- Center for Genetic Medicine Research, Children’s National Hospital, Washington, DC, United States
Many studies support the link between parental obesity and the predisposition to develop adult-onset metabolic syndromes that include obesity, high blood pressure, dyslipidemia, insulin resistance, and diabetes in the offspring. As the prevalence of obesity increases in persons of childbearing age, so does metabolic syndrome in their descendants. Understanding how parental obesity alters metabolic programs in the progeny, predisposing them to adult-onset metabolic syndrome, is key to breaking this cycle. This review explores the basis for altered metabolism of offspring exposed to overnutrition by focusing on critical developmental processes influenced by parental obesity. We draw from human and animal model studies, highlighting the adaptations in metabolism that occur during normal pregnancy that become maladaptive with obesity. We describe essential phases of development impacted by parental obesity that contribute to long-term alterations in metabolism in the offspring. These encompass gamete formation, placentation, adipogenesis, pancreas development, and development of brain appetite control circuits. Parental obesity alters the developmental programming of these organs in part by inducing epigenetic changes with long-term consequences on metabolism. While exposure to parental obesity during any of these phases is sufficient to alter long-term metabolism, offspring often experience multiple exposures throughout their development. These insults accumulate to increase further the susceptibility of the offspring to the obesogenic environments of modern society.
Introduction
The obesity epidemic is becoming one of the most significant public health challenges worldwide. The incidence of obesity has nearly tripled since 1975 (WHO, 2021). In 2016, 39% of adults over 18 years of age were overweight (1.9 billion adults), and 13% of adults were affected by obesity (650 million adults) (WHO, 2021). The number of children that are overweight or obese is rising, affecting 340 million children between the ages of 5–19 and 39 million children under the age of 5 worldwide (WHO, 2021). Likewise, obesity among women of childbearing age is increasing. For instance, in the United States, maternal obesity has increased by 14% since the 1990s (Poston, et al., 2016; Driscoll, 2020).
The idea that maternal nutritional status during early life can influence the long-term health of the offspring is now widely accepted (Godfrey et al., 2010; Hardikar et al., 2015). Originally known as the “Barker hypothesis,” this theory described the association between low birth weight and the development of cardiovascular disease in adulthood (Barker, 1990). The hypothesis proposes that undernutrition early in life increases susceptibility to adult-onset metabolic syndrome, including obesity, high blood pressure, dyslipidemia, and insulin resistance (Barker, 1990; Barker et al., 1990; Lecoutre et al., 2021). The theory was later renamed the Developmental Origins of Health and Disease (DOHaD) Hypothesis to include the influence of overnutrition (obesity) and to extend the critical exposure window from gamete formation to postnatal development (Wadhwa et al., 2009).
The DOHaD hypothesis explains the relationship between low or high birth weight and the increased risk for developing metabolic syndrome in adulthood. Defined as a full-term birth weight less than <2.5 kg, low birth weight can be due to intrauterine growth restriction (IUGR), placental insufficiency, or maternal undernutrition. Approximately 15% of births worldwide, or more than 20 million infants each year, fall into this category (Cutland et al., 2017). Changes in metabolism in the low-birthweight fetus are adaptive to survive in the nutrient-poor in utero environment (Fall, 2013; Bhowmik et al., 2019). Ironically, when exposed to an obesogenic environment later in life, these same adaptations increase the risk of the offspring to metabolic disorders (Yajnik and Yajnik, 2020).
Parental obesity likewise predisposes the offspring to develop metabolic syndromes in adulthood. For instance, several human observational studies suggest maternal obesity is associated with metabolic disease in the offspring (Godfrey et al., 2017). Additional cohort studies indicate that parental Body Mass Index (BMI) predicts the BMI in the offspring (O’Callaghan et al., 1997; Rooney et al., 2011; Laitinen et al., 2012; Kaseva et al., 2018). The underlying mechanisms have been experimentally explored in animal models, that include rodents, sheep, and non-human primates (Reynolds et al., 2017). Studies in rodents and sheep demonstrate that feeding a high-fat diet during the periconceptional period and throughout gestation predisposes the offspring to develop metabolic syndrome (Rattanatray et al., 2010; Tessier et al., 2013; Lecoutre et al., 2016; Akhaphong et al., 2021). Lactation is another critical period when developmental programming is sensitive to overnutrition (Srinivasan and Patel, 2008). For example, a recent meta-analysis of multiple human observational studies reveals that breastfeeding is inversely associated with childhood obesity (Qiao et al., 2020). In the rat model, reducing litter size results in over-nourishment of pups during lactation and predisposes the offspring to hyperphagia, obesity, and metabolic syndrome later in life (Patel and Srinivasan, 2011)).
Understanding how parental obesity alters metabolic programs, predisposing the offspring to adult-onset metabolic syndrome, is key to breaking this cycle (Fall, 2013; Wells et al., 2020). In this review, we explore, from a developmental perspective, how parental obesity influences metabolism in the offspring. We draw from studies of human populations and experimental animal model systems revealing critical development processes impacted by obesity that contribute to long-term alterations in metabolism. These encompass gamete formation, placentation, adipogenesis, pancreas development, and brain appetite control circuits.
Adiposopathy: Anatomic, endocrine, and metabolic dysfunction of adipose tissue in obesity
Obesity, adiposity, and adiposopathy
While obesity is linked to metabolic disease, not all individuals with obesity develop metabolic syndrome (Neri and Edlow, 2016; Pétursdóttir Maack et al., 2020). Shortcomings in the metrics used to define obesity contribute to this discrepancy (Borga et al., 2018). For instance, obesity is often determined based on BMI, calculated using a patient’s height and weight, but does not differentiate between muscle, adipose tissue, or the distribution of adipose tissue (Liu et al., 2010; Rospleszcz et al., 2019). Adiposity is suggested as a measure of obesity as it excludes muscle mass and incorporates total and compartment-specific measurements of the adipose tissue (Borga et al., 2018). However, neither BMI nor adiposity can fully account for adipose tissue dysfunction. Adiposopathy, or “sick fat,” is a term that describes adipose dysfunction that comprises multiple histopathologic and molecular changes (Bays, 2011). The physiologic and endocrine changes that occur with adiposopathy include changes in the distribution of adipose tissue, inflammation, lipotoxicity, fibrosis, insulin resistance, and changes in the secretion of adipokines and extracellular vesicles (EVs) from adipose tissue. These measures have become more relevant as predictors of obesity-related metabolic disease over the past several years than hypertrophy of adipose tissue (Mundi et al., 2010; Pincu et al., 2022).
Visceral fat accumulates around the intra-abdominal organs, and subcutaneous fat accumulates under the skin. Visceral adiposopathy contributes to metabolic syndrome, whereas subcutaneous accumulation is generally less harmful (Hoffstedt et al., 2010; Chait and den Hartigh, 2020). Furthermore, lower body subcutaneous fat is healthier with greater insulin sensitivity, and upper body subcutaneous fat is more insulin resistant (Chait and den Hartigh, 2020). The two main types of adipose tissue most relevant to obesity are brown adipose tissue (BAT) and white adipose tissue (WAT). BAT generates heat by burning calories, whereas WAT storages excess energy (Chait and den Hartigh, 2020). The mass and metabolic activity of BAT decrease with age and weight (Alcalá et al., 2019; Parra-Peralbo et al., 2021). With obesity, lipid accumulation and mitochondrial dysfunction shift the function of BAT from thermogenesis to lipid storage (Shimizu and Walsh, 2015). WAT also becomes dysfunctional (Figure 1). The energy storage capacity of WAT can expand by hyperplasia (cell number increase) or by hypertrophy (increased cell size). Hyperplasia involves the differentiation of adipocytes from pre-adipocytes in a process called adipogenesis. In contrast, hypertrophy of WAT results in increased release of inflammatory cytokines (e.g., TNF-α and IL-6) that promote insulin resistance (Makki et al., 2013). Hypertrophy of WAT with adiposopathy is accompanied by fibrosis, macrophage infiltration, hypoxia, and dysregulated secretion of adipokines and EVs, creating a pro-inflammatory environment (Ibrahim, 2010; Laforest et al., 2015).
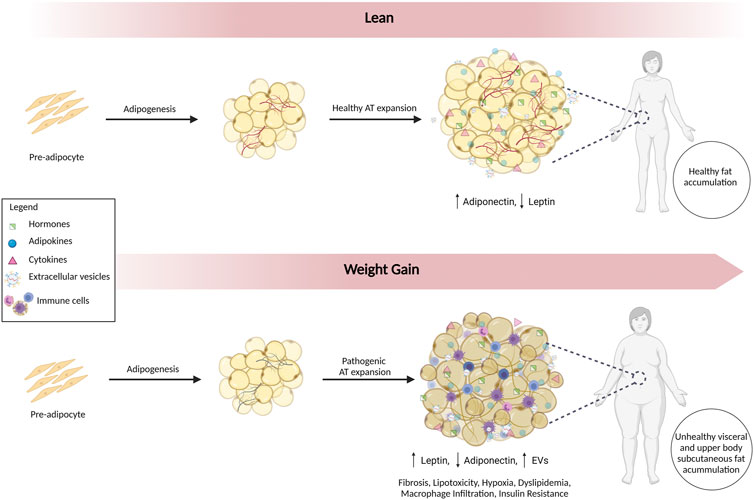
FIGURE 1. Alterations in Adipose Tissue in Adiposopathy. Adipogenesis involves the differentiation of adipocytes from pre-adipocytes. In lean individuals, healthy accumulation of subcutaneous fat predominates. With adiposopathy, WAT becomes dysfunctional, and expansion is accompanied by inflammation, fibrosis, lipotoxicity, hypoxia, dyslipidemia, macrophage infiltration, insulin resistance, dysregulated secretion of adipokines, and EVs. Fat accumulation occurs in metabolically unhealthy visceral and upper body subcutaneous depots.
Although excessive adipocyte hypertrophy is implicated in obesity/adiposopathy, recent studies suggested that adipocyte size and cell number can vary between individuals with obesity (Mundi et al., 2010; Suárez-Cuenca et al., 2021). The hypothesis that adipocyte size is a good predictor of the metabolic complications of obesity has been tested in animal models and human studies. In a lipodystrophic mouse model, reduced adipocyte number and size were associated with glucose intolerance, insulin resistance, increased serum triglycerides, and pro-inflammatory mediators (Sivasami et al., 2019). In patients with controlled obesity, small adipocytes are associated with inflammation in subcutaneous adipose tissue. This association was stronger in insulin-resistant than insulin-sensitive individuals (McLaughlin et al., 2007; McLaughlin et al., 2010). Moreover, Suaréz-Cuenca et al. (2021) described that adipocyte morphology and source are differentially related to insulin, adiponectin, blood glucose, and HbA1C levels in patients with obesity.
These data suggest that additional factors, not just adipocyte hypertrophy vs. hyperplasia, must be considered to characterize someone’s risk of obesity-related metabolic disease. One must characterize not just the size and distribution of adipose tissue but also adipose tissue function to understand the metabolic risks of obesity. Some have described the histopathologic and molecular changes in adipose tissue that correspond to the severity of obesity-related disease in individuals can be called “Obesity-related Adipose tissue Disease” or “OrAD.” These changes include adipocyte hypertrophy, inflammatory changes in the tissue, increasing fibrosis, and changes in the distribution of adipose tissue (Pincu et al., 2022). Accumulating dysfunctional ectopic visceral fat is metabolically harmful and is associated with insulin resistance, dyslipidemia, and type 2 diabetes (Neeland et al., 2019).
Changes in endocrine function of adipose tissue in obesity
Adipose tissue not only stores energy but is an active endocrine organ that secretes autocrine and paracrine factors to influence energy metabolism throughout the body (Funcke and Scherer, 2019). Adiposopathy is associated with increased leptin and decreased adiponectin, contributing to metabolic disease (Frühbeck et al., 2019; Longo et al., 2019). In lean individuals, leptin secretion from WAT increases after feeding to signal satiety and control energy balance by regulating glucose and fat metabolism. However, with adiposopathy, prolonged elevated leptin levels and reduced circulating soluble leptin receptors results in the development of a leptin-resistant state (Ogier et al., 2002; Holm et al., 2011). In addition to regulating satiety, leptin acts as an inflammatory cytokine to modulate the immune response, and the increased leptin levels with adiposopathy foster low-grade chronic inflammation (La Cava, 2017; Kumar et al., 2020).
Conversely, adiponectin is a protective adipokine and has an inverse relationship with insulin resistance, visceral adiposity, and inflammatory markers (Booth et al., 2016). Adiponectin regulates glucose and fat metabolism but is anti-inflammatory (Fu et al., 2005). Low adiponectin levels are driven by increased visceral adiposity and are associated with chronic low-grade inflammation, with elevated TNFα, C-reactive protein, and IL-6 (Longo et al., 2019).
Changes in extracellular vesicles in adiposopathy
EVs are another important secreted factor in adiposopathy (Camino et al., 2020; Huang and Xu, 2021). EVs are a heterogeneous group of cell-derived membranous particles with essential roles in intercellular communication. EVs contain proteins, lipids, and microRNAs that regulate the metabolism of multiple organs, including the liver, skeletal muscle, pancreas, and brain (Ferrante et al., 2015; Whitham et al., 2018; Barberio et al., 2019; Pérez-González et al., 2020). The contents and release of EVs are dramatically altered with adiposopathy (Akbar et al., 2019). Differences in miRNA contents of EVs are found in patients with obesity that can be improved with gastric bypass surgery (Koeck et al., 2014; Ferrante et al., 2015; Hubal et al., 2017). Interestingly, insulin resistance was one of the major pathways targeted by miRNAs in these EVs. Adiposopathy changes in EVs are likely functional; for instance, adipose-derived EVs from obese mice can stimulate inflammation and insulin resistance in lean mice (Deng et al., 2009). Conversely, EVs from lean mice improve insulin sensitivity in obese mice (Ying et al., 2017). Moreover, plasma EVs from women with obesity induce impaired insulin-stimulated glucose uptake in a human adipocyte cell line (Mleczko et al., 2018). These observations support the hypothesis that changes in the quantity and content of EVs are likely important mediators of dysfunction and inflammation that occur with adiposopathy (Ferrante et al., 2015; Huang-Doran et al., 2017).
Together, the changes in levels of EVs and adipokines that occur with adiposopathy play a central role in developing insulin resistance, type 2 diabetes, glucose intolerance, cardiovascular disease, and dyslipidemia (Landecho et al., 2019). As described in the next section, during pregnancy, these adiposopathy-associated metabolic changes collide with metabolic adaptations that prepare the mother for gestation and lactation. Thus, pregnancy-associated metabolic adaptations become pathological in women with obesity, altering not only the mother’s metabolism but the long-term metabolism of the developing fetus.
