrity and function of ER-mitochondria association through interaction with IP3R3-Grp75-VDAC1. Proc. Natl. Acad. Sci. U. S. A. 116, 25322–25328. doi:10.1073/pnas.1906565116
Lovell, M. A., Xiong, S., Xie, C., Davies, P., and Markesbery, W. R. (2004). Induction of hyperphosphorylated tau in primary rat cortical neuron cultures mediated by oxidative stress and glycogen synthase kinase-3. J. Alzheimers Dis. 6, 659–671. discussion 673-81. doi:10.3233/jad-2004-6610
Lúcio, M., Nunes, C., Gaspar, D., Ferreira, H., Lima, J. L. F. C., and Reis, S. (2009). Antioxidant activity of vitamin E and trolox: Understanding of the factors that govern lipid peroxidation studies in vitro. Food Biophys. 4, 312–320. doi:10.1007/s11483-009-9129-4
Ma, J. H., Shen, S., Wang, J. J., He, Z., Poon, A., Li, J., et al. (2017). Comparative proteomic analysis of the mitochondria-associated ER membrane (MAM) in a long-term type 2 diabetic rodent model. Sci. Rep. 7, 2062. doi:10.1038/s41598-017-02213-1
Magalhães Rebelo, A. P., Dal Bello, F., Knedlik, T., Kaar, N., Volpin, F., Shin, S. H., et al. (2020). Chemical modulation of mitochondria-endoplasmic reticulum contact sites. Cells 9, 1637. doi:10.3390/cells9071637
Magrì, A., Reina, S., and De Pinto, V. (2018). VDAC1 as pharmacological target in cancer and neurodegeneration: Focus on its role in apoptosis. Front. Chem. 6, 108. doi:10.3389/fchem.2018.00108
Mahase, E. (2021). Aducanumab: European agency rejects alzheimer’s drug over efficacy and safety concerns. BMJ 375, n3127. doi:10.1136/bmj.n3127
Manczak, M., and Reddy, P. H. (2012). Abnormal interaction of VDAC1 with amyloid beta and phosphorylated tau causes mitochondrial dysfunction in Alzheimer's disease. Hum. Mol. Genet. 21, 5131–5146. doi:10.1093/hmg/dds360
Martino Adami, P. V., Nichtová, Z., Weaver, D. B., Bartok, A., Wisniewski, T., Jones, D. R., et al. (2019). Perturbed mitochondria–ER contacts in live neurons that model the amyloid pathology of Alzheimer's disease. J. Cell. Sci. 132, jcs229906. doi:10.1242/jcs.229906
Matsuzaki, H., Fujimoto, T., Tanaka, M., and Shirasawa, S. (2013). Tespa1 is a novel component of mitochondria-associated endoplasmic reticulum membranes and affects mitochondrial calcium flux. Biochem. Biophys. Res. Commun. 433, 322–326. doi:10.1016/j.bbrc.2013.02.099
Maurice, T., Grégoire, C., and Espallergues, J. (2006). Neuro(active)steroids actions at the neuromodulatory sigma1 (sigma1) receptor: Biochemical and physiological evidences, consequences in neuroprotection. Pharmacol. Biochem. Behav. 84, 581–597. doi:10.1016/j.pbb.2006.07.009
Mehta, D., Jackson, R., Paul, G., Shi, J., and Sabbagh, M. (2017). Why do trials for alzheimer’s disease drugs keep failing? A discontinued drug perspective for 2010-2015. Expert Opin. Investig. Drugs 26, 735–739. doi:10.1080/13543784.2017.1323868
Minoshima, S., Giordani, B., Berent, S., Frey, K. A., Foster, N. L., and Kuhl, D. E. (1997). Metabolic reduction in the posterior cingulate cortex in very early Alzheimer's disease. Ann. Neurol. 42, 85–94. doi:10.1002/ana.410420114
Mishina, M., Ohyama, M., Ishii, K., Kitamura, S., Kimura, Y., Oda, K. I., et al. (2008). Low density of sigma1 receptors in early Alzheimer's disease. Ann. Nucl. Med. 22, 151–156. doi:10.1007/s12149-007-0094-z
