- Center for Biomedical Technology, Department for Biomedical Research, University for Continuing Education Krems, Krems, Austria
Activated platelets and platelet-derived extracellular vesicles (EVs) have emerged as central players in thromboembolic complications associated with severe coronavirus disease 2019 (COVID-19). Platelets bridge hemostatic, inflammatory, and immune responses by their ability to sense pathogens via various pattern recognition receptors, and they respond to infection through a diverse repertoire of mechanisms. Dysregulated platelet activation, however, can lead to immunothrombosis, a simultaneous overactivation of blood coagulation and the innate immune response. Mediators released by activated platelets in response to infection, such as antimicrobial peptides, high mobility group box 1 protein, platelet factor 4 (PF4), and PF4+ extracellular vesicles promote neutrophil activation, resulting in the release of neutrophil extracellular traps and histones. Many of the factors released during platelet and neutrophil activation are positively charged and interact with endogenous heparan sulfate or exogenously administered heparin via electrostatic interactions or via specific binding sites. Here, we review the current state of knowledge regarding the involvement of platelets and platelet-derived EVs in the pathogenesis of immunothrombosis, and we discuss the potential of extracorporeal therapies using adsorbents functionalized with heparin to deplete platelet-derived and neutrophil-derived mediators of immunothrombosis.
Introduction
Next to the lung inflammatory syndrome, thrombotic events and endothelial dysfunction are key pathogenic mechanisms of severe coronavirus disease-19 (COVID-19) caused by the severe acute respiratory syndrome coronavirus 2 (SARS-CoV-2) (Bonaventura et al., 2021; Hottz et al., 2020; Klok et al., 2020; Middeldorp et al., 2020; Middleton et al., 2020; Tang N. et al., 2020). Here, we focus on the involvement of platelets and platelet-derived extracellular vesicles (pEVs) in COVID-19-related immunothrombosis. We discuss the interaction of heparan sulfate and heparin with activated platelets and with mediators of immunothrombosis and highlight potential therapeutic implications of this interaction.
Immunothrombosis as a Hallmark of COVID-19
SARS-CoV-2 is an enveloped, single-stranded RNA virus (Masters, 2006; Zhou et al., 2020; Hu et al., 2021). Binding to its target cells depends mainly on the interaction of the viral spike protein with angiotensin-converting enzyme 2 (ACE2) on the surface of its target cells.
The clinical spectrum of COVID-19 ranges from asymptomatic or mild forms to severe disease requiring intensive care (Wiersinga et al., 2020). COVID-19 was first described as a pulmonary condition (Zhou et al., 2020; Zhu et al., 2020), but can be associated with gastrointestinal, cardiovascular, renal, or neurological dysfunctions alike (Gupta et al., 2020; Renu et al., 2020). Severe SARS-CoV-2 infection frequently induces a prothrombotic state, which can progress to multiorgan failure (Yang et al., 2020; Lopes-Pacheco et al., 2021). Laboratory parameters associated with a higher thrombotic risk in COVID-19 patients include elevated D-dimer levels, low fibrinogen, and low lymphocyte counts (Thomas and Scully, 2022).
The thrombotic events associated with severe COVID-19 are best described as immunothrombosis, a simultaneous overactivation of coagulation and the innate immune system (Engelmann and Massberg, 2013; Ackermann et al., 2020; Connors and Levy, 2020; Skendros et al., 2020). During immunothrombosis, deregulated complement activation enhances neutrophil activation and recruitment to the infected lungs, and promotes tissue factor (TF) expression, resulting in microvascular thrombosis and endothelial dysfunction (Bonaventura et al., 2021). The excessive release of neutrophil extracellular traps (NETs), which consist in DNA fibers associated with neutrophil elastase, antimicrobial peptides, TF citrullinated histone H3 (Nomura et al., 2019) as well as with high-mobility group box 1 protein (HMGB1) (Garcia-Romo et al., 2011) and activated platelets (Brinkmann et al., 2004; Li et al., 2020), further promotes thrombosis and tissue damage (Middleton et al., 2020). In addition to this complement/neutrophil/TF axis, platelets are centrally involved in initiating and propagating immunothrombosis in COVID-19 (Foley and Conway, 2016; Martinod and Deppermann, 2021), as discussed in detail below and shown in Figure 1. Altogether, the disproportionate activation of the innate immune response after infection with SARS-CoV-2 leads to an excessive release of inflammatory mediators (Fara et al., 2020; Huang et al., 2020), giving rise to a tissue-damaging environment (Elrobaa and New, 2021) and uncontrolled microthrombus formation (Helms et al., 2020; Iba et al., 2020; McFadyen et al., 2020).
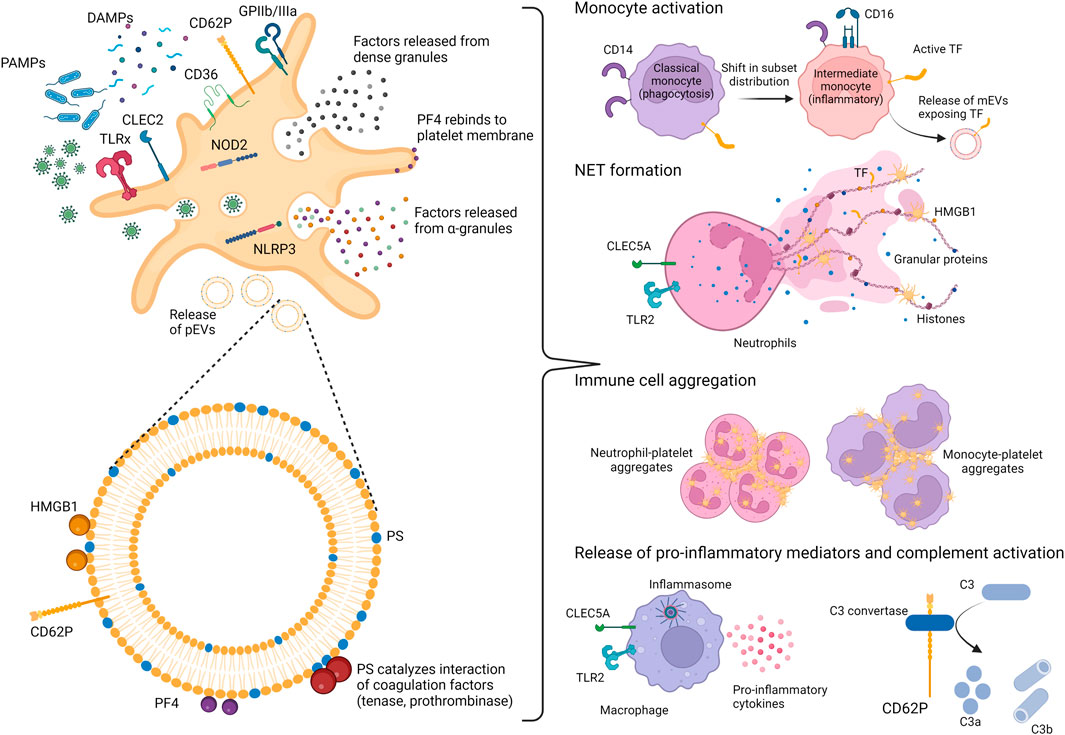
FIGURE 1. Platelets and platelet-derived EVs as mediators of immunothrombosis. Platelets act as sentinels by expressing pathogen-recognition receptors (PRRs), able to recognize pathogen-associated molecular patterns (PAMPs) or damage-associated molecular patterns (DAMPs). Upon activation, platelets expose on their surface activation markers (CD62P and GPIIb/IIIa) and release various mediators from their α-and dense-granules, including PF4 and HMGB1, both exerting affinity for heparin. Activated platelets also shed platelet-derived extracellular vesicles (pEVs), which expose phosphatidylserine (PS) as well as PF4 and HMGB1. Altogether, platelet activation and pEV release induce numerous processes supporting immunothrombosis, namely a shift in monocyte subsets towards inflammatory monocytes, the stimulation of neutrophil extracellular trap (NET) formation and enhanced interaction of platelets with other platelets, as well as with monocytes or neutrophils. TLR, Toll-like receptor; CLEC2, C-type lectin receptor 2; CLEC5A, C-type lectin receptor 5A; NOD2, nucleotide-binding oligomerization domain 2; NLRP3, nucleotide-binding domain rich leucine repeat containing protein; GP, glycoprotein; TF, tissue factor; PF4, platelet factor 4; HMGB1, high mobility group box 1 protein; mEVs, monocyte-derived EVs; C3, complement component 3.
Platelets as Mediators of Immunothrombosis
Platelets Are Sentinels of Endogenous and Exogenous Signals of Infection
Platelets are small (1–3 µm), short-lived (up to 9 days), anucleate entities derived from megakaryocytes residing in the bone marrow. With a concentration of 150,000 to 400,000 platelets per µL, they represent the second most abundant formed elements of blood (Ghoshal and Bhattacharyya, 2014). They are equipped with an array of surface and intracellular receptors to detect pathogens, enabling their function as intravascular sentinels. Platelets can contribute to the propagation of inflammatory processes through the release of mediators from their dense- and α-granules and through their interaction with other immune cells (Morrell et al., 2014).
SARS-CoV-2 can trigger an innate immune response via pathogen-associated molecular patterns (PAMPs) such as single-stranded RNA or the spike protein (Khan et al., 2021), and it stimulates the release of damage-associated molecular patterns (DAMPs) including HMGB1 (Chen et al., 2020). Platelets express with a wide range of pattern recognition receptors (PRRs) to sense PAMPs and DAMPs during viral infection. Human platelet PRRs include Toll-like receptors (TLRs) 1–10, which have all been detected in human platelets using RT-PCR (Koupenova et al., 2015). The presence of TLR1 (Shiraki et al., 2004), TLR2 (Cognasse et al., 2005; Aslam et al., 2006; Claushuis et al., 2019; Marín Oyarzún et al., 2020), TLR3 (Anabel et al., 2014), TLR4 (Cognasse et al., 2005; Claushuis et al., 2019; Marín Oyarzún et al., 2020), TLR5 (Claushuis et al., 2019), TLR6 (Shiraki et al., 2004; Aslam et al., 2006), TLR7 (Koupenova et al., 2014), TLR8 (Leroy et al., 2019), TLR9 (Cognasse et al., 2005; Aslam et al., 2006; Thon et al., 2012; Claushuis et al., 2019) in human platelets has been confirmed using Western blotting and/or flow cytometry.
Platelets have been shown to endocytose RNA viruses (Seyoum et al., 2018) and to recognize viral RNA via their endosomal TLR3, TLR7 and TLR8 (Hottz et al., 2018; Azkur et al., 2020). Viral recognition by platelet TLRs elicits signaling through the adaptor molecule MyD88, resulting in platelet activation, aggregation, granule secretion and interleukin (IL)-1 beta release (D’Atri and Schattner, 2017; Marín Oyarzún et al., 2020). Notably, the secretory profile of immuno-activated platelets appears to be distinct from the secretory profile during hemostatic platelet activation (Banerjee et al., 2020). While platelet activation mediated by G-protein-coupled receptors occurs rapidly, platelet activation mediated by PRRs in response to infection can be delayed and sustained (Vieira-de-Abreu et al., 2012).