Metabolic and endocrine changes in lean pregnancies
To understand how metabolism is altered in pregnancies with obesity, one must first appreciate the normal metabolic changes that occur during pregnancy (Figure 2). In lean pregnancies, maternal metabolism changes to accommodate increased energy needs for gestation and lactation. Lower body subcutaneous fat preferentially expands, but visceral fat later increases (Trivett et al., 2021). These changes occur in two phases (Zeng et al., 2017; Parrettini et al., 2020). In the first half of pregnancy, the anabolic phase enhances insulin sensitivity and expands fat stores in preparation for increased energy needs and lactation. The catabolic phase is characterized by insulin resistance which facilitates enhanced delivery of glucose and lipid to the developing fetus (Parrettini et al., 2020). This shift in metabolism results from changes to pancreatic β cells, liver hepatocytes, and adipocytes and in response to hormones, cytokines, and EVs secreted from the placenta and adipose tissue. (Jayabalan et al., 2017; Kampmann et al., 2019). Moreover, β cell mass, insulin secretion, and hepatic gluconeogenesis increase during pregnancy to enhance maternal weight gain and provide energy to the developing fetus (Banerjee, 2018; Salazar-Petres and Sferruzzi-Perri, 2022).
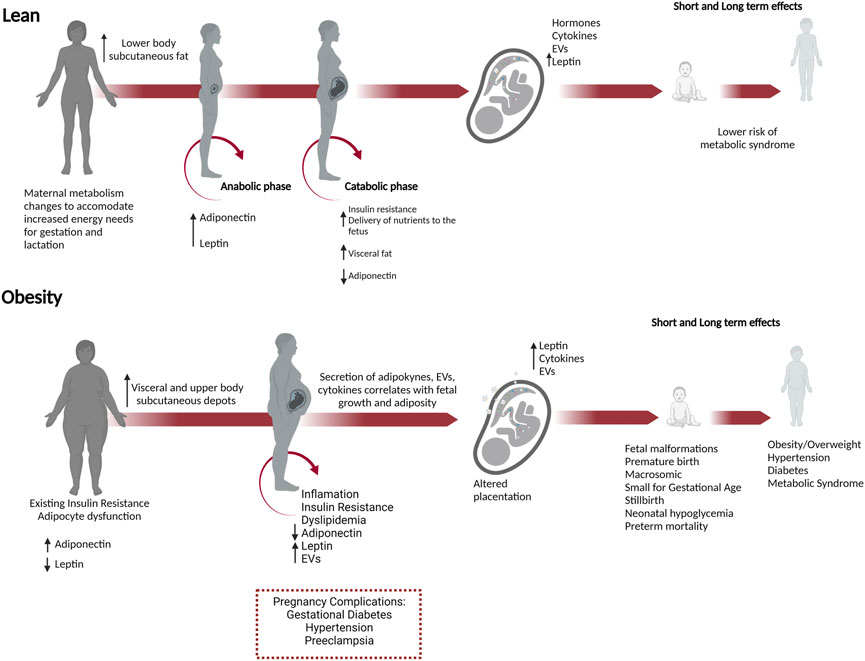
FIGURE 2. Obesity in pregnancy predisposes to short and long-term adverse outcomes in both the mother and offspring. Necessary metabolic adaptations occur during pregnancy that can go awry in women who enter pregnancy as overweight or obese. In lean pregnancies, maternal metabolism changes in anticipation of increased energy needs for gestation, and lactation. In the anabolic phase, increased insulin sensitivity and adiponectin promote the expansion of fat stores in lower body subcutaneous depots. In the catabolic phase during the second half of pregnancy, decreased adiponectin and insulin resistance occur to meet the increased energy needs and facilitate increased nutrient delivery to the developing fetus. These changes are managed in lean pregnancies and do not progress to metabolic dysfunction. In women with obesity, preexisting adipocyte dysfunction and insulin resistance result in fewer reserves for fat expansion and β cell adaptions. Visceral and upper body subcutaneous depots predominate, and fat is metabolically unhealthy with increased release of leptin, EVs, and decreased adiponectin. In lean women, lower body subcutaneous fat preferentially expands, whereas, in pregnancies with obesity, the growth of metabolically unfavorable visceral and upper body subcutaneous depots predominates. These changes have a greater likelihood of causing metabolic dysfunction in the mother, inducing insulin resistance, gestational diabetes, and hypertension. In the conceptus, placental dysfunction develops with increased leptin, cytokines, and EVs secretion, nutrient transporter expression. Together these changes increase fetal growth and adiposity, predisposing the offspring to metabolic syndrome (obesity, hypertension, and diabetes) in adulthood.
During human pregnancy, weight gain and increased fat mass results in elevated leptin secretion from both maternal adipocytes and the placenta (Masuzaki et al., 1997; Pérez-Pérez et al., 2018). These changes lead to increased leptin levels in the first and second trimesters of pregnancy and then a return to pre-pregnancy levels before birth (Briffa et al., 2015). Leptin resistance is an adaptation that occurs during pregnancy that allows for increases appetite and weight gain (Augustine et al., 2008). Furthermore, leptin is essential for implantation and placentation and plays a vital role in the development of the fetus (Pérez-Pérez et al., 2018). Adiponectin is secreted by adipose tissue during pregnancy and promotes insulin sensitivity, stimulating glucose uptake in maternal skeletal muscle (Aye et al., 2013). Adiponectin levels increase in the anabolic phase of pregnancy, supporting maternal weight gain, and then decrease during the catabolic phase, facilitating the allocation of nutrients to the fetus (Catalano et al., 2006; Mazaki-Tovi et al., 2007).
EVs are involved in maternal-fetal crosstalk during pregnancy, mediating an array of processes necessary for a successful pregnancy, including implantation, vascularization, decidualization, placentation, parturition, and modulation of the maternal immune response and metabolism (Jayabalan et al., 2019b; Bai et al., 2021; Condrat et al., 2021). In lean pregnancies, EVs are secreted from the placenta to influence maternal physiology and also signal from mother to fetus by interacting with the placenta and possibly the developing fetus (Czernek and Düchler, 2020). The total number of circulating EVs increases during pregnancy by approximately 50-fold (Salomon et al., 2014; Nakahara et al., 2020). Human placental-derived EVs are detected in maternal plasma as early as 6 weeks of gestation, with levels continuing to increase during gestation (Sarker et al., 2014). EVs may also reach the developing fetus. For instance, Cre-containing exosomes injected into the maternal circulation of cre-reporter mice, resulting in the recombination of the reporter in fetal membranes and the placenta (Sheller-Miller et al., 2019). Another study shows that DiI-labeled adipocyte-derived exosomes injected into the tail vein of pregnant mice can be found in the placenta and heart of embryos (Liu et al., 2021).
Maladaptive changes in metabolism in pregnancies with obesity
Numerous metabolic and physiologic adaptations occur during pregnancy to ensure the delivery of sufficient nutrients to the developing fetus and prepare the mother for delivery and breastfeeding. These changes can become pathogenic in women who enter pregnancy as overweight or with obesity (Figure 2). They adversely affect insulin resistance, glucose, and lipid homeostasis in the mother and contribute to adverse pregnancy outcomes, including gestational diabetes, hypertension, cesarean delivery, postpartum hemorrhage, stillbirth, and preeclampsia (Fitzsimons et al., 2009; Nelson et al., 2010; Felisbino-Mendes et al., 2014). Moreover, maternal obesity increases the risk for developing a range of health problems later in life for the offspring, including a tendency to become overweight or obese and to develop hypertension, type 2 diabetes, metabolic syndrome, asthma, and neurocognitive disorders (Neri and Edlow, 2016; Kelly et al., 2020; Chang et al., 2021).
In pregnancies with obesity, growth of metabolically unfavorable visceral and upper body subcutaneous depots predominates (Ehrenberg et al., 2003; Straughen et al., 2013). Lean women can better accommodate the expansion of existing adipocytes, but the expansion of adipocytes in women with obesity is limited. Adipocyte dysfunction exacerbates the low-grade inflammation, insulin resistance, and dyslipidemia that normally occur during pregnancy, affecting placentation and contributing to the development of gestational diabetes (Figure 2; Trivett et al., 2021; Salazar-Petres and Sferruzzi-Perri, 2022). Placental dysfunction in pregnancies with obesity further contributes to complications, influencing the metabolic state of the mother through the release of hormones, cytokines, and EVs (Jayabalan et al., 2017; Kampmann et al., 2019).
Maladaptive changes in adipokines and EVs secretions in pregnancies with obesity
While changes in adipokines and EVs are responsible for altering maternal metabolism to support pregnancy, in pregnancies affected by obesity, these changes contribute to metabolic dysfunction aggravating insulin resistance, hyperlipidemia, and inflammation (Christian and Porter, 2014; Corrales et al., 2021). Elevated leptin in pregnancies with obesity alters placentation, promotes inflammation, increases the risk for gestational diabetes, and correlates with increased fetal adiposity (Masuzaki et al., 1997; Pérez-Pérez et al., 2018). Conversely, reduced adiponectin further contributes to insulin resistance and gestational diabetes in both humans and rodents (Aye et al., 2013; Haghiac et al., 2014). For instance, in an obese rodent model, lower adiponectin levels in pregnancy increase the uptake of glucose and other nutrients by the placenta, that can be reversed by supplementing pregnant obese mice with adiponectin improving placental function, birthweight, and metabolism in the offspring (Aye et al., 2015; Paulsen et al., 2019; Vaughan et al., 2020).
Elevated levels of circulating placenta-derived EVs are found in both gestational diabetes and pregnancies with obesity (Salomon et al., 2016; Elfeky et al., 2017). Gestational diabetes is associated with increased levels of placenta-derived EVs which in turn increase the release of cytokines from endothelial cells contributing to fetoplacental endothelial dysfunction (Rice et al., 2015; Salomon et al., 2016; Sáez et al., 2018a, 2018b). Moreover, in gestational diabetes, EVs in maternal circulation are elevated with pathological changes in the composition and bioactivity of their contents that negatively affect energy production, inflammation, and metabolism (Salomon et al., 2016; Palma et al., 2022). For instance, differential expression of proteins related to insulin sensitivity is found in EVs in women with gestational diabetes (Jayabalan et al., 2019a). The miRNAs enriched in EVs from women with gestational diabetes target insulin and glucose regulatory pathways (Gillet et al., 2019). Moreover, placenta-derived EVs from pregnancies with gestational diabetes contain higher levels of inflammatory cytokines (Rice et al., 2015).
In summary, metabolic adaptations during pregnancy can become maladaptive in women with obesity. Changes in adiposity, adipokines, and EV composition and release contribute to the development of gestational diabetes and other pregnancy complications associated with obesity. These conditions negatively affect the metabolic programming of the offspring, predisposing them to metabolic syndrome later in life.
Changes in developmental programming in the offspring from parents with obesity
Pathogenic changes in adipokines and EVs, hyperlipidemia, insulin resistance, and elevated glucose in pregnant mothers with obesity alter the developmental programming of multiple organ systems in the offspring. These changes have lifelong effects on metabolism by modifying the epigenome and altering gene expression. Epigenetic mechanisms play critical roles in fetal programming, predisposing offspring to metabolic syndrome (Barouki et al., 2012; Neri and Edlow, 2016; Li, 2018). Deviations in developmental programming induced by parental obesity occur as early as gamete formation and continue through lactation. In the placenta, maternal obesity leads to changes in gene expression which not only alter placental hormone production but increase inflammation and nutrient transport to the developing fetus (Huynh et al., 2015; Gallo et al., 2017; Kelly et al., 2020). Augmented delivery of nutrients, lipids, and glucose to the developing fetus modify developmental plasticity and epigenetic programming of fetal adipocytes and pancreatic β cells. Postnatally, changes in the development of appetite control circuits that control satiety regulation led to hyperphagia (McMillen et al., 2005). Epigenetic changes affect long-term lipid metabolism and energy expenditure in the liver and skeletal muscle in the offspring (Friedman, 2018). Together, these deviations in developmental programming permanently alter the metabolism of the offspring, predisposing them to metabolic syndrome later in life.
Parental obesity modifies the epigenome
Epigenetic modifications are persistent and heritable changes in the DNA. They include DNA methylation, post-translational modifications of histones, and noncoding RNA such as microRNAs that regulate gene expression at both transcriptional and post-transcriptional levels (Skvortsova et al., 2018). DNA methylation requires the transfer of a methyl group from donors synthesized in the one-carbon metabolism cycle. Multiple micronutrient deficiencies are associated with obesity and can impact one-carbon metabolism affecting the availability of methyl donors required for epigenetic modifications providing a link between maternal diet and epigenetic regulation. For instance, obesity is associated with folate and B12 deficiency and elevated homocysteine in human populations (Sanchez-Margalet et al., 2002; Karatela and Sainani, 2009). In mouse models, feeding a high-fat diet alters epigenetic programming through the reduced availability of methyl donors (Vucetic et al., 2010). Differentiation of human adipocytes in low vitamin B12 conditions alters the expression of miRNAs involved in adipocyte differentiation and function altering expression of adipocyte-derived circulating miRNAs that target transcripts involved in adipogenesis (Adaikalakoteswari et al., 2017).
Vitamin D is another critical micronutrient with epigenetic consequences. Vitamin D deficiency is common in individuals with obesity as higher adiposity reduces circulating vitamin D (Hyppönen and Boucher, 2018). Children of vitamin D deficient mothers and offspring of vitamin D deficient mice have higher BMIs (Nascimento et al., 2013; Morales et al., 2015; Daraki et al., 2018). Moreover, vitamin D deficiency in childhood is linked to increased adiposity and metabolic syndrome in adulthood (Hyppönen and Boucher, 2018).
The association of obesity with imprinting syndromes such as Prader-Willi syndrome (PWS) and Albright hereditary osteodystrophy further implicates epigenetics in obesity (Shapira et al., 2005; Butler, 2009). Moreover, essential genes that regulate fetal size are regulated by imprinting. These include components of the insulin-like growth factor signaling pathway such as IGF2, expressed from the paternal allele to promote growth, and IGF2R, expressed from the maternal allele to inhibit growth (Smith et al., 2006). Several genome-wide association studies suggest that genetics likely only accounts for modest proportions of obesity at the population level (Loos, 2012). In this regard, epigenetic, rather than genetic mechanisms, are likely important in influencing developmental programming.