Missiroli, S., Patergnani, S., Caroccia, N., Pedriali, G., Perrone, M., Previati, M., et al. (2018). Mitochondria-associated membranes (MAMs) and inflammation. Cell. Death Dis. 9, 329. doi:10.1038/s41419-017-0027-2
Morris, G. P., Clark, I. A., and Vissel, B. (2014). Inconsistencies and controversies surrounding the amyloid hypothesis of Alzheimer's disease. Acta Neuropathol. Commun. 2, 135. doi:10.1186/s40478-014-0135-5
Mosconi, L. (2005). Brain glucose metabolism in the early and specific diagnosis of Alzheimer's disease: FDG-PET studies in MCI and AD. Eur. J. Nucl. Med. Mol. Imaging 32, 486–510. doi:10.1007/s00259-005-1762-7
Muñoz, F. J., Opazo, C., Gil-Gómez, G., Tapia, G., Fernández, V., Valverde, M. A., et al. (2002). Vitamin E but not 17beta-estradiol protects against vascular toxicity induced by beta-amyloid wild type and the Dutch amyloid variant. J. Neurosci. 22, 3081–3089. doi:10.1523/JNEUROSCI.22-08-03081.2002
Murakami, T., Ockinger, J., Yu, J., Byles, V., Mccoll, A., Hofer, A. M., et al. (2012). Critical role for calcium mobilization in activation of the NLRP3 inflammasome. Proc. Natl. Acad. Sci. U. S. A. 109, 11282–11287. doi:10.1073/pnas.1117765109
Nabavi, S. F., Braidy, N., Gortzi, O., Sobarzo-Sanchez, E., Daglia, M., Skalicka-Woźniak, K., et al. (2015). Luteolin as an anti-inflammatory and neuroprotective agent: A brief review. Brain Res. Bull. 119, 1–11. doi:10.1016/j.brainresbull.2015.09.002
Naia, L., Ly, P., Mota, S., Lopes, C., Maranga, C., Coelho, P., et al. (2021a). The Sigma-1 receptor mediates pridopidine rescue of mitochondrial function in Huntington Disease models. Neurotherapeutics 18, 1017–1038. doi:10.1007/s13311-021-01022-9
Naia, L., Pinho, C. M., Dentoni, G., Liu, J., Leal, N. S., Ferreira, D. M. S., et al. (2021b). Neuronal cell-based high-throughput screen for enhancers of mitochondrial function reveals luteolin as a modulator of mitochondria-endoplasmic reticulum coupling. BMC Biol. 19, 57. doi:10.1186/s12915-021-00979-5
Namgaladze, D., Khodzhaeva, V., and Brüne, B. (2019). ER-mitochondria communication in cells of the innate immune system. Cells 8, 1088. doi:10.3390/cells8091088
Paganoni, S., Berry, J. D., Quintana, M., Macklin, E., Saville, B. R., Detry, M. A., et al. (2022). Adaptive platform trials to transform amyotrophic lateral sclerosis therapy development. Ann. Neurol. 91, 165–175. doi:10.1002/ana.26285
Paillusson, S., Gomez-Suaga, P., Stoica, R., Little, D., Gissen, P., Devine, M. J., et al. (2017). α-Synuclein binds to the ER–mitochondria tethering protein VAPB to disrupt Ca2+ homeostasis and mitochondrial ATP production. Acta Neuropathol. 134, 129–149. doi:10.1007/s00401-017-1704-z
Paillusson, S., Stoica, R., Gomez-Suaga, P., Lau, D. H. W., Mueller, S., Miller, T., et al. (2016). There's something wrong with my MAM; the ER-mitochondria Axis and neurodegenerative diseases. Trends Neurosci. 39, 146–157. doi:10.1016/j.tins.2016.01.008
Pera, M., Larrea, D., Guardia-Laguarta, C., Montesinos, J., Velasco, K. R., Agrawal, R. R., et al. (2017). Increased localization of APP-C99 in mitochondria-associated ER membranes causes mitochondrial dysfunction in Alzheimer disease. EMBO J. 36, 3356–3371. doi:10.15252/embj.201796797