In addition to TLRs, platelets express nucleotide-binding oligomerization domain (NOD)-like receptors (NLRs), such as NOD2 which recognizes the bacterial component muramyl dipeptide (Zhang et al., 2015; Domínguez-Martínez et al., 2018) and viral RNA after internalization of SARS-CoV-2 by platelets. NOD2 acts via the mitochondrial antiviral signaling protein, a key mediator of the innate immune response to RNA viral infection (Vazquez and Horner, 2015; Fard et al., 2021), triggering the release of inflammatory cytokines.
Platelets also express the nucleotide-binding domain leucine rich repeat containing protein (NLRP3) (Hottz et al., 2015, 2013), a major sensor for the activation of the inflammasome by bacterial, viral or tissue damage signals.
In addition, they express C-type lectin receptor 2 (CLEC2) (Sung et al., 2019), which can recognize viral surface glycan patterns, as previously shown for human immunodeficiency virus (Chaipan et al., 2006) and dengue virus (Sung et al., 2019). CLEC2 might also interact with sialylated O-glycans of SARS-CoV-2 spike proteins (Gadanec et al., 2021), resulting in tyrosine-kinase-associated signal transduction and platelet activation (Meng et al., 2021).
Platelets can thus sense and respond to SARS-CoV-2 infection via different PRRs. There is no clear consensus, however, whether human platelets express the ACE2 on their surface, which has been identified as one of the main entry routes for SARS-CoV-2 into host cells (Lan et al., 2020). Some groups have described ACE2 expression on platelets (Zhang et al., 2020; Koupenova et al., 2021), while others have been unable to confirm its presence (Manne et al., 2020; Song et al., 2020; Zaid et al., 2020; Campbell et al., 2021a; Li et al., 2022). Platelets may also use other receptors to interact with SARS-CoV-2, including CD147 as well as glucose regulated protein 78 and kringle containing transmembrane protein 1 (Shen et al., 2021).
Platelet Activation in COVID-19
Following pathogen recognition and activation, platelets mediate and propagate the innate immune response by various mechanisms, such as the release of chemokines including platelet factor 4 (PF4), modulation of leukocyte migration (Pan et al., 2015; Chimen et al., 2020; Middleton et al., 2020), leukocyte recruitment to thrombi (Swystun and Liaw, 2016), induction of NET formation (Clark et al., 2007), monocyte expression of TF (Lindmark et al., 2000; Ivanov et al., 2019) as well as the release of extracellular vesicles (EVs) (Suades et al., 2022). In addition, platelets sustain inflammation via the release of mediators from their α- and dense granules (D’Atri and Schattner, 2017), triggering the release of pro-inflammatory cytokines and inflammasome assembly by neutrophils and macrophages (Chen et al., 2017), complement activation (del Conde et al., 2005a), and exposure of CD40L (Lievens et al., 2010) leading to endothelial activation (Cognasse et al., 2022).
PF4 is an abundant platelet α-granule chemokine released during platelet activation. In addition to its soluble form, PF4 is displayed on the surface of activated platelets and of pEVs. PF4 expression is strongly elevated following trauma as well as in sepsis (Maharaj and Chang, 2018; Wegrzyn et al., 2021) and COVID-19 (Comer et al., 2021). Due to its positive charge at physiological pH, PF4 binds to endogenous heparan sulfate and other glycosaminoglycans as well as to exogenously administered heparin with high affinity, thereby promoting blood coagulation (Kowalska et al., 2010). By neutralizing the negatively charged heparan sulfate side chains of glycosaminoglycans on the surface of platelets and endothelial cells, PF4 facilitates platelet aggregation and thrombus formation. Its affinity for heparan sulfate induces the re-binding of soluble PF4 to the surface of platelets, pEVs, and monocytes (George and Onofre, 1982; Witt and Lander, 1994; Rauova et al., 2006, 2010). Beyond its hemostatic activity, PF4 is responsible for neutrophil recruitment to sites of inflammation/infection and strongly induces the formation of NETs, which are central mediators of COVID-19-associated coagulopathy (Middleton et al., 2020; Zuo et al., 2020).
PF4 shares its affinity for glycosaminoglycans with HMGB1, which is expressed and released by activated platelets (Rouhiainen et al., 2000; Maugeri et al., 2012; Vogel et al., 2015). HMGB1 interacts with heparin via two positively charged domains, box A and box B (Martinotti et al., 2015), and via its heparin-binding domain (Xu et al., 2011). HMGB1 plasma levels are elevated in conditions associated with abnormal coagulation, including sepsis (Eichhorn et al., 2021) and COVID-19, where HMGB1 has been correlated with disease severity (Chen et al., 2020). The biological functions of HMGB1 resemble those of activated platelets, including the induction of DNA externalization in neutrophils (Maugeri et al., 2014; Hoste et al., 2019) and microvascular thrombosis (Ito et al., 2007). HMGB1 signals through agonist receptors, such as the receptor for advanced glycation end products (RAGE) as well as other PRRs, including TLR2, TLR4 and TLR9 (Park et al., 2006, 2004). There is evidence that HMGB1-induced NET formation depends on the integrity of RAGE. Interaction of HMGB1 with heparin induces a conformational change and decreases its affinity for RAGE (Ling et al., 2011), abrogating the ability of activated platelets to elicit NET formation (Maugeri et al., 2014).
Beyond elevated levels of PF4 and HMGB1, patients suffering from severe COVID-19 display elevated markers of platelet activation including thromboxane A2 (Hottz et al., 2020; Zhang et al., 2020), surface expressed P-selectin (CD62P) and CD63 (Hottz et al., 2020; Manne et al., 2020; Nicolai et al., 2020; Taus et al., 2020; Zhang et al., 2020), activated glycoprotein (GP) IIb/IIIa (Bongiovanni et al., 2021; Léopold et al., 2021), as compared to healthy controls or to patients suffering from other pulmonary infections. Variable and partially conflicting results regarding the expression of individual platelet activation markers obtained in different studies may partly be due to the heterogeneity of COVID-19 patients. Furthermore, studies investigating single time points of platelet activation can merely provide snapshots of a highly dynamic process. As an example, increased platelet-monocyte aggregate formation has been described to trigger TF expression and immunothrombosis in critically ill COVID-19 patients (Hottz et al., 2020; Manne et al., 2020; Zaid et al., 2020), whereas other studies found diminished levels of circulating platelet-leukocyte aggregates in fatally ill patients, linked to a hypo-responsive platelet phenotype with impaired GPIIb/IIIa activation (Schrottmaier et al., 2021).
Platelet-Derived EVs in Inflammation and Coagulation
EVs are membrane-enclosed vesicles released by almost all cell types and present in all body fluids (Lötvall et al., 2014; Yáñez-Mó et al., 2015). Platelet-derived EVs are the most abundant EV subset in the circulation (Arraud et al., 2014; Berckmans et al., 2019), and their release is enhanced under pathological conditions, including sepsis (Raeven et al., 2018) and COVID-19 (Cappellano et al., 2021; Krishnamachary et al., 2021; Traby et al., 2022). They are most commonly characterized in blood or plasma samples using flow cytometry. Typically, phosphatidylserine exposed on EVs is detected by staining with Annexin 5 or lactadherin, while CD41 is used as a marker for platelet origin (Weiss et al., 2018; Tripisciano et al., 2020).
Platelet activation mediated by PRRs as described above, or through platelet agonists, such as thrombin, ADP, or collagen triggers the release of pEVs (Heijnen et al., 1999; Taus et al., 2019). The release of pEVs from plasma membrane is associated with actin cytoskeletal rearrangement and depends on GPIIb/IIIa (Heinzmann et al., 2020). EV release alters the phospholipid composition of the plasma membrane monolayers, leading to an exposure of phosphatidylserine on the outer membrane of the EVs (Ståhl et al., 2019).
It is well established that the properties of pEVs depend on the agonists triggering their release and on their environment. Activation of platelets with ADP, thrombin, collagen, or with a combination of thrombin and collagen induces different responses in terms of surface protein patterns and EV cargo (Milioli et al., 2015), entailing functional differences of the resulting EV populations. As an example, pEVs enriched from platelet-rich plasma under physiological conditions support tissue regeneration (Wu et al., 2021), while pEVs released under pathological conditions sustain coagulation and immunothrombosis (Zaid and Merhi, 2022).
Platelet-Derived EVs Provide a Large Pro-coagulant Surface
The exposure of phosphatidylserine on EVs results in the formation of a pro-coagulant surface (Nieuwland et al., 2000; Freyssinet and Toti, 2010). In fact, it has been estimated that the pro-coagulant activity of EVs is 50–100 fold higher than that of platelets (Sinauridze et al., 2007). Phosphatidylserine forms a catalytic, negatively charged surface, facilitating the formation of the tenase (factors VIIIa, IXa) and prothrombinase (factors Va, Xa) complexes of the coagulation cascade (Furie and Furie, 1992; Tripisciano et al., 2020). Beyond their ability to propagate coagulation by exposing phosphatidylserine, EVs may be initiators of coagulation via their exposure of TF. This is well-established for EVs derived from activated monocytes or endothelial cells (Nieuwland et al., 2000; Tripisciano et al., 2017; Hell et al., 2021), whereas TF expression on activated platelets and pEVs has long been controversial (Zillmann et al., 2001; Siddiqui et al., 2002; Panes et al., 2007; Bouchard et al., 2010, 2012; Camera et al., 2012; Østerud and Bouchard, 2019). Our own data do not support TF exposure on EVs released upon activation of platelets from medical grade platelet concentrates in vitro (Tripisciano et al., 2017). Still, TF might be transferred from monocytes to platelets in vivo in settings of inflammation. We and others have previously shown that pEVs shed from activated platelets preferentially bind to monocytes (Fendl et al., 2018; Weiss et al., 2018; Chimen et al., 2020) in the circulation. This binding is mediated by the interaction of P-selectin on pEVs and P-selectin glycoprotein ligand-1 (PSGL-1) on monocytes (Chimen et al., 2020). The P-selectin/PSGL-1 interaction enhances the exposure of TF on monocytes (Ivanov et al., 2019) and induces the release of TF-bearing monocyte EVs that can bind to activated platelets via P-selectin/PSGL-1 interaction (del Conde et al., 2005b). Increased EV-TF activity associated with severe COVID-19 has consistently been described by several groups (Hottz et al., 2020; Campbell et al., 2021b; Guervilly et al., 2021; Krishnamachary et al., 2021; Rosell et al., 2021). The assays used in these studies, however, did not further differentiate the cellular origin of the TF-exposing EVs.