Parental obesity alters gametogenesis and preimplantation development of the offspring
From oocyte/sperm maturation to pre-and post-implantation development, the periconceptional period is a critical time in development where parental obesity can influence epigenetic changes with long-term metabolic consequences on the offspring (Huypens et al., 2016; Nicholas et al., 2016). Analysis of in vitro fertilization (IVF) data in humans and experiments in mice indicate that oocyte quality is influenced by maternal obesity, which impacts the metabolism of the offspring through epigenetic changes (Igosheva et al., 2010; Luzzo et al., 2012; Leary et al., 2015; Fleming et al., 2018). Transfer of IVF embryos from high-fat diet-fed mice with obesity to lean surrogates results in greater susceptibility to obesity and diabetes (Huypens et al., 2016). Interestingly, metabolic changes were more prominently inherited through maternal than paternal gametes, and female offspring were more susceptible than their male siblings (Huypens et al., 2016). The conclusion that epigenetic changes mediate these results is further supported by transgenerational inheritance, where the F2 generation (grandchildren) of the grandparents (F0) exposed to a high-fat rodent diet during gamete production were predisposed to metabolic syndromes (Anwer et al., 2022). Additional evidence of the importance of the preimplantation exposure window on the metabolism of the offspring comes from studies of IVF in humans. For instance, children conceived by IVF are at increased risk for imprinting disorders and metabolic diseases, including high blood pressure, insulin resistance, hyperlipidemia, and obesity (Ceelen et al., 2008; Scherrer et al., 2012; Valenzuela-Alcaraz et al., 2013; Gkourogianni et al., 2014). Similarly, studies in mice showed that the culture of preimplantation embryos before embryo transfer resulted in long-term metabolic changes in the offspring (Watkins et al., 2007).
Epigenetic changes in the sperm also contribute to the transgenerational inheritance of obesity and metabolic disorders (Craig et al., 2017). In humans, paternal obesity is associated with effects on placental development and gene expression in the liver and pancreas (Binder et al., 2012, 2015). Similarly, in rodent models, paternal obesity is linked to changes in DNA methylation and altered gene expression in the fetal liver and pancreas (Carone et al., 2010; Wei et al., 2014). Additional studies in rats connect paternal obesity to altered gene expression in sperm and long-term changes in the metabolism of female offspring (Ng et al., 2010, 2014). For instance, feeding male rats a high-fat diet alters DNA methylation patterns in the progeny, providing further evidence that paternal obesity is transmitted through epigenetic changes (Sinclair and Watkins, 2013; De Castro Barbosa et al., 2016; Fleming et al., 2018; Deshpande et al., 2021).
Maternal obesity and changes in the placenta
The placenta is essential for exchanging nutrients and waste between maternal and fetal circulations. It secretes hormones that support pregnancy and acts as a sensor, altering the expression of nutrient transporters to align the uptake of nutrients with maternal metabolism (Glazier et al., 1997; Constância et al., 2002, 2005; Dilworth et al., 2010; Huynh et al., 2015; Myatt and Maloyan, 2016). Significantly, maternal obesity and gestational diabetes induce multiple pathogenic changes in the placenta. These include increased placental weight, inflammation, and altered adipokine and EV secretions (Figure 3) (Aye et al., 2014; Myatt and Maloyan, 2016; Salomon et al., 2016; Howell and Powell, 2017). Additionally, hyperglycemia in gestational diabetes induces inflammation of the fetoplacental vasculature (Rice et al., 2015; Salomon et al., 2016; Elfeky et al., 2017; Echeverria et al., 2020).
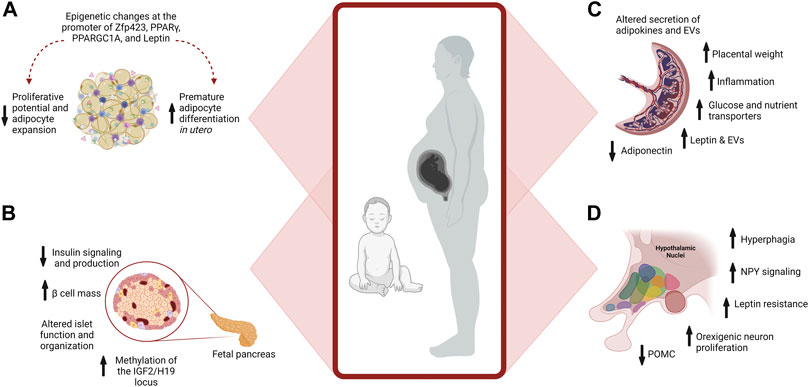
FIGURE 3. Maternal Obesity Alters Placenta, Adipocyte, and β cell Development. (A) Maternal obesity promotes premature differentiation of adipocytes, which depletes progenitors available for expansion in adulthood when excess energy storage may be needed. Epigenetic changes at the promoter of multiple genes further affect developmental plasticity. (B) Pancreatic development is influenced by maternal obesity. Exposure to hyperinsulinemia during fetal development increases Islet size and β cell mass, reducing insulin signaling and production. Epigenetic changes also occur, such as increased methylation of IGF2 in the pancreas, influencing growth and function. (C) In the placenta, maternal obesity alters the placental secretion of adipokines and EVs, increases inflammation, and placental weight, and modifies nutrient transport to the developing fetus. (D) Overfeeding postnatally alters hypothalamic circuits and food intake.
In a vicious cycle, placental dysfunction in obesity and gestational diabetes paradoxically leads to increased expression of placental glucose transporters, further increasing glucose uptake (Salomon et al., 2016; Plows et al., 2018). Increased insulin resistance affects early placental growth, altering gene expression, including genes that regulate lipid metabolism and mitochondrial activity (Parrettini et al., 2020). Gestational diabetes alters the expression of nutrient transporters in the placenta bringing excess nutrients to the developing fetus and promoting excessive fetal growth (Jansson et al., 2002, 2013; Castillo-Castrejon and Powell, 2017). Adipokines also regulate the expression of nutrient transporters in the placenta, and altered adipokine expression in obesity further contributes to placental dysfunction (Howell and Powell, 2017; Jayabalan et al., 2017; Corrales et al., 2021). Leptin increases nutrient availability to the fetus by stimulating the placental amino acid transport system promoting overnutrition of the fetus through increased transport of nutrients (Jansson et al., 2002, 2003; Von Versen-Höynck et al., 2009). Conversely, lower adiponectin levels in pregnancies with obesity upregulates glucose and amino acid transporters, resulting in increased transport of nutrient delivery by the placenta to further stimulate fetal growth (Jones et al., 2010; Rosario et al., 2012; Aye et al., 2013; Duval et al., 2016).
Maternal obesity alters adipocyte development in the offspring
Maternal obesity alters the developmental plasticity, distribution, and composition of adipose tissue in the offspring, predisposing them to adiposopathy in adulthood (Lecoutre and Breton, 2015; Lecoutre et al., 2021). Adipocytes are derived from multipotent mesenchymal stem cells, which become committed to the adipocyte lineage as pre-adipocytes. This commitment is influenced by a series of coordinated events that involve transcription factors, cofactors, and numerous signaling pathways (Rosen and MacDougald, 2006). Pre-adipocytes undergo clonal expansion and differentiate into functional adipocytes under the influence of leptin and adiponectin (Shin et al., 2020). These events occur between the 14th to 24th weeks of gestation in humans and mid-gestation to birth in rodents (Poissonnet et al., 1984; Lecoutre et al., 2018). Adipocyte numbers are typically established during childhood and adolescence but can expand if needed to store excess energy (Spalding et al., 2008). However, this is probably not absolute, since pre-adipocytes from elderly humans were found to differentiate in vitro (Hauner et al., 1989).
Our understanding of adipocyte development comes from both in vitro and animal studies and data on depot-specific aspects of differentiation are limited (Rosen and MacDougald, 2006). As such, much is still unknown about the precise developmental origins of fetal fat depots. It is proposed that subcutaneous and visceral adipocytes are specified at different stages of development, with subcutaneous fat specified in the fetus and visceral fat in the neonate (Desoye and Herrera, 2021). Furthermore, it is suggested that subcutaneous and visceral depots arise from distinct lineages (Ying and Simmons, 2021).
One-way maternal obesity influences fetal adipocyte development is by promoting premature differentiation of pre-adipocytes, depleting progenitors available for expansion in adulthood (Lecoutre et al., 2021). Depletion of progenitors alters the potential of the adipocyte lineage to expand in adulthood when excess energy storage may be needed (Berry et al., 2016). Reduced proliferative potential of adipocyte progenitors results in pathogenic expansion of adipocytes (Lecoutre et al., 2021) (Figure 3). Late gestation and lactation are critical times for expanding adipocytes and are periods when developmental programming is highly sensitive to overnutrition (Srinivasan and Patel, 2008).
Epigenetic alterations influence adipocyte remodeling in the offspring of mothers with obesity, and the persistence of these marks is a significant contributor to the long-term consequences of overnutrition during pregnancy (Lecoutre et al., 2021). For instance, increased leptin levels in the offspring of rats fed a high-fat diet during pregnancy are associated with the persistent epigenetic remodeling at vital regulatory regions of the leptin gene in adipocytes (Lecoutre et al., 2016, 2017). In humans, increased methylation of specific epigenetic sites in cord blood is observed in offspring of mothers with obesity and is correlated with adiposity in the offspring (Sharp et al., 2015). In rodents, maternal obesity alters expression via epigenetic changes at the promoters of key transcription factors involved in adipogenesis, including Zfp423, PPARγ, and PPARGC1A (Gemma et al., 2009; Borengasser et al., 2013; Yang et al., 2013; Lecoutre et al., 2018). Altered expression of microRNAs is another epigenetic modification that impacts adipose tissue metabolism in offspring of pregnancies with obesity. For instance, overexpression of miR-126 in adipocytes of male mice offspring targets the downregulation of insulin receptor substrate-1 (IRS-1), modulating insulin signaling (Figure 3) (Fernandez-Twinn et al., 2014; De Almeida-Faria et al., 2021). These epigenetic changes promote premature differentiation, attenuating the expansion of adipocytes and favoring metabolically adverse modes of expansion in adulthood.
Obesity alters pancreatic β cells development in the offspring
Changes in the developmental plasticity of insulin-producing pancreatic β cells are another critical long-term maladaptation in response to maternal obesity (Lecoutre et al., 2021). Insulin is produced from β cells of the pancreas in response to increased blood glucose levels and facilitates glucose uptake into cells. This feedback loop is essential for maintaining blood glucose levels in a healthy range.
Islets of the pancreas have a complex architecture and are composed of four cell types (α, β, D and F cells). α cells secrete glucagon, and β cells secrete insulin. β cells are highly susceptible to the deleterious effects of maternal obesity and gestational diabetes (Lecoutre et al., 2021). The development of β cells of the pancreas is best characterized in rodent models. The differentiation phase of β cell development occurs in the mouse embryo between embryonic days (E) E9.5 and E12.5 with the specification of multipotent endocrine progenitor-precursors from the embryonic endoderm (O’Dowd and Stocker, 2013). From E12.5 to birth, these progenitors differentiate into various endocrine cells, including β cells. The maturation phase occurs postnatally when β cells are immature, plastic, and actively proliferate until weaning when they mature and produce insulin (Lecoutre et al., 2021).
Differentiation and maturation phases of β cell development are most susceptible to the deleterious effects of maternal obesity and gestational diabetes (Lecoutre et al., 2021). Maternal obesity alters β cell size, function, and organization within the islets of the offspring (Figure 3). These changes are influenced by the sex, timing, and degree of glucose dysregulation. Pancreatic islet cells are arranged in a highly stereotypical fashion essential for their function. In rodent models of maternal obesity and gestational diabetes, changes in β cell development and organization are found in the offspring that alter insulin production and glucose regulation into adulthood (Lecoutre et al., 2021). Similarly, in humans, islet hypertrophy and hyperplasia of β cells are found in newborns from mothers with gestational diabetes (Holemans et al., 2003).
Offspring from mice fed an obesogenic diet with high sugar and fat levels throughout gestation and lactation exhibited elevated fasting glucose and insulin resistance (Samuelsson et al., 2008). In another study, exposure to a high-fat diet during gestation but not lactation resulted in hyperinsulinemia and increased β cell mass in male offspring (Gregorio et al., 2013). In contrast, maternal obesity without diabetes resulted in altered β cell function, increasing the risk of gestational diabetes and type 2 diabetes in the female but not male offspring (Han et al., 2005). Furthermore, in mice, a maternal high-fat diet alters islet structure in the male offspring, impairs the insulin-signaling pathway, increases FOXO1 protein expression, and decreases IRS1, PI3K, p-Akt, Pd-1, and GLUT2 protein expression in the pancreas (Bringhenti et al., 2016). The imprinted IGF2 locus is a critical developmental regulator of pancreas size and function and becomes hypermethylated in the pancreas of offspring from pregnant mice with gestational diabetes (Ding et al., 2012; Hammerle et al., 2020). These examples not only demonstrate the alterations in β cell development of the offspring induced by maternal obesity and the impact on glucose control in the offspring but also highlight the need for consideration of the diet used to induce obesity, the degree of glucose dysregulation, and sex differences of the offspring in rodent studies.
Obesity and altered development of appetite control
Maternal obesity and overfeeding postnatally have long-term consequences on appetite control in the offspring. Appetite is regulated centrally through a complex circuit involving the Hypothalamic-Pituitary-Adrenal Axis (HPA axis; Figure 4). The hypothalamus is the main integrator and processor of peripheral metabolic information. Hypothalamic nuclei control feeding and energy expenditure by the release of orexigenic (appetite-stimulating; neuropeptide Y [NPY], agouti-related protein [AgRP]) and anorexigenic (appetite inhibiting; pro-opiomelanocortin [POMC] and cocaine- and amphetamine-regulated transcript [CART]) peptides (Ross and Desai, 2014). There are several hypothalamic nuclei described in Figure 4, relevant to appetite control.
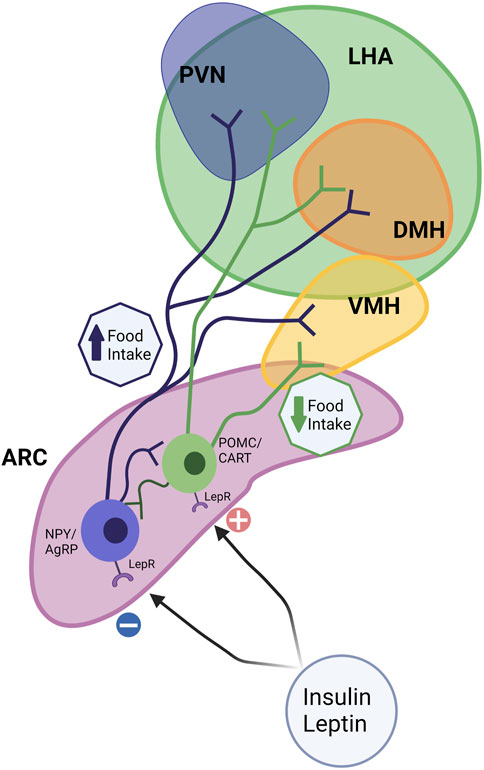
FIGURE 4. Hypothalamic circuits and Food Intake. The hypothalamus is considered the main integrator and processor of peripheral metabolic information. Among several hypothalamic nuclei such as the arcuate nucleus (ARC), paraventricular nucleus (PVN), the dorsomedial hypothalamus (DMH), ventromedial hypothalamus (VMH), and the lateral hypothalamic area (LHA), ARC and VMH are the predominant sites for the integration of signals. Hypothalamic nuclei control feeding and energy expenditure by the release of orexigenic (appetite-stimulating; neuropeptide Y [NPY] and agouti-related protein [AgRP]) and anorexigenic (appetite inhibiting; pro-opiomelanocortin [POMC] and cocaine- and amphetamine-regulated transcript [CART]) peptides. The ARC contains anorexigenic and orexigenic neurons, POMC/CART, and NPY/AgRP, which project extensively to other hypothalamic nuclei. Leptin and other signals upregulate anorexigenic neurons (POMC/CART) and reduce food intake. NPY neurons (orexigenic) are sensitive to peripheral nutrient and hormone fluctuations, such as insulin, and leptin, which promote food intake.