Pernicova, I., and Korbonits, M. (2014). Metformin—Mode of action and clinical implications for diabetes and cancer. Nat. Rev. Endocrinol. 10, 143–156. doi:10.1038/nrendo.2013.256
Perreault, S., Bousquet, O., Lauzon, M., Paiement, J., and Leclerc, N. (2009). Increased association between rough endoplasmic reticulum membranes and mitochondria in transgenic mice that express P301 L tau. J. Neuropathol. Exp. Neurol. 68, 503–514. doi:10.1097/NEN.0b013e3181a1fc49
Poston, C. N., Krishnan, S. C., and Bazemore-Walker, C. R. (2013). In-depth proteomic analysis of mammalian mitochondria-associated membranes (MAM). J. Proteomics 79, 219–230. doi:10.1016/j.jprot.2012.12.018
Puri, R., Cheng, X. T., Lin, M. Y., Huang, N., and Sheng, Z. H. (2019). Mul1 restrains Parkin-mediated mitophagy in mature neurons by maintaining ER-mitochondrial contacts. Nat. Commun. 10, 3645. doi:10.1038/s41467-019-11636-5
Radesäter, A. C., Johansson, S., Öberg, C., and Luthman, J. (2003). The vitamin-E analog trolox and the NMDA antagonist MK-801 protect pyramidal neurons in hippocampal slice cultures from IL-1beta-induced neurodegeneration. Neurotox. Res. 5, 433–442. doi:10.1007/BF03033173
Ramsey, C. P., Glass, C. A., Montgomery, M. B., Lindl, K. A., Ritson, G. P., Chia, L. A., et al. (2007). Expression of Nrf2 in neurodegenerative diseases. J. Neuropathol. Exp. Neurol. 66, 75–85. doi:10.1097/nen.0b013e31802d6da9
Raturi, A., Gutiérrez, T., Ortiz-Sandoval, C., Ruangkittisakul, A., Herrera-Cruz, M. S., Rockley, J. P., et al. (2016). TMX1 determines cancer cell metabolism as a thiol-based modulator of ER–mitochondria Ca2+ flux. J. Cell. Biol. 214, 433–444. doi:10.1083/jcb.201512077
Reddy, P. H., Mcweeney, S., Park, B. S., Manczak, M., Gutala, R. V., Partovi, D., et al. (2004). Gene expression profiles of transcripts in amyloid precursor protein transgenic mice: Up-regulation of mitochondrial metabolism and apoptotic genes is an early cellular change in Alzheimer's disease. Hum. Mol. Genet. 13, 1225–1240. doi:10.1093/hmg/ddh140
Regner-Nelke, L., Nelke, C., Schroeter, C. B., Dziewas, R., Warnecke, T., Ruck, T., et al. (2021). Enjoy carefully: The multifaceted role of vitamin E in neuro-nutrition. Int. J. Mol. Sci. 22, 10087. doi:10.3390/ijms221810087
Reilmann, R., Rosser, A., Kostyk, S., Geva, M., Mcgarry, A., Cohen, Y., et al. (2021). F41 the proof-hd phase 3 study: pridopidine’s outcome on function in huntington disease (PROOF). J. Neurology, Neurosurg. Psychiatry 92, A36. doi:10.1136/jnnp-2021-EHDN.84
Rizzuto, R., Brini, M., Murgia, M., and Pozzan, T. (1993). Microdomains with high Ca2+ close to IP3-sensitive channels that are sensed by neighboring mitochondria. Science 262, 744–747. doi:10.1126/science.8235595
Rizzuto, R., and Pozzan, T. (2006). Microdomains of intracellular Ca2+: Molecular determinants and functional consequences. Physiol. Rev. 86, 369–408. doi:10.1152/physrev.00004.2005
Roderick, H. L., Lechleiter, J. D., and Camacho, P. (2000). Cytosolic phosphorylation of calnexin controls intracellular Ca(2+) oscillations via an interaction with SERCA2b. J. Cell. Biol. 149, 1235–1248. doi:10.1083/jcb.149.6.1235