Platelet-Derived EVs Expose and Release Mediators of Immunothrombosis
Next to their pro-coagulant surface, pEVs expose and release mediators supporting immunothrombosis (Puhm et al., 2020). Like platelets, pEVs carry PF4 and HMGB1 on their surface, partially due to re-binding of soluble PF4 and HMGB1 to the pEV surface. Elevated levels of PF4+ pEVs have been reported in sepsis (Sartori et al., 2020), and there is evidence that HMGB1+ pEVs are significantly elevated in COVID-19 (Maugeri et al., 2022). In addition, oxidation-specific epitopes generated by lipid peroxidation in settings of inflammation and cell death (Miller et al., 2011; Weismann and Binder, 2012; Binder et al., 2016) have been identified on EVs (Tsiantoulas et al., 2015) and can further enhance immunothrombosis by acting as DAMPs.
Platelet-Derived EVs Support NET Formation and Influence Other Immune Cells
Given that PF4 (Carestia et al., 2016) and HMGB1 (Denning et al., 2019) induce NET formation, PF4+ and HMGB1+ pEVs contribute to NET-associated coagulopathy. Moreover, pEVs have been directly associated with induction of NETosis in viral infection. Interaction of dengue virus with platelet CLEC2 was shown to trigger the release of pEVs, activating neutrophils through heterocomplexes of TLR2 and CLEC5A (Sung et al., 2019). EVs released from platelets after CLEC2 activation during SARS-CoV-2 infection might enhance neutrophil activation by a similar mechanism (Sung and Hsieh, 2021, 2019).
Furthermore, pEVs can propagate and spread platelet activation via the interaction of phosphatidylserine on EVs and CD36 exposed on platelets (Nergiz-Unal et al., 2011). As a scavenger receptor, CD36 recognizes oxidized phospholipids and lipoproteins, and participates in the internalization of apoptotic cells, certain bacterial and fungal pathogens, as well as modified low-density lipoproteins, and there is evidence that signaling following the interaction of phosphatidylserine and CD36 mediates a prothrombotic phenotype in platelets (Ghosh et al., 2008; Ramakrishnan et al., 2016).
Studies from our own group and others have further indicated that activated platelets and pEVs can shift the distribution of monocyte subsets towards intermediate CD14+CD16+ monocytes, which possess inflammatory characteristics (Passacquale et al., 2011; Fendl et al., 2019, 2021; Lee et al., 2021). The CD16 induction on monocytes appears to be triggered by platelet-derived transforming growth factor-beta and monocyte derived IL-6, suggesting an important role of activated platelets and pEVs in modulating phenotypical and functional features of human monocytes.
Platelet-Derived EVs May Support Viral Propagation
There are indications that pEVs can propagate infection through the delivery of functional viral RNA from cell to cell (Valadi et al., 2007), which has already been described for several viruses (Bello-Morales et al., 2020; Nomura et al., 2020). Since SARS-CoV-2 RNA has been detected by reverse transcription-droplet digital polymerase chain reaction in exosomes isolated from plasma from COVID-19 patients (Barberis et al., 2021), it is conceivable that EVs might be involved in viral spreading.
Therapeutic Potential of Platelet-Derived EV Depletion During COVID-19
Extracorporeal blood purification has been proposed as a supportive measure for the treatment of COVID-19 (Rock et al., 2021; Ronco et al., 2021). Given the central role of immunothrombosis, removing mediators of excessive cellular activation from the circulation may indeed be beneficial (Morris et al., 2021; Tang L. et al., 2020).
Potential extracorporeal approaches include plasma exchange (Rock et al., 2021), depletion of cytokines using polystyrene based adsorbents (Nassiri et al., 2021; Ruiz-Rodríguez et al., 2021), as well as hemadsorption using beads functionalized with heparin. The Seraph-100 Microbind Affinity Blood Filter consists of ultra-high molecular weight polyethylene beads with endpoint-attached heparin (Seffer et al., 2021). It has been developed following a biomimetic approach with the assumption that bacterial as well as viral pathogens bind to immobilized heparin in the same way as they interact with cellular heparan sulfate. In fact, heparan sulfate is an essential cofactor in SARS-CoV-2 infection, as it modifies the conformation of the spike protein to facilitate its recognition by ACE2 (Clausen et al., 2020).
So far, Seraph-100 has been exploited for its ability to deplete carbapenem-resistant Enterobacteriaceae in vitro, where it yielded promising results (McCrea et al., 2014). First clinical case reports on the capacity of the Seraph-100 Microbind Affinity Blood Filter to eliminate Staphylococcus aureus from the circulation have been published recently (Seffer et al., 2020). There is evidence that the viral load is associated with increased disease severity and mortality in COVID-19 (Fajnzylber et al., 2020), and that heparan sulfate is a co-factor for viral entry, as discussed further above (Kalra and Kandimalla, 2021; Tandon et al., 2021). It was therefore obvious to consider the application of Seraph-100 as a supportive therapy in COVID-19. The Seraph-100 Microbind Affinity Blood Filter obtained emergency use authorization for COVID-19 by the FDA in 2020, followed by a case series assessing its use in SARS-CoV-2 infected patients early in critical illness (Rifkin and Stewart, 2022). This study however did not collect data on virus elimination from the circulation. A follow-up study provided evidence that treatment with Seraph-100 decreased the SARS-CoV-2 nucleocapsid protein in critically ill patients (Kielstein et al., 2021), while effects on clinically relevant outcome parameters remain to be further assessed. A recently published interim analysis of a multicenter observational study in 12 hospitals monitoring 102 treatment sessions in 82 patients confirmed that the treatment was well tolerated. Mortality was correlated with late initiation of the treatment as well as with bacterial super-infection (Schmidt et al., 2022). Although the efficacy of this approach has yet to be consistently demonstrated, current data suggest that it can be deployed as an adjunct measure until directed pharmacologic countermeasures are available (Chitty et al., 2022).
While this extracorporeal approach is currently mainly explored regarding pathogen depletion, we have provided evidence that heparin-immobilized adsorbents may exert beneficial effects by binding and depleting mediators of immunothrombosis, including activated platelets and PF4+ pEVs, as well as HMGB1 and histones/nucleosomes (Ebeyer-Masotta et al., 2022), as suggested in Figure 2, and we are currently assessing the ability of heparin-functionalized adsorbents to deplete NETs.
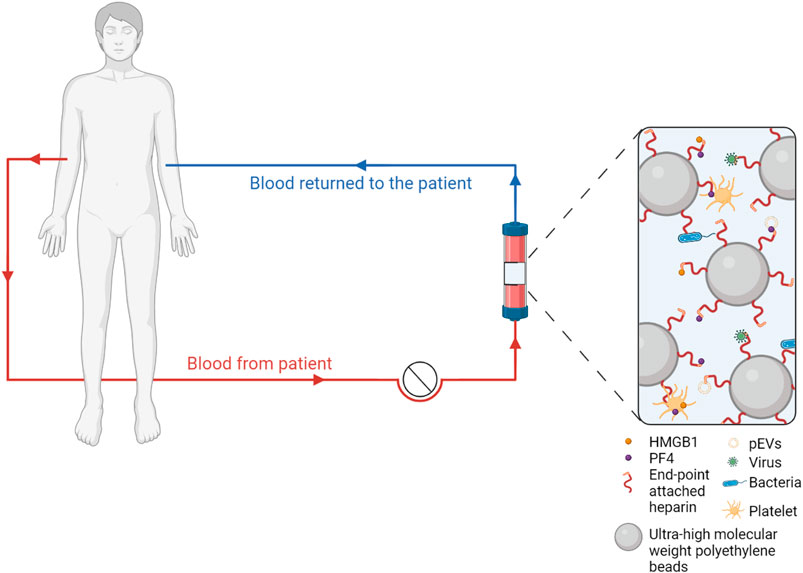
FIGURE 2. Extracorporeal blood purification with heparin-functionalized adsorbents. Human whole blood is recirculated over adsorbent columns containing beads functionalized with endpoint-attached heparin, which binds pathogens as well as mediators of immunothrombosis, including activated platelets, platelet-derived extracellular vesicles (EVs), platelet factor 4 (PF4) and high mobility group box 1 protein (HMGB1).
Conclusion
Platelets are sentinels of viral infection and can propagate immunothrombosis at multiple levels, e.g., by the release or exposure of mediators, by providing pro-coagulant surfaces, by inducing a shift towards inflammatory monocyte subsets, and by contributing to viral spread via EVs. Activated platelets and pEVs are markers and central mediators of immunothrombosis in COVID-19 (Puhm et al., 2021). Adverse outcome in COVID-19 patients appears to be linked to increased basal platelet activation and diminished platelet reactivity, which aggravates over the course of the disease.
Both, cellular heparan sulfate and exogenous heparin interact with activated platelets, pEVs, and with various mediators of immunothrombosis in many ways, and thus both, extracorporeal therapy with immobilized heparin and administration of heparin may provide approaches to alleviate excessive immunothrombosis.
Author Contributions
VW and ME-M wrote the manuscript; ME-M designed the figures using BioRender.com; TE, RW, and LL contributed to the manuscript by critical discussion and by providing feedback on the draft.
Funding
ME-M and TE received funding from the Lower Austrian Society for Research Funding Organization (FTI Call, project ID K3-F-744/005-2019). Open Access funding was provided by the University for Continuing Education Krems.
Conflict of Interest
The authors declare that the research was conducted in the absence of any commercial or financial relationships that could be construed as a potential conflict of interest.
Publisher’s Note
All claims expressed in this article are solely those of the authors and do not necessarily represent those of their affiliated organizations, or those of the publisher, the editors and the reviewers. Any product that may be evaluated in this article, or claim that may be made by its manufacturer, is not guaranteed or endorsed by the publisher.