Together these nuclei form an integrated circuit (Figure 4) that regulates food intake and appetite, integrating with peripheral signals such as leptin and insulin to control appetite (for review, see McMillen et al., 2005). ARC can be considered a predominant site for the integration of signals. The ARC contains both anorexigenic (POMC/CART) and orexigenic (NPY/AgRP) neurons that project extensively to other critical hypothalamic nuclei, such as the PVN, DMH, and LHA (McMillen et al., 2005). αMSH, a POMC-derived peptide, acts on the melanocortin receptor to suppress food intake. In addition, leptin upregulates POMC expression and therefore limits energy intake. The neuropeptide CART, co-expressed by POMC neurons, also inhibits food intake (Schwartz, 2001). NPY neurons are sensitive to peripheral nutrient and hormone fluctuations, such as glucose, insulin, and leptin (Gali Ramamoorthy et al., 2015). The ARC also expresses AgRP, an endogenous antagonist of the anorexigenic receptors in the PVN and other hypothalamic regions (Woods et al., 1998) (Figure 4).
The development of orexigenic and anorexigenic hypothalamic neurons starts during fetal life and continues its growth and remodeling in the neonatal period (Kagotani et al., 1989). In rodents, POMC becomes detectable at gestational day E12.5, and NPY neurons first appear in the ARC at E14.5 (Kagotani et al., 1989). Neuronal postnatal development is different between rodents, humans, and nonhuman primates, as hypothalamic neural projections develop almost entirely during fetal life in primates (Koutcherov et al., 2002). After hypothalamic neuroprogenitor cells (NPCs) differentiate into neurons, those cells differentiate to specific neuronal phenotypes: orexigenic (NPY) or anorexigenic (POMC), for example. NPCs proliferation and differentiation processes are regulated by a series of neuroregulatory basic helix-loop-helix (bHLH) transcription factors, including Hes1, Neurogenin (Ngn3), and Mash1 (Kageyama et al., 2008; Desai et al., 2020). Insulin and leptin regulate adult appetite behavior, but they also have important roles in appetite neurogenesis. For example, neurons treated with insulin exhibit cellular growth, and axonal growth was stimulated by insulin in a rat fetal brain cell culture. Whereas leptin-deficient or -insensitive animals displayed decreased brain size and development (Ross and Desai, 2014).
The timing of terminal differentiation defines a critical period during lactation for appetite regulation. In this context, male offspring from mice fed an HFD during pregnancy and lactation showed reduced hypothalamic bHLH factors, pAMPK/AMPK, and POMC while increased AgRP with NPCs showing preferential differentiation towards NPY (Desai et al., 2020). Feeding rats an obesogenic diet before mating until weaning induces long-term hyperphagia in the offspring (Samuelsson et al., 2008). Further studies define the perinatal period as critical, where high-fat diet exposure increases embryonic hypothalamic orexigenic cell proliferation in the PVN and LHA (Chang et al., 2008). Moreover, a high-fat diet during the perinatal period was also associated with reduced hypothalamic POMC neurons in postnatal age p1 and 12 months old male mice (Desai et al., 2020). In rodents, maternal obesity increases post-weaning high-fat diet-induced hyperphagia, hypothalamic NPY signaling, and leptin resistance in the offspring through adulthood (Chen et al., 2009). Rodent studies demonstrate that reducing litter size during lactation stimulates overfeeding and alters hypothalamic circuits to promote hyperphagia, predisposing offspring to increased body weight later in life (Patel and Srinivasan, 2011). Additionally, feeding rat pups milk from dams with gestational diabetes increased NPY and decreased POMC neurons in the ARC contributing to hyperphagia and later-onset metabolic syndrome (Fahrenkrog et al., 2004). Together these studies highlight the influence of overfeeding during late gestation/lactation on developing hypothalamic circuits and long-term appetite regulation.
Summary and future directions
With the growing obesity epidemic, the number of pregnancies affected is increasing, and so is the risk of metabolic disease in the offspring. Understanding how parental obesity alters metabolic programs in the progeny, predisposing them to adult-onset metabolic syndrome, is key to designing targeted interventions to break this cycle. This review synthesizes a broad literature on this topic to explore how parental obesity alters the development of the gametes, placenta, adipocytes, pancreatic β cells, and hypothalamic circuits that control feeding. We identify critical stages where parental obesity alters developmental programming (Figure 5). While not examined in isolation in all studies, select experiments in animal models demonstrate that exposure to parental obesity at distinct developmental phases is sufficient to alter certain aspects of the metabolism of the offspring. In humans, multiple stages of development are typically impacted, adding insult to injury to compound long-term changes in offspring metabolism.
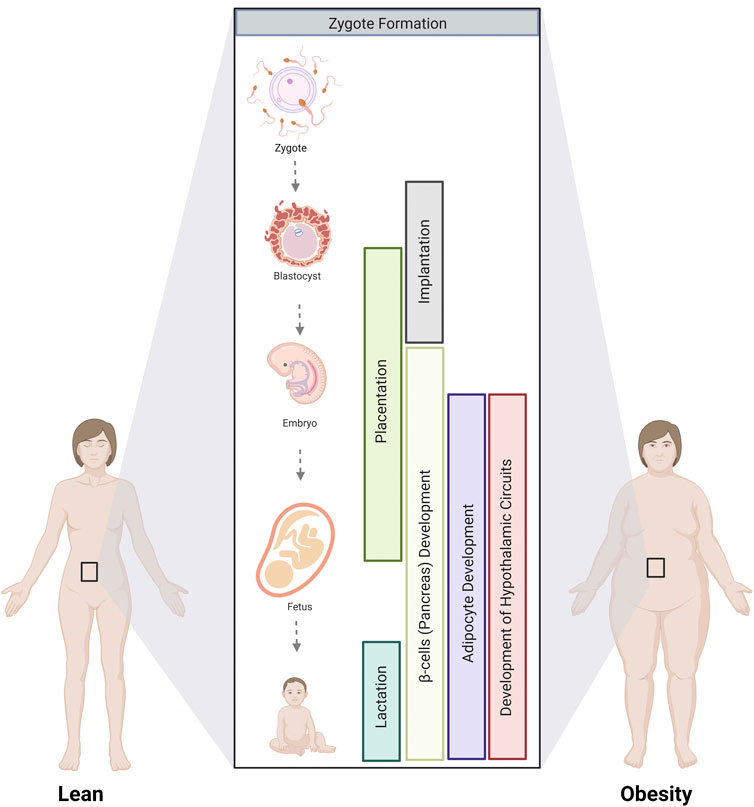
FIGURE 5. Critical stages where parental obesity influences developmental programming. The development of multiple tissues and organs involved in metabolism is altered by parental obesity from gamete formation until lactation. Critical stages involve gamete formation, placentation, and the development of adipocytes, the pancreas, and the hypothalamic circuits that control appetite that occurs during fetal and postnatal development.
Insulin resistance and glucose control are critical targets for intervention. In rodents, altering the carbohydrate and lipid composition of the maternal diet or increasing physical activity during pregnancy improves insulin resistance and glucose control in both mothers and their offspring (Vega et al., 2015; Gimpfl et al., 2017; Ramalingam et al., 2018; Rodrigo et al., 2022). Changes are most efficacious when started before pregnancy and continued through gestation (Stanford et al., 2015; Stanford et al., 2017; Harris et al., 2018; Summerfield et al., 2018; Kusuyama et al., 2020; Smits et al., 2021; Rodrigo et al., 2022). Humans are not always compliant with these changes, making modifying diet and exercise during pregnancy less successful (Dodd et al., 2014; Gimpfl et al., 2017; Rodrigo et al., 2022). One mechanism by which exercise could improve outcomes is by increasing circulating adiponectin, which affects the metabolism of the mother and the offspring by influencing placental vascularization, nutrient transport and fetal growth (Jones et al., 2010; Kelly et al., 2020; Mangwiro et al., 2018a; 2018b; Rosario et al., 2012; Son et al., 2019).
Adiponectin levels are reduced in pregnancies with obesity, and adiponectin supplementation may be an effective strategy to mitigate intergenerational effects. Supplementing pregnant dams with adiponectin counters the adverse effects of maternal obesity on placental function and fetal growth, ameliorating long-term metabolic syndrome in the offspring (Aye et al., 2015). Furthermore, adiponectin supplementation during gestation improves insulin sensitivity and reduces weight gain in the offspring of prenatal androgenized female adult offspring (Wu et al., 2021). Another study showed that administering adiponectin receptor agonist (AdipoRon) to pregnant diabetic rats improved insulin control in the mothers and long-term glucose tolerance in the offspring (Gázquez et al., 2021).
Another theme that emerges is the role of epigenetic changes in multiple organs that are essential for transmitting the long-term influence of parental obesity on the metabolism of the offspring. Obesity is associated with various micronutrient deficiencies, which are an attractive actionable target to alleviate some of these impacts of obesity. For instance, micronutrients involved in the one-carbon cycle generate the methyl groups that mediate epigenetic changes may be an actionable target. Supplementation with folic acid, Vitamin B12, and Vitamin D are promising strategies for reverting epigenetic modifications. Finally, postnatally, significant developmental programming of adipocytes, β cells, and hypothalamic circuits occurs. Targeted strategies delivered postnatally may prevent these changes from happening or even reverse some of the damage done in utero.
Author contributions
Conceptualization, LC, RB, RF, and IZ; Writing and literature review, LC, RB, and IZ; Critical revision of the manuscript, LC, RB, and IZ; Figures preparation, LC. All authors have read, edited, and approved the article’s final version.
Funding
This work is supported by a Collaborative Research Pilot Award from the Center for Genetic Medicine Research of the Children’s Research Institute (PI: RB and IZ), R01HD098861 from NICHD (PI: IZ), and the District of Columbia Intellectual and Developmental Disabilities Research Center (DC-IDDRC) Award P50HD105328 from NICHD (PI: V. Gallo).
Acknowledgments
The figures in this review were created with BioRender.com.
Conflict of interest
The authors declare that the research was conducted in the absence of any commercial or financial relationships that could be construed as a potential conflict of interest.
Publisher’s note
All claims expressed in this article are solely those of the authors and do not necessarily represent those of their affiliated organizations, or those of the publisher, the editors and the reviewers. Any product that may be evaluated in this article, or claim that may be made by its manufacturer, is not guaranteed or endorsed by the publisher.
References
Adaikalakoteswari, A., Vatish, M., Alam, M. T., Ott, S., Kumar, S., and Saravanan, P. (2017). Low vitamin B12 in pregnancy is associated with adipose-derived circulating miRs targeting PPARγ and insulin resistance. J. Clin. Endocrinol. Metab. 102, 4200–4209. doi:10.1210/jc.2017-01155
Akbar, N., Azzimato, V., Choudhury, R. P., and Aouadi, M. (2019). Extracellular vesicles in metabolic disease. Diabetologia 62, 2179–2187. doi:10.1007/s00125-019-05014-5
Akhaphong, B., Gregg, B., Kumusoglu, D., Jo, S., Singer, K., Scheys, J., et al. (2021). Maternal high-fat diet during pre-conception and gestation predisposes adult female offspring to metabolic dysfunction in mice. Front. Endocrinol. (Lausanne) 12, 780300. doi:10.3389/fendo.2021.780300
Alcalá, M., Calderon-Dominguez, M., Serra, D., Herrero, L., and Viana, M. (2019). Mechanisms of impaired Brown adipose tissue recruitment in obesity. Front. Physiol. 10, 94. doi:10.3389/fphys.2019.00094
Anwer, H., Morris, M. J., Noble, D. W. A., Nakagawa, S., and Lagisz, M. (2022). Transgenerational effects of obesogenic diets in rodents: A meta-analysis. Obes. Rev. 23, e13342. doi:10.1111/obr.13342
Augustine, R. A., Ladyman, S. R., and Grattan, D. R. (2008). From feeding one to feeding many: Hormone-induced changes in bodyweight homeostasis during pregnancy. J. Physiol. 586, 387–397. doi:10.1113/jphysiol.2007.146316
Aye, I. L. M. H., Lager, S., Ramirez, V. I., Gaccioli, F., Dudley, D. J., Jansson, T., et al. (2014). Increasing maternal body mass index is associated with systemic inflammation in the mother and the activation of distinct placental inflammatory pathways. Biol. Reprod. 90, 129. doi:10.1095/biolreprod.113.116186
Aye, I. L. M. H., Powell, T. L., and Jansson, T. (2013). Review: Adiponectin--the missing link between maternal adiposity, placental transport and fetal growth? Placenta 34 (1), S40–S45. doi:10.1016/j.placenta.2012.11.024
Aye, I. L. M. H., Rosario, F. J., Powell, T. L., and Jansson, T. (2015). Adiponectin supplementation in pregnant mice prevents the adverse effects of maternal obesity on placental function and fetal growth. Proc. Natl. Acad. Sci. U. S. A. 112, 12858–12863. doi:10.1073/pnas.1515484112
Bai, K., Li, X., Zhong, J., Ng, E. H. Y., Yeung, W. S. B., Lee, C.-L., et al. (2021). Placenta-derived exosomes as a modulator in maternal immune tolerance during pregnancy. Front. Immunol. 12, 671093. doi:10.3389/fimmu.2021.671093
Banerjee, R. R. (2018). Piecing together the puzzle of pancreatic islet adaptation in pregnancy. Ann. N. Y. Acad. Sci. 1411, 120–139. doi:10.1111/nyas.13552
Barberio, M. D., Kasselman, L. J., Playford, M. P., Epstein, S. B., Renna, H. A., Goldberg, M., et al. (2019). Cholesterol efflux alterations in adolescent obesity: Role of adipose-derived extracellular vesical microRNAs. J. Transl. Med. 17, 232. doi:10.1186/s12967-019-1980-6
Barker, D. J., Bull, A. R., Osmond, C., and Simmonds, S. J. (1990). Fetal and placental size and risk of hypertension in adult life. BMJ 301, 259–262.