Rodríguez, L. R., Calap-Quintana, P., Lapeña-Luzón, T., Pallardó, F. V., Schneuwly, S., Navarro, J. A., et al. (2020). Oxidative stress modulates rearrangement of endoplasmic reticulum-mitochondria contacts and calcium dysregulation in a Friedreich's ataxia model. Redox Biol. 37, 101762. doi:10.1016/j.redox.2020.101762
Rodríguez, L. R., Lapeña-Luzón, T., Benetó, N., Beltran-Beltran, V., Pallardó, F. V., Gonzalez-Cabo, P., et al. (2022). Therapeutic strategies targeting mitochondrial calcium signaling: A new hope for neurological diseases? Antioxidants (Basel, Switz. 11, 165. doi:10.3390/antiox11010165
Rohács, T., Tory, K., Dobos, A., and Spät, A. (1997). Intracellular calcium release is more efficient than calcium influx in stimulating mitochondrial NAD(P)H formation in adrenal glomerulosa cells. Biochem. J. 328 (2), 525–528. doi:10.1042/bj3280525
Rowland, A. A., and Voeltz, G. K. (2012). Endoplasmic reticulum-mitochondria contacts: Function of the junction. Nat. Rev. Mol. Cell. Biol. 13, 607–625. doi:10.1038/nrm3440
Ryskamp, D. A., Korban, S., Zhemkov, V., Kraskovskaya, N., and Bezprozvanny, I. (2019b). Neuronal sigma-1 receptors: Signaling functions and protective roles in neurodegenerative diseases. Front. Neurosci. 13, 862. doi:10.3389/fnins.2019.00862
Ryskamp, D., Wu, J., Geva, M., Kusko, R., Grossman, I., Hayden, M., et al. (2017). The sigma-1 receptor mediates the beneficial effects of pridopidine in a mouse model of Huntington disease. Neurobiol. Dis. 97, 46–59. doi:10.1016/j.nbd.2016.10.006
Ryskamp, D., Wu, L., Wu, J., Kim, D., Rammes, G., Geva, M., et al. (2019a). Pridopidine stabilizes mushroom spines in mouse models of Alzheimer’s disease by acting on the sigma-1 receptor. Neurobiol. Dis. 124, 489–504. doi:10.1016/j.nbd.2018.12.022
Sasaguri, H., Nilsson, P., Hashimoto, S., Nagata, K., Saito, T., De Strooper, B., et al. (2017). APP mouse models for Alzheimer's disease preclinical studies. EMBO J. 36, 2473–2487. doi:10.15252/embj.201797397
Sawmiller, D., Li, S., Shahaduzzaman, M., Smith, A. J., Obregon, D., Giunta, B., et al. (2014). Luteolin reduces alzheimer’s disease pathologies induced by traumatic brain injury. Int. J. Mol. Sci. 15, 895–904. doi:10.3390/ijms15010895
Schneeberger, M., Dietrich, M. O., Sebastian, D., Imbernon, M., Castano, C., Garcia, A., et al. (2013). Mitofusin 2 in POMC neurons connects ER stress with leptin resistance and energy imbalance. Cell. 155, 172–187. doi:10.1016/j.cell.2013.09.003
Schneider, L. S., Thomas, R. G., Hendrix, S., Rissman, R. A., Brewer, J. B., Salmon, D. P., et al. (2019). Safety and efficacy of edonerpic maleate for patients with mild to moderate alzheimer disease: A phase 2 randomized clinical trial. JAMA Neurol. 76, 1330–1339. doi:10.1001/jamaneurol.2019.1868
Schreiner, B., Hedskog, L., Wiehager, B., and Ankarcrona, M. (2015). Amyloid-beta peptides are generated in mitochondria-associated endoplasmic reticulum membranes. J. Alzheimers Dis. 43, 369–374. doi:10.3233/JAD-132543
Scorrano, L., De Matteis, M. A., Emr, S., Giordano, F., Hajnóczky, G., Kornmann, B., et al. (2019). Coming together to define membrane contact sites. Nat. Commun. 10, 1287. doi:10.1038/s41467-019-09253-3