References
Ackermann, M., Verleden, S. E., Kuehnel, M., Haverich, A., Welte, T., Laenger, F., et al. (2020). Pulmonary Vascular Endothelialitis, Thrombosis, and Angiogenesis in Covid-19. N. Engl. J. Med. 383, 120–128. doi:10.1056/NEJMoa2015432
Anabel, A.-S., Eduardo, P.-C., Pedro Antonio, H.-C., Carlos, S.-M., Juana, N.-M., Honorio, T.-A., et al. (2014). Human Platelets Express Toll-like Receptor 3 and Respond to Poly I:C. Hum. Immunol. 75, 1244–1251. doi:10.1016/j.humimm.2014.09.013
Arraud, N., Linares, R., Tan, S., Gounou, C., Pasquet, J.-M., Mornet, S., et al. (2014). Extracellular Vesicles from Blood Plasma: Determination of Their Morphology, Size, Phenotype and Concentration. J. Thromb. Haemost. 12, 614–627. doi:10.1111/jth.12554
Aslam, R., Speck, E. R., Kim, M., Crow, A. R., Bang, K. W. A., Nestel, F. P., et al. (2006). Platelet Toll-like Receptor Expression Modulates Lipopolysaccharide-Induced Thrombocytopenia and Tumor Necrosis Factor-α Production In Vivo. Blood 107, 637–641. doi:10.1182/blood-2005-06-2202
Azkur, A. K., Akdis, M., Azkur, D., Sokolowska, M., Veen, W., Brüggen, M. C., et al. (2020). Immune Response to SARS‐CoV‐2 and Mechanisms of Immunopathological Changes in COVID‐19. Allergy 75, 1564–1581. doi:10.1111/all.14364
Banerjee, M., Huang, Y., Joshi, S., Popa, G. J., Mendenhall, M. D., Wang, Q. J., et al. (2020). Platelets Endocytose Viral Particles and Are Activated via TLR (Toll-like Receptor) Signaling. Arterioscler. Thromb. Vasc. Biol. 40, 1635–1650. doi:10.1161/ATVBAHA.120.314180
Barberis, E., Vanella, V. V., Falasca, M., Caneapero, V., Cappellano, G., Raineri, D., et al. (2021). Circulating Exosomes Are Strongly Involved in SARS-CoV-2 Infection. Front. Mol. Biosci. 8, 632290. doi:10.3389/fmolb.2021.632290
Bello-Morales, R., Ripa, I., and López-Guerrero, J. A. (2020). Extracellular Vesicles in Viral Spread and Antiviral Response. Viruses 12, 623. doi:10.3390/v12060623
Berckmans, R. J., Lacroix, R., Hau, C. M., Sturk, A., and Nieuwland, R. (2019). Extracellular Vesicles and Coagulation in Blood from Healthy Humans Revisited. J. Extracell. Vesicles 8, 1688936. doi:10.1080/20013078.2019.1688936
Binder, C. J., Papac-Milicevic, N., and Witztum, J. L. (2016). Innate Sensing of Oxidation-specific Epitopes in Health and Disease. Nat. Rev. Immunol. 16, 485–497. doi:10.1038/nri.2016.63
Bonaventura, A., Vecchié, A., Dagna, L., Martinod, K., Dixon, D. L., Van Tassell, B. W., et al. (2021). Endothelial Dysfunction and Immunothrombosis as Key Pathogenic Mechanisms in COVID-19. Nat. Rev. Immunol. 21, 319–329. doi:10.1038/s41577-021-00536-9
Bongiovanni, D., Klug, M., Lazareva, O., Weidlich, S., Biasi, M., Ursu, S., et al. (2021). SARS-CoV-2 Infection Is Associated with a Pro-thrombotic Platelet Phenotype. Cell Death Dis. 12, 50. doi:10.1038/s41419-020-03333-9
Bouchard, B. A., Krudysz-Amblo, J., and Butenas, S. (2012). Platelet Tissue Factor Is Not Expressed Transiently after Platelet Activation. Blood 119, 4338–4339. doi:10.1182/blood-2012-01-403469
Bouchard, B. A., Mann, K. G., and Butenas, S. (2010). No Evidence for Tissue Factor on Platelets. Blood 116, 854–855. doi:10.1182/blood-2010-05-285627
Brinkmann, V., Reichard, U., Goosmann, C., Fauler, B., Uhlemann, Y., Weiss, D. S., et al. (2004). Neutrophil Extracellular Traps Kill Bacteria. Science 303, 1532–1535. doi:10.1126/science.1092385
Camera, M., Brambilla, M., Boselli, D., Facchinetti, L., Canzano, P., Rossetti, L., et al. (2012). Response: Functionally Active Platelets Do Express Tissue Factor. Blood 119, 4339–4341. doi:10.1182/blood-2012-02-410043
Campbell, R. A., Boilard, E., and Rondina, M. T. (2021a). Is There a Role for the ACE2 Receptor in SARS‐CoV‐2 Interactions with Platelets? J. Thromb. Haemost. 19, 46–50. doi:10.1111/jth.15156
Campbell, R. A., Hisada, Y., Denorme, F., Grover, S. P., Bouck, E. G., Middleton, E. A., et al. (2021b). Comparison of the Coagulopathies Associated with COVID‐19 and Sepsis. Res. Pract. Thromb. Haemost. 5, e12525. doi:10.1002/rth2.12525
Cappellano, G., Raineri, D., Rolla, R., Giordano, M., Puricelli, C., Vilardo, B., et al. (2021). Circulating Platelet-Derived Extracellular Vesicles Are a Hallmark of Sars-Cov-2 Infection. Cells 10, 85. doi:10.3390/cells10010085
Carestia, A., Kaufman, T., Rivadeneyra, L., Landoni, V. I., Pozner, R. G., Negrotto, S., et al. (2016). Mediators and Molecular Pathways Involved in the Regulation of Neutrophil Extracellular Trap Formation Mediated by Activated Platelets. J. Leukoc. Biol. 99, 153–162. doi:10.1189/jlb.3A0415-161R
Chaipan, C., Soilleux, E. J., Simpson, P., Hofmann, H., Gramberg, T., Marzi, A., et al. (2006). DC-SIGN and CLEC-2 Mediate Human Immunodeficiency Virus Type 1 Capture by Platelets. J. Virol. 80, 8951–8960. doi:10.1128/JVI.00136-06
Chen, R., Huang, Y., Quan, J., Liu, J., Wang, H., Billiar, T. R., et al. (2020). HMGB1 as a Potential Biomarker and Therapeutic Target for Severe COVID-19. Heliyon 6, e05672. doi:10.1016/j.heliyon.2020.e05672
Chen, S.-T., Li, F.-J., Hsu, T.-y., Liang, S.-M., Yeh, Y.-C., Liao, W.-Y., et al. (2017). CLEC5A Is a Critical Receptor in Innate Immunity against Listeria Infection. Nat. Commun. 8, 299. doi:10.1038/s41467-017-00356-3
Chimen, M., Evryviadou, A., Box, C. L., Harrison, M. J., Hazeldine, J., Dib, L. H., et al. (2020). Appropriation of GPIbα from Platelet-Derived Extracellular Vesicles Supports Monocyte Recruitment in Systemic Inflammation. Haematologica 105, 1248–1261. doi:10.3324/haematol.2018.215145
Chitty, S. A., Mobbs, S., Rifkin, B. S., Stogner, S. W., Lewis, M. S., Betancourt, J., et al. (2022). For the PURIFY InvestigatorsA Multicenter Evaluation of the Seraph 100 Microbind Affinity Blood Filter for the Treatment of Severe COVID-19. Crit. Care Explor. 4, e0662. doi:10.1097/CCE.0000000000000662
Clark, S. R., Ma, A. C., Tavener, S. A., McDonald, B., Goodarzi, Z., Kelly, M. M., et al. (2007). Platelet TLR4 Activates Neutrophil Extracellular Traps to Ensnare Bacteria in Septic Blood. Nat. Med. 13, 463–469. doi:10.1038/nm1565
Clausen, T. M., Sandoval, D. R., Spliid, C. B., Pihl, J., Perrett, H. R., Painter, C. D., et al. (2020). SARS-CoV-2 Infection Depends on Cellular Heparan Sulfate and ACE2. Cell 183, 1043–1057. e15. doi:10.1016/j.cell.2020.09.033
Claushuis, T. A. M., Van Der Veen, A. I. P., Horn, J., Schultz, M. J., Houtkooper, R. H., Van ’T Veer, C., et al. (2019). Platelet Toll-like Receptor Expression and Activation Induced by Lipopolysaccharide and Sepsis. Platelets 30, 296–304. doi:10.1080/09537104.2018.1445841
Cognasse, F., Duchez, A. C., Audoux, E., Ebermeyer, T., Arthaud, C. A., Prier, A., et al. (2022). Platelets as Key Factors in Inflammation: Focus on CD40L/CD40. Front. Immunol. 13, 825892. doi:10.3389/fimmu.2022.825892
Cognasse, F., Hamzeh, H., Chavarin, P., Acquart, S., Genin, C., and Garraud, O. (2005). Evidence of Toll‐like Receptor Molecules on Human Platelets. Immunol. Cell Biol. 83, 196–198. doi:10.1111/j.1440-1711.2005.01314.x
Comer, S. P., Cullivan, S., Szklanna, P. B., Weiss, L., Cullen, S., Kelliher, S., et al. (2021). COVID-19 Induces a Hyperactive Phenotype in Circulating Platelets. PLOS Biol. 19, e3001109. doi:10.1371/journal.pbio.3001109
Connors, J. M., and Levy, J. H. (2020). COVID-19 and its Implications for Thrombosis and Anticoagulation. Blood 135, 2033–2040. doi:10.1182/blood.2020006000
del Conde, I., Crúz, M. A., Zhang, H., López, J. A., and Afshar-Kharghan, V. (2005a). Platelet Activation Leads to Activation and Propagation of the Complement System. J. Exp. Med. 201, 871–879. doi:10.1084/jem.20041497
del Conde, I., Shrimpton, C. N., Thiagarajan, P., and López, J. A. (2005b). Tissue-factor-bearing Microvesicles Arise from Lipid Rafts and Fuse with Activated Platelets to Initiate Coagulation. Blood 106, 1604–1611. doi:10.1182/blood-2004-03-1095
Denning, N.-L., Aziz, M., Gurien, S. D., and Wang, P. (2019). DAMPs and NETs in Sepsis. Front. Immunol. 10, 2536. doi:10.3389/fimmu.2019.02536
Domínguez-Martínez, D. A., Núñez-Avellaneda, D., Castañón-Sánchez, C. A., and Salazar, M. I. (2018). NOD2: Activation during Bacterial and Viral Infections, Polymorphisms and Potential as Therapeutic Target. Rev. Investig Clin. 70, 18–28. doi:10.24875/RIC.17002327
D’ Atri, L. P., and Schattner, M. (2017). Platelet Toll-Like Receptors in Thromboinflammation. Front. Biosci. 22, 1867–1883. doi:10.2741/4576
Ebeyer-Masotta, M., Eichhorn, T., Weiss, R., Semak, V., Lauková, L., Fischer, M. B., et al. (2022). Heparin-Functionalized Adsorbents Eliminate Central Effectors of Immunothrombosis, Including Platelet Factor 4, High-Mobility Group Box 1 Protein and Histones. Int. J. Mol. Sci. 23, 1823. doi:10.3390/ijms23031823