Barouki, R., Gluckman, P. D., Grandjean, P., Hanson, M., and Heindel, J. J. (2012). Developmental origins of non-communicable disease: Implications for research and public health. Environ. Health 11, 42. doi:10.1186/1476-069X-11-42
Bays, H. E. (2011). Adiposopathy: Is “sick fat” a cardiovascular disease? J. Am. Coll. Cardiol. 57, 2461–2473. doi:10.1016/j.jacc.2011.02.038
Berry, D. C., Jiang, Y., and Graff, J. M. (2016). Emerging roles of adipose progenitor cells in tissue development, homeostasis, expansion and thermogenesis. Trends Endocrinol. Metab. 27, 574–585. doi:10.1016/j.tem.2016.05.001
Bhowmik, B., Siddique, T., Majumder, A., Mdala, I., Hossain, I. A., Hassan, Z., et al. (2019). Maternal BMI and nutritional status in early pregnancy and its impact on neonatal outcomes at birth in Bangladesh. BMC Pregnancy Childbirth 19, 413. doi:10.1186/s12884-019-2571-5
Binder, N. K., Beard, S. A., Kaitu’u-Lino, T. J., Tong, S., Hannan, N. J., and Gardner, D. K. (2015). Paternal obesity in a rodent model affects placental gene expression in a sex-specific manner. Reproduction 149, 435–444. doi:10.1530/REP-14-0676
Binder, N. K., Hannan, N. J., and Gardner, D. K. (2012). Paternal diet-induced obesity retards early mouse embryo development, mitochondrial activity and pregnancy health. PLoS One 7, e52304. doi:10.1371/journal.pone.0052304
Booth, A., Magnuson, A., Fouts, J., and Foster, M. T. (2016). Adipose tissue: An endocrine organ playing a role in metabolic regulation. Horm. Mol. Biol. Clin. Investig. 26, 25–42. doi:10.1515/hmbci-2015-0073
Borengasser, S. J., Zhong, Y., Kang, P., Lindsey, F., Ronis, M. J. J., Badger, T. M., et al. (2013). Maternal obesity enhances white adipose tissue differentiation and alters genome-scale DNA methylation in male rat offspring. Endocrinology 154, 4113–4125. doi:10.1210/en.2012-2255
Borga, M., West, J., Bell, J. D., Harvey, N. C., Romu, T., Heymsfield, S. B., et al. (2018). Advanced body composition assessment: From body mass index to body composition profiling. J. Investigative Med. 66, 1–9. doi:10.1136/jim-2018-000722
Briffa, J. F., McAinch, A. J., Romano, T., Wlodek, M. E., and Hryciw, D. H. (2015). Leptin in pregnancy and development: A contributor to adulthood disease? Am. J. Physiol. Endocrinol. Metab. 308, E335–E350. doi:10.1152/ajpendo.00312.2014
Bringhenti, I., Ornellas, F., Mandarim-de-Lacerda, C. A., and Aguila, M. B. (2016). The insulin-signaling pathway of the pancreatic islet is impaired in adult mice offspring of mothers fed a high-fat diet. Nutrition 32, 1138–1143. doi:10.1016/j.nut.2016.03.001
Butler, M. G. (2009). Genomic imprinting disorders in humans: A mini-review. J. Assist. Reprod. Genet. 26, 477–486. doi:10.1007/s10815-009-9353-3
Camino, T., Lago-Baameiro, N., Martis-Sueiro, A., Couto, I., Santos, F., Baltar, J., et al. (2020). Deciphering adipose tissue extracellular vesicles protein cargo and its role in obesity. Int. J. Mol. Sci. 21, E9366. doi:10.3390/ijms21249366
Carone, B. R., Fauquier, L., Habib, N., Shea, J. M., Hart, C. E., Li, R., et al. (2010). Paternally induced transgenerational environmental reprogramming of metabolic gene expression in mammals. Cell 143, 1084–1096. doi:10.1016/j.cell.2010.12.008
Castillo-Castrejon, M., and Powell, T. L. (2017). Placental nutrient transport in gestational diabetic pregnancies. Front. Endocrinol. (Lausanne) 8, 306. doi:10.3389/fendo.2017.00306
Catalano, P. M., Hoegh, M., Minium, J., Huston-Presley, L., Bernard, S., Kalhan, S., et al. (2006). Adiponectin in human pregnancy: Implications for regulation of glucose and lipid metabolism. Diabetologia 49, 1677–1685. doi:10.1007/s00125-006-0264-x
Ceelen, M., van Weissenbruch, M. M., Vermeiden, J. P. W., van Leeuwen, F. E., and Delemarre-van de Waal, H. A. (2008). Cardiometabolic differences in children born after in vitro fertilization: Follow-up study. J. Clin. Endocrinol. Metab. 93, 1682–1688. doi:10.1210/jc.2007-2432
Chait, A., and den Hartigh, L. J. (2020). Adipose tissue distribution, inflammation and its metabolic consequences, including diabetes and cardiovascular disease. Front. Cardiovasc Med. 7, 22. doi:10.3389/fcvm.2020.00022
Chang, G.-Q., Gaysinskaya, V., Karatayev, O., and Leibowitz, S. F. (2008). Maternal high-fat diet and fetal programming: Increased proliferation of hypothalamic peptide-producing neurons that increase risk for overeating and obesity. J. Neurosci. 28, 12107–12119. doi:10.1523/JNEUROSCI.2642-08.2008
Chang, R., Mei, H., Zhang, Y., Xu, K., Yang, S., and Zhang, J. (2021). Early childhood body mass index trajectory and overweight/obesity risk differed by maternal weight status. Eur. J. Clin. Nutr. 76 (3), 450–455. doi:10.1038/s41430-021-00975-6
Chen, H., Simar, D., and Morris, M. J. (2009). Hypothalamic neuroendocrine circuitry is programmed by maternal obesity: Interaction with postnatal nutritional environment. PLoS One 4, e6259. doi:10.1371/journal.pone.0006259
Christian, L. M., and Porter, K. (2014). Longitudinal changes in serum proinflammatory markers across pregnancy and postpartum: Effects of maternal body mass index. Cytokine 70, 134–140. doi:10.1016/j.cyto.2014.06.018
Condrat, C. E., Varlas, V. N., Duică, F., Antoniadis, P., Danila, C. A., Cretoiu, D., et al. (2021). Pregnancy-related extracellular vesicles revisited. Int. J. Mol. Sci. 22, 3904. doi:10.3390/ijms22083904
Constância, M., Angiolini, E., Sandovici, I., Smith, P., Smith, R., Kelsey, G., et al. (2005). Adaptation of nutrient supply to fetal demand in the mouse involves interaction between the Igf2 gene and placental transporter systems. Proc. Natl. Acad. Sci. U. S. A. 102, 19219–19224. doi:10.1073/pnas.0504468103
Constância, M., Hemberger, M., Hughes, J., Dean, W., Ferguson-Smith, A., Fundele, R., et al. (2002). Placental-specific IGF-II is a major modulator of placental and fetal growth. Nature 417, 945–948. doi:10.1038/nature00819
Corrales, P., Vidal-Puig, A., and Medina-Gómez, G. (2021). Obesity and pregnancy, the perfect metabolic storm. Eur. J. Clin. Nutr. 75, 1723–1734. doi:10.1038/s41430-021-00914-5
Craig, J. R., Jenkins, T. G., Carrell, D. T., and Hotaling, J. M. (2017). Obesity, male infertility, and the sperm epigenome. Fertil. Steril. 107, 848–859. doi:10.1016/j.fertnstert.2017.02.115
Cutland, C. L., Lackritz, E. M., Mallett-Moore, T., Bardají, A., Chandrasekaran, R., Lahariya, C., et al. (2017). Low birth weight: Case definition & guidelines for data collection, analysis, and presentation of maternal immunization safety data. Vaccine 35, 6492–6500. doi:10.1016/j.vaccine.2017.01.049
Czernek, L., and Düchler, M. (2020). Exosomes as messengers between mother and fetus in pregnancy. Int. J. Mol. Sci. 21, E4264. doi:10.3390/ijms21124264
Daraki, V., Roumeliotaki, T., Chalkiadaki, G., Katrinaki, M., Karachaliou, M., Leventakou, V., et al. (2018). Low maternal vitamin D status in pregnancy increases the risk of childhood obesity. Pediatr. Obes. 13, 467–475. doi:10.1111/ijpo.12267
De Almeida-Faria, J., Duque-Guimarães, D. E., Ong, T. P., Pantaleão, L. C., Carpenter, A. A., Loche, E., et al. (2021). Maternal obesity during pregnancy leads to adipose tissue ER stress in mice via miR-126-mediated reduction in Lunapark. Diabetologia 64, 890–902. doi:10.1007/s00125-020-05357-4
De Castro Barbosa, T., Ingerslev, L. R., Alm, P. S., Versteyhe, S., Massart, J., Rasmussen, M., et al. (2016). High-fat diet reprograms the epigenome of rat spermatozoa and transgenerationally affects metabolism of the offspring. Mol. Metab. 5, 184–197. doi:10.1016/j.molmet.2015.12.002
Deng, Z., Poliakov, A., Hardy, R. W., Clements, R., Liu, C., Liu, Y., et al. (2009). Adipose tissue exosome-like vesicles mediate activation of macrophage-induced insulin resistance. Diabetes 58, 2498–2505. doi:10.2337/db09-0216
Desai, M., Ferrini, M. G., Han, G., Narwani, K., and Ross, M. G. (2020). Maternal high fat diet programs male mice offspring hyperphagia and obesity: Mechanism of increased appetite neurons via altered neurogenic factors and nutrient sensor AMPK. Nutrients 12 (11), 3326.
Deshpande, S. S. S., Nemani, H., and Balasinor, N. H. (2021). High fat diet-induced- and genetically inherited- obesity differential alters DNA demethylation pathways in the germline of adult male rats. Reprod. Biol. 21, 100532. doi:10.1016/j.repbio.2021.100532
Desoye, G., and Herrera, E. (2021). Adipose tissue development and lipid metabolism in the human fetus: The 2020 perspective focusing on maternal diabetes and obesity. Prog. Lipid Res. 81, 101082. doi:10.1016/j.plipres.2020.101082
Dilworth, M. R., Kusinski, L. C., Cowley, E., Ward, B. S., Husain, S. M., Constância, M., et al. (2010). Placental-specific Igf2 knockout mice exhibit hypocalcemia and adaptive changes in placental calcium transport. Proc. Natl. Acad. Sci. U. S. A. 107, 3894–3899. doi:10.1073/pnas.0911710107
Ding, G.-L., Wang, F.-F., Shu, J., Tian, S., Jiang, Y., Zhang, D., et al. (2012). Transgenerational glucose intolerance with Igf2/H19 epigenetic alterations in mouse islet induced by intrauterine hyperglycemia. Diabetes 61, 1133–1142. doi:10.2337/db11-1314
Dodd, J. M., Turnbull, D., McPhee, A. J., Deussen, A. R., Grivell, R. M., Yelland, L. N., et al. (2014). Antenatal lifestyle advice for women who are overweight or obese: LIMIT randomised trial. Bmj 348.
Driscoll, A. K. (2020). Increases in prepregnancy obesity: United States, 2016–2019. United States, 8.
Duval, F., Santos, E. D., Poidatz, D., Sérazin, V., Gronier, H., Vialard, F., et al. (2016). Adiponectin inhibits nutrient transporters and promotes apoptosis in human villous cytotrophoblasts: Involvement in the control of fetal growth. Biol. Reprod. 94, 111. doi:10.1095/biolreprod.115.134544
Echeverria, C., Eltit, F., Santibanez, J. F., Gatica, S., Cabello-Verrugio, C., and Simon, F. (2020). Endothelial dysfunction in pregnancy metabolic disorders. Biochim. Biophys. Acta Mol. Basis Dis. 1866, 165414. doi:10.1016/j.bbadis.2019.02.009
Ehrenberg, H. M., Huston-Presley, L., and Catalano, P. M. (2003). The influence of obesity and gestational diabetes mellitus on accretion and the distribution of adipose tissue in pregnancy. Am. J. Obstet. Gynecol. 189, 944–948. doi:10.1067/s0002-9378(03)00761-0
Elfeky, O., Longo, S., Lai, A., Rice, G. E., and Salomon, C. (2017). Influence of maternal BMI on the exosomal profile during gestation and their role on maternal systemic inflammation. Placenta 50, 60–69. doi:10.1016/j.placenta.2016.12.020
Fahrenkrog, S., Harder, T., Stolaczyk, E., Melchior, K., Franke, K., Dudenhausen, J. W., et al. (2004). Cross-fostering to diabetic rat dams affects early development of mediobasal hypothalamic nuclei regulating food intake, body weight, and metabolism. J. Nutr. 134, 648–654. doi:10.1093/jn/134.3.648
Fall, C. H. (2013). Fetal malnutrition and long-term outcomes. Nestle Nutr. Inst. Workshop Ser. 74, 11–25. doi:10.1159/000348384
Felisbino-Mendes, M. S., Matozinhos, F. P., Miranda, J. J., Villamor, E., and Velasquez-Melendez, G. (2014). Maternal obesity and fetal deaths: Results from the Brazilian cross-sectional demographic health survey, 2006. BMC Pregnancy Childbirth 14, 5. doi:10.1186/1471-2393-14-5
Fernandez-Twinn, D. S., Alfaradhi, M. Z., Martin-Gronert, M. S., Duque-Guimaraes, D. E., Piekarz, A., Ferland-McCollough, D., et al. (2014). Downregulation of IRS-1 in adipose tissue of offspring of obese mice is programmed cell-autonomously through post-transcriptional mechanisms. Mol. Metab. 3, 325–333. doi:10.1016/j.molmet.2014.01.007
Ferrante, S. C., Nadler, E. P., Pillai, D. K., Hubal, M. J., Wang, Z., Wang, J. M., et al. (2015). Adipocyte-derived exosomal miRNAs: A novel mechanism for obesity-related disease. Pediatr. Res. 77, 447–454. doi:10.1038/pr.2014.202
Fitzsimons, K. J., Modder, J., and Greer, I. A. (2009). Obesity in pregnancy: Risks and management. Obstet. Med. 2, 52–62. doi:10.1258/om.2009.090009
Fleming, T. P., Watkins, A. J., Velazquez, M. A., Mathers, J. C., Prentice, A. M., Stephenson, J., et al. (2018). Origins of lifetime health around the time of conception: Causes and consequences. Lancet 391, 1842–1852. doi:10.1016/S0140-6736(18)30312-X
Friedman, J. E. (2018). Developmental programming of obesity and diabetes in mouse, monkey, and man in 2018: Where are we headed? Diabetes 67 (11), 2137–2151.