Selkoe, D. J., and Hardy, J. (2016). The amyloid hypothesis of Alzheimer's disease at 25 years. EMBO Mol. Med. 8, 595–608. doi:10.15252/emmm.201606210
Sheehan, J. P., Swerdlow, R. H., Miller, S. W., Davis, R. E., Parks, J. K., Parker, W. D., et al. (1997). Calcium homeostasis and reactive oxygen species production in cells transformed by mitochondria from individuals with sporadic Alzheimer's disease. J. Neurosci. 17, 4612–4622. doi:10.1523/jneurosci.17-12-04612.1997
Shilling, D., Müller, M., Takano, H., Mak, D. O., Abel, T., Coulter, D. A., et al. (2014). Suppression of InsP3 receptor-mediated Ca2+ signaling alleviates mutant presenilin-linked familial Alzheimer's disease pathogenesis. J. Neurosci. 34, 6910–6923. doi:10.1523/JNEUROSCI.5441-13.2014
Shoshan-Barmatz, V., Nahon-Crystal, E., Shteinfer-Kuzmine, A., and Gupta, R. (2018). VDAC1, mitochondrial dysfunction, and Alzheimer's disease. Pharmacol. Res. 131, 87–101. doi:10.1016/j.phrs.2018.03.010
Simmen, T., Aslan, J. E., Blagoveshchenskaya, A. D., Thomas, L., Wan, L., Xiang, Y., et al. (2005). PACS-2 controls endoplasmic reticulum-mitochondria communication and Bid-mediated apoptosis. Embo J. 24, 717–729. doi:10.1038/sj.emboj.7600559
Smilansky, A., Dangoor, L., Nakdimon, I., Ben-Hail, D., Mizrachi, D., and Shoshan-Barmatz, V. (2015). The voltage-dependent anion channel 1 mediates amyloid β toxicity and represents a potential target for alzheimer disease therapy. J. Biol. Chem. 290, 30670–30683. doi:10.1074/jbc.M115.691493
Sood, A., Jeyaraju, D. V., Prudent, J., Caron, A., Lemieux, P., Mcbride, H. M., et al. (2014). A Mitofusin-2–dependent inactivating cleavage of Opa1 links changes in mitochondria cristae and ER contacts in the postprandial liver. Proc. Natl. Acad. Sci. U. S. A. 111, 16017–16022. doi:10.1073/pnas.1408061111
Stoica, R., De Vos, K. J., Paillusson, S., Mueller, S., Sancho, R. M., Lau, K. F., et al. (2014). ER-mitochondria associations are regulated by the VAPB-PTPIP51 interaction and are disrupted by ALS/FTD-associated TDP-43. Nat. Commun. 5, 3996. doi:10.1038/ncomms4996
Sugiura, A., Nagashima, S., Tokuyama, T., Amo, T., Matsuki, Y., Ishido, S., et al. (2013). MITOL regulates endoplasmic reticulum-mitochondria contacts via Mitofusin2. Mol. Cell. 51, 20–34. doi:10.1016/j.molcel.2013.04.023
Swerdlow, R. H. (2012). Alzheimer's disease pathologic cascades: Who comes first, what drives what. Neurotox. Res. 22, 182–194. doi:10.1007/s12640-011-9272-9
Szabadkai, G., Bianchi, K., Várnai, P., De Stefani, D., Wieckowski, M. R., Cavagna, D., et al. (2006). Chaperone-mediated coupling of endoplasmic reticulum and mitochondrial Ca2+ channels. J. Cell. Biol. 175, 901–911. doi:10.1083/jcb.200608073
Taliou, A., Zintzaras, E., Lykouras, L., and Francis, K. (2013). An open-label pilot study of a formulation containing the anti-inflammatory flavonoid luteolin and its effects on behavior in children with autism spectrum disorders. Clin. Ther. 35, 592–602. doi:10.1016/j.clinthera.2013.04.006
Teng, J., Takei, Y., Harada, A., Nakata, T., Chen, J., and Hirokawa, N. (2001). Synergistic effects of MAP2 and MAP1B knockout in neuronal migration, dendritic outgrowth, and microtubule organization. J. Cell. Biol. 155, 65–76. doi:10.1083/jcb.200106025