Eichhorn, T., Linsberger, I., Lauková, L., Tripisciano, C., Fendl, B., Weiss, R., et al. (2021). Analysis of Inflammatory Mediator Profiles in Sepsis Patients Reveals that Extracellular Histones Are Strongly Elevated in Nonsurvivors. Mediat. Inflamm. 2021, 1–13. doi:10.1155/2021/8395048
Elrobaa, I. H., and New, K. J. (2021). COVID-19: Pulmonary and Extra Pulmonary Manifestations. Front. Public Health 9, 711616. doi:10.3389/fpubh.2021.711616
Engelmann, B., and Massberg, S. (2013). Thrombosis as an Intravascular Effector of Innate Immunity. Nat. Rev. Immunol. 13, 34–45. doi:10.1038/nri3345
Fajnzylber, J., Regan, J., Regan, J., Coxen, K., Corry, H., Wong, C., et al. (2020). SARS-CoV-2 Viral Load Is Associated with Increased Disease Severity and Mortality. Nat. Commun. 11, 5493. doi:10.1038/s41467-020-19057-5
Fara, A., Mitrev, Z., Rosalia, R. A., and Assas, B. M. (2020). Cytokine Storm and COVID-19: a Chronicle of Pro-inflammatory Cytokines. Open Biol. 10, 200160. doi:10.1098/rsob.200160
Fard, M. B., Fard, S. B., Ramazi, S., Atashi, A., and Eslamifar, Z. (2021). Thrombosis in COVID-19 Infection: Role of Platelet Activation-Mediated Immunity. Thromb. J. 19, 59. doi:10.1186/s12959-021-00311-9
Fendl, B., Eichhorn, T., Weiss, R., Tripisciano, C., Spittler, A., Fischer, M. B., et al. (2018). Differential Interaction of Platelet-Derived Extracellular Vesicles with Circulating Immune Cells: Roles of TAM Receptors, CD11b, and Phosphatidylserine. Front. Immunol. 9, 2797. doi:10.3389/fimmu.2018.02797
Fendl, B., Weiss, R., Eichhorn, T., Linsberger, I., Afonyushkin, T., Puhm, F., et al. (2021). Extracellular Vesicles Are Associated with C-Reactive Protein in Sepsis. Sci. Rep. 11, 6996. doi:10.1038/s41598-021-86489-4
Fendl, B., Weiss, R., Eichhorn, T., Spittler, A., Fischer, M. B., and Weber, V. (2019). Storage of Human Whole Blood, but Not Isolated Monocytes, Preserves the Distribution of Monocyte Subsets. Biochem. Biophys. Res. Commun. 517, 709–714. doi:10.1016/j.bbrc.2019.07.120
Flier, J. S., Underhill, L. H., Furie, B., and Furie, B. C. (1992). Molecular and Cellular Biology of Blood Coagulation. N. Engl. J. Med. 326, 800–806. doi:10.1056/NEJM199203193261205
Foley, J. H., and Conway, E. M. (2016). Cross Talk Pathways between Coagulation and Inflammation. Circ. Res. 118, 1392–1408. doi:10.1161/CIRCRESAHA.116.306853
Freyssinet, J.-M., and Toti, F. (2010). Formation of Procoagulant Microparticles and Properties. Thrombosis Res. 125, S46–S48. doi:10.1016/j.thromres.2010.01.036
Gadanec, L. K., McSweeney, K. R., Qaradakhi, T., Ali, B., Zulli, A., and Apostolopoulos, V. (2021). Can SARS-CoV-2 Virus Use Multiple Receptors to Enter Host Cells? Int. J. Mol. Sci. 22, 992. doi:10.3390/ijms22030992
Garcia-Romo, G. S., Caielli, S., Vega, B., Connolly, J., Allantaz, F., Xu, Z., et al. (2011). Netting Neutrophils Are Major Inducers of Type I IFN Production in Pediatric Systemic Lupus Erythematosus. Sci. Transl. Med. 3, 73ra20. doi:10.1126/scitranslmed.3001201
George, J., and Onofre, A. (1982). Human Platelet Surface Binding of Endogenous Secreted Factor VIII-Von Willebrand Factor and Platelet Factor 4. Blood 59, 194–197. doi:10.1182/blood.V59.1.194.194
Ghosh, A., Li, W., Febbraio, M., Espinola, R. G., McCrae, K. R., Cockrell, E., et al. (2008). Platelet CD36 Mediates Interactions with Endothelial Cell-Derived Microparticles and Contributes to Thrombosis in Mice. J. Clin. Invest. 118, 1934–1943. doi:10.1172/JCI34904
Ghoshal, K., and Bhattacharyya, M. (2014). Overview of Platelet Physiology: Its Hemostatic and Nonhemostatic Role in Disease Pathogenesis. Sci. World J. 2014, 1–16. doi:10.1155/2014/781857
Guervilly, C., Bonifay, A., Burtey, S., Sabatier, F., Cauchois, R., Abdili, E., et al. (2021). Dissemination of Extreme Levels of Extracellular Vesicles: Tissue Factor Activity in Patients with Severe COVID-19. Blood Adv. 5, 628–634. doi:10.1182/bloodadvances.2020003308
Gupta, A., Madhavan, M. V., Sehgal, K., Nair, N., Mahajan, S., Sehrawat, T. S., et al. (2020). Extrapulmonary Manifestations of COVID-19. Nat. Med. 26, 1017–1032. doi:10.1038/s41591-020-0968-3
Heijnen, H. F. G., Schiel, A. E., Fijnheer, R., Geuze, H. J., and Sixma, J. J. (1999). Activated Platelets Release Two Types of Membrane Vesicles: Microvesicles by Surface Shedding and Exosomes Derived from Exocytosis of Multivesicular Bodies and õ-Granules. Blood 94, 3791–3799. doi:10.1182/blood.v94.11.3791.423a22_3791_3799
Heinzmann, A. C. A., Karel, M. F. A., Coenen, D. M., Vajen, T., Meulendijks, N. M. M., Nagy, M., et al. (2020). Complementary Roles of Platelet αIIbβ3 Integrin, Phosphatidylserine Exposure and Cytoskeletal Rearrangement in the Release of Extracellular Vesicles. Atherosclerosis 310, 17–25. doi:10.1016/j.atherosclerosis.2020.07.015
Hell, L., Däullary, T., Burghart, V., Mauracher, L.-M., Grilz, E., Moser, B., et al. (2021). Extracellular Vesicle-Associated Tissue Factor Activity in Prostate Cancer Patients with Disseminated Intravascular Coagulation. Cancers 13, 1487. doi:10.3390/cancers13071487
Helms, J., Tacquard, C., Tacquard, C., Severac, F., Leonard-Lorant, I., Ohana, M., et al. (2020). High Risk of Thrombosis in Patients with Severe SARS-CoV-2 Infection: a Multicenter Prospective Cohort Study. Intensive Care Med. 46, 1089–1098. doi:10.1007/s00134-020-06062-x
Hoste, E., Maueröder, C., van Hove, L., Catrysse, L., Vikkula, H.-K., Sze, M., et al. (2019). Epithelial HMGB1 Delays Skin Wound Healing and Drives Tumor Initiation by Priming Neutrophils for NET Formation. Cell Rep. 29, 2689–2701. e4. doi:10.1016/j.celrep.2019.10.104
Hottz, E. D., Azevedo-Quintanilha, I. G., Palhinha, L., Teixeira, L., Barreto, E. A., Pão, C. R. R., et al. (2020). Platelet Activation and Platelet-Monocyte Aggregate Formation Trigger Tissue Factor Expression in Patients with Severe COVID-19. Blood 136, 1330–1341. doi:10.1182/blood.2020007252
Hottz, E. D., Bozza, F. A., and Bozza, P. T. (2018). Platelets in Immune Response to Virus and Immunopathology of Viral Infections. Front. Med. 5, 121. doi:10.3389/fmed.2018.00121
Hottz, E. D., Lopes, J. F., Freitas, C., Valls-de-Souza, R., Oliveira, M. F., Bozza, M. T., et al. (2013). Platelets Mediate Increased Endothelium Permeability in Dengue through NLRP3-Inflammasome Activation. Blood 122, 3405–3414. doi:10.1182/blood-2013-05-504449
Hottz, E. D., Monteiro, A. P. T., Bozza, F. A., and Bozza, P. T. (2015). Inflammasome in Platelets: Allying Coagulation and Inflammation in Infectious and Sterile Diseases? Mediat. Inflamm. 2015, 1–7. doi:10.1155/2015/435783
Hu, B., Guo, H., Zhou, P., and Shi, Z.-L. (2021). Characteristics of SARS-CoV-2 and COVID-19. Nat. Rev. Microbiol. 19, 141–154. doi:10.1038/s41579-020-00459-7
Huang, C., Wang, Y., Li, X., Ren, L., Zhao, J., Hu, Y., et al. (2020). Clinical Features of Patients Infected with 2019 Novel Coronavirus in Wuhan, China. Lancet 395, 497–506. doi:10.1016/S0140-6736(20)30183-5
Iba, T., Levy, J. H., Levi, M., Connors, J. M., and Thachil, J. (2020). Coagulopathy of Coronavirus Disease 2019. Crit. Care Med. 48, 1358–1364. doi:10.1097/CCM.0000000000004458
Ito, T., Kawahara, K., Nakamura, T., Yamada, S., Nakamura, T., Abeyama, K., et al. (2007). High-mobility Group Box 1 Protein Promotes Development of Microvascular Thrombosis in Rats. J. Thromb. Haemost. 5, 109–116. doi:10.1111/j.1538-7836.2006.02255.x
Ivanov, I. I., Apta, B. H. R., Bonna, A. M., and Harper, M. T. (2019). Platelet P-Selectin Triggers Rapid Surface Exposure of Tissue Factor in Monocytes. Sci. Rep. 9, 13397. doi:10.1038/s41598-019-49635-7
Kalra, R. S., and Kandimalla, R. (2021). Engaging the Spikes: Heparan Sulfate Facilitates SARS-CoV-2 Spike Protein Binding to ACE2 and Potentiates Viral Infection. Sig Transduct. Target Ther. 6, 39. doi:10.1038/s41392-021-00470-1
Khan, S., Shafiei, M. S., Longoria, C., Schoggins, J. W., Savani, R. C., and Zaki, H. (2021). SARS-CoV-2 Spike Protein Induces Inflammation via TLR2-dependent Activation of the NF-Κb Pathway. eLife 10, e68563. doi:10.7554/eLife.68563
Kielstein, J. T., Borchina, D.-N., Fühner, T., Hwang, S., Mattoon, D., and Ball, A. J. (2021). Hemofiltration with the Seraph 100 Microbind Affinity Filter Decreases SARS-CoV-2 Nucleocapsid Protein in Critically Ill COVID-19 Patients. Crit. Care 25, 190. doi:10.1186/s13054-021-03597-3
Klok, F. A., Kruip, M. J. H. A., van der Meer, N. J. M., Arbous, M. S., Gommers, D. A. M. P. J., Kant, K. M., et al. (2020). Incidence of Thrombotic Complications in Critically Ill ICU Patients with COVID-19. Thrombosis Res. 191, 145–147. doi:10.1016/j.thromres.2020.04.013
Koupenova, M., Corkrey, H. A., Vitseva, O., Tanriverdi, K., Somasundaran, M., Liu, P., et al. (2021). SARS-CoV-2 Initiates Programmed Cell Death in Platelets. Circ. Res. 129, 631–646. doi:10.1161/CIRCRESAHA.121.319117