Frühbeck, G., Catalán, V., Rodríguez, A., Ramírez, B., Becerril, S., Salvador, J., et al. (2019). Adiponectin-leptin ratio is a functional biomarker of adipose tissue inflammation. Nutrients 11, 454. doi:10.3390/nu11020454
Fu, Y., Luo, N., Klein, R. L., and Garvey, W. T. (2005). Adiponectin promotes adipocyte differentiation, insulin sensitivity, and lipid accumulation. J. Lipid Res. 46, 1369–1379. doi:10.1194/jlr.M400373-JLR200
Funcke, J.-B., and Scherer, P. E. (2019). Beyond adiponectin and leptin: Adipose tissue-derived mediators of inter-organ communication. J. Lipid Res. 60, 1648–1684. doi:10.1194/jlr.R094060
Gali Ramamoorthy, T., Begum, G., Harno, E., and White, A. (2015). Developmental programming of hypothalamic neuronal circuits: Impact on energy balance control. Front. Neurosci. 9, 00126. doi:10.3389/fnins.2015.00126
Gallo, L. A., Barrett, H. L., and Dekker Nitert, M. (2017). Review: Placental transport and metabolism of energy substrates in maternal obesity and diabetes. Placenta 54, 59–67. doi:10.1016/j.placenta.2016.12.006
Gázquez, A., Rodríguez, F., Sánchez-Campillo, M., Martínez-Gascón, L. E., Arnao, M. B., Saura-Garre, P., et al. (2021). Adiponectin agonist treatment in diabetic pregnant rats. J. Endocrinol. 251 (1), 1–13.
Gemma, C., Sookoian, S., Alvariñas, J., García, S. I., Quintana, L., Kanevsky, D., et al. (2009). Maternal pregestational BMI is associated with methylation of the PPARGC1A promoter in newborns. Obesity 17, 1032–1039. doi:10.1038/oby.2008.605
Gillet, V., Ouellet, A., Stepanov, Y., Rodosthenous, R. S., Croft, E. K., Brennan, K., et al. (2019). miRNA profiles in extracellular vesicles from serum early in pregnancies complicated by gestational diabetes mellitus. J. Clin. Endocrinol. Metab. 104, 5157–5169. doi:10.1210/jc.2018-02693
Gimpfl, M., Rozman, J., Dahlhoff, M., Kübeck, R., Blutke, A., Rathkolb, B., et al. (2017). Modification of the fatty acid composition of an obesogenic diet improves the maternal and placental metabolic environment in obese pregnant mice. Biochimica Biophysica Acta (BBA)-Molecular Basis Dis. 1863 (6), 1605–1614.
Gkourogianni, A., Kosteria, I., Telonis, A. G., Margeli, A., Mantzou, E., Konsta, M., et al. (2014). Plasma metabolomic profiling suggests early indications for predisposition to latent insulin resistance in children conceived by ICSI. PLoS One 9, e94001. doi:10.1371/journal.pone.0094001
Glazier, J. D., Cetin, I., Perugino, G., Ronzoni, S., Grey, A. M., Mahendran, D., et al. (1997). Association between the activity of the system A amino acid transporter in the microvillous plasma membrane of the human placenta and severity of fetal compromise in intrauterine growth restriction. Pediatr. Res. 42, 514–519. doi:10.1203/00006450-199710000-00016
Godfrey, K. M., Gluckman, P. D., and Hanson, M. A. (2010). Developmental origins of metabolic disease: Life course and intergenerational perspectives. Trends Endocrinol. Metab. 21, 199–205. doi:10.1016/j.tem.2009.12.008
Godfrey, K. M., Reynolds, R. M., Prescott, S. L., Nyirenda, M., Jaddoe, V. W. V., Eriksson, J. G., et al. (2017). Influence of maternal obesity on the long-term health of offspring. Lancet Diabetes Endocrinol. 5, 53–64. doi:10.1016/S2213-8587(16)30107-3
Gregorio, B. M., Souza-Mello, V., Mandarim-de-Lacerda, C. A., and Aguila, M. B. (2013). Maternal high-fat diet is associated with altered pancreatic remodelling in mice offspring. Eur. J. Nutr. 52, 759–769. doi:10.1007/s00394-012-0382-9
Haghiac, M., Basu, S., Presley, L., Serre, D., Catalano, P. M., and Hauguel-de Mouzon, S. (2014). Patterns of adiponectin expression in term pregnancy: Impact of obesity. J. Clin. Endocrinol. Metab. 99, 3427–3434. doi:10.1210/jc.2013-4074
Hammerle, C. M., Sandovici, I., Brierley, G. V., Smith, N. M., Zimmer, W. E., Zvetkova, I., et al. (2020). Mesenchyme-derived IGF2 is a major paracrine regulator of pancreatic growth and function. PLoS Genet. 16, e1009069. doi:10.1371/journal.pgen.1009069
Han, J., Xu, J., Epstein, P. N., and Liu, Y. Q. (2005). Long-term effect of maternal obesity on pancreatic beta cells of offspring: Reduced beta cell adaptation to high glucose and high-fat diet challenges in adult female mouse offspring. Diabetologia 48, 1810–1818. doi:10.1007/s00125-005-1854-8
Hardikar, A. A., Satoor, S. N., Karandikar, M. S., Joglekar, M. V., Puranik, A. S., Wong, W., et al. (2015). Multigenerational undernutrition increases susceptibility to obesity and diabetes that is not reversed after dietary recuperation. Cell Metab. 22, 312–319. doi:10.1016/j.cmet.2015.06.008
Harris, J. E., Baer, L. A., and Stanford, K. I. (2018). Maternal exercise improves the metabolic health of adult offspring. Trends Endocrinol. Metabolism 29 (3), 164–177.
Hauner, H., Entenmann, G., Wabitsch, M., Gaillard, D., Ailhaud, G., Negrel, R., et al. (1989). Promoting effect of glucocorticoids on the differentiation of human adipocyte precursor cells cultured in a chemically defined medium. J. Clin. Invest 84, 1663–1670. doi:10.1172/JCI114345
Hoffstedt, J., Arner, E., Wahrenberg, H., Andersson, D. P., Qvisth, V., Löfgren, P., et al. (2010). Regional impact of adipose tissue morphology on the metabolic profile in morbid obesity. Diabetologia 53, 2496–2503. doi:10.1007/s00125-010-1889-3
Holemans, K., Aerts, L., and Van Assche, F. A. (2003). Lifetime consequences of abnormal fetal pancreatic development. J. Physiol. 547, 11–20. doi:10.1113/jphysiol.2002.036582
Holm, J.-C., Gamborg, M., Ward, L. C., Gammeltoft, S., Kaas-Ibsen, K., Heitmann, B. L., et al. (2011). Tracking of leptin, soluble leptin receptor, and the free leptin index during weight loss and regain in children. Obes. Facts 4, 461–468. doi:10.1159/000335121
Howell, K. R., and Powell, T. L. (2017). Effects of maternal obesity on placental function and fetal development. Reproduction 153, R97–R108. doi:10.1530/REP-16-0495
Huang, Z., and Xu, A. (2021). Adipose extracellular vesicles in intercellular and inter-organ crosstalk in metabolic health and diseases. Front. Immunol. 12, 608680. doi:10.3389/fimmu.2021.608680
Huang-Doran, I., Zhang, C.-Y., and Vidal-Puig, A. (2017). Extracellular vesicles: Novel mediators of cell communication in metabolic disease. Trends Endocrinol. Metab. 28, 3–18. doi:10.1016/j.tem.2016.10.003
Hubal, M. J., Nadler, E. P., Ferrante, S. C., Barberio, M. D., Suh, J.-H., Wang, J., et al. (2017). Circulating adipocyte-derived exosomal MicroRNAs associated with decreased insulin resistance after gastric bypass. Obes. (Silver Spring) 25, 102–110. doi:10.1002/oby.21709
Huynh, J., Dawson, D., Roberts, D., and Bentley-Lewis, R. (2015). A systematic review of placental pathology in maternal diabetes mellitus. Placenta 36, 101–114. doi:10.1016/j.placenta.2014.11.021
Huypens, P., Sass, S., Wu, M., Dyckhoff, D., Tschöp, M., Theis, F., et al. (2016). Epigenetic germline inheritance of diet-induced obesity and insulin resistance. Nat. Genet. 48, 497–499. doi:10.1038/ng.3527
Hyppönen, E., and Boucher, B. J. (2018). Adiposity, vitamin D requirements, and clinical implications for obesity-related metabolic abnormalities. Nutr. Rev. 76, 678–692. doi:10.1093/nutrit/nuy034
Ibrahim, M. M. (2010). Subcutaneous and visceral adipose tissue: Structural and functional differences. Obes. Rev. 11, 11–18. doi:10.1111/j.1467-789X.2009.00623.x
Igosheva, N., Abramov, A. Y., Poston, L., Eckert, J. J., Fleming, T. P., Duchen, M. R., et al. (2010). Maternal diet-induced obesity alters mitochondrial activity and redox status in mouse oocytes and zygotes. PLoS One 5, e10074. doi:10.1371/journal.pone.0010074
Jansson, N., Greenwood, S. L., Johansson, B. R., Powell, T. L., and Jansson, T. (2003). Leptin stimulates the activity of the system A amino acid transporter in human placental villous fragments. J. Clin. Endocrinol. Metab. 88, 1205–1211. doi:10.1210/jc.2002-021332
Jansson, N., Rosario, F. J., Gaccioli, F., Lager, S., Jones, H. N., Roos, S., et al. (2013). Activation of placental mTOR signaling and amino acid transporters in obese women giving birth to large babies. J. Clin. Endocrinol. Metab. 98, 105–113. doi:10.1210/jc.2012-2667
Jansson, T., Ekstrand, Y., Björn, C., Wennergren, M., and Powell, T. L. (2002). Alterations in the activity of placental amino acid transporters in pregnancies complicated by diabetes. Diabetes 51, 2214–2219. doi:10.2337/diabetes.51.7.2214
Jayabalan, N., Lai, A., Nair, S., Guanzon, D., Scholz-Romero, K., Palma, C., et al. (2019a). Quantitative proteomics by SWATH-MS suggest an association between circulating exosomes and maternal metabolic changes in gestational diabetes mellitus. Proteomics 19, e1800164. doi:10.1002/pmic.201800164
Jayabalan, N., Lai, A., Ormazabal, V., Adam, S., Guanzon, D., Palma, C., et al. (2019b). Adipose tissue exosomal proteomic profile reveals a role on placenta glucose metabolism in gestational diabetes mellitus. J. Clin. Endocrinol. Metab. 104, 1735–1752. doi:10.1210/jc.2018-01599
Jayabalan, N., Nair, S., Nuzhat, Z., Rice, G. E., Zuñiga, F. A., Sobrevia, L., et al. (2017). Cross talk between adipose tissue and placenta in obese and gestational diabetes mellitus pregnancies via exosomes. Front. Endocrinol. (Lausanne) 8, 239. doi:10.3389/fendo.2017.00239
Jones, H. N., Jansson, T., and Powell, T. L. (2010). Full-length adiponectin attenuates insulin signaling and inhibits insulin-stimulated amino acid transport in human primary trophoblast cells. Diabetes 59 (5), 1161–1170.
Kageyama, R., Ohtsuka, T., and Kobayashi, T. (2008). Roles of Hes genes in neural development. Dev. growth & Differ. 50, S97–S103.
Kagotani, Y., Hashimoto, T., Tsuruo, Y., Kawano, H., Daikoku, S., and Chihara, K. (1989). Development of the neuronal system containing neuropeptide Y in the rat hypothalamus. Int. J. Dev. Neurosci. 7, 359–374. doi:10.1016/0736-5748(89)90057-9
Kampmann, U., Knorr, S., Fuglsang, J., and Ovesen, P. (2019). Determinants of maternal insulin resistance during pregnancy: An updated overview. J. Diabetes Res. 2019, 5320156. doi:10.1155/2019/5320156
Karatela, R. A., and Sainani, G. S. (2009). Plasma homocysteine in obese, overweight and normal weight hypertensives and normotensives. Indian Heart J. 61, 156–159.
Kaseva, N., Vääräsmäki, M., Matinolli, H.-M., Sipola-Leppänen, M., Tikanmäki, M., Heinonen, K., et al. (2018). Pre-pregnancy overweight or obesity and gestational diabetes as predictors of body composition in offspring twenty years later: Evidence from two birth cohort studies. Int. J. Obes. (Lond) 42, 872–879. doi:10.1038/ijo.2017.277
Kelly, A. C., Powell, T. L., and Jansson, T. (2020). Placental function in maternal obesity. Clin. Sci. (Lond) 134, 961–984. doi:10.1042/CS20190266
Koeck, E. S., Iordanskaia, T., Sevilla, S., Ferrante, S. C., Hubal, M. J., Freishtat, R. J., et al. (2014). Adipocyte exosomes induce transforming growth factor beta pathway dysregulation in hepatocytes: A novel paradigm for obesity-related liver disease. J. Surg. Res. 192, 268–275. doi:10.1016/j.jss.2014.06.050
Koutcherov, Y., Mai, J. K., Ashwell, K. W., and Paxinos, G. (2002). Organization of human hypothalamus in fetal development. J. Comp. Neurology 446 (4), 301–324.
Kumar, R., Mal, K., Razaq, M. K., Magsi, M., Memon, M. K., Memon, S., et al. (2020). Association of leptin with obesity and insulin resistance. Cureus 12, e12178. doi:10.7759/cureus.12178
Kusuyama, J., Alves-Wagner, A. B., Makarewicz, N. S., and Goodyear, L. J. (2020). Effects of maternal and paternal exercise on offspring metabolism. Nat. Metab. 2 (9), 858–872.