Tian, S., Lei, P., Zhang, J., Sun, Y., Li, B., and Shan, Y. (2021). Sulforaphane balances Ca(2+) homeostasis injured by excessive fat via mitochondria-associated membrane (MAM). Mol. Nutr. Food Res. 65, e2001076. doi:10.1002/mnfr.202001076
Triantafilou, K., Hughes, T. R., Triantafilou, M., and Morgan, B. P. (2013). The complement membrane attack complex triggers intracellular Ca2+ fluxes leading to NLRP3 inflammasome activation. J. Cell. Sci. 126, 2903–2913. doi:10.1242/jcs.124388
Tubbs, E., Axelsson, A. S., Vial, G., Wollheim, C. B., Rieusset, J., and Rosengren, A. H. (2018). Sulforaphane improves disrupted ER-mitochondria interactions and suppresses exaggerated hepatic glucose production. Mol. Cell. Endocrinol. 461, 205–214. doi:10.1016/j.mce.2017.09.016
Tubbs, E., Theurey, P., Vial, G., Bendridi, N., Bravard, A., Chauvin, M. A., et al. (2014). Mitochondria-associated endoplasmic reticulum membrane (MAM) integrity is required for insulin signaling and is implicated in hepatic insulin resistance. Diabetes 63, 3279–3294. doi:10.2337/db13-1751
Vance, J. E. (2014). MAM (mitochondria-associated membranes) in mammalian cells: Lipids and beyond. Biochim. Biophys. Acta 1841, 595–609. doi:10.1016/j.bbalip.2013.11.014
Vance, J. E. (1991). Newly made phosphatidylserine and phosphatidylethanolamine are preferentially translocated between rat-liver mitochondria and endoplasmic-reticulum. J. Biol. Chem. 266, 89–97. doi:10.1016/s0021-9258(18)52406-6
Vance, J. E. (1990). Phospholipid-synthesis in a membrane-fraction associated with mitochondria. J. Biol. Chem. 265, 7248–7256. doi:10.1016/s0021-9258(19)39106-9
Verma, M., Callio, J., Otero, P. A., Sekler, I., Wills, Z. P., and Chu, C. T. (2017). Mitochondrial calcium dysregulation contributes to dendrite degeneration mediated by PD/LBD-Associated LRRK2 mutants. J. Neurosci. 37, 11151–11165. doi:10.1523/JNEUROSCI.3791-16.2017
Wang, H., Wang, H., Cheng, H., and Che, Z. (2016). Ameliorating effect of luteolin on memory impairment in an Alzheimer's disease model. Mol. Med. Rep. 13, 4215–4220. doi:10.3892/mmr.2016.5052
Wang, W., Zhao, F., Ma, X., Perry, G., and Zhu, X. (2020). Mitochondria dysfunction in the pathogenesis of Alzheimer's disease: Recent advances. Mol. Neurodegener. 15, 30. doi:10.1186/s13024-020-00376-6
Wang, X., Su, B., Lee, H.-G., Li, X., Perry, G., Smith, M. A., et al. (2009). Impaired balance of mitochondrial fission and fusion in Alzheimer's disease. J. Neurosci. 29, 9090–9103. doi:10.1523/JNEUROSCI.1357-09.2009
Wang, X., Wen, Y., Dong, J., Cao, C., and Yuan, S. (2018). Systematic in-depth proteomic analysis of mitochondria-associated endoplasmic reticulum membranes in mouse and human testes. Proteomics 18, e1700478. doi:10.1002/pmic.201700478
Wasiak, S., Zunino, R., and Mcbride, H. M. (2007). Bax/Bak promote sumoylation of DRP1 and its stable association with mitochondria during apoptotic cell death. J. Cell. Biol. 177, 439–450. doi:10.1083/jcb.200610042
Weissig, V. (2020). Drug development for the therapy of mitochondrial diseases. Trends Mol. Med. 26, 40–57. doi:10.1016/j.molmed.2019.09.002