Koupenova, M., Mick, E., Mikhalev, E., Benjamin, E. J., Tanriverdi, K., and Freedman, J. E. (2015). Sex Differences in Platelet Toll-like Receptors and Their Association with Cardiovascular Risk Factors. Arterioscler. Thromb. Vasc. Biol. 35, 1030–1037. doi:10.1161/ATVBAHA.114.304954
Koupenova, M., Vitseva, O., MacKay, C. R., Beaulieu, L. M., Benjamin, E. J., Mick, E., et al. (2014). Platelet-TLR7 Mediates Host Survival and Platelet Count during Viral Infection in the Absence of Platelet-dependent Thrombosis. Blood 124, 791–802. doi:10.1182/blood-2013-11-536003
Kowalska, M. A., Rauova, L., and Poncz, M. (2010). Role of the Platelet Chemokine Platelet Factor 4 (PF4) in Hemostasis and Thrombosis. Thrombosis Res. 125, 292–296. doi:10.1016/j.thromres.2009.11.023
Krishnamachary, B., Cook, C., Kumar, A., Spikes, L., Chalise, P., and Dhillon, N. K. (2021). Extracellular Vesicle‐mediated Endothelial Apoptosis and EV‐associated Proteins Correlate with COVID‐19 Disease Severity. J. Extracell. Vesicles 10, e12117. doi:10.1002/jev2.12117
Lan, J., Ge, J., Yu, J., Shan, S., Zhou, H., Fan, S., et al. (2020). Structure of the SARS-CoV-2 Spike Receptor-Binding Domain Bound to the ACE2 Receptor. Nature 581, 215–220. doi:10.1038/s41586-020-2180-5
Lee, S. J., Yoon, B. R., Kim, H. Y., Yoo, S.-J., Kang, S. W., and Lee, W.-W. (2021). Activated Platelets Convert CD14+CD16- into CD14+CD16+ Monocytes with Enhanced FcγR-Mediated Phagocytosis and Skewed M2 Polarization. Front. Immunol. 11, 611133. doi:10.3389/fimmu.2020.611133
Léopold, V., Pereverzeva, L., Schuurman, A. R., Reijnders, T. D. Y., Saris, A., de Brabander, J., et al. (2021). Platelets Are Hyperactivated but Show Reduced Glycoprotein VI Reactivity in COVID-19 Patients. Thromb. Haemost. 121, 1258–1262. doi:10.1055/a-1347-5555
Leroy, J., Bortolus, C., Lecointe, K., Parny, M., Charlet, R., Sendid, B., et al. (2019). Fungal Chitin Reduces Platelet Activation Mediated via TLR8 Stimulation. Front. Cell. Infect. Microbiol. 9, 383. doi:10.3389/fcimb.2019.00383
Li, T., Yang, Y., Li, Y., Wang, Z., Ma, F., Luo, R., et al. (2022). Platelets Mediate Inflammatory Monocyte Activation by SARS-CoV-2 Spike Protein. J. Clin. Invest. 132, e150101. doi:10.1172/JCI150101
Li, T., Zhang, Z., Li, X., Dong, G., Zhang, M., Xu, Z., et al. (2020). Neutrophil Extracellular Traps: Signaling Properties and Disease Relevance. Mediat. Inflamm. 2020, 1–14. doi:10.1155/2020/9254087
Lievens, D., Zernecke, A., Seijkens, T., Soehnlein, O., Beckers, L., Munnix, I. C. A., et al. (2010). Platelet CD40L Mediates Thrombotic and Inflammatory Processes in Atherosclerosis. Blood 116, 4317–4327. doi:10.1182/blood-2010-01-261206
Lindmark, E., Tenno, T., and Siegbahn, A. (2000). Role of Platelet P-Selectin and CD40 Ligand in the Induction of Monocytic Tissue Factor Expression. Arterioscler. Thromb. Vasc. Biol. 20, 2322–2328. doi:10.1161/01.ATV.20.10.2322
Ling, Y., Yang, Z.-Y., Yin, T., Li, L., Yuan, W.-W., Wu, H.-S., et al. (2011). Heparin Changes the Conformation of High-Mobility Group Protein 1 and Decreases its Affinity toward Receptor for Advanced Glycation Endproducts In Vitro. Int. Immunopharmacol. 11, 187–193. doi:10.1016/j.intimp.2010.11.014
Lopes-Pacheco, M., Silva, P. L., Cruz, F. F., Battaglini, D., Robba, C., Pelosi, P., et al. (2021). Pathogenesis of Multiple Organ Injury in COVID-19 and Potential Therapeutic Strategies. Front. Physiol. 12, 593223. doi:10.3389/fphys.2021.593223
Lötvall, J., Hill, A. F., Hochberg, F., Buzás, E. I., Di Vizio, D., Gardiner, C., et al. (2014). Minimal Experimental Requirements for Definition of Extracellular Vesicles and Their Functions: a Position Statement from the International Society for Extracellular Vesicles. J. Extracell. Vesicles 3, 26913. doi:10.3402/jev.v3.26913
Maharaj, S., and Chang, S. (2018). Anti-PF4/heparin Antibodies Are Increased in Hospitalized Patients with Bacterial Sepsis. Thrombosis Res. 171, 111–113. doi:10.1016/j.thromres.2018.09.060
Manne, B. K., Denorme, F., Middleton, E. A., Portier, I., Rowley, J. W., Stubben, C., et al. (2020). Platelet Gene Expression and Function in Patients with COVID-19. Blood 136, 1317–1329. doi:10.1182/blood.2020007214
Marín Oyarzún, C. P., Glembotsky, A. C., Goette, N. P., Lev, P. R., De Luca, G., Baroni Pietto, M. C., et al. (2020). Platelet Toll-like Receptors Mediate Thromboinflammatory Responses in Patients with Essential Thrombocythemia. Front. Immunol. 11, 705. doi:10.3389/fimmu.2020.00705
Martinod, K., and Deppermann, C. (2021). Immunothrombosis and Thromboinflammation in Host Defense and Disease. Platelets 32, 314–324. doi:10.1080/09537104.2020.1817360
Martinotti, S., Patrone, M., and Ranzato, E. (2015). Emerging Roles for HMGB1 Protein in Immunity, Inflammation, and Cancer. ImmunoTargets Ther. 4, 101–109. doi:10.2147/ITT.S58064
Masters, P. S. (2006). The Molecular Biology of Coronaviruses. Adv. Virus Res. 66, 193–292. doi:10.1016/S0065-3527(06)66005-3
Maugeri, N., Campana, L., Gavina, M., Covino, C., De Metrio, M., Panciroli, C., et al. (2014). Activated Platelets Present High Mobility Group Box 1 to Neutrophils, Inducing Autophagy and Promoting the Extrusion of Neutrophil Extracellular Traps. J. Thromb. Haemost. 12, 2074–2088. doi:10.1111/jth.12710
Maugeri, N., De Lorenzo, R., Clementi, N., Antonia Diotti, R., Criscuolo, E., Godino, C., et al. (2022). Unconventional CD147‐dependent Platelet Activation Elicited by SARS‐CoV‐2 in COVID‐19. J Thrombosis Haemost 20, 434–448. doi:10.1111/jth.15575
Maugeri, N., Franchini, S., Campana, L., Baldini, M., Ramirez, G. A., Sabbadini, M. G., et al. (2012). Circulating Platelets as a Source of the Damage-Associated Molecular Pattern HMGB1 in Patients with Systemic Sclerosis. Autoimmunity 45, 584–587. doi:10.3109/08916934.2012.719946
McCrea, K., Ward, R., and LaRosa, S. P. (2014). Removal of Carbapenem-Resistant Enterobacteriaceae (CRE) from Blood by Heparin-Functional Hemoperfusion Media. PLoS ONE 9, e114242. doi:10.1371/journal.pone.0114242
McFadyen, J. D., Stevens, H., and Peter, K. (2020). The Emerging Threat of (Micro)Thrombosis in COVID-19 and its Therapeutic Implications. Circ. Res. 127, 571–587. doi:10.1161/CIRCRESAHA.120.317447
Meng, D., Luo, M., and Liu, B. (2021). The Role of CLEC-2 and its Ligands in Thromboinflammation. Front. Immunol. 12, 688643. doi:10.3389/fimmu.2021.688643
Middeldorp, S., Coppens, M., Haaps, T. F., Foppen, M., Vlaar, A. P., Müller, M. C. A., et al. (2020). Incidence of Venous Thromboembolism in Hospitalized Patients with COVID‐19. J. Thromb. Haemost. 18, 1995–2002. doi:10.1111/jth.14888
Middleton, E. A., He, X.-Y., Denorme, F., Campbell, R. A., Ng, D., Salvatore, S. P., et al. (2020). Neutrophil Extracellular Traps Contribute to Immunothrombosis in COVID-19 Acute Respiratory Distress Syndrome. Blood 136, 1169–1179. doi:10.1182/blood.2020007008
Milioli, M., Ibáñez-Vea, M., Sidoli, S., Palmisano, G., Careri, M., and Larsen, M. R. (2015). Quantitative Proteomics Analysis of Platelet-Derived Microparticles Reveals Distinct Protein Signatures when Stimulated by Different Physiological Agonists. J. Proteomics 121, 56–66. doi:10.1016/j.jprot.2015.03.013
Miller, Y. I., Choi, S.-H., Wiesner, P., Fang, L., Harkewicz, R., Hartvigsen, K., et al. (2011). Oxidation-Specific Epitopes Are Danger-Associated Molecular Patterns Recognized by Pattern Recognition Receptors of Innate Immunity. Circ. Res. 108, 235–248. doi:10.1161/CIRCRESAHA.110.223875
Morrell, C. N., Aggrey, A. A., Chapman, L. M., and Modjeski, K. L. (2014). Emerging Roles for Platelets as Immune and Inflammatory Cells. Blood 123, 2759–2767. doi:10.1182/blood-2013-11-462432
Morris, G., Bortolasci, C. C., Puri, B. K., Olive, L., Marx, W., O'Neil, A., et al. (2021). Preventing the Development of Severe COVID-19 by Modifying Immunothrombosis. Life Sci. 264, 118617. doi:10.1016/j.lfs.2020.118617
Nassiri, A. A., Hakemi, M. S., Miri, M. M., Shahrami, R., Koomleh, A. A., and Sabaghian, T. (2021). Blood Purification with CytoSorb in Critically Ill COVID‐19 Patients: A Case Series of 26 Patients. Artif. Organs 45, 1338–1347. doi:10.1111/aor.14024
Nergiz-Unal, R., Lamers, M. M. E., Van Kruchten, R., Luiken, J. J., Cosemans, J. M. E. M., Glatz, J. F. C., et al. (2011). Signaling Role of CD36 in Platelet Activation and Thrombus Formation on Immobilized Thrombospondin or Oxidized Low-Density Lipoprotein. J. Thromb. Haemost. 9, 1835–1846. doi:10.1111/j.1538-7836.2011.04416.x
Nicolai, L., Leunig, A., Brambs, S., Kaiser, R., Weinberger, T., Weigand, M., et al. (2020). Immunothrombotic Dysregulation in COVID-19 Pneumonia Is Associated with Respiratory Failure and Coagulopathy. Circulation 142, 1176–1189. doi:10.1161/CIRCULATIONAHA.120.048488