La Cava, A. (2017). Leptin in inflammation and autoimmunity. Cytokine 98, 51–58. doi:10.1016/j.cyto.2016.10.011
Laforest, S., Labrecque, J., Michaud, A., Cianflone, K., and Tchernof, A. (2015). Adipocyte size as a determinant of metabolic disease and adipose tissue dysfunction. Crit. Rev. Clin. Lab. Sci. 52, 301–313. doi:10.3109/10408363.2015.1041582
Laitinen, J., Jääskeläinen, A., Hartikainen, A.-L., Sovio, U., Vääräsmäki, M., Pouta, A., et al. (2012). Maternal weight gain during the first half of pregnancy and offspring obesity at 16 years: A prospective cohort study. BJOG 119, 716–723. doi:10.1111/j.1471-0528.2012.03319.x
Landecho, M. F., Tuero, C., Valentí, V., Bilbao, I., de la Higuera, M., and Frühbeck, G. (2019). Relevance of leptin and other adipokines in obesity-associated cardiovascular risk. Nutrients 11, E2664. doi:10.3390/nu11112664
Leary, C., Leese, H. J., and Sturmey, R. G. (2015). Human embryos from overweight and obese women display phenotypic and metabolic abnormalities. Hum. Reprod. 30, 122–132. doi:10.1093/humrep/deu276
Lecoutre, S., and Breton, C. (2015). Maternal nutritional manipulations program adipose tissue dysfunction in offspring. Front. Physiol. 6, 158. doi:10.3389/fphys.2015.00158
Lecoutre, S., Deracinois, B., Laborie, C., Eberlé, D., Guinez, C., Panchenko, P. E., et al. (2016). Depot- and sex-specific effects of maternal obesity in offspring’s adipose tissue. J. Endocrinol. 230, 39–53. doi:10.1530/JOE-16-0037
Lecoutre, S., Maqdasy, S., and Breton, C. (2021). Maternal obesity as a risk factor for developing diabetes in offspring: An epigenetic point of view. World J. Diabetes 12, 366. doi:10.4239/wjd.v12.i4.366
Lecoutre, S., Oger, F., Pourpe, C., Butruille, L., Marousez, L., Dickes-Coopman, A., et al. (2017). Maternal obesity programs increased leptin gene expression in rat male offspring via epigenetic modifications in a depot-specific manner. Mol. Metab. 6, 922–930. doi:10.1016/j.molmet.2017.05.010
Lecoutre, S., Petrus, P., Rydén, M., and Breton, C. (2018). Transgenerational epigenetic mechanisms in adipose tissue development. Trends Endocrinol. Metab. 29, 675–685. doi:10.1016/j.tem.2018.07.004
Li, Y. (2018). Epigenetic mechanisms link maternal diets and gut microbiome to obesity in the offspring. Front. Genet. 9, 342. doi:10.3389/fgene.2018.00342
Liu, J., Fox, C. S., Hickson, D. A., May, W. D., Hairston, K. G., Carr, J. J., et al. (2010). Impact of abdominal visceral and subcutaneous adipose tissue on cardiometabolic risk factors: The jackson heart study. J. Clin. Endocrinol. Metab. 95, 5419–5426. doi:10.1210/jc.2010-1378
Liu, Y., Wang, Y., Wang, C., Shi, R., Zhou, X., Li, Z., et al. (2021). Maternal obesity increases the risk of fetal cardiac dysfunction via visceral adipose tissue derived exosomes. Placenta 105, 85–93. doi:10.1016/j.placenta.2021.01.020
Longo, M., Zatterale, F., Naderi, J., Parrillo, L., Formisano, P., Raciti, G. A., et al. (2019). Adipose tissue dysfunction as determinant of obesity-associated metabolic complications. Int. J. Mol. Sci. 20, E2358. doi:10.3390/ijms20092358
Loos, R. J. F. (2012). Genetic determinants of common obesity and their value in prediction. Best Pract. Res. Clin. Endocrinol. Metabolism 26, 211–226. doi:10.1016/j.beem.2011.11.003
Luzzo, K. M., Wang, Q., Purcell, S. H., Chi, M., Jimenez, P. T., Grindler, N., et al. (2012). High fat diet induced developmental defects in the mouse: Oocyte meiotic aneuploidy and fetal growth retardation/brain defects. PLoS One 7, e49217. doi:10.1371/journal.pone.0049217
Makki, K., Froguel, P., and Wolowczuk, I. (2013). Adipose tissue in obesity-related inflammation and insulin resistance: Cells, cytokines, and chemokines. ISRN Inflamm. 2013, 139239. doi:10.1155/2013/139239
Mangwiro, Y. T., Briffa, J. F., Gravina, S., Mahizir, D., Anevska, K., Romano, T., et al. (2018a). Maternal exercise and growth restriction in rats alters placental angiogenic factors and blood space area in a sex-specific manner. Placenta 74, 47–54.
Mangwiro, Y. T., Cuffe, J. S., Briffa, J. F., Mahizir, D., Anevska, K., Jefferies, A. J., et al. (2018b). Maternal exercise in rats upregulates the placental insulin‐like growth factor system with diet‐and sex‐specific responses: Minimal effects in mothers born growth restricted. J. physiology 596 (23), 5947–5964.
Masuzaki, H., Ogawa, Y., Sagawa, N., Hosoda, K., Matsumoto, T., Mise, H., et al. (1997). Nonadipose tissue production of leptin: Leptin as a novel placenta-derived hormone in humans. Nat. Med. 3, 1029–1033. doi:10.1038/nm0997-1029
Mazaki-Tovi, S., Kanety, H., Pariente, C., Hemi, R., Wiser, A., Schiff, E., et al. (2007). Maternal serum adiponectin levels during human pregnancy. J. Perinatol. 27, 77–81. doi:10.1038/sj.jp.7211639
McLaughlin, T., Deng, A., Yee, G., Lamendola, C., Reaven, G., Tsao, P. S., et al. (2010). Inflammation in subcutaneous adipose tissue: Relationship to adipose cell size. Diabetologia 53 (2), 369–377.
McLaughlin, T., Sherman, A., Tsao, P., Gonzalez, O., Yee, G., Lamendola, C., et al. (2007). Enhanced proportion of small adipose cells in insulin-resistant vs insulin-sensitive obese individuals implicates impaired adipogenesis. Diabetologia 50 (8), 1707–1715.
McMillen, I. C., Adam, C. L., and Mühlhäusler, B. S. (2005). Early origins of obesity: Programming the appetite regulatory system. J. Physiology 565, 9–17. doi:10.1113/jphysiol.2004.081992
Mleczko, J., Ortega, F. J., Falcon‐Perez, J. M., Wabitsch, M., Fernandez‐Real, J. M., and Mora, S. (2018). Extracellular vesicles from hypoxic adipocytes and obese subjects reduce insulin‐stimulated glucose uptake. Mol. Nutr. Food Res. 62, 1700917. doi:10.1002/mnfr.201700917
Morales, E., Rodriguez, A., Valvi, D., Iñiguez, C., Esplugues, A., Vioque, J., et al. (2015). Deficit of vitamin D in pregnancy and growth and overweight in the offspring. Int. J. Obes. (Lond) 39, 61–68. doi:10.1038/ijo.2014.165
Mundi, M. S., Karpyak, M. V., Koutsari, C., Votruba, S. B., O'Brien, P. C., and Jensen, M. D. (2010). Body fat distribution, adipocyte size, and metabolic characteristics of nondiabetic adults. J. Clin. Endocrinol. Metabolism 95 (1), 67–73.
Myatt, L., and Maloyan, A. (2016). Obesity and placental function. Semin. Reprod. Med. 34, 42–49. doi:10.1055/s-0035-1570027
Nakahara, A., Nair, S., Ormazabal, V., Elfeky, O., Garvey, C. E., Longo, S., et al. (2020). Circulating placental extracellular vesicles and their potential roles during pregnancy. Ochsner J. 20, 439–445. doi:10.31486/toj.20.0049
Nascimento, F. A. M., Ceciliano, T. C., Aguila, M. B., and Mandarim-de-Lacerda, C. A. (2013). Transgenerational effects on the liver and pancreas resulting from maternal vitamin D restriction in mice. J. Nutr. Sci. Vitaminol. (Tokyo) 59, 367–374. doi:10.3177/jnsv.59.367
Neeland, I. J., Ross, R., Després, J.-P., Matsuzawa, Y., Yamashita, S., Shai, I., et al. (2019). Visceral and ectopic fat, atherosclerosis, and cardiometabolic disease: A position statement. Lancet Diabetes Endocrinol. 7, 715–725. doi:10.1016/S2213-8587(19)30084-1
Nelson, S. M., Matthews, P., and Poston, L. (2010). Maternal metabolism and obesity: Modifiable determinants of pregnancy outcome. Hum. Reprod. Update 16, 255–275. doi:10.1093/humupd/dmp050
Neri, C., and Edlow, A. G. (2016). Effects of maternal obesity on fetal programming: Molecular approaches. Cold Spring Harb. Perspect. Med. 6, a026591. doi:10.1101/cshperspect.a026591
Ng, S.-F., Lin, R. C. Y., Laybutt, D. R., Barres, R., Owens, J. A., and Morris, M. J. (2010). Chronic high-fat diet in fathers programs β-cell dysfunction in female rat offspring. Nature 467, 963–966. doi:10.1038/nature09491
Ng, S.-F., Lin, R. C. Y., Maloney, C. A., Youngson, N. A., Owens, J. A., and Morris, M. J. (2014). Paternal high-fat diet consumption induces common changes in the transcriptomes of retroperitoneal adipose and pancreatic islet tissues in female rat offspring. FASEB J. 28, 1830–1841. doi:10.1096/fj.13-244046
Nicholas, L. M., Morrison, J. L., Rattanatray, L., Zhang, S., Ozanne, S. E., and McMillen, I. C. (2016). The early origins of obesity and insulin resistance: Timing, programming and mechanisms. Int. J. Obes. (Lond) 40, 229–238. doi:10.1038/ijo.2015.178
O’Callaghan, M. J., Williams, G. M., Andersen, M. J., Bor, W., and Najman, J. M. (1997). Prediction of obesity in children at 5 years: A cohort study. J. Paediatr. Child. Health 33, 311–316. doi:10.1111/j.1440-1754.1997.tb01607.x
O’Dowd, J. F., and Stocker, C. J. (2013). Endocrine pancreatic development: Impact of obesity and diet. Front. Physiol. 4, 170. doi:10.3389/fphys.2013.00170
Ogier, V., Ziegler, O., Méjean, L., Nicolas, J. P., and Stricker-Krongrad, A. (2002). Obesity is associated with decreasing levels of the circulating soluble leptin receptor in humans. Int. J. Obes. Relat. Metab. Disord. 26, 496–503. doi:10.1038/sj.ijo.0801951
Palma, C., McIntyre, H. D., and Salomon, C. (2022). Extracellular vesicles-new players in cell-to-cell communication in gestational diabetes mellitus. Biomedicines 10, 462. doi:10.3390/biomedicines10020462
Parra-Peralbo, E., Talamillo, A., and Barrio, R. (2021). Origin and development of the adipose tissue, a key organ in physiology and disease. Front. Cell Dev. Biol. 9, 786129. doi:10.3389/fcell.2021.786129
Parrettini, S., Caroli, A., and Torlone, E. (2020). Nutrition and metabolic adaptations in physiological and complicated pregnancy: Focus on obesity and gestational diabetes. Front. Endocrinol. (Lausanne) 11, 611929. doi:10.3389/fendo.2020.611929
Patel, M. S., and Srinivasan, M. (2011). Metabolic programming in the immediate postnatal life. Ann. Nutr. Metab. 58 (2), 18–28. doi:10.1159/000328040
Paulsen, M. E., Rosario, F. J., Wesolowski, S. R., Powell, T. L., and Jansson, T. (2019). Normalizing adiponectin levels in obese pregnant mice prevents adverse metabolic outcomes in offspring. FASEB J. 33, 2899–2909. doi:10.1096/fj.201801015R
Pérez-González, R., Kim, Y., Miller, C., Pacheco-Quinto, J., Eckman, E. A., and Levy, E. (2020). Extracellular vesicles: Where the amyloid precursor protein carboxyl-terminal fragments accumulate and amyloid-β oligomerizes. FASEB J. 34, 12922–12931. doi:10.1096/fj.202000823R
Pérez-Pérez, A., Toro, A., Vilariño-García, T., Maymó, J., Guadix, P., Dueñas, J. L., et al. (2018). Leptin action in normal and pathological pregnancies. J. Cell. Mol. Med. 22, 716–727. doi:10.1111/jcmm.13369
Pétursdóttir Maack, H., Larsson, A., Axelsson, O., Olovsson, M., Wikström, A.-K., and Sundström Poromaa, I. (2020). Pregnancy in metabolic healthy and unhealthy obese women. Acta Obstetricia Gynecol. Scand. 99, 1640–1648. doi:10.1111/aogs.13929
Pincu, Y., Yoel, U., Haim, Y., Makarenkov, N., Maixner, N., Shaco-Levy, R., et al. (2022). Assessing obesity-related adipose tissue disease (OrAD) to improve precision medicine for patients living with obesity. Front. Endocrinol. (Lausanne) 13, 860799. doi:10.3389/fendo.2022.860799
Plows, J. F., Stanley, J. L., Baker, P. N., Reynolds, C. M., and Vickers, M. H. (2018). The pathophysiology of gestational diabetes mellitus. Int. J. Mol. Sci. 19, E3342. doi:10.3390/ijms19113342
Poissonnet, C. M., Burdi, A. R., and Garn, S. M. (1984). The chronology of adipose tissue appearance and distribution in the human fetus. Early Hum. Dev. 10, 1–11. doi:10.1016/0378-3782(84)90106-3
Poston, L., Caleyachetty, R., Cnattingius, S., Corvalán, C., Uauy, R., Herring, S., et al. (2016). Preconceptional and maternal obesity: Epidemiology and health consequences. Lancet Diabetes Endocrinol. 4, 1025–1036. doi:10.1016/S2213-8587(16)30217-0
Qiao, J., Dai, L.-J., Zhang, Q., and Ouyang, Y.-Q. (2020). A meta-analysis of the association between breastfeeding and early childhood obesity. J. Pediatr. Nurs. 53, 57–66. doi:10.1016/j.pedn.2020.04.024
Ramalingam, L., Menikdiwela, K. R., Clevenger, S., Eboh, T., Allen, L., Koboziev, I., et al. (2018). Maternal and postnatal supplementation of fish oil improves metabolic health of mouse male offspring. Obesity 26 (11), 1740–1748.
Rattanatray, L., MacLaughlin, S. M., Kleemann, D. O., Walker, S. K., Muhlhausler, B. S., and McMillen, I. C. (2010). Impact of maternal periconceptional overnutrition on fat mass and expression of adipogenic and lipogenic genes in visceral and subcutaneous fat depots in the postnatal lamb. Endocrinology 151, 5195–5205. doi:10.1210/en.2010-0501
Reynolds, C. M., Segovia, S. A., and Vickers, M. H. (2017). Experimental models of maternal obesity and neuroendocrine programming of metabolic disorders in offspring. Front. Endocrinol. (Lausanne) 8, 245. doi:10.3389/fendo.2017.00245
Rice, G. E., Scholz-Romero, K., Sweeney, E., Peiris, H., Kobayashi, M., Duncombe, G., et al. (2015). The effect of glucose on the release and bioactivity of exosomes from first trimester trophoblast cells. J. Clin. Endocrinol. Metab. 100, E1280–E1288. doi:10.1210/jc.2015-2270
Rodrigo, N., Saad, S., Pollock, C., and Glastras, S. J. (2022). Diet modification before or during pregnancy on maternal and foetal outcomes in rodent models of maternal obesity. Nutrients 14 (10), 2154.
Rooney, B. L., Mathiason, M. A., and Schauberger, C. W. (2011). Predictors of obesity in childhood, adolescence, and adulthood in a birth cohort. Matern. Child. Health J. 15, 1166–1175. doi:10.1007/s10995-010-0689-1
Rosario, F. J., Schumacher, M. A., Jiang, J., Kanai, Y., Powell, T. L., and Jansson, T. (2012). Chronic maternal infusion of full‐length adiponectin in pregnant mice down‐regulates placental amino acid transporter activity and expression and decreases fetal growth. J. Physiology 590 (6), 1495–1509.