Wilkinson, S. T., and Sanacora, G. (2019). A new generation of antidepressants: An update on the pharmaceutical pipeline for novel and rapid-acting therapeutics in mood disorders based on glutamate/GABA neurotransmitter systems. Drug Discov. Today 24, 606–615. doi:10.1016/j.drudis.2018.11.007
Wilson, E. L., and Metzakopian, E. (2021). ER-Mitochondria contact sites in neurodegeneration: Genetic screening approaches to investigate novel disease mechanisms. Cell. Death Differ. 28, 1804–1821. doi:10.1038/s41418-020-00705-8
Wruck, C. J., Claussen, M., Fuhrmann, G., Römer, L., Schulz, A., Pufe, T., et al. (2007). Luteolin protects rat PC 12 and C6 cells against MPP+ induced toxicity via an ERK dependent Keapl-Nrf2-ARE pathway. J. Neural Transm. Suppl. 72, 57–67. doi:10.1007/978-3-211-73574-9_9
Xu, S. L., Zhu, K. Y., Bi, C. W., Choi, R. C., Miernisha, A., Yan, A. L., et al. (2013). Flavonoids induce the expression of synaptic proteins, synaptotagmin, and postsynaptic density protein-95 in cultured rat cortical neuron. Planta Med. 79, 1710–1714. doi:10.1055/s-0033-1351023
Yabal, M., Calleja, D. J., Simpson, D. S., and Lawlor, K. E. (2019). Stressing out the mitochondria: Mechanistic insights into NLRP3 inflammasome activation. J. Leukoc. Biol. 105, 377–399. doi:10.1002/JLB.MR0318-124R
Yeo, H. K., Park, T. H., Kim, H. Y., Jang, H., Lee, J., Hwang, G.-S., et al. (2021). Phospholipid transfer function of PTPIP51 at mitochondria-associated ER membranes. EMBO Rep. 22, e51323. doi:10.15252/embr.202051323
Zampese, E., Fasolato, C., Kipanyula, M. J., Bortolozzi, M., Pozzan, T., and Pizzo, P. (2011). Presenilin 2 modulates endoplasmic reticulum (ER)-mitochondria interactions and Ca 2+ cross-talk. Proc. Natl. Acad. Sci. U. S. A. 108, 2777–2782. doi:10.1073/pnas.1100735108
Zheng, N., Yuan, P., Li, C., Wu, J., and Huang, J. (2015). Luteolin reduces BACE1 expression through NF-κB and through estrogen receptor mediated pathways in HEK293 and SH-SY5Y cells. J. Alzheimers Dis. 45, 659–671. doi:10.3233/JAD-142517
Keywords: Alzheimer’s disease, mitochondria–endoplasmic reticulum contact sites, small molecules, neurodegeneration, mitochondrial function
Citation: Dentoni G, Castro-Aldrete L, Naia L and Ankarcrona M (2022) The Potential of Small Molecules to Modulate the Mitochondria–Endoplasmic Reticulum Interplay in Alzheimer’s Disease. Front. Cell Dev. Biol. 10:920228. doi: 10.3389/fcell.2022.920228
Received: 14 April 2022; Accepted: 08 June 2022;
Published: 26 August 2022.
Edited by:
Francesco Ciscato, University of Padua, ItalyReviewed by:
Cláudia Pereira, University of Coimbra, PortugalSandra M. Martin-Guerrero, King’s College London, United Kingdom
Copyright © 2022 Dentoni, Castro-Aldrete, Naia and Ankarcrona. This is an open-access article distributed under the terms of the Creative Commons Attribution License (CC BY). The use, distribution or reproduction in other forums is permitted, provided the original author(s) and the copyright owner(s) are credited and that the original publication in this journal is cited, in accordance with accepted academic practice. No use, distribution or reproduction is permitted which does not comply with these terms.
*Correspondence: Maria Ankarcrona, TWFyaWEuQW5rYXJjcm9uYUBraS5zZQ==