Nieuwland, R., Berckmans, R. J., McGregor, S., Böing, A. N., Th. M. Romijn, F. P. H., Westendorp, R. G. J., et al. (2000). Cellular Origin and Procoagulant Properties of Microparticles in Meningococcal Sepsis. Blood 95, 930–935. doi:10.1182/blood.V95.3.930.003k46_930_935
Nomura, K., Miyashita, T., Yamamoto, Y., Munesue, S., Harashima, A., Takayama, H., et al. (2019). Citrullinated Histone H3: Early Biomarker of Neutrophil Extracellular Traps in Septic Liver Damage. J. Surg. Res. 234, 132–138. doi:10.1016/j.jss.2018.08.014
Nomura, S., Taniura, T., and Ito, T. (2020). Extracellular Vesicle-Related Thrombosis in Viral Infection. Int. J. Gen. Med. 13, 559–568. doi:10.2147/IJGM.S265865
Østerud, B., and Bouchard, B. A. (2019). Detection of Tissue Factor in Platelets: Why Is it So Troublesome? Platelets 30, 957–961. doi:10.1080/09537104.2019.1624708
Pan, D., Amison, R. T., Riffo-Vasquez, Y., Spina, D., Cleary, S. J., Wakelam, M. J., et al. (2015). P-Rex and Vav Rac-GEFs in Platelets Control Leukocyte Recruitment to Sites of Inflammation. Blood 125, 1146–1158. doi:10.1182/blood-2014-07-591040
Panes, O., Matus, V., Sáez, C. G., Quiroga, T., Pereira, J., and Mezzano, D. (2007). Human Platelets Synthesize and Express Functional Tissue Factor. Blood 109, 5242–5250. doi:10.1182/blood-2006-06-030619
Park, J. S., Gamboni-Robertson, F., He, Q., Svetkauskaite, D., Kim, J.-Y., Strassheim, D., et al. (2006). High Mobility Group Box 1 Protein Interacts with Multiple Toll-like Receptors. Am. J. Physiol. Cell Physiol. 290, C917–C924. doi:10.1152/ajpcell.00401.2005
Park, J. S., Svetkauskaite, D., He, Q., Kim, J.-Y., Strassheim, D., Ishizaka, A., et al. (2004). Involvement of Toll-like Receptors 2 and 4 in Cellular Activation by High Mobility Group Box 1 Protein. J. Biol. Chem. 279, 7370–7377. doi:10.1074/jbc.M306793200
Passacquale, G., Vamadevan, P., Pereira, L., Hamid, C., Corrigall, V., and Ferro, A. (2011). Monocyte-Platelet Interaction Induces a Pro-inflammatory Phenotype in Circulating Monocytes. PLoS ONE 6, e25595. doi:10.1371/journal.pone.0025595
Puhm, F., Boilard, E., and Machlus, K. R. (2020). Platelet Extracellular Vesicles: Beyond the Blood. Arterioscler. Thromb. Vasc. Biol. 41, 87–96. doi:10.1161/ATVBAHA.120.314644
Puhm, F., Flamand, L., and Boilard, E. (2021). Platelet Extracellular Vesicles in COVID‐19: Potential Markers and Makers. J. Leukoc. Bio 111, 63–74. doi:10.1002/JLB.3MIR0221-100R
Raeven, P., Zipperle, J., and Drechsler, S. (2018). Extracellular Vesicles as Markers and Mediators in Sepsis. Theranostics 8, 3348–3365. doi:10.7150/thno.23453
Ramakrishnan, D. P., Hajj-Ali, R. A., Chen, Y., and Silverstein, R. L. (2016). Extracellular Vesicles Activate a CD36-dependent Signaling Pathway to Inhibit Microvascular Endothelial Cell Migration and Tube Formation. Atvb 36, 534–544. doi:10.1161/ATVBAHA.115.307085
Rauova, L., Hirsch, J. D., Greene, T. K., Zhai, L., Hayes, V. M., Kowalska, M. A., et al. (2010). Monocyte-bound PF4 in the Pathogenesis of Heparin-Induced Thrombocytopenia. Blood 116, 5021–5031. doi:10.1182/blood-2010-03-276964
Rauova, L., Zhai, L., Kowalska, M. A., Arepally, G. M., Cines, D. B., and Poncz, M. (2006). Role of Platelet Surface PF4 Antigenic Complexes in Heparin-Induced Thrombocytopenia Pathogenesis: Diagnostic and Therapeutic Implications. Blood 107, 2346–2353. doi:10.1182/blood-2005-08-3122
Renu, K., Prasanna, P. L., and Valsala Gopalakrishnan, A. (2020). Coronaviruses Pathogenesis, Comorbidities and Multi-Organ Damage - A Review. Life Sci. 255, 117839. doi:10.1016/j.lfs.2020.117839
Rifkin, B. S., and Stewart, I. J. (2022). Seraph-100 Hemoperfusion in SARS-CoV-2-Infected Patients Early in Critical Illness: A Case Series. Blood Purif. 51, 317–320. doi:10.1159/000517430
Rock, G., Weber, V., and Stegmayr, B. (2021). Therapeutic Plasma Exchange (TPE) as a Plausible Rescue Therapy in Severe Vaccine-Induced Immune Thrombotic Thrombocytopenia. Transfus. Apher. Sci. 60, 103174. doi:10.1016/j.transci.2021.103174
Ronco, C., Bagshaw, S. M., Bellomo, R., Clark, W. R., Husain-Syed, F., Kellum, J. A., et al. (2021). Extracorporeal Blood Purification and Organ Support in the Critically Ill Patient during COVID-19 Pandemic: Expert Review and Recommendation. Blood Purif. 50, 17–27. doi:10.1159/000508125
Rosell, A., Havervall, S., von Meijenfeldt, F., Hisada, Y., Aguilera, K., Grover, S. P., et al. (2021). Patients with COVID-19 Have Elevated Levels of Circulating Extracellular Vesicle Tissue Factor Activity that Is Associated with Severity and Mortality-Brief Report. Arterioscler. Thromb. Vasc. Biol. 41, 878–882. doi:10.1161/ATVBAHA.120.315547
Rouhiainen, A., Imai, S., Rauvala, H., and Parkkinen, J. (2000). Occurrence of Amphoterin (HMG1) as an Endogenous Protein of Human Platelets that Is Exported to the Cell Surface upon Platelet Activation. Thromb. Haemost. 84, 1087–1094. doi:10.1055/s-0037-1614175
Ruiz-Rodríguez, J. C., Chiscano-Camón, L., Ruiz-Sanmartin, A., Palmada, C., Paola Plata-Menchaca, E., Franco-Jarava, C., et al. (2021). Cytokine Hemoadsorption as Rescue Therapy for Critically Ill Patients with SARS-CoV-2 Pneumonia with Severe Respiratory Failure and Hypercytokinemia. Front. Med. 8, 779038. doi:10.3389/fmed.2021.779038
Sartori, M. T., Zurlo, C., Bon, M., Bertomoro, A., Bendo, R., Bertozzi, I., et al. (2020). Platelet-Derived Microparticles Bearing PF4 and Anti-GAGS Immunoglobulins in Patients with Sepsis. Diagnostics 10, 627. doi:10.3390/diagnostics10090627
Schmidt, J. J., Borchina, D. N., van't Klooster, M., Bulhan-Soki, K., Okioma, R., Herbst, L., et al. (2022). Interim Analysis of the COSA (COVID-19 Patients Treated with the Seraph 100 Microbind Affinity Filter) Registry. Nephrol. Dial. Transpl. 37, 673–680. doi:10.1093/ndt/gfab347
Schrottmaier, W. C., Pirabe, A., Pereyra, D., Heber, S., Hackl, H., Schmuckenschlager, A., et al. (2021). Adverse Outcome in COVID-19 Is Associated with an Aggravating Hypo-Responsive Platelet Phenotype. Front. Cardiovasc. Med. 8, 795624. doi:10.3389/fcvm.2021.795624
Seffer, M.-T., Cottam, D., Forni, L. G., and Kielstein, J. T. (2021). Heparin 2.0: A New Approach to the Infection Crisis. Blood Purif. 50, 28–34. doi:10.1159/000508647
Seffer, M.-T., Eden, G., Engelmann, S., and Kielstein, J. T. (2020). Elimination of Staphylococcus aureus from the Bloodstream Using a Novel Biomimetic Sorbent Haemoperfusion Device. BMJ Case Rep. 13, e235262. doi:10.1136/bcr-2020-235262
Seyoum, M., Enawgaw, B., and Melku, M. (2018). Human Blood Platelets and Viruses: Defense Mechanism and Role in the Removal of Viral Pathogens. Thromb. J. 16, 16. doi:10.1186/s12959-018-0170-8
Shen, S., Zhang, J., Fang, Y., Lu, S., Wu, J., Zheng, X., et al. (2021). SARS-CoV-2 Interacts with Platelets and Megakaryocytes via ACE2-independent Mechanism. J. Hematol. Oncol. 14, 72. doi:10.1186/s13045-021-01082-6
Shiraki, R., Inoue, N., Kawasaki, S., Takei, A., Kadotani, M., Ohnishi, Y., et al. (2004). Expression of Toll-like Receptors on Human Platelets. Thrombosis Res. 113, 379–385. doi:10.1016/j.thromres.2004.03.023
Siddiqui, F. A., Desai, H., Amirkhosravi, A., Amaya, M., and Francis, J. L. (2002). The Presence and Release of Tissue Factor from Human Platelets. Platelets 13, 247–253. doi:10.1080/09537100220146398
Sinauridze, E., Kireev, D., Popenko, N., Pichugin, A., Panteleev, M., Krymskaya, O., et al. (2007). Platelet Microparticle Membranes Have 50- to 100-fold Higher Specific Procoagulant Activity Than Activated Platelets. Thromb. Haemost. 97, 425–434. doi:10.1160/TH06-06-0313
Skendros, P., Mitsios, A., Chrysanthopoulou, A., Mastellos, D. C., Metallidis, S., Rafailidis, P., et al. (2020). Complement and Tissue Factor-Enriched Neutrophil Extracellular Traps Are Key Drivers in COVID-19 Immunothrombosis. J. Clin. Invest. 130, 6151–6157. doi:10.1172/JCI141374
Song, X., Hu, W., Yu, H., Zhao, L., Zhao, Y., Zhao, X., et al. (2020). Little to No Expression of Angiotensin‐converting Enzyme‐2 on Most Human Peripheral Blood Immune Cells but Highly Expressed on Tissue Macrophages. Cytometry 24285. doi:10.1002/cyto.a.24285
Ståhl, A.-l., Johansson, K., Mossberg, M., Kahn, R., and Karpman, D. (2019). Exosomes and Microvesicles in Normal Physiology, Pathophysiology, and Renal Diseases. Pediatr. Nephrol. 34, 11–30. doi:10.1007/s00467-017-3816-z
Suades, R., Padró, T., Vilahur, G., and Badimon, L. (2022). Platelet-released Extracellular Vesicles: the Effects of Thrombin Activation. Cell. Mol. Life Sci. 79, 190. doi:10.1007/s00018-022-04222-4
Sung, P.-S., and Hsieh, S.-L. (2021). C-type Lectins and Extracellular Vesicles in Virus-Induced NETosis. J. Biomed. Sci. 28, 46. doi:10.1186/s12929-021-00741-7