Rosen, E. D., and MacDougald, O. A. (2006). Adipocyte differentiation from the inside out. Nat. Rev. Mol. Cell Biol. 7, 885–896. doi:10.1038/nrm2066
Rospleszcz, S., Lorbeer, R., Storz, C., Schlett, C. L., Meisinger, C., Thorand, B., et al. (2019). Association of longitudinal risk profile trajectory clusters with adipose tissue depots measured by magnetic resonance imaging. Sci. Rep. 9, 16972. doi:10.1038/s41598-019-53546-y
Ross, M. G., and Desai, M. (2014). Developmental programming of appetite/satiety. Ann. Nutr. Metab. 64 (1), 36–44. doi:10.1159/000360508
Sáez, T., de Vos, P., Kuipers, J., Sobrevia, L., and Faas, M. M. (2018a). Fetoplacental endothelial exosomes modulate high d-glucose-induced endothelial dysfunction. Placenta 66, 26–35. doi:10.1016/j.placenta.2018.04.010
Sáez, T., Salsoso, R., Leiva, A., Toledo, F., de Vos, P., Faas, M., et al. (2018b). Human umbilical vein endothelium-derived exosomes play a role in foetoplacental endothelial dysfunction in gestational diabetes mellitus. Biochimica Biophysica Acta (BBA) - Mol. Basis Dis. 1864, 499–508. doi:10.1016/j.bbadis.2017.11.010
Salazar-Petres, E. R., and Sferruzzi-Perri, A. N. (2022). Pregnancy-induced changes in β-cell function: What are the key players? J. Physiol. 600, 1089–1117. doi:10.1113/JP281082
Salomon, C., Scholz-Romero, K., Sarker, S., Sweeney, E., Kobayashi, M., Correa, P., et al. (2016). Gestational diabetes mellitus is associated with changes in the concentration and bioactivity of placenta-derived exosomes in maternal circulation across gestation. Diabetes 65, 598–609. doi:10.2337/db15-0966
Salomon, C., Torres, M. J., Kobayashi, M., Scholz-Romero, K., Sobrevia, L., Dobierzewska, A., et al. (2014). A gestational profile of placental exosomes in maternal plasma and their effects on endothelial cell migration. PLoS One 9, e98667. doi:10.1371/journal.pone.0098667
Samuelsson, A.-M., Matthews, P. A., Argenton, M., Christie, M. R., McConnell, J. M., Jansen, E. H. J. M., et al. (2008). Diet-induced obesity in female mice leads to offspring hyperphagia, adiposity, hypertension, and insulin resistance: A novel murine model of developmental programming. Hypertension 51, 383–392. doi:10.1161/HYPERTENSIONAHA.107.101477
Sanchez-Margalet, V., Valle, M., Ruz, F. J., Gascon, F., Mateo, J., and Goberna, R. (2002). Elevated plasma total homocysteine levels in hyperinsulinemic obese subjects. J. Nutr. Biochem. 13, 75–79. doi:10.1016/s0955-2863(01)00197-8
Sarker, S., Scholz-Romero, K., Perez, A., Illanes, S. E., Mitchell, M. D., Rice, G. E., et al. (2014). Placenta-derived exosomes continuously increase in maternal circulation over the first trimester of pregnancy. J. Transl. Med. 12, 204. doi:10.1186/1479-5876-12-204
Scherrer, U., Rimoldi, S. F., Rexhaj, E., Stuber, T., Duplain, H., Garcin, S., et al. (2012). Systemic and pulmonary vascular dysfunction in children conceived by assisted reproductive technologies. Circulation 125, 1890–1896. doi:10.1161/CIRCULATIONAHA.111.071183
Schwartz, M. W. (2001). Brain pathways controlling food intake and body weight. Exp. Biol. Med. (Maywood) 226, 978–981. doi:10.1177/153537020122601103
Shapira, N. A., Lessig, M. C., He, A. G., James, G. A., Driscoll, D. J., and Liu, Y. (2005). Satiety dysfunction in Prader-Willi syndrome demonstrated by fMRI. J. Neurol. Neurosurg. Psychiatry 76, 260–262. doi:10.1136/jnnp.2004.039024
Sharp, G. C., Lawlor, D. A., Richmond, R. C., Fraser, A., Simpkin, A., Suderman, M., et al. (2015). Maternal pre-pregnancy BMI and gestational weight gain, offspring DNA methylation and later offspring adiposity: Findings from the avon longitudinal study of parents and children. Int. J. Epidemiol. 44, 1288–1304. doi:10.1093/ije/dyv042
Sheller-Miller, S., Choi, K., Choi, C., and Menon, R. (2019). Cyclic-recombinase-reporter mouse model to determine exosome communication and function during pregnancy. Am. J. Obstet. Gynecol. 221, 502. e12. doi:10.1016/j.ajog.2019.06.010
Shimizu, I., and Walsh, K. (2015). The whitening of Brown fat and its implications for weight management in obesity. Curr. Obes. Rep. 4, 224–229. doi:10.1007/s13679-015-0157-8
Shin, S., Pang, Y., Park, J., Liu, L., Lukas, B. E., Kim, S. H., et al. (2020). Dynamic control of adipose tissue development and adult tissue homeostasis by platelet-derived growth factor receptor alpha. Elife 9, e56189. doi:10.7554/eLife.56189
Sinclair, K. D., and Watkins, A. J. (2013). Parental diet, pregnancy outcomes and offspring health: Metabolic determinants in developing oocytes and embryos. Reprod. Fertil. Dev. 26, 99–114. doi:10.1071/RD13290
Sivasami, P., Poudel, N., Munteanu, M. C., Hudson, J., Lovern, P., Liu, L., et al. (2019). Adipose tissue loss and lipodystrophy in xylosyltransferase II deficient mice. Int. J. Obes. 43 (9), 1783–1794.
Skvortsova, K., Iovino, N., and Bogdanović, O. (2018). Functions and mechanisms of epigenetic inheritance in animals. Nat. Rev. Mol. Cell Biol. 19, 774–790. doi:10.1038/s41580-018-0074-2
Smith, F. M., Garfield, A. S., and Ward, A. (2006). Regulation of growth and metabolism by imprinted genes. Cytogenet Genome Res. 113, 279–291. doi:10.1159/000090843
Smits, A., Marei, W. F., De Neubourg, D., and Leroy, J. L. (2021). Diet normalization or caloric restriction as a preconception care strategy to improve metabolic health and oocyte quality in obese outbred mice. Reproductive Biol. Endocrinol. 19 (1), 1–21.
Son, J. S., Liu, X., Tian, Q., Zhao, L., Chen, Y., Hu, Y., et al. (2019). Exercise prevents the adverse effects of maternal obesity on placental vascularization and fetal growth. J. physiology 597 (13), 3333–3347.
Spalding, K. L., Arner, E., Westermark, P. O., Bernard, S., Buchholz, B. A., Bergmann, O., et al. (2008). Dynamics of fat cell turnover in humans. Nature 453, 783–787. doi:10.1038/nature06902
Srinivasan, M., and Patel, M. S. (2008). Metabolic programming in the immediate postnatal period. Trends Endocrinol. Metab. 19, 146–152. doi:10.1016/j.tem.2007.12.001
Stanford, K. I., Lee, M. Y., Getchell, K. M., So, K., Hirshman, M. F., and Goodyear, L. J. (2015). Exercise before and during pregnancy prevents the deleterious effects of maternal high-fat feeding on metabolic health of male offspring. Diabetes 64 (2), 427–433.
Stanford, K. I., Takahashi, H., So, K., Alves-Wagner, A. B., Prince, N. B., Lehnig, A. C., et al. (2017). Maternal exercise improves glucose tolerance in female offspring. Diabetes 66 (8), 2124–2136.
Straughen, J. K., Trudeau, S., and Misra, V. K. (2013). Changes in adipose tissue distribution during pregnancy in overweight and obese compared with normal weight women. Nutr. Diabetes 3, e84. doi:10.1038/nutd.2013.25
Suárez-Cuenca, J. A., La Peña-Sosa, D., La Vega-Moreno, D., Banderas-Lares, D. Z., Salamanca-García, M., Martínez-Hernández, J. E., et al. (2021). Enlarged adipocytes from subcutaneous vs. visceral adipose tissue differentially contribute to metabolic dysfunction and atherogenic risk of patients with obesity. Sci. Rep. 11 (1), 1–11.
Summerfield, M., Zhou, Y., Zhou, T., Wu, C., Alpini, G., Zhang, K. K., et al. (2018). A long-term maternal diet transition from high-fat diet to normal fat diet during pre-pregnancy avoids adipose tissue inflammation in next generation. PLoS One 13 (12), e0209053.
Tessier, D. R., Ferraro, Z. M., and Gruslin, A. (2013). Role of leptin in pregnancy: Consequences of maternal obesity. Placenta 34, 205–211. doi:10.1016/j.placenta.2012.11.035
Trivett, C., Lees, Z. J., and Freeman, D. J. (2021). Adipose tissue function in healthy pregnancy, gestational diabetes mellitus and pre-eclampsia. Eur. J. Clin. Nutr. 75, 1745–1756. doi:10.1038/s41430-021-00948-9
Valenzuela-Alcaraz, B., Crispi, F., Bijnens, B., Cruz-Lemini, M., Creus, M., Sitges, M., et al. (2013). Assisted reproductive technologies are associated with cardiovascular remodeling in utero that persists postnatally. Circulation 128, 1442–1450. doi:10.1161/CIRCULATIONAHA.113.002428
Vaughan, O. R., Rosario, F. J., Powell, T. L., and Jansson, T. (2020). Normalisation of circulating adiponectin levels in obese pregnant mice prevents cardiac dysfunction in adult offspring. Int. J. Obes. (Lond) 44, 488–499. doi:10.1038/s41366-019-0374-4
Vega, C. C., Reyes-Castro, L. A., Bautista, C. J., Larrea, F., Nathanielsz, P. W., and Zambrano, E. (2015). Exercise in obese female rats has beneficial effects on maternal and male and female offspring metabolism. Int. J. Obes. 39 (4), 712–719.
Von Versen-Höynck, F., Rajakumar, A., Parrott, M. S., and Powers, R. W. (2009). Leptin affects system A amino acid transport activity in the human placenta: Evidence for STAT3 dependent mechanisms. Placenta 30, 361–367. doi:10.1016/j.placenta.2009.01.004
Vucetic, Z., Kimmel, J., Totoki, K., Hollenbeck, E., and Reyes, T. M. (2010). Maternal high-fat diet alters methylation and gene expression of dopamine and opioid-related genes. Endocrinology 151, 4756–4764. doi:10.1210/en.2010-0505
Wadhwa, P. D., Buss, C., Entringer, S., and Swanson, J. M. (2009). Developmental origins of health and disease: Brief history of the approach and current focus on epigenetic mechanisms. Semin. Reprod. Med. 27, 358–368. doi:10.1055/s-0029-1237424
Watkins, A. J., Platt, D., Papenbrock, T., Wilkins, A., Eckert, J. J., Kwong, W. Y., et al. (2007). Mouse embryo culture induces changes in postnatal phenotype including raised systolic blood pressure. Proc. Natl. Acad. Sci. U. S. A. 104, 5449–5454. doi:10.1073/pnas.0610317104
Wei, Y., Yang, C.-R., Wei, Y.-P., Zhao, Z.-A., Hou, Y., Schatten, H., et al. (2014). Paternally induced transgenerational inheritance of susceptibility to diabetes in mammals. Proc. Natl. Acad. Sci. U. S. A. 111, 1873–1878. doi:10.1073/pnas.1321195111
Wells, J. C., Sawaya, A. L., Wibaek, R., Mwangome, M., Poullas, M. S., Yajnik, C. S., et al. (2020). The double burden of malnutrition: Aetiological pathways and consequences for health. Lancet 395, 75–88. doi:10.1016/S0140-6736(19)32472-9
Whitham, M., Parker, B. L., Friedrichsen, M., Hingst, J. R., Hjorth, M., Hughes, W. E., et al. (2018). Extracellular vesicles provide a means for tissue crosstalk during exercise. Cell Metab. 27, 237–251. e4. doi:10.1016/j.cmet.2017.12.001
WHO (World Health Organization) (2021). Obesity and overweight”. Available at: https://www.who.int/news-room/fact-sheets/detail/obesity-and-overweight (Accessed June 15, 2022).
Woods, S. C., Seeley, R. J., Porte, D., and Schwartz, M. W. (1998). Signals that regulate food intake and energy homeostasis. Science 280, 1378–1383. doi:10.1126/science.280.5368.1378
Wu, Y., Chanclón, B., Micallef, P., Stener-Victorin, E., Wernstedt Asterholm, I., and Benrick, A. (2021). Maternal adiponectin prevents visceral adiposity and adipocyte hypertrophy in prenatal androgenized female mice. FASEB J. Apr 35 (4), e21299. doi:10.1096/fj.202002212R
Yajnik, C. S., and Yajnik, P. C. (2020). Fetal adiposity epidemic in the modern world: A thrifty phenotype aggravated by maternal obesity and diabetes. Am. J. Clin. Nutr. 112, 8–10. doi:10.1093/ajcn/nqaa122
Yang, Q.-Y., Liang, J.-F., Rogers, C. J., Zhao, J.-X., Zhu, M.-J., and Du, M. (2013). Maternal obesity induces epigenetic modifications to facilitate Zfp423 expression and enhance adipogenic differentiation in fetal mice. Diabetes 62, 3727–3735. doi:10.2337/db13-0433
Ying, T., and Simmons, R. A. (2021). The role of adipocyte precursors in development and obesity. Front. Endocrinol. (Lausanne) 11, 613606. doi:10.3389/fendo.2020.613606
Ying, W., Riopel, M., Bandyopadhyay, G., Dong, Y., Birmingham, A., Seo, J. B., et al. (2017). Adipose tissue macrophage-derived exosomal miRNAs can modulate in vivo and in vitro insulin sensitivity. Cell 171, 372–384. e12. doi:10.1016/j.cell.2017.08.035
Keywords: developmental programming, obesity, extracellular vesicles, placentation, adipogenesis, pancreatic developmnent, appetite regulation, epigenteics
Citation: Cechinel LR, Batabyal RA, Freishtat RJ and Zohn IE (2022) Parental obesity-induced changes in developmental programming. Front. Cell Dev. Biol. 10:918080. doi: 10.3389/fcell.2022.918080
Received: 12 April 2022; Accepted: 06 September 2022;
Published: 07 October 2022.
Edited by:
Patricia Ybot-Gonzalez, Institute of Biomedicine of Seville (CSIC), SpainReviewed by:
Claudia Kappen, Pennington Biomedical Research Center, United StatesBredford D. Kerr, San Sebastián University, Chile
Copyright © 2022 Cechinel, Batabyal, Freishtat and Zohn. This is an open-access article distributed under the terms of the Creative Commons Attribution License (CC BY). The use, distribution or reproduction in other forums is permitted, provided the original author(s) and the copyright owner(s) are credited and that the original publication in this journal is cited, in accordance with accepted academic practice. No use, distribution or reproduction is permitted which does not comply with these terms.
*Correspondence: Irene E. Zohn, aXpvaG5AY2hpbGRyZW5zbmF0aW9uYWwub3Jn