Sung, P.-S., and Hsieh, S.-L. (2019). CLEC2 and CLEC5A: Pathogenic Host Factors in Acute Viral Infections. Front. Immunol. 10, 2867. doi:10.3389/fimmu.2019.02867
Sung, P.-S., Huang, T.-F., and Hsieh, S.-L. (2019). Extracellular Vesicles from CLEC2-Activated Platelets Enhance Dengue Virus-Induced Lethality via CLEC5A/TLR2. Nat. Commun. 10, 2402. doi:10.1038/s41467-019-10360-4
Swystun, L. L., and Liaw, P. C. (2016). The Role of Leukocytes in Thrombosis. Blood 128, 753–762. doi:10.1182/blood-2016-05-718114
Tandon, R., Sharp, J. S., Zhang, F., Pomin, V. H., Ashpole, N. M., Mitra, D., et al. (2021). Effective Inhibition of SARS-CoV-2 Entry by Heparin and Enoxaparin Derivatives. J. Virol. 95, e01987–20. doi:10.1128/JVI.01987-20
Tang, L., Yin, Z., Hu, Y., and Mei, H. (2020). Controlling Cytokine Storm Is Vital in COVID-19. Front. Immunol. 11, 570993. doi:10.3389/fimmu.2020.570993
Tang, N., Li, D., Wang, X., and Sun, Z. (2020). Abnormal Coagulation Parameters Are Associated with Poor Prognosis in Patients with Novel Coronavirus Pneumonia. J. Thromb. Haemost. 18, 844–847. doi:10.1111/jth.14768
Taus, F., Meneguzzi, A., Castelli, M., and Minuz, P. (2019). Platelet-Derived Extracellular Vesicles as Target of Antiplatelet Agents. What Is the Evidence? Front. Pharmacol. 10, 1256. doi:10.3389/fphar.2019.01256
Taus, F., Salvagno, G., Canè, S., Fava, C., Mazzaferri, F., Carrara, E., et al. (2020). Platelets Promote Thromboinflammation in SARS-CoV-2 Pneumonia. Am. J. Physiol. Cell Physiol. 40, 2975–2989. doi:10.1161/ATVBAHA.120.315175
Thomas, M., and Scully, M. (2022). Clinical Features of Thrombosis and Bleeding in COVID-19. Blood blood, 2021012247. doi:10.1182/blood.2021012247
Thon, J. N., Peters, C. G., Machlus, K. R., Aslam, R., Rowley, J., Macleod, H., et al. (2012). T Granules in Human Platelets Function in TLR9 Organization and Signaling. J. Cell Biol. 198, 561–574. doi:10.1083/jcb.201111136
Traby, L., Kollars, M., Kussmann, M., Karer, M., Šinkovec, H., Lobmeyr, E., et al. (2022). Extracellular Vesicles and Citrullinated Histone H3 in Coronavirus Disease 2019 Patients. Thromb. Haemost. 122, 113–122. doi:10.1055/a-1522-4131
Tripisciano, C., Weiss, R., Eichhorn, T., Spittler, A., Heuser, T., Fischer, M. B., et al. (2017). Different Potential of Extracellular Vesicles to Support Thrombin Generation: Contributions of Phosphatidylserine, Tissue Factor, and Cellular Origin. Sci. Rep. 7, 6522. doi:10.1038/s41598-017-03262-2
Tripisciano, C., Weiss, R., Karuthedom George, S., Fischer, M. B., and Weber, V. (2020). Extracellular Vesicles Derived from Platelets, Red Blood Cells, and Monocyte-like Cells Differ Regarding Their Ability to Induce Factor XII-dependent Thrombin Generation. Front. Cell Dev. Biol. 8, 298. doi:10.3389/fcell.2020.00298
Tsiantoulas, D., Perkmann, T., Afonyushkin, T., Mangold, A., Prohaska, T. A., Papac-Milicevic, N., et al. (2015). Circulating Microparticles Carry Oxidation-specific Epitopes and Are Recognized by Natural IgM Antibodies. J. Lipid Res. 56, 440–448. doi:10.1194/jlr.P054569
Valadi, H., Ekström, K., Bossios, A., Sjöstrand, M., Lee, J. J., and Lötvall, J. O. (2007). Exosome-mediated Transfer of mRNAs and microRNAs Is a Novel Mechanism of Genetic Exchange between Cells. Nat. Cell Biol. 9, 654–659. doi:10.1038/ncb1596
Vazquez, C., and Horner, S. M. (2015). MAVS Coordination of Antiviral Innate Immunity. J. Virol. 89, 6974–6977. doi:10.1128/JVI.01918-14
Vieira-de-Abreu, A., Campbell, R. A., Weyrich, A. S., and Zimmerman, G. A. (2012). Platelets: Versatile Effector Cells in Hemostasis, Inflammation, and the Immune Continuum. Semin. Immunopathol. 34, 5–30. doi:10.1007/s00281-011-0286-4
Vogel, S., Bodenstein, R., Chen, Q., Feil, S., Feil, R., Rheinlaender, J., et al. (2015). Platelet-derived HMGB1 Is a Critical Mediator of Thrombosis. J. Clin. Invest. 125, 4638–4654. doi:10.1172/JCI81660
Wegrzyn, G., Walborn, A., Rondina, M., Fareed, J., and Hoppensteadt, D. (2021). Biomarkers of Platelet Activation and Their Prognostic Value in Patients with Sepsis-Associated Disseminated Intravascular Coagulopathy. Clin. Appl. Thromb. Hemost. 27, 107602962094330. doi:10.1177/1076029620943300
Weismann, D., and Binder, C. J. (2012). The Innate Immune Response to Products of Phospholipid Peroxidation. Biochim. Biophys. Acta 1818, 2465–2475. doi:10.1016/j.bbamem.2012.01.018
Weiss, R., Gröger, M., Rauscher, S., Fendl, B., Eichhorn, T., Fischer, M. B., et al. (2018). Differential Interaction of Platelet-Derived Extracellular Vesicles with Leukocyte Subsets in Human Whole Blood. Sci. Rep. 8, 6598. doi:10.1038/s41598-018-25047-x
Wiersinga, W. J., Rhodes, A., Cheng, A. C., Peacock, S. J., and Prescott, H. C. (2020). Pathophysiology, Transmission, Diagnosis, and Treatment of Coronavirus Disease 2019 (COVID-19). JAMA 324, 782. doi:10.1001/jama.2020.12839
Witt, D. P., and Lander, A. D. (1994). Differential Binding of Chemokines to Glycosaminoglycan Subpopulations. Curr. Biol. 4, 394–400. doi:10.1016/S0960-9822(00)00088-9
Wu, J., Piao, Y., Liu, Q., and Yang, X. (2021). Platelet‐rich Plasma‐derived Extracellular Vesicles: A Superior Alternative in Regenerative Medicine? Cell Prolif. 54. doi:10.1111/cpr.13123
Xu, D., Young, J., Song, D., and Esko, J. D. (2011). Heparan Sulfate Is Essential for High Mobility Group Protein 1 (HMGB1) Signaling by the Receptor for Advanced Glycation End Products (RAGE). J. Biol. Chem. 286, 41736–41744. doi:10.1074/jbc.M111.299685
Yáñez-Mó, M., Siljander, P. R.-M., Andreu, Z., Bedina Zavec, A., Borràs, F. E., Buzas, E. I., et al. (2015). Biological Properties of Extracellular Vesicles and Their Physiological Functions. J. Extracell. Vesicles 4, 27066. doi:10.3402/jev.v4.27066
Yang, X., Yang, Q., Wang, Y., Wu, Y., Xu, J., Yu, Y., et al. (2020). Thrombocytopenia and its Association with Mortality in Patients with COVID‐19. J. Thromb. Haemost. 18, 1469–1472. doi:10.1111/jth.14848
Zaid, Y., and Merhi, Y. (2022). Implication of Platelets in Immuno-Thrombosis and Thrombo-Inflammation. Front. Cardiovasc. Med. 9, 863846. doi:10.3389/fcvm.2022.863846
Zaid, Y., Puhm, F., Allaeys, I., Naya, A., Oudghiri, M., Khalki, L., et al. (2020). Platelets Can Associate with SARS-Cov-2 RNA and Are Hyperactivated in COVID-19. Circ. Res. 127, 1404–1418. doi:10.1161/CIRCRESAHA.120.317703
Zhang, S., Liu, Y., Wang, X., Yang, L., Li, H., Wang, Y., et al. (2020). SARS-CoV-2 Binds Platelet ACE2 to Enhance Thrombosis in COVID-19. J. Hematol. Oncol. 13, 120. doi:10.1186/s13045-020-00954-7
Zhang, S., Zhang, S., Hu, L., Zhai, L., Xue, R., Ye, J., et al. (2015). Nucleotide-Binding Oligomerization Domain 2 Receptor Is Expressed in Platelets and Enhances Platelet Activation and Thrombosis. Circulation 131, 1160–1170. doi:10.1161/CIRCULATIONAHA.114.013743
Zhou, P., Yang, X.-L., Wang, X.-G., Hu, B., Zhang, L., Zhang, W., et al. (2020). A Pneumonia Outbreak Associated with a New Coronavirus of Probable Bat Origin. Nature 579, 270–273. doi:10.1038/s41586-020-2012-7
Zhu, N., Zhang, D., Wang, W., Li, X., Yang, B., Song, J., et al. (2020). A Novel Coronavirus from Patients with Pneumonia in China, 2019. N. Engl. J. Med. 382, 727–733. doi:10.1056/NEJMoa2001017
Zillmann, A., Luther, T., Müller, I., Kotzsch, M., Spannagl, M., Kauke, T., et al. (2001). Platelet-Associated Tissue Factor Contributes to the Collagen-Triggered Activation of Blood Coagulation. Biochem. Biophysical Res. Commun. 281, 603–609. doi:10.1006/bbrc.2001.4399
Keywords: apheresis, coagulopathy, COVID-19, extracellular vesicles, heparin, immunothrombosis, platelets, platelet factor 4
Citation: Ebeyer-Masotta M, Eichhorn T, Weiss R, Lauková L and Weber V (2022) Activated Platelets and Platelet-Derived Extracellular Vesicles Mediate COVID-19-Associated Immunothrombosis. Front. Cell Dev. Biol. 10:914891. doi: 10.3389/fcell.2022.914891
Received: 07 April 2022; Accepted: 08 June 2022;
Published: 06 July 2022.
Edited by:
Roberto Bruzzone, Institut Pasteur, FranceReviewed by:
Shie-Liang Hsieh, Academia Sinica, TaiwanAngelo A. Manfredi, Vita-Salute San Raffaele University, Italy
Niloufar Kavian, Université Paris Descartes, France
Copyright © 2022 Ebeyer-Masotta, Eichhorn, Weiss, Lauková and Weber. This is an open-access article distributed under the terms of the Creative Commons Attribution License (CC BY). The use, distribution or reproduction in other forums is permitted, provided the original author(s) and the copyright owner(s) are credited and that the original publication in this journal is cited, in accordance with accepted academic practice. No use, distribution or reproduction is permitted which does not comply with these terms.
*Correspondence: Viktoria Weber, dmlrdG9yaWEud2ViZXJAZG9uYXUtdW5pLmFjLmF0